- 1Bioengineering and Biosystems Department, Karlsruhe Institute of Technology, Institute of Functional Interfaces, Eggenstein-Leopoldshafen, Germany
- 2Zweckverband Kläranlage Steinhäule, Neu-Ulm, Germany
Seven wastewater treatment plants (WWTPs) with different population equivalents and catchment areas were screened for the prevalence of the colistin resistance gene mcr-1 mediating resistance against last resort antibiotic polymyxin E. The abundance of the plasmid-associated mcr-1 gene in total microbial populations during water treatment processes was quantitatively analyzed by qPCR analyses. The presence of the colistin resistance gene was documented for all of the influent wastewater samples of the seven WWTPs. In some cases the mcr-1 resistance gene was also detected in effluent samples of the WWTPs after conventional treatment reaching the aquatic environment. In addition to the occurrence of mcr-1 gene, CTX-M-32, blaTEM, CTX-M, tetM, CMY-2, and ermB genes coding for clinically relevant antibiotic resistances were quantified in higher abundances in all WWTPs effluents. In parallel, the abundances of Acinetobacter baumannii, Klebsiella pneumoniae, and Escherichia coli were quantified via qPCR using specific taxonomic gene markers which were detected in all influent and effluent wastewaters in significant densities. Hence, opportunistic pathogens and clinically relevant antibiotic resistance genes in wastewaters of the analyzed WWTPs bear a risk of dissemination to the aquatic environment. Since many of the antibiotic resistance gene are associated with mobile genetic elements horizontal gene transfer during wastewater treatment can't be excluded.
Introduction
Antibiotic-resistant intestinal bacteria enter the environment through sewage water treatment plants. Some survive or even multiply during the waste water treatment and are capable of transferring genes to other microorganisms (Davies et al., 2006; Rizzo et al., 2013; Berendonk et al., 2015). There is a potential risk of people getting colonized with these bacteria, for example via contact with wastewater contaminated surface water (Ferrer et al., 2012). The resistant bacteria can cause infections which are difficult to treat because of the insensitivity to antibiotics. It is therefore in the interest of our society to quickly determine whether and how resistant bacteria spread via sewage water—and how this could be prevented.
The worldwide increase of antibiotic-resistant bacteria is considered as a major challenge by the World Health Organization (WHO, 2014), the US Centers for Disease Control and Prevention, and the German Antibiotic Resistance Strategy (DART, 2020; The Federal Ministry of Health, 2012). To minimize the leaking of antibiotics or antibiotic-resistant bacteria into the environment, the use of antibiotics in human and veterinary medicine needs to be reduced. Indications of the importance of sewage water and wastewater treatment plants (WWTP) for the spread of antibiotic-resistant pathogens were already described (Rizzo et al., 2013; Alexander et al., 2015, 2016).
A particular danger is represented by pathogens with a resistance against last resort antibiotics. It can be very difficult to cure patients that suffer from an infection with this kind of resistant bacteria. These serious concerns have been catalyzed by the rapid increase in carbapenemase-producing Enterobacteriaceae expressing enzymes such as KPC-2 (Klebsiella pneumoniae carbapenemase-2) and NDM-1 (New Dehli metallo-beta-lactamase; Kumarasamy et al., 2010; Munoz-Price et al., 2013). The global increase in such carbapenemase-producing Enterobacteriaceae has resulted in increased use of colistin with the risk of emerging resistance. The development of colistin resistance is also directly linked with the agricultural use of human antibiotics, where some countries have actively used colistin in animal production (Hao et al., 2014).
Colistin belongs to the family of polymyxins, cationic polypeptides, with broad-spectrum activity against Gram-negative bacteria, including most species of the family of Enterobacteriaceae. The two polymyxins currently in clinical use are polymyxin B and polymyxin E (colistin), which differ only by one amino acid from each other and have comparable biological activity. The mechanism of resistance to polymyxins is modification of lipid A, resulting in a reduction of polymyxin affinity (Liu et al., 2016). The polymyxin resistance can be traced back to the plasmid mediated mcr-1 gene. The plasmid carrying the mcr-1 is already characterized and can be mobilized by conjugation (Liu et al., 2016). The prevalence of mcr-1 in E. coli from livestock and food in Germany is documented by Irrgang et al. (2016), with the highest prevalence of mcr-1 positive isolates of Enterobacteriaceae from poultry food chains. Another report documented the presence of mcr-1 carrying plasmids in pig slurry in Estonia (Brauer et al., 2016). Furthermore, Ovejero et al. (2017) was able to isolate 30 mcr-1 positive isolates from sewage of two WWTP in Spain. Their analysis suggested, that mcr-1 in Spain besides E. coli has successfully transferred to K. pneumoniae. Bernasconi et al. (2016) also detected the mcr-1 gene in stool samples of travelers returning from India. This indicates the fact that humans can get colonized by mcr-1 resistant bacteria which are acquired through the food chain or from other environmental sources.
We report the prevalence of the plasmid mediated colistin resistance gene mcr-1 together with other clinically relevant antibiotic resistance genes during wastewater treatment at municipal WWTPs in Germany. Quantitative PCR (qPCR) was used for the detection of the antibiotic resistance genes in native wastewater populations aiming on a microbiological risk characterization concerning the dissemination of colistin and other resistance genes into the adjacent aquatic environment.
Materials and Methods
Sampling Sites
A number of seven different German WWTPs were investigated. These WWTPs possess different population equivalents with WWTP-1 440,000, WWTP-2 210,000, WWTP-3 23,500, WWTP-4 46,000, and WWTP-5 26,000, WWTP-6 45,000, and WWTP-7 8,000 (Table 1). All WWTPs performed mechanical separation, followed by activated sludge treatment, and sedimentation steps.
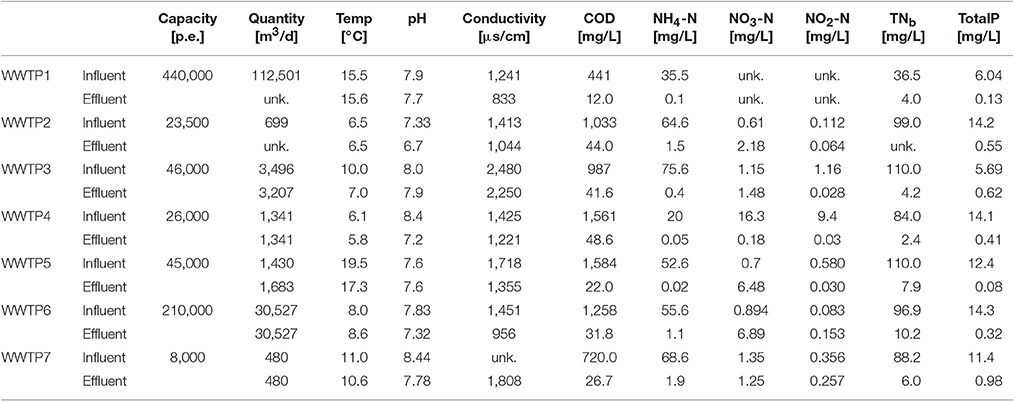
Table 1. List of all investigated wastewater treatment plants with their respective specifications (unk.: unknown).
