- LIPM, Université de Toulouse, INRA, CNRS, Castanet-Tolosan, France
The cAMP-dependent transcriptional regulator Clr of Sinorhizobium meliloti regulates the overall number of infection events on Medicago roots by a so-far unknown mechanism requiring smc02178, a Clr-target gene of unknown function. In order to shed light on the mode of action of Clr on infection and potentially reveal additional biological functions for Clr, we inventoried genomic Clr target genes by transcriptome profiling. We have found that Clr positively controls the synthesis of cAMP-dependent succinoglycan as well as the expression of genes involved in the synthesis of a so-far unknown polysaccharide compound. In addition, Clr activated expression of 24 genes of unknown function in addition to smc02178. Genes negatively controlled by Clr were mainly involved in swimming motility and chemotaxis. Functional characterization of two novel Clr-activated genes of unknown function, smb20495 and smc02177, showed that their expression was activated by the same plant signal as smc02178 ex planta. In planta, however, symbiotic expression of smc02177 proved independent of clr. Both smc02177 and smb20495 genes were strictly required for the control of secondary infection on M. sativa. None of the three smc02177, smc02178 and smb20495 genes were needed for plant signal perception. Altogether this work provides a refined view of the cAMP-dependent Clr regulon of S. meliloti. We specifically discuss the possible roles of smc02177, smc02178, smb20495 genes and other Clr-controlled genes in the control of secondary infection of Medicago roots.
Introduction
Sinorhizobium meliloti is a gram-negative bacterium that alternates between a free-living saprophytic life in the soil and the rhizosphere of plants, and an occasional symbiotic life within nodules of Medicago spp. Establishment of symbiosis requires the coordinated bacterial infection of the root epidermis and initiation of nodule organogenesis in the root cortex (Oldroyd, 2013). Neo-formed nodules are invaded intracellularly by bacteria that differentiate into nitrogen-fixing bacteroides. Infection of root hair cells takes place via specialized structures called epidermal Infection Threads (eIT) (Miri et al., 2016). Two bacterial effector molecules are required for eIT formation: Lipo-chitooligosaccharides (LCOs, also known as Nod factors), which are also needed for nodule organogenesis, and exopolysaccharides such as succinoglycan in S. meliloti (Cheng and Walker, 1998; Jones et al., 2007; Gibson et al., 2008).
Mechanisms negatively controlling the symbiotic interaction have also been identified. The best known is AON (autoregulation of nodulation) by which the plant systemically adjusts the number of nodules to its metabolic needs (Magori and Kawaguchi, 2009; Mortier et al., 2012). We recently obtained preliminary evidence that eIT formation was also negatively autoregulated in the S. meliloti–Medicago symbiosis (Tian et al., 2012). Specifically, we isolated S. meliloti mutants that displayed a hyper-infection phenotype on Medicago roots, resulting from a relaxed control of eIT formation. Whereas eIT formation by wild-type bacteria is transient, mutants kept infecting roots for an extended time-lapse, thus allowing increased secondary infection. Noteworthy, nodulation by hyper-infecting bacterial mutants was normal thus indicating that autoregulation of infection and nodulation are distinct mechanisms.
In S. meliloti, the control of secondary infection is mediated by a bacterial cAMP-cascade involving three receptor-like adenylate cyclases (ACs), CyaD1 (SMc02176), CyaD2 (SMc04307) and CyaK (SMb20776), a cAMP-dependent transcriptional regulator of the Crp family (Green et al., 2014), called Clr (SMc02175), and smc02178, a gene of unknown biochemical function located nearby cyaD1 and clr on the chromosome. In nodules, a so-far unknown plant signal activates cAMP production by CyaD1, CyaD2 and CyaK, which in turns allows activation of smc02178 transcription by Clr. This signal is also present in shoots but very low in roots (Tian et al., 2012). We and others have shown that purified Clr is as a 3′5′cAMP-dependent DNA-binding protein that binds a Clr-box in the promoter region of the smc02178 gene (Mathieu-Demaziere et al., 2013; Krol et al., 2016). Inactivation of clr, of smc02178 or of the three AC genes altogether, resulted in a hyper-infection phenotype on M. sativa (Tian et al., 2012). Noteworthy, individual mutants of any of the three ACs had no conspicuous hyper-infection phenotype thus indicating all three genes contribute full infection control, probably upon acting at different stages of the symbiotic interaction (Tian et al., 2012).
In order to shed light on the mechanism by which S. meliloti bacteria control secondary infection, we have identified here additional Clr targets by transcriptome profiling. We have found that Clr positively controls expression of 25 genes of unknown function as well the synthesis of succinoglycan and of a putative unknown polysaccharide. We compare our results with those recently obtained upon over-expression of CyaJ (Krol et al., 2016). Functional characterization of two genes of unknown function, smc02177 and smb20495, demonstrated their implication in the control of secondary infection. We discuss the possible roles of smc02177, smc02178, smb20495 and Clr-controlled surface polysaccharides in the control of secondary infection of Medicago roots.
Materials and Methods
Bacterial Strains and Culture Conditions
Bacterial strains used in this study are listed in Supplementary Table 1. Unless otherwise indicated, strains were grown at 28°C in VMG medium, i.e., Vincent minimal medium (Becker et al., 2004), supplemented with mannitol (1%w/vol) and glutamate (0.1%) as carbon and nitrogen sources, respectively. The concentrations of antibiotics used for S. meliloti were 200 μg/ml for streptomycin, 100 μg/ml for neomycin and 10 μg/ml for tetracycline in both liquid and solid media. Gentamicin was used at 10 μg/ml in liquid medium and 30 μg/ml on solid medium.
Primers used for DNA amplification are listed in Supplementary Table S2. S. meliloti Rm1021 was used as template for DNA amplification. The smb20495 and smc02177 single mutants were constructed by site-specific integration of the suicide pVO155 plasmid. smb20495 and smc02177 internal PCR fragments were amplified using 20495L-20495R, and L02177-R02177 primers (Supplementary Table S2), cloned into pCR2-1-TOPO and digested with BamHI and XbaI before cloning into pVO155. The resulting pVO155 derivatives were introduced into E. coli DH5α by transformation and then conjugated in S. meliloti using pRK600 as helper plasmid. All constructs were verified by PCR and Sanger sequencing in E. coli and by PCR in S. meliloti.
Plasmids Construction
Plasmids used in this study are listed in Supplementary Table S1. The clr-overexpressing construct pFA2175 was obtained after PCR amplification of the clr gene coding region using S. meliloti Rm1021 genomic DNA as template and the REco2175 and LBamH2175 primers (Supplementary Table S2). The PCR fragment was digested with BamHI and EcoRI and ligated into a BamHI-EcoRI digested pFAJ1708 plasmid. For pGD926-ExoY construction, the promoter region and the first 297nt of exoY coding region were PCR-amplified from pstb-LAFR5-ExoY plasmid DNA using the ExoY-fusHindIII and ExoY-fusBamHI primers. The PCR fragment was digested with BamHI and HindIII and ligated into a BamHI-HindIII digested pGD926 plasmid.
