- 1Department of Geography and Earth Sciences, Aberystwyth University, Aberystwyth, United Kingdom
- 2Nano-Science Center, Department of Chemistry, University of Copenhagen, Copenhagen, Denmark
A number of studies have highlighted that adsorption to minerals increases DNA longevity in the environment. Such DNA-mineral associations can essentially serve as pools of genes that can be stored across time. Importantly, this DNA is available for incorporation into alien organisms through the process of horizontal gene transfer (HGT). Here we argue that minerals hold an unrecognized potential for successfully transferring genetic material across environments and timescales to distant organisms and hypothesize that this process has significantly influenced the evolution of life. Our hypothesis is illustrated in the context of the evolution of early microbial life and the oxygenation of the Earth’s atmosphere and offers an explanation for observed outbursts of evolutionary events caused by HGT.
Introduction
Traditionally, we think of evolution as following the phylogenetic tree of life, where organisms principally evolve through vertical modification of genetic information by means of sexual reproduction (Figure 1, dark blue lines). However, genes also move between lineages, where DNA from one organism is incorporated into a different species through a horizontal transfer of genetic material. The introduction of foreign DNA into an organism through horizontal gene transfer (HGT) can effectively change the ecological character of the recipient species (Ochman et al., 2000; Johnson and Grossman, 2015) and tangle the traditional evolutionary phylogenetic relationships (Figure 1, light blue lines). Over the past years, an increasing amount of evidence has solidified that HGT was essential for the evolution of prokaryotic cells (Takeuchi et al., 2014) and played a large role in the evolution of eukaryotes (Keeling and Palmer, 2008). For prokaryotes, HGT is currently recognized as a key source of innovation and adaptation to new environments and lifestyles (Doolittle, 1999; Koonin et al., 2001; Gogarten et al., 2002; Gogarten and Townsend, 2005; O’Malley and Boucher, 2005) and Puigbò et al. (2014) found that ∼ two-thirds of accounted evolutionary events originated from HGT. In addition, recent evidence showed that the main processes driving the prokaryotic evolution and rapidly changing microbial genomes are HGT and gene loss (Puigbò et al., 2014).
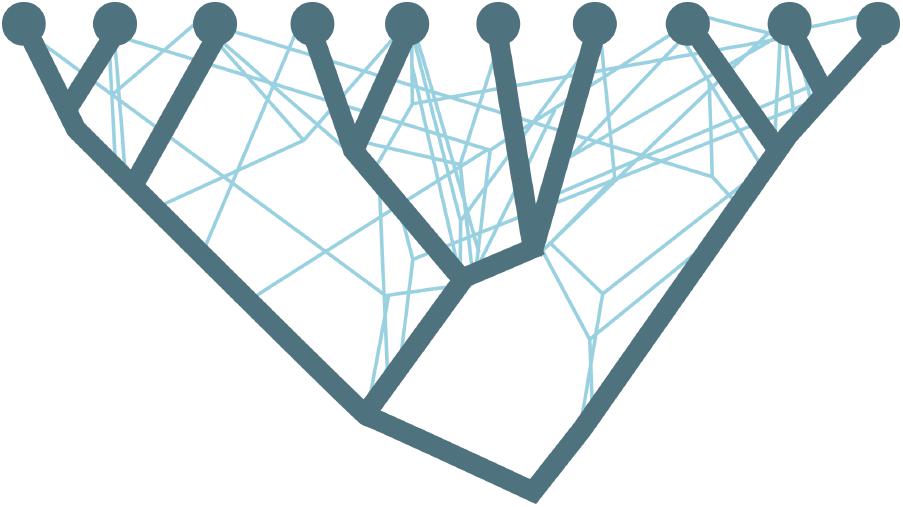
FIGURE 1. Schematics of the branches of the phylogenetic tree (dark blue lines) being crossed by the horizontal gene transfer (bright blue lines). Adapted from Puigbò et al., 2013.
In general, the evolution of an organism is tied to its ever-changing habitat and the successful processes of innovation and adaptation in which the geologic environment plays an indirect role by providing the physical setting. We propose that the distribution and availability of DNA for HGT could be facilitated by sedimentary processes and geologic events and we assign a much more direct role for the geologic environment in the evolution of life. Thus certain geologic processes and events could explain the dramatic outbursts of prokaryotic innovations caused by HGT (Puigbò et al., 2013).
“Free” or “extracellular” DNA are typically released during cell growth (Lorenz and Wackernagel, 1994; Lotareva and Prozorov, 2000), from a biofilm or cell remnants (Lorenz and Wackernagel, 1994; Cai et al., 2009) or through lysis of dead cells (Lorenz and Wackernagel, 1994; Nelson et al., 1999). However, DNA released into the aqueous environment or soil is subjected to degradation by dynamic biological, physical, and chemical factors (Levy-Booth et al., 2007) that decrease the preservation of free DNA and hence the probability of successful HGT. In sea- and freshwater environments, free DNA can only survive for weeks (Dejean et al., 2011; Thomsen et al., 2012a,b) but when associated with minerals, experimental work shows that the longevity of the DNA can be significantly prolonged (Aardema et al., 1983). E.g., it was found that DNA adsorbed to a sediment was considerable more resistant to enzymatic digestion than aqueous DNA (Lorenz et al., 1981). Compared to degradation of exogenous DNA, DNA adsorbed to clay minerals was shown to be 10 times more resistant (Khanna and Stotzky, 1992) and DNA adsorbed to sand grains required 100 times more DNase to be degraded (Romanowski et al., 1991). In addition, ∼14–550 thousand years old DNA retrieved from sediments confirm that minerals can protect adsorbed DNA in a natural setting and preserve it for geologically relevant time scales (Haile et al., 2007; Slon et al., 2017).
On a global scale, free DNA (not part of a dead biomass) found in the uppermost 10 cm of recent marine sediments corresponds to about 0.30–0.45 Gt of DNA and might represent the largest reservoir of DNA in the oceans (Dell’Anno and Danovaro, 2005). Free DNA is found in the majority of Earth’s surface ecosystems such as aqueous environments, soils and sediments (Danovaro et al., 2005; Dell’Anno and Danovaro, 2005; Levy-Booth et al., 2007). Up to 95% of this DNA is estimated to be in association with minerals (Gogarten et al., 2002) and the prolonged longevity provided by this association significantly contributes to the available pool of evolutionary traits in the aqueous environments. When geologic processes and events start acting on this mineral archive of genes, the relocated minerals act as shuttles through time and across environments and facilitate transfer of DNA and associated evolutionary traits, and can directly impact the evolution of life. HGT of DNA can occur though the transformation mechanism where the mineral adsorbed DNA are incorporated into the genome of a recipient organism (Lorenz and Wackernagel, 1988; Demanèche et al., 2001; Cai et al., 2007; Pietramellara et al., 2009; Yu et al., 2013) and even fragmented and damaged DNA are able to transfer the genetic information (Overballe-Petersen et al., 2013; Takeuchi et al., 2014).
There are three mechanisms by which genes can be transferred from one organism to another: (a) conjugation, where DNA is exchanged between bacteria in physical contact, (b) transduction, where DNA is transferred between organisms via a bacteriophage and (c) transformation, where the host organism absorbs free exogenous DNA. Conjugation requires a live donor bacterium and transduction requires an intermediate “messenger.” Neither of those would likely survive across a range of environments. Microbial motion across space is limited by a high nutrient heterogeneity in the oceanic (micro)environments (Stocker, 2012) and the high chance of encountering adverse conditions compromises cell integrity (Thomas and Nielsen, 2005) and result in cell lysis and subsequent DNA degradation or adsorption and stabilization at the mineral surface. In addition, the biomass of Archean oceans was likely lower compared to recent times (Kipp and Stüeken, 2017) highlighting that the contact between microbes from different ecological niches was more limited than it is today (Martiny et al., 2006). This suggests that conjugation and transduction are most effective for locally occurring HGT but they are not impossible at large distances. In contrast, minerals could facilitate distribution of DNA through different environments making the transfer mechanism dominant across time and space. The mechanism of microbial transformation is a complex process which depends on the properties of DNA and the competence of the recipient organism (Thomas and Nielsen, 2005). To our knowledge, it is not known if the DNA has to desorb from the mineral before being absorbed by the cell or the cell actively takes DNA directly from the mineral surface. Khanna et al. (1998) proposed that one end of DNA is attached to the mineral while the other is free in the interface region and ready to interact with the competent organism. However, the mechanism of uptake and the orientation and conformation of DNA at various mineral surfaces is still largely unknown. In the following, we advocate for the mineral facilitated HGT hypothesis and its impact on evolution of life by combining insight from DNA-mineral adsorption studies with metagenomic evidence and reports on early life and associated environmental settings. We summarize the main factors that give rise to our hypothesis and illustrate the potential impact of mineral facilitated HGT for the evolution of life. We describe a scenario that provides an explanation for detected outbursts of HGT (Puigbò et al., 2014) that caused dramatic evolutionary changes in prokaryotes (David and Alm, 2011; Puigbò et al., 2014) and link the processes of mineral facilitated HGT to the distribution of microbial metabolic traits 3.1 – 2.85 billion years (Ga) ago and the concomitant O2 buildup in the atmosphere.