Whereas, WWTP-1 and WWTP-2 treat wastewater from urban areas including clinical, household, and industrial wastewaters, the wastewaters of the other 4 WWTPs are mainly affected by agricultural catchment areas including animal and food farming. WWTP 7 only treats municipal wastewater without affection of agriculture or industry.
Sampling points were arranged at the influents of WWTPs after mechanical separation and at the effluent sites after sedimentation tanks before the purified wastewater is released into the receiving bodies.
Sample Preparation and DNA Extraction
Volumes of about 100 mL from the 24 h-composite of the WWTP influent samples and about 300 mL of the WWTP effluent samples from the 24 h-composite sample were used for DNA extraction. Wastewater samples were filtered using polycarbonate membranes with a diameter of 47 mm and a pore size of 0.2 μm (Whatman Nuclepore Track-Etched Membranes, Sigma-Aldrich, Munich, Germany). DNA extraction was performed using the Fast DNA spin kit for soil (MP Biomedical, Illkrich, France) utilizing the lysing matrix E according to the manufacturer's protocol for wastewater. The quantities and purities of the DNA extracts were measured by means of the Qubit fluorimetric quantitation (Thermo Scientific, Waldham, USA).
The yield of DNA obtained from the influent water samples ranged from 71 to 244 μg per mL (n = 14) and from 15 to 143 μg per mL (n = 14) for the effluent water samples. To determine the abundances of 7 clinically relevant antibiotic resistant genes including the mcr-1 colistin resistance gene, and the 16S rRNA gene as well as the gene markers for some Enterobacteriaceae qPCR analyses were performed.
Primer Design, qPCR Protocol, and Evaluation
All primers and references are listed in Table 2, which do also contains the accuracy (R2) as well as efficiency (%) values for each qPCR detection system. More specifically, the Escherichia coli NRZ-14408 reference strain is carrying the mcr-1 colistin resistance gene and was kindly provided from National Reference Center in Bochum, Germany. For mcr-1 primer design the NCBI Primer BLAST software was used. For the quantification of the mcr-1 gene in environmental water samples a colistin-resistant E. coli NRZ-14408 (hospital isolate, mcr-1 positive) reference bacterium was used to create a calibration curve, which is shown in Supplementary Material. An R2 value of 0.999 and an efficiency of 97.6% were determined, indicating the high specificity of the mcr-1 detection system.
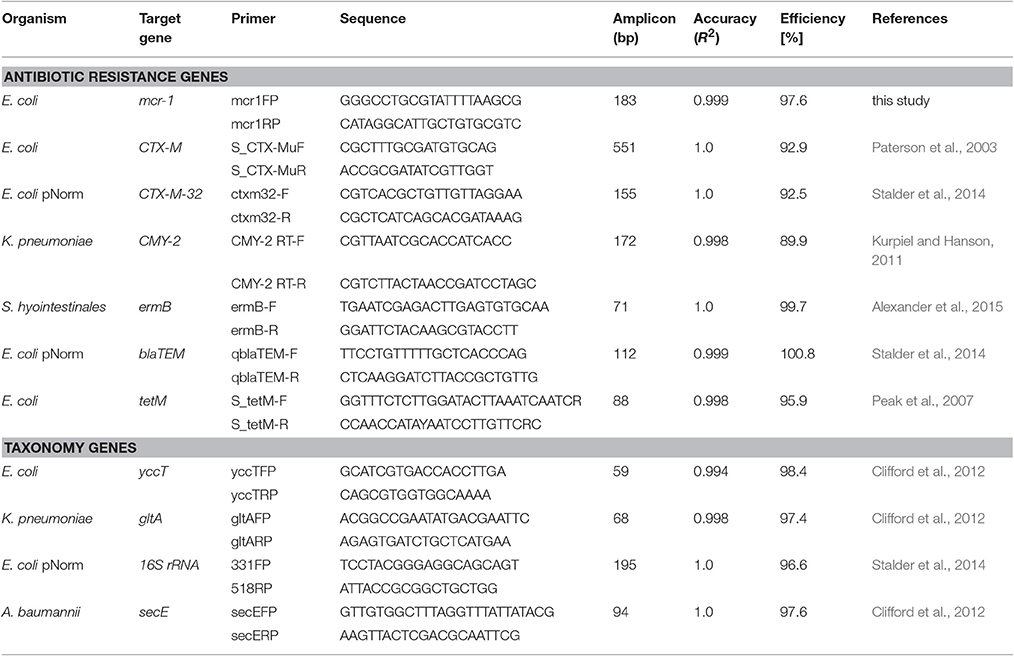
Table 2. List of all used primer systems with their corresponding target gene, expected amplicon size, accuracy, and efficiency of the used calibration curves.
The antibiotic resistance genes blaTEM, CTX-M, CTX-M-32, and CMY-2 are directed against ß-lactam antibiotics, whereas the tetM resistance gene is coding for the resistance against tetracycline and the ermB gene mediates the resistance against erythromycin. All primers, reference strains, and quality values of each detection system are listed in Table 2. In addition calibration and melting curves are given in Supplementary Material. The abundances of these ARGs were quantified in all WWTP effluent wastewater samples via qPCR approach using different reference strains carrying the mentioned resistance genes.
The yccT gene present in E. coli DSM 1103 was used as taxonomic gene marker as well as the gltA gene from K. pneumoniae DSM 30104 (Clifford et al., 2012). Total DNA of pure culture was extracted with the DNA extraction kit for soil (MP Biomedical, Illkrich, France) and subsequently used for the generation of target specific calibration curves. 16S rRNA primers were used to quantify eubacterial rDNA in the water samples for normalization. Here, an already cloned fragment of the eubacterial 16S rRNA gene in the pNORM plasmid of E. coli DH5α was used (Stalder et al., 2014). Plasmid-DNA was extracted with the GeneJet Plasmid Miniprep Kit (Thermo Scientific, Waldham, USA) and was used for the generation of the calibration curve which in turn was used for the calculation of the 16S rRNA gene copy number in water samples.
The mcr-1, ermB, tetM resistance genes, and the four ß-lactamase genes (CTX-M. CTX-M-32, CMY-2, blaTEM), the ribosomal 16S rRNA gene for Eubacteria, and the specific taxonomic gene markers of E. coli, K. pneumonia, and Acinetobacter baumannii were quantified in a SYBR Green qPCR approach. Reactions were run in volumes of 20 μL, containing 10 μL Maxima SYBR Green/ROX qPCR Master Mix (2x) (Thermo Scientific), 8.2 μL of nuclease-free water (Ambion, Life technologies, Karlsbad, Germany), 0.4 μL of the respective primers (stock concentration 10 μM, Table 2), and 1 μL of template DNA (20 ng μL−1). The qPCR protocol comprised 10 min at 95°C for activation of the DNA polymerase followed by 40 cycles of 15 s at 95°C and 1 min at appropriate temperature for primer annealing and elongation (see Supplementary Material). Each water sample was analyzed in technical triplicates. To determine the specificity of the amplification, a melting curve was recorded by raising the temperature from 60 to 95°C (1°C every 10 s). Data analysis was performed by using the Bio-Rad CFX Manager software.
