- 1Department of Conservative Dentistry and Periodontology, University Medical Center Regensburg, Regensburg, Germany
- 2Division of Oral Microbiology and Immunology, Clinic of Preventive Dentistry, Periodontology and Cariology, Center of Dental Medicine, University of Zurich, Zurich, Switzerland
- 3Division of Oral Diseases, Department of Dental Medicine, Karolinska Institutet, Solna, Sweden
- 4Department of Dermatology, University Medical Center Regensburg, Regensburg, Germany
In light of increasing resistance toward conventional antibiotics and antiseptics, antimicrobial photodynamic therapy (aPDT) may be a valuable alternative, especially for use in dentistry. In this regard, photosensitizers (PS) based on a phenalen-1-one structure seem to be especially favorable due to their high singlet oxygen quantum yield. However, the actual target structures of phenalen-1-one-mediated aPDT are still unclear. The aim of the present study was to investigate the antimicrobial efficacy of aPDT mediated by phenalen-1-one derivatives SAPYR and SAGUA for inactivation of a polymicrobial biofilm consisting of three putative periodontal pathogens in vitro and to get first insights in the mechanism of action of phenalen-1-one-mediated aPDT by assessing damage of cytoplasmic membranes. aPDT with SAPYR exhibited identical antimicrobial efficacy as compared to chlorhexidine (CHX) [4.4–6.1 log10 reduction of colony forming units (CFUs) depending on bacterial species] while aPDT with SAGUA was less effective (2.0–2.8 log10). Flow cytometric analysis combined with propidium iodide (PI) staining revealed no damage of cytoplasmic membranes after aPDT with both phenalen-1-one derivatives, which was confirmed by spectroscopic measurements for release of nucleic acids after treatment. Spectrophotometric PS-uptake measurements showed no uptake of SAPYR by bacterial cells. Despite the inability to pinpoint the actual target of phenalen-1-one-mediated aPDT, this study shows the high antimicrobial potential of phenalen-1-on mediated aPDT (especially when using SAPYR) and represents a first step for getting insights in the mechanism and damage patterns of aPDT with this class of PS.
Introduction
Periodontal disease is among the most prevalent diseases worldwide according to the most recent Global Burden of Disease study (1990–2015) affecting more than 537 million adults (GBD 2015 Disease and Injury Incidence and Prevalence Collaborators, 2016) and it is known to be the major cause for tooth loss (Tonetti et al., 2017). For patients suffering from aggressive periodontitis or severe or refractory forms of chronic periodontitis, it is common clinical practice to prescribe antibiotics (i.e., amoxicillin and metronidazole) adjunctively to subgingival debridement (van Winkelhoff et al., 1996; Zandbergen et al., 2013). However, this attracts critical voices nowadays (Preus et al., 2014), as more periodontal pathogens increasingly exhibit resistance toward these antibiotics (Rams et al., 2014). Rams et al. (2014) tested subgingival biofilm specimens from 400 adult patients suffering from chronic periodontitis in the United States and found one or more species resistant against either amoxicillin or metronidazole in approximately 43 or 30% of the patients, while 15% harbored subgingival periodontal pathogens resistant to both amoxicillin and metronidazole. Furthermore, recently first strains have been reported that exhibit resistance against the oral gold-standard antiseptic chlorhexidine (CHX) in vitro (Horner et al., 2012; Kitagawa et al., 2016), which may even be linked to multi-drug resistance (Saleem et al., 2016). Therefore, it has been recommended that its use should be limited to the applications “with a clear patient benefit” (i.e., in intensive care patients) in order to reduce the risk of inducing acquired resistances to CHX in pathogens (Kampf, 2016).
In the recent 2016 Review on Antimicrobial Resistance, the alarming scenario was built that the number of deaths due to antimicrobial resistance could increase to 10 million per year in 2050, potentially leading to cumulative economical costs of 100 trillion USD, if no action is taken immediately (O’Neill, 2016). Especially in dentistry, where usually no life-threatening diseases need to be treated, it should be a major goal to restrict the use of conventional antimicrobials and promote research on novel antimicrobial approaches with less risk of inducing resistance. In this light, antimicrobial photodynamic therapy (aPDT) may be a promising alternative (Gursoy et al., 2013). The principle of aPDT is based on irradiation of a per se non-toxic dye, the so-called photosensitizer (PS), by light of an appropriate wavelength in the presence of molecular oxygen. The absorption of light by the PS-molecule leads to its transition to an excited state, whereupon there are two mechanisms to let the PS regain its ground state: In the type I mechanism, charge is transferred to a substrate or molecular oxygen resulting in generation of reactive oxygen species (ROS) like superoxide anions (), hydrogen peroxide (H2O2), or free hydroxyl radicals (HO∙). In type II mechanism, energy (but no charge) is transferred directly to molecular oxygen, whereby singlet oxygen (1O2) emerges, which is regarded as the most effective ROS. Both processes can occur simultaneously in a PS, while the singlet oxygen quantum yield ΦΔ describes the proportion of type II mechanism (Maisch et al., 2007; Cieplik et al., 2014; Wainwright et al., 2017).
In general, aPDT-mediated killing of bacteria is based on unselective oxidative damage of bacterial proteins, lipids and nucleic acids (Almeida et al., 2015). Thereby, oxidative damage of two major cellular components, i.e., cytoplasmic membranes and DNA, has been proposed (Alves et al., 2014; Almeida et al., 2015), but is still a matter of debate since the actual target sites mostly hinge on the respective cellular localization of the PS, which in turn depends on its chemical structure (molecular weight, charge, lipophilicity) and the cell wall characteristics of the targeted bacteria (Alves et al., 2014; Kashef and Hamblin, 2017). For damage of DNA, a PS has to build up high intracellular concentrations (Cadet et al., 2010), wherefore it seems reasonable that external structures like cell walls and cytoplasmic membranes are first-line targets and nucleic acids may only be affected when these external structures have already been destroyed (Almeida et al., 2015). Accordingly, classic studies show that the extremophile bacterium Deinococcus radiodurans can be easily inactivated by aPDT despite its highly efficient DNA repair mechanisms (Schäfer et al., 1998; Nitzan and Ashkenazi, 1999).
Phenalen-1-one-mediated aPDT has already shown its high antimicrobial potential and may be particularly advantageous since PS based on a phenalen-1-one structure nearly quantitatively react according to the type II mechanism (singlet oxygen quantum yields ΦΔ ≥ 0.85) (Cieplik et al., 2013, 2015, 2016, 2018; Späth et al., 2014; Tabenski et al., 2016). However, target structures of aPDT with this class of PS are still unknown. Therefore, the aim of the present study was to combine traditional culture techniques and flow cytometry in order to (I) investigate the antimicrobial efficacy of phenalen-1-one-mediated aPDT for inactivation of a polymicrobial biofilm cultured from three putative periodontal pathogens in vitro and (II) to get first insights in its mechanism of action by assessing damage of cytoplasmic membranes.
Materials and Methods
Chemicals and Light Source
SAPYR (1-((1-Oxo-1H-phenalen-2-yl)methyl)-pyridinium chlo-ride) and SAGUA (1-((1-Oxo-1H-phenalen-2-yl)methyl)-1-methyl-guanidinium chloride) were provided by TriOptoTec GmbH (Regensburg, Germany), synthesized according to published protocols yielding a purity ≥99% (Cieplik et al., 2013; Späth et al., 2014; Tabenski et al., 2016). PS-suspensions were freshly prepared for the experiments in a concentration of 100 μM (dissolved in distilled water) and stored in the dark at 4°C for no longer than 2 weeks. For irradiation of both PS, a gas-discharge lamp (Waldmann PIB 3000; Waldmann Medizintechnik, Villingen-Schwenningen, Germany) was used (λem 380–600 nm). Irradiance was adjusted to 50 mW/cm2 at sample-level and irradiation was for a period of 10 min, resulting in an energy dose of 30 J/cm2.
Chlorhexidine digluconate (CHX) was obtained from the pharmacy department of the University Medical Center Regensburg in concentrations of 0.06 and 0.2% (w/v; dissolved in distilled water). Metronidazole (MET; Sigma-Aldrich, St. Louis, MO, United States) was diluted in distilled water yielding concentrations of 15 and 50 μg/mL.
Figure 1 shows the chemical structures of SAPYR, SAGUA, CHX, and MET.
