- Université de Lille, CNRS, INSERM, CHU Lille, Institut Pasteur de Lille, U1019 – UMR 8204 – Center for Infection and Immunity of Lille, Lille, France
Copper is an essential transition metal whose redox properties are used for a variety of enzymatic oxido-reductions and in electron transfer chains. It is also toxic to living beings, and therefore its cellular concentration must be strictly controlled. We have performed in silico analyses of the predicted proteomes of more than one hundred species of β proteobacteria to characterize their copper-related proteomes, including cuproproteins, i.e., proteins with active-site copper ions, copper chaperones, and copper-homeostasis systems. Copper-related proteomes represent between 0 and 1.48% of the total proteomes of β proteobacteria. The numbers of cuproproteins are globally proportional to the proteome sizes in all phylogenetic groups and strongly linked to aerobic respiration. In contrast, environmental bacteria have considerably larger proportions of copper-homeostasis systems than the other groups of bacteria, irrespective of their proteome sizes. Evolution toward commensalism, obligate, host-restricted pathogenesis or symbiosis is globally reflected in the loss of copper-homeostasis systems. In endosymbionts, defense systems and copper chaperones have disappeared, whereas residual cuproenzymes are electron transfer proteins for aerobic respiration. Lifestyle is thus a major determinant of the size and composition of the copper-related proteome, and it is particularly reflected in systems involved in copper homeostasis. Analyses of the copper-related proteomes of a number of species belonging to the Burkholderia, Bordetella, and Neisseria genera indicates that commensals are in the process of shedding their copper-homeostasis systems and chaperones to greater extents yet than pathogens.
Introduction
β proteobacteria form a large phylogenetic group mainly composed of environmental species and a few important pathogens, notably of the Burkholderia, Bordetella, and Neisseria genera. β proteobacteria also comprise a few known phytopathogens, commensals, endophytes, symbionts, and endosymbionts. Thus, members of this phylogenetic group represent a broad range of lifestyles, though information is scarce for most identified species.
Copper is a transition metal whose redox properties are widely used notably in electron transfer chains for respiration and photosynthesis, and in enzymes involved in oxido-reduction and hydrolytic reactions. Thus, bacteria need to acquire copper ions from the milieu (Stewart et al., 2019). However, Cu(I), which can cross the cytoplasmic membrane, is toxic at high concentrations (Fu et al., 2014; Djoko et al., 2015). Toxicity is thought to be caused by the reactive hydroxyl radical generated in Fenton and Haber-Weiss type reactions, and by copper displacing iron from its sites in metallo-proteins (Solioz, 2018). In particular, the biogenesis of 4Fe-4S centers is vulnerable to copper (Macomber and Imlay, 2009), and copper can also displace iron from assembled 4Fe-4S clusters (Azzouzi et al., 2013; Djoko and McEwan, 2013). As a consequence of this toxicity, bacteria have developed ways to strictly control intracellular copper concentrations (Nies, 2003; Ladomersky and Petris, 2015). A number of systems that ensure copper homeostasis, including extrusion of copper from the cytoplasm or from the periplasm and oxidation of Cu(I) into less toxic Cu(II), have been described in a few model bacteria including Escherichia coli, Salmonella typhimurium, Pseudomonas aeruginosa, Enterococcus hirae, and Mycobacterium tuberculosis (Wolschendorf et al., 2011; Fu et al., 2014). In contrast, little in known for most other bacteria (Solioz, 2018).
Expression of copper-related proteins is controlled by specific two-component signal transduction systems and transcriptional regulators. Typically, transcription of genes or operons involved in the export of copper or its detoxification is activated when excess copper is detected in the periplasm using two-component systems such as CusRS or CopRS, or in the cytoplasm using regulators of the MerR, ArsR, or CsoR families (Ma et al., 2009; Capdevila et al., 2017; Chandrangsu et al., 2017). Acquisition systems in case of copper starvation are less well-known. Few specific import systems of copper have been described, and they are mainly dedicated to the assembly of respiration and denitrification complexes (Ekici et al., 2012; Khalfaoui-Hassani et al., 2018).
β proteobacteria include Cupriavidus metallidurans, aptly named from its capacity to survive in environments heavily contaminated with transition metals, as it was first isolated from the sludge of a decantation tank in a zinc factory (Mergeay et al., 1985). This organism is extremely well equipped to deal with excessive concentrations of those elements including copper, either by efflux, complexation or reducing precipitation (Von Rozycki and Nies, 2009; Grosse et al., 2016; Herzberg et al., 2016). Rather far from this type of niche is the obligate, host-restricted pathogen Bordetella pertussis, the whooping cough agent (Melvin et al., 2014; Linz et al., 2019). B. pertussis lives in the respiratory mucosa of humans, mainly as an extracellular pathogen. It has no known environmental reservoir and is believed to be transmitted directly between humans. We have initiated the study of copper homeostasis in B. pertussis and discovered that it has considerably streamlined its defense against copper relative to model pathogenic bacteria. Bordetella bronchiseptica, a close relative of B. pertussis with a larger genome and a more promiscuous lifestyle, that can survive in the environment in addition to infecting mammals (Taylor-Mulneix et al., 2017), has more copper-regulated defense systems against excess of this metal than B. pertussis (our unpublished observations). This finding prompted us to analyze the predicted copper-related proteomes of a large range of β proteobacteria to investigate more broadly the links between their lifestyles and the homeostasis of this metal.
