- 1INRA, ISA, EA 7394-ICV Institut Charles Viollette, Université Lille, Université d’Artois, Université Littoral Côte d’Opale, Lille, France
- 2VF Bioscience, Parc Eurasanté, Loos-lez-Lille, France
To compensate for their amino acid auxotrophy, lactobacilli have developed the ability to hydrolyze proteins present in their environment. This proteolytic activity not only generates the free amino acids needed by the bacteria, but also a large variety of peptides, some of which are endowed with biological activities. These so-called “bioactive peptides” (BAPs) are interesting from a nutrition and healthcare perspective. The use of lactic acid bacteria (LAB) such as lactobacilli is an effective strategy for production and valorization of new BAPs. The proteolytic activity of lactobacilli is exerted in a strain- and species-dependent manner: each species exhibits different proteinase content, leading to a large variety of proteolytic activities. This underlines the high potential of Lactobacillus strains to produce novel hydrolysates and BAPs of major interest. This review aims at discussing the potential of different Lactobacillus species to release BAPs from fermentation media and processes. Strategies used for peptide production are presented. Additionally, we propose a methodology to select the most promising Lactobacillus strains as sources of BAPs. This methodology combines conventional approaches and in silico analyses.
Introduction
It is now recognized that diet plays a key role in the maintenance of our health status. More and more research projects aim at determining how diet could improve or restore health (Cornier et al., 2008) and a new discipline, Foodomics, was defined in 2009 as “a discipline that studies the Food and Nutrition domains through the application of advanced Omics technologies to improve consumer’s well-being, health and confidence” (Cifuentes, 2009; Braconi et al., 2017). Foodomics could, for instance, help identify approaches to reduce the prevalence of metabolic syndrome (O’Neill and O’Driscoll, 2015; Chen et al., 2018), which is defined as a cluster of symptoms (high blood pressure, high blood sugar, overweight, hyperlipidemia) ultimately increasing the risk of diabetes, stroke, cardiovascular diseases, or cancers (Cameron et al., 2004; Wilson et al., 2005; Cornier et al., 2008; Kassi et al., 2011).
Food proteins are currently being studied beyond their nutritional characteristics, for their positive impact on human health related to specific sequences encrypted into the native protein, called bioactive peptides (BAPs). Such sequences are inactive when present in the parental protein, but can be released after protein hydrolysis during gastro-intestinal digestion (GID), in vitro enzymatic hydrolysis, or microbial fermentation (Korhonen and Pihlanto, 2006; Korhonen, 2009). The BAPs display interesting biological functions such as angiotensin converting enzyme (ACE) inhibition, mineral binding, antidiabetic, satiating, immunomodulating, opioid, antioxidant, or antimicrobial activities (Nongonierma and FitzGerald, 2015; Park and Nam, 2015; Caron et al., 2017; Nielsen et al., 2017; Domenger et al., 2018). Research on BAPs provides insightful information on the impact of dietary proteins on health. Furthermore, industrial applications are emerging such as the production of functional foods (Korhonen and Pihlanto, 2006; Pihlanto, 2013; Hafeez et al., 2014) or the production of peptides to serve as active ingredients for pharmaceuticals or dietary supplement products.
Challenges for production and commercialization of BAPs include: (i) the identification and isolation of new BAPs, (ii) the elucidation of the mechanisms of action involved in their bioactivities, and (iii) the development of efficient bioprocesses for peptide production (Agyei et al., 2016; Giacometti and Buretić-Tomljanović, 2017; Nongonierma and FitzGerald, 2017b). Research on BAPs is progressing. The use of in silico methodologies facilitates the discovery and identification of new peptides (Sánchez-Rivera et al., 2014; Iwaniak et al., 2015). However, the industrial production of BAPs is still limited by the lack of suitable large-scale technologies and the high cost of enzymes used for protein hydrolysis (Wu et al., 2013).
Microbial fermentation represents a cost-effective method for BAP production (Daliri et al., 2017), already widely applied in the dairy industry for the functionalization of milk products and byproducts (Pihlanto, 2013; Hafeez et al., 2014). Fermented dairy products claiming functional health properties associated with BAPs already exist. Most of these products are obtained using LABs belonging to the Lactobacillus genus (Korhonen and Pihlanto, 2006; Hafeez et al., 2014).
The LAB constitute a diverse group of Gram-positive, catalase-negative bacteria producing lactic acid as the main end-product of carbohydrate fermentation (Felis and Dellaglio, 2007). With more than 231 valid species and 29 subspecies, Lactobacillus genus is certainly the main and most diverse LAB group. Lactobacilli are bacteria of major industrial interest, as it is used for the production of fermented foods (dairy, meat, or vegetable products), food biopreservation, or probiotic applications (Stefanovic et al., 2017). However, they are considered as fastidious microorganisms because of their auxotrophy for numerous amino acids. In order to find the amino acids required for their growth, lactobacilli hydrolyze proteins in their environment through their proteolytic system, and, more specifically, through the action of enzymes called cell envelope proteinases (CEPs). This leads to the release of peptides and free amino acids in the fermentation media (Savijoki et al., 2006). Besides contributing to changes in the organoleptic properties of the fermented product, these released peptides can display various biological activities (Hafeez et al., 2014). Numerous studies reported the presence of BAPs in ripened cheeses (Gouda, Cheddar, Swiss cheese varieties, Manchego) (Pihlanto, 2013) or in yogurts (Jin et al., 2016; Rutella et al., 2016). The most meaningful examples of BAPs produced by Lactobacillus species are the antihypertensive tripeptides, Ile-Pro-Pro and Val-Pro-Pro (also called lactotripeptides), generated from casein hydrolysis by different Lb. helveticus strains (Pihlanto, 2013). Fermented milk products containing antihypertensive BAPs produced by Lactobacillus strains are already being marketed (Korhonen and Pihlanto, 2006; Hafeez et al., 2014).
Lactobacilli are isolated from different ecological niches. Various studies have described large differences between species or even strains, at the genetic or physiological levels (Hutkins, 2006; Sun et al., 2015); it is established that different strains belonging to the same species can differently hydrolyze proteins (Jensen et al., 2009). This not only supports the idea that lactobacilli can produce a large variety of BAPs, but also highlights the need to carefully select the strains that will be used for BAP production.
This review describes the proteolytic system of Lactobacillus species, focusing on the aptitude of these microorganisms to hydrolyze dietary proteins in order to produce novel BAPs. A method for selection of BAP-producing Lactobacillus strains is proposed, based on a combination of conventional and in silico approaches. The strategies for BAP production using Lactobacillus species are presented, including (i) fermentation and functionalization of dietary products, (ii) extraction and purification of hydrolysates or BAPs, and (iii) use of purified CEPs. The advantages and drawbacks of each strategy are discussed. Finally, the current limitations and future challenges for BAP production by lactobacilli are discussed.
The Proteolytic System of Lactobacillus Species and Its Potential to Produce a Broad Variety of Peptides
Lactobacillus species are auxotrophic for numerous amino acids. An external source of nitrogen is required for their growth, particularly in milk where concentrations of amino acids are low. To fulfill their nitrogen requirement, Lactobacillus species have developed a proteolytic system, which hydrolyzes the proteins and supplies the amino acids. This system is composed of three major components (Figure 1A). Protein hydrolysis is initiated by CEPs that cleave the proteins into peptides ranging from 4 to 30 amino acids. As demonstrated by gene deletion experiments, CEPs are required for the strain’s growth in milk (Courtin et al., 2002). Peptides generated by CEP cleavage, released in the extracellular media, are then internalized by different transporters such as the oligopeptide permease (Opp), the ion-linked transporter (DtpT) for di- and tripeptides, and the ABC transporter (Dpp) for peptides containing 2 to 9 amino acid residues. Subsequently, the internalized peptides are degraded into amino acids by the combined action of numerous internal peptidases such as endopeptidases (PepO, PepF, PepE, and PepG), aminopeptidases (PepN, PepC, PepS, PepA, and PepL), tripeptidases (PepT), dipeptidases (PepD and PepV), and proline-specific peptidases (PepQ, PepI, PepR, PepX, and PepP) (for reviews see Savijoki et al., 2006; Sadat-Mekmene et al., 2011a; Griffiths and Tellez, 2013).
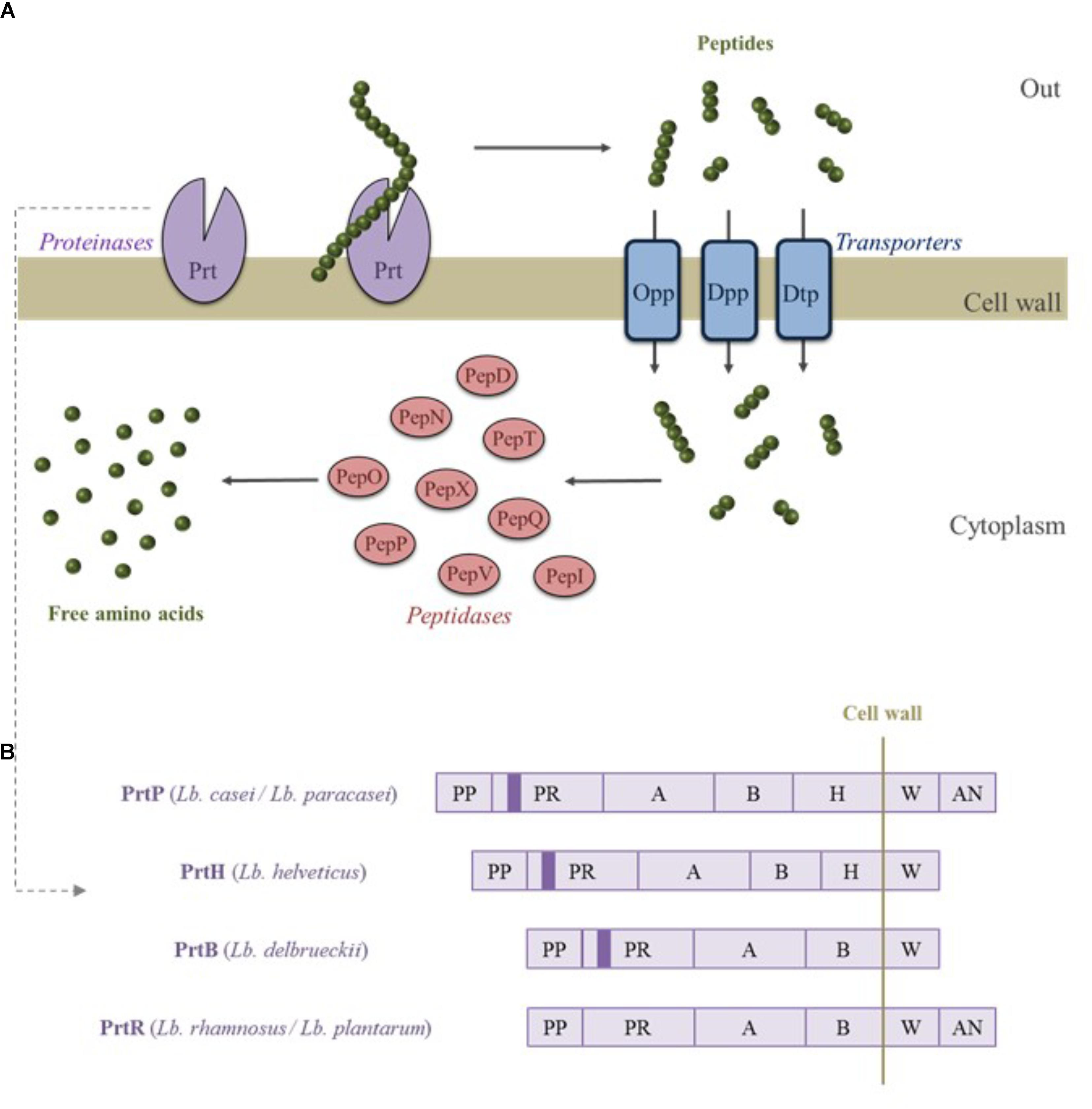
FIGURE 1. Schematic representation of the proteolytic system of Lactobacillus spp. (A). Protein hydrolysis is initiated by cell envelope proteinases (CEPs and Prt) and the resulting peptides are then transported inside the cell. Peptides are finally transformed into free amino acids by different peptidases. Diagrammatic representation of CEPs from different Lactobacillus species (B). PP, pro domain; PR, catalytic domain; A, A-domain; B, B-domain; H, helical domain; W, cell wall spacer domain; AN, membrane anchor domain. The plain color in the catalytic domain represent the insertion I domain. Adapted from Sadat-Mekmene et al. (2011a).