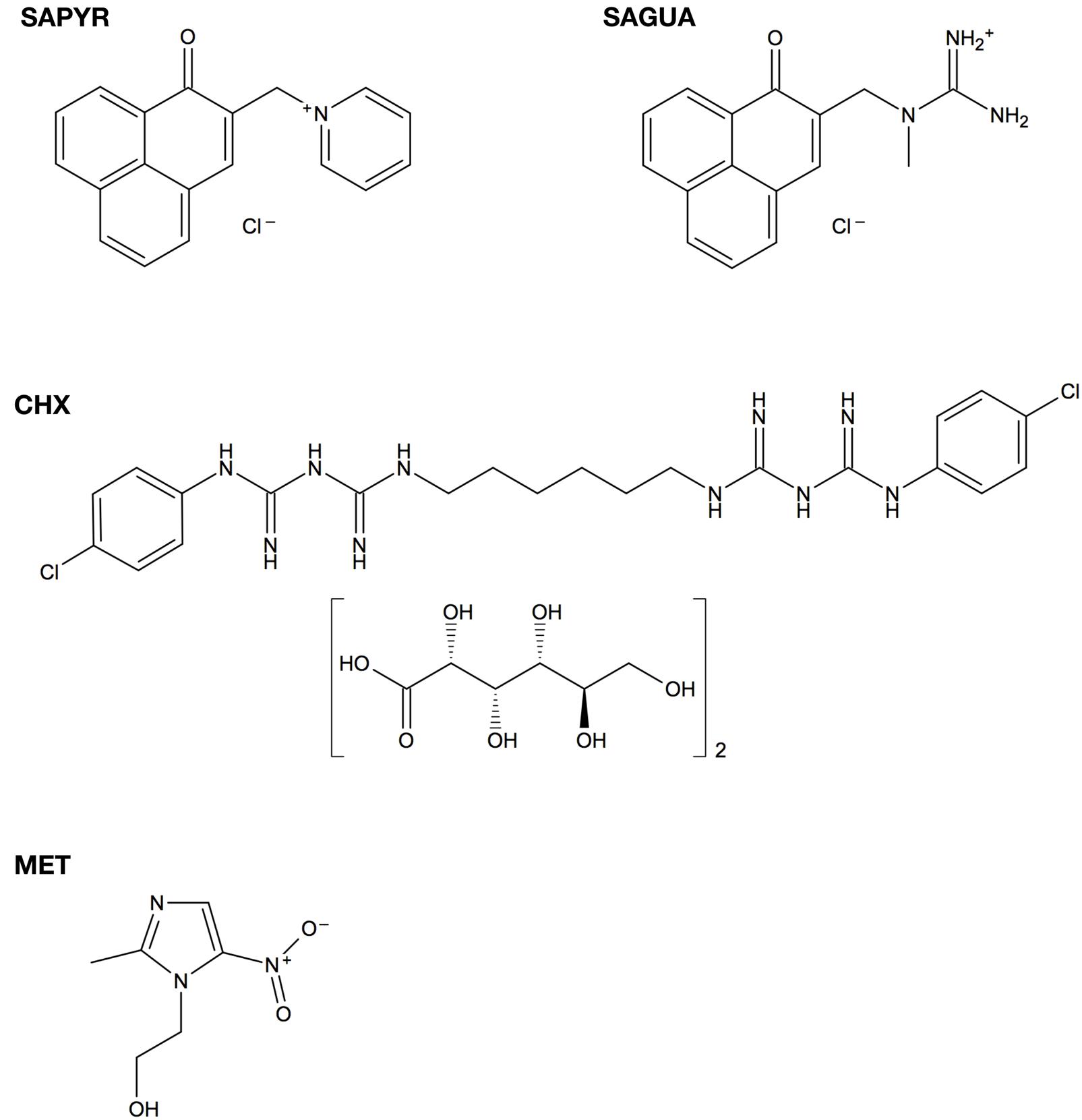
FIGURE 1. Chemical structures of SAPYR, SAGUA, chlorhexidine digluconate (CHX), and metronidazole (MET).
Bacterial Culture and Biofilm Formation
Three reference strains, Actinomyces naeslundii (DSM-43013), Fusobacterium nucleatum (DSM-20482), and Porphyromonas gingivalis (DSM-20709) were obtained from DSMZ (Deutsche Sammlung von Mikroorganismen und Zellkulturen, Braunschweig, Germany) to be used in this study. Bacteria were grown and maintained on Schaedler blood agar plates (provided by the Institute of Microbiology and Hygiene, University Medical Center Regensburg, Germany) in a microincubator (MI23NK, SCHOLZEN Microbiology Systems, St. Margrethen, Switzerland) under anaerobic conditions (80% N2, 10% CO2, and 10% H2). Modified Fluid Universal Medium (mFUM) supplemented with 67 mmol/L Sørensen’s buffer and containing 0.3% (w/v) glucose was employed as a basal liquid medium (Gmür and Guggenheim, 1983; Guggenheim et al., 2001). For preparation of planktonic cultures, colonies were picked and suspended in 5 mL of mFUM with 0.5 mL fetal bovine serum (FBS; Gibco® Life Technologies, Carlsbad, CA, United States) overnight under anaerobic conditions. From these 1 mL was transferred to fresh 5 mL of mFUM with 0.5 mL FBS and incubated for another 6 h under anaerobic conditions to obtain bacteria in the logarithmic phase of growth. Afterwards, suspensions were harvested by centrifugation (ROTINA 420 R, Hettich Lab Technology, Tuttlingen, Germany) and resuspended in mFUM yielding an optical density (OD) of 1.0, as measured by means of a spectrophotometer at 600 nm (Ultrospec 3300 pro, Amersham Biosciences, Amersham, United Kingdom). Bacterial suspensions then were diluted 1:9 in the biofilm culture medium (BCM) consisting of 50% mFUM, 10% FBS, and 40% whole unstimulated human saliva (saliva) that had been pooled from two volunteers (authors V-SS and DM; approved by the internal review board of the University of Regensburg) and filter-sterilized (Acrodisc® Syringe Filters, Pall, Newquay, United Kingdom) (Kalfas and Rundegren, 1991; Ruhl et al., 2011).
Polymicrobial Biofilms were formed in 96-well polystyrene culture plates (Corning® Costar®, Corning, NY, United States). Firstly, wells were incubated for 2 h with saliva for simulation of pellicle-coating. After that, saliva was discarded, and wells were filled with 200 μL of BCM containing A. naeslundii, F. nucleatum, and P. gingivalis and incubated under anaerobic conditions. After 24 and 48 h, medium was carefully removed and 200 μL fresh BCM was added. For all experiments, the total culture period was 72 h.
Antimicrobial Assay
After the total biofilm culture period of 72 h, medium was carefully discarded from the wells. Then, biofilms were either incubated with 50 μL phosphate buffered saline (PBS; Biochrom, Berlin, Germany; groups PS-L-, PS-L+) or 50 μL PS (100 μM SAPYR or SAGUA) in the dark for 20 min and then either illuminated for 10 min (groups SAPYR+L+, SAGUA+L+) or kept in the dark for 10 min (groups SAPYR+L-, SAGUA+L-). For positive controls, biofilms were incubated with CHX (0.06 and 0.2%) or MET (15 and 50 μg/mL) for a total of 30 min (50 μL each). Immediately afterwards, PBS, PS, CHX, or MET was carefully removed and the biofilm of each well was brought to suspension with 200 μL PBS and transferred to an Eppendorf tube. These were placed in an ultrasonic water-bath chamber (Sonorex Super RK 102 H, Bandelin, Berlin, Germany) obtaining a frequency of 35 kHz for 10 min and vortexed (REAX top, Heidolph Instruments, Schwabach, Germany) for 5 s to separate aggregated bacteria. Then 10-fold serial dilutions (10-2–10-7) were prepared in PBS and aliquots (180 μL) were plated on Schaedler blood agar and incubated anaerobically for 72 h. Afterwards, colony forming units (CFUs) were evaluated. Bacterial species on agar plates were discriminated by their unique colony morphology with the aid of a stereomicroscope.
Flow Cytometric Analysis for Cytoplasmic Membrane Damage
For flow cytometry, propidium iodide (PI; Sigma-Aldrich) was used as a fluorescent dye to evaluate integrity of cytoplasmic membranes (Petit et al., 1993). Biofilms were prepared, treated and brought to suspension as described above. After that, samples were centrifuged once at 8000 rpm for 3 min and resuspended in 1 mL PBS. Then, 10 μL of each sample were mixed with 985 μL PBS and 5 μL PI (5 μg/mL), incubated for 5 min in the dark at room temperature and immediately processed by a FACSCanto flow cytometer (Becton Dickinson, Franklin Lakes, NJ, United States) equipped with a 488 nm air-cooled solid-state laser with output of 20 mW. Red fluorescence emitted by PI was detected on FL3. Bacterial cells were gated on FSC/SSC dot plots from which FL3/FSC dot plots were derived. In all cases, 10,000 events were counted.
Spectroscopic Measurements for Release of Nucleic Acids
Damage of cytoplasmic membranes was further assessed by measuring the amount of nucleic acids released from the cytoplasm spectroscopically at 260 nm (Chen and Cooper, 2002). Biofilms were formed and treated as described above. As positive control for cytoplasmic membrane damage, biofilms were incubated with 100 μL lysozyme (40,000 units/mg; Sigma-Aldrich) for 30 min at 37°C. Then, 100 μL Proteinase K (7–14 units/mg; Sigma-Aldrich) and 200 μL sodium dodecyl sulfate (1%) were added and incubated for another 30 min at 37°C. Without discarding the supernatants, all samples were brought to suspension by adding 150 μL PBS and transferred to Eppendorf tubes. These were placed in an ultrasonic water-bath chamber (Sonorex Super RK 102 H; 35 kHz) for 10 min and centrifuged (13,000 rpm; 5 min). Afterwards, the supernatants were collected and assessed for release of nucleic acids by measuring the OD at 260 nm by means of a NanoDropTM 2000 spectrophotometer (PEQLAB, Erlangen, Germany).
Scanning Electron Microscopy (SEM)
Biofilms were prepared on Permanox® Chamber Slides (Nunc® Lab-Tek® Permanox®, 4.2 cm2/well, Sigma-Aldrich) and treated as described above. The samples were fixed by adding 2.5% glutaraldehyde buffered with Sørensen’s phosphate buffer (0.1 M; pH 7.4) at room temperature for 2 h. Each sample was washed twice with PBS and three times with distilled water for 15 min each. Then, the fixed samples were additionally dehydrated using 30, 50, 70, 80, 90, 96, and 100% (v/v) graded ethanol, 20 min each. After air-drying overnight in a desiccator, the growth-chambers were removed and the slides were stuck on SEM stubs (ø 25 mm). For coating, samples were purged with argon and sputtered with platinum for 30 s using a SCD 005 Sputter Coater (Bal-Tec, Balzers, Liechtenstein). Biofilms were examined using a Quanta 400 FEG scanning electron microscope (FEI Company, Hillsboro, OR, United States) in high vacuum mode at 2 kV with 6–7 mm working distance. Tilt and focus were adjusted to ensure optimum viewing. Images were taken from randomly selected fields on the slides.