Factors Influencing Mineral Facilitated HGT
Mineral associated DNA found in the environment does not simply mirror the diversity of the current active biota but represents a mixture of DNA from the overlying waters, surrounding sediments, surrounding habitats as well as dead biomass that accumulated over time. During early stages of the evolution of life, this mineral associated archive of genetic information in the water column and the seabed would have been relatively poor compared to present day simply because of less biomass (Kipp and Stüeken, 2017). Regardless, the factors impacting mineral facilitated HGT include (i) the longevity of mineral adsorbed DNA as defined by the stability of the DNA-mineral binding, (ii) the dynamics of the geologic environment in terms of events and sedimentary processes and (iii) the Earth’s near-surface mineralogy which relates to the abundance of minerals with a high DNA adsorption capacity. i, ii, and iii are closely interlinked but in contrast to i and ii which describe more or less uniform processes in the geologic history, iii has significantly changed over time. In the following sections we elaborate on each point.
Stability of the DNA-Mineral Binding
The longevity of mineral adsorbed DNA depends on a range of factors where in particular mineral species (Lorenz and Wackernagel, 1987; Cao et al., 2011; Michalkova et al., 2011; Feuillie et al., 2013; Canhisares-Filho et al., 2015; Pedreira-Segade et al., 2016), background ions (Lorenz and Wackernagel, 1987; Nguyen and Chen, 2007; Lu et al., 2010), salinity, and pH (Greaves and Wilson, 1969; Saeki et al., 2010; Michalkova et al., 2011; Feuillie et al., 2015; Maity et al., 2015) determine the DNA adsorption capacity of a mineral. Depending on the crystalline structure and composition, the surface charge of most minerals changes as a function of pH. In general, silicates have a low point of zero charge, i.e., they are negatively charged in wide pH range, whereas oxides and hydroxides have high point of zero charge, i.e., they are positively charged in a wide pH range (Figure 2). DNA interacts with minerals through its phosphate backbone (Figures 3A,B), and the nucleobases (Figure 3C) provide only a limited contribution to adsorption (Vuillemin et al., 2017). The phosphate moieties of DNA are positively charged below ∼pH 2 and can interact directly with negatively charged silicates and basal planes of clay minerals at such low pH values. Above ∼pH 2, the deprotonated and hence negatively charged phosphate moieties of the DNA can adsorb to negatively charged mineral surfaces through polyvalent cations (Ca2+, Mg2+, and Al3+) which make a “bridge” between two negative charges. These charge relationships between DNA and minerals as a function of pH make adsorption and desorption of DNA very sensitive to the geochemical characteristics of the environment (solution composition, oxygen fugacity, temperature, and pH) (Cao et al., 2011; Michalkova et al., 2011; Feuillie et al., 2013; Pedreira-Segade et al., 2016; Biondi et al., 2017) and at neutral pH conditions clay edges and oxides can be considered to have a stronger electrostatic interaction with the negatively charged DNA moieties. These charge relationships are also likely to affect degradation by biological factors. A strong adsorption to a mineral could well prevent the unzipping of the double helix or change the conformation of the DNA so it cannot be recognized by degrading enzymes. In addition, the longevity of DNA is originally assumed to be most efficient in anoxic depositional environments (Coolen et al., 2002). Yet, the unambiguous finding of ancient DNA within sediments deposited under oxic conditions (Willerslev et al., 2003; Corinaldesi et al., 2008) suggest that oxic environments also offer preservation. The experimental aims and setups of reported studies of DNA-mineral interactions vary significantly between studies making it very difficult to quantitatively address the causal effects on longevity from available data. In the following, we therefore generalize trends primarily based on grain size and established trends for DNA adsorption capacities.
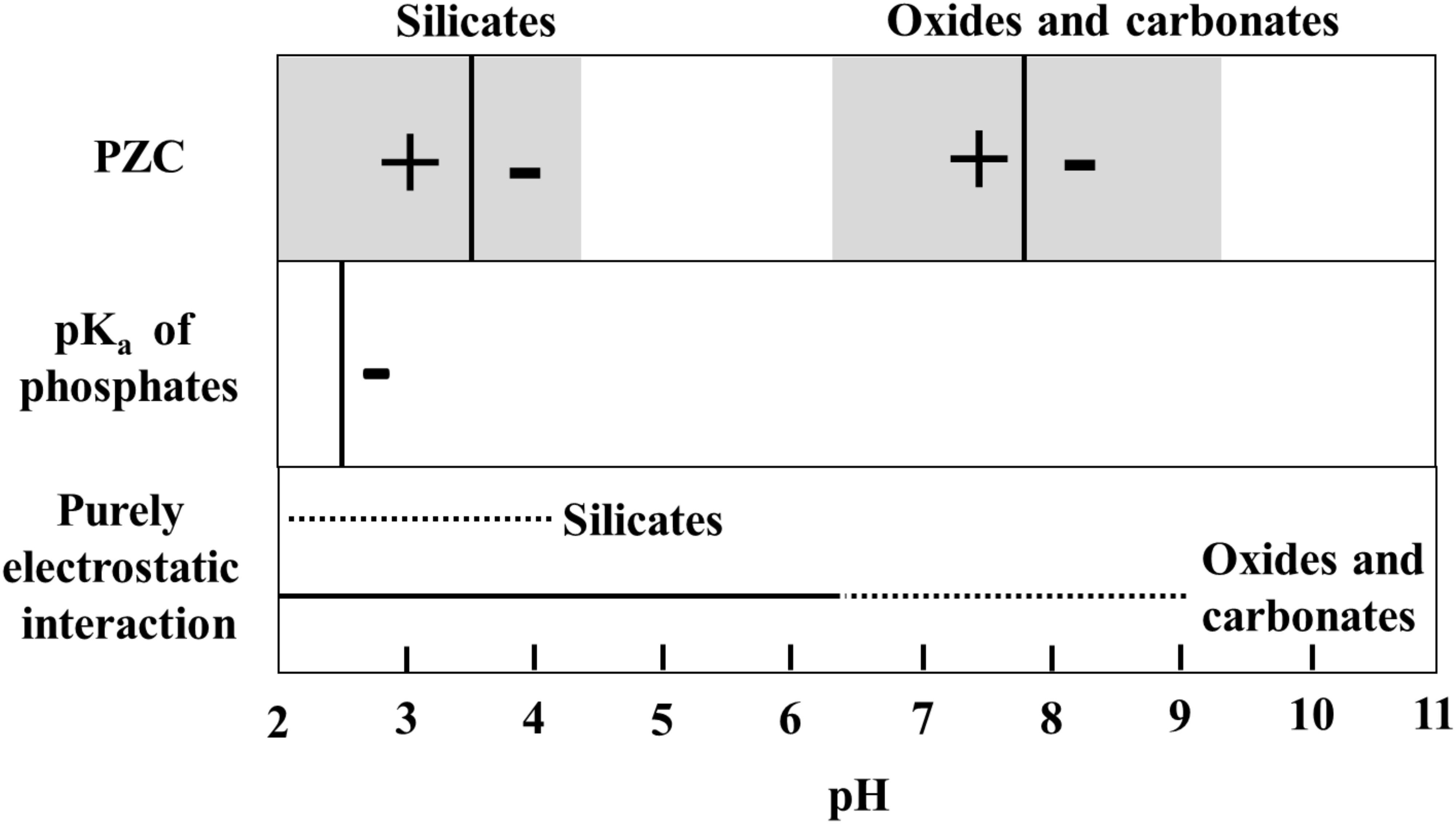
FIGURE 2. Schindler diagram showing the pH range where the interaction between minerals and phosphate groups of DNA can be purely electrostatic. For silicates (clay minerals, quartz and feldspars), the mode value of point of zero charge (PZC-black vertical line) is taken from Oelkers et al. (2009) and Kosmulski (2011) and for oxides and carbonates, from Kosmulski (2011). The gray shaded area in the PZC box represents a range of PZC’s for minerals in silicate, oxide or carbonate classes. Outside the range of purely electrostatic interaction, DNA adsorption will depend on aqueous ions and the formation of cation bridges.