Materials and Methods
Retrieval of β Proteobacterial Proteomes
All β proteobacterial species with genomic sequences in the NCBI database (release Nov 2018) were collected, resulting in 465 distinct species. Among them, those for which the genomes are completely sequenced were selected. A single bacterial species was selected for most genera, based on the numbers of publications available in the Pubmed database on each species. However, we selected one representative isolate of all the species of the three β proteobacterial genera – Bordetella, Burkholderia and Neisseria- that include important pathogens. The RefSeq genome files of the selected species were retrieved whenever available, and assembled genome files were used in the other cases. We generated the predicted proteomes of the selected species by translating all their annotated open reading frames. The proteins thus obtained were analyzed in the CLC main package to predict protein domains according to the Pfam nomenclature.
In silico Searches for Cu-Related Domains
An exhaustive search was conducted to identify all types of known copper-related protein domains that can be found in β proteobacteria. Instead of using a Blast approach to retrieve putative members of known copper-related protein families, we used family signatures as found in Pfam. Firstly, all proteins whose three-dimensional structures contain a copper ion were retrieved from the metal-specific MetalPDB database (Putignano et al., 2018) as described previously (Sharma et al., 2018). The 1397 distinct 3-dimensional structures of proteins with bound Cu were found to correspond to 4391 protein sequences, as some entries include several polypeptide chains. Pfam predictions were performed for all sequences, and 233 distinct Pfam domains were identified in that set. Among those, we determined which domains provide amino acyl residues that coordinate copper. The binding sites of Cu are mainly formed by the side chains of His, Cys, and Met residues (Rubino et al., 2011), as well as Asp and Glu according to MetalPDB. A metal binding site was considered plausible if all coordinating residues belong to a single predicted Pfam domain, yielding 27 potential Cu-binding domains. Mismetallated domains and eukaryote-specific domains were discarded.
Secondly, we used the BACMET database to identify additional Cu-related Pfam domains absent from MetalDB as described (Li et al., 2019). In BACMET, 97 proteins are reported to be involved in copper resistance in eubacteria, but this list is highly redundant. This additional search identified two new domains, CopD and CopB. BACMET also led to the identification of domains involved in metal sensing, in particular histidine sensor-kinases of two-component systems and cytoplasmic transcriptional regulators of the MerR family. However, we chose to disregard metal-sensing domains of regulation systems in our analyses, because of the difficulty to determine their metal specificity based on sequence alone (Ibanez et al., 2015). Functional studies and three-dimensional structures are generally required to establish selectivity (Pennella et al., 2003; Ma et al., 2009).
Thirdly, we searched the Pfam database by text mining for copper-related domains that might have been missed in the first two approaches. A few additional domains were found, namely CutC, NosL, Cu-oxidase_4, and NnrS.
After having identified all known copper-related domains, we searched for their presence in each of our predicted β proteobacterial proteomes using hmmsearch1. For most domains, there is no ambiguity regarding their specificity for copper. However, for others further analyses were necessary. To identify metal-specific exporters, we retrieved all the putative proteins identified as P1-type ATPases (domain E1-E2_ATPases) and RND (domain ACR-tran) transporters according to Pfam in our set of proteins. We performed BlastP analyses against the 17545 proteins of the TCDB database2 (link: TCDB FastA Sequences), which provides subcategories of transporters according to their substrates. Only hits with E values of 0.0 were selected, namely the copper resistance ATPases (TCDB category 3.A.3.5) and the metal-specific RND HME transporters (heavy metal efflux; TCDB 2.A.6.1). Note that ATPases providing copper to respiratory complexes (Gonzalez-Guerrero et al., 2010; Hassani et al., 2010) are part of a distinct TCDB category (3.A.3.27) that we did not consider in our analysis.
Multicopper oxidases (MCO) harbor at least two copper-containing domains that can also be found in other types of proteins of various functions. X-ray structures of bona fide MCOs were analyzed to determine their specific domain organizations, which are Cu-oxidase_3/Cu-oxidase/Cu-oxidase_2; Cu-oxidase_3/Cu-oxidase_2; and Cu-oxidase_3//Cu-oxidase_2. The same approach was used for copper-containing nitrite reductases, yielding the following domain organizations:
Cu-oxidase_3/Cu-oxidase; Cu-oxidase_3//Cytochrome_CB B3; Copper-bind/Cu-oxidase_3; Copper-bind/Cu-oxidase_3/Cu-oxidase_2. For nitrous oxide reductases (N2OR), the nos_propeller domain was used as the defining signature according to MetalPDB.