PS-Uptake Measurements
The amount of PS-uptake was measured by spectrophotometric measurement of the supernatants of bacterial cultures after incubation with the respective PS, as described earlier (Maisch et al., 2007; George et al., 2009). Planktonic cultures of A. naeslundii, F. nucleatum, and P. gingivalis were grown overnight as described above and adjusted to an OD of 1.0. Then, these suspensions were incubated in the dark for 20 min with 100 μM SAPYR or with 100 μM Methylene Blue (Sigma-Aldrich, St. Louis, MO, United States), which acted as a positive control. After incubation, cells were centrifuged (13,000 rpm; 5 min) and the supernatants were measured spectrophotometrically (SPECORD® 50 PLUS, Analytik Jena, Jena, Germany) to record absorption of PS not bound to bacterial cells. Bacteria without PS and PS-solutions served as controls. This experiment was repeated thrice.
Data Analysis
All CFU results are shown as medians, 1st and 3rd quartiles and were calculated using SPSS for Windows, version 25 (SPSS Inc., Chicago, IL, United States) from the values of at least six independent experiments, each performed in duplicate. Horizontal solid and dashed lines represent reductions of 3 and 5 log10 steps of CFU, respectively, compared to the untreated control group PS-L-. Medians on or below these lines demonstrate an antimicrobial efficacy of 99.9% (3 log10) or 99.999% (5 log10), at least, which is declared as biologically relevant antimicrobial activity or disinfectant effect according to guidelines of infection control (Boyce and Pittet, 2002).
Flow cytometric data were analyzed using FACSDivaTM software, version 5.0.2 (Becton Dickinson). The percentages of unstained and PI-stained bacteria were calculated as medians, 1st and 3rd quartiles using SPSS from the values of six independent experiments, each performed in duplicate.
Results from the spectroscopic measurements for release of nucleic acids are shown as medians, 1st and 3rd quartiles, calculated from the values of seven independent experiments using SPSS.
Results
Antimicrobial Assay
Untreated biofilms (PS-L-) showed a slightly higher growth of A. naeslundii (1.1 × 107) of about 1 log10-step as compared to F. nucleatum and P. gingivalis (both: 1.8 × 106), as shown as absolute CFU values in the left panel of Figure 2. The right panel of Figure 2 depicts relative CFU data (CFU [%]) with untreated controls (groups PS-L-) set to 100% separately for each bacterial strain.
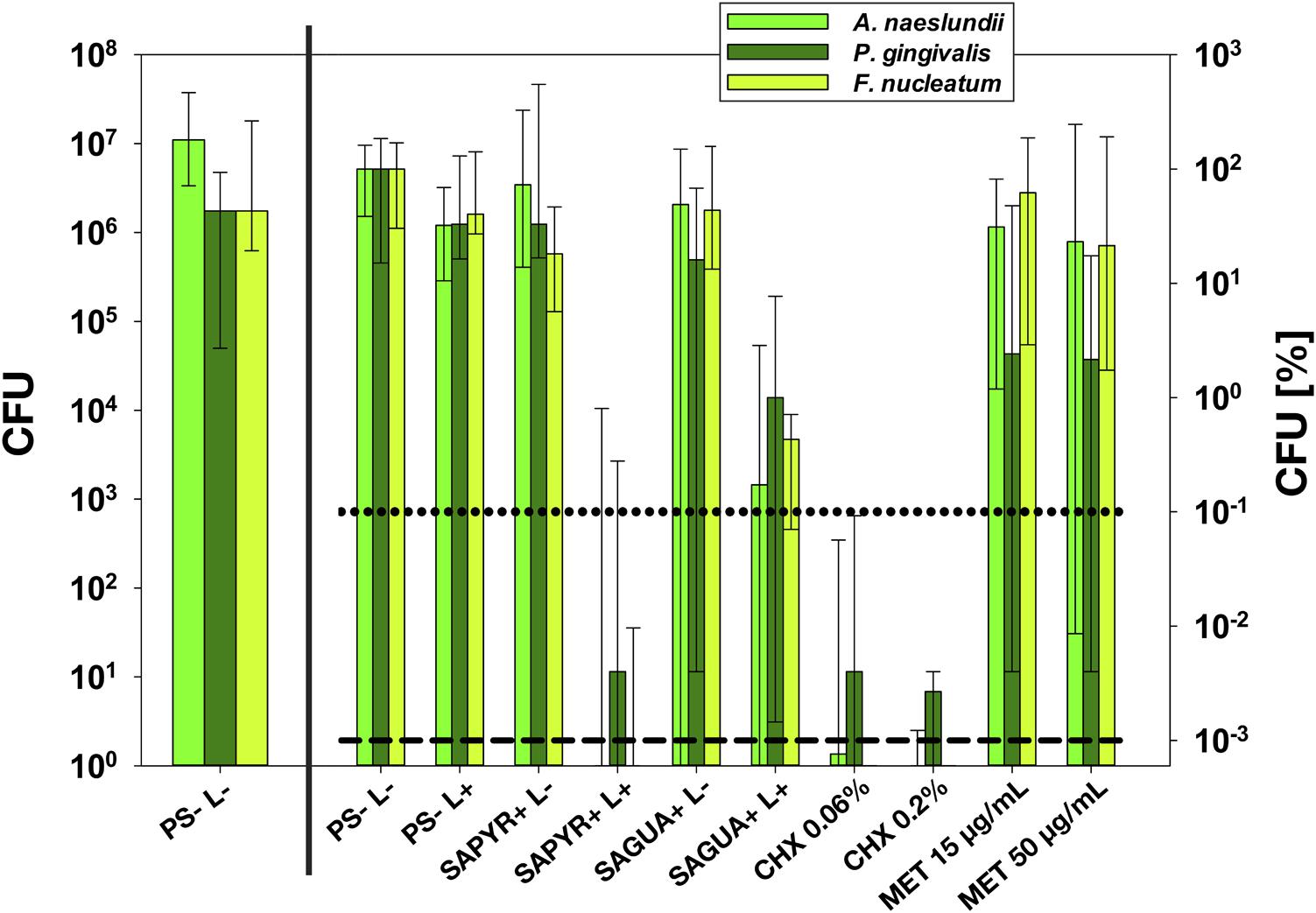
FIGURE 2. Antimicrobial assay. All results are depicted as medians, 1st and 3rd quartiles from six independent experiments in duplicates on a log10-scaled ordinate. (Left) shows untreated control group PS-L– as absolute colony forming units (CFU) values. (Right) shows relative CFU data (CFU [%]) with untreated control group (PS-L–) set to 100% for each bacterial strain. Horizontal dotted and dashed lines represent CFU-reductions of 3 log10 and 5 log10, respectively.
Antimicrobial photodynamic therapy with SAPYR reduced CFU of A. naeslundii and F. nucleatum by 6.0 and 6.1 log10 and P. gingivalis by 4.4 log10. In contrast, aPDT with SAGUA led to reductions of 2.8 log10 against A. naeslundii, 2.4 log10 against F. nucleatum and 2.0 log10 against P. gingivalis only. In all cases, there was no effect of treatment with PS or light only.
Chlorhexidine 0.06 and 0.2% led to reductions of 4.4–6.1 log10 or 4.6–6.1 log10, respectively, with P. gingivalis representing the less susceptible species. In contrast, P. gingivalis was the only species that could be inactivated by treatment with MET by >0.5 log10 (1.6 or 1.8 log10 at concentrations of 15 μg/mL or 50 μg/mL, respectively).
Flow Cytometric Analysis for Cytoplasmic Membrane Damage
Flow cytometry with PI as fluorescent dye was employed to evaluate damage of the cytoplasmic membrane as cells with intact membranes are not permeable to PI (Petit et al., 1993). When determining the region of interest (ROI) in untreated controls, three cell populations indicative for the three bacterial species could be clearly determined on dot plots FSC vs. SSC depending on the respective size and granularity of the bacterial cells (Figure 3A).
