- Section for Microbiology, Department of Bioscience, Aarhus University, Aarhus, Denmark
Hydrogen may accumulate to micromolar concentrations in cyanobacterial mat communities from various environments, but the governing factors for this accumulation are poorly described. We used newly developed sensors allowing for simultaneous measurement of H2S and H2 or O2 and H2 within the same point to elucidate the interactions between oxygen, sulfate reducing bacteria, and H2 producing microbes. After onset of darkness and subsequent change from oxic to anoxic conditions within the uppermost ∼1 mm of the mat, H2 accumulated to concentrations of up to 40 μmol L-1 in the formerly oxic layer, but with high variability among sites and sampling dates. The immediate onset of H2 production after darkening points to fermentation as the main H2 producing process in this mat. The measured profiles indicate that a gradual disappearance of the H2 peak was mainly due to the activity of sulfate reducing bacteria that invaded the formerly oxic surface layer from below, or persisted in an inactive state in the oxic mat during illumination. The absence of significant H2 consumption in the formerly oxic mat during the first ∼30 min after onset of anoxic conditions indicated absence of active sulfate reducers in this layer during the oxic period. Addition of the methanogenesis inhibitor BES led to increase in H2, indicating that methanogens contributed to the consumption of H2. Both H2 formation and consumption seemed unaffected by the presence/absence of H2S.
Introduction
Photosynthetic microbial mats are highly diverse communities both physiologically and phylogenetically. The mats are stratified communities where different functional groups exploit the microniches created by steep gradients in light and chemistry. They form on top of solid substrates where the photosynthetic primary producers fuel heterotrophic and chemolithoautotrophic bacteria. Environments with little or no disruption from benthic fauna are a prerequisite for formation of microbial mats, and they are thus best developed where fauna is absent such as in hypersaline and high-temperature environments (Stal, 1995; Fenchel, 1998; Bolhuis et al., 2014).
Photosynthetic microbial mats are autotrophic and thus reduce CO2 to organic matter while oxidizing an electron donor. Under certain conditions they may, however, also produce H2. Hydrogen production by photosynthetic bacteria has been reported numerous times (Gest et al., 1962; Benemann and Weare, 1974; Warthmann et al., 1992; Moezelaar and Stal, 1997; Hoehler et al., 2001; Burow et al., 2012; Lee et al., 2014; Nielsen et al., 2015), and such microbial H2 production has recently gained considerable interest due to its potential use in renewable energy supply (Lee et al., 2010).
Photosynthetic bacteria can produce H2 by three different pathways: as a by-product during nitrogen fixation (Benemann and Weare, 1974; Warthmann et al., 1992), by hydrogenases during anaerobic fermentation (Moezelaar and Stal, 1997; Burow et al., 2012), and by photoproduction through the action of hydrogenases when there is an excess of energy (Warthmann et al., 1992; Appel et al., 2000). Hydrogen is a favorable electron donor for aerobic and anaerobic respiration as well as for anoxygenic photosynthesis (Drutschmann and Klemme, 1985; Hoehler et al., 1998). Even in anoxic environments the H2 concentration is normally kept at few nanomolar levels due to the tight coupling between production and consumption (Lovley et al., 1982; Hoehler et al., 1998; Stams and Plugge, 2009). A substantial H2 accumulation has, however, been observed in the cyanobacterial layer of both hypersaline (Burow et al., 2012), marine (Nielsen et al., 2015) and hot spring (Anderson et al., 1987; Revsbech et al., 2016) microbial mats during dark incubations following periods with illumination. The H2 production in photosynthetic mats has been suspected to be due to nitrogenase activity, but the H2 production in hypersaline mats seems to be mainly due to fermentation, where the photosynthetic bacteria ferment the photosynthate fixed during the day as a nighttime metabolism (Hoehler et al., 2001; Burow et al., 2012; Lee et al., 2014). The simultaneous production of other fermentation products supports this hypothesis (Burow et al., 2012; Lee et al., 2014). Analysis of the gene expression of bidirectional hydrogenases pointed to cyanobacteria of the genus Microcoleus as the main hydrogenogens in these mats (Burow et al., 2012; Lee et al., 2014). Sulfate is a dominant terminal electron acceptor in marine sediment (Jørgensen, 1982) and the sulfate reducing bacteria Desulfobacterales have been identified as the dominant hydrogenotrophs in hypersaline microbial mats (Burow et al., 2014). In hypersaline and marine sediments sulfate reduction typically outcompetes methanogenesis due to high sulfate concentrations. Some methane production has been observed in saline photosynthetic mats (Hoehler et al., 2001), but methanogenesis appeared to be an unimportant H2 sink as compared to sulfate reduction in a thoroughly investigated hypersaline mat (Burow et al., 2012, 2014). It is generally assumed that the upper limit for sulfate reduction and hence sulfide production is governed by the depth of O2 penetration, although some reports suggest that sulfate reduction may occur under oxic conditions (Canfield and Des Marais, 1991; Fründ and Cohen, 1992). During the day when oxygenic photosynthesis is producing O2, sulfate reduction will thus be confined to the deeper anoxic part of the sediment. After darkening when O2 production is replaced with consumption, sulfide moves closer to the surface and may actually reach the very surface (Revsbech et al., 1983). Sulfate reducing bacteria may contain enzymes protecting them against reactive oxygen species, which reflect their frequent presence close to the oxic zone (Fournier et al., 2003; Dolla et al., 2006), and massive presence of especially Desulfonema has been observed in oxic mat layers sampled during illumination (Minz et al., 1999). Occurrence of nighttime sulfate reduction in layers that were oxic during the day may thus be due to either migration of sulfate reducing bacteria into the formerly oxic zone or resumption of activity by resident sulfate reducers. Tolerance to reactive oxygen species has also been found in methanogens (Horne and Lessner, 2013; Jasso-Chávez et al., 2015).
In this study the H2 dynamics in a photosynthetic microbial mat were described by applying combined H2 – H2S and H2 – O2 microsensors, yielding fine scale measurements and aligned profiles in this system. The alignment of profiles measured during light-dark cycles allowed for accurate positioning of the H2 producing layer relative to the photosynthetically active layer that was oxic during illumination, and also to the occurrence of free sulfide. The application of these sensors was essential, as previous data seem to show that the main H2 producing zone in cyanobacterial mats is at the lower boundary or even below the photosynthetic zone (e.g., Figure 7 in Nielsen et al., 2015), and we hypothesized that this was due to erroneous alignment of profiles measured with different sensors.
Materials and Methods
Sampling Site
Samples were collected from Løgstør Bredning in Limfjorden close to the village of Aggersund (57°00′04.4″N 9°17′18.6″E). The place is subject to changing water levels where the sediment may be either covered by water or exposed to air for hours or days. Due to these harsh conditions the mats can develop without being grazed by larger invertebrates. Sampling was done by coring with Plexiglas cylinders having inner diameters of 3.6 cm and a length of 8 cm. Sampling was done in November 2014, January 2015, June 2015, September 2016, and December 2016. During each sampling campaign ∼10 samples were collected and selected cores with well-developed cyanobacterial communities were profiled.
Samples were kept moist and stored at 5°C under a halogen lamp (100 μmol photons m-2 s-1) with a timer mimicking a diurnal cycle until experiments could be conducted in an aquarium kept at 20°C. Experiments were conducted between 1 and 17 days after sampling.
Sensors
A combined H2 and H2S microsensor was applied for measuring H2 and H2S profiles in the mat. This type of sensor has been developed very recently (Maegaard et al., 2017, submitted) and allowed for perfectly aligned profiles of H2 and H2S, as both species enter the sensor through the same membrane-covered opening at the 20–50 μm wide tip. The physical design is identical to the STOX oxygen sensor (Revsbech et al., 2009), with a Clark-type H2 microsensor (obtained from Unisense A/S) housed in an outer sensor capillary with a tip silicone membrane. A gold anode placed in front of the H2 microsensor and bathed in a ferricyanide containing electrolyte (Jeroschewski et al., 1996) serves to quantify the H2S entering the sensor assembly and also prevents H2S interference on the H2 signal. The H2 part of the sensors applied had a sensitivity of 1.2–7.7 pA μmol-1 L-1 and the H2S part of the sensors had a sensitivity of 16.3–32.5 pA μmol-1 L-1. The calibration of the sulfide part of the sensors was done in 0.1 M HCl where O2 had been removed by flushing with N2. A 20 mmol L-1 Na2S solution was prepared from a washed crystal of Na2Sx9H2O from which fresh calibration standards of 0, 50, 100, and 200 μmol L-1 H2S were made. No pH profiles were recorded in the mat, and the total pool of dissolved sulfide thus cannot be calculated. The pKa of H2S is about 7 (varying somewhat with salinity (Millero et al., 1988) and the total pool of dissolved sulfide was thus substantially higher than the measured H2S concentration. The calibration of the H2 part of the sensor was performed in water from the sampling site to which demineralized water saturated with H2 was added to final concentrations of 0, 50, 100, and 200 μmol L-1. The concentration of the H2 saturated stock solution was calculated according to Wiesenburg and Guinasso (1979) to contain 805 μmol H2 L-1. In addition to the combined H2 and H2S sensor, an O2 microsensor (Revsbech, 1989) with a tip diameter of 10 μm was applied. The O2 sensor was calibrated assuming O2 saturation (252 μmol L-1 at 20°C and 20‰ salinity) in the aerated aquarium. The second calibration point for the linear calibration curve was the zero reading obtained in deep anoxic sediment. Further, a combined H2 and O2 sensor was constructed according to the same principle as the combined H2 and H2S sensor, but where the H2S anode and ferricyanide electrolyte were substituted with a gold O2 cathode and bicarbonate electrolyte (Revsbech, 1989). The sensitivity was 5.7–21.8 pA μmol-1 L-1 for O2 and 0.7–1.0 pA μmol-1 L-1 for H2. The large current for O2 is associated with a large stirring sensitivity, amounting to an about 10% signal difference between stirred and stagnant water. Oxygen data obtained with such sensors should thus not be used for calculation of fluxes and rates near mat-water interfaces. The H2 and O2 calibrations were performed as described above. Solubility of O2 in water and diffusion coefficients for O2, H2 and H2S in water were obtained from the tables at http://www.unisense.com/files/PDF/Diverse/Seawater & Gases table.pdf.