The CEPs are serine proteinases that belong to the subtilisin family. These enzymes are synthesized as preproteins of 2,000 amino acids and composed of 7 functional domains (Figure 1B). From the N terminus side, CEPs include the protein pro domain (PP), the catalytic domain (PR), an insertion domain (I) that possibly modulates substrate specificity, the domain A (unknown function), the domain B probably involved in CEP stabilization, the helix domain (H) that keeps the CEPs outside the cell, and a hydrophilic domain (W) (cell wall spacer or cell wall binding domain) (Savijoki et al., 2006). An anchor domain (AN) is present at the C-terminus of the protein except for the CEPs of Lb. helveticus and Lb. delbrueckii subsp. bulgaricus species (Griffiths and Tellez, 2013).
Four different CEPs from Lactobacillus species have so far been characterized. They include PrtB from Lb. delbrueckii subsp. bulgaricus (Hou et al., 2015), PrtP from Lb. casei and Lb. paracasei (Kojic et al., 1991; Ikram-ul-Haq and Mukhtar, 2006; Alcántara et al., 2016), PrtR from Lb. rhamnosus and Lb. plantarum (Vukotić, 2016), and PrtH from Lb. helveticus (Pederson et al., 1999; Griffiths and Tellez, 2013). While most Lactobacillus species possess only one CEP, the presence of four different paralogs of PrtH (called PrtH1, PrtH2, PrtH3, and PrtH4) has been observed in Lb. helveticus and their distribution is strain-dependent (Broadbent et al., 2011; Miyamoto et al., 2015). The presence of several CEP paralogs, exhibiting different specificities, make Lb. helveticus the most proteolytic species among the Lactobacillus genus and obviously the most efficient at generating a great diversity of BAPs (Genay et al., 2009; Stefanovic et al., 2017). Moreover, the proteolytic system of Lb. helveticus is the most studied due to its wide utilization for the production of dairy products, mainly cheese and fermented milk (Broadbent et al., 2011).
Proteolytic activities and protein hydrolysis patterns differ widely from one strain to another (Jensen et al., 2009). This is probably due to multiple factors like differences in CEP gene expression, CEP gene mutations, differences in optimal conditions for enzymatic activity, although the methodology used to assess the hydrolysis pattern of CEPs may also somewhat introduce variability in the reported results (Sadat-Mekmene et al., 2011a; Patel and Hati, 2018). In the case of Lb. helveticus strains, the genetic diversity of CEPs explains at least in part the differences in protein hydrolysis patterns (Sadat-Mekmene et al., 2011b). Broadbent et al. (2011) reported that among 51 strains of Lb. helveticus of dairy origin, 6, 4, 21, and 20 of them possessed 4, 3, 2, or 1 CEP paralogs, respectively. The predominant paralog was PrtH3. Miyamoto et al. (2015) studied 6 strains isolated in Mongolia and reported different protein hydrolysis patterns than in strains isolated in European or North American countries. The only paralog detected in the 6 Mongolian strains was PrtH1. Hydrolysis pattern differences were also observed between different Lb. delbrueckii subsp. bulgaricus strains (Oberg et al., 2002; Oommen et al., 2002), although this species is known to possess only one CEP (PrtB).
As expected, the differences in proteolytic activities and protein hydrolysis patterns are even more visible when comparing different Lactobacillus species. The caseinolytic specificities of Lb. helveticus strains and Lb. delbrueckii subsp. lactis CRL581 strain are different (Hebert et al., 2008). A similar observation was made when comparing 14 strains of Lb. delbrueckii subsp. bulgaricus with 8 strains of Lb. helveticus (Oberg et al., 2002).
All these results show that Lactobacillus strains have different hydrolytic specificities for proteins and consequently may release very different BAPs. Some investigations are still needed to fully appreciate the diversity in CEP activities. A recent comparative genomic study of 213 Lactobacillus genomes allowed the detection of 60 different genes presenting significant homology with the currently known CEP genes (amino acid identities ranging from 100 to 20%). This high level of divergence shows the potential of Lactobacillus species to generate a large variety of different peptides (Sun et al., 2015).
Another source of BAP diversity is the type of protein used as substrate by the bacteria. Lactobacilli can indeed hydrolyze different types of proteins and BAP release could be tightly matrix dependent (Ayyash et al., 2017). Overall, Lactobacillus strains are mostly used for milk fermentation and most of the BAPs characterized so far were isolated from milk cultures (Dziuba and Dziuba, 2014). However, even milk proteins are not identical, and the same Lactobacillus strain will generate different peptides when hydrolysing caseins from cow milk, goat milk, camel milk, or mare milk. Moreover, Lactobacillus strains are used to hydrolyze proteins from other sources than milk, such as fish (Yang et al., 2016) or plants (Rizzello et al., 2017; Singh and Vij, 2017), and this can also lead to the release of specific BAPs. For example, fermentation of pea seed by Lb. plantarum 299v leads to the release of ACE inhibitor peptides (Jakubczyk et al., 2013).
In conclusion, Lactobacillus species clearly present a great potential as producers of new BAPs, due to (i) the presence of different CEPs, with species- and strain-specific activities, able to generate a large variety of peptides of different molecular weights and sequences and (ii) their capacity to hydrolyze different types of proteins. Studying CEP activities and specificities will lead to a better understanding of the health potential of dietary proteins.
Selection of BAP-Producing Lactobacillus Strains
The basic strategy for the production and the characterization of new BAPs consists in the selection of a substrate and a proteolytic enzyme or microbial/bacterial strain, followed by hydrolysis under specific conditions (Korhonen, 2009). The resulting hydrolysate is assessed in vitro for biological activity and identification of BAPs. However, this strategy presents some limitations such as the empirical selection of substrate and enzyme as well as a lack of standardized protocols for the in vitro assessment of the biological activities. Recently, the development of dedicated software and computational tools has led to the development of more targeted/specific strategies, offering cost-effective and time-saving alternatives to conventional approaches (Sánchez-Rivera et al., 2014; Nongonierma and FitzGerald, 2017b). These approaches seem reasonably accurate, at least when predicting the outcome of hydrolysis by purified enzymes (Iwaniak et al., 2015). For example, PeptideCutter software (Gasteiger et al., 2005) is used to mimic protein/peptide hydrolysis and predict the sequence of the released peptides. Such predictions can help selecting the appropriate enzyme or protein source to generate specific BAPs (Carrasco-Castilla et al., 2012).
Regarding the production of BAPs using Lactobacillus species, the inter- and intra-genus variability of proteolytic activity and specificity make strain selection difficult. A methodology for selection of BAP-producing Lactobacillus strains is proposed (Figure 2). This selection procedure combines the use of a conventional approach with targeted strategies based on in silico analysis that were so far mostly used for BAPs produced by purified enzymes or gastro-intestinal enzymes (Nongonierma and FitzGerald, 2017a).
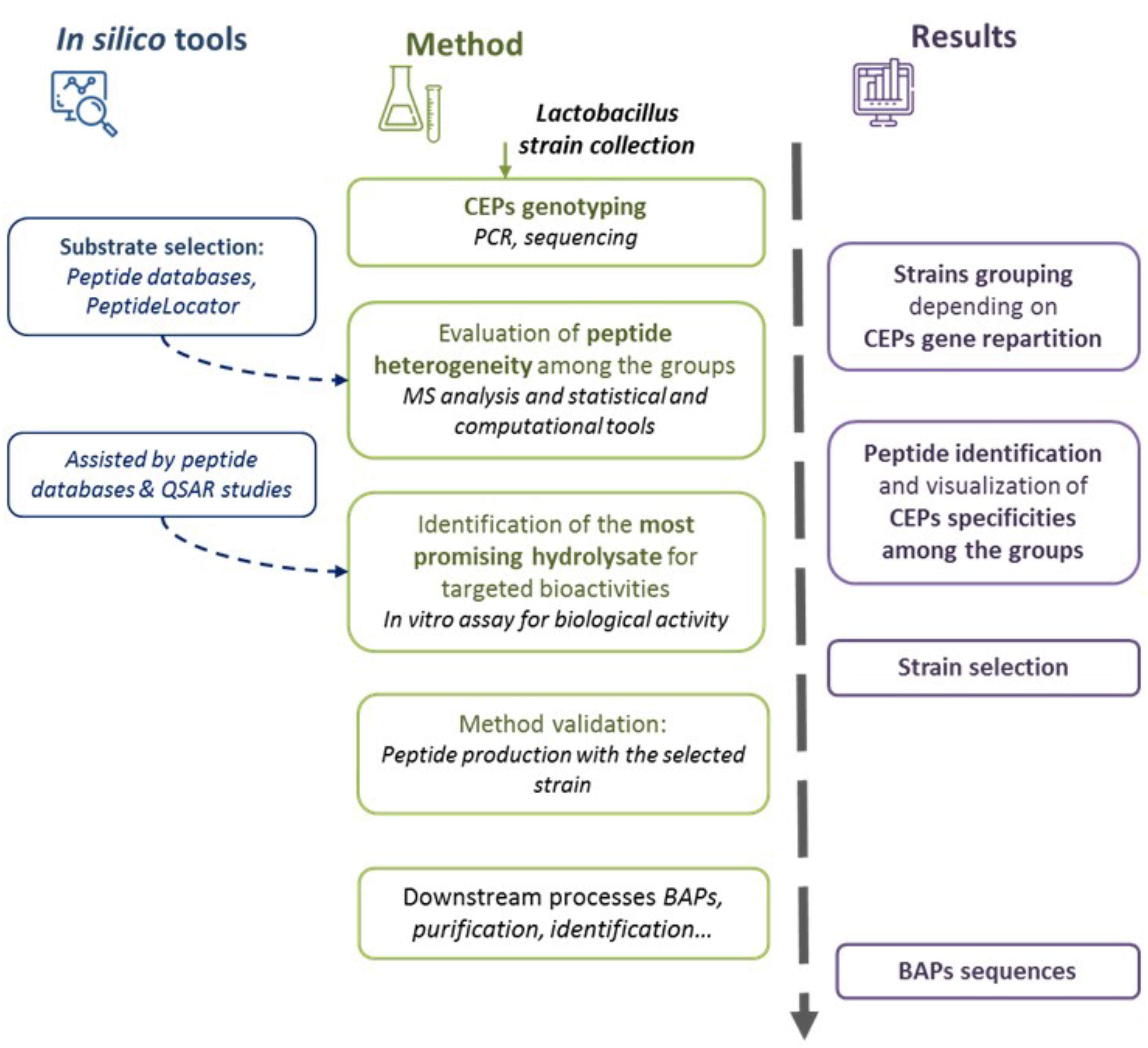
FIGURE 2. Schematic representation of the proposed methodology of Lactobacillus strains selection to produce bioactive peptides (BAPs). Cell envelope proteinase (CEPs) genotyping is performed, followed by the evaluation of the heterogeneity of the produced peptides. The most promising hydrolysate is selected depending on the targeted application. Finally, the selected strains are tested for method validation. Different in silico tools could be used during the methodology.
