- 1Department of Biology, University of Pennsylvania, Philadelphia, PA, United States
- 2Zentrum für Biochemie und Molekulare Zellforschung (ZBMZ), Institut für Biochemie und Molekularbiologie, Medizinische Fakultät, Albert-Ludwigs-Universität Freiburg, Freiburg, Germany
The thiol-disulfide oxidoreductase DsbA carries out oxidative folding of extra-cytoplasmic proteins by catalyzing the formation of intramolecular disulfide bonds. It has an important role in various cellular functions, including cell division. The purple non-sulfur bacterium Rhodobacter capsulatus mutants lacking DsbA show severe temperature-sensitive and medium-dependent respiratory growth defects. In the presence of oxygen, at normal growth temperature (35°C), DsbA− mutants form colonies on minimal medium, but they do not grow on enriched medium where cells elongate and lyse. At lower temperatures (i.e., 25°C), cells lacking DsbA grow normally in both minimum and enriched media, however, they do not produce the cbb3-type cytochrome c oxidase (cbb3-Cox) on enriched medium. Availability of chemical oxidants (e.g., Cu2+ or a mixture of cysteine and cystine) in the medium becomes critical for growth and cbb3-Cox production in the absence of DsbA. Indeed, addition of Cu2+ to the enriched medium suppresses, and conversely, omission of Cu2+ from the minimal medium induces, growth and cbb3-Cox defects. Alleviation of these defects by addition of redox-active chemicals indicates that absence of DsbA perturbs cellular redox homeostasis required for the production of an active cbb3-Cox, especially in enriched medium where bioavailable Cu2+ is scarce. This is the first report describing that DsbA activity is required for full respiratory capability of R. capsulatus, and in particular, for proper biogenesis of its cbb3-Cox. We propose that absence of DsbA, besides impairing the maturation of the c-type cytochrome subunits, also affects the incorporation of Cu into the catalytic subunit of cbb3-Cox. Defective high affinity Cu acquisition pathway, which includes the MFS-type Cu importer CcoA, and lower production of the c-type cytochrome subunits lead together to improper assembly and degradation of cbb3-Cox.
Introduction
The Gram-negative, purple non-sulfur, facultative photosynthetic bacterium Rhodobacter capsulatus exhibits versatile growth modes, including anoxygenic photosynthesis (Ps) and aerobic respiration (Res) (Zannoni, 2004). Several c-type cytochromes (cyts) and cytochrome (cyt) complexes are key electron transfer components in these energy transduction pathways. Ps growth requires an active photochemical reaction center (RC), an ubihydroquinone: cyt c oxidoreductase (cyt bc1) and an electron carrier (i.e., soluble cyt c2 or membrane-anchored cy) (Jenney et al., 1994; Figure 1). Respiratory growth pathways rely on the NADH and succinate dehydrogenases to reduce the quinone (Q) pool that feeds two different terminal oxidases (La Monica and Marrs, 1976; Hochkoeppler et al., 1995). One of these oxidases is a bd-type quinol oxidase (bd-Qox) that uses hydroquinone (QH2) to reduce O2 to H2O (Giuffrè et al., 2014). The other terminal oxidase is a high O2 affinity cbb3-type cyt c oxidase (cbb3-Cox), which is important for aerobic Res and for the onset of anoxygenic Ps growth (Gray et al., 1994; Hassani et al., 2010). The cbb3-Cox is a member of a large superfamily of heme-Cu oxygen reductases (García-Horsman et al., 1994; Pereira et al., 2001). It receives electrons from the cyts c2 or cy, which are reduced by cyt bc1 (Hochkoeppler et al., 1995; Daldal et al., 2001), to catalyze O2 reduction to H2O, while pumping protons across the cytoplasmic membrane (Han et al., 2011; Figure 1). The cbb3-Cox is composed of four structural subunits (CcoN, CcoO, CcoQ, and CcoP) (Koch et al., 1998), of which CcoO and CcoP are mono- and di-heme c-type cyts, respectively (Gray et al., 1994). The catalytic site where O2 is converted to H2O is located in CcoN, which harbors a low-spin heme b and a binuclear center composed of the high-spin heme b3 and a Cu atom (CuB) (Zufferey et al., 1998; Rauhamäki et al., 2009; Buschmann et al., 2010). CcoQ has no cofactor but is important for the stability of the enzyme (Peters et al., 2008). In wild type R. capsulatus, both cbb3-Cox and bd-Qox are active, and either one of them is sufficient to sustain aerobic Res growth (La Monica and Marrs, 1976; Hochkoeppler et al., 1995). Mutants defective for cyt c maturation (Ccm) (i.e., covalent addition of heme to the apocyt c) (Sanders et al., 2010; Verissimo and Daldal, 2014) do not produce any c-type cyt (Sanders et al., 2010; Verissimo and Daldal, 2014), and are unable to grow by Ps, or by Res via the cbb3-Cox dependent branch. They cannot catalyze the Nadi reaction (α-naphthol + dimethyl-phenylenediamine → indophenol blue + H2O) (Nadi− phenotype), which is a visual indicator for the cbb3-Cox activity (Koch et al., 1998). However, they can still grow by Res via the bd-Qox dependent branch, which contains no c-type cyt (Aygun-Sunar et al., 2006).
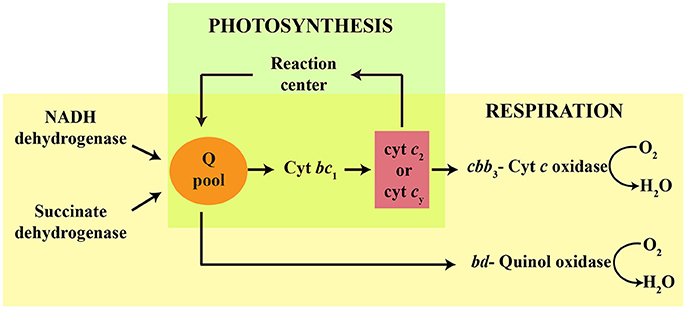
Figure 1. Schematic representation of R. capsulatus growth pathways and electron carriers involved in respiration and photosynthesis. The quinol pool (Q pool) is reduced via either the reaction center, or the respiratory NADH and succinate dehydrogenases. During photosynthesis (green), electrons are transferred from the Q pool to cytochrome bc1 (cyt bc1). The membrane anchored cyt cy and soluble periplasmic cyt c2 carry electrons from the cyt bc1 to the reaction center. During aerobic respiration (yellow), the cbb3-type cyt c oxidase is reduced by cyt bc1 via the electron carrier cyts c2 or cy. A cyt bc1-independent pathway also occurs in R. capsulatus, in which the cyt bd-quinol oxidase is reduced directly by the Q pool.
DsbA is a periplasmic thiol-disulfide oxidoreductase that belongs to the thioredoxin family of proteins with a CxxC conserved domain (Shouldice et al., 2011). It facilitates oxidative folding of extra-cytoplasmic proteins by catalyzing the formation of intramolecular disulfide bonds between two reactive Cys residues of its substrates, which include the c-type apocyts with their conserved CxxCH heme-binding sites. Reduced DsbA is re-oxidized by its recycling partner DsbB, which then transfers the reducing equivalents to the Q pool, and eventually to the electron transport chain (Inaba and Ito, 2008). In E. coli, mutants lacking DsbA are unable to grow under anaerobiosis (Meehan et al., 2017a) and during aerobic growth exhibit a myriad of pleotropic phenotypes, including cell division defects (Meehan et al., 2017b). R. capsulatus DsbA− mutants also exhibit complex growth phenotypes (Deshmukh et al., 2003). Under anaerobiosis, they can grow by Ps (Ps+), but do not form colonies under aerobiosis on enriched medium at normal growth temperature (35°C). Yet, they revert frequently to bypass this growth defect by acquiring additional mutation(s) (Deshmukh et al., 2003). Comparative proteomic studies showed that the periplasmic protease DegP, which is overproduced in the absence of DsbA, is drastically decreased in these bypass revertants (Onder et al., 2008). These findings indicated that overproduction of DegP is correlated with the growth defect observed in the DsbA− mutants (Spiess et al., 1999; Rizzitello et al., 2001; Onder et al., 2008).
In R. capsulatus, and in other organisms, DsbA− mutants are proficient for the Ccm process, but they produce low amounts of c-type cyts (Metheringham et al., 1995; Kojima et al., 2005; Turkarslan et al., 2008; Mavridou et al., 2012). In wild type cells, the apocyts c are substrates for DsbA. The disulfide bonds formed at their heme-binding sites (CxxCH) are then reduced by a thioredoxin (CcmG)/thiol-disulfide oxidoreductase (CcdA) reductive pathway to allow heme ligation. During this process, reduced apocyts c form mixed-disulfide complexes with another thiol-disulfide oxidoreductase (CcmH), which is also likely to be oxidized by DsbA (Verissimo et al., 2017). It is thought that the formation of a disulfide bond at the heme-binding site by DsbA promotes partial folding and confers increased stability to the apocyts c, enhancing Ccm efficiency to yield high amounts of c-type cyts. In Ccm-deficient mutants, apocyts c do not accumulate because they are substrates for the periplasmic protease DegP (Gao and O'Brian, 2007). In the absence of DsbA, the apocyts c remain reduced and the CcmG/CcdA-catalyzed reductive pathway becomes unnecessary for Ccm (Deshmukh et al., 2003; Turkarslan et al., 2008). However, as the absence of DsbA leads to the overproduction of the periplasmic protease DegP, a fraction of the apocyts c are most likely degraded before they are able to ligate heme, resulting in ~50% decrease in the steady-state amounts of the c-type cyts (Onder et al., 2008; Turkarslan et al., 2008).
Proper assembly of cbb3-Cox does not only require a functional Ccm system but is also dependent on Cu availability. How Cu is acquired from the environment and incorporated into the active site of cbb3-Cox during its biogenesis is not well understood. In R. capsulatus a high affinity Cu uptake pathway has been described. It involves various transporters, such as CcoA (Ekici et al., 2014) and CcoI (Koch et al., 2000), and chaperones, including PccA (Trasnea et al., 2016) and SenC (Swem et al., 2005; Lohmeyer et al., 2012). Mutants lacking any of these components have very small amounts of cbb3-Cox, and in most cases, this defect can be palliated by addition of Cu2+ to the growth medium (Ekici et al., 2012a). Moreover, a low affinity Cu uptake pathway was also observed, but its components remain unknown (Ekici et al., 2014).