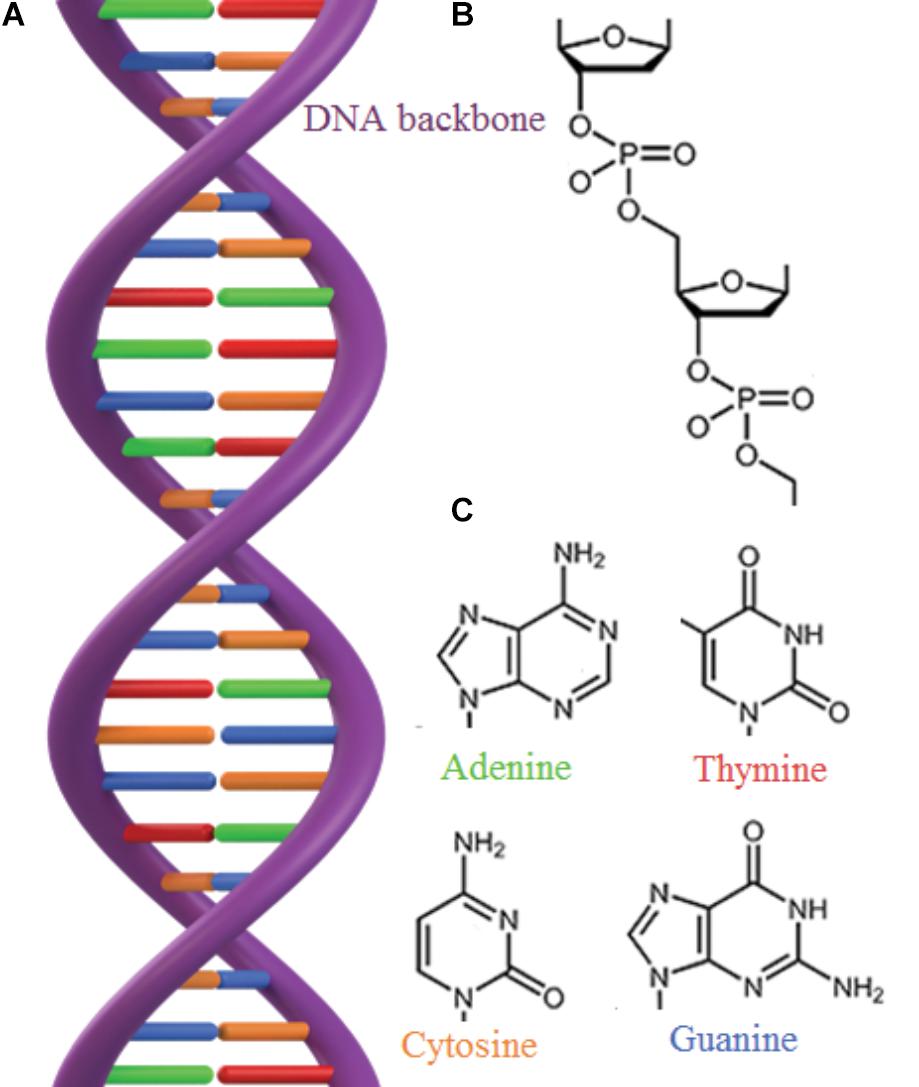
FIGURE 3. (A) The double stranded structure of DNA. (B) The DNA backbone consists of phosphate and sugar groups. (C) DNA contains 4 different nucleobases bound to the sugar ring: adenine, thymine, cytosine and guanine, that are bound in pairs through hydrogen bonds (H) which give rise to the double helix.
Dynamics of the Geological Environment
The dynamics of the environment is a result of a variety of geological events such as seawater transgressions and regressions, weathering rates as well as the acting local sedimentation processes. The latter includes distance to shore, climate zone, depth, ocean currents, energy levels etc. These dynamic processes can facilitate transport of the DNA-mineral association across time and space. Additionally, if the association is suspended rather than deposited on the seabed they can be distributed by smaller scale processes such as local currents. The rate of deposition is primarily determined by the retention time of the suspended particles. Retention times depend on the particle size and density (and the acting energy levels). The particles with dimensions of a few micrometers or less, i.e., the clay fraction (less than 0.002 mm), have the longest retention times and thus the higher potential to shuttle DNA across environments. The adsorption capacity of DNA depends on the surface area as well as the forces between DNA, the mineral and solution composition (as discussed in Section 2a). The clay fraction is enriched in clay minerals and iron oxyhydroxides which, beside their high surface areas, have the highest adsorption capacity for DNA (tens of micrograms of DNA per gram of mineral) (Lorenz and Wackernagel, 1994). In contrast, silt (0.002–0.05 mm) or sand (above 0.05 mm) sized particles typically have a hundred-fold lower capacity primarily because of a smaller surface area (Lorenz and Wackernagel, 1994) and electrostatic effects (DNA-mineral-solution interaction). The point is that minerals in the clay fraction such as clay minerals, iron oxides and hydroxides are more likely to meet suspended DNA, adsorb it and transport it to distant sedimentary environments than, e.g., sand fraction minerals. Considering the amount of DNA associated with minerals in the seabed, redistribution of those would increase the probability for HGT downstream as the DNA-mineral shuttles would be resuspended and be more accessible for microbes.
Near-Surface Mineralogy
In terms of the Earth’s near-surface mineralogy, clay minerals, oxides, hydroxides and carbonates represent a significant fraction of the mineral surfaces of suspended sediments in recent water columns (Van Der Gaast, 1991). However, on the early Earth, prior to the Great Oxidation Event (GOE), the mineralogy was less varied and marine suspended particles were likely dominated by sand fraction silicates produced by weathering which have shorter retention time compared to clay fraction.
Prior to the buildup of atmospheric oxygen ∼2.4–2.3 Ga ago (Anbar et al., 2007; Luo et al., 2016), the occurrence of oxides, hydroxides and clay minerals in sediments was mostly restricted to environments where physical erosion and aqueous weathering of (ultra)mafic and granitic rocks operated (Hazen et al., 2008). Well before the GOE, sedimentary iron oxides occurred in the form of the iron formations which are iron oxide rich rocks whose global and abundant occurrence is one of the signatures of the onset of the GOE. Iron formations were forming already ∼3.75 Ga ago (Rosing et al., 1996) as a consequence of the mantle plume breakout events (Isley and Abbott, 1999) and well after the GOE [for a thorough review see, e.g., Bekker et al. (2014)] but those deposits are rare and volumetrically restricted compared to their Neoarchean and Paleoproterozoic counterparts (Konhauser et al., 2017). Hence, their importance for the HGT was most likely negligible. Recently new evidence revealed a distinct terrestrial biosphere as early as ∼3 Ga ago (Rye and Holland, 2000; Stüeken et al., 2017; Homann et al., 2018; Stüeken and Buick, 2018) which would have influenced the weathering of continental masses and the resulting formation of secondary minerals. However, we still know little about this biosphere and its properties.
The only volumetrically important amounts of clay minerals in the oceans were most likely produced by serpentinization of oceanic crust around hydrothermal vents (Hazen et al., 2013). However, there would have been some amount of terigenic clay minerals that were transported to the early oceans by rivers or winds. These would have been suspended in the water column prior to sedimentation and could well have been acting as DNA carriers. Globally, the near-surface mineralogy of the Earth changed after the GOE (Folinsbee, 1982; Hazen et al., 2008) when about 2500 new minerals came to existence (Hazen et al., 2008). Iron oxyhydroxides such as ferrihydrite and hematite are particularly important products of the GOE because they precipitated in significant amounts from slightly oxygenated upper part of the ocean as a consequence of lower solubility of Fe3+ compared to the Fe2+. In addition to the higher abundance of clay fraction minerals, the proportion of clay minerals increased because of the associated onset of Fe3+-rich clay mineral formation on the continental shelf (Hazen et al., 2013) combined with the increased weathering from continents that started to change the composition from mafic to felsic at ∼3 Ga ago (Tang et al., 2016) at ∼3 Ga ago. We propose that the sudden abundance in numbers of these major DNA carriers shortly prior to (Anbar et al., 2007; Frei et al., 2009; Crowe et al., 2013) and following the GOE added to the success and magnitude of mineral facilitated HGT. During buildup of free O2, the emergence of new ecological niches was high and would have boosted the demand for new evolutionary traits and innovations. In contrast, the emerging oxic conditions most likely decreased the DNA longevity as it has been observed for the recent anoxic lake sediments (Coolen et al., 2002). Unfortunately, there are major gaps in current knowledge about the longevity of mineral bound DNA and a quantitative assessment of the interplay between these factors is a still-standing frontier that needs to be approached.
