- 1Teagasc Moorepark Food Research Centre, Fermoy, Ireland
- 2School of Microbiology, University College Cork, Cork, Ireland
- 3Donvis Ltd., Palmerston North, New Zealand
- 4AgResearch Limited, Grasslands Research Centre, Palmerston North, New Zealand
- 5APC Microbiome Ireland, University College Cork, Cork, Ireland
Enteric fermentation in ruminants is the single largest anthropogenic source of agricultural methane and has a significant role in global warming. Consequently, innovative solutions to reduce methane emissions from livestock farming are required to ensure future sustainable food production. One possible approach is the use of lactic acid bacteria (LAB), Gram positive bacteria that produce lactic acid as a major end product of carbohydrate fermentation. LAB are natural inhabitants of the intestinal tract of mammals and are among the most important groups of microorganisms used in food fermentations. LAB can be readily isolated from ruminant animals and are currently used on-farm as direct-fed microbials (DFMs) and as silage inoculants. While it has been proposed that LAB can be used to reduce methane production in ruminant livestock, so far research has been limited, and convincing animal data to support the concept are lacking. This review has critically evaluated the current literature and provided a comprehensive analysis and summary of the potential use and mechanisms of LAB as a methane mitigation strategy. It is clear that although there are some promising results, more research is needed to identify whether the use of LAB can be an effective methane mitigation option for ruminant livestock.
Introduction
While ruminant animals play an important role in sustainable agricultural systems (Eisler et al., 2014) they are also an important source of greenhouse gas (GHG) emissions (Reisinger and Clark, 2018). Regardless of the ruminant species, the largest source of GHG emissions from ruminant production is methane (CH4), with more than 90 percent of emissions originating from enteric fermentation (Opio et al., 2013). Enteric fermentation is a digestive process by which a community of microbes present in the forestomach of ruminants (the reticulo-rumen) break down plant material into nutrients that can be used by the animal for the production of high-value proteins that include milk, meat and leather products. Hydrogen (H2) and methyl-containing compounds generated as fermentation end products of this process are used by different groups of rumen methanogenic archaea to form CH4, which is belched and exhaled from the lungs via respiration from the animal and released to the atmosphere. In the coming decades, livestock farmers will face numerous challenges and the development of technologies and practices which support efficient sustainable food production while moderating greenhouse gas emissions are urgently required. More than 100 countries have committed to reducing agricultural GHG emissions in the 2015 Paris Agreement of the United Nations Framework Convention on Climate Change, however, known agricultural practices could deliver just 21–40% of the needed reduction, even if implemented fully at scale (Wollenberg et al., 2016). New technical mitigation options are needed. Reviews of CH4 mitigation strategies consistently discuss the possibility that lactic acid bacteria (LAB) could be used to modulate rumen microbial communities thus providing a practical and effective on-farm approach to reducing CH4 emissions from ruminant livestock (Hristov et al., 2013; Takahashi, 2013; Knapp et al., 2014; Jeyanathan et al., 2014; Varnava et al., 2017). This review examines the possible contribution of LAB in the development of an on-farm CH4 mitigating strategy.
Results and Discussion
General Characteristics of Lactic Acid Bacteria
Lactic acid bacteria are Gram positive, acid tolerant, facultatively anaerobic bacteria that produce lactic acid as a major end-product of carbohydrate fermentation (Stilez and Holzapfel, 1997). Biochemically they include homofermenters that produce primarily lactic acid, and heterofermenters that also give a variety of other fermentation end-products such as acetic acid, ethanol and CO2. LAB have long been used as starter cultures for a wide range of dairy, meat and plant fermentations, and this history of use in human and animal foods has resulted in most LAB having Qualified Presumption of Safety (QPS) status in the European Union or Generally Recognized as Safe (GRAS) status in the United States. The main LAB genera used as starter cultures are Lactobacillus, Lactococcus, Leuconostoc, and Pediococcus (Bintsis, 2018) together with some species of Enterococcus and Streptococcus.
In addition to their contribution to the development of food flavor and texture, LAB have an important role in inhibiting the growth of spoilage organisms through the production of inhibitory compounds. These compounds include fermentation products such as organic acids and hydrogen peroxide as well as ribosomally synthesized peptides known as bacteriocins (Cotter et al., 2013). In many cases, the physiological role of bacteriocins is unclear but they are thought to offer the producing organism a competitive advantage, via their ability to inhibit the growth of other microorganisms, particularly in complex microbial communities. Some strains also produce other compounds such as non-ribosomally synthesized peptides which may have additional antimicrobial activity (Mangoni and Shai, 2011).
In recent years much interest has been shown in the use of LAB as probiotic organisms and in their potential contribution to human health and well-being. LAB have also been advocated as probiotics to improve food animal production and as alternatives to antibiotics used as growth promotors (Vieco-Saiz et al., 2019).
LAB and the Rumen
LAB are members of the normal gastrointestinal tract microbiota, however, in ruminants these organisms are generally only prevalent in young animals before the rumen has properly developed (Stewart et al., 1988). LAB are unable to initiate the metabolism of plant structural polysaccharides and are not regarded as major contributors to rumen fermentation. In the Global Rumen Census project (Henderson et al., 2015) which profiled the microbial community of 684 rumen samples collected from a range of ruminant species, only members of the genus Streptococcus were found in a majority of samples (63% prevalence, 0.5% abundance). Nevertheless, LAB can be readily isolated from the rumen, with some species such as Lactobacillus ruminis and Streptococcus equinus (formerly S. bovis) being regarded as true rumen inhabitants while others (Lactobacillus plantarum and Lactococcus lactis) are likely to be transient bacteria that have been introduced with the feed (Stewart, 1992). Several obligately anaerobic rumen bacteria also produce lactate as a fermentation end product and two of these are included in this review. These organisms (Kandleria vitulina and Sharpea azabuensis) are both members of the family Erysipelotrichaceae within the phylum Firmicutes, although Kandleria vitulina was formerly known as Lactobacillus vitulinus (Salvetti et al., 2011). Sharpea and Kandleria are a significant component of the rumen microbiome in low CH4 yield animals in which rapid heterofermentative growth results in lactate production (Kamke et al., 2016).
Table 1 lists the rumen LAB together with strains of Kandleria and Sharpea that have been genome sequenced along with potential antimicrobial biosynthetic clusters predicted from the genome sequence data. The majority (81%) of genome sequenced strains from rumen members of the Streptococcaceae encode antimicrobial biosynthetic clusters, and previous studies have also reported that rumen streptococci can produce a range of bacteriocins (Iverson and Mills, 1976; Mantovani et al., 2001; Whitford et al., 2001). Conversely, antimicrobial biosynthetic genes have not been identified from the species Kandleria vitulina and Sharpea azabuensis.
