- 1National Reference Laboratory of Antibiotic Resistances and Healthcare Associated Infections, Department of Infectious Diseases, National Institute of Health Doutor Ricardo Jorge, Lisbon, Portugal
- 2Centre for the Studies of Animal Science, Institute of Agrarian and Agri-Food Sciences and Technologies, University of Porto, Oporto, Portugal
- 3Innovation and Technology Unit, Human Genetics Department, National Institute of Health Doutor Ricardo Jorge, Lisbon, Portugal
- 4Laboratory of Microbiology, Hospital Professor Doutor Fernando Fonseca, Amadora, Portugal
A new QepA4 variant was detected in an O86:H28 ST156-fimH38 Escherichia coli, showing a multidrug-resistance phenotype. PAβN inhibition of qepA4-harboring transconjugant resulted in increase of nalidixic acid accumulation. The qepA4 and catA1 genes were clustered in a 26.0-kp contig matching an IncF-type plasmid, and containing a Tn21-type transposon with multiple mobile genetic elements. This QepA variant is worrisome because these determinants might facilitate the selection of higher-level resistance mutants, playing a role in the development of resistance, and/or confer higher-level resistance to fluoroquinolones in association with chromosomal mutations.
Introduction
Quinolones are broad-spectrum antibiotics that have been used in medical practice for the treatment of severe or resistant infections (Kim and Hooper, 2014). This class of antibiotics is fully synthetic and used widely in both human and veterinary medicine.
Bacterial resistance to fluoroquinolones has emerged quickly and has conventionally been attributed to chromosomally encoded mechanisms that allow the alteration of quinolone targets (QRDR, quinolone resistance-determining regions): DNA gyrase and topoisomerase IV (Jacoby et al., 2014). However, the discovery of plasmid-borne determinants has increased the genetic background on the mechanisms of quinolone resistance, such as the plasmid-mediated fluoroquinolone resistance (PMQR) determinants.
The qepA gene is a PMQR gene encoding a 14-transmembrane-segment efflux pump of the major facilitator superfamily (MFS). Unlike other MFS efflux pumps that typically export various antimicrobial agents and dyes, QepA shows substrate specificity directed to the fluoroquinolones ciprofloxacin and norfloxacin (Jacoby et al., 2014; Rodríguez-Martínez et al., 2016).
QepA1 was described in Escherichia coli clinical isolates from Japan and Belgium in 2007 (Périchon et al., 2007; Yamane et al., 2007). Since then two new variants have been described: QepA2, in a CTX-M-15-producing E. coli isolated from urine and blood samples of a patient suffering from pyelonephritis, in France, in 2007 (Cattoir et al., 2008); and, more recently, QepA3, in an Enterobacter aerogenes isolated in 2011 from wound pus of a patient admitted at a Chinese hospital (Wang et al., 2015). These QepA2 and QepA3 variants differ from QepA1 by two (Ala99Gly and Val134Ile) and five (Ala235Glu, Pro274Leu, Trp318Cys, Met372Lys, and Ala445Thr) amino acids, respectively (Table 1).
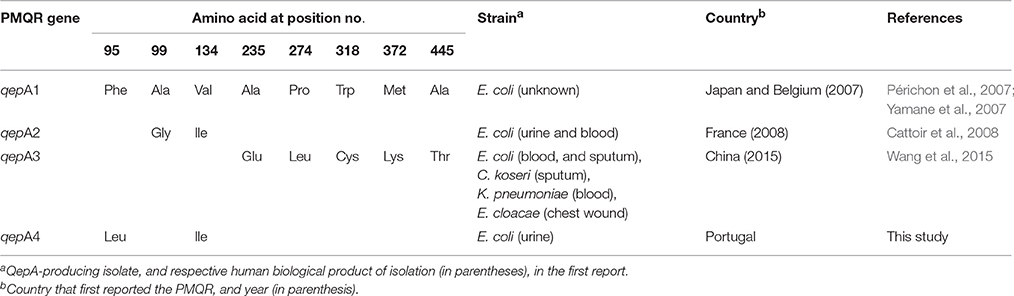
Table 1. Comparison of amino acid substitutions, and epidemiology of first reports of clinical QepA-producing strains.
