- 1Key Laboratory of Bio-resource and Eco-environment, Ministry of Education, College of Life Sciences, Sichuan University, Chengdu, China
- 2Animal Disease Prevention and Food Safety Key Laboratory of Sichuan Province, Chengdu, China
The aim of this study was to evaluate the influence of apramycin administration on the development of antibiotic resistance in Escherichia coli (E. coli) strains isolated from chicken feces and houseflies under field conditions. Chickens in the medicated group (n = 25,000) were given successive prophylactic doses (0.5 mg/l) of apramycin in their drinking water from Days 1 to 5, while no antibiotics were added to the un-medicated groups drinking water (n = 25,000). Over 40 days, a total of 1170 E. coli strains were isolated from fecal samples obtained from medicated and un-medicated chickens and houseflies from the same chicken farm. Apramycin MIC90 values for E. coli strains obtained from the medicated group increased 32–128 times from Days 2 to 6 (256–1024 μg/ml) when compared to those on Day 0 (8 μg/ml). Strains isolated from un-medicated chickens and houseflies had consistently low MIC90 values (8–16 μg/ml) during the first week, but showed a dramatic increase from Days 8 to 10 (128–1024 μg/ml). The apramycin resistance gene aac(3)-IV was detected in E. coli strains from medicated (n = 71), un-medicated (n = 32), and housefly groups (n = 42). All strains positive for aac(3)-IV were classified into 12 pulsed-field gel electrophoresis (PFGE) types. PFGE types A, E, and G were the predominant types in both the medicated and housefly groups, suggesting houseflies play an important role in spreading E. coli-resistant strains. Taken together, our study revealed that apramycin administration could facilitate the occurrence of apramycin-resistant E. coli and the apramycin resistance gene acc(3)-IV. In turn, these strains could be transmitted by houseflies, thus increasing the potential risk of spreading multi-drug-resistant E. coli to the public.
Introduction
Antimicrobial resistance emerges from the use of antimicrobials in animals and the subsequent transfer of resistance bacteria from those animals to the broader environment (Berendonk et al., 2015). The influence of antimicrobial usage on the prevalence of resistant strains in animals is of great concern for wider public health (da Costa et al., 2008; Martins da Costa et al., 2011; Sato et al., 2014).
Apramycin is an aminoglycoside antibiotic that has been used in animal husbandry since the early 1980s. It is still used in several European countries and it was approved for use in China in 1999 (Zhang et al., 2009). It is used to treat or prevent infections caused by Gram-negative bacteria such as colibacillosis, salmonellosis, and bacterial enteritis in poultry, swine, and calves (Antunes et al., 2011). Epidemiological investigations of apramycin-resistant bacteria from food producing animals showed differential prevalence of apramycin resistance in different animals (Choi et al., 2011). To date, there are two known resistance genes that confer resistance to apramycin in E. coli. One is the most prevalent apramycin resistance gene, aac(3)-IV, which codes for an aminoglycoside 3-N-acetyltransferase type-IV enzyme (Davies and Oconnor, 1978). The other is npmA, which was identified in a clinical E. coli strain in 2007 and subsequently found to encode for a 16S rRNA m1A1408 methyltransferase (Wachino et al., 2007).
According to a previous study in China, apramycin-resistant E. coli are not only resistant to apramycin itself, such strains have also been found to be multi-resistant to several other antimicrobial agents (Zhang et al., 2009). This could complicate therapeutic options for bacteriosis treatment in both farm animals and humans (Zhang et al., 2009). A few studies have shown that apramycin treatment caused significant selective pressure in prevalence of resistance E. coli in swine (Mathew et al., 2003; Jensen et al., 2006). However, its influence on E. coli found in chicken has not yet been investigated.
The risk of flies disseminating resistant bacteria from livestock and poultry farms to the public has been a subject of increasing concern. Flies captured from different animal rearing facilities had been shown to be vectors for different microorganisms, some of which may be foodborne pathogens that are potentially threatening to human health (Forster et al., 2007). Moreover, flies also function as transmission vehicles for ESBL-producing E. coli from cattle (Usui et al., 2013) as well as laying hens and broilers (Blaak et al., 2014). However, the influence of apramycin administration on the development of antibiotic resistance in E. coli from chicken feces and houseflies has not been fully investigated.
Given this, our study was designed to evaluate three questions: (i) the influence on the development and persistence of apramycin resistance in E. coli isolated from fecal and houseflies in a chicken farm after preventive use of apramycin; (ii) the relationships between apramycin-resistant E. coil isolated from chicken feces and houseflies; and (iii) the characterization of apramycin-resistant E. coli found in houseflies.
Materials and Methods
Study Setting
This study was conducted in a chicken farm with two different poultry houses (1000 m2 each). The two houses were separated about 50 m to each other. After hatching, 50,000 chickens were equally and randomly allocated into two poultry houses (Day 0). Chickens in the medicated group (n = 25,000) were given successive prophylactic doses (0.5 mg/l) of apramycinsulfate (Shandong Qilu King-phar Pharmaceutical Co., Ltd., Shandong, China) in their drinking water from Days 1 to 5. In comparison, the un-medicated group (n = 25,000) was given drinking water without apramycin. No other antibiotics were used during the study period. Add antibiotic to drinking water for 5 days is the normal production behavior of the laying hens company. This study was carried out without any additional interference with the growth of the chickens. The protocol was approved by the Animal Ethics Committee of Sichuan University. We confirm that the best practice veterinary care and informed consent has been granted by the owners.
Samples were taken from each group as described in Table 1. Specifically, 15 cloacal swabs were collected from both the medicated and un-medicated groups at Day 0 and placed separately into sterile plastic bags. Fifteen sterilized plates were randomly placed under selected cages along two main diagonals of the poultry house containing both the medicated and un-medicated groups. Plates were placed at 12:00 am and withdrawn at 3:00 pm to allow for the collection of fresh fecal samples. Collections occurred on Days 1, 2, 3, 4, 5, 6, 8, 10, 15, 20, 30, and 40. Flies were captured using a sweep net on each sampling day from both of the two houses and approximately 30 flies were individually placed into sterile tubes for later morphological classification. All samples were placed into cool boxes containing ice packs and transported to the lab within 4 h for immediate bacterial isolation.
Bacterial Isolation
The cloacal swabs (n = 30) were separately put into 10 ml phosphate-buffered saline (PBS) and thoroughly vortexed. The resulting suspension was then 10-fold serial diluted with PBS and 100 μl of the dilution was plated onto eosin methylene blue (EMB) agar (Hangzhou Microbial Reagent Co., Ltd., Hangzhou, China) and incubated at 37°C overnight.
