- 1UMR INTERTRYP, Institut de Recherche pour le Développement-CIRAD, CIRAD TA A-17/G, Montpellier, France
- 2Parasitology and Ecology Laboratory, Department of Animal Biology and Physiology, Faculty of Science, University of Yaounde 1, Yaounde, Cameroon
- 3UMR MIVEGEC, Institut de Recherche pour le Développement 224-Centre National de la Recherche Scientifique 5290, Montpellier, France
- 4UMR 7138, Systématique Adaptation Evolution, Université de Nice-Sophia Antipolis, Nice, France
- 5Institut National de la Recherche Agronomique, UMR 1309 ASTRE, Montpellier, France
- 6CIRAD, UMR ASTRE, Montpellier, France
Glossina sp. the tsetse fly that transmits trypanosomes causing the Human or the Animal African Trypanosomiasis (HAT or AAT) can harbor symbiotic bacteria that are known to play a crucial role in the fly's vector competence. We hypothesized that other bacteria could be present, and that some of them could also influence the fly's vector competence. In this context the objectives of our work were: (a) to characterize the bacteria that compose the G. palpalis palpalis midgut bacteriome, (b) to evidence possible bacterial community differences between trypanosome-infected and non-infected fly individuals from a given AAT and HAT focus or from different foci using barcoded Illumina sequencing of the hypervariable V3-V4 region of the 16S rRNA gene. Forty G. p. palpalis flies, either infected by Trypanosoma congolense or uninfected were sampled from three trypanosomiasis foci in Cameroon. A total of 143 OTUs were detected in the midgut samples. Most taxa were identified at the genus level, nearly 50% at the species level; they belonged to 83 genera principally within the phyla Actinobacteria, Bacteroidetes, Firmicutes, and Proteobacteria. Prominent representatives included Wigglesworthia (the fly's obligate symbiont), Serratia, and Enterobacter hormaechei. Wolbachia was identified for the first time in G. p. palpalis. The average number of bacterial species per tsetse sample was not significantly different regarding the fly infection status, and the hierarchical analysis based on the differences in bacterial community structure did not provide a clear clustering between infected and non-infected flies. Finally, the most important result was the evidence of the overall very large diversity of intestinal bacteria which, except for Wigglesworthia, were unevenly distributed over the sampled flies regardless of their geographic origin and their trypanosome infection status.
Introduction
Animal African Trypanosomiasis (AAT), or Nagana, is primarily caused by Trypanosoma brucei brucei (Tbb), Trypanosoma vivax, and Trypanosoma congolense, which are transmitted to their mammalian hosts by tsetse flies belonging to the species Glossina palpalis and Glossina morsitans. This disease is responsible for significant economic losses in areas where cattle are exposed to parasites (Shaw et al., 2013). Human African Trypanosomiasis (HAT), or sleeping sickness, is endemic to Sub-Saharan Africa, where it causes significant health and economic impacts (Welburn and Maudlin, 2012). The chronic form of HAT is caused by Trypanosoma brucei gambiense (Tbg), whereas Trypanosoma brucei rhodesiense (Tbr) causes the acute form; the two forms are transmitted to humans by G. palpalis and G. morsitans, respectively.
Currently, about 60 million people continue to be at risk for HAT in 36 Sub-Saharan African countries. Several factors complicate the treatment of HAT. The medications that are currently used to treat human patients can generate many side effects (reviewed in Geiger et al., 2011a). In addition, the emergence of drug-resistant trypanosomes was recently reported (Baker et al., 2013). These factors are pertinent, since epidemiological modeling of the impact of global change on HAT expansion predicts that 46–70 million more people will be at risk by 2090 (Moore et al., 2012). Thus, the search for new control strategies is a priority to impede the spread of the disease. In this context, identifying the factors involved in fly vector competence (as well as understanding how they work) may open new perspectives.
Trypanosomes ingested by the tsetse fly during a blood meal on an infected host passively reach the tsetse intestine, where they differentiate into their procyclic form, mature, and multiply. Then, depending on the species, the trypanosomes migrate toward either the fly's salivary glands (in the case of T. brucei sp.) or the mouth parts, where they undergo the maturation step into their infective metacyclic form. The trypanosomes may then be transmitted to a mammalian host during a subsequent blood meal ingested by the fly (Vickerman et al., 1988). This ability of tsetse flies to acquire trypanosomes and to subsequently favor their maturation and transmit them to a vertebrate host, known as the fly's vector competence, depends on several factors including the production of antimicrobial peptides (e.g., cecropin and attacin; Hu and Aksoy, 2006), reactive oxygen species (MacLeod et al., 2007), EP-protein [Glutamic acid (E)—Proline (P) rich protein, an immune responsive protein], (Haines et al., 2010), intestinal lectin (Welburn et al., 1994), as well as the peritrophic matrix (Weiss et al., 2013, 2014; Rose et al., 2014; Aksoy et al., 2016).
As previously reported, tsetse flies can harbor three different symbionts that play a crucial role in the physiology of their host. First, the obligate Enterobacteria Wigglesworthia glossinidia is present intracellularly in the bacteriocytes, specialized cells of the intestine that form the bacteriome (Aksoy, 1995). This ancient host association (Chen et al., 1999) is involved in the tsetse fly's fertility, nutrition, and the development of its immune system (Akman et al., 2002; Weiss et al., 2011; Rio et al., 2012). Second, the facultative symbiont Sodalis glossinidius is present in the cells of the intestine, but it can also be found extracellularly and in other tissues of the fly (Cheng and Aksoy, 1999; Balmand et al., 2013). There is substantial evidence that certain S. glossinidius genotypes favor the establishment of trypanosomes in the fly's gut (Geiger et al., 2007; Farikou et al., 2010a; Hamidou Soumana et al., 2014). W. glossinidia and S. glossinidius are both transmitted maternally to the progeny via milk secretions during the intra-uterine development of the larvae (Aksoy, 1995). Third, the facultative symbiont Wolbachia can be detected very early in oocytes, embryos and larvae (Cheng et al., 2000). This symbiont acts on the reproductive process of tsetse flies by inducing cytoplasmic incompatibility (Alam et al., 2011). Wolbachia infection has been negatively correlated with the prevalence of trypanosome and of salivary gland hypertrophy virus (Alam et al., 2012). This indicates that Wolbachia may have a role in preventing infection of tsetse flies from trypanosomes (Aksoy et al., 2013), which interestingly has not been revealed for plasmodium infection in mosquitoes that harbor Wolbachia (Zélé et al., 2014). This symbiont is trans-ovary transmitted to the progeny (Cheng and Aksoy, 1999).
The presence of bacteria (other than symbionts) in the gut of field-collected tsetse flies has been assessed by bacterial isolation and culture approaches (Geiger et al., 2009, 2011b; Lindh and Lehane, 2011), as well as by direct molecular identification methods. Using deep sequencing of the V4 hypervariable region of the 16S rRNA gene, Aksoy et al. (2014) revealed the limited diversity of gut-specific microbiota in wild tsetse flies from Uganda. Surprisingly, bacteria belonging to the genera Enterobacter, Acinetobacter, and Enterococcus were found in the gut of the blood-sucking fly, despite the fly feeds on animals which blood is normally devoid of any bacteria. The source of these bacteria is unknown, but their presence could be due to the fact that tsetse flies can occasionally feed on nutrients other than blood that may be contaminated by diverse bacteria (Colman et al., 2012). Flies may also ingest bacteria present on the skin surface of the hosts when taking their bloodmeal (Poinar et al., 1979). Finally, differences in their diet that may depend on the environmental conditions could lead to differences in the fly's gut bacteriome as it was shown for other insects (Colman et al., 2012). Finding such bacteria in an organ where trypanosomes are localized raised the question whether these bacteria could be involved, besides the known symbionts, in fly infection by trypanosomes, inasmuch as certain bacteria species have been shown to produce antiparasitic molecules (Lazaro et al., 2002; Moss, 2002). In this context, a novel bacterial species, Serratia glossinae, has been identified in the gut of insects reared in insectarium (Geiger et al., 2010). This bacterium is related to Serratia marcescens (Enterobacteriaceae) a bacterium involved in the production of pigments that are toxic for Trypanosoma cruzi (Azambuja et al., 2004). In Anopheles, the abundance of intestinal Enterobacteriaceae has been associated to the presence of the parasite Plasmodium falciparum (Boissière et al., 2012). This illustrates the need for a better understanding of the composition of the tsetse fly bacteriome and its potential variations, since differences in the environment could induce differences in the bacteriome composition which, in turn, could induce differences in the fly vector competence.
Using bar-coded Illumina sequencing of the hypervariable V3-V4 region of the 16S rRNA gene, we aimed to characterize bacterial communities in the midgut of G. p. palpalis sampled from three different sleeping sickness foci in Cameroon, and to verify the hypotheses that the composition of the midgut bacterial communities of trypanosome-infected G. p. palpalis might be different from that of non-infected flies across these three HAT foci. Finally we aimed to identify bacteria that could be associated with the infection status of the fly. Those, if any, associated with fly refractoriness would be of particular interest inasmuch if they would be maternally transmitted to offspring. In the case they would not be maternally transferred, their trypanocidal compound(s) should be identified and possibly delivered via the paratransgenic approach described by Rio et al. (2004). Both approaches may offer the possibility to block the fly vector competence, hence the spread of the sleeping disease.
Materials and Methods
Sampling Areas
The study was performed in three sleeping sickness foci (Bipindi, Campo, and Fontem) located in the forest region of southern-Cameroon (Figure 1).
Bipindi (3°2′N, 10°22′E) is a historical sleeping sickness focus originally described in 1920 (Grébaut et al., 2001). It is situated between Lolodorf and Kribi, 75 km from the Atlantic Ocean. Although, a 2004 survey identified four tsetse fly species (Grébaut et al., 2004), G. p. palpalis was the only species found in this focus since 2007 (Farikou et al., 2010a,b; Melachio et al., 2011; Tchouomene-Labou et al., 2014). Bipindi is surrounded by hills and covers several villages, mainly located along the roads. The focus has a typical forest bioecological environment, including equatorial forest and farmland along the roads and surrounding the villages. Bipindi is home to highly diverse wild fauna (Njiokou et al., 2004, 2006) and it has a dense network of rivers crossing cocoa farms, all of which offer suitable habitats for tsetse flies.
Campo (2°20′N, 9°52′E) is a hypo-endemic focus where several cases of sleeping sickness are diagnosed every year. It is located along the Ntem River, which separates Cameroon from Equatorial Guinea. Several tsetse fly species (including G. p. palpalis, and to a lesser extent G. pallicera, G. caliginea, and G. nigrofusca) can be encountered in this focus (Simo et al., 2008). Several biotopes are found in the Campo focus, including farmland, marshes, swampy areas, and rainy forest.
Fontem (5°40′N, 9°55′E), in the Southwest Region of Cameroon, has been recognized as a sleeping sickness focus since 1949. Since that time, the prevalence of HAT has decreased considerably. The landscape is characterized by the presence of hills and valleys that are crossed by rivers with fast currents. G. p. palpalis is the only tsetse fly species found in this area (Simo et al., 2008). The focus is divided into the North, Center and South sub-foci. The Center sub-focus (comprising the villages of Menji, Fotabong, Nsoko, and Azi), where flies were sampled, has only had a few sleeping sickness cases in the past decade. In the Northern sub-focus (comprising the villages of Bechati, Folepi, and Besali) no new HAT patients have been diagnosed in the last 20 years (Ebo'o Eyenga, personal communication), although 15% of all pigs were infected with T. b. gambiense group 1 in 1999 (Nkinin et al., 2002).