Profiling
The mat was placed in an aerated aquarium filled with seawater from the sampling site (20‰ salinity) with the temperature kept at 20°C. The water depth above the sample was ∼2 cm. The depth position of the sensor tips was controlled by a motorized micromanipulator connected to a PC with the data acquisition software Sensor Trace Pro (Unisense A/S). The program also served to collect the A/D converted signals from the sensors. The signal currents from all types of sensors were measured by custom-made picoammeters connected to an 8-channel A/D converter (Unisense A/S). The sensor signals were recorded for every 50 μm depth interval. The recording of a H2/H2S profile took about 5 min and recording of a H2/O2 profile took about 15 min. The times for recording profiles mentioned in the following are for the start of a profile.
Experiments
The surface of the sediment cores was illuminated at an irradiance of 600 μmol photons m-2 s-1 in the 400–700 nm range using a halogen lamp equipped with a heat filter. This light intensity corresponds to about a third of maximum sunlight intensity at noon during mid-summer. For all H2 accumulation experiments the samples received 3 h of light before onset of darkness. Profiles of O2, H2 and H2S were recorded both during illumination and during darkness. O2 profiles were only recorded at steady-state light and dark conditions, whereas combined H2/H2S profiles and H2/O2 profiles were measured after 3 h of illumination and at about 20 min intervals throughout the dark period following illumination. For clarity of illustration only some of the measured profiles are shown on figures.
Inhibition of Sulfate Reduction and Methanogenesis in Intact Sediment Core
Sulfate reduction was inhibited by addition of 10 mmol L-1 Na2MoO4 during the illuminated period. The molybdate was allowed to diffuse into the mat for 2 h before illumination ceased. When the H2 profile during the dark period was approaching a steady-state in the presence of molybdate, 20 mmol L-1 2-bromoethanesulfonic acid (BES) was added to inhibit methanogenesis (Scholten et al., 2000). Profiling ceased when a new steady state was approached.
Hydrogen Dynamics in Isolated Cyanobacterial Layer
To prevent migration of organisms from deeper anoxic layers to the photosynthetic zone of the mat during dark conditions, a thin slice of the mat was placed on top of seawater agar, and the mat was fastened with multiple glass needles. The about 1-mm thick mat slice was cut off the sediment core with a razor blade after 3 h of illumination. Profiling of this thin mat slice during illumination and darkness was performed as described above for the intact sediment core. The importance of sulfate reduction and methanogenesis in the separated mat was evaluated by adding sodium molybdate and BES as described above for the intact sediment core. In addition H2 profiling was performed on a mat slice where molybdate was added when the H2 concentration during dark incubation had decreased to about half of the maximum value.
Consumption of External Hydrogen during Illumination and Darkness
Hydrogen was added to the water phase by bubbling with a gas stream controlled by a gas mixer (Brooks Instruments 0260 with Thermal Mass Flow Controllers and Meter GF 40). Profiles were recorded during light and dark conditions until steady-state profiles were observed.
Calculations and Modeling
Profiles of reaction rates of O2 and H2 were obtained by use of the diffusion-reaction program “Profile” (Berg et al., 1998). The porosity in this type of mat can be assumed to be 0.75 according to Glud et al. (1995). We estimated the effective diffusion coefficient in the mat (Ds) asuming Ds= φ D (Berg et al., 1998), where φ is porosity and D the diffusion coefficient in water. For the values of D for O2 and H2 we used the Unisense table values of 2.0 and 3.9.10-5 cm-2 s-1, respectively. The boundary conditions were a H2 or O2 flux of zero in the water column and below the consumption zone. Calculation of activity distributions from H2 and O2 profiles were only possible when the profiles were relatively stable, and we were thus unable to do calculations on data obtained just after a light-dark shift. The presence of iron minerals oxidizing and precipitating sulfide and the lack of pH data prevented modeling of H2S production profiles. It should be stressed that the recording of a H2/H2S profile required about 5 min, which further stresses the need of near-steady state of modeled profiles. Fick’s first law of diffusion (Crank, 1975) was applied for calculation of water – mat diffusional exchange of O2, assuming that the steepest gradient was in a loose surface layer with diffusion coefficient for O2 close to the one in water.
Results
Visual Impressions
The sampled mats were 1–2 mm thick rigid, dark-green structures formed on top of sandy sediment. Microscopic examination showed that Microcoleus sp. were dominating the oxygenic photosynthetic community, but other filamentous cyanobacteria and diatoms were present as well. The underlying sediment was black indicating sulfidic conditions. During illumination O2 bubbles were formed on top of the mat. A white layer of colorless sulfur bacteria was observed at the surface after several hours of dark incubation, indicating that the O2/H2S interface was at the very surface of the mat (Jørgensen and Revsbech, 1983).
Oxygen Profiles during Light and Dark Conditions
Oxygen concentrations peaked at ∼0.1 mm during illumination and O2 penetrated to a depth of ∼1 mm. Modeling of the profile shown in Figure 1 indicated a net O2 production zone from 0 to 0.2 mm and a net consumption zone from 0.2 to 1.1 mm. The net O2 production was calculated to be 0.59 nmol cm-2 s-1 and the net O2 consumption below 0.2 mm depth was 0.17 nmol cm-2 s-1 (Figure 1). Oxygen profiles were measured at 3 additional positions of the mat, and all showed O2 penetrations of 1.0–1.1 mm and comparable O2 productions in the zones with net O2 production. The exact figure for net O2 production is, however, very dependent on the diffusivity at the mat/water interface. If we assume that the steepest O2 gradient is in the overlying water, which will be true with a diffuse water-sediment interface, then the estimate of O2 production in the zone with net production (Figure 1) increases to 0.79 nmol cm-2s-1, and the average from all 4 profiles was 0.86 ± 0.16 (SD) nmol cm-2 s-1.
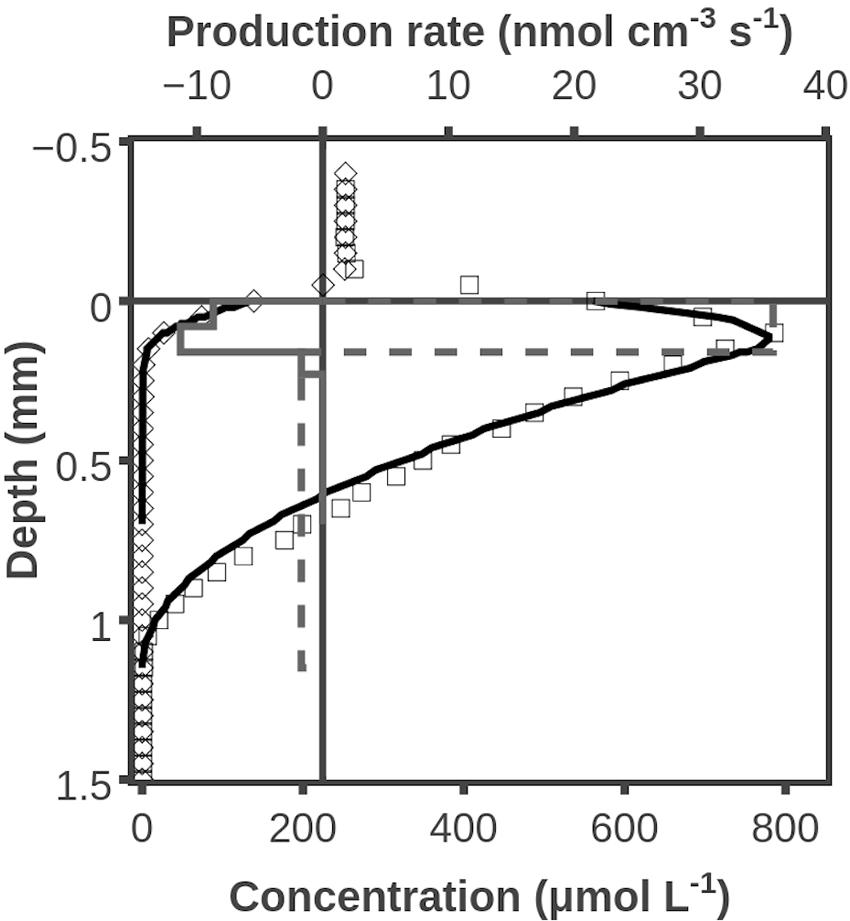
FIGURE 1. Example of O2 concentration profile and O2 production rates under dark- and light conditions. Shown are measured O2 concentrations during darkness (◇) and illumination (□). Also shown are modeled O2 concentration profiles (―), assuming transformation rates (during darkness: fully drawn box diagram; during illumination: with broken line) suggested by curve fitting. Data obtained by use of O2 microsensor.