Fecal samples were collected from medicated (n = 15) and un-medicated groups (n = 15) at each sampling time. From these fresh fecal samples, 0.1 g was put into 10 ml PBS and thoroughly vortexed. The resulting suspension was 10-fold serial diluted with PBS and 100 μl was plated onto EMB agar and incubated at 37°C overnight.
Houseflies were collected at each sampling time, as previously described. Collected houseflies were morphologically identified using a stereomicroscope and 15 houseflies were randomly chosen for subsequent E. coli isolation. Each housefly was put into 10 ml PBS and thoroughly vortexed. The resulting suspension was 10 times gradient diluted with PBS, 100 μl was plated onto EMB agar, then incubated at 37°C overnight.
After overnight incubation, two colonies from each plate were selected for each sample. All isolates were then confirmed as being E. coli using a biochemical identification kit for Enterobacteriaceae (Hangzhou Microbial Reagent Co. Ltd., Hangzhou, China). All the confirmed E. coli isolates were kept frozen (-70°C) with 25% glycerol pending further analysis.
Antimicrobial Susceptibility Testing
The minimum inhibitory concentration (MIC) of apramycinsulfate (China Institute of Veterinary Drugs Control, Beijing, China) for all E. coli isolates was determined using the agar dilution method following the guidelines of the Clinical and Laboratory Standards Institute [CLSI] (2012a). In short, E. coli strains were subcultured on Luria Bertani (LB) agar at 37°C for 12 h. A clearly separate colony of the E. coli isolate was picked and a suspension of each strain in saline solution was adjusted to match the 0.5 McFarland standard. Mueller–Hinton (MH) plates that contain different apramycinsulfate concentration (0.125–1024 μg/ml) were seeded with a multipoint inoculum replicator and incubated at 35°C for 16–18 h. E. coli ATCC 25922 was used as the quality control strain. MIC data were only accepted if MICs of the control strains were within the required reference ranges. MIC90 (the MIC that ≥90% tested bacteria were inhibited for each sampling group) was used to evaluate the changes trend of apramycin resistance.
Apramycin Resistance Gene Detection
For detection of apramycin resistance genes, genomic DNA was prepared using a QIAamp DNA Mini Kit according to the manufacturer’s instructions (Qiagen Inc., Valencia, CA, United States). Apramycin resistance genes aac(3)-IV and npmA were screened for all E. coli isolates as previously described (Yates et al., 2004; Zhou et al., 2010).
Pulsed-Field Gel Electrophoresis (PFGE) Typing of aac(3)-IV-Positive Strains
The clonal relatedness of aac(3)-IV-positive isolates were typed by PFGE as previously described (Gautom, 1997). Briefly, 145 aac(3)-IV-positive isolates were subcultured on LB agar at 37°C for 12 h. A single colony of each isolate was suspended with cotton swab in about 2 ml of TE buffer. The cell suspensions were adjusted to 20% transmittance by using a bioMérieux Vitek (Hazelwood, MO, United States). Proteinase K and lysozyme were added into 100 ml cell suspensions at final concentration of 1 mg/ml each and then incubated at 37°C for 10–15 min. Following the lysozyme–proteinase K incubation, 7 ml of 20% sodium dodecyl sulfate (50°C) and 140 ml of 1.2% InCert Agarose (50°C) were mixed with each bacterial suspension. Then the mixture was immediately added to plug molds (Bio-Rad Laboratories). After that, each solid plug was transferred to 2-ml round-bottom tubes with 1.5 ml of ESP buffer and incubated at 55°C for 2 h in a water bath. Then five times washes with 8–10 ml TE buffer (50°C) each in a shaker water bath for 15 min were carried out. For restriction endonuclease digestion, two 1-mm-thick slices of each plug were incubated at 37°C for 3 h with 50 U of XbaI enzyme. The plugs were then soaked in standard 0.5 Tris–borate–EDTA (TBE) prior to electrophoresis. The electrophoretic conditions used were as follows: initial switch time, 2.16 s; final switch time, 54.17 s; run time, 22 h; angle, 120°; gradient, 6.0 V/cm; temperature, 14°C; ramping factor, linear. PFGE profiles were analyzed using the BioNumerics Program (Applied Maths, Sint-Martens-Latem, Belgium) as previously described (Yates et al., 2004). The clonal clusters with a similarity cutoff value of 80% were used in this study.
Antimicrobial Resistance Phenotype and Genotype of aac(3)-IV-Positive Strains
To investigate the antimicrobial resistance patterns and resistance genes of aac(3)-IV-positive isolates belonging to different PFGE types, we tested one isolate of each PFGE type for susceptibility to 22 antimicrobial agents. This process was conducted using the disk diffusion method according to CLSI guidelines (Clinical and Laboratory Standards Institute [CLSI], 2012b). Briefly, MH agar plate was inoculated with suspensions of bacteria, equivalent to standard 0.5 McFarland. Subsequently, the disks of different antimicrobial agents were placed on media and then incubated at 35°C for 16–18 h. The tested antimicrobial agents were as follows: ampicillin (10 μg), piperacillin (100 μg), cefazolin (30 μg), ceftazidime (30 μg), cefotaxime (30 μg), ceftriaxone (30 μg), cefepime (30 μg), amoxicillin/clavulanic acid (20/10 μg), ampicillin/sulbactam (10/10 μg), piperacillin/tazobactam (100/10 μg), aztreonam (30 μg), imipenem (10 μg), meropenem (10 μg), tetracycline (30 μg), doxycycline (30 μg), ciprofloxacin (5 μg), levofloxacin (5 μg), gentamicin (10 μg), amikacin (30 μg), sulfamethoxazole/trimethoprim (1.25/23.75 μg), chloramphenicol (30 μg), and florfenicol (30 μg). All tested antimicrobial agents were obtained from Oxoid (Basingstoke, United Kingdom). E. coli ATCC 25922 was used as the control strain. The obtained data were interpreted according to CLSI recommendations (Clinical and Laboratory Standards Institute [CLSI], 2016).
