- Jiangsu Key Laboratory for Microbes and Functional Genomics, Jiangsu Engineering and Technology Research Center for Microbiology, College of Life Sciences, Nanjing Normal University, Nanjing, China
The proper timing and positioning of cytokinesis/septation is crucial for hyphal growth and conidiation in Aspergillus nidulans. The septation initiation network (SIN) components are a conserved spindle pole body (SPB) localized signaling cascade and the terminal kinase complex SidB-MobA, which must localize on the SPB in this pathway to trigger septation/cytokinesis. The regulatory subunit of phosphatase PP2A-ParA has been identified to be a negative regulator capable of inactivating the SIN. However, little is known about how ParA regulates the SIN pathway and whether ParA regulates the septum formation process through affecting the SPB-localized SIN proteins. In this study, through RNA-Seq and genetic approaches, we identified a new positive septation regulator, a putative mitotic-spindle organizing protein and a yeast Mzt1 homolog MztA, which acts antagonistically toward PP2A-ParA to coordinately regulate the SPB-localized SIN proteins SidB-MobA during septation. These findings imply that regulators, phosphatase PP2A-ParA and MztA counteract the septation function probably through balancing the polymerization and depolymerization of microtubules at the SPB.
Introduction
In all eukaryotic organisms, cytokinesis is the cell division process after the nuclear division of mitosis in which the cytoplasm of a cell is physically partitioned into two. Although organisms of different kingdoms have developed unique mechanisms to execute cytokinesis, signals that trigger the onset of cytokinesis are evolutionarily conserved (Wolkow et al., 1996; Krapp and Simanis, 2008; Seiler and Justa-Schuch, 2010). Many lines of evidence have identified that the conserved mitotic exit network (MEN) components, which are tightly connected with cytokinesis, exist in the budding yeast Saccharomyces cerevisiae and mammalian systems (Foltman and Sanchez-Diaz, 2017; Guo and Segal, 2017; Renicke et al., 2017; Scarfone and Piatti, 2017). A pathway homologous to the MEN, termed the septation initiation network (SIN), has also been found to couple mitotic exit with cytokinesis in the fission yeast, Schizosaccharomyces pombe and the model filamentous fungus A. nidulans (Barr and Gruneberg, 2007; Csikasz-Nagy et al., 2007; Zhong et al., 2012; Simanis, 2015). Genetic analysis has identified that the SIN components are a conserved spindle pole body (SPB) localized signaling cascade, which uses SPB, the functional counterpart of the centrosome in yeasts as a scaffold from which to initiate signaling (Magidson et al., 2006; Lengefeld et al., 2017; Rüthnick et al., 2017). The core SIN network is composed of a GTPase, three protein kinases, an inhibitory GAP complex, and a scaffold complex that anchors the pathway to SPBs (Johnson et al., 2012). Among them, Sid2-Mob1 is the terminal kinase complex in the pathway, which transitions from SPB to the cell division site in anaphase to drive cytokinesis. During the completion of mitosis, SidB and MobA appear at both the SPB and the division site. As the transfer factors, SidB and MobA have the capacity to form a ring to complete septation initiation at the SPB (Harris et al., 1994; Sparks et al., 1999; Kim et al., 2006; Eslami et al., 2014). Therefore, SPB-localized SIN proteins especially Sid2-Mob1 in S. pombe and SidB-MobA in A. nidulans must transmit signals through the cascade to trigger cytokinesis so that the SPB works as a signaling hub for this process.
In addition, the SPB also serves as a microtubule-organizing center (MTOC), to seed the polymerization of the mitotic spindle and to provide the nucleating machinery for regulating microtubule attachment and dynamics and establishing microtubule polarity (Zekert et al., 2010; Takeshita and Fischer, 2011; Kilmartin, 2014; Wieczorek et al., 2015). The major regulator of microtubules (MTs) nucleation is the γ-tubulin ring complex (γ-TuRC), which caps the minus ends of the MTs and facilitates directional MTs nucleation. Thus, MTs are dynamic polymers transiting between polymerization and depolymerization (Xiong and Oakley, 2009; Suri et al., 2014; Walia et al., 2014). Moreover, signals transmitting SPB-localized SIN proteins through the cascade to trigger cytokinesis need spatial and temporal control of MT-dependent cellular restructuring events (Robinson and Spudich, 2004; Rankin and Wordeman, 2010; Zhou et al., 2010; Ngo et al., 2016). In human tissue, a protein called the mitotic-spindle organizing protein (MOZART1) that interacts with γ-TuRC has been identified to promote the polymerization of the microtubule and then give rise to branched microtubules (Hutchins et al., 2010; Teixidó-Travesa et al., 2010; Cukier et al., 2017). However, the knowledge of whether the major regulator γ -TuRC of MTs nucleation affects cytokinesis is still limited.
SIN signaling requires three protein kinases for its function and thus the phosphorylation/dephosphorylation reactions play important roles by regulating protein activity and subcellular localization in the septum formation processes (Bruno et al., 2001; Sharpless and Harris, 2002; Ramsubramaniam et al., 2014; Rusin et al., 2017). Three main classical protein phosphatases including serine/threonine phosphatases, protein tyrosine phosphatases and the aspartate-based catalysis protein phosphatase coordinate and regulate the septation signaling pathway through counteracting several protein kinases (Son and Osmani, 2009). Phosphatase PP2A is a major intracellular protein phosphatase, which contains three subunits, namely, a catalytic subunit, a structural subunit and several regulatory subunits. Previous studies indicate that the regulatory subunits PP2A-Par1 and PP2A-Pab1 in yeasts are negative regulators that inactivate the SIN (Jiang and Hallberg, 2001; Lahoz et al., 2010; Goyal and Simanis, 2012). However, unlike in yeasts, the regulatory subunit of PP2A-ParA counteracts PabA during the septation process. In addition, ParA localizes to the septum site and the deletion of parA causes hyperseptation, while the overexpression of parA abolishes septum formation (Zhong et al., 2014). This suggests that ParA functions as a negative factor in regulating the SIN pathway. However, little is known about how ParA regulates the SIN pathway and whether ParA regulates septum formation process by affecting the SPB-localized SIN proteins.
In this study, through RNA-Seq and genetic approaches, we identified a new positive septation regulator, MztA, which acts antagonistically toward ParA to coordinately regulate SPB-localized SIN proteins SidB-MobA during septation in the filamentous fungus A. nidulans.
Materials and Methods
Strains, Media, and Culture Conditions
A list of A. nidulans strains and oligonucleotides used in this study is provided in Tables 1, 2. YAG (5 g/L Yeast extract + 1 mL/L Trace element + 20 g/L Glucose), YUU (YAG + 1.2 g/L Uridine + 1.1 g/L Uracil), MMPGR (50 mL/L Salt + 10 mL/L Glycerol + 0.5 mg/L Pyridoxine + 2.5 mg/L Riboflavin + 1 mL/L Trace element), MMPGRTUU (MMPGR + 1.2 g/L Uridine + 1.1 g/L Uracil + 11.9 g/L Threonine) and MMPPGRTUU (MMPGRUU with 100 mM p-Aminobenzoic acid). These media have been described in previous works (Gupta et al., 1976; Käfer, 1977). Growth conditions, crosses, and induction conditions for alcA(p)-driven expression were as described previously (Liu et al., 2003). Overexpression of tagged genes under the control of the alcA promoter were induced with threonine (Zhong et al., 2014). Standard DNA transformation procedures were used for A. nidulans (Osmani et al., 1988).
Quantitative Real-Time PCR Analysis
Conidia of parA-overexpressing mutant and the parental wild type (TN02A7) were cultured in MMPGRTUU for 18 h at 37°C with a rotary shaker at 220 rpm and then the collected dried hyphae were pulverized to a fine powder in the presence of liquid nitrogen. The total RNA was extracted using TRIzol (Roche) following the manufacturer's instructions. The samples were treated with DNase I (TaKaRa), and cDNA was generated using an iScript Select cDNA synthesis kit (Bio-Rad). Real-time PCR was performed using an ABI one-step fast thermocycler (Applied Biosystems), and the reaction products were detected with SYBR green (TaKaRa). PCR was accomplished by a 10-min denaturation step at 95°C followed by 40 cycles of 95°C-30 s, 60°C-30 s, 72°C-30 s. Transcript levels were calculated by the comparative CT method and normalized against the expression of the actin gene in A. nidulans (Alam et al., 2012; Long et al., 2016). Primer information is provided in Table 2.
