- 1Department of Microbiology, Faculty of Science and Informatics, University of Szeged, Szeged, Hungary
- 2Department of Botany and Microbiology, College of Science, King Saud University, Riyadh, Saudi Arabia
Various Trichoderma species possess significance in agricultural systems as biofertilizers or biocontrol agents (BCAs). Besides these beneficial features, certain Trichoderma species can also act as agricultural pests, causing the green mold disease of cultivated mushrooms. This double-faced nature of the genus in agricultural environments points at the importance of proper monitoring tools, which can be used to follow the presence and performance of candidate as well as patented and/or registered biocontrol strains, to assess the possible risks arising from their application, but also to track harmful, unwanted Trichoderma species like the green molds in mushroom growing facilities. The objective of this review is to discuss the molecular tools available for the species- and strain-specific monitoring of Trichoderma, ranging from immunological approaches and fingerprinting tools to exogenous markers, specific primers used in polymerase chain reaction (PCR) as well as “omics” approaches.
Introduction
Members of the filamentous fungal genus Trichoderma commonly occur in the soil and the rhizosphere of various plants. The genus has been a popular subject of basic and applied mycology research since the 1930s (Weindling, 1932), which is mainly due to the fact that Trichoderma species play important roles in various agricultural environments ranging from field and forest soil ecosystems to substrate materials used for mushroom production. Several members of the genus have the potential to control plant pathogenic fungi and nematodes by antagonistic action based on competition, antibiosis and/or parasitism, furthermore, the biostimulant ability of certain Trichoderma species enables to enhance the uptake of nutrients by plants, promote plant growth, increase crop productivity and induce systemic resistance in plants, which can also be exploited within the frames of environmentally friendly agricultural practices (Harman et al., 2004; Nawrocka and Małolepsza, 2013; Gupta et al., 2014; López-Bucio et al., 2015; Contreras-Cornejo et al, 2016). The original view considering Trichoderma species as biocontrol agents has recently evolved to the concept that they are avirulent, endophytic plant symbionts capable of long-lasting colonization and penetration of roots and providing the plants with various beneficial effects (Harman et al., 2004; Lorito et al., 2010). However, besides the positive implications of the genus, Trichoderma species may also be harmful for agriculture, like in the case of mushroom production, where Trichoderma occurs as the causal agent of green mold disease severely affecting cultivated mushrooms (Hatvani et al., 2008, 2017; Kredics et al., 2010). Moreover, certain species of the genus are known as opportunistic human pathogens, and the causal agents of different diseases may also originate from agricultural environments (Druzhinina et al., 2008).
Ecological fitness is an important trait of any Trichoderma strain to establish in agricultural habitats like soil, plant rhizosphere or compost materials (Weaver et al., 2005; Cordier et al., 2007). The survival and spread of Trichoderma in an agricultural habitat depend on its interactions with the environmental parameters as well as the biotic and abiotic components of the local ecosystem (Kredics et al., 2003). An increased knowledge about the inoculum source, survival, spread and general population dynamics of harmful Trichoderma species in the substrates of mushroom production may enable the development of more efficient control measures to avoid green mould outbreaks in mushroom farms. On the other hand, during the development of a beneficial Trichoderma strain to a BCA, a crucial step is the collection of basic information about its abilities to colonize, persist, and spread under the conditions characteristic to the environment where it will be applied (Longa et al., 2009). Monitoring the fate, behavior and population dynamics of Trichoderma strains released as BCAs into the environment is therefore of special importance. In Europe, the registration of new, Trichoderma-based biocontrol products requires the use of specific monitoring tools capable of accurately identifying and quantifying the released strain and tracking its population dynamics over time (Savazzini et al., 2008). Therefore, it is a challenge of increasing importance to differentiate the newly introduced Trichoderma strains from the natural Trichoderma populations occurring in an agricultural habitat (Dodd et al., 2004b).
The traditional microbiological approach in Trichoderma population studies is the use of Trichoderma-selective or semi-selective agar media (Elad et al., 1981; Chung and Hoitink, 1990; Williams et al., 2003; Vargas Gil et al., 2009) for dilution plating of environmental samples, which is followed by the calculation of colony forming units (CFU). However, only the total amount of Trichoderma propagules can be assessed by this technique, it does not enable the distinction of an introduced strain from the Trichoderma populations resident in the investigated environment. Neither morphological, nor nutritional criteria are suitable markers to reliably verify the identity of the colonies (Hermosa et al., 2001), and for the large number of samples examined during population studies, a colony-by-colony barcoding based on the sequence analysis of the ITS (internal transcribed spacer) region (Druzhinina et al., 2005) or a part of the translation elongation factor 1α (tef1) gene (Kopchinskiy et al., 2005) would be extremely expensive and time-consuming. Furthermore, the quantification of more than one strain by dilution plating is limited if one of the strains grows faster and completely covers the agar plate before all colonies could be counted (Dodd et al., 2004b).
Biochemical characterization of Trichoderma strains may be applicable for species identification or studying the diversity within and between certain species of the genus. Methods of isoenzyme analysis have been succesfully applied, e.g., for the differentiation between morphologically undistinguishable strains belonging to the T. harzianum species complex (Grondona et al., 1997), or for the characterization of winter wheat rhizosphere-derived Trichoderma strains belonging to various species based on their well-defined, characteristic isoenzyme patterns (Kredics et al., 2012). Metabolic profiling by BIOLOG Phenotype MicroArray on 95 carbon sources has also been applied to differentiate among Trichoderma strains at the species level (Hoyos-Carvajal et al., 2009), furthermore, the diversity of certain taxa, in particular the T. harzianum species complex, including isolates with antagonistic potential against the plant pathogenic fungus Sclerotinia sclerotiorum, was also found to be clearly reflected in their BIOLOG metabolic profiles (Lopes et al., 2012). However, although these approaches proved to be applicable to investigate the biochemical diversity between—and in some cases also within—distinct species of the genus Trichoderma, they are not suited well enough for monitoring purposes.
Although benomyl resistant mutants were succesfully used to examine the rhizosphere competence of T. harzianum strains in an early study (Ahmad and Baker, 1987), mutants might behave differently from the wild type strain in agricultural habitats, therefore methods enabling the monitoring of the wild type strain's population dynamics would be more preferable (Green and Jensen, 1995). The availability of the highly sensitive molecular biology tools summarized below, some of them being also highly specific, may fulfill the emerging need for high-throughput, rapid, and cheap monitoring techniques to detect beneficial or harmful strains and species from the genus Trichoderma in various agricultural environments.
Immunological Approaches
Immunological assays provide a range of possibilities for studying the growth dynamics of Trichoderma species during their antagonistic interactions with their saprotrophic target fungi in complex natural environments. Immunoassays as fast and sensitive detection methods were used to precisely identify and quantify Trichoderma species in soil or other complex environments. The introduction of hybridoma technology resulted in the exploration and application of specific monoclonal antibodies (MAbs) from animals (e.g., mice) to quantify Trichoderma biomass in soil. Enzyme-linked immunosorbent assays (ELISA) or immunofluorescence was employed to detect fungi in soil. MAbs of the immunoglobulin sub-class IgG2a produced by the cell line Th.HD33 from murine splenocytes could specifically recognize an antigen, a protein epitope which is part of a glycoprotein molecule from a number of Trichoderma species. This MAbs-based immunological assay has been used for the specific identification of living T. harzianum mycelia colonizing peat-bran medium (Carter and Lynch, 1991; Thornton et al., 1994).
More importantly, immunological assays can not only discriminate between mixed populations but also between different growth states of Trichoderma species. In the study of Thornton et al. (1994), a MAb-based ELISA was used to detect the active mycelium of T. harzianum. In contrast, murine MAbs produced from a hybridoma cell line, GH5, can only recognize antigens from phialoconidia of T. harzianum and T. viride isolates but not from chlamydospores or mycelium (Thornton and Dewey, 1996). MAbs-based assays that quantify the biomass of Trichoderma strains and detect their different components can be employed in the quantitative analysis of population dynamics of BCAs. MAbs can also be used to detect and quantify the growth of soil borne pathogens, such as Rhizoctonia solani and their growth dynamics under the influence of the hyperparasitic T. harzianum artificially introduced into composts (Thornton et al., 1999). The high level of specificity of the monoclonal antibody MF2 (raised against the constitutive glucoamylase enzyme) as a molecular marker for the recovery and detection of Trichoderma species has been demonstrated in the study of Thornton et al. (2002). MF2 specific for Trichoderma species and a group of their close relatives prepared from a commercial β-1,3-glucanase allowed the fast detection and visualization of Trichoderma species in soil or other complex matrices containing mixed fungal populations. The examined Trichoderma species have been shown to greatly inhibit the saprotrophic growth of R. solani in composts. By immunofluorescence micoscopy using MF2, Trichoderma isolate S-B2 was shown to grow toward and coil around the hyphae of the host in dual cultures of Trichoderma and Rhizoctonia (Thornton et al., 2002).
Immunological methods are also able to distinguish between rapid mycelial growth of Trichoderma and other components such as conidia and spores. In the study of Thornton (2004), the Trichoderma-specific monoclonal antibody MF2 was used to quantitatively assess the population dynamics of BCAs from the species T. harzianum and T. asperellum in antagonistic interaction with the pathogen R. solani in microcosm studies. A R. solani-specific monoclonal antibody (EH2) recognized an extracellular glycoprotein secreted from the growing tip of Rhizoctonia mycelia. Standard curves were constructed from extracts of lyophilized mycelium containing quantifiable and repeatable sources of the antigens. By using these curves, lyophilized mycelial biomass could be calculated, which allowed to comparatively estimate the mycelial growth of Trichoderma species and R. solani. Trichoderma species were able to prevent R. solani growth by competing with the pathogen for nutrients (Thornton, 2004).
The above studies indicate that the development of immunological assays allows the species-level detection and identification of Trichoderma strains, however, a shortage of these techniques is that serology is not specific at the isolate level.
Introduction of Exogenous Marker Genes
Genetic engineering of BCAs with exogenous markers, e.g., the green fluorescent protein (gfp) (Figure 1), hygromycin B phosphotransferase (hygB), and/or β-glucuronidase (GUS) encoding genes is a powerful strategy to detect and monitor individual Trichoderma strains in agricultural habitats (Green and Jensen, 1995; Lo et al., 1998; Bae and Knudsen, 2000).
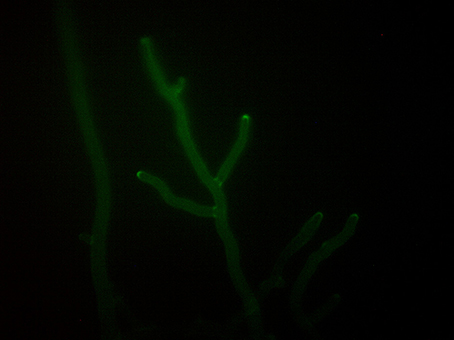
Figure 1. Hyphae of Trichoderma pleuroti expressing the green fluorescent protein (photo: Lóránt Hatvani).