Finally, we screened for the presence of 25 additional types of resistance genes and integron integrates genes in the 12 aac(3)-IV-positive isolates were screened using primers and PCR conditions as previously described: blaTEM, blaSHV, blaOXA-1-like, blaCTX-M-group1, blaCTX-M-group2, blaCTX-M-group9, blaCTX-M-group8/25 (Dallenne et al., 2010), tetA, tetB, tetM (Ng et al., 2001), qnrA, qnrB, qnrC, qnrD (Schink et al., 2012), aac(3)-IIa, aac(6′)-Ib, ant(3″)-Ia, aph(3′)-IIa (Zhang et al., 2012), sulI, sulII (Kerrn et al., 2002), cfr, cmlA, floR (Keyes et al., 2000; Kehrenberg and Schwarz, 2006), IntI, and IntII (Ishikawa, 2011).
Statistical Analysis
Statistical analysis was performed using SPSS software for Windows, version 18.0 (SPSS Inc., Chicago, IL, United States). Data were analyzed using descriptive statistics and χ2 tests. A P-value < 0.05 was considered statistically significant.
Results
Bacterial Isolation
Over the course of the 40-day testing period, a total of 585 samples were collected. Two E. coli strains were selected from each sample. As shown in Table 1, a total of 1170 E. coli isolates from the medicated group (n = 390), un-medicated group (n = 390), and housefly group (n = 390) were obtained. Prior to apramycin administration (Day 0), 90 E. coli strains were collected from the included samples, 450 E. coli strains were collected during apramycin administration (Days 1–5), and 630 E. coli strains were collected after apramycin administration.
The Changes of MIC90 for Apramycin
Minimum inhibitory concentration for apramycin was tested for all 1170 E. coli isolates. MIC90 was used to evaluate the changes trend of apramycin resistance (Figure 1).
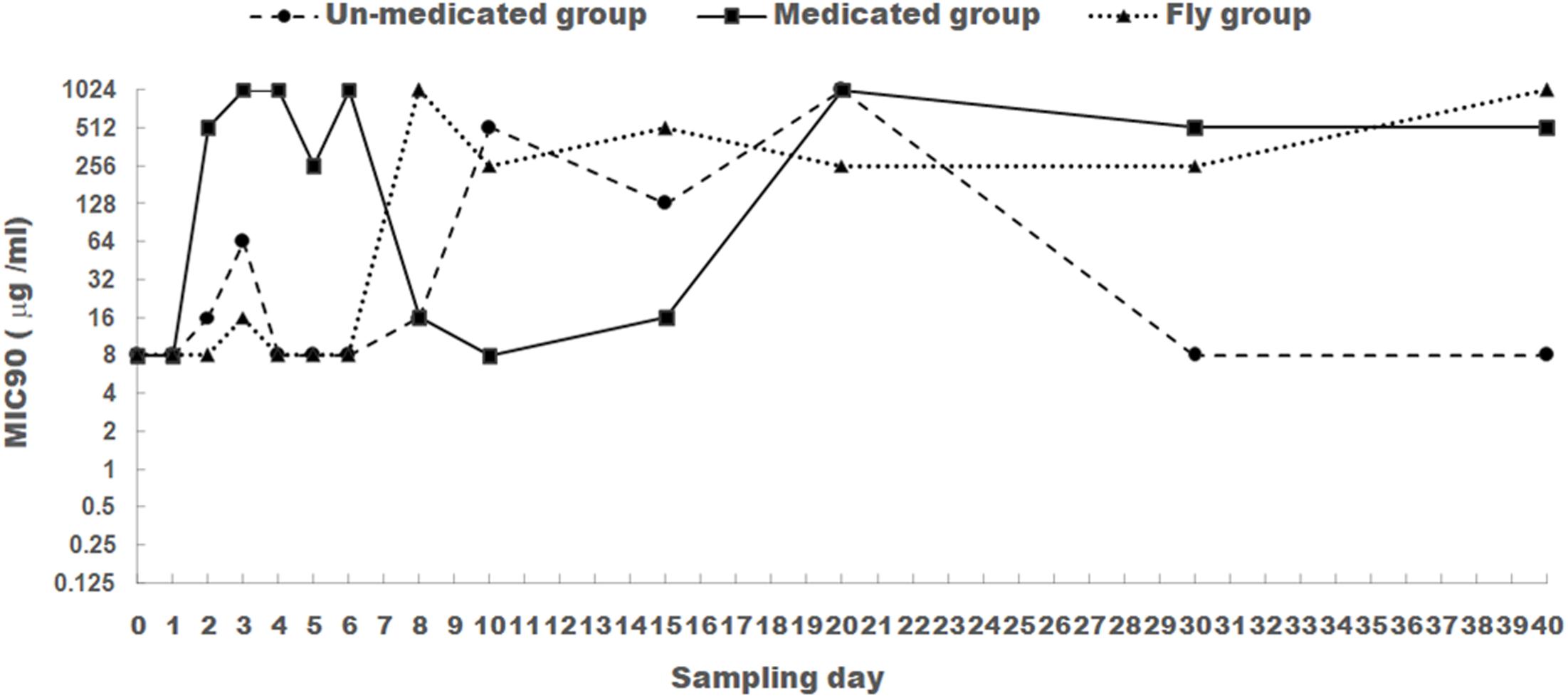
FIGURE 1. The changes of MIC90 for apramycin of E. coli isolated from chicken feces (medicated and un-medicated groups) and houseflies. Apramycin was administrated from Days 1 to 5 in their drinking water (0.5 mg/l) for the medicated group.
For E. coli isolates obtained from the medicated group, apramycin MIC90 was at a low level (8 μg/ml) prior to apramycin administration (Day 0). After the addition of apramycin, MIC90 increased significantly from Days 2 to 6 and was maintained above 512 μg/ml compared to that in Day 0 and Day 1 (P < 0.05). This was with the exception of Day 5, which sustained a level of 256 μg/ml. However, ending apramycin administration resulted in a substantial decrease in MIC90 (8–16 μg/ml) from Days 8 to 15. To our surprise, MIC90 increased again (above 512 μg/ml) from Days 20 to 40.
For E. coli isolates obtained from the un-medicated group, apramycin MIC90 was remained at low level (8–16 μg/ml) from Days 0 to 8. This was with the exception of Day 3, which sustained a level of 64 μg/ml. Days 10–20 saw a dramatic increase (128–1024 μg/ml), but a subsequent decrease to 8 μg/ml from Days 30 to 40. Significant difference was found for the MIC90 values between E. coli isolates from the un-medicated group and medicated group (P < 0.05).