Construction of Mutant Strains
For construction of the OE::parA ΔmztA and the ΔmztA mutants, the A. nidulans AnpyroA gene, which is required for biosynthesis of pyridoxine, was amplified from plasmid pQa-pyroA with the primer pairs pyroA-5′/ pyroA-3′, and used as a selectable nutritional marker for fungal transformation. The upstream and downstream regions of the mztA gene were amplified with primers P1-mztA/P3-mztA and P4-mztA/P6-mztA from the A. nidulans TN02A7 genomic DNA, respectively. Purified linearized upstream and downstream DNA fragments plus the pyroA gene fragment were mixed and used in a double joint PCR with primers P2-mztA/P5-mztA (Yu et al., 2004). The resulting fusion fragment was then cloned into the pEasy-Blunt Zero (TransGen Biotech) vector for generating plasmid pJP01. Finally, the pJP01 plasmid was respectively transformed into the OE::parA strain and the parental wild-type strain to generate strains OE::parA ΔmztA (JPA01) and ΔmztA (JPA02), A similar strategy was used to construct the OE::parAΔpdbAor ΔpdaAor ΔpamAor Δcdc5A and ΔpdbA (ΔpdaA or ΔpamA or Δcdc5A) strains. Briefly, as shown in Figure S1A, upstream and downstream regions of these five genes were amplified from gDNA with primer pairs P1/P3 and P4/P6. Each two regions were then fused to the AnpyroA gene cassette with the nested primer pair P2/P5 and cloned into plasmid pEasy-Blunt Zero. The resulting plasmids were further transformed into OE::parA strain and the parental wild type (TN02A7) to generate related strains. Oligonucleotides used in this study are listed in Table 2. To construct the ΔparAΔmztA mutant, the ΔmztA mutant was crossed with the ΔparA mutant. All progeny were screened according to a standard protocol (Todd et al., 2007).
For constructions of the overexpressed aforementioned five genes in the background of parA-overexpressing, the A. nidulans AnpyroA gene, a selectable nutritional marker, was amplified with primer pair NotI-pyroA-5′/SpeI-pyroA-3′. Then the AnpyroA fragment was cloned into the reconstitute vector pBARGPE1 using the ClonExpress II One Step Cloning Kit (VazymeTM, C112-02), this strain was designated pBARGPE1-1, which contains the AngpdA promoter. Using gDNA as a template, the ORF fragments of those five genes were amplified with primer pairs mztA-ClaI-5′/mztA-ClaI-3′, pdbA-ClaI-5′/pdbA -ClaI-3′, pdbA-SmaI-5′/pdbA-SmaI-3′, pamA-ClaI-5′/ pamA-ClaI-3′, cdc5A-ClaI-5′/cdc5A-ClaI-3′, respectively, and subsequently were cloned into pBARGPE1-1. The resulting relevant plasmids were transformed into the OE::parA strain.
For generation of strains WTmztA, ΔmztAmztA, OE::parAmztA, the parental wild-type mztA gene driven by the endogenous promoter was introduced into WT, ΔmztA, OE::parA, respectively. An 1876-bp AfpyrG fragment was amplified with primer pairs Af-pyrG-5′/Af-pyrG-3′ from plasmid pXDRFP4. The 1759-bp AnpyroA gene was amplified with primer pair pyroA-5′/pyroA-3′ from plasmid pQa-pyroA. AfpyrG and AnpyroA were chosen as two selectable nutritional markers, so fragments of mztA gene with endogenous promoter was amplified from the A. nidulans TN02A7 genomic DNA with primers n-mztA-5′/n-mztA-3′ and n-mztA-5′/n-mztA-2-3′, respectively, referred as fragments 1 and 2. Then the fragment 1 was fused with AfpyrG and PCR cassette was cloned into plasmid pEasy-Blunt Zero and transformed into strain TN02A7, ΔmztA to generate WTmztA, ΔmztAmztA. Fragment 2 subsequently was fused with AnpyroA and the resulting fusion product was cloned into plasmid pEasy-Blunt Zero then transformed into strain OE::parA to obtain the OE::parAmztA. For WTOE::mztA, ΔmztAOE::mztA construction, the plasmid containing mztA gene controlled by gpdA promoter and selective markers pyroA or riboB genes were co-transformed into the indicated mutants.
To generate an alcA(p)-gfp-mobA fusion construct, a 594-bp fragment of mobA was amplified from TN02A7 genomic DNA by use of primers mobA-NotI-5′ /mobA-XbaI-3′ (see Table 2) and then mobA was cloned into the corresponding sites of pLB01, yielding pLB-mobA (Liu et al., 2003). This plasmid was transformed into the receipt strain R21. Homologous recombination of this plasmid into the mobA locus should result in an N-terminal green fluorescent protein (GFP) fusion with the product of the entire mobA gene under the control of the alcA promoter. This strain was referred as alc(p)-mobA-GFP. For generation of OE::parAmobA−GFP, OE::mztAmobA−GFP and OE::parA OE::mztAmobA−GFP strains, alc(p)-mobA-GFP was crossed with the OE::parA, OE::mztA and OE::parA OE::mztA mutants, respectively.
Microscopy and Image Processing
To visualize the process of septation, conidia of indicated strains were incubated in indicated liquid medium on sterile glass coverslips, respectively, at related temperatures prior to observation under a microscope. The signal of septum was observed in live cells by placing the coverslips on a glass slide. Then removed the media from coverslips and washed three times by phosphate buffered saline (PBS). After that, 4% paraformaldehyde (Polyscience, Warrington, PA) was used to fix the cells and Calcofluor white (CFW) (Sigma-Aldrich, St. Louis, MO) was added for septum and chitin staining for keeping in dark for 10 min. After staining, the staining solution was removed and washed with PBS for three times. Finally, above coverslips were placed on a glass slide and sealed with nail oil. Microscopic images of cells were collected with a Zeiss Axio Imager A1 microscope (Zeiss, Jena, Germany). These images were then collected and analyzed with a Sensicam QE cooled digital camera system (Cooke Corporation, Germany) with the MetaMorph/MetaFluor combination software package (Universal Imaging, West Chester, PA), and the results were assembled in Adobe Photoshop 7.0 (Adobe, San Jose, CA). A similar approach was taken to visualize the localization of MobA-GFP in related strains. 4′,6-diamidino-2-phenylindole (DAPI) (Sigma-Aldrich, St. Louis, MO) was used to stain DNA.
RNA Isolation for Northern Analysis
All test mutants were grown in the liquid medium-MMPGRTUU shaken on a rotary shaker at 220 rpm at 37°C. G7 is referred as the harvest detection time-point when conidia were cultured for 7 h. V12 and V18 represent the vigorous hyphal growth time-point when conidia were cultured for 12 or 18 h. S24 presents the sporulation time-point when conidia were cultured for 18 h and then the liquid cultures were transferred to the solid culture plate for 24 h. Pulverized the mycelia of those strains to fine powder in the presence of liquid nitrogen. RNA purification and Northern blot analysis were performed as described. Briefly, the total RNA was extracted using TRIzol (Roche) following the manufacturer's instructions. 10 μg of RNA was used for electrophoresis on 1.1% formaldehyde agarose gels and subsequently blotted onto a nylon membrane (Bio-Rad). Probed are labeled with DIG (digoxigenin)-labeled oligonucleotide probes complementary to the mRNA of mztA or parA. Primer information is provided in Table 2. The blots are pre-hybridized with high-SDS for at least 5–7 h at 42°C. Late in the afternoon the DIG-probes are heated up to 65°C for 10 min and the high-SDS solution in the tube containing the membrane was exchanged with the probe. This is then further incubated overnight at 42°C. Finally, signals of the RNA bands were showed by using an CSPD(Sigma) (Misslinger et al., 2017).