Antibiotic resistance genes are frequently associated to mobile genetic elements (MGE), such as insertion sequences (ISs), phages, transposons and plasmids, which enhance their ability to efficiently spread among different bacterial species (Stokes and Gillings, 2011). The occurrence of MGE harboring multiple antibiotic resistance genes is also frequent, and enables the development of bacterial multidrug-resistance (MDR), which may be responsible for therapeutic failures (Poirel et al., 2012; Kim and Hooper, 2014). Indeed, PMQRs are commonly described in isolates co-producing plasmid-mediated β-lactamases (PMAβ) and extended-spectrum β-lactamases (ESBL) (Jacoby et al., 2014).
In this study, we have identified and characterized the fourth variant of the QepA determinant-QepA4, which is responsible for the increased levels of resistance to clinically important quinolones. Furthermore, this is also, at our knowledge, the first description of the co-production of QepA and both the PMAβ CMY-2 and the penicillinase TEM-1. The study highlights the need of surveillance of this resistance mechanism and reinforces a more careful use of quinolones.
Methods
Antibiotic Susceptibility Testing and Molecular Characterization
Minimum inhibitory concentrations (MICs) of E. coli INSRA6015 isolated from a Portuguese healthcare facility were determined by microdilution and E-test methods according to European Committee on Antimicrobial Susceptibility Testing (EUCAST) guidelines (Table 2). Interpretation of the results was done according to the EUCAST epidemiological cut-off values (http://mic.eucast.org/Eucast2/). Detection and identification of β-lactamase- and PMQR-encoding genes, as well as the analysis of the quinolone-resistance-determining region (QRDR) was performed as previously described (Jones-Dias et al., 2013).
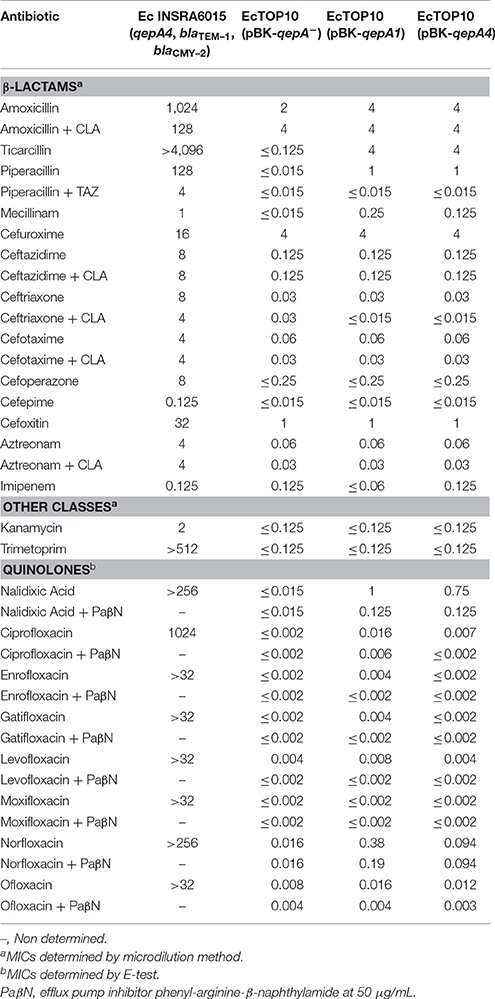
Table 2. MICs (mg/L) of antibiotics for the E. coli (Ec) strains: clinical INSRA6015, EcTOP10 (pBK-qepA1), and EcTOP10 (pBK-qepA4) transformants, and the recipient EcTOP10 (pBK-qepA−).
Gene Transfer Experiments
In order to characterize the QepA4 determinant, transformants were obtained by amplifying qepA4 with primers qepA-F (5′–CGTTAAAGCATTCTTGTCCCGG–3′) and qepA-R (5′–ATGTCCGCCACGCTCCACG–3′), cloning it in the pBK-CMV phagemid vector (Stratagene), and transforming it into TOP10 OneShot chemically competent E. coli cells (Invitrogen). Susceptibility of transformants to an assortment of fluoroquinolones was tested alone and in the presence of 50 μg/mL of the efflux pump inhibitor phenyl-arginine-β-naphthylamide (PAβN) (Sigma-Aldrich) (Périchon et al., 2007), as mentioned above (Table 2). EcTOP10 (pBK-qepA1) strain was used as control.