Genomic Evolution and O2 Buildup on Early Earth
Genomic research has identified an Archaean expansion (AE) as a period of intensive genetic innovations between 3.3–2.9 Ga ago that coincides with a rapid diversification of bacterial lineages (David and Alm, 2011). David and Alm (2011) generated a detailed evolutionary history for ∼4,000 major gene families where they account for events caused by HGT. Specifically, they compared individual gene phylogenies with the phylogeny of organisms (the “tree of life”). They show that gene histories reveal marked changes in the rates of gene birth, gene duplication, gene loss and HGT over geological timescales (Figure 4). Interestingly, during the decline in the genetic expansion there is an outburst of HGT driven evolution (Figure 4 arrow), which stabilizes at a maximum ∼2.7 Ga ago and remains relatively constant until present times. Genes born during the AE were found likely to be associated with an expansion in capabilities of microbial respiration and electron transfer capabilities, with an enrichment in oxygen-using genes toward the end of the expansion (David and Alm, 2011). In addition, genes arising after the expansion show increasing use of free oxygen in metabolic processes (David and Alm, 2011).
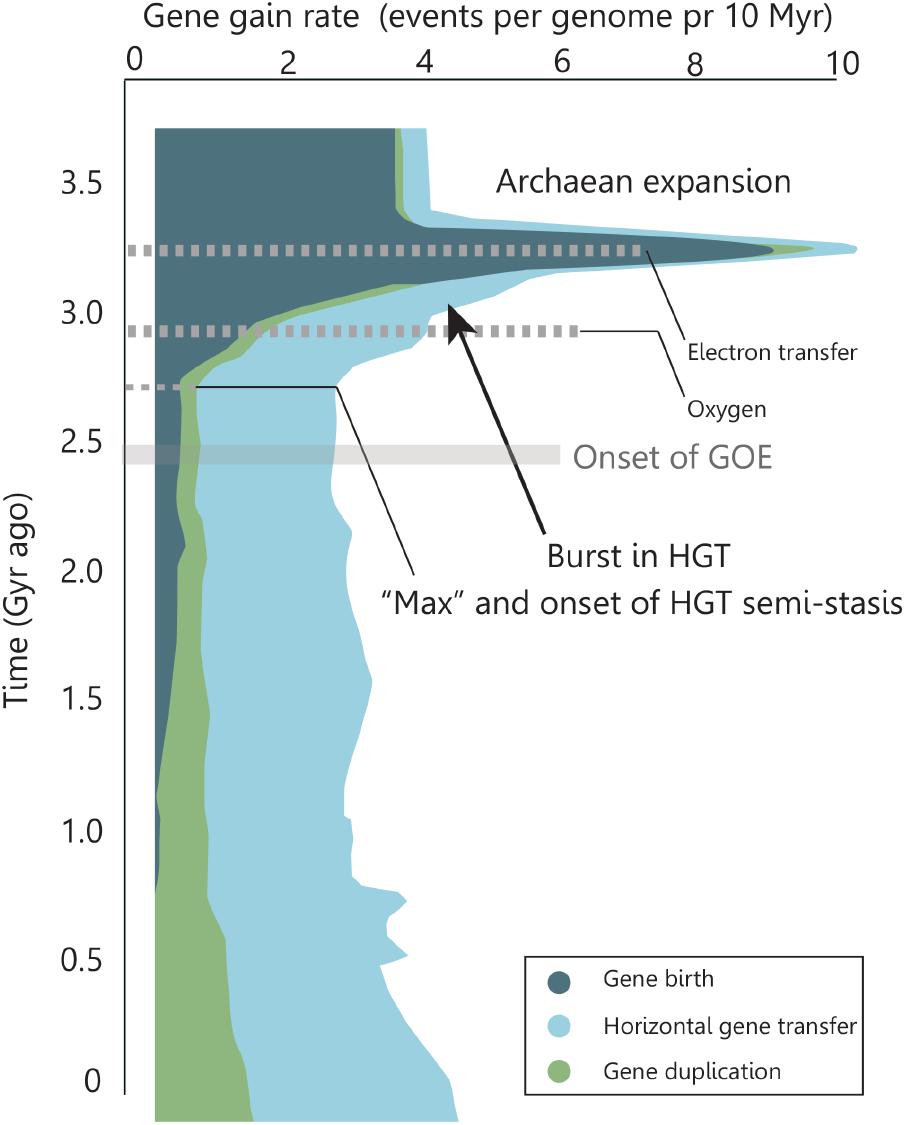
FIGURE 4. Rate of genomic macroevolutionary events over time. The Archaean expansion marks a rapid diversification of bacterial lineages. During the expansion, the metabolic complexities of respiratory pathways evolved to include electron transfers and, after the expansion, arising genes showed increasing use of molecular oxygen. At the decline of the expansion a significant amount of gene rates are caused by HGT. This contribution reaches a maximum and remains semi-constant until present time. Adapted by permission from Macmillan Publishers Ltd: Nature [Rapid evolutionary innovation during an Archaean genetic expansion, David and Alm (2011)].
The process of photosynthesis is a mosaic of various subprocesses (Hohmann-Marriott and Blankenship, 2011) and, according to the “fusion model” (Blankenship, 1992; Leonova et al., 2011), the evolution of photosynthetic organisms is complicated by insertion of genes that encode these reactions via HGT. The fusion model was recently supported by the discovery of Oxyphotobacteria that gained photosynthetic subprocesses through HGT (Shih et al., 2017; Soo et al., 2017). Oxygenic photosynthesis is the only significant source of free oxygen in the hydrosphere and atmosphere and the dominant metabolic pathway for life on Earth. Before the invention of photosynthesis, life was solely dependent upon chemical sources of reducing power such as hydrothermal activity and weathering (Lyons et al., 2014). Photosynthesis might have evolved prior to 3.8 Ga ago (Rosing and Frei, 2004) and evidence suggest whiffs of atmospheric oxygen (Anbar et al., 2007) occurring as early as 3 Ga ago (Crowe et al., 2013). This means that, at least locally, pockets of O2 were present in the Earth’s near surface environments as a product of sparse photosynthetic life prior to the build-up of appreciable amounts of free atmospheric O2. At that time, several processes have competed for the free O2 including oxidation of sulfides, reduced geothermal outflow and oxidation of organic matter as well as limited formation of oxides and hydroxides. We propose that mineral facilitated HGT have had a higher impact in suboxic compared to anoxic conditions, i.e., the combination of (i) increased local development of clay fraction minerals with associated high DNA adsorption capacities, (ii) the overall anoxic conditions (enhancing longevity) and (iii) the local whiffs of O2 would have been offering compelling sites for archiving DNA on mineral surfaces and a demand for innovations. Despite the steady levels of free atmospheric O2, recent data imply that for much of the Proterozoic Eon (2.5–0.5 Ga ago), Earth’s oceans were moderately oxic at the surface and still anoxic at depth (Canfield, 1998; Reinhard et al., 2013) which would have favored DNA preservation in the upper parts of the water column but beneficial in the lower parts where the majority of mineral bound DNA would end up.
We find it plausible that the variation in rates of macroevolutionary events caused by HGT during and post the AE (Figure 4) is related to the interdependent factors of increasing O2 levels, abundance of minerals with a high DNA adsorption capacity, geologic environments, and new opportune niches for innovations and DNA longevity. The initial rise of gene transfer events in the AE is dominated by gene births and the emerging role of HGT could well be caused by development of life, a higher frequency of DNA transfer combined with the need for evolutionary innovation. The subsequent rise and the following stasis of HGT contribution to the genomic evolution could represent a balance between the need for innovations, the development of oxic environments (decreasing preservation potential for free DNA) and the promoted formation of minerals which preserve the DNA (enhancing longevity) as well as abundance and higher adsorption capacities as determined by the local geology and O2 levels. Although, considering the present-day amount of DNA in sediments, we do not consider anoxic conditions to be as important condition for DNA longevity as is the stabilization of DNA at mineral surfaces and we suggest that the main agent controlling the longevity is the favorable mineral-DNA binding.