Fly Sampling and Experimental Design
Entomological surveys were conducted in the Campo focus in March 2008, and in the Bipindi and Fontem foci in November 2013. Pyramidal traps (Gouteux and Lancien, 1986) were placed for 4 consecutive days in suitable tsetse fly biotopes of each village located within each focus. The geographical position of the sampling sites was determined by GPS. Trapped tsetse flies were harvested twice a day (before 12 a.m. and before 3 p.m.).
The Glossina species were first identified according to morphological criteria (Grébaut et al., 2004), and then sorted into two groups of teneral (young flies that had never taken a blood meal) and non-teneral flies, respectively. Among the 599 non-teneral flies that were caught in the Campo focus, 200 were randomly sampled, their midguts dissected (see below) and observed under a light microscope (magnification x100) to detect the presence of motile trypanosomes (infected flies) or the absence of trypanosomes (non-infected flies). The trypanosomes from infected flies were subsequently identified (species or sub-species) by PCR using specific primers (Table 1). The absence of any trypanosomes (negative PCR assay) was confirmed in all non-infected flies using the same specific primers. Among the 300 flies caught in Bipindi, 200 were sampled and processed as for the Campo flies. Only 27 non-teneral flies could be caught in Fontem; as shown after dissection, microscopy, and PCR analyses, they all were non-infected by trypanosomes.
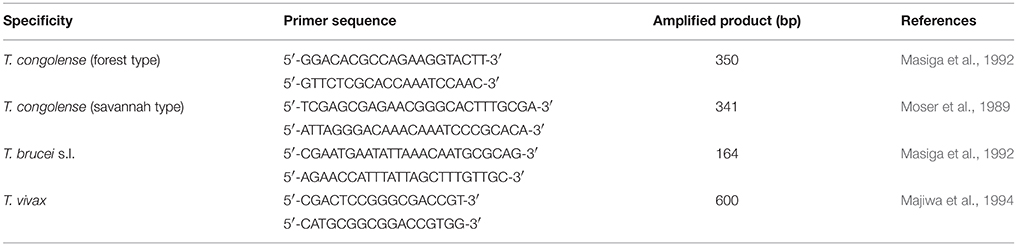
Table 1. Primers used for PCR amplification of trypanosome DNA (Farikou et al., 2010a).
The microbiota analysis was performed on G. p. palpalis flies that had undergone further selection. The midguts from the 200 sampled flies from Campo were separated into two groups composed, respectively, of trypanosome infected and non-infected midguts. Among the infected midguts, only those harboring T. congolense (either the forest or savannah type; mixed infections were discarded) were taken into account. Finally, from the non-infected group and from the T. congolense infected group, respectively, eight midguts were randomly selected and identified by numbering. The 200 samples from Bipindi were processed similarly. Among the midguts from the 27 flies from Fontem, eight were randomly selected, which formed a unique group of non-infected samples. In total, five groups were formed that differed from each other regarding the infection status of the flies and/or their geographic origin. The “sex ratio” (12.5, 37.5, 37.5, 75, and 25% male flies in, respectively group 1–5) was recorded but not taken into account in the subsequent analyses. The characteristics of each group, including sample numbering, are the followings: “Group 1/Campo infected flies: number 1–8; Group 2/Campo non-infected flies: number 9–16; Group 3/Bipindi infected flies: number 17–24; Group 4/Bipindi non-infected flies: number 25–32; Group5/Fontem non-infected flies: number 33–40 (Figure 2).
Dissection of Tsetse Flies, DNA Extraction, and Trypanosome PCR Amplification
The non-teneral flies were surface-sterilized (once with 5% sodium hypochlorite for 10 min and twice with 70% ethanol, each for 10 min) and the midgut of each fly was dissected under sterile conditions using a Bunsen burner, and in sterile NaCl 0.9%. The instruments used were carefully cleaned after the dissection of each fly to prevent cross-contamination. Midguts were recovered and then separately transferred into tubes containing 95° ethanol for further DNA extraction. During the field surveys (10 days for each mission), tubes containing the samples were maintained at −20°C; thereafter, in the laboratory, the midgut samples were immediately processed and subjected to DNA extraction using the DNeasy kit (Qiagen, Paris, France) according to Geiger et al. (2007). Genomic DNA was quantified using NanoDrop (Thermo Scientific, Paris).
PCR amplification was performed as previously described (Farikou et al., 2010a) using trypanosome-specific primers (Table 1; Moser et al., 1989; Masiga et al., 1992; Majiwa et al., 1994; Herder et al., 2002). This procedure allowed detection of T. brucei s.l., T. congolense (both forest and savannah types), and T. vivax.
Meta-Barcoding Analysis
Amplification of the highly variable V3-V4 region of the 16S rRNA gene, using the forward primer 5′-CCTACGGGNGGCWGCAG-3′ and the reverse primer 5′-GACTACHVGGGTHTCTAATCC-3′ (N representing A, C, G, or T; V representing A, C, or G; H representing A, C, or T; W representing A or T; IUPAC codes), was performed to evaluate the bacterial communities of tsetse midguts on the Illumina MiSeq (MR DNA Laboratory-http://www.mrdnalab.com/shallowater, USA). The primers were specifically designed by one of the co-authors of the current study. As previously described in the method of Porter et al. (2016), each PCR amplifying medium contained 2 μL DNA template, 17.5 μL molecular biology-grade water, 2.5 μL 10× reaction buffer, 1 μL 50× MgCl2 (50 mM), 0.5 μL dNTPs mix (10 mM), 0.5 μL forward primer (10 mM), 0.5 μL reverse primer (10 mM), and 0.5 μL Invitrogen Platinum Taq polymerase (5 U/μL) in a total volume of 25 μL. The amplification program was: 95°C for 5 min, followed by 25 cycles of 94°C for 40 s, 55°C for 1 min, and 72°C for 30 s, and lasted at 72°C for 5 min. Amplicons were purified using MiniElute PCR purification columns (Qiagen, Paris, France) and were eluted in 50 μL molecular biology-grade water. The purified amplicons from the first PCR round served as templates for the second PCR round, using Illumina adaptor-tailed primers in a 10-cycle amplification regime. All PCR reactions were performed in an Eppendorf Mastercycler ep gradient S thermal cycler. Negative controls (containing all reagents except the midgut DNA that was replaced by water) were included in all experiments. Amplicons were quantified using a Bioanalyzer (Agilent Technologies, Paris) and then the KAPA Quantification Kit (Roche, Paris). Equimolar amounts of the generated libraries were dual-indexed, combined, and sequenced on an Illumina MiSeq platform (MRDNA Laboratory, Shallowater, USA) using MiSeq Reagent kits (300 cycles), following the 2 × 300-bp paired-end sequencing protocol. The generated sequences have been deposited in the EMBL-EBI (Study accession number PRJEB14010; Secondary study accession number ERP015605 and Locus tag prefix BN6929).
Quality Filtering and Taxonomic Classification of Sequencing Data
Illumina MiSeq reads were analyzed using in-house pipelines (Richard Christen; described in Boissière et al., 2012; Hartmann et al., 2012; Gimonneau et al., 2014; Massana et al., 2015). Briefly, Silva 119 NR (analyses performed in 2014) was used as the reference database for taxonomic identification (Quast et al., 2012). An in silico extraction of Silva amplicons using forward: 5′-CCTACGGGNGGCWGCAG-3′ and reverse: 5′-GACTACHVGGGTHTCTAATCC-3′ primers, followed by an analysis by length/number of amplicons, yielded the following results: 1–50/0; 51–100/0; 101–150/2; 151–200/1; 201–250/1; 251–300/11; 301–350/40; 351–400/21,669; 401–450/448,575; 451–500/450; 501–550/192; 551–600/1,198; 601–650/0. This demonstrates that most amplicons were between 350 and 450 nucleotides in length. The extracted database is referenced below as the refseq. Each sequence identifier was reformatted to eight taxonomic fields as in PR2 (Guillou et al., 2012) making it easier to use a pipeline for analyses.
In order to assemble paired-end reads, the software programs PEAR (Zhang et al., 2014) and FLASH (Magoc and Salzberg, 2011) were both tested. PEAR using default parameters merged more pairs, and paired-end reads were therefore assembled and quality filtered (using Illumina quality scores) with PEAR. 94.26% (3,622,233 reads) of the total reads (3,842,672 reads) were assembled; 0.003% were discarded and 5.734% were not assembled.
Reads were then sorted by length using a dedicated python script, yielding the following results: 0–0/0; 1–50/3; 51–100/1,556; 101–150/1,435; 151–200/1,535; 201–250/1,578; 251–300/2,251; 301–350/9,401; 351–400/38,323; 401–450/132,487; 451–500/3,433,239; 501–550/381; 551–600/44; 601–650/0. Reads shorter than 300 nucleotides (0.23% out of the overall number of reads) were discarded, resulting in the extraction of 3,613,875 reads. The fasta file was then demultiplexed using a dedicated C++ program. Primer trimming was performed using CutAdapt v1.8.1 (Martin, 2011).
Each file was dereplicated, sorted by decreasing abundance, chimera checked with UCHIME (Edgar et al., 2011) and then clustered using Crunchclust (Mondani et al., 2011; Gimonneau et al., 2014; Massana et al., 2015; Tchioffo et al., 2016; available from https://github.com/dumaatravaie/crunchclust/blob/master/Documentation; at a Levenstein distance of 5). After this clustering step, clusters that contained <2 reads were discarded as artifacts (see Boissière et al., 2012). All these steps resulted in 2,562,144 high-quality sequences contained in clusters with at least two reads (70.73% of the total assembled reads) that were subsequently used for taxonomic assignment. In each cluster, the most abundant sequence was kept as the representative one, since it was assumed to have the least errors in a cluster. Taxonomic assignment was done as in Pawlowski et al. (2011). Briefly, a Needleman–Wunsch algorithm to search for the 30 most similar sequences to each representative sequence from the refseq was employed. The reference sequences with the highest percentage were then used, and taxonomy to a given level was obtained. When more than one result emerged, the two highest hits were reported. When similarity was <80%, sequences were not assigned. Abundance matrices were generated for statistical analyses at each taxonomic level. Several abundant Operational Taxonomic Units (OTUs) could not be identified satisfactorily down to the genus or species level. In these cases (rough estimation: 1%), reads sequences and similar refseq sequences were selected and then aligned using ClustalO (Sievers et al., 2011) and SeaView (Gouy et al., 2010). Trees were plotted using TreeDyn (Chevenet et al., 2006) or MetaPhlAn (Segata et al., 2012), and distinct robust subtrees were annotated as distinct species whenever possible.
