- 1Departamento de Bioquímica y Biología Molecular, Campus de Excelencia Internacional Agroalimentario CeiA3, Universidad de Córdoba, Córdoba, Spain
- 2Institute of Biocomputation and Physics of Complex Systems (BIFI), Joint Units BIFI-IQFR-CSIC and GBsC-BIFI-CSIC, Universidad de Zaragoza, Zaragoza, Spain
- 3Aragon Institute for Health Research (IIS Aragon), Zaragoza, Spain
- 4Instituto Aragonés de Ciencias de la Salud, Zaragoza, Spain
- 5Centro de Investigación Biomédica en Red en el Área Temática de Enfermedades Hepáticas y Digestivas, Barcelona, Spain
- 6Fundación ARAID, Gobierno de Aragón, Zaragoza, Spain
- 7Professur für Mikrobiologie, Department Biologie, Friedrich-Alexander-Universität Erlangen-Nürnberg, Erlangen, Germany
Previous studies showed differences in the regulatory response to C/N balance in Prochlorococcus with respect to other cyanobacteria, but no information was available about its causes, or the ecological advantages conferred to thrive in oligotrophic environments. We addressed the changes in key enzymes (glutamine synthetase, isocitrate dehydrogenase) and the ntcA gene (the global nitrogen regulator) involved in C/N metabolism and its regulation, in three model Prochlorococcus strains: MED4, SS120, and MIT9313. We observed a remarkable level of diversity in their response to azaserine, a glutamate synthase inhibitor which increases the concentration of the key metabolite 2-oxoglutarate, used to sense the C/N balance by cyanobacteria. Besides, we studied the binding between the global nitrogen regulator (NtcA) and the promoter of the glnA gene in the same Prochlorococcus strains, and its dependence on the 2-oxoglutarate concentration, by using isothermal titration calorimetry, surface plasmon resonance, and electrophoretic mobility shift. Our results show a reduction in the responsiveness of NtcA to 2-oxoglutarate in Prochlorococcus, especially in the MED4 and SS120 strains. This suggests a trend to streamline the regulation of C/N metabolism in late-branching Prochlorococcus strains (MED4 and SS120), in adaptation to the rather stable conditions found in the oligotrophic ocean gyres where this microorganism is most abundant.
Introduction
The extraordinary abundance of Prochlorococcus in the oligotrophic areas of the oceans has been reported by different studies since its discovery (Chisholm et al., 1988), and it is considered that Prochlorococcus contributes significantly to the global primary production (Goericke and Welschmeyer, 1993; Liu et al., 1997; Casey et al., 2007), conferring this organism an outstanding importance in marine ecology (Biller et al., 2015). Recent reports suggest it might become even more widespread due to global warming in the near future (Flombaum et al., 2013). Different explanations have been proposed for the abundance of Prochlorococcus, including the very small size of the cells, the high surface/volume ratio, or the streamlining of the genome (and consequently of the metabolic pathways), leading to low energetic requirements for survival and cell division (Partensky et al., 1999; Giovannoni et al., 2014; Biller et al., 2015).
Adaptation to oligotrophic oceans involves the development of adaptive mechanisms to cope with the availability of several key elements, which are scarce in those regions. Nitrogen is one of them. This fact has led to different strategies to optimize its utilization in marine cyanobacteria, providing enough nitrogen for growth with an affordable energetic expense, such as the use of specific sets of N-assimilating genes depending on the strain (López-Lozano et al., 2002; Moore et al., 2002; Dufresne et al., 2003, 2008; Palenik et al., 2003; Rocap et al., 2003; García-Fernández et al., 2004; Scanlan et al., 2009; Berube et al., 2014; Biller et al., 2014).
The cyanobacterial regulatory mechanisms to control the C/N balance are present in Prochlorococcus, but showing some striking variations with respect to freshwater strains: despite possessing the genes involved in standard carbon/nitrogen regulation in cyanobacteria (namely ntcA, glnB, and pipX encoding, respectively, NtcA, PII, and PipX), they do not seem to work as previously described (Lindell et al., 2002; Palinska et al., 2002; García-Fernández et al., 2004; López-Lozano et al., 2009; Domínguez-Martín et al., 2014). Besides, the regulation of enzymes such as glutamine synthetase (GS) (El Alaoui et al., 2001, 2003; Gómez-Baena et al., 2001) and isocitrate dehydrogenase (ICDH) (López-Lozano et al., 2009; Domínguez-Martín et al., 2014) is clearly different from their counterparts in other cyanobacteria, showing a lack of response to nitrogen starvation or darkness. Furthermore, comparative transcriptomic studies have shown clear changes in two of the main model Prochlorococcus strains, such as MED4 and MIT9313, suggesting that they integrate N and C metabolism in fundamentally different ways (Tolonen et al., 2006). However, the molecular underpinnings of these changes have not been studied thus far.
We studied the role of 2-oxoglutarate (2OG) in the control of the C/N balance in Prochlorococcus, in order to check whether there are differences with respect to other model cyanobacteria (Forchhammer and Tandeau De Marsac, 1994; Vazquez-Bermudez et al., 2002; Muro-Pastor and Florencio, 2003; Flores and Herrero, 2005; Muro-Pastor et al., 2005; Luque and Forchhammer, 2008). Besides, we addressed the regulatory differences between Prochlorococcus strains by studying the enzymes glutamine synthetase (GS) and isocitrate dehydrogenase (ICDH) and also the interaction between the NtcA protein and the promoter of the glnA gene (encoding GS). To this goal, we measured enzymatic activities by spectrophotometric methods, enzyme concentrations by Western blotting, gene expression by qRT-PCR, intracellular 2OG concentration by an enzymatic method and the interaction of NtcA with glnA promoter DNA by isothermal titration calorimetry (ITC), surface plasmon resonance (SPR) and electrophoretic mobility shift assay (EMSA).
Azaserine is an inhibitor of glutamate synthase (GOGAT) (Pinkus, 1977), the enzyme that together with GS compose the main pathway for nitrogen assimilation in cyanobacteria. We have previously shown that azaserine induces clear changes in enzyme activities related to nitrogen metabolism of several Prochlorococcus strains (El Alaoui et al., 2001; López-Lozano et al., 2009; Rangel et al., 2009; Domínguez-Martín et al., 2014). Furthermore, we have recently shown that azaserine addition induces an increase in the concentration of 2OG in Prochlorococcus SS120, mimicking the effect of nitrogen starvation (Domínguez-Martín et al., 2017); this leads to extensive changes in the proteome of this strain (Domínguez-Martín et al., 2017). Hence we decided to study the effects of azaserine addition to cultures of different Prochlorococcus strains.
In this study we compared three different strains: MED4, representative member of ecotypes adapted to live near the ocean surface, and MIT9313 and SS120 belonging to ecotypes adapted to live at depth. Despite both strains living at depth, MIT9313 and SS120 are very different in evolutionary terms, as MIT9313 was early branching in the Prochlorococcus phylogenetic tree (Biller et al., 2014). Our goal was to test if differences in the regulation of the C/N balance might be related to the different habitats of strains, and/or to their phylogeny.
Materials and Methods
Prochlorococcus Strains and Growth Conditions
Prochlorococcus spp. strains MED4 (high-irradiance adapted), SS120 and MIT9313 (low-irradiance adapted) were cultured as described (El Alaoui et al., 2001). Cultures were grown in a culture room set at 24°C under continuous blue irradiances: 40 μE/m2/s for high-light adapted ecotypes and 4 μE/m2/s for low-light adapted ecotypes using neon Sylvania F18W/154-ST Daylight, covered with a filter Moonlight blue L183 from Lee Filters.
Cell Extracts
Cells were centrifuged at 26,000 g for 8 min at 4°C using an Avanti J-25 Beckman centrifuge equipped with a JA-14 rotor. After pouring most of the supernatant and carefully pipetting out the remaining medium, the pellet was directly resuspended in the corresponding volume of buffer (generally 1 mL per liter of culture). For Western blotting the proportion was 0.5 mL per liter of culture. For protein assays (enzymatic and Western blotting) the solution was 50 mM Tris-HCl pH 7.5 and for RNA analysis the pellet was resuspended in 10 mM sodium acetate (pH 4.5), 200 mM sucrose and 5 mM EDTA.
After thawing, the cell suspensions were broken in a French pressure cell (SLM/Aminco model FA-079) at 16,000 psi; the obtained extracts were centrifuged for 10 min at 16,900 g and 4°C in an Eppendorf microfuge 5418R. The supernatant was transferred to a new tube.
Determination of Protein Concentration
Protein concentration was determined using the Bio-Rad Protein Assay kit, according to the manufacturer instructions.
Determination of Intracellular Concentration of 2OG
After thawing the samples on ice, the extracts were centrifuged for 10 min at 16,900 g and 4°C. The supernatants were used for the determination of 2OG as previously described (Domínguez-Martín et al., 2014).
Determination of Enzymatic Activities
Glutamine synthetase transferase activity and NADP-isocitrate dehydrogenase activity were determined as previously described (El Alaoui et al., 2001; Domínguez-Martín et al., 2014).
Detection of Glutamine Synthetase and Isocitrate Dehydrogenase by Western Blotting
Western blotting was carried out as described elsewhere (Domínguez-Martín et al., 2014), by using anti-GS and anti-ICDH antibodies raised against both enzymes from Synechocystis sp. PCC 6803, kindly provided by Dr. M.I. Muro-Pastor and Prof. F.J. Florencio (Instituto de Bioquímica Vegetal y Fotosíntesis, Sevilla, Spain).
RNA Extraction
RNA was isolated from 500 mL culture samples. Cells were harvested as described above. Total RNA was extracted using TRIsure RNA Isolation Reagent (Bioline) following the manufacturer's recommendations, and resuspended in 200 μL sterile MilliQ-grade water, with the addition of 133 μL 8 M LiCl, an additional precipitation step included at the end of the procedure to improve the RNA quality. RNA was treated with Rnase-free DnaseI (Ambion) according to the manufacturer instructions, and the absence of contaminating genomic DNA was assessed using a PCR control test.
Design and Production of Oligonucleotides
The sequences of cyanobacterial genes were obtained from CYORF (Cyanobacteria gene annotation database, http://cyano.genome.ad.jp/). The oligonucleotides used in this work were designed using the program Oligo v4.05 (National Biosciences Inc. Plymouth), Primer3 (University of Massachusetts Medical School, http://bioinfo.ut.ee/primer3/) and refined manually. Integrated DNA Technologies, Sigma, and Invitrogen provided the oligonucleotides. The list of oligonucleotides used is shown in Supplementary Table 1.
Quantitation of Gene Expression by QRT-PCR
qRT-PCR was performed as previously described (López-Lozano et al., 2009), by using the rnpB expression as internal control for quantitation. The sequences of the utilized primers are shown in Supplementary Table 1.
Construction of a ntcA Expression Plasmid
Oligonucleotides used for ntcA cloning from MED4, SS120, and MIT9313 are listed in the Supplementary Table 1. To obtain the expression plasmid, ntcA sequences were PCR amplified with high fidelity polymerases (Fermentas and Bioline) following the manufacturers instructions. The resulting fragment was cloned into pGEM®-T (Promega) or pSpark® (Canvax). From the resulting plasmid the NdeI-BamHI fragment containing ntcA was cloned into the NdeI-BamHI site of pET-15b, giving pET-15b-ntcA. Each step was checked by sequencing using the services from the SCAI (Servicio Central de Apoyo a la Investigación) at the University of Córdoba.