Three complexes of aerobic respiration, cytochrome bo ubiquinol oxidases, cytochrome C oxidases aa3, and cytochrome C oxidases cbb3, use copper and contain a COX1 domain. Cytochrome C oxidases cbb3 can be defined by the presence of a FixO Pfam domain in one of their components. TIGRFAM3 was used to determine the identities of the other two complexes. Cytochrome bo ubiquinol oxidases have a component with a CyoB domain signature (TIGR02843). For cytochrome oxidase aa3 identification, the TIGRFAM signature QoxB (TIGR02882) was used. As some nitric oxide reductases also harbor this domain, we used the KEGG oxidative phosphorylation and nitrogen metabolism reference pathways to assign unambiguously the cytochrome C oxidases aa3 in our set of proteomes.
For Copper Storage Proteins (CSPs) (Dennison et al., 2018), the only available signature is a domain of unknown function (DUF326; PF02860), and therefore we created a new, unique CSP signature. A DUF326 domain is found in 18 proteins of our set that are annotated in GenBank RefSeq files as “four-helix bundle copper-binding protein.” Using those keywords, 22 additional proteins were identified. Sequence alignments of the 40 proteins with ClustalW was used to create a new CSP profile, called CSP.hmm (Supplementary File S1), using hmmbuild1. Searching for this profile in our proteomes retrieved seven additional proteins. As CSPs can be periplasmic or cytoplasmic, they were distinguished based on the prediction of a Tat signal peptide using the Tatfind server4. Eight proteins belong to the extracytoplasmic group (Csp1/2_Ecsp), and the other 39 are cytoplasmic (Csp3_Ccsp).
Hierarchical Clustering Analyses
Hierarchical clustering was performed using the Cluster 3.0 software5 to group bacteria based on the occurrence and abundance of the various types of copper-related proteins. We used medians of copy numbers of each type of proteins in each species and dispersion of the values around the medians for hierarchical clustering, with the Correlation (uncentered) similarity metric parameter. All other parameters were kept at their default values. Hierarchical clustering was also used to identify co-occurrences of the various proteins in our bacterial set without centering around medians. Note that those analyses were performed on 86 species, as only one species was selected for the Burkholderia, Bordetella, and Neisseria genera to avoid their overrepresentation in our bacterial set. The dendrograms were exported to Figtree for visualization6.
Phylogenetic Analyses
A phylogenetic tree was built based on 16S RNA sequences using one species for each genus (86 sequences). They were aligned with cmalign of the Infernal package7 using the bacterial small subunit ribosomal RNA profile (SSU_rRNA_bacteria, RF0077). The MEGA X software8 (Kumar et al., 2018) was used to build a Neighbor-joining-tree using the Maximum likelihood algorithm with the Tamura-Nei nucleotide substitution model (Tamura and Nei, 1993) and 1000 replicates using the Bootstrap method (Felstenstein, 1985). The phylogenetic tree was visualized with FigTree.
Results
Diversity of β Proteobacteria
After collecting all β proteobacterial species whose genomes are completely sequenced, we selected one species for each genus in order to obtain as wide a range of lineages as possible while keeping the analyses to a manageable level. For the Bordetella, Neisseria, and Burkholderia genera, we selected one isolate as representative of each species. Altogether, this yielded 119 distinct species of β proteobacteria, with the largest possible variety of genera and lifestyles, including a few unclassified species (Supplementary Table S1). As no completely sequenced genomes from the Ferritrophicales, Ferrovales, and Procabacteriales orders were available at the time of our analyses, no representatives of those phylogenetic groups were included.
Many species in our set are described to live in natural milieus such as water and soils and will be called hereafter environmental bacteria, although limited information is available in many cases. The environmental species reported to frequently cause infections in specific conditions were placed in a category called environmental/opportunists. Bona fide pathogens were sorted according to their types of hosts, yielding animal pathogens and phytopathogens. The single species of fungus pathogen was included in the group of environmental bacteria. We also identified a few endophytes, symbionts, endosymbionts, and commensal species. Commensals responsible for opportunistic infections were placed in a separate category.
We generated the complete predicted proteomes of all selected isolates and determined the Pfam domain(s) present in each protein. The largest proteome sizes in the various categories of bacteria are those of opportunists, environmental/opportunists and pathogens, although there are considerable variations within each of those groups (Figure 1A). Endosymbionts are at the other end of the spectrum, as expected. Strikingly, the proteome size of Verminephrobacter eisiniae, a heritable extracellular earthworm symbiont, is similar to those of environmental β Proteobacteria (Pinel et al., 2008; Lund et al., 2014).
