- 1Department of Earth and Environmental Sciences, University of Michigan, Ann Arbor, MI, USA
- 2Department of Earth Sciences, University of Minnesota, Minneapolis, MN, USA
- 3BioTechnology Institute, University of Minnesota, Minneapolis, MN, USA
- 4Annis Water Resources Institute, Grand Valley State University, Muskegon, MI, USA
- 5NOAA-Great Lakes Environmental Research Laboratory, Ann Arbor, MI, USA
Little is known about large sulfur bacteria (LSB) that inhabit sulfidic groundwater seeps in large lakes. To examine how geochemically relevant microbial metabolisms are partitioned among community members, we conducted metagenomic analysis of a chemosynthetic microbial mat in the Isolated Sinkhole, which is in a deep, aphotic environment of Lake Huron. For comparison, we also analyzed a white mat in an artesian fountain that is fed by groundwater similar to Isolated Sinkhole, but that sits in shallow water and is exposed to sunlight. De novo assembly and binning of metagenomic data from these two communities yielded near complete genomes and revealed representatives of two families of LSB. The Isolated Sinkhole community was dominated by novel members of the Beggiatoaceae that are phylogenetically intermediate between known freshwater and marine groups. Several of these Beggiatoaceae had 16S rRNA genes that contained introns previously observed only in marine taxa. The Alpena fountain was dominated by populations closely related to Thiothrix lacustris and an SM1 euryarchaeon known to live symbiotically with Thiothrix spp. The SM1 genomic bin contained evidence of H2-based lithoautotrophy. Genomic bins of both the Thiothrix and Beggiatoaceae contained genes for sulfur oxidation via the rDsr pathway, H2 oxidation via Ni-Fe hydrogenases, and the use of O2 and nitrate as electron acceptors. Mats at both sites also contained Deltaproteobacteria with genes for dissimilatory sulfate reduction (sat, apr, and dsr) and hydrogen oxidation (Ni-Fe hydrogenases). Overall, the microbial mats at the two sites held low-diversity microbial communities, displayed evidence of coupled sulfur cycling, and did not differ largely in their metabolic potentials, despite the environmental differences. These results show that groundwater-fed communities in an artesian fountain and in submerged sinkholes of Lake Huron are a rich source of novel LSB, associated heterotrophic and sulfate-reducing bacteria, and archaea.
Introduction
The discovery of chemosynthetic communities at deep-sea hydrothermal vents fundamentally changed views of how energy and carbon flows through ecosystems (Jannasch and Wirsen, 1979). Although this marked the first known example of complex communities based on inorganic energy sources, the concept of chemosynthesis dates back far earlier, to Winogradsky’s studies of large sulfur bacteria (LSB) (Ackert, 2006). Chemosynthetic microbial communities have now been well studied in many marine and terrestrial environments. Particularly prominent are LSB that form dense mats at redox boundaries where sulfide meets oxygen (Jørgensen, 2010). These organisms are geographically widespread, feature extensive morphological diversity, and provide a rich platform for studying microbial evolution and physiology (Salman et al., 2013). LSB thrive in marine seeps (Larkin et al., 1994; Joye et al., 2004; Jones et al., 2015), mud volcanoes (Girnth et al., 2011), deep-sea hydrothermal systems (McKay et al., 2012), organic-rich sediments, terrestrial hydrothermal vents (Konkol et al., 2010) and sulfide springs (Caldwell et al., 1975; Nelson and Castenholz, 1981; Larkin et al., 1994; Teske, 2006; Chaudhary et al., 2009), sulfidic caves (Engel et al., 2001; Macalady et al., 2006) and phototrophic mats (Hinck et al., 2011). Although Thioploca spp. have been studied in some large lakes (Zemskaya et al., 2009), little is known about chemosynthetic communities or LSB in large freshwater lakes such as the Laurentian Great Lakes (Biddanda et al., 2006).
In submerged sinkholes of Lake Huron, venting of low-oxygen, saline, sulfate-rich, sulfidic groundwater supports chemosynthetic microbial communities (Biddanda et al., 2006). The geochemistry of this groundwater is shaped by water-rock interactions in Silurian-Devonian carbonate-limestone bedrock aquifers, in which evaporites including gypsum and anhydrite are dissolved (Ruberg et al., 2008). Dissolution of the limestone has produced both onshore and offshore karst formations in Alpena County, including sinkholes in the lake bottom where groundwater emerges (Biddanda et al., 2006). The sinkholes were first scientifically discovered during a 2001 shipwreck survey in the Thunder Bay National Marine Sanctuary (Coleman, 2002). During subsequent surveys in 2002 and 2003, active groundwater intrusion was observed at the Middle Island, Isolated, and Misery Bay sinkholes (Biddanda et al., 2006).
Several microbial mat communities that are not typically found elsewhere in the Great Lakes populate these sinkholes. At the Middle Island Sinkhole, mat communities in the photic zone at 23 m water depth are dominated by metabolically versatile cyanobacteria that can conduct both oxygenic and anoxygenic photosynthesis (Nold et al., 2010; Voorhies et al., 2012). In communities beneath the cyanobacterial mats chemosynthetic primary production is fueled by redox reactions of an active sulfur cycle driven by sulfur-oxidizing and sulfate-reducing bacteria (Nold et al., 2010; Voorhies et al., 2012).
The Isolated Sinkhole sits in the aphotic zone at a water depth of 93 m and covers an area of approximately 55 m × 40 m, as described in detail in Biddanda et al. (2006). Groundwater seeps into the sinkhole and is characterized by high chlorine, sulfate, phosphorus, particulate organic matter, and conductivity. Dissolved oxygen levels are low, ranging from between 1.28 and 5.12 mg L-1 throughout the year. A 1–2 m thick turbid “sinkhole plume” with elevated biomass sits near the bottom of the sinkhole. Two distinct microbial mat morphotypes cover the floor of the sinkhole: one is white, irregularly shaped, and cohesive and the other is brown, non-cohesive, and sometimes associated with pink ‘fingers’ (Biddanda et al., 2006). Previous sampling and microscopic analysis of the Isolated Sinkhole characterized the microbial mat community as low diversity, with just a few dominant bacterial species (Biddanda et al., 2006). Evidence of chemoautotrophic primary production at this site led to the conclusion that the microbial community generates most of its organic matter in situ rather than relying on organic material settling down from above. This study also predicted that dissimilatory sulfate reduction would be important in the sinkhole ecosystem, but the specific metabolisms and organisms have, until now, not been confirmed.
Groundwater seeps in this area also occur in sinkholes on land and at the Alpena County Library fountain, where an artesian well draws groundwater from the same aquifers that feed the sinkholes (Snider et al., 2017). The fountain consists of a lower basin that has brown cyanobacterial mats and an upper basin that has white chemosynthetic mats. A complete description of the fountain site is provided in Snider et al. (2017). Major differences in microbial mat habitat between this site and the Isolated Sinkhole include the following: (i) the fountain is exposed to direct sunlight, (ii) groundwater at the fountain is in closer contact with atmospheric oxygen but does not interact with lake water as at Isolated Sinkhole, (iii) mats in the fountain have no underlying sediment as at Isolated Sinkhole, (iv) the rate of water flow at the fountain is higher than at the Isolated Sinkhole.
In this study, we sought to understand the interaction between groundwater geochemistry and microbial community composition and metabolism in two contrasting settings, the Isolated Sinkhole and the Alpena fountain. We used a metagenomic approach to identify genes that underpin energy metabolisms, focusing on sulfur metabolism, hydrogen oxidation, aerobic respiration, denitrification, and carbon fixation. Our results indicate that these two communities are driven by similar suites of largely sulfur-based metabolic pathways held within different taxa at the two sites.
Materials and Methods
Sample Collection, Chemical Analyses, DNA Extraction, and Metagenomic Sequencing
A sample was collected from the whole white mat, which was directly attached to the fountain substrate, in the upper basin of the Alpena library fountain site (45 3.747′N, 83 25.872′W) on July 24th, 2012, and preserved in RNAlater (Ambion) as instructed by the manufacturer. Sulfide concentrations were determined by the methylene blue method (Cline, 1969) using Procedure 1 described by Reese et al. (2011). Dissolved oxygen was measured by a YSI Pro Dissolved Oxygen probe (Digital Professional Series). At the Isolated Sinkhole site (45 10.711′N, 83 09.173′W), a sample of white mat was skimmed off of sediment from a box core collected on September 6th 2008 on board the R/V Laurentian using a 60 ml syringe with attached flexible Tygon tubing, and immediately frozen at -20°C. After thawing, the white mat was manually separated from surrounding sediment and washed gently with a filter-sterilized NaCl solution to remove visible sediment particles. We note that even such gentle washing could reduce the diversity detected, and since it was only applied to the Isolated Sinkhole sample (where attached sediments were present), comparisons between the two samples could theoretically have been affected. However, it is unlikely to influence the results of our main focus, the large sulfur-oxidizing bacteria. DNA from both samples was extracted using the FastDNA Spin Kit for Soil (MP Biomedicals). Both samples were then sequenced in September of 2012 by standard methods using the Illumina HiSeq with paired-end 100 base-length reads at the University of Michigan DNA Sequencing Core. Sequence reads were deposited to the NCBI Sequence Read Archive (see Supplementary Table S1 for accession numbers).
rRNA Gene Assembly and Phylogenetic Analysis
16S rRNA gene sequences were assembled from the metagenomic datasets using EMIRGE (Miller et al., 2011). EMIRGE assemblies were performed with 120 iterations against the Silva small subunit reference database v. 111 (Pruesse et al., 2007) using quality filtered and trimmed reads as described below. Nine of the resulting 22 EMIRGE sequences had top BLAST hits within the Beggiatoaceae. These sequences were screened for chimeric sequences using Pintail (Ashelford et al., 2005) in mothur v. 1.33 (Schloss et al., 2009), and no chimeras were detected. Initial difficulties in resolving the phylogeny of one the EMIRGE sequences suggested the possible presence of an intron. The EMIRGE sequences were aligned with 16S rRNA gene sequences from taxa within the Beggiatoaceae that contain introns, as well as taxa whose 16S rRNA gene sequences do not contain introns, using Geneious (9.1.3) (Kearse et al., 2012). This alignment identified homologs to introns observed by Salman et al. (2012). The introns were manually removed and the 16S rRNA genes were aligned using the SINA aligner (Pruesse et al., 2012). The alignment was manually corrected in ARB (Ludwig et al., 2004) before phylogenetic analyses. Alignments were end-clipped so that all sequences were of equal length and positions with >50% gaps were removed (final alignment 28- 1271 E. coli base positions). Tree building was performed within Geneious. A neighbor joining phylogenetic tree with Jukes-Cantor distance correction (Jukes and Cantor, 1969; Saitou and Nei, 1987) and a heuristic search maximum parsimony tree (Fitch, 1971) were constructed with PAUP 4.0a148 (Swofford, 2003). Both trees were bootstrapped (Felsenstein, 1985) with 1000 replicates and consensus trees were generated. A consensus Bayesian interference phylogenic tree was constructed with MrBayes 3.2.6 with 1,000,000 generational runs (Huelsenbeck and Ronquist, 2001) using six substitution categories (aka. the general time reversible (GTR) model), gamma distribution and an invariant site nucleotide substitution rate (G++I). The trees were saved every 100 generations and posterior probabilities calculated after discarding the first 20% of trees. For maximum likelihood analyses, the GTR nucleotide substitution model with G+I rate variation was selected with JModelTest v.2.1.10 (Darriba et al., 2012). The Maximum-Likelihood (ML) analysis was computed in RAxML v.7.2.8 (Stamatakis, 2006) with 1000 bootstrap replicates. The ML tree was selected for the phylogenic distance tree. Branches were collapsed when ML bootstrap values fell below 50%.