Results
Identification for the Suppressors of ParA in Septation and Conidiation
Our previous data confirmed that ParA, a regulatory subunit of protein phosphatase 2A (PP2A), is a negative regulator of septation since deletion of parA causes a hyperseptation phenotype while overexpressing parA results in abolished septum formation in hyphal cells (Zhong et al., 2014). To further address how overexpressing parA causes completely abolished septation, RNA-Seq analysis was performed in the parA-overexpressing mutant compared to that of its parental wild-type strain (TN02A7). A total of 551 mRNAs had an altered abundance in the parA-overexpressing mutant compared with the parental wild-type strain when using a 2-fold change as a cutoff. Among them 453 genes were up-regulated and 98 genes were down-regulated. To analyze the affected gene expression induced by overexpressing parA, we selected candidate genes based on a putative septation-related function in S. pombe as well as having a ratio>5 for their fold change. Based on these two criteria, five candidate genes were obtained with up-regulation of 9.8-, 9.1-, 8.9-, 7.9-, and 7.6-fold the OE::parA mutant. As queries in the NCBI and CADRE databases in A. nidulans, those five genes are referred as mztA (AN1361.4, Accession:Q5BDL9.1), pdbA (AN8559.4,Accession: CBF80798.1), pdaA (AN1726.4,Accession: CBF85442.1), pamA (AN3493.4,Accession: CBF76042.1) and cdc5A (AN7174.4,Accession: CBF78928.1), respectively. To verify that overexpressing parA caused the changed expression of those five genes as seen in the RNA-Seq data, a real-time PCR was further carried out to visualize the mRNA abundance level of these five genes in the OE::parA strain. As shown in Figure 1A, the expression level of mztA, pdbA, pdaA, pamA and cdc5A was increased 8.03-, 7.30-, 7.00-, 7.02-, and 4.88-fold compared to that of its reference strain, respectively, which was comparable to that of RNA-Seq data for some content. Therefore, we hypothesize that one of reasons for completely abolished septation in the parA-overexpressing strain might be the result of the overexpression of the aforementioned five genes. To check whether deleting those five genes could rescue the septation defect of the OE::parA strain, we constructed mztA-, pdbA-, pdaA-, pamA-, and cdc5A-null mutants in the background of OE::parA and the parental wild-type strains. Diagnostic PCR analysis showed that those deletion mutants had the correct insertion of the AnpyroA disruption cassette in the relative gene coding sequence loci, and no original ORF of those five genes could be detected, indicating that the relative genes were fully deleted in the background of OE::parA and the parental wild-type strains (Figure S1). As shown in the upper panel of Figure 1B, none of those deletions could suppress the nearly aconidial colony morphology in the parA-overexpressing mutant. Likewise, under the liquid culture conditions, four of them could not rescue the septation defect eourourxcept for pdbA deletion, which caused a septum restoration in the background of the OE::parA mutant (Figure 2A). These data suggest that completely abolished septation in the parA-overexpressing strain might not directly result from the overexpression of the aforementioned four genes. While overexpressed pdbA may be related to the defective septation in the parA-overexpressing strain. In addition, ΔmztA and ΔpdbA exhibited a slightly attenuated conidiaton, plus decreased colony diameters of approximately 70 and 50%, respectively, of that of its parental wild type (Figure 1B), while the pdaA deletion mutant only showed decreased conidiation with almost normal colony size. In comparison, ΔpamA and Δcdc5A showed WT-like colony phenotypes.
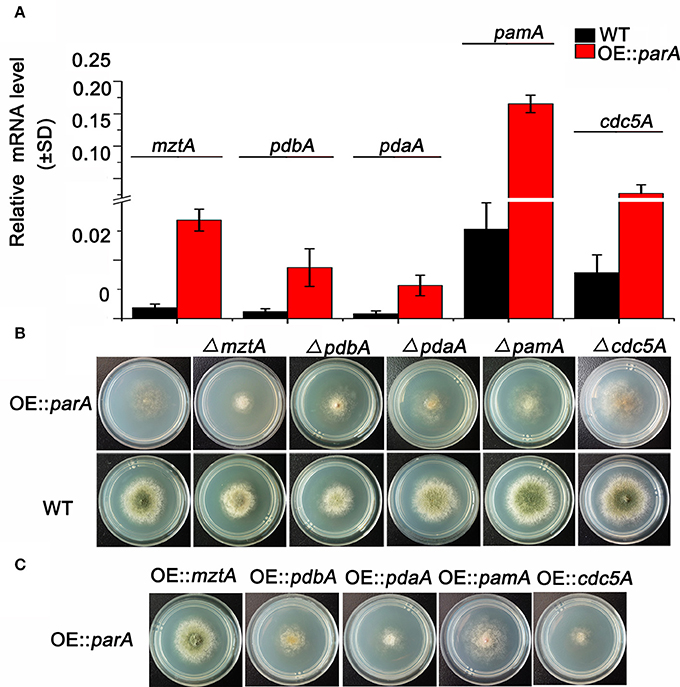
Figure 1. Identification of suppressors of parA in septation and conidiation. (A) The relative mRNA levels of mztA, pdbA, pdaA, pamA, and cdc5A, respectively, using real-time RT-PCR assays in the parA-overexpressing mutant and parental wild type (TN02A7). Those two strains cultured in liquid MMPGRTUU medium at 37°C at 220 rpm for 18 h. The measured quantity of the mRNA in each of the treated samples was normalized using C T values obtained for the internal reference actin (AN3696.4). (B) Phenotypic characterization of mztA, pdbA, pdaA, pamA, and cdc5A deletion mutants in the background of the parA-overexpressing mutant and the parental wild type (TN02A7), respectively. All the strains were cultured on solid MMPGRTUU medium for 2.5 days. (C) Colony morphologies of the overexpressed mutants for mztA, pdbA, pdaA, pamA and cdc5A genes in the background of the parA-overexpressing mutant. All the strains were cultured on solid MMPGRTUU medium for 2.5 days.
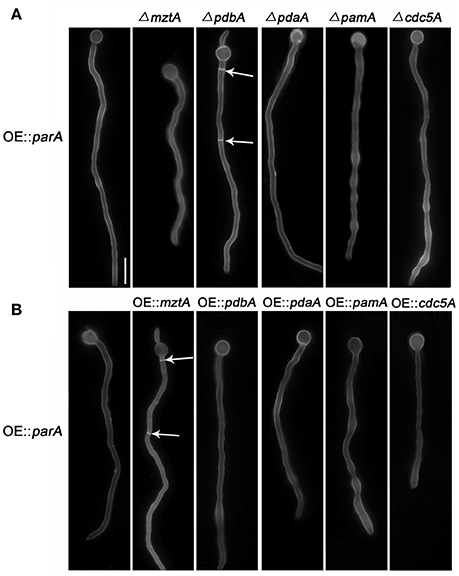
Figure 2. Hyphal morphologies of the mztA, pdbA, pdaA, pamA, and cdc5A deletions (A) and their overexpression (B) in the background of the parA-overexpressing mutant cultured in liquid MMPGRTUU media and stained with Calcofluor white (septa). All the strains were cultured at 37°C for 11 h. Arrows indicate the locations of septa. Bars, 10 μm.
In contrast, we next wondered that whether overexpressing those five genes could rescue the sick morphology of the parA-overexpressing mutant. We then over-expressed them by an AngpdA-driven constitutive promoter in the background of the parA-overexpressing strain. Diagnostic PCR revealed those related strains that were constructed successfully (Figure S2). Notably, over-expression of mztA significantly restored the OE::parA mutant to a phenotype with WT-like conidiation and radial hyphal growth as shown in Figure 1C. In comparison, over-expression of the other four genes could not rescue the aconidial colony to the wild-type phenotype. Consistently, liquid cultural observation demonstrated that OE::mztA could rescue the septation defect of over-expressed parA, while there were no obvious differences for septation between the other four over-expression strains and its parental wild-type strain (Figure 2B). Therefore, those results suggest that mztA works as a suppressor of parA in septation and conidiation in A. nidulans.
Septation and Conidiation Defects Induced by Overexpressing parA Are Rescued by Overexpressing mztA in a Dose-Dependent Way
The abovementioned phenomena led us to question whether the restoration of the defective phenotype in the OE::parA mutant by overexpressing mztA is in a dose-dependent. To test this hypothesis, we constructed six complementation strains by introducing the parental wild-type mztA gene driven by the endogenous promoter and the constitutive promoter (gpdA) into ΔmztA, OE::parA and the parental wild-type strains, respectively (Figure 3A). Diagnostic PCR analysis indicated that all the strains were constructed successfully (Figure S3). To further ensure that the expression level of mztA in these complementation strains was truly over-expressed, Northern blotting was used to detected the mRNA expressions and 28 and 18 S RNA as the loading control shown in Figure 3C. As expected, the mRNA abundance level of the mztA gene driven by an endogenous promoter in WTmztA, ΔmztAmztA and OE::parAmztA were separately increased 4.65-, 7.25-, and 12.75-fold changes compared with the parental wild-type. Meanwhile, the mztA transcript level driven by the constitutive promoter (gpdA) in WTOE::mztA, mztAOE::mztA and OE::parAOE::mztA was up-regulated 20.6-, 19.55-, 27.8-fold times compared with the WT. These data indicated that introducing mztA into ΔmztA, OE::parA and the parental wild-type strains driven by the self-promoter and AngpdA promoter were truly resulted in the relative overexpression of mztA. As shown in Figures 3A,B, phenotypic analysis suggests that introducing either low copies or high copies of the mztA gene into WT, ΔmztA receipt strains had no obvious difference in colony size and conidia production compared to that of the parental wild type. Furthermore, the aconidial defect phenotype in the OE::parA mutant could be dramatically restored by over-expressed mztA gene under the control of the gpdA promoter to nearly the levels of the parental wild type strain and partially rescue of the conidiation defect was observed on the solid media of OE::parAmztA, which was controlled by native promoter (Figures 3A,B). Interestingly, in submerged liquid culture, we found that, in spite of low copies of the mztA gene, it could not completely restore the aconidiation defect of OE::parA, but it truely recovered the sepetation of OE::parA (Figure 3D). Collectively, the above results showed that restoration of the defective phenotypes of the OE::parA mutant showed a dose-dependent response to the over-expressed mztA gene.