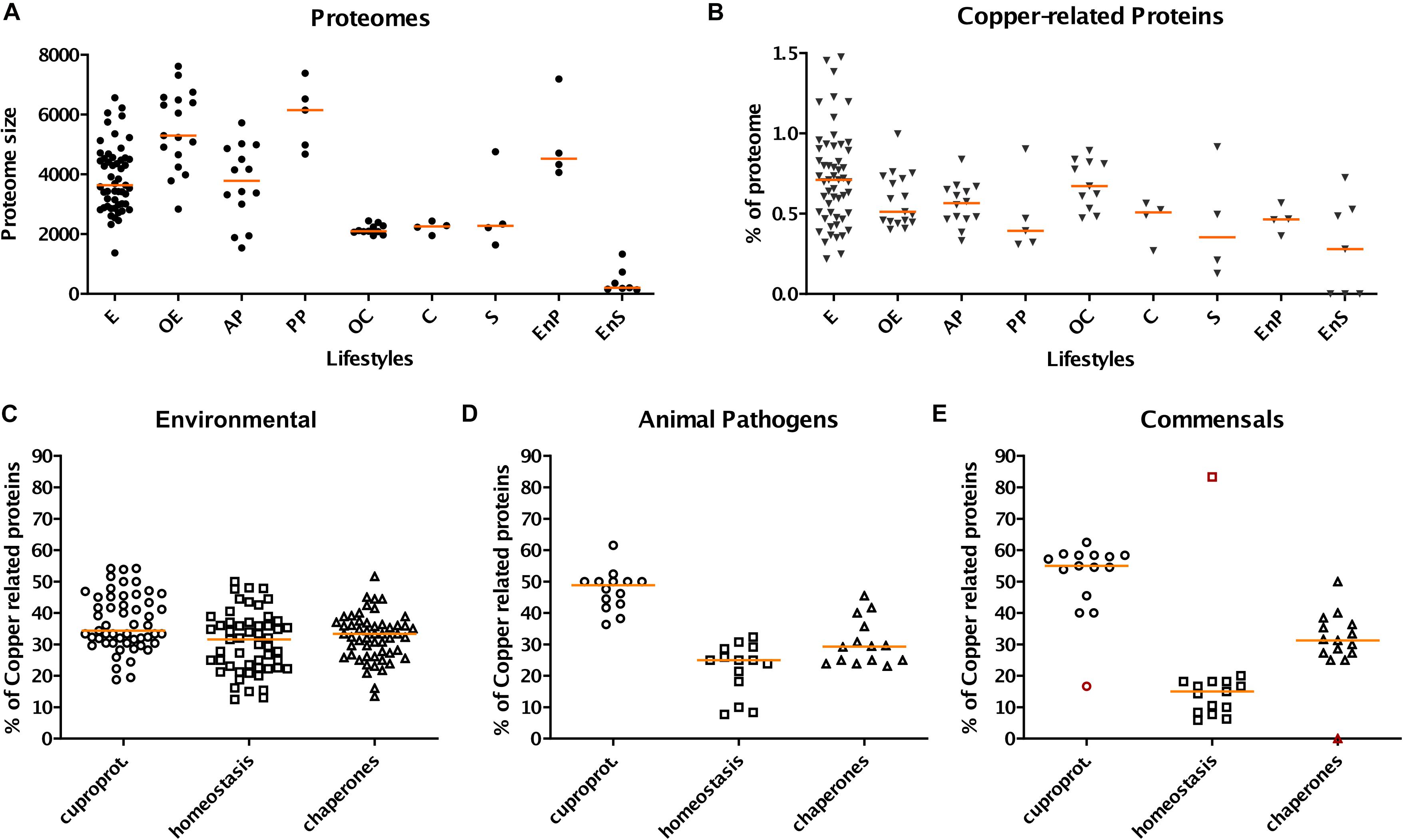
Figure 1. Profiles of copper-related proteomes as a function of the lifestyles of β proteobacteria. The bacteria were grouped according to their lifestyles, and various data were extracted from their predicted proteomes. E, OE, AP, PP, OC, C, S, EnP, and EnS represent environmental, opportunistic/environmental, animal pathogen, phytopathogen, opportunistic/commensal, commensal, symbiotic, endophytic, and endosymbiotic bacteria, respectively. (A) Sizes (in numbers of proteins) of the total proteomes as a function of the lifestyles of β proteobacteria. (B) Proportions (in%) of the copper-related proteomes relative to the entire proteomes. The copper-related proteomes include all predicted proteins of the three categories described in the text (cuproproteins, copper-homeostasis proteins, and copper chaperones). (C–E) Proportions of cuproproteins, copper-homeostasis proteins, and copper chaperones relative to the copper-related proteomes in selected bacterial groups, namely environmental bacteria (C), animal pathogens (D), and commensals (E). Note that in panel E both commensals and commensals/opportunists were included in order to increase the number of species in that group. The red symbols in panel E correspond to O. formigenes. In each panel, the different species are each represented by one symbol. The orange horizontal lines represent the medians of the values for the various groups.