Phylogenetic Analysis of rpoB
rpoB phylogenies were calculated using full-length or nearly full-length rpoB gene sequences from the metagenomes, and from freshwater and marine Beggiatoaceae available from isolate genomes and metagenomes at the Joint Genome Institute Integrated Microbial Genomes and Metagenomics (IMG/M) website (Markowitz et al., 2008). Nucleotide sequences were translated in ARB (Ludwig et al., 2004). Protein sequences were aligned using M-Coffee (Moretti et al., 2007), re-imported into ARB, and the corresponding nucleotide sequences re-aligned according to the amino acid alignments. Additional sequences were aligned with the ARB integrated aligner. Maximum likelihood analyses were computed using the rapid bootstrap algorithm (1000 replicates) in RAxML v.8.0.24 (Stamatakis, 2006). All positions with missing information and gaps were masked from the alignments prior to analysis, for final lengths of 989 and 2967 columns for the protein and nucleotide alignments, respectively. Evolutionary models were selected using the corrected Akaike information criterion (AICc) with ProtTest v.2.4 (Abascal et al., 2005) and JModelTest v2.1.5 (Darriba et al., 2012). Maximum likelihood analyses of protein sequences were calculated with the LG amino acid replacement matrix (Le and Gascuel, 2008) and gamma-distributed rates, with proportions of invariant sites and the alpha parameter estimated from the data. Maximum likelihood analyses of nucleotide sequences were calculated with the general time reversible model, gamma-distributed rates, and proportions of invariant sites and the alpha parameter estimated from the data.
Whole Genome Assembly and Annotation
Sequence reads were dereplicated (100% identity over 100% lengths), then trimmed with the adaptive read trimmer, Sickle (Joshi and Fass, 2011). De novo assembly of both metagenomic data sets was done using IDBA UD with a minimum kmer of 52, maximum kmer of 92, and a step size of 8 (Peng et al., 2012). Emergent self-organizing maps of tetranucleotide frequencies were then used to sort scaffolds of length greater than 4,000 bp into genomic bins as described previously (Dick et al., 2009). The Joint Genome Institute Integrated Microbial Genomes and Metagenomics (IMG/M) system was used to annotate genes on assembled scaffolds in the bins of interest (Markowitz et al., 2008). Annotations of genes of interest in these bins were confirmed manually using BLASTp to the National Center for Biotechnology Information non-redundant database with cutoffs of 150 for bitscore and 30% for percent identity (Pruitt et al., 2005), and results were evaluated manually. Scaffold sequences and gene annotations were deposited to NCBI (BioProject PRJNA340051 and PRJNA340052) and Integrated Microbial Genomes (Genome ID 3300003868 and 3300003874), respectively (see Supplementary Table S1 for accession numbers of individual genome bins).
Bin Completeness, Taxonomic Identification, and Mapping
Bin completeness was estimated by searching each bin for 36 conserved phylogenetic markers (Ciccarelli et al., 2006). The percentage completeness reported is the number of these conserved phylogenetic markers present out of the expected 36. The taxonomic identification of each bin was determined through a combination of methods including 16S identity, IMG/M, and PhyloSift (Darling et al., 2014). We first conducted a BLASTn search of scaffolds against the Silva Small Subunit RNA database release 119 using cutoffs of 700bp for alignment length and 80% for percent identity (Quast et al., 2013). Abundance of unbinned 16S rRNA genes identified through this BLASTn was then determined through a BLASTn search of all reads against 16S rRNA gene containing portions of scaffolds (Quast et al., 2013). This was then compared to the bin abundances of corresponding taxonomy in order to match unbinned 16S rRNA genes to bins. All bins were further identified using conserved phylogenetic markers as annotated by IMG/M (Ciccarelli et al., 2006). The most resolved taxonomy with ≥50% of gene sequences matching to it was reported (except in the case of Bin A7, in which Rhodoferax ferrireducens was reported due to its co-binning with that reference genome). These bin identities were confirmed and resolved using PhyloSift database of 37 universal, conserved gene families (Darling et al., 2014). The percent identity reported for Phylosift analyses refers to the percent of scaffolds that were identified as that taxonomy in the PhyloSift database. Bin abundance was found by mapping all reads to scaffolds of each bin using BWA with default parameters in order to determine coverage (Li and Durbin, 2009). The coverage of each scaffold was normalized for scaffold length and averaged to get mean genome coverage for the bin, which was used as a measure of abundance. In addition, scaffolds from Bin A8 were mapped to a published Thiothrix lacustris genome (NCBI accession NZ_JHYQ01000054.1) (Chernousova et al., 2009) using the same method (Li and Durbin, 2009).
Results and Discussion
We shotgun sequenced white microbial mat communities that are bathed in similar groundwater at two contrasting locations: the library fountain in the town of Alpena, Michigan (Figure 1A; Snider et al., 2017) and the Isolated Sinkhole, which is submerged at a water depth of 93 m in Lake Huron (Figure 1B; Biddanda et al., 2006). The emerging groundwater at these two sites is sourced from the same Silurian-Devonian aquifer and has similar conductivity and chemistry (Biddanda et al., 2012). The concentration of H2S in water samples taken from the upper basin of the Alpena Fountain, in which the white mats sat, ranged from 17 to 31 μM depending on the exact location and time sampled. Dissolved O2 concentrations in the same samples varied from 24 to 28 μM, and the water temperature was 11.4°C. It is unusual to have such relatively high concentrations of H2S and dissolved O2 in the same water sample. We suspect that this was the result of rapid entrainment of O2 from the atmosphere during vigorous mixing that occurs in the fountain. In emerging groundwater of Isolated Sinkhole the dissolved O2 concentration ranged from 6 to 44 μM and temperature was 6–7°C.
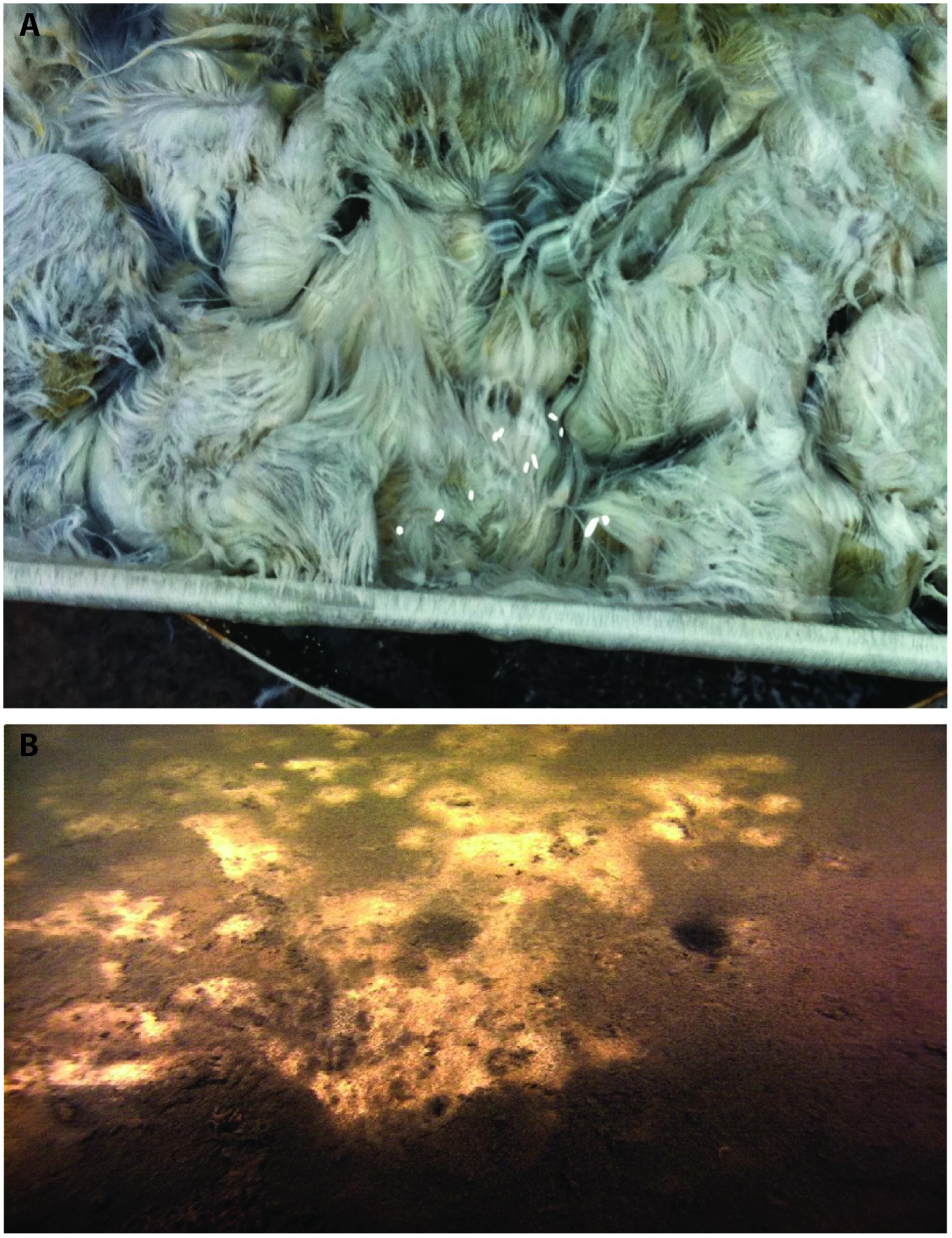
FIGURE 1. Images of white microbial mats in the Alpena fountain (A) and at the Isolated Sinkhole in Lake Huron (B). (A) Close-up photograph of Thiothrix filaments in the top reservoir of the Alpena fountain. Note that the bright white spots (bottom, center) are an artifact of reflected light in the photograph. (B) High-definition video still images of the Isolated Sinkhole taken by the ROV Little Hercules, courtesy of Dwight Coleman, Institute for Exploration.
De novo assembly and binning yielded eight genomic bins from the Alpena fountain and three from Isolated Sinkhole (Figure 2). These genomic bins, which are of varying degrees of completeness, were identified by Phylosift (Darling et al., 2014) and analysis of 16S rRNA genes, and their relative abundance was assessed by mapping reads to binned scaffolds (Table 1).
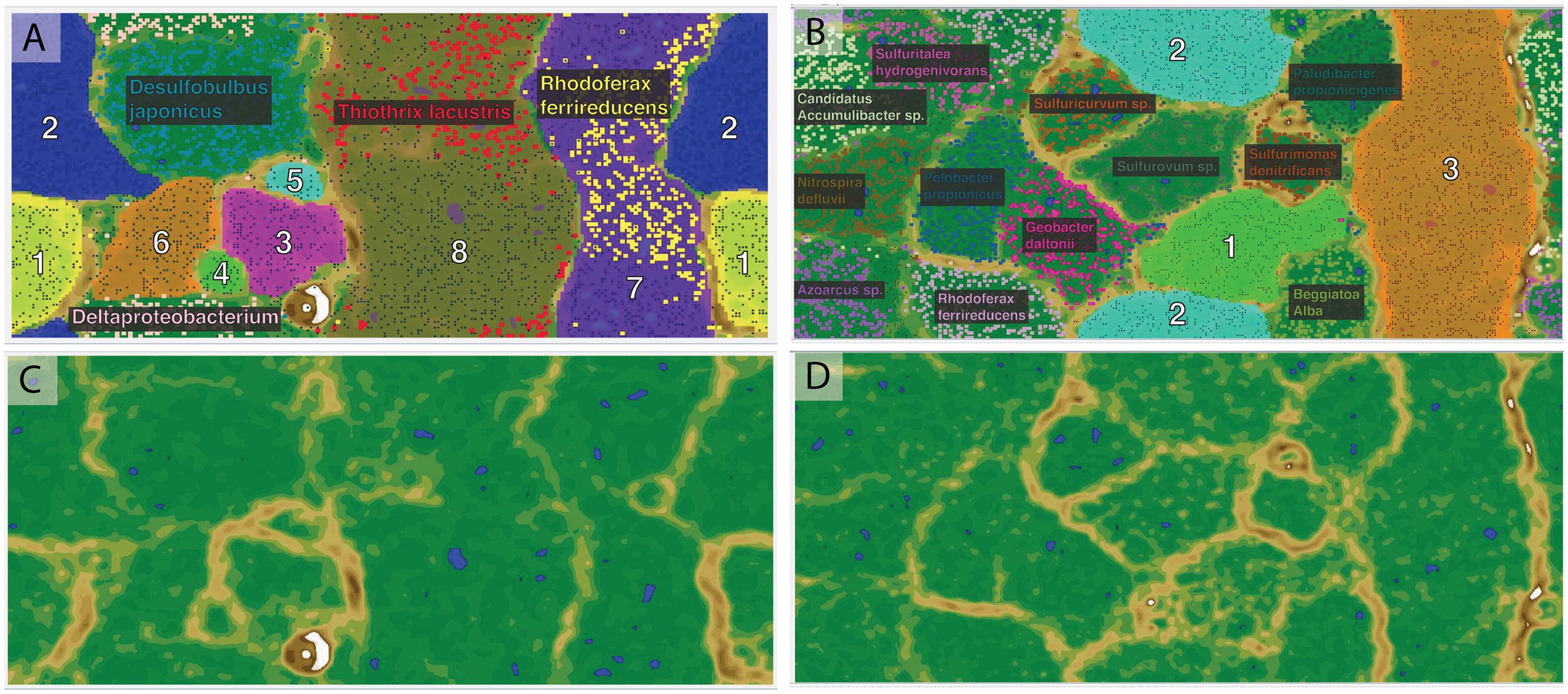
FIGURE 2. Emergent self-organizing maps used for binning of scaffolds by tetranucleotide frequency. The left panels (A,C) are for Alpena fountain and the right panels are for Isolated Sinkhole. The top panels (A,B) show the assigned bins indicated with numbers and fill color, with small data points representing sequence fragments. The species names indicate publicly available genomes used as references, with their sequence fragments shown in bold. The bottom panels (C,D) show the ESOM map background topography, which indicates the difference in tetranucleotide patterns between data points on the map. “High topography” (brown and white) indicates large differences and represents borders between bins, whereas “low topography” (green and blue) indicates similar tetranucleotide frequency patterns and represents areas within genomic bins.