Overexpression and Purification of Recombinant NtcA from Prochlorococcus
Since NtcA-glnA promoter interaction studies were done using two different techniques (ITC and SPR), in collaboration with two different laboratories, the overexpression and purification procedures were optimized for each technique, and thus are described separately.
Overexpression and Purification of Recombinant NtcA from Prochlorococcus sp. MED4, SS120, and MIT9313 to Perform ITC Determinations
Escherichia coli HB101 served as the host strain for construction and propagation of pGEM®-T (Promega) or pSpark® (Canvax) carrying the ntcA gene. Expression of recombinant NtcA (His6) proteins from Prochlorococcus sp. MED4, MIT9313, and SS120 were carried in E. coli BL21 (DE3). Strains were grown in Luria broth (LB) supplemented with filtered ampicillin (100 μg/mL). An overnight 150 mL culture was diluted in 4.5 L of LB supplemented with 100 μg/mL ampicillin and grown at 37°C to an OD600 of 0.5–0.6. Subsequently, heterologous expression was induced with 0.5 mM IPTG (isopropyl ß-D-1-thiogalactopyranoside) and the culture was transferred to 37°C for 4 h. Thereafter, cells were harvested at 3,800 g for 10 min at room temperature. The pellet was resuspended in buffer A (50 mM NaH2PO4, 300 mM NaCl, 60 mM Imidazole) containing lysozyme (0.3 mg/mL). Disruption of cells was carried out on ice by sonication and 30 μL of Dnase I (10 mg/mL) and 30 μL of RNase (5 mg/mL) were added and incubated on ice for 20 min. Cell debris was removed by centrifugation at 48,000 g (JA-20 rotor) for 40 min at 4°C and the supernatant was collected. The purification was carried out with a Pierce Centrifuge Column, 10 mL (ThermoFisher Scientific), adding 4 mL of Ni-NTA agarose (ThermoFisher Scientific) according to the manufacturer instructions. All steps were performed at 4°C. The column was charged with the supernatant and subsequently washed with 4 column volumes of wash buffer (50 mM NaH2PO4, 300 mM NaCl, 80 mM Imidazole). The attached proteins were eluted by ligand exchange employing buffer B (50 mM NaH2PO4, 300 mM NaCl, 250 mM Imidazole). Fractions containing specific NtcA were visualized by coomassie-stained 12% SDS-PAGE. The buffer exchange of the eluted proteins to the buffer used for the isothermal titration calorimetry (50 mM Hepes, 350 mM NaCl, pH 7.5) was carried out with a Spectra/Por 2 Regenerated Cellulose Dialysis Membrane (Spectrum Labs) according to the manufacturer's instructions.
Overexpression and Purification of Recombinant NtcA from Prochlorococcus sp. SS120 and MIT9313 to Perform SPR Determinations
E. coli HB101 and DH5α served as the host strain for construction and propagation of pGEM®-T (Promega) or pSpark® (Canvax) with ntcA gene cloned. Expression of recombinant NtcA (His6) proteins from Prochlorococcus MIT9313 and SS120 were carried in E. coli BL21 (DE3) (Supplementary Figure 1). Since the SPR studies were carried out earlier than those using ITC, at that time we focused our attention on two low-light adapted Prochlorococcus strains with very different evolutionary histories, as MIT9313 and SS120, and therefore this interaction was not studied by SPR in the strain MED4. Strains were grown in Luria broth (LB) supplemented with filtered ampicillin (100 μg/mL). An overnight 100 mL culture was diluted in 6 L of LB and grown at 37°C to an OD600 of 0.5–0.6. Subsequently, heterologous expression was induced with 0.5 mM IPTG (isopropyl β-D-1-thiogalactopyranoside) and the culture was transferred to 37°C for 4 h. Thereafter, cells were harvested at 3,800 g for 5 min at room temperature. The pellet was resuspended in buffer A (50 mM NaH2PO4, 300 mM NaCl, 30–80 mM imidazole) containing protease inhibitor (1 tablet in 2 mL MilliQ-purified water). Disruption of cells was carried out on ice by sonication and 30 μL of DNase I (10 mg/mL) and 30 μL of RNase (5 mg/mL) were added and incubated on ice for 20 min. Cell debris were removed by centrifugation at 48,000 g (JA-25.50 rotor) for 40 min at 4°C and the supernatant was collected. The purification was carried out with an Äkta Prime System (GE Healthcare) with 5 mL of Ni-NTA according to the manufacturer's instructions. All steps were performed at 4°C. The column was charged with the supernatant and subsequently washed with 15 column volumes of buffer A. The attached proteins were eluted by ligand exchange employing a step gradient of 10, 20, 30, 50, and finally 100% of buffer B (50 mM NaH2PO4, 300 mM NaCl, 500 mM imidazole). Fractions containing specific NtcA were visualized by Coomassie-stained 12% SDS-PAGE.
Molecular Size Exclusion Chromatography
The protein solution was concentrated to a volume of 5 mL by centrifugation with Centriprep filters (MWCO 10 or 30 kDa) (Millipore) at 1,500 g and 4°C. The concentrated sample was loaded on a Sephadex75 size exclusion chromatography column for further purification. Pure protein fractions were further concentrated and stored in HBS-E buffer at 4°C not exceeding 4 weeks for SPR measurements, or in HBS-E with 50% (v/v) glycerol at −20°C for longer terms storage.
Isothermal Titration Calorimetry
All dsDNA samples were prepared from 1 mM solutions for each oligonucleotide (Integrated DNA Technologies) including the wild-type or mutated binding site sequence for NtcA from the different Prochlorococcus strains. The specific DNA fragments contained the binding site for NtcA from the promoter region of glnA and the reference oligonucleotides contained this binding site with mutations (Table 1). Each pair of oligonucleotides was mixed in an equimolar solution and subjected to 5 min at 95°C, followed by a 3-h temperature gradient from 95 to 25°C, decreasing 0.5°C each step. 0.5 mM dsDNA stock solutions were obtained for each NtcA binding site sequence.
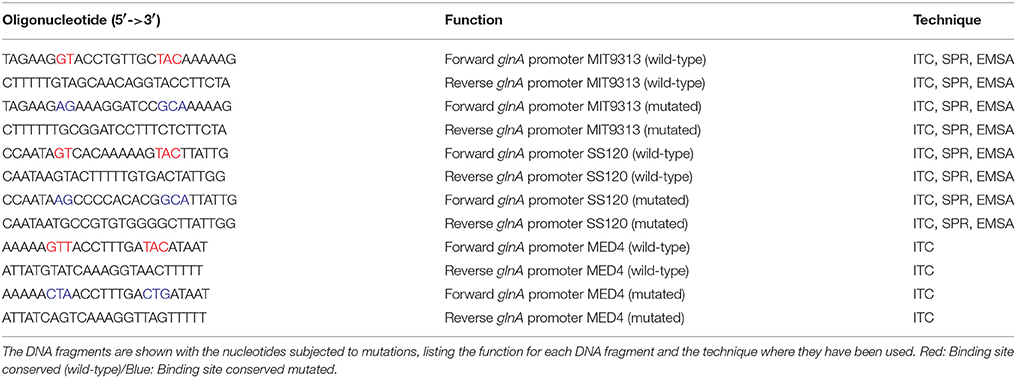
Table 1. Wild-type and mutated oligonucleotides corresponding to the promoter of the glnA gene in Prochlorococcus sp. strains MIT9313, SS120, and MED4.
The interaction between the different NtcA variants and dsDNA was characterized using an Auto-iTC200 microcalorimeter (MicroCal, Malvern). Titrations were performed at 25°C. Protein in the calorimetric cell at 5–10 μM in a monomer basis (5 μM for NtcA SS120 and NtcA MED4 experiments, 10 μM for MIT9313 experiments) was titrated with dsDNA at 50–100 μM (50 μM for NtcA SS120 and NtcA MED4 experiments, 100 μM for MIT9313 experiments). All solutions were degassed at 15°C for 2 min before each assay. A sequence of 2 μL-injections of titrant solution every 150 s was programmed and the stirring speed was set to 750 rpm. Different experiments increasing 2OG concentration (from 0 to 10 mM) in the calorimetric cell were performed. Each single experiment provided the apparent dissociation constant for NtcA interacting with dsDNA at a certain 2OG concentration (Supplementary Figure 2). Dissociation constants were estimated through non-linear least squares regression analysis of the experimental data employing a single ligand binding site model (1:1 dsDNA:dimer stoichiometry) implemented in Origin (OriginLab).
From each titration, the apparent dissociation constant for the NtcA-dsDNA interaction in the presence of 2OG, , was determined. If the binding of dsDNA is affected by 2OG, the dissociation constant may increase (negative cooperativity) or decrease (positive cooperativity). Considering the ternary equilibrium between NtcA, dsDNA, and 2OG, the for the NtcA-dsDNA interaction will explicitly depend on the concentration of 2OG:
where Kd is the (intrinsic) dissociation constant for the NtcA-dsDNA interaction in the absence of 2OG, [oxo] is the concentration of 2OG, Kd,oxo is the dissociation constant for the NtcA-2OG interaction, and α is the cooperativity interaction constant (α > 1 indicates positive cooperativity, whereas α < 1 indicates negative cooperativity) (Velazquez-Campoy et al., 2006). The limit value for the dissociation constant at very high 2OG concentration is Kd,eff = Kd/α. The cooperative effect of both ligands is reciprocal, and, therefore, the dissociation constant for 2OG will also be affected by the presence of dsDNA. Thus, the limit value for the dissociation constant Kd,oxo at very large dsDNA concentration is equal to Kd,oxo/α. This last value can be observed in the vs. [oxo] plot as the effective 2OG concentration, Kd,oxo,eff, at which half-maximal change in is observed. Then, Kd,oxo,eff = Kd,oxo/α is the effective Kd,oxo, that is, the concentration of 2OG at which the cooperative effect (reduction of for dsDNA due to the presence of 2OG) is observed at half extent (or the dissociation constant for the NtcA/2OG interaction at very high dsDNA concentration). as a function of 2OG concentration can be fitted using the previous equation in order to estimate Kd, Kd,oxo, and α as adjustable parameters through non-linear least squares regression. The cooperative effect of 2OG and dsDNA is reciprocal: if 2OG increases the binding affinity of dsDNA, then, dsDNA increases the binding affinity of 2OG in the same extent.
Surface Plasmon Resonance
All SPR measurements were carried out on a BIACORE X (GE Healthcare) instrument at 25°C. All 26 nt oligonucleotides were purchased from MWG Eurofins/Ebersberg. The forward strand was biotinylated at the 5′end. The DNA fragments used are summarized in Table 1.
Equal amounts of complementary strands (5 μM) were mixed in PCR tubes and hybridized in the heat block for 20 min at 80°C. Afterwards, the heat block was set at 60°C, then cooled down (ca. 20 min) and finally switched off. For the visualization of the hybridization, a 20% polyacrylamide gel was used.