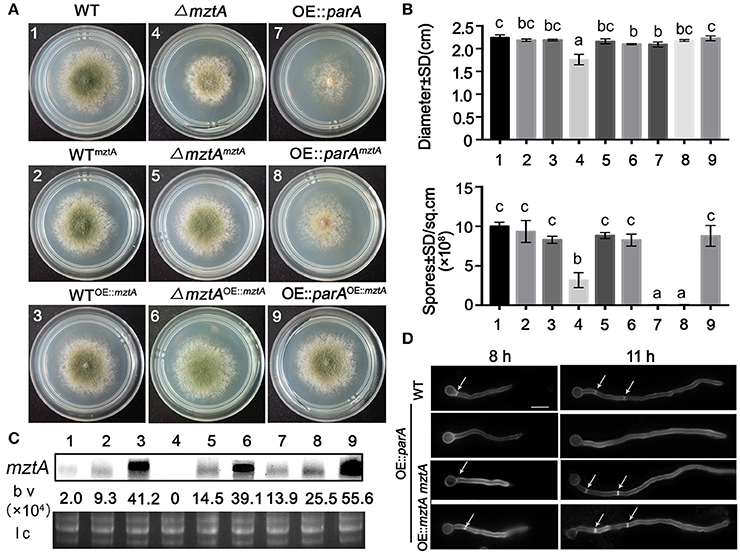
Figure 3. Septation and conidiation defects induced by overexpressed parA are rescued by overexpressed mztA in a dose-dependent manner. (A) The colony morphology of the overexpressed mztA mutant under the control of its endogenous promoter or a constitutive promoter “Angpd(p)” in the parental wild type (TN02A7), ΔmztA and parA-overexpressing strains, respectively. The superscript “mztA” represent the overexpression mztA controlled by the endogenous promoter. The superscript “OE::mztA” represents the overexpression of mztA controlled by the “Angpd(p)” promoter. (B) Quantitative data for the diameter and quantitative number of spores for the strains shown in panel A. The number under the histogram indicates the WT, WTmztA, WTOE::mztA,ΔmztA,ΔmztAmztA, ΔmztAOE::mztA, OE::parA, OE::parAmztA, and OE::parAOE::mztA. Error bars represent the standard deviation from three replicates. Different lowercase letters on the bars of each group represent significant differences among the strains. P < 0.05. (C) Expression level of mztA mRNA was detected by Northern blotting analysis with RNA extracted from the strains shown in panel A. The capital letters,1-9, indicate the WT, WTmztA, WTOE::mztA,ΔmztA,ΔmztAmztA, ΔmztAOE::mztA, OE::parA, OE::parAmztA, and OE::parAOE::mztA. All the strains cultured in liquid MMPGRTUU at 37°C 220 rpm for 18 h. Equal loading of the RNA samples were evaluated by 28 S and 18 S RNA bands. Numbers below the blots denoted the relative density of each band normalized as calculated using ImageJ. b v: band volume; l c: loading control. (D) Comparison of the septum formation in hyphal cells between the parental wild type (TN02A7), OE::parA, OE::parAmztA, and OE::parAOE::mztA. All the strains were cultured in liquid MMPGRTUU medium for 8 h and 11 h, respectively. Arrows indicate the locations of septa. Bars, 10 μm.
Reduced Septa in ΔmztA Are Suppressed by Deleted parA
With the aim of understanding the function of MztA during septation, the ΔmztA strain was analyzed under a dissecting microscope used to analyze the Calcofluor white staining shown in Figure 4A. When conidial spores were inoculated into liquid medium for 10, 11, and 12 h at 37°C, and hyphae were stained with CFW, the mutant displayed an abnormal reduced septa distribution in ΔmztA mature cells compared to that of the parental wild type (Figure 4A). The distance between the septa in the ΔmztA mutant was 41.29 ± 4.32 μm (n = 105) instead of the distance of 20.54 ± 6.51 μm (n = 105) seen in the parental wild type of the same hyphae length (Figure 4B). Thus, according to the average distance between the septa, we conclude that the ΔmztA mutant had a reduced or delayed septation defect phenotype. To better understand the relationship between parA and mztA, Northern blotting was carried out to visualize the mRNA abundance levels of these two genes in various developmental stages (Figure 4C). The 28 S and 18 S RNA were the loading control for Northern blotting. As a result, parA was abundantly expressed in all indicated stages, but mztA was strongly expressed in G7 and V12, and then weakly expressed in V18 and almost vanished in S24, suggesting that the expression pattern for mztA differs substantially from that of parA. Since the aforementioned data indicated that over-expressed mztA had a reverse effect on the overexpression of parA during septation, we further tested whether this suppressed phenotype for septation could be happen due to the double deletions of parA and mztA. By crossing the ΔparA strain with the ΔmztA mutant as described in the Material and Methods section, a ΔparAΔmztA double deletion strain was generated. Diagnostic PCR showed that both coding sequences of parA and mztA have not been detected in the double deletion mutant, suggesting that those strains were constructed successfully (Figure S4). As shown in Figure 5A, a microscopic study found that the single deletion of parA had a hyperseptation phenotype, while the mztA deletion mutant had a reduced-septa defect, and the double deletion strain showed almost normal septation formation compared to that of the parental wild type. Quantified analysis identified that the mature hyphal cells of ΔparA mutant were 14.21 ± 6.17 μm (n = 110), while the ΔparAΔmztA double-deletion mutant had almost the normal septum formation with a septa distance of 20.65 ± 5.01 μm (n = 100; Figure 5B). These data suggest that the mztA deletion could suppress the hyperseptation of the ΔparA mutant. We also observed the morphology of the conidiophores in ΔparA, ΔmztA, ΔparAΔmztA, and the parental wild type strains. mztA and parA single deletion strains could develop a few irregular metulae and phialides with some conidia, whereas in the parA and mztA double deletion mutant there were more severely defects of metulae, and most of the hyphal cells in the ΔparAΔmztA mutant were unable to form these metulae structures. Interestingly, the parA deletion strain showed abnormal multiple septa in the stalk compared to the parental wild type strain having no any septa in the stalk, further indicating that parA is a negative regulator. In comparison, under the same culture condition, ΔmztA also showed a non-septum phenotype in the stalk. However, deletion of mztA could restore the abnormal multiple septa of the stalk in the deletion of parA to the wild-type non-septum phenotype (Figure 5C). Meanwhile, compared to the ΔparA or ΔmztA single mutant, the ΔparAΔmztA double-deletion mutant showed more severe radical growth and conidiation defects with very tiny and aconidial colony but it was not the synthetic lethality phenotype (Figure 5D). All the data indicate that the mztA deletion was capable of suppressing the septation defect of the parA deletion and both parA and mztA are simultaneously required for hyphal growth, conidiation and septation.
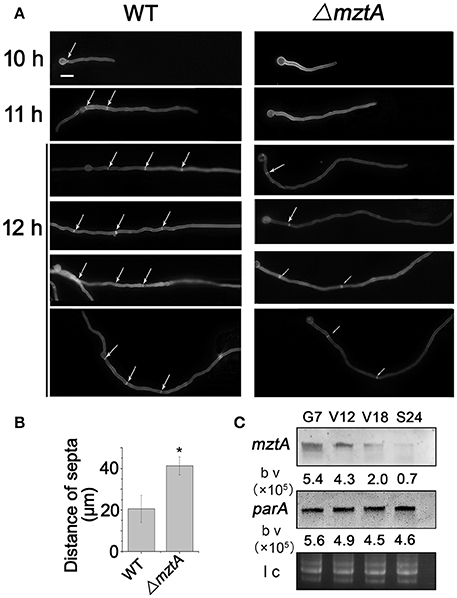
Figure 4. Abnormal distribution of the septa in the mztA deletion mutant and the mRNA levels for the mztA and parA genes during different development stages in the wild type (TN02A7). (A) Comparison of the septum distribution in hyphal cells between the parental wild type (TN02A7) and ΔmztA mutant. Those two strains were grown in MMPGRTUU medium at 37°C for 10,11, and 12 h. Septa were stained by Calcofluor white. Arrows indicate the locations of the septa. Bars, 10 μm. (B) Quantitative data for the septum distance of the WT and ΔmztA mutant under the same cultural condition and time. Statistical significance was determined by Student's test, with P < 0.05 (*). (C) Expression levels of mztA and parA mRNA were shown by Northern blotting analysis with RNA extracted from the wild-type strain (TN02A7) throughout the four developmental time-points. The capital letters, G7, V12, V18, and S24, indicate the germination time point (G7), the vigorous hyphal growth time point (V12 and V18) and the sporulation time point (S24), respectively. Equal loadings of RNA samples were evaluated by 28 and 18 S RNA bands (l c: loading control). Numbers below the blots denoted the relative density of each band normalized as calculated using ImageJ (b v: band volume).