Data and Statistical Analysis
Statistical analyses were performed using the R package vegan. Rarefaction curves (Figure S1) were performed prior to comparative analyses between infected and uninfected flies, and between the sampling sites. Significant differences in bacterial richness between the infected and uninfected flies, and between the three sampling sites were tested using non-parametric Kruskal–Wallis test. We used vegdist and hclust using single, average, and complete linkage methods for hierarchical clustering and then compared them for the presence of sub-trees. Nonmetric multidimensional scaling (nMDS) were generated using the R packages ggplot2 and phyloseq (McMurdie and Holmes, 2013).
Results
Distribution of Tsetse Flies by Infected vs. Non-infected Status
The distribution of trypanosome species and/or subspecies in the midgut of tsetse flies from Campo (200 flies) and Bipindi (200 flies) is displayed in Figure 3. Nearly half of all flies, whether from Campo (51.4%) or from Bipindi (48.6%), were infected with at least one of the four studied trypanosome species and/or subspecies. The prevalence of the four trypanosome species was highly dependent on the sampling site. In flies sampled from Campo, T. congolense (both forest and savannah types) displayed the highest prevalence, accounting for 83% of all infected flies (including mixed infections), while T. brucei sensu lato displayed the lowest prevalence (12%, including mixed infections). In contrast, T. brucei s.l. displayed the highest prevalence (43%) in infected flies from Bipindi, with T. congolense accounting for 42%. As for the Fontem focus, the low number of collected flies (27 flies) prevented any reliable statistical analysis regarding the prevalence of the infected flies in this focus, inasmuch as all of the 27 flies were uninfected (which does not imply that the overall population of flies is uninfected).
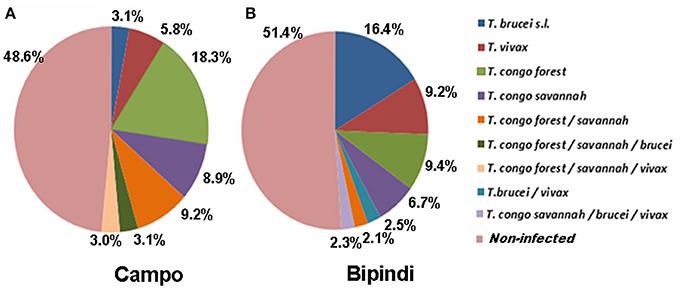
Figure 3. Prevalence of the different trypanosome species or subspecies infecting tsetse flies collected in the Campo (A) and Bipindi (B) foci. Single as well as mixed infections have been considered.
Bacterial Communities in the G. p. palpalis Midgut
Illumina sequencing of 16S rDNA gene generated a total of 2,562,144 high-quality sequence reads across the V3–V4 region in 40 tsetse flies, for the 40 samples randomly selected. The average number of tags/sample for the V3–V4 region was 12,988 (ranging from 6,525 to 19,694 per gut), with read length varying from 300 to 600 nucleotides. After taxonomic assignation, a total of 13 phyla were identified from the 40 gut samples (Table S1); 12 phyla belonged to the Bacterial kingdom and one phylum belonged to the Archaea. Proteobacteria were present in all 40 samples and had a relative abundance higher than 68% in each sample (even higher than 97% in 37 out of 40 samples), while the other phyla (as well as their respective relative abundances) were unevenly distributed among the different samples. The Bacteroidetes phylum was detected in 26 samples, Firmicutes was observed in 19 samples, Proteobacteria/Actinobacteria was detected in 14 samples, and Actinobacteria was found in 7 samples. The 7 other phyla could only be detected in 4 samples or less. Samples from groups 1 and 2 (infected and non-infected midguts from Campo; sample numbers 1–16) harbored 1 to 4 different phyla; samples from group 3 (infected midguts from Bipindi; sample numbers 17–24) contained 2–5 different phyla; samples from group 4 (non-infected midguts from Bipindi; sample numbers 25–32) contained 3–7 different phyla; and samples from group 5 (non-infected midguts from Fontem; sample numbers 33–40) harbored 1–4 different phyla (Table S1). A heat map analysis of the distribution and abundance of the bacterial phyla showed the sampled midgut did not cluster together, confirming that bacterial communities at the phylum level were unevenly distributed among the samples (Figure 4).
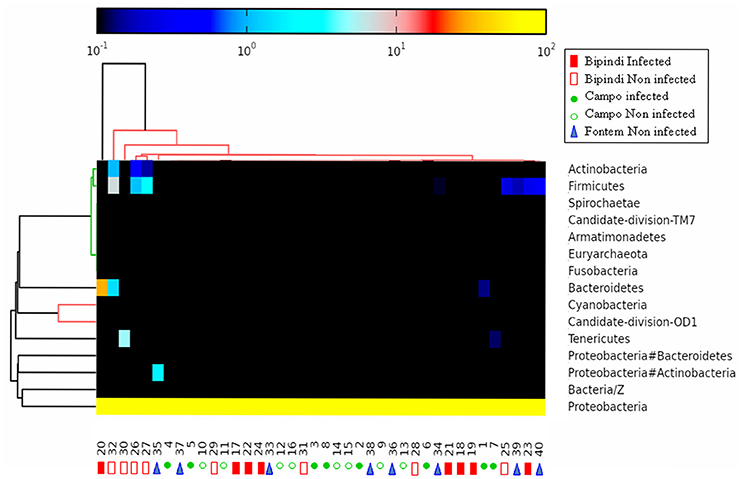
Figure 4. Heat map analysis of the distribution and abundance of the bacterial phyla in midgut samples. The samples did not cluster together, indicating highly variable bacterial communities. Numbers 1–8: infected samples from the Campo focus; Numbers 9–16: non-infected samples from the Campo focus; Numbers 17–24: infected samples from the Bipindi focus; Numbers 25–32: non-infected samples from the Bipindi focus; Numbers 33–40: non infected samples from the Fontem focus.
The distribution of bacterial taxa among the 40 samples at the class and genus levels and their relative abundance in each sample are reported in Table S1. Twenty-four classes and 83 genera could be distinguished. The gut microbiota presented a large inter-individual variability, although only a few taxa were prominent.
Bacteria Classes
As reported above, all 40 samples contained Proteobacteria, accounting for more than 97% of all microbiota in 37 samples. The Gammaproteobacteria, present in all 40 samples and accounting for more than 95% of the total microbiota in 38 samples, was the most prominent class in this phylum. Alpha-proteobacteria (maximal abundance per sample: 11.08%) were found in 32 samples. In contrast, Beta-proteobacteria were present in only 7 samples (maximal abundance per sample: 2.38%). Flavobacteria were present in 26 samples (maximum abundance per sample: 31.09%). Bacteroidia, Mollicutes, Actinobacteria/Gammaproteobacteria, and Bacilli were present in 1, 4, 14, and 19 samples, respectively [maximum abundance (%) of the total microbiota per sample: 0.6, 4.96, 1.88, and 4.11, respectively]. Finally, Actinobacteria were present in 7 samples (maximum abundance per sample: 0.19%). The heat map analysis in Figure 5 shows the distribution of the most abundant classes (those that were present in at least 7 samples) among the different samples. The other identified classes, such as Negativicutes, Rubrobacteria, Sphingobacteriia, and Clostridia, were present in <7 samples at a frequency of <0.25% per sample. In general, bacterial classes were very unevenly distributed among the samples. For example, whereas the abundance of Gammaproteobacteria was 100% in sample 15 and sample 32, sample 20 harbored representatives of Gammaproteobacteria, Flavobacteria, and Alphaproteobacteria (respective abundance: 57.74, 31.11, and 11.10%).
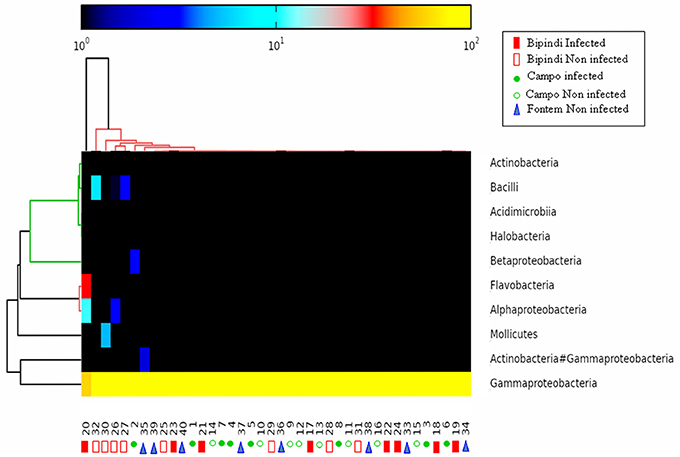
Figure 5. Heat map analysis of the distribution and abundance of the bacterial classes in midgut samples. The samples did not cluster together, indicating highly variable bacterial communities. Sample numbering as in Figure 4.
Bacterial Genera
Out of the 40 samples, 83 different bacteria genera (Table S1) were detected. The heat map analysis (Figure S2) performed on genera present in at least four samples, displays the distribution of genera, and their respective abundance, among the 40 samples. Midgut bacteria were unevenly distributed among individual tsetse flies and between the different sampled localities. Several genera were found in all (or most of) tsetse flies, possibly representing the midgut core microbiota. This was the case for the genus Wigglesworthia, as well as for several genera belonging to the Enterobacteriaceae family. The relative abundance of Wigglesworthia ranged from 34.5 to 99.8% across the 40 samples. Other genera widely distributed included two Enterobacter that were present, respectively, in 40 (relative abundance: 0.02–61.1%) and in 21 samples (relative abundance: 0.02–1.11%); Candidatus Sulcia muelleri, present in 26 samples (relative abundance: 0.01–31.09%); two Serratia present in 29 samples (relative abundance: 0.01–14%) and in 20 samples (relative abundance: 0.01–4.95%). Furthermore, Wolbachia was present in 25 samples (relative abundance: 0.01–11.08%), while Sodalis was only present in 4 samples, with a maximum relative abundance of 0.06%.
These results indicate that the midgut bacterial flora was mainly composed of gram-negative communities. Gram-positive bacteria belonged to the Bacilli, Mollicutes, Actinobacteria, Rubrobacteria, Clostridia, Fusobacteriia, Acidimicrobiia, and Thermoleophilia classes, and represented 10% of the total bacteria.
Finally, a total of 103 bacterial species (Table S1) and one Miscellaneous Euryarchaeotic Group (MEG, archeae) were detected. Only a few genera were shown to include more than one species, including Bacillus, Burkholderia, Enterobacter, Neisseria, Prevoltella, Providencia, and Serratia.
Of note, sequences assigned to W. glossinidia showed a relative abundance higher than 80% in all except two samples (samples 20 and 35, relative abundance 51.27 and 34.55%, respectively; Figure S3). A high number of sequences assigned to the Pseudomonas, Sphingomonas, and Hyphomicrobiaceae were detected in sample 26, and 74 species were found in sample 32.
Bacterial Communities between Infected and Uninfected Flies from the Different Geographic Located HAT
The average number of bacterial species per tsetse sample was not significantly different regarding their group (Kruskal–Wallis p-value = 0.342), their geographical origin (Kruskal–Wallis p-value = 0.167), or their status (infected vs. non-infected; Kruskal–Wallis p-value = 0.836).