Trichoderma harzianum TF3, a propiconazole-resistant strain with the ability of survival at relatively high densities in the phylloplane of tomato and grapevine and high antagonistic potential against Botrytis cinerea on these crops was transformed to hygromycin B resistance by high-voltage electric pulse with the vector pHATα (a derivative of pAN7-1) containing the hygromycin B phosphotransferase (hph) gene of E. coli, which confers hygromycin B resistance as a selectable marker (Migheli et al., 1994). All transformants showed higher survival rates on tomato phylloplane than the wild type strain, all of them being mitotically stable after several passages in the absence of selective pressure and on tomato plants during 2 weeks, without losing the ability to grow in the presence of both hygromycin B and propiconazole. Thrane et al. (1995) used the polyethylen-glycol method to transform the same T. harzianum strain with both pNOM102 and pAN7-l carrying the Escherichia coli β-glucuronidase (GUS) and hygB resistance genes, respectively. The resulting transformants could be visualized under an epifluorescence microscope. Three methods were used to assay the GUS activity with 5-bromo-4-chloro-3-indolyl-β-D-glucuronide: the transformant strains were tested in a microtiter plate by incubating fresh mycelium, non-sporulating transformants were assayed for GUS activity on plates by wounding the mycelium, or the GUS activities of mycelial extracts were measured. Three of the transformants, T. harzianum T3a, T3b, and T3c proved to be mitotically stable for 8 months with respect to both genes. Green and Jensen (1995) studied the population activity and stability of the GUS-transformed T. harzianum strain T3a in the rhizosphere of sphagnum peat-grown cucumber plants. This was the first study using the GUS marker to monitor the presence, population dynamics and activities of a T. harzianum strain introduced into the rhizosphere. The authors proved the genetic stability of the transformant during its growth in a natural potting mixture, and observed a high GUS-production in conidia preparing for germination as well as during subsequent mycelial growth. The results demonstrate the applicability of a GUS-transformed strain to detect the presence and follow the population dynamics and activities of a specific T. harzianum strain in the environment. Bowen et al. (1996) transformed another T. harzianum strain, M1057, shown to be effective against Sclerotium rolfsii, with the plasmids pAN7-l and pNOM102. Two transformants proved to be mitotically stable and their growth rates were not different from the wild type. In order to evaluate the effect of transformation on biocontrol ability, the parental strain and the selected transformants were compared for their abilities to protect lentil (Lens culinaris) from the parasitic attack of S. rolfsii. Both the wild type and the transformant T. harzianum strains could rapidly colonize the soil with fast growth at the surface and inhibit S. rolfsii growth (Bowen et al., 1996).
To monitor the hyphal growth, activities and survival of a T. harzianum strain, Bae and Knudsen (2000) transformed strain ThzID1 with plasmids carrying the gfp (pTEFEGFP), GUS (pNOM102), and hygB (pAN7-2) genes. The mitotic stability of the cotransformants and their capacity to colonize the resting sclerotia of the plant pathogen Sclerotinia sclerotiorum in soil were investigated. The cotransformant ThzID1-M3 proved to be the only strain showing stable expression of all three introduced exogenous marker genes. In order to confirm the genomic integration of the introduced plasmid DNAs, the total genomic DNAs of ThzID1-M3 and the parental strain ThzID1 were tested by Southern hybridization with a pTEFEGFP fragment (HindIII-BamHI), a pNOM102 fragment (NcoI-NcoI), and a pAN7-2 fragment (HindIII-EcoRI) to confirm the genomic integration of the introduced marker genes. According to the results of this study, cotransformation with GUS and gfp may provide a useful tool to detect and monitor specific T. harzianum strains released into the soil. ThzID1-M3 was the most succesful, colonizing ~60% of the sclerotia. A subsequent study examined, whether Aphelenchoides sp. (a fungivorous nematode) affects the growth and proliferation of ThzID1-M3 in soil (Bae and Knudsen, 2001). Soil treatment with a ThzID1-M3 formulation was found to stimulate the growth of fungivorous and other nematode populations, and a fungivorous nematode population could significantly reduce the colonization of S. sclerotiorum sclerotia by ThzID1-M3 in soil. Fungivorous nematodes migrate to soil locations with large quantities of available nutrients. Based on the results of the study, the Trichoderma strain introduced into the soil as a pellet formulation formed a hyphal net of high-density, which may be able to attract nematodes by serving as an appropriate nutrition source. Orr and Knudsen (2004) used the GUS- and gfp-labeled T. harzianum strain ThzID1-M3 to quantitatively measure the biomass changes of a fungal BCA in nonsterile soil, where the populations of indigenous bacteria and fungi interfere with traditional biomass determination procedures. The marker genes GUS and gfp allowed differentiation between the introduced BCA and the indigenous Trichoderma populations, and demonstrated that the monitoring of gfp-labeled T. harzianum by epifluorescence microscopy was a useful method for distinguishing active hyphal biomass functional for biological control from inactive chlamydospores and conidia enumerated by plate counts. Kim and Knudsen (2008) developed a set of specific PCR primers for ThzID1-M3 with high reproducibility and precision. Methods applying real-time PCR and epifluorescence microscopy-based image analysis were subsequently refined and evaluated in order to quantify the colonization dynamics of S. sclerotiorum sclerotia by ThzID1-M3 (Kim and Knudsen, 2011). In a recent study, Contina et al. (2017) hypothesized that the use of T. harzianum ThzID1-M3 would allow direct observation of the biocontrol mechanisms employed by strain ThzID1-M3 to reduce infection and reproduction of the potato cyst nematode Globodera pallida. Strain ThzID1-M3 proved to be useful to monitor the biocontrol interactions between T. harzianum and the nematode: the BCA significantly reduced G. pallida infection and reproduction in potato roots.
Lu et al. (2004) introduced the gfp gene into T. atroviride strain P1, which is able to suppress diseases caused by Pythium ultimum and R. solani. The authors proved that transformation with the gfp gene had no affect on the biocontrol activity of the T. atroviride P1 strain. Kowsari et al. (2014) transformed the T. harzianum strain ABRIICC T8-7MK, resulting from protoplast fusion, with the amdS and gfp genes, and selected a cotransformant which was subsequently tested against R. solani. The cotransformant was able to colonize potato tubers affected by black scurf and planted into agricultural soil treated with the Trichoderma strain. After 20 days of incubation, Trichoderma was found to completely colonize R. solani sclerotia and produce conidia totally covering the black scurf.
Although green fluorescent protein, hygromycin B resistance or GUS activity enable the development of simple tools for the monitoring of biocontrol strains (Bowen et al., 1996), the application of organisms genetically modified with exogenous markers as BCAs is a very tedious alternative due to the public concern about the release of GMOs into natural environments, which also needs specific permission in several countries including the European Union (Dodd et al., 2004b). Therefore, there is a constant need for the development of molecular tools based on endogenous DNA markers enabling the specific monitoring of distinct, genetically unmodified biocontrol Trichoderma strains in agricultural environments.
Fingerprinting Techniques
In general sense, fingerprinting techniques are DNA-based methods relying on PCR, digestion with restriction enzymes and/or Southern hybridization, generating banding patterns that can be compared with each other and allow the identification of characteristic, strain-specific DNA fragments.
The specific tool known as DNA fingerprinting was used by Schlick et al. (1994) to prove that different patented strains of T. harzianum and their gamma-ray induced mutants can be distinguished by using oligonucleotides (CAC)5, (GTG)5, GGCATCGGCC, and M13 sequence GAGGGTGGCGGTTCT as PCR primers and the wild-type M13 phage oligonucleotides (GTG)5 and (CT)8 as hybridization probes. The authors investigated T. reesei and strains of T. harzianum including LC-1 (a biocontrol strain), as well as LC-2 and LC-3 (its two irradiation mutant derivatives). As a result, discriminative fingerprint patterns were obtained, and all examined strains could be succesfully distinguished from each other.
The first technique that proved to be capable of distinguishing a commercially used biocontrol strain, T. harzianum T-39 used as a BCA against B. cinerea, from other Trichoderma strains was designed by Zimand et al. (1994). All examined Trichoderma strains could be distinguished based on their unique banding profiles generated by a set of 10 mer primers used in a random amplified polymorphic DNA (RAPD) procedure. This technique has some advantages when compared to morphology-based methods: it is less time-consuming and can be conducted using small amounts of DNA.
Instead of random sequences, Arisan-Atac et al. (1995) used wild-type M13 phage DNA GAGGTGGNGGNTCT, (GACA)4 and (GTG)5–previously used for RFLP fingerprinting—for the PCR generation of banding patterns, which was named RAPD fingerprinting. The authors analyzed 11 strains of T. viride, 2 strains of its teleomorph Hypocrea rufa and 9 isolates representing further Trichoderma species (T. citrinoviride, T. parceramosum, T. harzianum, T. koningii, T. longibrachiatum, T. piluliferum, T. polysporum, T. reesei, T. saturnisporum) which were screened for their antagonistic activities against Cryphonectria parasitica. The results showed that all three primers amplified 12–15 fragments characteristic to each strain.
To successfully differentiate the commercially used T. harzianum strain C65—a strain with biocontrol activity against B. cinerea causing kiwifruit stem-end rot—from other closely related Trichoderma species including isolates of T. koningii, T. viride, T. reesei, T. atroviride, and T. aureoviride. Bowen et al. (1996) designed a restriction fragment length polymorphism (RFLP) assay using the HindIII enzyme. Southern blot hybridisation probes for the RFLP analysis were generated by RAPD using the DNA of T. harzianum C65 as template. The method was capable to detect the BCA in the commonly isolated mycota. To facilitate the screening of a large number of samples, Dodd et al. (2004a) combined this isolate-specific RFLP marker with a dot-blot assay, which was used to monitor the spread and survival of T. atroviride C65 on leaves of kiwifruit both in shadehouse and in the orchard over two subsequent seasons. By this procedure, the authors have successfully proved that strain C65 is an ideal BCA, as it did not only survive but also spread to uninoculated trees and fruits, protecting them from post-harvest fruit rot.
Bulat et al. (1998) evaluated the ability of universally primed (UP) PCR and ITS-ribotyping to discriminate closely related Trichoderma and Gliocladium strains and to identify markers for fast and reliable detection assays. Several isolates of T. harzianum, T. hamatum, T. koningii, T. polysporum, T. virens, T. viride, and G. roseum were tested in a cross dot-blot hybridisation carried out using UP-PCR amplification products, and similarity among the strains was determined. The results suggested that the combination of UP-PCR and ITS-ribotyping techniques may become a valuable tool for intra-species distinction.
Forty-two isolates of T. harzianum and T. viride from Phillippine rice fields were analyzed by Cumagun et al. (2000) and characterized using rDNA-ITS1 analysis and UP-PCR with the 16–21 mer primers L45, 3-2, AA2M2, AS4, AS15, AS15inv, L21, Fok1, L15/AS19, and 0.3-1. Strains were characterized to the species level based on UP-PCR banding profiles and the length and Sau3A restriction patterns of the ribosomal DNA. The authors have concluded that UP-PCR combined with the analysis of rDNA-ITS1 is a useful tool for the identification of isolates that are indistinguishable on the basis of their morphology. The combination of UP-primers L21 and Fok1 could distinguish the promising biocontrol strain T. harzianum 94-016 from other Trichoderma isolates, therefore having the potential to be used for monitoring purposes.