In the following, we illustrate the advocated framework for mineral facilitated HGT and its proposed impact on the evolution of early life. The relative timing of events and likely evolutionary order of the metabolic mechanisms are adapted from those proposed by David and Alm (2011) and accounted for in Figure 4. Because the genomic data from David and Alm (2011) are poorly geologically restrained and difficult to compare to the absolutely dated (pre)GOE events, we illustrate a qualitative and hypothetic scenario that offers a simple solution to observations on the relatively coinciding events of increasing HGT and changes in the near-surface mineralogy in Mesoarchean and Neoarchean. The hypothetic scenario is illustrated in Figure 5 and shows a mineral facilitated distribution of metabolic traits and the subsequent evolution that is supported by the fusion model (Hohmann-Marriott and Blankenship, 2011). The purpose of Figure 5 is to illustrate likely processes and the proposed significance of mineral facilitated HGT for the evolution of life.
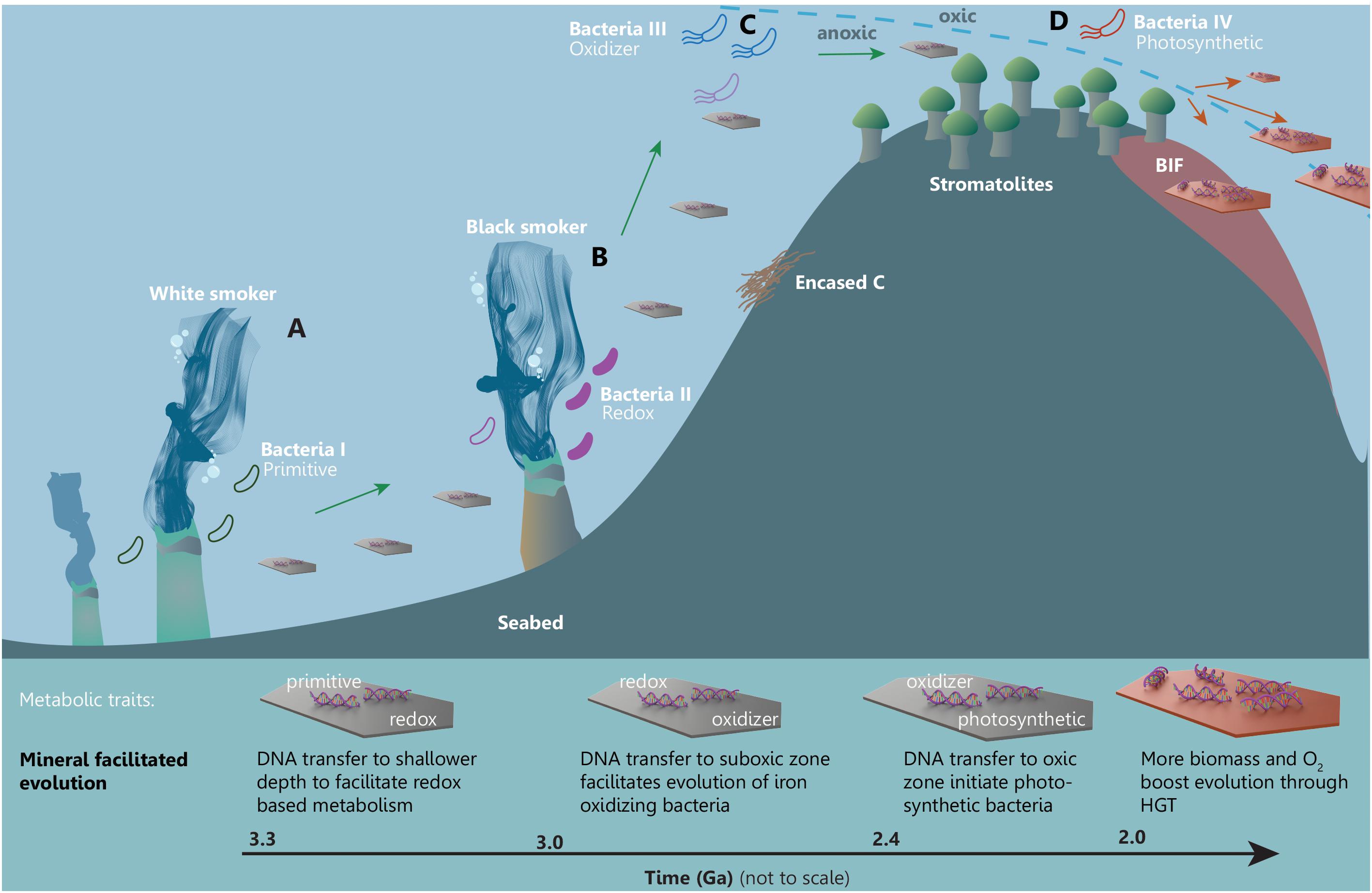
FIGURE 5. A simplified framework illustrating mineral facilitated HGT and proposed impact for the evolution of early life. Minerals are distributing adsorbed metabolic traits across time and space facilitating evolution of increasingly advanced metabolic pathways in a marine setting starting from (A) a white smoker environment through to (B) black smoker habitats, towards (C) the subphotic and finally (D) the photic zone.
Hypothetical Framework for Mineral Facilitated HGT and the Impact for Early Life
Likely candidates for the earliest habitable environments are anoxic submarine-hydrothermal vents (Dodd et al., 2017) such as the white smoker and the black smoker habitats in Figure 5, where heat and chemical gradients in aqueous solutions rich in hydrogen, methane, carbon dioxide and sulfur would have sustained early life with primitive metabolism. PreGOE white and black smokers had some of the largest deposits of, e.g., carbonates and serpentine group clay minerals. DNA from the microbes dwelling in these habitats were able to adsorb to carbonates through electrostatic interactions and to serpentines through ion bridging (Figure 2), which would protect the DNA and facilitate its transport to distant environments. In Figure 5, genes encoding a primitive metabolic pathway are carried from the white smoker (a) to a shallower black smoker habitat (b), which has a higher content of reduced Fe and S. The “newly” arrived DNA enables the evolution of electron transfer mechanism (e.g., chemolithotrophs). Minerals with adsorbed DNA that originated around the black smoker are subsequently carrying genes that encode electron transfer metabolism to a locally oxygenated pocket in the subphotic zone where early photosynthesis is occurring (Figure 5C). The suboxic environment and the new traits result in evolution of Fe-oxidizers such as microaerophilic Gallionella type filamentous bacteria. The bacteria oxidize Fe2+ precipitating iron oxyhydroxides on their filaments causing them to sink to the anoxic zone and cause drawdown of carbon (and DNA) which, in turn, enhances the buildup of free O2 (Lalonde et al., 2012). DNA from the Fe-oxidizing bacteria is carried toward shallower conditions into the photic zone where cyanobacterial mats, which produce stromatolites, are generating the buildup of free O2 (Figure 5D). Here, the new environment and the presence of both stromatolitic cyanobacteria and the DNA transported by minerals facilitate the evolution of new photosynthetic bacteria. At this point in time, the increasing amount of free O2 in the upper part of the ocean leads to precipitation of, e.g., banded iron formations and an increasing amount of clay minerals, oxides and hydroxides that can carry DNA. The new opportune conditions for life and innovations would have sparked a demand for new traits which could be propagated across time and space with the increased amount of DNA carriers. It is noteworthy to emphasize that the dynamic geologic processes such as marine regression or sea currents could facilitate the above presented scenario. E.g., currents could bring mineral bound DNA kept in sediments to completely new habitats or a marine regression could bring previously deep-sea sediments to shallower environments.