For Lsmc02178-lacZ construction (pGMI50322), the smc02178 coding region including signal peptide was amplified using the 2178H and 2178BamHIlacZ primers. The HindIII-BamHI resulting PCR product was subsequently cloned into HindIII and BamHI-digested pGD926. For the phoA fusions, the signal-peptide containing fusion (Lsmc02178-phoA) was obtained using the primers 2178H and 2178EcoRVphoAL whereas the short construction (Ssmc02178-phoA) lacking endogenous signal peptide was obtained using the 2178H and 2178EcoRVphoAc primers. In parallel, the phoA gene from E. coli genomic DNA was amplified using the primers phoAEcoRV and phoABamHI and cloned into pGEM plasmid. The phoA and Lsmc02178 or Ssmc02178 fragments were ligated together, PCR-amplified, digested by Hind III and BamHI before cloning into HindIII/BamHI digested-pGD926. Constructs were conjugated into a phoA S. meliloti derivative (Rm8002) to minimize phosphatase alkaline background activity. For the construction of pGD20495, a 934 bp PCR fragment encompassing the smb20495 promoter region (full intergenic region) and 26 nucleotides of the smb20495 gene was amplified using the SMb20495-HindIII and SMb20495-BamHI primers, digested with BamHI and HindIII and cloned in-frame with lacZ in the translational fusion plasmid pGD926 For pGD2177, a 303 bp PCR fragment encompassing the full intergenic region between smc02177 and smc02178 and 32 nucleotides of the smc02177 gene was amplified using the p2177HindIII and p2177BamHI primers, digested with BamHI and HindIII and cloned in the in-frame orientation at the same sites of the lacZ translational fusion plasmid pGD926.
Transcriptome and Quantitative PCR Analyses
For transcriptome experiments, S. meliloti clr- (GMI11567) and clr-overexpressing (GMI11896) strains (Table 1) were grown overnight at 28°C in VMG medium, diluted to an OD600 of 0.12 in 20 ml of VMG medium and incubated under either oxic or microoxic (2%O2) conditions in the presence of caffeine (2.5 mM), to inhibit endogenous phosphodiesterase activity (Essayan, 2001). Microoxic conditions were achieved as described before (David et al., 1988). When OD600 reached 0.3, 15 ml were filtered on Supor® Membrane Disk Filters (Pall), and stored at -80°C. RNA preparations were as described in Bobik et al. (2006).
Transcriptome analyses were performed using 70-mer oligonucleotide microarrays representative of 6,207 predicted open reading frames (ORFs) of S. meliloti Rm1021. The protocol was as described before (Bobik et al., 2006). Microarray raw data have been deposited in the ArrayExpress database under accession number E-MTAB-4780.
For exocAMP-induction assays of exo and flaB gene expression (Figure 1 and Supplementary Figure S2, respectively), overnight cultures of S. meliloti strains at 28°C in VMG medium were diluted to an OD600 of 0.12 in 20 ml of same medium and supplemented with 5 mM (final concentration) of 3′-5′-cAMP (Sigma) when cultures reached an OD600 of 0.3. When exocAMP-treated cultures reached an OD600 of 0.6, 15 ml were filtered on Supor® Membrane Disk Filters (Pall) and cells were frozen in liquid nitrogen and stored at -80°C. All experiments were performed in triplicate.
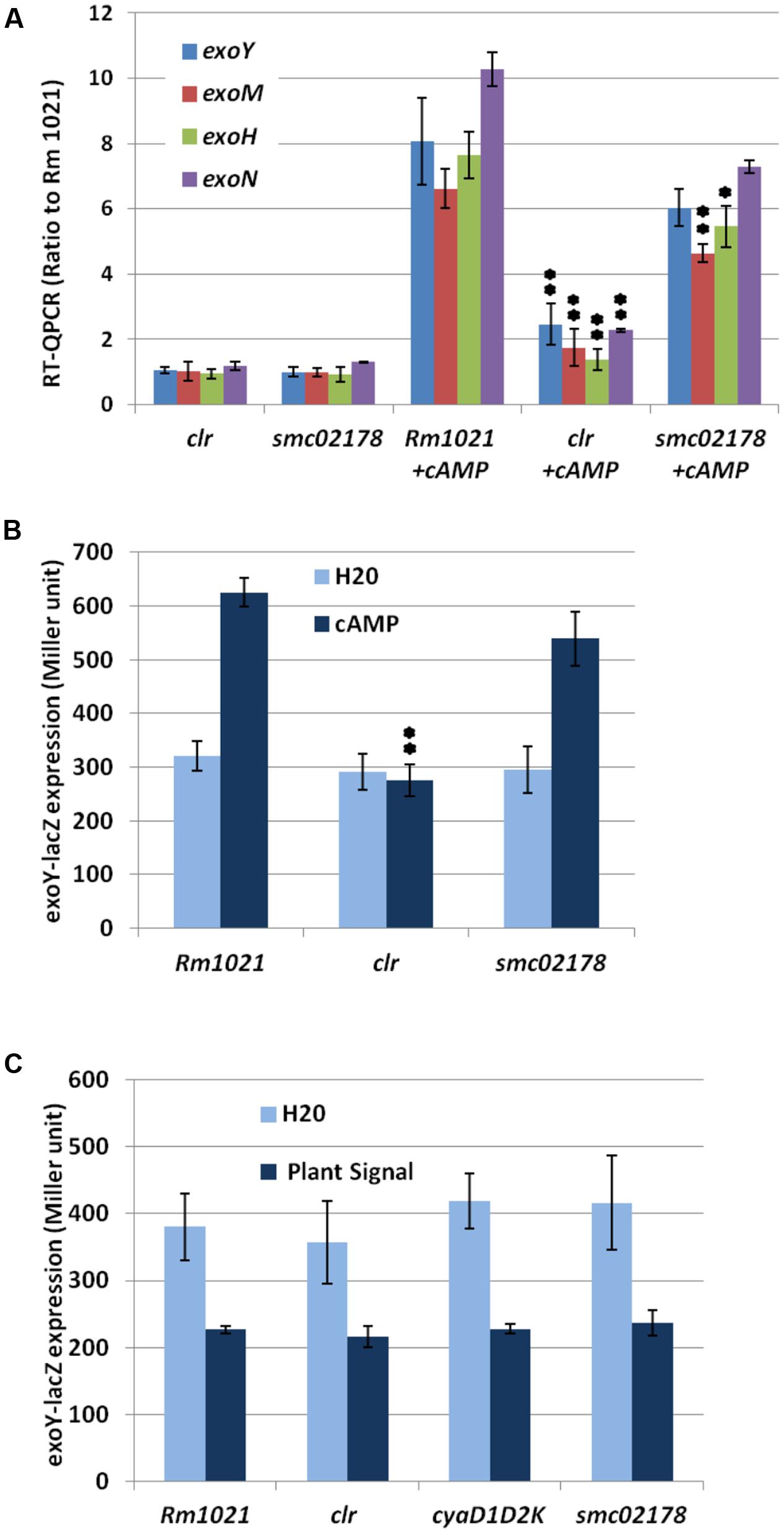
FIGURE 1. Clr and cAMP control succinoglycan production. (A) RT-qPCR analysis of exo genes expression in response to 5 mM exocAMP, expressed as ratio to Rm1021 wt strain. (B) Expression of a translational exoY-lacZ reporter fusion in the presence of 10 mM exocAMP. (C) Expression of a translational exoY-lacZ reporter fusion in the presence of plant shoot extract. smc02178-lacZ expression was included as an internal control for plant shoot extract activity but data were omitted in (C) for clarity. ∗ and ∗∗ indicate statistical significance at p ≤ 0.05 and p < 0.01, respectively, with respect to Rm1021+cAMP.
Reverse transcriptions were performed from 1 μg of RNA using the Transcriptor Reverse Transcriptase (Roche), and random hexamers as primers. cDNAs were used for running real-time PCR on a LightCycler 1.5 system (Roche) using the FastStart DNA Master PLUS SYBRGreen I kit (Roche) according to the manufacturer’s instructions. The rplM gene was used as reference gene for data normalization. Gene expression was assessed using the second derivative maximum analysis.