For E. coli isolated from houseflies, apramycin MIC90 remained at a low level (8–16 μg/ml) from Days 0 to 6, then increased and fluctuated between 256 and 1024 μg/ml from Days 8 to 40. MIC90 values for apramycin were significantly different between 1–6 days and 8–40 days for E. coli isolated from houseflies (P < 0.05).
Detection Rates of Apramycin Resistance Gene
Apramycin resistance genes aac(3)-IV and npmA were screened for all 1170 E. coli isolates. Aac(3)-IV was detected in 32, 71, and 42 E. coli isolates from the un-medicated, medicated, and housefly groups, respectively. npmA gene was not detected in any samples from this study. The change of aac(3)-IV frequency is shown in Figure 2.
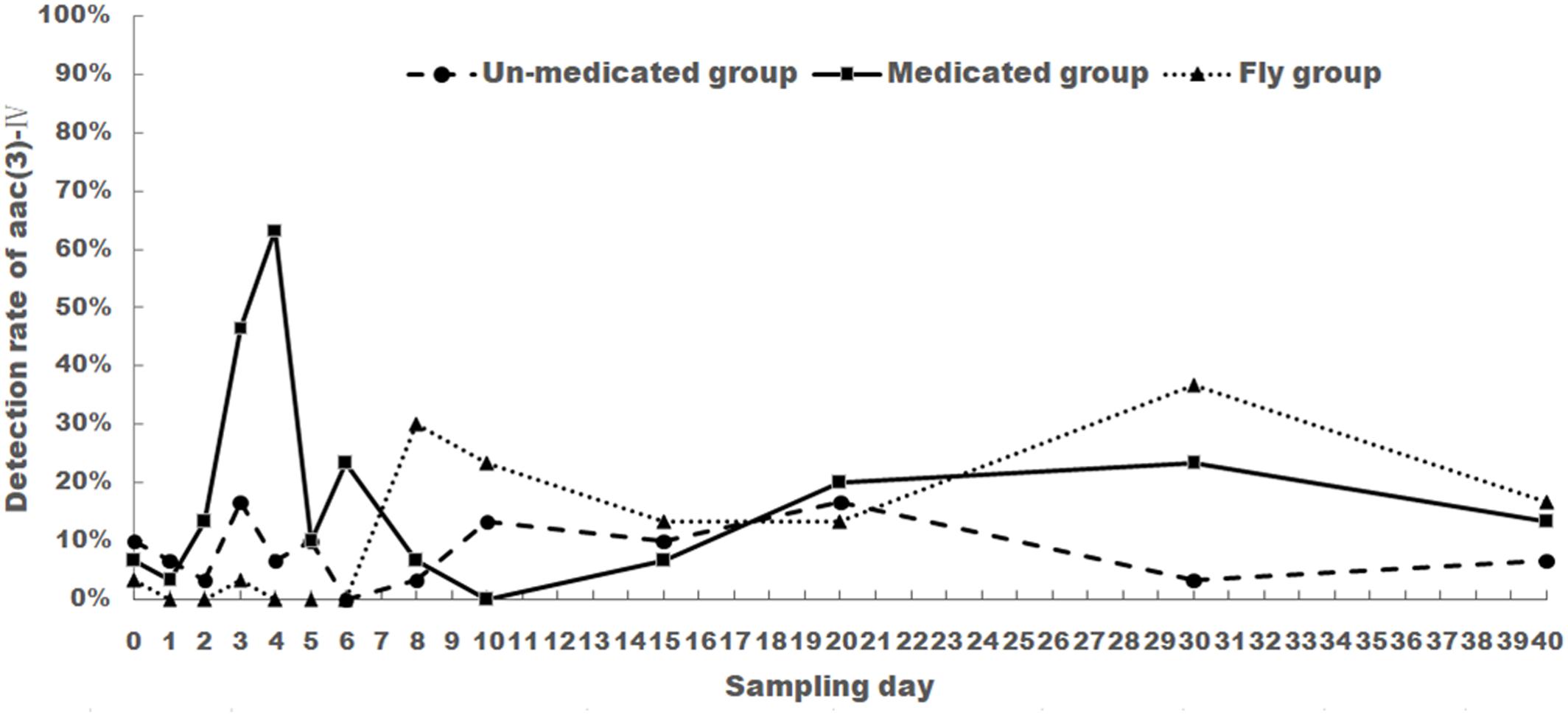
FIGURE 2. The changes of aac(3)-IV detection rate of E. coli isolated from chicken feces (medicated and un-medicated groups) and houseflies. Apramycin was administrated from Days 1 to 5 in their drinking water (0.5 mg/l) for the medicated group.
For the medicated group, aac(3)-IV detection rate was 6.67% before treatment (Day 0) and showed a steady increase from Day 1 (3.33%) to Day 4 (63.33%). Rates then decreased and fluctuated between 0 and 23.33% from Days 5 to 40. Noticeably, aac(3)-IV detection rates were still higher than Day 0. This rate held even 35 days after treatment (Day 40).
For the un-medicated group, aac(3)-IV detection rate showed no drastic change when compared to Day 0. Rates fluctuated between 3.33 and 16.67% for the entirety of the experiment.
For the housefly group, aac(3)-IV detection rate was low from Days 0 to 6 (0–3.33%), then increased and fluctuated between 13.33 and 36.67% from Days 8 to 40.
The aac(3)-IV detection rate was significantly different between medicated group and un-medicated group from days 3 to 4 (P < 0.05). No significant difference was found between un-medicated group and housefly group (P > 0.5).
PFGE Typing of aac(3)-IV-Positive Strains
A total of 145 aac(3)-IV-positive E. coli isolates from the un-medicated (n = 32), medicated (n = 71), and housefly groups (n = 42) were analyzed using PFGE and 12 PFGE types were characterized (Figure 3). Among these, the three predominant PFGE types that emerged in the un-medicated group were types A (n = 12), B (n = 4), and D (n = 5). In the medicated group, the three major types were types A (n = 8), E (n = 39), and G (n = 9) and the housefly group were types A (n = 7), E (n = 11), and G (n = 19). PFGE types A, E, and G were the predominant types in both the medicated and housefly groups, suggesting houseflies play an important role in the spread of antibiotic-resistant E. coli.