In this study, we analyzed the properties of R. capsulatus DsbA− mutants. We found that they form filamentous and osmosensitive cells at 35°C under Res growth conditions on enriched medium, where bioavailable Cu is limited. In contrast, these mutants grow normally at 25°C, but they produce very low levels of cbb3-Cox. However, upon supplementation of the growth media with redox-active chemicals, they can grow normally and produce active cbb3-Cox. Our results showed that overproduction of the Cu importer CcoA partially restores the cbb3-Cox defect, suggesting defective Cu incorporation into this enzyme in the absence of DsbA. This is the first description of DsbA being required for respiratory capabilities of R. capsulatus, and in particular, for efficient cbb3-Cox biogenesis under conditions where bioavailable Cu2+ is scarce.
Materials and Methods
Bacterial Strains, Plasmids, and Growth Conditions
The bacterial strains and plasmids used in this work are described in Table 1. E. coli strains were grown aerobically at 37°C in LB medium, supplemented with ampicillin (Amp), kanamycin (Kan), spectinomycin (Spe) or tetracycline (Tet) as needed, at final concentrations of 100, 50, 50, or 12.5 μg per ml, respectively. R. capsulatus strains were grown chemoheterotrophically (i.e., by Res in the dark) at either 35°C or 25°C, in Sistrom's minimal medium A (MedA) (Sistrom, 1960) or in enriched medium (MPYE, mineral-peptone-yeast extract), supplemented with 10, 10 and 2.5 μg per ml of Kan, Spe or Tet, respectively (Darrouzet et al., 2002). As needed, up to 20 μM CuSO4 was added to MPYE to obtain “MPYE+Cu,” and the 1.5 μM CuSO4, which is normally present in MedA, was omitted from MedA to obtain “MedA-Cu” media (Ekici et al., 2012b). Alternatively, mixtures containing different concentrations of cysteine and cystine were used as redox supplements (Turkarslan et al., 2008). For induction of CcoA expression in the DsbA− mutant carrying a plasmid containing wild type ccoA (BK69/MD20, Table 1), 1% L-arabinose was added to the medium.
The cbb3-Cox activity on colonies was visualized using the Nadi staining reaction (formation of indophenol blue) by overlaying them with a mixture containing 1:1 (v/v) of 35 mM α-naphthol and 30 mM N, N, N',N'-dimethyl-p-phenylene diamine (Marrs et al., 1972). Colonies with cbb3-Cox activity exhibited dark blue staining (Nadi+) within 30 s to 1 min, while those lacking it showed no staining (Nadi−) up to 15 min (Aygun-Sunar et al., 2006).
To obtain photomicrographs of R. capsulatus cells, overnight cultures were sub-cultured on enriched medium at 25°C for ~6 h under Res conditions, and then switched to 35°C for 4 h. A 5 μl culture sample was deposited on a microscope slide, and cells were immobilized under a cover slip with a drop of 1% agarose. Slides were examined using an Olympus IX81 microscope at a magnification of 1,000× (10× objective and 100× ocular) and representative field images were acquired with a SensiCam QE camera (Cooke Corporation, Romulus, MI) and IPLab version 4.0 software (Scanalytics, Fairfax, VA).
Molecular Genetic Techniques
Molecular genetic techniques were performed according to standard protocols (Sambrook and Russell, 2001). Conjugal transfer of plasmids from E. coli to R. capsulatus, and interposon mutagenesis via the gene transfer agent (GTA) (Yen et al., 1979) using the SpeR gene cassette from pHP45Ω (Prentki and Krisch, 1984), were carried out as described earlier (Daldal et al., 1986). The DsbA− cbb3-Cox− and DsbA− bd-Qox− double mutants were obtained by GTA crosses using as donors the ccoNO::spe or cydAB::spe alleles carried by the plasmids pAiK32 (Koch et al., 1998) or pKZ2 (Zhang and Daldal, unpublished), respectively (Table 1). Strain MD20 (ΔdsbA::kan) (Deshmukh et al., 2003) was used as a recipient, selecting for antibiotic resistance under growth permissive conditions (e.g., MPYE+Cu plates at 25°C). The double mutants thus obtained were tested for their temperature sensitive Res growth (ResTs) and Cu2+-suppressible phenotypes on MPYE at 35°C. Appropriate merodiploids were constructed by introducing the plasmids pDsbA, pSenC and pBK69 (CcoA) carrying wild type alleles of dsbA, senC and ccoA, respectively into the DsbA− and DsbA− SenC−mutants (Table 1) using triparental crosses as described earlier (Valkova-Valchanova et al., 1998).
Biochemical Techniques
As required, R. capsulatus total cell extracts were prepared using cells collected from freshly grown plates of enriched medium to avoid any undesired reversion event. Cells were resuspended in 25 mM Tris pH 7.5, 150 mM NaCl, 2 mM 4-(2-Aminoethyl) benzenesulfonyl fluoride hydrochloride (AEBSF), 1% N-dodecyl-β-D-maltoside (DDM), 0.2 mg/mL lysozyme and 0.05 mg/mL DNase lysis buffer and incubated at room temperature for 20 min. Clarified extracts were obtained after centrifugation at 15,000 × g for 15 min. Chromatophore (i.e., intracytoplasmic) membranes were prepared from cells grown in liquid enriched medium under Res conditions for ~24 h, and stored at −80°C until use. Cells were disrupted using a French pressure cell in 50 mM MOPS pH 7.0, 10 mM EDTA, 1 mM phenylmethylsulfonylfluoride (PMSF), 0.1 mM ε-aminocaproic acid buffer, as described earlier (Sanders et al., 2008; Verissimo et al., 2011). After 2 h centrifugation at 138,000 × g and 4°C, membrane pellets were resuspended in different buffers as needed. R. capsulatus chromatophore membranes were resuspended to a final concentration of 1–5 mg/ml in 10 mM Tris-HCl pH 7.0, 120 mM KCl buffer and dispersed with a protein: detergent ratio of 1:1 (w/w) of DDM. Protein concentrations were determined according to the Bradford (Bio-Rad) or the BCA (Sigma-Fisher) methods, using bovine serum albumin as a standard. Protein samples were resolved using 12.5% Tris-Glycine (Laemmli, 1970) or 16.5% Tris-Tricine (Schägger and von Jagow, 1987) SDS-PAGE or blue native (BN)-PAGE (Schägger and von Jagow, 1991). The CcoN subunit of cbb3-Cox was identified by incubating immunoblots with rabbit polyclonal antibodies against R. capsulatus CcoN (Koch et al., 2000), following Tris-Glycine SDS-PAGE and electrotransfer onto Immobilon-PVDF membranes (Millipore, MA). Horseradish peroxidase-conjugated anti-rabbit IgG (GE Healthcare) was used as a secondary antibody, and detection was done using the SuperSignal West Pico Chemiluminescent Substrate (Thermo Scientific, Inc.). The presence of c-type cyts in the protein samples was detected via the endogenous peroxidase activity of their covalently attached hemes, using 3,3′,5,5-tetramethylbenzidine (TMBZ) following Tris-Tricine SDS-PAGE (Thomas et al., 1976). Semi-quantitative estimation of the amount of c-type cyts and CcoN subunits of cbb3-Cox was done using the ImageJ software.
Enzymatic Assays
The cbb3-Cox and cyt bc1 activities were measured using a Hitachi U-3210 spectrophotometer by monitoring oxidation, or reduction, of horse heart cyt c (Sigma, MO), respectively, in a stirred cuvette at 25°C. Horse heart cyt c (1 mM) was reduced with sodium dithionite, and excess of reductant removed using a PD-10 desalting column (GE Healthcare). Determination of cbb3-Cox activity was done using cells grown either in solid enriched medium at 25°C under Res condition, or in liquid enriched medium for ~24 h at 25°C under Res conditions. The assays were initiated by adding to the assay buffer containing 50 μM of reduced cyt c either 100–200 μg of R. capsulatus total protein extracts (prepared from freshly grown cells in solid medium) or 1–10 μg of DDM-dispersed chromatophore membrane proteins (prepared from cells grown in liquid medium and stored at −80°C until breakage using a French pressure cell). The decrease in absorbance at 550 nm due to oxidation of cyt c, was recorded for 2 min. The specificity of the assay for cbb3-Cox activity was confirmed by addition of 0.1 mM KCN to the assay mixture, which immediately stopped cyt c oxidation (Gray et al., 1994). Note that the cbb3-Cox is the only Cox enzyme present in R. capsulatus membranes (Hochkoeppler et al., 1995). Cyt bc1 activity was measured using 40 μM decylbenzoquinol (DBH2) as an electron donor and 50 μM cyt c as an electron acceptor as described elsewhere (Atta-Asafo-Adjei and Daldal, 1991). The reaction was initiated by addition of 50–250 μg of DDM-dispersed chromatophore membrane proteins to the assays mixture and the increase in absorbance at 550 nm due to cyt c reduction was monitored for 1 min. The portion of the initial rate that was inhibited by addition of 150 μM famoxadone was used as the specific cyt bc1 activity.
O2 consumption rates exhibited by R. capsulatus membranes were determined polarographically at 25°C using a Clark-type oxygen electrode (Yellow Springs Instruments, Co) and appropriate electron donors and inhibitors. All assays were carried out using 2 ml of 50 mM MOPS, 5 mM MgCl2, pH 7.2 assay buffer, containing 50–300 μg of DDM-dispersed membrane proteins from cells grown in liquid MPYE enriched medium for ~24 h at 25°C under Res conditions (Valkova-Valchanova et al., 1998). Depending on the segment of the respiratory chain to be assayed, the O2 consumption reaction was initiated by addition of 1 mM NADH (for NADH dehydrogenase), 200 μM DBH2 (for bd-Qox) or 250 μM N,N,N',N'-tetramethyl-p-phenylenediamine (TMPD) together with 10 mM sodium ascorbate (for cbb3-Cox) (Hochkoeppler et al., 1995). For bd-Qox activity measurements, chromatophore membranes were previously incubated with 150 μM famoxadone and 100 μM KCN to inhibit the cyt bc1 and cbb3-Cox activities, respectively. In all assays, the respiratory chain independent O2 consumption activity was determined by using 2 mM KCN, which completely inhibits both cbb3-Cox and bd-Qox dependent O2 reductase activities, and subtracted from the respiratory rates observed. Enzymatic activities were expressed in μmoles of O2 reduced per min, per mg of total protein, considering that air-saturated buffer at 25°C contains 0.237 mM O2. The temperature sensitivity of a chosen respiratory enzyme was tested by incubating chromatophore membranes for 2 h at 35°C prior to O2 consumption assays.