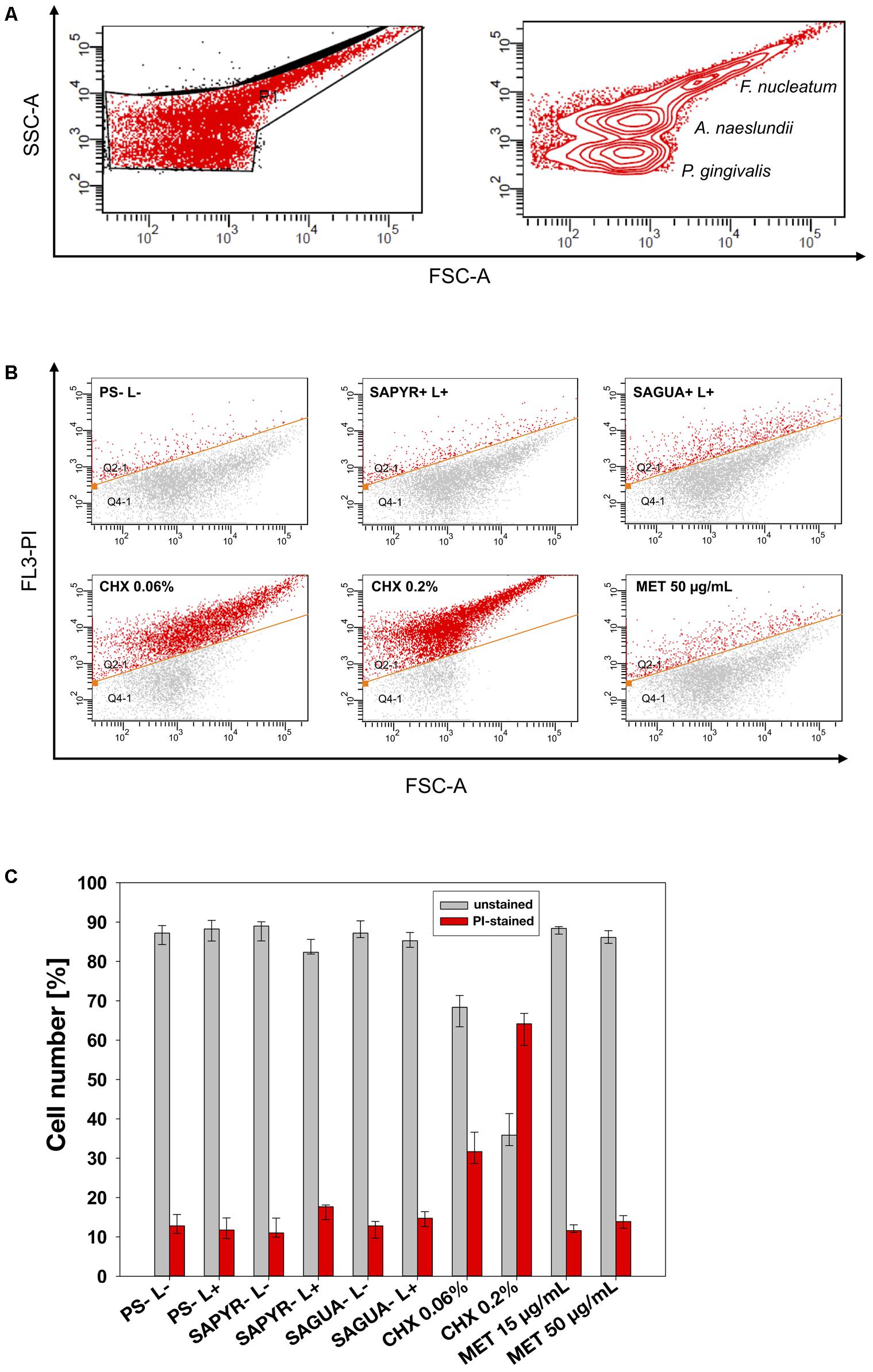
FIGURE 3. Flow cytometric analysis for cytoplasmic membrane damage. (A) Bacterial cell populations gated on dot plot FSC vs. SSC with P1 showing the chosen region of interest (ROI). Three populations could be discriminated (circles show high number of reads). (B) Exemplary dot plots for untreated controls (PS-L–) and groups treated with aPDT (SAPYR+L+; SAGUA+L+), CHX (CHX 0.06%; CHX 0.2%) or MET (MET 50 μg/mL), respectively. The gate below the orange line indicates unstained cells with intact membrane, while the gate above the orange line indicates PI-stained cells with damaged membrane. (C) Summarized median percentages, 1st and 3rd quartiles of unstained (gray) as well as PI-stained bacterial cells (red) are shown for all experimental groups. Membrane damage could only be observed after treatment with CHX in a concentration-dependent manner.
Exemplary dot plots are shown in Figure 3B for selected groups. Figure 3C shows summarized percentages of unstained as well as PI-stained bacterial cells for all groups. Untreated biofilms (PS-L-) showed a proportion of PI-positive cells of 13%. Neither treatment with PS or light alone, nor aPDT with SAPYR and SAGUA nor MET at both concentrations led to an increase of PI-positive cells as compared to untreated controls (median of PI-positive cells: 11–15%). In contrast, treatment with CHX led to a concentration-dependent median increase of PI-positive cells to 32% (CHX 0.06%) or 64% (CHX 0.2%), respectively.
Spectroscopic Measurements for Release of Nucleic Acids
Damage of cytoplasmic membranes was further assessed by measuring the release of nucleic acids spectroscopically at 260 nm. As compared to the untreated control group (PS-L-), neither treatment with light or PS alone nor aPDT with SAPYR or SAGUA led to an increase in OD at 260 nm (median OD values ranging from 0.15 to 0.19). In contrast, in the positive control group comprising lysis with lysozyme following Proteinase K digestion a median OD of 0.91 was found (Figure 4).
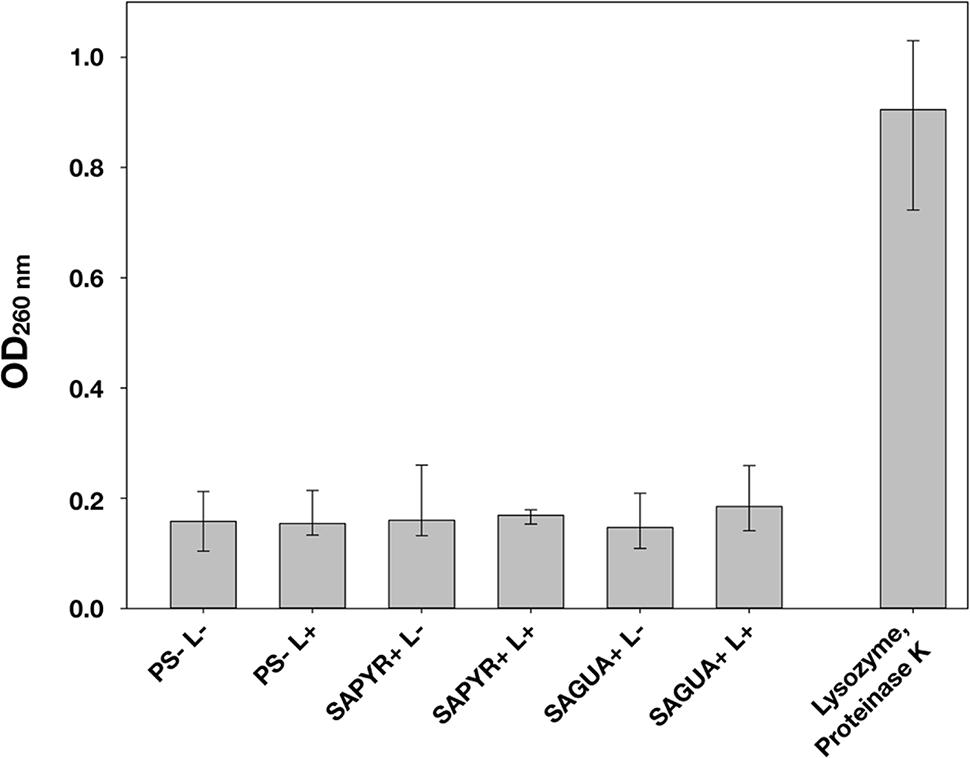
FIGURE 4. Spectroscopic measurements for release of nucleic acids. OD medians, 1st and 3rd quartiles of the supernatants of biofilms treated with phenalen-1-one mediated aPDT (groups: PS-L–, PS-L+, SAPYR+L-, SAPYR+L+, SAGUA+L-, and SAGUA+L+) or positive control (lysozyme treatment followed by Proteinase K digestion), as measured at 260 nm for release of nucleic acids.
Scanning Electron Microscopy (SEM)
Figure 5 shows exemplary SEM images taken from randomly selected fields of untreated biofilms (group PS-L-) and biofilms treated with aPDT with SAPYR (SAPYR+L+) or SAGUA (SAGUA+L+) or CHX (CHX 0.2%). Untreated biofilms showed a multi-layered biofilm structure with typical voids. While aPDT with SAPYR resulted in no visible impact on overall biofilm structure or bacterial cell morphology, in CHX-treated samples debris most likely from killed cells was detectable on the biofilm surface.
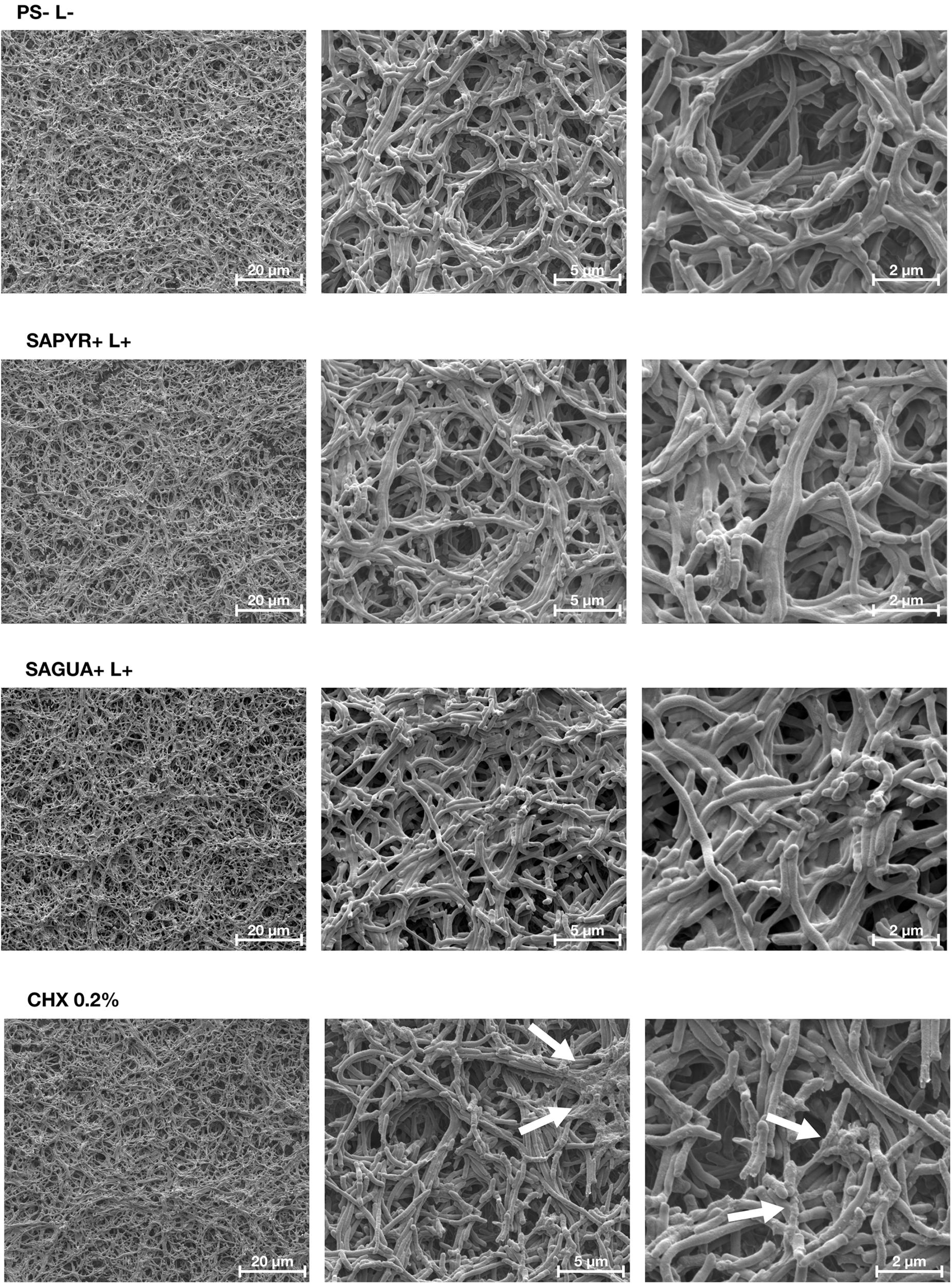
FIGURE 5. Exemplary visualization of polymicrobial biofilms by means of scanning electron microscopy. Exemplary SEM visualization of randomly selected fields of untreated biofilms (PS-L–), biofilms treated with aPDT using SAPYR (SAPYR+L+) or SAGUA (SAGUA+L+) or treated with CHX (CHX 0.2%) in 3,000-fold, 12,000-fold, and 24,000-fold magnification. In the CHX-treated biofilms white arrows show debris most likely originating from killed cells on the top layer of the biofilms.
