- 1Department of Microbiology, Defence Food Research Laboratory, Mysore, India
- 2Department of Biotechnology, Vignan’s Foundation for Science, Technology and Research University, Guntur, India
Safety and protective efficacy of recombinant multi-epitope subunit vaccine (r-AK36) was evaluated in a mouse model. Recombinant AK36 protein comprised of immunodominant antigens from outer membrane proteins (Omp’s) of Klebsiella pneumoniae namely OmpA and OmpK36. r-AK36 was highly immunogenic and the hyperimmune sera reacted strongly with native OmpA and OmpK36 proteins from different K. pneumoniae strains. Hyperimmune sera showed cross-reactivity with Omp’s of other Gram-negative organisms. Humoral responses showed a Th2-type polarized immune response with IgG1 being the predominant antibody isotype. Anti-r-AK36 antibodies showed antimicrobial effect during in vitro testing with MIC values in the range of 25–50 μg/ml on different K. pneumoniae strains. The recombinant antigen elicited three fold higher proliferation of splenocytes from immunized mice compared to those with sham-immunized mice. Anti-r-AK36 antibodies also exhibited in vitro biofilm inhibition property. Subunit vaccine r-AK36 immunization promoted induction of protective cytokines IL-2 and IFN-γ in immunized mice. When r-AK36-immunized mice were challenged with 3 × LD100 dose, ∼80% of mice survived beyond the observation period. Passive antibody administration to naive mice protected them (67%) against the lethal challenge. Since the targeted OMPs are conserved among all K. pneumoniae serovars and due to the strong nature of immune responses, r-AK36 subunit vaccine could be a cost effective candidate against klebsiellosis.
Introduction
Klebsiella pneumoniae is a Gram-negative opportunistic pathogen that belongs to family Enterobacteriaceae. It is the most common cause of nosocomial respiratory tract infections and second most frequent cause of bacteraemia and urinary tract infections (Center for Disease Control [CDC], 1974; Ahmad et al., 2012; Huang et al., 2015). K. pneumoniae is also an important pathogen causing severe morbidity and mortality (>50%) in intensive care units, pediatric and surgical wards (Rice et al., 1990; Coovadia et al., 1992; Palusiak, 2015). K. pneumoniae is recognized as a major health threat worldwide and the treatment options of Klebsiella infections are limited owing to high incidence of multiple drug resistance and adverse antibiotic reactions (Breecher, 2007; Vieira et al., 2016). A novel and distinct K. pneumoniae variant called hypervirulent K. pneumoniae (hvKP) has emerged which is predicted to become a major threat in Asia and Western countries (Diago-Navarro et al., 2017). Infections caused by such strains are reported to be associated with higher morbidity and mortality even with antibiotic sensitive strains. In comparison to classical K. pneumoniae, hvKP strains exhibit enhanced virulence in terms of over production of CPS, possess anti-phagocytosis and severe metastases, cause severe infections such as pyogenic liver abscess, pneumonia and endophthalmitis (Wang et al., 2017). Management of hvKP infections is going to be extremely challenging due to multidrug resistance including acquisition of extended-spectrum β-lactamases, carbapenemases and recently described NDM-1 (Elemam et al., 2009). Control of K. pneumoniae is also hampered by additional mechanisms such as endotoxin production that induces septic shock, CPS that inhibits phagocytosis and resistance to compliment-mediated killing (Merino et al., 1992; Podschun and Ullmann, 1998; Kim et al., 2002). This has directed attention toward controlling K. pneumoniae infections through bacterial clearance from body employing alternate strategies to improve immune defenses such as probiotics (Vieira et al., 2016) or more specific means such as vaccination and antibody therapy (Jain et al., 2015; Lee et al., 2015).
At present, the knowledge regarding the pathogenic mechanisms utilized by K. pneumoniae to develop an infection is limited. However, five main classes of virulence factors were identified namely capsule, LPS, siderophores, adhesins (pili, fimbriae, OMPs) and exotoxins. K. pneumoniae might not be simple to control by regular vaccine approaches due to its high degree of antigenic variation among strains including CPS and LPS antigens. Clearance of K. pneumoniae from the host system requires effective host defense mechanisms, to which bacterial surface plays a major role. Three components of bacterial surface are suspected in the development of immunity: CPS, LPS and OMPs. Capsular antigens of K. pneumoniae have been implicated as important virulence factors and help the bacteria in preventing the killing by serum and escaping from the host immune system by avoiding phagocytosis. Studies have indicated a direct correlation between capsule size and pathogenicity (Simoons-Smit et al., 1986). Till date common vaccines used against Klebsiella infections are based on CPS and also LPS to a certain extent (Cryz, 1983, 1990; Yadav et al., 2005). However, CPS and LPS provide only type-specific protection against these infections since at least seventy seven capsular (K) antigen types and eight LPS (O) antigen serotypes exist. OMPs are surface proteins which exist as trimers and act as water-filled channels that allow the hydrophilic molecules across the membrane. These are involved in antibiotic resistance mechanisms and contribute to the virulence of the organism. OMPs of K. pneumoniae are considered safe as subunit vaccines and have been tested and shown to induce host specific antibodies and found effective without the need of additional adjuvants in animal models (Sun et al., 2014). OmpK36 is produced by the majority of extended-spectrum beta-lactamase-producing (ESBL) K. pneumoniae members and it is also reported to contribute to resistance or reduced susceptibility to carbapenems in ESBL-producing K. pneumoniae strains (Mena et al., 2006; Skurnik et al., 2010). OmpA is one of the major proteins of outer membranes of Gram-negative bacteria and is highly conserved among the members of Enterobacteriaceae (Pichavant et al., 2003). OmpA has been shown to possess adjuvant properties and also induce specific humoral and anti-tumor cytotoxic responses when coupled with tumor antigens (Jeannin et al., 2002). Individually OmpA and OmpK36 as DNA vaccines were shown to confer protective immune responses in mouse model (Kurupati et al., 2011). Therefore, OmpA and OmpK36 could be ideal targets for design of anti-Klebsiella vaccine since these targets are carried by all the strains unlike O and K antigens.
For effective control of K. pneumoniae infections in the wake of widespread multiple antibiotic resistance mechanisms, preventive measures by active and passive immunization approaches appear to be promising. Therefore, in the present study we constructed a chimeric gene comprising immunodominant regions of OmpA and OmpK36 fused together by a glycine linker followed by evaluation of its protective efficacy in BALB/c mouse model. Hyperimmune serum from immunized mice was characterized for its in vitro neutralization ability against virulent K. pneumoniae strains and in vivo protective efficacy against lethal challenge.
Materials and Methods
Materials
Dehydrated media and supplements were procured from HiMedia laboratories, India. Taq DNA polymerase, dNTPs, IPTG and adjuvants were from Sigma–Aldrich, India. Pfu DNA polymerase was from Fermentas, United States. pRSET vector series, Escherichia coli DH5α and BL21DE3 strains are from Invitrogen, United States. K. pneumoniae ATCC 13883 and ATCC 10031 strains were procured from MicroBiologics Inc., United States. All the cell culture reagents were from Sigma–Aldrich, India. Specific pathogen-free BALB/c mice used in the study were received from Central Animal Housing Facility of Defence Food Research Laboratory (DFRL), Mysore city, India.
Bacterial Strains
Genomic DNA of K. pneumoniae ATCC 13883 strain was used for amplification of ompK36 and ompA genes for chimeric gene construction. Additionally, K. pneumoniae E-1716 a clinical isolate belonging to K1 serotype isolated from sputum sample collected from a patient admitted at Sri Dharmasthala Manjunatheshwara (SDM) College of medical science and hospital, Dharwad, India was used for challenge experiments based on its higher virulence (lower LD50 values) potential. For animal experiments, K. pneumoniae was cultured from glycerol stocks in BHI broth and incubated overnight at 37°C with vigorous shaking. Following centrifugation, bacterial cells were washed two times and suspended in 1x PBS solution. LD50values were determined by intraperitoneal administration of different serial dilutions of three K. pneumoniae strains.
Cloning, Expression and Purification
Primers were designed for amplification of ompA and ompK36 genes based on gene sequences available at GenBank (Table 1). The individual genes were fused using PCR by ‘gene splicing by overlap extension’ (SOE-PCR) method employing the primers shown in Table 1. Restriction sites (BamHI and Hind III) were added to 5′ end of primers so as to clone the resulting fusion gene in-frame with the coding sequence of pRSETA vector. The recombinant plasmid was transferred to E. coli DH5α and BL21(DE3) strains. A single transformed BL21(DE3) clone was subjected to induction with IPTG followed by testing for chimeric protein expression by SDS-PAGE analysis and Western blotting with anti-histidine antibodies. For bulk purification, recombinant clone was cultured in 500 ml Luria-Bertani broth followed by purification of histidine-tagged (6xHis) protein using Ni-NTA resin (Qiagen) by gravity flow protocol as per manufacturer’s instructions.
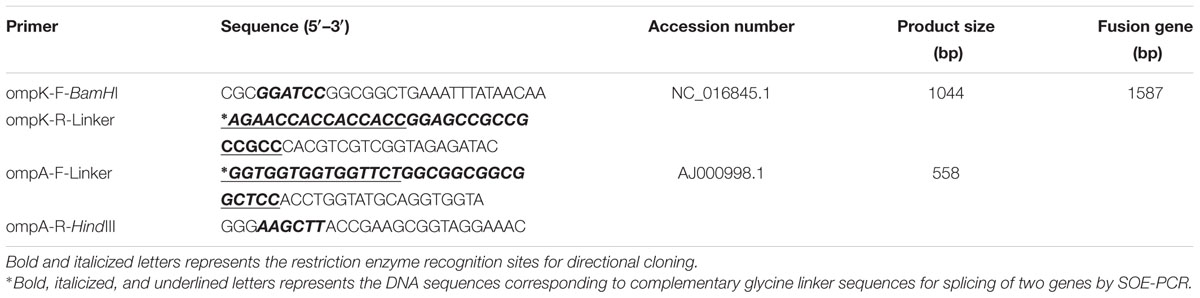
TABLE 1. List of primers, their sequences and accession numbers used for construction of chimeric rAK36 gene.