SPR with Sensor Chip CM5 with Neutroavidin
Surface preparation
The Sensor Chip CM5 (GE Healthcare) was equilibrated at room temperature for 15–30 min in order to prevent condensation of water vapor on the chip surface. The BIACORE instrument was prepared with HBS-EP running buffer. The Sensor Chip was docked in the instrument. The next step was the coupling of the Neutroavidin in both flow cells (1 and 2). First, the chip was activated injecting 50 μL of EDC (1-ethyl-3-(3-dimethylaminopropyl)-carbodiimide) and 50 μL of NHS (N-hydroxysuccionimide). Then, 50 μL of 5 μM Neutroavidin (1 mg/mL) dissolved in 10 mM Na-Acetate was injected, followed by 35 μL of 1 M ethanolamin.
Sample injection
The samples containing NtcA from Prochlorococcus sp. SS120 and MIT9313 were diluted from the stock to 1 μM with HBS-EP buffer. To minimize sample dispersion at the beginning and the end of the injection, it is recommended to introduce a small volume of air with the sample (about 5 μL each) into the pipette tip before loading. This will separate the sample from running buffer and prevent mixing. The protein was injected with a flow rate of 5 μL/min. The interaction was analyzed with the program Biacore X Control Software, and the results evaluated using the software BIAevaluation.
To analyze the effect of 2OG on the binding between NtcA and the glnA promoter, 20 mL of 2OG were prepared in HBS-EP buffer as the concentration required, adjusting the pH to 7.4 with 5% of NaOH. The system was equilibrated with new buffer prior protein injection.
Electrophoretic Mobility Shift Assay (EMSA)
EMSA studies were carried out as described (Napolitano et al., 2012). Gels were stained with SyberSafe (Invitrogen) following the manufacturer instructions. The images were captured with a ChemiDoc system (Bio-Rad).
Genomic Sequences and Phylogenetic Analysis
Selected cyanobacterial ntcA sequences were retrieved from CYORF (http://cyano.genome.jp/), Cyanobase (http://genome.microbedb.jp/cyanobase/), Integrated Microbial Genomes (https://img.jgi.doe.gov) and NCBI (http://www.ncbi.nlm.nih.gov/) databases. Protein BLAST analysis were performed using NCBI BLASTp tool (http://blast.ncbi.nlm.nih.gov/Blast.cgi?PAGE=Proteins). The software MEGA 7 (Kumar et al., 2016) was used to align the deduced protein sequences by using the MUSCLE method (using default settings) and a phylogenetic tree was obtained by using the Maximum Likelihood method. Further details are provided in the legend of Figure 6.
Statistical Analysis
The results are shown with error bars corresponding to the standard deviation. Significance of data was assessed by using the Student's T-test, and indicated in figures with asterisks: * means p ≤ 0.05; ** means p ≤ 0.01.
Results
2OG is the molecule responsible for the control of the C/N balance in cyanobacteria (Herrero et al., 2001; Muro-Pastor et al., 2001; Tanigawa et al., 2002; Vazquez-Bermudez et al., 2002; Flores and Herrero, 2005; Flores et al., 2005; Luque and Forchhammer, 2008). We have described previously its role in Prochlorococcus sp. PCC 9511, a strain genetically identical to MED4 (Domínguez-Martín et al., 2014). However, no specific study has been carried out thus far addressing the possible diversity of the role of 2OG within a cyanobacterial genus. Therefore, we decided to assess how this metabolite might impact the balance of C/N in different strains of Prochlorococcus under diverse conditions. To this goal, we studied first the azaserine effects on key enzymes and genes involved in the C/N metabolism in cyanobacteria; then proceeded to examine the possible diversity in the requirements of 2OG for the interaction between the NtcA transcriptional factor and the promoter of the glnA gene. Three model Prochlorococcus strains were selected for this work: MED4 (high light-adapted), SS120 and MIT9313 (both low light-adapted), with different evolutionary origins, as described above.
Effect of Azaserine on the Metabolism of Prochlorococcus sp. SS120, MIT9313 and MED4
Effect of Azaserine on the Regulation of GS and ICDH
GS (EC 6.3.1.2) catalyzes the first step of the central pathway for ammonium assimilation in most organisms, including cyanobacteria; i.e., the ATP-dependent synthesis of glutamine from glutamate and ammonia. The enzyme isocitrate dehydrogenase (ICDH; EC 1.1.1.42) catalyzes the oxidative decarboxylation of isocitrate to produce 2OG. The possible variability between Prochlorococcus strains with respect to their regulatory mechanisms was assessed by studying the effect of different concentration of azaserine on these two key enzymes, GS and ICDH, using three different strains: MED4, MIT9313, and SS120.
Figure 1 shows that the response of GS transferase activity (Figure 1A) and the amount of GS (Figure 1B) to the different concentrations of azaserine is quite different depending on the strain. In the case of SS120, the activity increased with the concentration of azaserine (maximum level at 100 μM azaserine, p = 0.0018), while the other strains show a quite different pattern. In Prochlorococcus sp. MIT9313, the activity did not change with the concentration of azaserine. In MED4, GS activity only showed a significant increase after 20 μM azaserine addition.
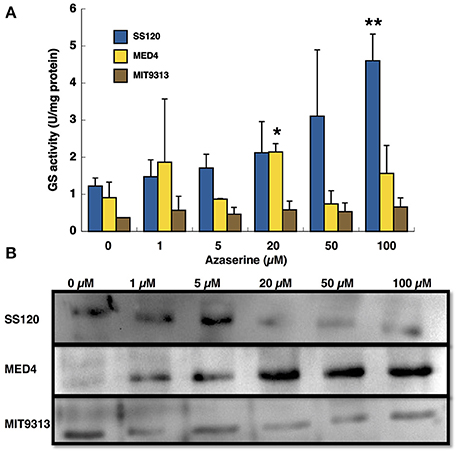
Figure 1. Effect of different concentration of azaserine on GS activity (A) and GS protein concentration (B) in different strains from Prochlorococcus. The different concentrations of azaserine were added to the cultures and cells were collected after 24 h of treatment. (A) is a representation of three independent biological replicates of each strain. Error bars correspond to standard deviation. (B) corresponds to a Western blotting using anti-GS antibodies.
GS concentration measured by Western blotting also showed three different patterns (Figure 1B): in MED4 the enzyme concentration increased with the concentration of the inhibitor but in contrast, GS from SS120 decreased. In MIT9313 the concentration of the enzyme showed little changes, in good agreement with the results observed for GS activity.
ICDH activity was measured under the same conditions like GS (Figure 2A). The pattern was similar in the case of Prochlorococcus sp. SS120 and MED4, the activity being higher at low concentration of azaserine, although it was not reflected in the Western blotting for SS120 shown in Figure 2B. In the case of MIT9313, the activity increased significantly with azaserine concentration up to 5 μM (p = 0.0001; Figure 2A), and the same was observed for the concentration of the enzyme (Figure 2B).
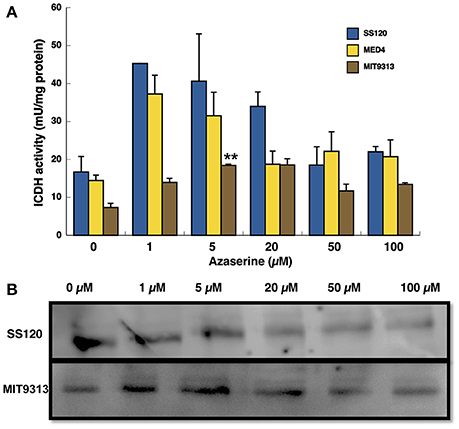
Figure 2. Effect of different concentration of azaserine on ICDH activity (A) and ICDH protein concentration (B) in different strains of Prochlorococcus. The different concentrations of azaserine were added to the cultures and cells were collected after 24 h of treatment. (A) is a representation of three independent biological replicates of each strain. Error bars correspond to standard deviation. (B) corresponds to a Western blotting using anti-ICDH antibodies.
The high amino acid sequence conservation of both GS and ICDH from the three Prochlorococcus strains studied here (Supplementary Figures 3, 4, respectively) suggests that the differences described above are not due to changes in structure or physico-chemicals properties of the enzymes, but to different regulatory mechanisms, probably derived from evolutionary pressures.
Effect of Azaserine on ntcA Expression
Since azaserine had a remarkable diversity of effects on enzyme regulation, we further studied this process by measuring the expression of the global nitrogen regulator, ntcA (encoding the transcriptional factor NtcA, which controls the expression of both glnA and icd, coding for glutamine synthetase and isocitrate dehydrogenase, respectively). We measured its expression by qRT-PCR in culture samples collected 24 h after the addition of different azaserine concentrations (0–100 μM).
The ntcA expression pattern was very different depending on the studied Prochlorococcus strain (Figure 3). While in SS120 the expression did not significantly change with the concentration of azaserine, in MED4, ntcA expression peaked at 20 μM azaserine (p = 0.0001), with significant increases also at 5 and 50 μM (p = 0.0279 and p = 0.0001, respectively). It is worth noting that the increase in ntcA expression observed in this strain was much higher than either in SS120 or MIT9313 (i.e., almost 5-fold higher than in MIT9313). In MIT9313 the expression of ntcA increased with the concentration of the inhibitor, being significantly higher at 50 and 100 μM (p = 0.0047 and p = 0.0059, respectively).
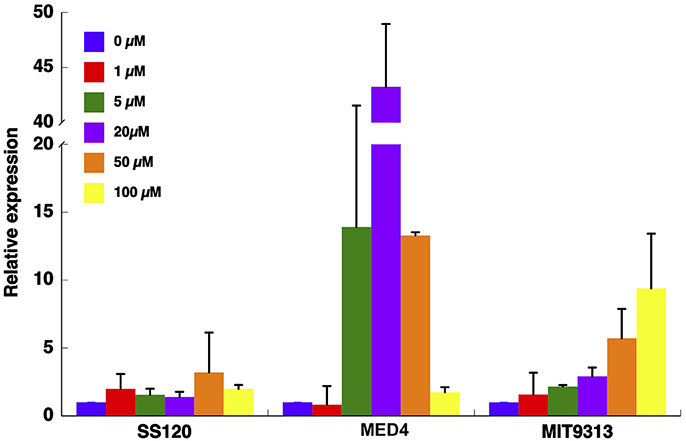
Figure 3. Effect of different concentration of azaserine on ntcA expression in different strains of Prochlorococcus. The different concentrations of azaserine were added to cultures. Samples were collected after 24 h, and gene expression was measured by qRT-PCR. Data are the average of four independent biological replicates. Error bars correspond to standard deviation.
Study of the Interaction of NtcA with glnA Promoter DNA in Prochlorococcus sp. MED4, SS120, and MIT9313
Since the enzymatic and gene expression results described above pointed out to differences in the regulation of the C/N balance between the studied Prochlorococcus strains, we decided to carry out a comparative study of the interaction between the global nitrogen regulator protein in cyanobacteria, NtcA, and one of the promoters subjected to its regulation, that of the glnA gene. To this goal, we performed the heterologous overexpression of ntcA from Prochlorococcus sp. MED4, SS120, and MIT9313. The overexpressed NtcA proteins were used to carry out isothermal titration calorimetry (ITC), surface plasmon resonance (SPR), and electrophoretic mobility assays (EMSA) studies, in order to determine the properties of the NtcA-glnA promoter interaction and their dependence on 2OG concentration in each Prochlorococcus strain.