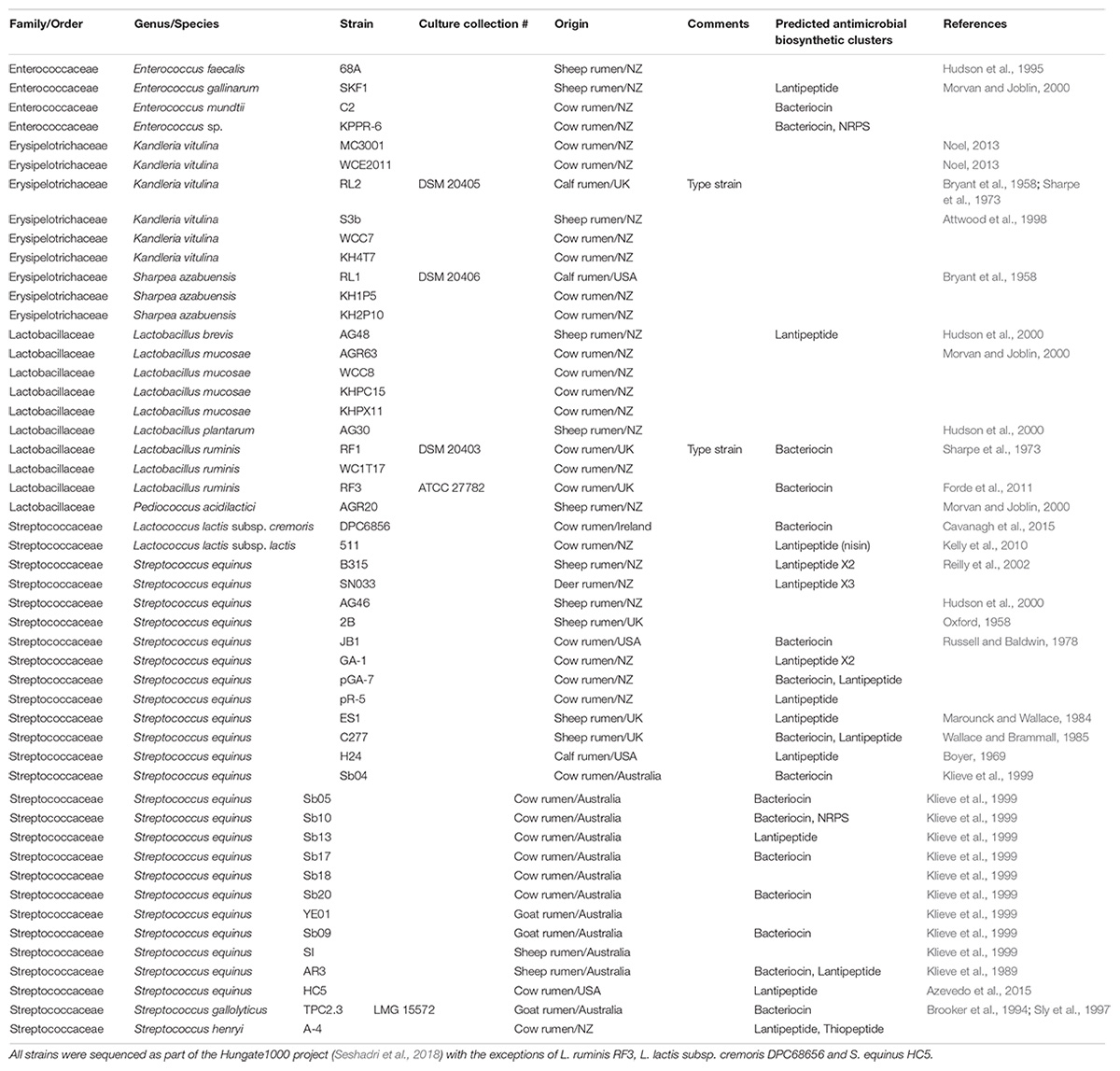
Table 1. List of rumen LAB cultures in addition to a further two species of obligately anaerobic rumen bacteria (Kandleria and Sharpea) also known to produce lactate as a fermentation end product.
How Are LAB Used in Ruminant Agriculture?
On-farm, LAB are used as direct-fed microbials (DFMs), probiotics and as silage inoculants. The terms DFM and probiotic are used interchangeably in animal nutrition and refer to any type of live microbe-based feed additive. Although the products have different purposes, there is considerable overlap in the bacterial species used.
The efficacy of DFMs containing LAB has been studied mostly in pre-ruminants where their reported benefits include a reduction in the incidence of diarrhea, a decrease in fecal shedding of coliforms, promotion of ruminal development, improved feed efficiency, increased body weight gain, and reduction in morbidity (Krehbiel et al., 2003). A meta-analysis of randomized controlled trials of LAB supplementation in young calves has shown that LAB can exert a protective effect and reduce the incidence of diarrhea (Signorini et al., 2012) and can increase body weight gain and improve feed efficiency (Frizzo et al., 2011). The meta-analysis further revealed that LAB can induce further beneficial effects if administered with whole milk and as a single strain inoculum. The use of DFM supplementation in young ruminants is expanding as farmers look to use natural alternatives to antibiotics to help improve calf health and promote growth.
In the adult ruminant, there is limited research available on the efficacy of LAB DFMs. Their use is targeted at improving the health and performance of animals (Table 2). With regard to health, a meta-analysis of trials evaluating the use of DFMs (predominantly Lactobacillus) to reduce the prevalence of Escherichia coli O157 fecal shedding in beef cattle has shown LAB supplementation to be efficacious (Wisener et al., 2015). Administration of Lactococcus lactis has been shown to be as effective as common antibiotics in the treatment of bovine mastitis (Klostermann et al., 2008). LAB DFMs have also been shown to minimize the risk of ruminal acidosis in some instances (Ghorbani et al., 2002; Lettat et al., 2012). A recent review by Rainard and Foucras (2018) appraised the use of probiotics for mastitis control. The authors concluded that based on the lack of scientific data the use of probiotics to prevent or treat mastitis is not currently recommended. However, use of teat apex probiotics deserves further research. The results from a small number of trials using only LAB supplementation treatment groups to enhance animal performance are mixed (Table 2). Studies where beneficial effects have been reported include an increase in milk yield, change in milk fat composition, improved feed efficiency, and increased daily weight gain but equally there have been studies where no change has been reported (see Table 2). Although responses to DFMs have been positive in some experiments, the basic mechanisms underlying these beneficial effects are not well defined or clearly understood.
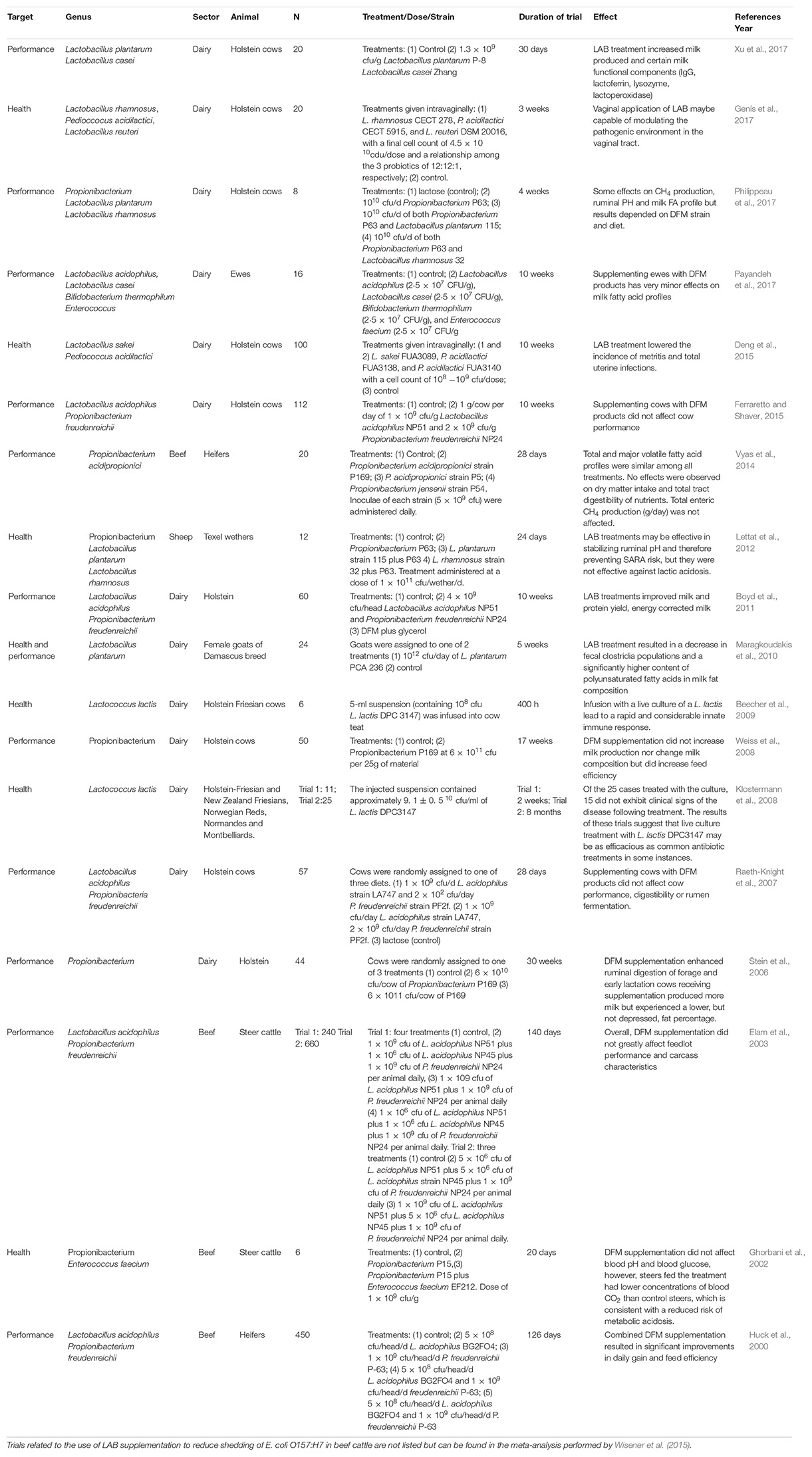
Table 2. Animal trials which studied the effect of DFM supplementation containing LAB only on ruminant performance and health.