Immunization and Hyperimmune Sera Generation
Six to seven-week-old female BALB/c mice were grouped into three batches of 10 mice each. Blood was drawn from all the mice 2 days prior to the start of immunization schedule and the pooled serum served as negative control in all the experiments. Two groups of mice received 50 μg of recombinant protein subcutaneously in emulsion with Freund’s complete adjuvant followed by three booster doses at 10 days interval with the same quantity of protein in Freund’s incomplete adjuvant. Third group served as a control receiving only adjuvants in 1x PBS by intraperitoneal route. Blood was drawn 2 days prior to first immunization, 1 day prior to the second booster and 7 days past the last immunization and the pooled sera were used for evaluation of antibody titers and isotypes. Of the 20 immunized mice, two sets of 6 mice each were used in active challenge and memory groups. Three mice were used for splenocyte stimulation experiments. Remaining mice served as a backup in case of accidental deaths. Of the 10 sham-immunized mice, 6 were used in active challenge and 3 were used for splenocyte proliferation assay. For passive immunization and challenge naïve mice were used.
Humoral Response and Antibody Isotyping
Antibody titers of the pooled serum were measured after the second and the third booster immunizations by indirect ELISA employing r-AK36 protein as antigen. Maximum dilution at which the mean absorbance (OD600) was at least two times more or equals the mean value of negative control was considered as endpoint titers. Serum samples were diluted 1:500 in PBS followed by antibody isotype determination employing the mouse monoclonal antibody isotyping reagents (Sigma, United States) as per manufacturer’s recommendations. Sham-immunized serum served as controls during isotype determination.
Western Blot Analysis
Western blot analysis was performed to test the reactivity of r-AK36 hyperimmune sera with native OmpA and OmpK36 proteins from various K. pneumoniae strains along with other Enterobacteriaceae members. Whole-cell lysates of various bacterial strains were separated on 12% SDS-PAGE gels followed by electrotransfer on to nitrocellulose membrane. Membranes were blocked in 5% skim milk solution followed by washing in PBS-Tween 20 (0.05%) and probing with 1:1000 dilutions of anti-r-AK36 sera. After washing, membranes were treated with 1:3000 dilutions of goat anti-mouse IgG conjugated with horseradish peroxidase (HRP). Blots were developed either with diaminobenzidine tetrahydrochloride (Sigma–Aldrich, India) and 30% H2O2 or ready to use tetramethylbenzidine (Bangalore Genei, India) solution.
Lymphocyte Proliferation Assay and Cytokine Analysis
Seven days after the last immunization, aseptically isolated spleens from immunized and sham-immunized mice were gently ruptured on a wire mesh using sterile glass rod with round edges to break splenic capsule and separating connective tissue followed by flushing with sterile DMEM. The resultant splenocytes were treated with ACK buffer (150 mM NH4Cl, 10 mM KHCO3 and 0.1 mM EDTA) to remove RBCs. The splenocytes were resuspended in Dulbecco’s Modified Eagle’s medium (DMEM) supplemented with 10% heat-inactivated fetal bovine serum (Hyclone, Thermo scientific, United States), 5 mM glutamine, 50 U/ml penicillin, 50 μg/ml of streptomycin and 0.2% NaHCO3. This experiment was performed three times with three mice from active and sham-immunized groups. The number of splenocytes used for the stimulation studies was 106 cells/well. The antigen (recombinant protein) was used at a concentration of 2, 4, 6, 8, and 10 μg/well. Concanavalin A (Sigma–Aldrich, United States) was included as a mitogen for positive control at a concentration of 10 μg/ml. The experiment was performed in triplicates. The plates were incubated at 37°C in 5% CO2 for 72 h. The proliferation was measured using MTT reagent (Sigma, India) as described elsewhere (Reddy et al., 2015). Lymphocyte proliferation was calculated as stimulation index employing the following formula: Stimulation index = OD570 with antigen/OD570 without antigen. The supernatants from cultured splenocytes were evaluated for cytokine estimation (IL-2, IL-4, IL-10, IFN-γ, and TNF-α) employing commercial kits (MabTech, Nacka, Sweden) based on sandwich ELISA.
In Vitro Antimicrobial Susceptibility Testing
To determine the antibacterial activity of r-AK36 antibodies against K. pneumoniae, microtiter plate-based in vitro antimicrobial susceptibility test was carried out as per CLSI recommendations. In brief, 1 mg/ml of antibody stock was serially diluted in PBS to 100, 75, 50, 25, and 10 μg/ml concentrations and 100 μl volumes of each dilution were tested for antimicrobial activity in triplicates. Approximately 100 μl of 106/ml CFU of K. pneumoniae ATCC 13883, K. pneumoniae ATCC 10031 and K. pneumoniae E-1716 strain diluted in double-strength Mueller-Hinton broth were added to each antibody dilution in three replicates. Only the PBS with no antibody and K. pneumoniae in MH broth served as negative control. Only PBS along with MH broth served as a blank. Rifampicin antibiotic served as positive control during antimicrobial testing. Absorbance at OD600 was recorded before the start of experiment followed by incubation of plates at 37°C overnight. The lowest antibody dilution at which there was no increase in turbidity after overnight incubation was considered as MIC of the polyclonal antibodies.
Biofilm Inhibition Assay
Effect of sub-inhibitory concentrations of r-AK36 antibodies on biofilm formation was assessed by microtiter plate method as described by Sun et al. (2005) with minor modifications. K. pneumoniae standard and clinical isolates were grown overnight in BHI broth at 37°C. Bacterial cells were harvested and the cell count was adjusted to 106 CFU/ml using sterile double-strength BHI broth (with 1% glucose) and 100 μl was mixed with 100 μl of 50 μg/ml polyclonal antibodies and incubated at 37°C overnight with shaking. Post-incubation, plate was washed two times in PBS to remove non-adherent cells. Adherent biofilm was fixed by adding 200 μl of methanol for 10 min. After drying the plates, 250 μl of 1% crystal violet was added to each well and incubated for 5 min. Plates were washed under running water, dried and the adherent biofilm was solubilised by adding 250 μl of 33% glacial acetic acid. OD was measured at 570 nm in Tecan multimode plate reader. A control was included for each strain with only BHI broth and glucose without antibodies. Each sample was tested in triplicates. The percent inhibition of biofilm formation of each K. pneumoniae strain was determined using the formula: (OD570 in control – OD570 in treatment)/(OD570 in control) × 100.
Challenge Studies
For studying the protective efficacy of the recombinant protein, the mice were challenged with a hypervirulent strain (K1 serotype) of K. pneumoniae whose lethal dose (LD50) had been determined to be 3 × 105 CFU. Two groups of six mice each of both immunized and sham-immunized were challenged intraperitoneally 10 days after the last immunization with 106 CFU in 100 μL of 0.9% saline. The protective efficacy of the recombinant protein was checked by evaluating the survival rates after 15 days of challenge. Every day the animals were observed for signs of illness, such as weight loss, change in coat appearance, and lack of movement. On day 15 the remaining mice were terminated to collect blood for antibacterial assay and organs were collected for testing bacterial load. Similar challenge experiments were performed to test the persistence of immunization and memory response 120 days after the last immunization and observed for survival rates.
Bacterial Load in Organ Systems
After the 15 days observation period following bacterial challenge, two mice were humanely euthanized by CO2 sedation followed by cervical dislocation. Before excising the organs, the surface of the viscera was swiped with sterile cotton swabs to check the bacterial translocation and cultured in BHI broth. Further kidney, spleen, liver, and lungs were excised and transferred to sterile preweighed Eppendorf tubes. The tissues from organs were macerated by plastic tissue grinder and the resultant homogenate and blood samples were plated on plate count agar. The colony count was measured following incubation at 37°C for 24 h.
Passive Immunotherapy
The in vivo prophylactic and neutralization efficacy of the hyperimmunepolysera was determined by performing passive antibody administration where in 6-week-old naïve female BALB/c mice (n = 6) were injected intraperitoneally with 200 μL of polysera and left for 24 h followed by challenge with 2 × LD100 (4 × 105 CFU) of K. pneumoniae E-1716 strain. A control group (n = 6) was injected with same quantity of PBS alone followed by challenge with same quantity of lethal dosage. Mice were observed for a period of 14 days for any possible deaths. Survival of mice post-challenge during the observation period is represented by Kaplan–Meier graphs using GraphPad Prism 5.0 version.
Statistical Analysis
The data were presented either as mean ± SD or individual values+grand median. Mantel-Cox (log-rank) test was used to compare the survival curves and Student’s t-test was used for other statistical comparisons. All graphical illustrations were constructed by GraphPad Prism 5 software. Statistical difference between different groups were analyzed by univariate ANOVA using Minitab 17. Significance (P)-value summary: ∗P ≤ 0.05, ∗∗P ≤ 0.01, ∗∗∗P ≤ 0.001; no significance #.
Animal Ethics
All the animal experiments, biosafety procedures and genetic manipulations undertaken in this study have been performed with permission and following the guidelines laid by the Institutional Animal Ethics Committee (IAEC) and the Institutional Bio-Safety Committee (IBSC) of Defence Food Research Laboratory, Mysore. Permission for using the experimental animals was approved by Institutional Animal Ethical Committee during the meeting held on 4th December, 2015 at DFRL, Mysore. Animals were received from DFRL animal housing facility (28/1999/CPCSEA dt. 11 March, 1999) and maintained under hygienic conditions in animal house of Microbiology Department of DFRL, Mysore. The clinical isolate used for challenge experiments in this study was received as pure culture from the culture collection of SDM hospital after taking mutual consents of Head of microbiology departments of SDM hospital and DFRL. Direct sampling of clinical specimens was not carried out in this study. The IAEC of DFRL approved the protocols employed.