PS-Uptake Measurements
The amount of PS-uptake was determined spectrophotometrically for SAPYR and Methylene Blue by measuring the absorption of the respective PS remaining in the supernatants of planktonic cultures after incubation for 30 min. Figure 6 shows absorption spectra for SAPYR and the supernatants of bacteria incubated with SAPYR (Figure 6A) or Methylene Blue and the supernatants of bacteria incubated with Methylene Blue (Figure 6B), respectively. For Methylene Blue, there was a clear decrease in absorption in the supernatants as compared to the PS-suspension indicating PS-uptake or strong attachment to the bacterial cells. In contrast, for SAPYR no uptake or attachment of the PS to the bacterial cells was found as shown by the absent decrease in absorption in the supernatants.
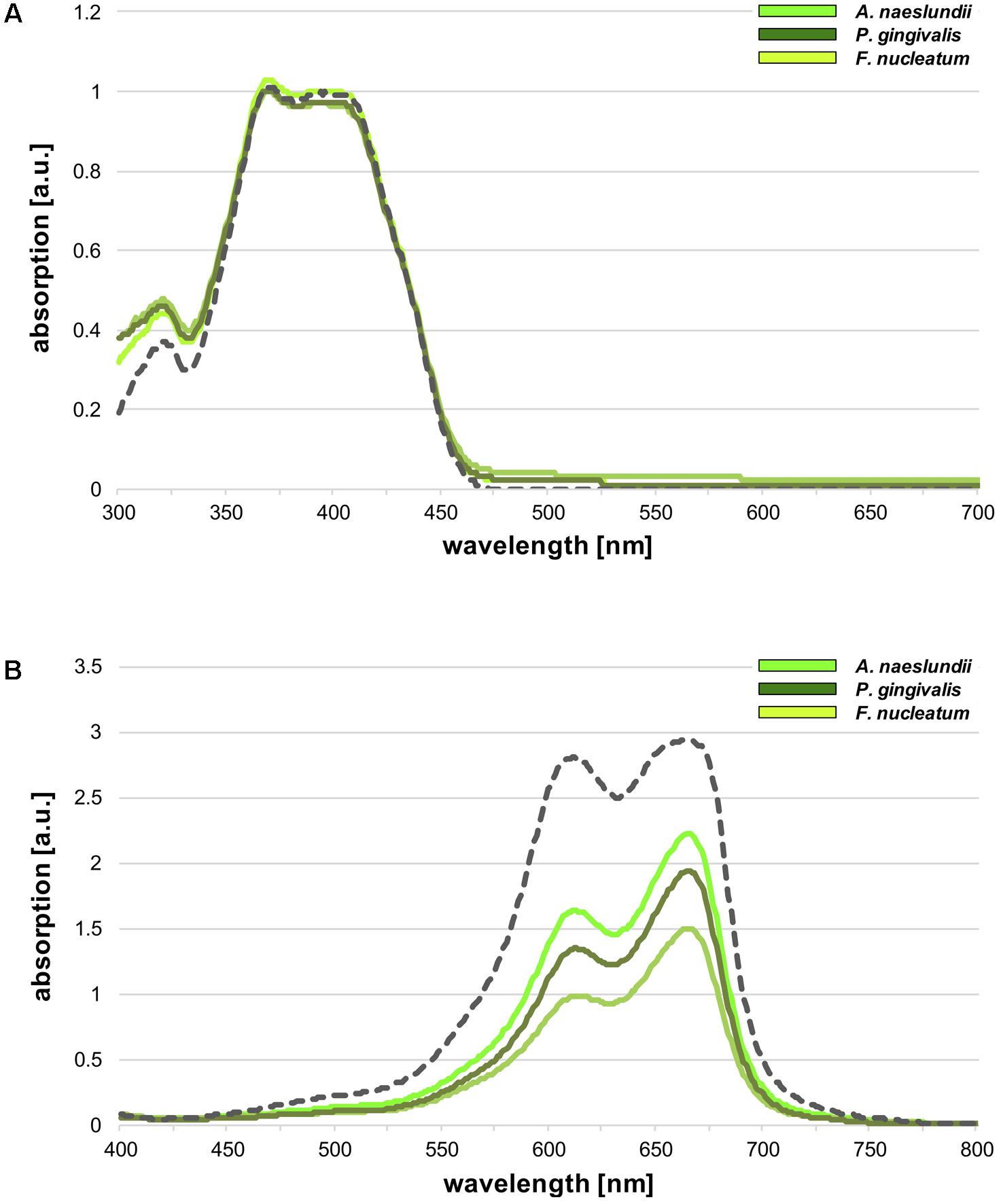
FIGURE 6. Photosensitizers (PS)-uptake measurements. Spectrophotometrical evaluation of PS-uptake for (A) SAPYR and (B) Methylene Blue. Gray dotted lines show absorption spectra of the PS solutions (100 μM), while green solid lines show absorption spectra of the supernatants of bacteria incubated with the respective PS (neon green: Actinomyces naeslundii; yellowish green: Fusobacterium nucleatum; dark green: Porphyromonas gingivalis).
Discussion
Traditionally, culture-based methods (like CFU assays) have been employed for evaluating the efficacy of given antimicrobial approaches. Hereby, “death” of bacterial cells is defined by their inability to replicate and form colonies on solid agar medium (Berney et al., 2007). This means that the effect of an antimicrobial approach can only be evaluated retrospectively after a given incubation period as several cell divisions are needed for forming a visible colony on agar (Nebe-von Caron et al., 1998). CFU assays facilitate standardization of results from distinct studies, as the calculated log10 reduction rates can easily be compared. On the other hand, this technique does not permit insights to the mechanisms of action of given antimicrobials. In this instance, a complementary technique may be flow cytometry which allows for measurements of different vital parameters within a cell when combined with appropriate fluorescent dyes (Novo et al., 2000; Kennedy et al., 2011). This may be especially valuable in narrowing down to the mechanism of novel antimicrobial approaches, such as aPDT, and revealing their main target structures. As for phenalen-1-one-mediated aPDT incubation periods in the seconds range are sufficient to reach disinfecting effects (≥5 log10 steps reduction of CFU) against planktonic bacteria irrespective of their Gram-staining characteristics (Späth et al., 2014; Tabenski et al., 2016; Muehler et al., 2017), we proposed that damage of cytoplasmic membranes rather than of DNA may be the main mechanism of aPDT with this class of PS. Therefore, in the present study we combined traditional culture techniques and flow cytometry to investigate the antimicrobial efficacy of phenalen-1-one-mediated aPDT when applied to biofilms formed by three putative periodontal pathogens in vitro in order to get first insights in its mechanism of action by evaluating the damage of cytoplasmic membranes.
For this purpose, a polymicrobial biofilm was established in vitro from Actinomyces naeslundii, Fusobacterium nucleatum, and Porphyromonas gingivalis, whereby culture conditions were derived and slightly modified from the well-known Zurich biofilm model (Guggenheim et al., 2001; Belibasakis and Thurnheer, 2014). In this model, A. naeslundii represents an essential early colonizer (Dige et al., 2009), while F. nucleatum is known to act as a bridge between early and late colonizers (Bolstad et al., 1996) and P. gingivalis is a late colonizer and keystone pathogen promoting the formation of dysbiotic periodontitis-associated microbial communities (Hajishengallis and Lamont, 2016). In contrast to the original Zurich biofilm model, biofilms were not cultured on hydroxyapatite disks, but in flat-bottomed 96-well plates to allow for high-throughput screening and, particularly, for facilitating standardized irradiation procedures which would be compromised with biofilms forming on both sides of the hydroxyapatite disks. Exemplary SEM visualization of untreated biofilms revealed a multi-layered biofilm architecture with typical voids, similarly to naturally occurring oral biofilms (Rudney et al., 2012). As positive control for antimicrobial efficacy, CHX was used as gold-standard antiseptic in typical concentrations applied in oral care (0.06% as well as 0.2%). Further, MET was included as standard antibiotic in clinical periodontal practice for inactivation of anaerobes (Slots, 2004). Here, the concentration of 15 μg/mL was chosen as it can be typically reached in plasma, gingival crevicular fluid and saliva after systemic administration (Pähkla et al., 2005; Belibasakis and Thurnheer, 2014), while 50 μg/mL was additionally used as slightly higher concentration.