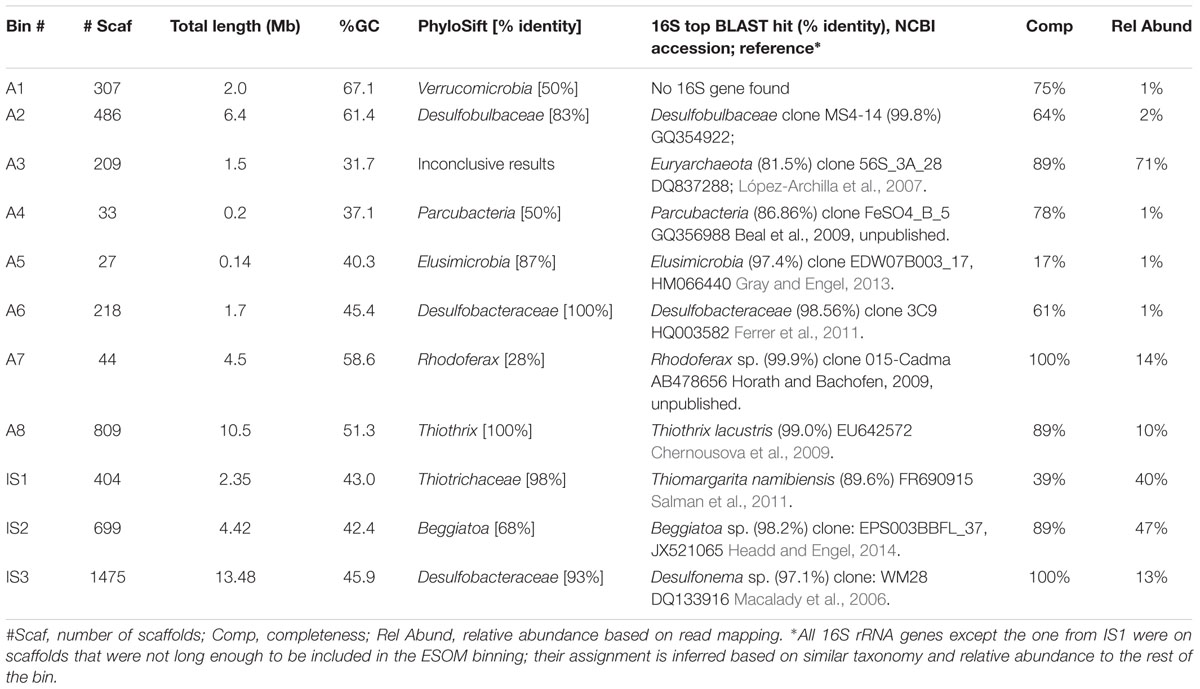
TABLE 1. Properties and taxonomic identification of genomic bins from the Alpena fountain and Isolated Sinkhole.
Community Composition
The metagenomes of both mat communities contained abundant members of known groups of LSB. The Isolated Sinkhole metagenome was dominated by two LSB genomic bins. One bin (IS2) represents a near-complete genome that was classified as Beggiatoa by both PhyloSift and 16S rRNA gene similarity (Table 1). The second (IS1) was classified by Phylosift as family Thiotrichaceae, but a fragment (1407 bp) of the 16S rRNA gene sequence had a closest match (89% sequence identity) to Thiomargarita namibiensis (Salman et al., 2011), which belongs to one of 8 currently recognized genera within the Beggiatoaceae. The 16S rRNA gene sequences associated with these two bins shared 84% sequence identity. The PhyloSift classification uses NCBI taxonomy, based on the system of Garrity et al. (2005), which proposed the Thiotrichaceae as a new polyphyletic family that encompasses the three traditional families of LSB, Leucotrichaceae, Achromatiaceae, and Beggiatoaceae. However, more recent work supports the traditional three family system (Salman et al., 2011, 2013) and thus would consider both bins IS1 and IS2 as members of the Beggiatoaceae.
One of the abundant genomic bins in the Alpena fountain (Bin A8) was closely related to T. lacustris (Table 1), an LSB genus known to form long filaments by attaching to surfaces in habitats with sulfidic flowing water (Larkin and Strohl, 1983), such as the Alpena fountain (Figure 1). This identification was supported by PhyloSift classification, by the co-binning of these scaffolds with the T. lacustris reference genome (Figure 2B), and by mapping of the reads to the T. lacustris genome at 157x average coverage. An unbinned 16S gene was found to have a 99% sequence similarity to T. lacustris (Chernousova et al., 2009), indicating it is the same species (Yarza et al., 2014).
In addition to LSB, the Isolated Sinkhole and Alpena fountain also had putative sulfate-reducing Deltaproteobacteria. Bins assigned to Desulfobacteraceae and Desulfobulbaceae were at moderate (Bin IS3; 13%) to low (Bins A2 and A6; 2% and 1%) relative abundances (Table 1). Members of these two families are strict anaerobes that reduce sulfate to sulfide (Kuever, 2014a,b). Other members of the microbial mat communities were different between the two sites. The Alpena fountain community is dominated by a well-defined genomic bin (A3; Figure 2) whose taxonomic identity could not be resolved by PhyloSift or IMG/M taxonomic profiling. Twenty-six percentage of its gene sequences matched to Archaea and 19% matched to Bacteria. Of the archaeal matches, the most common hits (24%) were to Euryarchaeota. An unbinned 16S gene with 97% sequence similarity to an uncultured SM1 euryarchaeon from cold sulfur springs that is highly divergent from cultivated archaea (Rudolph et al., 2001) was found to dominate the 16S rRNA gene sequence reads (Supplementary Figure S1) of the fountain, paralleling the dominance of genomic bin A3 of the fountain metagenome (Table 1). Members of this lineage, which was recently proposed to be a new euryarchaeal order, Candidatus Altiarchaeles (Probst et al., 2014b), form a ‘string of pearls’ morphology and are closely associated with Thiothrix filaments (Moissl et al., 2003) by attachment with nano-grappling structures (Moissl et al., 2005; Perras et al., 2014). Given the simplicity of the Alpena fountain community and the parallel dominance of the 16S gene and whole-genome scaffolds, this genomic bin can be confidently assigned as SM1 euryarchaeon.
Also present at high abundance in the Alpena fountain microbial mat was a genomic bin related to R. ferrireducens (Bin A7), a facultatively anaerobic betaproteobacterium that oxidizes organic carbon with the reduction of Fe(III) (Finneran et al., 2003). At lower abundance were two unknown groups of bacteria that PhyloSift analyses suggest are Verrucomicrobia (Bin1, 50% identity) and Elusimicrobia (Bin5, 87% identity) (Table 1). Also present at low abundance was a genomic bin for candidate division OD1 (Bin A4) (Harris et al., 2004), which has been found mainly in sulfur-rich, anoxic environments (Peura et al., 2012) and was recently proposed to be named Parcubacteria (Rinke et al., 2013).
Phylogenetic Analysis of LSB
To better identify the LSB, phylogenetic analysis was conducted on 16S rRNA and rpoB gene sequences assembled from shotgun metagenomic data. Initial alignments revealed the presence of two different large introns in two of the 16S rRNA genes (Figure 3). These introns have high sequence similarity to those previously identified in the marine LSB T. namibiensis (Salman et al., 2012). Such self-splicing introns are widespread among marine LSB, likely hinder PCR amplification of 16S rRNA genes, and may be horizontally transferred (Salman et al., 2012; MacGregor et al., 2013a; Flood et al., 2016). The introns found here are the first to be identified in non-marine LSB, and given their high sequence similarity to T. namibiensis, they are likely the result of horizontal gene transfer. After removal of the introns the remainder of the 16S rRNA gene sequence was most similar to other sequences from Isolated Sinkhole that may represent a novel group in the Beggiatoaceae (see below).
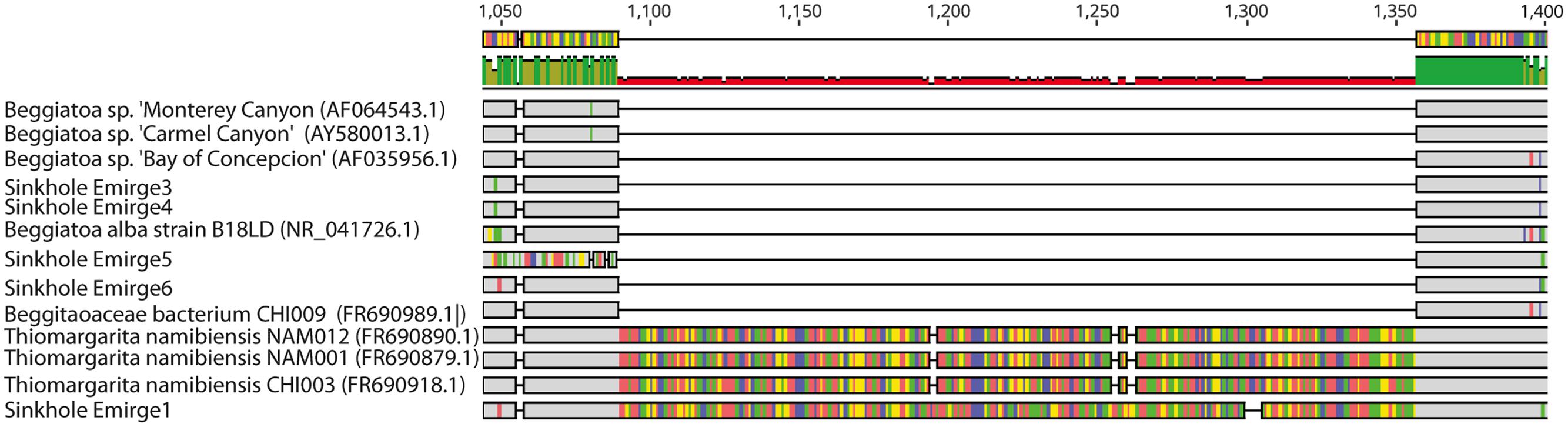
FIGURE 3. Geneious screenshot of alignments of 16S rRNA sequences from LSB, showing introns from an Isolated Sinkhole sequence that are homologous to those from Thiomargarita namibiensis. Bases that are dissimilar from other bases in each alignment column are shown in color. Numbers at top indicate the position on the 16S rRNA gene.