We investigated potential relationships between the structure of the gut microbial communities of tsetse flies from Campo, Bipindi, and Fontem foci and the fly's trypanosome infection status. A hierarchical clustering using the Bray–Curtis indice did not discriminate unambiguously the different groups (Figure 6). It however showed a trend of infected flies from a given focus to be separated from non-infected flies of the same focus. One can also notice that infected flies from Bipindi are closely related to non-infected flies from Campo and vice versa. In contrast, the non-infected samples from Fontem were distributed over the different clusters. These results were reinforced by the nonmetric multidimensional scaling plots shown in Figures S4A,B.
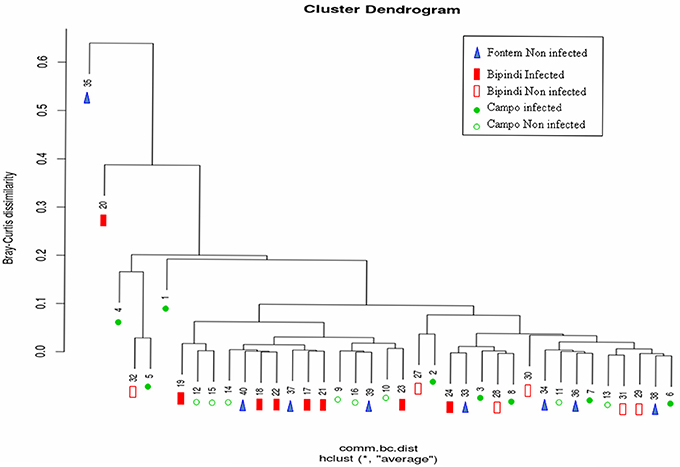
Figure 6. Hierarchical cluster dendrogram based on Bray-Curtis Index values, showing the relationship between different samples (represented by numbers) and foci. Sample numbering as in Figure 4.
Novel Bacteria Taxa
A phylogenetic tree was inferred to further investigate the potential dissimilarities between the bacteria sequences identified in this study and the sequences currently available in public databases, thereby illustrating the taxonomic relationships between the representative OTU sequences (Figures S5A–D). The most abundant OTU present in each of the 40 samples showed sequence similarities with Wigglesworthia sp. We identified 60 different genotypes of Wigglesworthia, similar to those from G. morsitans or G. brevipalpis. Depending on the sample, there were one to four genotypes per sample. However, most samples harbored one genotype, except those from Fontem that harbored two to four different genotypes in the same sample.
Some OTU-representative sequences did not match known targets in the NCBI database (analyses performed in 2014) and had to be annotated manually. Sample 20 (Figure 7) was shown to harbor four different genotypes of several bacteria species, including Candidatus Nasuia sp., Wigglesworthia sp., and Wolbachia sp. Similarly, four different genotypes of Enterobacteriaceae sp., Wigglesworthia sp., Wolbachia sp., and Spiroplasma sp. were detected in sample 30 (Figure 8).
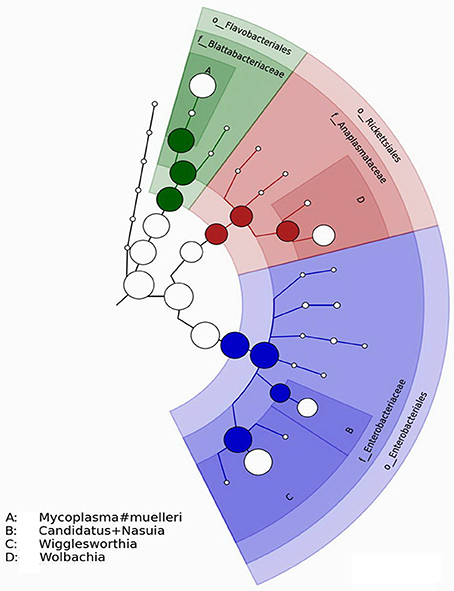
Figure 7. Phylogenetic diversity of bacterial 16S rRNA sequences found in sample 20 (infected tsetse fly midguts from Bipindi).
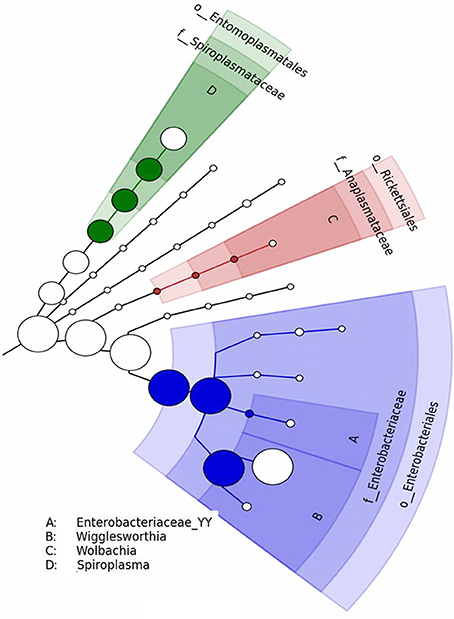
Figure 8. Phylogenetic diversity of bacterial 16S rRNA sequences found in sample 30 (uninfected tsetse fly midguts from Bipindi).
Presence of Symbionts
Special attention was paid to symbionts, due to their role in tsetse physiology and their interaction with trypanosomes. Wigglesworthia glossinidia was once again characterized by its high density in most samples and groups; this was assessed by the high percentages (more than 80% in each sample), except in two samples from groups 4 and 5 (Bipindi and Fontem, non-infected flies). Wolbachia sp. sequences were also present in all groups, but in smaller amounts. Nevertheless, a very high number of Wolbachia sequences were observed in sample 20 (11.08%). In addition, we detected, at low relative abundance, the presence of the symbiont S. glossinidius in four samples distributed over all groups, except Bipindi-uninfected (group 4).
Discussion
The main objective of this study was to characterize the bacterial communities inhabiting the midgut of field-collected tsetse flies from sleeping sickness foci in Cameroon using deep sequencing. Trypanosome-infected and non-infected flies were sampled in three sleeping sickness foci in Cameroon (Bipindi, Campo, and Fontem) that differ in their natural environment, thus offering the possibility to investigate the potential association between specific bacterial communities composition and the environment and/or the ability of the flies to be infected by trypanosomes. In this study the targeted trypanosome species was T. congolense (causing AAT) since, as shown in Figure 3, its prevalence was large enough in both Campo and Bipindi sampled flies. In contrast, T. brucei (sensu lato, Tb sl hereafter) had a much lower prevalence. Indeed, only 6 Tb sl infected flies were recorded in the sampled sites which, furthermore, included the two sub-species, T. brucei brucei and T. brucei gambiense—the former causing AAT and the second being responsible for the chronic form of HAT.
The Campo sampling campaign was performed in 2008, while the ones regarding Bipindi and Fontem were performed in 2013. A reduced but significant gene flow, due to fly migration, was previously reported between Campo and Bipindi (Farikou et al., 2011b), raising the question whether the 5 years delay between the sampling periods could affect the fly infection status and the microbiota profiles. One may consider that in the case of an important and/or continuous migration flow for years, differences in fly population diversity between the two foci would have been reduced or even suppressed. This was clearly not the case, and was never recorded (Farikou et al., 2010a,b). Although, the possibility that the 5 years delay could marginally affect the comparison between the 3 foci cannot be totally excluded, it could not affect the intra-foci comparison between trypanosome infected and non-infected flies.
Diversity of the Bacterial Communities and Its Origin
We detected a very large, unexpected number of different bacterial taxa (103 species), among which 26 were found in more than one sample. The number of different species identified in the present study was clearly much higher than in previous reports. For instance, three species were identified in G. p. palpalis in Angola (Geiger et al., 2009); one, three and eight species were, respectively, found in G. nigrofusca, G. pallicera, and G. p. palpalis in Cameroon (Geiger et al., 2011b); one species was identified in G. p. gambiensis; and 24 species were found in G. f. fuscipes in Kenya (Lindh and Lehane, 2011). With the exception of two bacterial species, all species detected in these previous studies were identified using culture-dependent methods. Many bacteria species are difficult to culture and the density of some species may be too low for proper isolation. This could explain the low number of bacteria identified in other studies as compared to the high number of species we characterized in the gut of tsetse flies using molecular approach.
A more recent study (Aksoy et al., 2014) identified a high number of bacteria species in the gut microbiota of several tsetse fly species collected in Uganda using molecular approaches, although the bacteriome of Uganda flies was less diverse than the one of flies from Cameroon.
Compared to the bacterial communities of flies from Uganda, flies from Cameroon revealed both similarities (namely Enterobacteriaceae and an unexpected high abundance of Wigglesworthia) and differences (bacteria belonging to the genus Delftia, Stenotrophomonas, Thermoanaerobacterium, Proteus, and Ochrobactrum, among others, were not found in flies from Cameroon). These differences may be due to differences in the respective investigated fly species, G. f. fuscipes, G. m. morsitans, G pallidipes vs. G. p. palpalis, and/or the respective environmental factors and the geographic locations of the HAT/AAT foci in Uganda vs. Cameroon.
Aside from the question of what effect these gut bacteria may have on tsetse fly physiology and/or on their ability to become trypanosomes infected (i.e., their vector competence), there is also the question of how tsetse flies can acquire such a large diversity of bacterial species. In their report, Aksoy et al. (2014) mentioned the possibility that some of the low abundant bacteria “may have arisen from contamination from environmental sources, such as during field-based dissection processes,….” Although, the occurrence of contamination can never be totally excluded, our data do not support this comment since it would be expected, in such case, that several bacteria species from the environment would be detected, at low abundance, in all or most of the samples, inasmuch the 40 fly were processed at the same time. As shown in Table S1, this is not the case; most of the low abundant bacteria (74 out of 103) are present in only one sample and, in contrast, one of the samples (number 32) was shown to harbor 59 species. Nevertheless, further independent studies should be undertaken to confirm the finding. An alternative explanation could account for the complexity of the microbiota as it was recently reported, that is, tsetse flies are not strictly hematophagous; they can occasionally feed on nectar from a broad range of plants growing within their different ecological environments, and, thus, may ingest bacteria colonizing this nutriment (Colman et al., 2012; Solano et al., 2015). Such effect of diet differences and their links to environmental conditions, on bacteriome community structure of insects has been documented by Colman et al. (2012). Finally, as reported by Poinar et al. (1979), adult flies may be contaminated with bacteria present on the epidermis of humans or animals they bite during blood meal. This could lead to a large diversity of the fly bacteriome since tsetse flies are known to ingest their blood meals from a variety of vertebrates (Moloo et al., 1988; Simo et al., 2008; Farikou et al., 2010b). Thus, despite the viviparous reproduction of tsetse flies (in contrast to other dipterans) that prevent fly contamination at the larval stage (except for the symbionts that are vertically transmitted from the mother to the larva via milk gland secretions), the modes of acquiring “exogenous” bacteria by tsetse flies seems to be more diverse than previously believed and could explain the recorded large microbiota diversity herein.
One however has to keep in mind that in addition to true tsetse gut inhabitants, it is likely that some—or many—of the 143 OTUs are solely opportunistic, simply in transit or possibly present only as DNA fragments. Since dead bacteria provide the same DNA signature as do living bacteria, further investigations should aim to isolate bacteria of interest; only such approach will provide best evidence of their presence as a living organism in the tsetse gut.