Genomic Characterization of QepA4-Producing E. coli
The QepA4-producing E. coli was characterized by whole-genome sequencing (WGS), as previously described (Manageiro et al., 2015). Briefly, genomic DNA was extracted using DNeasy Blood and Tissue Kit (Qiagen) and quantified using Qubit 1.0 Fluorometer (Invitrogen). The Nextera XT DNA Sample Preparation Kit (Illumina) was used to prepare sequencing libraries from 1 ng of genomic DNA according to the manufacturer's instructions. WGS was performed using 150 bp paired-end reads on a MiSeq (Illumina). Sequence reads were trimmed and filtered according to quality criteria, and de novo assembled into contigs by means of CLC Genomics Workbench 10.0 (QIAGEN). PathogenFinder 1.1, ResFinder 2.1, VirulenceFinder 1.4, SerotypeFinder 1.1, MLST 1.8, pMLST 1.4, and ISSaga were used to estimate the pathogenicity determinants, acquired antibiotic resistance genes, virulence factors, serotype, MLST, plasmid MLST, and insertion sequence regions, respectively in the genomes of PMQR-producing E. coli (Manageiro et al., 2015).
Results and Discussion
INSRA6015 showed non-susceptibility to fluoroquinolones (E-test method) and to third-generation cephalosporins, aztreonam, and cefoxitin (microdilution method); no synergy with clavulanate was detected. The E. coli isolate remained susceptible to piperacillin/tazobactam and imipenem (Table 2).
The β-lactamase-encoding genes blaTEM–1 and blaCMY–2, and the PMQR-encoding gene qepA were detected by molecular methods. Sequence analysis of the QepA variant showed amino acid substitutions Phe95Leu and Val134Ile, justifying the new name QepA4 (Table 1). Sequencing of QRDR revealed the presence of three amino acid substitutions in the correspondent proteins: Ser83Leu and Asp87Asn in GyrA subunit of DNA-gyrase, and Glu84Lys in the ParC subunit of topoisomerase-IV. These results are consistent with the detected high levels of resistance to fluoroquinolones (MIC >32 mg/L) (Table 2).
EcTOP10 (pBK-qepA4) strain revealed susceptibility to all fluoroquinolones and remaining antibiotics classes tested. In fact, susceptibility levels were similar to the ones showed by EcTOP10 (pBK-qepA1) strain, with exception of non-susceptibility to norfloxacin (0.094 vs. 0.38 mg/L, respectively). However, the qepA4-encoding transformant showed higher MIC values to nalidixic acid (≥6-fold), ciprofloxacin (≥2-fold) and norfloxacin (3-fold) than the qepA-negative strain. Moreover, the levels of susceptibility to nalidixic acid upon QepA with and without inhibition by PAβN were 3-fold higher for qepA4- and qepA1-encoding transformants (Table 2). These results show variability in the level of qepA expression, as previously discussed (Rodríguez-Martínez et al., 2016).
WGS allowed the characterization of the qepA4 genetic background. The analysis yielded 91 contigs (from 203 to 358,909 bp), with a minimum 64-fold coverage. The draft genome contained a total assembly length of 4,770,076 bp, with a mean coverage of about 160-fold; the GC content was 50.9%. All de novo contigs were searched against the GenBank complete plasmids database using Megablast, with 14/91 contigs mapping against plasmid sequences therein deposited. Full details of these contigs are available in Table 3.