β-Galactosidase and Alkaline Phosphatase Assays
Sinorhizobium meliloti strains carrying the pGD926-ExoY, pGD2178, pGD20495 or pGD2177 plasmids were grown at 28°C in VMG medium. Overnight cultures were diluted to an OD600 of 0.1 in 10 ml Vincent medium and additionally grown for 2 h. Cultures were then supplemented with 10 mM exocAMP for pGD926-ExoY (Figure 1B) and grown for an additional 24 h. S. meliloti strains carrying plasmids pGMI50322, pGMI50323, pGMI50324, and pGD2178 were grown overnight in 1 ml-cultures in VMG medium supplemented with 100 μl of Medicago shoot signal fresh extract prepared as described in Tian et al. (2012). Although a plant shoot extract is slightly less active than a nodule extract (Tian et al., 2012) it was preferred as it is easier to prepare ex temporaneously.
The assays for beta-galactosidase activity were carried out using the protocol of Miller (1972), whereas alkaline phosphatase was assayed in a S. meliloti phoA background as described before (Brickman and Beckwith, 1975). All experiments were performed in triplicate.
Symbiotic detection of beta-galactosidase activity in Medicago nodules was conducted as described before (Tian et al., 2012).
Calcofluor Dye Detection of Succinoglycan
Sinorhizobium meliloti strains were grown at 28°C in LB-MC (LB+2.5 mM MgCl2 and 2.5 mM CaCl2). Overnight cultures were diluted to an OD600 of 0.15 and 10 μl were dropped off as a spot on LB-MC plates supplemented with 0.02% calcofluor white (Fluorescent Brightener 28, Sigma). After 2 and 13 days incubation, the fluorescence was monitored under UV light with a G:BOX BioImaging System (Syngene).
Motility Assays
104 cells (ca 5 μl) from overnight cultures in LB-MC medium were jabbed on top of soft (0.2%) agar plates. Motility (i.e., size of the colony) was measured after 5 days at 28°C.
Results
Transcriptome Profiling of the Clr Regulon
We compared the transcriptomes of a S. meliloti strain (GMI11896) expressing clr from a constitutive nptII promoter on a low copy-number plasmid and of a nearly isogenic clr null mutant strain (GMI11567). Experiments were performed under both free-living aerobic and microoxic conditions, as low oxygen is a known symbiotic signal (Soupene et al., 1995). Both experiments, performed in biological duplicate, revealed a similar list of genes with the same extent of induction or repression thus indicating that oxygen did not affect Clr activity. We identified 72 genes displaying at least a twofold change in gene expression in both oxic and microoxic conditions, i.e., in 4 independent assays (Table 1). Most of these genes are probably indirect Clr targets (see below).
Sinorhizobium meliloti has a tripartite genome consisting of one main chromosome (3.65 Mb) and two symbiotic replicons called pSymB (1.68 Mb) and pSymA (1.35 Mb) (Galibert et al., 2001). The 42 genes activated by Clr were all located on pSymB and chromosome with the exception of the pSymA-located nodP1 gene which was likely a false positive that was detected because of its very strong (99.7%) sequence similarity with nodP2 (see below).
Targets included six genes involved in succinoglycan synthesis (exoN, Y, H, F1, W, U), an exopolysaccharide that plays a key role in IT formation and stress adaptation.
Seven genes belong to a large ca. 30-kb cluster on pSymB between genes nodP2 and smb21248 that contains many genes involved in polysaccharide metabolism (see S. meliloti genome browser1), (Galibert et al., 2001).
Clr-targets in this cluster encompass two categories of genes: three genes involved in activated sulfate (PAPS) biosynthesis and sulfate transfer (NodP2, NodQ2, SMb21243) and four genes involved in sugar/polysaccharide metabolism including a glycosyl transferase (SMb21242), a beta-glucosidase (SMb21069), a sugar (GlcNAc-containing) deacetylase (SMb21247) and Smb21240, a protein with a tyrosine kinase domain resembling surface exopolysaccharide export protein of the ExoP family. Altogether, Clr target genes in this cluster may contribute synthesis of a sulfated surface polysaccharide (see Discussion).
Additional Clr targets included the lpsS-encoded sulfotransferase (Cronan and Keating, 2004), cyaF2 encoding an adenylate/guanylate cyclase of unknown function, as well as 25 genes of unknown function (including smc02178), most of which were located on the main chromosome.
Thirty genes were repressed by Clr under both oxic and microoxic conditions, most of which (25) were chromosomal (Table 1). Ten genes belonged to a large chemotaxis/motility cluster (from smc03004 to smc03072) involved in flagella synthesis, swimming motility and chemotaxis. Related genes were smc01104 (mcpX), smc01468 (cheW2) and smc04114 (pilA1). We also detected two genes involved in nitrogen metabolism (glnK, glnII) and 11 genes of unknown function. We identified a conspicuous Clr-box in the promoter region of 12 Clr target genes (Table 1 and Supplementary Table S3, see Discussion).
Clr and cAMP Drive Succinoglycan Synthesis
RT-qPCR analysis of four exo genes (exoH, exoM, exoN, and exoY) in S. meliloti wt and clr mutant cells supplemented with exogenous cAMP (abbreviated thereafter as exocAMP) (Figure 1A) confirmed that the clr gene indeed mediated exocAMP-activation of these genes. Inactivation of the direct Clr-target smc02178 had no significant effect on exoY and exoN expression but slightly decreased exoM and exoH gene expression (Figure 1A). Expression of a translational exoY-lacZ reporter fusion (Figure 1B) confirmed the clr-dependency and smc02178-independency of the exocAMP-mediated exoY-lacZ expression. Noteworthy, exoY-lacZ ex planta expression was not induced by a plant shoot extract (Figure 1C) nor nodule extract thus indicating that cAMP-dependent exo gene expression and smc02178 expression are under different genetic control. Instead a plant extract had a negative effect on exoY expression that was, however, independent of Clr.
In order to directly assess the implication of Clr in succinoglycan biosynthesis, we monitored fluorescence of bacterial colonies on agar plates containing the calcofluor white dye which is specific for succinoglycan in strain Rm1021 (Jones, 2012). Under conditions where wt S. meliloti showed only background fluorescence, bright fluorescence was triggered (Figure 2) in a wt strain carrying the pGMI50127 plasmid that constitutively synthesizes endogenous cAMP (endocAMP) (Tian et al., 2012). Bright fluorescence was almost completely abolished in the clr mutant in agreement with previous results (Krol et al., 2016) whereas inactivation of the smc02178 gene had no detectable effect on overall succinoglycan synthesis (Figure 2).