Bacterial Species Richness of the Samples (Alpha Diversity)
Although, the analyzed midgut bacteriome of adult tsetse flies included 103 species belonging to 13 phyla, only 4 of them were dominant (Proteobacteria, Bacteroidetes, Actinobacteria, and Firmicutes), showing some similarities with gut microbial communities from other invertebrates such as cattle tick (Andreotti et al., 2011), several species of Anopheles (Rani et al., 2009; Boissière et al., 2012) and of Aedes (Zouache et al., 2011; Terenius et al., 2012); Acridid (Dillon et al., 2008); Asian longhorned beetle (Geib et al., 2009); Lutzomyia longipalpis (Gouveia et al., 2008); honey and bumble bees (Martinson et al., 2011). However, there is a major difference between the bacteriome of all these invertebrates and those of tsetse flies, namely the presence, in the latter, of W. glossinidia in all samples. W. glossinidia's presence is not surprising as it is the tsetse fly's obligate symbiont; its abundance in the different samples was however remarkable as the mean relative abundance over the 40 samples was as high as 95.3% (even higher than 99% in all but five samples—see below), while that of Enterobacter homaechei, also present in all samples, was only 1.8%. Such an “overabundance” of Wigglesworthia was previously reported by Aksoy et al. (2014) who estimate that it may disrupt proper identification of low abundant bacteria thus leading to an underestimation of the alpha diversity (species richness). In order to decrease the abundance of this bacterium—and thus overcoming the difficulty related to its overabundance—the group of Aksoy et al. (2014) excised part of the bacteriome organ harboring Wigglesworthia and was able to discover some bacteria previously non-identified when analyzing the whole bacteriome. In our study, five samples showed smaller Wigglesworthia relative abundance than the 35 others; these are [sample number and (relative Wigglesworthia abundance)] sample 2 (88.83%), 4 (80.52%), 20 (51.27%), 32 (86.96%), and 35 (34.55%) while the numbers of hosted bacteria were, respectively, 16, 9, 8, 73, 10 (Wigglesworthia excluded). Thus, concerning our study, it seems that there was no relationship between the Wigglesworthia relative abundance and the species richness of the samples [for example, sample 32 hosted the largest number of bacteria although the relative abundance of Wigglesworthia was rather high (86.96%)]. Thus, as suggested by Aksoy et al. (2014), further investigations on the tsetse fly bacteriome should be performed using genomic/complementary functional approaches.
Microbiome Composition and the Possible Role of Identified Bacteria
Most of the sequence tags corresponded to few taxa. Only 16 species displayed a maximum relative abundance per sample higher than 1%, whereas 78 species had a maximum relative abundance lower than 0.2%. Nevertheless, some members of the latter group of bacteria are potentially of great interest.
The Symbionts
This group of bacteria includes in particular three species W. glossinidia, S. glossinidius, and Wolbacchia. Besides its prominent role as an obligate symbiont of the tsetse fly, Wigglesworthia differs from the other bacteria by the fact that 60 genotypes of this species have been identified, whereas most other bacteria were represented by only one genotype, or at least by a limited number of genotypes. In samples from Uganda, differences were also detected in the sequences of the Wigglesworthia V4 region (Aksoy et al., 2014) that confirmed the existence of several genotypes, although the authors reported small amounts of Wigglesworthia carryover between the different tsetse species sampled; thus they do not exclude completely the occurrence of a cross contamination. Nevertheless, the occurrence of multiple genotypes of a given species is not a surprise and may not be considered as an artifact generated along the technical process. For example, we have previously identified number of S. glossinidius genotypes when using other approaches than amplicon sequencing (Illumina MiSeq) such as AFLP (Geiger et al., 2005, 2007) or microsatellite (Farikou et al., 2011a,b) genotyping on either insectary-reared or field sampled tsetse flies. This finding could be of great interest, as until now only the density of Wigglesworthia, not its potential genetic diversity, was considered to be involved in the modulation of tsetse fly infection by trypanosomes (Wang et al., 2009). In fact, the genetic diversity within populations of W. glossinidia that infect tsetse flies could play a prominent role, as previously demonstrated for the facultative symbiont S. glossinidius (Geiger et al., 2007). However, investigating a possible association between trypanosome infection and specific W. glossinidia genotypes (60 have been identified) will require a much larger sampling size.
Attention was paid to Sodalis that was previously shown to be involved in modulating the tsetse fly's ability to acquire trypanosomes (Farikou et al., 2010a; Hamidou Soumana et al., 2014). In the present study this symbiont was identified in 10% of the samples, a finding markedly different from the 30% average prevalence we found previously in nearly the same sampling sites (Farikou et al., 2010a), and from that recorded for example by Aksoy et al. (2014) who suggest the variations could be a matter of sensitivity of the analytical approach. However, this could be only part of an explanation since we used in our study similar analytical approaches and instruments (including the Illumina MiSeq platform). Overall, these data confirm the existence of a very high symbiont variability in natural populations of flies, as previously reported by Farikou et al. (2010a). These variations could be linked to a multitude of factors including differences in tsetse individual flying times, field environment modifications or, as suggested by Aksoy et al. (2014) the competition between Sodalis and other intestinal bacteria which diversity and respective abundance may vary.
Wolbachia sp. was previously characterized in field populations of G. morsitans morsitans, G. morsitans centralis, G. austeni flies, G. brevipalpis, G. pallidipes, and G. p. gambiensis (Doudoumis et al., 2012). Interestingly, Wolbachia has not been characterized in G. fuscipes fuscipes or G. tachinoides and, until now in G. p. palpalis. The bacterium was detected in 25 out of 40 flies (62.5%) and was distributed over the 5 groups (thus across the three sampling sites, and the fly trypanosome infection status): 5, 4, 7, 5, and 4 Wolbachia positive flies in groups 1–5, respectively. At this stage of the investigation we cannot suggest any explanation for its presence; we can only report its relatively high prevalence. The fact that until now Wolbacchia was never characterized in G. palpalis palpalis does not mean such infection does not occur in other fly individuals; further studies should focus on surveys of larger G. p. palpalis populations from the studied HAT foci, or on populations from HAT foci located elsewhere than in Cameroon (for example flies from HAT foci located in RDC—République Démocratique du Congo—or in Angola). In a recent report, Ji et al. (2015) highlight the extreme variability (from 1.54 to 66.67%) of the prevalence of Wolbacchia across 25 Bemisia tabaci populations from China. The frequency of Bemisia infection was shown to be affected by a large panel of factors (putative B. tabaci species, geographic location, sex of the host …). Finally, a cystoplasmic incompatibility occurs when a Wolbacchia negative (W−) female fly mates with a Wolbacchia positive (W+) male, and the embryos degenerate (Alam et al., 2011). In contrast, when a (W+) female mates with a (W+) or a (W−) male, the offspring is not only fertile but also more numerous than in the case of a (W−) female with (W−) male mating. As Wolbacchia is transmitted by the female fly to its offspring, the presence of Wolbacchia in a female gives them a reproductive advantage. Could this explain the high prevalence of Wolbacchia in the studied Gpp populations?
In contrast to Anopheles gambiae whose susceptibility to Plasmodium infection increased with the presence of Wolbachia under natural conditions (Zélé et al., 2014); the presence of this symbiont was not found to be associated with the infection status of the tsetse flies.
We also identified in tsetse fly midguts the presence of bacteria that are known to be symbionts when infecting other organisms. This is the case of Candidatus Sulcia muelleri (Flavobacteriaceae), which displayed an ancient symbiotic relationship with the hemipteran Auchenorrhyncha (Moran et al., 2005). The identified bacterium Candidatus Zinderia is also a symbiont of the tick Haemaphysalis longicornis (McCutcheon and Moran, 2010). Finally, we observed the cohabitation of four symbionts in some of our analyzed samples, although it is unknown if they develop a synergistic effect on the fly's physiology. Given the current state of our investigation, large-scale analyses (including attempts to isolate the two Candidatus species) deserve to be undertaken, as until now only three symbionts have been described in tsetse flies.
Other Bacteria of Interest
S. marcescens and Serratia odorifera (Enterobacteriaceae) were present in more than 50% of the samples. Both species are considered to be opportunistic pathogens for humans (Chmel, 1988; Hejazi and Falkiner, 1997; Grimont and Grimont, 2015). Serratia marcescens is also responsible for the increased mortality in tsetse flies (Poinar et al., 1979) such as Glossina pallidipes (Gonzalez-Ceron et al., 2003); it secretes trypanolytic compounds and reduces the establishment of T. cruzi in the midgut of its vector, Rhodnius prolixus (Triatominae), vector of T. cruzi causing the Chagas disease; Azambuja et al., 2004). One previous work reported the presence of the novel species S. glossinae in the gut of insectary-reared G. palpalis gambiensis flies (Geiger et al., 2010), although this species has never been identified in field-collected Glossina flies. Surprisingly, the same situation was observed for mosquitoes where the bacterium Elizabethkingia was found only in insectary-reared Anopheles sp. (Boissière et al., 2012; Gimonneau et al., 2014). However, this phenomenon cannot be currently explained. The presence of Serratia in the guts of G. f. fuscipes flies from western Kenya has also been reported (Lindh and Lehane, 2011). In Anopheles gambiae mosquito midguts, multiple strains of Serratia have been identified, some of which are able to reduce mosquito infections by Plasmodium (Bando et al., 2013). Serratia has also been identified as a newly acquired symbiotic partner in aphids (Burke and Moran, 2011). Currently, we did not evidence a clear association between the presence of some bacterial and trypanosome infection. However, as suggested previously by Aksoy et al. (2014), understanding the association of Serratia with tsetse flies and its functional role in tsetse physiology could provide critical knowledge about trypanosome transmission dynamics in tsetse flies. Similar investigations could be performed with other bacteria.
In addition to Serratia, other identified bacteria have the ability to produce antiparasitic compounds, including members of Acinetobacter. This genus was previously characterized in the gut of tsetse flies from Cameroon (Geiger et al., 2009). The presence of Acinetobacter (guillouiae and spp.) was observed in 6 samples in the present study.
Association between the Composition of the Intestinal Communities and the Fly Infection Status and/or the Environmental Differences
The characterization of the composition of the intestinal bacterial communities was an important objective of the work. However, the statistical analyses of the identified bacteria in this study did not reveal any significant differences in the sample bacterial species richness between the different groups (Campo and Bipindi infected and uninfected flies as well as Fontem uninfected flies), or the status of the flies (infected vs. uninfected). A hierarchical clustering neither discriminate properly the different groups, nor the infected flies from the non-infected flies of the same focus. At the level of individual bacteria, some of them seem to display an association with a given group or a sampling site. However, most often they concern species that are poorly represented. The possibly that environmental differences between the three sampling sites (especially the climate, wildlife, and flora) were too weak to induce significant differences in the flies' microbiome composition may be considered. These data suggest future fly microbiome research should aim for greater numbers of flies and target more ecologically contrasted sites, in order to identify bacteria involved in (or at least associated with) the fly vector competence.