Isothermal Titration Calorimetry Studies
Wild-type and mutated versions of the promoter of glnA (to alter the canonical NtcA binding site) from Prochlorococcus sp. MED4, MIT9313, and SS120 were chemically generated so that the mutated promoters avoided the NtcA binding (Table 1). The dissociation constant Kd for the NtcA—glnA promoter interaction was determined in the three strains (Figure 4, Supplementary Figure 2), using four concentrations of 2OG (0, 1, 5, and 10 mM). We observed clear differences between MIT9313, SS120, and MED4 (Table 2, Figure 4): in the three strains, NtcA interacts with the glnA promoter in the absence of 2OG (Figure 4). The binding affinity of NtcA for the promoter in MIT9313 (Kd = 0.97 μM; the lower Kd, the higher the binding affinity) is higher than in SS120 (Kd = 6.7 μM) and MED4 (Kd = 4.8 μM). Also in the three strains, NtcA interacts with 2OG. The binding affinity for NtcA/2OG (reflected by Kd,oxo; the lower Kd,oxo, the higher the binding affinity) is also higher in MIT9313 (Table 2). This means that the effect of 2OG on NtcA interacting with the glnA promoter will be observed at lower 2OG concentrations in MIT9313 than in SS120 and MED4.
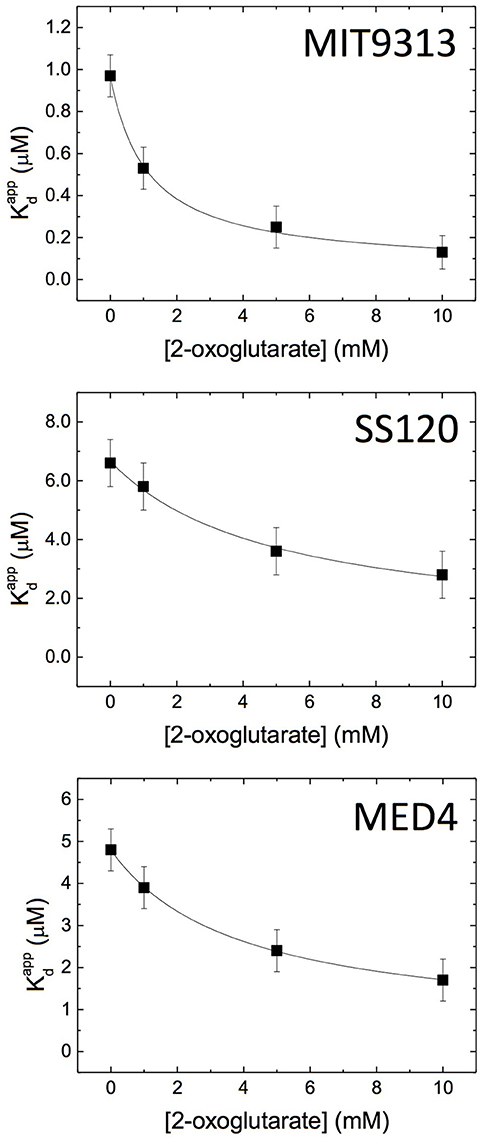
Figure 4. Isothermal titration calorimetry study of the NtcA-PglnA interaction in the Prochlorococcus MED4, MIT9313 and SS120 strains. Apparent dissociation constant () of the interaction of NtcA with the wild-type glnA promoter DNA was determined in the presence of different concentrations of 2OG. Further details are shown in Table 2.
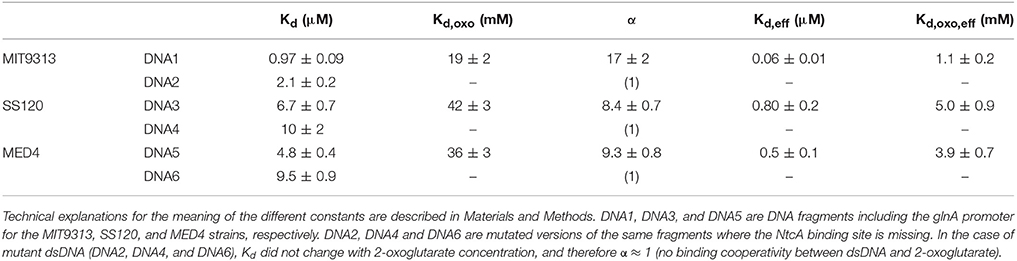
Table 2. Isothermal titration calorimetry parameters of the NtcA—glnA promoter interaction in Prochlorococcus MIT9313, SS120, and MED4.
NtcA shows a positive cooperativity effect (α > 1; Table 2) between 2OG and the glnA promoter in all studied strains. NtcA from MIT9313 is more sensitive to 2OG, showing a higher binding affinity for 2OG (lower Kd,oxo) and a larger cooperativity effect (higher α), resulting in a higher effective affinity to glnA promoter and 2OG in the presence of both ligands. In contrast, NtcA from both SS120 and MED4 behave similarly: they have lower binding affinity and lower cooperative effect from 2OG, compared to MIT9313. It is worth noting that the value of Kd for SS120 and MED4 is roughly 7- and 5-fold higher, respectively, than for MIT9313; these are significant changes, stressing the strong difference of the NtcA responsiveness to 2OG between these strains. For each NtcA variant, the binding affinity for the mutated promoter DNA is lower (higher Kd) than that for the wild-type promoter DNA, and the cooperative effect from 2OG is absent (i.e., α ≈ 1, and increasing 2OG concentration hardly affects the binding affinity of mutated dsDNA; Table 2).
Surface Plasmon Resonance Studies
The differences in the interaction of NtcA with glnA promoter DNA were also studied by Surface Plasmon Resonance, restricted in this case to the two low-light adapted Prochlorococcus strains (MIT9313 and SS120), since the behaviour of MED4 was very similar to SS120, as observed with ITC. As in the case of ITC, promoters of glnA from MIT9313 and SS120 were chemically generated (Table 1) and ntcA from Prochlorococcus sp. SS120 and MIT9313 were heterologously overexpressed (Supplementary Figure 1). The first step was the hybridization of the oligonucleotides to the glnA promoter DNA for each strain, as shown in Supplementary Figure 5. To confirm that 2OG does not bind directly to the chip, a control experiment using 2OG without the protein was performed, and no change in the SPR relative units was observed.
The interaction NtcA-glnA promoter DNA was analyzed with two effector molecules, glutamine and 2OG. The results with different millimolar concentration of glutamine did not show any positive effect on the binding. On the contrary, 2OG had a positive effect on the binding, although it is clearly different between SS120 and MIT9313 (Figure 5). The binding appeared in MIT9313 with 1 mM 2OG and increased with the concentration of 2OG, however a higher level of 2OG was required for the binding in SS120. Although the shape of the sensorgrams hint to an influence of the charge of 2OG on the SPR measurements and consequently these results have to be interpreted with caution, they support the observations of our ITC studies, showing a lower responsiveness to 2OG in Prochlorococcus sp. SS120 strain with respect to MIT9313.
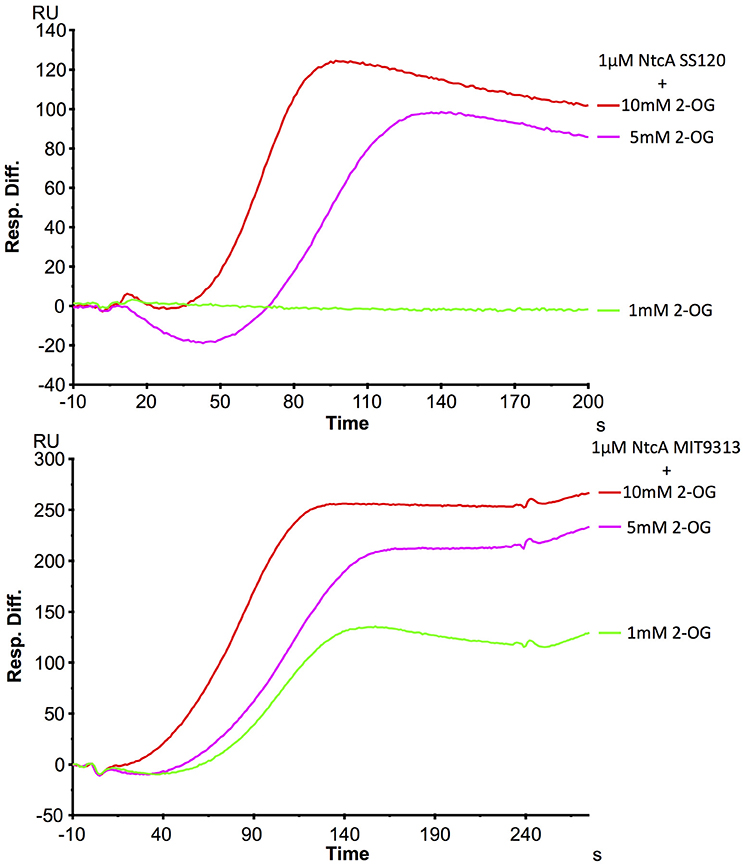
Figure 5. SPR analysis of the interaction between NtcA(His)6 and the promoter for glnA in Prochlorococcus sp. SS120 and MIT9313. This sensorgram shows the interaction of 1 μM of NtcA(His)6 with wild-type glnA promoter DNA and a rising concentration of 2OG. Relative units show the signal difference from FC2 (glnA) and an unspecific oligonucleotide coupled to the reference cell FC1. 1 μM NtcA was used in all assays, in the presence of 1 mM (green), 5 mM (pink), and 10 mM (red) 2OG.
Electrophoretic Mobility Shift Assays
In order to confirm the ITC and SPR results described above, we used EMSA under the same conditions and with the same purified proteins used in the SPR measurements.
Figure 6 shows that the EMSA results of the interaction were in good agreement to those obtained with ITC and SPR: the binding of NtcA from MIT9313 (Figure 6A) was enhanced with 2OG, being stronger when the concentration rises from 1 to 10 mM. In the case of NtcA from SS120, the binding needed a higher concentration of 2OG (Figure 6C). Figure 6B (MIT9313) and D (SS120) show the interaction between NtcA from both strains and the mutated glnA promoter DNA (Table 1). Our EMSA results showed no binding with the mutated promoter (Figures 6B,D), indicating that the GTAN8/9TAC sequence is specific for NtcA from Prochlorococcus. Nevertheless ITC studies, due to their higher sensitivy, showed a small level of binding between NtcA and the mutated promoter (Table 2).