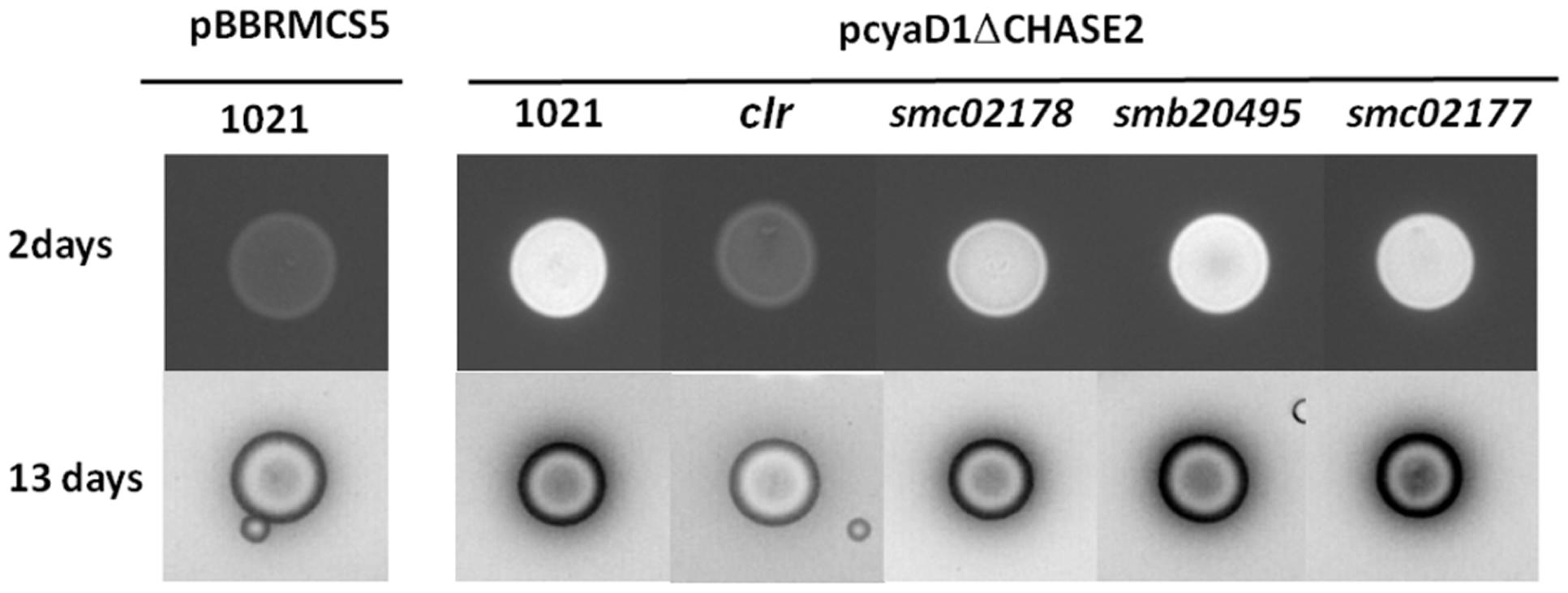
FIGURE 2. Calcofluor white detection of endocAMP-dependent succinoglycan production in different S. meliloti genetic backgrounds after 2 and 13 days incubation of plates at 28°C. The pCyaD1ΔCHASE2 plasmid produces endocAMP constitutively. Pictures at 13 days are inversed images that better show the halo of LMW succinoglycan. The pictures shown have been taken from one of 3 independent replicates. Original images are shown in Supplementary Figure S1.
The presence of a halo of similar size corresponding to diffusible low molecular weight succinoglycan around the wt and smc02178 bacterial colonies indicated that succinoglycan secretion was not impaired in the smc02178 mutant (Figure 2).
clr Negatively Controls Motility
exo and fla genes show opposite regulation in many biological instances, including symbiosis and stress responses (Mendrygal and Gonzalez, 2000; Ruberg et al., 2003; Hellweg et al., 2009). We confirmed by RT-qPCR that clr regulates flaB transcription negatively, in accordance with transcriptome experiments (Supplementary Figure S2). A swimming motility assay showed a reduced swim radius of the wild type strain producing endocAMP that was not due a reduced growth of the strain carrying plasmid pGMI50127 (Supplementary Figure S2). Inhibition of motility by endocAMP was indeed mediated by clr as observed before (Krol et al., 2016).
Two Novel Clr-Target Genes Involved in the Control of Secondary Infection
Among the 25 genes of unknown biological function activated by Clr we characterized two of them; smb20495, which was the most highly-induced gene in the transcriptome experiment (Table 1) and smc02177, the gene located next to smc02178 on the chromosome.
We first tested activation of smb20495- and smc02177-lacZ translational gene fusions in free-living cultures supplemented by a plant shoot extract known to activate smc02178 expression (Tian et al., 2012). We found that free-living expression of both genes was activated by the plant signal in a clr- and cyaD1D2K-dependent manner, although to a lower level as compared to smc02178 (Figure 3A).
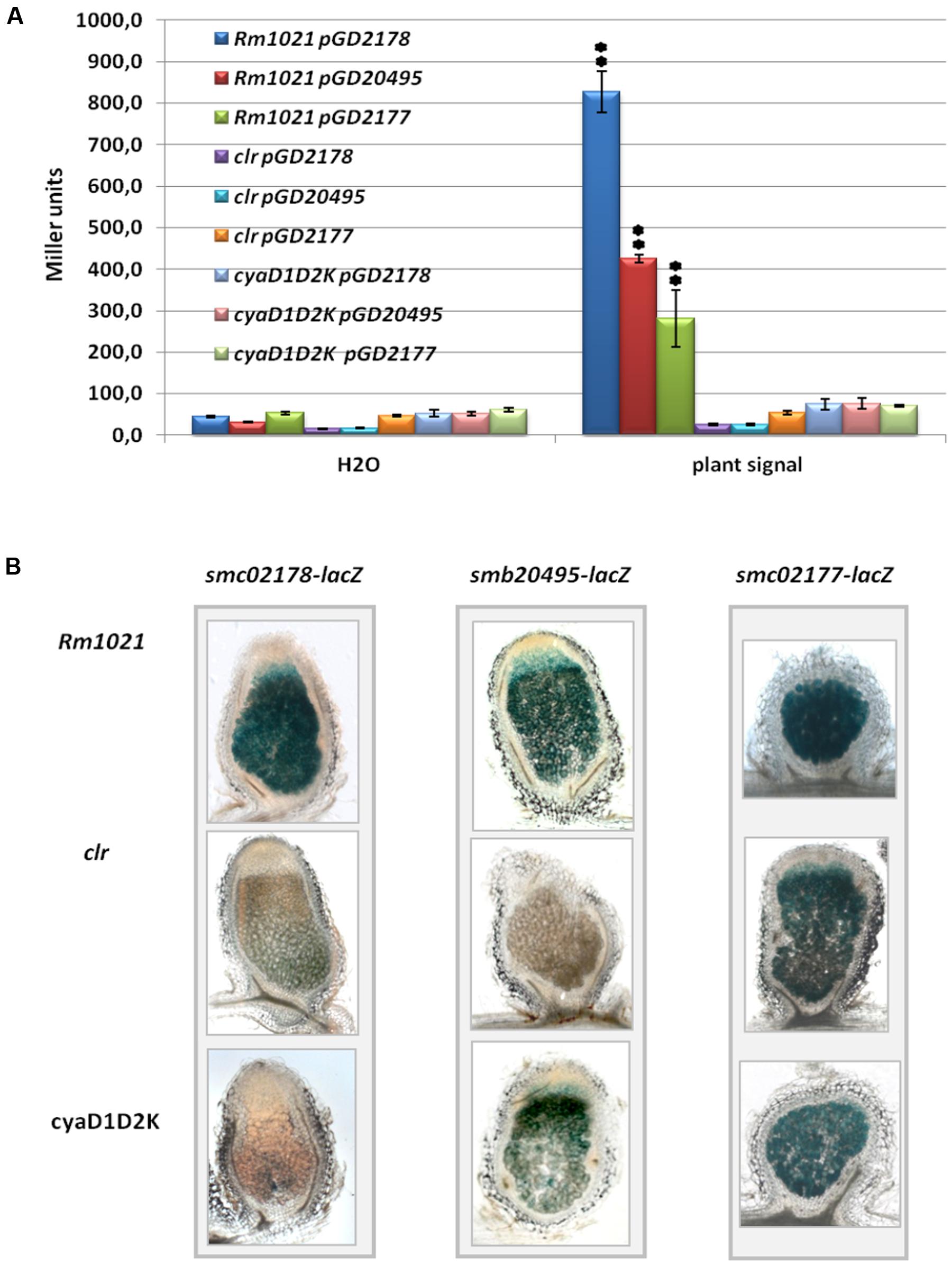
FIGURE 3. smc02177 and smb20495 gene expression. (A) Activation of smc02178-lacZ, smb20495-lacZ and smc02177-lacZ fusions by a Medicago shoot extract ex planta. ∗∗Indicates statistical significance at p < 0.01 with respect to water control. (B) smc02178-lacZ, smb20495-lacZ and smc02177-lacZ expression in planta, respectively. The images featuring smc02178-lacZ expression have been retrieved from Tian et al. (2012) for comparison.