There are many imaginable scenarios for the impact and determining processes of mineral facilitated HGT on the evolution of life. Because of the major gaps in our understanding of the interplay between minerals and DNA, we cannot currently quantitatively predict the longevity of mineral associated DNA in various environmental conditions nor assess the impact on evolutionary events from the acting local sedimentation processes or geologic events (marine trans/regressions, tectonics etc.). Consequently, we need more information to pinpoint geochemical, phylogenetic, sedimentological or mineralogic signatures that could identify an event of mineral facilitated HGT in the geologic record. Regardless, the high rates of HGT over time since ∼3 Ga ago highlight the significance of HGT on evolutionary innovations and we find it timely to combine geologic and genomic efforts to address the contribution of minerals in the evolution of life. We advocate that the high amount of DNA associated with minerals can function as an archive for genes and evolutionary innovations which could be distributed across environments and timescales at a rate that depends on mineral assemblages, sedimentary processes and geochemical conditions. The subsequent effect in terms of species evolution would depend on the need for innovative adaptions and the availability of recipient organisms. Overall, we would encourage future studies to investigate the correlation between larger and smaller geologic events and the prokaryotic evolutionary outbursts. We find that the changes in the mineralogy of the geological setting would affect the longevity of mineral bound DNA and its availability for HGT. Mineral facilitated HGT holds a potential for a new principle for the evolution of life that calls for integrated studies of mineralogy, geology and evolutionary biology in a multidisciplinary effort.
Author Contributions
KS and SJ developed the hypothesis. KS wrote the manuscript. SJ participated in manuscript editing and approved of its submission.
Funding
KS received funding from the European Union’s Horizon 2020 research and innovation program under the Marie Skłodowska-Curie grant agreement No. 663830 and the Welsh Government and Higher Education Funding Council for Wales through the Sêr Cymru National Research Network for Low Carbon, Energy and Environment.
Conflict of Interest Statement
The authors declare that the research was conducted in the absence of any commercial or financial relationships that could be construed as a potential conflict of interest.
Acknowledgments
We sincerely thank Dr. Agnete Steenfelt and Anne Rath Nielsen for valuable comments on this manuscript, and Illustrations Elaborated for 3D models.
References
Aardema, B. W., Lorenz, M. G., and Krumbein, W. E. (1983). Protection of sediment-adsorbed transforming DNA against enzymatic inactivation. Appl. Environ. Microbiol. 46, 417–420.
Anbar, A. D., Duan, Y., Lyons, T. W., Arnold, G. L., Kendall, B., Creaser, R. A., et al. (2007). A whiff of oxygen before the great oxidation event? Science 317, 1903–1906. doi: 10.1126/science.1140325
Bekker, A., Planavsky, N. J., Krapež, B., Rasmussen, B., Hofmann, A., Slack, J. F., et al. (2014). “Iron formations: their origins and implications for ancient seawater chemistry”, in Treatise on Geochemistry, 2nd Edn, eds H. D. Holland and K. K. Turekian (Oxford: Elsevier), 561–628. doi: 10.1016/B978-0-08-095975-7.00719-1
Biondi, E., Furukawa, Y., Kawai, J., and Benner, S. A. (2017). Adsorption of RNA on mineral surfaces and mineral precipitates. Beilstein J. Org. Chem. 13, 393–404. doi: 10.3762/bjoc.13.42
Blankenship, R. E. (1992). Origin and early evolution of photosynthesis. Photosynth. Res. 33, 91–111. doi: 10.1007/BF00039173
Cai, P., Huang, Q., Lu, Y., Chen, W., Jiang, D., and Liang, W. (2007). Amplification of plasmid DNA bound on soil colloidal particles and clay minerals by the polymerase chain reaction. J. Environ. Sci. China 19, 1326–1329. doi: 10.1016/S1001-0742(07)60216-2
Cai, P., Zhu, J., Huang, Q., Fang, L., Liang, W., and Chen, W. (2009). Role of bacteria in the adsorption and binding of DNA on soil colloids and minerals. Colloids Surf. B Biointerfaces 69, 26–30. doi: 10.1016/j.colsurfb.2008.10.008
Canfield, D. E. (1998). A new model for Proterozoic ocean chemistry. Nature 396, 450–453. doi: 10.1038/24839
Canhisares-Filho, J. E., Carneiro, C. E., de Santana, H., Urbano, A., da Costa, A. C., Zaia, C. T., et al. (2015). Characterization of the adsorption of nucleic acid bases onto ferrihydrite via fourier transform infrared and surface-enhanced Raman spectroscopy and X-ray Diffractometry. Astrobiology 15, 728–738. doi: 10.1089/ast.2015.1309
Cao, Y., Wei, X., Cai, P., Huang, Q., Rong, X., and Liang, W. (2011). Preferential adsorption of extracellular polymeric substances from bacteria on clay minerals and iron oxide. Colloids Surf. B Biointerfaces 83, 122–127. doi: 10.1016/j.colsurfb.2010.11.018
Coolen, M. J. L., Cypionka, H., Sass, A. M., Sass, H., and Overmann, J. (2002). Ongoing modification of Mediterranean Pleistocene sapropels mediated by prokaryotes. Science 296, 2407–2410. doi: 10.1126/science.1071893
Corinaldesi, C., Beolchini, F., and Dell’anno, A. (2008). Damage and degradation rates of extracellular DNA in marine sediments: implications for the preservation of gene sequences. Mol. Ecol. 17, 3939–3951. doi: 10.1111/j.1365-294X.2008.03880.x
Crowe, S. A., Døssing, L. N., Beukes, N. J., Bau, M., Kruger, S. J., Frei, R., et al. (2013). Atmospheric oxygenation three billion years ago. Nature 501, 535–538. doi: 10.1038/nature12426
Danovaro, R., Corinaldesi, C., Luna, G. M., and Dell’Anno, A. (2005). “Molecular tools for the analysis of DNA in marine environments,” in Marine Organic Matter: Biomarkers, Isotopes and DNA, ed. J. K. Volkman (Berlin: Springer), 105–126. doi: 10.1007/698_2_004
David, L. A., and Alm, E. J. (2011). Rapid evolutionary innovation during an Archaean genetic expansion. Nature 469, 93–96. doi: 10.1038/nature09649
Dejean, T., Valentini, A., Duparc, A., Pellier-Cuit, S., Pompanon, F., Taberlet, P., et al. (2011). Persistence of Environmental DNA in Freshwater Ecosystems. PLoS One 6:e23398. doi: 10.1371/journal.pone.0023398
Dell’Anno, A., and Danovaro, R. (2005). Extracellular DNA plays a key role in deep-sea ecosystem functioning. Science 309:2179. doi: 10.1126/science.1117475
Demanèche, S., Jocteur-Monrozier, L., Quiquampoix, H., and Simonet, P. (2001). Evaluation of biological and physical protection against nuclease degradation of clay-bound plasmid DNA. Appl. Environ. Microbiol. 67, 293–299. doi: 10.1128/AEM.67.1.293-299.2001
Dodd, M. S., Papineau, D., Grenne, T., Slack, J. F., Rittner, M., Pirajno, F., et al. (2017). Evidence for early life in Earth’s oldest hydrothermal vent precipitates. Nature 543:nature21377. doi: 10.1038/nature21377
Doolittle, W. F. (1999). Lateral genomics. Trends Cell Biol. 9, M5–M8. doi: 10.1016/S0962-8924(99)01664-5
Feuillie, C., Daniel, I., Michot, L. J., and Pedreira-Segade, U. (2013). Adsorption of nucleotides onto Fe–Mg–Al rich swelling clays. Geochim. Cosmochim. Acta 120, 97–108. doi: 10.1016/j.gca.2013.06.021
Feuillie, C., Sverjensky, D. A., and Hazen, R. M. (2015). Attachment of Ribonucleotides on α-Alumina as a Function of pH, Ionic Strength, and Surface Loading. LANGMUIR 31, 240–248. doi: 10.1021/la504034k
Folinsbee, R. E. (1982). “Variations in the distribution of mineral deposits with time,” in Mineral Deposits and the Evolution of the Biosphere Dahlem Workshop Report, eds H. D. Holland and M. Schidlowski (Berlin: Springer), 219–236. doi: 10.1007/978-3-642-68463-0_12
Frei, R., Gaucher, C., Poulton, S. W., and Canfield, D. E. (2009). Fluctuations in Precambrian atmospheric oxygenation recorded by chromium isotopes. Nature 461, 250–253. doi: 10.1038/nature08266
Gogarten, J. P., Doolittle, W. F., and Lawrence, J. G. (2002). Prokaryotic evolution in light of gene transfer. Mol. Biol. Evol. 19, 2226–2238. doi: 10.1093/oxfordjournals.molbev.a004046
Gogarten, J. P., and Townsend, J. P. (2005). Horizontal gene transfer, genome innovation and evolution. Nat. Rev. Microbiol. 3, 679–687. doi: 10.1038/nrmicro1204
Greaves, M. P., and Wilson, M. J. (1969). The adsorption of nucleic acids by montmorillonite. Soil Biol. Biochem. 1, 317–323. doi: 10.1016/0038-0717(69)90014-5