First of all, it is important to evaluate the inter- or intraspecific diversity of CEPs in a given Lactobacillus collection. The repartitioning of CEPs will indeed impact the proteolytic pattern diversities (Sadat-Mekmene et al., 2011b). This is particularly the case for Lb. helveticus isolates in which four different CEP paralogs can be present (Broadbent et al., 2011; Miyamoto et al., 2015). A rapid way to assess this diversity is the detection of CEP-encoding genes by PCR (Genay et al., 2009), by multiplex PCR (Broadbent et al., 2011), or even by genomic sequencing (Sun et al., 2015). It must be noted that when seven different CEPs have so far been characterized for Lactobacillus species (PrtB, PrtP, PrtR, and 4 paralogs of PrtH), it cannot be excluded that other, not yet described variants do exist in certain species (as noted earlier, searches based on gene homology have already identified 60 CEP gene candidates). Further work must be undertaken to characterize new CEPs from different species. In any case, strains presenting the same CEP genotype can be grouped together as likely to generate fairly similar proteolytic patterns.
The protein source must then be selected. A number of software can be useful at this stage. PeptideCutter is not relevant for use in the context of Lactobacillus research because CEPs are not yet covered by this software, probably due to a lack of sufficient enzymatic characterization and difficulties to model CEPs specificities. Protein selection should be done using BAP databases to search for bioactive sequences in different proteins of interest. Numerous BAP databases exist, usually more or less related to specific activities or sources (for review see Iwaniak et al., 2015; Blanco-Míguez et al., 2017; Giacometti and Buretić-Tomljanović, 2017; especially for milk proteins see Nielsen et al., 2017). The PeptideLocator software (Mooney et al., 2013) is dedicated to the prediction of BAPs in protein sequences. The ToxinPred software could also be used for predicting toxic peptide sequences and avoid selection of a highly cytotoxic protein (Gupta et al., 2013).
Following incubation of the selected protein with different CEP-harboring Lactobacillus strains (or extracted CEPs), the released peptides can be identified using mass spectrometry. This peptidomics analysis allows to assess the proteolytic potential of the selected strains or their CEPs (Hernández-Ledesma et al., 2004; Dallas et al., 2016; Giacometti and Buretić-Tomljanović, 2017). A peptide coverage diagram can be constructed, which highlights the protein areas that are preferentially hydrolyzed (Dallas et al., 2015). The Peptigram software could be used for peptidomics data visualization (Manguy et al., 2017). Moreover, different computational representations or statistical analyses can be used to assess peptide distribution among different samples or conditions as the Venn diagram, the principal component analysis (PCA), multiple linear regression (MLR), artificial neural networks (ANNs), or partial least square-discriminant analysis (PLS-DA) (Iwaniak et al., 2015; Dallas et al., 2016; Jin et al., 2016; Li et al., 2017). Therefore, peptidomics will be able to establish correlations between the CEP genotype of the strains and the profile of peptides they generate.
The selection of Lactobacillus strains is based on the bioactive potential of the produced peptides. Bioinformatics tools can be used to predict the biological activities of peptides. Through BAP databases, bioactive sequences can be easily identified by homology search against known functional peptide sequences (Iwaniak et al., 2015; Blanco-Míguez et al., 2017; Giacometti and Buretić-Tomljanović, 2017; Nielsen et al., 2017). The PeptideRanker server was designed for the prediction of BAPs activities (Mooney et al., 2012). Numerous studies have used quantitative structure activity relationship (QSAR) modeling to achieve peptide activity prediction (Carrasco-Castilla et al., 2012). The QSAR models analyze the correlations between the structural characteristics of molecules and their biological activities in order to predict (even quantitatively) the bioactivity of different peptides. The development of a QSAR model (for review see Iwaniak et al., 2015; Nongonierma and Fitzgerald, 2016a) is first conducted by the construction of a BAPs library composed of known functional sequences with quantitative biological data (such as half maximal inhibitory/effective concentration, IC50/EC50). The BAPs are then described by the creation of different descriptors such as physicochemical properties or amino acid scalar descriptors. Once all the BAPs from the library are described, the QSAR is mathematically modeled using computational methods and statistical analysis (for example PCA, MLR, ANNs, or PLS). Finally, the QSAR model is cross-validated by a test set of BAPs and by confirmatory studies with synthetic peptides. Different QSAR models have not only been developed mainly for ACE inhibition activity (for review see Jahangiri et al., 2014), but also for dipeptidyl dipeptidase IV (DPP-IV) activity (Nongonierma and Fitzgerald, 2016b) and α-glucosidase inhibition (Mollica et al., 2018). The use of QSAR modeling is an interesting approach. Although there is a risk of missing novel peptides with unknown bioactive properties since the method only takes into consideration the sequences that already exist in peptide databases. The main limitation of QSAR is related to the BAP dataset construction. BAPs should preferentially be identified and characterized by the same in vitro methods (Nongonierma and Fitzgerald, 2016a). Furthermore, there is also a need to develop more sophisticated amino acid and peptide descriptors as well as to improve the mathematical expression and algorithms for QSAR modeling (Agyei et al., 2016).
Bioinformatics tools can, therefore, be very useful to select the most promising strains, protein sources, and even predict the activities of the generated peptides. However, it is obvious that an in vitro assessment of the predicted BAP activity must be ultimately undertaken to validate in silico predictions (Lafarga et al., 2015).
Methods for BAPs Production by Lactobacillus Species
Different strategies have already been described for the production of BAPs by Lactobacillus species or their CEPs. These include functionalization of food products by fermentation; the purification of peptides from fermentation broth; and finally, the use of purified or partially purified Lactobacillus CEPs.
In this section, these strategies are presented and updated based on recent technological progress. Each strategy has different advantages and drawbacks, which are presented in Table 1. The choice will mostly depend on the targeted application. Different applications of these methodologies are reviewed in Table 2.
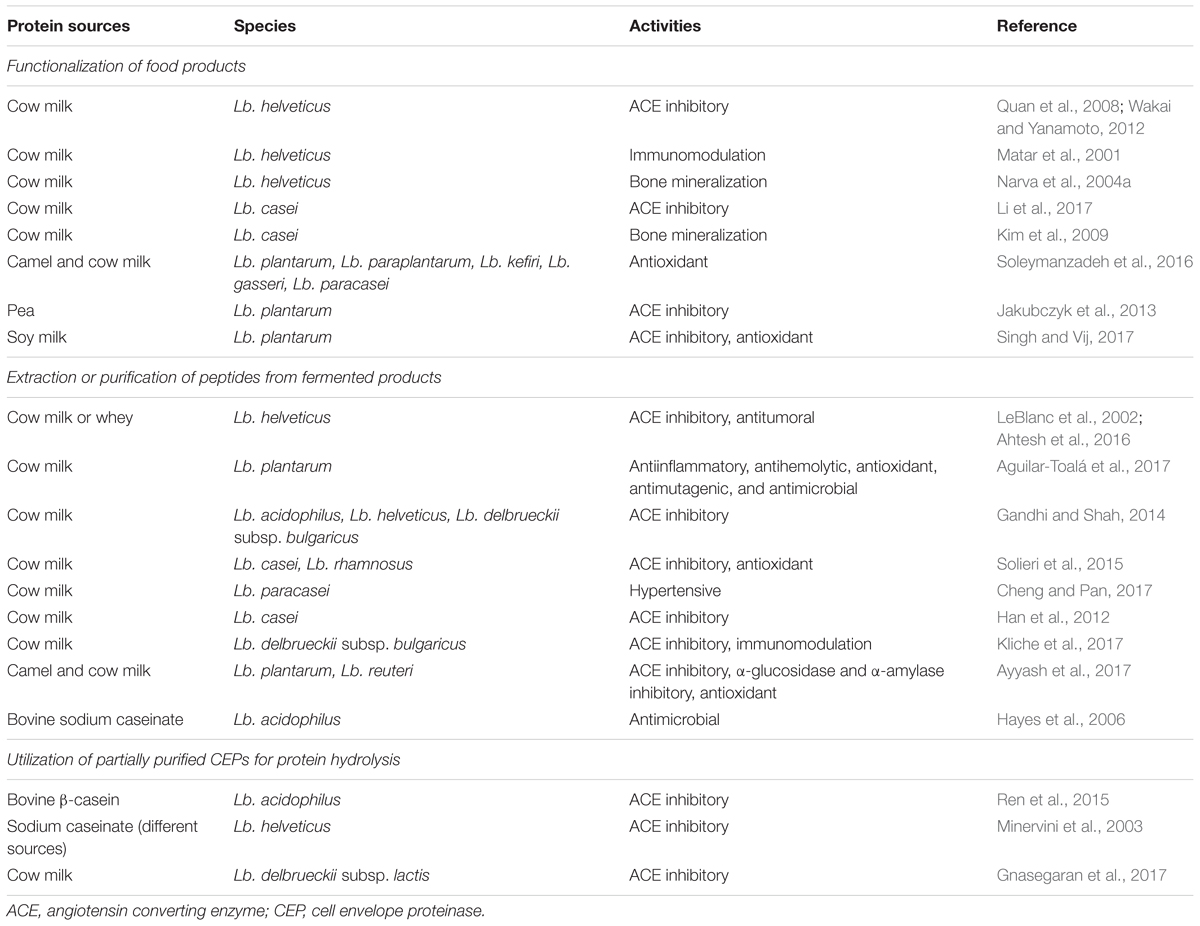
TABLE 2. Applications of BAPs produced by Lactobacillus species depending on the production strategy.