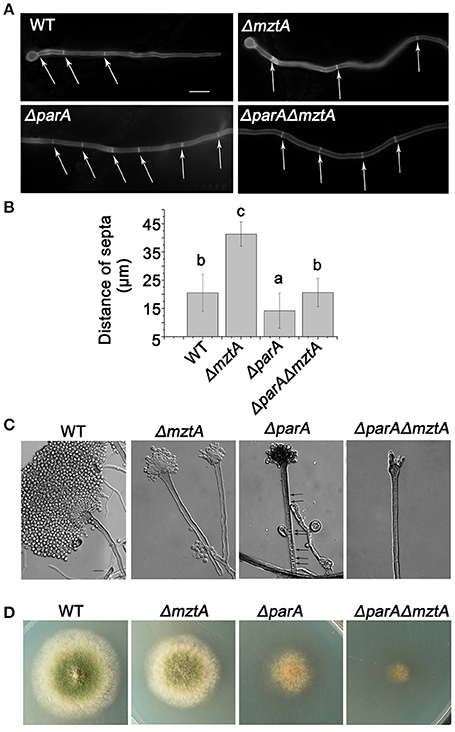
Figure 5. Reduced septa in ΔmztA are suppressed by deleted parA. (A) Comparison of the septum distribution in hyphal cells between the parental wild type (TN02A7), ΔmztA, ΔparA and ΔparAΔmztA. All the strains were cultured in liquid MMPGRTUU medium at 37 °C for 12 h. Arrows indicate the locations of septa. Bars, 10 μm. (B) Quantitative data for the septum distance of the strains in (A). Different lowercase letters on the bars of each group represent significant differences among strains. P < 0.05. (C) Conidiophores of the parent control strains (TN02A7), and the ΔmztA, ΔparA, and ΔparAΔmztA mutants. Arrows indicate the locations of septa. Bars, 10 μm. (D) Colony morphologies of the parental wild type (TN02A7), and the ΔmztA, ΔparA, and ΔparAΔmztA strains cultured on YUU at 37°C for 2 days.
parA and mztA Coordinately Affect MobA Localization
According to the above results, we next asked how the function of mztA could suppress parA during septation. We speculated that parA and mztA may coordinately affect the recruiment of the components in the septation initiation network (SIN) during septation. We next found that the most well-known component of SIN, MobA, always appeared at the top of nuclei, which was known to be the position of spindle pole body (SPB). During septation, MobA localized to the septation site forming a ring and then accumulated gradually to the central region of the septation site. First, we constructed a conditional strain named alc(p)-GFP-mobA, in which the expression of mobA was able to be repressed by glucose on YAG medium, nonrepressed by glycerol on MMPGR, and induced by glycerol plus threonine on MMPGR. Diagnostic PCR indicated that the gene cassette was integrated into the predicted site in this conditional strain (Figure S5). Microscopic observation showed that GFP-MobA indeed localized at the SPB and septum site in the mature cell under induced condition. Based on the localization and function of MobA, we hypothesized that MobA might play an important role in ParA and MobA coordinately control of the SIN pathway. Thus, we generated strain JPA24, JPA25 and JPA26 which expressed the MobA protein as a GFP-tagged fusion protein on the background of OE::mztA, OE::parA and double overexpression mztA and parA strains by genetic-crossing alc(p)-GFP-mobA with relative strains, respectively. Microscopic observation showed that for the wild-type and the OE::mztA strains, during septation, MobA localized to the septation site forming a ring and then accumulated gradually to the central region of the septation site. However, GFP-MobA in the OE::parA mutant exhibited cytosol localization with no detectable signal at the SPB and the septum. Most interestingly, the double overexpression of parA and mztA strain showed a wild-type GFP-MobA signal localized at the SPB and the septation site to those in the alc-GFP-mobA mutant (Figures 6A,B). Our finding demonstrated that overexpressing mztA could rescued the abnormal localization of GFP-MobA induced by overexpressing parA.
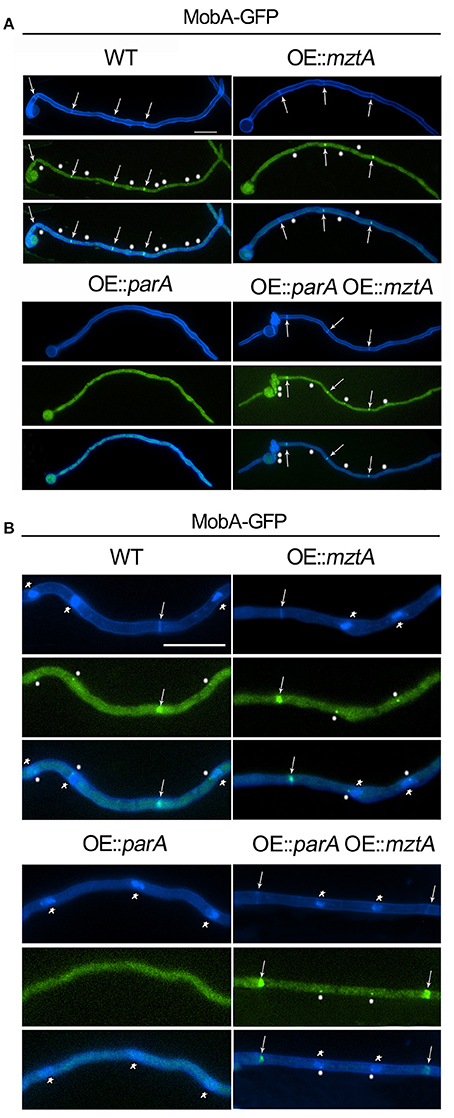
Figure 6. Localization patterns of GFP-tagged MobA in the parental wild type (R21), mztA-overexpressing, parA-overexpressing, and mztA and parA-double overexpressing mutants. Calcofluor white(CFW) was used to visualize the septa. (A,B) show different enlargement. All the strains were cultured in liquid medium MMPPGRTUU at 37°C for 12 h. Arrows indicate the location of septa, asterisk indicate the location of the spindle pole body (SPB) and polygon indicate the locations of nuclei. Bars, 10 μm.
Discussion
Our previous data have confirmed that ParA, a regulatory subunit of protein phosphatase 2A (PP2A), is a negative regulator so that overexpression of PP2A-ParA causes complete abolished septation in A. nidulans (Zhong et al., 2014). MztA was reported to be a putative mitotic-spindle organizing protein and functioned in connecting the ring of γ-tubulin complex to the SPB (Masuda et al., 2013; Batzenschlager et al., 2014). Here, we found it is a new regulator of septation as a suppressor of PP2A-ParA in A. nidulans.
Using the RNA-Seq technique to systematically check the fold changes of mRNA expression, we found that overexpressing parA led to the up-regulation of a series of genes. According to the homolog information in S. pombe for the selected 5 genes, all indicated their functions might be related to septation. Therefore, by RT-PCR analysis, we further verified whether expression of these five genes was truly affected by overexpression of parA. As shown in Figure 1A, expression of the aforementioned five genes were significantly up-regulated in the overexpressed parA strain. However, deleting four of them (mztA, pdaA, pamA, cdc5A) could not rescue the conidiation and septation defects induced by overexpressing parA, suggesting completely abolished septation in the parA-overexpressing strain, which might not directly result from the overexpression of the aforementioned four genes. In comparison, the deletion of another gene, pdbA, a homologous gene of pdb1 in S. pombe, which is a putative beta subunit of the branched chain alpha-keto acid dehydrogenase E1-beta subunit, caused a septum restoration in the parA-overexpressing background mutant. In S. pombe, it has been identified that the pdb1 deletion mutant was lethal after spore germination without cell division and with elongated germ tubes (Cavan and MacDonald, 1994; Beltrao et al., 2009; Kettenbach et al., 2015). Additionally, a previous study has identified that pdb1 and par1 are both detected in purified Paa1p-TAP complex by using LC-MS/MS while the A-subunit of the PP2A holoenzyme, Paa1p which has a function of controlling the asymmetric protein localization to old mitotic SPB, is included in SIN-inhibitory phosphatase complex(Singh et al., 2011; Johnson et al., 2012). These data suggest that pdb1 and par1 in S. pombe might be coordinately regulate the SIN-inhibitory phosphatase complex. Most notably, our data indicate that the pdbA deletion in the background of parA-overexpressing strain displayed a phenotype of septum restoration under the liquid cultural condition. This finding suggests that the pdbA might be also involved in the function of co-regulating cytokinesis with parA in the filamentous fungus A. nidulans, which has not previously been reported. The possible explanation for this phenotypic phenomenon is that ParA and PdbA might work in a concerted fashion to keeping the SIN proteins on the old SPB. Overexpressing of parA may break this asymmetric localization pattern in the old mitotic SPB. In contrast, deletion pdbA could rescue the asymmetric protein re-localization on the old SPB resulted from overexpressed parA, suggesting pdbA has a parallel complementary function with parA during septation.