LAB are the dominant silage inoculant in many parts of the world. LAB are used not only for their convenience and safety, but also because they are effective in controlling microbial events during silage fermentation (Muck et al., 2018). In the ensiling process, a succession of LAB ferment the available soluble sugars in cut plant material to produce organic acids, including lactic acid. As a result, the pH drops, preventing further microbial degradation of the plant material and preserving it as silage. The efficacy of adding LAB inoculants in enhancing the natural silage preservation process is well established. In addition, silage inoculants containing homofermentative LAB have not only improved silage quality and reduced fermentation losses but have also improved animal performance by increasing milk yield, daily gain and feed efficiency (Kung et al., 1993; Weinberg and Muck, 1996, 2013; Kung and Muck, 1997; Muck et al., 2018). The mechanism(s) behind the additional benefits in animal performance from feeding inoculated silage are not understood.
LAB DFMs and silage inoculants are microbial based technologies which are widely accepted and actively used in modern farming systems today. If LAB can be found to reduce ruminant CH4 production effectively then both DFMs and inoculants provide a practical and useful mitigation option on-farm.
Methanogens and the Rumen
Rumen methanogenic archaea are much less diverse than rumen bacteria (Henderson et al., 2015), and members of two clades of the genus Methanobrevibacter (referred to as M. gottschalkii and M. ruminantium) make up ∼75% of the archaeal community (Janssen and Kirs, 2008; Henderson et al., 2015). Cultivated members of both of these methanogen clades are hydrogenotrophic and use H2 and CO2 for CH4 formation. Their cell walls contain pseudomurein and have similarities to those found in Gram positive bacteria which may be relevant to their sensitivity to antimicrobial agents (Varnava et al., 2017). Other significant members of the methanogen community in the rumen are methylotrophs, producing CH4 from methyl-containing substrates, particularly methylamines and methanol. These include strains of the genus Methanosphaera and members of the family Methanomassiliicoccaceae. The former have pseudomurein-containing cell walls, while the cell envelope surrounding the Methanomassiliicoccaceae has not been characterized. The ability of rumen bacteria to produce the H2 or methyl-containing substrates required for methanogenesis has been determined from culture studies, or is able to be inferred from genome sequences, but it is not yet known which bacteria are the most important contributors in the rumen.
How could LAB reduce ruminant CH4 production? It is hypothesized that LAB could influence ruminal methanogenesis in three possible ways (Figure 1): (1) use of LAB or their metabolites to shift the rumen fermentation so that there is a corresponding decrease in CH4 production, (2) use of LAB or their metabolites to directly inhibit rumen methanogens and (3) use of LAB or their metabolites to inhibit specific rumen bacteria that produce H2 or methyl-containing compounds that are the substrates for methanogenesis.
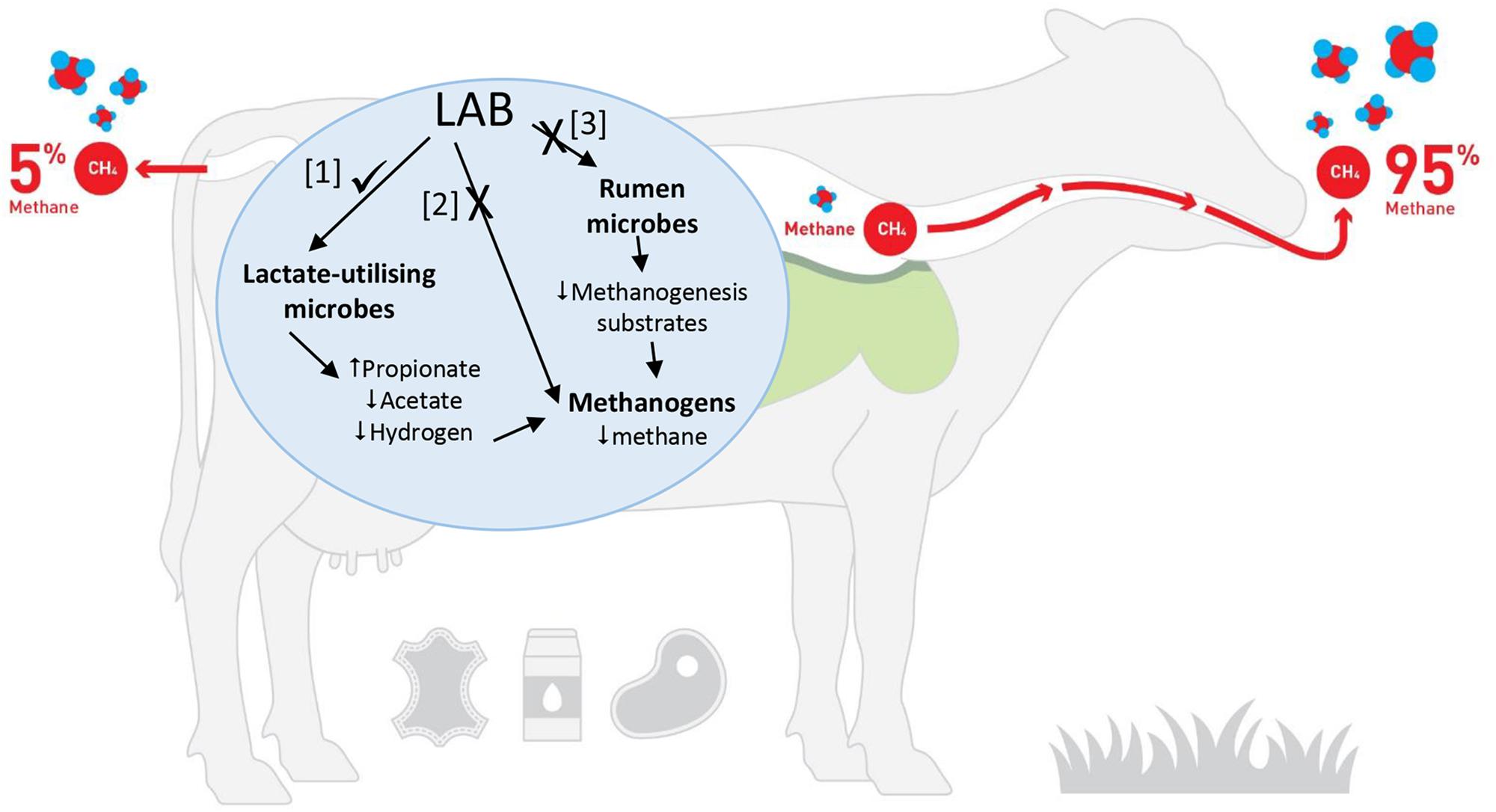
Figure 1. Potential pathways that could be modulated by LAB to decrease CH4 production [Adapted from FAO (2019). Image is being used with the permission of the copyright holder, New Zealand Agricultural Greenhouse Gas Research Centre (www.nzagrc.org.nz)].
How Have LAB Been Shown to Affect Ruminant CH4 Production?