After illumination ceased a new steady state O2 profile was approached within 5 min, and O2 penetrated to a depth of only ∼0.2 mm. The modeled O2 consumption in the zone from 0 to 0.2 mm was 0.17 nmol cm-2 s-1 (Figure 1). With a similar argumentation about the steepest gradient found in a layer with a diffusivity close to the one in water, then the estimate of total diffusive uptake increases to 0.25 ± 0.04 (SD, n = 4) nmol cm-2 s-1. It should be stressed that the relatively large differences between surface gradient and profile modeling obtained estimates of reaction rates are due to the ill-defined boundary between mat and water. Modeling of rates in deeper layers are not subject to a similar uncertainty.
Hydrogen and Hydrogen Sulfide Profiles under Dark Conditions
Many sets of combined H2/H2S profiles were measured in the Aggersund microbial mat, and an example is shown in Figure 2. The extent of H2 accumulation was highly variable between samples with the maximum H2 levels after darkening varying from 0 to 40 μmol L-1 [average 12 ± 12 (SD, n = 13)]. We suspect that the variation was caused by differences in the species composition of the cyanobacterial community. The average depth of the H2 peak was 0.5 ± 0.2 (SD, n = 12) mm. The average H2 production rate was 0.031 ± 0.027 nmol cm-2 s-1 (SD, n = 6). Mats that appeared to be very similar could have very different levels of H2 accumulation, but a prerequisite for substantial H2 accumulation seemed to be a well-developed cyanobacterial community. The data in Figure 2 show H2 accumulation higher than average, but the shapes and positions of the profiles are representative. After only 1 min of dark incubation H2 concentrations of up to ∼15 μmol L-1 were observed in the formerly oxic layer of the mat (Figure 2A). Hydrogen sulfide was present from a depth of ∼1.3 mm, and in this layer H2 was consumed as well. Hydrogen and hydrogen sulfide profiles after 20 min of darkening are shown in Figure 2B. Here the H2 accumulation peaked with a concentration of ∼40 μmol L-1 and H2S was present from a depth of ∼0.8 mm. Modeling of the H2 profile showed a production zone from 0.5 to 1 mm and a consumption zone from 1 to 1.3 mm. The H2 production in the zone with net production was 0.060 nmol cm-2 s-1 and the H2 consumption in the layers with measurable H2 and net consumption was 0.043 nmol cm-2 s-1, with the difference of 0.017 nmol cm-2 s-1 diffusing out of the mat. The peak H2 concentrations had decreased to ∼20 μmol L-1 after 120 min of darkening, and H2S was present at the surface of the mat (Figure 2C). Modeling of the H2 profile now showed a production zone from 0.5 to 0.8 mm and a consumption zone from 0.8 to 0.9 mm, where the net H2 production was 0.032 nmol cm-2 s-1 and the H2 consumption was 0.023 nmol cm-2 s-1, respectively. At 120 min, 0.09 nmol H2 cm-2 s-1 diffused out of the mat. After 240 min (Figure 2D), there was no H2 accumulation and H2S was present to the very surface of the mat.
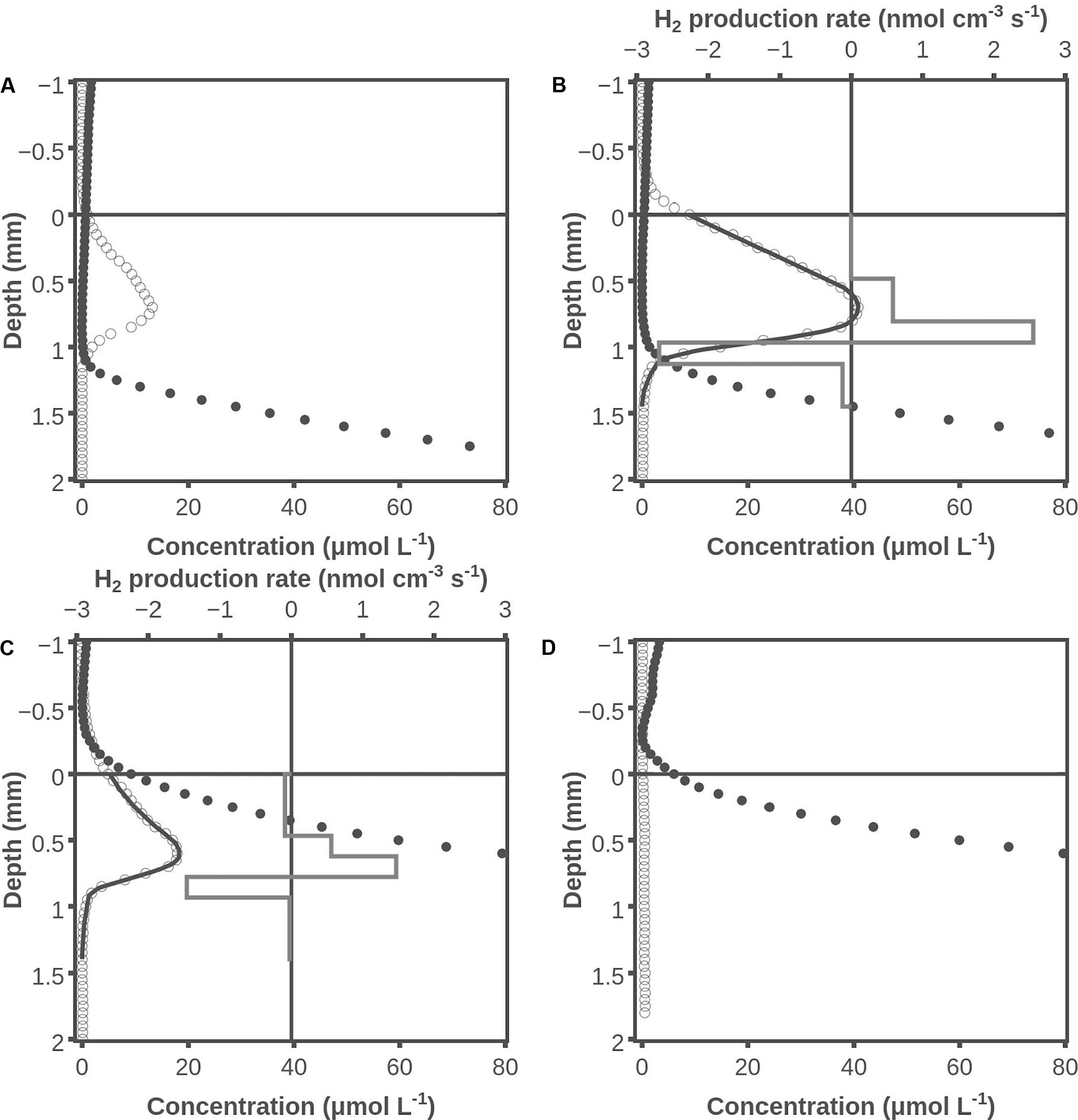
FIGURE 2. Examples of hydrogen and hydrogen sulfide concentration profiles under dark conditions. One minute after darkening (A), 20 min after darkening (B), 120 min after darkening (C), and 240 min after darkening (D). Shown are measured H2 concentrations (○), H2S concentrations (●), modeled H2 concentration profile (―), and modeled H2 production rate (box diagram). Data obtained by use of the combined H2 - H2S sensor.
Overlap of H2 and O2 under Illumination and Darkness
To investigate how O2 affected H2 metabolism, the mats were analyzed with combined H2-O2 microsensors. An example of perfectly aligned data for O2 and H2 obtained with such sensors is shown in Figure 3. During illumination, O2 was produced in a zone from ∼0-0.4 mm and consumed in a zone from ∼0.4-1.0 mm, and no H2 was detected (Figure 3). The set of profiles initiated 1 min after darkening showed that O2 now only penetrated to ∼0.25 mm, and H2 was already accumulating with maximum concentrations of 26 μmol L-1 (Figure 3). It is not possible to model the rates of H2 metabolism as the data were obtained 1–5 min after darkening and thus are far from a steady state. However, as seen in Figure 2 the peak of H2 occurs in the layer that was oxic during illumination, and the linear H2 profile through the ∼0.25 mm thick oxic zone indicates no net transformation in this layer. Due to sulfide poisoning of the sensor it was not possible to get H2 profiles for extended periods of dark incubation.