Cell Equivalent Calculation
To calculate the gene copy number of the respective antibiotic resistance gene, the 16S rRNA gene, and taxon-specific gene marker, reference strains carrying the genetic targets of interest were used. With regard to the already known genome sizes of the reference bacteria it is possible to calculate the cell copies. The following equation with an average molecular weight for one base pair of about 650 g/mol, Avogardo's number with 6.022 × 1023 molecules/mol, and a converting factor of 109 ng/g was used.
Serial dilution of DNA stocks were prepared and used for calculation of the calibration curves starting with 500 ng DNA (see Supplementary Material). The coefficient of determination of all standard curves was above 0.994 in all experiments (Table 2), indicating minimal variability within the linear data range.
Using these curves, the measured Ct values of the mcr-1 gene, the 16S ribosomal RNA gene or the taxon-specific gene markers from water samples can be used for copy number calculations (Alexander et al., 2015, 2016). The abundances of antibiotic resistance genes (ARGs) and opportunistic bacteria were quantified in each water sample and normalized to 100 ng total extracted DNA (cell equivalents per 100 ng DNA). In addition, the qPCR data was also normalized to the 16S rRNA gene abundances of the corresponding water samples.
Data Statistics and Presentation
In total 14 biological samples from the influents and effluents of 7 WWTPs were obtained from two individual sampling campaigns in autumn and spring time. For data presentation the standardized box plot diagram was used displaying the distribution of data based on the five number summaries: minimum, first quartile, median, third quartile, and maximum. In the box plot the central rectangle spans the first quantile (P = 25) to the third quantile (P = 75). A segment inside the rectangle shows the median and whiskers above and below the box show the minimum and maximum values. Thus, the box plot displays the full range of variation (from min to max), the likely range of variation, and a typical value (the median). In addition, student's t-test calculations were performed to identify the significance of reduction of gene targets during conventional wastewater treatments.
Propidium Monoazide (PMA) Treatment
PMA can enter dead or injured bacteria due to the loss of their membrane integrities, intercalate with the intracellular and extracellular DNA. The presence of the PMA-DNA complex blocks the polymerase activity at the target sites. In consequence, no PCR product is generated. The protocol is in accordance with that of our previous study (Villarreal et al., 2013) and based on the publications of Nocker et al. (2007a,b) and Nocker and Camper (2009), where the method was optimized also for natural mixed populations. To separate the living bacterial population from dead or injured bacteria during conventional wastewater treatment, the PhAST Blue Photo-Activation System (GenIUL, Barcelona, Spain) was used. After filtration of the influent and effluent wastewater samples originating from WWTP 1 (n = 3), polycarbonate membranes (Nucleopore) were submerged in 300 μL of a 25 μM propidium monoazide (PMA; Biotium, Hayward, California, USA) solution and put into a 1.5 mL colorless tube (SafeSeal tubes, Carl Roth, Karlsruhe, Germany). After incubation in the dark at 4°C for 5 min, samples were exposed to the LED light of the photoactivation system at 100% intensity for 15 min. After PMA treatment, samples were prepared for DNA extraction using the Fast DNA spin kit for soil according to the manufacturer's protocol for wastewater.
Agarose Gel Electrophoresis and Sequencing of mcr-1 Amplicons
The mcr-1 amplicons were separated on a 1% agarose gel to examine the primers and PCR protocols for proper specificity. The results demonstrated that mcr-1 positive water samples generated amplicons with the expected length of 183 bp (Figure 2). Sequence analyses confirmed the mcr-1 identity (see Supplementary Material). For that, the mcr-1 PCR products were purified with ExoSAPit (GE Healthcare, Munich, Germany) before sequencing. The sequencing reactions were performed in a 10 μL reaction volume, including 2 μL of Premix (BigDye® Terminator v1.1 Cycle Sequencing Kit, Applied Biosystems), 0.5 μL primer 517R (10 pM), and 1 μL of purified, 1:3 diluted PCR product. This volume was filled up to 10 μL with sterile water. The temperature profile of the sequencing reaction was as followed: 5 min at 96°C, 25 cycles of 10 s at 96°C, 5 s at 55°C, and 1 min at 60°C, afterwards cooling down to 4°C. For deleting excessive dye, the sequence products were purified with DyeEx2.0 Spin Kit (Qiagen, Hilden, Germany). Three microliters of this product were added to 12 μL of Hi-Di-Formamid (Applied Biosystems) and analyzed in the ABI Prism 310 Genetic Analyser (Applied Biosystems) using POP4 Polymer (Applied Biosystems) and 47 cm × 50 μm capillaries (Applied Biosystems) according to the manufacturer‘s instructions. For database analyses, NCBI (National Center for Biotechnology Information) Blast alignments were performed.
Results
Feasibility of the Detection System Targeting mcr-1 Resistance Gene in Wastewater Population Demonstrated at WWTP-1
The accuracy of the novel qPCR detection system targeting the mcr-1 colistin resistance gene in wastewater samples was demonstrated with the extracted total DNA of original water samples. Here, total DNA from the WWTP-1 with the highest population equivalent of 440.000 was used. Neither irregular amplification results were observed during qPCR nor unexpected shoulders seen during melting curve analyses (Figures 1A,B). After qPCR amplification the amplicons were separated in an agarose gel and resulted in one specific DNA band positioned at the expected product size of 183 bp (Figure 2). The amplicons were also sequenced. The evaluation of the BLAST search confirmed the 99% identity with the mcr-1 resistance gene from E. coli (LC191581), K. pneumoniae (KX377410), Salmonella enterica (KX257482), and Cronobacter sakazakii (KX505142) strain. As a first result of this study it could be shown that this newly developed qPCR system is suited for the reliable quantification of the mcr-1 gene in wastewater populations.
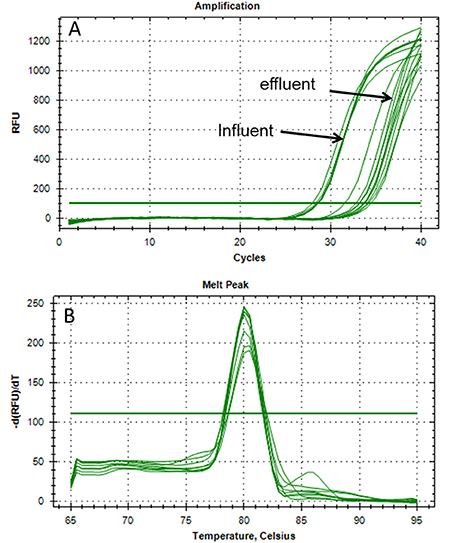
Figure 1. qPCR fluorescence signal of mcr-1 amplification from total extracted DNA. All samples were from influent and effluent wastewater of the WWTP-1. No abnormal amplification results could be observed (A). Change of fluorescence units over increasing temperature (melting curve) of mcr-1 amplicons. No unexpected shoulders were detected above the threshold (B).