In planta, the pattern of smb20495-lacZ expression in nodules was similar to the one previously described for smc02178 (Figure 3B) and expression strictly depended on clr. However, although 30% of the nodules induced by the cyaD1D2K mutant were colorless, 70% stained pale blue. Probably one of the many ACs in the S. meliloti genome (25 besides CyAD1CyaD2CyaK; Galibert et al., 2001) allows low level of cyaD1D2K-independent expression of the smb20495 gene in planta.
The situation was even more contrasted for the smc02177-lacZ fusion. Although smc02177 displayed very little clr-independent expression ex planta (Figure 3A), its expression in mature nodules was essentially clr and cyaD1D2K-independent (Figure 3B). A likely explanation is that a Clr-independent promoter gets strongly activated in nodules.
Null mutants of S. meliloti in smb20495 and smc02177 genes displayed a full hyper-infection phenotype on M. sativa (Figure 4) comparable to the one previously observed for a smc02178 or clr mutant (Tian et al., 2012). However, no difference in nodule number was observed (Figure 4A). Nodules were pink and leaves were green thus indicating that the mutants were not affected for nitrogen fixation, as demonstrated before for a triple cyaD1D2K mutant (Tian et al., 2012). Both mutants synthesized endocAMP-dependent HMW and diffusible LMW succinoglycan on calcofluor white dye plates, similar to the smc02178 mutant (Figure 2).
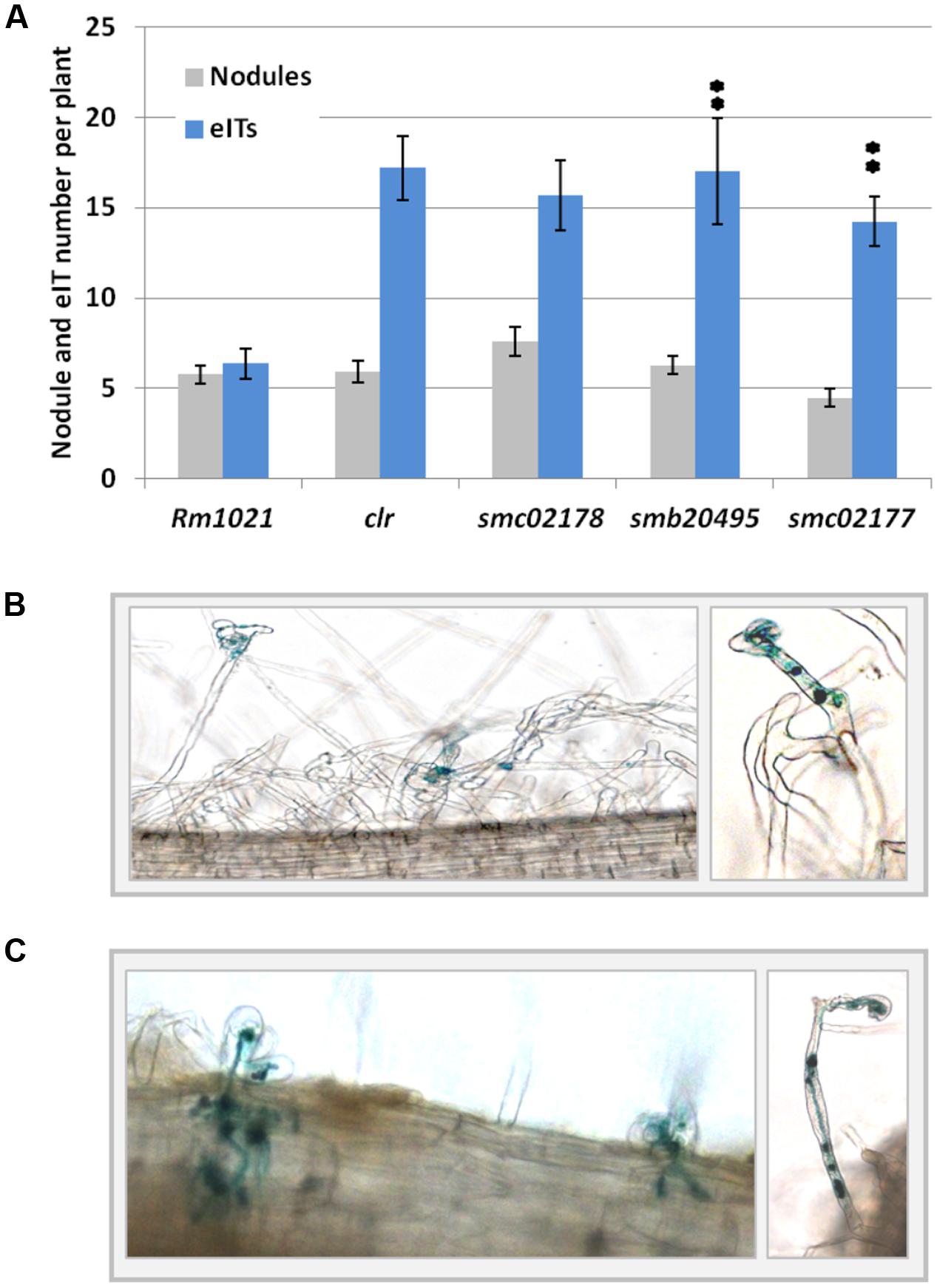
FIGURE 4. smb20495 and smc02177 control secondary infection. (A) Nodule and eITs number counting. ∗∗Indicates statistical significance at p < 0.01 with respect to Rm1021. Data for clr and smc02178 have been retrieved here from Tian et al. (2012) and are shown here for comparison. (B,C) Medicago sativa roots hyperinfected by smb20495 and smc02177 mutants, respectively. Note occasional defense reactions and aborted eITs.
Altogether these results indicate that smb20495 and smc02177 expression are prone to plant signal regulation under clr and cyaD1D2K control. Nevertheless smc02177 showed a high-level of plant signal-independent symbiotic expression in nodules. Both genes were required for the control of secondary infection.
Sequence Analysis of the SMc02177, SMc02178 and SMb20495 Proteins
PSORTb 3.0 analysis (Yu et al., 2010) predicted a non-cytoplasmic location for all three SMc02177, SMc02178 and SMb20495 proteins without specifying more precisely their subcellular localization. SignalP 4.1 analysis (Petersen et al., 2011) indicated the presence of a cleavable signal peptide in SMc02178 and SMb20495. As for SMc02178, we experimentally demonstrated the localization of the main portion of the protein in the bacterial periplasm using a combination of lacZ and phoA reporter fusions (Supplementary Figure S3).
Contrary to SMc02178 for which sequence inspection gave no structural or functional clues, we identified a FecR domain (IPR006860, PF04773) in SMc02177. In the E. coli full-length FecR protein, the FecR domain interacts with the amino-terminal periplasmic part of the FecA outer membrane protein involved in iron citrate signaling. Sequence inspection of the large SMb20495 protein indicated the presence of several TPR domains (IPR019734) known to mediate protein–protein interactions.