The β-galactosidase activities of strains carrying the ccoN::lacZ were visualized qualitatively using enriched medium plates containing 40 μg/ml of the chromogenic indicator 5-bromo-4-chloro-3-indolyl-β-D-galactopyranoside (X-Gal). The β-galactosidase activities were also assayed at 420 nm using liquid cultures grown in enriched medium at 25°C, with or without addition of 5 μM CuSO4, as described earlier (Khalfaoui-Hassani et al., 2016a). Briefly, bacteria were centrifuged and resuspended in 1 ml of Z-buffer (60 mM Na2HPO4 pH 7.0, 40 mM NaH2PO4, 10 mM KCl, 1 mM MgSO4 and 50 mM β-mercaptoethanol), and their OD600 nm was recorded. The cells were permeabilized using 100 μl of chloroform and 50 μl of 0.1% SDS. The reaction was started by adding 0.2 ml of o-nitrophenyl-β-galactoside (ONPG; 4 mg/ml), incubated at 30°C for 20 min, and stopped by adding 0.5 ml of 1 M Na2CO3. The absorbance at 420 nm resulting from the pigments of R. capsulatus cells was recorded before the addition of ONPG, and subtracted from the final absorbance read at 420 nm at the end of incubation. The β-galactosidase activity was expressed as μmoles of ONPG hydrolyzed per minute per OD600 of cells according to ΔOD420 × 106/4,860/min/OD600 of cells, as done earlier (Khalfaoui-Hassani et al., 2016a).
Chemicals
All chemicals were of highest purity and HPLC spectral grades, and purchased from commercial sources.
Results
R. capsulatus DsbA− Mutants Are Temperature Sensitive for Respiratory Growth
Previously, we had observed that R. capsulatus mutants lacking DsbA were able to grow via Ps, albeit at a slower rate, on both enriched and minimal growth media, but could grow by Res only on minimal, and not on enriched medium, at normal temperature (35°C) (Figure 2A; Deshmukh et al., 2003; Turkarslan et al., 2008). Moreover, the DsbA− mutants reverted readily on enriched medium at 35°C to regain Res growth ability. Proteomic analyses showed that in the absence of DsbA the protease DegP was overproduced, and that the revertants contained mutations that lowered DegP activity (Onder et al., 2008). DegP is usually less abundant and acts as a chaperone at lower temperatures (Spiess et al., 1999). Considering this unusual functional switch, we tested the growth ability of R. capsulatus DsbA− mutant at 25°C in enriched medium. We found that a DsbA− mutant formed colonies at this lower temperature, indicating that the absence of DsbA rendered R. capsulatus cells temperature sensitive for Res growth on enriched medium (ResTs) (Figure 2B, right side and Table 2). Upon microscopic examination of liquid cultures, we also observed that the DsbA− mutants exhibited defective cell division. Cells growing by Res in enriched medium at 25°C stopped dividing when shifted to 35°C, yielding elongated cells that eventually lysed (Figure 2C, left side).
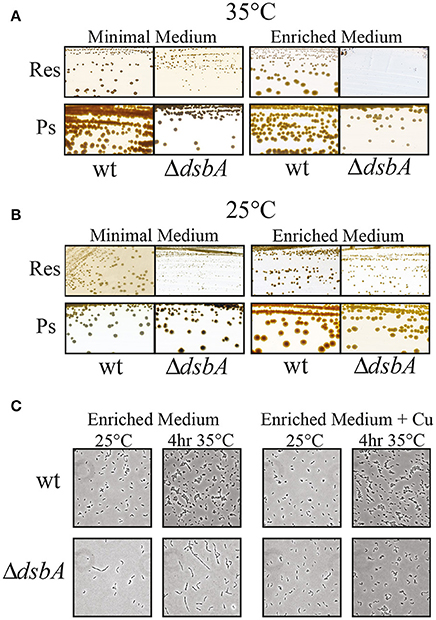
Figure 2. The Res growth of DsbA− mutants is temperature-sensitive. R. capsulatus wild type strain and ΔdsbA mutant grown in minimal (MedA) and in enriched medium (MPYE) at 35°C (A) and 25°C (B). The respiratory growth (Res) of ΔdsbA is defective when the cells are grown at 35°C on enriched medium. DsbA− mutant is still able to grow on minimal medium and under Ps growth conditions, albeit at lower rate than wild type. (C) Microscopic examination of wild type and ΔdsbA mutant cells with or without addition of 5 μM of Cu2+ (+Cu) in the enriched medium. Cell division is severely affected in the ΔdsbA mutant, producing filaments that are longer than the wild type cells. This growth phenotype is suppressed by the addition of Cu2+ into the growth medium.
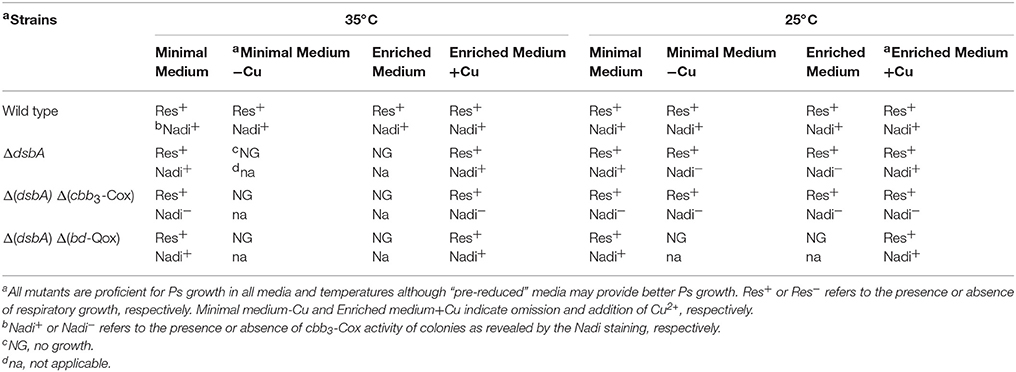
Table 2. Growth phenotypes of R. capsulatus DsbA− single and double mutants in minimal and enriched medium, with or without supplementation with Cu2+, grown at 25 or 35°C.
R. capsulatus Respiratory Chain Is Not Temperature Sensitive in the Absence of DsbA
In order to probe whether the ResTs phenotype of R. capsulatus DsbA− mutants originated from temperature sensitivity of their respiratory chain component(s), we polarographically monitored the O2 consumption rates of wild type and ΔdsbA strains. Cells were grown under Res conditions in liquid enriched medium (MPYE) at 25°C for 24 h and stored at −80°C. Chromatophore membranes were isolated and solubilized with DDM, and the rate of O2 consumption was assayed at 25°C (Materials and Methods). The measurements were repeated using solubilized membranes that were incubated for 2 h at 35°C prior to the assays. NADH oxidase, DBH2 oxidase (bd-Qox, in the presence of a cyt bc1 inhibitor) and TMPD/Ascorbate-dependent O2 reductase (cbb3-Cox) activities were measured using appropriate substrates and inhibitors. The data showed that in such cells the respiratory capabilities of the DsbA− mutant were variable but always lower than those of the wild type strain, and the bd-Qox and cbb3-Cox activities were affected to a greater extent than the NADH dehydrogenase activity (Figure 3). When membranes were incubated at 35°C and then assayed at 25°C, the NADH dehydrogenase and bd-Qox activities decreased similarly in both strains (Figures 3A,B). However, the cbb3-Cox activity remained unchanged both in wild type and DsbA− strains, indicating that this enzyme was not temperature sensitive (Figure 3C). Thus, although the absence of DsbA decreased the overall activities of the respiratory enzymes, it did not render them more temperature sensitive than their wild type counterparts. Hence, the ResTs phenotype of the DsbA− mutant was not correlated with the temperature sensitivity of the respiratory chain components.
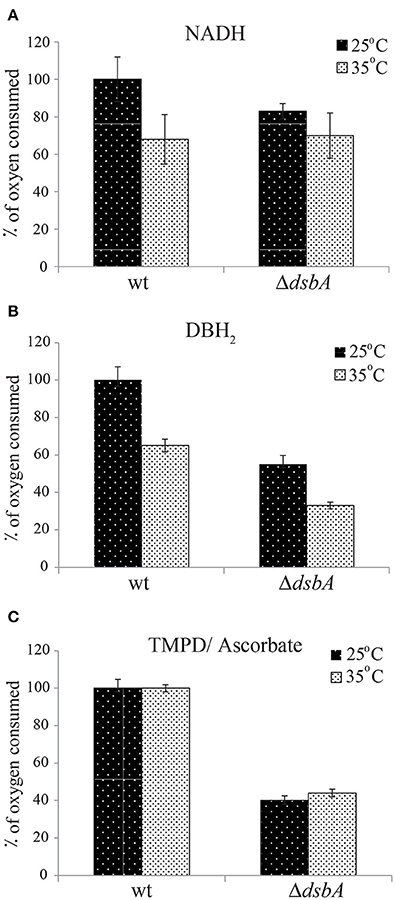
Figure 3. Enzymatic activities of the respiratory complexes are not temperature-sensitive in the absence of DsbA. The rates of oxygen consumption were determined polarographically at 25°C using a Clark-type oxygen electrode, in DDM-solubilized chromatophore membranes from cells grown in liquid enriched medium at 25°C under Res conditions. All assays were carried out using 2 ml of 50 mM MOPS, 5 mM MgCl2, pH 7.2 assay buffer, containing 50–300 μg of DDM-dispersed membrane proteins [protein: detergent (w/w) of 1:1] from wild type and ΔdsbA strains. Depending on the segment of the respiratory chain to be assayed, the O2 consumption reaction was initiated by addition of 1 mM NADH (for NADH dehydrogenase) (A), 200 μM DBH2 (for bd-Qox) (B) or 250 μM N,N,N',N'-tetramethyl-p-phenylenediamine (TMPD) together with 10 mM sodium ascorbate (for cbb3-Cox) (C). The same measurements at 25°C were also repeated after incubation of the membranes at 35°C for 2 h (gray bars) to assess the temperature response of the activities. The rates were determined as μmoles of O2 reduced/min/mg total protein, and are shown as % of oxygen consumed taking as 100% the rate of the wild type strain. Average values of at least 3 independent measurements are shown. Error bars represent the range of values (maximum and minimum) obtained for each condition.
Respiratory Growth at 25°C of a DsbA− Mutant Is Mediated by bd-Qox Enzyme
We further examined the Res growth pathways of the DsbA− mutant at 25°C in enriched medium, to assess whether growth was sustained either by the cbb3-Cox, or bd-Qox, or both of these terminal oxidases (Figure 1). R. capsulatus double mutants lacking both DsbA and either of the cbb3-Cox or the bd-Qox were constructed under the permissive growth conditions (Materials and Methods). As expected, neither strain could grow on enriched medium at 35°C, confirming their DsbA− phenotypes. However, the DsbA− cbb3-Cox− double mutant was able to grow at 25°C on both enriched and minimal media, whereas the DsbA− bd-Qox− double mutant could do so only on minimal, but not on enriched medium (Table 2). Thus, the bd-Qox activity sustained the Res growth on enriched medium at 25°C, indicating that in the absence of DsbA, the cbb3-Cox activity became defective even under growth permissive conditions. Together with the temperature insensitivity of the bd-Qox activity described above, this finding implied that the Res growth defect observed in the DsbA− mutant was not due to a defective bd-Qox activity.