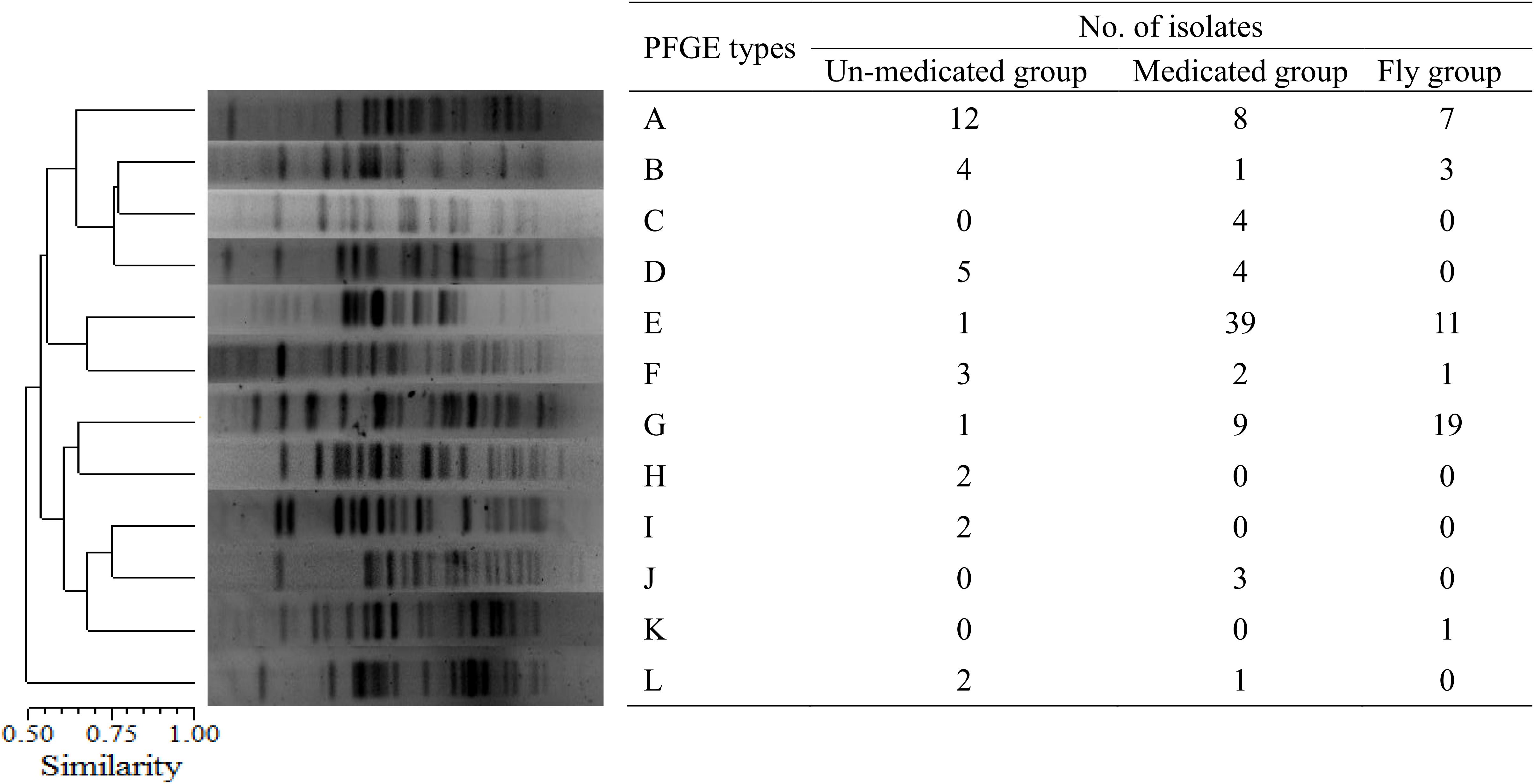
FIGURE 3. PFGE analysis of aac(3)-IV-positive E. coli isolates. A total of 145 aac(3)-IV-positive E. coli isolates from the un-medicated (n = 32), medicated (n = 71), and housefly groups (n = 42) were characterized into 12 PFGE types.
Characterization of Antimicrobial Resistance Phenotype and Genotype of aac(3)-IV-Positive Strains
Antimicrobial resistance profiles of the 12 E. coli isolates from each PFGE type are shown in Table 2. All tested isolates were multi-resistant, showing an antimicrobial-resistant phenotype to 10–18 antibiotics. Furthermore, all 12 isolates were co-resistant to the following antibiotics: ampicillin, tetracycline, doxycycline, ciprofloxacin, levofloxacin, gentamicin, and sulfamethoxazole/trimethoprim. They showed sensitivity to piperacillin/tazobactam, imipenem, meropenem, and amikacin. The number of isolates resistant to other antimicrobials ranged from 4 to 11 (Table 2).
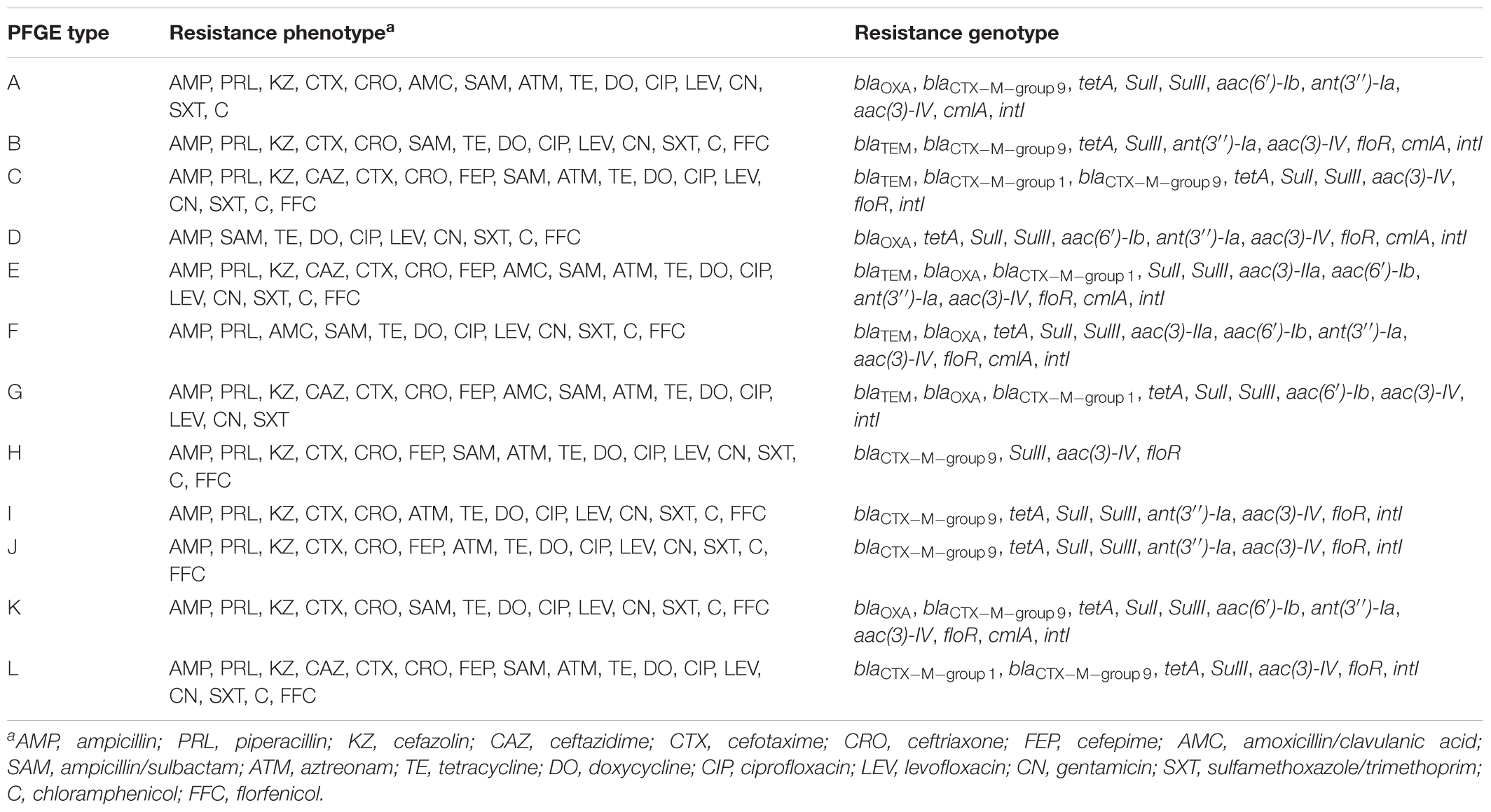
TABLE 2. Antimicrobial resistance profile of aac(3)-IV-positive E. coli isolates of different PFGE types.
Resistance gene screening results showed multiple resistance genes co-existed in all 12 different E. coli isolates from each PFGE type (Table 2). The isolates among the 12 different PFGE types harboring resistance genes other than aac(3)-IV are shown in Table 2. Remarkably, 10 isolates harbored at least one ESBL genes (blaCTX-M-group1or9). Moreover, among the 12 isolates, 11 were positive for the type I integrase gene intI.
Discussion
Increasing attention has been paid to verify whether the extensive uses of antibiotics in food animals poses a risk to human health. Studies regarding the association between antibiotic administration and the development and persistence of resistant bacteria may provide guidance for more accurate antibiotic usage in animal husbandry.
Previous studies have suggested that apramycin administration can promote resistance E. coli isolated from swine (Mathew et al., 2003; Jensen et al., 2006). However, the influence of apramycin administration on E. coli resistance in chicken has not yet been reported. In this study, we demonstrated that the use of apramycin could facilitate E. col I resistance from the first day after administration to 1 day after cessation. Apramycin MIC90 dropped to a relatively low level 3 days after cessation, but increased again from Days 20 to 40 after cessation. Some studies have investigated the influence of other antibiotics on resistance changes of E. coli isolated from different farm animals (Smith et al., 2007; Martins da Costa et al., 2011; Sato et al., 2014). These previous studies have also demonstrated that antimicrobials caused selective pressure and resulted in increased resistance to bacteria originating from animals.