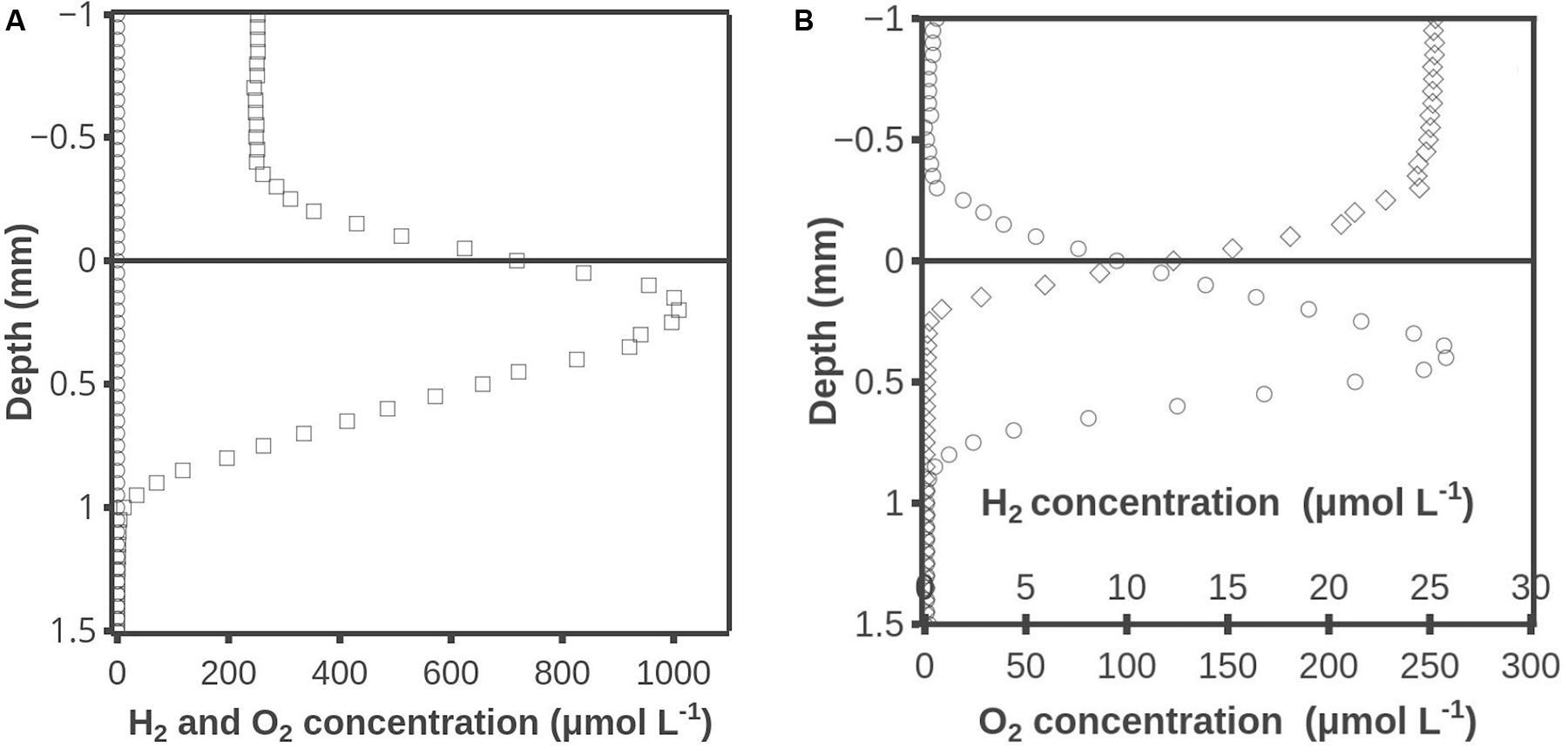
FIGURE 3. O2 and H2 concentration profiles during illumination (A) and 1 min after darkening (B). Shown are measured H2 concentrations (○) and O2 concentrations during illumination (□) and darkness (◇). Note different scale on horizontal axis. Results obtained with the combined H2-O2 sensor.
Consumption of External Hydrogen during Light and Dark
A series of experiments were conducted where H2 was added to the overlying water, and an example of data from these experiments is shown in Figure 4. Illumination resulted in O2 being produced in a zone from 0 to 0.55 mm at a rate of 0.94 nmol cm-2 s-1 and O2 being consumed in a zone from 0.55 to 0.65 mm at a rate of 0.38 nmol cm-2 s-1. The main H2 consumption zone was from 0.5 to 0.7 mm, and H2 was consumed at a rate of 0.03 nmol cm-2 s-1 (Figure 4). There was no indication of any H2 consumption in the oxic photosynthetic zone, but a high rate of H2 consumption at the oxic-anoxic interface. This consumption could be due to both photosynthetic (Drutschmann and Klemme, 1985) and non-photosynthetic prokaryotes. Oxygen was consumed in a zone from 0 to 0.15 mm at a rate of 0.17 nmol cm-2 s-1 during darkness. Hydrogen was consumed down to 0.5 mm at a uniform low rate integrating up to 0.05 nmol cm-2 s-1 (Figure 4). It is not possible to judge if the oxic zone actually had lower activity than the anoxic zone, but modeling of the profile with zero activity in the oxic zone gave just as good a curve fit (data not shown) as the activity distribution shown in Figure 4B.
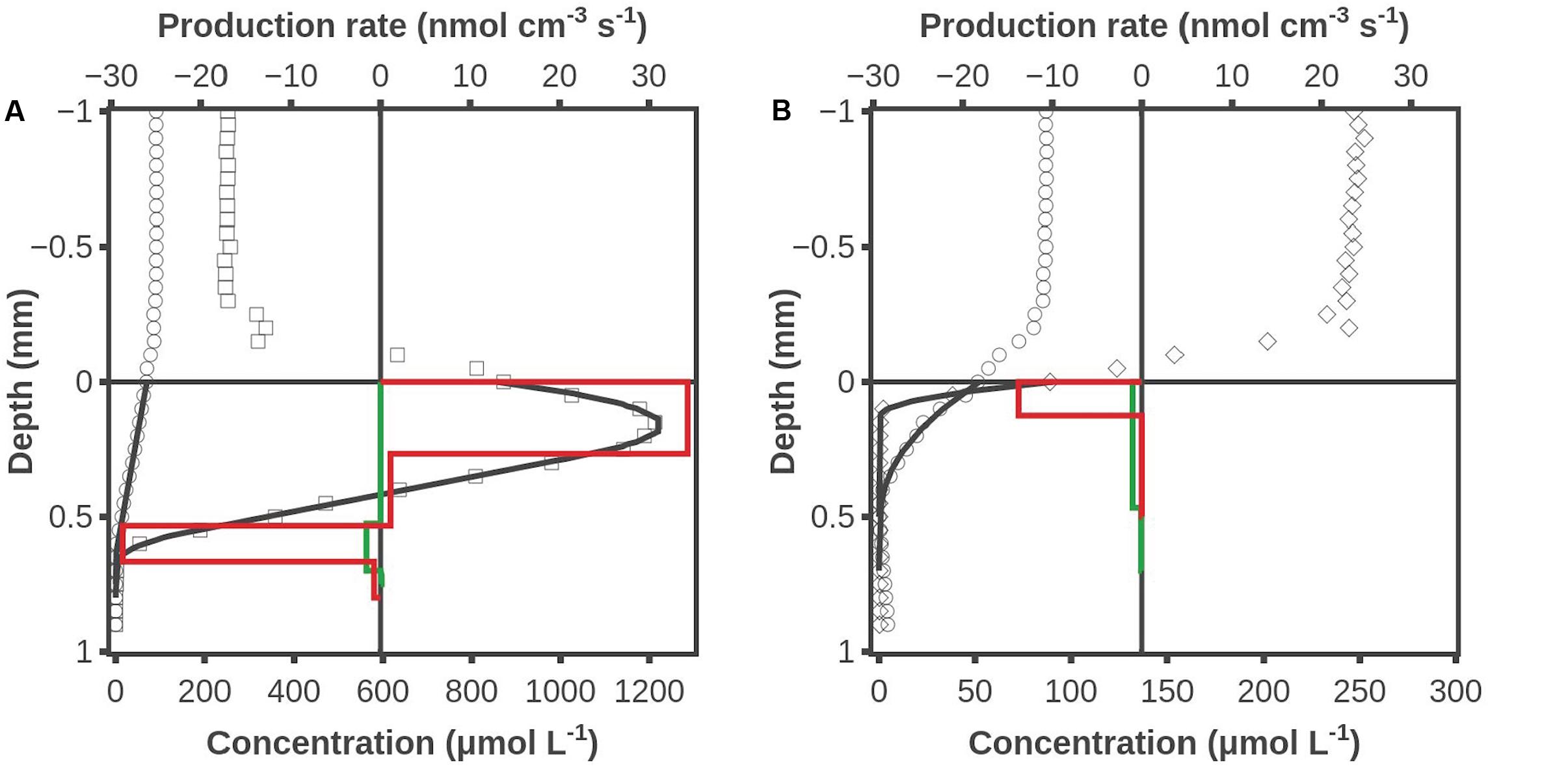
FIGURE 4. O2 and H2 concentration profiles when H2 was added to the water phase. (A) After 2 h of illumination, (B) after 2 h of dark incubation. Shown are measured H2 concentrations (○), O2 concentrations during illumination (□), O2 concentration during darkness (◇), modeled H2 and O2 concentrations (―), modeled H2 production rate (green box diagram) and modeled O2 production rate (red box diagram). Data obtained by combined H2 – O2 sensor.