Phylogenetic analysis showed that the LSB sequences from Alpena Fountain and Isolated Sinkhole fell within two different clades (Figure 4). As expected, the Alpena fountain sequences clustered with Thiothrix sequences. The Isolated Sinkhole sequences formed novel clades within the Beggiatoaceae. Several branches were unresolved, consistent with previous phylogenetic analyses of these groups (Salman et al., 2013), but results suggest that the Isolated Sinkhole sequences represent new genera that are intermediate between the freshwater and marine Beggiatoaceae (Figure 4). Similar results were obtained from phylogenetic analysis of rpoB gene and protein sequences (Supplementary Figure S2). This phylogenetic placement might reflect the salinity of the groundwater fluids at the Alpena fountain and Isolated Sinkhole, which is ∼5% of seawater (Biddanda et al., 2012). However, the absence of such phylogenetically distinct LSB in other environments with comparable salinity, such as the Frasassi and Acquasanta cave systems (Macalady et al., 2008; Jones et al., 2010; Hamilton et al., 2015), suggests that other environmental factors may select for such organisms. Hereafter we refer to these organisms as Isolated Sinkhole Beggiatoaceae (ISB).
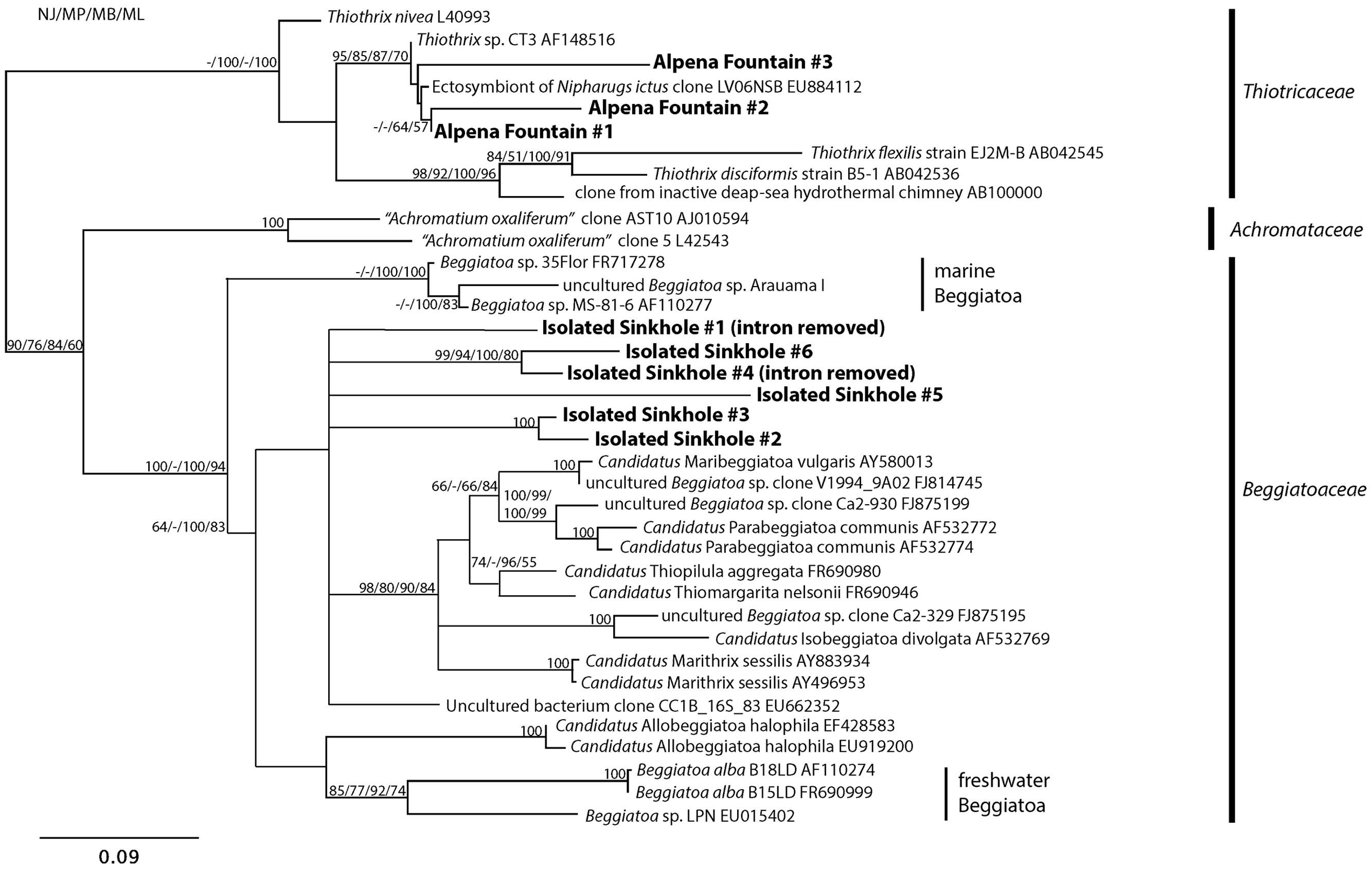
FIGURE 4. Phylogenetic tree of the 16S rRNA gene of selected large sulfur bacteria. Sequences from the Alpena Fountain and Isolated Sinkhole are shown in bold.
Energy Metabolism of LSB
Genes for dissimilatory sulfur metabolism were prevalent in the metagenomes of microbial mats at both sites. LSB are characterized by their capability to oxidize reduced sulfur compounds for chemolithoautotrophic or mixotrophic growth (Teske and Nelson, 2006). As expected, sulfur oxidation pathways were encoded in the genome bins of Thiothrix (A8) and ISB (IS1 and IS2). The Thiothrix bin had genes for flavocytochrome c sulfide dehydrogenase (fcc), sulfide quinone oxidoreductase (sqr), the sox system (soxABXYZ) and the reverse dissimilatory sulfite reductase pathway (dsr) (Supplementary Table S2 and Figure 5), consistent with the ability of T. lacustris to use either sulfide, thiosulfate, or intracellular elemental sulfur globules as electron donors during lithotrophic growth (Chernousova et al., 2009). Bin A8 also contains genes for polysulfide reductase (psr), respiratory tetrathionate reductase and thiosulfate reductase (phs), which disproportionates thiosulfate into sulfide and sulfite during the respiration of thiosulfate (Akegawa et al., 1985; Stoffels et al., 2012). Thiosulfate reductase genes are also present in Beggiatoa genomes (Mußmann et al., 2007), and thiosulfate disproportionation is a key process in some environments with active sulfur cycling (Jørgensen and Bak, 1991). The presence of genes for both oxidation (sox) and respiration/disproportionation (phs) suggests that it may be a key intermediate in sulfur cycling in the Thiothrix mats studied here.
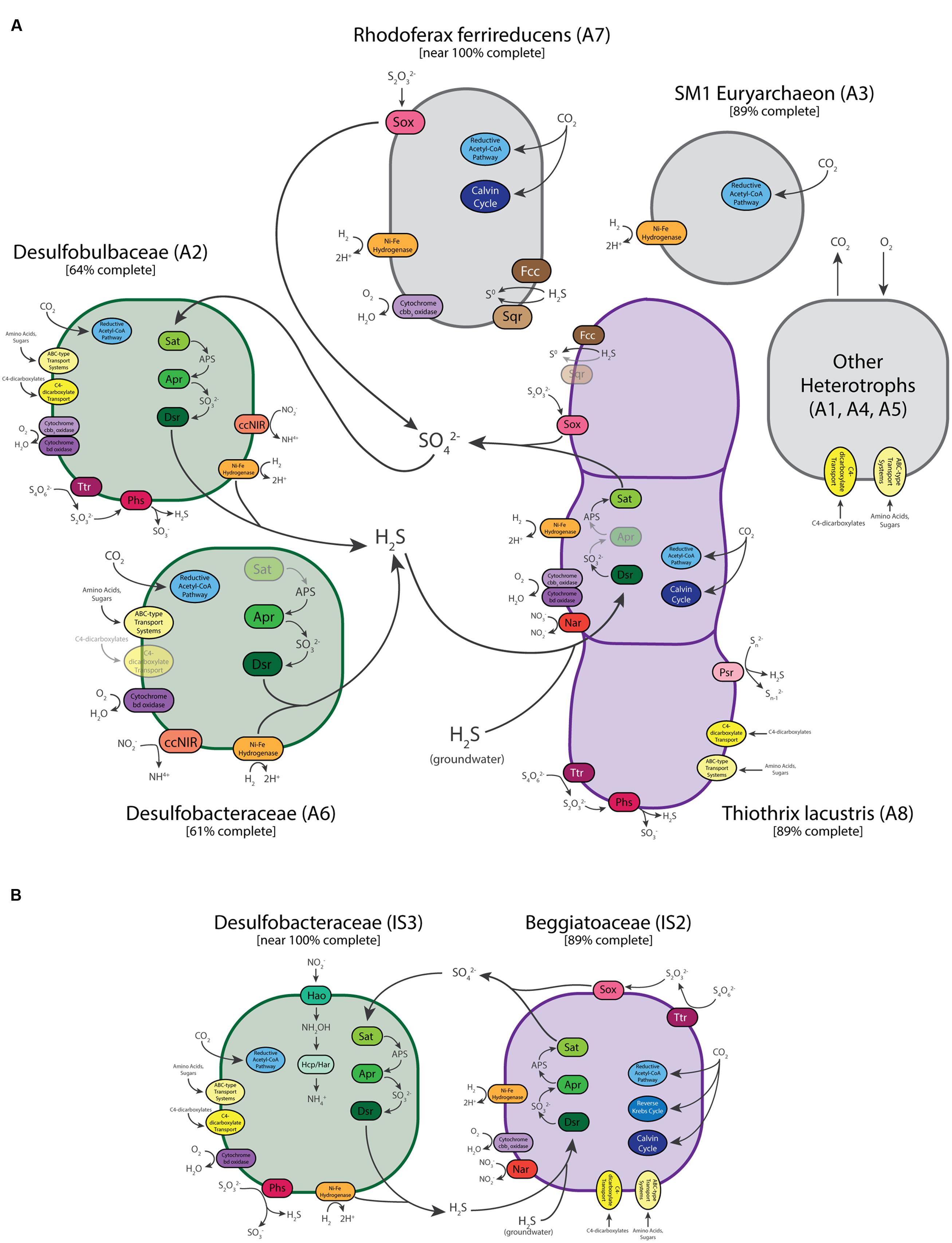
FIGURE 5. Schematic illustration of the predicted metabolisms and metabolic interactions of dominant community members of the Alpena fountain (A) and Isolated Sinkhole (B). Enzymes and reactions for which genes are expected to be present are missing are shown gray/dull. See text for abbreviations.
Only one of the two ISB bins from the Isolated Sinkhole (IS2) was near complete (Table 1). This genomic bin also contained genes for oxidation of sulfide (sqr and fcc), thiosulfate (soxABXY) and the reverse Dsr pathway (dsr, and also sat and apr) (Supplementary Table S2 and Figure 5), consistent with previously sequenced genomes of the Beggiatoaceae (Mußmann et al., 2007; MacGregor et al., 2013b; Flood et al., 2016; Winkel et al., 2016). It also had genes annotated as tetrathionate reductase. The availability of multiple pathways for oxidation of reduced sulfur species in the LSB at both the Alpena fountain and Isolated Sinkhole is consistent with a flexible lifestyle suited to a niche of varying availability of electron donors and acceptors (Klatt and Polerecky, 2015; Winkel et al., 2016).