In conclusion the meta-barcoding analysis that we performed on DNA extracted from G. p. palpalis collected from three different HAT foci in Cameroon resulted in the detection of 143 OTUs belonging to 83 genera and 13 phyla. Part of these bacteria could be identified at the species level (103 annotated OTUs). However, some species were not identifiable since the corresponding sequences did not match any available sequence libraries; they may correspond to novel species, and need to undergo further identification process. The presence of such a variety of bacteria was unexpected and raises many questions regarding how they are acquired by tsetse flies, and the role they may play regarding the fly's physiology and vector competence. In addition, it would be of interest, in a following investigation campaign, to enlarge the sampling scheme and include teneral flies as a supplementary control besides non-infected non-teneral flies. The former are expected to host a more limited microbiota and to have a less developed immunity compared to the latter. On the other hand, it should be noted that, when teneral flies undergo artificial infection process, about 70% do not develop trypanosome infection (Geiger et al., 2007). This suggests that within a collection of teneral flies most of them, even considered as “naïve,” are physiologically and genetically “programmed” to be/become refractory to trypanosome infection.
Author Contributions
Conceived and designed the experiments: FJ, AG. Performed the experiments: FJ, TM, GN, FN, AG. Analyzed the data: FJ, RC, AG. Contributed reagents/materials/analysis tools: AG. Wrote the paper: GG, FN, LA, JR, AG.
Conflict of Interest Statement
The authors declare that the research was conducted in the absence of any commercial or financial relationships that could be construed as a potential conflict of interest.
Acknowledgments
The authors thank the “Région Languedoc-Roussillon—Appel d'Offre Chercheur d'Avenir 2011,” the “Institut de Recherche pour le Développement” and the International Atomic Energy Agency (IAEA) for their support.
Supplementary Material
The Supplementary Material for this article can be found online at: http://journal.frontiersin.org/article/10.3389/fmicb.2017.01464/full#supplementary-material
Table S1. Classification of the total bacteria detected in the 40 tsetse samples from the Campo, Bipindi, and Fontem foci in Cameroon. The table provides, for each sample, the relative abundance (in %) of each bacterium present in this sample. Several of the detected bacteria could not be identified at the species level. The suffix “X or Y” refers to “unclassified” at that rank.
Figure S1. Rarefaction analysis on the studied samples (Renyi diversity plots); (A) Samples 1–24 and 27–40; (B) Samples 25 and 26 that were processed apart.
Figure S2. Heat map analysis of the distribution and abundance of the bacterial genera in midgut samples. The samples did not cluster together, indicating that the bacterial community among them is highly variable. Sample numbering as in Figure 4.
Figure S3. Heat map analysis of the repartition and abundance of bacterial species in midgut samples. The samples did not cluster together, indicating that the bacterial community among them is highly variable. Sample numbering as in Figure 4.
Figure S4. (A) NMDS plot comparing bacterial OTUs in tsetse fly midguts of different foci (Bipindi, Campo, Fontem) using Bray-Curtis dissimilarity indice. (B) NMDS plot comparing bacterial OTUs of infected vs. non infected tsetse fly midguts using Bray-Curtis dissimilarity indice.
Figure S5. Phylogenetic diversity of bacterial 16S rRNA sequences in tsetse fly midguts. NJ tree clustering of OTU representatives based on homology with known sequences is shown. The width of the branch is proportional to the number of samples exhibiting a given OTU. Colors correspond to different phyla. (A–D) Expended scale of four regions from this figure in order to make it easier to read (the corresponding regions are indicated in this figure).
References
Akman, L., Yamashita, A., Watanabe, H., Oshima, K., Shiba, T., Hattori, M., et al. (2002). Genome sequence of the endocellular obligate symbiont of tsetse flies, Wigglesworthia glossinidia. Nat. Genet. 32, 402–407. doi: 10.1038/ng986
Aksoy, E., Telleria, E. L., Echodu, R., Wu, Y., Okedi, L. M., Weiss, B. L., et al. (2014). Analysis of multiple tsetse fly populations in Uganda reveals limited diversity and species-specific gut microbiota. Appl. Environ. Microbiol. 80, 4301–4312. doi: 10.1128/AEM.00079-14
Aksoy, E., Vigneron, A., Bing, X., Zhao, X., O'Neill, M., Wu, Y. N., et al. (2016). Mammalian African trypanosome VSG coat enhances tsetse's vector competence. Proc. Natl. Acad. Sci. U.S.A. 113, 6961–6966. doi: 10.1073/pnas.1600304113
Aksoy, S. (1995). Wigglesworthia gen. nov. and Wigglesworthia glossinidia sp. nov., taxa consisting of the mycetocyte-associated, primary endosymbionts of tsetse flies. Int. J. Syst. Bacteriol. 45, 848–851. doi: 10.1099/00207713-45-4-848
Aksoy, S., Caccone, A., Galvani, A. P., and Okedi, L. M. (2013). Glossina fuscipes populations provide insights for human African trypanosomiasis transmission in Uganda. Trends Parasitol. 29, 394–406. doi: 10.1016/j.pt.2013.06.005
Alam, U., Hyseni, C., Symula, R. E., Brelsfoard, C., Wu, Y., Kruglov, O., et al. (2012). Implications of microfauna-host interactions for trypanosome transmission dynamics in Glossina fuscipes fuscipes in Uganda. Appl. Environ. Microbiol. 78, 4627–4637. doi: 10.1128/AEM.00806-12
Alam, U., Medlock, J., Brelsfoard, C., Pais, R., Lohs, C., Balmand, S., et al. (2011). Wolbachia symbiont infections induce strong cytoplasmic incompatibility in the tsetse fly Glossina morsitans. PLoS Pathog. 7:e1002415. doi: 10.1371/journal.ppat.1002415
Andreotti, R., Pérez de León, A. A., Dowd, S. E., Guerrero, F. D., Bendele, K. G., and Scoles, G. A. (2011). Assessment of bacterial diversity in the cattle tick Rhipicephalus (Boophilus) microplus through tag-encoded pyrosequencing. BMC Microbiol. 11:6. doi: 10.1186/1471-2180-11-6
Azambuja, P., Feder, D., and Garcia, E. S. (2004). Isolation of Serratia marcescens in the midgut of Rhodnius prolixus: impact on the establishment of the parasite Trypanosoma cruzi in the vector. Exp. Parasitol. 107, 89–96. doi: 10.1016/j.exppara.2004.04.007
Baker, N., de Koning, H. P., Mäser, P., and Horn, D. (2013). Drug resistance in African trypanosomiasis: the melarsoprol and pentamidine story. Trends Parasitol. 29, 110–118. doi: 10.1016/j.pt.2012.12.005
Balmand, S., Lohs, C., Aksoy, S., and Heddi, A. (2013). Tissue distribution and transmission routes for the tsetse fly endosymbionts. J. Invertebr. Pathol. 112, S116–S122. doi: 10.1016/j.jip.2012.04.002
Bando, H., Okado, K., Guelbeogo, W. M., Badolo, A., Aonuma, H., Nelson, B., et al. (2013). Intra-specific diversity of Serratia marcescens in Anopheles mosquito midgut defines Plasmodium transmission capacity. Sci. Rep. 3:1641. doi: 10.1038/srep01641
Boissière, A., Tchioffo, M. T., Bachar, D., Abate, L., Marie, A., Nsango, S. E., et al. (2012). Midgut microbiota of the malaria mosquito vector Anopheles gambiae and interactions with Plasmodium falciparum infection. PLoS Pathog. 8:e1002742. doi: 10.1371/journal.ppat.1002742
Burke, G. R., and Moran, N. A. (2011). Massive genomic decay in Serratia symbiotica, a recently evolved symbiont of aphids. Genome Biol. Evol. 3, 195–208. doi: 10.1093/gbe/evr002
Chen, X., Song, L., and Aksoy, S. (1999). Concordant evolution of a symbiont with its host insect species: molecular phylogeny of genus Glossina and its bacteriome associated endosymbiont, Wigglesworthia glossinidia. J. Mol. Evol. 48, 49–58. doi: 10.1007/PL00006444
Cheng, Q., and Aksoy, S. (1999). Tissue tropism, transmission and expression of foreign genes in vivo in midgut symbionts of tsetse flies. Insect Mol. Biol. 8, 125–132. doi: 10.1046/j.1365-2583.1999.810125.x
Cheng, Q., Ruel, T. D., Zhou, W., Moloo, S. K., Majiwa, P., O'Neill, S. L., et al. (2000). Tissue distribution and prevalence of Wolbachia infections in tsetse flies, Glossina spp. Med. Vet. Entomol. 14, 44–50. doi: 10.1046/j.1365-2915.2000.00202.x
Chevenet, F., Brun, C., Ba-uls, A. L., Jacq, B., and Christen, R. (2006). TreeDyn: towards dynamic graphics and annotations for analyses of trees. BMC Bioinformatics 7:439. doi: 10.1186/1471-2105-7-439
Chmel, H. (1988). Serratia odorifera biogroup 1 causing an invasive human infection. J. Clin. Microbiol. 26, 1244–1245.