Identification of Copper-Related Proteins in the Selected Set of β Proteobacteria
We inventoried all predicted proteins that harbor Cu-related domain(s). As several of those domains are frequently associated with one another, some proteins or protein complexes in our set include more than one Cu-related domain (Table 1). Copper-related proteins were sorted in several categories. The first encompasses cuproproteins, i.e., proteins that harbor copper ion(s) necessary for their activity. Fourteen distinct families of cuproproteins are present in β proteobacteria. The second group corresponds to proteins mediating copper homeostasis, in particular defense against copper. Resistance to excess copper mainly consists in transporting it from the cytoplasm to the periplasm via P1B-type copper ATPases (Migocka, 2015) and from the periplasm to the external milieu via heavy metal exporters (HME) of the resistance/nodulation/division (RND) superfamily of transporters (Kim et al., 2011). An additional line of defense is to detoxify Cu(I) by oxidation to Cu(II) using multi-copper oxidases (MCOs) (Singh et al., 2004; Kaur et al., 2019). Note that in addition to mediating Cu homeostasis, MCOs are bona fide cuproproteins.
Regarding the other facet of copper homeostasis, i.e., import of copper as a micronutrient, few systems have been described. Reports that specific P1B-type copper ATPases might import rather than expel copper have thus far not been convincingly substantiated (Solioz, 2018). Specific major facilitator superfamily (MFS) importers take up copper across the cytoplasmic membrane, notably for cytochrome C oxidase assembly (Ekici et al., 2012; Khalfaoui-Hassani et al., 2018). TonB-dependent receptors (TBDR) that transport copper across the outer membrane have also been reported, notably in Pseudomonas (Yoneyama and Nakae, 1996; Wunsch et al., 2003). However, as the MFS and TBDR families include many paralogs but no clear signatures specify copper transporters, we chose to not include those two classes of transporters in this analysis. Other proteins potentially involved in copper homeostasis include the periplasmic and inner membrane proteins CopC and CopD, respectively, reported to form a system that sequesters copper in the periplasm (Cooksey, 1994), and other proteins of ill-defined functions, the outer membrane protein CopB (Lawaree et al., 2016) and the periplasmic protein CopK (Monchy et al., 2006).
The third category includes copper-specific chaperones (Robinson and Winge, 2010; Capdevila et al., 2017), most of which play more than one role. Cu chaperones transfer copper for cuproprotein assembly or participate in copper extrusion by handing copper over to exporters. They also buffer copper, which may contribute to limiting its toxicity (Corbett et al., 2011; Osman et al., 2013; Vita et al., 2016; Solioz, 2018; Novoa-Aponte et al., 2019; Utz et al., 2019). We chose to place Csp3_Ccsp (cytosolic copper storage proteins) both in the homeostasis and copper chaperone categories, based on its putative functions (Dennison et al., 2018).
A first observation is that the proportion of copper-related proteins in a given species is not necessarily related to its proteome size (Figures 1A,B). Among those, cuproproteins account for 40% of copper-related proteins, homeostasis proteins for 28% and copper chaperones for 32%, using medians. However, those proportions vary depending on the lifestyles. Thus, pathogens, commensals and symbionts have greater proportions of cuproproteins and chaperones and lower proportions of homeostasis systems than environmental bacteria (Figures 1C–E, Supplementary Figure S1 and Supplementary Table S2). Endophytes and symbionts have few copper-related proteins, which are mostly cuproproteins, and hardly any homeostasis systems (Supplementary Figure S1 and Supplementary Table S2). For phytopathogens, the dispersion of the values is too large and the size of the samples is too small to identify trends.
In environmental bacteria, by far our largest sample, the situation is rather contrasted, likely depending on their respective niches. Using medians, copper-related proteins make up 0.71% of their proteomes, of which 34, 33, and 33% are cuproproteins, copper-homeostasis proteins, and copper chaperones, respectively (Figures 1B,C). Nitrosomonadales have particularly high proportions of proteins of all three categories relative to the sizes of their proteomes (Supplementary Table S2). Some Burkholderiales including C. metallidurans are well equipped to deal with excess copper. The champion is Herminiimonas arsenicoxydans, with 1.48% of its medium-size proteome made of copper-related proteins (Figure 2).