In addition to lithotrophic growth on reduced sulfur compounds, genes for Ni-Fe hydrogenase in both the Thiothrix and ISB bins suggest that these organisms can also use hydrogen as an electron donor. These genes are commonly present in a wide range of organisms and habitats (Greening et al., 2016), including sulfur-oxidizing bacteria (Anantharaman et al., 2013). Members of the Beggiatoaceae are known to use H2 as a supplemental energy source (Schmidt et al., 1987; Kreutzmann and Schulz-Vogt, 2016; Winkel et al., 2016). H2 is produced by fermentation and may be present in terrestrial sulfidic springs (Aragno, 1992).
Both the Thiothrix and ISB genomic bins contained evidence of potential for aerobic respiration and nitrate reduction. Two different types of high affinity terminal oxidases for respiration at low-O2 concentrations were identified: cytochrome cbb3 oxidase and cytochrome bd oxidase (Morris and Schmidt, 2013). The Thiothrix bin (A8) contained both of these terminal oxidases, whereas the ISB bin contained only the cbb3-type oxidase (Supplementary Table S2 and Figure 5). The presence of these high-affinity terminal oxidases suggest that LSB at each of these sites conduct aerobic respiration at low concentrations of O2, which is likely introduced through diffusion and mixing with the atmosphere (Alpena fountain) or with overlying lake water (Isolated Sinkhole). These results are consistent with the microoxic lifestyle of many Beggiatoa spp. (Nelson et al., 1986b). To evaluate the possibility that these LSB are also capable of denitrification, we also searched for genes for reduction of nitrate, nitrite, and nitrous and nitric oxides (Supplementary Table S3; Philippot, 2002). Nar genes for dissimilatory nitrate reduction were found in the LSB genomic bins of both sites (ISB in the Isolated Sinkhole and T. lacustris in the fountain), as were norD and norQ genes for nitric oxide reduction. However, a homolog of the norB gene was only found in the Thiothrix bin (A8), and genes for dissimilatory reduction of nitrite or nitrous oxide were not found. The pathway for dissimilatory reduction of nitrate to ammonia (nirS and cytochrome c nitrite reductase), which is present in Thiomargarita along with genes for classical denitrification to N2 (Flood et al., 2016), was also not present in the LSB genomes analyzed here. Thus, we find genomic evidence for only partial denitrification, including reduction of nitrate and potentially nitric oxide. The resulting accumulation of nitrite could necessitate the detoxifying nitrite-reducing enzymes in the sulfate-reducing bacteria (see below). In culture, the type strain for T. lacustris is capable of nitrate reduction (Chernousova et al., 2009) but anaerobic growth on nitrate only occurs in the presence of thiosulfate, not organic substrates (Trubitsyn et al., 2013).
Carbon Metabolism of Large Sulfur Bacteria
Large sulfur bacteria including Thiothrix and Beggiatoa species have been shown to grow by autotrophy, heterotrophy, and mixotrophy (Nelson and Jannasch, 1983; Nelson et al., 1986a; Schmidt et al., 1987; Chernousova et al., 2009). In low oxygen, sulfidic environments, Beggiatoa prefer lithoautotrophic sulfide oxidation (Schmidt et al., 1987). We found genetic evidence of multiple carbon fixation pathways (Berg et al., 2010) in the LSB genomic bins recovered here (Supplementary Table S2 and Figure 5). The Thiothrix bin had genetic markers of the Calvin cycle (rbc genes) and reductive acetyl-CoA pathway (acyl-CoA synthetase). The reductive acetyl-CoA pathway contains O2-sensitive enzymes and is only used by anaerobic or microaerophilic microbes (Hügler and Sievert, 2011). The IS2 ISB bin contained key genes for the 3-hydroxypropionate bicycle (malonyl-CoA reductase, propionyl-CoA synthase and acetyl-CoA carboxylase) and the Calvin cycle (rbc). We also found numerous genes annotated as organic carbon transporters in these bins, including transporters for acetate and dicarboxylates. Also present were genes for acetate kinase and acetyl CoA synthetase, which could channel acetate into the general metabolism and are found in Beggiatoa genomes (Mußmann et al., 2007; MacGregor et al., 2013b; Flood et al., 2016; Winkel et al., 2016). Genes for oxidation of glycolate, a common photosynthetic exudate, were also found, suggesting an interaction with cyanobacteria (Mußmann et al., 2007). Although no cyanobacteria were detected at Isolated Sinkhole, close relatives of these LSB are tightly associated with cyanobacteria at the Middle Island Sinkhole (Voorhies et al., 2012). Alternatively, glycolate oxidases have been implicated in the consumption of glycolate produced through the oxygenase activity of RubisCO in LSB (Winkel et al., 2016). Many glycolate-related genes were also found in the Thiothrix genomic bin (Supplementary Table S2). Overall, these results are consistent with the LSB being capable of both autotrophy and heterotrophy, and perhaps mixotrophy.
Sulfate-Reducing Bacteria
Abundant members of both communities were found to have pathway for dissimilatory sulfate reduction via the sat, apr, and dsr genes (Supplementary Table S2). This suggests that in addition to sulfide present in the groundwater as it emerges from the subsurface, sulfide for the LSB could be sourced from the bacterial reduction of sulfate and/or intermediate sulfur species within the mat (Figure 5). The genomic bins that contained genes for sulfate reduction were from Deltaproteobacteria groups well known to reduce sulfate. In the Alpena fountain, the genome bin (A2) identified as Desulfobulbaceae was the only genomic bin with the entire pathway for reduction of sulfate to sulfide. In addition to producing sulfide via the Dsr pathway, genes for tetrathionate reductase and thiosulfate reductase suggest that sulfide can also be produced via alternative routes involving the reduction and disproportionation of sulfur intermediates (Figure 5). It also contained genes for the use of O2 as an electron acceptor, H2 as an electron donor, a wide variety of organic carbon substrates as a carbon source (Supplementary Table S2). This bin also had genes potentially encoding the reductive acetyl-CoA pathway (Wood-Ljungdahl) for carbon fixation (Supplementary Table S2). In addition to using this pathway for carbon fixation, it can also be run in reverse and coupled to sulfate reduction to generate energy (Ragsdale and Pierce, 2008). A partial bin of Desulfobacteraceae in the Alpena fountain (A6) also had the genes for sulfite and O2 reduction, H2 oxidation, carbon fixation, and heterotrophy, but it did not have the sat or apr genes for sulfate reduction. However, a near-complete genomic bin of Desulfobacteraceae in the Isolated Sinkhole (IS3) contained the complete pathway along with genes for disproportionation of thiosulfate and the same complement of genes for H2 oxidation, O2 reduction, carbon fixation, and heterotrophy (Figure 5). This bin also contained a 16S rRNA gene sequence that was 97% similar to a sequence from an uncultured deltaproteobacterium of the Frasassi Cave biofilms. This sequence clusters phylogenetically with Desulfonema and appears to be filamentous and to have close physical associations with Beggiatoa and Thiothrix (Macalady et al., 2006). Desulfobacteraceae and Desulfobulbaceae have also been observed at the Middle Island Sinkhole, in mat consortia of cyanobacteria and LSB (Nold et al., 2010; Kinsman-Costello et al., 2017). Both Deltaproteobacteria bins from the Alpena fountain (A2 and A6) had genes for cytochrome c nitrite reductase, which reduces nitrite to ammonium and is used by sulfate-reducing bacteria for detoxification of nitrite, often in association with sulfide-oxidizing, nitrate-reducing bacteria (Haveman et al., 2004). Bin IS3 also had the complete pathway for nitrate reduction to ammonium via the reverse hydroxylamine:ubiquinone reductase module pathway (Supplementary Table S2; Hanson et al., 2013).
The genome-predicted phenotypes of H2-based autotrophy, organoheterotrophy, respiration of O2 have all been observed in cultured sulfate-reducing bacteria including Desulfobulbaceae and Desulfobacteraceae (Kuever, 2014a,b). Ni-Fe hydrogenase oxidizes H2, which is an important electron donor for anaerobic sulfate-reducing bacteria such as the deltaproteobacterium Desulfovibrio (Eidsness et al., 1989). Genes for cytochrome oxidases may indicate that the sulfate-reducing bacteria can use oxygen as a terminal electron acceptor for carbon metabolism (Canfield and Des Marais, 1991). Generally this process is linked to ATP production, but not growth (Dilling and Cypionka, 1990; Dannenberg et al., 1992; Sass et al., 2002), although weak growth has been observed (Marschall et al., 1993; Sigalevich and Cohen, 2000).
SM1 Euryarchaeota
Whereas the Isolated Sinkhole metagenome was dominated by just the two LSB bins and one sulfate-reducing bacterium bin, the Alpena fountain featured additional near-complete genomes from other community members. The genomic bin from the SM1 euryarchaeon accounted for 71% of all mapped metagenomic reads from the fountain (Table 1). This lineage is widespread is sulfide springs (Rudolph et al., 2004) but its physiology and potential metabolism have been enigmatic (Probst et al., 2013). Based on close physical associations, syntrophic relationships with Thiothrix (Moissl et al., 2002) and sulfate reducing bacteria (Probst et al., 2013, 2014a) have been proposed. Recent genomic data derived from SM1 populations in flowing, oxygen-depleted groundwater in Germany and stratified suboxic/anoxic groundwater in the USA indicate that SM1 can fix carbon via a novel reductive acetyl-CoA (Wood-Ljungdahl) pathway, but no clear genetic evidence of electron donors or acceptors for energy metabolism was uncovered (Probst et al., 2014a). The Alpena fountain SM1 population also has key genes for the reductive acetyl-CoA pathway for carbon fixation, including acetyl-coenzyme A synthetase and CO dehydrogenase/acetyl-CoA synthease (Supplementary Table S2). We also found genes for a Ni,Fe-hydrogenase, suggesting an electron donor for lithotrophy, and genes putatively annotated as transporters for amino acids, polysaccharides, and C4-dicarboxylates, suggesting potential for heterotrophy as well (Figure 5 and Supplementary Table S2). Experimental studies are required to confirm these genome-generated hypotheses.
Other Community Members and Metabolisms
The next most abundant bin in the Alpena fountain was A7, which accounted for 14% of mapped metagenomic reads and was identified as a Rhodoferax species based on PhyloSift, 16S rRNA gene sequence, and co-binning with the R. ferrireducens genome (Figure 2). Consistent with the known metabolic versatility of R. ferrireducens (Risso et al., 2009; Yao et al., 2013), we found genes for transporters of organic compounds, sulfide and thiosulfate oxidation, aerobic respiration, dissimilatory nitrate reduction, and autotrophy (Supplementary Table S2). Other genomic bins (A1, A4, A5) were present at low relative abundance and the few genes of functional significance noted were related to uptake of organic carbon compounds (Supplementary Table S2).
Conclusion
Metagenomics revealed that the Alpena fountain and Isolated Sinkhole both held relatively low-diversity microbial mat communities supported by sulfur-based chemolithoautotrophy. While taxonomic composition was different, genes underpinning sulfur metabolism, oxygen utilization, carbon fixation, hydrogen oxidation, and denitrification were similar between the two sites. Differences in light availability and water depth do not appear to differentiate the dominant metabolisms of the two communities, but the higher rate of water flow in the Alpena fountain relative to the Isolated Sinkhole is likely a key determinant of which LSB are present. Hence availability of electron donors and acceptors, which is similar between the two sites, likely explains the similar overall metabolisms, while other environmental factors (light, flow) govern differences in richness and phylogenetic diversity. The phylogenetic affinity of Isolated Sinkhole LSB for marine taxa, both in terms of phylogeny and in similarity of introns within the 16S rRNA genes, reveal promising avenues for investigating the biogeography, evolution, and ecology of the Beggiatoaceae. Abundant and co-occurring sulfate-reducing organisms alongside the LSB suggest tightly coupled sulfur cycling, echoing microbial metabolic interactions that have been well documented in other environments. Likewise, the discovery that the SM1 euryarchaeon dominates biofilms and co-occurs with Thiothrix and sulfate-reducing bacteria in an accessible fountain just outside a city library highlights the potential of this site for studying the biology of this archaeon and its bacterial partners. Overall, this study provides new windows into the diversity and distribution of LSB and their associations with other bacteria as they occur in a lacustrine setting.
