- 1Área de Microbiología, Departamento de Ciencias de la Salud, Facultad de Ciencias Experimentales, Universidad de Jaén, Jaén, Spain
- 2Centro Andaluz de Biología del Desarrollo – Consejo Superior de Investigaciones Cientificas, Universidad Pablo de Olavide, Sevilla, Spain
- 3Department of Civil and Environmental Engineering, University of Strathclyde, Glasgow, United Kingdom
- 4Área de Estadística e Investigación Operativa, Departamento de Estadística e Investigación Operativa, Facultad de Ciencias Experimentales, Universidad de Jaén, Jaén, Spain
Lactobacillus pentosus MP-10 is a potential probiotic lactic acid bacterium originally isolated from naturally fermented Aloreña green table olives. The entire genome sequence was annotated to in silico analyze the molecular mechanisms involved in the adaptation of L. pentosus MP-10 to the human gastrointestinal tract (GIT), such as carbohydrate metabolism (related with prebiotic utilization) and the proteins involved in bacteria–host interactions. We predicted an arsenal of genes coding for carbohydrate-modifying enzymes to modify oligo- and polysaccharides, such as glycoside hydrolases, glycoside transferases, and isomerases, and other enzymes involved in complex carbohydrate metabolism especially starch, raffinose, and levan. These enzymes represent key indicators of the bacteria’s adaptation to the GIT environment, since they involve the metabolism and assimilation of complex carbohydrates not digested by human enzymes. We also detected key probiotic ligands (surface proteins, excreted or secreted proteins) involved in the adhesion to host cells such as adhesion to mucus, epithelial cells or extracellular matrix, and plasma components; also, moonlighting proteins or multifunctional proteins were found that could be involved in adhesion to epithelial cells and/or extracellular matrix proteins and also affect host immunomodulation. In silico analysis of the genome sequence of L. pentosus MP-10 is an important initial step to screen for genes encoding for proteins that may provide probiotic features, and thus provides one new routes for screening and studying this potentially probiotic bacterium.
Introduction
The Lactobacillus genus belongs to the LAB group, which currently comprises of 222 species described in List of Prokaryotic Names with Standing in Nomenclature “LPSN”1 (February 2017). In this context, Lactobacillus represents a highly heterogeneous taxonomic group encompassing species with various physiological, biochemical and genetic characteristics that reflect their capacity to colonize many ecological niches and to respond to several environmental stresses (De Angelis and Gobbetti, 2004; Pot et al., 2014). Lactobacilli have been isolated from different sources [e.g., plants, foods, and the mucosal surfaces (i.e., from oral, gastrointestinal, and reproductive tracts) of mammalian hosts], and they have widely been used as starter cultures in food fermentations, due to their safe-history of use, and also as protective cultures because of their production of antimicrobial substances (e.g., bacteriocins, peroxide, diacetyl, among others) (Leroy and de Vuyst, 1999; Heller, 2001; Hansen, 2002; Holzapfel, 2002; Giraffa et al., 2010; Franz et al., 2011; Garrigues et al., 2013). Thus, the Food and Drug Administration and European Food Safety Authority certify some Lactobacillus species as Generally Recognized As Safe (GRAS) or having a Qualified Presumption of Safety (QPS), respectively (Bernardeau et al., 2008). Furthermore, many Lactobacillus species represent main components of the global probiotic market: L. acidophilus, L. bulgaricus, L. plantarum, L. brevis, L. reuteri, L. johnsonii, L. casei, L. rhamnosus, and L. salivarius. Specifically, some L. pentosus strains have exerted probiotic effects such as the acceleration of IgA secretion in saliva and the enhancement of IgA production in the small intestine (Kotani et al., 2010; Izumo et al., 2011), which have aroused great interest due to vegetal origin (Pérez Montoro et al., 2016). Generic mechanisms for underlying probiotic effects can be linked to taxonomic groups (genus or species); however, specific mechanisms tend to be strain-specific (Hill et al., 2014). As such, whole genome sequencing (WGS) remains the best way to better understand the genetic and metabolic potential of each species/strain, to demonstrate the plasticity of their phylogenetic relationships, metabolic pathways, adaptation, fitness and safety (Jolley and Maiden, 2010; Maiden et al., 2013).
Lactobacillus pentosus MP-10 is a potential probiotic LAB isolated from naturally fermented Aloreña green table olives (Abriouel et al., 2011) and has exhibited several probiotic capacities when tested in vitro such as good growth and survival capacities under simulated gastro-intestinal conditions, ability to auto-aggregate, and co-aggregate with pathogenic bacteria, adherence to intestinal and vaginal cell lines, antagonistic activity against pathogens and fermentation of several prebiotics and lactose (Pérez Montoro et al., 2016). However, the putative health-promoting capacities of this strain may depend on genetic characteristics and the interactions within its ecological niche (O’Sullivan et al., 2009); for this reason, the whole-genome sequence obtained by Abriouel et al. (2016) and the subsequent annotation will improve our knowledge about the functionality of this strain, its adaptation to the human gastrointestinal tract (GIT) and its interaction within the host. As such, we carried out in silico analysis of L. pentosus MP-10’s carbohydrate metabolism and the factors that affect their interaction with the host with the aim to identify genes as potential probiotic markers.
Results and Discussion
General Metabolic Features of a Probiotic Lactobacillus pentosus MP-10
Figure 1 shows the frequency of KEGG functional annotations obtained by BlastKOALA (KEGG tool; last updated March 4, 2016), which assigned approximately half (45.7%) of the genes to KEGG annotations corresponding to environmental information processing (443 genes), genetic information processing (413 genes), carbohydrate metabolism (279), amino acid metabolism (173), cellular processes (164 genes), nucleotide metabolism (90 genes), energy metabolism (87 genes), metabolism of cofactors and vitamins (87 genes), human disease factors (70 genes), lipid metabolism (62 genes), among others.
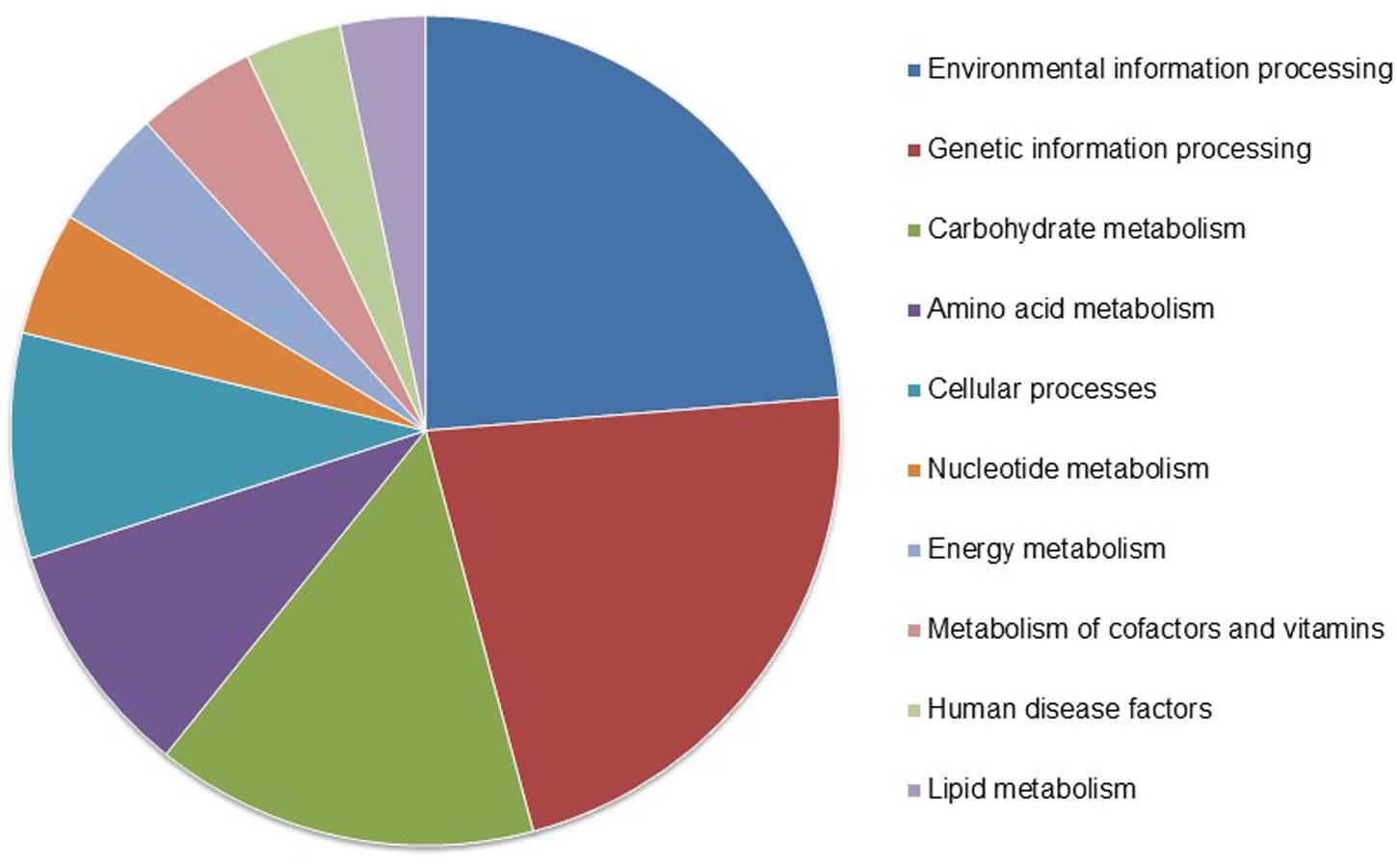
FIGURE 1. BlastKOALA results of functional categories predicted in Lactobacillus pentosus MP-10 genome and their frequencies.