Most interestingly, we found that overexpression of mztA could remarkably restore the defective phenotype of the parA-overexpressing strain to the wild-type-like septation and conidiation. Moreover, there is evidence that defects induced by overexpressed parA are rescued by overexpressed mztA in a dose-dependent manner, and deletion of mztA restores the abnormal multiple septa of the stalk in the deletion of parA to the wild-type non-septum phenotype, which further demonstrates that mztA works as a suppressor of parA during septation and conidiation in A. nidulans.
Additionally, the mztA single deletion strain showed a significantly reduced septa phenotype, implying that mztA itself may act as a positive regulator for septation in A. nidulans. Previous studies in S. pombe, Drosophila melanogaster, Xenopus laevis, and Homo sapiens have demonstrated that MztA homologous protein could function as a component of the γ-TuRCs (γ-tubulin ring complexes), which is essential for its recruitment to the microtubule-organizing centers (Raff et al., 1993; Stearns and Kirschner, 1994; Hutchins et al., 2010; Dhani et al., 2013). In contrast, γ-TuRC, which consists of γ-tubulin and γ-tubulin complex proteins 2–6 (GCP2–6), possesses a potent microtubule nucleation activity (Stearns and Kirschner, 1994; Gard et al., 1995; Zheng et al., 1995; Masuda et al., 2013). Meanwhile, SPB can act as an MTOC to initiate microtubule nucleation, where the microtubule serves as a track for SIN proteins transporting from SPB to the septum site. The process of γ-TuRCs anchors to SPB and the transportation of the SIN signal pathway to SPB are both required for the septation in A. nidulans (Zekert et al., 2010; Johnson et al., 2012; Zhang et al., 2017). Therefore, the abovementioned information suggests that the SPB-localized MztA may also affect cytokinesis/septation through regulating the microtubule nucleation activity. Through Northern blotting we found parA was abundantly expressed in all indicated stages, but mztA was strongly expressed in G7 and V12, and then weakly expressed in V18 and almost vanished in S24, suggesting that the expression pattern for mztA differs substantially from that of parA (Figure 4C). Moreover, based on data from Figure 5, deletion of mztA showed an impact on conidiophore morphology and colony growth. Therefore, we hypothesize that MztA may regulate the upstream genes of sporulation pathway. Previous studies have identified that not all genes such as fphA (phytochrome), lreA and lreB (blue-light sensor), laeA (methyltransferase) that affect the production of spores will be highly expressed during the process of conidiation (Bok and Keller, 2004; Purschwitz et al., 2008; Etxebeste et al., 2010).
On the other hand, microtubules require polymerization and depolymerization to ensure the balance of the diverse cellular structures and processes in eukaryotes. Previous studies have demonstrated that PP2A, a serine–threonine protein phosphatase, also associates with microtubules. For example, in Caenorhabditis elegans embryos, PP2A and its regulatory subunit SUR-6, together with the cortically directed microtubule pulling force, actively disassemble PCM (pericentriolar material) during mitotic exit (Enos et al., 2018). Likewise, in COS cells, okadaic acid, an inhibitor of protein PP2A, could impair the binding ability of tubulin carboxypeptidase to microtubules thereby affecting the stability of MTs (Hiraga and Tamura, 2000; Contín et al., 2003; Nunbhakdi-Craig et al., 2007; Yoon et al., 2008). Thus, based on recent literature and our findings, we conclude that ParA and MztA may cooperate to control the stability of microtubules. MztA may strengthen the stability of MTs to ensure the exact localization of SIN members to SPB, whereas ParA may be able to depolymerize MTs through increasing tubulin carboxypeptidase activity (Hiraga and Tamura, 2000; Tar et al., 2006). To clarify this hypothesis, we further observed the localization of GFP-MobA, a core member of SIN, which has been demonstrated to appear at the spindle pole body (SPB) and the septum site in previous studies. Clearly, the overexpression of parA truly causes the abnormal non-SPB localization of GFP-MobA with a cytoplasmic distribution throughout the hyphal cells. In comparison, the double overexpression of parA and mztA strain showed a wild-type GFP-MobA signal at the SPB and septation site, indicating that the abnormal localization of GFP-MobA induced by over-activated PP2A-ParA, can be rescued by over-expressed MztA. This phenomenon is coincided with the phenotypic rescue experiment observed in the double over-expressed parA and mztA strain. These findings imply that regulators, which may affect the stability of microtubules, could change the correct localization of the SIN components in the SPB. Consequently, overexpressing mztA can suppress the defected septation phenotype induced by overexpressing parA probably through maintaining the microtubule balance of polymerization and depolymerization at SPB/MTOC.
Author Contributions
PJ and LL: conception and design of the investigation. PJ and SZ: completion of the experiments. PJ and LL: evaluation and analysis of the results and manuscript writing. PJ, SZ, and LL: final approval of the manuscript.
Conflict of Interest Statement
The authors declare that the research was conducted in the absence of any commercial or financial relationships that could be construed as a potential conflict of interest.
Acknowledgments
This work was financially supported by the National Natural Science Foundation of China (NSFC31370112), the Priority Academic Program Development (PAPD) of Jiangsu Higher Education Institutions and the Research to LL, and the Special Fund for the Doctoral Program of High Education of China to PJ (no. 1812000002152); the plasmid pFNO3 including GFP gene were purchased from FGSC (http://www.fgsc.net). A. nidulans strain TN02A7 (AJM68) was a gift of Christopher J. Staiger (Purdue University, West Lafayette).
Supplementary Material
The Supplementary Material for this article can be found online at: https://www.frontiersin.org/article/10.3389/fmicb.2018.00988/full#supplementary-material
References
Alam, M. K., El-Ganiny, A. M., Afroz, S., Sanders, D. A., Liu, J., and Kaminskyj, S. G. (2012). Aspergillus nidulans galactofuranose biosynthesis affects antifungal drug sensitivity. Fungal Genet. Biol. 49, 1033–1043. doi: 10.1016/j.fgb.2012.08.010
Barr, F. A., and Gruneberg, U. (2007). Cytokinesis: placing and making the final cut. Cell 131, 847–860. doi: 10.1016/j.cell.2007.11.011
Batzenschlager, M., Herzog, E., Houlné, G., Schmit, A. C., and Chabouté, M. E. (2014). GIP/MZT1 proteins orchestrate nuclear shaping. Front. Plant Sci. 5:29. doi: 10.3389/fpls.2014.00029
Beltrao, P., Trinidad, J. C., Fiedler, D., Roguev, A., Lim, W. A., Shokat, K. M., et al. (2009). Evolution of phosphoregulation: comparison of phosphorylation patterns across yeast species. PLoS Biol. 7:e1000134. doi: 10.1371/journal.pbio.1000134
Bok, J. W., and Keller, N. P. (2004). LaeA, a regulator of secondary metabolism in Aspergillus spp. Eukaryotic Cell 3, 527–535. doi: 10.1128/EC.3.2
Bruno, K. S., Morrell, J. L., Hamer, J. E., and Staiger, C. J. (2001). SEPH, a Cdc7p orthologue from Aspergillus nidulans, functions upstream of actin ring formation during cytokinesis. Mol. Microbiol. 42, 3–12. doi: 10.1046/j.1365-2958.2001.02605.x
Cavan, G., and MacDonald, D. (1994). Mutations which reduce levels of pyruvate dehydrogenase in Schizosaccharomyces pombe cause a requirement for arginine or glutamine. FEMS Microbiol. Lett. 124, 361–365.