Lübeck and Jensen (2002) combined dilution plating and UP-PCR with the above listed set of primers for monitoring the commercial biocontrol Trichoderma strains T. harzianum KRL-AG2 (Bio-Trek 22G), Supresivit (Supresivit), T39 (TRICHODEX), ATCC20476 (Binab T, type 1) and Promot 1 (Promot), T. polysporum ATCC20475 (Binab T, type 2), and T. koningii Promot 2 (Promot). Several primers generated characteristic banding patterns for each strain, allowing the commercially used strains to be differentiated from others. The results have shown that this method is suitable for monitoring the species composition in different glasshouses. The spread of the biocontrol strains could also be monitored, and the necessity of reintroduction could be easily detected.
Freeman et al. (2002) conducted arbitrarily primed PCR (ap-PCR) in an experiment to assess the use of the strains T. harzianum T39 (TRICHODEX) as well as T161 and T166 for controlling B. cinerea and Colletotrichum acutatum in strawberry, and to investigate the population survival of these 3 T. harzianum strains as well as T. asperellum T-105 on strawberry leaves. The primers (GACAC)3, (GACA)4, (TGTC)4, and (CAG)5 used for ap-PCR were derived from microsatellite or repeat sequences. The PCR banding patterns generated by ap-PCR proved to be suitable for grouping the isolates at the species level.
Fanti et al. (2003) evaluated fingerprinting with random, minisatellite and microsatellite primers for the environmental monitoring of two promising biocontrol Trichoderma strains effective against Cytospora canker of peach and found that the M13 minisatellite revealed unique fingerprints for both strains. Four months after the application to the soil under the canopy of peach trees, the antagonists could be recovered from above plant parts but not from soil, while after 1 year they were lost.
Naef et al. (2006) used a microsatellite-based method to determine the fungal biomass in decomposing plant material. PCR was carried out with fluorescent primers, which co-amplify fragments of different length from the reference and target strains. The primer pair MS-Ta4 generated a 132 or 142 bp long fragment from the investigated Trichoderma strains. T. atroviride P1 and T. harzianum RAC616 possess the alleles of 132 bp (13 TG repeats) and 142 bp (18 TG repeats), respectively. The fragments were separated by a capillary sequencer with fluorescent detection and the biomass of the fungi could be calculated by target/reference ratio of the fluorescent signal.
The RAPD-PCR method combined with benomyl resistance improved the identification of biocontrol strains from field samples. Tachapattaworakul et al. (2008) used this technique for monitoring of T. atroviride strains CBS 470.94, CBS 350.93, L2, and OB. The RAPD procedure with primer OPC8 generated a fragment of 3,000 bp specific to the biocontrol strain L2. This strain showed tenfold level of resistance to benomyl when compared to the other strains examined, which made its isolation from soil easier. The applied method allowed the recovery and identification of strain L2 from the soil 4 months after its inoculation.
Although the PCR-based fingerprinting tools represent simple and flexible methods, they are not always strain-specific and their reproducibility may be affected by the low annealing temperature and short primer size (Hermosa et al., 2001). However, strain-specific fragments generated by such techniques have the potential to be converted into monitoring markers based on SCARs (sequence characterized amplified regions) (Paran and Michelmore, 1993).
Species-Specific PCR
The use of specific primers is a powerful tool for distinguishing individual Trichoderma species in agricultural environments. Chen et al. (1999) developed a species-specific primer-based test to identify T. harzianum biotypes Th2 and Th4 (redescribed later as T. aggressivum f. europaeum and T. aggressivum f. aggressivum, Samuels et al., 2002), causing green mold diseases on Agaricus bisporus crops in Europe and North America, respectively. The specific primers were designed from the sequence of a 1,150 bp RAPD-PCR product generated by primer 232, which proved to be unique to T. aggressivum. The primer pair Th-F/Th-R (Table 1) gave no product from the DNA samples of T. harzianum, several biocontrol Trichoderma strains, or from 31 other genera and species of fungi. The method can be used efficiently for the detection of T. aggressivum in green mold-affected mushroom compost. Kredics et al. (2009) described a multiplex PCR assay allowing the detection of T. pleuroticola and T. pleuroti (formerly T. pleurotum) (Park et al., 2006), the green mold pathogens of oyster mushroom. Primers FPforw1, FPrev1, and PSrev1 (Table 1) were designed based on DNA sequences located within the 4th and 5th introns of the translation elongation factor 1 α (tef1) gene. By the use of the three-primer set in a multiplex PCR, a 447 bp fragment is amplified from both T. pleuroti and T. pleuroticola, while in the case of T. pleuroti an additional, 218 bp specific product is also formed. The primers showed no cross reaction with other Trichoderma species or species from other fungal genera. The technique proved to be suitable for the detection of T. pleuroti and T. pleuroticola directly from green mold-affected Pleurotus substrate without the need of strain isolation, furthermore, it could also be used to demonstrate the presence of T. pleuroticola in the growing substrates and on the surface of the fruiting bodies of wild oyster mushroom.
Meena (2009) described a technique for the detecion of the species T. harzianum and T. hamatum, known as potential biocontrol agents of different plant pathogenic fungi. The author designed SCAR primers (Table 1) based on the sequence of species-specific RAPD fragments. A similar method was developed by Pérez et al. (2014) (Table 1), which enables monitoring the growth and colonization of T. cf. harzianum in experimental communities, however, due to the cosmopolitan and diverse nature of the species the authors did not suggest the use of this tool for assessing the presence of T. cf. harzianum in complex environmental samples such as soil.
In order to monitor and quantify T. harzianum, López-Mondéjar et al. (2010) designed a primer set and a TaqMan probe for the ITS region (Table 1). The quantity of ITS copies was calculated based on real-time PCR results and a correlation of 0.76 was obtained between ITS copy number and the fungal biomass determined by optical microscopy and image analysis. The authors suggest that quantification of fungi in soil samples can be performed precisely using real-time PCR data. The primers and the probe were designed for pure cultures of T. harzianum, however, they might be applied for the detection and quantification of the fungus in soils and other organic materials. This method was successfully used by Beaulieu et al. (2011) to monitor the population of T. harzianum in peat and green compost.
Geistlinger et al. (2015) introduced a tool based on the use of simple sequence repeats (SSRs) using Touchdown PCR to track and quantify the species T. virens. Primers were designed for 12 different loci. The results confirmed the root-endophytic lifestyle of this species in tomato plants: the fungal biomass in the plant tissues was quantified and co-colonization of the roots by different strains of T. virens could also be observed.
Skoneczny et al. (2015) examined the genetic diversity of environmental T. atroviride isolates using cleaved amplified polymorphic sequence (CAPS) markers. Following the amplification of RAPD regions by PCR primers (Table 1), the obtained amplicons were digested with defined restriction enzymes (BslI, DraI, TaqI). Three CAPS markers were found to distinguish T. atroviride from other species; therefore they have the potential to be used for the identification and monitoring of T. atroviride, particularly in environmental specimens.
Recently, Oskiera et al. (2017) described a multiplex PCR assay for monitoring the population levels of T. atroviride and T. harzianum applied as biopreparations in the field. For the detection in soil, a maximum of 3 primer pairs targeting markers TaQ (Q01_3F/Q01_3R) or TaX (X18_1F/X18_3R) (Table 1), the Trichoderma chitinase-specific chit42_1af/chit42_2ar (Kullnig-Gradinger et al., 2002) and ITS primers (5.8SR/LR6) specific to fungi were combined for the detection of T. atroviride, while two markers, TVD (TVD_If/TVD_Ir) (Bhat, 2007) and ThQ (QTh_5F/QTh_4R) (Table 1) were combined in multiplex-PCR for T. harzianum detection. Primers targeting the β-tubulin gene of Trichoderma spp. (β-tub-F, β-tub-R) (Chen et al., 1999) served as a control. The results of the study confirmed the increased presence of T. atroviride and T. harzianum in the soil even 2 years after the application of biopreparations, while these species were not found in untreated soil samples (Oskiera et al., 2017).
Design of Strain-Specific PCR Primers
Although the above mentioned species- and genus-specific detection tools are very valuable for studying the population dynamics or epidemiology of certain Trichoderma species, due to the lack of strain specificity they are not the most reliable options for the environmental monitoring of particular biocontrol strains.
Nucleic acid-based endogenous markers are unique DNA sequences distinguishing a particular strain from all others. Such markers have the potential to be exploited in highly specific and sensitivite PCR-based assays. SCARs based on strain-specific fragments generated by fingerprinting methods allow the development of conventional and real-time PCR-based strategies for the differentiation of a particular biocontrol Trichoderma strain from other strains of Trichoderma present in the field environment, even from those belonging to the same species.
The first strain-specific SCAR marker-based tool of Trichoderma monitoring was developed by Abbasi et al (1999) for the precise detection and monitoring of T. hamatum strain 382, a BCA effective in compost-amended substrates. The authors performed RAPD analysis and identified 3 diagnostic fragments of 0.35, 0.6, and 0.65 kb in the patterns generated by primers OPE-16, OPH-19, and OPH20, respectively. The fragments were cloned into vector, sequenced, and primer pairs were designed for each of them (Table 2). The resulting 3 SCAR primer-pairs could be successfully used to indicate the presence of T. hamatum 382 in 9 different compost, soil and potting mix samples fortified with the BCA, while 274 Trichoderma isolates deriving from 9 non-fortified samples gave negative results. The method proved to be applicable also for the detection of the SCAR fragments from crude DNA preparations extracted directly from compost-amended substrates.
The same strategy was applied by Hermosa et al. (2001), who aimed to develop a PCR technique for the monitoring of the patented T. atroviride 11 strain showing biocontrol activity against plant pathogenic fungi including Acremonium cucurbitacearum, Aphanomyces cochlioides, Phoma betae, and R. solani. RAPD analysis with primer OPF10 revealed two fragments (11-A1: 996 bp, 11-A2: 361 bp) from the DNA of T. atroviride 11, which were absent from the closely related T. atroviride 260 strain. The fragments were cloned, sequenced and subjected to nucleotide and protein BLAST analysis, which revealed no significant homology. Although primers 11-A1 and 11-A1c (Table 2) designed based on the sequence of the SCAR 11-A1 fragment proved to distinguish strain T. atroviride 11 from 26 other Trichoderma strains belonging to 7 species (Hermosa et al., 2001), later it turned out that they were not strain-specific as they also yielded the PCR product from 5 of the 9 T. atroviride strains examined by Cordier et al. (2007). In a subsequent study (Rubio et al., 2005), the same primer pair was tested against the DNA of further 27 Trichoderma strains, and it was also found to amplify a larger, 1.5 kb fragment from T. harzianum strain 2413. This fragment was cloned, and the primers BR1 and BR2 (Table 2), amplifying a 837 bp fragment from T. harzianum 2413 were designed based on the sequence information of the SCAR-fragment. The primers were tested on the DNA from 27 Trichoderma strains and 22 untreated soils, and proved to be specific to T. harzianum 2413. In addition, primers Q2413f and Q2413r (Table 2) along with a TaqMan fluorogenic probe were also designed based on the sequence of the 837 bp SCAR marker, in order to improve the sensitivity of the monitoring tool by the application of real-time PCR. The developed assay was able to quantify the DNA of the BCA from sterile soil fortified with 108 conidia/g of soil but failed to detect the strain from a Trichoderma mixture introduced into the soil (Rubio et al., 2005). In a similar study, Cordier et al. (2007) developed a SCAR-based real-time PCR to specifically identify and study the population dynamics of T. atroviride strain T1. The authors of this study also applied the primers 11-A1 and 11-A1c to generate a fragment from strain T1, which was converted to a SCAR marker by sequence analysis. Among the 3-primer sets designed for the SCAR fragment, the primer pair PF74/PR88 (Table 2) generated the expected amplicon of 141 bp only from strains T1 and 1571. RAPD and AFLP (amplified fragment length polymorphism) fingerprints have shown that these two strains are identical for all the molecular characters tested and are therefore probably the parallel deposits of the same isolate. The same primer pair was used to develop a real-time PCR assay with SYBR Green detection, which proved to be applicable for monitoring the population dynamics of strain T1 in complex soil environments: detection of the BCA was possible at levels of 103 CFU/g of nonsterile soil containing autochthonous Trichoderma populations (Cordier et al., 2007).