Treatment with CHX resulted in reductions of about 6 log10 against A. naeslundii and F. nucleatum and about 4.5 log10 against P. gingivalis irrespective of the applied concentration. Identical antimicrobial efficacy was found for aPDT employing SAPYR as a PS. This is noteworthy because despite equal total treatment periods (30 min) for CHX and aPDT, the period of actual antimicrobial activity of aPDT is limited to the period of light-activation (here: 10 min), while the preceding pre-incubation period (here: 20 min) just ensures sufficient penetration of the PS throughout the extracellular polymeric substance (EPS) of the biofilm (Mah and O’Toole, 2001). The antimicrobial photodynamic efficacy of SAGUA (2.0–2.8 log10) was clearly inferior as compared to SAPYR (4.4–6.1 log10) although the opposite was found in a previous study for inactivation of planktonic bacteria (Tabenski et al., 2016). This may be explained by the nature of the positively charged guanidinium group of SAGUA which can establish hydrogen bonds with phosphate and carboxylate groups (Tabenski et al., 2016). This may improve the attachment of SAGUA toward bacterial cell walls in planktonic cultures, but otherwise hamper diffusion of SAGUA through the EPS of a biofilm by interacting with negatively charged EPS molecules. Another point may be the inferior singlet oxygen quantum yield ΦΔ of SAGUA as compared to SAPYR (0.86 vs. 0.99) (Tabenski et al., 2016).
MET reduced only CFU of P. gingivalis in a concentration-dependent manner by 1.6 log10 or 1.8 log10, while A. naeslundii and F. nucleatum were not affected at all. This is in line with literature data, where Belibasakis and Thurnheer (2014) found no CFU-reductions after applying 15 μg/mL MET even for an incubation period of 24 h in the Zurich subgingival biofilm model. Furthermore, Wang et al. (2015) reported a minimum biofilm eradication concentration of 800 μg/mL MET for a double-species in vitro biofilm of F. nucleatum and P. gingivalis after treatment for 24 h. Despite in vitro data, this is further evidence that any administration of antibiotics in clinical periodontal treatment is obsolete without mechanical disruption of the subgingival biofilms (Belibasakis and Thurnheer, 2014). Accordingly, minimum bactericidal concentrations of MET are in a much lower range for planktonic cultures of periodontal pathogens [e.g., 25 and 10 μg/mL for F. nucleatum or P. gingivalis, respectively (Wang et al., 2015)], which is clinically achievable in saliva and gingival crevicular fluid (Pähkla et al., 2005).
Although there are some studies that utilized flow cytometry as a simple measure for antimicrobial efficacy of aPDT (Bulit et al., 2014; Manoil et al., 2014; Marinic et al., 2015), the present study is the first one employing flow cytometry for getting insights into the mechanism of action of aPDT. We employed PI, a positively charged nucleic acid stain that is only able to enter bacterial cells through permeabilized membranes, in order to assess cytoplasmic membrane damage (Petit et al., 1993; Joux and Lebaron, 2000). Here, CHX served as a positive control because it is well known to damage the bacterial cytoplasmic membrane followed by leakage of cytoplasmic constituents (McDonnell and Russell, 1999). MET served as a negative control as its antimicrobial effect is based on inducing DNA strand breaks, but not on cytoplasmic membrane damage (Edwards, 1979).
In the chosen ROI, three cell populations could be clearly discriminated according to the respective size and granularity of the bacterial cells. As the small size of bacteria (especially of P. gingivalis) complicates distinguishing between small bacterial cells and abiotic cellular debris (Ambriz-Aviña et al., 2014), the correctness of the chosen ROI was confirmed by additional nucleic acid staining with SYBR green (data not shown). The populations indicative for F. nucleatum and P. gingivalis and the shape of their respective dot plots are in accordance to those recently reported by Ciandrini et al. (2014) for single-species biofilms of these species.
As expected, flow cytometry revealed a clear and concentration-dependent increase in the percentage of PI-positive cells after treatment with CHX and no increase after treatment with MET. At first glance, a proportion of 64% PI-positive cells after treatment with 0.2% CHX seems not matching a reduction of 4.6–6.1 log10 steps of CFU, but this phenomenon just shows that very distinct vital parameters, i.e., integrity of cytoplasmic membranes or ability of bacterial cells to replicate, are measured by means of these techniques. Therefore, a bacterial cell with not completely permeabilized cytoplasmic membrane may still not be able to replicate due to the total incurred extent of damage. This phenomenon is known as “viable, but not culturable” (VBNC) state (Joux and Lebaron, 2000), in which bacteria usually show decreased metabolic activity and little or no ability to replicate (Tamburini et al., 2014). In contrast to CHX, aPDT led to no increase in PI-positive cells neither when employing SAPYR nor SAGUA.
For further assessment of cytoplasmic membrane damage after aPDT with SAPYR or SAGUA, release of nucleic acids after treatment was measured spectroscopically at 260 nm, which has been described to serve as a reliable indicator for assessing membrane integrity (Chen and Cooper, 2002). As CHX was not applicable for these experiments due to its characteristic absorption maximum at 260 nm (Hiraishi et al., 2008), a lysis step with lysozyme followed by Proteinase K digestion served as positive control for cytoplasmic membrane damage. In accordance with the results from flow cytometry, there was no increased release of nucleic acids after aPDT with SAPYR or SAGUA as compared to the untreated control group. These findings are in line with the exemplary SEM visualizations, where no impact on overall biofilm structure or bacterial cell morphology could be detected after treatment with aPDT using SAPYR or SAGUA as PS. In contrast, in CHX-treated samples debris was visible on the biofilm surface, most likely originating from disrupted cells (Wang et al., 2017).
However, these results do not necessarily implicate that cytoplasmic membranes are not targeted at all by phenalen-1-one-mediated aPDT, as it is usually assumed that permeability to “exclusion dyes” like PI (molecular weight 668.4 g/mol) is associated with large and irreparable gaps in the cytoplasmic membrane (Novo et al., 2000; Falcioni et al., 2008). Likewise, leakage of large molecules like nucleic acids occurs only after pronounced damage of cytoplasmic membranes, while small ions like potassium or phosphate tend to leach out earlier (Chen and Cooper, 2002). Consequently, the oxidative burst induced by aPDT potentially may not lead to these large gaps in the cytoplasmic membranes, but to a depolarization of the membrane potential only.
For evaluating the cellular localization of SAPYR, PS-uptake was measured spectrophotometrically. Here, Methylene Blue served as a positive control because this PS seems to locate intracellularly as its photodynamic efficacy can be enhanced by addition of efflux pump inhibitors (Tegos et al., 2008). Accordingly, a clear decrease in absorption could be found in the supernatants of bacterial cells after incubation with Methylene Blue, indicating cellular uptake or strong attachment to the bacteria. In contrast, the supernatants of bacterial cells incubated with SAPYR exhibited no decrease in absorption at all. Therefore, cellular uptake of SAPYR seems very improbable. As it is known that a PS has to build up high intracellular concentrations for resulting in DNA damage upon irradiation (Cadet et al., 2010), DNA may not be the target structure of aPDT with SAPYR. It is rather plausible that SAPYR is just electrostatically attracted to bacterial cell surfaces by its positive charge but does not directly attach toward these cell surfaces.
This study represents a first step in investigating the bacterial target structures of the oxidative burst mediated by aPDT with phenalene-1-one derivatives. For deeper insights into the mechanism and damage patterns of aPDT, future studies must combine distinct methods for resolving these complex mechanisms. For example, so-called multi-parameter flow cytometry employing many fluorescent markers for distinct vital parameters may represent a valuable approach for assessing the effects of aPDT on distinct cellular structures (Novo et al., 2000; Ambriz-Aviña et al., 2014).
Furthermore, potential harmful effects on mammalian tissues must be precluded before phenalen-1-one-mediated aPDT can be applied clinically. In a recent study, aPDT with various phenalen-1-one derivatives was investigated for its antimicrobial efficacy toward dermal pathogens and for its eukaryotic toxicity toward keratinocytes as compared to the biocide benzalkonium chloride for assessing potential effective concentration ranges (i.e., ≥5 log10 reduction of CFU while ≥80% survival of keratinocytes). For aPDT with SAPYR a broad effective concentration range was found, while for benzalkonium chloride there was no effective concentration range at all (Muehler et al., 2017). These encouraging results must now be corroborated in further biocompatibility studies.
Conclusion
• Antimicrobial photodynamic therapy with SAPYR is as effective as CHX 0.2% when applied to an in vitro biofilm comprising A. naeslundii, F. nucleatum, and P. gingivalis leading to reduction rates of 4.4–6.1 log10 steps while aPDT with SAGUA is less effective.
• In contrast to CHX, phenalen-1-one-mediated aPDT leads to no damage of cytoplasmic membranes as revealed by flow cytometry using PI as a fluorescent dye. Likewise, no release of nucleic acids could be detected after aPDT with SAPYR or SAGUA.
• SAPYR exhibits neither uptake nor strong attachment toward bacterial cells as opposed to Methylene Blue.