Colman, D. R., Toolson, E. C., and Takacs-Vesbach, C. D. (2012). Do diet and taxonomy influence insect gut bacterial communities? Mol. Ecol. 21, 5124–5137. doi: 10.1111/j.1365-294X.2012.05752.x
Dillon, R. J., Webster, G., Weightman, A. J., Dillon, V. M., Blanford, S., and Charnley, A. K. (2008). Composition of Acridid gut bacterial communities as revealed by 16S rRNA gene analysis. J. Invertebr. Pathol. 97, 265–272. doi: 10.1016/j.jip.2007.09.010
Doudoumis, V., Tsiamis, G., Wamwiri, F., Brelsfoard, C., Alam, U., Aksoy, E., et al. (2012). Detection and characterization of Wolbachia infections in laboratory and natural populations of different species of tsetse flies (genus Glossina). BMC Microbiol. 12(Suppl. 1):S3. doi: 10.1186/1471-2180-12-S1-S3
Edgar, R. C., Haas, B. J., Clemente, J. C., Quince, C., and Knight, R. (2011). UCHIME improves sensitivity and speed of chimera detection. Bioinformatics 27, 2194–2200. doi: 10.1093/bioinformatics/btr381
Farikou, O., Njiokou, F., Cuny, G., and Geiger, A. (2011a). Microsatellite genotyping reveals diversity within populations of Sodalis glossinidius, the secondary symbiont of tsetse flies. Vet. Microbiol. 150, 207–210. doi: 10.1016/j.vetmic.2011.01.021
Farikou, O., Njiokou, F., Mbida Mbida, J. A., Njitchouang, G. R., Djeunga, H. N., Asonganyi, T., et al. (2010a). Tripartite interactions between tsetse flies, Sodalis glossinidius and trypanosomes–an epidemiological approach in two historical human African trypanosomiasis foci in Cameroon. Infect. Genet. Evol. 10, 115–121. doi: 10.1016/j.meegid.2009.10.008
Farikou, O., Njiokou, F., Simo, G., Asonganyi, T., Cuny, G., and Geiger, A. (2010b). Tsetse fly blood meal modification and trypanosome identification in two sleeping sickness foci in the forest of southern Cameroon. Acta Trop. 116, 81–88. doi: 10.1016/j.actatropica.2010.06.002
Farikou, O., Thevenon, S., Njiokou, F., Allal, F., Cuny, G., and Geiger, A. (2011b). Genetic diversity and population structure of the secondary symbiont of tsetse flies, Sodalis glossinidius, in sleeping sickness foci in Cameroon. PLoS Negl. Trop. Dis. 5:e1281. doi: 10.1371/journal.pntd.0001281
Geib, S. M., Jimenez-Gasco Mdel, M., Carlson, J. E., Tien, M., and Hoover, K. (2009). Effect of host tree species on cellulase activity and bacterial community composition in the gut of larval Asian longhorned beetle. Environ. Entomol. 38, 686–699. doi: 10.1603/022.038.0320
Geiger, A., Cuny, G., and Frutos, R. (2005). Two Tsetse fly species, Glossina palpalis gambiensis and Glossina morsitans morsitans, carry genetically distinct populations of the secondary symbiont Sodalis glossinidius. Appl. Environ. Microbiol. 71, 8941–8943. doi: 10.1128/AEM.71.12.8941-8943.2005
Geiger, A., Fardeau, M. L., Falsen, E., Ollivier, B., and Cuny, G. (2010). Serratia glossinae sp. nov., isolated from the midgut of the tsetse fly Glossina palpalis gambiensis. Int. J. Syst. Evol. Microbiol. 60, 1261–1265. doi: 10.1099/ijs.0.013441-0
Geiger, A., Fardeau, M. L., Grebaut, P., Vatunga, G., Josénando, T., Herder, S., et al. (2009). First isolation of Enterobacter, Enterococcus, and Acinetobacter spp. as inhabitants of the tsetse fly (Glossina palpalis palpalis) midgut. Infect. Genet. Evol. 9, 1364–1370. doi: 10.1016/j.meegid.2009.09.013
Geiger, A., Fardeau, M. L., Njiokou, F., Joseph, M., Asonganyi, T., Ollivier, B., et al. (2011b). Bacterial diversity associated with populations of Glossina spp. from Cameroon and distribution within the Campo sleeping sickness focus. Microb. Ecol. 62, 632–643. doi: 10.1007/s00248-011-9830-y
Geiger, A., Ravel, S., Mateille, T., Janelle, J., Patrel, D., Cuny, G., et al. (2007). Vector competence of Glossina palpalis gambiensis for Trypanosoma brucei s.l. and genetic diversity of the symbiont Sodalis glossinidius. Mol. Biol. Evol. 24, 102–109. doi: 10.1093/molbev/msl135
Geiger, A., Simo, G., Grébaut, P., Peltier, J. B., Cuny, G., and Holzmuller, P. (2011a). Transcriptomics and proteomics in human African trypanosomiasis: current status and perspectives. J. Proteomics 74, 1625–1643. doi: 10.1016/j.jprot.2011.01.016
Gimonneau, G., Tchioffo, M. T., Abate, L., Boissière, A., Awono-Ambéné, P. H., Nsango, S. E., et al. (2014). Composition of Anopheles coluzzii and Anopheles gambiae microbiota from larval to adult stages. Infect. Genet. Evol. 28, 715–724. doi: 10.1016/j.meegid.2014.09.029
Gonzalez-Ceron, L., Santillan, F., Rodriguez, M. H., Mendez, D., and Hernandez-Avila, J. E. (2003). Bacteria in midguts of field-collected Anopheles albimanus block Plasmodium vivax sporogonic development. J. Med. Entomol. 40, 371–374. doi: 10.1603/0022-2585-40.3.371
Gouteux, J. P., and Lancien, J. (1986). Le piège pyramidal à tsé-tsé (Diptera: Glossinidae) pour la capture et la lutte. Essais comparatifs et description de nouveaux systèmes de capture. Trop. Med. Parasitol. 37, 61–66.
Gouveia, C., Asensi, M. D., Zahner, V., Rangel, E. F., and Oliveira, S. M. (2008). Study on the bacterial midgut microbiota associated to different Brazilian populations of Lutzomyia longipalpis (Lutz & Neiva) (Diptera: Psychodidae). Neotrop. Entomol. 37, 597–601. doi: 10.1590/S1519-566X2008000500016
Gouy, M., Guindon, S., and Gascuel, O. (2010). SeaView version 4: a multiplatform graphical user interface for sequence alignment and phylogenetic tree building. Mol. Biol. Evol. 27, 221–224. doi: 10.1093/molbev/msp259
Grébaut, P., Mbida, J. A., Kondjio, C. A., Njiokou, F., Penchenier, L., and Laveissiere, C. (2004). Spatial and temporal patterns of human African trypanosomiasis (HAT) transmission risk in the Bipindi focus, in the forest zone of southern Cameroon. Vector Borne Zoonotic Dis. 4, 230–238. doi: 10.1089/vbz.2004.4.230
Grébaut, P., Wang, S., Bodo, J. M., Ebo'o Eyenga, V., Binzouli, J. J., Ndong Ngoe, C., et al. (2001). Aspects épidémiologiques d'un foyer de la maladie du sommeil mal connu: le foyer de Bipindi au Cameroun. Bull. Liaison Document. de l'OCEAC. 33, 16.
Grimont, F., and Grimont, P. A. (2015). Serratia. Bergey's Manual of Systematics of Archaea and Bacteria. (Oxford: John Wiley & Sons, Inc., in association with Bergey's Manual Trust).
Guillou, L., Bachar, D., Audic, S., Bass, D., Berney, C., Bittner, L., et al. (2012). The Protist Ribosomal Reference database (PR2): a catalog of unicellular eukaryote small sub-unit rRNA sequences with curated taxonomy. Nucleic Acids Res. 41, D597–D604. doi: 10.1093/nar/gks1160
Haines, L. R., Lehane, S. M., Pearson, T. W., and Lehane, M. J. (2010). Tsetse EP protein protects the fly midgut from trypanosome establishment. PLoS Pathog. 6:e1000793. doi: 10.1371/journal.ppat.1000793
Hamidou Soumana, I., Loriod, B., Ravel, S., Tchicaya, B., Simo, G., Rihet, P., et al. (2014). The transcriptional signatures of Sodalis glossinidius in the Glossina palpalis gambiensis flies negative for Trypanosoma brucei gambiense contrast with those of this symbiont in tsetse flies positive for the parasite: possible involvement of a Sodalis-hosted prophage in fly Trypanosoma refractoriness? Infect. Genet. Evol. 24, 41–56. doi: 10.1016/j.meegid.2014.03.005
Hartmann, M., Howes, C. G., VanInsberghe, D., Yu, H., Bachar, D., Christen, R., et al. (2012). Significant and persistent impact of timber harvesting on soil microbial communities in Northern coniferous forests. ISME J. 6, 2199–2218. doi: 10.1038/ismej.2012.84
Hejazi, A., and Falkiner, F. R. (1997). Serratia marcescens. J. Med. Microbiol. 46, 903–912. doi: 10.1099/00222615-46-11-903
Herder, S., Simo, G., Nkinin, S., and Njiokou, F. (2002). Identification of trypanosomes in wild animals from southern Cameroon using the polymerase chain reaction (PCR). Parasite 9, 345–349. doi: 10.1051/parasite/2002094345
Hu, C., and Aksoy, S. (2006). Innate immune responses regulate trypanosome parasite infection of the tsetse fly Glossina morsitans morsitans. Mol. Microbiol. 60, 1194–1204. doi: 10.1111/j.1365-2958.2006.05180.x
Ji, H. L., Qi, L. D., Hong, X. Y., Xie, H. F., and Li, Y. X. (2015). Effects of host sex, plant species, and putative host species on the prevalence of wolbachia in natural populations of Bemisia tabaci (Hemiptera: Aleyrodidae): a modified nested PCR study. J. Econ. Entomol. 108, 210–218. doi: 10.1093/jee/tou004
Lazaro, J. E., Nitcheu, J., Predicala, R. Z., Mangalindan, G. C., Nesslany, F., Marzin, D., et al. (2002). Heptyl prodigiosin, a bacterial metabolite, is antimalarial in vivo and non-mutagenic in vitro. J. Nat. Toxins 11, 367–377.
Lindh, J. M., and Lehane, M. J. (2011). The tsetse fly Glossina fuscipes fuscipes (Diptera: Glossina) harbours a surprising diversity of bacteria other than symbionts. Antonie Van Leeuwenhoek 99, 711–720. doi: 10.1007/s10482-010-9546-x
MacLeod, E. T., Maudlin, I., Darby, A. C., and Welburn, S. C. (2007). Antioxidants promote establishment of trypanosome infections in tsetse. Parasitology 134, 827–831. doi: 10.1017/S0031182007002247
Magoč, T., and Salzberg, S. L. (2011). FLASH: fast length adjustment of short reads to improve genome assemblies. Bioinformatics 27, 2957–2963. doi: 10.1093/bioinformatics/btr507
Majiwa, P. A. O., Thatthi, R., Moloo, S. K., Nyeko, J. H. P., Otieno, L. H., and Maloo, S. (1994). Detection of trypanosome infections in the saliva of tsetse flies and buffy-coat samples from antigenaemic but aparasitaemic cattle. Parasitology 108, 313–322. doi: 10.1017/S0031182000076150
Martin, M. (2011). Cutadapt removes adapter sequences from high-throughput sequencing reads. EMBnet J. 17.1, 1–3. doi: 10.14806/ej.17.1.200
Martinson, V. G., Danforth, B. N., Minckley, R. L., Rueppell, O., Tingek, S., and Moran, N. A. (2011). A simple and distinctive microbiota associated with honey bees and bumble bees. Mol. Ecol. 20, 619–628. doi: 10.1111/j.1365-294X.2010.04959.x
Masiga, D. K., Smyth, A. J., Ayes, P., Bromidge, T. J., and Gibson, W. C. (1992). Sensitive detection of trypanosomes in tsetse flies by DNA amplification. Int. J. Parasitol. 22, 909–918. doi: 10.1016/0020-7519(92)90047-O
Massana, R., Gobet, A., Audic, S., Bass, D., Bittner, L., Boutte, C., et al. (2015). Marine protist diversity in European coastal waters and sediments as revealed by high-throughput sequencing. Environ. Microbiol. 17, 4035–4049. doi: 10.1111/1462-2920.12955
McCutcheon, J. P., and Moran, N. A. (2010). Functional convergence in reduced genomes of bacterial symbionts spanning 200 My of evolution. Genome Biol. Evol. 2, 708–718. doi: 10.1093/gbe/evq055
McMurdie, P. J., and Holmes, S. (2013). phyloseq: an R package for reproducible interactive analysis and graphics of microbiome census data. PLoS ONE 8:e61217. doi: 10.1371/journal.pone.0061217
Melachio, T. T., Simo, G., Ravel, S., De Meeûs, T., Causse, S., Solano, P., et al. (2011). Population genetics of Glossina palpalis palpalis from central African sleeping sickness foci. Parasit. Vectors 4:140. doi: 10.1186/1756-3305-4-140
Moloo, S. K., Grootenhuis, J. G., Kar, S. K., and Karstad, L. (1988). Survival and reproductive performance of female Glossina morsitans morsitans when maintained on the blood of different species of wild mammals. Med. Vet. Entomol. 2, 347–350. doi: 10.1111/j.1365-2915.1988.tb00207.x
Mondani, L., Benzerara, K., Carrière, M., Christen, R., Mamindy-Pajany, Y., Février, L., et al. (2011). Influence of uranium on bacterial communities: a comparison of natural uranium-rich soils with controls. PLoS ONE 6:e25771. doi: 10.1371/journal.pone.0025771
Moore, S., Shrestha, S., Tomlinson, K. W., and Vuong, H. (2012). Predicting the effect of climate change on African trypanosomiasis: integrating epidemiology with parasite and vector biology. J. R. Soc. Interface 9, 817–830. doi: 10.1098/rsif.2011.0654
Moran, N. A., Tran, P., and Gerardo, N. M. (2005). Symbiosis and insect diversification: an ancient symbiont of sap-feeding insects from the bacterial phylum Bacteroidetes. Appl. Environ. Microbiol. 71, 8802–8810. doi: 10.1128/AEM.71.12.8802-8810.2005
Moser, D. R., Cook, G. A., Ochs, D. E., Bailey, C. P., McKane, M. R., and Donelson, J. E. (1989). Detection of Trypanosoma congolense and Trypanosoma brucei subspecies by DNA amplification using the polymerase chain reaction. Parasitology 99, 57–66. doi: 10.1017/S0031182000061023
Njiokou, F., Laveissière, C., Simo, G., Nkinin, S., Grébaut, P., Cuny, G., et al. (2006). Wild fauna as a probable animal reservoir for Trypanosoma brucei gambiense in Cameroon. Infect. Genet. Evol. 6, 147–153. doi: 10.1016/j.meegid.2005.04.003
Njiokou, F., Simo, G., Nkinin, S. W., Laveissière, C., and Herder, S. (2004). Infection rate of Trypanosoma brucei s.l., T. vivax, T. congolense ”forest type,” and T. simiae in small wild vertebrates in south Cameroon. Acta Trop. 92, 139–146. doi: 10.1016/j.actatropica.2004.04.011
Nkinin, S. W., Njiokou, F., Penchenier, L., Grébaut, P., Simo, G., and Herder, S. (2002). Characterization of Trypanosoma brucei s.l subspecies by izoenzymes in domestic pigs from the Fontem sleeping sickness focus of Cameroon. Acta Trop. 81, 225–232. doi: 10.1016/S0001-706X(01)00213-3
Pawlowski, J., Christen, R., Lecroq, B., Bachar, D., Shahbazkia, H. R., Amaral-Zettler, L., et al. (2011). Eukaryotic richness in the abyss: insights from pyrotag sequencing. PLoS ONE 6:e18169. doi: 10.1371/journal.pone.0018169
Poinar, G. O. Jr., Wassink, H. J., Leegwater-van der Linden, M. E., and van der Geest, L. P. (1979). Serratia marcescens as a pathogen of tsetse flies. Acta Trop. 36, 223–227.