To highlight the molecular mechanisms involved in the adaptation of L. pentosus MP-10 to the human GIT, we focused the in silico analysis on carbohydrate metabolism related to prebiotic utilization and the proteins involved in host interactions, since the adaptation of probiotics is mainly represented by the enrichment of mucus-binding proteins and enzymes involved in breakdown of complex carbohydrates (Ventura et al., 2012).
In silico analysis has some limitations related with the prediction accuracy which in turn depends on the algorithm used and the phenotype data from experiments (Ng and Henikoff, 2006); however, to avoid incorrect predictions all the annotations made in the present study were curated manually.
Carbohydrate Metabolism Related with Prebiotic Utilization
Over 8% of the identified genes in L. pentosus MP-10 genome are involved in carbohydrate metabolism (279 of 3558 genes), which is similar to the most-studied bifidobacterial genomes and 30% higher than other gastrointestinal (GIT)-resident bacteria (Ventura et al., 2009). The abundance of carbohydrate metabolism genes in L. pentosus MP-10 is important with respect to its possible adaptation to the microhabitats of gastrointestinal environment and its interaction with human host, and thus may enhance its survival, competitiveness and persistence.
Lactobacillus pentosus MP-10 is a facultatively hetero-fermentative LAB, and its genome possesses genes for both the phosphoketolase and Embden-Meyerhof pathways (EMP). Thus, it can potentially ferment carbohydrates mainly via the EMP, utilizing glucose, and converting it to pyruvate and then to lactate (glycolysis). However, in the absence of six-carbon sugars (e.g., glucose, et al.), L. pentosus MP-10 would possibly ferment five-carbon carbohydrates such as xylose, xylulose, arabinose, or ribose via the phosphoketolase pathway (PK), as reported for other L. pentosus strains (Bustos et al., 2005). Analysis by BlastKOALA indicated that EMP (complete pathway), pentose phosphate pathway (PP) (both oxidative and non-oxidative complete pathways), and galactose degradation pathway (complete Leloir pathway) form the central core of carbohydrate metabolism in L. pentosus MP-10; however, the Entner-Doudoroff pathway (ED) appears incomplete.
Lactobacillus pentosus MP-10 has been shown to be able to ferment in vitro a variety of carbohydrates such as glucose, galactose, fructose, lactose, saccharose, and lactulose (Pérez Montoro et al., 2016). In silico analysis of the annotated genome sequence of L. pentosus MP-10 also predicted its capacity to ferment several simple carbohydrates of both five-carbon and six-carbon sugars such as mannose, inositol, ribose, arabinose, rhamnose, maltose, xylose, xylulose, and trehalose; furthermore, we also predicted its ability to use complex carbohydrates such as cellulose, xylan (hemicellulose), starch, raffinose, chitin, and levan (Figure 2). These carbohydrates can either be dietary compounds or carbon sources derived from the metabolism of the gastrointestinal microbiota (Korakli et al., 2002). Ultimately, 15 carbohydrate utilization pathways were predicted in L. pentosus MP-10 genome sequence: glycolysis/gluconeogenesis, citrate cycle, PP pathway, pentose, and glucuronate interconversions, fructose and mannose metabolism, galactose metabolism, ascorbate, and aldarate metabolism, starch and sucrose metabolism, amino sugar and nucleotide sugar metabolism, pyruvate metabolism, glyoxylate and dicarboxylate metabolism, propanoate metabolism, butanoate metabolism, C5-branched dibasic acid metabolism and inositol phosphate metabolism. As such, the wide repertoire of enzymes involved in the fermentation of various carbohydrate substrates is reflected in its relatively large genome size, which is also corroborated by the significantly abundant number of genes for the phosphoenolpyruvate- (PEP) dependent sugar phosphotransferase system (PTS) (77 genes) and the presence of specific genes or gene clusters involved in carbohydrate utilization by L. pentosus MP-10.
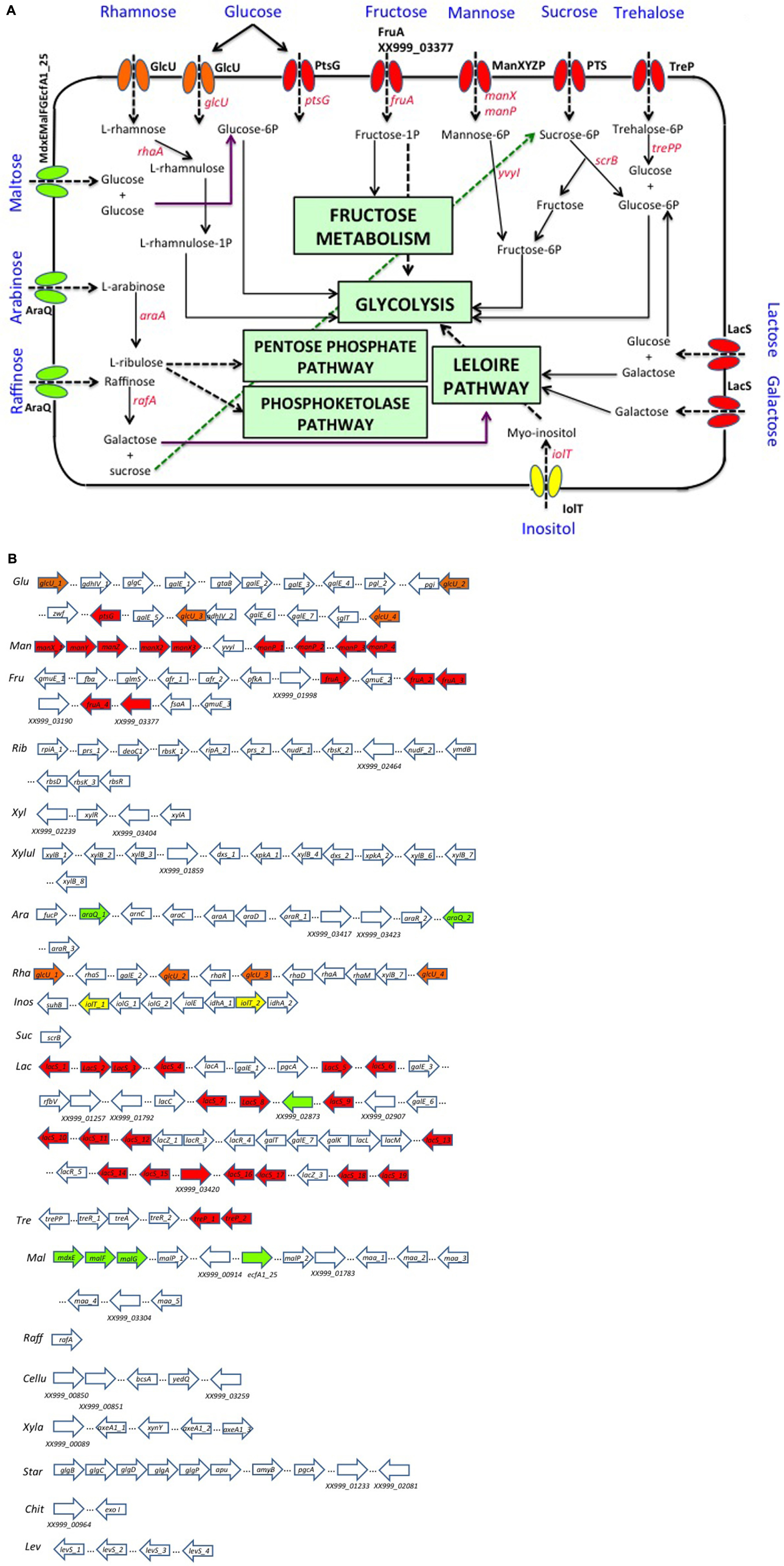
FIGURE 2. Organization of gene clusters encoding proteins predicted to be involved in carbohydrate utilization as prebiotics by L. pentosus MP-10. (A) Pathway reconstruction as predicted by genome annotation: PTS (phosphotransferase system), red; MFS (Major Facilitator Superfamily), yellow; ABC Transporter, green; GRP (Glucose/Ribose Porter Family), orange. (B) Genetic loci of interest: Ara, arabinose; Cellu, cellulose; Chit, chitin; Fru, fructose; Glu, glucose; Inos, inositol; Lac, lactose–galactose loci; Lev, levan; Mal, maltose; Man, mannose; Raff, raffinose; Rha, rhamnose; Rib, ribose; Star, starch; Suc, sucrose; Tre, trehalose; Xyl, xylose; Xyla, xylan; Xylul, xylulose.