The idea that LAB can be used to reduce CH4 production in ruminant livestock is not new. Reviews of CH4 mitigation strategies consistently refer to this possibility (Hristov et al., 2013; Takahashi, 2013; Jeyanathan et al., 2014; Knapp et al., 2014; Varnava et al., 2017). However, research on the topic has been limited and convincing data from animal trials to support this concept are lacking. Jeyanathan et al. (2016) screened 45 bacteria, including strains of LAB, bifidobacteria and propionibacteria, in 24h rumen in vitro batch incubations for their ability to reduce methanogenesis. Three strains were selected for in vivo trials in sheep (n = 12), and one strain (Lactobacillus pentosus D31) showed a 13% reduction in CH4 production (g CH4/kg/DMI) over 4 weeks when dosed at 6 × 1010 cfu/animal/day. The mechanism of action was not determined in this study, but the ability of introduced bacterial strains to persist in the rumen environment was highlighted as an important factor. Subsequent work by Jeyanathan et al. (2019) using the same strains has shown no ability to reduce CH4 emissions in dairy cows. A further two studies which examined LAB supplementation on CH4 production have had mixed results. Mwenya et al. (2004) assessed the effect of feeding Leuconostoc mesenteroides subsp. mesenteroides to sheep (n = 4). Supplementation with this strain was found to increase CH4 production (g CH4/kg/DMI) in vivo. The authors did not offer any discussion as to how a LAB strain could increase CH4 production in vivo. Astuti et al. (2018) evaluated 14 strains of L. plantarum in rumen in vitro experiments and identified strain U32 which had the lowest CH4 production value when compared to the other LAB treatment groups. The authors hypothesized the addition of LAB may have stimulated the growth of lactic utilizing bacteria leading to increased production of propionic acid and a subsequent decrease in the hydrogen availability for methane production (Astuti et al., 2018).
Research conducted on bacteriocins and their ability to reduce ruminal CH4 production has been minimal. The few bacteriocins and preparations from bacteriocin-producing lactic acid bacteria that have been examined have displayed promising results both in vitro and in vivo. Callaway et al. (1997) tested the effect of the Lactococcus lactis bacteriocin nisin on rumen fermentation in vitro and reported a 36% reduction in CH4 production. However, later work has shown nisin to be susceptible to rumen proteases limiting its potential efficacy in vivo (Russell and Mantovani, 2002). One in vivo trial has, however, reported a 10% decrease in CH4 emissions (g/kg DMI) in sheep (n = 4) fed this bacteriocin (Santoso et al., 2004). The trial was conducted for 15 days and the authors surmised that the reduction in CH4 was due to the inhibition of growth of the methanogenic microbes. Nollet et al. (1998) examined the addition of the cell-free supernatant of Lactobacillus plantarum 80 (LP80) to ruminal samples in vitro and noted an 18% decrease in CH4 production and a 30.6% reduction in CH4 when the supernatant was combined with an acetogenic culture, Peptostreptococcus productus ATCC 35244. The effect of the LP80 supernatant in combination with P. productus was also studied in vivo using two rams and it was concluded that inhibition of methanogenesis (80% decrease; mmol/6 h) occurred during the first 3 days but the effect did not persist. Compounds (PRA1) produced by L. plantarum TUA1490L were tested in vitro and found to decrease methanogenesis by 90% (Asa et al., 2010). Further work with PRA1 confirmed its ability to maintain an antimicrobial effect even after incubation with proteases but the hypothesis that the inhibition mechanism of PRA1 may relate to the production of hydrogen peroxide has not been proven (Takahashi, 2013). Bovicin HC5, a bacteriocin produced by Streptococcus equinus HC5, inhibited CH4 production by 53% in vitro (Lee et al., 2002), while more recently the bacteriocin pediocin produced by Pediococcus pentosaceus 34 was shown to reduce CH4 production in vitro by 49% (Renuka et al., 2013). The possibility of using bacteriocins from rumen streptococci for CH4 mitigation has recently been reviewed (Garsa et al., 2019). Currently, it is not clear whether the bacteriocins affect the methanogens themselves, or whether they affect the other rumen microbes that produce substrates necessary for methanogenesis. The only evidence that bacteriocins affect methanogens directly is a single article (Hammes et al., 1979) in which nisin was shown to inhibit a non-rumen methanogen, Methanobacterium, using an agar diffusion assay to determine the inhibitory effect. Recently, Shen et al. (2017) used in vitro assays and 16S rRNA gene analysis to assess the effect nisin has on rumen microbial communities and fermentation characteristics. Results demonstrate that nisin treatments can reduce populations of total bacteria, fungi and methanogens resulting in a decrease in the ratio of acetate to propionate concentrations. A similar class of compounds (antimicrobial peptides such as human catelicidin) have also been shown to be strongly inhibitory to a range of methanogens (Bang et al., 2012, 2017). There is no standardized approach to screening methanogen cultures for their susceptibility to bacteriocins, however, the method developed to facilitate screening of small molecule inhibitors (Weimar et al., 2017) should be useful. This employs the rumen methanogen strain AbM4 (a strain of Methanobrevibacter boviskoreani) which grows without H2 in the presence of ethanol and methanol (Leahy et al., 2013).
Many LAB silage inoculants possess antibacterial and/or antifungal activity and in some cases this activity is imparted into the inoculated silage (Gollop et al., 2005). The inhibitory activity has been shown to inhibit detrimental micro-organisms in silage (Flythe and Russell, 2004; Marciňáková et al., 2008; Amado et al., 2012) and has been postulated to do the same in the rumen, but the role of specific silage inoculants in CH4 mitigation has received little attention. Thus far, research has demonstrated that LAB included in freeze-dried silage inoculants can survive in rumen fluid (Weinberg et al., 2003) and that LAB survive passage from silage into rumen fluid in vitro (Weinberg et al., 2004). Several studies have demonstrated that in vitro rumen fermentation can be altered by some LAB strains. Muck et al. (2007) made silages using a range of inoculants and showed in vitro that some of the inoculated silages had reduced gas production compared with the untreated silage suggesting a shift in fermentation had occurred. Cao et al. (2010a) investigated the effect of L. plantarum Chikuso-1 on an ensiled total mixed ration (TMR) and showed CH4 production decreased by 8.6% and propionic acid increased by 4.8% compared with untreated TMR silage. Cao et al. (2011) found similar results with the same inoculant strain in vegetable residue silage with the inoculated silage having higher in vitro dry matter digestibility and lower CH4 production (46.6% reduction). Further work with this LAB strain in vivo showed that the inoculated TMR silage increased digestibility and decreased ruminal CH4 (kg DMI) emissions (24.7%) in sheep (n = 4) compared with a non-inoculated control (Cao et al., 2010b). Although more research is required in this area, the results suggest that some LAB strains are capable of altering ruminal fermentation leading to downstream effects such as reduced CH4 production.
Conclusion and Future Perspectives
Literature on the use of LAB to reduce CH4 production in ruminants is limited. In the small number of studies available, in vitro, LAB can reduce CH4 production effectively. The effect is clearly strain dependent and it is not understood whether the LAB or their metabolites affect the methanogens themselves, or whether they affect the other rumen microbes that produce substrates necessary for methanogenesis. In vivo, the lack of robust animal trials (appropriate animal numbers, relevant treatment groups, trial period, and strain efficacy) investigating LAB supplementation and CH4 mitigation make it impossible at this time to make a comprehensive conclusion. Much more research is needed to understand the mechanisms behind the use of LAB as rumen modifiers. However, if appropriate LAB cultures can be identified, and proven to be effective in vivo then a range of delivery options that are already accepted in the global farming system such as DFMs and silage inoculants are available. This represents an alternative approach to CH4 mitigation research and one that can be used in combination with other mitigation options such as vaccines (Wedlock et al., 2013) and CH4 inhibitors (Dijkstra et al., 2018) which are currently under development. Ruminant production systems with low productivity lose more energy per unit of animal product than those with high productivity. In systems where farm management practices result in an increase in performance per animal (e.g., kg milk solids per cow, kg lamb slaughtered per ewe, kg beef slaughtered per cow), and combined with a reduction in stocking rates, then absolute CH4 emissions can be reduced. LAB supplementation and use of silage inoculants can contribute to these on-farm management options that reduce agricultural GHG emissions through increases in animal productivity and improved health. LAB supplementation could offer a practical, effective and natural approach to reducing CH4 emissions from ruminant livestock and contribute to the on-farm management practices that can be used to reduce CH4 emissions.
Author Contributions
SL, WK, and GA conceived the research. ND, PM, WK, YL, and SL performed the analysis and wrote the manuscript. RR, CS, and GA reviewed the final manuscript.