Redox Active Chemicals Palliate Growth and cbb3-Cox Defects of DsbA− Mutants
We observed frequently that the R. capsulatus DsbA− mutants that were grown on minimal medium showed partial growth at 35°C when transferred to enriched medium. However, this growth ability was not sustained for subsequent cultures in the enriched medium, suggesting that carryover of a chemical(s) beneficial to growth, from the minimal to the enriched medium might be responsible for these observations. Systematic supplementation of the enriched medium with the constituents of the minimal medium, as well as omission of these constituents from the minimal medium, identified Cu2+ as the culprit. Indeed, addition of 5 μM Cu2+ to the enriched medium allowed normal cell division (Figure 2C, right side, bottom row), restoring growth and cbb3-Cox defects at 35°C. Similarly, omission of Cu2+ from the minimal medium (normally containing 1.5 μM Cu2+) rendered the DsbA− mutants ResTs at 35°C (Figure 4A), and addition of Cu2+ to enriched medium rendered it Nadi+ at 25°C (Figure 4B). Interestingly, addition of 10 mM of the Cu chelator bathocuproine disulfonate (BCS) to the minimal medium also rendered wild type cells Nadi− without affecting their growth ability at 25°C, thus mimicking the phenotype of a DsbA− mutant (Figure 4C). These findings suggested that in the absence of DsbA, Cu2+ incorporation into cbb3-Cox might be defective.
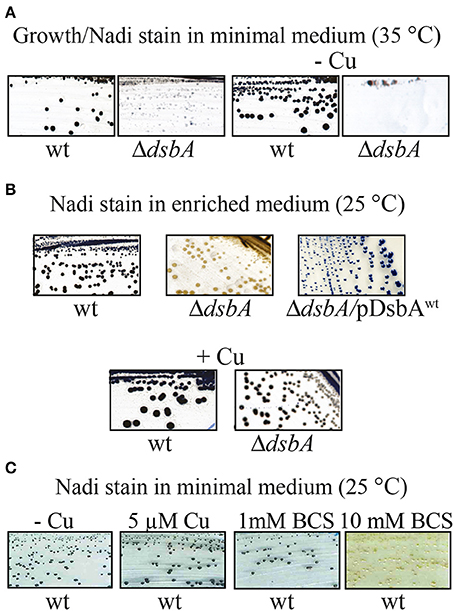
Figure 4. The NADI phenotype of a DsbA− mutant in R. capsulatus. (A) Wild type and ΔdsbA mutant cells grown on minimal medium (MedA) at 35°C. The ΔdsbA grows at lower rate than wild type, and when Cu2+ is omitted from the minimal medium (-Cu) the ΔdsbA mutant does not grow. Both wild type and ΔdsbA mutant cells become blue after Nadi staining. (B) Nadi staining of wild type and ΔdsbA mutant in enriched medium (MPYE) at 25°C. The ΔdsbA mutant does not show any NADI staining and this phenotype is suppressed by addition of Cu2+. Complementation of the ΔdsbA mutant with wild type dsbA restores Nadi staining, indicating restored production of cbb3-Cox. (C) Nadi staining of wild type cells grown on minimal medium without Cu2+ (-Cu), with addition of 5 μM of Cu2+, or with addition of 1 mM or 10 mM of the Cu chelator bathocuproine disulfonate (BCS). A wild type strain becomes Nadi− (no blue stain), like the ΔdsbA or Δcbb3-Cox mutants, when Cu2+ in the medium is chelated by BCS.
Suppression of growth and cbb3-Cox defects was specific to Cu2+ ions only, as no other component of the minimal medium, including several other transition metal ions (e.g., Fe3+, Ni2+, and Mn2+) could remedy these defects (data not shown). Titration of the Cu2+ amount added to the enriched medium (between 0 and 500 μM) or the minimal medium (0–10 μM) at 25 and 35°C showed that higher amounts of Cu2+ were required in enriched medium compared with the minimal medium to suppress the DsbA− mutant growth and cbb3-Cox defects (Table 3). These observations were in accordance with the restricted bio-availability of Cu2+ in enriched medium (MPYE) containing yeast extract (rich in metal chelators), as previously reported (Park et al., 1998). Interestingly, while addition of lower amounts of Cu2+ in both media was sufficient to rescue growth at 35°C, higher amounts were needed to restore the cbb3-Cox activity (i.e., Nadi+) (Table 3). Similarly, when enriched medium was supplemented with a mixture of cysteine/cystine, lower concentrations allowed cell growth but higher concentrations were needed to rescue the Nadi phenotype (Table 4). We also noted that, like the E. coli DsbA− mutants (Hiniker et al., 2005), the R. capsulatus DsbA− mutants were more sensitive to Cu2+ than other strains (Table 3).
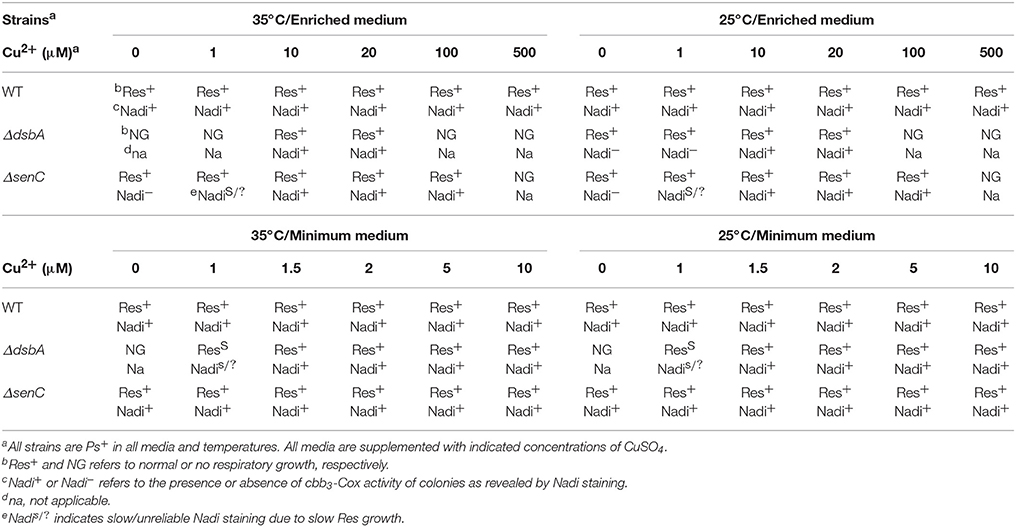
Table 3. Respiratory growth and Nadi phenotypes of various R. capsulatus strains grown in minimal or enriched media at 25 or 35°C supplemented with increasing Cu2+ concentrations.
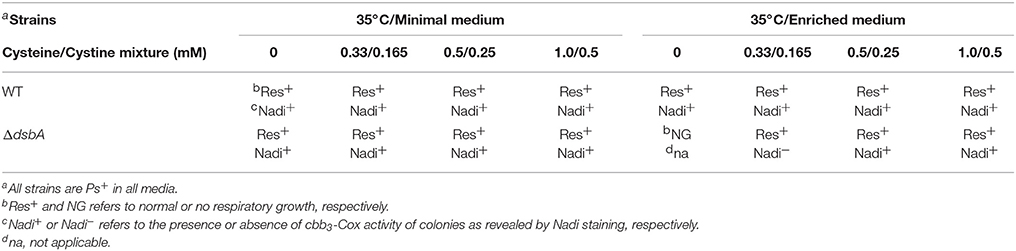
Table 4. Res growth and Nadi phenotypes of various R. capsulatus strains grown in minimal or enriched media at 35°C supplemented with increasing concentrations (mM) of a mixture of cysteine/cystine.
When tested immediately after growth in agar containing (i.e., solid) enriched medium (MPYE) at 25°C without Cu2+ supplementation, the DsbA− mutants showed very low cbb3-Cox activity (i.e., Nadi−) (Figure 2B, Table 5), unlike the cells grown in liquid medium (Figure 3 and Table 5). However, upon storage of plates at room temperature and exposed to air, or after growth in liquid medium they regained partially cbb3-Cox activity (Figure 3 and Table 5). The molecular basis of this difference was not investigated, but it might be caused by “phenotypic and/or genotypic” reversion of the DsbA− mutants in liquid cultures (Onder et al., 2008). The amount of oxygen present in shaken liquid cultures grown under Res conditions might partially alleviate the oxidative defect due to the absence of DsbA. Conversely, the presence of agar on solid media might exacerbate this defect. Indeed, complementation of the DsbA− mutant with wild type DsbA (pDsbAwt), or supplementation of the enriched medium with Cu2+ (Figure 4B) or cysteine/cystine mixture (data not shown) acting as chemical oxidants allowed growth and mitigated the cbb3-Cox defect at 35°C (i.e., Nadi+). The DsbA− mutants grown at 25°C in enriched medium in the presence of ~0.66 mM cysteine and 0.33 mM cystine or 5–10 μM Cu2+ contained higher cbb3-Cox activity than those grown without any supplement, which relied uniquely on oxygen to compensate for the absence of DsbA (Table 5). We also noted that the DsbA− mutants had lower cyt bc1 activity under Res growth conditions, despite their ability to grow by Ps under anaerobiosis, and this defect was also palliated by addition of chemical oxidants (Table 5). Thus, the DsbA− mutants exhibited a general redox imbalance that impaired multiple cellular functions, including its respiratory activities and cell division.
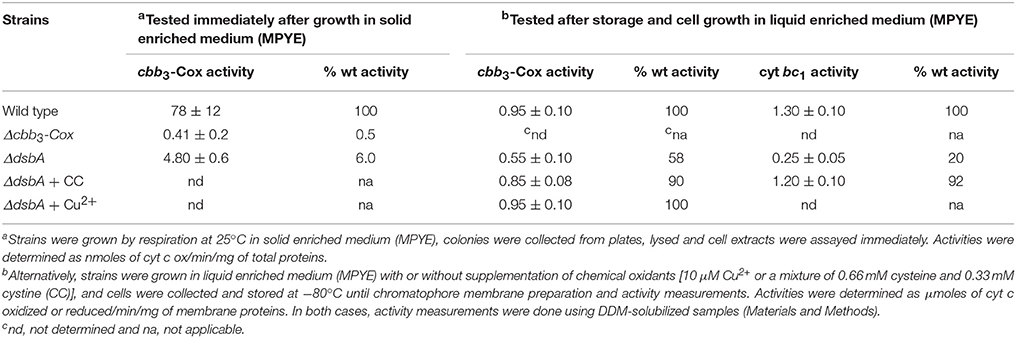
Table 5. Cyt c: oxygen oxidoreductase activity of cbb3-Cox and DBH2: cyt c oxidoreductase activity of cyt bc1 of R. capsulatus wild type, cbb3-Cox− and DsbA− strains.