The smc02177, smc02178 and smb20495 Genes Are Not Required for Plant Signal Perception
The predicted extra-cytoplasmic location of the three proteins and the presence of protein–protein interaction motifs in two of them suggested to us the possibility that these proteins were involved in the perception/transduction of the plant signal that activates the CyaD1, CyaD2 and CyaK ACs (Tian et al., 2012). We therefore monitored plant shoot signal-dependent expression of plasmidic smc02177- and smc02178-lacZ fusions in the three mutant backgrounds. For the smc02177-lacZ fusion no statistically significant difference in expression was observed in any mutant background as compared to wild-type. For the smc02178 mutation a weak but significant (p < 0.05) effect was observed on smc02178-lacZ expression (Figure 5).
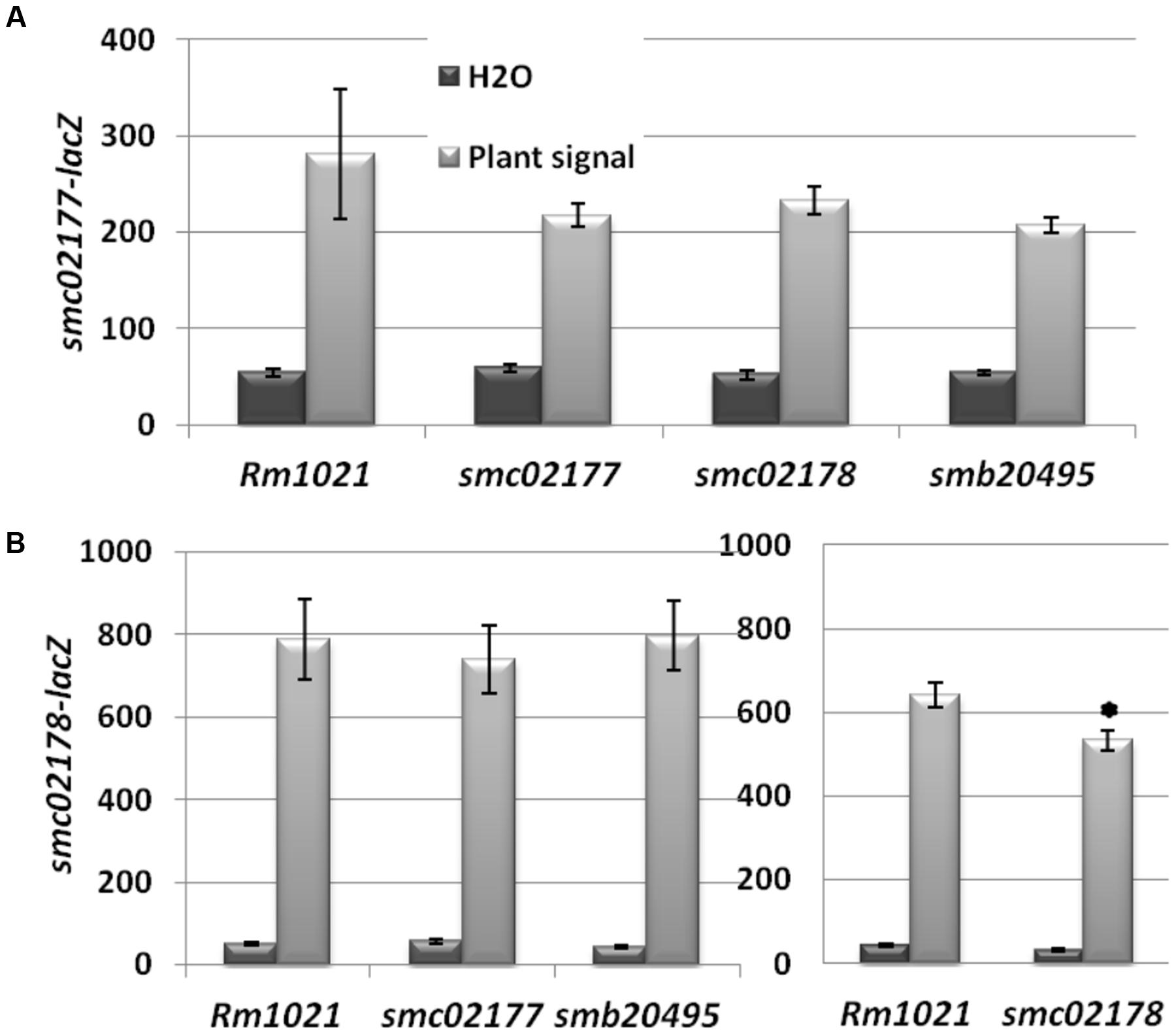
FIGURE 5. smc02177, smc02178 and smb20495 are not involved in plant signal perception. (A) smc02177-lacZ expression (expressed as Miller Units). (B) smc02178-lacZ expression. ∗Indicates a statistically significant difference at p < 0.05 with respect to Rm1021 control.
Altogether, none of the genes under test had a significant effect on plant signal perception that could account for its hyper-infection associated phenotype (see Discussion).
Discussion
The Clr Regulon
In this work, we compared the transcriptome of a strain moderately overexpressing clr (6- to 8-fold, Table 1) to that of a clr null mutant. As a result we identified 72 genes that showed at least a twofold up- or down-regulation in two biological replicates of the two biological conditions tested, i.e., aerobic and microaerobic (2% O2) conditions.
Krol et al. (2016) recently determined the transcriptome of a strain overexpressing the CyaJ AC to a control strain carrying an empty vector plasmid with the purpose of identifying cAMP-dependent genes in S. meliloti. Of the 42 genes induced by Clr in both aerobic and microaerobic conditions in our experiments, 27 were also identified as being activated by CyaJ using similar thresholds (M > 1 and p < 0.05). These 27 genes are thus bona fide cAMP-dependent Clr targets. Five additional genes in our experiments were found in the immediate vicinity of these bona fide targets. cyaF2 is a Clr target solely detected in our experiments but for which a Clr-binding site was experimentally identified (Krol et al., 2016). CyaF2 is thus probably a genuine Clr (direct) target too. Altogether, we believe that at least 32 genes (over 42) detected here are bona fide Clr- and cAMP-activated targets.
In contrast the list of Clr-repressed genes in our experiments and in previous (Krol et al., 2016) experiments (33 and 82 genes, respectively) showed a very limited overlap; 7 genes all involved in chemotaxis and swimming motility. Metabolic genes evidenced by manipulating intracellular cAMP concentration (Krol et al., 2016) were not detected here and thus may not be Clr targets.
Our data also provide direct evidence that the Clr regulon is wider than the cyaD1D2K symbiotic signaling cascade since Clr targets such as exo and fla genes are not regulated by the plant signal nor its cognate ACs, CyaD1D2K. This is best explained by the fact that they are 28 ACs in the S. meliloti genome beside CyaD1D2K (Galibert et al., 2001). Altogether this suggests that Clr is a central transcriptional regulator that integrates different environmental signals via different ACs, both in the free-living and symbiotic life of S. meliloti.
We initially identified a functional palindromic (TGTTN8AACA) Clr-box in the smc02178 promoter region (Tian et al., 2012). Based on DNA-binding assays, Krol et al. (2016) identified a relaxed consensus (HGTYHCNNNNGRWACA) that we have found in the promoter regions of nine predicted Clr targets [smb20906–smb20908, smc00864, smc00925, smc01136, smc01210, smc02177, smc03985 (cyaF2), smc04164 and smc04190]. Upon studying smb20495 expression, we have observed that a promoter extending 500 bp upstream of the start ATG codon and carrying the Clr-like box (HGTYHCNNNNGRWACT) was indeed inducible by Clr and cAMP (Figure 3A) whereas a shorter promoter version (285 bp upstream of ATG) lacking this putative Clr-box was not (Krol et al., 2016). On this ground we tentatively propose a novel HGTYHCNNNNGRWACW Clr-box consensus that we have also found in the smb21329 promoter (Table 1 and Supplementary Table S3). We acknowledge that a functional binding assay is needed to validate this prediction. No functional (e.g., nCRNA) or structural element (e.g., RIME) was identified so far in the 500 nt region between the Clr-box and the smb20495 start codon2.