Results
Synthesis of Chimeric Recombinant Protein r-AK36
Fusion gene comprising immunodominant regions of K. pneumoniae ompA and ompK36 genes was generated by SOE-PCR (Figures 1A,B) and cloned into pRSETA vector. The chimeric r-AK36 gene was sequenced and was found not to possess any deletions or point mutations when compared to the expected sequence. Transformation of recombinant plasmid in E. coli host was followed by induction with IPTG which resulted in the expression of a protein at 61 kDa region as observed in SDS-PAGE (Figure 1C) and Western blot analysis (Figure 1D). This was in accordance with the predicted size of the protein deduced from the fusion gene sequence in vector. Histidine-tagged (6xhis) recombinant protein in inclusion bodies was extracted and purified from 500 ml induced culture by immobilized metal affinity chromatography under denaturing conditions (Figure 1E). Purified protein was subjected to step dialysis with decreasing concentrations of urea under reducing environment. Final protein concentration was quantified and adjusted to 2.5 mg/ml by Lowry’s calorimetric assay against BSA standard.
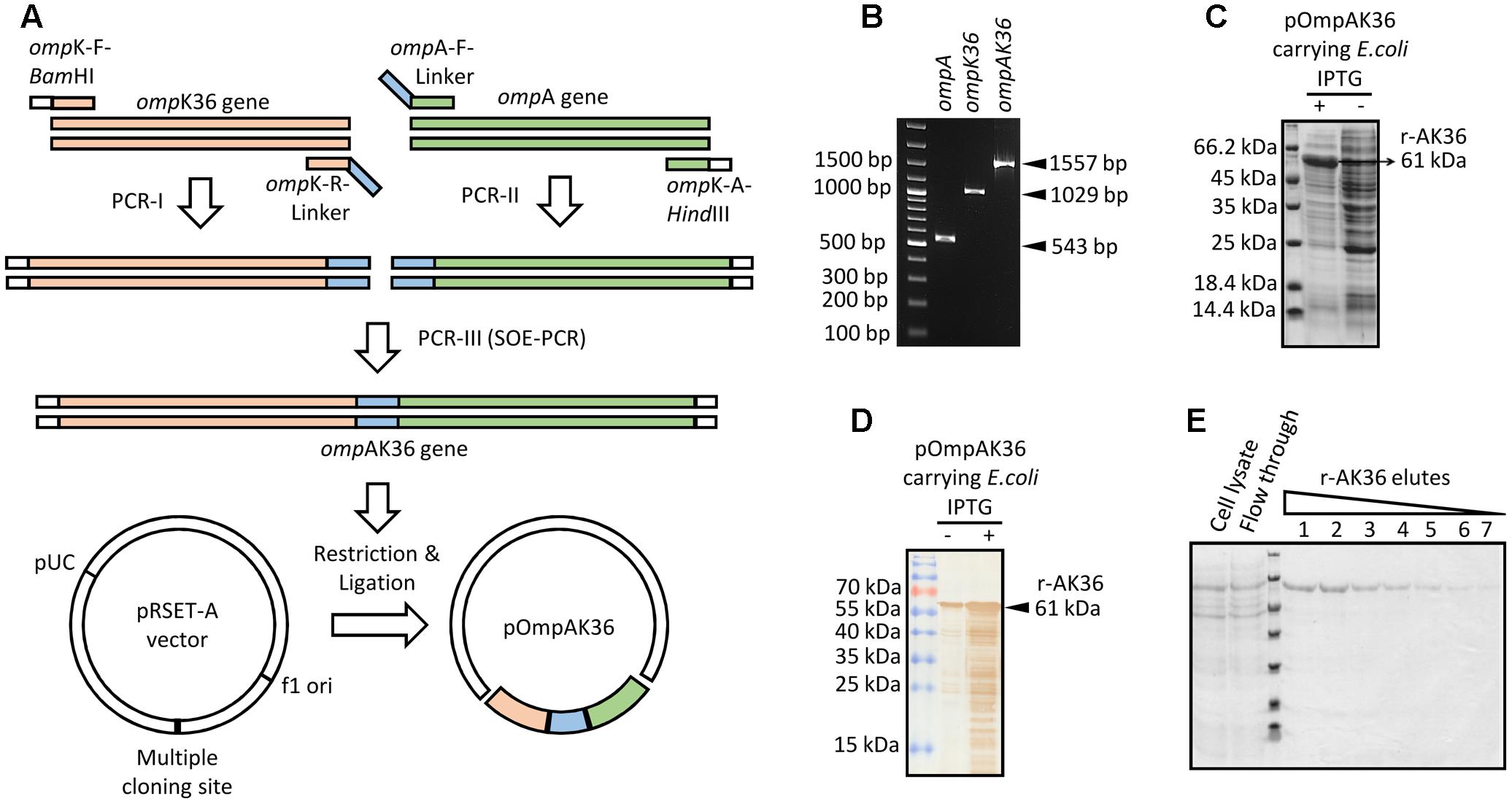
FIGURE 1. Cloning and expression of r-AK36 fusion protein in pRSETA vector. (A) Schematic representation of PCR amplification, splicing and cloning of chimeric r-AK36 gene in pRSETA vector. (B) Agarose gel showing PCR amplified ompA and ompK36 genes and ompAK36 spliced gene. (C) Stained SDS-PAGE gel showing expressed rAK36 protein upon IPTG induction. (D) Western blot probed with anti-histidine antibodies showing 6x-His tagged r-AK36 protein. (E) SDS-PAGE gel showing r-AK36 protein from different elutions following IMAC purification with Ni-NTA agarose.
Immunogenicity of r-AK36 in BALB/c Mice
In immunized mice, a progressive and significant increase of anti-r-AK36 antibodies was observed. After three booster doses (Figure 2A), the end-point antibody titers reached 1:64000, while the control sera from sham-immunized mice showed no reactivity with r-AK36 in indirect ELISA (Figure 2B). Pooled sera from r-AK36-immunized mice, when subjected to isotype analysis, a Th2-type immune response was observed indicated by the predominance of IgG1 immunoglobulin. Surprisingly, IgG2b levels also predominated the immune response indicating Th1 polarization. IgM antibodies also persisted in serum even after last immunization and Ig class switching (Figure 2C). Despite significant IgG2b levels, the relative degree of contribution by Th2- and Th1-type humoral immune responses determined as IgG1:IgG2a ratio is >1, indicating r-AK36 in CFA/IFA adjuvant system induced primarily Th2-type antibody response in BALB/c mice.
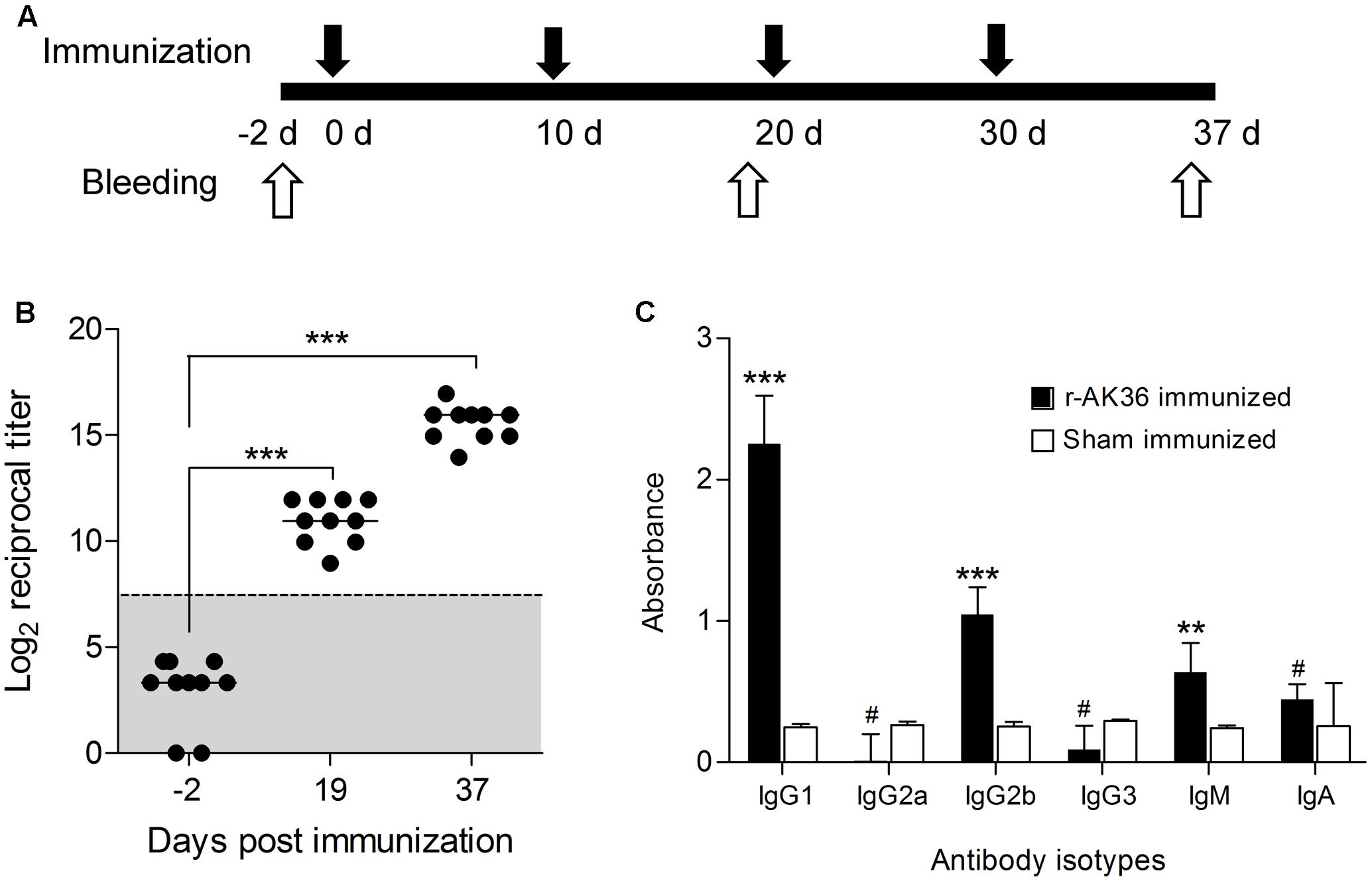
FIGURE 2. Determination of antibody titers and antibody isotypes from r-AK36 evoked anti-r-AK36 serum in BALB/c mice. (A) Schematic representation of immunization and blood collection schedules of r-AK36 antigen. (B) Estimation of antibody titers of anti-r-AK36 serum induced by r-AK36 subunit vaccine at different time points of immunization. (C) Antibody isotypes induced by r-AK36 was evaluated by ELISA using isotype-specific monoclonal antibodies for IgG1, IgG2a, IgG2b, IgG3, IgM and IgA. Data are represented in mean ± SD (n = 8).Significance (P)-value summary analyzed by univariate ANOVA: ∗P ≤ 0.05, ∗∗P ≤ 0.01; ∗∗∗P ≤ 0.001; no significance #.