Funding
METHLAB – Refining direct fed microbials (DFM) and silage inoculants for reduction of CH4 emissions from ruminants has been funded by FACCE ERA-GAS, an EU ERA-NET Cofund program whereby national money is pooled to fund transnational projects, and the European Commission also provides co-funding for the action. FACCE ERA-GAS is the ERA-NET Cofund for Monitoring and Mitigation of Greenhouse Gases from Agri- and Silvi-culture, and comprises funding agencies and project partners from 19 organizations across 13 European countries. Teagasc, the Agriculture and Food Development Authority in Ireland, is the overall coordinator of the ERA-NET. ND is in receipt of Teagasc Walsh Fellowship. The New Zealand contribution to the FACCE ERA-GAS METHLAB project is funded by the New Zealand Government in support of the objectives of the Livestock Research Group of the Global Research Alliance on Agricultural Greenhouse Gases.
Conflict of Interest
WK was employed by the company Donvis Ltd.
The remaining authors declare that the research was conducted in the absence of any commercial or financial relationships that could be construed as a potential conflict of interest.
References
Amado, I. R., Fuciños, C., Fajardo, P., Guerra, N. P., and Pastrana, L. (2012). Evaluation of two bacteriocin-producing probiotic lactic acid bacteria as inoculants for controlling Listeria monocytogenes in grass and maize silages. Anim. Feed Sci. Technol. 175, 137–149. doi: 10.1016/j.anifeedsci.2012.05.006
Asa, R., Tanaka, A., Uehara, A., Shinzato, I., Toride, Y., Usui, N., et al. (2010). Effects of protease-resistent antimicrobial substances produced by lactic acid bacteria on rumen methanogenesis. Asian-Australa. J. Anim. Sci. 23, 700–707. doi: 10.5713/ajas.2010.90444
Astuti, W. D., Wiryawan, K. G., Wina, E., Widyastuti, Y., Suharti, S., and Ridwan, R. (2018). Effects of selected Lactobacillus plantarum as probiotic on in vitro ruminal fermentation and microbial population. Pak. J. Nutr. 17, 131–139. doi: 10.3923/pjn.2018.131.139
Attwood, G. T., Klieve, A. V., Ouwerkerk, D., and Patel, B. K. C. (1998). Ammonia-hyperproducing bacteria from New Zealand ruminants. Appl. Environ. Microbiol. 64, 1796–1804.
Azevedo, A. C., Bento, C. B., Ruiz, J. C., Queiroz, M. V., and Mantovani, H. C. (2015). Draft genome sequence of Streptococcus equinus (Streptococcus bovis) HC5, a lantibiotic producer from the bovine rumen. Genome Announc. 3:e00085-e15. doi: 10.1128/genomeA.00085-15
Bang, C., Schilhabel, A., Weidenbach, K., Kopp, A., Goldmann, T., Gutsmann, T., et al. (2012). Effects of antimicrobial peptides on methanogenic archaea. Antimicrobial. Agents Chemother. 56, 4123–4130. doi: 10.1128/aac.00661-12
Bang, C., Vierbuchen, T., Gutsmann, T., Heine, H., and Schmitz, R. A. (2017). Immunogenic properties of the human gut-associated archaeon Methanomassiliicoccus luminyensis and its susceptibility to antimicrobial peptides. PLoS One 12:e0185919. doi: 10.1371/journal.pone.0185919
Beecher, C., Daly, M., Berry, D. P., Klostermann, K., Flynn, J., Meaney, W., et al. (2009). Administration of a live culture of Lactococcus lactis DPC 3147 into the bovine mammary gland stimulates the local host immune response, particularly IL-1 and IL-8 gene expression. J. Dairy Res. 76, 340–348. doi: 10.1017/S0022029909004154
Bintsis, T. (2018). Lactic acid Bacteria as starter cultures: an update in their metabolism and genetics. AIMS Microbiol. 4, 665–684. doi: 10.3934/microbiol.2018.4.665
Boyd, J., West, J. W., and Bernard, J. K. (2011). Effects of the addition of direct-fed microbials and glycerol to the diet of lactating dairy cows on milk yield and apparent efficiency of yield. J. Dairy Sci. 94, 4616–4622. doi: 10.3168/jds.2010-3984
Boyer, E. W. (1969). Amylolytic Enzymes and Selected Physiological Properties of Streptococcus bovis and Streptococcus equinus. Ph.D. thesis, Iowa State University: Ames, IA.
Brooker, J. D., O’Donovan, L. A., Skene, I., Clarke, K., Blackall, L., and Muslera, P. (1994). Streptococcus caprinus sp. nov., a tannin-resistent ruminal bacterium from feral goats. Lett. Appl. Microbiol. 18, 313–318. doi: 10.1111/j.1472-765x.1994.tb00877.x
Bryant, M. P., Small, S. N., Bouma, C., and Robinson, I. (1958). Studies on the composition of the ruminal flora and fauna of young calves. J. Dairy Sci. 41, 1747–1767. doi: 10.3168/jds.s0022-0302(58)91160-3
Callaway, T. R., Carneiro De Melo, A. M. S., and Russell, J. B. (1997). The effect of nisin and monensin on ruminal fermentations in vitro. Curr. Microbiol. 35, 90–96. doi: 10.1007/s002849900218
Cao, Y., Cai, Y., Takahashi, T., Yoshida, N., Tohno, M., Uegaki, R., et al. (2011). Effect of lactic acid bacteria inoculant and beet pulp addition on fermentation characteristics and in vitro ruminal digestion of vegetable residue silage. J. Dairy Sci. 94, 3902–3912. doi: 10.3168/jds.2010-3623
Cao, Y., Takahashi, T., Horiguchi, K., and Yoshida, N. (2010a). Effect of adding lactic acid bacteria and molasses on fermentation quality and in vitro ruminal digestion of total mixed ration silage prepared with whole crop rice. Grassl. Sci. 56, 19–25. doi: 10.1111/j.1744-697x.2009.00168.x
Cao, Y., Takahashi, T., Horiguchi, K., Yoshida, N., and Cai, Y. (2010b). Methane emissons from sheep fed fermented or non-fermented total mixed ration containing whole-crop rice and rice bran. Anim. Feed Sci. Tech. 157, 72–78. doi: 10.1016/j.anifeedsci.2010.02.004
Cavanagh, D., Casey, A., Altermann, E., Cotter, P. D., Fitzgerald, G. F., and McAuliffe, O. (2015). Evaluation of Lactococcus lactis isolates from nondairy sources with potential dairy applications reveals extensive phenotype-genotype disparity and implications for a revised species. Appl. Environ. Microbiol. 81, 3961–3972. doi: 10.1128/AEM.04092-14
Cotter, D., Ross, P., and Hill, C. (2013). Bacteriocins — a viable alternative to antibiotics? Nat. Rev. Microbiol. 11, 95–102. doi: 10.1038/nrmicro2937
Deng, Q., Odhiambo, J. F., Farooq, U., Lam, T., Dunn, S. M., and Ametaj, B. N. (2015). Intravaginal lactic acid bacteria modulated local and systemic immune responses and lowered the incidence of uterine infections in periparturient dairy cows. PLoS One 10:e0124167. doi: 10.1371/journal.pone.0124167
Dijkstra, J., Bannink, A., France, J., Kebreab, E., and van Gastelen, S. (2018). Antimethanogenic effects of 3-nitrooxypropanol depend on supplementation dose, dietary fiber content, and cattle type. J. Dairy Sci. 101, 9041–9047. doi: 10.3168/jds.2018-14456
Eisler, M. C., Lee, M. R., Tarlton, J. F., Martin, G. B., Beddington, J., Dungait, J. A., et al. (2014). Agriculture: steps to sustainable livestock. Nature 507, 32–34.
Elam, N. A., Gleghorn, J. F., Rivera, J. D., Galyean, M. L., Defoor, P. J., Brashears, M. M., et al. (2003). Effects of live cultures of Lactobacillus acidophilus (strains NP45 and NP51) and Propionibacterium freudenreichii on performance, carcass, and intestinal characteristics, and Escherichia coli strain O157 shedding of finishing beef steers. J. Anim. Sci. 81, 2686–2698. doi: 10.2527/2003.81112686x
FAO, (2019). The State of the World’s Biodiversity for Food and Agriculture, eds J. Belanger, and D. Pilling, (Rome: FAO Commission on Genetic Resources for Food and Agriculture Assessments), 572.