Dodd et al. (2004b) also used RAPD, and with primer OPAW04 they could identify a fragment unique to T. virens GV4, a strain with biocontrol abilities against both the vegetable pathogen S. sclerotiorum and the onion white rot pathogen Sclerotium cepivorum. Among the primers designed based on the sequence of the fragment, the primer pair F116/R331 (Table 2) gave the most consistent results during tests with further 5 T. virens isolates. The test was developed further to a duplex PCR system by including ITS4 and ITS5 (White et al., 1990), two universal primers targeting the ITS region as positive control to detect amplifiable fungal DNA, and was able to detect strain GV4 down to a concentration of 10 spores per gram nonsterile agricultural soil (Dodd et al., 2004b).
To quantify the presence of the biocontrol strains Hypocrea parapilulifera Tp039 and T. atroviride Ta040, Feng et al. (2011) developed primer pairs for strain-specific SCAR markers identified by RAPD primers OPX02 for Tp039 and OPX13 for Ta040. The primer pairs TpX021F/TpX021R and TaX131R/TaX131R amplified a 637 and a 2,029 bp fragment from strains Tp039 and Ta040, respectively (Table 2). Additional primers replacing the original forward or reverse primer were also designed. The TpX021F2/TpX021R combination amplifying a 160 bp fragment and the TaX131F/TaX131R2 combination forming a 157 bp fragment, discriminated Ta040 and Tp09 from all other strains tested. Fluorophore-labeled probes distinguishing the two strains were also designed and succesfully used in combination with the SCAR primers in multiplex real-time PCR for the examination of artificially inoculated soils as well as samples derived from a golf green which has been treated with a product containing both strains. The results revealed that the two biocontrol strains were not able to establish on the golf green and its surrounding area (Feng et al., 2011).
Instead of RAPD, Naeimi et al. (2011) performed UP-PCR with 5 universal primers both separately, and in pairwise combinations in order to search for endogenous markers specific to T. harzianum AS12-2, a strain with the potential to control the sheath blight disease of rice caused by R. solani. UP primers are primarily targeting more variable intergenic regions of the genome, therefore, this technique is especially useful in detecting intraspecific variations (Bulat et al., 1998). The application of UP primers AS15inv, AA2M2, as well as their pairwise combinations resulted in the amplification of unique fragments (905, 684, and 842 bp, respectively) from the genomic DNA of the T. harzianum AS12-2 strain. The sequences of the cloned fragments were used to design 14 oligonucleotide primers. Two primer pairs, 15invFL1/15invRL1 and 15invF2/15invRL1 (Table 2), designed from the sequence of the 905 bp SCAR marker, amplified fragments of the expected size (796 and 627 bp, respectively) from the DNA of T. harzianum AS12-2, but not from the DNA of further 111 T. harzianum isolates tested or of 84 further strains belonging to 5 other Trichoderma species occurring in rice fields (Naeimi et al., 2011).
Specific PCR markers for the monitoring of particular strains of a species can also be developed from sequences with known functions. In genetically modified strains, the sequences of the introduced genes may serve as targets of primer development. Baek and Kenerley (1998) developed a quantitative PCR method for the detection and quantitation of the T. virens strain GvT6, which carries the bacterial organophosphate degrading (opd) gene, therefore being potentially applicable for the bioremediation of soils contaminated with organophosphate pesticides. The assay based on the amplification of an opd fragment with opd-specific primers can be quantified by the application of a DNA standard containing the 1.5 kb gpd gene with 20 bp opd primer-binding sequences at both ends. The detection limit of the PCR assay from soil samples was 10–1,000 times lower than that of traditional dilution plating (Baek and Kenerley, 1998). The same Q-PCR method was used by Weaver et al. (2005) for a genetically modified derivative of T. virens Gv29-8, constructed by transformation with plasmid pCL1 carrying the hygB and the opd genes. The authors evaluated the genetic stability and ecological persistence of the strain over 243 days in soil mesocosms and found that both the genetically modified and the wild type strain were able to rapidly and efficiently colonize freshly added substrates even after the long-term incubation, indicating a high level of responsiveness (Weaver et al., 2005). A similar monitoring tool has been developed for studying the environmental fate of T. reesei strain QM6A, an efficient cellulase producer of biotechnological significance (Providenti et al., 2004). The strain was transformed with the hygromycin B resistance gene and specifically monitored in intact soil-core microcosms with primers targeting the introduced marker. Results of this study indicated that T. reesei may persist for at least 2 seasons in soil followed by its release from fermentation plants as spent biomass.
The increasing availability of Trichoderma sequences with known functions allows the identification of single-nucleotide polymorphisms applicable for the design of strain-specific monitoring tools. Savazzini et al. (2008) used the ech42 endochitinase gene sequence to monitor T. atroviride strain SC1, a BCA against root rot pathogens, primarily Armillaria species in soils of orchards and vineyards, for which the development of RAPD-based SCAR markers was unsuccessful. The authors amplified the target gene from the BCA with primers Ech42 Fw and Ech42 Rv (Table 2), and compared its sequence with 34 other ech42 sequences, which was followed by the design of the TaqMan probe Ech42 P (Table 2) targeting 2 nucleotide mutations within the first intron of the ech42 gene of strain SC1. A second primer set and TaqMan probe to a common sequence of tga3, a gene encoding the G protein α subunit, were also designed to verify the presence of Trichoderma DNA in specificity tests and microcosm experiments. The detection and quantification limits of the resulting low throughput time, real-time PCR procedure were 6,000 and 20,000 haploid genome copies per gram of soil, suggesting that the method could be useful to monitor the fate of T. atroviride SC1 applied as an open-field BCA (Savazzini et al., 2008). This monitoring tool was incorporated into an intact soil microcosm technique developed to evaluate both the survival and the vertical dispersal of the SC1 strain (Longa and Pertot, 2009). Another study applying this monitoring tool revealed that strain SC1 tolerated both low temperature values during winter and fluctuations of humidity in soil after applications in a vineyard, could move beyond the treated soil area, migrated passively to the lower leaves of grapevine and became an integral part of the local microbiota (Longa et al., 2009).
Horn et al. (2016) performed genome mining to identify putative, new regions enabling the differentiation of various T. harzianum strains, and subsequently developed a multiplex Q-PCR assay for the monitoring of T. harzianum strain T22, an efficient BCA against Calonectria pauciramosa. The region upstream the coding part of the L-amino acid oxidase gene aox1 proved to have sufficient genetic variation to discriminate between different strains of T. harzianum including T22, therefore a primer pair was designed for this region along with TaqMan probes specific to particular T. harzianum strains including T22 (Table 2). Strain T22 could be successfully monitored by the developed Q-PCR assay on the roots of greenhouse-cultivated tomato plants inoculated with the BCA (Horn et al., 2016).
The main problem during the use of DNA-based, strain-specific monitoring tools for the direct tracking of Trichoderma strains in agricultural environments is the difficulty in reproducing the results, which may be due to the presence of PCR inhibitors, such as humic acids and tannins—co-extracted with nucleic acids in the case of soil environments—and the presence of large amounts of DNA from other microorganisms in the examined habitats (Dodd et al., 2004b; Cordier et al., 2007). The inhibition of PCR in combination with the varying efficiency of DNA extraction between conidia and mycelia (Fredricks et al., 2005) may lead to an underestimation (Cordier et al., 2007), while the lack of discrimination between DNA from living and dead cells may result in an overestimation of the populations present (Pujol et al., 2006). It cannot be excluded either that a SCAR marker may be lost during field trials, however, this problem can be circumvented by the development and application of several independent strain-specific markers for the monitoring of a single strain (Hermosa et al., 2001).
“OMICS” Approaches in the Service of Trichoderma Monitoring
Since the release of the first complete Trichoderma genome sequence in 2008 belonging to the industrially important cellulase producer T. reesei (Martinez et al., 2008) and its subsequent comparative analysis with the genomes of the biocontrol species T. virens and T. atroviride (Kubicek et al., 2011; Schmoll et al, 2016), the number of full genome sequences publicly available in databases for Trichoderma strains is growing rapidly (Baroncelli et al., 2015, 2016; Kuo et al., 2015; Shi-Kunne et al., 2015; Compant et al., 2017), which opens the way for using comparative genome analysis to identify specific endogenous markers. An example is the study of Mahfooz et al. (2016), who performed a comparative in silico analysis of SSR sequences in the genomes of T. reesei and three biocontrol species of the genus, and developed 6-6 primer pairs for the amplification of polymorphic markers from T. atroviride and T. harzianum. In another study, Lange et al. (2016) compared the genome sequence of the remarkably effective biocontrol agent T. cf. atroviride strain LU132 with that of the less efficient strain T. cf. atroviride LU140 and identified a single functional nucleotide polymorphism (SNP), which proved useful for the development of an RFLP technique applicable for the differentiation of the two strains from each other.
The approach of full metagenome sequencing in the case of samples from agricultural habitats has the potential to reveal important information not just about Trichoderma but the entire microbiome, however, it requires sequencing platforms providing a very high coverage as well as substantial bioinformatics capacities. An alternate approach is metabarcoding, which may also provide valuable tools for the investigation of Trichoderma populations in agricultural and natural soil systems. Hagn et al. (2007) developed a set of primers targeting the ITS region for the cultivation independent detection and monitoring of Trichoderma species. The analysis of a clone library from PCR products amplified from arable soil revealed that Trichoderma clades comprising many species of biocontrol relevance were covered by amplification with the designed primer set. For Trichoderma diversity analysis, Meincke et al. (2010) developed another Trichoderma-specific primer pair targeting the ITS region and a semi-nested PCR to amplify fragments suitable for denaturing gradient gel electrophoresis (DGGE), which was tested on Trichoderma communities in the rhizosphere of various potato genotypes. However, the reverse primer used in this study binds to a still polymorphic region of ITS2, making several species undetectable, therefore Friedl and Druzhinina (2012) worked out an ITS-based metabarcoding approach based on six reverse primers used in combination with the forward primer ITS5, that amplify the entire diagnostic ITS1 and 2 region from all representatives of the genus. This culture independent PCR-based tool was succesfully used to reveal the restricted diversity of the genus Trichoderma in temperate soil of a riparian forest.