Contín, M. A., Purro, S. A., Bisig, C. G., Barra, H. S., and Arce, C. A. (2003). Inhibitors of protein phosphatase 1 and 2A decrease the level of tubulin carboxypeptidase activity associated with microtubules. Eur. J. Biochem. 270, 4921–4929. doi: 10.1046/j.1432-1033.2003.03893.x
Csikász-Nagy, A., Kapuy, O., Gyorffy, B., Tyson, J. J., and Nová, B. (2007). Modeling the septation initiation network (SIN) in fission yeast cells. Curr. Genet. 51, 245–255. doi: 10.1007/s00294-007-0123-4
Cukier, C. D., Tourdes, A., El-Mazouni, D., Guillet, V., Nomme, J., Mourey, L., et al. (2017). NMR secondary structure and interactions of recombinant human MOZART1 protein, a component of the gamma-tubulin complex. Protein Sci. 26, 2240–2248. doi: 10.1002/pro.3282
Dhani, D. K., Goult, B. T., George, G. M., Rogerson, D. T., Bitton, D. A., Miller, C. J., et al. (2013). Mzt1/Tam4, a fission yeast MOZART1 homologue, is an essential component of the gamma-tubulin complex and directly interacts with GCP3(Alp6). Mol. Biol. Cell 24, 3337–3349. doi: 10.1091/mbc.E13-05-0253
Enos, S. J., Dressler, M., Gomes, B. F., Hyman, A. A., and Woodruff, J. B. (2018). Phosphatase PP2A and microtubule-mediated pulling forces disassemble centrosomes during mitotic exit. Biol. Open 7:bio029777. doi: 10.1242/bio.029777
Eslami, H., Khorramizadeh, M. R., Pourmand, M. R., Moazeni, M., and Rezaie, S. (2014). Down-regulation of sidB gene by use of RNA interference in Aspergillus nidulans. Iran. Biomed. J. 18, 55–59. doi: 10.6091/ibj.1217.2013
Etxebeste, O., Garzia, A., Espeso, E. A., and Ugalde, U. (2010). Aspergillus nidulans asexual development: making the most of cellular modules. Trends Microbiol. 18, 569–576. doi: 10.1016/j.tim.2010.09.007
Foltman, M., and Sanchez-Diaz, A. (2017). Studying the role of the mitotic exit network in cytokinesis. Methods Mol. Biol. 1505, 245–262. doi: 10.1007/978-1-4939-6502-1_18
Gard, D. L., Affleck, D., and Error, B. M. (1995). Microtubule organization, acetylation, and nucleation in Xenopus laevis oocytes: II. A developmental transition in microtubule organization during early diplotene. Dev Biol 168, 189–201. doi: 10.1006/dbio.1995.1071
Goyal, A., and Simanis, V. (2012). Characterization of ypa1 and ypa2, the Schizosaccharomyces pombe orthologs of the peptidyl proyl isomerases that activate PP2A, reveals a role for Ypa2p in the regulation of cytokinesis. Genetics 190, 1235–1250. doi: 10.1534/genetics.111.138040
Guo, Z., and Segal, M. (2017). Analysis of the localization of MEN components by live cell imaging microscopy. Methods Mol. Biol. 1505, 151–166. doi: 10.1007/978-1-4939-6502-1_12
Gupta, S. K., Maggon, K. K., and Venkitasubramanian, T. A. (1976). Effect of zinc on adenine nucleotide pools in relation to aflatoxin biosynthesis in Aspergillus parasiticus. Appl. Environ. Microbiol. 32, 753–756
Harris, S. D., Morrell, J. L., and Hamer, J. E. (1994). Identification and characterization of Aspergillus nidulans mutants defective in cytokinesis. Genetics 136, 517–532.
Hiraga, A., and Tamura, S. (2000). Protein phosphatase 2A is associated in an inactive state with microtubules through 2A1-specific interaction with tubulin. Biochem. J. 346(Pt 2), 433–439. doi: 10.1042/bj3460433
Hutchins, J. R., Toyoda, Y., Hegemann, B., Poser, I., Hériché, J. K., Sykora, M. M., et al. (2010). Systematic analysis of human protein complexes identifies chromosome segregation proteins. Science 328, 593–599. doi: 10.1126/science.1181348
Jiang, W., and Hallberg, R. L. (2001). Correct regulation of the septation initiation network in Schizosaccharomyces pombe requires the activities of par1 and par2. Genetics 158, 1413–1429.
Johnson, A. E., McCollum, D., and Gould, K. L. (2012). Polar opposites: fine-tuning cytokinesis through SIN asymmetry. Cytoskeleton (Hoboken) 69, 686–699. doi: 10.1002/cm.21044
Käfer, E. (1977). Meiotic and mitotic recombination in Aspergillus and its chromosomal aberrations. Adv. Genet. 19, 33–131.
Kettenbach, A. N., Deng, L., Wu, Y., Baldissard, S., Adamo, M. E., Gerber, S. A., et al. (2015). Quantitative phosphoproteomics reveals pathways for coordination of cell growth and division by the conserved fission yeast kinase pom1. Mol. Cell. Proteomics 14, 1275–1287. doi: 10.1074/mcp.M114.045245
Kilmartin, J. V. (2014). Lessons from yeast: the spindle pole body and the centrosome. Philos. Trans. R. Soc. Lond,. B. Biol. Sci. 369:20130456. doi: 10.1098/rstb.2013.0456
Kim, J. M., Lu, L., Shao, R., Chin, J., and Liu, B. (2006). Isolation of mutations that bypass the requirement of the septation initiation network for septum formation and conidiation in Aspergillus nidulans. Genetics 173, 685–696. doi: 10.1534/genetics.105.054304
Krapp, A., and Simanis, V. (2008). An overview of the fission yeast septation initiation network (SIN). Biochem. Soc. Trans. 36(Pt 3), 411–415. doi: 10.1042/BST0360411
Lahoz, A., Alcaide-Gavilán, M., Daga, R. R., and Jimenez, J. (2010). Antagonistic roles of PP2A-Pab1 and Etd1 in the control of cytokinesis in fission yeast. Genetics 186, 1261–1270. doi: 10.1534/genetics.110.121368
Lengefeld, J., Hotz, M., Rollins, M., Baetz, K., and Barral, Y. (2017). Budding yeast Wee1 distinguishes spindle pole bodies to guide their pattern of age-dependent segregation. Nat. Cell Biol. 19, 941–951. doi: 10.1038/ncb3576
Liu, B., Xiang, X., and Lee, Y. R. (2003). The requirement of the LC8 dynein light chain for nuclear migration and septum positioning is temperature dependent in Aspergillus nidulans. Mol. Microbiol. 47, 291–301. doi: 10.1046/j.1365-2958.2003.03285.x
Long, N., Xu, X., Qian, H., Zhang, S., and Lu, L. (2016). A putative mitochondrial iron transporter MrsA in Aspergillus fumigatus plays important roles in azole-, oxidative stress responses and virulence. Front. Microbiol. 7:716. doi: 10.3389/fmicb.2016.00716
Magidson, V., Chang, F., and Khodjakov, A. (2006). Regulation of cytokinesis by spindle-pole bodies. Nat. Cell Biol. 8, 891–893. doi: 10.1038/ncb1449
Masuda, H., Mori, R., Yukawa, M., and Toda, T. (2013). Fission yeast MOZART1/Mzt1 is an essential gamma-tubulin complex component required for complex recruitment to the microtubule organizing center, but not its assembly. Mol. Biol. Cell 24, 2894–2906. doi: 10.1091/mbc.E13-05-0235
Misslinger, M., Gsaller, F., Hortschansky, P., Müller, C., Bracher, F., Bromley, M. J., et al. (2017). The cytochrome b5 CybE is regulated by iron availability and is crucial for azole resistance in A. fumigatus. Metallomics 9, 1655–1665. doi: 10.1039/c7mt00110j
Nayak, T., Szewczyk, E., Oakley, C. E., Osmani, A., Ukil, L., Murray, S. L., et al. (2006). A versatile and efficient gene-targeting system for Aspergillus nidulans. Genetics 172, 1557–1566. doi: 10.1534/genetics.105.052563
Ngo, T., Miao, X., Robinson, D. N., and Zhou, Q. Q. (2016). An RNA-binding protein, RNP-1, protects microtubules from nocodazole and localizes to the leading edge during cytokinesis and cell migration in Dictyostelium cells. Acta Pharmacol. Sin. 37, 1449–1457. doi: 10.1038/aps.2016.57
Nunbhakdi-Craig, V., Schuechner, S., Sontag, J. M., Montgomery, L., Pallas, D. C., Juno, C., et al. (2007). Expression of protein phosphatase 2A mutants and silencing of the regulatory B alpha subunit induce a selective loss of acetylated and detyrosinated microtubules. J. Neurochem. 101, 959–971. doi: 10.1111/j.1471-4159.2007.04503.x
Osmani, S. A., Pu, R. T., and Morris, N. R. (1988). Mitotic induction and maintenance by overexpression of a G2-specific gene that encodes a potential protein kinase. Cell 53, 237–244.
Purschwitz, J., Müller, S., Kastner, C., Schöser, M., Haas, H., Espeso, E. A., et al. (2008). Functional and physical interaction of blue- and red-light sensors in Aspergillus nidulans. Curr. Biol. 18, 255–259. doi: 10.1016/j.cub.2008.01.061
Raff, J. W., Kellogg, D. R., and Alberts, B. M. (1993). Drosophila gamma-tubulin is part of a complex containing two previously identified centrosomal MAPs. J. Cell Biol. 121, 823–835.