Functionalization of Food Products for Improved Health Benefit
Fermentation with Lactobacillus bacteria has major applications in the food industry. In addition to modifying the organoleptic properties of the product, fermentation (i) improves its nutritional value, by increasing vitamin, amino acid, polysaccharide contents; (ii) decreases the immuno-reactivity of proteins by hydrolyzing allergenic epitopes, a phenomenon that was, for instance, studied on milk proteins hydrolyzed by Lb. fermentum and Lb. delbrueckii subsp. bulgaricus strains (El-Ghaish et al., 2011; Zheng et al., 2013; Pescuma et al., 2015); and (iii) brings extra benefits such as health-promoting properties, through generation of BAPs. Numerous BAPs have been found in fermented products, presenting different functional activities such as antihypertensive, immunomodulatory, hypocholesterolemic, antioxidative, antimicrobial, mineral binding, opioid, and bone mineralization activities (Marco et al., 2017). Many studies focused on ACE inhibitor peptides, probably due to the ease of use of in vitro anti-ACE assays. The well-known Val-Pro-Pro and Ile-Pro-Pro peptides are produced during milk fermentation by some Lb. helveticus strains. The resulting fermented milk showed antihypertensive activities in animal and human clinical studies, with a significant decrease in systolic blood pressure (Wakai and Yanamoto, 2012). An additional ACE inhibitory peptide sequence (Ala-Ile-Pro-Pro-Lys-Lys-Asn-Gln-Asp) was also identified in milk fermented by Lb. helveticus 130B4 strain isolated in Mongolia, demonstrating the potential of this species to release novel ACE inhibitor peptides (Quan et al., 2008). Moreover, other Lactobacillus species may also release new ACE inhibitory peptides, as reported for some Lb. casei strains (Li et al., 2017).
Other types of biological activities were also observed in fermented milk. Milk fermented by Lb. helveticus showed immunomodulating effect after administration to mice. This activity was not observed when mice were treated with milk fermented by a non-proteolytic variant of the same Lb. helveticus strain (Matar et al., 2001). Antioxidant activities were reported from cow and camel milk fermented by specific strains of Lb. plantarum, Lb. paraplantarum, Lb. kefiri, Lb. gasseri, and Lb. paracasei (Soleymanzadeh et al., 2016). Furthermore, a clinical study showed the possible impact of a milk fermented by Lb. helveticus on sleep efficiency in elderly subjects (Yamamura et al., 2009). Numerous animal or human clinical studies reported a positive impact of fermented milk on bone metabolism, probably because of the high calcium concentration and bioavailability in these products (Rizzoli and Biver, 2017). Indeed, fermented milk by Lb. casei 393 strain showed positive impact on bone metabolism in ovariectomized rats (Kim et al., 2009). A comparable effect was observed with milk fermented by Lb. helveticus strains on rats (Narva et al., 2004b) and even on calcium metabolism in postmenopausal women (Narva et al., 2004c). It was also demonstrated that a high intake of yogurt and dairy products was associated with antioxidative effects and a lowered risk of type 2 diabetes (Hove et al., 2015; Jin et al., 2016; Rutella et al., 2016; Fardet and Rock, 2017).
Because of their abilities to hydrolyze different proteins, Lactobacillus species are also used to produce fermented food from non-dairy sources. For instance, several Lb. plantarum strains were used for fermentation of pea seeds and soy milk, and the resulting products displayed ACE inhibition and antioxidative properties (Jakubczyk et al., 2013; Singh and Vij, 2017).
Fermentation by Lactobacillus species can, therefore, lead to the development of health-promoting food, allowing simultaneous functionalization and production. Actually, it must be noted that numerous metabolites are produced by Lactobacillus species during fermentation: not only BAPs, but also lactate, vitamins, or exopolysaccharides. All of these can contribute to the health- promoting effect (Fardet and Rock, 2017; Marco et al., 2017).
Another issue in BAPs research is that it is sometimes difficult to quantify one specific peptide in a whole hydrolysate or fermented food, and correlate its presence with the activity observed in vitro or in vivo. Actually, bioactivities of hydrolysates often result from the combined effects of several peptides.
Finally, a last issue to be kept in mind is that as a product designed for direct consumption, fermented food will be submitted to GID. Proteolytic enzymes (pepsin and pancreatic enzymes) present in the digestive tract will alter the peptide profile of a fermented food and change its activities in vivo (Daliri et al., 2017). The impact of GID on a fermented food must, therefore, be assessed to get an accurate prediction of the real in vivo activities of the food. In fact, this impact can be positive. GID is even, by itself, one of the main processes for BAP production from food proteins (Korhonen and Pihlanto, 2006). Usually, for ACE inhibitory peptides, GID increases the release of BAPs from fermented food, as proteins that were not hydrolyzed during the fermentation will be digested during GID. This was reported for fermented milk (Matar et al., 1996; Hernández-Ledesma et al., 2004; Jin et al., 2016). Furthermore, the Val-Pro-Pro and Ile-Pro-Pro sequences generated during in vitro/industrial fermentation are not altered by GID (Ohsawa et al., 2008). The impact of GID can be evaluated in vitro by treating the fermented product with gastric and pancreatic enzymes, and comparing the activity of the resulting product with the activity of a non-fermented sample.
Extraction and Purification of Peptides From Fermented Media
The BAPs can be extracted and purified from fermentation broth. The resulting product will then be used for various applications (food, pharmaceutical, or cosmetic applications) as a functional or nutraceutical ingredient.
This approach involves production of a broth, from food products or byproducts, through fermentation with Lactobacillus species. The use of a non-proliferative medium, for instance, a casein suspension, is possible (Hebert et al., 2008). An advantage is that substrates other than food can be used as protein sources, especially industrial byproducts. For instance, Lactobacillus species can be used for the valorization of whey proteins from the cheese industry (Ahtesh et al., 2016).
Peptides generated during the fermentation are then isolated by a downstream process of extraction and/or purification. Methods for extraction and purification of peptides have already been reviewed (Korhonen and Pihlanto, 2006; Agyei et al., 2016). Selective precipitation is a fast, cost-effective, and easy to scale-up approach to separate peptides from proteins; trichloroacetic acid (TCA), ethanol, or ammonium sulfate (NH4)2SO4 are usually used as precipitating agents. For instance, TCA precipitation was used to obtain protein hydrolysates from Lb. plantarum-fermented milk (Aguilar-Toalá et al., 2017). The TCA was also used for the isolation of peptides from different milks fermented by Lactobacillus species (Lb. helveticus, Lb. acidophilus, Lb. delbrueckii susbp. bulgaricus, Lb. casei, and Lb. rhamnosus). The resulting hydrolysates displayed ACE inhibition or antioxidative activities (Gandhi and Shah, 2014; Solieri et al., 2015). Ethanol precipitation was used to purify fermentation broths of Lb. paracasei, which were used in animal studies for treatment of hypertension (Cheng and Pan, 2017). Approaches based on chemical precipitation, however, suffer from a major drawback: they require a cleaning step, such as chromatography or affinity columns, to remove the precipitating agent.
Another possibility is to take advantage of Lactobacillus species metabolism. Indeed, these bacteria produce lactic acid during fermentation, leading to a decrease of pH and, therefore, to the precipitation of proteins. Following fermentation, the water soluble extract (WSE) containing peptides can be readily separated by centrifugation (Ayyash et al., 2017). This approach is used preferentially because it does not require the use of chemical agents (Solanki et al., 2017; Taha et al., 2017).
Membrane ultra- and nanofiltration techniques can also be used for peptide extraction and purification (Coutte et al., 2017). Again, the addition of a chemical agent is not required. These procedures are easy to scale-up and can be combined with other processes. A membrane reactor can be used to allow a continuous process where protein hydrolysis and peptide separation will occur simultaneously (Wu et al., 2013; Hernández-Ledesma et al., 2014). Ultrafiltration was used for peptide separation from both fermented milk and casein suspensions. The cut-off threshold ranged from 3 to 14 kDa depending on the targeted peptides (Hayes et al., 2006; Han et al., 2012; Aguilar-Toalá et al., 2017).
Finally, chromatographic methods can be used to achieve peptide purification. These methods include size-exclusion chromatography and ion-exchange chromatography. They are highly selective, with a high-resolution separation. The main issue, especially for industrial applications, is the very high cost of these techniques. Furthermore, chromatography leads to peptide dilution and accumulation of solvent wastes (Agyei et al., 2016). For research applications such as peptidomics, chromatographic techniques are widely used. As an example, the purification and the preparation of antitumoral peptides were performed by gel filtration chromatography from a milk fermented by Lb. helveticus (LeBlanc et al., 2002). Due to its high resolution, this technique of purification is also particularly suitable for identification of the specific peptides involved in a given bioactivity. Peptide identification is indeed important to properly demonstrate that a given activity is associated with a specific BAP. Full identification and sequencing of active peptides are anyway necessary for pharmaceutical applications (Jin et al., 2016; Georgalaki et al., 2017; Giacometti and Buretić-Tomljanović, 2017).
Since the yield of BAP production using the proteolytic activity of Lactobacillus strains is relatively low compared with what is achieved using purified enzymes (Daliri et al., 2017), purification and extraction of BAPs will be useful as a tool for peptide enrichment in the final product. For example, a WSE of fermented milk by a strain of Lb. delbrueckii subsp. bulgaricus, presenting ACE inhibitory and immunomodulatory capacities, was lyophilized and used as a nutraceutical for yogurt (Kliche et al., 2017).
A major issue with this strategy is the cost of extraction and purification. It was estimated that about 70% of total peptide production costs are dedicated to downstream processes (Agyei et al., 2016). However, these steps are needed for research studies focusing on discovery and identification of BAPs. Moreover, it is also advisable to separate peptides from the fermentation broth to avoid interferences with other molecules (Daliri et al., 2017). Purification is also needed for specific nutraceutical or pharmaceutical applications where high-quality finished products are required.