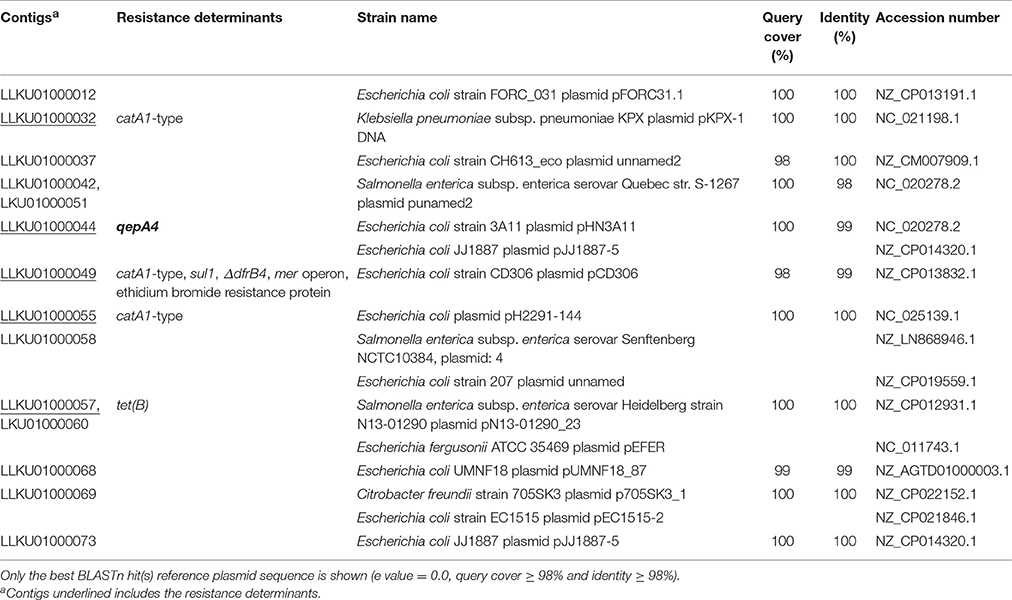
Table 3. Assembled contigs representing plasmids identified after BLASTn searched against the NCBI plasmid database.
The qepA4 gene was found in a 25,957 bp length contig, which enclosed a composite mercury resistance Tn21-like transposon (Figure 1), showing 99% identity with previously described IncFII NR1 low-copy-number natural plasmid (Williams et al., 2006), over 80% length coverage. The region located between urf2 and tnpM (Figure 1A) was interrupted by a complete In227 (Figure 1B), which included IS1353 inserted into IS1326, thus interrupting tniB gene (Figure 1C). The variable region harbored a ΔdfrB4 and a qepA4 gene downstream of an ISCR3 element, which was flanked upstream by In211. Downstream of the Tn21-like transposition region, two acetyltransferase genes (catA1, ybjA) were detected. Unlike qepA1 or qepA3, this qepA4 gene was not genetically associated to the rmtB gene encoding a plasmid-mediated ribosomal methylase, which is consistent with susceptibility to aminoglycosides showed by INSRA6015. The remaining sequence of the contig matched with previously described qepA-harboring IncFII plasmids, such as pJJ1887-5 and pHN3A11, reported in the USA and China, respectively (Chen et al., 2014; Johnson et al., 2016), which correlates with PCR-based replicon results obtained (Carattoli et al., 2005). Horizontal transfer of the qepA4 gene was not achieved either by bacterial conjugation or through the direct transformation of plasmid DNA (data not shown), suggesting the presence of a non-transferable plasmid.