Mutants Lacking DsbA Have Lower Amounts of the cbb3-Cox Subunits
Given that Cu2+ supplementation of a DsbA− mutant increased its cbb3-Cox activity, and that chelation by BCS eliminated this activity in a wild type strain, we inquired whether Cu2+ affected the expression of the ccoNOQP operon, encoding the structural genes of cbb3-Cox. A transcriptional-translational ccoN::lacZ fusion, containing the promoter region of ccoNOQP and the first eight amino acids of CcoN (Koch et al., 2000) was introduced into the wild type and DsbA− strains. At 25°C, these strains formed colonies of comparable blue colors on 5-bromo-4-chloro-3-indoyl β-D-galactopyranoside (X-gal) containing enriched medium plates (data not shown). Similarly, in liquid cultures they produced comparable amounts of β-galactosidase activities in the absence or presence of 5 μM Cu2+ (Table 6). Thus, neither the addition of Cu2+, nor the absence of DsbA significantly affected ccoNOQP expression, suggesting that the lack of cbb3-Cox activity was not due to impaired transcription or initiation of translation of its CcoN subunit.
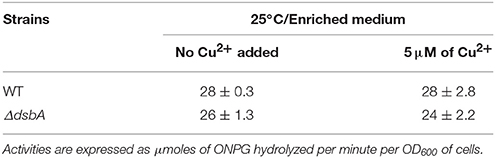
Table 6. β-galactosidase activity of R. capsulatus wild type and DsbA− strains determined in cell cultures, grown by respiration at 25°C in enriched medium (MPYE) in the presence or absence of 5 μM of Cu2+.
In order to further investigate why the production of an active cbb3-Cox was defective in the absence of DsbA, chromatophore membranes of cells grown at 25°C in enriched medium (MPYE) were analyzed by native BN-PAGE and by denaturing SDS-PAGE. Activity staining of native gels using the Nadi reaction for cbb3-Cox revealed an intense band of ~230 kDa, corresponding to cbb3-Cox activity in the wild type and in the cbb3-Cox− mutant complemented with a plasmid carrying wild type ccoNOQP genes (Δcox/pCox) (Kulajta et al., 2006), but not in the cbb3-Cox− (Δcox) or DsbA− mutants (Figure 5A). Similarly, denaturing gels followed by TMBZ staining showed in wild type membranes four c-type cyts, cp, c1, cy, and co (Figure 5B). In the absence of DsbA, the amounts of the cyts c1, cy, cp, and co decreased drastically in freshly grown cells to 40, 18, 10, and 8% of the wild type levels, respectively, as indicated by semi-quantitative analysis using ImageJ software. Lastly, immunoblot analysis using R. capsulatus anti-CcoN polyclonal antibodies showed that the steady-state level of the catalytic subunit CcoN (with a CuB center and no c-type cyts) was also very low in a DsbA− mutant (about 15% of the wild type) (Figure 5C), similar to the other subunits of cbb3-Cox. Upon complementation of the DsbA− mutant with a plasmid carrying wild type DsbA (ΔdsbA/pDsbA), the levels of CcoN increased to wild type levels (Figure 5C). While we anticipated decreased amounts of the c-type cyts in the absence of DsbA, due to its role during Ccm (Deshmukh et al., 2003; Turkarslan et al., 2008), finding a decrease in the Cu containing CcoN, which is not a c-type cyt, was unexpected. Considering that CcoN alone is highly stable in the absence of its c-type cyt partners (Ekici et al., 2013), provided that CcoQ is present (Peters et al., 2008), these observations further suggested that R. capsulatus DsbA− mutants were defective in Cu2+ incorporation into cbb3-Cox. The very low levels of this enzyme were then a consequence of the overproduction of DegP, as indicated by the nature of the suppressor mutations that lowered this protease activity (Onder et al., 2008).
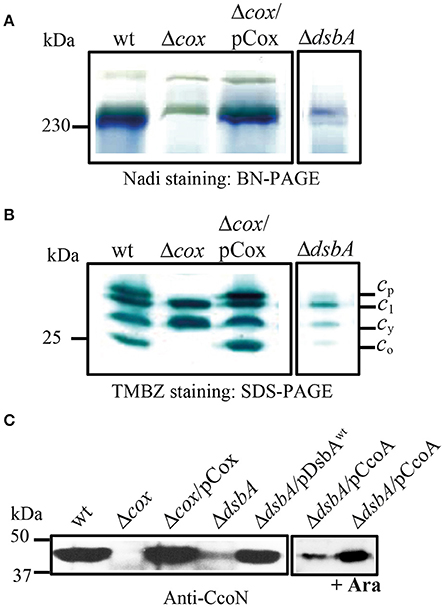
Figure 5. DsbA is required for cbb3-Cox activity and maturation/assembly of its subunits. (A) Detection of active cbb3-Cox by BN-PAGE stained with NADI in chromatophore membranes prepared from the wild type (wt), Δcbb3-Cox (Δcox), Δcbb3-Cox overproducing cbb3-Cox (Δcox/pCox) and ΔdsbA. The active 230 kDa band corresponding to cbb3-Cox complex is present in the wild type and Δcox/pCox strains. In the absence of DsbA, activity of the cbb3-Cox is severely affected. (B) Chromatophore membranes were separated by SDS-PAGE and stained by TMBZ for detection of the c-type cyts. The wild type and Δcox/pCox showed cyt c1 of cyt bc1 complex, the electron carrier cy, and the cyts cp and co subunits of cbb3-Cox, which are absent in Δcbb3-Cox. The levels of all c-type cyts c are decreased in ΔdsbA due to its effect on the Ccm process, and the cyts cp and co subunits of cbb3-Cox were present at ~10 and 8% of the wild type levels, respectively. (C) Immunoblot analysis of chromatophore membranes separated by SDS-PAGE using polyclonal antibodies against the CcoN subunit of cbb3-Cox. The amount of CcoN is severely reduced in the ΔdsbA mutant to ~15% of wild type levels, but it increases by roughly three folds when CcoA is overproduced upon induction with L-arabinose.
DsbA− and Several cbb3-Cox Biogenesis Mutants Exhibit Similar Phenotypes
We noticed that the cbb3-Cox related properties of the DsbA− mutants were reminiscent of some of the cbb3-Cox biogenesis mutants such as those lacking the Cu importer CcoA (Ekici et al., 2012b) or the Cu chaperones SenC and PccA (Lohmeyer et al., 2012; Trasnea et al., 2016; Table 3). Earlier studies indicated that CcoA, PccA, and SenC are required for Cu incorporation into cbb3-Cox at low Cu availability, and mutants lacking these components had small amounts of cbb3-Cox, which could be restored by supplementation of the growth medium with Cu2+ (Ekici et al., 2012a). The Cu chaperone PccA has no cysteine residues, but SenC which is homologous to the mitochondrial Sco proteins involved in Cox biogenesis, contains two cysteines required for Cu binding (Thompson et al., 2012; Trasnea et al., 2016). Similarly, CcoA also has several cysteine residues and at least one is critical for its function (unpublished data). These resemblances led us to probe for possible functional link(s) between DsbA and CcoA or SenC, provided that these proteins could be substrates of DsbA. We constructed the pDsbA+/SenC− and pSenC+/DsbA− as well as the pDsbA+/CcoA− and pCcoA+/DsbA− (pBK69/MD20) merodiploids that carried multiple copies of wild type dsbA, senC or ccoA alleles (Table 1). Indeed, no functional complementation was seen between DsbA and SenC or DsbA and CcoA with respect to the ResTs phenotype, consistent with the ResTs defect being unrelated to cbb3-Cox biogenesis. Interestingly, although no complementation was observed between SenC and DsbA (pSenC/MD20 or pDsbA/LS01), in respect to the Nadi− phenotype at 25°C in enriched medium, a partial complementation was detected when CcoA was overproduced in the DsbA− mutant upon L-Ara induction of wild type ccoA carried by a plasmid (pBK69/MD20) (Khalfaoui-Hassani et al., 2016a) (i.e., the Nadi phenotype became NadiS) (Table 1). Considering that CcoA is important for Cu incorporation into the CcoN subunit of cbb3-Cox, immunoblot analyses were carried out using CcoN antibodies. The data showed that, the overproduction of CcoA as a result of arabinose induction in the DsbA− mutant increased about three times the amount of CcoN in the membranes (as determined using ImageJ software) consistent with the enhanced Nadi staining of this merodiploid strain (Figure 5C). This finding implied that the cbb3-Cox defect was at least partly due to a shortage of active CcoA and compromised Cu incorporation into its catalytic site. Thus, DsbA has a hitherto unknown role during cbb3-Cox biogenesis, especially under restricted Cu availability conditions.
Discussion
Previously, we have shown that R. capsulatus DsbA− mutants do not grow in enriched medium (MPYE) by Res although they are proficient for Ps growth (Deshmukh et al., 2003). We investigated the properties of such mutants, and found that their growth defects were linked to cellular redox imbalance and resulting defective oxidative protein folding, which led to decreased c-type cyt maturation and impaired cbb3-Cox biogenesis (Figure 6A, in red). Improperly folded proteins are targets to the periplasmic protease DegP, which is overproduced in the DsbA− mutant. At 35°C, cell division in a DsbA− mutant stopped, yielding elongated cells that eventually lysed. The observation that R. capsulatus cells could grow at 35°C in minimal medium, but not in enriched medium, led us to find that exposure to oxygen and to redox-active chemicals (e.g., cysteine/cystine mixture or Cu2+ ions) restored the redox imbalance due to the absence of DsbA (Figure 6B, in blue). Supplementation of the growth medium with Cu2+ enhanced disulfide bond formation in DsbA− mutants. However, since the chemically catalyzed process is less specific than the DsbA-catalyzed disulfide bond formation, cells relied on the disulfide bond isomerase DsbC for survival (Hiniker et al., 2005), and became more Cu2+ sensitive. Additionally, lowering the growth temperature (from 35 to 25°C) also alleviated the growth, but not the cbb3-Cox, defect of the R. capsulatus DsbA− mutant (Figure 6B, in green). The ability to grow at low temperature indicated that decreased DegP activity and oxygen mediated basal disulfide bond formation were sufficient for Res growth via the bd-Qox pathway. Accordingly, addition of small amounts of redox chemicals was sufficient to rescue the growth defect, but higher amounts were needed to observe wild type-like cbb3-Cox biogenesis.