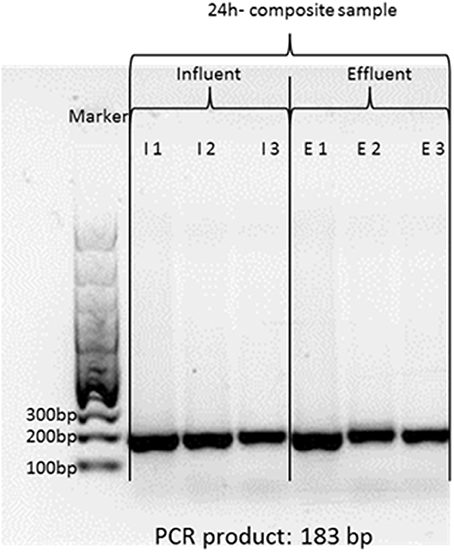
Figure 2. One percent agarose gel of mcr-1 amplicons from influent and effluent of WWTP-1. Theoretical amplification length is 183 base pairs. The gel showed only one specific band at the expected length.
The Ct-values from qPCR were used for cell equivalent calculations normalized to 100 ng total DNA, which is a relative quantification related to the population microbiome. It is independent from the sample volume. Additionally, the cell equivalents from the calibration curve were normalized to the 16S rRNA gene copies as an alternative eubacterial biomass gene marker. It became obvious that the mcr-1 gene was present in influent samples of WWTP-1 with a calculated abundance of 8.11 × 101 cell equivalents per 100 ng total DNA. The median values of cell equivalent per 100 ng DNA of the effluent water samples were quantified with 7.0 × 101. Alternatively, the cell equivalents were also referred to 16S rRNA gene copies and corresponded with 2.64 × 10−8 cell equivalents in the influent and 1.30 × 10−9 cell equivalents in the effluent water samples, respectively. The data from WWTP-1 is indicating the stable presence of mcr-1 gene within the microbiome of the wastewaters of this large WWTP.
Additionally, influent and effluent wastewater samples of WWTP-1 were treated with PMA to discriminate living bacteria from dead bacteria or extracellular DNA (eDNA) prior to DNA extraction. The presence of eDNA, which might be released from dead or injured bacteria, is known to be a promoting factor for horizontal gene transfer (HGT) (Davies et al., 2006; Aminov, 2011). In case of the colistin resistance the mcr-1 gene is located on a conjugative plasmid, which is already described to transfer the colistin resistance among Enterobacteriaceae (e.g., Liu et al., 2016). After normalization to 100 ng DNA and 16S rRNA gene copies slightly reduced cell equivalents were found in case of PMA treatment in influent and effluent samples (influent PMA treated: 6.2 × 100 cell equivalents per 100 ng DNA and 8.92 × 10−8 per 16S rRNA gene copy; effluent PMA treated: 6.2 × 100 cell equivalents per 100 ng DNA; and 3.66 × 10−8 cell equivalents per 16S rRNA gene copy). Comparing PMA-treated with untreated samples of the influent and effluent in maximum one order of magnitude difference became obvious. These results are indicating the presence of low amounts of eDNA or distinct numbers of injured/dead bacteria in wastewater samples, which might have a relevance for transformation and therefore HGT.
Nevertheless, it could be shown that the mcr-1 colistin resistance gene is present in water samples from WWTP-1 and still living bacteria carrying the mcr-1 gene are released to the receiving adjacent body.
Comparing Different WWTPs According to the Presence of mcr-1 Gene
As mentioned before, the influent and effluent wastewaters of 6 additional WWTPs were investigated for the abundance of the mcr-1 gene coding for the colistin resistance in Enterobacteriaceae. In contrast to WWTP-1 the population equivalents of these WWTPs were much lower ranging from 8,000 to 210,000 p.e. The mcr-1 gene was quantified in the influent wastewater samples of all six WWTPs. These mcr-1 positive tested WWTPs treat urban and rural wastewaters including hospitals, livestock, and food industries. Here, the cell equivalents ranged from 4.45 × 101 to 2.01 × 102 in 100 ng total DNA, and 9.89 × 10−8 to 3.44 × 10−7 per 16S rRNA gene copy number (Figure 3).
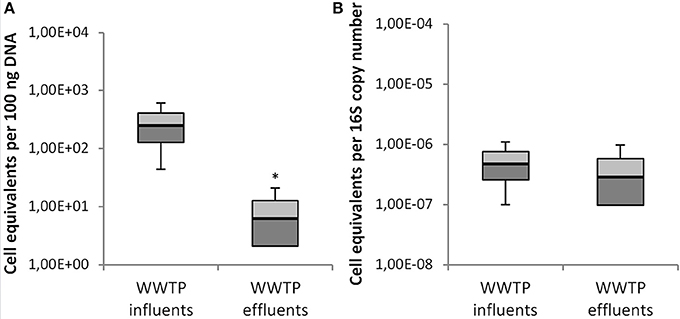
Figure 3. Abundance of mcr-1 cell equivalents in influent and effluent of all 7 WWTP per 100 ng DNA (A) and per 16S rRNA copy number (B). Displayed are the median values as well as the quantils [p = 0.25 (dark), p = 0.75 (bright)] and the standard deviations (n = 14). Significance of reduction is assessed by student's t-test with p ≤ 0.05 and are indicated by asterisk.
Compared to WWTP-1 (440,000 p.e.), the influent waters of these WWTPs showed higher abundances of the mcr-1 gene (Figure 3), which might result from the agricultural or food industry impacts. Even in influent samples of the smallest WWTP-7 (8,000 p.e.) mcr-1 gene copies were detected despite the fact that no hospitals and no intensive animal farms or food industry released wastewaters to this WWTP.
Besides WWTP-1, the mcr-1 gene was detected in the effluent water samples of WWTP-2 and WWTP-3 with 8.4 × 100 to 1.3 × 101 cell equivalents per 100 ng total DNA and 3.88 × 10−7 and 1.55 × 10−7 per 16S rRNA.
Hence, the mcr-1 gene was detected both in the influent waters of all seven WWTPs and in the effluent water samples of WWTP-1, WWTP-2, and WWTP-3.
These WWTPs differed in population equivalents (Table 1), which gives hints that the dimension of the WWTPs does not significantly impact the survival and persistence of mcr-1 gene carrying bacteria. For the mcr-1 gene a maximum reduction of 1 Log scale was determined in WWTP-1, WWTP-2, and WWTP-3.
Detection of Other Clinically Relevant Antibiotic Resistance Genes
Besides the mcr-1 colistin resistance gene, 6 other clinically relevant antibiotic resistance genes were quantified in effluent wastewater samples of the 7 WWTPs. Both the relative quantification and the cell equivalents per 16S rRNA gene copy number are shown in Figures 4, 5. The targeted genes are the ermB gene coding for the erythromycin resistance, the tetracycline resistance gene tetM, and 4 different ß-lactam resistance genes (CTX-M-32, blaTEM, CMY-2, and CTX-M). All resistance genes were distinctly present in all wastewater effluents samples. The most abundant resistance gene was ermB with a median value of 2.39 × 105 cell equivalents in 100 ng DNA or 3.08 × 10−3 cell equivalents per 16S rRNA gene copy number. The second most abundant gene was the tetracycline resistance gene tetM with 1.26 × 104 cell equivalents in 100 ng DNA and 1.68 × 10−4 per 16S rRNA gene copy number. The abundances of the blaTEM, and CTX-M-32 genes were found in a similar range slightly below the tetM gene. The median values for the CMY-2 and CTX-M ß-lactamase genes were detected in a lower abundance of 1.0 × 102 in 100 ng DNA and 8.7 × 10−7 per 16S rRNA gene copy number.