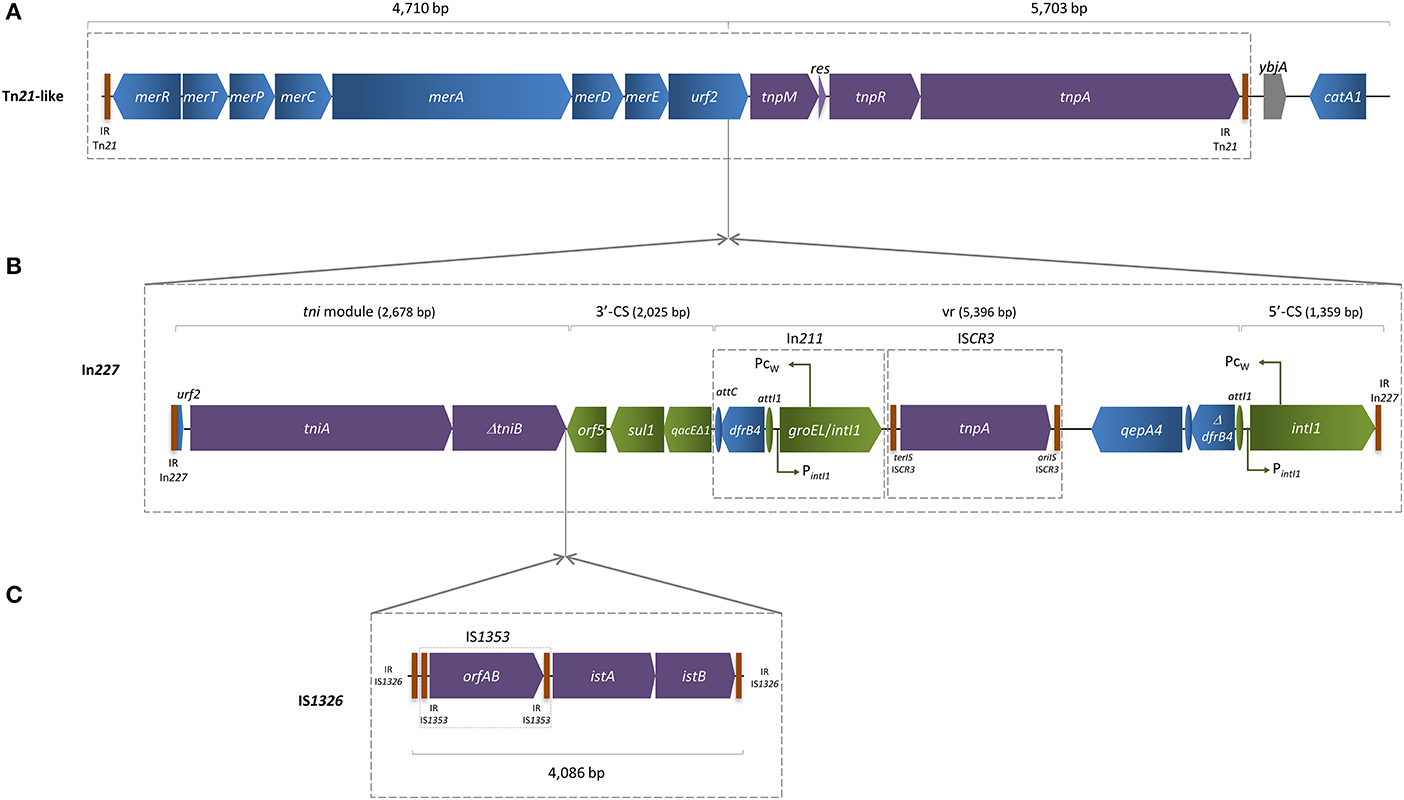
Figure 1. Schematic representation of qepA4-harboring contig assembled using LLKU01000032, LLKU01000034, LLKU01000049, and LLKU01000073, with mean coverage of 134-fold and 59.0% of GC content. (A) The Tn21-like transposon included the transposition (tnp) region [comprising the transposase (tnpA), the resolvase (tnpR), and the putative transposition regulator (tnpM)]; the resolution site (res); and the mercury resistance (mer) operon [with the regulatory genes merR and merD, the structural genes merT, merP, merC, and merA, and two unknown reading frames: urf1 (also called merE) and urf2, downstream of merD]; (B) The urf2 gene was interrupted by a complete In227 integron, which variable region (vr) harbored a Δ dfrB4 and a qepA4 gene downstream an ISCR3 element, flanked upstream by an In211 integron; (C) Two ISs (IS1353 inserted into IS1326) interrupted the tniB (transposition of the integron) gene. IR, flanking inverted repeats; oriIS and terIS, origin and terminus of ISCR elements, respectively; vertical bars indicate IR of transposons (Tn), integrons (In) and insertion sequences (IS). Arrows indicate the direction of transcription of the various genes. Truncated genes are indicated by a Δ symbol.
Further analysis revealed the presence of genes conferring resistance to β-lactams (blaTEM–1, blaCMY–2), tetracycline [tet(B)], phenicol (catA1-type), sulphonamides (sul1) and trimethoprim (dfrB4-type). CMY-2- and TEM-1-encoding genes (LLKU01000025 and LLKU01000032 contigs, respectively), contrarily to the other genes found, were not detected within contigs that mapped against plasmid sequences (with ≥98% of query coverage and ≥98% of identity) (Table 3). According with the methodology used, the strain does not have other typeable plasmids.