While PCR methods targeting genomic DNA amplify products from both active (e.g., growing hyphae) and inactive fungal elements (e.g., dead mycelia and dormant conidia), which may result in the overestimation of Trichoderma populations, transcriptomic approaches by the isolation of total RNA, its reverse transcription and the subsequent detection of cDNA have the advantage of allowing the detection of active Trichoderma populations. The previously mentioned method of López-Mondéjar et al. (2010) detecting genomic ITS sequences was further developed to a quantitative reverse transcribed PCR (qRT-PCR) tool by Beaulieu et al. (2011) to monitor the active population of T. harzianum in peat and green compost. The results of this study revealed a significant correlation between qRT-PCR and the parallelly applied serial dilution technique. In a more recent study, Mendoza-Mendoza et al. (2015) identified key genes involved in the morphogenesis of Trichoderma sp. “atroviride type B” and developed molecular marker-based assays to follow the development of the strain in soil environment. The authors developed an efficient total RNA isolation method from soil and followed the expression profiles of Trichoderma genes specific to different developmental stages (dormant conidia, germination stage, and vegetative hyphae) by qRT-PCR. Microarray technology offers further perspectives for studying Trichoderma populations in natural environments by following the expression changes of several genes. With the availability of field-portable microarray analysis systems (Chandler et al., 2010), this approach not only enables the monitoring of active Trichoderma populations, but may also provide information about the structure and dynamics of the entire microbial community, as well as about the physiological status of Trichoderma populations in the soil environment. For the selection of target genes appropriate for monitoring by microarrays, the results deriving from transcriptomic studies and comparative transcriptome analyses performed in vitro or in soil microcosm systems for Trichoderma-fungus (Atanasova et al., 2013; Steindorff et al., 2014; Morán-Diez et al., 2015; Perazzolli et al., 2016; Shaw et al., 2016) and Trichoderma-plant (Chacón et al., 2007; Samolski et al., 2009; Rubio et al., 2012; Shaw et al., 2016) interactions are highly valuable. Metatranscriptome analysis of agricultural habitats after the introduction of Trichoderma as a biocontrol agent has further potential to reveal important information about the changes of the entire, active microbiome, however, similarly to the sequencing of full metagenome, this approach is also expensive and time-consuming due to the necessity of appropriate, high-throughput sequencing and computational platforms, furthermore, the choice of an appropriate RNA isolation method is crucial due to the RNA's high susceptibility to degradation by RNase enzymes.
Besides substantially aiding the process of Trichoderma strain selection as well as the development of application strategies in the case of BCAs, the increasing amount of data available in the literature about the proteome, metabolome, and secretome of Trichoderma strains from different species (Sharma et al., 2009; Lorito et al., 2010; Druzhinina et al., 2012; Contreras-Cornejo et al, 2016) may also contribute to the development of new monitoring strategies based on the detection of specific cellular or secreted proteins or secondary metabolites. For example, the non-ribosomally synthesized peptaibols have the potential to form the basis of mass spectrometry-based, species-specific monitoring strategies, as particular Trichoderma species produce a unique peptaibiome which is different even from that of closely related species (Marik et al., 2017).
Conclusions
During the past decades, the methods for monitoring Trichoderma populations in agricultural environments evolved from genus-specific, classical microbiological tools through species-specific immunological and fingerprinting techniques to high throughput, strain-specific real-time PCR methodologies. Table 3 shows a critical evaluation of the reviewed methods available for Trichoderma monitoring (detection and quantification) in agricultural environments, with special focus on their strengths and limitations. An ideal tool for monitoring Trichoderma species/strains in agricultural environments is expected to be cheap, highly sensitive, and reproducible, specific at strain (or at least at species) level, able to accurately quantify fungal densities by detecting only the active fungal elements, applicable to commercial BCAs, as well as easy to perform, without the need of extensive knowledge of classical taxonomy or expensive equipments. Although none of the discussed methods meet all these requirements, certain tools like the application of qRT PCR, strain-specific primers developed based on SCAR markers or comparative omics approaches possess a wide scale of advantages, making them suitable as parts of strategies aimed at Trichoderma monitoring in agricultural environments. Furthermore, it can be expected that the era of bioinformatically supported genomic, transcriptomic and metabolomic approaches will further increase our understanding of the population dynamics and ecology of Trichoderma in agricultural environments, thereby delivering useful information for the optimization of application strategies in the case of BCAs, and also supporting the development of adequate and efficient control methods for the harmful members of the genus.
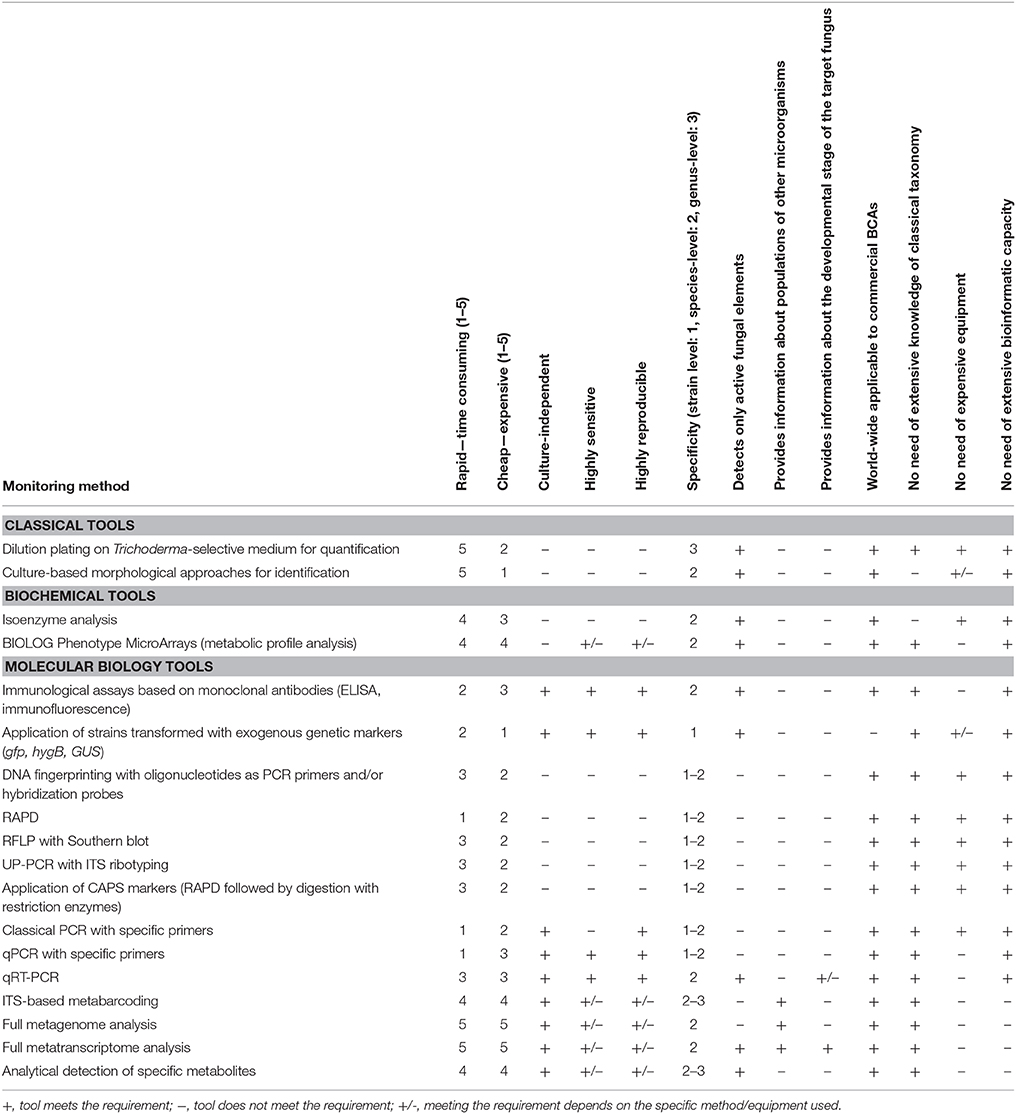
Table 3. Critical evaluation of the advantages and limitations of techniques applicable for monitoring (detection and quantification) Trichoderma species/strains in agricultural environments.
Author Contributions
LC and CV reviewed the immunological approaches. OK and JK reviewed the introduction of exogenous marker genes. RB and NA reviewed fingerprinting techniques. LH and HA reviewed species-specific PCR and metagenomics tools. VN and LK reviewed the design of strain-specific PCR primers. LK and CV prepared the abstract, introduction and conclusions, and assembled the manuscript. Critical reviewing of all content and editing of the manuscript were performed by LK, CV, JK, NA, and LH.
Funding
CV was supported by the Hungarian Government and the European Union within the frames of the Széchenyi 2020 Programme through grant GINOP-2.3.2-15-2016-00052. LK was supported by grants GINOP-2.2.1-15-2016-00006 (Széchenyi 2020 Programme), NKFI K-116475 (National Research, Development and Innovation Office), and the János Bolyai Research Scholarship (Hungarian Academy of Sciences). The authors extend their appreciation to the International Scientific Partnership Program (ISPP) at King Saud University for supporting the preparation of this review through ISPP#0105.
Conflict of Interest Statement
The authors declare that the research was conducted in the absence of any commercial or financial relationships that could be construed as a potential conflict of interest.
The reviewer FV and handling editor declared their shared affiliation at time of review.
Acknowledgments
The gfp-labeled Trichoderma pleuroti strain depicted in Figure 1 was kindly provided by Irina S. Druzhinina and Jian Zhang (Microbiology and Applied Genomics Group, Research Area Biochemical Technology, Institute of Chemical, Environmental & Bioscience Engineering, TU Wien, Vienna, Austria).
References
Abbasi, P. A., Miller, S. A., Meulia, T., Hoitink, H. A., and Kim, J. M. (1999). Precise detection and tracing of Trichoderma hamatum 382 in compost-amended potting mixes by using molecular markers. Appl. Environ. Microbiol. 65, 5421–5426.
Ahmad, J. S., and Baker, R. (1987). Competitive saprophytic ability and cellulolytic activity of rhizosphere-competent mutants of Trichoderma harzianum. Phytopathology 77, 358–362.
Arisan-Atac, I., Heidenreich, E., and Kubicek, C. P. (1995). Randomly amplified polymorphic DNA fingerprinting identifies subgroups of Trichoderma viride and other Trichoderma sp. capable of chestnut blight biocontrol. FEMS Microbiol. Lett. 126, 249–255. doi: 10.1016/0378-1097(95)00017-Y
Atanasova, L., Le Crom, S., Gruber, S., Coulpier, F., Seidl-Seiboth, V., Kubicek, C. P., et al. (2013). Comparative transcriptomics reveals different strategies of Trichoderma mycoparasitism. BMC Genomics 14:121. doi: 10.1186/1471-2164-14-121
Bae, Y. S., and Knudsen, G. R. (2000). Cotransformation of Trichoderma harzianum with β-glucuronidase and green fluorescent protein genes provides a useful tool for monitoring fungal growth and activity in natural soils. Appl. Environ. Microbiol. 66, 810–815.