Hydrogen Accumulation When Sulfate Reduction Was Inhibited by Molybdate and Methanogenesis Was Inhibited by BES
Substantial H2 concentrations persisted for a long time in the mat when sulfate reduction was inhibited by molybdate (Figure 5). After 120 min the H2 accumulation thus peaked with a concentration of ∼13 μmol L-1. This concentration is much lower than the levels shown in Figure 2, but this may be due to a less developed mat. After 210 min the maximum H2 concentration had decreased to ∼6 μmol L-1, and the methanogensis inhibitor BES was added. Inhibition of methanogenesis resulted in the maximum H2 concentration increasing to ∼10 μmol L-1. Hydrogen sulfide profiles are not shown since sulfide forms a complex with molybdate (Newport and Nedwell, 1988). A positive effect of BES on H2 accumulation was also seen in two similar experiments with an average increase in maximum H2 concentrations of 53 ± 9% (SD, n = 3).
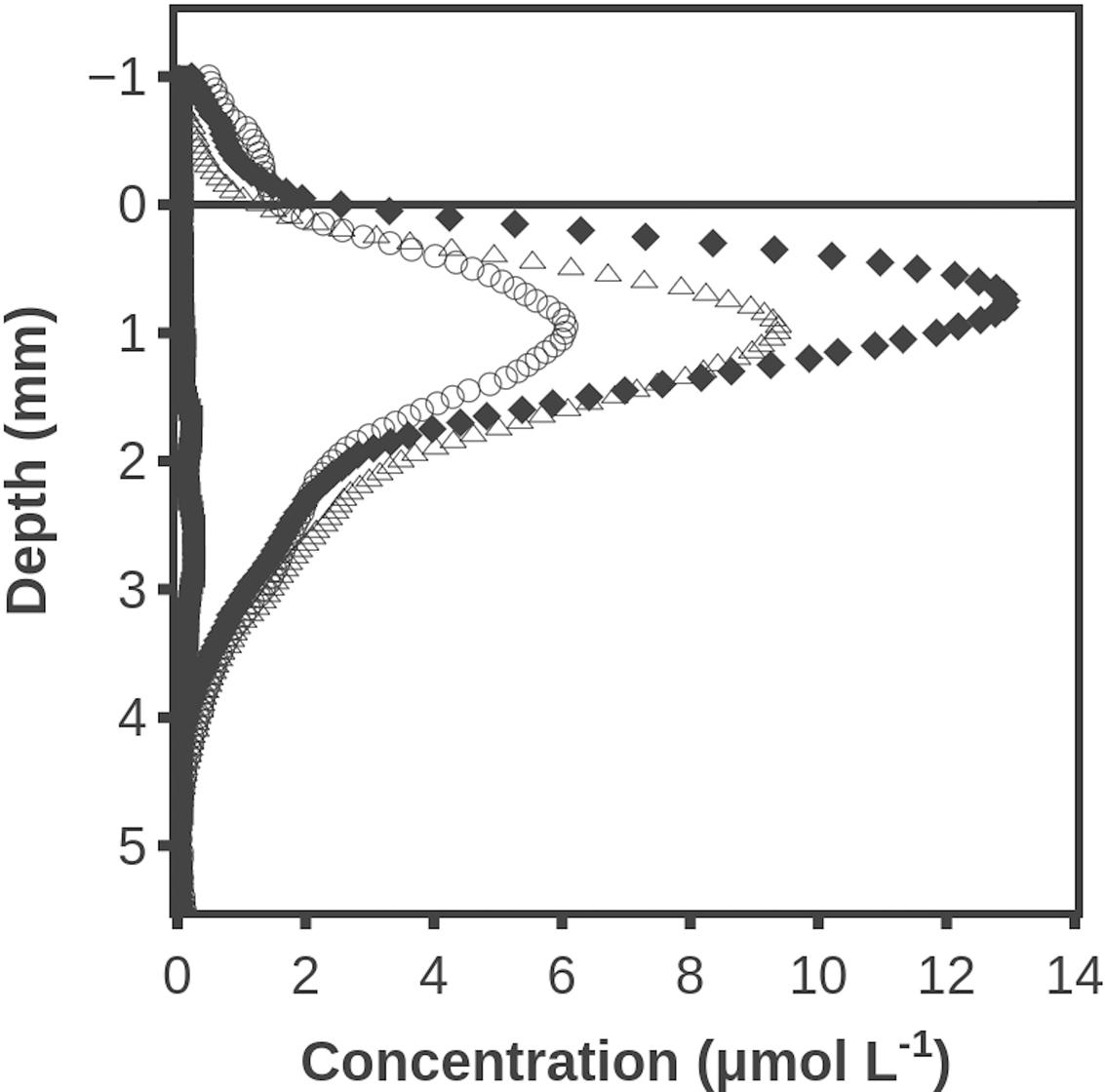
FIGURE 5. Hydrogen accumulation after darkening when sulfate reduction was inhibited with molybdate and after 210 min where methanogenesis was additionally inhibited by addition of BES. Time after darkening: 1 min (■), 120 min (◆), 210 min (○) and 240 min (△). Data obtained by a combined H2 – H2S sensor.
Hydrogen and Hydrogen Sulfide Profiles When Microbial Migration from Anoxic Layers to the Oxic Zone Was Prevented
Hydrogen accumulation after darkening also occurred when the photosynthetically active part of the mat was placed on agar (Figure 6A). The H2 accumulation peaked after ∼30 min and H2 was barely detectable after 450 min. No H2S was detected at any time (data not shown). Oxygen profiles were measured at several locations and all data showed that the mat was oxic in all layers during the illumination but became anoxic below about 0.2 mm after 5 min of darkening (data not shown). In another experiment with the surface ∼1 mm of the mat placed on agar, the H2 accumulation peaked at ∼15 μmol L-1 after 20 min of darkness (Figure 6B). When the maximum concentration had decreased to ∼4 μmol L-1 at 165 min, molybdate was added to inhibit sulfate reduction. This resulted in a sizable stimulation of the H2 accumulation so that a maximum concentration of 27 μmol L-1 was reached. Sequential addition of inhibitor was also tested on a new slice of mat by adding molybdate 2 h before darkening and adding BES after 210 min of darkening. The maximum level of H2 of 13 μmol L-1 in the molybdate inhibited mat occurred after 20 min of darkening, after which H2 decreased to a maximum of 5 μmol L-1 at 195 min. When BES was added, it led to an increase of the maximum H2 concentration to 15 μmol L-1 at 315 min (data not shown).