Noticeably, a high MIC90 was persistent even after stopping antibiotic treatment in the medicated group (Days 20–40). This value was higher than prior to antibiotic treatment, results that have also been found in a separate study (Smith et al., 2007). These findings could be due to the clonal dissemination of resistant strains and the capacity of E. coli to exchange resistance genes (da Costa et al., 2009). One of the potential reasons could be due to the dissemination of resistant strains by flies. Because according to the results of MIC90 of the flies group (Figure 1), the MIC90 values remained at a high level (256–1024 mg/ml) from days 20 to 40 in the housefly group.
MIC90 in the un-medicated group also increased at Day 3 and again from Days 10 to 20. This change in antibiotic resistance has also been observed in other studies featuring no antimicrobial treatment (Diarra et al., 2007; da Costa et al., 2009). These findings might be due to the influence of resistant strains in the farm environment and animal feed on microbial composition in the chicken gut (Apajalahti et al., 2004; Martins da Costa et al., 2011). We also hypothesized that the change of resistant phenotype of the un-medicated group was due to the spread of the resistant strains from the medicated group to un-medicated group through environmental factors (e.g., air, dust, mice, and flies). There are two reasons for this: first, compared with medicated group, the increase of MIC90 values of the un-medicated group was relatively delayed. Second, the trend of drug-resistant phenotype of the un-medicated group and housefly group was very similar, which suggested the resistant strains might be spread from the medicated group to un-medicated group by houseflies.
Furthermore, the influence of antimicrobial administration on resistance phenotype and genotype of E. coli isolated from houseflies captured from a poultry farm was investigated for the first time. Our study found that apramycin administration also promoted resistance of E. coli isolated from houseflies. However, the change of apramycin resistance in E. coli isolated from houseflies group was not as synchronous as that seen in the medicated group. To this end, MIC90 values rose from Days 2 to 6 (except for Day 5) in the medicated group, but remained at a low level (8–16 μg/ml) in the housefly group. Furthermore, while MIC90 values dropped from Days 8 to 15 in the medicated group, values rose above 256 μg/ml in the housefly group.
Pulsed-field gel electrophoresis analysis of aac(3)-IV-positive E. coli isolates indicated that the same strains were present in both fecal samples and houseflies. Furthermore, the predominant three PFGE types in the medicated group (A, E, and G) were also the predominant three PFGE types in the housefly group. This suggests that houseflies are transmission vehicles from chicken feces for resistant bacterial strains. Therefore, as the use of antimicrobials increases the presence of resistant strains in food producing animals, it will also likely increase the potential for further dissemination by houseflies to the public. Similar results have been found in pig farms, as E. coli isolates from flies and pigs showed the same resistance phenotype, genes, and PFGE profiles (Literak et al., 2009).