Author Contributions
FC, TM, and WB conceived and designed the experiments. V-SS and DM performed the experiments. TT and GB helped with setting up the biofilm model. FC, V-SS, DM, K-AH, TM, and WB analyzed the data. FC wrote the manuscript with input from all the other authors. All authors reviewed the manuscript.
Funding
This study was funded by a grant from the Deutsche Forschungsgemeinschaft (German Research Foundation; CI 263/1-1).
Conflict of Interest Statement
The authors declare that the research was conducted in the absence of any commercial or financial relationships that could be construed as a potential conflict of interest.
The reviewer TR and handling Editor declared their shared affiliation.
Acknowledgments
TriOptoTec GmbH and Dr. Andreas Späth are gratefully thanked for providing the photosensitizers to be used in this study. Helga Ebensberger and Gerlinde Ferstl are thanked for their excellent technical support in SEM experiments.
References
Almeida, A., Faustino, M. A. F., and Tomé, J. P. (2015). Photodynamic inactivation of bacteria: finding the effective targets. Future Med. Chem. 7, 1221–1224. doi: 10.4155/fmc.15.59
Alves, E., Faustino, M. A., Neves, M. G., Cunha, A., Tome, J., and Almeida, A. (2014). An insight on bacterial cellular targets of photodynamic inactivation. Future Med. Chem. 6, 141–164. doi: 10.4155/fmc.13.211
Ambriz-Aviña, V., Contreras-Garduño, J. A., and Pedraza-Reyes, M. (2014). Applications of flow cytometry to characterize bacterial physiological responses. Biomed Res. Int. 2014:461941. doi: 10.1155/2014/461941
Belibasakis, G. N., and Thurnheer, T. (2014). Validation of antibiotic efficacy on in vitro subgingival biofilms. J. Periodontol. 85, 343–348. doi: 10.1902/jop.2013.130167
Berney, M., Hammes, F., Bosshard, F., Weilenmann, H.-U., and Egli, T. (2007). Assessment and interpretation of bacterial viability by using the LIVE/DEAD BacLight kit in combination with flow cytometry. Appl. Environ. Microbiol. 73, 3283–3290. doi: 10.1128/AEM.02750-06
Bolstad, A. I., Jensen, H. B., and Bakken, V. (1996). Taxonomy, biology, and periodontal aspects of Fusobacterium nucleatum. Clin. Microbiol. Rev. 9, 55–71.
Boyce, J. M., and Pittet, D. (2002). Guideline for hand hygiene in health-care settings: recommendations of the healthcare infection control practices advisory committee and the HICPAC/SHEA/APIC/IDSA hand hygiene task force. Infect. Control Hosp. Epidemiol. 23, 3–40. doi: 10.1086/503164
Bulit, F., Grad, I., Manoil, D., Simon, S., Wataha, J. C., Filieri, A., et al. (2014). Antimicrobial activity and cytotoxicity of 3 photosensitizers activated with blue light. J. Endod. 40, 427–431. doi: 10.1016/j.joen.2013.12.001
Cadet, J., Douki, T., and Ravanat, J.-L. (2010). Oxidatively generated base damage to cellular DNA. Free Radic. Biol. Med. 49, 9–21. doi: 10.1016/j.freeradbiomed.2010.03.025
Chen, C. Z., and Cooper, S. L. (2002). Interactions between dendrimer biocides and bacterial membranes. Biomaterials 23, 3359–3368. doi: 10.1016/S0142-9612(02)00036-4
Ciandrini, E., Campana, R., Federici, S., Manti, A., Battistelli, M., Falcieri, E., et al. (2014). In vitro activity of Carvacrol against titanium-adherent oral biofilms and planktonic cultures. Clin. Oral Investig. 18, 2001–2013. doi: 10.1007/s00784-013-1179-9
Cieplik, F., Pummer, A., Leibl, C., Regensburger, J., Schmalz, G., Buchalla, W., et al. (2016). Photodynamic inactivation of root canal bacteria by light activation through human dental hard and simulated surrounding tissue. Front. Microbiol. 7:929. doi: 10.3389/fmicb.2016.00929
Cieplik, F., Pummer, A., Regensburger, J., Hiller, K.-A., Späth, A., Tabenski, L., et al. (2015). The impact of absorbed photons on antimicrobial photodynamic efficacy. Front. Microbiol. 6:706. doi: 10.3389/fmicb.2015.00706
Cieplik, F., Späth, A., Regensburger, J., Gollmer, A., Tabenski, L., Hiller, K.-A., et al. (2013). Photodynamic biofilm inactivation by SAPYR–an exclusive singlet oxygen photosensitizer. Free Radic. Biol. Med. 65, 477–487. doi: 10.1016/j.freeradbiomed.2013.07.031
Cieplik, F., Tabenski, L., Buchalla, W., and Maisch, T. (2014). Antimicrobial photodynamic therapy for inactivation of biofilms formed by oral key pathogens. Front. Microbiol. 5:405. doi: 10.3389/fmicb.2014.00405
Cieplik, F., Wimmer, F., Muehler, D., Thurnheer, T., Belibasakis, G. N., Hiller, K.-A., et al. (2018). Phenalen-1-one-mediated antimicrobial photodynamic therapy and chlorhexidine applied to a novel caries biofilm model. Caries Res. 52, 447–453. doi: 10.1159/000487815
Dige, I., Raarup, M. K., Nyengaard, J. R., Kilian, M., and Nyvad, B. (2009). Actinomyces naeslundii in initial dental biofilm formation. Microbiology 155, 2116–2126. doi: 10.1099/mic.0.027706-0
Edwards, D. I. (1979). Mechanism of antimicrobial action of metronidazole. J. Antimicrob. Chemother. 5, 499–502. doi: 10.1093/jac/5.5.499
Falcioni, T., Papa, S., and Gasol, J. M. (2008). Evaluating the flow-cytometric nucleic acid double-staining protocol in realistic situations of planktonic bacterial death. Appl. Environ. Microbiol. 74, 1767–1779. doi: 10.1128/AEM.01668-07
GBD 2015 Disease and Injury Incidence and Prevalence Collaborators (2016). Global, regional, and national incidence, prevalence, and years lived with disability for 310 diseases and injuries, 1990–2015: a systematic analysis for the Global Burden of Disease Study 2015. Lancet 388, 1545–1602. doi: 10.1016/S0140-6736(16)31678-6
George, S., Hamblin, M. R., and Kishen, A. (2009). Uptake pathways of anionic and cationic photosensitizers into bacteria. Photochem. Photobiol. Sci. 8, 788–795. doi: 10.1039/B809624D
Gmür, R., and Guggenheim, B. (1983). Antigenic heterogeneity of Bacteroides intermedius as recognized by monoclonal antibodies. Infect. Immun. 42, 459–470.
Guggenheim, B., Giertsen, E., Schüpbach, P., and Shapiro, S. (2001). Validation of an in vitro biofilm model of supragingival plaque. J. Dent. Res. 80, 363–370. doi: 10.1177/00220345010800011201
Gursoy, H., Ozcakir-Tomruk, C., Tanalp, J., and Yilmaz, S. (2013). Photodynamic therapy in dentistry: a literature review. Clin. Oral Investig. 17, 1113–1125. doi: 10.1007/s00784-012-0845-7
Hajishengallis, G., and Lamont, R. J. (2016). Dancing with the stars: how choreographed bacterial interactions dictate nososymbiocity and give rise to keystone pathogens, accessory pathogens, and pathobionts. Trends Microbiol. 24, 477–489. doi: 10.1016/j.tim.2016.02.010
Hiraishi, N., Yiu, C. K. Y., King, N. M., Tay, F. R., and Pashley, D. H. (2008). Chlorhexidine release and water sorption characteristics of chlorhexidine-incorporated hydrophobic/hydrophilic resins. Dent. Mater. 24, 1391–1399. doi: 10.1016/j.dental.2008.03.011
Horner, C., Mawer, D., and Wilcox, M. (2012). Reduced susceptibility to chlorhexidine in staphylococci: is it increasing and does it matter? J. Antimicrob. Chemother. 67, 2547–2559. doi: 10.1093/jac/dks284
Joux, F., and Lebaron, P. (2000). Use of fluorescent probes to assess physiological functions of bacteria at single-cell level. Microbes Infect. 2, 1523–1535. doi: 10.1016/S1286-4579(00)01307-1
Kalfas, S., and Rundegren, J. (1991). Biological qualities of saliva sterilized by filtration or ethylene oxide treatment. Oral Microbiol. Immunol. 6, 182–186. doi: 10.1111/j.1399-302X.1991.tb00474.x
Kampf, G. (2016). Acquired resistance to chlorhexidine - is it time to establish an “antiseptic stewardship” initiative? J. Hosp. Infect. 94, 213–227. doi: 10.1016/j.jhin.2016.08.018
Kashef, N., and Hamblin, M. R. (2017). Can microbial cells develop resistance to oxidative stress in antimicrobial photodynamic inactivation? Drug Resist. Updat. 31, 31–42. doi: 10.1016/j.drup.2017.07.003
Kennedy, D., Cronin, U. P., and Wilkinson, M. G. (2011). Responses of Escherichia coli, Listeria monocytogenes, and Staphylococcus aureus to simulated food processing treatments, determined using fluorescence-activated cell sorting and plate counting. Appl. Environ. Microbiol. 77, 4657–4668. doi: 10.1128/AEM.00323-11
Kitagawa, H., Izutani, N., Kitagawa, R., Maezono, H., Yamaguchi, M., and Imazato, S. (2016). Evolution of resistance to cationic biocides in Streptococcus mutans and Enterococcus faecalis. J. Dent. 47, 18–22. doi: 10.1016/j.jdent.2016.02.008
Mah, T.-F. C., and O’Toole, G. A. (2001). Mechanisms of biofilm resistance to antimicrobial agents. Trends Microbiol. 9, 34–39. doi: 10.1016/S0966-842X(00)01913-2
Maisch, T., Baier, J., Franz, B., Maier, M., Landthaler, M., Szeimies, R.-M., et al. (2007). The role of singlet oxygen and oxygen concentration in photodynamic inactivation of bacteria. Proc. Natl. Acad. Sci. U.S.A. 104, 7223–7228. doi: 10.1073/pnas.0611328104
Manoil, D., Filieri, A., Gameiro, C., Lange, N., Schrenzel, J., Wataha, J. C., et al. (2014). Flow cytometric assessment of Streptococcus mutans viability after exposure to blue light-activated curcumin. Photodiagnosis Photodyn. Ther. 11, 372–379. doi: 10.1016/j.pdpdt.2014.06.003
Marinic, K., Manoil, D., Filieri, A., Wataha, J. C., Schrenzel, J., Lange, N., et al. (2015). Repeated exposures to blue light-activated eosin Y enhance inactivation of E. faecalis biofilms, in vitro. Photodiagnosis Photodyn. Ther. 12, 393–400. doi: 10.1016/j.pdpdt.2015.06.004
McDonnell, G., and Russell, A. D. (1999). Antiseptics and disinfectants: activity, action, and resistance. Clin. Microbiol. Rev. 12, 147–179.