Utilization of Partially Purified CEPs for Protein Hydrolysis
The CEPs initiate protein breakdown in the extracellular media during fermentation. Fermentation, however, is not needed to achieve peptide production, the CEPs can be used as purified enzymes for in vitro hydrolysis. A controlled media for optimal enzymatic activity is requested, while optimal conditions for CEP activity can be different from those typically used for bacterial growth (Gnasegaran et al., 2017). Therefore, higher peptide concentrations can be reached in comparison with those obtained with fermentation approaches. Furthermore, the use of partially purified CEPs allows to generate peptides without production of other fermentation products, thus avoiding biological activity interferences (Daliri et al., 2017).
Obviously, a process of CEP purification is necessary before protein hydrolysis (Sadat-Mekmene et al., 2011a) as well as the assessment of optimal conditions for CEP activity (Ayyash et al., 2012). For example, different CEPs were isolated and purified or partially purified from Lb. helveticus strains. The resulting enzymes displayed a broad range of characteristics including a temperature optimum ranging from 40 to 50°C, a pH optimum ranging from 5.5 to 8, and, sometimes, a requirement for calcium ions as activators (Sadat-Mekmene et al., 2011a).
Depending on the species, CEPs are either anchored to the cell wall or released in the media. The CEPs from a Lb. zeae are secreted in the extracellular media, while several strains of Lb. plantarum seem to possess cell wall-bound CEPs (Vukotić, 2016). This feature determines which extraction strategy must be followed. Cell wall-bound CEPs will require proteinase extraction. For instance, CEP extraction from Lb. helveticus cell wall was carried out using proteinase treatment in Tris-EDTA buffer (Elfahri et al., 2014). The resulting crude CEP extract is then subjected to further enzyme purification process, like ion-exchange chromatography (Minervini et al., 2003). Bacterial culture conditions can be optimized to increase CEP production and extraction yields; this was done for Lactobacillus species such as Lb. plantarum (Kurniati et al., 2015), Lb. delbrueckii subsp. bulgaricus (Nour et al., 2014), or Lb. delbrueckii subsp. lactis (Agyei et al., 2012).
In vitro protein hydrolysis was carried out with extracted CEPs. The CEPs extracted from Lb. acidophilus JQ-1 were used for β-casein hydrolysis, leading to the release of ACE inhibitors (Ren et al., 2015). The CEPs from the Lb. helveticus PR4 strain were partially purified and used to hydrolyze six sodium caseinates from different milks (bovine, sheep, goat, pig, buffalo, or human milk). Among the generated hydrolysates, numerous ACE inhibitor peptides were identified as well as one antibacterial peptide derived from human β-casein (Minervini et al., 2003). Another study used the Lb. delbrueckii subsp. lactis ATCC7830 strain to produce CEPs (Agyei et al., 2012). This strain was also used for the development of an integrated process to produce ACE inhibitory peptides from milk. The process combined CEP production and milk hydrolysis followed by a downstream process of peptide purification. Although the process is expensive, the obtained results are promising for further industrial development (Gnasegaran et al., 2017).
Various strategies have also been tried to increase the yield of peptide production by CEPs. For instance, enzymatic immobilization (cross-linked CEP aggregates) was developed to increase activity and stability of CEPs (Agyei and He, 2015). The use of a combination of Lactobacillus CEPs and commercial enzyme is also another possible approach (Hafeez et al., 2014; Ahtesh et al., 2016).
BAP Production by Lactobacillus Species: Limitations and Future Challenges
The use of Lactobacillus strains for BAP production is a strategy that still suffers from limitations.
First of all, CEPs are still poorly characterized enzymes. Their specificities are not clearly elucidated, and some of them probably remain to be discovered. There is a need for a comprehensive and comparative data analysis of fermentation potentials from different Lactobacillus strains, for example, through genomic comparison (Sun et al., 2015). To date, even a full comparative genomic study on different Lb. helveticus strains has yet not been performed (Stefanovic et al., 2017), although this species is considered as the most proteolytic among the Lactobacillus genus.
Peptidomics tools (chromatography, mass spectrometry, and bioinformatics) allow to analyze more rapidly and more accurately the heterogeneity of peptides produced through fermentation. Using computational methods (PCA, MLR, ANNs, and PLS-DA) (Dallas et al., 2016; Jin et al., 2016; Li et al., 2017), it is possible to visualize which protein regions are preferentially hydrolyzed by different lactobacilli and their CEPs. However, statistical tools are needed to deal with the large datasets generating by peptidomics as thousands of peptides can be identified in a single sample (Dupont, 2017). The collected data can then be assembled to create databases of fermented products (Giacometti and Buretić-Tomljanović, 2017), particularly, for milk proteins. which have been extensively studied.
The main limitation of Lactobacillus species use for BAP production is their low capacity to release peptides, compared with the activity of purified enzymes (Hernández-Ledesma et al., 2004; Daliri et al., 2017). This limitation could be addressed by designing improved fermentation media. For instance, a chemically defined medium was developed to increase the proteolytic activity of Lb. delbrueckii subsp. bulgaricus 761N strain (Nour et al., 2014).
Identification of improved strain variants or even metabolic engineering is another approach. Changes in metabolic pathways of different Lactobacillus strains were conducted successfully for different metabolisms like exopolysaccharides, lactic acid, vitamins, diacetyl, or even sorbitol (Papagianni, 2012; Stefanovic et al., 2017). However, to date, there are no studies on the metabolic engineering of the proteolytic system from lactobacilli. Research is needed to assess whether proteolytic capacities of promising Lactobacillus strains could be improved by CEP overexpression or coexpression of several CEPs by the same strain, which could increase the quantity and diversity of peptides produced upon fermentation.
These optimizations could be assisted by bioinformatics tools such as design of experiments (DOE) followed by response surface methodology (RSM) to improve BAPs release (Sánchez-Rivera et al., 2014; Nongonierma and FitzGerald, 2017a) or CEP production (Kurniati et al., 2015).
Conclusion
This review highlights the great potential of Lactobacillus species to release numerous peptides (and potentially BAPs) during fermentation of proteins. Three different strategies were described to produce BAPs using Lactobacillus strains: (i) production and functionalization of dietary products by fermentation; (ii) extraction or purification of peptides from a fermented broth; and (iii) utilization of partially purified CEPs for protein hydrolysis. The choice between these strategies depends mostly on the targeted application.
In this review, a methodology was proposed for selecting efficient BAP-producing Lactobacillus strains (Figure 2). This method uses a combination of conventional (or empirical) approaches and in silico tools. By describing different strategies for BAP production and a method for strain selection, this work aims at delivering a general workflow for development of various BAP-containing products (nutraceuticals or functionalized foods) using lactobacilli.
Production of BAPs by Lactobacillus species is relatively inexpensive, compared to the use of purified enzymes. It also has a greater potential to generate new peptide sequences and, therefore, new BAPs with specific biological properties. However, there are still some challenges for further industrial exploitation of lactobacilli, such as the poor characterization of CEPs from different species and the low yields of peptide production by fermentation. Metabolic engineering of the proteolytic system of lactobacilli could overcome these challenges.
Author Contributions
CR participated in all steps of preparation of this manuscript. BC, FC, CF, MF, DD, and PD participated in the editing of the manuscript and revised it critically.
Funding
This research was supported by a grant from BPI France/Métropole Européenne de Lille/Région Hauts de France (DOS0020745) and by a grant from Association Nationale de la Recherche et de la Technologie (ANRT).
Conflict of Interest Statement
CR and MF are employed by the company VF Bioscience SAS, France.
The remaining authors declare that the research was conducted in the absence of any commercial or financial relationships that could be construed as a potential conflict of interest.
Acknowledgments
The authors thank the ALIBIOTECH program funding administered by the Hauts-de-France Region.
Abbreviations
ACE, angiotensin converting enzyme; BAPs, bio active peptides; CEP, cell envelope proteinase; DOE, design of experiment; DPP-IV, di peptidyl peptidase IV; EDTA, ethylene diamine tetra acetic acid; GID, gastro-intestinal digestion; LAB, lactic acid bacteria; MLR, multiple linear regression; PCA, principal component analysis; PLS-DA, partial least square discriminant analysis; QSAR, quantitative structural activity relationship; RSM, response surface methodology; TCA, tri chloro acetic acid; WSE, water soluble extract.