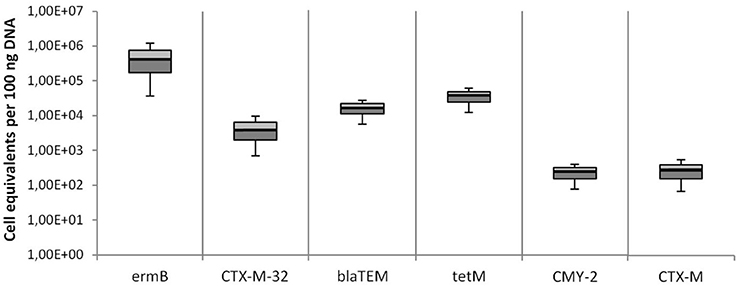
Figure 4. Abundances of antibiotic resistance cell equivalents per 100 ng DNA derived from total extracted DNA of all 7 WWTPs effluents. Erythromycin resistance was detected by ermB gene abundance, ß-lactam resistance genes were detected by CTX-M32, blaTEM, CMY-2, and CTX-M gene abundances, and tetracycline resistance was detected by tetM gene abundance. Displayed are the median values as well as the quantils [p = 0.25 (dark), p = 0.75 (bright)] and the standard deviations (n = 14).
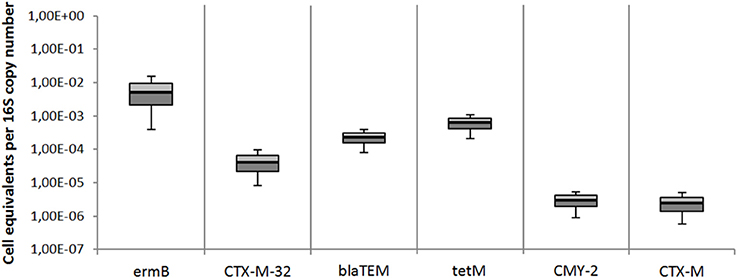
Figure 5. Abundances of antibiotic resistance genes per 16S rRNA gene copy number derived from total extracted DNA of all 7 WWTPs effluents. Erythromycin resistance was detected by ermB gene abundance, ß-lactam resistance genes were detected by CTX-M32, blaTEM, CMY-2, and CTX-M gene abundances, and tetracycline resistance was detected by tetM gene abundance. Displayed are the median values as well as the quantils [p = 0.25 (dark), p = 0.75 (bright)] and the standard deviations (n = 14). Student's t-test values were all >0.05.
In comparison to mcr-1 gene abundances, these resistance genes were much more frequently found and were quantified in higher concentrations in all wastewater effluents released to the aquatic environment. Data from the influent samples of WTTPs demonstrated a reduction of the mentioned resistance genes ranging from 1 Log to maximum of 2 Logs during wastewater treatment (data not shown), which is in accordance with the data targeting the mcr-1 gene.
Opportunistic Pathogens in the Wastewater Samples
The abundance of specific taxonomic gene markers was quantified targeting A. baumannii and the Enterobacteriaceae K. pneumoniae and E coli. These three bacterial species are described to belong to the network of horizontal gene transfer with clinical relevance. All of them are opportunistic pathogens and were shown to hold resistances to colistin by previous studies (Hua et al., 2017; Jeannot et al., 2017). To address the growing concern of emerging colistin resistant pathogens, the abundance of these possible carrier bacteria are also analyzed in the German WWTP of this study.
The Figures 6, 7 summarize the data of all seven investigated WWTPs (influent/effluent) according to the two different normalization approaches, i.e., per 100 ng total DNA (Figures 6A–C) and per 16S rRNA gene copy (Figures 7A–C). Both figures show the abundances of taxonomic gene markers specific for A. baumannii (secE), K. pneumoniae (gltA), and E. coli (yccT). Calibration curves and melting curve analyses for each parameter are documented in Supplementary Material. The obtained Ct values matched with the linear ranges of the calibration curves.
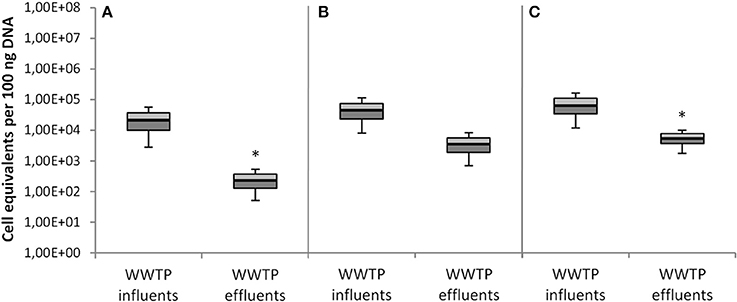
Figure 6. Abundances of taxonomical marker genes per 100 ng of total extracted DNA from all 7 WWTPs. (A) A. baumannii was detected by secE gene abundance, (B) K. pneumoniae was detected by gltA gene abundance, and (C) E. coli was detected by yccT gene abundance. Displayed are the median values as well as the quantils [p = 0.25 (dark), p = 0.75 (bright)] and the standard deviations (n = 14). Significance of reduction is assessed by student's t-test with p ≤ 0.05 and are indicated by asterisk.
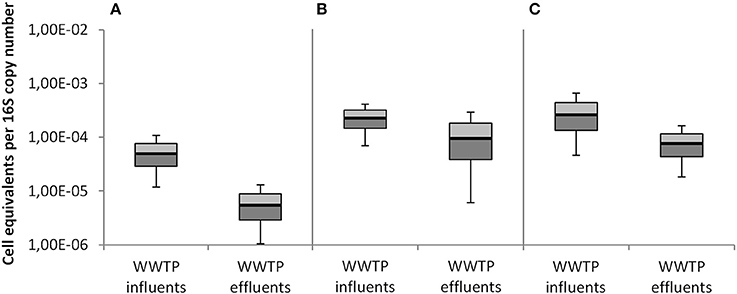
Figure 7. Abundances of taxonomical marker genes per 16S rRNA gene copy number derived from total extracted DNA of all seven WWTPs. (A) A. baumannii was detected by secE gene abundance, (B) K. pneumoniae was detected by gltA gene abundance, and (C) E. coli was detected by yccT gene abundance. Displayed are the median values as well as the quantils [p = 0.25 (dark), p = 0.75 (bright)] and the standard deviations (n = 14). Student's t-test values were all >0.05.
The median values of the influent samples were calculated for the specific taxonomic genes with 1.12 × 104 for A. baumannii, 2.15 × 104 for K. pneumoniae, and 2.96 × 104 for E. coli cell equivalents per 100 ng total DNA. Decreased median values per 100 ng DNA resulted from the effluent samples for all three target genes, i.e., 1.05 × 102 for A. baumannii, 1.60 × 103 for K. pneumoniae, and 1.80 × 103 for E. coli (Figure 6). In total, the abundances of these taxonomic gene markers decreased during conventional wastewater treatment with 1–2 orders of magnitudes, but are still present in significant amounts in effluent samples.
Figure 7 illustrates the abundances of taxonomical marker genes referred to the 16S rRNA copy number. The median values were analyzed and resulted in 2.17 × 10−5 cell equivalents for A. baumannii, 8.25 × 10−5 for K. pneumoniae, and 1.3 × 10−4 for E. coli in the influents samples. The investigation of cell equivalents in the effluent samples resulted in a median of 2.60 × 10−6 for A. baumannii, 5.86 × 10−5 for K. pneumoniae, and 3.29 × 10−5 for E. coli.