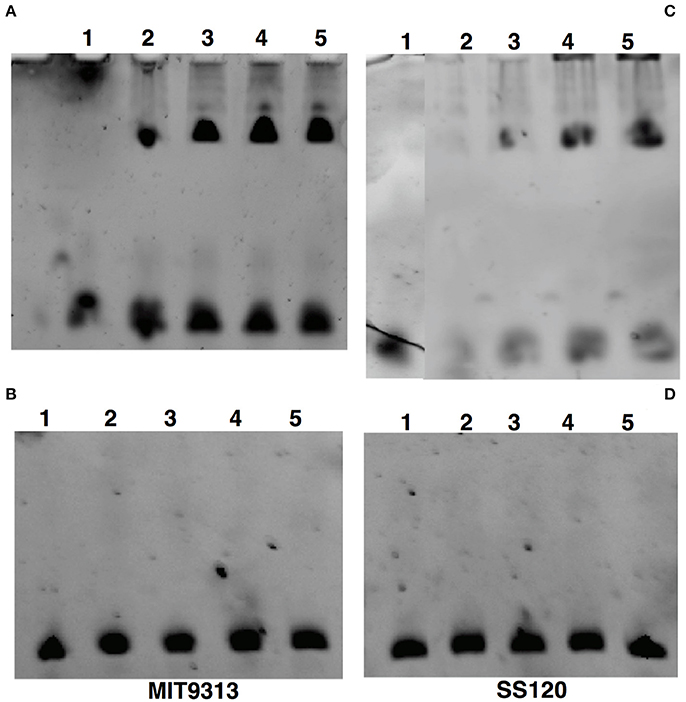
Figure 6. EMSA analysis of the specific binding of NtcA to the glnA promoter of Prochlorococcus sp. MIT9313 (A,B) and SS120 (C,D). (A,C) The binding of 1 μM NtcA to the wild-type glnA promoter DNA was examined by EMSA analysis in the presence of the indicated concentrations of 2OG. (B,D) The binding of 1 μM NtcA in the presence of the indicated concentrations of 2OG to mutated glnA promoter. The samples had the following composition: Lane 1, glnA-promoter; lane 2, glnA-promoter + NtcA (1 μM); lane 3, glnA-promoter + NtcA (1 μM) + 2OG (1 mM); lane 4, glnA-promoter + NtcA (1 μM) + 2OG (5 mM); lane 5, glnA-promoter + NtcA (1 μM) + 2OG (10 mM).
Phylogeny of NtcA in Marine Picocyanobacteria
In order to study the evolution of the ntcA gene in the marine picocyanobacterial radiation, we retrieved a set of ntcA genes from marine Synechococcus and Prochlorococcus strains, together with sequences from freshwater model cyanobacterial strains, which were used as outgroup (i.e., Synechocystis sp. strain PCC 6803, Synechococcus elongatus strain PCC 7942, Nostoc sp. strain PCC 7120). The genes were translated to their amino acid sequence, aligned by using the MUSCLE method, and a phylogenetic tree was constructed with the MEGA 7 software, following the procedures described in the legend of Figure 7. The results are consistent with previous phylogenetic studies on ntcA (Penno et al., 2006), showing the progressive appearance of the different ecotypes in Prochlorococcus and Synechococcus (Figure 7).
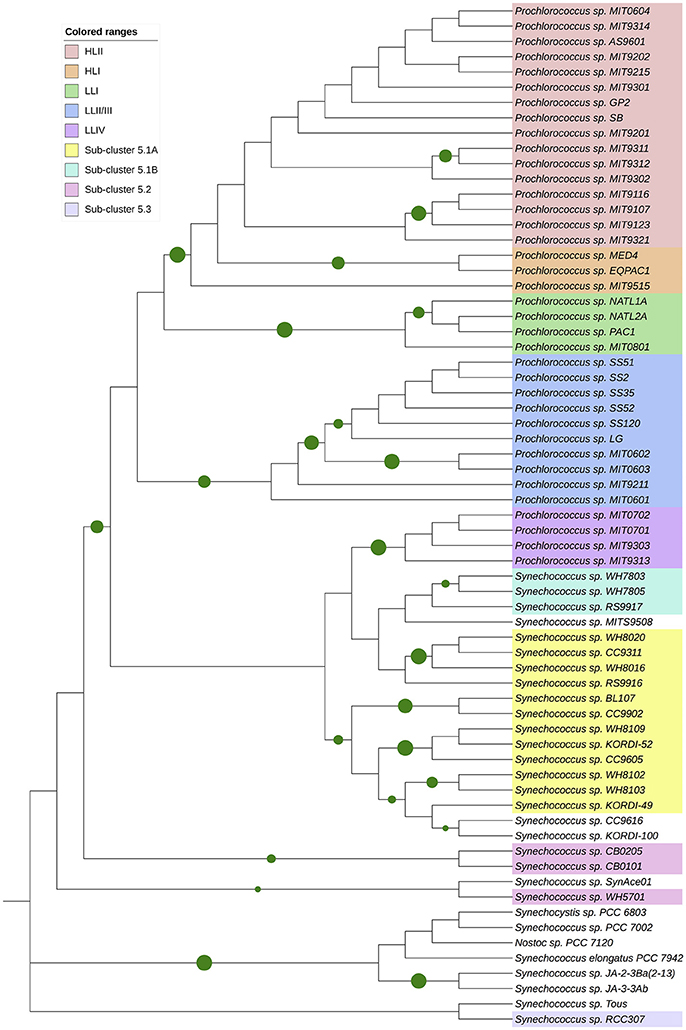
Figure 7. Phylogenetic tree based on cyanobacterial NtcA sequences. Molecular phylogenetic analysis by Maximum Likelihood method. The evolutionary history was inferred by using the Maximum Likelihood method based on the Whelan and Goldman model (Whelan and Goldman, 2001). The tree with the highest log likelihood (−3239.73) is shown. Initial tree(s) for the heuristic search were obtained automatically by applying Neighbor-Join and BioNJ algorithms to a matrix of pairwise distances estimated using a JTT model, and then selecting the topology with superior log likelihood value. Circle sizes correspond to bootstrap value (only values higher than 0.75 are shown). The analysis involved 67 amino acid sequences. All positions containing gaps and missing data were eliminated. There were a total of 210 positions in the final dataset. Evolutionary analyses were conducted in MEGA7 (Kumar et al., 2016), and the tree edited with iTOL (Letunic and Bork, 2016).
Interestingly, the phylogeny of ntcA is in good agreement with previous models established for the evolution of the Prochlorococcus and Synechococcus genera (Moore et al., 1998; Rocap et al., 2002; Dufresne et al., 2008; Scanlan et al., 2009; Biller et al., 2015): both are closely related but show a clear separation.
A striking aspect of this phylogeny was the grouping of four Prochlorococcus strains (including MIT9313) of the LLIV clade with one of the subbranches corresponding to marine Synechococcus strains of the sub-clusters 5.1A and 5.1B, such as WH7803, highlighting the common origin of both genera and the earlier origin of MIT9313, which is considered as one of the oldest Prochlorococcus strains (Rocap et al., 2002). By contrast, the ntcA sequences of Prochlorococcus sp. strains MED4 and SS120 appear in different branches (Figure 7; Penno et al., 2006), corresponding to strains which diverged later in the Prochlorococcus phylogeny (Rocap et al., 2002; Penno et al., 2006).
Discussion
The main goal of this work was to further study the differences in the regulatory response to the C/N balance between Prochlorococcus strains and to understand the molecular underpinnings of the process. 2OG is the molecule utilized by cyanobacteria to perceive the balance between C and N (Muro-Pastor et al., 2001). The binding affinity of the nitrogen control transcription factor, NtcA, is modulated by 2OG (Tanigawa et al., 2002; Vazquez-Bermudez et al., 2002). The role of 2OG as master regulator metabolite is supported by a large body of evidence in bacteria, archaea, and also eukaryotes (Huergo and Dixon, 2015). Therefore, we used several strategies to assess the effects of different 2OG concentration in the regulation of the C/N balance in three model strains of Prochlorococcus.
Early results from our team suggested that azaserine provoked differential changes depending on the Prochlorococcus strain (El Alaoui et al., 2001). The response of the three studied strains to increasing concentrations of azaserine was clearly different (Figures 1–3): MIT9313 showed little changes in the enzymatic activities, while SS120 responded with clear increases. By contrast, the expression of the gene encoding the global nitrogen regulator NtcA was increased in response to the 2OG concentrations in MIT9313, but was very little affected in SS120 (Figure 3). The strain MED4 showed an intermediate behavior. Overall, these results suggested that Prochlorococcus strains have different sensitivities to the increases in the 2OG concentration promoted by the azaserine addition.
NtcA interacts with the binding site GTA N(8/9) TAC (Tolonen et al., 2006) in the glnA promoter DNA from Prochlorococcus. Our results showed that 2OG enhances the interaction between the transcription factor and DNA (Figures 4–6, Table 2), in agreement with the results described in other cyanobacteria (Luque et al., 1994; Tanigawa et al., 2002; Vazquez-Bermudez et al., 2002; Espinosa et al., 2006; Valladares et al., 2008; Kuniyoshi et al., 2011; Forcada-Nadal et al., 2014). Previous studies have addressed this kind of interaction, usually in a single freshwater model strain, such as S. elongatus PCC 7942 (Tanigawa et al., 2002; Vazquez-Bermudez et al., 2002), Synechocystis sp. PCC 6803 (Muro-Pastor et al., 2001; Forcada-Nadal et al., 2014), Nostoc sp. PCC 7120 (Laurent et al., 2005; Valladares et al., 2008; Zhao et al., 2010), or Microcystis aeruginosa PCC 7806 (Kuniyoshi et al., 2011). The modulation of the binding activity of nitrogen transcriptional regulators has also been shown in other bacterial groups (Beckers et al., 2005; Hasselt et al., 2011). However, our results showed that the sensitivity of NtcA to the 2OG binding differs in MIT9313 vs SS120 and MED4. Interestingly, by using EMSA and SPR, we could detect almost no binding at 1 mM 2OG in the case of the SS120 strain, while in MIT9313 the level of binding was already very significant (Figures 5, 6). When this interaction was monitored by ITC (Figure 4, Table 2), we observed a significantly lower equilibrium dissociation constant for the binding NtcA–glnA promoter DNA in MIT9313 than in both SS120 and MED4. Furthermore, the cooperativity effect of 2OG with NtcA in the binding (α in Table 2) was rougly 2-fold higher in MIT9313 than in SS120 or MED4. Besides, the Kd,oxo,eff constant (concentration of 2OG at which the cooperative effect is observed at half extent) shows that the requirements of 2OG for binding are also significantly lower in MIT9313 than in SS120/MED4.
These results evidence an important level of diversity in a critical process of transcriptional regulation, as the NtcA control in cyanobacteria. To our knowledge, such a diverse response in members of the same cyanobacterial genus has not been reported before, and suggests that even central mechanisms of regulation (as the system controlling the C/N balance of cyanobacteria, involving the proteins NtcA-PII-PipX) are subjected to fine tuning by evolutionary pressure.
Previous studies described the Kd constant (in the absence of 2OG) for the NtcA-glnA promoter binding in freshwater cyanobacterial strains, by using either EMSA (Reyes et al., 1997; Vazquez-Bermudez et al., 2002) or SPR (Forcada-Nadal et al., 2014). The reported Kd values were 0.052 μM in S. elongatus PCC 7942 (Vazquez-Bermudez et al., 2002), 0.025 μM (Reyes et al., 1997), and 0.057 μM (Forcada-Nadal et al., 2014) in Synechocystis PCC 6803 (compared to 0.97, 6.7, and 4.8 μM for Prochlorococcus sp. MIT9313, SS120 and MED4, respectively; Table 2). This means that both S. elongatus PCC 7942 and Synechocystis sp. PCC 6803 are more sensitive to 2OG than the three Prochlorococcus strains here studied, and it fits nicely in our hypothesis of progressive loss of responsiveness to 2OG.