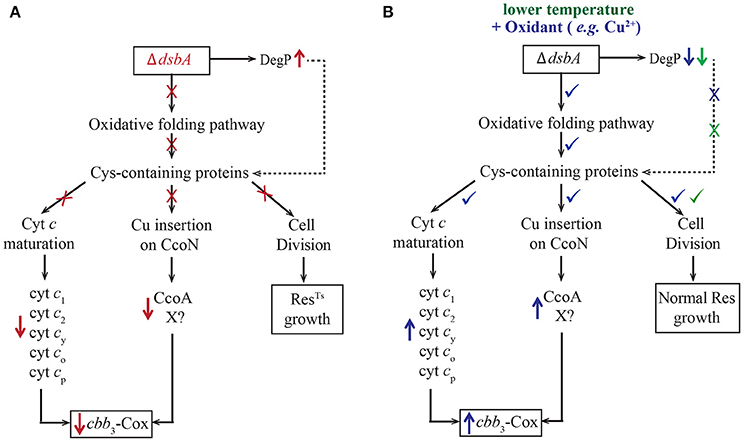
Figure 6. Absence of DsbA has multiple effects on R. capsulatus respiratory growth. (A) In the absence of DsbA (shown in red), the oxidative folding pathway is defective, causing improper folding of many Cys-containing proteins. These proteins are important for many cellular functions ranging from cyt c maturation (Ccm) (left), Cu incorporation into CcoN (middle) and cell division (right) that become defective (marked with ×). In addition, in the absence of DsbA, the periplasmic protease DegP is overproduced (marked with ↑), and degrades rapidly misfolded DsbA substrates. The impairment of cell division leads to the ResTS growth phenotype (right). The defect on Ccm results in lower steady-state amounts of the c-type cyts (c1, c2, cy, co, cp) and their complexes, including the cyt bc1 and cbb3-Cox (left) (marked with ↓). Other Cys-containing proteins, such as CcoA and hypothetical components (X) involved in Cu incorporation into the catalytic subunit CcoN, also become targets of DegP, further decreasing cbb3-Cox production (marked with ↓) (middle). (B) Lowering the growth temperature (shown in green) decreases the protease activity of DegP (marked with ↓), improving cell division (marked with ✓), but not cbb3-Cox biogenesis. However, addition of a chemical oxidant, such as Cu2+ or a mixture of cysteine/cysteine (shown in blue), repairs the redox imbalance due to the absence of DsbA, decreases the levels of DegP (marked with ↓) and improves folding of Cys-containing proteins (marked with ✓). This in turn allows growth at normal temperature (35°C) (right), efficient Ccm (left) and enhances cbb3-Cox biogenesis (marked with ✓) (middle). In addition, use of high concentrations of Cu2+ also activates the low affinity Cu uptake pathway, which facilitates Cu insertion into the CcoN subunit (not shown).
Remarkably, the growth defects associated with a DsbA− mutant of R. capsulatus (a soil/aquatic organism) were not identical to those observed with an analogous E. coli (an enteric organism) mutant. The DsbA− mutants of both species exhibited cell division defects under aerobic Res conditions where residual protein disulfide bond formation presumably relied on oxygen (Meehan et al., 2017b). Recently, it was shown that the E. coli cell division protein FtsN, which has a structural disulfide bond, is rapidly degraded in the absence of DsbA, leading to cell filamentation (Meehan et al., 2017b). FtsN is present only in a few bacterial species, and is absent in R. capsulatus (https://biocyc.org), although it is plausible that a functional analog might exist in this organism. Furthermore, an E. coli DsbA− mutant is unable to grow under anaerobic conditions, possibly due to defective outer membrane biosynthesis (Meehan et al., 2017b) and deficient cyt c maturation (Metheringham et al., 1995). In contrast, the R. capsulatus DsbA− mutant is proficient for anaerobic growth in the presence of light (i.e., photosynthesis) (Deshmukh et al., 2003). Indeed, the two species have different anaerobic growth modes, while E. coli relies mainly on glycolysis, R. capsulatus performs anoxygenic photosynthesis (Hunter et al., 2009).
Perhaps the most salient finding of this work is the decrease of the respiratory capacity observed in a mutant lacking DsbA (Figure 6A). In particular, the cyt bc1 and cbb3-Cox activities were much lower in these cells that grew by Res via the bd-Qox enzyme. It is known that DsbA is not essential for Ccm, but its absence decreases the steady-state levels of c-type cyts by ~50% (Turkarslan et al., 2008). Occurrence of a thio-redox loop (oxidation of an apocyt c by DsbA followed by its reduction by CcmG) might protect the unfolded apocyt c against degradation via the holdase role of CcmG (Turkarslan et al., 2008). Our recent work showed that CcmG interacts with CcmH and other Ccm components involved in heme ligation. In a DsbA− mutant, CcmH that is responsible for the stereo-specificity of heme ligation may not be rapidly oxidized. This would compromise the formation of correct thioether bonds, leading to degradation of non-native cyts c (Verissimo et al., 2017). In addition to the lower efficiency of the Ccm process, we also note that two of the cyt bc1 subunits, the FeS protein (Valkova-Valchanova et al., 1998) and the cyt c1 (Gray et al., 1992) contain disulfide bonds that are essential for their stability and function. Thus, the lower cyt bc1 activity found in a DsbA− mutant could be due in part to incomplete formation of these structural disulfide bonds. Although two of the cyt cbb3-Cox subunits are c-type cyts, they do not contain any structural disulfide bond (Koch et al., 2000). Thus, the decreased Ccm efficiency was not sufficient to rationalize why cbb3-Cox was quasi-absent in a DsbA− mutant. Moreover, it was unclear why the CcoN subunit of cbb3-Cox, which is matured independently of the Ccm process, and stable in the absence of the cyts co and cp subunits (Ekici et al., 2013), was barely detectable in the absence of DsbA. In addition, we did not observe in native gels the presence of a fully-assembled, but inactive cyt cbb3-Cox, in contrast to what was observed in cbb3-Cox biogenesis mutants lacking CcoS (Koch et al., 2000; Kulajta et al., 2006). These observations, combined with the unaffected expression of the ccoNOQP cluster and the remedial of cbb3-Cox defect by decreased DegP activity (Onder et al., 2008) implied that the cbb3-Cox subunits were absent in a DsbA− mutant due to their rapid degradation (Figure 6A). Our earlier comparative proteomics study showed that R. capsulatus cells lacking DsbA contained lower amounts of several multi-cysteine containing periplasmic proteins compared to wild type, suggesting that without the formation of their critical disulfide bonds, these proteins remain unfolded, leading to their elimination via increased DegP activity (Onder et al., 2008). While some of these DsbA-dependent proteins are important for essential cellular functions such as osmo-protection, outer membrane synthesis or lipid biosynthesis (Onder et al., 2008), conceivably, other yet to be identified components (X) might be involved in cbb3-Cox biogenesis (Figure 6A).
The presence of small quantities of CcoN in membranes is a common characteristic of cbb3-Cox biogenesis mutants that are defective for incorporation of Cu into cbb3-Cox at low Cu2+ availability (Ekici et al., 2012a; Khalfaoui-Hassani et al., 2016b). Cells lacking the MFS-type Cu importer CcoA and the periplasmic Cu chaperones SenC and PccA (Koch et al., 2000; Lohmeyer et al., 2012; Ekici et al., 2014; Trasnea et al., 2016) contain very low levels of cbb3-Cox subunits and activity, and like the DsbA− mutants (Hiniker et al., 2005), are phenotypically rescued by addition of Cu2+. Aside from PccA, both SenC (Lohmeyer et al., 2012) and CcoA contain Cys residues required for their activity (unpublished data), possibly forming structurally or functionally important disulfide bonds. In the absence of DsbA, the active forms of these, and probably other, proteins may be quasi-absent, leading to impaired Cu incorporation into cbb3-Cox especially at low Cu2+ bioavailability (Figure 6A). Consistent with this possibility is the fact that a wild type strain of R. capsulatus also becomes impaired in cbb3-Cox biogenesis when grown in the presence of BCS, which restricts Cu availability.
How Cu is incorporated into the CcoN subunit of cbb3-Cox is not well understood. A high affinity Cu uptake pathway involving at least CcoA and SenC, and a CcoA-independent low affinity Cu uptake pathway have been described (Ekici et al., 2012a). Our data suggests that, in the absence of DsbA, the naturally bioavailable Cu2+ present in enriched medium is not efficiently incorporated into the cbb3-Cox, indicating that the CcoA-dependent high affinity (i.e., nM Cu) pathway including CcoA, is compromised even at growth-permissive low temperature. Supplementation of the growth medium with a redox active chemical (e.g., cysteine/cystine mixture) restores this high affinity Cu uptake pathway. On the other hand, addition of high concentrations (~ μM) of Cu2+ activates the low affinity (μM) CcoA-independent Cu uptake pathway, allowing Cu2+ incorporation into cbb3-Cox (Ekici et al., 2014; Figure 6B). Conceivably, besides CcoA, other component(s) (X) important for cbb3-Cox biogenesis may also be compromised in the absence of DsbA.
As summarized in Figure 6, this work showed that the respiratory chain of R. capsulatus becomes deficient in the absence of DsbA due to multiple defects, including decreased Ccm efficiency and impaired Cu incorporation into the active site of cbb3-Cox via the CcoA-dependent high affinity Cu uptake pathway. This biogenesis defect could be alleviated either by lowering DegP protease activity, or by providing chemical oxidants that restore the high affinity Cu uptake pathway, or by supplying high amounts of Cu2+ that activates a low affinity CcoA-independent Cu uptake pathway. Future studies will hopefully identify the R. capsulatus DsbA-dependent biogenesis component(s) that functions during the incorporation of Cu to cbb3-Cox.
Author Contributions
All authors have given approval to the final version of the manuscript. OO designed and performed experiments and analyzed data. AV designed and performed experiments, analyzed data and wrote the manuscript. BK-H and AP performed experiments. H-GK did critical revision of the manuscript. FD managed the project, supervised the study and wrote the manuscript.
Funding
This work is supported partly (proteins and respiratory activity characterizations) by the Division of Chemical Sciences, Geosciences and Biosciences, Office of Basic Energy Sciences of Department of Energy [DOE DE-FG02-91ER20052] to FD, and partly (mutant construction and characterization) by the National Institute of Health [NIH GM 38237] to FD. Supports of the Deutsche Forschungsgemeinschaft to H-GK (IRTG 1478 and RTG 2202) are gratefully acknowledged.
Conflict of Interest Statement
The authors declare that the research was conducted in the absence of any commercial or financial relationships that could be construed as a potential conflict of interest.
Acknowledgments
We thank Dr. C. Khatchikian and the Core facilities of Border Biomedical Research Center (BBRC) at the University of Texas at El Paso for use of equipment.
Abbreviations
MPYE, Mineral-peptone-yeast extract enriched medium; MedA, Sistrom's minimal medium A; Res, Respiration; Ps, Photosynthesis; cyt c, cytochrome c; cbb3-Cox, cbb3-type cytochrome c oxidase; bd-Qox, bd-type quinol oxidase; NADI, a staining procedure for cytochrome c oxidase activity in colonies; GTA, gene transfer agent; Xgal, 5-bromo-4-chloro-3-indolyl-β-D-galactopyranoside; ONPG, o-nitrophenyl-β-galactoside; DBH2, decylbenzoquinol; PMFS, phenyl methyl sulfonyl fluoride; AEBSF, 4-(2-Aminoethyl) benzenesulfonyl fluoride hydrochloride.