Discussion
To the best of our knowledge, this is the first study that demonstrated the occurrence of the colistin resistance mcr-1 gene in bacterial populations of wastewater. The mcr-1 gene was detected in influent samples of all seven WWTPs and was not eliminated during wastewater treatment reaching the aquatic environment. The overall abundances, expressed as cell equivalents per 100 ng DNA or eubacterial 16S rRNA gene copy numbers are still low and live/dead analyses demonstrated that the mcr-1 gene was present in living bacteria, released to receiving bodies.
The colistin resistance can be traced back to the plasmid carrying the mcr-1 gene (Liu et al., 2016). This plasmid is already characterized and can be mobilized by conjugation. Regarding wastewater systems a potential high risk for horizontal gene transfer of the mcr-1 carrying conjugative plasmid is present, since many factors promoting horizontal gene transfer are occurring in water samples from WWTPs (Bellanger et al., 2014). The combination of high cell densities in activated sludge tanks, increased nutrient availability, co-selections by heavy metals and selective pressures like complex mixtures of low-concentrated xenobiotics (e.g., antibiotics, biocids, disinfectants, pharmaceuticals) is hypothesized to promote horizontal gene transfer and therefore the persistence of certain antibiotic resistant bacteria in wastewater environments (Rizzo et al., 2013; Berendonk et al., 2015). This is of major concern because it has been shown, that some human pathogens are involved in high rates of horizontal gene transfer like K. pneumoniae (Hu et al., 2016; Navon-Venezia et al., 2017) and could enhance the dissemination of the mcr-1 plasmid. Potential mcr-1 carrying opportunistic pathogens, i.e., A. baumannii, E. coli, and K. pneumoniae were quantified in higher abundances even at effluent sampling sites.
Cultivation experiments of specific mcr-1 positive Enterobacteriaceae from wastewater populations failed due to the low abundances of the target bacteria and due to the high abundance of accompanying bacterial flora, which overgrew the agar plates supplemented with polymyxin for colistin resistant Enterobacteriaceae.
The dissemination of colistin resistant bacteria via municipal wastewaters is only one way of dissemination of these antibiotic resistant bacteria. Other important dissemination pathways of antibiotic resistant pathogens were already mentioned (Vaz-Moreira et al., 2014). It needs to be studied how far the abundance of the mcr-1 gene target is changing over times. An increasing number of this gene target, especially in clarified wastewater samples released to the aquatic environment, is then directly linked with an increasing microbiological risk potential and would underline the requirements of additional treatment steps to eliminate these opportunistic and antibiotic resistant bacteria.
According to the results of this study, the release of antibiotic resistance genes and opportunistic pathogens from WWTPs in significant amounts to the environment was demonstrated and underlines the requirement of an expanded wastewater treatment (Rizzo et al., 2013; Alexander et al., 2015). Most available data for the selected antibiotic resistance genes derived from clinical studies. Here, the occurrence of blaTEM, CTX-M, CTX-M-32, and CMY-2, all present in the investigated wastewater samples, leads to resistance against ß-lactam antibiotics. ß-lactams made up 30% of all prescript antibiotics in Germany (ECDC, 2015). As a consequence, the high prescription may lead to an increase resistance gene evolution and finally dissemination to the wastewaters. The blaTEM gene, quantified in high abundance of the WWTP effluents under investigation, is responsible for high percentages of ampicillin resistance in E. coli and is also prevalent in K. pneumoniae (Cooksey et al., 1990). It is considered to be a precursor of the extended-spectrum-ß-lactamases (ESBL) (Emery and Weymouth, 1997). CTX-M type ß-lactamases are the most widespread types of ESBL (Bonnet, 2004). Plasmids containing blaCTX-M genes often contain also blaTEM as well as blaOXA genes (Poirel et al., 2005). The CTX-M-32 gene mediates a high resistance in pseudomonads and renders ceftazidime completely ineffective (Fernández et al., 2007). In addition, the CMY-2 gene represents a resistance gene against carbapenems. Carbapenems are used when therapies with other ß-lactam antibiotics failed (Bauernfeind et al., 1996).
It became obvious, that these ß-lactam resistance genes were still present in all effluent samples indicating an insufficient reduction during conventional wastewater treatment. This was also demonstrated by the t-test calculations indicated by values with p < 0.05. In addition, the ermB gene provides resistance against erythromycin, which is a macrolide antibiotic used as substitute for penicillin for patients with allergies against penicillin or infections with resistant bacteria to ß-lactams (Jelić and Antolović, 2016). Macrolides are used for respiratory or gastrointestinal infections and erythromycin made up to 16% of all antibiotics in Germany (ECDC, 2015). Finally, the tetM resistance gene mediates resistance against tetracycline, the second most common antibiotic in the world and currently used as food additive in animal feeding (Gu and Karthikeyan, 2005). Tetracycline and erythromycin often reach WWTP in sub-letal concentrations which might promote the spread of the corresponding resistances through horizontal gene transfer (Alexander et al., 2015).
In consequence, the integration of oxidative, chemical-physical, or membrane-based technologies for an adjusted wastewater treatment is a necessity to interrupt dissemination pathways of ARBs and ARGs to the aquatic environments. The fate of antibiotic resistant and opportunistic pathogens in different environmental habitats depends on species and genetic backgrounds. Some opportunistic pathogens are highly adaptable to stress situation and are able to activate effective stress responses to survive adverse growth conditions (nutrient limitation, low temperatures, etc.; Alexander et al., 2015, 2016). Hence, these microorganisms are able to persist in uncomfortable situation and will not die. The risk of contamination via direct contact of humans with insufficient cleaned wastewater or via vegetables irrigated with contaminated water (direct or indirect re-use) can't be excluded. The degree of environmental contamination via wastewater effluents depends on (1) the densities of WWTPs in a specific area, (2) the dimension of conditioned wastewaters released to the receiving bodies, and (3) the catchment area of the WWTP. It became clear by this study, that the size of the WWTP is not an adequate parameter to discuss a possible reduction of antibiotic resistance. The reduction was limited to only 1 to a maximum of 2 Logs during conventional treatment in all WTTPs ranging from low to high population equivalents. Thus, WWTPs should include an effective disinfection technique to avoid an environmental contamination with clinically relevant microbes to stop the dissemination and evolution of antibiotic resistances. Such an effort would also contribute to an effective protection of reservoirs for drinking water production even in industrialized countries. In fact, no national or international regulations or threshold values are available that mention the dissemination of clinically relevant microbes including antibiotic resistances via WWTPs to the aquatic environments. In fact, integrated evaluation concepts to assess the efficiency of advanced wastewater treatment processes for the elimination of antibiotic resistant bacteria and micro-pollutants are already published (Ternes et al., 2017) and are supposed to be a useful tool to initialize regulation processes.
Author Contributions
TS: Experimental organization, preparing the manuscript. NH, JA, and FS: Authors performed experiments and generate the scientific data. Co-authors of the manuscript. CH: Local support at the municipal wastewater treatment plants, sampling procedures, providing WWTP specific data. ER: Providing sequencing data.