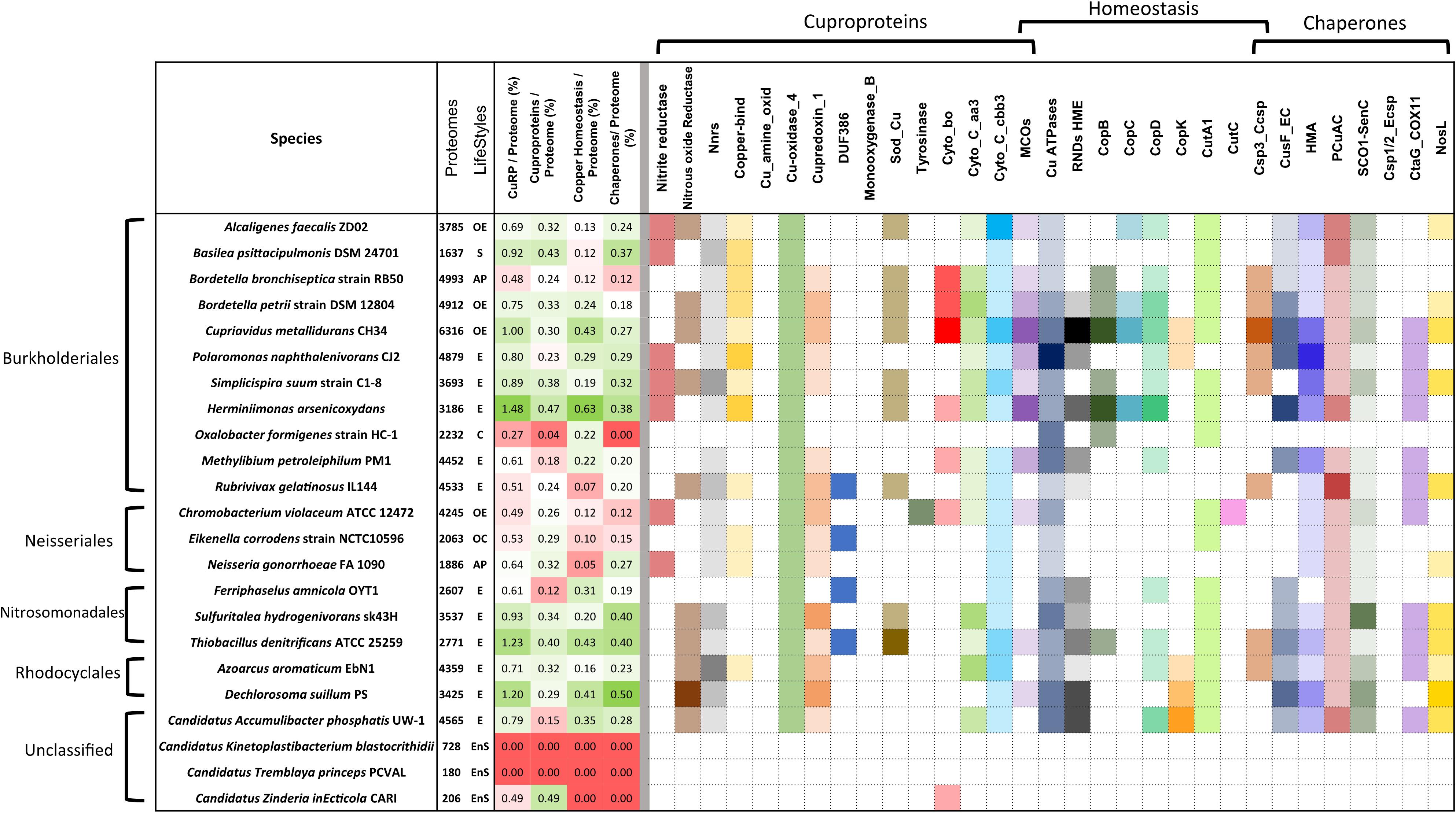
Figure 2. Copper-related proteomes in representative species of β proteobacteria. A limited number of species was selected that represent various orders of β proteobacteria (Burkholderiales, Neisseriales, Rhodocyclales, Nitrosomonadales, and unclassified) and various lifestyles. The full set of data describing the 119 species is provided in Supplementary Figure S1. The sizes of the proteomes (numbers of proteins) and the lifestyles are given in the second vertical panel, with the same abbreviations as in the legend to Figure 1. In the third vertical panel are shown the proportions (in%) of the copper-related proteomes (CuRP) relative to the complete proteomes for each species, and the proportions (in%) of the proteins in the three functional categories (cuproproteins, copper-homeostasis proteins, and copper chaperones) relative to the complete proteomes. In that panel, the white, green, and red background colors represent average values, above-average values, and below-average values, respectively, relative to the averages for the 119 species. The intensities of the colors are proportional to the distances to the average values. The abundances of each type of proteins in each bacterial species are represented in the last panel. Note that MCOs are both cuproproteins and copper-homeostasis proteins, and Csp3_Ccsps (cytoplasmic copper storage proteins) are both copper-homeostasis proteins and copper chaperones. The intensities of the colors increase with the number of paralogs in each bacterial species, with white indicating the absence of the protein. The absolute numbers of proteins of each type in each bacterial species can be found in Supplementary Table S2.
Analysis by Types of Proteins
Use of copper in β proteobacteria is largely linked to aerobic respiration, as the most represented cuproproteins are cytochrome C oxidases aa3 and cbb3 (Figure 2 and Supplementary Table S2 and Supplementary Figure S1). Very few species in our set lack both types of cytochrome C oxidases, including one uncharacterized Bordetella species. Cytochromes bo ubiquinol oxidase complexes are present in half of our bacterial set. The only bacteria totally devoid of genes for aerobic respiration are Oxalobacter formigenes, a commensal of the human digestive tract, and two endosymbiont candidates with minute proteomes, Tremblaya princeps and Vidania fulgoroideae.