Ramsubramaniam, N., Harris, S. D., and Marten, M. R. (2014). The phosphoproteome of Aspergillus nidulans reveals functional association with cellular processes involved in morphology and secretion. Proteomics 14, 2454–2459. doi: 10.1002/pmic.201400063
Rankin, K. E., and Wordeman, L. (2010). Long astral microtubules uncouple mitotic spindles from the cytokinetic furrow. J. Cell Biol. 190, 35–43. doi: 10.1083/jcb.201004017
Renicke, C., Allmann, A. K., Lutz, A. P., Heimerl, T., and Taxis, C. (2017). The mitotic exit network regulates spindle pole body selection during sporulation of Saccharomyces cerevisiae. Genetics 206, 919–937. doi: 10.1534/genetics.116.194522
Robinson, D. N., and Spudich, J. A. (2004). Mechanics and regulation of cytokinesis. Curr. Opin. Cell Biol. 16, 182–188. doi: 10.1016/j.ceb.2004.02.002
Rusin, S. F., Adamo, M. E., and Kettenbach, A. N. (2017). Identification of candidate casein kinase 2 substrates in mitosis by quantitative phosphoproteomics. Front Cell Dev Biol 5:97. doi: 10.3389/fcell.2017.00097
Rüthnick, D., Neuner, A., Dietrich, F., Kirrmaier, D., Engel, U., Knop, M., et al. (2017). Characterization of spindle pole body duplication reveals a regulatory role for nuclear pore complexes. J. Cell Biol. 216, 2425–2442. doi: 10.1083/jcb.201612129
Scarfone, I., and Piatti, S. (2017). Asymmetric localization of components and regulators of the mitotic exit network at spindle pole bodies. Methods Mol. Biol. 1505, 183–193. doi: 10.1007/978-1-4939-6502-1_14
Seiler, S., and Justa-Schuch, D. (2010). Conserved components, but distinct mechanisms for the placement and assembly of the cell division machinery in unicellular and filamentous ascomycetes. Mol. Microbiol. 78, 1058–1076. doi: 10.1111/j.1365-2958.2010.07392.x
Sharpless, K. E., and Harris, S. D. (2002). Functional characterization and localization of the Aspergillus nidulans formin SEPA. Mol. Biol. Cell 13, 469–479. doi: 10.1091/mbc.01-07-0356
Simanis, V. (2015). Pombe's thirteen - control of fission yeast cell division by the septation initiation network. J. Cell Sci. 128, 1465–1474. doi: 10.1242/jcs.094821
Singh, N. S., Shao, N., McLean, J. R., Sevugan, M., Ren, L., Chew, T. G., et al. (2011). SIN-inhibitory phosphatase complex promotes Cdc11p dephosphorylation and propagates SIN asymmetry in fission yeast. Curr. Biol. 21, 1968–1978. doi: 10.1016/j.cub.2011.10.051
Son, S., and Osmani, S. A. (2009). Analysis of all protein phosphatase genes in Aspergillus nidulans identifies a new mitotic regulator, fcp1. Eukaryotic Cell 8, 573–585. doi: 10.1128/EC.00346-08
Sparks, C. A., Morphew, M., and McCollum, D. (1999). Sid2p, a spindle pole body kinase that regulates the onset of cytokinesis. J. Cell Biol. 146, 777–790.
Stearns, T., and Kirschner, M. (1994). In vitro reconstitution of centrosome assembly and function: the central role of gamma-tubulin. Cell 76, 623–637.
Suri, C., Hendrickson, T. W., Joshi, H. C., and Naik, P. K. (2014). Molecular insight into gamma-gamma tubulin lateral interactions within the gamma-tubulin ring complex (gamma-TuRC). J. Comput. Aided Mol. Des. 28, 961–972. doi: 10.1007/s10822-014-9779-2
Takeshita, N., and Fischer, R. (2011). On the role of microtubules, cell end markers, and septal microtubule organizing centres on site selection for polar growth in Aspergillus nidulans. Fungal Biol. 115, 506–517. doi: 10.1016/j.funbio.2011.02.009
Tar, K., Csortos, C., Czikora, I., Olah, G., Ma, S. F., Wadgaonkar, R., et al. (2006). Role of protein phosphatase 2A in the regulation of endothelial cell cytoskeleton structure. J. Cell. Biochem. 98, 931–953. doi: 10.1002/jcb.20829
Teixidó-Travesa N., Villén J., Lacasa, C., Bertran, M. T., Archinti, M., Gygi, S. P., et al. (2010). The gammaTuRC revisited: a comparative analysis of interphase and mitotic human gammaTuRC redefines the set of core components and identifies the novel subunit GCP8. Mol. Biol. Cell 21, 3963–3972. doi: 10.1091/mbc.E10-05-0408
Todd, R. B., Davis, M. A., and Hynes, M. J. (2007). Genetic manipulation of Aspergillus nidulans: meiotic progeny for genetic analysis and strain construction. Nat. Protoc. 2, 811–821. doi: 10.1038/nprot.2007.112
Walia, A., Nakamura, M., Moss, D., Kirik, V., Hashimoto, T., and Ehrhardt, D. W. (2014). GCP-WD mediates gamma-TuRC recruitment and the geometry of microtubule nucleation in interphase arrays of Arabidopsis. Curr. Biol. 24, 2548–2555. doi: 10.1016/j.cub.2014.09.013
Wieczorek, M., Bechstedt, S., Chaaban, S., and Brouhard, G. J. (2015). Microtubule-associated proteins control the kinetics of microtubule nucleation. Nat. Cell Biol. 17, 907–916. doi: 10.1038/ncb3188
Wolkow, T. D., Harris, S. D., and Hamer, J. E. (1996). Cytokinesis in Aspergillus nidulans is controlled by cell size, nuclear positioning and mitosis. J. Cell Sci. 109(Pt 8), 2179–2188.
Xiong, Y., and Oakley, B. R. (2009). In vivo analysis of the functions of gamma-tubulin-complex proteins. J Cell Sci. 122(Pt 22), 4218–4227. doi: 10.1242/jcs.059196
Yoon, S. Y., Choi, J. E., Choi, J. M., and Kim, D. H. (2008). Dynein cleavage and microtubule accumulation in okadaic acid-treated neurons. Neurosci. Lett. 437, 111–115. doi: 10.1016/j.neulet.2008.03.083
Yu, J. H., Hamari, Z., Han, K. H., Seo, J. A., Reyes-Domínguez, Y., and Scazzocchio, C. (2004). Double-joint PCR: a PCR-based molecular tool for gene manipulations in filamentous fungi. Fungal Genet. Biol. 41, 973–981. doi: 10.1016/j.fgb.2004.08.001
Zekert, N., Veith, D., and Fischer, R. (2010). Interaction of the Aspergillus nidulans microtubule-organizing center (MTOC) component ApsB with gamma-tubulin and evidence for a role of a subclass of peroxisomes in the formation of septal MTOCs. Eukaryotic Cell 9, 795–805. doi: 10.1128/EC.00058-10
Zhang, Y., Gao, X., Manck, R., Schmid, M., Osmani, A. H., Osmani, S. A., et al. (2017). Microtubule-organizing centers of Aspergillus nidulans are anchored at septa by a disordered protein. Mol. Microbiol. 106, 285–303. doi: 10.1111/mmi.13763
Zheng, Y., Wong, M. L., Alberts, B., and Mitchison, T. (1995). Nucleation of microtubule assembly by a gamma-tubulin-containing ring complex. Nature 378, 578–583. doi: 10.1038/378578a0
Zhong, G., Wei, W., Guan, Q., Ma, Z., Wei, H., Xu, X., et al. (2012). Phosphoribosyl pyrophosphate synthetase, as a suppressor of the sepH mutation in Aspergillus nidulans, is required for the proper timing of septation. Mol. Microbiol. 86, 894–907. doi: 10.1111/mmi.12026
Zhong, G. W., Jiang, P., Qiao, W. R., Zhang, Y. W., Wei, W. F., and Lu, L. (2014). Protein phosphatase 2A (PP2A) regulatory subunits ParA and PabA orchestrate septation and conidiation and are essential for PP2A activity in Aspergillus nidulans. Eukaryotic Cell 13, 1494–1506. doi: 10.1128/EC.00201-14
Keywords: ParA, MztA, PP2A, suppressor, septation, Aspergillus nidulans
Citation: Jiang P, Zheng S and Lu L (2018) Mitotic-Spindle Organizing Protein MztA Mediates Septation Signaling by Suppressing the Regulatory Subunit of Protein Phosphatase 2A-ParA in Aspergillus nidulans. Front. Microbiol. 9:988. doi: 10.3389/fmicb.2018.00988
Received: 06 February 2018; Accepted: 27 April 2018;
Published: 08 May 2018.
Edited by:
Hector Mora Montes, Universidad de Guanajuato, MexicoReviewed by:
Praveen Rao Juvvadi, Duke University, United StatesFrank Ebel, Ludwig-Maximilians-Universität München, Germany
Copyright © 2018 Jiang, Zheng and Lu. This is an open-access article distributed under the terms of the Creative Commons Attribution License (CC BY). The use, distribution or reproduction in other forums is permitted, provided the original author(s) and the copyright owner are credited and that the original publication in this journal is cited, in accordance with accepted academic practice. No use, distribution or reproduction is permitted which does not comply with these terms.
*Correspondence: Ling Lu, bGluZ2x1QG5qbnUuZWR1LmNu