The possible adaptation and enrichment of L. pentosus MP-10 in GIT could be predicted by the presence of genes encoding various carbohydrate-modifying enzymes able to modify oligo- and polysaccharides. These enzymes are produced by intestinal microbial communities and are required for the metabolism of plant- and host-derived carbohydrates (e.g., cellulose, xylan, and pectin), since mammals have limited evolved abilities to hydrolyze complex polysaccharides for digestion (Cantarel et al., 2012). Among these enzymes, many were predicted in L. pentosus MP-10 genome and belong to several CAZY “Carbohydrate-Active Enzymes” families (Table 1): glycoside hydrolases or glycosylases (15 genes); hexosyl- (15 genes), pentosyl- (13 genes) and phospho-transferases (13 genes); and also isomerases (24 genes).
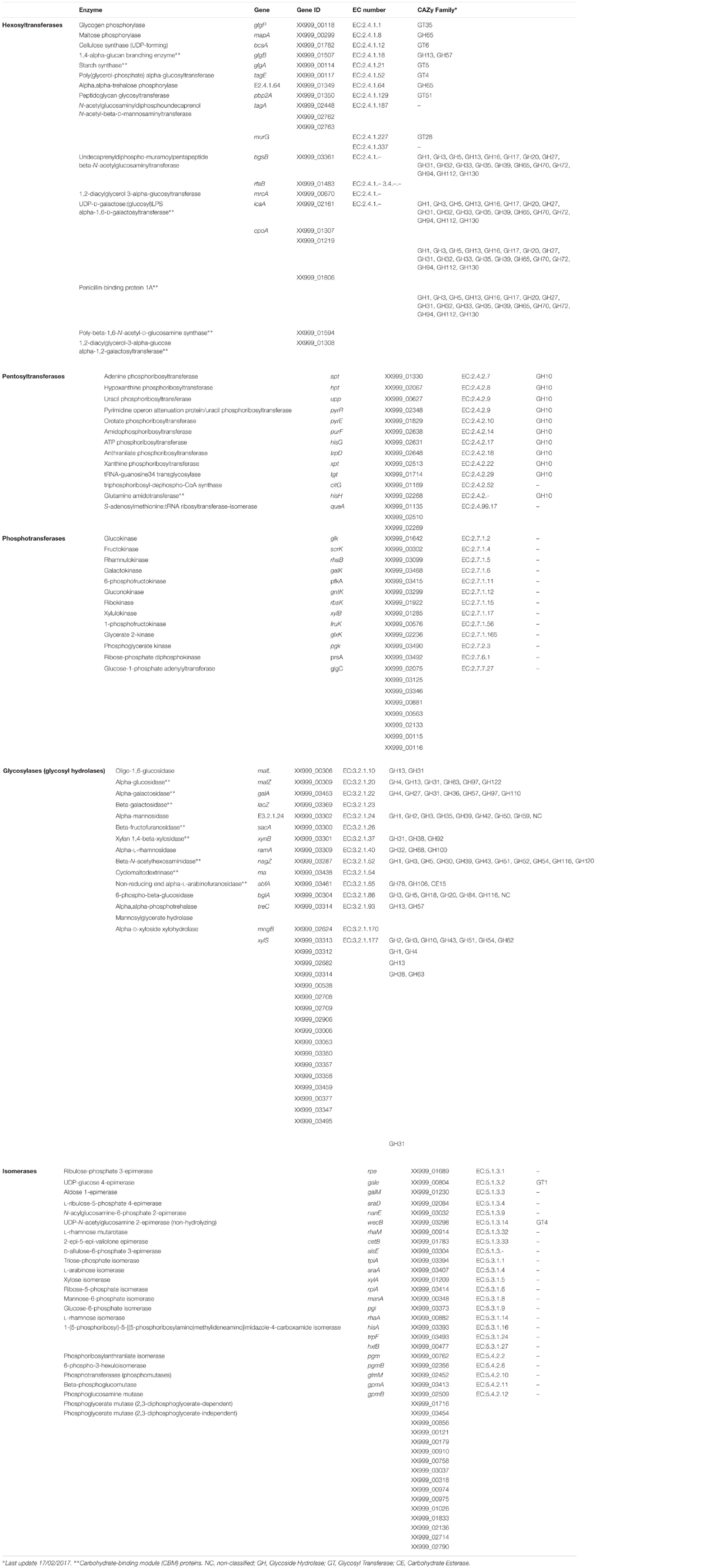
TABLE 1. Putative carbohydrate-modifying enzymes identified in the genome sequence of Lactobacillus pentosus MP-10.
Furthermore, the presence of sugar ABC transporters, carbohydrate esterases, glycosyl transferases, polysaccharide lyases, permeases, and PEP-PTS (PEP; PTS) components required for the uptake and metabolism of plant and host-derived carbohydrates were predicted in the L. pentosus MP-10 genome, as similarly reported for the probiotic Bifidobacterium (Kim et al., 2009). This arsenal of genes coding for carbohydrate-modifying enzymes predicted in L. pentosus MP-10 genome could represent a key indicator of this bacterium’s adaptation to the GIT environment, as these genes are involved in the metabolism and transport of carbohydrates non-digestible by human enzymes. Glycosyl (hexosyl-, pentosyl-, and phospho-) transferases are involved in the biosynthesis of disaccharides, oligosaccharides and polysaccharides by transferring sugar moieties from an activated donor to a specific substrate (Lairson et al., 2008); the resulting glycoconjugates (as part of the glycome) play an important role in the establishment of environment- and host-specific interactions (Kay et al., 2010). Glycoside hydrolases are able to hydrolyze the glycosidic bond between two or more carbohydrates, and also between carbohydrate and non-carbohydrate moieties. The most common predicted genes found in L. pentosus MP-10 were coding for oligo-1,6-glucosidase, beta-galactosidase, alpha-L-rhamnosidase, and 6-phospho-beta-glucosidase among others (with several GH families), playing a key role not only in carbohydrate hydrolysis but also their action as retaining enzymes involved in the synthesis of oligosaccharides that may be selectively used as prebiotics by L. pentosus MP-10 and other gastrointestinal probiotic bacteria (Table 1).
Regarding isomerases, we observed several carbohydrate isomerases involved in the glycolytic pathway; however, the presence of different copies of phosphoglycerate mutase may indicate that gene-products may accomplish other functions as a moonlighting protein (Candela et al., 2007).
Complex Carbohydrate Metabolism
Lactobacillus pentosus MP-10 has the capacity to metabolize complex carbohydrates (e.g., starch, cellulose, galactan, xylan, pullulan, pectins, and gums). For example, glycogen metabolism plays an important role in survival and fitness of LAB in their ecological niche by contributing to cellular processes such as carbohydrate metabolism, energy production, stress response, and cell–cell communication (Eydallin et al., 2007, 2010). The glycogen metabolism operon (glg) predicted in L. pentosus MP-10 is encoded by a 9608-base chromosomal region and consists of glgBCDAP-apu genes (XX999_00114 to XX999_00119), which are co-transcribed as polycistronic mRNA (Table 2). The organization of the core genes (glgBCDAP) is identical to many bacteria, with the exception of two additional glycogen synthase genes exclusive to L. pentosus MP-10 (XX999_01233 and XX999_02081) which are homologous with Bacillus subtilis 168 and Mycobacterium tuberculosis CDC 1551, respectively (Table 2). Furthermore, genes amyB and pgcA coding for alpha-amylase 2 and phosphoglucomutase, respectively, are distantly located from the glg operon (Table 2 and Figure 2B). According to Goh and Klaenhammer (2014), the glycogen gene cluster organization might differ depending on the bacterial species and origin; in this study, the glycogen gene cluster is composed of glgBCDAP-apu-amyB-pgcA genes and the other two glycogen synthase genes (XX999_01233 and XX999_02081). Glycogen metabolism is predicted as an additional trait in L. pentosus MP-10, as it will contribute to probiotic activities and the retention of this bacterium in highly competitive and dynamic niches, such as the gastrointestinal environment, similarly as the probiotic L. acidophilus (Goh and Klaenhammer, 2013). The presence of more than one glycogen synthase gene in L. pentosus MP-10 indicates the capacity of these bacteria to store carbohydrates in the form of glycogen.