Overall, 53 putative ORFs related to ISs were found within the INSRA6015 genome: 21 complete, 28 partial, and 4 uncategorized (including IS1, IS1380, IS21, IS3ssgrIS3, ISAs1, and Tn3 with 100% of similarity with those described in the ISsaga database. Three virulence factors (iss, lpfA, gap) were identified. Moreover, this O86:H28 E. coli isolate displayed a prediction of 93.4% for being a human pathogen, based on the probability scores assigned by PathogenFinder.
In addition, QepA4-producing E. coli presented a set of genetic features crucial to support their own successful dissemination (Figure 1), such as multiple antibiotic resistance genes carried by MGE, virulence factors and numerous other pathogenicity factors. These features enlarge bacteria ability for transboundary dissemination among bacteria from different environments (Stokes and Gillings, 2011; Caniça et al., 2015).
The QepA4-producing INSRA6015 E. coli isolate belonged to the ST156 lineage (UCC scheme) and ST119 (Pasteur scheme), and showed the fimH38 allele upon fimH typing. This ST has been reported associated with different antibiotic-resistance genes, namely PMAβ and ESBL, NDM carbapenemases, and 16S rRNA methylases, both in clinical and colonizing human-associated E. coli isolates collected in different countries, and in water samples from Bangladesh (Corvec et al., 2010; Mushtaq et al., 2011; Pan et al., 2013; Sáez-López et al., 2014; Rashid et al., 2015). Indeed, the qepA4 variant identified in this clinical isolate matched a partial QepA-type sequence (Accession Number LK934678) detected in a TEM-1 and CTX-M-15-producing E. coli strain recovered in a raw wastewater sample in Portugal, but from a different ST (ST443) (Varela et al., 2015).
The PMQR determinants confer low-level quinolone resistance that, in some cases, does not exceed the clinical breakpoint for susceptibility as demonstrated in this study and by others (Jacoby et al., 2014). However, its presence may facilitate higher-level resistance under selective pressure from antimicrobial agents at therapeutic levels, mostly due to chromosomal mechanisms, which makes infection by pathogens containing PMQR harder to treat (Poirel et al., 2012). Fortunately, the qepA4 gene detected in this study was not in a genetic linkage to rmtB gene as has been demonstrated; this suggests that are no potential for selection of the QepA4 determinant by the use of aminoglycosides.
Conclusion
In conclusion, qepA4 was here first identified in an rmtB-negative clinical isolate, and genetically characterized within a composite mercury resistance Tn21-like transposon, harboring other different mobile genetic elements. This report represents an important finding about a plasmid-mediated resistance mechanism, which contributes with other quinolone resistance mechanisms to increase therapeutic failures, and understanding of this resistance in different reservoirs (Poirel et al., 2012; Yan et al., 2017).
Data Access
This draft genome has been deposited at DDBJ/EMBL/GenBank under the accession LLKU00000000. The version described in this paper is version LLKU01000000. The qepA4 nucleotide sequence from this study was submitted to the GenBank Database with accession number KX686116.
Author Contributions
VM designed the study, performed molecular experiments, bioinformatics analysis, analyzed the data and wrote the manuscript. DF, DJ, and EF performed microbiological and molecular experiments, and analyzed the data. DS and LV performed Illumina genome sequencing experiments. LS acquired laboratory data. MC designed the study, wrote and reviewed the manuscript. All authors read and approved the final manuscript.
Funding
VM was supported by FCT fellowship (grant SFRH/BPD/77486/2011), financed by the European Social Funds (COMPETE-FEDER) and national funds of the Portuguese Ministry of Education and Science (POPH-QREN). The authors thank Fundação para a Ciência e a Tecnologia (FCT) for project grant PEst-OE/AGR/UI0211/2011-2014, Strategic Project UI211-2011-2014.
Conflict of Interest Statement
The authors declare that the research was conducted in the absence of any commercial or financial relationships that could be construed as a potential conflict of interest.