Porter, T. M., Shokralla, S., Baird, D., Golding, G. B., and Hajibabaei, M. (2016). Ribosomal, DNA, and plastid markers used to sample fungal and plant communities from wetland soils reveals complementary biotas. PLoS ONE 11:e0142759. doi: 10.1371/journal.pone.0142759
Quast, C., Pruesse, E., Yilmaz, P., Gerken, J., Schweer, T., Yarza, P., et al. (2012). The SILVA ribosomal RNA gene database project: improved data processing and web-based tools. Nucleic Acids Res. 41, D590–D596. doi: 10.1093/nar/gks1219
Rani, A., Sharma, A., Rajagopal, R., Adak, T., and Bhatnagar, R. K. (2009). Bacterial diversity analysis of larvae and adult midgut microflora using culture-dependent and culture-independent methods in lab-reared and field-collected Anopheles stephensi-an Asian malarial vector. BMC Microbiol. 9:96. doi: 10.1186/1471-2180-9-96
Rio, R. V., Hu, Y., and Aksoy, S. (2004). Strategies of the home-team: symbioses exploited for vector-borne disease control. Trends Microbiol. 12, 325–336. doi: 10.1016/j.tim.2004.05.001
Rio, R. V. M., Symula, R. E., Wang, J., Lohs, C., Wu, Y. N., Snyder, A. K., et al. (2012). Insight into the transmission biology and species-specific functional capabilities of tsetse (Diptera: Glossinidae) obligate symbiont Wigglesworthia. MBio 3, e00240–e00211. doi: 10.1128/mBio.00240-11
Rose, C., Belmonte, R., Armstrong, S. D., Molyneux, G., Haines, L. R., Lehane, M. J., et al. (2014). An investigation into the protein composition of the teneral Glossina morsitans morsitans peritrophic matrix. PLoS Negl. Trop. Dis. 8:e2691. doi: 10.1371/journal.pntd.0002691
Segata, N., Waldron, L., Ballarini, A., Narasimhan, V., Jousson, O., and Huttenhower, C. (2012). Metagenomic microbial community profiling using unique clade-specific marker genes. Nat. Methods 9, 811–814. doi: 10.1038/nmeth.2066
Shaw, A. P., Torr, S. J., Waiswa, C., Cecchi, G., Wint, G. R., Mattioli, R. C., et al. (2013). Estimating the costs of tsetse control options: an example for Uganda. Prev. Vet. Med. 110, 290–303. doi: 10.1016/j.prevetmed.2012.12.014
Sievers, F., Wilm, A., Dineen, D., Gibson, T. J., Karplus, K., Li, W., et al. (2011). Fast, scalable generation of high-quality protein multiple sequence alignments using Clustal Omega. Mol. Syst. Biol. 7:539. doi: 10.1038/msb.2011.75
Simo, G., Njiokou, F., Mbida Mbida, J. A., Njitchouang, G. R., Herder, S., Asonganyi, T., et al. (2008). Tsetse fly host preference from sleeping sickness foci in Cameroon. Epidemiological implications. Infect. Genet. Evol. 8, 34–39. doi: 10.1016/j.meegid.2007.09.005
Solano, P., Salou, E., Rayaisse, J.-B., Ravel, S., Gimonneau, G., Traore, I., et al. (2015). “Do tsetse flies only feed on blood?” Infect. Genet. Evol. 36, 184–189. doi: 10.1016/j.meegid.2015.09.016
Tchioffo, M. T., Boissière, A., Abate, L., Nsango, S. E., Bayibéki, A. N., Awono-Ambéné, P. H., et al. (2016). Dynamics of bacterial community composition in the malaria mosquito's Epithelia. Front. Microbiol. 6:1500. doi: 10.3389/fmicb.2015.01500
Tchouomene-Labou, J., Nana-Djeunga, H., Simo, G., Njitchouang, G. R., Cuny, G., Asonganyi, T., et al. (2014). Spatial and temporal variations relevant to tsetse control in the Bipindi focus of southern Cameroon. Parasit. Vectors 6:193. doi: 10.1186/1756-3305-6-193
Terenius, O., Lindh, J. M., Eriksson-Gonzales, K., Bussière, L., Laugen, A. T., Bergquist, H., et al. (2012). Midgut bacterial dynamics in Aedes aegypti. FEMS Microbiol. Ecol. 80, 556–565. doi: 10.1111/j.1574-6941.2012.01317.x
Vickerman, K., Tetley, L., Hendry, A., and Turner, C. M. (1988). Biology of African trypanosomes in the tsetse fly. Biol. Cell 64, 109–119. doi: 10.1016/0248-4900(88)90070-6
Wang, J., Wu, Y., Yang, G., and Aksoy, S. (2009). Interactions between mutualist Wigglesworthia and tsetse peptidoglycan recognition protein (PGRP-LB) influence trypanosome transmission. Proc. Natl. Acad. Sci. U.S.A. 106, 12133–12138. doi: 10.1073/pnas.0901226106
Weiss, B. L., Savage, A. F., Griffith, B. C., Wu, Y., and Aksoy, S. (2014). The peritrophic matrix mediates differential infection outcomes in the tsetse fly gut following challenge with commensal, pathogenic, and parasitic microbes. J. Immunol. 193, 773–782. doi: 10.4049/jimmunol.1400163
Weiss, B. L., Wang, J., and Aksoy, S. (2011). Tsetse immune system maturation requires the presence of obligate symbionts in larvae. PLoS Biol. 9:e1000619. doi: 10.1371/journal.pbio.1000619
Weiss, B. L., Wang, J., Maltz, M. A., Wu, Y., and Aksoy, S. (2013). Trypanosome infection establishment in the tsetse fly gut is influenced by microbiome-regulated host immune barriers. PLoS Pathog. 9:e1003318. doi: 10.1371/journal.ppat.1003318
Welburn, S. C., and Maudlin, I. (2012). Priorities for the elimination of sleeping sickness. Adv. Parasitol. 79, 299–337. doi: 10.1016/B978-0-12-398457-9.00004-4
Welburn, S. C., Maudlin, I., and Molyneux, D. H. (1994). Midgut lectin activity and sugar specificity in teneral and fed tsetse. Med. Vet. Entomol. 8, 81–87. doi: 10.1111/j.1365-2915.1994.tb00391.x
Zélé, F., Nicot, A., Berthomieu, A., Weill, M., Duron, O., and Rivero, A. (2014). Wolbachia increases susceptibility to Plasmodium infection in a natural system. Proc. Biol. Sci. 281:20132837. doi: 10.1098/rspb.2013.2837
Zhang, J., Kobert, K., Flouri, T., and Stamatakis, A. (2014). PEAR: a fast and accurate Illumina Paired-End reAd mergeR. Bioinformatics 30, 614–620. doi: 10.1093/bioinformatics/btt593
Zouache, K., Raharimalala, F. N., Raquin, V., Tran-Van, V., Raveloson, L. H., Ravelonandro, P., et al. (2011). Bacterial diversity of field-caught mosquitoes, Aedes albopictus and Aedes aegypti, from different geographic regions of Madagascar. FEMS Microbiol. Ecol. 75, 377–389. doi: 10.1111/j.1574-6941.2010.01012.x
Keywords: Glossina, trypanosome, microbiome, meta-taxonomics, sleeping sickness, nagana
Citation: Jacob F, Melachio TT, Njitchouang GR, Gimonneau G, Njiokou F, Abate L, Christen R, Reveillaud J and Geiger A (2017) Intestinal Bacterial Communities of Trypanosome-Infected and Uninfected Glossina palpalis palpalis from Three Human African Trypanomiasis Foci in Cameroon. Front. Microbiol. 8:1464. doi: 10.3389/fmicb.2017.01464
Received: 14 April 2017; Accepted: 20 July 2017;
Published: 03 August 2017.
Edited by:
Alexandre Morrot, Federal University of Rio de Janeiro, BrazilReviewed by:
Brice Rotureau, Institut Pasteur, FranceFrédéric Mahé, Centre de Coopération Internationale en Recherche Agronomique Pour le Développement (CIRAD), France
Copyright © 2017 Jacob, Melachio, Njitchouang, Gimonneau, Njiokou, Abate, Christen, Reveillaud and Geiger. This is an open-access article distributed under the terms of the Creative Commons Attribution License (CC BY). The use, distribution or reproduction in other forums is permitted, provided the original author(s) or licensor are credited and that the original publication in this journal is cited, in accordance with accepted academic practice. No use, distribution or reproduction is permitted which does not comply with these terms.
*Correspondence: Anne Geiger, YW5uZS5nZWlnZXJAaXJkLmZy