The specific reactivity of anti-r-AK36 antibodies with the native OMPs of various K. pneumoniae and other bacterial species was assessed by Western blot analysis. Anti-r-AK36 antibodies showed strong reactivity with native OmpA (37 kDa) and OmpK36 (39.6 kDa) proteins from different K. pneumoniae standard and isolated strains (Figure 3). Other Gram-negative rods of Enterobacteriaceae family showed varying reactivities at different molecular sizes. This is not surprising considering the conserved nature of epitopes on OmpA and OmpK36 proteins among Enterobacteriaceae members. Reactivities of anti-r-AK36 antibodies with different bacterial species are provided in Table 2.
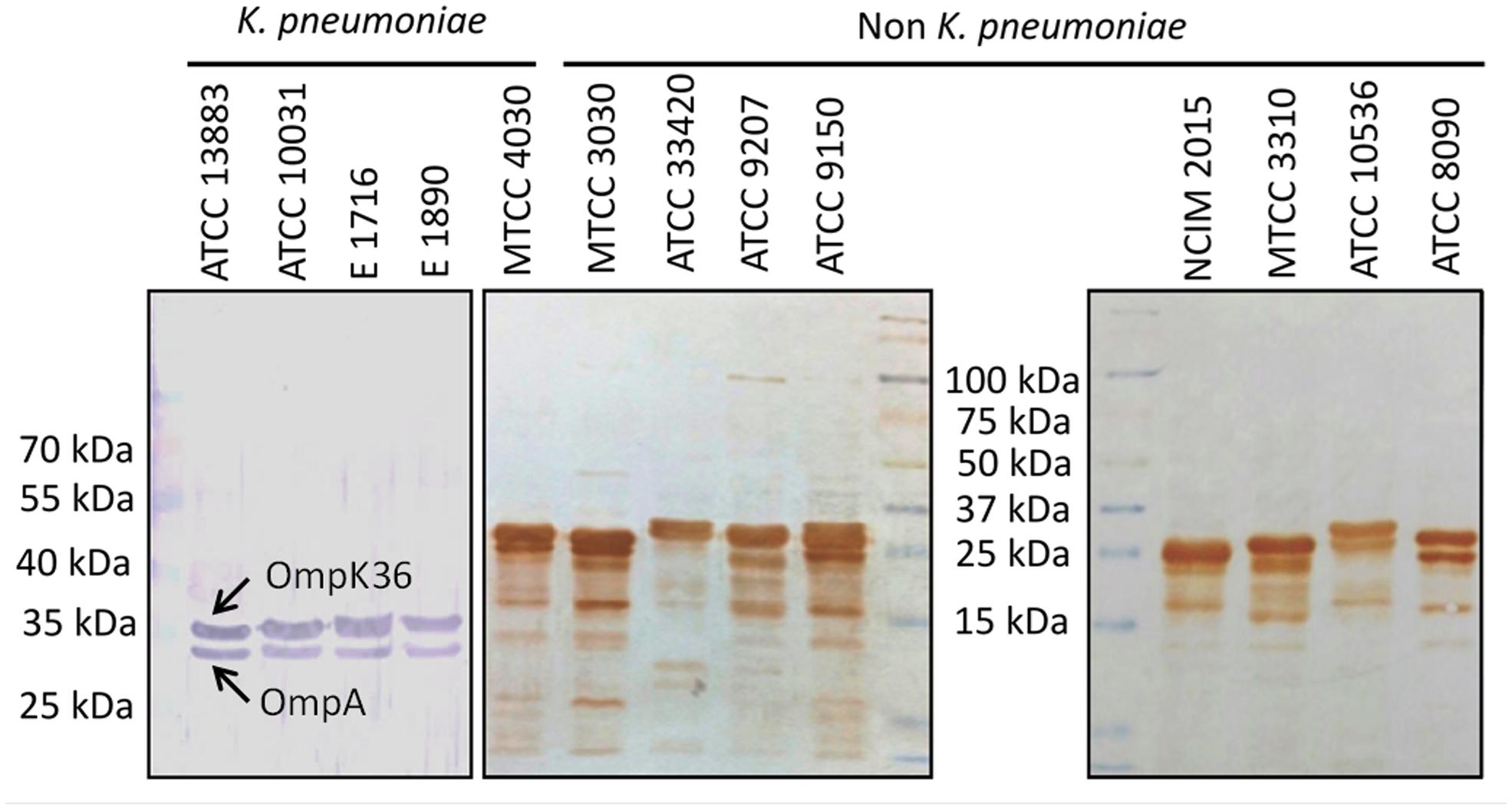
FIGURE 3. Western blot analysis for testing reactivity of anti-r-AK36 antibodies with different Klebsiella pneumoniae and related Enterobacteriaceae members. Ant-r-AK36 serum reacted clearly with native OmpA and OmpK36 proteins of K. pneumoniae strains, while same serum cross-reacted with other members of Enterobacteriaceae at different regions.
In Vitro Proliferation of Lymphocytes from r-AK36-Immunized Mice and Cytokine Analysis
The r-AK36 antigen induced a significant proliferation of splenocytes (whole lymphocytes) from r-AK36-immunized mice upon reinduction by the same antigen at a concentration of 4 μg per million cells. When splenocytes from sham-immunized mice were induced with r-AK36, no significant proliferation was observed (Figure 4A). As a positive control, 10 μg/well of Concanavalin A was used and a significant increase in proliferation was observed in the lymphocyte population, confirming the efficiency of the cell purification process.
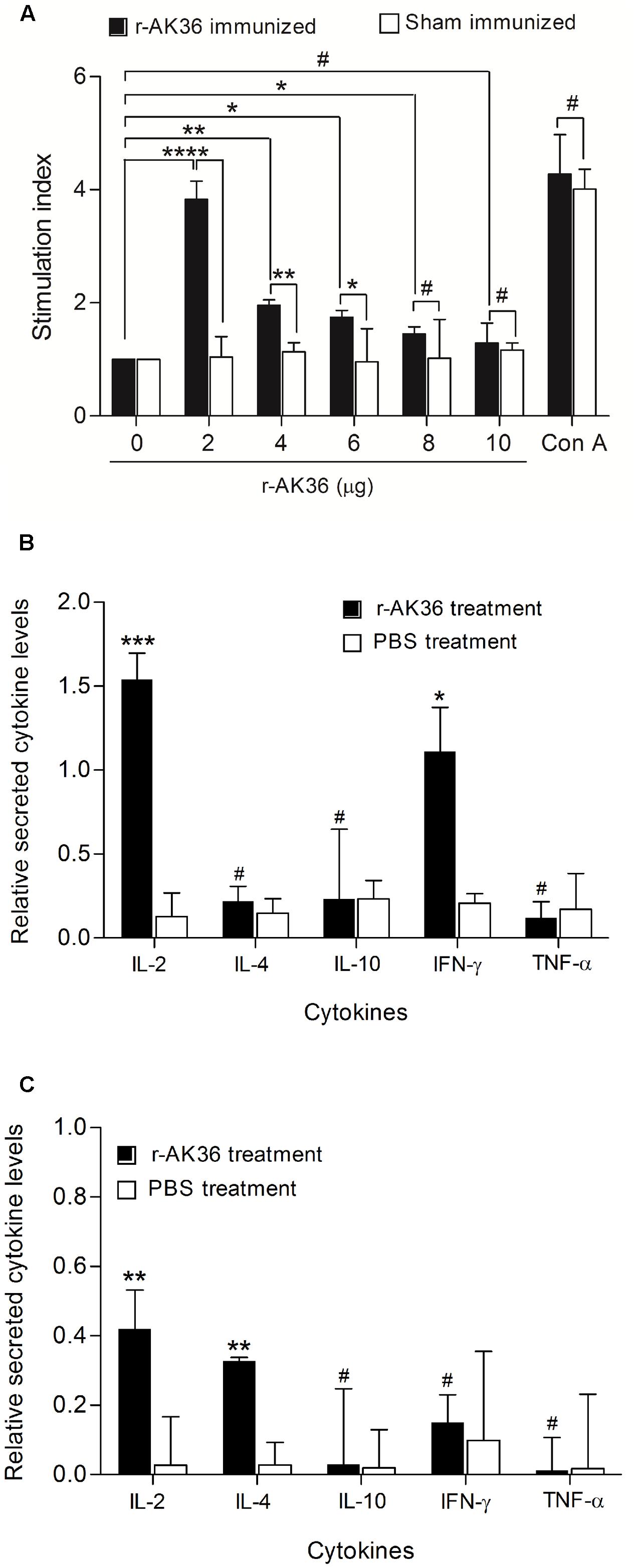
FIGURE 4. Lymphocyte proliferation and cytokine analysis from splenocytes of r-AK36-immunized and sham-immunized mice. (A) Proliferation of lymphocytes isolated from r-AK36-immunized and sham-immunized BALB/c mice and treated with r-AK36 protein at different concentrations for 72 h. (B) Bar graphs showing mean cytokine levels relative to positive controls in conditioned media of splenocytes isolated from r-AK36 immunized BALB/c mice, after stimulation for 3 days with r-AK36 protein. (C) Bar graphs showing mean cytokine levels relative to positive controls in conditioned media of splenocytes isolated from sham-immunized BALB/c mice, after stimulation for 3 days with r-AK36 protein. All results are the average of three independent experiments. Significance (P)-value summary analyzed by univariate ANOVA: ∗P ≤ 0.05, ∗∗P ≤ 0.01, ∗∗∗P ≤ 0.001, ∗∗∗∗P ≤ 0.0001; no significance #.