Ferraretto, L. F., and Shaver, R. D. (2015). Effect of direct-fed microbial supplementation on lactation performance and total-tract starch digestibility by midlactation dairy cows. Prof. Anim. Sci. 31, 63–67. doi: 10.15232/pas.2014-01369
Flythe, M. D., and Russell, J. B. (2004). The effect of pH and a bacteriocin (bovicin HC5) on Clostridium sporogenes MD1, a bacterium that has the ability to degrade amino acids in ensiled plant materials. FEMS Microbiol. Ecol. 47, 215–222. doi: 10.1016/S0168-6496(03)00259-9
Forde, B. M., Neville, B. A., O’Donnell, M. M., Riboulet-Bisson, E., Claesson, M. J., Coghlan, A., et al. (2011). Genome sequences and comparative genomics of two Lactobacillus ruminis strains from the bovine and human intestinal tracts. Microb. Cell Fact. 10(Suppl. 1):S13. doi: 10.1186/1475-2859-10-S1-S13
Frizzo, L. S., Soto, L. P., Zbrun, M. V., Signorini, M. L., Bertozzi, E., Sequeira, G., et al. (2011). Effect of lactic acid bacteria and lactose on growth performance and intestinal microbial balance of artificially reared calves. Livest. Sci. 140, 246–252. doi: 10.1016/j.livsci.2011.04.002
Garsa, A. K., Choudhury, P. K., Puniya, A. K., Dhewa, T., Malik, R. K., and Tomar, S. K. (2019). Bovicins: the bacteriocins of streptococci and their potential in methane mitigation. Probiotics Antimicrob Proteins doi: 10.1007/s12602-018-9502-z [Epub ahead of print].
Genís, S., Bach, À, and Arís, A. (2017). Effects of intravaginal lactic acid bacteria on bovine endometrium: implications in uterine health. Vet. Microbiol. 204, 174–179. doi: 10.1016/j.vetmic.2017.04.025
Ghorbani, G. R., Morgavi, D. P., Beauchemin, K. A., and Leedle, J. A. Z. (2002). Effects of bacterial direct-fed microbials on ruminal fermentation, blood variables, and the microbial populations of feedlot cattle. J. Anim. Sci. 80, 1977–1985. doi: 10.2527/2002.8071977x
Gollop, N., Zakin, V., and Weinberg, Z. G. (2005). Antibacterial activity of lactic acid bacteria included in inoculants for silage and in silages treated with these inoculants. J. Appl. Microbiol. 98, 662–666. doi: 10.1111/j.1365-2672.2004.02504.x
Hammes, W. P., Winter, J., and Kandler, O. (1979). The sensitivity of the pseudomurein-containing genus Methanobacterium to inhibitors of murein synthesis. Arch. Microbiol. 123, 275–279. doi: 10.1007/bf00406661
Henderson, G., Cox, F., Ganesh, S., Jonker, A., and Young, W., Global Rumen Census Collaborators, et al. (2015). Rumen microbial community composition varies with diet and host, but a core microbiome is found across a wide geographical range. Sci. Rep. 5:14567. doi: 10.1038/srep14567
Hristov, A. N., Oh, J., Firkins, J. L., Dijkstra, J., Kebreab, E., Waghorn, G., et al. (2013). Special topics–Mitigation of methane and nitrous oxide emissions from animal operations: I. A review of enteric methane mitigation options. J. Anim. Sci. 91, 5045–5069. doi: 10.2527/jas.2013-6583
Huck, G. L., Kreikemeier, K. K., and Ducharme, G. A. (2000). Effects of feeding two microbial additives in sequence on growth performance and carcass characteristics of finishing heifers. Kansas Agric. Exp. Station Res. Rep. Available at: http://hdl.handle.net/2097/4655
Hudson, J. A., Cai, Y., Corner, R. J., Morvan, B., and Joblin, K. N. (2000). Identification and enumeration of oleic acid and linoleic acid hydrating bacteria in the rumen of sheep and cows. J. Appl. Microbiol. 88, 286–292. doi: 10.1046/j.1365-2672.2000.00968.x
Hudson, J. A., MacKenzie, C. A. M., and Joblin, K. N. (1995). Conversion of oleic acid to 10-hydroxystearic acid by two species of ruminal bacteria. Appl. Microbiol. Biotechnol. 44, 1–6. doi: 10.1007/s002530050511
Iverson, W. G., and Mills, N. F. (1976). Bacteriocins of Streptococcus bovis. Can. J. Microbiol. 22, 1040–1047. doi: 10.1139/m76-151
Janssen, P. H., and Kirs, M. (2008). Structure of the archaeal community of the rumen. Appl. Environ. Microbiol. 74, 3619–3625. doi: 10.1128/aem.02812-07
Jeyanathan, J., Martin, C., Eugène, M., Ferlay, A., Popova, M., and Morgavi, D. P. (2019). Bacterial direct-fed microbials fail to reduce methane emissions in primiparous lactating dairy cows. J. Ani. Sci Biotech. 10:41. doi: 10.1186/s40104-019-0342-9
Jeyanathan, J., Martin, C., and Morgavi, D. P. (2014). The use of direct-fed microbials for mitigation of ruminant methane emissions: a review. Animal 8, 250–261. doi: 10.1017/S1751731113002085
Jeyanathan, J., Martin, C., and Morgavi, D. P. (2016). Screening of bacterial direct-fed microbials for their antimethanogenic potential in vitro and assessment of their effect on ruminal fermentation and microbial profiles in sheep. J. Anim. Sci. 94, 739–750. doi: 10.2527/jas2015-9682
Kamke, J., Kittelmann, S., Soni, P., Li, Y., Tavendale, M., Ganesh, S., et al. (2016). Rumen metagenome and metatranscriptome analyses of low methane yield sheep reveals a Sharpea-enriched microbiome characterised by lactic acid formation and utilisation. Microbiome 4:56.
Kelly, W. J., Ward, L. J. H., and Leahy, S. C. (2010). Chromosomal diversity in Lactococcus lactis and the origin of dairy starter cultures. Genome Biol. Evol. 2, 729–744. doi: 10.1093/gbe/evq056
Klieve, A. V., Heck, G. L., Prance, M. A., and Shu, Q. (1999). Genetic homogeneity and phage susceptibility of ruminal strains of Streptococcus bovis isolated in Australia. Lett. Appl. Microbiol. 29, 108–112. doi: 10.1046/j.1365-2672.1999.00596.x
Klieve, A. V., Hudman, J. F., and Bauchop, T. (1989). Inducible bacteriophages from ruminal bacteria. Appl. Environ. Microbiol. 55, 1630–1634.
Klostermann, K., Crispie, F., Flynn, J., Ross, R. P., Hill, C., and Meaney, W. (2008). Intramammary infusion of a live culture of Lactococcus lactis for treatment of bovine mastitis: comparison with antibiotic treatment in field trials. J. Dairy Res. 75, 365–373. doi: 10.1017/S0022029908003373
Knapp, J. R., Laur, G. L., Vadas, P. A., Weiss, W. P., and Tricarico, J. M. (2014). Invited review: enteric methane in dairy cattle production: quantifying the opportunities and impact of reducing emissions. J. Dairy Sci. 97, 3231–3261. doi: 10.3168/jds.2013-7234
Krehbiel, C. R., Rust, S. R., Zhang, G., and Gilliland, S. E. (2003). Bacterial direct-fed microbials in ruminant diets: performance response and mode of action. J. Anim. Sci. 81, 120–132.
Kung, L., Chen, J. H., Creck, E. M., and Knusten, K. (1993). Effect of microbial inoculants on the nutritive value of corn silage for lactating dairy cows. J. Dairy Sci. 76, 3763–3770. doi: 10.3168/jds.s0022-0302(93)77719-x
Kung, L., and Muck, R. E. (1997). Animal Response to Silage Additives. In: Silage: Field to Feedbunk, NRAES-99. New York, NY: Northeast Regional Agricultural Engineering Service, 200–210.