References
Aguilar-Toalá, J. E., Santiago-López, L., Peres, C. M., Peres, C., Garcia, H. S., Vallejo-Cordoba, B., et al. (2017). Assessment of multifunctional activity of bioactive peptides derived from fermented milk by specific Lactobacillus plantarum strains. J. Dairy Sci. 100, 65–75. doi: 10.3168/jds.2016-11846
Agyei, D., and He, L. (2015). Evaluation of cross-linked enzyme aggregates of Lactobacillus cell-envelope proteinases, for protein degradation. Food Bioprod. Process. 94, 59–69. doi: 10.1016/j.fbp.2015.01.004
Agyei, D., Ongkudon, C. M., Wei, C. Y., Chan, A. S., and Danquah, M. K. (2016). Bioprocess challenges to the isolation and purification of bioactive peptides. Food Bioprod. Process. 98, 244–256. doi: 10.1016/j.fbp.2016.02.003
Agyei, D., Potumarthi, R., and Danquah, M. K. (2012). Optimisation of batch culture conditions for cell-envelope-associated proteinase production from Lactobacillus delbrueckii subsp. lactis ATCC 7830. Appl. Biochem. Biotechnol. 168, 1035–1050. doi: 10.1007/s12010-012-9839-9
Ahtesh, F., Stojanovska, L., Shah, N., and Mishra, V. K. (2016). Effect of flavourzyme on angiotensin-converting enzyme inhibitory peptides formed in skim milk and whey protein concentrate during fermentation by Lactobacillus helveticus. J. Food Sci. 81, M135–M143. doi: 10.1111/1750-3841.13177
Alcántara, C., Bäuerl, C., Revilla-Guarinos, A., Pérez-Martínez, G., Monedero, V., and Zúñiga, M. (2016). Peptide and amino acid metabolism is controlled by an OmpR-family response regulator in Lactobacillus casei. Mol. Microbiol. 100, 25–41. doi: 10.1111/mmi.13299
Ayyash, M., Al-Nuaimi, A. K., Al-Mahadin, S., and Liu, S. Q. (2017). In vitro investigation of anticancer and ACE-inhibiting activity, α-amylase and α-glucosidase inhibition, and antioxidant activity of camel milk fermented with camel milk probiotic: a comparative study with fermented bovine milk. Food Chem. 239, 588–597. doi: 10.1016/j.foodchem.2017.06.149
Ayyash, M. M., Sherkat, F., and Shah, N. P. (2012). The impact of NaCl substitution with KCl on proteinase activities cell-free extract and cell-free supernatant at different ph levels and salt concentrations: Lactobacillus delbrueckii ssp. bulgaricus and Streptococcus thermophilus. J. Food Sci. 77, 490–498. doi: 10.1111/j.1750-3841.2012.02802.x
Blanco-Míguez, A., Gutiérrez-Jácome, A., Fdez-Riverola, F., Lourenço, A., and Sánchez, B. (2017). MAHMI database: a comprehensive MetaHitbased resource for the study of the mechanism of action of the human microbiota. Database 2017:baw157. doi: 10.1093/database/baw157
Braconi, D., Bernardini, G., Millucci, L., and Santucci, A. (2017). Foodomics for human health: current status and perspectives. Expert Rev. Proteomics 15, 153–164. doi: 10.1080/14789450.2018.1421072
Broadbent, J. R., Cai, H., Larsen, R. L., Hughes, J. E., Welker, D. L., De Carvalho, V. G., et al. (2011). Genetic diversity in proteolytic enzymes and amino acid metabolism among Lactobacillus helveticus strains. J. Dairy Sci. 94, 4313–4328. doi: 10.3168/jds.2010-4068
Cameron, A. J., Shaw, J. E., and Zimmet, P. Z. (2004). The metabolic syndrome: prevalence in worldwide populations. Endocrinol. Metab. Clin. North Am. 33, 351–375. doi: 10.1016/j.ecl.2004.03.005
Caron, J., Domenger, D., Dhulster, P., Ravallec, R., and Cudennec, B. (2017). Protein digestion-derived peptides and the peripheral regulation of food intake. Front. Endocrinol. 8:85. doi: 10.3389/fendo.2017.00085
Carrasco-Castilla, J., Hernández-Álvarez, A. J., Jiménez-Martínez, C., Gutiérrez-López, G. F., and Dávila-Ortiz, G. (2012). Use of proteomics and peptidomics methods in food bioactive peptide science and engineering. Food Eng. Rev. 4, 224–243. doi: 10.1007/s12393-012-9058-8
Chen, J.-P., Chen, G.-C., Wang, X.-P., Qin, L., and Bai, Y. (2018). Dietary fiber and metabolic syndrome: a meta-analysis and review of related mechanisms. Nutrients 10:24. doi: 10.3390/nu10010024
Cheng, M. C., and Pan, T. M. (2017). Prevention of hypertension-induced vascular dementia by Lactobacillus paracasei subsp. paracasei NTU 101-fermented products. Pharm. Biol. 55, 487–496. doi: 10.1080/13880209.2016.1253109
Cifuentes, A. (2009). Food analysis and foodomics. J. Chromatogr. A 1216:7109. doi: 10.1016/j.chroma.2009.09.018
Cornier, M. A., Dabelea, D., Hernandez, T. L., Lindstrom, R. C., Steig, A. J., Stob, N. R., et al. (2008). The metabolic syndrome. Endocr. Rev. 29, 777–822. doi: 10.1210/er.2008-0024
Courtin, P., Monnet, V., and Rul, F. (2002). Cell-wall proteinases PrtS and PrtB have a different role in Streptococcus thermophilus/Lactobacillus bulgaricus mixed cultures in milk. Microbiology 148, 3413–3421. doi: 10.1099/00221287-148-11-3413
Coutte, F., Lecouturier, D., Firdaous, L., Kapel, R., Bazinet, L., Cabassud, C., et al. (2017). “Recent trends in membrane bioreactors,” in Current Developments in Biotechnology and Bioengineering, eds A. Pandey, C. Larroche, and C. Soccol (Amsterdam: Elsevier), 279–311. doi: 10.1016/B978-0-444-63663-8.00010-0
Dallas, D. C., Citerne, F., Tian, T., Silva, V. L. M., Kalanetra, K. M., Frese, S. A., et al. (2016). Peptidomic analysis reveals proteolytic activity of kefir microorganisms on bovine milk proteins. Food Chem. 197, 273–284. doi: 10.1016/j.foodchem.2015.10.116
Dallas, D. C., Guerrero, A., Parker, E. A., Robinson, R. C., Gan, J., German, J. B., et al. (2015). Current peptidomics: applications, purification, identification, quantification, and functional analysis. Proteomics 15, 1026–1038. doi: 10.1002/pmic.201400310.Current
Domenger, D., Cudennec, B., Kouach, M., Touche, V., Landry, C., Lesage, J., et al. (2018). Food-derived hemorphins cross intestinal and blood-brain barriers in vitro. Front. Endocrinol. 9:159. doi: 10.3389/fendo.2018.00159
Dupont, D. (2017). Peptidomic as a tool for assessing protein digestion. Curr. Opin. Food Sci. 16, 53–58. doi: 10.1016/j.cofs.2017.08.001
Dziuba, B., and Dziuba, M. (2014). Milk proteins-derived bioactive peptides in dairy products: molecular, biological and methodological aspects. Acta Sci. Pol. Technol. Aliment. 13, 5–25. doi: 10.17306/J.AFS.2014.1.1
Elfahri, K. R., Donkor, O. N., and Vasiljevic, T. (2014). Potential of novel Lactobacillus helveticus strains and their cell wall bound proteases to release physiologically active peptides from milk proteins. Int. Dairy J. 38, 37–46. doi: 10.1016/j.idairyj.2014.03.010
El-Ghaish, S., Rabesona, H., Choiset, Y., Sitohy, M., Haertlé, T., and Chobert, J.-M. (2011). Proteolysis by Lactobacillus fermentum IFO3956 isolated from Egyptian milk products decreases immuno-reactivity of αS1-casein. J. Dairy Res. 78, 203–210. doi: 10.1017/S0022029911000100
Fardet, A., and Rock, E. (2017). In vitro and in vivo antioxidant potential of milks, yoghurts, fermented milks and cheeses: a narrative review of evidence. Nutr. Res. Rev. 31, 52–70. doi: 10.1017/S0954422417000191
Felis, G. E., and Dellaglio, F. (2007). Taxonomy of Lactobacilli and Bifidobacteria. Curr. Issues Intest. Microbiol. 8, 44–61.
Gandhi, A., and Shah, N. P. (2014). Cell growth and proteolytic activity of Lactobacillus acidophilus, Lactobacillus helveticus, Lactobacillus delbrueckii ssp. bulgaricus, and Streptococcus thermophilus in milk as affected by supplementation with peptide fractions. Int. J. Food Sci. Nutr. 65, 937–941. doi: 10.3109/09637486.2014.945154
Gasteiger, E., Hoogland, C., Gattiker, A., Duvaud, S., Wilkins, M. R., Appel, R. D., et al. (2005). “Protein identification and analysis tools on the ExPASy server,” in The Proteomics Protocols Handbook, ed. J. M. Walker (New York, NY: Humana Press), 571–607. doi: 10.1385/1592598900
Genay, M., Sadat, L., Gagnaire, V., and Lortal, S. (2009). PrtH2, Not prtH, Is the ubiquitous cell wall proteinase gene in Lactobacillus helveticus. Appl. Environ. Microbiol. 75, 3238–3249. doi: 10.1128/AEM.02395-08
Georgalaki, M., Zoumpopoulou, G., Mavrogonatou, E., Van Driessche, G., Alexandraki, V., Anastasiou, R., et al. (2017). Evaluation of the antihypertensive angiotensin-converting enzyme inhibitory (ACE-I) activity and other probiotic properties of lactic acid bacteria isolated from traditional Greek dairy products. Int. Dairy J. 75, 10–21. doi: 10.1016/j.idairyj.2017.07.003
Giacometti, J., and Buretić-Tomljanović, A. (2017). Peptidomics as a tool for characterizing bioactive milk peptides. Food Chem. 230, 91–98. doi: 10.1016/j.foodchem.2017.03.016
Gnasegaran, G. K., Agyei, D., Pan, S., Sarethy, I. P., Acquah, C., and Danquah, M. K. (2017). “Process development for bioactive peptide production,” in Food Bioactives: Extraction and Biotechnology Applications, ed. M. Puri (Cham: Springer International Publishing AG), 91–110. doi: 10.1007/978-3-319-51639-4
Griffiths, M. W., and Tellez, A. M. (2013). Lactobacillus helveticus: the proteolytic system. Front. Microbiol. 4:30. doi: 10.3389/fmicb.2013.00030
Gupta, S., Kapoor, P., Chaudhary, K., Gautam, A., Kumar, R., and Raghava, G. P. S. (2013). In silico approach for predicting toxicity of peptides and proteins. PLoS One 8:e73957. doi: 10.1371/journal.pone.0073957
Hafeez, Z., Cakir-Kiefer, C., Roux, E., Perrin, C., Miclo, L., and Dary-Mourot, A. (2014). Strategies of producing bioactive peptides from milk proteins to functionalize fermented milk products. Food Res. Int. 63, 71–80. doi: 10.1016/j.foodres.2014.06.002
Han, Z., Luo, X., Tian, H., Men, Y., Wang, N., Jiang, Y., et al. (2012). “Separation of angiotensin-converting enzyme inhibitors from fermented milk prepared with Lactobacillus casei HZ1,” in Smart Materials and Nanotechnology in Engineering, ed. J. L. Zhong (Zurich: Trans Tech Pubn), 161–167. doi: 10.4028/www.scientific.net/AMR.345.161
Hayes, M., Ross, R. P., Fitzgerald, G. F., Hill, C., and Stanton, C. (2006). Casein-derived antimicrobial peptides generated by Lactobacillus acidophilus DPC6026. Appl. Environ. Microbiol. 72, 2260–2264. doi: 10.1128/AEM.72.3.2260
Hebert, E. M., Mamone, G., Picariello, G., Raya, R. R., Savoy, G., Ferranti, P., et al. (2008). Characterization of the pattern of αs1- and β-casein breakdown and release of a bioactive peptide by a cell envelope proteinase from Lactobacillus delbrueckii subsp. lactis CRL 581. Appl. Environ. Microbiol. 74, 3682–3689. doi: 10.1128/AEM.00247-08
Hernández-Ledesma, B., Amigo, L., Ramos, M., and Recio, I. (2004). Application of high-performance liquid chromatography-tandem mass spectrometry to the identification of biologically active peptides produced by milk fermentation and simulated gastrointestinal digestion. J. Chromatogr. A 1049, 107–114. doi: 10.1016/j.chroma.2004.07.025
Hernández-Ledesma, B., García-Nebot, M. J., Fernández-Tomé, S., Amigo, L., and Recio, I. (2014). Dairy protein hydrolysates: peptides for health benefits. Int. Dairy J. 38, 82–100. doi: 10.1016/j.idairyj.2013.11.004
Hou, J.-C., Liu, F., Ren, D.-X., Han, W.-W., and Du, Y.-O. (2015). Effect of culturing conditions on the expression of key enzymes in the proteolytic system of Lactobacillus bulgaricus. J. Zhejiang Univ. Sci. B 16, 317–326. doi: 10.1631/jzus.B1400230
Hove, K. D., Brons, C., Faerch, K., Lund, S. S., Rossing, P., and Vaag, A. (2015). Effects of 12 weeks of treatment with fermented milk on blood pressure, glucose metabolism and markers of cardiovascular risk in patients with type 2 diabetes: a randomised double-blind placebo-controlled study. Eur. J. Endocrinol. 172, 11–20. doi: 10.1530/EJE-14-0554
Hutkins, R. W. (2006). “Microbiology and technology of fermented foods,” in Chapter 2: Microorganisms and Metabolism, ed. R. W. Hutkins (Hoboken, NJ: John Wiley & Sons, Inc.), 15–66. doi: 10.1007/s13398-014-0173-7.2
Ikram-ul-Haq, and Mukhtar, H. (2006). Biosynthesis of protease from Lactobacillus paracasei: kinetic analysis of fermentation parameters. Indian J. Biochem. Biophys. 43, 377–381.