Neisseriales have few cytochrome C oxidases or ubiquinol oxidases, but they possess complexes for respiration on oxidized nitrogen species two of which, nitrite reductase (Mellies et al., 1997) and N2OR, are cuproproteins. Genes for those proteins are also found in some environmental bacteria, including ammonium-oxidizing bacteria (Casciotti and Ward, 2001), some Rhodocyclacles and some Burkholderiales (Figure 2 and Supplementary Figure S1). NnrS is a cuproprotein linked to nitrosative stress (Patra et al., 2019). It is present in most β proteobacteria, at several copies in Rhodocyclales, Nitrosomonadales, Neisseriales, and some Burkholderiales.
Small copper-containing plastocyanin-like electron transfer proteins and cupredoxins are also broadly present in β Proteobacteria, with genes found at 1–3 copies in more than half of the genomes. Largest numbers are in environmental bacteria (Supplementary Table S2).
Periplasmic MCOs are both cuproproteins and proteins involved in copper homeostasis, using O2 to mediate oxidation of Cu(I) to Cu(II) as a means to reduce its toxicity. The numbers of MCO-coding genes vary from 0 in symbionts, some animal pathogens and anaerobes, to four or five in C. metallidurans, H. arsenicoxidans, Ralstonia solanacearum, and Nitrosomonas europaea (Supplementary Table S2).
Cu, Zn superoxide dismutases (SodC) protect the cell from superoxide stress, either exogenous or endogenous (Battistoni, 2003; Broxton and Culotta, 2016; Larosa and Remacle, 2018). sodC genes are found in half of the species, with two copies in a few environmental species. Most endosymbionts and a majority of Neisseriales are devoid of sodC, consistent with micro-aerophilic environments (Figure 2 and Supplementary Figure S1).
A single gene copy for laccase (multicopper polyphenol oxidase) is found in most β proteobacteria. Finally, other categories of cuproproteins are present in more limited numbers of species, most likely for specific metabolisms (Figure 2 and Supplementary Figure S1).
Cu Homeostasis in β Proteobacteria
Resistance to copper is mediated by several mechanisms involving Cu-specific ATPases, RND transporters, MCOs, in some cases coupled with export across the outer membrane (Lawaree et al., 2016), and sequestration by copper-binding proteins, including copper chaperones, in the two compartments. As chaperones also participate in Cu traffic within cells, they are considered separately.
Genes for copper-specific P1B-type ATPases are found in 106 species from our set, making those systems the most widespread mechanisms of defense against copper in β proteobacteria. The largest numbers, up to 5, are found in specific environmental bacteria (Figure 3B). Genes for RND-mediated export by heavy metal exporters (HME) are present in 62 species. Up to 10 RND HME-coding genes are present in some environmental bacteria, irrespective of the size of their proteome, a strong indication that those bacteria are exposed to transition metals in their environment (Figure 3A). It is, however, difficult to determine which of those might be involved in copper efflux. In contrast, other environmental bacteria, symbionts, and animal pathogens do not possess such genes at all, indicating that this defense feature is strongly correlated with lifestyle. For instance, Neisseriales and most animal pathogens have no HME genes but have at least one Cu-ATPase gene (Figure 2 and Supplementary Figure S1). As the two types of systems expel copper from different cellular compartments, its removal from the cytoplasm might be sufficient for bacteria that have no environmental phase in their life cycles.
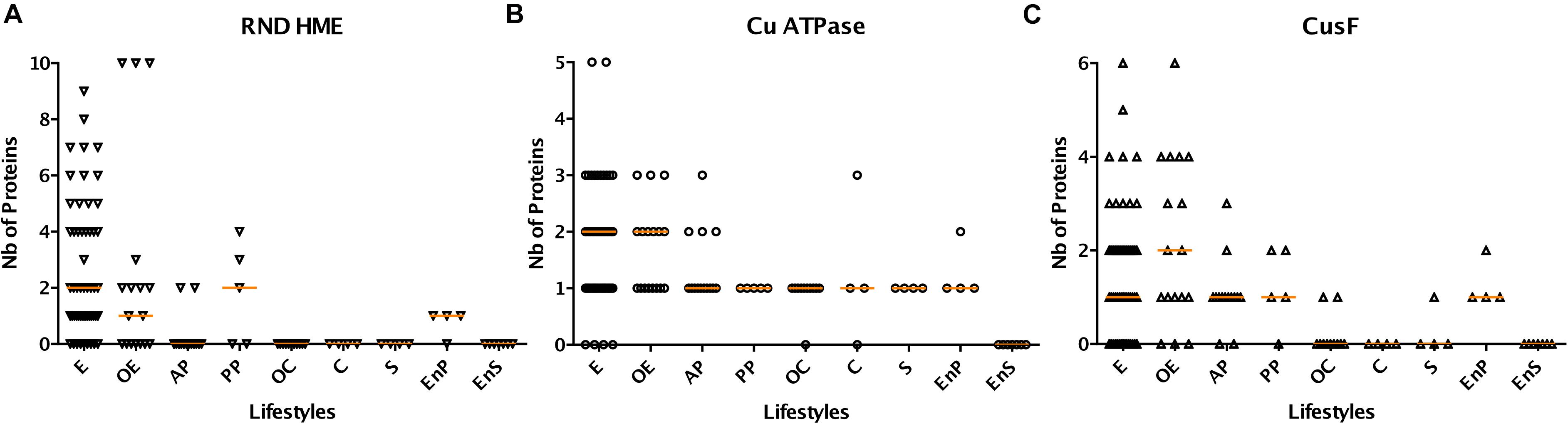
Figure 3. Abundances of selected classes of copper-related proteins (RND HME in A, Cu ATPase in B and CusF in C) in β proteobacteria as a function of their lifestyles. Absolute numbers are shown here, and the horizontal orange lines represent the medians of the values for each group. E, environmental bacteria; OE, opportunistic/environmental bacteria; C, commensal bacteria; OC, opportunistic/commensal bacteria; AP, animal pathogens; PP, phytopathogens; EnP, endophytes; S, symbionts; EnS, endosymbionts. The orange horizontal lines represent the medians of the values for the various groups.