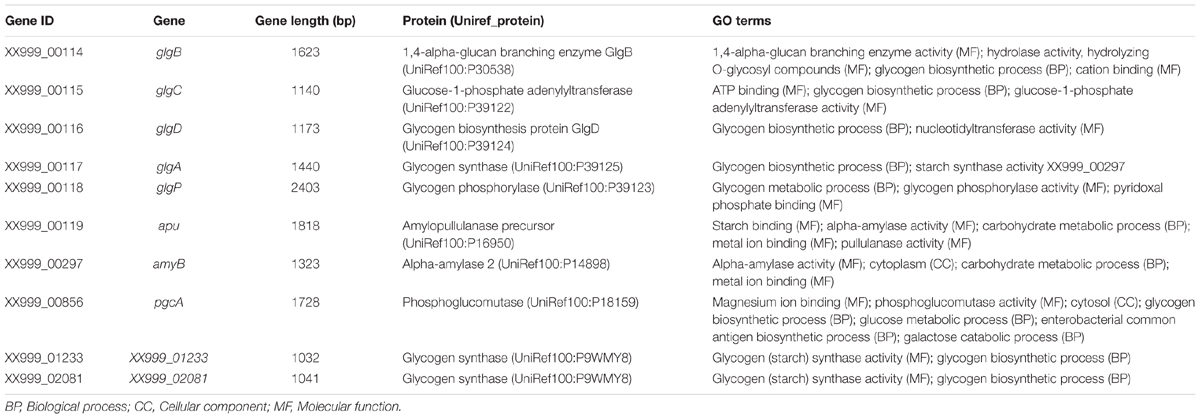
TABLE 2. Genes necessary for the glycogen metabolism in Lactobacillus pentosus MP-10 isolated from naturally fermented Aloreña table olives.
Lactobacillus pentosus MP-10 possesses genes predicted as levansucrase (levS_1, levS_2, levS_3, and levS_4) with identities ranging from 44.07 to 62.4% with levS gene from L. sanfranciscensis (Table 3; Rhee et al., 2002; Tieking et al., 2005), which are responsible for levan polymers [fructan polymers composed of β(2,6)-linked fructose units] and the fructo-oligosaccharide (FOS) 1-kestose production with prebiotic effects. This bacterium is capable to produce levan [with β-2,6 glycosidic bonds, produced by levansucrases (E.C. 2.4.1.10)] but not inulin-fructan types as no inulosucrase genes were detected in L. pentosus MP-10 genome. This is the first report of levansucrase in L. pentosus; this enzyme has only been reported in other LAB (L. sanfranciscensis L. reuteri, L. johnsonii, L. gasseri, L. crispatus, L. plantarum, L delbrueckii, and L. vaginalis among others). Alignments of the amino acid sequence of LevS proteins of L. pentosus MP-10 (LevS1, LvS2, LevS3, and LevS4) with levansucrase proteins of other lactic acid bacteria revealed less similarity and formed a separate cluster in the phylogenetic tree (Figure 3).
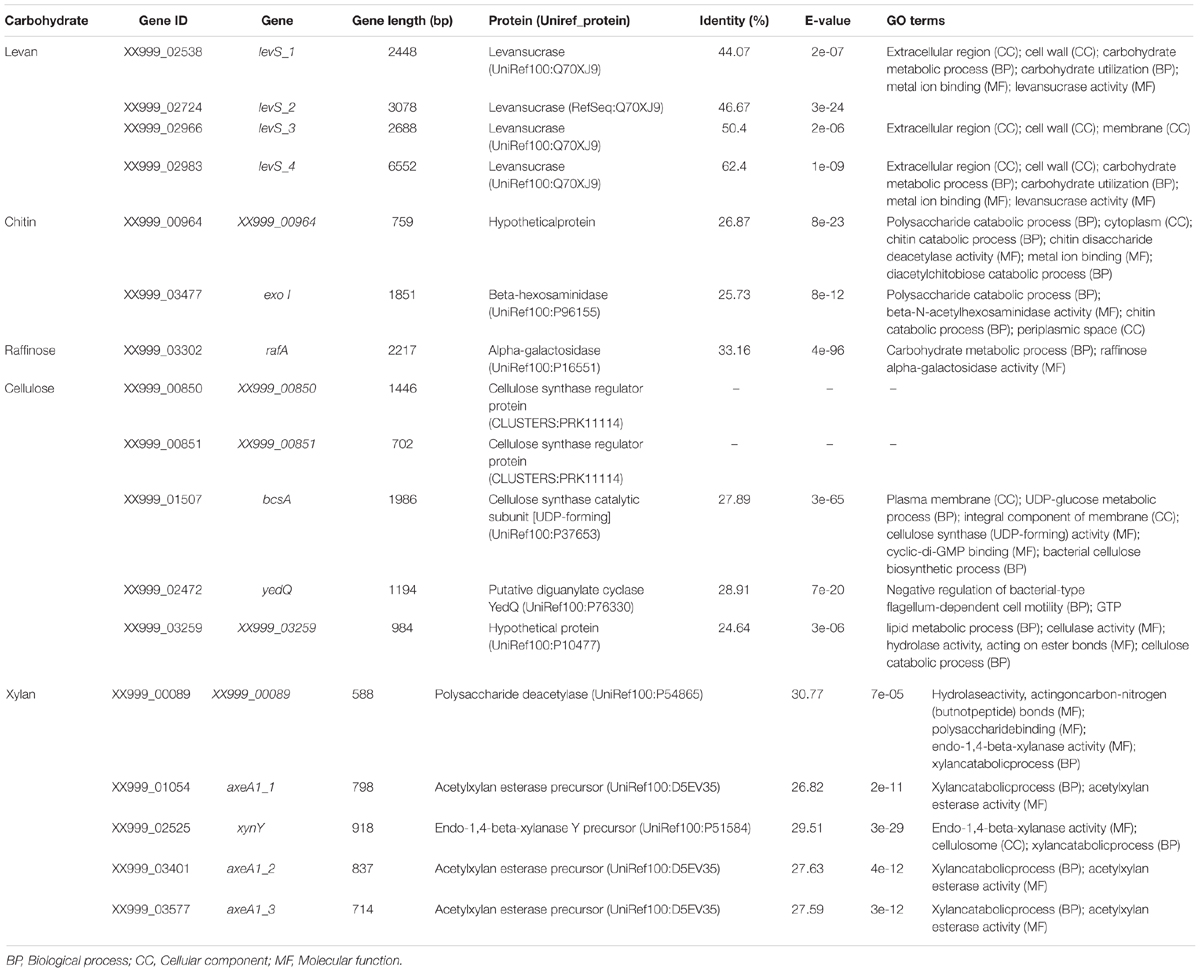
TABLE 3. Genes necessary for complex carbohydrate metabolism in Lactobacillus pentosus MP-10 isolated from naturally fermented Aloreña table olives.