In this view, we suggest that transcriptional regulation carried out by NtcA in a 2OG-dependent manner appeared early in the cyanobacterial radiation, since the branch leading to Synechococcus elongatus strain PCC 7942 diverged at the end of the Paleoproterozoic period (ca. 1750 millions of years ago), according to recent phylogenetic studies (Sanchez-Baracaldo et al., 2014). Synechocystis sp. strain PCC 6803 diverged later (in the Neoproterozoic era, ca. 750 million years ago; Sanchez-Baracaldo et al., 2014). Since this strain lives in changing freshwater habitats, it has conserved a similar 2OG-dependent NtcA system (with roughly the same Kd observed in PCC 7942).
The marine picocyanobacterial radiation occurred later in the evolution, giving place to the large diversity observed in the Synechococcus and Prochlorococcus genera (Scanlan et al., 2009). During that radiation, picocyanobacterial strains had to get adapted to different kinds of marine environments, from coastal—subjected to changing conditions—to rather stable oligotrophic gyres of the oceans. Presumably, the more diverse Synechococcus genera would include a range of regulatory C/N systems mediated by NtcA: from ecotypes adapted to variable habitats (where the NtcA system maintains a 2OG responsiveness similar to that of freshwater cyanobacterial strains), to oligotrophic habitats (where the NtcA system is gradually losing responsiveness to 2OG, as the Synechococcus ecotypes are more and more adapted to stable conditions). Future NtcA interaction studies in Synechococcus might allow to verify if this hypothesis holds true.
The whole Prochlorococcus genus appeared quite recently, in evolutive terms, and rapidly evolved a number of genetic features to adapt to oligotrophic, stable ocean environments. This is consistent with the phylogeny of ntcA (Figure 7; Penno et al., 2006), where the NtcA sequence of Prochlorococcus sp. MIT9313 sits next to early branching freshwater strains, while NtcA sequences from both MED4 and SS120 are located in late-branching positions of recently evolved strains.
In this context, we propose that one of the molecular adaptations in Prochlorococcus was to decrease the NtcA responsiveness to 2OG (Figures 4–6, Table 2), compared to the freshwater cyanobacterial ancestors. Specifically, the strain MIT9313 (corresponding to one of the earliest branching Prochlorococcus ecotypes, LLIV) diverged earlier than strain SS120 (ecotype LLII), while MED4 (ecotype HLI) is considered as one of the most recently evolved Prochlorococcus strains (Biller et al., 2015). The progressive loss of NtcA responsiveness to 2OG fits with this evolutive model, leading to a similar response in two rather different (SS120 is adapted to live at depth, while MED4 thrives near the ocean surface) but modern strains. The difference of habitats is reflected in the clearly different genotypes between these model Prochlorococcus strains (Dufresne et al., 2003; Rocap et al., 2003). The recent evolution of both SS120 and MED4 is illustrated by their similar, decreased responsiveness of their NtcA proteins to 2OG, when compared to the older MIT9313. This is consistent with previous work suggesting that the eMIT9313 clade has more complexity in their ability to respond to various stimuli (Kettler et al., 2007).
It is however difficult to establish a direct relationship between our observations in enzymes regulation (Figures 1, 2) and ntcA expression (Figure 3), with the results obtained in the NtcA interaction studies in the three Prochlorococcus strains (Figures 4–6). While an important level of diversity was detected in all those experiments, we found more changes in GS and ICDH in Prochlorococcus SS120 and MED4 than in MIT9313 (Figures 1, 2). In the experiments addressing ntcA expression, MED4 showed the most remarkable changes (with a peak at intermediate azaserine concentrations), while MIT9313 had a progressive increase with the maximum expression at the highest tested azaserine concentration (Figure 3).
This might be partially explained by the involvement of several key regulatory proteins in the C/N balance regulation of Prochlorococcus, as PII (Palinska et al., 2002) and PipX (Espinosa et al., 2014), which have not been studied here and might contribute significantly to our observations. Another important factor might be the relatively short times of our studies (with samples taken 24 h after the beginning of the experiment), which might not be fully representative of the transcriptomic changes relevant in the context of a slow growing organism as Prochlorococcus, which divides once per day in the ocean (Vaulot et al., 1995). A global transcriptomic study addressing the effects of different N sources in Prochlorococcus (Tolonen et al., 2006) showed that the strain MED4 transcriptional response to N deprivation was rapid and transient, whereas the MIT9313 response was slower and sustained. This is coherent with our observations: given that the growth of Prochlorococcus is quite slow, a hypothesis is that it might take a long time to reach a 2OG concentration triggering the NtcA response to N deprivation, explaining the slow but continued transcriptional reply in MIT9313. The rapid but transient response to N limitation in MED4 might be mediated by other regulatory proteins as well (for instance, the high increase in glnB expression, which was not observed in MIT9313; Tolonen et al., 2006). Our team has studied the effect of nitrogen limitation in Prochlorococcus sp. strain SS120 by redox proteomics (Mcdonagh et al., 2012) and quantitative proteomics (Domínguez-Martín et al., 2017); future studies on the two other Prochlorococcus main model strains (MED4 and MIT9313) will allow to elucidate whether the transcriptional interstrain differences are also translated to the proteome.
The results here reported, together with different studies on the regulation of N metabolism in Prochlorococcus (El Alaoui et al., 2001, 2003; Gómez-Baena et al., 2001, 2015; Lindell et al., 2002; Tolonen et al., 2006; López-Lozano et al., 2009; Rangel et al., 2009; Gilbert and Fagan, 2010; Mcdonagh et al., 2012; Domínguez-Martín et al., 2014, 2017), suggest that the regulatory simplification observed in this cyanobacterium (Rocap et al., 2003; García-Fernández and Diez, 2004; García-Fernández et al., 2004; Steglich et al., 2008) is a complex process, involving the loss of regulatory proteins (Kettler et al., 2007) and the modulation in their sensitivity to key metabolites, such as exemplified here: reducing the NtcA responsiveness to 2OG means that Prochlorococcus triggers the N-stress response upon a higher threshold of 2OG (compared to other freshwater cyanobacterial strains), thus saving energy required for production of the N-stress induced proteins. These adaptive changes would mean lower energetic costs involved in the production and maintenance of the sensing and regulatory mechanisms, which would ultimately improve the fitness of Prochlorococcus in very oligotrophic environments.
A recent study proposed a decrease in nutrient flux density during the evolution of Prochlorococcus (Braakman et al., 2017), suggested by a decrease in its maximal growth rate and an increased efficiency in its use of limiting nutrients. Our results are consistent with this later feature: besides other strategies to increase efficiency in the use of low N concentrations (such as reducing the size of the genome and the guanine-cytosine content, Dufresne et al., 2005), the decreased use of amino acids with side chains rich in N (Grzymski and Dussaq, 2011), or the loss of genes required to assimilate oxidized forms of N (López-Lozano et al., 2002; Moore et al., 2002; Rocap et al., 2003), evolving a regulatory NtcA system less responsive to 2OG would optimize the utilization of N in oligotrophic oceans where conditions are rather stable.
Concluding Remarks
The results described in this paper show that a key aspect underlying the diversity of regulation of the C/N balance in Prochlorococcus is the decrease in responsiveness of NtcA to 2OG observed in recently evolved strains. However, besides NtcA, there are other regulatory cyanobacterial proteins involved in the control of N and C, such as the PII protein (Forchhammer and Tandeau De Marsac, 1994), or PipX, which is another global regulator in cyanobacteria (Espinosa et al., 2014). Further studies should address the role of those additional players in the diversity of reponses from Prochlorococcus.
Author Contributions
MAD-M, AL-L, RC-G, AV-C, and GS performed research; MAD-M, AL-L, RC-G, AV-C, GS, AB, JD, and JG-F designed research, analyzed data, wrote, and approved the final version of the manuscript.
Funding
This work was supported by grants BFU2013-44767-P, BFU2013-47064-P, BFU2016-76227-P, and BFU2016-78232-P (Spanish Ministerio de Economía y Competitividad, cofunded by the European Social Fund from the European Union), P12-BIO-2141 (Proyectos de Excelencia, Junta de Andalucía), Universidad de Córdoba (Programa Propio de Investigación and Programa de Fortalecimiento de las Capacidades en I+D+I), Fondo de Investigaciones Sanitarias (PI15/00663), and Centro de Investigacion Biomedica en Red en Enfermedades Hepaticas y Digestivas (CIBERehd). MAD-M received a grant funded by projects BFU-2009-08008/BMC and P07-CVI-3055, and a grant funded by Universidad de Córdoba to work during a short stay in the laboratory of Prof. Andreas Burkovski. RC-G received a grant funded by the Spanish Ministerio de Educacion, Cultura y Deporte.
Conflict of Interest Statement
The authors declare that the research was conducted in the absence of any commercial or financial relationships that could be construed as a potential conflict of interest.
Acknowledgments
We thank the cyanobacterial Pasteur Culture Collection (Institut Pasteur, Paris, France), the Roscoff Culture Collection (Station Biologique, Roscoff, France, through the ASSEMBLE project, grant agreement no. 227799, from the “Capacities” program, 7th Framework Program, European Union) and the MIT Culture Collection (Massachusetts Institute of Technology, Cambridge, Massachusetts, USA) for providing Prochlorococcus strains. We acknowledge the kind collaboration of Instituto Español de Oceanografía for supplying the seawater. We thank Dr. I. Luque (Instituto de Bioquímica Vegetal y Fotosíntesis, Sevilla, Spain) and Prof. M. Fillat (Universidad de Zaragoza, Spain) for helpful discussions and suggestions regarding EMSAs assays. We thank Servicio Centralizado de Apoyo a la Investigación (SCAI, Universidad de Córdoba) for DNA sequencing.
Supplementary Material
The Supplementary Material for this article can be found online at: https://www.frontiersin.org/articles/10.3389/fmicb.2017.02641/full#supplementary-material
Supplementary Figure 1. ITC titration of NtcA with dsDNA promoter of the glnA gene in the Prochlorococcus sp. strains MIT9313, SS120 and MED4. Calorimetric titrations for NtcA interacting with dsDNA sequences corresponding to the glnA promoter from the wild strains (left) MIT9313, (middle) SS120 and (right) MED4, in the absence of 2-oxoglutarate. Calorimetric thermograms (thermal power as a function of time) are shown in the upper plots, and binding isotherms (ligand normalized heat effects as a function of the molar ratio) are shown in the lower plots.
Supplementary Figure 2. Heterologous NtcA(His)6 purified from Prochlorococcus sp. SS120 and MIT9313. 1 and 3 μg of the purified protein were analyzed on a 12% polyacrylamide gel-SDS and stained with Coomassie solution.
Supplementary Figure 3. Multiple sequence alignment of GS from Prochlorococcus MIT9313, SS120 and MED4. The sequences in FASTA format were taken from CYORF and ClustalW2 from EMBL-EBI was used to make the alignment. (∗) Indicates positions which have a single, fully conserved residue; (:) indicates conservation between groups of strongly similar properties and (.) indicates conservation between groups of weakly similar properties. Highlighted amino acids residues involved in the biosynthetic reaction.