References
Atta-Asafo-Adjei, E., and Daldal, F. (1991). Size of the amino acid side chain at position 158 of cytochrome b is critical for an active cytochrome bc1 complex and for photosynthetic growth of Rhodobacter capsulatus. Proc. Natl. Acad. Sci. U.S.A. 88, 492–496. doi: 10.1073/pnas.88.2.492
Aygun-Sunar, S., Mandaci, S., Koch, H. G., Murray, I. V., Goldfine, H., and Daldal, F. (2006). Ornithine lipid is required for optimal steady-state amounts of c-type cytochromes in Rhodobacter capsulatus. Mol. Microbiol. 61, 418–435. doi: 10.1111/j.1365-2958.2006.05253.x
Buschmann, S., Warkentin, E., Xie, H., Langer, J. D., Ermler, U., and Michel, H. (2010). The structure of cbb3 cytochrome oxidase provides insights into proton pumping. Science 329, 327–330. doi: 10.1126/science.1187303
Daldal, F., Cheng, S., Applebaum, J., Davidson, E., and Prince, R. C. (1986). Cytochrome c2 is not essential for photosynthetic growth of Rhodopseudomonas capsulata. Proc. Natl. Acad. Sci. U.S.A. 83, 2012–2016. doi: 10.1073/pnas.83.7.2012
Daldal, F., Mandaci, S., Winterstein, C., Myllykallio, H., Duyck, K., and Zannoni, D. (2001). Mobile cytochrome c2 and membrane-anchored cytochrome cy are both efficient electron donors to the cbb3- and aa3-type cytochrome c oxidases during respiratory growth of Rhodobacter sphaeroides. J. Bacteriol. 183, 2013–2024. doi: 10.1128/JB.183.6.2013-2024.2001
Darrouzet, E., Valkova-Valchanova, M., and Daldal, F. (2002). The [2Fe-2S] cluster E(m) as an indicator of the iron-sulfur subunit position in the ubihydroquinone oxidation site of the cytochrome bc1 complex. J. Biol. Chem. 277, 3464–3470. doi: 10.1074/jbc.M107973200
Deshmukh, M., Turkarslan, S., Astor, D., Valkova-Valchanova, M., and Daldal, F. (2003). The dithiol:disulfide oxidoreductases DsbA and DsbB of Rhodobacter capsulatus are not directly involved in cytochrome c biogenesis, but their inactivation restores the cytochrome c biogenesis defect of CcdA-null mutants. J. Bacteriol. 185, 3361–3372. doi: 10.1128/JB.185.11.3361-3372.2003
Ditta, G., Schmidhauser, T., Yakobson, E., Lu, P., Liang, X. W., Finlay, D. R., et al. (1985). Plasmids related to the broad host range vector, pRK290, useful for gene cloning and for monitoring gene expression. Plasmid 13, 149–153. doi: 10.1016/0147-619X(85)90068-X
Ekici, S., Jiang, X., Koch, H. G., and Daldal, F. (2013). Missense mutations in cytochrome c maturation genes provide new insights into Rhodobacter capsulatus cbb3-type cytochrome c oxidase biogenesis. J. Bacteriol. 195, 261–269. doi: 10.1128/JB.01415-12
Ekici, S., Pawlik, G., Lohmeyer, E., Koch, H. G., and Daldal, F. (2012a). Biogenesis of cbb3-type cytochrome c oxidase in Rhodobacter capsulatus. Biochim. Biophys. Acta 1817, 898–910. doi: 10.1016/j.bbabio.2011.10.011
Ekici, S., Turkarslan, S., Pawlik, G., Dancis, A., Baliga, N. S., Koch, H. G., et al. (2014). Intracytoplasmic copper homeostasis controls cytochrome c oxidase production. MBio 5:e01055-13. doi: 10.1128/mBio.01055-13
Ekici, S., Yang, H., Koch, H. G., and Daldal, F. (2012b). Novel transporter required for biogenesis of cbb3-type cytochrome c oxidase in Rhodobacter capsulatus. MBio 3:e00293-11. doi: 10.1128/mBio.00293-11
Gao, T., and O'Brian, M. R. (2007). Control of DegP-dependent degradation of c-type cytochromes by heme and the cytochrome c maturation system in Escherichia coli. J. Bacteriol. 189, 6253–6259. doi: 10.1128/JB.00656-07
García-Horsman, J. A., Barquera, B., Rumbley, J., Ma, J., and Gennis, R. B. (1994). The superfamily of heme-copper respiratory oxidases. J. Bacteriol. 176, 5587–5600. doi: 10.1128/jb.176.18.5587-5600.1994
Giuffrè, A., Borisov, V. B., Arese, M., Sarti, P., and Forte, E. (2014). Cytochrome bd oxidase and bacterial tolerance to oxidative and nitrosative stress. Biochim. Biophys. Acta 1837, 1178–1187. doi: 10.1016/j.bbabio.2014.01.016
Gray, K. A., Davidson, E., and Daldal, F. (1992). Mutagenesis of methionine-183 drastically affects the physicochemical properties of cytochrome c1 of the bc1 complex of Rhodobacter capsulatus. Biochemistry 31, 11864–11873. doi: 10.1021/bi00162a027
Gray, K. A., Grooms, M., Myllykallio, H., Moomaw, C., Slaughter, C., and Daldal, F. (1994). Rhodobacter capsulatus contains a novel cb-type cytochrome c oxidase without a CuA center. Biochemistry 33, 3120–3127. doi: 10.1021/bi00176a047
Han, H., Hemp, J., Pace, L. A., Ouyang, H., Ganesan, K., Roh, J. H., et al. (2011). Adaptation of aerobic respiration to low O2 environments. Proc. Natl. Acad. Sci. U.S.A. 108, 14109–14114. doi: 10.1073/pnas.1018958108
Hassani, B. K., Steunou, A. S., Liotenberg, S., Reiss-Husson, F., Astier, C., and Ouchane, S. (2010). Adaptation to oxygen: role of terminal oxidases in photosynthesis initiation in the purple photosynthetic bacterium, Rubrivivax gelatinosus. J. Biol. Chem. 285, 19891–19899. doi: 10.1074/jbc.M109.086066
Hiniker, A., Collet, J. F., and Bardwell, J. C. (2005). Copper stress causes an in vivo requirement for the Escherichia coli disulfide isomerase DsbC. J. Biol. Chem. 280, 33785–33791. doi: 10.1074/jbc.M505742200
Hochkoeppler, A., Jenney, F. E. Jr., Lang, S. E., Zannoni, D., and Daldal, F. (1995). Membrane-associated cytochrome cy of Rhodobacter capsulatus is an electron carrier from the cytochrome bc1 complex to the cytochrome c oxidase during respiration. J. Bacteriol. 177, 608–613. doi: 10.1128/jb.177.3.608-613.1995
Hunter, C. N., Daldal, F., Thurnauer, M. C., and Beatty, J. T. (2009). The Purple Phototrophic Bacteria. Dordrecht: Springer.
Inaba, K., and Ito, K. (2008). Structure and mechanisms of the DsbB-DsbA disulfide bond generation machine. Biochim. Biophys. Acta 1783, 520–529. doi: 10.1016/j.bbamcr.2007.11.006
Jenney, F. E. Jr., Prince, R. C., and Daldal, F. (1994). Roles of the soluble cytochrome c2 and membrane-associated cytochrome cy of Rhodobacter capsulatus in photosynthetic electron transfer. Biochemistry 33, 2496–2502. doi: 10.1021/bi00175a019
Keen, N. T., Tamaki, S., Kobayashi, D., and Trollinger, D. (1988). Improved broad-host-range plasmids for DNA cloning in gram-negative bacteria. Gene 70, 191–197. doi: 10.1016/0378-1119(88)90117-5
Khalfaoui-Hassani, B., Verissimo, A. F., Koch, H. G., and Daldal, F. (2016a). Uncovering the transmembrane metal binding site of the novel bacterial major facilitator superfamily-type copper importer CcoA. MBio 7:e01981-15. doi: 10.1128/mBio.01981-15
Khalfaoui-Hassani, B., Verissimo, A. F., Shroff, N., Ekici, S., Trasnea, P.-I., Utz, M., et al. (2016b). “Biogenesis of cytochrome c complexes: from insertion of redox cofactors to assembly of different subunits,” in Cytochrome Complexes: Evolution, Structures, Energy Transduction, and Signaling, eds W. Cramer and T. Kallas (Dordrecht: Springer), 527–546.
Koch, H. G., Hwang, O., and Daldal, F. (1998). Isolation and characterization of Rhodobacter capsulatus mutants affected in cytochrome cbb3 oxidase activity. J. Bacteriol. 180, 969–978.