Author Contributions
GD, BB, and AS conceived of this study. GD, DM, BB, and SR collected the samples. AS, BF, JB, DJ, DM, and GD analyzed the data. All authors contributed to writing the manuscript.
Funding
This work was supported by NSF grants EAR-1637066 and EAR-1035955 to BB and GD and an Alfred P. Sloan Foundation Fellowship in Ocean Sciences to GD.
Conflict of Interest Statement
The authors declare that the research was conducted in the absence of any commercial or financial relationships that could be construed as a potential conflict of interest.
Acknowledgments
We thank the University of Michigan DNA Sequencing Core for DNA sequencing and Sunit Jain for bioinformatics assistance. We would also like to thank members of the sampling expedition including Tom Johengen (UM-CILER), Val Klump and Rob Paddock (UW-Milwaukee), who were responsible for ROV video and collections and provided Isolated Sinkhole environmental data, and the captain and crew of NOAA’s R/V Laurentian provided ship support.
Supplementary Material
The Supplementary Material for this article can be found online at: http://journal.frontiersin.org/article/10.3389/fmicb.2017.00791/full#supplementary-material
FIGURE S1 | Relative abundance of 16S genes as identified by a BLASTn of all reads against portions of scaffolds containing 16S genes found using the Silva119ssu database for (A) Isolated Sinkhole and (B) Alpena fountain communities.
FIGURE S2 | Maximum likelihood phylograms of full-length or nearly full-length (A) RpoB protein and (B) rpoB nucleotide sequences. The tree is rooted at the branch separating the Thiothrix clade from the other sequences. Numbers indicate bootstrap support for nodes (>50).
TABLE S1 | NCBI and IMG accession numbers for Alpena Fountain and Isolated Sinkhole metagenomes and individual bins.
TABLE S2 | Annotations and IMG locus tags for key metabolic genes identified across bins.
TABLE S3 | Key metabolic pathways and genes searched for across bins.
References
Abascal, F., Zardoya, R., and Posada, D. (2005). ProtTest: selection of best-fit models of protein evolution. Bioinformatics 21, 2104–2105. doi: 10.1093/bioinformatics/bti263
Ackert, L. T. (2006). The role of microbes in agriculture: Sergei Vinogradskii’s discovery and investigation of chemosynthesis, 1880-1910. J. Hist. Biol. 39, 373–406. doi: 10.1007/s10739-006-0008-2
Akegawa, J., Kobayahshi, K., and Ishimoto, M. (1985). Purification and properties of thiosulfate reductase. J. Biochem. 97, 1025–1032. doi: 10.1093/oxfordjournals.jbchem.a135144
Anantharaman, K., Breier, J. A., Sheik, C. S., and Dick, G. J. (2013). Evidence for hydrogen oxidation and metabolic plasticity in widespread deep-sea sulfur-oxidizing bacteria. Proc. Natl. Acad. Sci. U.S.A. 110, 330–335. doi: 10.1073/pnas.1215340110
Aragno, M. (1992). Thermophilic, Aerobic, Hydrogen-Oxidizing (Knallgas) Bacteria. The Prokaryotes. Berlin: Springer, 3917–3933. doi: 10.1007/978-1-4757-2191-1_55
Ashelford, K. E., Chuzhanova, N. A., Fry, J. C., Jones, A. J., and Weightman, A. J. (2005). At least 1 in 20 16S rRNA sequence records currently held in public repositories is estimated to contain substantial anomalies. Appl. Environ. Microbiol. 71, 7724–7736. doi: 10.1128/AEM.71.12.7724-7736.2005
Berg, I. A., Kockelkorn, D., Ramos-Vera, W. H., Say, R. F., Zarzycki, J., Hügler, M., et al. (2010). Autotrophic carbon fixation in archaea. Nat. Rev. Microbiol. 8, 447–460. doi: 10.1038/nrmicro2365
Biddanda, B. A., Coleman, D. F., Johengen, T. H., Ruberg, S. A., Meadows, G. A., Van Sumeren, H. W., et al. (2006). Exploration of a submerged sinkhole ecosystem in Lake Huron. Ecosystems 9, 828–842. doi: 10.1007/s10021-005-0057-y
Biddanda, B. A., Nold, S. C., Dick, G. J., Kendall, S. T., Vail, J. H., Ruberg, S. A., et al. (2012). Rock, water, microbes: underwater sinkholes in Lake Huron are habitats for ancient microbial life. Nat. Educ. Knowl. 2, 9.
Caldwell, D. E., Caldwell, S. J., and Tiedje, J. M. (1975). An ecological study of the sulfur- oxidizing bacteria from the littoral zone of a Michigan lake and a sulfur spring in Florida. Plant Soil 43, 101–114. doi: 10.1007/BF01928479
Canfield, D. E., and Des Marais, D. J. (1991). Aerobic sulfate reduction in microbial mats. Science 251, 1471–1473. doi: 10.1126/science.11538266
Chaudhary, A., Haack, S. K., Duris, J. W., and Marsh, T. L. (2009). Bacterial and archaeal phylogenetic diversity of a cold sulfur-rich spring on the shoreline of Lake Erie, Michigan. Appl. Environ. Microbiol. 75, 5025–5036. doi: 10.1128/AEM.00112-09
Chernousova, E., Gridneva, E., Grabovich, M., Dubinina, G., Akimov, V., Rossetti, S., et al. (2009). Thiothrix caldifontis sp. nov. and Thiothrix lacustris sp. nov., gammaproteobacteria isolated from sulfide springs. Int. J. Syst. Evol. Microbiol. 59, 3128–3135. doi: 10.1099/ijs.0.009456-0
Ciccarelli, F. D., Doerks, T., Von Mering, C., Creevey, C. J., Snel, B., and Bork, P. (2006). Toward automatic reconstruction of a highly resolved tree of life. Science 311, 1283–1287. doi: 10.1126/science.1123061
Cline, J. D. (1969). Spectrophotometric determination of hydrogen sulfide in natural waters. Limnol. Oceanogr. 14, 454–458. doi: 10.4319/lo.1969.14.3.0454
Coleman, D. F. (2002). Underwater archaeology in thunder bay national marine sanctuary, lake huron—preliminary results from a shipwreck mapping survey. Mar. Technol. Soc. J. 36, 33–44. doi: 10.4031/002533202787913387
Dannenberg, S., Kroder, M., Dilling, W., and Cypionka, H. (1992). Oxidation of H2, organic compounds and inorganic sulfur compounds coupled to reduction of O2 or nitrate by sulfate-reducing bacteria. Arch. Microbiol. 158, 93–99. doi: 10.1007/BF00245211
Darling, A. E., Jospin, G., Lowe, E., Matsen, F. A. IV, Bik, H. M., and Eisen, J. A. (2014). PhyloSift: phylogenetic analysis of genomes and metagenomes. PeerJ 2:e243. doi: 10.7717/peerj.243
Darriba, D., Taboada, G. L., Doallo, R., and Posada, D. (2012). jModelTest 2: more models, new heuristics and parallel computing. Nat. Methods 9:772. doi: 10.1038/nmeth.2109
Dick, G. J., Andersson, A. F., Baker, B. J., Simmons, S. L., Thomas, B. C., Yelton, A. P., et al. (2009). Community-wide analysis of microbial genome sequence signatures. Genome Biol. 10:R85. doi: 10.1186/gb-2009-10-8-r85
Dilling, W., and Cypionka, H. (1990). Aerobic respiration in sulfate-reducing bacteria. FEMS Microbiol. Lett. 71, 123–127. doi: 10.1111/j.1574-6968.1990.tb03809.x
Eidsness, M. K., Scott, R. A., Prickril, B. C., DerVartanian, D. V., Legall, J., Moura, I., et al. (1989). Evidence for selenocysteine coordination to the active site nickel in the [NiFeSe] hydrogenases from Desulfovibrio baculatus. Proc. Natl. Acad. Sci. U.S.A. 86, 147–151. doi: 10.1073/pnas.86.1.147
Engel, A. S., Porter, M. L., Kinkle, B. K., and Kane, T. C. (2001). Ecological assessment and geological significance of microbial communities from Cesspool Cave, Virginia. Geomicrobiol. J. 18, 259–274. doi: 10.1080/01490450152467787
Felsenstein, J. (1985). Confidence limits on phylogenies: an approach using the bootstrap. Evolution 39, 783–791. doi: 10.2307/2408678
Ferrer, M., Guazzaroni, M.-E., Richter, M., García-Salamanca, A., Yarza, P., Suárez-Suárez, A., et al. (2011). Taxonomic and functional metagenomic profiling of the microbial community in the anoxic sediment of a sub-saline shallow lake (Laguna de Carrizo, Central Spain). Microb. Ecol. 62, 824–837. doi: 10.1007/s00248-011-9903-y
Finneran, K. T., Johnsen, C. V., and Lovley, D. R. (2003). Rhodoferax ferrireducens sp. nov., a psychrotolerant, facultatively anaerobic bacterium that oxidizes acetate with the reduction of Fe (III). Int. J. Syst. Evol. Microbiol. 53, 669–673. doi: 10.1099/ijs.0.02298-0
Fitch, W. M. (1971). Toward defining the course of evolution: minimum change for a specific tree topology. Syst. Zool. 20, 406–416. doi: 10.2307/2412116
Flood, B. E., Fliss, P., Jones, D. S., Dick, G. J., Jain, S., Kaster, A.-K., et al. (2016). Single-cell (meta-)genomics of a dimorphic Candidatus Thiomargarita nelsonii reveals genomic plasticity. Front. Microbiol. 7:603. doi: 10.3389/fmicb.2016.00603
Garrity, G. M., Bell, J. A., and Lilburn, T. (2005). “Family I. Thiotrichaceae fam. Nov,” in Bergey’s Manual of Systematic Bacteriology, Part B, Vol. 2, eds G. M. Garrity, D. J. Brenner, N. R. Krieg, and J. T. Staley (New York, NY: Springer), 131–179. doi: 10.1007/0-387-28022-7_5
Girnth, A. C., Grünke, S., Lichtschlag, A., Felden, J., Knittel, K., Wenzhöfer, F., et al. (2011). A novel, mat-forming Thiomargarita population associated with a sulfidic fluid flow from a deep-sea mud volcano. Environ. Microbiol. 13, 495–505. doi: 10.1111/j.1462-2920.2010.02353.x
Gray, C. J., and Engel, A. S. (2013). Microbial diversity and impact on carbonate geochemistry across a changing geochemical gradient in a karst aquifer. ISME J. 7, 325–337. doi: 10.1038/ismej.2012.105
Greening, C., Biswas, A., Carere, C. R., Jackson, C. J., Taylor, M. C., Stott, M. B., et al. (2016). Genomic and metagenomic surveys of hydrogenase distribution indicate H2 is a widely utilised energy source for microbial growth and survival. ISME J. 10, 761–777. doi: 10.1038/ismej.2015.153
Hamilton, T. L., Jones, D. S., Schaperdoth, I., and Macalady, J. L. (2015). Metagenomic insights into S(0) precipitation in a terrestrial subsurface lithoautotrophic ecosystem. Front. Microbiol. 5:756. doi: 10.3389/fmicb.2014.00756
Hanson, T. E., Campbell, B. J., Kalis, K. M., Campbell, M. A., and Klotz, M. G. (2013). Nitrate ammonification by Nautilia profundicola AmH: experimental evidence consistent with a free hydroxylamine intermediate. Front. Microbiol. 4:180. doi: 10.3389/fmicb.2013.00180
Harris, J. K., Kelley, S. T., and Pace, N. R. (2004). New perspective on uncultured bacterial phylogenetic division OP11. Appl. Environ. Microbiol. 70, 845–849. doi: 10.1128/AEM.70.2.845-849.2004
Haveman, S. A., Greene, E. A., Stilwell, C. P., Voordouw, J. K., and Voordouw, G. (2004). Physiological and gene expression analysis of inhibitition of Desulfovibrio vulgaris Hildenborough by nitrite. J. Bacteriol. 186, 7944–7950. doi: 10.1128/JB.186.23.7944-7950.2004
Headd, B., and Engel, A. S. (2014). Biogeographic congruency among bacterial communities from terrestrial sulfidic springs. Front. Microbiol. 5:473. doi: 10.3389/fmicb.2014.00473
Hinck, S., Mußmann, M., Salman, V., Neu, T. R., Lenk, S., de Beer, D., et al. (2011). Vacuolated Beggiatoa-like filaments from different hypersaline environments form a novel genus. Environ. Microbiol. 13, 3194–3205. doi: 10.1111/j.1462-2920.2011.02513.x
Huelsenbeck, J. P., and Ronquist, F. (2001). MRBAYES: Bayesian inference of phylogenetic trees. Bioinformatics 17, 754–755. doi: 10.1093/bioinformatics/17.8.754
Hügler, M., and Sievert, S. M. (2011). Beyond the calvin cycle: autotrophic carbon fixation in the ocean. Mar. Sci. 3, 261–289. doi: 10.1146/annurev-marine-120709-142712
Jannasch, H. W., and Wirsen, C. O. (1979). Chemosynthetic primary production at East Pacific sea floor spreading centers. Bioscience 29, 592–598. doi: 10.2307/1307765
Jones, D. S., Flood, B. E., and Bailey, J. V. (2015). Metatranscriptomic analysis of diminutive Thiomargarita-like bacteria (“Candidatus Thiopilula” spp.) from abyssal cold seeps of the Barbados accretionary prism. Appl. Environ. Microbiol. 81, 3142–3156. doi: 10.1128/AEM.00039-15
Jones, D. S., Tobler, D. J., Schaperdoth, I., Mainiero, M., and Macalady, J. L. (2010). Community structure of subsurface biofilms in the thermal sulfidic caves of Acquasanta Terme, Italy. Appl. Environ. Microbiol. 76, 5902–5910. doi: 10.1128/AEM.00647-10
Jørgensen, B. B., and Bak, F. (1991). Pathways and microbiology of thiosulfate transformations and sulfate reduction in a marine sediment (Kattegat, Denmark). Appl. Environ. Microbiol. 57, 847–856.