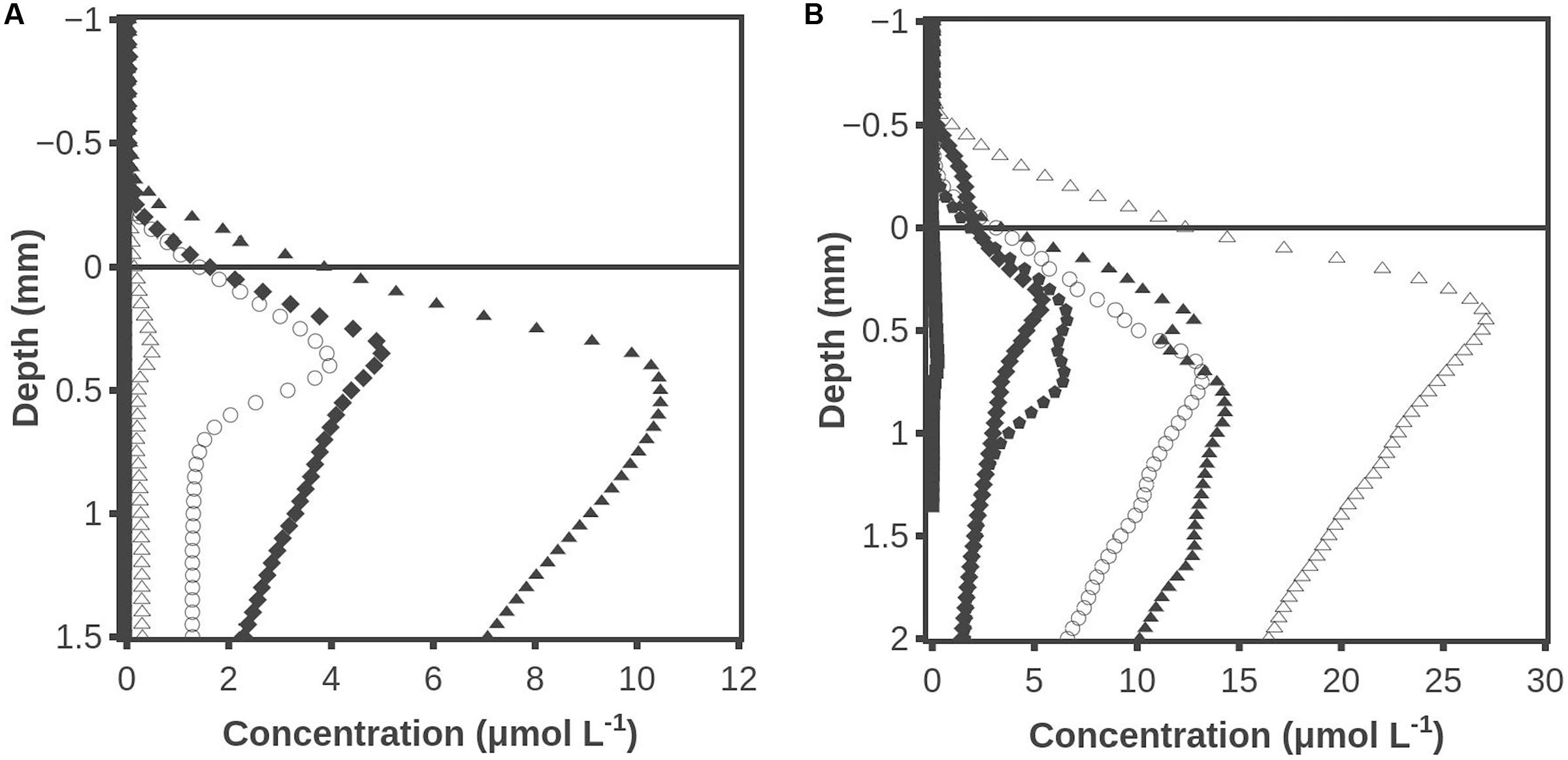
FIGURE 6. (A) Hydrogen accumulation when the top ∼1 mm cyanobacterial layer of the mat was placed on agar. Time after darkening: 1 min (■), 10 min (◆), 30 min (▲), 60 min (○) and 450 min (△). (B) Hydrogen concentration profiles when the surface ∼1 mm of the mat was placed on agar. After 165 min molybdate was added to inhibit sulfate reduction. Time after darkening: 1 min (■), 10 min (◆), 20 min (▲), 30 min (), 170 min (○) and 225 min (△). Data obtained by the combined H2 – H2S sensor.
Discussion
When comparing stratification of chemical species and types of metabolism in compact microbial communities it is very important to align profiles obtained by different methods at the same depth scale. Various procedures have been used to get a reasonable alignment of microsensor profiles, including gluing sensors together with tips very close to each other (e.g., Revsbech et al., 1983) and visual determination of “surface” by observing the individual sensor tips during insertion. Until now only the combined O2/N2O sensor (Revsbech et al., 1988) has allowed for simultaneous measurement of two gasses in the same point. We here present the first comprehensive study of H2 metabolism in a microbial mat where we have totally aligned profiles of H2/H2S and H2/O2 so that interactions between H2, O2 and sulfur cycling can be precisely evaluated.
Position of H2 Producing Layer
The microbial mat was oxic down to about 1 mm during illumination, but after darkening O2 only penetrated to about 0.2 mm. When darkened, H2 accumulated only within the formerly oxic zone of the intact sediment cores, where photosynthates formed during illumination were available for fermentation. The zone of net H2 formation as measured by the combined H2 – H2S sensor was in anoxic layers below 0.4–0.5 mm depth and thus relatively far below the depth of maximum net O2 production as measured by a separate O2 sensor. When the combined H2 – O2 sensor was used we observed, on the other hand, a perfect alignment between the concentration peak of H2 and photosynthetic layers below 0.2 mm that became anoxic by darkening. It thus seems that our surface definition was about 0.2 mm wrong when we estimated the surface from combined H2-H2S data. This illustrates the problems of aligning profiles from separate sensors and the strength of using sensors with dual chemical sensing as used here. By use of the two types of sensors with dual chemical sensing it was possible to determine at very high resolution how H2 production and consumption were associated with the presence of both O2 and H2S. The problem of aligning profiles from different sensors was in our case probably aggravated by the very different tip shapes and dimensions of O2 and combined sensors, where the O2 sensor had a slimline-tip of 10 μm and the combined sensors had blunt tips of 50 μm. Insertion of a microsensor changes the flow pattern above the mat and also the thickness of the diffusive boundary layer (Glud et al., 1994), and this effect is very dependent on sensor dimensions and shape.
Position of H2 Consuming Layers
The results shown in Figures 3, 4 were obtained by use of combined H2-O2 sensors. Microbial mat overlaid with air-saturated water was analyzed in Figure 3, and the dark profile initiated 1 min after darkening showed no sign of H2 consumption in the 0.2-mm thick oxic surface layer. Further proof for the very low H2 consumption potential is provided by the data in Figure 4, obtained after saturating the overlying water with a mixture of ∼10% H2 gas and air. No H2 consumption could be seen in the oxic 0.7-mm layer of the illuminated mat (Figure 4A). When the mat had been dark incubated for 2 h there was a uniform low rate of H2 consumption throughout the formerly oxic zone (Figure 4B). These data are important as it has previously been suggested that sulfate reduction may occur at high rates in oxic cyanobacterial mats (Canfield and Des Marais, 1991). The absence of any significant H2 oxidation potential in oxic mat counter-indicates that there are active sulfate reducers in the oxic mat, as sulfate reducing bacteria generally use H2 as electron donor (Jørgensen, 1982).
The data obtained with combined H2 – H2S sensors shortly after darkening, but sufficiently long after darkening to approach diffusional equilibrium, all have almost linear profiles between the H2 production zone and the overlying water (e.g., Figure 2B). This indicates that not only oxic mat layers, but also mat layers that have a recent history of being oxic are characterized by very little H2 consumption as illustrated by Figure 4B. A low H2 oxidation potential of oxic cyanobacterial mats was also suggested by Hoehler et al. (2002).
A high H2 consumption potential was found in the layer that formed the oxic-anoxic interface during illumination (Figure 2). This indicates that anaerobic bacteria are the main hydrogenotrophs in the mat, and this is in accordance with the findings of Lee et al. (2014) who identified sulfate reducing bacteria as the main hydrogen consumers. The zone of intense H2 consumption moved 0.2 mm upward from 20 to 120 min after darkening (Figures 2B,C), indicating that the anaerobic bacteria were able to migrate. At 120 min there was also a low rate of H2 consumption in the surface layers that were now highly sulfidic, indicating that some anaerobic bacteria had reached these layers by migration, or that dormant bacteria permanently inhabiting these layers had become active. The presence of sulfate reducing bacteria in oxic microbial mat has previously been reported by Minz et al. (1999). The question of migration of bacteria versus revival of inactive hydrogenotrops in the layer that was oxic during the light period was addressed by slicing off the top 1 mm and placing the slice on agar (Figures 6A,B). When molybdate was added to the sliced mat after 165 min of dark incubation (Figure 6B) there was a rapid and significant increase in H2 concentration, indicating that resident sulfate reducing bacteria had become active although all layers of the mat slice were oxic during the preceding light period. The effect of molybdate could also be explained by change in the fermentative pattern, but we are not aware of reports indicating such an effect of molybdate. So after >1 h of dark incubation it seems that both migration from below and revival of resident anaerobic hydrogenotrophic microorganisms caused hydrogen consumption in the surface layers of intact mats.
Sulfate Reduction versus Methanogenesis as H2 Sinks
Previous investigations (Lee et al., 2014; Nielsen et al., 2015) indicate that sulfate reduction is the major hydrogenotrophic process in dark-incubated cyanobacterial mats, although methanogenesis may also occur in saline environments (Oremland and Taylor, 1978). We investigated the possible role of methanogens as hydrogenotrophs in the mat by sequentially adding molybdate and BES to both intact (Figure 5) and sliced mat (data not shown). Data from experiments with addition of inhibitors should always be interpreted with care as no inhibitor is totally specific. Molybdate thus complexes with H2S (and thereby lowers the H2S activity), and it also forms complexes with volatile fatty acids (VFAs) (Finke et al., 2007). BES addition caused a significant increase in H2 concentration, indicating that significant numbers of active methanogens were present. As expected the effect of BES was smaller than the effect of molybdate, in accordance with the conclusions of Lee et al. (2014) that sulfate reducers are the main hydrogenotrophs in such mats. The high H2 concentrations in a dark-incubated mat might, however, make methanogenesis locally and temporally favorable (Hoehler et al., 2002). The effect of BES in the sliced mat provides evidence that methanogens like sulfate reducers could survive long periods of oxic conditions in the mat, as previously described for a hot spring microbial mat (Revsbech et al., 2016) and soils (Angel et al., 2012).