The Th2polarization of immune response suggested a role of IL-4 lymphokine in mediating IgG1 production. Also, significant proliferative index observed in r-AK36-immunized mice splenocytes prompted us to assess the levels of pro-inflammatory and anti-inflammatory cytokines from conditioned media after lymphocyte proliferation. No increase in the production of IL-4 cytokine was observed from r-AK36-immunized mice splenocytes but a significant rise in the same was observed when sham-immunized mice splenocytes were induced with r-AK36 protein (Figures 4B,C). On the other hand, IL-2, a cytokine that promotes differentiation of T-cells to effector and memory T-cells was significantly produced from r-AK36-immunized mice. IL-2 plays a role in the development of T cell immunologic memory by expanding the number and function of antigen-selected T cell clones. Also, IFN-γ was found to be produced significantly from the r-AK36-immunized mice splenocytes (Figure 4B). IFN-γ was found to be crucial for controlling pulmonary pneumoniae caused by K. pneumoniae. IFN-γ is involved in host immunological defense against K. pneumoniae infection (Yoshida et al., 2001). Splenocytes from sham-immunized mice upon induction by r-AK36 produced significant levels of IL-2 cytokine (Figure 4C).
Challenge Studies
To challenge the immunized mice with K. pneumoniae, firstly we ventured into determining LDs of various strains. The LD (LD100 and LD50) of different K. pneumoniae strains varied significantly (Table 3). In general, these values were lower for strains recovered from clinical sources rather than the standard cultures indicating their higher virulence potential. Groups of 4 mice were used for each dilution during LD determination. K. pneumoniae E-1716 strain isolated from sputum sample from an infected patient had lower LD50 values than K. pneumoniae ATCC 13883 and ATCC 10031 strains. The clinical strain was also different in terms of pathology induced post-challenge and the rapidity of mortality. The clinical strain induced acute respiratory distress, lethargy and rapid death between 24 and 48 h of pathogen administration. On the other hand, mice challenged with standard strains showed reduced activity and dullness in movement and delayed death beyond 48 h of administration.
Groups of six r-AK36-immunized and sham-immunized mice were challenged with ∼106 CFU (3 × LD100) of virulent K. pneumoniae E-1716 strain by intraperitoneal route 7 days after the last booster dose immunization. At least 83% of mice survived beyond the observation period with only one death on day 11 (Figure 5). None of the sham-immunized mice survived beyond 72 h indicating the protective role of r-AK36 subunit vaccine. Gross pathology of lungs of challenged sham-immunized mice showed enlarged, swollen and dull gray regions affecting entire lobes with edema.
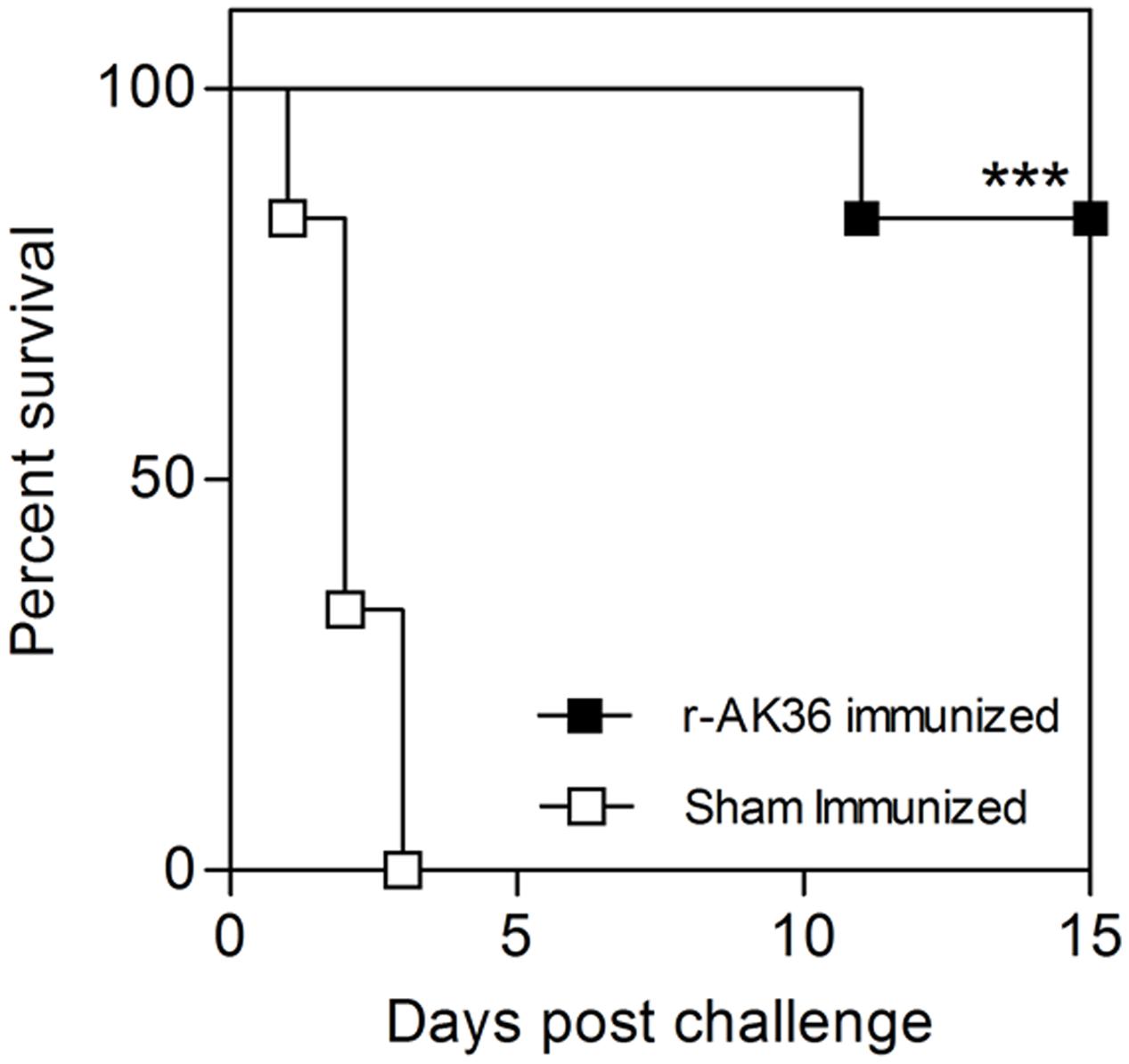
FIGURE 5. Challenge studies in r-AK36-immunized mice. Kaplan–Meier’s survival graph for r-AK36-immunized and sham-immunized mouse challenged with LD of K. pneumoniae E-1716 strain. One animal succumbed to death (83% survival) in active immunized group, but none survived in sham-immunized group. Significance (P)-value summary: ∗∗∗P ≤ 0.001.
Antibacterial Activity of Anti-r-AK36 Antibodies
To assess the antibacterial activity of anti-r-AK36 antibodies, three assays were utilized, viz., MIC, biofilm inhibition and antibacterial protection conferred by passive transfer of antibodies. The MIC values were moderately uniform over a different range of K. pneumoniae strains. The MIC value of r-AK36 antibodies collected 7 days past the last booster dose immunization was determined to be in the range of 60–100 μg/ml. The inhibition of bacteria is also time and concentration dependant as seen from the Figure 6A.