Conflict of Interest Statement
The authors declare that the research was conducted in the absence of any commercial or financial relationships that could be construed as a potential conflict of interest.
Acknowledgments
We thank the BMBF for financial support within the framework of the HyReKA-project (02WRS1377B) as part of the RiSKWa-program. The HyReKA research project is headed by Prof. Dr. Martin Exner (University Clinics Bonn, Germany). We acknowledge support by Deutsche Forschungsgemeinschaft and Open Access Publishing Fund of Karlsruhe Institute of Technology. TS is supporting the COST Nereus and the NORMAN WG5. We also thank Dr. Chistophe Merlin (University of Lorraine, Nancy) providing the E. coli reference strain carrying the pNORM plasmid.
Supplementary Material
The Supplementary Material for this article can be found online at: http://journal.frontiersin.org/article/10.3389/fmicb.2017.01282/full#supplementary-material
References
Alexander, J., Bollmann, A., Seitz, W., and Schwartz, T. (2015). Microbiological characterization of aquatic microbiomes targeting taxonomical marker genes and antibiotic resistance genes of opportunistic bacteria. Sci. Total Environ. 512–513, 316–325. doi: 10.1016/j.scitotenv.2015.01.046
Alexander, J., Knopp, G., Dötsch, A., Wieland, A., and Schwartz, T. (2016). Ozone treatment of conditioned wastewater selects antibiotic resistance genes, opportunistic bacteria, and induce strong population shifts. Sci. Total Environ. 559, 103–112. doi: 10.1016/j.scitotenv.2016.03.154
Aminov, R. I. (2011). Horizontal gene exchange in environmental microbiota. Front. Microbiol. 2:158. doi: 10.3389/fmicb.2011.00158
Bauernfeind, A., Stemplinger, I., Jungwirth, R., and Giamarellou, H. (1996). Characterization of the plasmidic beta-lactamase CMY-2, which is responsible for cephamycin resistance. Antimicrob. Agents Chemother. 40, 221–224.
Bellanger, X., Guilloteau, H., Bonot, S., and Merlin, C. (2014). Demonstrating plasmid-based horizontal gene transfer in complex environmental matrices: a practical approach for a critical review. Sci. Total Environ. 493, 872–882. doi: 10.1016/j.scitotenv.2014.06.070
Berendonk, T. U., Manaia, C. M., Merlin, C., Fatta-Kassinos, D., Cytryn, E., Walsh, F., et al. (2015). Tackling antibiotic resistance: the environmental framework. Nat. Rev. Microbiol. 13, 310–317. doi: 10.1038/nrmicro3439
Bernasconi, O. J., Kuenzli, E., Pires, J., Tinguely, R., Carattoli, A., Hatz, C., et al. (2016). Travelers can import colistin-resistant Enterobacteriaceae, including those possessing the plasmid-mediated mcr-1 gene. Antimicrob. Agents Chemother. 60, 5080–5084. doi: 10.1128/AAC.00731-16
Bonnet, R. (2004). Growing group of extended-spectrum β-lactamases: the CTX-M enzymes. Antimicrob. Agents Chemother. 48, 1–14. doi: 10.1128/AAC.48.1.1-14.2004
Brauer, A., Telling, K., Laht, M., Kalmus, P., Lutsar, I., Remm, M., et al. (2016). Plasmid with colistin resistance gene mcr-1 in extended-spectrum-β-lactamase-producing Escherichia coli strains isolated from pig slurry in Estonia. Antimicrob. Agents Chemother. 60, 6933–6936. doi: 10.1128/AAC.00443-16
Clifford, R. J., Milillo, M., Prestwood, J., Quintero, R., Zurawski, D. V., Kwak, Y. I., et al. (2012). Detection of bacterial 16S rRNA and identification of four clinically important bacteria by real-time PCR. PLoS ONE 7:e48558. doi: 10.1371/journal.pone.0048558
Cooksey, R., Swenson, J., Clark, N., Gay, E., and Thornsberry, C. (1990). Patterns and mechanisms of beta-lactam resistance among isolates of Escherichia coli from hospitals in the United States. Antimicrob. Agents Chemother. 34, 739–745. doi: 10.1128/AAC.34.5.739
Davies, J., Spiegelman, G. B., and Yim, G. (2006). The world of subinhibitory antibiotic concentrations. Curr. Opin. Microbiol. 9, 445–453. doi: 10.1016/j.mib.2006.08.006
ECDC (2015). European Center of Disease Prevention and Control. Distribution of Antibacterials for Systemic Use (ATC group J01) in the Community (Primary Care Sector) in Germany, Reporting Year 2015. Available online at: http://ecdc.europa.eu/en/healthtopics/antimicrobial-resistance-and-consumption/antimicrobial-consumption/esac-net-database/Pages/consumption-rates-by-country.aspx
Emery, C. L., and Weymouth, L. A. (1997). Detection and clinical significance of extended-spectrum beta-lactamases in a tertiary-care medical center. J. Clin. Microbiol. 35, 2061–2067.
Fernández, A., Gil, E., Cartelle, M., Pérez, A., Beceiro, A., Mallo, S., et al. (2007). Interspecies spread of CTX-M-32 extended-spectrum ß-lactamase and the role of the insertion sequence IS1 in down-regulating bla CTX−M gene expression. J. Antimicob. Chemother. 59, 841–847. doi: 10.1093/jac/dkm030
Ferrer, A., Nguyen-Viet, H., and Zinsstag, J. (2012). Quantification of diarrhea risk related to wastewater contact in Thailand. Ecohealth 9, 49–59. doi: 10.1007/s10393-012-0746-x
Gu, C., and Karthikeyan, K. G. (2005). Interaction of tetracycline with aluminum and iron hydrous oxides. Environ. Sci. Technol. 39, 2660–2667. doi: 10.1021/es048603o
Hao, H., Cheng, G., Iqbal, Z., Ai, X., Hussain, H. I., Huang, L., et al. (2014). Benefits and risks of antimicrobial use in food-producing animals. Front. Microbiol. 5:288. doi: 10.3389/fmicb.2014.00288
Hu, Y., Yang, X., Li, J., Lv, N., Liu, F., Wu, J., et al. (2016). The bacterial mobile resistome transfer network connecting the animal and human microbiomes. Appl. Environ. Microbiol. 82, 6672–6681. doi: 10.1128/AEM.01802-16
Hua, X., Liu, L., Fang, Y., Shi, Q., Li, X., Chen, Q., et al. (2017). Colistin resistance in Acinetobacter baumannii MDR-ZJ06 revealed by a multiomics approach. Front. Cell. Infect. Microbiol. 7:45. doi: 10.3389/fcimb.2017.00045
Irrgang, A., Roschanski, N., Tenhagen, B. A., Grobbel, M., Skladnikiewicz-Ziemer, T., Thomas, K., et al. (2016). Prevalence of mcr-1 in E. coli from livestock and food in Germany, 2010-2015. PLoS ONE 11:e159863. doi: 10.1371/journal.pone.0159863
Jeannot, K., Bolard, A., and Plésiat, P. (2017). Resistance to polymyxins in Gram-negative organisms. Int. J. Antimicrob. Agents 49, 526–535. doi: 10.1016/j.ijantimicag.2016.11.029