Haile, J., Holdaway, R., Oliver, K., Bunce, M., Gilbert, M. T. P., Nielsen, R., et al. (2007). Ancient DNA chronology within sediment deposits: are paleobiological reconstructions possible and is DNA leaching a factor? Mol. Biol. Evol. 24, 982–989. doi: 10.1093/molbev/msm016
Hazen, R. M., Papineau, D., Bleeker, W., Downs, R. T., Ferry, J. M., McCoy, T. J., et al. (2008). Mineral evolution. Am. Mineral. 93, 1693–1720. doi: 10.2138/am.2008.2955
Hazen, R. M., Sverjensky, D. A., Azzolini, D., Bish, D. L., Elmore, S. C., Hinnov, L., et al. (2013). Clay mineral evolution. Am. Mineral. 98, 2007–2029. doi: 10.2138/am.2013.4425
Hebsgaard, M. B., Phillips, M. J., and Willerslev, E. (2005). Geologically ancient DNA: fact or artefact? Trends Microbiol. 13, 212–220. doi: 10.1016/j.tim.2005.03.010
Hohmann-Marriott, M. F., and Blankenship, R. E. (2011). Evolution of photosynthesis. Annu. Rev. Plant Biol. 62, 515–548. doi: 10.1146/annurev-arplant-042110-103811
Homann, M., Sansjofre, P., Zuilen, M. V., Heubeck, C., Gong, J., Killingsworth, B., et al. (2018). Microbial life and biogeochemical cycling on land 3,220 million years ago. Nat. Geosci. 11, 665–671. doi: 10.1038/s41561-018-0190-9
Isley, A. E., and Abbott, D. H. (1999). Plume-related mafic volcanism and the deposition of banded iron formation. J. Geophys. Res. 104, 15461–15477. doi: 10.1029/1999JB900066
Johnson, C. M., and Grossman, A. D. (2015). Integrative and conjugative elements (ICEs): what they do and how they work. Annu. Rev. Genet. 49, 577–601. doi: 10.1146/annurev-genet-112414-055018
Keeling, P. J., and Palmer, J. D. (2008). Horizontal gene transfer in eukaryotic evolution. Nat. Rev. Genet. 9, 605–618. doi: 10.1038/nrg2386
Khanna, M., and Stotzky, G. (1992). Transformation of Bacillus subtilis by DNA bound on montmorillonite and effect of DNase on the transforming ability of bound DNA. Appl. Environ. Microbiol. 58, 1930–1939.
Khanna, M., Yoder, M., Calamai, L., and Stotzky, G. (1998). X-ray diffractometry and electron microscopy of DNA from Bacillus subtilis bound on clay minerals. Sci. Soils 3, 1–10. doi: 10.1007/s10112-998-0001-3
Kipp, M. A., and Stüeken, E. E. (2017). Biomass recycling and Earth’s early phosphorus cycle. Sci. Adv. 3:eaao4795. doi: 10.1126/sciadv.aao4795
Konhauser, K. O., Planavsky, N. J., Hardisty, D. S., Robbins, L. J., Warchola, T. J., Haugaard, R., et al. (2017). Iron formations: a global record of Neoarchaean to Palaeoproterozoic environmental history. Earth Sci. Rev. 172, 140–177. doi: 10.1016/j.earscirev.2017.06.012
Koonin, E. V., Makarova, K. S., and Aravind, L. (2001). Horizontal gene transfer in prokaryotes: quantification and classification. Annu. Rev. Microbiol. 55, 709–742. doi: 10.1146/annurev.micro.55.1.709
Kosmulski, M. (2011). The pH-dependent surface charging and points of zero charge: V. Update. J. Colloid Interface Sci. 353, 1–15. doi: 10.1016/j.jcis.2010.08.023
Lalonde, K., Mucci, A., Ouellet, A., and Gélinas, Y. (2012). Preservation of organic matter in sediments promoted by iron. Nature 483, 198–200. doi: 10.1038/nature10855
Leonova, M. M., Fufina, T. Y., Vasilieva, L. G., and Shuvalov, V. A. (2011). Structure-function investigations of bacterial photosynthetic reaction centers. Biochem. Biokhimiia 76, 1465–1483. doi: 10.1134/S0006297911130074
Levy-Booth, D. J., Campbell, R. G., Gulden, R. H., Hart, M. M., Powell, J. R., Klironomos, J. N., et al. (2007). Cycling of extracellular DNA in the soil environment. Soil Biol. Biochem. 39, 2977–2991. doi: 10.1016/j.soilbio.2007.06.020
Lorenz, M. G., Aardema, B. W., and Krumbein, W. E. (1981). Interaction of marine sediment with DNA and DNA availability to nucleases. Mar. Biol. 64, 225–230. doi: 10.1007/BF00397113
Lorenz, M. G., and Wackernagel, W. (1987). Adsorption of DNA to sand and variable degradation rates of adsorbed DNA. Appl. Environ. Microbiol. 53, 2948–2952.
Lorenz, M. G., and Wackernagel, W. (1988). “Impact of mineral surfaces on gene transfer by transformation in natural bacterial environments,” in Risk Assessment for Deliberate Releases, ed. W. Klingmüller (Berlin: Springer), 110–119. doi: 10.1007/978-3-642-73419-9_13
Lorenz, M. G., and Wackernagel, W. (1994). Bacterial gene transfer by natural genetic transformation in the environment. Microbiol. Rev. 58, 563–602.
Lotareva, O. V., and Prozorov, A. A. (2000). Effect of the clay minerals montmorillonite and kaolinite on the genetic transformation of competent Bacillus subtilis cells. Microbiology 69, 571–574. doi: 10.1007/BF02756810
Lu, N., Zilles, J. L., and Nguyen, T. H. (2010). Adsorption of extracellular chromosomal DNA and its effects on natural transformation of Azotobacter vinelandii. Appl. Environ. Microbiol. 76, 4179–4184. doi: 10.1128/AEM.00193-10
Luo, G., Ono, S., Beukes, N. J., Wang, D. T., Xie, S., and Summons, R. E. (2016). Rapid oxygenation of Earth’s atmosphere 2.33 billion years ago. Sci. Adv. 2:e1600134. doi: 10.1126/sciadv.1600134
Lyons, T. W., Reinhard, C. T., and Planavsky, N. J. (2014). The rise of oxygen in Earth/’s early ocean and atmosphere. Nature 506, 307–315. doi: 10.1038/nature13068
Maity, S., Zanuy, D., Razvag, Y., Das, P., Alemán, C., and Reches, M. (2015). Elucidating the mechanism of interaction between peptides and inorganic surfaces. Phys. Chem. Chem. Phys. 17, 15305–15315. doi: 10.1039/C5CP00088B
Martiny, J. B. H., Bohannan, B. J. M., Brown, J. H., Colwell, R. K., Fuhrman, J. A., Green, J. L., et al. (2006). Microbial biogeography: putting microorganisms on the map. Nat. Rev. Microbiol. 4, 102–112. doi: 10.1038/nrmicro1341
Michalkova, A., Robinson, T. L., and Leszczynski, J. (2011). Adsorption of thymine and uracil on 1:1 clay mineral surfaces: comprehensive ab initio study on influence of sodium cation and water. Phys. Chem. Chem. Phys. 13, 7862–7881. doi: 10.1039/C1CP00008J
Nelson, K. E., Clayton, R. A., Gill, S. R., Gwinn, M. L., Dodson, R. J., Haft, D. H., et al. (1999). Evidence for lateral gene transfer between Archaea and bacteria from genome sequence of Thermotoga maritima. Nature 399, 323–329. doi: 10.1038/20601
Nguyen, T. H., and Chen, K. L. (2007). Role of divalent cations in plasmid DNA adsorption to natural organic matter-coated silica surface. Environ. Sci. Technol. 41, 5370–5375. doi: 10.1021/es070425m
Ochman, H., Lawrence, J. G., and Groisman, E. A. (2000). Lateral gene transfer and the nature of bacterial innovation. Nature 405, 299–304. doi: 10.1038/35012500
Oelkers, E. H., Golubev, S. V., Chairat, C., Pokrovsky, O. S., and Schott, J. (2009). The surface chemistry of multi-oxide silicates. Geochim. Cosmochim. Acta 73, 4617–4634. doi: 10.1016/j.gca.2009.05.028
O’Malley, M. A., and Boucher, Y. (2005). Paradigm change in evolutionary microbiology. Stud. Hist. Philos. Sci. Part C Stud. Hist. Philos. Biol. Biomed. Sci. 36, 183–208. doi: 10.1016/j.shpsc.2004.12.002
Overballe-Petersen, S., Harms, K., Orlando, L. A. A., Mayar, J. V. M., Rasmussen, S., Dahl, T. W., et al. (2013). Bacterial natural transformation by highly fragmented and damaged DNA. Proc. Natl. Acad. Sci. U.S.A. 110, 19860–19865. doi: 10.1073/pnas.1315278110
Pedreira-Segade, U., Feuillie, C., Pelletier, M., Michot, L. J., and Daniel, I. (2016). Adsorption of nucleotides onto ferromagnesian phyllosilicates: significance for the origin of life. Geochim. Cosmochim. Acta 176, 81–95. doi: 10.1016/j.gca.2015.12.025
Pietramellara, G., Ascher, J., Borgogni, F., Ceccherini, M. T., Guerri, G., and Nannipieri, P. (2009). Extracellular DNA in soil and sediment: fate and ecological relevance. Biol. Fertil. Soils 45, 219–235. doi: 10.1007/s00374-008-0345-8
Puigbò, P., Lobkovsky, A. E., Kristensen, D. M., Wolf, Y. I., and Koonin, E. V. (2014). Genomes in turmoil: quantification of genome dynamics in prokaryote supergenomes. BMC Biol. 12:66. doi: 10.1186/s12915-014-0066-4
Puigbò, P., Wolf, Y. I., and Koonin, E. V. (2013). Seeing the tree of life behind the phylogenetic forest. BMC Biol. 11:46. doi: 10.1186/1741-7007-11-46
Reinhard, C. T., Planavsky, N. J., Robbins, L. J., Partin, C. A., Gill, B. C., Lalonde, S. V., et al. (2013). Proterozoic ocean redox and biogeochemical stasis. Proc. Natl. Acad. Sci. U.S.A. 110, 5357–5362. doi: 10.1073/pnas.1208622110
Romanowski, G., Lorenz, M. G., and Wackernagel, W. (1991). Adsorption of plasmid DNA to mineral surfaces and protection against DNase I. Appl. Environ. Microbiol. 57, 1057–1061.