Supplementary Figure 4. Multiple sequence alignment of ICDH from Prochlorococcus MIT9313, SS120 and MED4. The sequences in FASTA format were taken from CYORF and ClustalW2 from EMBL-EBI was used to make the alignment. (∗) Indicates positions which have a single, fully conserved residue; (:) indicates conservation between groups of strongly similar properties and (.) indicates conservation between groups of weakly similar properties.
Supplementary Figure 5. Hybridization of glnA promoter. The 26 nt long oligonucleotides were analyzed on a 20% polyacrylamide gel. As control besides the Peqlab ultra low range DNA standard (0.01-0.3 kb) in the lane named MW, the single strand Forward (F) and Reverse (R) were loaded. The successfully hybridized samples are shown in lanes named 1, 2, and 3.
Supplementary Table 1. Oligonucleotides used for cloning and qRT-PCR.
Abbreviations
EMSA, electrophoretic mobility shift assay; ITC, isothermal titration calorimetry; SPR, surface plasmon resonance; 2OG, 2-oxoglutarate.
References
Beckers, G., Strosser, J., Hildebrandt, U., Kalinowski, J., Farwick, M., Kramer, R., et al. (2005). Regulation of AmtR-controlled gene expression in Corynebacterium glutamicum: mechanism and characterization of the AmtR regulon. Mol. Microbiol. 58, 580–595. doi: 10.1111/j.1365-2958.2005.04855.x
Berube, P., Biller, S., Kent, A., Berta-Thompson, J., Roggensack, S., Roache-Johnson, K., et al. (2014). Physiology and evolution of nitrate acquistion in Prochlorococcus. ISME J. 9, 1195–1207. doi: 10.1038/ismej.2014.211
Biller, S., Berube, P., Berta-Thompson, J., Kelly, L., Roggensack, S., Awad, L., et al. (2014). Genomes of diverse isolates of the marine cyanobacterium Prochlorococcus. Sci. Data 1:140034. doi: 10.1038/sdata.2014.34
Biller, S., Berube, P., Lindell, D., and Chisholm, S. (2015). Prochlorococcus: the structure and function of collective diversity. Nat. Rev. Microbiol. 13, 13–27. doi: 10.1038/nrmicro3378
Braakman, R., Follows, M. J., and Chisholm, S. W. (2017). Metabolic evolution and the self-organization of ecosystems. Proc. Natl. Acad. Sci. U.S.A. 114, E3091–E3100. doi: 10.1073/pnas.1619573114
Casey, J., Lomas, M., Mandecki, J., and Walker, D. (2007). Prochlorococcus contributes to new production in the Sargasso Sea deep chlorophyll maximum. Geophys. Res. Lett. 34:L10604. doi: 10.1029/2006GL028725
Chisholm, S., Olson, R., Zettler, E., Goericke, R., Waterbury, J., and Welschmeyer, N. (1988). A novel free living prochlorophyte abundant in the oceanic euphotic zone. Nature 334, 340–343. doi: 10.1038/334340a0
Domínguez-Martín, M. A., Gómez-Baena, G., Diez, J., López-Grueso, M. J., Beynon, R. J., and García-Fernández, J. M. (2017). Quantitative proteomics shows extensive remodeling induced by N limitation in Prochlorococcus sp. SS120. mSystems 2, e0008–0017. doi: 10.1128/mSystems.00008-17
Domínguez-Martín, M. A., López-Lozano, A., Diez, J., Gómez-Baena, G., Rangel-Zú-iga, O., and García-Fernández, J. M. (2014). Physiological regulation of isocitrate dehydrogenase and the role of 2-oxoglutarate in Prochlorococcus sp. strain PCC 9511. PLoS ONE 9:e103380. doi: 10.1371/journal.pone.0103380
Dufresne, A., Garczarek, L., and Partensky, F. (2005). Accelerated evolution associated to genome reduction in a free-living prokaryote. Genome Biol. 6:R14. doi: 10.1186/gb-2005-6-2-r14
Dufresne, A., Ostrowski, M., Scanlan, D., Garczarek, L., Mazard, S., Palenik, B., et al. (2008). Unraveling the genomic mosaic of a ubiquitous genus of marine cyanobacteria. Genome Biol. 9:R90. doi: 10.1186/gb-2008-9-5-r90
Dufresne, A., Salanoubat, M., Partensky, F., Artiguenave, F., Axmann, I., Barbe, V., et al. (2003). Genome sequence of the cyanobacterium Prochlorococcus marinus SS120, a nearly minimal oxyphototrophic genome. Proc. Natl. Acad. Sci. U.S.A. 100, 10020–10025. doi: 10.1073/pnas.1733211100
El Alaoui, S., Diez, J., Humanes, L., Toribio, F., Partensky, F., and García-Fernández, J. (2001). In vivo regulation of glutamine synthetase activity in the marine chlorophyll b-containing cyanobacterium Prochlorococcus sp. strain PCC 9511 (Oxyphotobacteria). Appl. Environ. Microbiol. 67, 2202–2207. doi: 10.1128/AEM.67.5.2202-2207.2001
El Alaoui, S., Diez, J., Toribio, F., Gómez-Baena, G., Dufresne, A., and García-Fernández, J. (2003). Glutamine synthetase from the marine cyanobacteria Prochlorococcus spp.: characterization, phylogeny and response to nutrient limitation. Environ. Microbiol. 5, 412–423. doi: 10.1046/j.1462-2920.2003.00433.x
Espinosa, J., Forchhammer, K., Burillo, S., and Contreras, A. (2006). Interaction network in cyanobacterial nitrogen regulation: PipX, a protein that interacts in a 2-oxoglutarate dependent manner with PII and NtcA. Mol. Microbiol. 61, 457–469. doi: 10.1111/j.1365-2958.2006.05231.x
Espinosa, J., Rodríguez-Mateos, F., Salinas, P., Lanza, V., Dixon, R., De La Cruz, F., et al. (2014). PipX, the coactivar of NtcA, is a global regulator in cyanobacteria. Proc. Natl. Acad. Sci. U.S.A. 111, 2423–2430. doi: 10.1073/pnas.1404097111
Flombaum, P., Gallegos, J. L., Gordillo, R. A., Rincón, J., Zabala, L. L., Jiao, N., et al. (2013). Present and future global distributions of the marine cyanobacteria Prochlorococcus and Synechococcus. Proc. Natl. Acad. Sci. U.S.A. 110, 9824–9829. doi: 10.1073/pnas.1307701110
Flores, E., Frías, J., Rubio, L., and Herrero, A. (2005). Photosynthetic nitrate assimilation in cyanobacteria. Photosynth. Res. 83, 117–133. doi: 10.1007/s11120-004-5830-9
Flores, E., and Herrero, A. (2005). Nitrogen assimilation and nitrogen control in cyanobacteria. Biochem. Soc. Trans. 33, 164–167. doi: 10.1042/BST0330164
Forcada-Nadal, A., Forchhammer, K., and Rubio, V. (2014). SPR analysis of promoter binding of Synechocystis PCC6803 transcription factors NtcA and CRP suggests cross-talk and sheds light on regulation by effector molecules. FEBS Lett. 588, 2270–2276. doi: 10.1016/j.febslet.2014.05.010
Forchhammer, K., and Tandeau De Marsac, N. (1994). The PII protein in the cyanobacterium Synechococcus sp. strain PCC 7942 is modified by serine phosphorylation and signals the cellular N-status. J. Bacteriol. 176, 84–91. doi: 10.1128/jb.176.1.84-91.1994
García-Fernández, J., and Diez, J. (2004). Adaptive mechanisms of the nitrogen and carbon assimilatory pathways in the marine cyanobacteria Prochlorococcus. Res. Microbiol. 155, 795–802. doi: 10.1016/j.resmic.2004.06.009
García-Fernández, J., Tandeau De Marsac, N., and Diez, J. (2004). Streamlined regulation and gene loss as adaptive mechanisms in Prochlorococcus for optimized nitrogen utilization in oligotrophic environments. Microbiol. Mol. Biol. Rev. 68, 630–638. doi: 10.1128/MMBR.68.4.630-638.2004
Gilbert, J., and Fagan, W. (2010). Contrasting mechanisms of proteomic nitrogen thrift in Prochlorococcus. Mol. Ecol. 20, 92–104. doi: 10.1111/j.1365-294X.2010.04914.x
Giovannoni, S. J., Cameron Thrash, J., and Temperton, B. (2014). Implications of streamlining theory for microbial ecology. ISME J. 8, 1553–1565. doi: 10.1038/ismej.2014.60
Goericke, R., and Welschmeyer, N. (1993). The marine prochlorophyte Prochlorococcus contributes significantly to phytoplankton biomass and primary production in the Sargasso Sea. Deep Sea Res. I Oceanogr. Res. Pap. 40, 2283–2294. doi: 10.1016/0967-0637(93)90104-B
Gómez-Baena, G., Diez, J., García-Fernández, J., El Alaoui, S., and Humanes, L. (2001). Regulation of glutamine synthetase by metal-catalyzed oxidative modification in the marine oxyphotobacterium Prochlorococcus. Biochim. Biophys. Acta 1568, 237–244. doi: 10.1016/S0304-4165(01)00226-4
Gómez-Baena, G., Domínguez-Martín, M., Donaldson, R., García-Fernández, J., and Diez, J. (2015). Nutrient starvation makes glutamine synthetase more sensitive to oxidative modification in Prochlorococcus marinus PCC 9511. PLoS ONE 1568, 237–244. doi: 10.1371/journal.pone.0135322
Grzymski, J., and Dussaq, A. (2011). The significance of nitrogen cost minimization in proteomes of marine microorganisms. ISME J. 6, 71–80. doi: 10.1038/ismej.2011.72
Hasselt, K., Rankl, S., Worsch, S., and Burkovski, A. (2011). Adaptation of AmtR-controlled gene expression by modulation of AmtR binding activity in Corynebacterium glutamicum. J. Biotechnol. 154, 156–162. doi: 10.1016/j.jbiotec.2010.09.930
Herrero, A., Muro-Pastor, A., and Flores, E. (2001). Nitrogen control in cyanobacteria. J. Bacteriol. 183, 411–425. doi: 10.1128/JB.183.2.411-425.2001
Huergo, L. F., and Dixon, R. (2015). The emergence of 2-oxoglutarate as a master regulator metabolite. Microbiol. Mol. Biol. Rev. 79, 419–435. doi: 10.1128/MMBR.00038-15
Kettler, G., Martiny, A., Huang, K., Zucker, J., Coleman, M., Rodrigue, S., et al. (2007). Patterns and implications of gene gain and loss in the evolution of Prochlorococcus. PLoS Genet. 3:e231. doi: 10.1371/journal.pgen.0030231
Kumar, S., Stecher, G., and Tamura, K. (2016). MEGA7: Molecular Evolutionary genetics analysis version 7.0 for bigger datasets. Mol. Biol. Evol. 33, 1870–1874. doi: 10.1093/molbev/msw054
Kuniyoshi, T. M., Gonzalez, A., Lopez-Gomollon, S., Valladares, A., Bes, M. T., Fillat, M. F., et al. (2011). 2-oxoglutarate enhances NtcA binding activity to promoter regions of the microcystin synthesis gene cluster. FEBS Lett. 585, 3921–3926. doi: 10.1016/j.febslet.2011.10.034
Laurent, S., Chen, H., Bedu, S., Ziarelli, F., Peng, L., and Zhang, C. (2005). Nonmetabolizable analogue of 2-oxoglutarate elicits heterocyst differentiation under repressive conditions in Anabaena sp. PCC 7120. Proc. Natl. Acad. Sci. U.S.A. 102, 9907–9912. doi: 10.1073/pnas.0502337102
Letunic, I., and Bork, P. (2016). Interactive tree of life (iTOL) v3: an online tool for the display and annotation of phylogenetic and other trees. Nucleic Acids Res. 44, W242–W245. doi: 10.1093/nar/gkw290
Lindell, D., Erdner, D., Marie, D., Prasil, O., Koblizek, M., Le Gall, F., et al. (2002). Nitrogen stress response of Prochlorococcus strain PCC 9511 (Oxyphotobacteria) involves contrasting regulation of ntcA and amt1. J. Phycol. 38, 1113–1124. doi: 10.1046/j.1529-8817.2002.01205.x
Liu, H., Nolla, H., and Campbell, L. (1997). Prochlorococcus growth rate and contribution to primary production in the equatorial and subtropical North Pacific ocean. Aquat. Microb. Ecol. 12, 39–47. doi: 10.3354/ame012039
López-Lozano, A., Diez, J., El Alaoui, S., Moreno-Vivián, C., and García-Fernández, J. (2002). Nitrate is reduced by heterotrophic bacteria but not transferred to Prochlorococcus in non axenic cultures. FEMS Microbiol. Ecol. 41, 151–160. doi: 10.1016/S0168-6496(02)00297-0
López-Lozano, A., Gómez-Baena, G., Mu-oz-Marín, M., Rangel, O., Diez, J., and García-Fernández, J. (2009). Expression of genes involved in nitrogen assimilation and the C/N balance sensing in Prochlorococcus sp. strain SS120. Gene Exp. 14, 279–289. doi: 10.3727/105221609788681204
Luque, I., Flores, E., and Herrero, A. (1994). Molecular mechanism for the operation of nitrogen control in cyanobacteria. EMBO J. 13, 2862–2869.