Jelić, D., and Antolović, R. (2016). From erythromycin to azithromycin and new potential ribosome-binding antimicrobials. Antibiotics 5:29. doi: 10.3390/antibiotics5030029
Kumarasamy, K. K., Toleman, M. A., Walsh, T. R., Bagaria, J., Butt, F., Balakrishnan, R., et al. (2010). Emergence of a new antibiotic resistance mechanism in India, Pakistan, and the UK: a molecular, biological, and epidemiological study. Lancet Infect. Dis. 10, 597–602. doi: 10.1016/S1473-3099(10)70143-2
Kurpiel, P. M., and Hanson, N. D. (2011). Association of IS5 with divergent tandem blaCMY-2 genes in clinical isolates of Escherichia coli. J. Antimicrob. Chemother. 66, 1734–1738. doi: 10.1093/jac/dkr212
Liu, Y. Y., Wang, Y., Walsh, T. R., Yi, L. X., Zhang, R., Spencer, J., et al. (2016). Emergence of plasmid-mediated colistin resistance mechanism MCR-1 in animals and human beings in China: a microbiological and molecular biological study. Lancet Infect. Dis. 16, 161–168. doi: 10.1016/S1473-3099(15)00424-7
Munoz-Price, L. S., Poirel, L., Bonomo, R. A., Schwaber, M. J., Daikos, G. L., Cormican, M., et al. (2013). Clinical epidemiology of the global expansion of Klebsiella pneumoniae carbapenemases. Lancet Infect. Dis. 13, 785–796. doi: 10.1016/S1473-3099(13)70190-7
Navon-Venezia, S., Kondratyeva, K., and Carattoli, A. (2017). Klebsiella pneumoniae: a major worldwide source and shuttle for antibiotic resistance. FEMS Microbiol. Rev. 41, 252–275. doi: 10.1093/femsre/fux013
Nocker, A., and Camper, A. K. (2009). Novel approaches toward preferential detection of viable cells using nucleic acid amplification techniques. FEMS Microbiol. Lett. 291, 137–142. doi: 10.1111/j.1574-6968.2008.01429.x
Nocker, A., Sossa, K. E., and Camper, A. K. (2007a). Molecular monitoring of disinfection efficacy using propidium monoazide in combination with quantitative PCR. J. Microbiol. Methods 70, 252–260. doi: 10.1016/j.mimet.2007.04.014
Nocker, A., Sossa-Fernandez, P., Burr, M. D., and Camper, A. K. (2007b). Use of propidium monoazide for live/dead distinction in microbial ecology. Appl. Environ. Microbiol. 73, 5111–5117. doi: 10.1128/AEM.02987-06
Ovejero, C. M., Delgado-Blas, J. F., Calero-Caceres, W., Muniesa, M., and Gonzalez-Zorn, B. (2017). Spread of mcr-1-carrying Enterobacteriaceae in sewage water from Spain. J. Antimicrob. Chemother. 72, 1050–1053. doi: 10.1093/jac/dkw533
Paterson, D. L., Hujer, K. M., Hujer, A. M., Yeiser, B., Bonomo, M. D., Rice, L. B., et al. (2003). Extended-spectrum β-lactamases in Klebsiella pneumoniae bloodstream isolates from seven countries: dominance and widespread prevalence of SHV- and CTX-M-type β-lactamases. Antimicrob. Agents Chemother. 47, 3554–3560. doi: 10.1128/aac.47.11.3554-3560.2003
Peak, N., Knapp, C. W., Yang, R. K., Hanfelt, M. M., Smith, M. S., Aga, D. S., et al. (2007). Abundance of six tetracycline resistance genes in wastewater lagoons at cattle feedlots with different antibiotic use strategies. Environ. Microbiol. 9, 143–151. doi: 10.1111/j.1462-2920.2006.01123.x
Poirel, L., Lartigue, M.-F., Decousser, J.-W., and Nordmann, P. (2005). ISEcp1B-mediated transposition of blaCTX-M in Escherichia coli. Antimicrob. Agents Chemother. 49, 447–450. doi: 10.1128/AAC.49.1.447-450.2005
Rizzo, L., Manaia, C., Merlin, C., Schwartz, T., Dagot, C., Ploy, M. C., et al. (2013). Urban wastewater treatment plants as hotspots for antibiotic resistant bacteria and genes spread into the environment: a review. Sci. Total Environ. 447, 345–360. doi: 10.1016/j.scitotenv.2013.01.032
Stalder, T., Barraud, O., Jové, T., Casellas, M., Gaschet, M., Dagot, C., et al. (2014). Quantitative and qualitative impact of hospital effluent on dissemination of the integron pool. ISME J. 8, 768–777. doi: 10.1038/ismej.2013.189
Ternes, T. A., Prasse, C., Eversloh, C. L., Knopp, G., Cornel, P., Schulte-Oehlmann, U., et al. (2017). Integrated evaluation concept to assess the efficiency of advanced wastewater treatment processes for the elimination of micropollutants and pathogens. Environ. Sci Technol. 51, 308–319. doi: 10.1021/acs.est.6b04855
The Federal Ministry of Health (2012). DART 2020 Fighting Antibiotic Resistance for the Good of Both Humans and Animals.
Vaz-Moreira, I., Nunes, O. C., and Manaia, C. M. (2014). Bacterial diversity and antibiotic resistance in water habitats: searching the links with the human microbiome. FEMS Microbiol. Rev. 38, 761–778. doi: 10.1111/1574-6976.12062
Villarreal, J. V., Jungfer, C., Obst, U., and Schwartz, T. (2013). DNase I and Proteinase K eliminate DNA from injured or dead bacteria but not from living bacteria in microbial reference systems and natural drinking water biofilms for subsequent molecular biology analyses. J. Microbiol. Methods 94, 161–169. doi: 10.1016/j.mimet.2013.06.009
WHO (2014). Antimicrobial Resistance: Global Report on Surveillance 2014. World Health Organization. Available online at: http://www.who.int/en/ (Accessed February 24, 2017).
Keywords: colistin resistance, antibiotic resistance genes, opportunistic bacteria, wastewater treatment, qPCR
Citation: Hembach N, Schmid F, Alexander J, Hiller C, Rogall ET and Schwartz T (2017) Occurrence of the mcr-1 Colistin Resistance Gene and other Clinically Relevant Antibiotic Resistance Genes in Microbial Populations at Different Municipal Wastewater Treatment Plants in Germany. Front. Microbiol. 8:1282. doi: 10.3389/fmicb.2017.01282
Received: 17 March 2017; Accepted: 26 June 2017;
Published: 11 July 2017.
Edited by:
David W. Graham, Newcastle University, United KingdomCopyright © 2017 Hembach, Schmid, Alexander, Hiller, Rogall and Schwartz. This is an open-access article distributed under the terms of the Creative Commons Attribution License (CC BY). The use, distribution or reproduction in other forums is permitted, provided the original author(s) or licensor are credited and that the original publication in this journal is cited, in accordance with accepted academic practice. No use, distribution or reproduction is permitted which does not comply with these terms.
*Correspondence: Thomas Schwartz, dGhvbWFzLnNjaHdhcnR6QGtpdC5lZHU=
†These authors have contributed equally to this work.