Iwaniak, A., Minkiewicz, P., Darewicz, M., Protasiewicz, M., and Mogut, D. (2015). Chemometrics and cheminformatics in the analysis of biologically active peptides from food sources. J. Funct. Foods 16, 334–351. doi: 10.1016/j.jff.2015.04.038
Jahangiri, R., Soltani, S., and Barzegar, A. (2014). A review of QSAR studies to predict activity of ACE peptide inhibitors. Pharm. Sci. 20, 122–129.
Jakubczyk, A., Karaś, M., Baraniak, B., and Pietrzak, M. (2013). The impact of fermentation and in vitro digestion on formation angiotensin converting enzyme (ACE) inhibitory peptides from pea proteins. Food Chem. 141, 3774–3780. doi: 10.1016/j.foodchem.2013.06.095
Jensen, M. P., Vogensen, F. K., and Ardö, Y. (2009). Variation in aminopeptidase and aminotransferase activities of six cheese related Lactobacillus helveticus strains. Int. Dairy J. 19, 661–668. doi: 10.1016/j.idairyj.2009.09.007
Jin, Y., Yu, Y., Qi, Y., Wang, F., Yan, J., and Zou, H. (2016). Peptide profiling and the bioactivity character of yogurt in the simulated gastrointestinal digestion. J. Proteomics 141, 24–46. doi: 10.1016/j.jprot.2016.04.010
Kassi, E., Pervanidou, P., Kaltsas, G., and Chrousos, G. (2011). Metabolic syndrome: definitions and controversies. BMC Med. 9:48. doi: 10.1186/1741-7015-9-48
Kim, J. G., Lee, E., Kim, S. H., Whang, K. Y., Oh, S., and Imm, J. Y. (2009). Effects of a Lactobacillus casei 393 fermented milk product on bone metabolism in ovariectomised rats. Int. Dairy J. 19, 690–695. doi: 10.1016/j.idairyj.2009.06.009
Kliche, T., Li, B., Bockelmann, W., Habermann, D., Klempt, M., de Vrese, M., et al. (2017). Screening for proteolytically active lactic acid bacteria and bioactivity of peptide hydrolysates obtained with selected strains. Appl. Microbiol. Biotechnol. 101, 7621–7633. doi: 10.1007/s00253-017-8369-3
Kojic, M., Fira, D., Banina, A., and Topisirovic, L. (1991). Characterization of the cell wall-bound proteinase of Lactobacillus casei HN14. Appl. Environ. Microbiol. 57, 1753–1757.
Korhonen, H. (2009). Milk-derived bioactive peptides: from science to applications. J. Funct. Foods 1, 177–187. doi: 10.1016/j.jff.2009.01.007
Korhonen, H., and Pihlanto, A. (2006). Bioactive peptides: production and functionality. Int. Dairy J. 16, 945–960. doi: 10.1016/j.idairyj.2005.10.012
Kurniati, N., Mubarik, N. R., and Desniar, C. (2015). Optimization of production of protease by Lactobacillus plantarum SK (5) from bekasam with response surface methodology. Pak. J. Biotechnol. 12, 123–130.
Lafarga, T., Rai, D. K., OConnor, P., and Hayes, M. (2015). A bovine fibrinogen-enriched fraction as a source of peptides with in vitro renin and angiotensin-I-converting enzyme inhibitory activities. J. Agric. Food Chem. 63, 8676–8684. doi: 10.1021/acs.jafc.5b03167
LeBlanc, J. G., Matar, C., Valdéz, J. C., LeBlanc, J., and Perdigon, G. (2002). Immunomodulating effects of peptidic fractions issued from milk fermented with Lactobacillus helveticus. J. Dairy Sci. 85, 2733–2742. doi: 10.3168/jds.S0022-0302(02)74360-9
Li, C., Kwok, L.-Y., Mi, Z., Bala, J., Xue, J., Yang, J., et al. (2017). Characterization of the angiotensin-converting enzyme inhibitory activity of fermented milks produced with Lactobacillus casei. J. Dairy Sci. 100, 9495–9507. doi: 10.3168/jds.2017-12970
Manguy, J., Jehl, P., Dillon, E. T., Davey, N. E., Shields, D. C., and Holton, T. A. (2017). Peptigram: a web-based application for peptidomics data visualization. J. Proteome Res. 16, 712–719. doi: 10.1021/acs.jproteome.6b00751
Marco, M. L., Heeney, D., Binda, S., Cifelli, C. J., Cotter, P. D., Foligné, B., et al. (2017). Health benefits of fermented foods: microbiota and beyond. Curr. Opin. Biotechnol. 44, 94–102. doi: 10.1016/j.copbio.2016.11.010
Matar, C., Amiot, J., Savoie, L., and Goulet, J. (1996). The effect of milk fermentation by Lactobacillus helveticus on the release of peptides during in vitro digestion. J. Dairy Sci. 79, 971–979. doi: 10.3168/jds.S0022-0302(96)76448-2
Matar, C., Valdez, J. C., Medina, M., Rachid, M., and Perdigon, G. (2001). Immunomodulating effects of milks fermented by Lactobacillus helveticus and its non-proteolytic variant. J. Dairy Res. 68, 601–609. doi: 10.1017/S0022029901005143
Minervini, F., Algaron, F., Rizzello, C. G., Fox, P. F., Monnet, V., and Gobbetti, M. (2003). Angiotensin I-converting-enzyme-inhibitory and antibacterial peptides from Lactobacillus helveticus PR4 proteinase-hydrolyzed caseins of milk from six species. Appl. Environ. Microbiol. 69, 5297–5305. doi: 10.1128/AEM.69.9.5297-5305.2003
Miyamoto, M., Ueno, H. M., Watanabe, M., Tatsuma, Y., Seto, Y., Miyamoto, T., et al. (2015). Distinctive proteolytic activity of cell envelope proteinase of Lactobacillus helveticus isolated from airag, a traditional Mongolian fermented mare’s milk. Int. J. Food Microbiol. 197, 65–71. doi: 10.1016/j.ijfoodmicro.2014.12.012
Mollica, A., Zengin, G., Durdagi, S., Ekhteiari Salmas, R., Macedonio, G., Stefanucci, A., et al. (2018). Combinatorial peptide library screening for discovery of diverse α-glucosidase inhibitors using molecular dynamics simulations and binary QSAR models. J. Biomol. Struct. Dyn. 1102, 1–15. doi: 10.1080/07391102.2018.1439403
Mooney, C., Haslam, N. J., Holton, T. A., Pollastri, G., and Shields, D. C. (2013). PeptideLocator: prediction of bioactive peptides in protein sequences. Bioinformatics 29, 1120–1126. doi: 10.1093/bioinformatics/btt103
Mooney, C., Haslam, N. J., Pollastri, G., and Shields, D. C. (2012). Towards the improved discovery and design of functional peptides: common features of diverse classes permit generalized prediction of bioactivity. PLoS One 7:e45012. doi: 10.1371/journal.pone.0045012
Narva, M., Collin, M., Jauhiainen, T., Vapaatalo, H., and Korpela, R. (2004a). Effects of Lactobacillus helveticus fermented milk and its bioactive peptides on bone parameters in spontaneously hypertensive rats. Milchwissenschaft 59, 359–363.
Narva, M., Collin, M., Lamberg-Allardt, C., Kärkkäinen, M., Poussa, T., Vapaatalo, H., et al. (2004b). Effects of long-term intervention with Lactobacillus helveticus-fermented milk on bone mineral density and bone mineral content in growing rats. Ann. Nutr. Metab. 48, 228–234. doi: 10.1159/000080455
Narva, M., Nevala, R., Poussa, T., and Korpela, R. (2004c). The effect of Lactobacillus helveticus fermented milk on acute changes in calcium metabolism in postmenopausal women. Eur. J. Nutr. 43, 61–68. doi: 10.1007/s00394-004-0441-y
Nielsen, S. D., Beverly, R. L., Qu, Y., and Dallas, D. C. (2017). Milk bioactive peptide database: a comprehensive database of milk protein-derived bioactive peptides and novel visualization. Food Chem. 232, 673–682. doi: 10.1016/j.foodchem.2017.04.056
Nongonierma, A. B., and FitzGerald, R. J. (2015). The scientific evidence for the role of milk protein-derived bioactive peptides in humans: a review. J. Funct. Foods 17, 640–656. doi: 10.1016/j.jff.2015.06.021
Nongonierma, A. B., and Fitzgerald, R. J. (2016a). Learnings from quantitative structure–activity relationship (QSAR) studies with respect to food protein-derived bioactive peptides: a review. RSC Adv. 6, 75400–75413. doi: 10.1039/C6RA12738J
Nongonierma, A. B., and Fitzgerald, R. J. (2016b). Structure activity relationship modelling of milk protein-derived peptides with dipeptidyl peptidase IV (DPP-IV) inhibitory activity. Peptides 79, 1–7. doi: 10.1016/j.peptides.2016.03.005
Nongonierma, A. B., and FitzGerald, R. J. (2017a). Enhancing bioactive peptide release and identification using targeted enzymatic hydrolysis of milk proteins. Anal. Bioanal. Chem. 410, 3407–3423. doi: 10.1007/s00216-017-0793-9
Nongonierma, A. B., and FitzGerald, R. J. (2017b). Strategies for the discovery and identification of food protein-derived biologically active peptides. Trends Food Sci. Technol. 69, 289–305. doi: 10.1016/j.tifs.2017.03.003
Nour, I., Fattouh, F., and El-Adawi, H. (2014). Chemically defined medium for optimization of proteolytic activity of Lactobacillus bulgaricus 761N. Int. J. Life Sci. Med. Res. 4, 46–56. doi: 10.5963/LSMR0404002
Oberg, C. J., Broadbent, J. R., Strickland, M., and McMahon, D. J. (2002). Diversity in specificity of the extracellular proteinases in Lactobacillus helveticus and Lactobacillus delbrueckii subsp. bulgaricus. Lett. Appl. Microbiol. 34, 455–460. doi: 10.1046/j.1472-765X.2002.01123.x
Ohsawa, K., Satsu, H., Ohki, K., Enjoh, M., Takano, T., and Shimizu, M. (2008). Producibility and digestibility of antihypertensive?β-casein tripeptides, Val-Pro-Pro and Ile-Pro-Pro, in the gastrointestinal tract: analyses using an in vitro model of mammalian gastrointestinal digestion. J. Agric. Food Chem. 56, 854–858. doi: 10.1021/jf072671n
O’Neill, S., and O’Driscoll, L. (2015). Metabolic syndrome: a closer look at the growing epidemic and its associated pathologies. Obes. Rev. 16, 1–12. doi: 10.1111/obr.12229
Oommen, B. S., McMahon, D. J., Oberg, C. J., Broadbent, J. R., and Strickland, M. (2002). Proteolytic specificity of Lactobacillus delbrueckii subsp. bulgaricus influences functional properties of Mozzarella Cheese. J. Dairy Sci. 85, 2750–2758. doi: 10.3168/jds.S0022-0302(02)74362-2
Papagianni, M. (2012). Metabolic engineering of lactic acid bacteria for the production of industrially important compounds. Comput. Struct. Biotechnol. J. 3, 1–8. doi: 10.5936/csbj.201210003
Park, Y. W., and Nam, M. S. (2015). Bioactive peptides in milk and dairy products: a review. Korean J. Food Sci. Anim. Resour. 35, 831–840. doi: 10.5851/kosfa.2015.35.6.831
Patel, R., and Hati, S. (2018). Production of antihypertensive (angiotensin I-converting enzyme inhibitory) peptides derived from fermented milk supplemented with WPC70 and Calcium caseinate by Lactobacillus cultures. Rev. Med. Microbiol. 29, 30–40. doi: 10.1097/MRM.0000000000000119
Pederson, J. A., Mileski, G. J., Weimer, B. C., and Steele, J. L. (1999). Genetic characterization of a cell envelope-associated proteinase from Lactobacillus helveticus CNRZ32. J. Bacteriol. 181, 4592–4597.