Bae, Y. S., and Knudsen, G. R. (2001). Influence of a fungus-feeding nematode on growth and biocontrol efficacy of Trichoderma harzianum. Phytopathology 91, 301–306. doi: 10.1094/PHYTO.2001.91.3.301
Baek, J. M., and Kenerley, C. M. (1998). Detection and enumeration of a genetically modified fungus in soil environments by quantitative competitive polymerase chain reaction. FEMS Microbiol. Ecol. 25, 419–428. doi: 10.1111/j.1574-6941.1998.tb00493.x
Baroncelli, R., Piaggeschi, G., Fiorini, L., Bertolini, E., Zapparata, A., Pè, M. E., et al. (2015). Draft whole-genome sequence of the biocontrol agent Trichoderma harzianum T6776. Genome Announc. 3:e00647–15. doi: 10.1128/genomeA.00647-15
Baroncelli, R., Zapparata, A., Piaggeschi, G., Sarrocco, S., and Vannacci, G. (2016). Draft whole-genome sequence of Trichoderma gamsii T6085, a promising biocontrol agent of Fusarium head blight on wheat. Genome Announc. 4:e01747–15. doi: 10.1128/genomeA.01747-15
Beaulieu, R., López-Mondéjar, R., Tittarelli, F., Ros, M., and Pascual, J. A. (2011). qRT-PCR quantification of the biological control agent Trichoderma harzianum in peat and compost-based growing media. Biores. Technol. 102, 2793–2798. doi: 10.1016/j.biortech.2010.09.120
Bhat, G. (2007). Cloning and Functional Characterization of Endochitinase Gene From Trichoderma spp. MSc. thesis, University of Agricultural Sciences, Dharwad.
Bowen, J. K., Franicevic, S. C., Crowhurst, R. N., Templeton, M. D., and Stewart, A. (1996). Differentiation of a specific Trichoderma biological control agent by restriction fragment length polymorphism (RFLP) analysis. N. Z. J. Crop Hort. Sci. 24, 201–211. doi: 10.1080/01140671.1996.9513955
Bulat, S. A., Lübeck, M., Mironenko, N. V., Jensen, D. F., and Lübeck, P. S. (1998). UP-PCR analysis and ITS1 ribotyping of Trichoderma and Gliocladium fungi. Mycol. Res. 102, 933–943. doi: 10.1017/S0953756297005686
Carter, J. P., and Lynch, J. M. (1991). Substrate-dependent variation in the protein profile and antigens of Trichoderma harzianum. Enzyme Microb. Technol. 13, 537–543. doi: 10.1016/0141-0229(91)90088-R
Chacón, M. R., Rodríguez-Galán, O., Benítez, T., Sousa, S., Rey, M., Llobell, A., et al. (2007). Microscopic and transcriptome analyses of early colonization of tomato roots by Trichoderma harzianum. Int. Microbiol. 10, 19–27. doi: 10.2436/20.1501.01.4
Chandler, D. P., Kukhtin, A., Mokhiber, R., Knickerbocker, C., Ogles, D., Rudy, G., et al. (2010). Monitoring microbial community structure and dynamics during in situ U(VI) bioremediation with a field-portable microarray analysis system. Environ. Sci. Technol. 44, 5516–5522. doi: 10.1021/es1006498
Chen, X., Romaine, C. P., Ospina-Giraldo, M. D., and Royse, D. J. (1999). A polymerase chain reaction-based test for the identification of Trichoderma harzianum biotypes 2 and 4, responsible for the worldwide green mold epidemic in cultivated Agaricus bisporus. Appl. Microbiol. Biotechnol. 52, 246–250. doi: 10.1007/s002530051516
Chung, Y. R., and Hoitink, H. A. J. (1990). Interactions between thermophilic fungi and Trichoderma hamatum in suppression of Rhizoctonia damping-off in a bark compost-amended container medium. Phytopathology 80, 73–77. doi: 10.1094/Phyto-80-73
Compant, S., Gerbore, J., Antonielli, L., Brutel, A., and Schmoll, M. (2017). Draft genome sequence of the root-colonizing fungus Trichoderma harzianum B97. Genome Announc. 5:A.00137-17. doi: 10.1128/genomeA.00137-17
Contina, J. B., Dandurand, L. M., and Knudsen, G. R. (2017). Use of GFP-tagged Trichoderma harzianum as a tool to study the biological control of the potato cyst nematode Globodera pallida. Appl. Soil Ecol. 115, 31–37. doi: 10.1016/j.apsoil.2017.03.010
Contreras-Cornejo, H. A., Macías-Rodríguez, L., del-Val, E., and Larsen, J (2016) Ecological functions of Trichoderma spp. their secondary metabolites in the rhizosphere: interactions with plants. FEMS Microbiol. Ecol. 92:fiw036. doi: 10.1093/femsec/fiw036
Cordier, C., Edel-Hermann, V., Martin-Laurent, F., Blal, B., Steinberg, C., and Alabouvette, C. (2007). SCAR-based real time PCR to identify a biocontrol strain (T1) of Trichoderma atroviride and study its population dynamics in soils. J. Microbiol. Methods 68, 60–68. doi: 10.1016/j.mimet.2006.06.006
Cumagun, J. C. R., Hockenhull, J., and Lübeck, M. (2000). Characterization of Trichoderma isolates from Philippine rice fields by UP-PCR and rDNA-ITS1 analysis: identification of UP-PCR Markers. J. Phytopathol. 148, 109–115. doi: 10.1046/j.1439-0434.2000.00467.x
Dodd, S. L., Hill, R. A., and Stewart, A. (2004a). Monitoring the survival and spread of the biocontrol fungus Trichoderma atroviride (C65) on kiwifruit using a molecular marker. Austr. Plant Pathol. 33, 189–196. doi: 10.1071/AP03070
Dodd, S. L., Hill, R. A., and Stewart, A. (2004b). A duplex-PCR bioassay to detect a Trichoderma virens biocontrol isolate in non-sterile soil. Soil Biol. Biochem. 36, 1955–1965. doi: 10.1016/j.soilbio.2004.03.012
Druzhinina, I. S., Komon-Zelazowska, M., Kredics, L., Hatvani, L., Antal, Z., Belayneh, T., et al. (2008). Alternative reproductive strategies of Hypocrea orientalis and genetically close but clonal Trichoderma longibrachiatum, both capable of causing invasive mycoses of humans. Microbiology 154, 3447–3459. doi: 10.1099/mic.0.2008/021196-0
Druzhinina, I. S., Kopchinskiy, A. G., Komon, M., Bissett, J., Szakacs, G., and Kubicek, C. P. (2005). An oligonucleotide barcode for species identification in Trichoderma and Hypocrea. Fungal Genet. Biol. 42, 813–828. doi: 10.1016/j.fgb.2005.06.007
Druzhinina, I. S., Shelest, E., and Kubicek, C. P. (2012). Novel traits of Trichoderma predicted through the analysis of its secretome. FEMS Microbiol. Lett. 337, 1–9. doi: 10.1111/j.1574-6968.2012.02665.x
Elad, Y., Chet, I., and Henis, Y. (1981). A selective medium for improving quantitative isolation of Trichoderma spp. from soil. Phytoparasitica 9, 59–67. doi: 10.1007/BF03158330
Fanti, S., Barbieri, A., and Vannacci, G. (2003). “Environmental monitoring of antagonistic Trichoderma”, in Abstracts of the 1st Federation of European Microbiological Societies (FEMS) Congress of European Microbiologists (Ljubljana: FEMS), 428.
Feng, X. M., Holmberg, A.-I. J, Sundh, I., Ricard, T., and Melin, P. (2011). Specific SCAR markers and multiplex real-time PCR for quantification of two Trichoderma biocontrol strains in environmental samples. Biol. Control 56, 903–913. doi: 10.1007/s10526-011-9365-7
Fredricks, D. N., Smith, C., and Meier, A. (2005). Comparison of six DNA extraction methods for recovery of fungal DNA as assessed by quantitative PCR. J. Clin. Microbiol. 43, 5122–5128. doi: 10.1128/JCM.43.10.5122-5128.2005
Freeman, S., Kolesnik, I., Barbul, O., Zveibil, A., Maymon, M., Nitzani, Y., et al. (2002). Use of Trichoderma spp. for biocontrol of Colletotrichum acutatum (anthracnose) and Botrytis cinerea (grey mould) in strawberry, and study of biocontrol population survival by PCR. IOBC WPRS Bull. 25, 167–170.
Friedl, M. A., and Druzhinina, I. S. (2012). Taxon-specific metagenomics of Trichoderma reveals a narrow community of opportunistic species that regulate each other's development. Microbiology 158, 69–83. doi: 10.1099/mic.0.052555-0
Geistlinger, J., Zwanzig, J., Heckendorff, S., and Schellenberg, I. (2015). SSR markers for Trichoderma virens: their evaluation and application to identify and quantify root-endophytic strains. Diversity 7, 360–384. doi: 10.3390/d7040360
Green, H., and Jensen, D. F. (1995). A tool for monitoring Trichoderma harzianum: II. the use of a GUS transformant for ecological studies in the rhizosphere. Phytopathology 85, 1436–1440. doi: 10.1094/Phyto-85-1436
Grondona, I., Hermosa, R., Tejada, M., Gomis, M. D., Mateos, P. F., Bridge, P. D., et al. (1997). Physiological and biochemical characterization of Trichoderma harzianum, a biological control agent against soilborne fungal plant pathogens. Appl. Environ. Microbiol. 63, 3189–3198.
Gupta, V. K., Schmoll, M., Herrera-Estrella, A., Upadhyay, R. S., Druzhinina, I., and Tuohy, M. G (Eds.). (2014). Biotechnology and Biology of Trichoderma. Amsterdam: Elsevier.
Hagn, A., Wallisch, S., Radl, V., Charles Munch, J., and Schloter, M. (2007). A new cultivation independent approach to detect and monitor common Trichoderma species in soils. J. Microbiol. Methods 69, 86–92. doi: 10.1016/j.mimet.2006.12.004
Harman, G. E., Howell, C. R., Viterbo, A., Chet, I., and Lorito, M. (2004). Trichoderma species: opportunistic, avirulent plant symbionts. Nat. Rev. Microbiol. 2, 43–56. doi: 10.1038/nrmicro797
Hatvani, L., Kocsubé, S., Manczinger, L., Antal, Z., Szekeres, A., Druzhinina, I. S., et al. (2008). “The green mould disease global threat to the cultivation of oyster mushroom (Pleurotus ostreatus): a review,” in Science and Cultivation of Edible and Medicinal Fungi: Mushroom Science XVII: Proceedings of the 17th Congress of the International Society for Mushroom Science, ed M. Gruening (Cape Town: ISMS), 485–495.