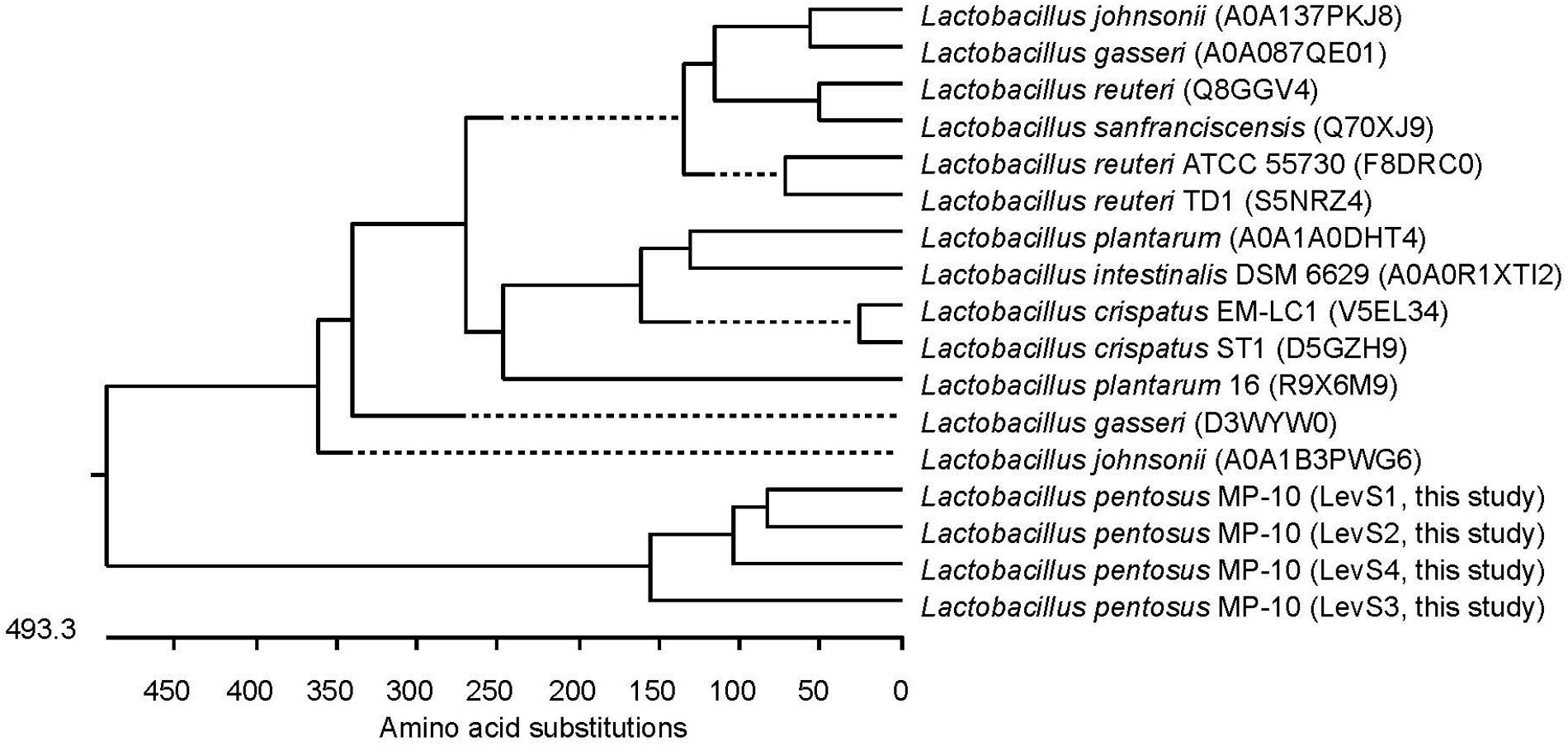
FIGURE 3. Phylogenetic relationships of L. pentosus MP-10 and other Lactobacillus sp. inferred from the alignment of levansucrase proteins. The sequences were aligned and the most parsimonious phylogenetic trees were constructed using the CLUSTAL W of Lasergene program, version 14 (MegAlign 14, Inc., Madison, WI, USA). The scale below indicates the number of amino acid substitutions. Accession numbers are indicated in parentheses.
Regarding other enzymes involved in complex carbohydrate degradation, we found genes coding for a protein similar to chitooligosaccharide deacetylase of E. coli K12 and beta-hexosaminidase involved in chitin degradation pathway as part of glycan degradation. Further, several genes coding for enzymes involved in the degradation of plant structural polysaccharides such as cellulose, ß-glucan, and xylan were predicted in L. pentosus MP-10 genome (Table 3). In this context, a gene coding for a protein similar to cellulase/esterase CelE from Clostridium thermocellum ATCC 27405, which is a multifunctional enzyme involved in the degradation of plant cell wall polysaccharides, was identified in L. pentosus MP-10 genome necessary for cellulose and xylan digestion by both human and animals (Table 3). Moreover, endo-1,4-beta-xylanase, acetylxylan esterase (three genes) and polysaccharide deacetylase were predicted in L. pentosus MP-10 genome sequence being involved in xylan catabolic pathway. Alpha-galactosidase coding gene was also detected in L. pentosus MP-10 genome sequence and is involved in raffinose degradation (Table 3), which was previously shown in vitro by Pérez Montoro et al. (2016). Furthermore, L. pentosus MP-10 also had genes coding for cellulose synthase (two genes exclusive to L. pentosus MP-10 and two other genes) involved in cellulose synthesis (Table 3), which could accumulate cellulose on the cell wall surface as an extracellular matrix for cell adhesion and biofilm formation to protect the bacteria. Cellulose production has been reported in lactic acid bacteria (Adetunji and Adegoke, 2007); however, no reports were found of cellulase production, although some Lactobacillus sp. genomes exhibit cellulase genes such as L. delbrueckii subsp. bulgaricus CNCM I-1519 (UniProtKB-G6F519) and L. plantarum (UniProtKB – A0A1C9HK74). For probiotic bacteria, such as E. coli Nissle 1917, cellulose production is required for adhesion of bacteria to the gastrointestinal epithelial cell line HT-29, to the mouse epithelium in vivo, and for enhanced cytokine production (Monteiro et al., 2009). Thus, the role of cellulose production in L. pentosus MP-10 must be investigated in depth.
Overall, the repertoire of enzymes coding genes identified in L. pentosus MP-10 genome highlight the attractiveness of this bacterium as potential probiotic for human and animal.
Molecular Mechanisms Involved in the Interaction with the Host
Probiotic lactobacilli can mimic the same mechanisms used by the pathogens in the colonization process, thus they can express cell surface proteins such as key probiotic ligands that interact with host receptors resulting in several probiotic effects—thus inducing signaling pathways in the host (Voltan et al., 2008). The identification and characterization of these proteins, often strain-specific, involved in the molecular communication or interaction with the host are necessary to evaluate a priori the probiotic potential of Lactobacillus sp. candidates. Here, the possible interaction between L. pentosus MP-10 and the intestinal host cells, the target of most interactions with probiotics (Lebeer et al., 2010), may be bioinformatically predicted from the genome sequence. For example, several extracellular proteins (reviewed by Sánchez et al., 2008) were predicted in L. pentosus MP-10 to be involved in mucus adhesion: MucBP domain protein (codified by two genes determined in this study), lipoprotein signal peptidase (lspA gene) and moonlighting proteins such as glutamine-binding periplasmic protein (glnH genes) and elongation factor Tu (tuf gene) (Table 4). The high genetic heterogeneity of MucBP proteins among Lactobacillus species (and strains) was reported by Mackenzie et al. (2010) for MUB and MUB-like proteins in L. reuteri. MucBP domain proteins are bacterial peptidoglycan-bound proteins, which are ligands or effector molecules contributing to specific properties such as adherence to the host, auto-aggregation and/or co-aggregation with pathogenic bacteria (Pérez Montoro et al., 2016)—as reported by Mackenzie et al. (2010) for MUB in L. reuteri. However, this should be further investigated for L. pentosus MP-10 under different conditions. Adhesion to mucus has been attributed to other molecules such as the Lactobacillus surface protein A (LspA), reported as mucus binding protein in L. salivarius UCC118 (van Pijkeren et al., 2006), which was also found in L. pentosus MP-10 (Table 4). Mucus binding proteins in L. pentosus MP-10 may have a dual role: (1) being involved in the adhesion of this bacterium to the host cells and thus reinforcing the protection of the mucosal barrier and the competitive exclusion of pathogens, and (2) these proteins could also be implicated in the induction of mucin secretion by the host as reported for other lactobacilli (Mack et al., 2003). These finding are corroborated by the fact that L. pentosus MP-10 was able to adhere to Caco-2 and HeLa 229 cell lines and also co-aggregate with different pathogens (Escherichia coli, Staphylococcus aureus, Listeria innocua, and Salmonella Enteritidis) (Pérez Montoro et al., 2016) by means of cell-wall surface molecules. However, further studies are required to demonstrate the target cell-wall surface molecules involved in such adhesion to intestinal cells.