Joshi, N. A., and Fass, J. N. (2011). Sickle: A Sliding-Window, Adaptive, Quality-Based Trimming Tool for FastQ files (Version 1.33) [Software]. Available at https://github.com/najoshi/sickle.
Joye, S. B., Boetius, A., Orcutt, B. N., Montoya, J. P., Schulz, H. N., Erickson, M. J., et al. (2004). The anaerobic oxidation of methane and sulfate reduction in sediments from Gulf of Mexico cold seeps. Chem. Geol. 205, 219–238. doi: 10.1016/j.chemgeo.2003.12.019
Jukes, T. H., and Cantor, C. R. (1969). “Evolution of protein molecules,” in Mammalian Protein Metabolism, ed. H. N. Munro (New York, NY: Academic Press), 21–132. doi: 10.1016/b978-1-4832-3211-9.50009-7
Kearse, M., Moir, R., Wilson, A., Stones-Havas, S., Cheung, M., Sturrock, S., et al. (2012). Geneious Basic: an integrated and extendable desktop software platform for the organization and analysis of sequence data. Bioinformatics 28, 1647–1649. doi: 10.1093/bioinformatics/bts199
Kinsman-Costello, L. E., Sheik, C. S., Sheldon, N. D., Burton, G. A., Costello, D. M, Marcus, D. N., et al. (2017). Groundwater shapes sediment biogeochemistry and microbial diversity in a submerged Great Lake sinkhole. Geobiology 15, 225–239. doi: 10.1111/gbi.12215
Klatt, J. M., and Polerecky, L. (2015). Assessment of the stoichiometry and efficiency of CO2 fixation coupled to reduced sulfur oxidation. Front. Microbiol. 6:484. doi: 10.3389/fmicb.2015.00484
Konkol, N. R., Bruckner, J. C., Aguilar, C., Lovalvo, D., and Maki, J. S. (2010). Dominance of epiphytic filamentous Thiothrix spp. on an aquatic macrophyte in a hydrothermal vent flume in Sedge Bay, Yellowstone Lake, Wyoming. Microb. Ecol. 60, 528–538. doi: 10.1007/s00248-010-9656-z
Kreutzmann, A.-C., and Schulz-Vogt, H. N. (2016). Oxidation of molecular hydrogen by a chemolithoautotrophic Beggiatoa strain. Appl. Environ. Microbiol. 82, 2527–2536. doi: 10.1128/AEM.03818-15
Kuever, J. (2014a). “The family Desulfobacteraceae,” in The Prokaryotes, eds E. Rosenberg, E. F. DeLong, S. Lory, E. Stackebrandt, and F. Thompson (Berlin: Springer), 45–73.
Kuever, J. (2014b). “The family Desulfobulbacaea,” in The Prokaryotes, eds E. Rosenberg, E. F. DeLong, S. Lory, E. Stackebrandt, and F. Thompson (Berlin: Springer), 75–86.
Larkin, J., Henk, M. C., and Aharon, P. (1994). Beggiatoa in microbial mats at hydrocarbon vents in the Gulf of Mexico and Warm Mineral Springs, Florida. Geo Mar. Lett. 14, 97–103. doi: 10.1007/BF01203720
Larkin, J. M., and Strohl, W. R. (1983). Beggiatoa, Thiothrix, and Thioploca. Annu. Rev. Microbiol. 37, 341–367. doi: 10.1146/annurev.mi.37.100183.002013
Le, S. Q., and Gascuel, O. (2008). An improved general amino acid replacement matrix. Mol. Biol. Evol. 25, 1307–1320. doi: 10.1093/molbev/msn067
Li, H., and Durbin, R. (2009). Fast and accurate short read alignment with burrows- wheeler transform. Bioinformatics 25, 1754–1760. doi: 10.1093/bioinformatics/btp324
López-Archilla, A. I., Moreira, D., Velasco, S., and López-Garca, P. (2007). Archaeal and bacterial community composition of a pristine coastal aquifer in Donana National Park, Spain. Aquat. Microb. Ecol. 47, 123–139. doi: 10.3354/ame047123
Ludwig, W., Strunk, O., Westram, R., Richter, L., Meier, H., Yadhukumar, et al. (2004). ARB: a software environment for sequence data. Nucleic Acids Res. 32, 1363–1371. doi: 10.1093/nar/gkh293
Macalady, J. L., Dattagupta, S., Schaperdoth, I., Jones, D. S., Druschel, G. K., and Eastman, D. (2008). Niche differentiation among sulfur-oxidizing bacterial populations in cave waters. ISME J. 2, 590–601. doi: 10.1038/ismej.2008.25
Macalady, J. L., Lyon, E. H., Koffman, B., Albertson, L. K., Meyer, K., Galdenzi, S., et al. (2006). Dominant microbial populations in limestone-corroding stream biofilms, Frasassi cave system, Italy. Appl. Environ. Microbiol. 72, 5596–5609. doi: 10.1128/AEM.00715-06
MacGregor, B. J., Biddle, J. F., and Teske, A. (2013a). Mobile elements in a single-filament Orange Guaymas Basin Beggiatoa (“Candidatus Maribeggiatoa”) sp. draft genome: Evidence for genetic exchange with Cyanobacteria. Appl. Environ. Microbiol. 79, 3974–3985. doi: 10.1128/AEM.03821-12
MacGregor, B. J., Biddle, J. F., Harbort, C., Matthysse, A. G., and Teske, A. (2013b). Sulfide oxidation, nitrate respiration, carbon acquisition, and electron transport pathways suggested by the draft genome of a single orange Guaymas Basin Beggiatoa (Cand. Maribeggiatoa) sp. filament. Mar. Genomics 11, 53–65. doi: 10.1016/j.margen.2013.08.001
Markowitz, V. M., Ivanova, N. N., Szeto, E., Palaniappan, K., Chu, K., Dalevi, D., et al. (2008). IMG/M: a data management and analysis system for metagenomes. Nucleic Acids Res. 36(Suppl. 1), D534–D538.
Marschall, C., Frenzel, P., and Cypionka, H. (1993). Influence of oxygen on sulfate reduction and growth of sulfate-reducing bacteria. Arch. Microbiol. 159, 168–173. doi: 10.1007/BF00250278
McKay, L. J., MacGregor, B. J., Biddle, J. F., Albert, D. B., Mendlovitz, H. P., Hoer, D. R., et al. (2012). Spatial heterogeneity and underlying geochemistry of phylogenetically diverse orange and white Beggiatoa mats in Guaymas Basin hydrothermal sediments. Deep Sea Res. 67, 21–31. doi: 10.1016/j.dsr.2012.04.011
Miller, C. S., Baker, B. J., Thomas, B. C., Singer, S. W., and Banfield, J. F. (2011). EMIRGE: reconstruction of full-length ribosomal genes from microbial community short read sequencing data. Genome Biol. 12:R44. doi: 10.1186/gb-2011-12-5-r44
Moissl, C., Rachel, R., Briegel, A., Engelhardt, H., and Huber, R. (2005). The unique structure of archaeal ‘hami’, highly complex cell appendages with nano-grappling hooks. Mol. Microbiol. 56, 361–370. doi: 10.1111/j.1365-2958.2005.04294.x
Moissl, C., Rudolph, C., and Huber, R. (2002). Natural communities of novel archaea and bacteria with a string-of-pearls-like morphology: molecular analysis of the bacterial partners. Appl. Environ. Microbiol. 68, 933–937. doi: 10.1128/AEM.68.2.933-937.2002
Moissl, C., Rudolph, C., Rachel, R., Koch, M., and Huber, R. (2003). In situ growth of the novel SM1 euryarchaeon from a string-of-pearls-like microbial community in its cold biotope, its physical separation and insights into its structure and physiology. Arch. Microbiol. 180, 211–217. doi: 10.1007/s00203-003-0580-1
Moretti, S., Armougom, F., Wallace, I. M., Higgins, D. G., Jongeneel, C. V., and Notredame, C. (2007). The M-Coffee web server: a meta-method for computing multiple sequence alignments by combining alternative alignment methods. Nucleic Acids Res. 35(Suppl. 2), W645–W648. doi: 10.1093/nar/gkm333
Morris, R. L., and Schmidt, T. M. (2013). Shallow breathing: bacterial life at low O2. Nat. Rev. Microbiol. 11, 205–212. doi: 10.1038/nrmicro2970
Mußmann, M., Hu, F. Z., Richter, M., de Beer, D., Preisler, A., Jørgensen, B. B., et al. (2007). Insights into the genome of large sulfur bacteria revealed by analysis of single filaments. PLoS Biol. 5:e230. doi: 10.1371/journal.pbio.0050230
Nelson, D. C., and Castenholz, R. W. (1981). Organic nutrition of Beggiatoa sp. J. Bacteriol. 147, 236–247.
Nelson, D. C., and Jannasch, H. W. (1983). Chemoautotrophic growth of a marine Beggiatoa in sulfide-gradient cultures. Arch. Microbiol. 136, 262–269. doi: 10.1007/BF00425214
Nelson, D. C., Jørgensen, B. B., and Revsbech, N. P. (1986a). Growth pattern and yield of a chemoautotrophic Beggiatoa sp. in oxygen-sulfide microgradients. Appl. Environ. Microbiol. 52, 225–233.
Nelson, D. C., Revsbech, N. P., and Jørgensen, B. B. (1986b). Microoxic-anoxic niche of Beggiatoa spp.: microelectrode survey of marine and freshwater strains. Appl. Environ. Microbiol. 52, 161–168.