Koch, H. G., Winterstein, C., Saribas, A. S., Alben, J. O., and Daldal, F. (2000). Roles of the ccoGHIS gene products in the biogenesis of the cbb3-type cytochrome c oxidase. J. Mol. Biol. 297, 49–65. doi: 10.1006/jmbi.2000.3555
Kojima, N., Yamanaka, M., Ichiki, S., and Sambongi, Y. (2005). Unexpected elevated production of Aquifex aeolicus cytochrome c555 in Escherichia coli cells lacking disulfide oxidoreductases. Biosci. Biotechnol. Biochem. 69, 1418–1421. doi: 10.1271/bbb.69.1418
Kulajta, C., Thumfart, J. O., Haid, S., Daldal, F., and Koch, H. G. (2006). Multi-step assembly pathway of the cbb3-type cytochrome c oxidase complex. J. Mol. Biol. 355, 989–1004. doi: 10.1016/j.jmb.2005.11.039
Laemmli, U. K. (1970). Cleavage of structural proteins during the assembly of the head of bacteriophage T4. Nature 227, 680–685. doi: 10.1038/227680a0
La Monica, R. F., and Marrs, B. L. (1976). The branched respiratory system of photosynthetically grown Rhodopseudomonas capsulata. Biochim. Biophys. Acta 423, 431–439. doi: 10.1016/0005-2728(76)90198-5
Lohmeyer, E., Schröder, S., Pawlik, G., Trasnea, P. I., Peters, A., Daldal, F., et al. (2012). The ScoI homologue SenC is a copper binding protein that interacts directly with the cbb3-type cytochrome oxidase in Rhodobacter capsulatus. Biochim. Biophys. Acta 1817, 2005–2015. doi: 10.1016/j.bbabio.2012.06.621
Marrs, B., Stahl, C. L., Lien, S., and Gest, H. (1972). Biochemical physiology of a respiration-deficient mutant of the photosynthetic bacterium Rhodopseudomonas capsulata. Proc. Natl. Acad. Sci. U.S.A. 69, 916–920. doi: 10.1073/pnas.69.4.916
Mavridou, D. A., Ferguson, S. J., and Stevens, J. M. (2012). The interplay between the disulfide bond formation pathway and cytochrome c maturation in Escherichia coli. FEBS Lett. 586, 1702–1707. doi: 10.1016/j.febslet.2012.04.055
Meehan, B. M., Landeta, C., Boyd, D., and Beckwith, J. (2017a). The disulfide bond formation pathway is essential for anaerobic growth of Escherichia coli. J. Bacteriol. 199(16):e00120-17. doi: 10.1128/JB.00120-17
Meehan, B. M., Landeta, C., Boyd, D., and Beckwith, J. (2017b). The essential cell division protein FtsN contains a critical disulfide bond in a non-essential domain. Mol. Microbiol. 103, 413–422. doi: 10.1111/mmi.13565
Metheringham, R., Griffiths, L., Crooke, H., Forsythe, S., and Cole, J. (1995). An essential role for DsbA in cytochrome c synthesis and formate-dependent nitrite reduction by Escherichia coli K-12. Arch. Microbiol. 164, 301–307. doi: 10.1007/BF02529965
Onder, O., Turkarslan, S., Sun, D., and Daldal, F. (2008). Overproduction or absence of the periplasmic protease DegP severely compromises bacterial growth in the absence of the dithiol: disulfide oxidoreductase DsbA. Mol. Cell. Proteomics 7, 875–890. doi: 10.1074/mcp.M700433-MCP200
Park, S. H., Choi, C. W., Lee, K. S., and Kim, C. J. (1998). Influence of quercetin, a bioflavonoid, on the toxicity of copper to Fusarium culmorum. Lett. Appl. Microbiol. 26, 363–366. doi: 10.1046/j.1472-765X.1998.00351.x
Pereira, M. M., Santana, M., and Teixeira, M. (2001). A novel scenario for the evolution of haem-copper oxygen reductases. Biochim. Biophys. Acta 1505, 185–208. doi: 10.1016/S0005-2728(01)00169-4
Peters, A., Kulajta, C., Pawlik, G., Daldal, F., and Koch, H. G. (2008). Stability of the cbb3-type cytochrome oxidase requires specific CcoQ-CcoP interactions. J. Bacteriol. 190, 5576–5586. doi: 10.1128/JB.00534-08
Prentki, P., and Krisch, H. M. (1984). In vitro insertional mutagenesis with a selectable DNA fragment. Gene 29, 303–313. doi: 10.1016/0378-1119(84)90059-3
Rauhamäki, V., Bloch, D. A., Verkhovsky, M. I., and Wikström, M. (2009). Active site of cytochrome cbb3. J. Biol. Chem. 284, 11301–11308. doi: 10.1074/jbc.M808839200
Rizzitello, A. E., Harper, J. R., and Silhavy, T. J. (2001). Genetic evidence for parallel pathways of chaperone activity in the periplasm of Escherichia coli. J. Bacteriol. 183, 6794–6800. doi: 10.1128/JB.183.23.6794-6800.2001
Sambrook, J., and Russell, D. W. (2001). Molecular Cloning: A Laboratory Manual, NY: Cold Spring Harbor Laboratory Press.
Sanders, C., Turkarslan, S., Lee, D. W., and Daldal, F. (2010). Cytochrome c biogenesis: the Ccm system. Trends Microbiol. 18, 266–274. doi: 10.1016/j.tim.2010.03.006
Sanders, C., Turkarslan, S., Lee, D. W., Onder, O., Kranz, R. G., and Daldal, F. (2008). The cytochrome c maturation components CcmF, CcmH, and CcmI form a membrane-integral multisubunit heme ligation complex. J. Biol. Chem. 283, 29715–29722. doi: 10.1074/jbc.M805413200
Schägger, H., and von Jagow, G. (1987). Tricine-sodium dodecyl sulfate-polyacrylamide gel electrophoresis for the separation of proteins in the range from 1 to 100 kDa. Anal. Biochem. 166, 368–379. doi: 10.1016/0003-2697(87)90587-2
Schägger, H., and von Jagow, G. (1991). Blue native electrophoresis for isolation of membrane protein complexes in enzymatically active form. Anal. Biochem. 199, 223–231. doi: 10.1016/0003-2697(91)90094-A
Scolnik, P. A., Walker, M. A., and Marrs, B. L. (1980). Biosynthesis of carotenoids derived from neurosporene in Rhodopseudomonas capsulata. J. Biol. Chem. 255, 2427–2432.
Shouldice, S. R., Heras, B., Walden, P. M., Totsika, M., Schembri, M. A., and Martin, J. L. (2011). Structure and function of DsbA, a key bacterial oxidative folding catalyst. Antioxid. Redox Signal. 14, 1729–1760. doi: 10.1089/ars.2010.3344
Sistrom, W. R. (1960). A requirement for sodium in the growth of Rhodopseudomonas spheroides. J. Gen. Microbiol. 22, 778–785. doi: 10.1099/00221287-22-3-778
Spiess, C., Beil, A., and Ehrmann, M. (1999). A temperature-dependent switch from chaperone to protease in a widely conserved heat shock protein. Cell 97, 339–347. doi: 10.1016/S0092-8674(00)80743-6
Swem, D. L., Swem, L. R., Setterdahl, A., and Bauer, C. E. (2005). Involvement of SenC in assembly of cytochrome c oxidase in Rhodobacter capsulatus. J. Bacteriol. 187, 8081–8087. doi: 10.1128/JB.187.23.8081-8087.2005
Thomas, P. E., Ryan, D., and Levin, W. (1976). An improved staining procedure for the detection of the peroxidase activity of cytochrome P-450 on sodium dodecyl sulfate polyacrylamide gels. Anal. Biochem. 75, 168–176. doi: 10.1016/0003-2697(76)90067-1
Thompson, A. K., Gray, J., Liu, A., and Hosler, J. P. (2012). The roles of Rhodobacter sphaeroides copper chaperones PCu(A)C and Sco (PrrC) in the assembly of the copper centers of the aa3-type and the cbb3-type cytochrome c oxidases. Biochim. Biophys. Acta 1817, 955–964. doi: 10.1016/j.bbabio.2012.01.003
Trasnea, P. I., Utz, M., Khalfaoui-Hassani, B., Lagies, S., Daldal, F., and Koch, H. G. (2016). Cooperation between two periplasmic copper chaperones is required for full activity of the cbb3-type cytochrome c oxidase and copper homeostasis in Rhodobacter capsulatus. Mol. Microbiol. 100, 345–361. doi: 10.1111/mmi.13321
Turkarslan, S., Sanders, C., Ekici, S., and Daldal, F. (2008). Compensatory thio-redox interactions between DsbA, CcdA and CcmG unveil the apocytochrome c holdase role of CcmG during cytochrome c maturation. Mol. Microbiol. 70, 652–666. doi: 10.1111/j.1365-2958.2008.06441.x
Valkova-Valchanova, M. B., Saribas, A. S., Gibney, B. R., Dutton, P. L., and Daldal, F. (1998). Isolation and characterization of a two-subunit cytochrome bc1 subcomplex from Rhodobacter capsulatus and reconstitution of its ubihydroquinone oxidation (Qo) site with purified Fe-S protein subunit. Biochemistry 37, 16242–16251. doi: 10.1021/bi981651z
Verissimo, A. F., and Daldal, F. (2014). Cytochrome c biogenesis System I: an intricate process catalyzed by a maturase supercomplex? Biochim. Biophys. Acta 1837, 989–998. doi: 10.1016/j.bbabio.2014.03.003
Verissimo, A. F., Khalfaoui-Hassani, B., Hwang, J., Steimle, S., Selamoglu, N., Sanders, C., et al. (2017). The thioreduction component CcmG confers efficiency and the heme ligation component CcmH ensures stereo-specificity during cytochrome c maturation. J. Biol. Chem. 292, 13154–13167. doi: 10.1074/jbc.M117.794586
Verissimo, A. F., Yang, H., Wu, X., Sanders, C., and Daldal, F. (2011). CcmI subunit of CcmFHI heme ligation complex functions as an apocytochrome c chaperone during c-type cytochrome maturation. J. Biol. Chem. 286, 40452–40463. doi: 10.1074/jbc.M111.277764
Yen, H. C., Hu, N. T., and Marrs, B. L. (1979). Characterization of the gene transfer agent made by an overproducer mutant of Rhodopseudomonas capsulata. J. Mol. Biol. 131, 157–168. doi: 10.1016/0022-2836(79)90071-8
Yen, H. C., and Marrs, B. (1976). Map of genes for carotenoid and bacteriochlorophyll biosynthesis in Rhodopseudomonas capsulata. J. Bacteriol. 126, 619–629.
Zannoni, D. (2004). Respiration in Archaea and Bacteria: Diversity of Prokaryotic Respiratory Systems. Dordrecht: Springer.
Keywords: disulfide bond formation, copper homeostasis, cbb3-type cytochrome c oxidase biogenesis, Rhodobacter capsulatus, respiration, photosynthesis
Citation: Onder O, Verissimo AF, Khalfaoui-Hassani B, Peters A, Koch H-G and Daldal F (2017) Absence of Thiol-Disulfide Oxidoreductase DsbA Impairs cbb3-Type Cytochrome c Oxidase Biogenesis in Rhodobacter capsulatus. Front. Microbiol. 8:2576. doi: 10.3389/fmicb.2017.02576
Received: 26 September 2017; Accepted: 11 December 2017;
Published: 21 December 2017.
Edited by:
Wendy Schluchter, University of New Orleans, United StatesReviewed by:
Jonathan Hosler, University of Mississippi Medical Center, United StatesPia Ädelroth, Stockholm University, Sweden
Mehmet Berkmen, New England Biolabs, United States
Copyright © 2017 Onder, Verissimo, Khalfaoui-Hassani, Peters, Koch and Daldal. This is an open-access article distributed under the terms of the Creative Commons Attribution License (CC BY). The use, distribution or reproduction in other forums is permitted, provided the original author(s) or licensor are credited and that the original publication in this journal is cited, in accordance with accepted academic practice. No use, distribution or reproduction is permitted which does not comply with these terms.
*Correspondence: Fevzi Daldal, ZmRhbGRhbEBzYXMudXBlbm4uZWR1
†Present Address: Ozlem Onder, Department of Pathology and Laboratory Medicine, Perelman School of Medicine, University of Pennsylvania, Philadelphia, PA, United States
Bahia Khalfaoui-Hassani, Department of IBEAS-UFR Science et Techniques, Equipe Environement et Microbiology, Univestité de Pau et des Pays de l'Andour, Pau, France
‡These authors have contributed equally to this work and Co first authors.