Luque, I., and Forchhammer, K. (2008). “Nitrogen assimilation and C/N balance sensing,” in The Cyanobacteria. Molecular Biology, Genomics and Evolution, eds A. Herrero and E. Flores (Norfolk: Caister Academic Press), 335–385.
Mcdonagh, B., Domínguez-Martín, M. A., Gómez-Baena, G., López-Lozano, A., Diez, J., Bárcena, J. A., et al. (2012). Nitrogen starvation induces extensive changes in the redox proteome of Prochlorococcus sp. SS120. Environ. Microbiol. Rep. 4, 257–267. doi: 10.1111/j.1758-2229.2012.00329.x
Moore, L., Post, A., Rocap, G., and Chisholm, S. (2002). Utilization of different nitrogen sources by the marine cyanobacteria Prochlorococcus and Synechococcus. Limnol. Oceanogr. 47, 989–996. doi: 10.4319/lo.2002.47.4.0989
Moore, L., Rocap, G., and Chisholm, S. (1998). Physiology and molecular phylogeny of coexisting Prochlorococcus ecotypes. Nature 393, 464–467. doi: 10.1038/30965
Muro-Pastor, M., and Florencio, F. (2003). Regulation of ammonium assimilation in cyanobacteria. Plant Physiol. Biochem. 41, 595–603. doi: 10.1016/S0981-9428(03)00066-4
Muro-Pastor, M., Reyes, J., and Florencio, F. (2001). Cyanobacteria perceive nitrogen status by sensing intracellular 2-oxoglutarate levels. J. Biol. Chem. 276, 38320–38328. doi: 10.1074/jbc.M105297200
Muro-Pastor, M., Reyes, J., and Florencio, F. (2005). Ammonium assimilation in cyanobacteria. Photosyn. Res. 83, 135–150. doi: 10.1007/s11120-004-2082-7
Napolitano, M., Rubio, M. A., Santamaria-Gomez, J., Olmedo-Verd, E., Robinson, N., and Luque, I. (2012). Characterization of the response to zinc deficiency in the cyanobacterium Anabaena sp. strain PCC7120. J. Bacteriol. 194, 2426–2436. doi: 10.1128/JB.00090-12
Palenik, B., Brahamsha, B., Larimer, F., Land, M., Hauser, L., Chain, P., et al. (2003). The genome of a motile marine Synechococcus. Nature 424, 1037–1042. doi: 10.1038/nature01943
Palinska, K. A., Laloui, W., Bedu, S., Loiseaux-De Goer, S., Castets, A., Rippka, R., et al. (2002). The signal transducer PII and bicarbonate acquisition in Prochlorococcus marinus PCC 9511, a marine cyanobacterium naturally deficient in nitrate and nitrite assimilation. Microbiology 148, 2405–2412. doi: 10.1099/00221287-148-8-2405
Partensky, F., Hess, W., and Vaulot, D. (1999). Prochlorococcus, a marine photosynthetic prokaryote of global significance. Microbiol. Mol. Biol. Rev. 63, 106–127.
Penno, S., Lindell, D., and Post, A. (2006). Diversity of Synechococcus and Prochlorococcus populations determined from DNA sequences of the N-regulatory gene ntcA. Environ. Microbiol. 8, 1200–1211. doi: 10.1111/j.1462-2920.2006.01010.x
Pinkus, L. (1977). Glutamine binding sites. Methods Enzymol. 46, 414–427. doi: 10.1016/S0076-6879(77)46049-X
Rangel, O., Gómez-Baena, G., López-Lozano, A., Diez, J., and García-Fernández, J. (2009). Physiological role and regulation of glutamate dehydrogenase in Prochlorococcus MIT9313. Environ. Microbiol. Rep. 1, 56–64. doi: 10.1111/j.1758-2229.2008.00005.x
Reyes, J., Muro-Pastor, M., and Florencio, F. (1997). Transcription of glutamine synthetase genes (glnA and glnN) from the cyanobacterium Synechocystis sp. strain PCC 6803 is differently regulated in response to nitrogen availability. J. Bacteriol. 179, 2678–2689. doi: 10.1128/jb.179.8.2678-2689.1997
Rocap, G., Distel, D., Waterbury, J., and Chisholm, S. (2002). Resolution of Prochlorococcus and Synechococcus ecotypes by using 16S-23S ribosomal DNA internal transcribed spacer sequences. Appl. Environ. Microbiol. 68, 1180–1191. doi: 10.1128/AEM.68.3.1180-1191.2002
Rocap, G., Larimer, F., Lamerdin, J., Malfatti, S., Chain, P., Ahlgren, N., et al. (2003). Genome divergence in two Prochlorococcus ecotypes reflects oceanic niche differentiation. Nature 424, 1042–1047. doi: 10.1038/nature01947
Sanchez-Baracaldo, P., Ridgwell, A., and Raven, J. A. (2014). A neoproterozoic transition in the marine nitrogen cycle. Curr. Biol. 24, 652–657. doi: 10.1016/j.cub.2014.01.041
Scanlan, D., Ostrowski, M., Mazard, S., Dufresne, A., Garczarek, L., Hess, W., et al. (2009). Ecological genomics of marine picocyanobacteria. Microbiol. Mol. Biol. Rev. 73, 249–299. doi: 10.1128/MMBR.00035-08
Steglich, C., Futschik, M., Lindell, D., B, V., Chisholm, S., and Hess, W. (2008). The challenge of regulation in a minimal photoautotroph: non-coding RNAs in Prochlorococcus. PLoS Genet. 4:e1000173. doi: 10.1371/journal.pgen.1000173
Tanigawa, R., Shirokane, M., Maeda Si, S., Omata, T., Tanaka, K., and Takahashi, H. (2002). Transcriptional activation of NtcA-dependent promoters of Synechococcus sp. PCC 7942 by 2-oxoglutarate in vitro. Proc. Natl. Acad. Sci. U.S.A. 99, 4251–4255. doi: 10.1073/pnas.072587199
Tolonen, A., Aach, J., Lindell, D., Johnson, Z., Rector, T., Steen, R., et al. (2006). Global gene expression of Prochlorococcus ecotypes in response to changes in nitrogen availability. Mol. Syst. Biol. 2:53. doi: 10.1038/msb4100087
Valladares, A., Flores, E., and Herrero, A. (2008). Transcription activation by NtcA and 2-oxoglutarate of three genes involved in heterocyst differentiation in the cyanobacterium Anabaena sp. strain PCC 7120. J. Bacteriol. 190, 6126–6133. doi: 10.1128/JB.00787-08
Vaulot, D., Marie, D., Olson, R., and Chisholm, S. (1995). Growth of Prochlorococcus, a photosynthetic prokaryote, in the equatorial Pacific ocean. Science 268, 1480–1482. doi: 10.1126/science.268.5216.1480
Vazquez-Bermudez, M. F., Herrero, A., and Flores, E. (2002). 2-oxoglutarate increases the binding affinity of the NtcA (nitrogen control) transcription factor for the Synechococcus glnA promoter. FEBS Lett. 512, 71–74. doi: 10.1016/S0014-5793(02)02219-6
Velazquez-Campoy, A., Goni, G., Peregrina, J. R., and Medina, M. (2006). Exact analysis of heterotropic interactions in proteins: characterization of cooperative ligand binding by isothermal titration calorimetry. Biophys. J. 91, 1887–1904. doi: 10.1529/biophysj.106.086561
Whelan, S., and Goldman, N. (2001). A general empirical model of protein evolution derived from multiple protein families using a maximum-likelihood approach. Mol. Biol. Evol. 18, 691–699. doi: 10.1093/oxfordjournals.molbev.a003851
Keywords: Prochlorococcus, cyanobacteria, 2-oxoglutarate, streamlined regulation, C/N balance
Citation: Domínguez-Martín MA, López-Lozano A, Clavería-Gimeno R, Velázquez-Campoy A, Seidel G, Burkovski A, Díez J and García-Fernández JM (2018) Differential NtcA Responsiveness to 2-Oxoglutarate Underlies the Diversity of C/N Balance Regulation in Prochlorococcus. Front. Microbiol. 8:2641. doi: 10.3389/fmicb.2017.02641
Received: 27 July 2017; Accepted: 19 December 2017;
Published: 09 January 2018.
Edited by:
George S. Bullerjahn, Bowling Green State University, United StatesReviewed by:
Lisa Moore, University of Southern Maine, United StatesBranko Rihtman, University of Warwick, United Kingdom
Copyright © 2018 Domínguez-Martín, López-Lozano, Clavería-Gimeno, Velázquez-Campoy, Seidel, Burkovski, Díez and García-Fernández. This is an open-access article distributed under the terms of the Creative Commons Attribution License (CC BY). The use, distribution or reproduction in other forums is permitted, provided the original author(s) or licensor are credited and that the original publication in this journal is cited, in accordance with accepted academic practice. No use, distribution or reproduction is permitted which does not comply with these terms.
*Correspondence: José M. García-Fernández, am1nYXJjaWFAdWNvLmVz