In summary, 10 to 12 Clr-activated genes identified in this work could be direct Clr targets. Nine of them are chromosomal and three located on pSymB. Noteworthy pSymB-located exo genes and the gene cluster encoding the putative polysaccharide (nodP2-smb21248) may be indirect Clr targets. Similarly, none of the Clr-repressed genes involved in flagellin synthesis and chemotaxis showed a potential Clr-box matching the [HGTYHCNNNNGRWACW] consensus. So, Clr-repressed genes are likely indirect targets. Although more work is needed to precisely define the full Clr (direct) regulon our data suggest that the Clr-regulon mainly consists of chromosome-located genes positively regulated by Clr.
Clr-Mediated Control of Legume Root Secondary Infection
We have described here two novel Clr-target genes strictly required for the control of secondary plant infection. Inactivation of any of these two genes led to a hyperinfection phenotype similar to the one observed with a smc02178, clr or a triple cyaD1cyaD2cyaK mutant. Hence, both genes have a unique and essential role in the control of secondary infection. Similar to sm02178, smb20495 and smc02177 were expressed at a very low level ex planta in standard culture conditions and were activated by the plant signal ex planta in a cyaD1D2K- and clr-dependent manner. All three genes were expressed in nodules. Yet smc02177 expression in mature nodules was essentially independent of clr and cyaD1cyaD2cyaK (Figure 3B), likely reflecting a dual regulation of this gene.
The mechanism by which the three Clr-targets restrict secondary infection now needs to be elucidated. No enzymatic function was predicted for any of the corresponding proteins. Instead two of them displayed protein–protein interaction motifs (TPR) or domain (FecR).
We speculated that (some of) these proteins could be involved in plant signal perception. Our present results, however, do not lend support to this possibility (Figure 5). However, the observation that the CyaD1, CyaD2 and CyaK ACs act at successive stages of nodule development suggested that different signal molecules could be associated with them (Tian et al., 2012) and indeed we have obtained genetic evidence for other(s) signal in addition to the one evidenced so far in nodules and shoots. Hence the role of the sm02178, smb20495 and smc02177 genes in signal sensing would have to be reevaluated if more signals were discovered.
A second possibility is that the sm02178, smb20495 and smc02177 genes are involved in the secretion of an effector molecule or MAMP whose recognition by a plant receptor may dampen root susceptibility to infection. In this respect, the transcriptome profiling experiment pointed to two candidate MAMP molecules. The first candidate is cAMP-dependent succinoglycan. Considering the well-established (positive) role of succinoglycan in primary infection it is tempting to speculate that succinoglycan may play a negative role during secondary infection as well. Two lines of evidence, however, argue against implication of cAMP-dependent succinoglycan in the control of secondary infection: (i) the individual smc02177, smc02178 and smb20495 mutants -although displaying a full hyperinfection phenotype- were not affected in cAMP-dependent succinoglycan synthesis nor secretion (Figure 2) (ii) cAMP-dependent exoY gene did not depend on the plant shoot signal (Figure 1C) for expression ex planta, contrary to genes (smc02177, smc02178, and smb20495) involved in infection control. Altogether the data suggest that succinoglycan by itself is not the MAPM molecule but does not exclude succinoglycan is part of the MAMP biosynthetic or export pathway.
The second candidate molecule is a polysaccharide compound putatively encoded by a ca. Thirty kilobyte nodP2-smb21248 gene cluster in which we identified at least seven potential Clr target genes by transcriptome profiling. This cluster altogether encompasses 13 glycosyltransferases, 2 sugar epimerases (SMb21228 and SMb21232), 2 tyrosine kinases (SMb21240 and ExoP2) and 3 succinoglycan-related transport proteins (Wzx1, ExoF2, and ExoF3) potentially involved in surface polysaccharide synthesis. Activation by Clr of nodP2Q2 involved in sulfate activation as well as three sulfotransferases (SMb21237, SMb21243, and SMb21249) in this large cluster as well as the lpsS-encoded sulfotransferase (Cronan and Keating, 2004) on the chromosome suggests a sulfated polysaccharide. Both (cAMP-independent) sulfated LPS and KPS have been described in S. meliloti (Cronan and Keating, 2004; Townsend et al., 2006). Although highly unlikely, the synthesis of a modified Nod factor molecule cannot be completely excluded at this stage.
The structural characterization of cAMP-dependent polysaccharide molecule and their possible implication in the control of secondary infection is now under investigation. This together with the precise subcellular localization of the SMc02177 and SMb20495 proteins as well as the identification of their interacting partners should shed further light to the control of secondary infection in the S. meliloti–Medicago symbiosis.
Author Contributions
LZ, AG, CMD, FS and AMG performed experiments. AMG, JB and CMB designed the research. CMB, AMG and JB wrote the manuscript.
Funding
LZ was supported by a CSC scholarship and FS was partly supported by a Post-doctoral AGREENSKILLS fellowship. This work was funded in part by the ANR “RhizocAMP” (ANR-10-BLAN-1719), the ANR “AOI” (ANR-15-CE20-0004-01) and the Pôle de Compétitivité “Agri Sud Ouest Innovation”. This work is part of the “Laboratoire d’Excellence” (LABEX) entitled TULIP (ANR-10-LABX-41).
Conflict of Interest Statement
The authors declare that the research was conducted in the absence of any commercial or financial relationships that could be construed as a potential conflict of interest.
Acknowledgments
We thank Prof. GC Walker (MIT) and Prof. K. Jones (FSU) for sending the Rm8002 strain and pstb-LAFR5-ExoY plasmid, respectively. We also thank the Florimond-Desprez company (Cappelle en Perche, France) for generous gift of Medicago seeds.
Supplementary Material
The Supplementary Material for this article can be found online at: http://journal.frontiersin.org/article/10.3389/fmicb.2017.01236/full#supplementary-material
Footnotes
- ^ https://iant.toulouse.inra.fr/bacteria/annotation/cgi/rhime.cgi
- ^ https://iant.toulouse.inra.fr/bacteria/annotation/cgi/rhime.cgi
References
Becker, A., Berges, H., Krol, E., Bruand, C., Ruberg, S., Capela, D., et al. (2004). Global changes in gene expression in Sinorhizobium meliloti 1021 under microoxic and symbiotic conditions. Mol. Plant Microbe Interact. 17, 292–303. doi: 10.1094/MPMI.2004.17.3.292
Bobik, C., Meilhoc, E., and Batut, J. (2006). FixJ: a major regulator of the oxygen limitation response and late symbiotic functions of Sinorhizobium meliloti. J. Bacteriol. 188, 4890–4902. doi: 10.1128/JB.00251-06
Brickman, E., and Beckwith, J. (1975). Analysis of regulation of Escherichia coli alkaline-phosphatase synthesis using deletions and Phi-80 transducing phages. J. Mol. Biol. 96, 307–316. doi: 10.1016/0022-2836(75)90350-2
Cheng, H. P., and Walker, G. C. (1998). Succinoglycan is required for initiation and elongation of infection threads during nodulation of alfalfa by Rhizobium meliloti. J. Bacteriol. 180, 5183–5191.