Hatvani, L., Kredics, L., Allaga, H., Manczinger, L., Vágvölgyi, C., Kuti, K., et al. (2017). First report of Trichoderma aggressivum f. aggressivum green mold on Agaricus bisporus in Europe. Plant Dis. 101, 1052–1053. doi: 10.1094/PDIS-12-16-1783-PDN
Hermosa, M. R., Grondona, I., Díaz-Mínguez, J. M., Iturriaga, E. A., and Monte, E. (2001). Development of a strain-specific SCAR marker for the detection of the Trichoderma atroviride 11, a biological control agent against soilborne fungal plant pathogens. Curr. Genet. 38, 343–350. doi: 10.1007/s002940000173
Horn, I. R., van Rijn, M., Zwetsloot, T. J., Basmagi, S., Dirks-Mulder, A., van Leeuwen, W. B., et al. (2016). Development of a multiplex Q-PCR to detect Trichoderma harzianum Rifai strain T22 in plant roots. J. Microbiol. Methods 121, 44–49. doi: 10.1016/j.mimet.2015.12.014
Hoyos-Carvajal, L., Orduz, S., and Bissett, J. (2009). Genetic and metabolic biodiversity of Trichoderma from Colombia and adjacent neotropic regions. Fungal Genet. Biol. 46, 615–631. doi: 10.1016/j.fgb.2009.04.006
Kim, T. G., and Knudsen, G. R. (2008). Quantitative real-time PCR effectively detects and quantifies colonization of sclerotia of Sclerotinia sclerotiorum by Trichoderma spp. Appl. Soil Ecol. 40, 100–108. doi: 10.1016/j.apsoil.2008.03.013
Kim, T. G., and Knudsen, G. R. (2011). Comparison of real-time PCR and microscopy to evaluate sclerotial colonisation by a biocontrol fungus. Fungal Biol. 115, 317–325. doi: 10.1016/j.funbio.2010.12.008
Kopchinskiy, A., Komon, M., Kubicek, C. P., and Druzhinina, I. S. (2005). TrichoBLAST: a multilocus database for Trichoderma and Hypocrea identifications. Mycol. Res. 109, 658–660. doi: 10.1017/S0953756205233397
Kowsari, M., Motallebi, M., and Zamani, R. M. (2014). Construction of new GFP-tagged fusants for Trichoderma harzianum with enhanced biocontrol activity. J. Plant Prot. Res. 54, 122–131. doi: 10.2478/jppr-2014-0020.
Kredics, L., Antal, Z., Manczinger, L., Szekeres, A., Kevei, F., and Nagy, E. (2003). Influence of environmental parameters on Trichoderma strains with biocontrol potential. Food Technol. Biotechnol. 41, 37–42.
Kredics, L., García Jimenez, L., Naeimi, S., Czifra, D., Urbán, P., Manczinger, L., et al. (2010). “A challenge to mushroom growers: the green mould disease of cultivated champignons,” in Current Research, Technology and Education Topics in Applied Microbiology and Microbial Biotechnology, ed A. Méndez-Vilas (Badajoz: Formatex), 295–305.
Kredics, L., Kocsubé, S., Nagy, L., Komon-Zelazowska, M., Manczinger, L., Sajben, E., et al. (2009). Molecular identification of Trichoderma species associated with Pleurotus ostreatus and natural substrates of the oyster mushroom. FEMS Microbiol. Lett. 300, 58–67. doi: 10.1111/j.1574-6968.2009.01765.x
Kredics, L., Láday, M., Körmöczi, P., Manczinger, L., Rákhely, G., Vágvölgyi, C., et al. (2012). Genetic and biochemical diversity among Trichoderma isolates in soil samples from winter wheat fields of the Great Hungarian Plain. Acta Biol. Szeged. 56, 141–149.
Kubicek, C. P., Herrera-Estrella, A., Seidl-Seiboth, V., Martinez, D. A., Druzhinina, I. S., Thon, M., et al. (2011). Comparative genome sequence analysis underscores mycoparasitism as the ancestral life style of Trichoderma. Genome Biol. 12:R40. doi: 10.1186/gb-2011-12-4-r40
Kullnig-Gradinger, C. M., Szakacs, G., and Kubicek, C. P. (2002). Phylogeny and evolution of the genus Trichoderma: a multigene approach. Mycol. Res. 106, 757–767. doi: 10.1017/S0953756202006172
Kuo, H. C., Wang, T. Y., Chen, P. P., Chen, R. S., and Chen, T. Y. (2015). Genome sequence of Trichoderma virens FT-333 from tropical marine climate. FEMS Microbiol. Lett. 362:fnv036. doi: 10.1093/femsle/fnv036
Lange, C., Weld, R. J., Cox, M. P., Bradshaw, R. E., McLean, K. L., Stewart, A., et al. (2016). Genome-scale investigation of phenotypically distinct but nearly clonal Trichoderma strains. PeerJ 4:e2023. doi: 10.7717/peerj.2023
Lo, C. T., Nelson, E. B., Hayes, C. K., and Harman, G. E. (1998). Ecological studies of transformed Trichoderma harzianum strain 1295-22 in rhizosphere and on the phylloplane of creeping bentgrass. Phytopathology 88, 129–136. doi: 10.1094/PHYTO.1998.88.2.129
Longa, C. M., Savazzini, F., Tosi, S., Elad, Y., and Pertot, I. (2009). Evaluating the survival and environmental fate of the biocontrol agent Trichoderma atroviride SC1 in vineyards in northern Italy. J. Appl. Microbiol. 106, 1549–1557. doi: 10.1111/j.1365-2672.2008.04117.x
Longa, C. M., and Pertot, I. (2009). An intact soil-core microcosm method to evaluate the survival and vertical dispersal of Trichoderma atroviride SC1. Lett. Appl. Microbiol. 49, 609–614. doi: 10.1111/j.1472-765X.2009.02715.x
Lopes, F. A., Steindorff, A. S., Geraldine, A. M., Brandão, R. S., Monteiro, V. N., Lobo, M., et al. (2012). Biochemical and metabolic profiles of Trichoderma strains isolated from common bean crops in the Brazilian Cerrado, and potential antagonism against Sclerotinia sclerotiorum. Fungal Biol. 116, 815–824. doi: 10.1016/j.funbio.2012.04.015
López-Bucio, J., Pelagio-Flores, R., and Herrera-Estrella, A. (2015). Trichoderma as biostimulant: exploiting the multilevel properties of a plant beneficial fungus. Sci. Hortic. 196, 109–123. doi: 10.1016/j.scienta.2015.08.043
López-Mondéjar, R., Antón, A., Raidl, S., Ros, M., and Pascual, J. A. (2010). Quantification of the biocontrol agent Trichoderma harzianum with real-time TaqMan PCR and its potential extrapolation to the hyphal biomass. Biores. Technol. 101, 2888–2891. doi: 10.1016/j.biortech.2009.10.019
Lorito, M., Woo, S. L., Harman, G. E., and Monte, E. (2010). Translational research on Trichoderma: from 'omics to the field. Annu. Rev. Phytopathol. 48, 395–417. doi: 10.1146/annurev-phyto-073009-114314
Lübeck, M., and Jensen, D. F. (2002). Monitoring of biocontrol agents based on Trichoderma strains following their application to glasshouse crops by combining dilution plating with UP-PCR fingerprinting. Biocontrol Sci. Technol. 12, 371–380. doi: 10.1080/09583150220128158
Lu, Z., Tombolini, R., Woo, S., Zeilinger, S., Lorito, M., and Jansson, J. K. (2004). In vivo study of Trichoderma-pathogen-plant interactions, using constitutive and inducible green fluorescent protein reporter systems. Appl. Environ. Microbiol. 70, 3073–3081. doi: 10.1128/AEM.70.5.3073-3081.2004
Mahfooz, S., Singh, S. P., Rakh, R., Bhattacharya, A., Mishra, N., Singh, P. C., et al. (2016). A comprehensive characterization of simple sequence repeats in the sequenced Trichoderma genomes provides valuable resources for marker development. Front. Microbiol. 7:575. doi: 10.3389/fmicb.2016.00575
Marik, T., Urbán, P., Tyagi, C., Szekeres, A., Leitgeb, B., Vágvölgyi, M., et al. (2017). Diversity profile and dynamics of peptaibols produced by green mould Trichoderma species in interactions with their hosts Agaricus bisporus and Pleurotus ostreatus. Chem. Biodivers. 14:e1700033. doi: 10.1002/cbdv.201700033
Martinez, D., Berka, R. M., Henrissat, B., Saloheimo, M., Arvas, M., Baker, S. E., et al. (2008). Genome sequencing and analysis of the biomass-degrading fungus Trichoderma reesei (syn. Hypocrea jecorina). Nat. Biotechnol. 26, 553–560. doi: 10.1038/nbt1403
Meena, S. N. (2009). Development of Species-Specific SCAR Markers for the Identification of Trichoderma Species. Master's thesis, Department of Biotechnology, College of Agriculture, Dharwad University of Agricultural Sciences, Dharwad.