Absence of H2S from Sliced Mat
It should be noticed that sulfide did not accumulate in the sliced 1-mm mat layer during the dark incubation (Figures 6A,B). That might seem to counter-indicate H2 consumption by sulfate reduction, suggested by the increase in H2 after addition of molybdate, but the data in Figure 2 may offer an explanation. Here H2S was found in high concentrations in layers deeper than 1.1 mm after 1–6 min (the time to record a profile was about 5 min) of darkness, and the situation was basically unchanged after 20–26 min of darkness. The mean diffusion time for a H2S molecule over a distance of 1 mm is less than 10 min, assuming a diffusion coefficient of 1 × 10-5 cm2s-1 within the mat (Jørgensen, 2000). By diffusion alone the whole mat should thus have been highly sulfidic already after a few minutes, and that points to extensive sulfide consumption within the previously oxic mat. The consumption of free sulfide may be due to oxidation and precipitation by iron and manganese minerals. The buffer capacity of the iron pool should be very high if it could consume the high sulfide flux from below in the intact mat for more than 20 min. The iron pool might thus be able to keep an isolated 1-mm top layer free of dissolved sulfide for a long time. An alternative explanation for the absence of free sulfide is oxidation by cable bacteria (Pfeffer et al., 2012). The mats from Aggersund have been found to be rich in cable bacteria (L.P. Nielsen, unpublished), and these may be able to oxidize H2S within the upper few mm of the mat by transporting the electrons to the surface of mat. In intact mat they may not be able to oxidize all the sulfide diffusing up from below, but the much lower amount formed in a thin slice might all be oxidized.
Rate of H2 Formation as Compared to Net Photosynthesis
The rates of net H2 production deduced from Figure 2B were 0.06 nmol cm-2 s-1 when they were at the highest. This is about 10% of the rate of net O2 production in the same mat (Figure 1). Even in the presence of molybdate there is some decrease in H2 production with time (Figure 5), but the illumination periods were also limited, so the figures are roughly comparable. One O2 corresponds to two H2 molecules in terms of electron transfer, so about 5% of the reducing equivalents stored in organic compounds during the light period were liberated as H2. Fermentation of glucose by a Clostridium that was studied because of a high rate of H2 formation was found to yield up to 2 moles of H2 per mole of glucose (Jo and Kim, 2016). Assuming a loss of glucose equivalents as fast as the rate of build-up during photosynthesis, the 0.6–0.9 nmol O2 cm-2 s-1 corresponds to a formation rate of 0.2–0.3 nmol H2 cm-2 s-1. The loss rate of glucose in the dark should actually be lower than the rate of glucose production by photosynthesis in the light, and we thus also see only 20–30% of the H2 production calculated above. The mass balance considerations do indicate a substantial loss of photosynthates by fermentation during the dark period. This is in accordance with previous estimates of extreme rates of sulfate reduction is such mats (Jørgensen and Cohen, 1977), as the sulfate reducing bacteria utilize the fermentation products.
Based on our data we cannot rule out that there was H2 production due to nitrogenase activity, which would invalidate the H2 budget outlined above. However, Burow et al. (2012) and Lee et al. (2014) found no effect on H2 accumulation in a similar mat when nitrogen fixation was inhibited. Both studies observed a production of other fermentation products as well as H2, which support fermentation and not nitrogen fixation as the H2 source. The immediate rise in H2 after darkening observed in our study also points to fermentation as the major H2 forming process, as de novo synthesis of the O2 sensitive nitrogenase would require some time.
Conclusion
The analyzed microbial mat produced high concentrations of H2 by fermentation after onset of darkness. The layers that had the highest photosynthetic activity in the light and became anoxic in the dark had the highest rate of H2 production. Results from addition of inhibitors suggest that sulfate reducing bacteria were the main hydrogenotrophs of the mat, but that methanogenic archaea also contributed to the disappearance of the H2 peak. Both sulfate reducing bacteria and methanogens were apparently able to survive the high O2 concentrations within the mat during illumination and become active again within less than 2 h of anoxia. Oxic conditions in the mat during illumination resulted in low H2 consumption potential, and this low potential persisted at least 30 min after anoxia was reached during subsequent darkening. This indicates that the anaerobes in the mat could survive the oxic conditions during illumination, but in a dormant stage.
Author Contributions
KM did all measurements and was leading in the writing of the manuscript. NR supervised the study, was active in the development of new sensors, and participated in the writing of the manuscript. LN co-supervised the study and participated in the writing of the manuscript.
Funding
The study was supported by the EU FP7 project Senseocean, 614141, and by Innovation Fund Denmark, grant Electrogas 4106-00017B.
Conflict of Interest Statement
The authors declare that the research was conducted in the absence of any commercial or financial relationships that could be construed as a potential conflict of interest.
Acknowledgment
We are grateful for the help of Lars Borregaard Pedersen and Preben Sørensen in the construction of microsensors.
References
Anderson, K. L., Tayne, T. A., and Ward, D. M. (1987). Formation and fate of fermentation products in hot spring cyanobacterial mats. Appl. Environ. Microbiol. 53, 2343–2352.
Angel, R., Claus, P., and Conrad, R. (2012). Methanogenic archaea are globally ubiquitous in aerated soils and become active under wet anoxic conditions. ISME J. 6, 847–862. doi: 10.1038/ismej.2011.141
Appel, J., Phunpruch, S., Steinmüller, K., and Schulz, R. (2000). The bidirectional hydrogenase of Synechocystis sp. PCC 6803 works as an electron valve during photosynthesis. Arch. Microbiol. 173, 333–338. doi: 10.1007/s002030000139
Benemann, J. R., and Weare, N. M. (1974). Hydrogen evolution by nitrogen-fixing Anabaena cylindrica cultures. Science 184, 174–175. doi: 10.1126/science.184.4133.174
Berg, P., Risgaard-Petersen, N., and Rysgaard, S. (1998). Interpretation of measured concentration profiles in sediment pore water. Limnol. Oceanogr. 43, 1500–1510. doi: 10.4319/lo.1998.43.7.1500
Bolhuis, H., Cretoiu, M. S., and Stal, L. J. (2014). Molecular ecology of microbial mats. FEMS Microbiol. Ecol. 90, 335–350. doi: 10.1111/1574-6941.12408
Burow, L. C., Woebken, D., Bebout, B. M., McMurdie, P. J., Singer, S. W., Pett-Ridge, J., et al. (2012). Hydrogen production in photosynthetic microbial mats in the Elkhorn Slough estuary, Monterey Bay. ISME J. 6, 863–874. doi: 10.1038/ismej.2011.142
Burow, L. C., Woebken, D., Marshall, I. P. G., Singer, S. W., Pett-Ridge, J., Prufert-Bebout, L., et al. (2014). Identification of Desulfobacterales as primary hydrogenotrophs in a complex microbial mat community. Geomicrobiology 12, 221–230. doi: 10.1111/gbi.12080
Canfield, D. E., and Des Marais, D. J. (1991). Aerobic sulfate reduction in microbial mats. Science 251, 1471–1473. doi: 10.1126/science.11538266
Dolla, A., Fournier, M., and Dermoun, Z. (2006). Oxygen defense in sulfate-reducing bacteria. J. Biotechnol. 126, 87–100. doi: 10.1016/j.jbiotec.2006.03.041
Drutschmann, M., and Klemme, J.-H. (1985). Sulfide-repressed, membrane-bound hydrogenase in the thermophilic facultative phototroph, Chloroflexus aurantiacus. FEMS Microbiol. Lett. 28, 231–235. doi: 10.1111/j.1574-6968.1985.tb00797.x
Fenchel, T. (1998). Formation of laminated cyanobacterial mats in the absence of benthic fauna. Aquat. Microb. Ecol. 14, 235–240. doi: 10.3354/ame014235
Finke, N., Vandieken, V., and Jørgensen, B. B. (2007). Acetate, lactate, propionate, and isobutyrate as electron donors for iron and sulfate reduction in Arctic marine sediments, Svalbard. FEMS Microbiol. Ecol. 59, 10–22. doi: 10.1111/j.1574-6941.2006.00214.x
Fournier, M., Zhang, Y., Wildschut, J. D., Dolla, A., Voordouw, J. K., Schriemer, D. C., et al. (2003). Function of oxygen resistance proteins in the anaerobic, sulfate-reducing bacterium Desulfovibrio vulgaris Hildenborough. J. Bacteriol. 185, 71–79. doi: 10.1128/JB.185.1.71-79.2003
Fründ, C., and Cohen, Y. (1992). Diurnal cycles of sulfate reduction under oxic conditions in cyanobacterial mats. Appl. Environ. Microbiol. 58, 70–77.