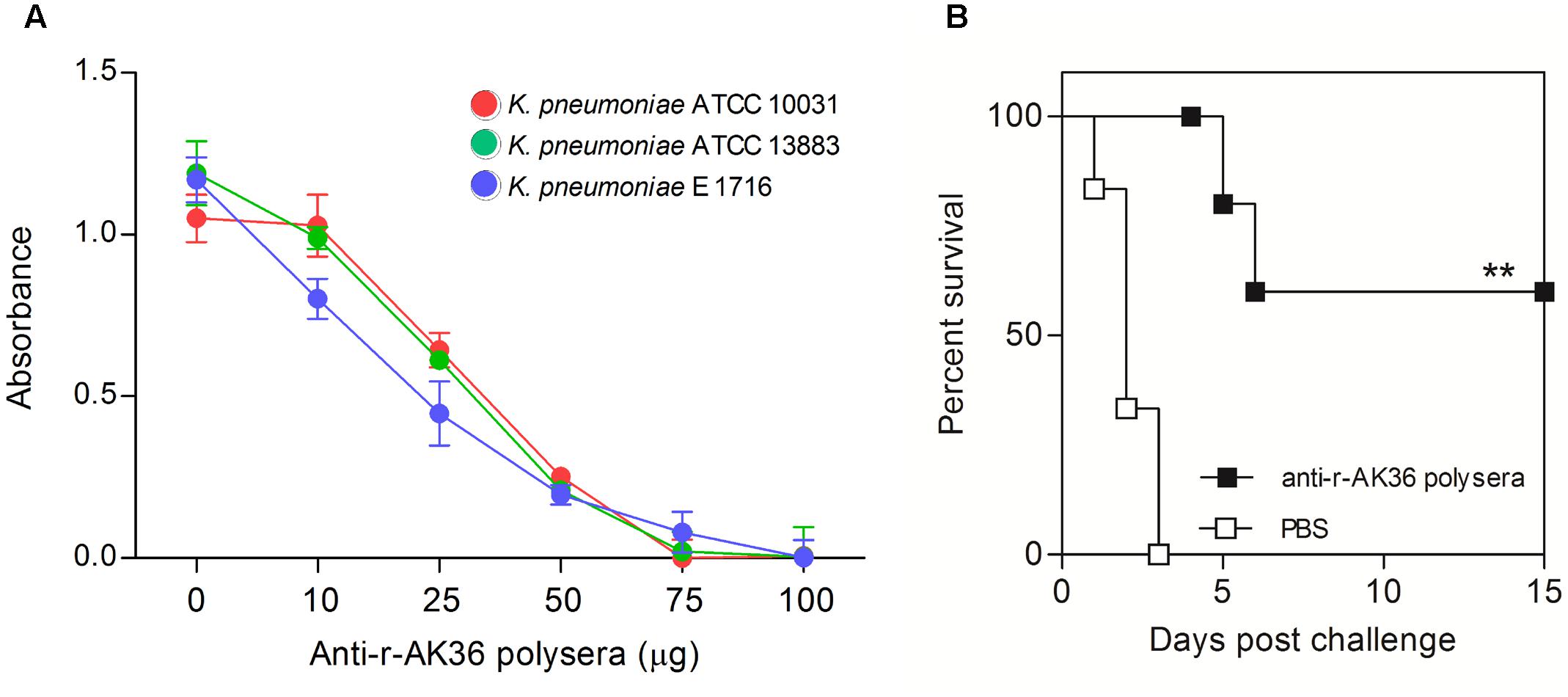
FIGURE 6. Determination of MIC values of anti-r-AK36 serum and challenge studies on passive immunized mice. (A) MIC values were found to be in the range of 25–50 μg/ml of polyclonal antibodies against different strains. (B) Survival graph showing passively immunized mice challenged with r-AK36 antigen. Two mice succumbed to death (67% survival) from passive immunized mice compared all six from sham-immunized group. Significance (P)-value summary: ∗∗P ≤ 0.01.
Antibodies against surface proteins can inhibit biofilm formation by coating the pathogens surface and targeting for destruction. Anti-r-AK36 antibodies at subinhibitory concentrations reduced the ability of different K. pneumoniae strains belonging to various serotypes to form biofilm in vitro. Since the ability of K. pneumoniae to form biofilm varies between strains, a preliminary test was carried out to ascertain the biofilm forming potential. The percent reduction of biofilm varied significantly between different strains. The amount of biofilm formation quantified by crystal violet staining with and without addition of antibody and the percent inhibition of biofilm formation is provided in Table 4.
Passive administration of anti-r-AK36 antibodies protected the mice significantly from mortality upon challenge by 4 × 105 CFU of K. pneumoniae E-1716. Approximately 67% of mice survived beyond the observation period post-challenge (Figure 6B).
Immunological Memory Assessment
Persistence of immunity was evaluated by subjecting r-AK36-immunized mice to lethal challenge with K. pneumoniae E-1716 strain 120 days past final booster. Approximately 50% of the challenged mice survived beyond the observation period in memory group (Figure 7).
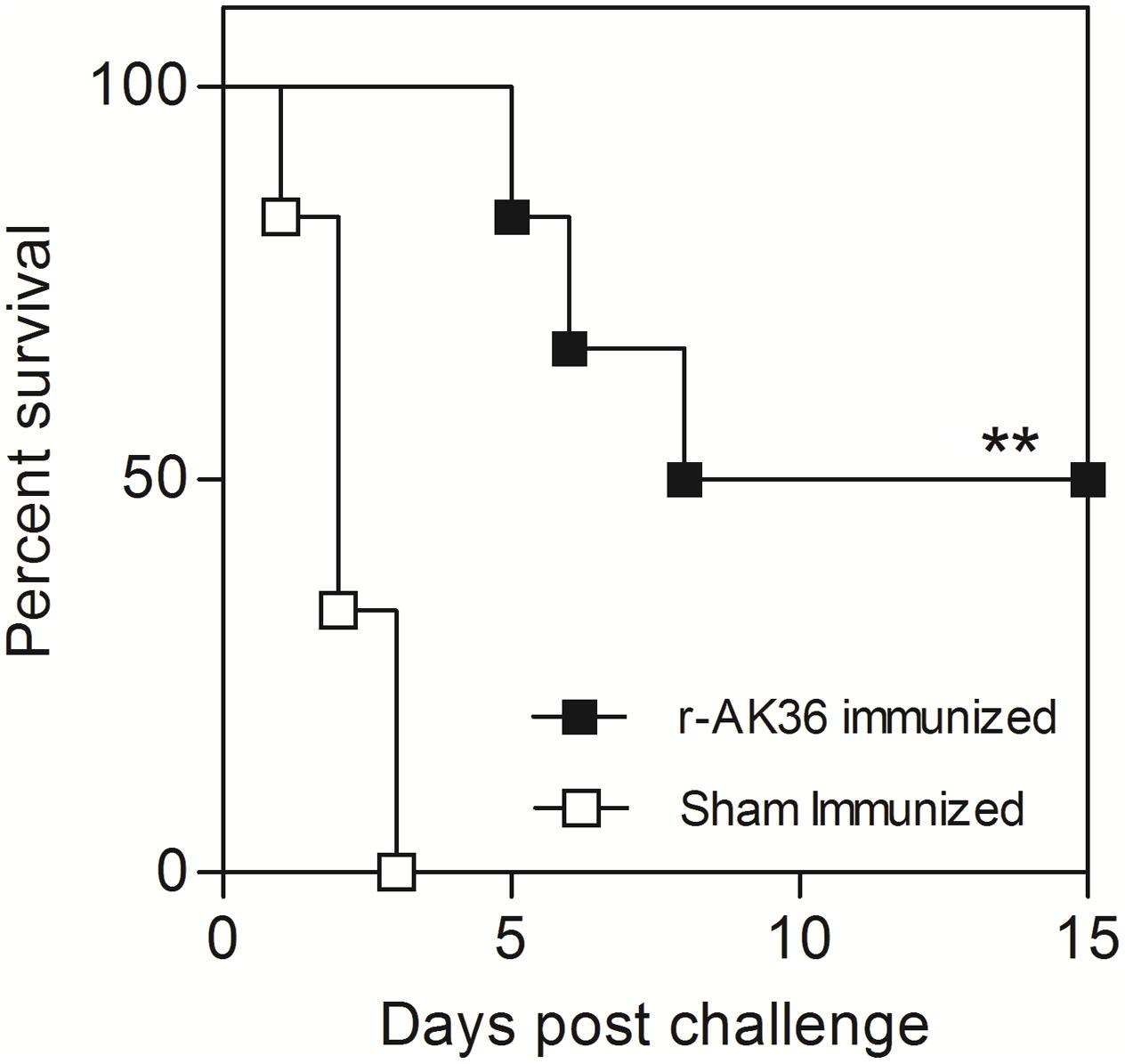
FIGURE 7. Challenge studies in r-AK36-immunized mice 120 days post last immunization. Three mice succumbed to death (50% survival) in active immunized mice while no survivors were observed in sham-immunized group of mice. Significance (P)-value summary: ∗∗P ≤ 0.01.
Viable bacterial counts from various organs such as lungs, spleen, kidney, and liver were evaluated from challenged mice sacrificed after the observation period. Results indicated that lungs of sham-immunized mice showed higher infiltration of K. pneumoniae while lungs of r-AK36-immunized mice showed significantly reduced bacterial counts. The same trend in CFU counts was seen with other organs namely kidney, liver, and spleen. Bacterial infiltration was highest in lungs followed by liver, kidney, and colon (Table 5).