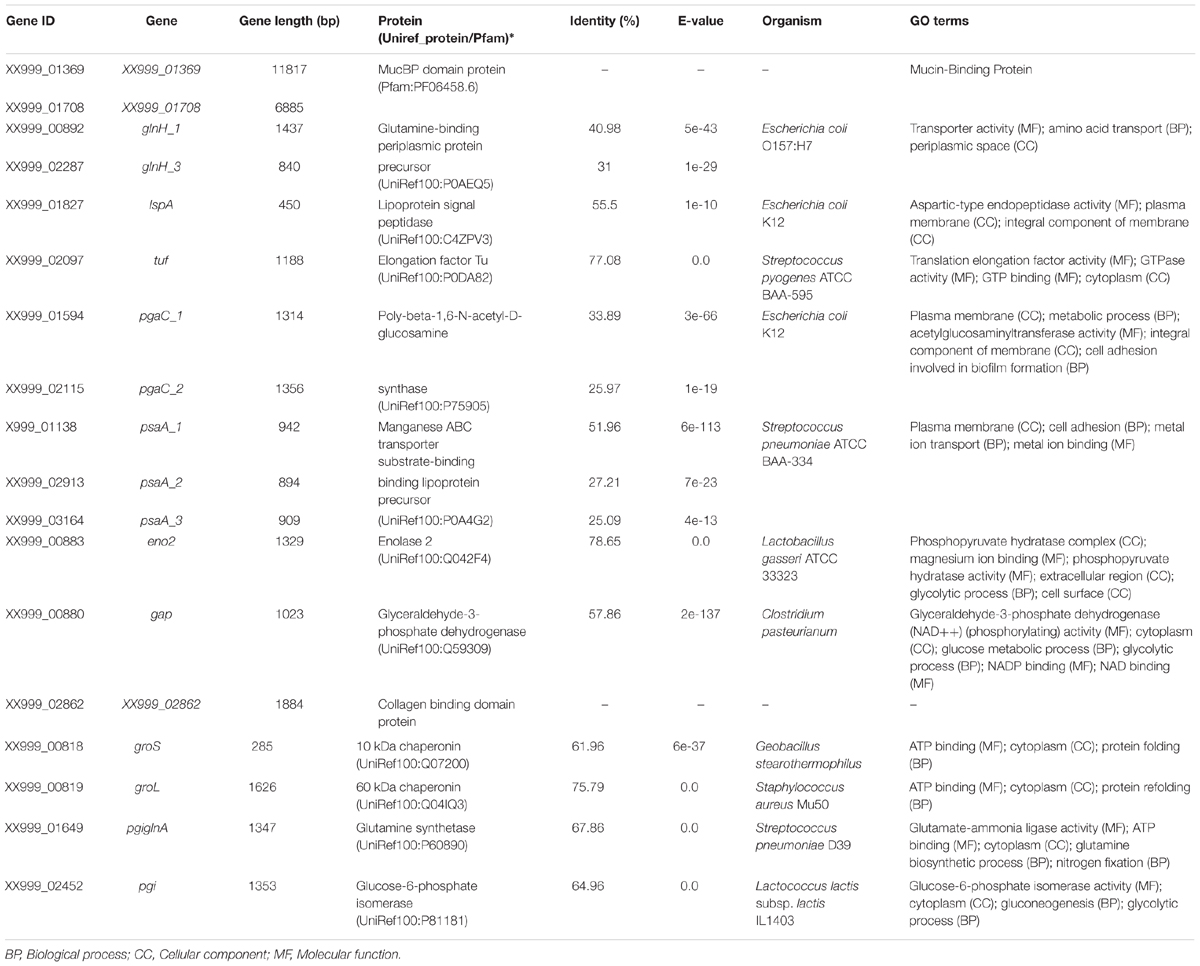
TABLE 4. Genes coding for extracellular proteins with roles in adhesion or interaction with the host as predicted from genome annotation of Lactobacillus pentosus MP-10 isolated from naturally fermented Aloreña table olives.
Other proteins predicted to be involved in adhesion to epithelial cells or extracellular matrix include: poly-beta-1,6-N-acetyl-D-glucosamine synthase, collagen binding protein, manganese ABC transporter substrate-binding lipoprotein precursor and moonlighting proteins such as elongation factor Tu, glyceraldehyde-3-phosphate dehydrogenase, 10 and 60 kDa chaperonins, enolase, 2 glutamine synthetase, and glucose-6-phosphate isomerase (Table 4). The poly-beta-1,6-N-acetyl-D-glucosamine synthase encoded by L. pentosus MP-10 was similar to E. coli K12 (33.89% identity), and it has been reported to be a surface polysaccharide involved in biofilm formation by this strain (Matthysse et al., 2008). However, the role of this protein in lactobacilli has not been determined. Furthermore, we predicted the presence of collagen-binding protein specific to L. pentosus MP-10, which could be involved in their adhesion to epithelial cells/extracellular matrix proteins similarly as shown other lactobacilli such as L. reuteri NCIB 11951 (Roos et al., 1996) and L. fermentum RC-14 (Heinemann et al., 2000). Thus, this could be of vital importance for effective colonization and also competitive displacement of gut pathogens (Yadav et al., 2013).
On the other hand, the manganese ABC transporter substrate-binding lipoprotein precursor predicted in L. pentosus MP-10, similar to Streptococcus pneumoniae ATCC BAA-334 (51.96% identity), has been described as an important factor in pathogenesis and infection, since it acts as an adhesin involved on adherence to extracellular matrix (Dintilhac et al., 1997). Furthermore, the manganese ABC transporter substrate-binding lipoprotein precursor has also been detected in different Lactobacillus sp. such as L. plantarum, L. rhamnosus, and L. delbrueckii among others being involved in cell adhesion (UniprotKB).
The moonlighting proteins, or multifunctional proteins such as elongation factor Tu and chaperonin GroEL, have been involved in the adhesion to epithelial cells and/or extracellular matrix proteins and also in host immunomodulation in L. johnsonii NCC 533 (Granato et al., 2004; Bergonzelli et al., 2006; Sánchez et al., 2008), while α-enolase has been involved in adhesion to epithelial cells and/or extracellular matrix proteins and also plasma components in L. crispatus ST1 (Antikainen et al., 2007). Glyceraldehyde-3-phosphate dehydrogenase and phosphoglycerate mutase have been involved in the adhesion to plasma components in L. crispatus ST2 (Antikainen et al., 2007; Candela et al., 2007). Furthermore, Kainulainen et al. (2012) showed that glutamine synthetase and glucose-6-phosphate isomerase have also been involved in adhesion to epithelial cells. However, the role of these moonlighting proteins in L. pentosus MP-10 has not yet been determined, requiring for this purpose further mutation or proteomic studies.
Conclusion
Lactobacillus pentosus MP-10 has harbored in its genome several genes putatively involved in their adaptation to the human GIT—particularly those involved in carbohydrate metabolism related to prebiotic utilization, and also the proteins involved in the interaction with host tissues. Enzymes involved in carbohydrate modification and complex-carbohydrate metabolism are highly represented in L. pentosus MP-10 genome, which may enhance their survival, competitiveness, and persistence in a competitive GIT niche. Furthermore, we found genes encoding mucus-binding proteins—involved in the adhesion to mucus, epithelial cells or extracellular matrix, to plasma components—and also moonlighting proteins, or multifunctional proteins, predicted to be involved in their adhesion to epithelial cells and/or extracellular matrix proteins and also involved in host immunomodulation. In conclusion, in silico analysis of the L. pentosus MP-10 genome sequence highlights the attractiveness of this bacterium as a potential probiotic for human and animal hosts, and offers opportunities for further investigation of novel routes for screening and studying these bacteria.
Materials and Methods
Genomic DNA Sequence of L. pentosus MP-10
The complete genome sequence of L. pentosus MP-10, obtained by using PacBio RS II technology (Abriouel et al., 2016) and deposited at the EMBL Nucleotide Sequence Database under accession numbers FLYG01000001 to FLYG01000006, was annotated as described by Abriouel et al. (in press). Briefly, the assembled genome sequences were annotated using the Prokka annotation pipeline, version 1.11 (Seemann, 2014), which predicted tRNA, rRNA, and mRNA genes and signal peptides in the sequences using Aragorn, RNAmmer, Prodigal, and SignalP, respectively (Laslett and Canback, 2004; Lagesen et al., 2007; Hyatt et al., 2010).
In Silico Analysis of Carbohydrate Metabolism in L. pentosus MP-10
The annotated genome sequence was used to detect the putative genes involved in carbohydrate metabolism, their products, and the associated GO terms. Furthermore, the carbohydrate metabolic pathways were reconstructed by using BlastKOALA (last update March 4, 2016) as part of the KEGG (Kyoto Encyclopedia of Genes and Genome) tool in the pathway database2 for annotating genomes; here, we used the annotated genes predicted in L. pentosus MP-10 genome as the input query.
In Silico Analysis of Proteins Involved in Interaction with Host
The annotated genome sequence was screened for mucus-binding proteins, proteins involved in adhesion to epithelial/extracellular matrix proteins, plasma components, and host immunomodulation as described in the literature (Roos et al., 1996; Heinemann et al., 2000; Granato et al., 2004; Bergonzelli et al., 2006; van Pijkeren et al., 2006; Antikainen et al., 2007; Candela et al., 2007; Sánchez et al., 2008; Mackenzie et al., 2010; Kainulainen et al., 2012).
Author Contributions
HA, NB, CK, and AG drafted the manuscript. HA, NB, BPM, CC-S, APP, NCG, SC-G, and ME-M analyzed the data; All authors discussed the results, commented on the manuscript, and approved the final version.
Conflict of Interest Statement
The authors declare that the research was conducted in the absence of any commercial or financial relationships that could be construed as a potential conflict of interest.
Acknowledgments
We acknowledge research grants AGL2013-43571-P (Ministerio de Economía y Competitividad, MINECO, FEDER), UJA2014/07/02 (Plan Propio UJA) and Research Team (EI_BIO01_2017).
Footnotes
References
Abriouel, H., Benomar, N., Lucas, R., and Gálvez, A. (2011). Culture-independent study of the diversity of microbial populations in brines during fermentation of naturally fermented Aloreña green table olives. Int. J. Food Microbiol. 144, 487–496. doi: 10.1016/j.ijfoodmicro.2010.11.006
Abriouel, H., Pérez Montoro, B., Casado Muñoz, M. C., Knapp, C. W., Gálvez, A., and Benomar, N. (in press). Genomic insights into safety aspects and defense mechanisms of a potentially probiotic Lactobacillus pentosus MP-10 isolated from brines of naturally fermented Aloreña green table olives. PLoS ONE.