Gest, H., Ormerod, J. G., and Ormerod, K. S. (1962). Photometabolism of Rhodospirillum rubrum: light-dependent dissimilation of organic compounds to carbon dioxide and molecular hydrogen by an anaerobic citric acid cycle. Arch. Biochem. Biophys. 97, 21–33. doi: 10.1016/0003-9861(62)90039-5
Glud, R. N., Gundersen, J. K., Revsbech, N. P., and Jørgensen, B. B. (1994). Effects on the benthic diffusive boundary layer imposed by microelectrodes. Limnol. Oceanogr. 39, 462–467. doi: 10.4319/lo.1994.39.2.0462
Glud, R. N., Jensen, K., and Revsbech, N. P. (1995). Diffusivity in surficial sediments and benthic mats determined by use of a combined N2O-O2 microsensor. Geochim. Cosmochim. Acta 59, 231–237. doi: 10.1016/0016-7037(94)00321-C
Hoehler, T. M., Albert, D. B., Alperin, M. J., Bebout, B. M., Martens, C. S., and Des Marais, D. J. (2002). Comparative ecology of H2 cycling in sedimentary and phototrophic ecosystems. Antonie Van Leeuwenhoek 81, 575–585. doi: 10.1023/A:1020517924466
Hoehler, T. M., Alperin, M. J., Albert, D. B., and Martens, C. S. (1998). Thermodynamic control on hydrogen concentrations in anoxic sediments. Geochim. Cosmochim. Acta 62, 1745–1756. doi: 10.1016/S0016-7037(98)00106-9
Hoehler, T. M., Bebout, B. M., and Des Marais, D. J. (2001). The role of microbial mats in the production of reduced gases on the early Earth. Nature 412, 324–327. doi: 10.1038/35085554
Horne, A. J., and Lessner, D. J. (2013). Assessment of the oxidant tolerance of Methanosarcina acetivorans. FEMS Microbiol. Lett. 343, 13–19. doi: 10.1111/1574-6968.12115
Jasso-Chávez, R., Santiago-Martiìnez, M. G., Lira-Silva, E., Pineda, E., Zepeda-Rodriìguez, A., Belmont-Diìaz, J., et al. (2015). Air-adapted Methanosarcina acetivorans shows high methane production and develops resistance against oxygen stress. PLOS ONE 10:e0117331. doi: 10.1371/journal.pone.0117331
Jeroschewski, P., Steuckart, C., and Kühl, M. (1996). An amperometric microsensor for determination of H2S in aquatic environments. Anal. Chem. 24, 4351–4357. doi: 10.1021/ac960091b
Jo, J. H., and Kim, W. (2016). Carbon material distribution and flux analysis under varying glucose concentrations in hydrogen-producing Clostridium tyrobutyricum JM1. J. Biotechnol. 228, 103–111. doi: 10.1016/j.jbiotec.2016.04.051
Jørgensen, B. B. (1982). Mineralization of organic matter in the sea bed – The role of sulphate reduction. Nature 296, 643–645. doi: 10.1038/296643a0
Jørgensen, B. B. (2000). “Bacteria and marine biogeochemistry,” in Marine Geochemistry, eds H. D. Schulz and M. Zabel (Heidelberg: Springer), 169–206.
Jørgensen, B. B., and Cohen, Y. (1977). Solar Lake (Sinai). 5. The sulfur cycle of the benthic cyanobacterial mats. Limnol. Oceanogr. 22, 657–666. doi: 10.4319/lo.1977.22.4.0657
Jørgensen, B. B., and Revsbech, N. P. (1983). Colorless sulfur bacteria, Beggiatoa spp. and Thiovulum spp., in O2 and H2S microgradients. Appl. Environ. Microbiol. 45, 1261–1270.
Lee, H., Vermaas, W. F. J., and Rittmann, B. E. (2010). Biological hydrogen production: prospects and challenges. Trends Biotechnol. 28, 262–271. doi: 10.1016/j.tibtech.2010.01.007
Lee, J. Z., Burow, L. C., Woebken, D., Everroad, R. C., Kubo, M. C., Spormann, A. M., et al. (2014). Fermentation couples Chloroflexi and sulfate-reducing bacteria to Cyanobacteria in hypersaline microbial mats. Front. Microbiol. 5:61. doi: 10.3389/fmicb.2014.00061
Lovley, D. R., Dwyer, D. F., and Klug, M. J. (1982). Kinetic-analysis of competition between sulfate reducers and methanogens for hydrogen in sediments. Appl. Environ. Microbiol. 43, 1373–1379.
Millero, F. J., Plese, T., and Fernandez, M. (1988). The dissociation of hydrogen-sulfide in seawater. Limnol. Oceanogr. 33, 269–274. doi: 10.1021/es402956r
Minz, D., Fishbain, S., Green, S. J., Muyzer, G., Cohen, Y., Rittmann, B. E., et al. (1999). Unexpected population distribution in a microbial mat community: sulfate-reducing bacteria localized to the highly oxic chemocline in contrast to a eukaryotic preference for anoxia. Appl. Environ. Microbiol. 65, 4659–4665.
Moezelaar, R., and Stal, L. (1997). A comparison of fermentation in the cyanobacterium Microcystis PCC7806 grown under a light/dark cycle and continuous light. Eur. J. Phycol. 32, 373–378. doi: 10.1080/09670269710001737309
Newport, P. J., and Nedwell, D. B. (1988). The mechanisms of inhibition of Desulfovibrio and Desulfotomaculum species by selenate and molybdate. J. Appl. Bacteriol. 65, 419–423. doi: 10.1111/j.1365-2672.1988.tb01911.x
Nielsen, M., Revsbech, N. P., and Kühl, M. (2015). Microsensor measurements of hydrogen gas dynamics in cyanobacterial microbial mats. Front. Microbiol. 6:726. doi: 10.3389/fmicb.2015.00726
Oremland, R. S., and Taylor, B. F. (1978). Sulfate reduction and methanogenesis in marine sediments. Geochim. Acta 42, 209–214. doi: 10.1016/0016-7037(78)90133-3
Pfeffer, C., Larsen, S., Song, J., Dong, M., Besenbacher, F., Meyer, R. L., et al. (2012). Filamentous bacteria transport electrons over centimetre distances. Nature 491, 218–221. doi: 10.1038/nature11586
Revsbech, N. P. (1989). An oxygen microsensor with a guard cathode. Limnol. Oceanogr. 34, 474–478. doi: 10.4319/lo.1989.34.2.0474
Revsbech, N. P., Jørgensen, B. B., and Blackburn, T. H. (1983). Microelectrode studies of the photosynthesis and O2, H2S, and pH profiles of a microbial mat. Limnol. Oceanogr. 28, 1062–1074. doi: 10.4319/lo.1983.28.6.1062
Revsbech, N. P., Larsen, L. H., Gundersen, J., Dalsgaard, T., Ulloa, O., and Thamdrup, B. (2009). Determination of ultra-low oxygen concentrations in oxygen minimum zones by the STOX sensor. Limnol. Oceanogr. Methods 7, 371–381. doi: 10.4319/lom.2009.7.371
Revsbech, N. P., Nielsen, L. P., Christensen, P. B., and Sørensen, J. (1988). Combined oxygen and nitrous oxide microsensor for denitrification studies. Appl. Environ. Microbiol. 54, 2245–2249.
Revsbech, N. P., Trampe, E., Lichtenberg, M., Ward, D. M., and Kühl, M. (2016). In situ hydrogen dynamics in a hot spring microbial mat during a diel cycle. Appl. Environ. Microbiol. 82, 4209–4217. doi: 10.1128/AEM.00710-16
Scholten, J. C., Conrad, R., and Stams, A. J. (2000). Effect of 2-bromo-ethane sulfonate, molybdate and chloroform on acetate consumption by methanogenic and sulfate-reducing populations in freshwater sediment. FEMS Microbiol. Ecol. 32, 35–42. doi: 10.1111/j.1574-6941.2000.tb00696.x
Stal, L. (1995). Physiological ecology of cyanobacteria in microbial mats and other communities. New Phytol. 131, 1–32. doi: 10.1111/j.1469-8137.1995.tb03051.x
Stams, A. J. M., and Plugge, C. M. (2009). Electron transfer in syntrophic communities of anaerobic bacteria and archaea. Nat. Rev. Microbiol. 7, 568–577. doi: 10.1038/nrmicro2166
Warthmann, R., Cypionka, H., and Pfennig, N. (1992). Photoproduction of H2 from acetate by syntrophic cocultures of green sulfur bacteria and sulfur-reducing bacteria. Arch. Microbiol. 157, 342–348. doi: 10.1007/BF00248679
Keywords: sulfate reducing bacteria, methanogens, survival, fermentation, sediment, microsensor
Citation: Maegaard K, Nielsen LP and Revsbech NP (2017) Hydrogen Dynamics in Cyanobacteria Dominated Microbial Mats Measured by Novel Combined H2/H2S and H2/O2 Microsensors. Front. Microbiol. 8:2022. doi: 10.3389/fmicb.2017.02022
Received: 24 August 2017; Accepted: 04 October 2017;
Published: 18 October 2017.
Edited by:
Brian T. Glazer, University of Hawaii, United StatesReviewed by:
Heather Craig Olins, Boston College, United StatesDirk De Beer, Max Planck Society (MPG), Germany
Copyright © 2017 Maegaard, Nielsen and Revsbech. This is an open-access article distributed under the terms of the Creative Commons Attribution License (CC BY). The use, distribution or reproduction in other forums is permitted, provided the original author(s) or licensor are credited and that the original publication in this journal is cited, in accordance with accepted academic practice. No use, distribution or reproduction is permitted which does not comply with these terms.
*Correspondence: Niels P. Revsbech, revsbech@bios.au.dk