Discussion
Multidrug-resistant K. pneumoniae is responsible for a variety of nosocomial and community acquired infections. Clinical trials addressing MDR infections are focused mainly on antimicrobials, neglecting vaccine development. Efforts onto discovery of non-traditional approaches to combat K. pneumoniae have been hampered by poor knowledge of immunological correlates. On-going investigations on preclinical studies have shown that Th17-mediated immunity can confer broad protection against MDR Gram-negative infections. Approaches to enhance host immunity and for reducing bacterial infection such as adenoviral gene therapy for over production of IL-2, using TNF-α pro-inflammatory cytokine and cyclic di-GMP have shown promise in boosting immunity and reduced bacterial infections. But none of these molecules could reach market due to their high price, being non-specific and no clinical study support. In these difficult times, promising alternatives to antibiotics seems to be pathogen-specific active vaccination and passive antibody therapy (Babb and Pirofski, 2017). Th17 cells are shown to confer serotype independent immunity by recognizing conserved antigens among Enterobacteriaceae members. A recombinant OmpX protein cloned from K. pneumoniae was recognized by Klebsiella immune serum and Th17 cells from Klebsiella immunized mice. Intranasal administration of purified r-OmpX induced strong mucosal Th17 responses necessary for protection against infectious diseases (Chen et al., 2014). Intranasal administration of mouse with heat-killed K. pneumoniae induced IL-17 production in lungs and conferred protection in immunized mice during live K. pneumoniae challenge. It was also reported that oral immunization reduced the bacterial burden during pulmonary infection through recruitment of Th17 cells (Chen et al., 2011a). Among the recently reported immunotherapies, one group reported that monoclonal antibodies generated against the antigen MrkA, a major protein in fimbrial III complex reduced biofilm formation, and bacterial burden following challenge and protection in multiple mouse pneumoniae models (Wang et al., 2016). Protective efficacy of MAb’s 4C5 (IgG1) and 19A10 (IgG3) generated against K1-serotype CPS (K1-CPS) was demonstrated in murine model of sepsis and pulmonary infection. After MAb treatment, reduced dissemination of K. pneumoniae from gut to mesenteric lymph nodes and organs, efficient opsonophagocytosis and clearance of bacteria from liver were noted (Diago-Navarro et al., 2017). Apart from these, a humanized MAb (A1102) specific for the conserved LPS O-antigen was found effective for endotoxin neutralization. Passive administration of A1102 showed efficacy more than that of polymyxin B by three orders of magnitude and afforded significant protection in a galactosamine-sensitized mouse model of endotoxemia or upon challenge with live bacteria (Szijártó et al., 2017). Other passive immunotherapy approach to reduce bacterial counts and delayed onset of infection was tried mainly using the antibodies directed against CPSs (Cryz et al., 1985), inactivated whole cells (Cooper and Rowley, 1982), ribosomal preparations (Riottot et al., 1979), cell surface preparations (Fournier et al., 1981), cytotoxins (Singh and Sharma, 2001) and conjugate vaccine preparations (Chhibber and Bajaj, 1995). However, most of these studies were limited to single capsular serotype or single strain. Due to rampant spread of multidrug resistance and the severe nature of K. pneumoniae infections, highly protective and specific active immunization and passive immunotherapy approaches should be considered for prophylactic or therapeutic approach for treatment of K. pneumoniae infections. Vaccination with OMPs elicits a strong Th17 response against broad-spectrum of K. pneumoniae strains along with recently described metallo-beta-lactamase-I producing strains (Chen et al., 2011b). Targeting pathogen-associated molecular pattern molecules (PAMP’s) such as OMPs (OmpA and OmpK36) and antibodies raised against these molecules would form effective bactericidal defense mechanisms against bacterial clearance from the system. Therefore, purified outer membrane preparations could be ideal targets to provide specific and targeted protection against most K. pneumoniae strains independent of serotype.
In the present study, immunization with recombinant r-AK36 candidate subunit vaccine comprising highly conserved portions of OmpA and OmpK36 proteins of K. pneumoniae conferred protection against intraperitoneal lethal challenge with K. pneumoniae E-1716 strain belonging to virulent K2 serotype in murine model. For this model, BALB/c mice that are traditionally used for vaccine studies were utilized despite Th2-skewed immune response. On the other hand, one can speculate that Th2- polarized immune responses render BALB/c mice ineffective to assess cell-mediated immunity. But previous studies in influenza vaccine studies have shown that primary immunization of vaccine induces Th1 immune responses in BALB/c mouse model. Considering these factors we utilized BALB/c mice in this study for vaccination and challenge studies.
Anti-r-AK36 antibodies reacted strongly with all K. pneumoniae strains along with many other Gram-negative rods such as Salmonella sp., Shigella sp., E. coli, and Proteus sp. indicating a possibility of cross-immunity and cross-protection properties. The antibodies showed significant antimicrobial activity against K. pneumoniae strains. Microbial biofilm represents a major virulence mechanism of pathogenic Klebsiella sps. associated with chronic and recurrent infections aiding in resistance from chemical and environmental agents (Di Domenico et al., 2016). Anti-r-AK36 antibodies also inhibited the biofilm formation in different K. pneumoniae strains belonging to different serotypes. In vitro experiments demonstrated that r-AK36 antibodies possessed strong neutralizing and inhibitory properties. Therefore, we presumed that they could offer protection in a K. pneumoniae infection model in mice. Passive antibody administration in naive mice conferred partial protection against the lethal challenge. Low viable counts of K. pneumoniae were recovered from the vital organs such as lungs, liver, kidney, and spleen of vaccinated animals rather than from the control mice indicating the protective efficacy of the subunit vaccine preparation. Immune response generated by r-AK36 antigen was long lived as seen from the reasonable protection conferred 120 days past last vaccination. The r-AK36 subunit vaccine preparation is more advantageous over reported vaccines due to its protective efficacy, long lived immunity and less or no local anaphylactic reaction at the site of antigen administration. Results obtained from our studies on r-AK36 subunit vaccine were in agreement with some of results obtained by immunizing OmpA and OmpK36 as DNA vaccines in mouse model (Kurupati et al., 2011). Additionally, immunization of K. pneumoniae-derived extracellular vesicles (EVs) comprising OMPs also produced similar results as in our studies (Lee et al., 2015). They report that three vaccinations with EV protected against bacterial challenge.
Conclusion
The data presented in this study identified a novel multicomponent r-AK36 subunit vaccine molecule effective in combating K. pneumoniae infectious diseases. This molecule induced both humoral and cell-mediated memory immune responses. This molecule might offer broad-spectrum protection against a majority of K. pneumoniae serotypes due to the universal presence of these antigens in all strains irrespective of serotype and their highly conserved nature.
Author Contributions
LB participated in conceiving, designing, performing the experiments and writing of manuscript. SU helped in writing the manuscript, statistical analysis, revision and proofreading of manuscript. MS helped in work design, planning, providing reagents and other logistics and manuscript evaluation. PR helped in performing experiments, analysis and interpretation of results, writing and critically reviewing the manuscript.
Funding
This work was supported by DFRL institution funds sanctioned by Defence Research and Development Organization (DRDO), India.
Conflict of Interest Statement
The authors declare that the research was conducted in the absence of any commercial or financial relationships that could be construed as a potential conflict of interest.
Acknowledgment
PR is a SERB-National Post-Doctoral Fellow (NPDF) funded by Department of Science and Technology, India.
Abbreviations
CPS, capsular polysaccharide; LD, lethal dose; LPS, lipopolysaccharide; MIC, minimum inhibitory concentration; OMP, outer membrane protein.
References
Ahmad, T. A., El-Sayed, L. H., Haroun, M., Hussein, A. A., and El Ashry el, S. H. (2012). Development of immunization trials against Klebsiella pneumoniae. Vaccine 30, 2411–2420. doi: 10.1016/j.vaccine.2011.11.027
Babb, R., and Pirofski, L. A. (2017). Help is on the way: monoclonal antibody therapy for multi-drug resistant bacteria. Virulence doi: 10.1080/21505594.2017.1306620 [Epub ahead of print].
Breecher, M. M. (2007). IDSA: Achilles Tendon Rupture after Use of Antibiotics. San Diego, CA: Doctor’s Guide.
Center for Disease Control [CDC] (1974). National Nosocomial Infections Study Report. Atlanta: Center for Disease Control.
Chen, K., Fisher, C., Zheng, M., Weaver, C., and Kolls, J. (2011a). Immunization of heat-killed Klebsiella pneumoniae promotes strong mucosal Th17 responses and protects mice from live infection (59.4). J. Immunol. 186(Suppl. 1), 59.4.
Chen, K., McAleer, J. P., Lin, Y., Paterson, D. L., Zheng, M., Alcorn, J. F., et al. (2011b). Th17 cells mediate clade-specific, serotype-independent mucosal immunity. Immunity 35, 997–1009. doi: 10.1016/j.immuni.2011.10.018
Chen, K., Goodwin, M., McAleer, J., Nguyen, N., Way, E., and Kolls, J. (2014). Recombinant outer membrane protein: a potential candidate for Th17 based vaccine against Klebsiella pneumoniae. (VAC7P. 967). J. Immunol. 192 (Suppl. 1), 141.12.
Chhibber, S., and Bajaj, J. (1995). Polysaccharide-iron—regulated cell surface protein conjugate vaccine: its role in protection against Klebsiella pneumoniae-induced lobar pneumonia. Vaccine 13, 179–184. doi: 10.1016/0264-410X(95)93133-T
Cooper, J. M., and Rowley, D. (1982). Resistance to Klebsiella pneumoniae and the importance of two bacterial antigens. Aust. J. Exp. Biol. Med. Sci. 60, 629–641. doi: 10.1038/icb.1982.65
Coovadia, Y. M., Johnson, A. P., Bhana, R. H., Hutchinson, G. R., George, R. C., and Hafferjee, I. E. (1992). Multiresistant Klebsiella pneumoniae in a neonatal nursery: the importance of maintenance of infection control policies and procedures in the prevention of outbreaks. J. Hosp. Infect. 22, 197–205. doi: 10.1016/0195-6701(92)90044-M
Cryz, S. J. (1983). Progress in immunization against Klebsiella infections. Eur. J. Clin. Microbiol. Infect. Dis. 2, 523–528. doi: 10.1007/BF02016559
Cryz, S. J., Fürer, E., and Germanier, R. (1985). Safety and immunogenicity of Klebsiella pneumoniae K1 capsular polysaccharide vaccine in humans. J. Infect. Dis. 151, 665–671. doi: 10.1093/infdis/151.4.665
Di Domenico, E. G., Toma, L., Provot, C., Ascenzioni, F., Sperduti, I., Prignano, G., et al. (2016). Development of an in vitro assay, based on the biofilm ring test®, for rapid profiling of biofilm-growing bacteria. Front. Microbiol. 7:1429. doi: 10.3389/fmicb.2016.01429
Diago-Navarro, E., Calatayud-Baselga, I., Sun, D., Khairallah, C., Mann, I., Ulacia-Hernando, A., et al. (2017). Antibody-based immunotherapy to treat and prevent infection with hypervirulent Klebsiella pneumoniae. Clin. Vaccine Immunol. 24:e00456-16. doi: 10.1128/CVI.00456-16
Elemam, A., Rahimian, J., and Mandell, W. (2009). Infection with pan resistant Klebsiella pneumoniae: a report of 2 cases and a brief review of the literature. Clin. Infect. Dis. 49, 271–274. doi: 10.1086/600042
Fournier, J. M., Jolivet-Reynaud, C., Riottot, M. M., and Jouin, H. (1981). Murine immunoprotective activity of Klebsiella pneumoniae cell surface preparations: comparative study with ribosomal preparations. Infect. Immun. 32, 420–426.