Meincke, R., Weinert, N., Radl, V., Schloter, M., Smalla, K., and Berg, G. (2010). Development of a molecular approach to describe the composition of Trichoderma communities. J. Microbiol. Methods 80, 63–69. doi: 10.1016/j.mimet.2009.11.001
Mendoza-Mendoza, A., Steyaert, J., Nieto-Jacobo, M. F., Holyoake, A., Braithwaite, M., and Stewart, A. (2015). Identification of growth stage molecular markers in Trichoderma sp. 'atroviride type B' and their potential application in monitoring fungal growth and development in soil. Microbiology 161, 2110–2126. doi: 10.1099/mic.0.000167
Migheli, Q., Herrera-Estrella, A., Avataneo, M., and Gullino, M. L. (1994). Fate of transformed Trichoderma harzianum in the phylloplane of tomato plants. Mol. Ecol. 3, 153–159. doi: 10.1111/j.1365-294X.1994.tb00116.x
Morán-Diez, M. E., Trushina, N., Lamdan, N. L., Rosenfelder, L., Mukherjee, P. K., Kenerley, C. M., et al. (2015). Host-specific transcriptomic pattern of Trichoderma virens during interaction with maize or tomato roots. BMC Genomics 16:8. doi: 10.1186/s12864-014-1208-3
Naef, A., Senatore, M., and Defago, G. (2006). A microsatellite-based method for quantification of fungi in decomposing plant material elucidates the role of Fusarium graminearum DON production in saprophytic competition with Trichoderma atroviride in maize tissue microcosms. FEMS Microbiol. Ecol. 55, 211–220. doi: 10.1111/j.1574-6941.2005.00023.x
Naeimi, S., Kocsubé, S., Antal, Z., Okhovvat, S. M., Javan-Nikkhah, M., Vágvölgyi, C., et al. (2011). Strain-specific SCAR markers for the detection of Trichoderma harzianum AS12-2, a biological control agent against Rhizoctonia solani, the causal agent of rice sheath blight. Acta Biol. Hung. 62, 73–84. doi: 10.1556/ABiol.61.2011.1.8
Nawrocka, J., and Małolepsza, U. (2013). Diversity in plant systemic resistance induced by Trichoderma. Biol. Control 67, 149–156. doi: 10.1016/j.biocontrol.2013.07.005
Orr, K. A., and Knudsen, G. R. (2004). Use of green fluorescent protein and image analysis to quantify proliferation of Trichoderma harzianum in nonsterile soil. Phytopathology 94, 1383–1389. doi: 10.1094/PHYTO.2004.94.12.1383
Oskiera, M., Szczech, M., Stepowska, A., Smolinska, U., and Bartoszewski, G. (2017). Monitoring of Trichoderma species in agricultural soil in response to application of biopreparations. Biol. Control 113, 65–72. doi: 10.1016/j.biocontrol.2017.07.005
Paran, I., and Michelmore, R. W. (1993). Development of reliable PCR-based markers linked to downy mildew resistance genes in lettuce. Theor. Appl. Genet. 85, 985–993. doi: 10.1007/BF00215038
Park, M. S., Bae, K. S., and Yu, S. H. (2006). Two new species of Trichoderma associated with green mold of oyster mushroom cultivation in Korea. Mycobiology 34, 111–113. doi: 10.4489/MYCO.2006.34.3.111
Perazzolli, M., Herrero, N., Sterck, L., Lenzi, L., Pellegrini, A., Puopolo, G., et al. (2016). Transcriptomic responses of a simplified soil microcosm to a plant pathogen and its biocontrol agent reveal a complex reaction to harsh habitat. BMC Genomics 17:838. doi: 10.1186/s12864-016-3174-4
Pérez, G., Verdejo, V., Gondim-Porto, C., Orlando, J., and Carú, M. (2014). Designing a SCAR molecular marker for monitoring Trichoderma cf. harzianum in experimental communities. J. Zhejiang Univ. Sci. B Biomed. Biotechnol. 15, 966–978. doi: 10.1631/jzus.B1400063
Providenti, M. A., Mautner, S. I., Chaudhry, O., Bombardier, M., Scroggins, R., Gregorich, E., et al. (2004). Determining the environmental fate of a filamentous fungus, Trichoderma reesei, in laboratory-contained intact soil-core microcosms using competitive PCR and viability plating. Can. J. Microbiol. 50, 623–631. doi: 10.1139/w04-053
Pujol, M., Badosa, E., Manceau, C., and Montesinos, E. (2006). Assessment of the environmental fate of the biological control agent of fire blight, Pseudomonas fluorescens EPS62e, on apple by culture and real-time PCR methods. Appl. Environ. Microbiol. 72, 2421–2427. doi: 10.1128/AEM.72.4.2421-2427.2006
Rubio, M. B., Domínguez, S., Monte, E., and Hermosa, R. (2012). Comparative study of Trichoderma gene expression in interactions with tomato plants using high-density oligonucleotide microarrays. Microbiology 158, 119–128. doi: 10.1099/mic.0.052118-0
Rubio, M. B., Hermosa, M. R., Keck, E., and Monte, E. (2005). Specific PCR assays for the detection and quantification of DNA from the biocontrol strain Trichoderma harzianum 2413 in soil. Microb. Ecol. 49, 25–33. doi: 10.1007/s00248-003-0171-3
Samolski, I., de Luis, A., Vizcaíno, J. A., Monte, E., and Suárez, M. B. (2009). Gene expression analysis of the biocontrol fungus Trichoderma harzianum in the presence of tomato plants, chitin, or glucose using a high-density oligonucleotide microarray. BMC Microbiol. 9:217. doi: 10.1186/1471-2180-9-217
Samuels, G. J., Dodd, S. L., Gams, W., Castlebury, L. A., and Petrini, O. (2002) Trichoderma species associated with the green mold epidemic of commercially grown Agaricus bisporus. Mycologia 94, 146–170. doi: 10.2307/3761854
Savazzini, F., Longa, C. M. O., Pertot, I., and Gessler, C. (2008). Real-time PCR for detection and quantification of the biocontrol agent Trichoderma atroviride strain SC1 in soil. J. Microbiol. Methods 73, 185–194. doi: 10.1016/j.mimet.2008.02.004
Schlick, A., Kuhls, K., Meyer, W., Lieckfeldt, E., Börner, T., and Messner, K. (1994). Fingerprinting reveals gamma-ray induced mutations in fungal DNA: implications for identification of patent strains of Trichodenna harzianum. Curr. Genet. 26, 74–78. doi: 10.1007/BF00326307
Schmoll, M., Dattenböck, C., Carreras-Villaseñor, N., Mendoza-Mendoza, A., Tisch, D., and Alemán, M. I (2016) The genomes of three uneven siblings: footprints of the lifestyles of three Trichoderma species. Microbiol. Mol. Biol. Rev. 80, 205–327. doi: 10.1128/MMBR.00040-15.
Sharma, K., Mishra, A. K., and Misra, R. J. (2009). Morphological, biochemical and molecular characterization of Trichoderma harzianum isolates for their efficacy as biocontrol agents. J. Phytopathol. 157, 51–56. doi: 10.1111/j.1439-0434.2008.01451.x
Shaw, S., Le Cocq, K., Paszkiewicz, K., Moore, K., Winsbury, R., de Torres Zabala, M., et al. (2016). Transcriptional reprogramming underpins enhanced plant growth promotion by the biocontrol fungus Trichoderma hamatum GD12 during antagonistic interactions with Sclerotinia sclerotiorum in soil. Mol. Plant Pathol. 17, 1425–1441. doi: 10.1111/mpp.12429
Shi-Kunne, X., Seidl, M. F., Faino, L., and Thomma, B. P. H. J. (2015). Draft genome sequence of a strain of cosmopolitan fungus Trichoderma atroviride. Genome Announc. 3:e00287–15. doi: 10.1128/genomeA.00287-15
Skoneczny, D., Oskiera, M., Szczech, M., and Bartoszewski, G. (2015). Genetic diversity of Trichoderma atroviride strains collected in Poland and identification of loci useful in detection of within-species diversity. Folia Microbiol. 60, 297–307. doi: 10.1007/s12223-015-0385-z
Steindorff, A. S., Ramada, M. H., Coelho, A. S., Miller, R. N., Pappas, G. J Jr, et al. (2014). Identification of mycoparasitism-related genes against the phytopathogen Sclerotinia sclerotiorum through transcriptome and expression profile analysis in Trichoderma harzianum. BMC Genomics. 15:204. doi: 10.1186/1471-2164-15-204
Tachapattaworakul, T., Fontana-Tachon, A., Guiraud, J. P., and Ratomahenina, R. (2008). Application of benomyl resistance and RAPD-PCR for detecting a Trichoderma atroviride strain used as biocontrol agent. Biol. Agric. Hortic. 25, 369–377. doi: 10.1080/01448765.2008.9755062
Thornton, C. R. (2004). An immunological approach to quantifying the saprotrophic growth dynamics of Trichoderma species during antagonistic interactions with Rhizoctonia solani in a soil-less mix. Environ. Microbiol. 6, 323–334. doi: 10.1111/j.1462-2920.2004.00574.x
Thornton, C. R., and Dewey, F. M. (1996). Detection of phialoconidia of Trichoderma harzianum in peat-bran by monoclonal antibody-based enzyme-linked immunosorbent assay. Mycol. Res. 100, 217–222. doi: 10.1016/S0953-7562(96)80126-2
Thornton, C. R., Dewey, F. M., and Gillican, C. A. (1994). Development of a monoclonal antibody-based enzyme-linked immunosorbent assay for the detection of live propagules of Trichoderma harzianum in a peat-bran medium. Soil Biol. Biochem. 26, 909–920. doi: 10.1016/0038-0717(94)90307-7
Thornton, C. R., O'Neill, T. M., Hilton, G., and Gilligan, C. A. (1999). Detection and recovery of Rhizoctonia solani in naturally infested glasshouse soils using a combined baiting, double monoclonal antibody ELISA. Plant Pathol. 48, 627–634. doi: 10.1046/j.1365-3059.1999.00386.x
Thornton, C. R., Pitt, D., Wakley, G. E., and Talbot, N. J. (2002). Production of a monoclonal antibody specific to the genus Trichoderma and closely related fungi, and its use to detect Trichoderma spp. in naturally infested composts. Microbiology 148, 1263–1279. doi: 10.1099/00221287-148-5-1263
Thrane, C., Lübeck, M., Green, H., Degefu, Y., Allerup, S., Thrane, U., et al. (1995). A tool for monitoring Trichoderma harzianum: I. transformation with the GUS gene by protoplast technology. Phytopathology 85, 1428–1435. doi: 10.1094/Phyto-85-1428
Vargas Gil, S., Pastor, S., and March, G. J. (2009). Quantitative isolation of biocontrol agents Trichoderma spp., Gliocladium spp. and actinomycetes from soil with culture media. Microbiol. Res. 164, 196–205. doi: 10.1016/j.micres.2006.11.022
Weaver, M., Vedenyapina, E., and Kenerley, C. M. (2005). Fitness, persistence, and responsiveness of a genetically engineered strain of Trichoderma virens in soil mesocosms. Appl. Soil Ecol. 29, 125–134. doi: 10.1016/j.apsoil.2004.11.006
Weindling, R. (1932). Trichoderma lignorum as a parasite of other soil fungi. Phytopathology 22, 837–845.
White, T. J., Bruns, T., Lee, S., and Taylor, J. (1990). “Amplification and direct sequencing of fungal ribosomal RNA genes for phylogenetics,” in PCR Protocols: A Guide to Methods and Applications, eds M. A. Innes, D. H. Gelfand, J. J. Sninsky, and T. J. White (San Diego, CA: Academic Press), 315–322.
Williams, J., Clarkson, J. M., Mills, P. R., and Cooper, R. M. (2003). A selective medium for quantitative reisolation of Trichoderma harzianum from Agaricus bisporus compost. Appl. Environ. Microbiol. 69, 4190–4191. doi: 10.1128/AEM.69.7.4190-4191.2003
Keywords: biocontrol agent, fingerprinting, marker, monitoring, PCR, specific detection, transformation, Trichoderma
Citation: Kredics L, Chen L, Kedves O, Büchner R, Hatvani L, Allaga H, Nagy VD, Khaled JM, Alharbi NS and Vágvölgyi C (2018) Molecular Tools for Monitoring Trichoderma in Agricultural Environments. Front. Microbiol. 9:1599. doi: 10.3389/fmicb.2018.01599
Received: 19 January 2018; Accepted: 27 June 2018;
Published: 25 July 2018.
Edited by:
Raffaella Balestrini, Consiglio Nazionale Delle Ricerche (CNR), ItalyReviewed by:
Francesco Vinale, Istituto per la Protezione Sostenibile delle Piante (CNR), ItalyRoberto Silva, Universidade de São Paulo, Brazil
Copyright © 2018 Kredics, Chen, Kedves, Büchner, Hatvani, Allaga, Nagy, Khaled, Alharbi and Vágvölgyi. This is an open-access article distributed under the terms of the Creative Commons Attribution License (CC BY). The use, distribution or reproduction in other forums is permitted, provided the original author(s) and the copyright owner(s) are credited and that the original publication in this journal is cited, in accordance with accepted academic practice. No use, distribution or reproduction is permitted which does not comply with these terms.
*Correspondence: László Kredics, kredics@bio.u-szeged.hu