Rosing, M. T., and Frei, R. (2004). U-rich Archaean sea-floor sediments from Greenland – indications of > 3700 Ma oxygenic photosynthesis. Earth Planet. Sci. Lett. 217, 237–244. doi: 10.1016/S0012-821X(03)00609-5
Rosing, M. T., Rose, N. M., Bridgwater, D., and Thomsen, H. S. (1996). Earliest part of Earth’s stratigraphic record: a reappraisal of the > 3.7 Ga Isua (Greenland) supracrustal sequence. Geology 24, 43–46. doi: 10.1130/0091-7613 (1996)024<0043:EPOESS>2.3.CO;2
Rye, R., and Holland, H. D. (2000). Life associated with a 2.76 Ga ephemeral pond? evidence from Mount Roe #2 paleosol. Geology 28, 483–486. doi: 10.1130/0091-7613 (2000)28<483:LAWAGE>2.0.CO;2
Saeki, K., Sakai, M., and Wada, S.-I. (2010). DNA adsorption on synthetic and natural allophanes. Appl. Clay Sci. 50, 493–497. doi: 10.1016/j.clay.2010.09.015
Shih, P. M., Hemp, J., Ward, L. M., Matzke, N. J., and Fischer, W. W. (2017). Crown group Oxyphotobacteria postdate the rise of oxygen. Geobiology 15, 19–29. doi: 10.1111/gbi.12200
Slon, V., Hopfe, C., Weiß, C. L., Mafessoni, F., Rasilla, M., de la Lalueza-Fox, C., et al. (2017). Neandertal and Denisovan DNA from Pleistocene sediments. Science 356, 605–608. doi: 10.1126/science.aam9695
Soo, R. M., Hemp, J., Parks, D. H., Fischer, W. W., and Hugenholtz, P. (2017). On the origins of oxygenic photosynthesis and aerobic respiration in Cyanobacteria. Science 355, 1436–1440. doi: 10.1126/science.aal3794
Stocker, R. (2012). Marine microbes see a sea of gradients. Science 338, 628–633. doi: 10.1126/science.1208929
Stüeken, E. E., and Buick, R. (2018). Environmental control on microbial diversification and methane production in the Mesoarchean. Precambrian Res. 304, 64–72. doi: 10.1016/j.precamres.2017.11.003
Stüeken, E. E., Buick, R., Anderson, R. E., Baross, J. A., Planavsky, N. J., and Lyons, T. W. (2017). Environmental niches and metabolic diversity in Neoarchean lakes. Geobiology 15, 767–783. doi: 10.1111/gbi.12251
Takeuchi, N., Kaneko, K., and Koonin, E. V. (2014). Horizontal gene transfer can rescue prokaryotes from Muller’s Ratchet: benefit of DNA from dead cells and population subdivision. G3 Genes Genomes Genet. 4, 325–339. doi: 10.1534/g3.113.009845
Tang, M., Chen, K., and Rudnick, R. L. (2016). Archean upper crust transition from mafic to felsic marks the onset of plate tectonics. Science 351, 372–375. doi: 10.1126/science.aad5513
Thomas, C. M., and Nielsen, K. M. (2005). Mechanisms of, and barriers to, horizontal gene transfer between bacteria. Nat. Rev. Microbiol. 3, 711–721. doi: 10.1038/nrmicro1234
Thomsen, P. F., Kielgast, J., Iversen, L. L., Møller, P. R., Rasmussen, M., and Willerslev, E. (2012a). Detection of a diverse marine fish fauna using environmental DNA from seawater samples. PLoS One 7:e41732. doi: 10.1371/journal.pone.0041732
Thomsen, P. F., Kielgast, J., Iversen, L. L., Wiuf, C., Rasmussen, M., Gilbert, M. T. P., et al. (2012b). Monitoring endangered freshwater biodiversity using environmental DNA. Mol. Ecol. 21, 2565–2573. doi: 10.1111/j.1365-294X.2011.05418.x
Van Der Gaast, S. (1991). “Mineralogical analysis of marine particles by X-Ray powder diffraction,” in Marine Particles: Analysis and Characterization, eds D. C. Hurd and D. W. Spencer (Washington, DC: American Geophysical Union), 343–362. doi: 10.1029/GM063p0343
Vuillemin, A., Horn, F., Alawi, M., Henny, C., Wagner, D., Crowe, S. A., et al. (2017). Preservation and Significance of Extracellular DNA in Ferruginous Sediments from Lake Towuti, Indonesia. Front. Microbiol. 8:1440. doi: 10.3389/fmicb.2017.01440
Willerslev, E., Hansen, A. J., Binladen, J., Brand, T. B., Gilbert, M. T. P., Shapiro, B., et al. (2003). Diverse plant and animal genetic records from holocene and pleistocene sediments. Science 300, 791–795. doi: 10.1126/science.1084114
Keywords: nucleic acid, lateral gene transfer, metagenomics, mineral-DNA interaction, mineral evolution, evolution of life, great oxidation event
Citation: Sand KK and Jelavić S (2018) Mineral Facilitated Horizontal Gene Transfer: A New Principle for Evolution of Life? Front. Microbiol. 9:2217. doi: 10.3389/fmicb.2018.02217
Received: 18 April 2018; Accepted: 30 August 2018;
Published: 25 September 2018.
Edited by:
Martin G. Klotz, Washington State University Tri-Cities, United StatesReviewed by:
Eric Boyd, Montana State University, United StatesEva Stueeken, University of St Andrews, United Kingdom
Copyright © 2018 Sand and Jelavić. This is an open-access article distributed under the terms of the Creative Commons Attribution License (CC BY). The use, distribution or reproduction in other forums is permitted, provided the original author(s) and the copyright owner(s) are credited and that the original publication in this journal is cited, in accordance with accepted academic practice. No use, distribution or reproduction is permitted which does not comply with these terms.
*Correspondence: Karina Krarup Sand, a2FzODRAYWJlci5hYy51aw==