Muehler, D., Sommer, K., Wennige, S., Hiller, K.-A., Cieplik, F., Maisch, T., et al. (2017). Light-activated phenalen-1-one bactericides: efficacy, toxicity and mechanism compared with benzalkonium chloride. Future Microbiol. 12, 1297–1310. doi: 10.2217/fmb-2016-0229
Nebe-von Caron, G., Stephens, P., and Badley, R. A. (1998). Assessment of bacterial viability status by flow cytometry and single cell sorting. J. Appl. Microbiol. 84, 988–998. doi: 10.1046/j.1365-2672.1998.00436.x
Nitzan, Y., and Ashkenazi, H. (1999). Photoinactivation of Deinococcus radiodurans: an unusual gram-positive microorganism. Photochem. Photobiol. 69, 505–510. doi: 10.1111/j.1751-1097.1999.tb03320.x
Novo, D. J., Perlmutter, N. G., Hunt, R. H., and Shapiro, H. M. (2000). Multiparameter flow cytometric analysis of antibiotic effects on membrane potential, membrane permeability, and bacterial counts of Staphylococcus aureus and Micrococcus luteus. Antimicrob. Agents Chemother. 44, 827–834. doi: 10.1128/AAC.44.4.827-834.2000
O’Neill, J. (2016). The Review on Antimicrobial Resistance. Tackling Drug-Resistant Infections Globally: Final Report and Recommendations. Available at: https://amr-review.org
Pähkla, E.-R., Koppel, T., Saag, M., and Pähkla, R. (2005). Metronidazole concentrations in plasma, saliva and periodontal pockets in patients with periodontitis. J. Clin. Periodontol. 32, 163–166. doi: 10.1111/j.1600-051X.2005.00653.x
Petit, J. M., Denis-Gay, M., and Ratinaud, M. H. (1993). Assessment of fluorochromes for cellular structure and function studies by flow cytometry. Biol. Cell 78, 1–13. doi: 10.1016/0248-4900(93)90109-R
Preus, H. R., Scheie, A. A., and Baelum, V. (2014). Letter to the editor: Re: The clinical effect of scaling and root planing and the concomitant administration of systemic amoxicillin and metronidazole: a systematic review; Re: Effectiveness of systemic amoxicillin/metronidazole as adjunctive therapy to scaling and root planing in the treatment of chronic periodontitis: a systematic review and meta-analysis; Re: Effectiveness of systemic amoxicillin/metronidazole as an adjunctive therapy to full-mouth scaling and root planing in the treatment of aggressive periodontitis: a systematic review and meta-analysis. J. Periodontol. 85, 374–384. doi: 10.1902/jop.2014.130379
Rams, T. E., Degener, J. E., and van Winkelhoff, A. J. (2014). Antibiotic resistance in human chronic periodontitis microbiota. J. Periodontol. 85, 160–169. doi: 10.1902/jop.2013.130142
Rudney, J. D., Chen, R., Lenton, P., Li, J., Li, Y., Jones, R. S., et al. (2012). A reproducible oral microcosm biofilm model for testing dental materials. J. Appl. Microbiol. 113, 1540–1553. doi: 10.1111/j.1365-2672.2012.05439.x
Ruhl, S., Berlenbach, P., Langenfelder, S., Hörl, D., Lehn, N., Hiller, K.-A., et al. (2011). Integrity of proteins in human saliva after sterilization by gamma irradiation. Appl. Environ. Microbiol. 77, 749–755. doi: 10.1128/AEM.01374-10
Saleem, H. G. M., Seers, C. A., Sabri, A. N., and Reynolds, E. C. (2016). Dental plaque bacteria with reduced susceptibility to chlorhexidine are multidrug resistant. BMC Microbiol. 16:214. doi: 10.1186/s12866-016-0833-1
Schäfer, M., Schmitz, C., and Horneck, G. (1998). High sensitivity of Deinococcus radiodurans to photodynamically-produced singlet oxygen. Int. J. Radiat. Biol. 74, 249–253. doi: 10.1080/095530098141636
Slots, J. (2004). Systemic antibiotics in periodontics. J. Periodontol. 75, 1553–1565. doi: 10.1902/jop.2004.75.11.1553
Späth, A., Leibl, C., Cieplik, F., Lehner, K., Regensburger, J., Hiller, K.-A., et al. (2014). Improving photodynamic inactivation of bacteria in dentistry: highly effective and fast killing of oral key pathogens with novel tooth-colored type-II photosensitizers. J. Med. Chem. 57, 5157–5168. doi: 10.1021/jm4019492
Tabenski, I., Cieplik, F., Tabenski, L., Regensburger, J., Hiller, K.-A., Buchalla, W., et al. (2016). The impact of cationic substituents in phenalen-1-one photosensitizers on antimicrobial photodynamic efficacy. Photochem. Photobiol. Sci. 15, 57–68. doi: 10.1039/c5pp00262a
Tamburini, S., Foladori, P., Ferrentino, G., Spilimbergo, S., and Jousson, O. (2014). Accurate flow cytometric monitoring of Escherichia coli subpopulations on solid food treated with high pressure carbon dioxide. J. Appl. Microbiol. 117, 440–450. doi: 10.1111/jam.12528
Tegos, G. P., Masago, K., Aziz, F., Higginbotham, A., Stermitz, F. R., and Hamblin, M. R. (2008). Inhibitors of bacterial multidrug efflux pumps potentiate antimicrobial photoinactivation. Antimicrob. Agents Chemother. 52, 3202–3209. doi: 10.1128/AAC.00006-08
Tonetti, M. S., Jepsen, S., Jin, L., and Otomo-Corgel, J. (2017). Impact of the global burden of periodontal diseases on health, nutrition and wellbeing of mankind: a call for global action. J. Clin. Periodontol. 44, 456–462. doi: 10.1111/jcpe.12732
van Winkelhoff, A. J., Rams, T. E., and Slots, J. (1996). Systemic antibiotic therapy in periodontics. Periodontol. 2000, 45–78. doi: 10.1111/j.1600-0757.1996.tb00068.x
Wainwright, M., Maisch, T., Nonell, S., Plaetzer, K., Almeida, A., Tegos, G. P., et al. (2017). Photoantimicrobials-are we afraid of the light? Lancet Infect. Dis. 17, e49–e55. doi: 10.1016/S1473-3099(16)30268-7
Wang, H.-Y., Cheng, J.-W., Yu, H.-Y., Lin, L., Chih, Y.-H., and Pan, Y.-P. (2015). Efficacy of a novel antimicrobial peptide against periodontal pathogens in both planktonic and polymicrobial biofilm states. Acta Biomater. 25, 150–161. doi: 10.1016/j.actbio.2015.07.031
Wang, Z., Shen, Y., and Haapasalo, M. (2017). Antibiofilm peptides against oral biofilms. J. Oral Microbiol. 9:1327308. doi: 10.1080/20002297.2017.1327308
Keywords: biofilm, periodontal, photodynamic, phenalen-1-one, chlorhexidine, flow cytometry, propidium iodide, cytoplasmic membrane
Citation: Cieplik F, Steinwachs V-S, Muehler D, Hiller K-A, Thurnheer T, Belibasakis GN, Buchalla W and Maisch T (2018) Phenalen-1-one-Mediated Antimicrobial Photodynamic Therapy: Antimicrobial Efficacy in a Periodontal Biofilm Model and Flow Cytometric Evaluation of Cytoplasmic Membrane Damage. Front. Microbiol. 9:688. doi: 10.3389/fmicb.2018.00688
Received: 21 January 2018; Accepted: 23 March 2018;
Published: 06 April 2018.
Edited by:
Octavio Luiz Franco, Universidade Católica de Brasília, BrazilReviewed by:
Taia Maria Berto Rezende, Universidade Católica de Brasília, BrazilNejat Duzgunes, Arthur A. Dugoni School of Dentistry, United States
Copyright © 2018 Cieplik, Steinwachs, Muehler, Hiller, Thurnheer, Belibasakis, Buchalla and Maisch. This is an open-access article distributed under the terms of the Creative Commons Attribution License (CC BY). The use, distribution or reproduction in other forums is permitted, provided the original author(s) and the copyright owner are credited and that the original publication in this journal is cited, in accordance with accepted academic practice. No use, distribution or reproduction is permitted which does not comply with these terms.
*Correspondence: Fabian Cieplik, ZmFiaWFuLmNpZXBsaWtAdWtyLmRl