Abriouel, H., Peìrez Montoro, B., Casado Munþoz, M. D. C., Lavilla Lerma, L., Hidalgo Pestanþa, M., Caballero Goìmez, N., et al. (2016). Complete genome sequence of a potential probiotic, Lactobacillus pentosus MP-10, isolated from fermented Alorenþa table olives. Genome Announc. 4:e854-16. doi: 10.1128/genomeA.00854-16
Adetunji, V. O., and Adegoke, G. O. (2007). Bacteriocin and cellulose production by lactic acid bacteria isolated from West African soft cheese. Afr. J. Biotechnol. 6, 2616–2619.
Antikainen, J., Kuparinen, V., Lähteenmaki, K., and Korhonen, T. K. (2007). pH-dependent association of enolase and glyceraldehyde-3-phosphate dehydrogenase of Lactobacillus crispatus with the cell wall and lipoteichoic acids. J. Bacteriol. 189, 4539–4543.
Bergonzelli, G. E., Granato, D., Pridmore, R. D., Marvin-Guy, L. F., Donnicola, D., and Corthésy-Theulaz, I. E. (2006). GroEL of Lactobacillus johnsonii La1 (NCC 533) is cell surface associated: potential role in interactions with the host and the gastric pathogen Helicobacter pylori. Infect. Immun. 74, 425–434.
Bernardeau, M., Vernoux, J. P., Henri-Dubernet, S., and Gueguen, M. (2008). Safety assessment of dairy microorganisms: the Lactobacillus genus. Int. J. Food Microbiol. 126, 278–285.
Bustos, G., Moldes, A. B., Cruz, J. M., and Domiìnguez, J. M. (2005). Influence of the metabolism pathway on lactic acid production from hemicellulosic trimming vine shoots hydrolyzates using Lactobacillus pentosus. Biotechnol. Prog. 21, 793–798.
Candela, M., Bergmann, S., Vici, M., Vitali, B., Turroni, S., Eikmanns, B. J., et al. (2007). Binding of human plasminogen to Bifidobacterium. J. Bacteriol. 189, 5929–5936.
Cantarel, B. L., Lombard, V., and Henrissat, B. (2012). Complex carbohydrate utilization by the healthy human microbiome. PLoS ONE 7:e28742. doi: 10.1371/journal.pone.0028742
De Angelis, M., and Gobbetti, M. (2004). Environmental stress responses in Lactobacillus: a review. Proteomics 4, 106–122.
Dintilhac, A., Alloing, G., Granadel, C., and Claverys, J.-P. (1997). Competence and virulence of Streptococcus pneumoniae: Adc and PsaA mutants exhibit a requirement for Zn and Mn resulting from inactivation of putative ABC metal permeases. Mol. Microbiol. 25, 727–739.
Eydallin, G., Montero, M., Almagro, G., Sesma, M. T., Viale, A. M., Munoz, F. J., et al. (2010). Genome-wide screening of genes whose enhanced expression affects glycogen accumulation in Escherichia coli. DNA Res. 17, 61–71. doi: 10.1093/dnares/dsp028
Eydallin, G., Viale, A. M., Moran-Zorzano, M. T., Munoz, F. J., Montero, M., Baroja-Fernandez, E., et al. (2007). Genome-wide screening of genes affecting glycogen metabolism in Escherichia coli K-12. FEBS Lett. 581, 2947–2953.
Franz, C. M. A. P., Cho, G. Y., and Holzapfel, W. H. (2011). “Probiotics: taxonomy and technological features,” in Probiotic and Prebiotic Foods: Technology, Stability and Benefits to Human Health, eds N. P. Shah, A. Gomes da Cruz, and J. de Assis Fonseca Faria (Hauppauge, NY: Nova Science Publishers), 1–23.
Garrigues, C., Johansen, E., and Crittenden, R. (2013). Pangenomics—an avenue to improved industrial starter cultures and probiotics. Curr. Opin. Biotechnol. 24, 187–191. doi: 10.1016/j.copbio.2012.08.009
Giraffa, G., Chanishvili, N., and Widyastuti, Y. (2010). Importance of lactobacilli in food and feed biotechnology. Res. Microbiol. 161, 480–487. doi: 10.1016/j.resmic.2010.03.001
Goh, Y. J., and Klaenhammer, T. R. (2013). A functional glycogen biosynthesis pathway in Lactobacillus acidophilus: expression and analysis of the glg operon. Mol. Microbiol. 89, 1187–1200. doi: 10.1111/mmi.12338
Goh, Y. J., and Klaenhammer, T. R. (2014). Insights into glycogen metabolism in Lactobacillus acidophilus: impact on carbohydrate metabolism, stress tolerance and gut retention. Microb. Cell Fact. 13:94. doi: 10.1186/s12934-014-0094-3
Granato, D., Bergonzelli, G. E., Pridmore, R. D., Marvin, L., Rouvet, M., and Corthésy-Theulaz, I. E. (2004). Cell surface-associated elongation factor Tu mediates the attachment of Lactobacillus johnsonii NCC533 (La1) to human intestinal cells and mucins. Infect. Immun. 72, 2160–2169.
Hansen, E. B. (2002). Commercial bacterial starter cultures for fermented foods of the future. Int. J. Food Microbiol. 78, 119–131.
Heinemann, C., van Hylckama Vlieg, J. E. T., Janssen, D. B., Busscher, H. J., van der Mei, H. C., and Reid, G. (2000). Purification and characterization of a surface-binding protein from Lactobacillus fermentum RC-14 that inhibits adhesion of Enterococcus faecalis 1131. FEMS Microbiol. Lett. 190, 177–180.
Heller, K. J. (2001). Probiotic bacteria in fermented foods: product characteristics and starter organisms. Am. J. Clin. Nutr. 73, 374s–379s.
Hill, C., Guarner, F., Reid, G., Gibson, G. R., Merenstein, D. J., Pot, B., et al. (2014). The International Scientific Association for Probiotics and Prebiotics consensus statement on the scope and appropriate use of the term probiotic. Nat. Rev. Gastroenterol. Hepatol. 11, 506–514. doi: 10.1038/nrgastro.2014.66
Holzapfel, W. H. (2002). Appropriate starter culture technologies for small-scale fermentation in developing countries. Int. J. Food Microbiol. 75, 197–212.
Hyatt, D., Chen, G.-L., LoCascio, P. F., Land, M. L., Larimer, F. W., and Hauser, L. J. (2010). Prodigal: prokaryotic gene recognition and translation initiation site identification. BMC Bioinformatics 11:119. doi: 10.1186/1471-2105-11-119
Izumo, T., Izumi, F., Nakagawa, I., Kitagawa, Y., Shibata, H., and Kiso, Y. (2011). Influence of Lactobacillus pentosus S-PT84 ingestion on the mucosal immunity of healthy and Salmonella Typhimurium-infected mice. Biosci. Microflora 30, 27–35. doi: 10.12938/bifidus.30.27
Jolley, K. A., and Maiden, M. C. (2010). BIGSdb: scalable analysis of bacterial genome variation at the population level. BMC Bioinformatics 11:595. doi: 10.1186/1471-2105-11-595
Kainulainen, V., Loimaranta, V., Pekkala, A., Edelman, S., Antikainen, J., Kylväjä, R., et al. (2012). Glutamine synthetase and glucose-6-phosphate isomerase are adhesive moonlighting proteins of Lactobacillus crispatus released by epithelial cathelicidin LL-37. J. Bacteriol. 194, 2509–2519. doi: 10.1128/JB.06704-11
Kay, E., Lesk, V. I., Tamaddoni-Nezhad, A., Hitchen, P. G., Dell, A., Sternberg, M. J., et al. (2010). Systems analysis of bacterial glycomes. Biochem. Soc. Trans. 38, 1290–1293. doi: 10.1042/BST0381290
Kim, J. F., Jeong, H., Yu, D. S., Choi, S.-H., Hur, C.-G., Park, M.-S., et al. (2009). Genome sequence of the probiotic bacterium Bifidobacterium animalis subsp. lactis AD011. J. Bacteriol. 191, 678–679. doi: 10.1128/JB.01515-08
Korakli, M., Gänzle, M. G., and Vogel, R. F. (2002). Metabolism by bifidobacteria and lactic acid bacteria of polysaccharides from wheat and rye, and exopolysaccharides produced by Lactobacillus sanfranciscensis. J. Appl. Microbiol. 92, 958–965.