Cronan, G. E., and Keating, D. H. (2004). Sinorhizobium meliloti sulfotransferase that modifies lipopolysaccharide. J. Bacteriol. 186, 4168–4176. doi: 10.1128/JB.186.13.4168-4176.2004
David, M., Daveran, M. L., Batut, J., Dedieu, A., Domergue, O., Ghai, J., et al. (1988). Cascade regulation of nif gene expression in Rhizobium meliloti. Cell 54, 671–683. doi: 10.1016/S0092-8674(88)80012-6
Essayan, D. M. (2001). Cyclic nucleotide phosphodiesterases. J. Allergy Clin. Immunol. 108, 671–680. doi: 10.1067/mai.2001.119555
Galibert, F., Finan, T. M., Long, S. R., Puhler, A., Abola, P., Ampe, F., et al. (2001). The composite genome of the legume symbiont Sinorhizobium meliloti. Science 293, 668–672. doi: 10.1126/science.1060966
Gibson, K. E., Kobayashi, H., and Walker, G. C. (2008). Molecular determinants of a symbiotic chronic infection. Annu. Rev. Genet. 42, 413–441. doi: 10.1146/annurev.genet.42.110807.091427
Green, J., Stapleton, M. R., Smith, L. J., Artymiuk, P. J., Kahramanoglou, C., Hunt, D. M., et al. (2014). Cyclic-AMP and bacterial cyclic-AMP receptor proteins revisited: adaptation for different ecological niches. Curr. Opin. Microbiol. 18, 1–7. doi: 10.1016/j.mib.2014.01.003
Hellweg, C., Puhler, A., and Weidner, S. (2009). The time course of the transcriptomic response of Sinorhizobium meliloti 1021 following a shift to acidic pH. BMC Microbiol. 9:37. doi: 10.1186/1471-2180-9-37
Jones, K. M. (2012). Increased production of the exopolysaccharide succinoglycan enhances Sinorhizobium meliloti 1021 symbiosis with the host plant Medicago truncatula. J. Bacteriol. 194, 4322–4331. doi: 10.1128/JB.00751-12
Jones, K. M., Kobayashi, H., Davies, B. W., Taga, M. E., and Walker, G. C. (2007). How rhizobial symbionts invade plants: the Sinorhizobium-Medicago model. Nat. Rev. Microbiol. 5, 619–633. doi: 10.1038/nrmicro1705
Krol, E., Klaner, C., Gnau, P., Kaever, V., Essen, L. O., and Becker, A. (2016). Cyclic mononucleotide- and Clr-dependent gene regulation in Sinorhizobium meliloti. Microbiology 162, 1840–1856. doi: 10.1099/mic.0.000356
Magori, S., and Kawaguchi, M. (2009). Long-distance control of nodulation: molecules and models. Mol. Cells 27, 129–134. doi: 10.1007/s10059-009-0016-0
Mathieu-Demaziere, C., Poinsot, V., Masson-Boivin, C., Garnerone, A. M., and Batut, J. (2013). Biochemical and functional characterization of SpdA, a 2′, 3′ cyclic nucleotide phosphodiesterase from Sinorhizobium meliloti. BMC Microbiol. 13:268. doi: 10.1186/1471-2180-13-268
Mendrygal, K. E., and Gonzalez, J. E. (2000). Environmental regulation of exopolysaccharide production in Sinorhizobium meliloti. J. Bacteriol. 182, 599–606. doi: 10.1128/JB.182.3.599-606.2000
Miller, G. (1972). Experiments in Molecular Genetics. Cold Spring Harbor, NY: Cold Spring Harbor Laboratory Press.
Miri, M., Janakirama, P., Held, M., Ross, L., and Szczyglowski, K. (2016). Into the root: how cyokinin controls rhizooial infection. Trends Plant Sci. 21, 178–186. doi: 10.1016/j.tplants.2015.09.003
Mortier, V., Holsters, M., and Goormachtig, S. (2012). Never too many? How legumes control nodule numbers. Plant Cell Environ. 35, 245–258. doi: 10.1111/j.1365-3040.2011.02406.x
Oldroyd, G. E. D. (2013). Speak, friend, and enter: signalling systems that promote beneficial symbiotic associations in plants. Nat. Rev. Microbiol. 11, 252–263. doi: 10.1038/nrmicro2990
Petersen, T. N., Brunak, S., von Heijne, G., and Nielsen, H. (2011). SignalP 4.0: discriminating signal peptides from transmembrane regions. Nat. Methods 8, 785–786. doi: 10.1038/nmeth.1701
Ruberg, S., Tian, Z. X., Krol, E., Linke, B., Meyer, F., Wang, Y. P., et al. (2003). Construction and validation of a Sinorhizobium meliloti whole genome DNA microarray: genome-wide profiling of osmoadaptive gene expression. J. Biotechnol. 106, 255–268. doi: 10.1016/j.jbiotec.2003.08.005
Soupene, E., Foussard, M., Boistard, P., Truchet, G., and Batut, J. (1995). Oxygen as a key developmental regulator of Rhizobium meliloti N2-fixation gene expression within the alfalfa root nodule. Proc. Natl. Acad. Sci. U.S.A. 92, 3759–3763. doi: 10.1073/pnas.92.9.3759
Tian, C. F., Garnerone, A. M., Mathieu-Demaziere, C., Masson-Boivin, C., and Batut, J. (2012). Plant-activated bacterial receptor adenylate cyclases modulate epidermal infection in the Sinorhizobium meliloti-Medicago symbiosis. Proc. Natl. Acad. Sci. U.S.A. 109, 6751–6756. doi: 10.1073/pnas.1120260109
Townsend, G. E., Forsberg, L. S., and Keating, D. H. (2006). Mesorhizobium loti produces nodPQ-dependent sulfated cell surface polysaccharides. J. Bacteriol. 188, 8560–8572. doi: 10.1128/JB.01035-06
Yu, N. Y., Wagner, J. R., Laird, M. R., Melli, G., Rey, S., Lo, R., et al. (2010). PSORTb 3.0: improved protein subcellular localization prediction with refined localization subcategories and predictive capabilities for all prokaryotes. Bioinformatics 26, 1608–1615. doi: 10.1093/bioinformatics/btq249
Keywords: rhizobium, legume, symbiosis, infection, adenylate cyclase, cAMP, transcriptome
Citation: Zou L, Gastebois A, Mathieu-Demazière C, Sorroche F, Masson-Boivin C, Batut J and Garnerone A-M (2017) Transcriptomic Insight in the Control of Legume Root Secondary Infection by the Sinorhizobium meliloti Transcriptional Regulator Clr. Front. Microbiol. 8:1236. doi: 10.3389/fmicb.2017.01236
Received: 18 May 2017; Accepted: 19 June 2017;
Published: 06 July 2017.
Edited by:
Alessio Mengoni, University of Florence, ItalyReviewed by:
George Colin DiCenzo, University of Florence, ItalyPeter Mergaert, Centre National de la Recherche Scientifique (CNRS), France
Copyright © 2017 Zou, Gastebois, Mathieu-Demazière, Sorroche, Masson-Boivin, Batut and Garnerone. This is an open-access article distributed under the terms of the Creative Commons Attribution License (CC BY). The use, distribution or reproduction in other forums is permitted, provided the original author(s) or licensor are credited and that the original publication in this journal is cited, in accordance with accepted academic practice. No use, distribution or reproduction is permitted which does not comply with these terms.
*Correspondence: Jacques Batut, jacques.batut@inra.fr Anne-Marie Garnerone, anne-marie.garnerone@inra.fr
†Present address: Amandine Gastebois, GEIHP UPRES EA 3142, Institut de Biologie en Santé PBH-IRIS, Centre Hospitalier Universitaire, Angers, France Céline Mathieu-Demazière, Laboratoire de Microbiologie et Génétique Moléculaires, Centre de Biologie Intégrative, Université de Toulouse, CNRS, UPS, Toulouse, France