Resistance profiles of the aac(3)-IV-positive isolates of different PFGE types indicated multi-drug resistance was very common, which is consistent with other studies (da Costa et al., 2009; Zhang et al., 2009). Therefore, apramycin administration does not only cause selective effects on resistance itself, but also to other antimicrobials. Noticeably, among these apramycin-resistant isolates, the ESBL-producing strains were very common (10/12). More critically, some of these ESBL-producing strains also existed in houseflies. This would only increase their disseminating opportunity, posing a great potential risk to public health. Other studies have also shown that flies were capable of spreading ESBL-producing E. coli from poultry and cattle (Usui et al., 2013; Blaak et al., 2014).
Conclusion
Our study found that apramycin administration increased the occurrence of aac(3)-IV-resistant isolates from chicken feces and houseflies. Moreover, houseflies transmitted resistant bacteria from chicken feces, thus increasing the potential risk of spreading these multi-resistant isolates to the public. Critical management strategies of antimicrobial usage in animal husbandry and pest control should be undertaken to better control and reduce this risk.
Author Contributions
AZ and HW designed the study. ZG, YY, and YL carried out the sampling work. ZG, HT, and DL performed the experiments. AZ, CX, and CL analyzed the data. AZ and ZG drafted the manuscript. All authors have read and approved the final manuscript.
Funding
This work was supported by the National Key Research and Development Program of China (2016YFD0501608), “973” National Basic Research Program of China (2013CB127200), the General Program of National Natural Science Foundation of China (31572548 and 31572547), Earmarked Fund for Modern Agro-industry Technology Research System (CARS-41-K09), and the Outstanding Young Scholars of Sichuan University (2015SCU04A24).
Conflict of Interest Statement
The authors declare that the research was conducted in the absence of any commercial or financial relationships that could be construed as a potential conflict of interest.
References
Antunes, E. D. B., Lourenco, F. R., and Pinto, T. D. A. (2011). Determination of apramycin in oral soluble powder by a HPLC method using pre-column derivatization with o-phthalaldehyde and UV detection. Braz. J. Pharm. Sci. 47, 261–268. doi: 10.1590/S1984-82502011000200007
Apajalahti, J., Kettunen, A., and Graham, H. (2004). Characteristics of the gastrointestinal microbial communities, with special reference to the chicken. World Poult. Sci. J. 60, 223–232. doi: 10.1079/Wps200415
Berendonk, T. U., Manaia, C. M., Merlin, C., Fatta-Kassinos, D., Cytryn, E., Walsh, F., et al. (2015). Tackling antibiotic resistance: the environmental framework. Nat. Rev. Microbiol. 13, 310–317. doi: 10.1038/nrmicro3439
Blaak, H., Hamidjaja, R. A., van Hoek, A. H., de Heer, L., de Roda Husman, A. M., and Schets, F. M. (2014). Detection of extended-spectrum beta-lactamase (ESBL)-producing Escherichia coli on flies at poultry farms. Appl. Environ. Microbiol. 80, 239–246. doi: 10.1128/AEM.02616-13
Choi, M. J., Lim, S. K., Nam, H. M., Kim, A. R., Jung, S. C., and Kim, M. N. (2011). Apramycin and gentamicin resistances in indicator and clinical Escherichia coli isolates from farm animals in Korea. Foodborne Pathog. Dis. 8, 119–123. doi: 10.1089/fpd.2010.0641
Clinical and Laboratory Standards Institute [CLSI] (2012a). Methods for Dilution Antimicrobial Susceptibility Tests for Bacteria that Grow Aerobically; Approved Standard, 9rd Edn. Wayne, PA: CLSI.
Clinical and Laboratory Standards Institute [CLSI] (2012b). Performance Standards for Antimicrobial Disk Susceptibility Tests; Approved Standard, 11th Edn. Wayne, PA: CLSI.
Clinical and Laboratory Standards Institute [CLSI] (2016). Performance Standards for Antimicrobial Susceptibility Testing; Twenty-sixth Informational Supplement M100S. Wayne, PA: CLSI.
da Costa, P. M., Belo, A., Goncalves, J., and Bernardo, F. (2009). Field trial evaluating changes in prevalence and patterns of antimicrobial resistance among Escherichia coli and Enterococcus spp. isolated from growing broilers medicated with enrofloxacin, apramycin and amoxicillin. Vet. Microbiol. 139, 284–292. doi: 10.1016/j.vetmic.2009.06.006
da Costa, P. M., Bica, A., Vaz-Pires, P., and Bernardo, F. (2008). Effects of antimicrobial treatment on selection of resistant Escherichia coli in broiler fecal flora. Microb. Drug Resist. 14, 299–306. doi: 10.1089/mdr.2008.0859
Dallenne, C., Da Costa, A., Decre, D., Favier, C., and Arlet, G. (2010). Development of a set of multiplex PCR assays for the detection of genes encoding important beta-lactamases in Enterobacteriaceae. J. Antimicrob. Chemother. 65, 490–495. doi: 10.1093/jac/dkp498
Davies, J., and Oconnor, S. (1978). Enzymatic modification of aminoglycoside antibiotics - 3-N-acetyltransferase with broad specificity that determines resistance to novel aminoglycoside apramycin. Antimicrob. Agents Chemother. 14, 69–72. doi: 10.1128/AAC.14.1.69
Diarra, M. S., Silversides, F. G., Diarrassouba, F., Pritchard, J., Masson, L., Brousseau, R., et al. (2007). Impact of feed supplementation with antimicrobial agents on growth performance of broiler chickens, Clostridium perfringens and Enterococcus counts, and antibiotic resistance phenotypes and distribution of antimicrobial resistance determinants in Escherichia coli isolates. Appl. Environ. Microbiol. 73, 6566–6576. doi: 10.1128/AEM.01086-07
Forster, M., Klimpel, S., Mehlhorn, H., Sievert, K., Messler, S., and Pfeffer, K. (2007). Pilot study on synanthropic flies (e.g. Musca, Sarcophaga, Calliphora, Fannia, Lucilia, Stomoxys) as vectors of pathogenic microorganisms. Parasitol. Res. 101, 243–246. doi: 10.1007/s00436-007-0522-y
Gautom, R. K. (1997). Rapid pulsed-field gel electrophoresis protocol for typing of Escherichia coli O157:H7 and other gram-negative organisms in 1 day. J. Clin. Microbiol. 35, 2977–2980.