Huang, Y., Li, J., Gu, D., Fang, Y., Chan, E. W., Chen, S., et al. (2015). Rapid detection of K1 hypervirulent Klebsiella pneumoniae by MALDI-TOF MS. Front. Microbiol. 6:1435. doi: 10.3389/fmicb.2015.01435
Jain, R. R., Mehta, M. R., Bannalikar, A. R., and Menon, M. D. (2015). Alginate microparticles loaded with lipopolysaccharide subunit antigen for mucosal vaccination against Klebsiella pneumoniae. Biologicals 43, 195–201. doi: 10.1016/j.biologicals.2015.02.001
Jeannin, P., Magistrelli, G., Goetsch, L., Haeuw, J. F., Thieblemont, N., Bonnefoy, J. Y., et al. (2002). Outer membrane protein A (OmpA): a new pathogen-associated molecular pattern that interacts with antigen presenting cells-impact on vaccine strategies. Vaccine 20(Suppl. 4), A23–A27. doi: 10.1016/S0264-410X(02)00383-3
Kim, Y. K., Pai, H., Lee, H. J., Park, S. E., Choi, E. H., Kim, J., et al. (2002). Bloodstream infections by extended-spectrum β-lactamase-producing Escherichia coli and Klebsiella pneumoniae in children: epidemiology and clinical outcome. Antimicrob. Agents Chemother. 46, 1481–1491. doi: 10.1128/AAC.46.5.1491.2020
Kurupati, P., Ramachandran, N. P., and Poh, C. L. (2011). Protective efficacy of DNA vaccines encoding outer membrane protein A and OmpK36 of Klebsiella pneumoniae in mice. Clin. Vaccine Immunol. 18, 82–88. doi: 10.1128/CVI.00275-10
Lee, W. H., Choi, H. I., Hong, S. W., Kim, K. S., Gho, Y. S., and Jeon, S. G. (2015). Vaccination with Klebsiella pneumoniae-derived extracellular vesicles protects against bacteria-induced lethality via both humoral and cellular immunity. Exp. Mol. Med. 47, e183. doi: 10.1038/emm.2015.59
Mena, A., Plasencia, V., García, L., Hidalgo, O., Ayestarán, J. I., Alberti, S., et al. (2006). Characterization of a large outbreak by CTX-M-1-producing Klebsiella pneumoniae and mechanisms leading to in vivo carbapenem resistance development. J. Clin. Microbiol. 44, 2831–2837. doi: 10.1128/JCM.00418-06
Merino, S., Camprubí, S., Albertí, S., Benedí, V. J., and Tomás, J. M. (1992). Mechanisms of Klebsiella pneumoniae resistance to complement-mediated killing. Infect. Immun. 60, 2529–2535.
Palusiak, A. (2015). The antigens contributing to the serological cross-reactions of Proteus antisera with Klebsiella representatives. Mol. Immunol. 64, 228–234. doi: 10.1016/j.molimm.2014.11.016
Pichavant, M., Delneste, Y., Jeannin, P., Fourneau, C., Brichet, A., Tonnel, A. B., et al. (2003). Outer membrane protein A from Klebsiella pneumoniae activates bronchial epithelial cells: implication in neutrophil recruitment. J. Immunol. 171, 6697–6705. doi: 10.4049/jimmunol.171.12.6697
Podschun, R., and Ullmann, U. (1998). Klebsiella spp. as nosocomial pathogens: epidemiology, taxonomy, typing methods, and pathogenicity factors. Clin. Microb. Rev. 11, 589–603.
Reddy, P. N., Paul, S., Sripathy, M. H., and Batra, H. V. (2015). Evaluation of recombinant SEA-TSST fusion toxoid for protection against superantigen induced toxicity in mouse model. Toxicon 103, 106–113. doi: 10.1016/j.toxicon.2015.06.008
Rice, L. B., Willey, S. H., Papanicolaou, G. A., Medeiros, A. A., Eliopoulos, G. M., Moellering, R. C., et al. (1990). Outbreak of ceftazidime resistance caused by extended-spectrum beta-lactamases at a Massachusetts chronic-care facility. Antimicrob. Agents Chemother. 34, 2193–2199. doi: 10.1128/AAC.34.11.2193
Riottot, M., Fournier, J. M., and Pillot, J. (1979). Capsular serotypic specificity of the protection conferred on mice by Klebsiella pneumoniae ribosomal preparations. Infect. Immun. 24, 476–482.
Simoons-Smit, A. M., Verweij-van Vught, A. M. J. J., and MacLaren, D. M. (1986). The role of K antigens as virulence factors in Klebsiella. J. Med. Microbiol. 21, 133–137. doi: 10.1099/00222615-21-2-133
Singh, B. R., and Sharma, V. D. (2001). Potential of Klebsiella pneumoniae cytotoxin toxoid as vaccine against klebsiellosis in rabbits and mice. Vaccine 19, 4505–4510. doi: 10.1016/S0264-410X(01)00187-6
Skurnik, D., Lasocki, S., Bremont, S., Muller-Serieys, C., Kitzis, M. D., Courvalin, P., et al. (2010). Development of ertapenem resistance in a patient with mediastinitis caused by Klebsiella pneumoniae producing an extended-spectrum β-lactamase. J. Med. Microbiol. 59, 115–119. doi: 10.1099/jmm.0.012468-0
Sun, D., Accavitti, M. A., and Bryers, J. D. (2005). Inhibition of biofilm formation by monoclonal antibodies against Staphylococcus epidermidis RP62A accumulation associated protein. Clin. Diagn. Lab. Immunol. 12, 93–100. doi: 10.1016/j.micinf.2013.10.019
Sun, W. S. W., Syu, W. J., Ho, W. L., Lin, C. N., Tsai, S. F., and Wang, S. H. (2014). SitA contributes to the virulence of Klebsiella pneumoniae in a mouse infection model. Microb. Infect. 16, 161–170. doi: 10.1016/j.micinf.2013.10.019
Szijártó, V., Guachalla, L. M., Hartl, K., Varga, C., Badarau, A., Mirkina, I., et al. (2017). Endotoxin neutralization by an O-antigen specific monoclonal antibody: a potential novel therapeutic approach against Klebsiella pneumoniae ST258. Virulence doi: 10.1080/21505594.2017.1279778 [Epub ahead of print].
Vieira, A. T., Rocha, V. M., Tavares, L., Garcia, C. C., Teixeira, M. M., Oliveira, S. C., et al. (2016). Control of Klebsiella pneumoniae pulmonary infection and immunomodulation by oral treatment with the commensal probiotic Bifidobacterium longum 51A. Microb. Infect. 18, 180–189. doi: 10.1016/j.micinf.2015.10.008
Wang, L., Shen, D., Wu, H., and Ma, Y. (2017). Resistance of hypervirulent Klebsiella pneumoniae to both intracellular and extracellular killing of neutrophils. PLOS ONE 12:e0173638. doi: 10.1371/journal.pone.0173638
Wang, Q., Chang, C. S., Pennini, M., Pelletier, M., Rajan, S., Zha, J., et al. (2016). Target-agnostic identification of functional monoclonal antibodies against Klebsiella pneumoniae multimeric MrkA fimbrial subunit. J. Infect. Dis. 213, 1800–1808. doi: 10.1093/infdis/jiw021
Yadav, V., Sharma, S., Harjai, K., Mohan, H., and Chhibber, S. (2005). Lipopolysaccharide-mediated protection against Klebsiella pneumoniae-induced lobar pneumonia: intranasal vs. intramuscular route of immunization. Folia Microbiol. 50, 83–86. doi: 10.1007/BF02931298
Yoshida, K., Matsumoto, T., Tateda, K., Uchida, K., Tsujimoto, S., Iwakura, Y., et al. (2001). Protection against pulmonary infection with Klebsiella pneumoniae in mice by interferon-γ through activation of phagocytic cells and stimulation of production of other cytokines. J. Med. Microbiol. 50, 959–964. doi: 10.1099/0022-1317-50-11-959
Keywords: Klebsiella pneumoniae, subunit vaccine, OmpA, OmpK36, animal challenge
Citation: Babu L, Uppalapati SR, Sripathy MH and Reddy PN (2017) Evaluation of Recombinant Multi-Epitope Outer Membrane Protein-Based Klebsiella pneumoniae Subunit Vaccine in Mouse Model. Front. Microbiol. 8:1805. doi: 10.3389/fmicb.2017.01805
Received: 09 February 2017; Accepted: 05 September 2017;
Published: 20 September 2017.
Edited by:
José Roberto Mineo, Federal University of Uberlandia, BrazilReviewed by:
Masaaki Miyazawa, Kindai University, JapanVenkataramana Mudili, DRDO-Bharathiar University Centre for Life Sciences, India
Chandra Nayak, University of Mysore, India
Copyright © 2017 Babu, Uppalapati, Sripathy and Reddy. This is an open-access article distributed under the terms of the Creative Commons Attribution License (CC BY). The use, distribution or reproduction in other forums is permitted, provided the original author(s) or licensor are credited and that the original publication in this journal is cited, in accordance with accepted academic practice. No use, distribution or reproduction is permitted which does not comply with these terms.
*Correspondence: Prakash N. Reddy, cHJha2FzaHJlZGR5LjM2OUBnbWFpbC5jb20=