Nold, S. C., Pangborn, J. B., Zajack, H. A., Kendall, S. T., Rediske, R. R., and Biddanda, B. A. (2010). Benthic bacterial diversity in submerged sinkhole ecosystems. Appl. Environ. Microbiol. 76, 347–351. doi: 10.1128/AEM.01186-09
Peng, Y., Leung, H. C. M., Yiu, S. M., and Chin, F. Y. L. (2012). IDBA-UD: a de novo assembler for single-cell and metagenomic sequencing data with highly uneven depth. Bioinformatics 28, 1420–1428. doi: 10.1093/bioinformatics/bts174
Perras, A. K., Wanner, G., Klingl, A., Mora, M., Auerbah, A. K., Heinz, V., et al. (2014). Grappling archaea: ultrastructural analyses of an uncultivated, cold-loving archaeon, and its biofilm. Front. Microbiol. 5:397. doi: 10.3389/fmicb.2014.00397
Peura, S., Eiler, A., Bertilsson, S., Nykänen, H., Tiirola, M., and Jones, R. I. (2012). Distinct and diverse anaerobic bacterial communities in boreal lakes dominated by candidate division OD1. ISME J. 6, 1640–1652. doi: 10.1038/ismej.2012.21
Philippot, L. (2002). Denitrifying genes in bacterial and archaeal genomes. Biochim. Biophys. Acta 1577, 355–376. doi: 10.1016/S0167-4781(02)00420-7
Probst, A. J., Birarda, G., Holman, H. Y. N., DeSantis, T. Z., Wanner, G., Andersen, G. L., et al. (2014a). Coupling genetic and chemical microbiome profiling reveals heterogeneity of archaeome and bacteriome in subsurface biofilms that are dominated by the same archaeal species. PLoS ONE 9:e99801. doi: 10.1371/journal.pone.0099801
Probst, A. J., Weinmaier, T., Raymann, K., Perras, A., Emerson, J. B., Rattei, T., et al. (2014b). Biology of a widespread uncultivated archaeon that contributes to carbon fixation in the subsurface. Nat. Commun. 5:5497. doi: 10.1038/ncomms6497
Probst, A. J., Holman, H. N., DeSantis, T. Z., Andersen, G. L., Birarda, G., Bechtel, H. A., et al. (2013). Tackling the minority: sulfate-reducing bacteria in an archaea-dominated subsurface biofilm. ISME J. 7, 635–651. doi: 10.1038/ismej.2012.133
Pruesse, E., Peplies, J., and Glöckner, F. O. (2012). SINA: accurate high-throughput multiple sequence alignment of ribosomal RNA genes. Bioinformatics 28, 1823–1829. doi: 10.1093/bioinformatics/bts252
Pruesse, E., Quast, C., Knittel, K., Fuchs, B. M., Ludwig, W., Peplies, J., et al. (2007). SILVA: a comprehensive online resource for quality checked and aligned ribosomal RNA sequence data compatible with ARB. Nucleic Acids Res. 35, 7188–7196. doi: 10.1093/nar/gkm864
Pruitt, K. D., Tatusova, T., and Maglott, D. R. (2005). NCBI Reference Sequence (RefSeq): a curated non-redundant sequence database of genomes, transcripts and proteins. Nucleic Acids Res. 33, D501–D504. doi: 10.1093/nar/gki025
Quast, C., Pruesse, E., Yilmz, P., Gerken, J., Schweer, T., Yarza, P., et al. (2013). The Silva ribosomal RNA gene database project: improved data processing and web-based tools. Nucleic Acids Res. 41, D590–D596. doi: 10.1093/nar/gks1219
Ragsdale, S. W., and Pierce, E. (2008). Acetogenesis and the Wood–Ljungdahl pathway of CO2 fixation. Biochim. Biophys. Acta 1784, 1873–1898. doi: 10.1016/j.bbapap.2008.08.012
Reese, B. K., Finneran, D. W., Mills, H. J., Zhu, M., and Morse, J. W. (2011). Examination and refinement of the determination of aqueous hydrogen sulfide by the methylene blue method. Aquat. Geochem. 17, 567–582. doi: 10.1007/s10498-011-9128-1
Rinke, C., Schwientek, P., Sczyrba, A., Ivanova, N. N., Anderson, I. J., Cheng, J. F., et al. (2013). Insights into the phylogeny and coding potential of microbial dark matter. Nature 499, 431–437. doi: 10.1038/nature12352
Risso, C., Sun, J., Zhuang, K., Mahadevan, R., DeBoy, R., Ismail, W., et al. (2009). Genome-scale comparison and constraint-based metabolic reconstruction of the facultative anaerobic Fe (III)-reducer Rhodoferax ferrireducens. BMC Genomics 10:447. doi: 10.1186/1471-2164-10-447
Ruberg, S. A., Kendall, S. T., Biddanda, B. A., Black, T., Nold, S. C., Lusardi, W. R., et al. (2008). Observations of the middle island sinkhole in lake huron–a unique hydrogeologic and glacial creation of 400 million years. Mar. Technol. Soc. J. 42, 12–21. doi: 10.4031/002533208787157633
Rudolph, C., Moissl, C., Henneberger, R., and Huber, R. (2004). Ecology and microbial structures of archaeal/bacterial strings-of-pearls communities and archaeal relatives thriving in cold sulfidic springs. FEMS Microbiol. Ecol. 50, 1–11. doi: 10.1016/j.femsec.2004.05.006
Rudolph, C., Wanner, G., and Huber, R. (2001). Natural communities of novel archaea and bacteria growing in cold sulfurous springs with a string-of-pearls-like morphology. Appl. Environ. Microbiol. 67, 2336–2344. doi: 10.1128/AEM.67.5.2336-2344.2001
Saitou, N., and Nei, M. (1987). The neighbor-joining method: a new method for reconstructing phylogenetic trees. Mol. Biol. Evol. 4, 406–425.
Salman, V., Amann, R., Girnth, A. C., Polerecky, L., Bailey, J. V., Høgslund, S., et al. (2011). A single-cell sequencing approach to the classification of large, vacuolated sulfur bacteria. Syst. Appl. Microbiol. 34, 243–259. doi: 10.1016/j.syapm.2011.02.001
Salman, V., Amann, R., Shub, D. A., and Schulz-Vogt, H. N. (2012). Multiple self-splicing introns in the 16S rRNA genes of giant sulfur bacteria. Proc. Natl. Acad. Sci. U.S.A. 109, 4203–4208. doi: 10.1073/pnas.1120192109
Salman, V., Bailey, J. V., and Teske, A. (2013). Phylogenetic and morphologic complexity of giant sulphur bacteria. Antonie Van Leeuwenhoek 104, 169–186. doi: 10.1007/s10482-013-9952-y
Sass, A., Rütters, H., Cypionka, H., and Sass, H. (2002). Desulfobulbus mediterraneus sp. nov., a sulfate-reducing bacterium growing on mono-and disaccharides. Arch. Microbiol. 177, 468–474. doi: 10.1007/s00203-002-0415-5
Schloss, P. D., Westcott, S. L., Ryabin, T., Hall, J. R., Hartmann, M., Hollister, E. B., et al. (2009). Introducing mothur: open-source, platform-independent, community-supported software for describing and comparing microbial communities. Appl. Environ. Microbiol. 75, 7537–7541. doi: 10.1128/AEM.01541-09
Schmidt, T. M., Arieli, B., Cohen, Y., Padan, E., and Strohl, W. R. (1987). Sulfur metabolism in Beggiatoa alba. J. Bacteriol. 169, 5466–5472. doi: 10.1128/jb.169.12.5466-5472.1987
Sigalevich, P., and Cohen, Y. (2000). Oxygen-dependent growth of the sulfate-reducing bacterium Desulfovirbrio oxyclinae in coculture with Marinobacter sp. Strain MB in an aerated sulfate-depleted chemostat. Appl. Environ. Microbiol. 66, 5019–5023. doi: 10.1128/AEM.66.11.5019-5023.2000
Snider, M. J., Biddanda, B. A., Lindback, M., Grim, S. L., and Dick, G. J. (2017). Versatile photophysiology of compositionally similar cyanobacterial mat communities inhabiting submerged sinkholes of Lake Huron. Aquat. Microb. Ecol. 79, 63–78. doi: 10.3354/ame01813
Stamatakis, A. (2006). RAxML-VI-HPC: maximum likelihood-based phylogenetic analyses with thousands of taxa and mixed models. Bioinformatics 22, 2688–2690. doi: 10.1093/bioinformatics/btl446
Stoffels, L., Krehenbrink, M., Berks, B. C., and Unden, G. (2012). Thiosulfate reduction in Salmonella enterica is driven by the proton motive force. J. Bacteriol. 194, 475–485. doi: 10.1128/JB.06014-11
Swofford, D. L. (2003). Phylogenetic Analysis Using Parsimony (∗and Other Methods). Version 4.b10. Sunderland, MA: Sinauer Associates.
Teske, A., and Nelson, D. C. (2006). The Genera Beggiatoa and Thioploca The prokaryotes. Berlin: Springer, 784–810. doi: 10.1007/0-387-30746-X_27
Teske, A. P. (2006). Microbial communities of deep marine subsurface sediments: molecular and cultivation surveys. Geomicrobiol. J. 23, 357–368. doi: 10.1080/01490450600875613
Trubitsyn, I. V., Andreevskikh, Z. G., Yurevich, L. I., Belousova, E. V., Tutukina, M. N., Merkel, A. Y., et al. (2013). Capacity for nitrate respiration as a new aspect of metabolism of the filamentous sulfur bacteria of the genus Thiothrix. Microbiology 82, 15–21. doi: 10.1134/S0026261713010153
Voorhies, A. A., Biddanda, B. A., Kendall, S. T., Jain, S., Marcus, D. N., Nold, S. C., et al. (2012). Cyanobacterial life at low O2: community genomics and function reveal metabolic versatility and extremely low diversity in a Great Lakes sinkhole mat. Geobiology 10, 250–267. doi: 10.1111/j.1472-4669.2012.00322.x
Winkel, M., Carvalho, V., Woyke, T., Richter, M., Schulz-Vogt, H. N., Flood, B., et al. (2016). Single-cell sequencing of Thiomargarita reveals genomic flexibility for adaptation to dynamic redox conditions. Front. Microbiol. 7:964. doi: 10.3389/fmicb.2016.00964
Yao, S., Ni, J., Chen, Q., and Borthwick, A. G. (2013). Enrichment and characterization of a bacteria consortium capable of heterotrophic nitrification and aerobic denitrification at low temperature. Bioresour. Technol. 127, 151–157. doi: 10.1016/j.biortech.2012.09.098
Yarza, P., Yilmaz, P., Pruesse, E., Glöckner, F. O., Ludwig, W., Schleifer, K. H., et al. (2014). Uniting the classification of cultured and uncultured bacteria and archaea using 16S rRNA gene sequences. Nat. Rev. Microbiol. 12, 635–645. doi: 10.1038/nrmicro3330
Keywords: chemosynthesis, dissimilatory sulfate reduction, sulfur oxidation, archaea, archaeal genomics, microbial mat, Beggiatoa, Thiothrix
Citation: Sharrar AM, Flood BE, Bailey JV, Jones DS, Biddanda BA, Ruberg SA, Marcus DN and Dick GJ (2017) Novel Large Sulfur Bacteria in the Metagenomes of Groundwater-Fed Chemosynthetic Microbial Mats in the Lake Huron Basin. Front. Microbiol. 8:791. doi: 10.3389/fmicb.2017.00791
Received: 17 August 2016; Accepted: 18 April 2017;
Published: 08 May 2017.
Edited by:
Andreas Teske, University of North Carolina at Chapel Hill, USAReviewed by:
Barbara J. Campbell, Clemson University, USAVirginia P. Edgcomb, Woods Hole Oceanographic Institution, USA
Copyright © 2017 Sharrar, Flood, Bailey, Jones, Biddanda, Ruberg, Marcus and Dick. This is an open-access article distributed under the terms of the Creative Commons Attribution License (CC BY). The use, distribution or reproduction in other forums is permitted, provided the original author(s) or licensor are credited and that the original publication in this journal is cited, in accordance with accepted academic practice. No use, distribution or reproduction is permitted which does not comply with these terms.
*Correspondence: Gregory J. Dick, Z2RpY2tAdW1pY2guZWR1