Ishikawa, S. (2011). Simultaneous PCR detection of multiple classes of integron integrase genes for determining the presence of multidrug-resistant bacteria in environmental samples. Curr. Microbiol. 62, 1677–1681. doi: 10.1007/s00284-011-9913-5
Jensen, V. F., Jakobsen, L., Emborg, H. D., Seyfarth, A. M., and Hammerum, A. M. (2006). Correlation between apramycin and gentamicin use in pigs and an increasing reservoir of gentamicin-resistant Escherichia coli. J. Antimicrob. Chemother. 58, 101–107. doi: 10.1093/jac/dkl201
Kehrenberg, C., and Schwarz, S. (2006). Distribution of florfenicol resistance genes fexA and cfr among chloramphenicol-resistant Staphylococcus isolates. Antimicrob. Agents Chemother. 50, 1156–1163. doi: 10.1128/AAC.50.4.1156-1163.2006
Kerrn, M. B., Klemmensen, T., Frimodt-Moller, N., and Espersen, F. (2002). Susceptibility of Danish Escherichia coli strains isolated from urinary tract infections and bacteraemia, and distribution of sul genes conferring sulphonamide resistance. J. Antimicrob. Chemother. 50, 513–516. doi: 10.1093/jac/dkf164
Keyes, K., Hudson, C., Maurer, J. J., Thayer, S., White, D. G., and Lee, M. D. (2000). Detection of florfenicol resistance genes in Escherichia coli isolated from sick chickens. Antimicrob. Agents Chemother. 44, 421–424. doi: 10.1128/AAC.44.2.421-424.2000
Literak, I., Dolejska, M., Rybarikova, J., Cizek, A., Strejckova, P., Vyskocilova, M., et al. (2009). Highly variable patterns of antimicrobial resistance in commensal Escherichia coli isolates from pigs, sympatric rodents, and flies. Microb. Drug Resist. 15, 229–237. doi: 10.1089/mdr.2009.0913
Martins da Costa, P., Oliveira, M., Ramos, B., and Bernardo, F. (2011). The impact of antimicrobial use in broiler chickens on growth performance and on the occurrence of antimicrobial-resistant Escherichia coli. Livest. Sci. 136, 262–269. doi: 10.1016/j.livsci.2010.09.016
Mathew, A. G., Arnett, D. B., Cullen, P., and Ebner, P. D. (2003). Characterization of resistance patterns and detection of apramycin resistance genes in Escherichia coli isolated from swine exposed to various environmental conditions. Int. J. Food Microbiol. 89, 11–20. doi: 10.1016/S0168-1605(03)00124-7
Ng, L. K., Martin, I., Alfa, M., and Mulvey, M. (2001). Multiplex PCR for the detection of tetracycline resistant genes. Mol. Cell. Probes 15, 209–215. doi: 10.1006/mcpr.2001.0363
Sato, T., Okubo, T., Usui, M., Yokota, S., Izumiyama, S., and Tamura, Y. (2014). Association of veterinary third-generation cephalosporin use with the risk of emergence of extended-spectrum-cephalosporin resistance in Escherichia coli from dairy cattle in Japan. PLoS One 9:e96101. doi: 10.1371/journal.pone.0096101
Schink, A. K., Kadlec, K., and Schwarz, S. (2012). Detection of qnr genes among Escherichia coli isolates of animal origin and complete sequence of the conjugative qnrB19-carrying plasmid pQNR2078. J. Antimicrob. Chemother. 67, 1099–1102. doi: 10.1093/jac/dks024
Smith, J. L., Drum, D. J., Dai, Y., Kim, J. M., Sanchez, S., Maurer, J. J., et al. (2007). Impact of antimicrobial usage on antimicrobial resistance in commensal Escherichia coli strains colonizing broiler chickens. Appl. Environ. Microbiol. 73, 1404–1414. doi: 10.1128/AEM.01193-06
Usui, M., Iwasa, T., Fukuda, A., Sato, T., Okubo, T., and Tamura, Y. (2013). The role of flies in spreading the extended-spectrum beta-lactamase gene from cattle. Microb. Drug Resist. 19, 415–420. doi: 10.1089/mdr.2012.0251
Wachino, J., Shibayama, K., Kurokawa, H., Kimura, K., Yamane, K., Suzuki, S., et al. (2007). Novel plasmid-mediated 16S rRNA m1A1408 methyltransferase, NpmA, found in a clinically isolated Escherichia coli strain resistant to structurally diverse aminoglycosides. Antimicrob. Agents Chemother. 51, 4401–4409. doi: 10.1128/AAC.00926-07
Yates, C. M., Pearce, M. C., Woolhouse, M. E., and Amyes, S. G. (2004). High frequency transfer and horizontal spread of apramycin resistance in calf faecal Escherichia coli. J. Antimicrob. Chemother. 54, 534–537. doi: 10.1093/jac/dkh353
Zhang, T., Wang, C. G., Jiang, G. E., Lv, J. C., and Zhong, X. H. (2012). Molecular epidemiological survey on aminoglycoside antibiotics-resistant genotype and phenotype of avian Escherichia coli in North China. Poult. Sci. 91, 2482–2486. doi: 10.3382/ps.2012-02400
Zhang, X. Y., Ding, L. J., and Fan, M. Z. (2009). Resistance patterns and detection of aac(3)-IV gene in apramycin-resistant Escherichia coli isolated from farm animals and farm workers in northeastern of China. Res. Vet. Sci. 87, 449–454. doi: 10.1016/j.rvsc.2009.05.006
Keywords: apramycin resistance genes, Escherichia coli, PFGE, chicken feces, housefly
Citation: Zhang A, Li Y, Guan Z, Tuo H, Liu D, Yang Y, Xu C, Lei C and Wang H (2018) Characterization of Resistance Patterns and Detection of Apramycin Resistance Genes in Escherichia coli Isolated from Chicken Feces and Houseflies after Apramycin Administration. Front. Microbiol. 9:328. doi: 10.3389/fmicb.2018.00328
Received: 11 August 2017; Accepted: 12 February 2018;
Published: 27 February 2018.
Edited by:
Gilberto Igrejas, University of Trás-os-Montes and Alto Douro, PortugalReviewed by:
Liang Li, Los Angeles Biomedical Research Institute, United StatesSunil D. Saroj, Symbiosis International (Deemed University), India
Copyright © 2018 Zhang, Li, Guan, Tuo, Liu, Yang, Xu, Lei and Wang. This is an open-access article distributed under the terms of the Creative Commons Attribution License (CC BY). The use, distribution or reproduction in other forums is permitted, provided the original author(s) and the copyright owner are credited and that the original publication in this journal is cited, in accordance with accepted academic practice. No use, distribution or reproduction is permitted which does not comply with these terms.
*Correspondence: Hongning Wang, d2hvbmduaW5nQDE2My5jb20=