References
Caniça, M., Manageiro, V., Jones-Dias, D., Clemente, L., Gomes-Neves, E., Poeta, P., et al. (2015). Current perspectives on the dynamics of antibiotic resistance in different reservoirs. Res. Microbiol. 166, 594–600. doi: 10.1016/j.resmic.2015.07.009
Carattoli, A., Bertini, A., Villa, L., Falbo, V., Hopkins, K. L., and Threlfall, E. J. (2005). Identification of plasmids by PCR-based replicon typing. J. Microbiol. Methods 63, 219–228. doi: 10.1016/j.mimet.2005.03.018
Cattoir, V., Poirel, L., and Nordmann, P. (2008). Plasmid-mediated quinolone resistance pump QepA2 in an Escherichia coli isolate from France. Antimicrob. Agents Chemother. 52, 3801–3804. doi: 10.1128/AAC.00638-08
Chen, X., He, L., Li, Y., Zeng, Z., Deng, Y., Liu, Y., et al. (2014). Complete sequence of a F2:A-:B- plasmid pHN3A11 carrying rmtB and qepA, and its dissemination in China. Vet. Microbiol. 174, 267–271. doi: 10.1016/j.vetmic.2014.08.023
Corvec, S., Crémet, L., Leprince, C., Dauvergne, S., Reynaud, A., Lepelletier, D., et al. (2010). Epidemiology of Escherichia coli clinical isolates producing AmpC plasmidic beta-lactamase during a 5-year period in a French teaching Hospital. Diagn. Microbiol. Infect. Dis. 67, 277–281. doi: 10.1016/j.diagmicrobio.2010.02.007
Jacoby, G. A., Strahilevitz, J., and Hooper, D. C. (2014). Plasmid-mediated quinolone resistance. Microbiol. Spectr. 2:PLAS-006-2013, doi: 10.1128/microbiolspec.PLAS-0006-2013
Johnson, T. J., Aziz, M., Liu, C. M., Sokurenko, E., Kisiela, D. I., Paul, S., et al. (2016). Complete genome sequence of a CTX-M-15-producing Escherichia coli strain from the H30Rx subclone of sequence type 131 from a patient with recurrent urinary tract infections, closely related to a lethal urosepsis isolate from the patient's sister. Genome Announc. 4:e00334–e00316. doi: 10.1128/genomeA.00334-16
Jones-Dias, D., Manageiro, V., Francisco, A. P., Martins, A. P., Domingues, G., Louro, D., et al. (2013). Assessing the molecular basis of transferable quinolone resistance in Escherichia coli and Salmonella spp. from food-producing animals and food products. Vet. Microbiol. 167, 523–531. doi: 10.1016/j.vetmic.2013.08.010
Kim, E. S., and Hooper, D. C. (2014). Clinical importance and epidemiology of quinolone resistance. Infect. Chemother. 46, 226–238. doi: 10.3947/ic.2014.46.4.226
Manageiro, V., Sampaio, D. A., Pereira, P., Rodrigues, P., Vieira, L., Palos, C., et al. (2015). Draft genome sequence of the first NDM-1-producing Providencia stuartii strain isolated in Portugal. Genome Announc. 3, e01077–e01015. doi: 10.1128/genomeA.01077-15
Mushtaq, S., Irfan, S., Sarma, J. B., Doumith, M., Pike, R., Pitout, J., et al. (2011). Phylogenetic diversity of Escherichia coli strains producing NDM-type carbapenemases. J. Antimicrob. Chemother. 66, 2002–2005. doi: 10.1093/jac/dkr226
Pan, Y. S., Liu, J. H., Hu, H., Zhao, J. F., Yuan, L., Wu, H., et al. (2013). Novel arrangement of the blaCTX−M−55gene in an Escherichia coli isolate coproducing 16S rRNA methylase. J. Basic Microbiol. 53, 928–933. doi: 10.1002/jobm.201200318
Périchon, B., Courvalin, P., and Galimand, M. (2007). Transferable resistance to aminoglycosides by methylation of G1405 in 16S rRNA and to hydrophilic fluoroquinolones by QepA-mediated efflux in Escherichia coli. Antimicrob. Agents Chemother. 51, 2464–2469. doi: 10.1128/AAC.00143-07