Pescuma, M., Hébert, E. M., Haertlé, T., Chobert, J.-M., Mozzi, F., and Font de Valdez, G. (2015). Lactobacillus delbrueckii subsp. bulgaricus CRL 454 cleaves allergenic peptides of β-lactoglobulin. Food Chem. 170, 407–414. doi: 10.1016/j.foodchem.2014.08.086
Pihlanto, A. (2013). “Lactic fermentation and bioactive peptides,” in Lactic Acid Bacteria – R & D for Food, Health and Livestock Purposes, ed. M. Kongo (Rijeka: InTech Prepress), 310–331. doi: 10.5772/51692
Quan, S., Tsuda, H., and Miyamoto, T. (2008). Angiotensin I-converting enzyme inhibitory peptides in skim milk fermented with Lactobacillus helveticus 130B4 from camel milk in inner Mongolia, China. J. Sci. Food Agric. 88, 2688–2692. doi: 10.1002/jsfa
Ren, X., Pan, D., Wu, Z., Zeng, X., Sun, Y., Cao, J., et al. (2015). Limited hydrolysis of β-casein by cell wall proteinase and its effect on hydrolysates’s conformational and structural properties. Int. J. Food Sci. Technol. 50, 55–61. doi: 10.1111/ijfs.12705
Rizzello, C. G., Lorusso, A., Russo, V., Pinto, D., Marzani, B., and Gobbetti, M. (2017). Improving the antioxidant properties of quinoa flour through fermentation with selected autochthonous lactic acid bacteria. Int. J. Food Microbiol. 241, 252–261. doi: 10.1016/j.ijfoodmicro.2016.10.035
Rizzoli, R., and Biver, E. (2017). Effects of fermented milk products on bone. Calcif. Tissue Int. 102, 489–500. doi: 10.1007/s00223-017-0317-9
Rutella, G. S., Solieri, L., Martini, S., and Tagliazucchi, D. (2016). Release of the antihypertensive tripeptides valine-proline-proline and isoleucine-proline-proline from bovine milk caseins during in vitro gastrointestinal digestion. J. Agric. Food Chem. 64, 8509–8515. doi: 10.1021/acs.jafc.6b03271
Sadat-Mekmene, L., Genay, M., Atlan, D., Lortal, S., and Gagnaire, V. (2011a). Original features of cell-envelope proteinases of Lactobacillus helveticus. A review. Int. J. Food Microbiol. 146, 1–13. doi: 10.1016/j.ijfoodmicro.2011.01.039
Sadat-Mekmene, L., Jardin, J., Corre, C., Mollé, D., Richoux, R., Delage, M. M., et al. (2011b). Simultaneous presence of PrtH and PrtH2 proteinases in Lactobacillus helveticus strains improves breakdown of the pure αs1-casein. Appl. Environ. Microbiol. 77, 179–186. doi: 10.1128/AEM.01466-10
Sánchez-Rivera, L., Martínez-Maqueda, D., Cruz-Huerta, E., Miralles, B., and Recio, I. (2014). Peptidomics for discovery, bioavailability and monitoring of dairy bioactive peptides. Food Res. Int. 63, 170–181. doi: 10.1016/j.foodres.2014.01.069
Savijoki, K., Ingmer, H., and Varmanen, P. (2006). Proteolytic systems of lactic acid bacteria. Appl. Microbiol. Biotechnol. 71, 394–406. doi: 10.1007/s00253-006-0427-1
Singh, B. P., and Vij, S. (2017). Growth and bioactive peptides production potential of Lactobacillus plantarum strain C2 in soy milk: a LC-MS/MS based revelation for peptides biofunctionality. Food Sci. Technol. 86, 293–301. doi: 10.1016/j.lwt.2017.08.013
Solanki, D., Hati, S., and Sakure, A. (2017). In silico and in vitro analysis of novel angiotensin I-converting enzyme (ACE) inhibitory bioactive peptides derived from fermented camel milk (Camelus dromedarius). Int. J. Pept. Res. Ther. 23, 441–459. doi: 10.1007/s10989-017-9577-5
Soleymanzadeh, N., Mirdamadi, S., and Kianirad, M. (2016). Antioxidant activity of camel and bovine milk fermented by lactic acid bacteria isolated from traditional fermented camel milk (Chal). Dairy Sci. Technol. 96, 443–457. doi: 10.1007/s13594-016-0278-1
Solieri, L., Rutella, G. S., and Tagliazucchi, D. (2015). Impact of non-starter lactobacilli on release of peptides with angiotensin-converting enzyme inhibitory and antioxidant activities during bovine milk fermentation. Food Microbiol. 51, 108–116. doi: 10.1016/j.fm.2015.05.012
Stefanovic, E., Fitzgerald, G., and McAuliffe, O. (2017). Advances in the genomics and metabolomics of dairy lactobacilli: a review. Food Microbiol. 61, 33–49. doi: 10.1016/j.fm.2016.08.009
Sun, Z., Harris, H. M. B., McCann, A., Guo, C., Argimón, S., Zhang, W., et al. (2015). Expanding the biotechnology potential of lactobacilli through comparative genomics of 213 strains and associated genera. Nat. Commun. 6:8322. doi: 10.1038/ncomms9322
Taha, S., El Abd, M., De Gobba, C., Abdel-Hamid, M., Khalil, E., and Hassan, D. (2017). Antioxidant and antibacterial activities of bioactive peptides in buffalo’s yoghurt fermented with different starter cultures. Food Sci. Biotechnol. 26, 1325–1332. doi: 10.1007/s10068-017-0160-9
Vukotić, G., Strahinić, I., Begović, J., Lukić, J., Kojić, M., and Fira, D. (2016). Survey on proteolytic activity and diversity of proteinase genes in mesophilic lactobacilli. Microbiology 85, 33–41. doi: 10.1134/S002626171601015X
Wakai, T., and Yanamoto, N. (2012). “Antihypertensive peptides specific to Lactobacillus helveticus fermented milk,” in Biotechnology - Molecular Studies and Novel Applications for Improved Quality of Human Life, ed. R. Sammour (London: IntechOpen Limited), 159–172. doi: 10.5772/711
Wilson, P. W. F., D’Agostino, R. B., Parise, H., Sullivan, L., and Meigs, J. B. (2005). Metabolic syndrome as a precursor of cardiovascular disease and type 2 diabetes mellitus. Circulation 112, 3066–3072. doi: 10.1161/CIRCULATIONAHA.105.539528
Wu, S., Qi, W., Li, T., Lu, D., Su, R., and He, Z. (2013). Simultaneous production of multi-functional peptides by pancreatic hydrolysis of bovine casein in an enzymatic membrane reactor via combinational chromatography. Food Chem. 141, 2944–2951. doi: 10.1016/j.foodchem.2013.05.050
Yamamura, S., Morishima, H., Kumano-go, T., Suganuma, N., Matsumoto, H., Adachi, H., et al. (2009). The effect of Lactobacillus helveticus fermented milk on sleep and health perception in elderly subjects. Eur. J. Clin. Nutr. 63, 100–105. doi: 10.1038/sj.ejcn.1602898
Yang, F., Xia, W.-S., Zhang, X.-W., Xu, Y.-S., and Jiang, Q.-X. (2016). A comparison of endogenous and microbial proteolytic activities during fast fermentation of silver carp inoculated with Lactobacillus plantarum. Food Chem. 207, 86–92. doi: 10.1016/j.foodchem.2016.03.049
Keywords: bioactive peptides, fermentation, hydrolysis, Lactobacillus, strain screening
Citation: Raveschot C, Cudennec B, Coutte F, Flahaut C, Fremont M, Drider D and Dhulster P (2018) Production of Bioactive Peptides by Lactobacillus Species: From Gene to Application. Front. Microbiol. 9:2354. doi: 10.3389/fmicb.2018.02354
Received: 22 June 2018; Accepted: 13 September 2018;
Published: 17 October 2018.
Edited by:
Aldo Corsetti, Università degli Studi di Teramo, ItalyReviewed by:
Victor Ladero, Consejo Superior de Investigaciones Científicas (CSIC), SpainDenis Colum Shields, University College Dublin, Ireland
Giorgio Giraffa, Centro di Zootecnia e Acquacoltura (CREA-ZA), Italy
Copyright © 2018 Raveschot, Cudennec, Coutte, Flahaut, Fremont, Drider and Dhulster. This is an open-access article distributed under the terms of the Creative Commons Attribution License (CC BY). The use, distribution or reproduction in other forums is permitted, provided the original author(s) and the copyright owner(s) are credited and that the original publication in this journal is cited, in accordance with accepted academic practice. No use, distribution or reproduction is permitted which does not comply with these terms.
*Correspondence: Benoit Cudennec, YmVub2l0LmN1ZGVubmVjQHVuaXYtbGlsbGUuZnI=; YmVub2l0LmN1ZGVubmVjQHVuaXYtbGlsbGUxLmZy