Kotani, Y., Shinkai, S., Okamatsu, H., Toba, M., Ogawa, K., Yoshida, H., et al. (2010). Oral intake of Lactobacillus pentosus strain b240 accelerates salivary immunoglobulin A secretion in the elderly: a randomized, placebo-controlled, double-blind trial. Immun. Ageing 7:11. doi: 10.1186/1742-4933-7-11
Lagesen, K., Hallin, P., Rødland, E. A., Stærfeldt, H.-H., Rognes, T., and Ussery, D. W. (2007). RNAmmer: consistent and rapid annotation of ribosomal RNA genes. Nucleic Acids Res. 35, 3100–3108.
Lairson, L. L., Henrissat, B., Davies, G. J., and Withers, S. G. (2008). Glycosyltransferases: structures, functions, and mechanisms. Annu. Rev. Biochem. 77, 521–555.
Laslett, D., and Canback, B. (2004). ARAGORN, a program to detect tRNA genes and tmRNA genes in nucleotide sequences. Nucleic Acids Res. 32, 11–16.
Lebeer, S., Vanderleyden, J., and De Keersmaecker, S. C. J. (2010). Host interactions of probiotic bacterial surface molecules: comparison with commensals and pathogens. Nat. Rev. Microbiol. 8, 171–184. doi: 10.1038/nrmicro2297
Leroy, F., and de Vuyst, L. (1999). Temperature and pH conditions that prevail during fermentation of sausages are optimal for production of the antilisterial bacteriocin sakacin K. Appl. Environ. Microbiol. 65, 974–981.
Mack, D. R., Ahrne, S., Hyde, L., Wei, S., and Hollingsworth, M. A. (2003). Extracellular MUC3 mucin secretion follows adherence of Lactobacillus strains to intestinal epithelial cells in vitro. Gut 52, 827–833.
Mackenzie, D. A., Jeffers, F., Parker, M. L., Vibert-Vallet, A., Bongaerts, R. J., Roos, S., et al. (2010). Strain-specific diversity of mucus-binding proteins in the adhesion and aggregation properties of Lactobacillus reuteri. Microbiol. 156, 3368–3378. doi: 10.1099/mic.0.043265-0
Maiden, M. C., Jansen van Rensburg, M. J., Bray, J. E., Earle, S. G., Ford, S. A., Jolley, K. A., et al. (2013). MLST revisited: the gene-by-gene approach to bacterial genomics. Nat. Rev. Microbiol. 11, 728–736. doi: 10.1038/nrmicro3093
Matthysse, A. G., Deora, R., Mishra, M., and Torres, A. G. (2008). Polysaccharides cellulose, poly-β-1,6-N-acetyl-D-glucosamine, and colanic acid are required for optimal binding of Escherichia coli O157:H7 strains to alfalfa sprouts and K-12 strains to plastic but not for binding to epithelial cells. Appl. Environ. Microbiol. 74, 2384–2390. doi: 10.1128/AEM.01854-07
Monteiro, C., Saxena, I., Wang, X., Kader, A., Bokranz, W., Simm, R., et al. (2009). Characterization of cellulose production in Escherichia coli Nissle 1917 and its biological consequences. Environ. Microbiol. 11, 1105–1116. doi: 10.1111/j.1462-2920.2008.01840.x
Ng, P. C., and Henikoff, S. (2006). Predicting the effects of amino acid substitutions on protein function. Annu. Rev. Genomics Hum. Genet. 7, 61–80.
O’Sullivan, O., O’Callaghan, J., Sangrador-Vegas, A., McAuliffe, O., Slattery, L., Kaleta, P., et al. (2009). Comparative genomics of lactic acid bacteria reveals a niche-specific gene set. BMC Microbiol. 9:50. doi: 10.1186/1471-2180-9-50
Pérez Montoro, B., Benomar, N., Lavilla Lerma, L., Castillo Gutiérrez, S., Gálvez, A., and Abriouel, H. (2016). Fermented Aloreña table olives as a source of potential probiotic Lactobacillus pentosus strains. Front. Microbiol. 7:1583.
Pot, B., Felis, G. E., De Bruyne, K., Tsakalidou, E., Papadimitriou, K., Leisner, J., et al. (2014). “The genus Lactobacillus,” in Lactic Acid Bacteria: Biodiversity and Taxonomy, eds W. P. Holzapfel and B. J. B. Wood (Hoboken, NJ: John Wiley & Sons, Inc), 249–353.
Rhee, S.-K., Song, K. B., Kim, C. H., Park, B. S., Jang, E. K., and Jang, K. H. (2002). “Levan,” in Biopolymers. Polysaccharides I: Polysaccharides from Prokaryotes, eds A. Steinbüchel, S. D. Baets, and E. H. Vandamme (Weinheim: Wiley VCH), 351–378.
Roos, S., Aleljung, P., Robert, N., Lee, B., Wadstrom, T., Lindberg, M., et al. (1996). A collagen binding protein from Lactobacillus reuteri is part of an ABC transporter system? FEMS Microbiol. Lett. 144, 33–38.
Sánchez, B., Bressollier, P., and Urdaci, M. C. (2008). Exported proteins in probiotic bacteria: adhesion to intestinal surfaces, host immunomodulation and molecular cross-talking with the host. FEMS Immunol. Med. Microbiol. 54, 1–17. doi: 10.1111/j.1574-695X.2008.00454.x
Seemann, T. (2014). Prokka: rapid prokaryotic genome annotation. Bioinformatics 30, 2068–2069. doi: 10.1093/bioinformatics/btu153
Tieking, M., Ehrmann, M. A., Vogel, R. F., and Ganzle, M. G. (2005). Molecular and functional characterization of a levansucrase from the sourdough isolate Lactobacillus sanfranciscensis TMW 1.392. Appl. Microbiol. Biotechnol. 66, 655–663.
van Pijkeren, J.-P., Canchaya, C., Ryan, K. A., Li, Y., Claesson, M. J., Sheil, B., et al. (2006). Comparative and functional analysis of sortase-dependent proteins in the predicted secretome of Lactobacillus salivarius UCC118. Appl. Environ. Microbiol. 72, 4143–4153.
Ventura, M., Turroni, F., Canchaya, C., Vaughan, E. E., O’toole, P. W., and Sinderen, D. V. (2009). Microbial diversity in the human intestine and novel insights from metagenomics. Front. Biosci. 14:3214–3221.
Ventura, M., Turroni, F., Motherway, M. O. C., Macsharry, J., and Van Sinderen, D. (2012). Host-microbe interactions that facilitate gut colonization by commensal bifidobacteria. Trends Microbiol. 20, 467–476. doi: 10.1016/j.tim.2012.07.002
Voltan, S., Martines, D., Elli, M., Brun, P., Longo, S., Porzionato, A., et al. (2008). Lactobacillus crispatus M247-derived H2O2 acts as a signal transducing molecule activating peroxisome proliferator activated receptor-γ in the intestinal mucosa. Gastroenterol. 135, 1216–1227. doi: 10.1053/j.gastro.2008.07.007
Yadav, A. K., Tyagi, A., Kaushik, J. K., Saklani, A. C., Grover, S., and Batish, V. K. (2013). Role of surface layer collagen binding protein from indigenous Lactobacillus plantarum 91 in adhesion and its anti-adhesion potential against gut pathogen. Microbiol. Res. 168, 639–645. doi: 10.1016/j.micres.2013.05.003
Keywords: Aloreña table olives, Lactobacillus pentosus, probiotics, in silico analysis, carbohydrate metabolism, host interaction
Citation: Abriouel H, Pérez Montoro B, Casimiro-Soriguer CS, Pérez Pulido AJ, Knapp CW, Caballero Gómez N, Castillo-Gutiérrez S, Estudillo-Martínez MD, Gálvez A and Benomar N (2017) Insight into Potential Probiotic Markers Predicted in Lactobacillus pentosus MP-10 Genome Sequence. Front. Microbiol. 8:891. doi: 10.3389/fmicb.2017.00891
Received: 23 March 2017; Accepted: 02 May 2017;
Published: 22 May 2017.
Edited by:
Joaquin Bautista-Gallego, Consejo Superior de Investigaciones Cientificas (CSIC), SpainReviewed by:
Konstantinos Papadimitriou, Agricultural University of Athens, GreeceStefania De Domenico, Consiglio Nazionale delle Ricerche – Istituto di Scienze delle Produzioni Alimentari, Italy
Copyright © 2017 Abriouel, Pérez Montoro, Casimiro-Soriguer, Pérez Pulido, Knapp, Caballero Gómez, Castillo-Gutiérrez, Estudillo-Martínez, Gálvez and Benomar. This is an open-access article distributed under the terms of the Creative Commons Attribution License (CC BY). The use, distribution or reproduction in other forums is permitted, provided the original author(s) or licensor are credited and that the original publication in this journal is cited, in accordance with accepted academic practice. No use, distribution or reproduction is permitted which does not comply with these terms.
*Correspondence: Hikmate Abriouel, aGlrbWF0ZUB1amFlbi5lcw==