Poirel, L., Cattoir, V., and Nordmann, P. (2012). Plasmid-mediated quinolone resistance; interactions between human, animal, and environmental ecologies. Front. Microbiol. 3:24. doi: 10.3389/fmicb.2012.00024
Rashid, M., Rakib, M. M., and Hasan, B. (2015). Antimicrobial-resistant and ESBL-producing Escherichia coli in different ecological niches in Bangladesh. Infect. Ecol. Epidemiol. 5:26712. doi: 10.3402/iee.v5.26712
Rodríguez-Martínez, J. M., Machuca, J., Cano, M. E., Calvo, J., Martínez-Martínez, L., and Pascual, A. (2016). Plasmid-mediated quinolone resistance: two decades on. Drug Resist. Updat. 29, 13–29. doi: 10.1016/j.drup.2016.09.001
Sáez-López, E., Guiral, E., López, Y., Montero, I., Bosch, J., Vila, J., et al. (2014). Characterization of CTX-M-14 and CTX-M-15 producing Escherichia coli strains causing neonatal sepsis. Microb. Drug Resist. 20, 281–284. doi: 10.1089/mdr.2013.0190
Stokes, H. W., and Gillings, M. R. (2011). Gene flow, mobile genetic elements and the recruitment of antibiotic resistance genes into Gram negative pathogens. FEMS Microbiol. Rev. 35, 790–819. doi: 10.1111/j.1574-6976.2011.00273.x
Varela, A. R., Macedo, G. N., Nunes, O. C., and Manaia, C. M. (2015). Genetic characterization of fluoroquinolone resistant Escherichia coli from urban streams and municipal and hospital effluents. FEMS Microbiol. Ecol. 91. doi: 10.1093/femsec/fiv015
Wang, D., Huang, X., Chen, J., Mou, Y., Li, H., and Yang, L. (2015). Characterization of genetic structures of the qepA3 gene in clinical isolates of Enterobacteriaceae. Front. Microbiol. 6:1147. doi: 10.3389/fmicb.2015.01147
Williams, L. E., Detter, C., Barry, K., Lapidus, A., and Summers, A. O. (2006). Facile recovery of individual high-molecular-weight, low-copy-number natural plasmids for genomic sequencing. Appl. Environ. Microbiol. 72, 4899–4906. doi: 10.1128/AEM.00354-06
Yamane, K., Wachino, J., Suzuki, S., Kimura, K., Shibata, N., Kato, H., et al. (2007). New plasmid-mediated fluoroquinolone efflux pump, QepA, found in an Escherichia coli clinical isolate. Antimicrob. Agents Chemother. 51, 3354–3360. doi: 10.1128/AAC.00339-07
Keywords: QepA4, quinolone resistance, genetic characterization, PaβN, WGS
Citation: Manageiro V, Félix D, Jones-Dias D, Sampaio DA, Vieira L, Sancho L, Ferreira E and Caniça M (2017) Genetic Background and Expression of the New qepA4 Gene Variant Recovered in Clinical TEM-1- and CMY-2-Producing Escherichia coli. Front. Microbiol. 8:1899. doi: 10.3389/fmicb.2017.01899
Received: 26 July 2017; Accepted: 15 September 2017;
Published: 09 October 2017.
Edited by:
Miklos Fuzi, Semmelweis University, HungaryReviewed by:
Séamus Fanning, University College Dublin, IrelandFelipe C. Cabello, New York Medical College, United States
Copyright © 2017 Manageiro, Félix, Jones-Dias, Sampaio, Vieira, Sancho, Ferreira and Caniça. This is an open-access article distributed under the terms of the Creative Commons Attribution License (CC BY). The use, distribution or reproduction in other forums is permitted, provided the original author(s) or licensor are credited and that the original publication in this journal is cited, in accordance with accepted academic practice. No use, distribution or reproduction is permitted which does not comply with these terms.
*Correspondence: Manuela Caniça, bWFudWVsYS5jYW5pY2FAaW5zYS5taW4tc2F1ZGUucHQ=