Leahy, S. C., Kelly, W. J., Li, D., Li, Y., Altermann, E., Lambie, S. C., et al. (2013). The complete genome sequence of Methanobrevibacter sp. ABM4. Stand. Genom. Sci. 8, 215–227. doi: 10.4056/sigs.3977691
Lee, S. S., Hsu, J. T., Mantovani, H. C., and Russell, J. B. (2002). The effect of bovicin HC5, a bacteriocin from Streptococcus bovis HC5, on ruminal methane production in vitro. FEMS Microbiol. Lett. 217, 51–55. doi: 10.1016/S0378-1097(02)01044-3
Lettat, A., Nozière, P., Silberberg, M., Morgavi, D. P., Berger, C., and Martin, C. (2012). Rumen microbial and fermentation characteristics are affected differently by bacterial probiotic supplementation during induced lactic and subacute acidosis in sheep. BMC Microbiol. 12:142. doi: 10.1186/1471-2180-12-142
Mangoni, M., and Shai, Y. (2011). Short native antimicrobial peptides and engineered ultrashort lipopeptides: similarities and differences in cell specificities and modes of action. Cell. Mol. Life Sci. 68, 2267–2280. doi: 10.1007/s00018-011-0718-2
Mantovani, H. C., Kam, D. K., Ha, J. K., and Russell, J. B. (2001). The antibacterial activity and sensitivity of Streptococcus bovis strains isolated from the rumen of cattle. FEMS Microbiol. Ecol. 37, 223–229. doi: 10.1016/s0168-6496(01)00166-0
Maragkoudakis, P. A., Mountzouris, K. C., Rosu, C., Zoumpopoulou, G., Papadimitriou, K., Dalaka, E., et al. (2010). Feed supplementation of Lactobacillus plantarum PCA 236 modulates gut microbiota and milk fatty acid composition in dairy goats–a preliminary study. Int. J. Food Microbiol. 141(Suppl. 1), S109–S116. doi: 10.1016/j.ijfoodmicro.2010.03.007
Marciňáková, M., Lauková, A., Simonová, M., Strompfová, V., Koréneková, B., and Nad, P. (2008). A new probiotic and bacteriocin-producing strain of Enterococcus faecium EF9296 and its use in grass ensiling. Czech J. Anim. Sci. 53, 335–344. doi: 10.17221/348-cjas
Marounck, M., and Wallace, R. J. (1984). Influence of culture Eh on the growth and metabolism of the rumen bacteria Selenomonas ruminantium, Bacteroides amylophilus, Bacteroides succinogenes and Streptococcus bovis in batch culture. J. Gen. Microbiol. 130, 223–229. doi: 10.1099/00221287-130-2-223
Morvan, B., and Joblin, K. N. (2000). Hydration of oleic acid by Enterococcus gallinarum, Pediococcus acidilactici and Lactobacillus sp. Anaerobe 5, 605–611. doi: 10.1006/anae.1999.0306
Muck, R. E., Filya, I., and Contreras-Govea, F. E. (2007). Inoculant effects on alfalfa silage: In vitro gas and volatile fatty acid production. J. Dairy Sci. 90, 5115–5125. doi: 10.3168/jds.2006-878
Muck, R. E., Nadeau, E. M. G., McAllister, T. A., Contreras-Govea, F. E., Santos, M. C., and Kung, L. (2018). Silage review: recent advances and future uses of silage additives. J. Dairy Sci. 101, 3980–4000. doi: 10.3168/jds.2017-13839
Mwenya, B., Santoso, B., Sar, C., Gamo, Y., Kobayashi, T., Arai, I., et al. (2004). Effects of including β1-4 galacto-oligosaccharides, lactic acid bacteria or yeast culture on methanogenesis as well as energy and nitrogen metabolism in sheep. Anim. Feed Sci. Tech. 115, 313–326. doi: 10.1016/j.anifeedsci.2004.03.007
Noel, S. (2013). Cultivation and Community Composition Analysis of Plant-adherent Rumen Bacteria. Ph.D. thesis, Massey University: Palmerston North.
Nollet, L., Mbanzamihigo, L., Demeyer, D., and Verstraete, W. (1998). Effect of the addition of Peptostreptococcus productus ATCC 35244 on reductive acetogenesis in the ruminal ecosystem after inhibition of methanogenesis by cell-free supernatant of Lactobacillus plantarum 80. Anim. Feed Sci. Tech. 71, 49–66. doi: 10.1016/s0377-8401(97)00135-1
Opio, C., Gerber, P., Mottet, A., Falcucci, A., Tempio, G., MacLeod, M., et al. (2013). Greenhouse Gas Emissions from Ruminant Supply Chains – A Global Life Cycle Assessment. Rome: Food and Agriculture Organization of the United Nations (FAO).
Oxford, A. E. (1958). The nutritional requirements of rumen strains of Streptococcus bovis considered in relation to dextran synthesis from sucrose. J. Gen. Microbiol. 19, 617–623. doi: 10.1099/00221287-19-3-617
Payandeh, S., Kafilzadeh, F., Juárez, M., De La Fuente, M. A., Ghadimi, D., and Martínez Marín, A. L. (2017). Probiotic supplementation effects on milk fatty acid profile in ewes. J. Dairy Res. 84, 128–131. doi: 10.1017/S0022029917000115
Philippeau, C., Lettat, A., Martin, C., Silberberg, M., Morgavi, D. P., Ferlay, A., et al. (2017). Effects of bacterial direct-fed microbials on ruminal characteristics, methane emission, and milk fatty acid composition in cows fed high- or low-starch diets. J. Dairy Sci. 100, 2637–2650. doi: 10.3168/jds.2016-11663
Raeth-Knight, M. L., Linn, J. G., and Jung, H. G. (2007). Effect of direct-fed microbials on performance, diet digestibility, and rumen characteristics of Holstein dairy cows. J. Dairy Sci. 90, 1802–1809. doi: 10.3168/jds.2006-643
Rainard, P., and Foucras, G. (2018). A critical appraisal of probiotics for mastitis control. Front. Vet. Sci. 5:251. doi: 10.3389/fvets.2018.00251
Reilly, K., Carruthers, V. R., and Attwood, G. T. (2002). Design and use of 16S ribosomal DNA-directed primers in competitive PCRs to enumerate proteolytic bacteria in the rumen. Microb. Ecol. 43, 259–270. doi: 10.1007/s00248-001-1052-2
Reisinger, A., and Clark, H. (2018). How much do direct livestock emissions actually contribute to global warming? Glob. Change Biol. 24, 1749–1761. doi: 10.1111/gcb.13975
Renuka, Puniya, M., Sharma, A., Malik, R., Upadhyay, R. C., and Puniya, A. K. (2013). Influence of pediocin and enterocin on in-vitro methane, gas production and digestibility. Int. J. Curr. Microbiol. Appl. Sci. 2, 132–142.
Russell, J. B., and Baldwin, R. L. (1978). Substrate preferences in rumen bacteria: evidence of catabolite regulatory mechanisms. Appl. Environ. Microbiol. 36, 319–329.
Russell, J. B., and Mantovani, H. C. (2002). The bacteriocins of ruminal bacteria and their potential as an alternative to antibiotics. J. Mol. Microbiol. Biotechnol. 4, 347–355.
Salvetti, E., Felis, G. E., Dellaglio, F., Castioni, A., Torriani, S., and Lawson, P. A. (2011). Reclassification of Lactobacillus catenaformis (Eggerth 1935) Moore and Holdeman 1970 and Lactobacillus vitulinus Sharpe et al., 1973 as Eggerthia catenaformis gen. nov., comb. nov. and Kandleria vitulina gen. nov., comb. nov., respectively. Int. J. Syst. Evol. Microbiol. 61, 2520–2524. doi: 10.1099/ijs.0.029231-0
Santoso, B., Mwenya, B., Sar, C., Gamo, Y., Kobayashi, T., Morikawa, R., et al. (2004). Effects of supplementing galacto-oligosaccharides, Yucca schidigera or nisin on ruminal methanogenesis, nitrogen and energy metabolism in sheep. Livest. Prod. Sci. 91, 209–217. doi: 10.1016/j.livprodsci.2004.08.004
Seshadri, R., Leahy, S. C., Attwood, G. T., Teh, K. H., Lambie, S. C., Cookson, A. L., et al. (2018). Cultivation and sequencing of rumen microbiome members from the Hungate1000 Collection. Nat. Biotechnol. 36, 359–367. doi: 10.1038/nbt.4110
Sharpe, M. E., Latham, M. J., Garvie, E. I., Zirngibl, J., and Kandler, O. (1973). Two new species of Lactobacillus isolated from the bovine rumen, Lactobacillus ruminis sp. nov. and Lactobacillus vitulinus sp. nov. J. Gen. Microbiol. 77, 37–49. doi: 10.1099/00221287-77-1-37
Shen, J., Liu, Z., Yu, Z., and Zhu, W. (2017). Monensin and nisin affect rumen fermentation and microbiota differently in vitro. Front. Microbiol. 8:111. doi: 10.3389/fmicb.2017.01111
Signorini, M. L., Soto, L. P., Zbrun, M. V., Sequeira, G. J., Rosmini, M. R., and Frizzo, L. S. (2012). Impact of probiotic administration on the health and fecal microbiota of young calves: a meta-analysis of randomized controlled trials of lactic acid bacteria. Res. Vet. Sci. 93, 250–258. doi: 10.1016/j.rvsc.2011.05.001
Sly, L. I., Cahill, M. M., Osawa, R., and Fujisawa, T. (1997). The tannin-degrading species Streptococcus gallolyticus and Streptococcus caprinus are subjective synonyms. Int. J. Syst. Bacteriol. 47, 893–894. doi: 10.1099/00207713-47-3-893
Stein, D. R., Allen, D. T., Perry, E. B., Bruner, J. C., Gates, K. W., Rehberger, T. G., et al. (2006). Effects of feeding propionibacteria to dairy cows on milk yield, milk components, and reproduction. J. Dairy Sci. 89, 111–125. doi: 10.3168/jds.S0022-0302(06)72074-4
Stewart, C. S. (1992). “Lactic acid bacteria in the rumen,” in The Lactic Acid Bacteria, ed. B. J. B. Wood, (Boston, MA: Springer), 1.
Stewart, C. S., Fonty, G., and Gouet, P. (1988). The establishment of rumen microbial communities. Anim. Feed Sci. Technol. 21, 69–97. doi: 10.1016/0377-8401(88)90093-4
Stilez, M. E., and Holzapfel, W. (1997). Lactic acid bacteria of foods and their current taxonomy. Int. J. Food Microbiol. 36, 1–29. doi: 10.1016/s0168-1605(96)01233-0
Takahashi, J. (2013). “Lactic acid bacteria and mitigation of GHG emission from ruminant livestock,” in Lactic Acid Bacteria - R & D for Food, Health and Livestock Purposes, ed. M. Kongo, (London: IntechOpen).
Varnava, K. G., Ronimus, R. S., and Sarojini, V. (2017). A review on comparative mechanistic studies of antimicrobial peptides against archaea. Biotechnol. Bioeng. 114, 2457–2473. doi: 10.1002/bit.26387
Vieco-Saiz, N., Belguesmia, Y., Raspoet, R., Auclair, E., Gancel, F., Kempf, I., et al. (2019). Benefits and inputs from Lactic Acid Bacteria and their bacteriocins as alternatives to antibiotic growth promoters during food-animal production. Front. Microbiol. 10:57. doi: 10.3389/fmicb.2019.00057
Vyas, D., McGeough, E. J., McGinn, S. M., McAllister, T. A., and Beanchemin, K. A. (2014). Effect of Propionibacterium spp. on ruminal fermentation, nutrient digestibility, and methane emissions in beef heifers fed a high-forage diet. J. Anim. Sci. 92, 2192–2201. doi: 10.2527/jas2013-7492
Wallace, R. J., and Brammall, M. L. (1985). The role of different species of bacteria in the hydrolysis of protein in the rumen. Microbiology 131, 821–832. doi: 10.1099/00221287-131-4-821
Wedlock, D. N., Janssen, P. H., Leahy, S. C., Shu, D., and Buddle, B. M. (2013). Progress in the development of vaccines against rumen methanogens. Animal 7:(Suppl 2), 244–252. doi: 10.1017/S1751731113000682
Weimar, M. R., Cheung, J., Dey, D., McSweeney, C., Morrison, M., Kobayashi, Y., et al. (2017). Development of multiwell-plate methods using pure cultures of methanogens to identify new inhibitors for suppressing ruminant methane emissions. Appl. Environ. Microbiol. 83:e00396-e17. doi: 10.1128/AEM.00396-17
Weinberg, Z. G., and Muck, R. E. (1996). New trends and opportunities in the development and use of inoculants for silage. FEMS Microbiol. Rev. 19, 53–68. doi: 10.1016/0168-6445(96)00025-3
Weinberg, Z. G., and Muck, R. E. (2013). “Potential probiotic effects of lactic acid bacteria on ruminant performance,” in Proceedings of the III International Symposium on Forage Quality and Conservation, (Piracicaba, SP: FEALQ), 47–68.
Weinberg, Z. G., Muck, R. E., and Weimer, P. J. (2003). The survival of silage inoculant lactic acid bacteria in rumen fluid. J. Appl. Microbiol. 94, 1066–1071. doi: 10.1046/j.1365-2672.2003.01942.x
Weinberg, Z. G., Muck, R. E., Weimer, P. J., Chen, Y., and Gamburg, M. (2004). Lactic acid bacteria used in inoculants for silage as probiotics for ruminants. Appl. Biochem. Biotechnol. 118, 1–10.
Weiss, W. P., Wyatt, D. J., and McKelvey, T. R. (2008). Effect of feeding propionibacteria on milk production by early lactation dairy cows. J. Dairy Sci. 91, 646–652. doi: 10.3168/jds.2007-0693
Whitford, M. F., McPherson, M. A., Forster, R. J., and Teather, R. M. (2001). Identification of bacteriocin-like inhibitors from rumen Streptococcus spp. and isolation and characterization of bovicin 255. Appl. Environ. Microbiol. 67, 569–574. doi: 10.1128/aem.67.2.569-574.2001
Wisener, L. V., Sargeant, J. M., O’Conner, A. M., Faires, M. C., and Glass-Kaastra, S. K. (2015). The use of direct-fed microbials to reduce shedding of Escherichia coli O157 in beef cattle: a systematic review and meta-analysis. Zoonoses Public Health 62, 75–89. doi: 10.1111/zph.12112
Wollenberg, E., Richards, M., Smith, P., Havlik, P., Obersteiner, M., Tubiello, F. N., et al. (2016). Reducing emissions from agriculture to meet the 2 °C target. Glob. Chang. Biol. 22, 3859–3864. doi: 10.1111/gcb.13340
Keywords: lactic acid bacteria, methane, methanogens, bacteriocins, direct-fed microbials, silage inoculants, mitigation
Citation: Doyle N, Mbandlwa P, Kelly WJ, Attwood G, Li Y, Ross RP, Stanton C and Leahy S (2019) Use of Lactic Acid Bacteria to Reduce Methane Production in Ruminants, a Critical Review. Front. Microbiol. 10:2207. doi: 10.3389/fmicb.2019.02207
Received: 12 April 2019; Accepted: 09 September 2019;
Published: 01 October 2019.
Edited by:
David R. Yanez-Ruiz, Estación Experimental del Zaidín (CSIC), SpainReviewed by:
Elvira Maria Hebert, National Scientific and Technical Research Council (CONICET), ArgentinaTimothy John Snelling, Harper Adams University, United Kingdom
Copyright © 2019 Doyle, Mbandlwa, Kelly, Attwood, Li, Ross, Stanton and Leahy. This is an open-access article distributed under the terms of the Creative Commons Attribution License (CC BY). The use, distribution or reproduction in other forums is permitted, provided the original author(s) and the copyright owner(s) are credited and that the original publication in this journal is cited, in accordance with accepted academic practice. No use, distribution or reproduction is permitted which does not comply with these terms.
*Correspondence: Sinead Leahy, c2luZWFkLmxlYWh5QGFncmVzZWFyY2guY28ubno=
†These authors have contributed equally to this work