Among other proteins reported to mediate Cu homeostasis, several copies of CopC and CopD are found in bacteria that live in metal-rich environments and in some Burkholderiae, whereas symbionts and most animal and phytopathogens are devoid of them (Figure 2). This suggests a role for defense against copper, in line with early reports (Silver and Ji, 1994). Other poorly characterized proteins, including CopB, CopK, CutA1, and CutC are absent from the genomes of most β Proteobacteria or present in low copy numbers, indicating that they likely represent specific adaptations of a limited number of genera.
Cu Metallochaperones
The isolated HMA domain, called CopZ in model bacteria, has several roles: it sequesters Cu in the cytoplasm and also hands Cu(I) to Cu-ATPases, either as a means of defense or to supply copper for cytochrome C oxidase assembly (Corbett et al., 2011; Utz et al., 2019). Among the single-domain HMA proteins in our set, greatest numbers are found in environmental bacteria including C. metallidurans, H. arsenicoxydans, and Polaromonas naphtalenivorans. Very few β proteobacteria do not have any, supporting its role for copper homeostasis in that phylogenetic group.
CusF is a periplasmic Cu-binding protein that transfers copper to the RND HME transporter CusABC for extrusion of Cu to the external milieu in model bacteria. Largest numbers of cusF genes are found in B. multivorans, Alicycliphilus denitrificans, H. arsenicoxydans, Dechloromonas suillum, Polaromonas naphtalenivorans, i.e., opportunists or environmental bacteria that possess multiple RND HME systems (Figure 3C). However, cusF is present in a number of species devoid of RND HMEs, in particular in animal pathogens including all pathogenic Bordetellae (Figure 2 and Supplementary Figure S1). It must thus fulfill another role in the absence of RND HME systems.
The PCuAC and ScoI-SenC chaperones are involved in the assembly of respiration or photosynthetic complexes or of nitrite reductase (Jen et al., 2015), and they have also been reported to participate in copper homeostasis (Trasnea et al., 2016). They are found in most β proteobacterial proteomes, except for O. formigenes, Candidatus Symbiobacter mobilis, endosymbionts and others that have few or no genes for aerobic respiration (Supplementary Figure S1). NosL is a Cu chaperone involved in the assembly of N2OR (Zumft, 2005), and accordingly it is mostly found in species with that enzyme. Finally, genes for copper storage proteins (Dennison et al., 2018) Csp3_Ccsp (cytoplasmic Cu storage) are present in a number of environmental bacteria as well as in several Bordetellae. As for Csp1/2_Ecsp (periplasmic Cu storage) proteins, they are only found in a few species, including several Neisseriales (Supplementary Figure S1).
Co-occurring Copper-Related Proteins in β Proteobacteria
Hierarchical clustering was performed on 86 species with a single representative of each bacterial genus, including Burkholderia cepacia, B. bronchiseptica, and N. gonorrheae. Most of the co-occurrences of copper-related proteins in that bacterial set revealed by those analyses were expected based on the functions of the respective proteins (Figure 4). Thus, RND HME exporters and CusF, which form export systems across the outer membrane, are often found together. Similarly, isolated HMA domains have been described to transfer copper to Cu-ATPases for export from the cytoplasm, and accordingly, the two proteins cluster in our set. We also observed co-occurrence of RND HME and Cu-ATPases. This is in good agreement with the report that the two systems can synergize for the defense against copper (Padilla-Benavides et al., 2014). The chaperone NosL cluster with N2OR, as expected from its role for N2OR assembly. Other associations revealed by our analyses include MCO with the OMP CopB, cupredoxin with SenC, Csp3 with Cyto_bo, PcuAC and Cu-oxidase_4, and Csp1/2 with DUF386. Some of those may provide indications on the putative functions of little characterized copper-related proteins, e.g., for the assembly of specific complexes.
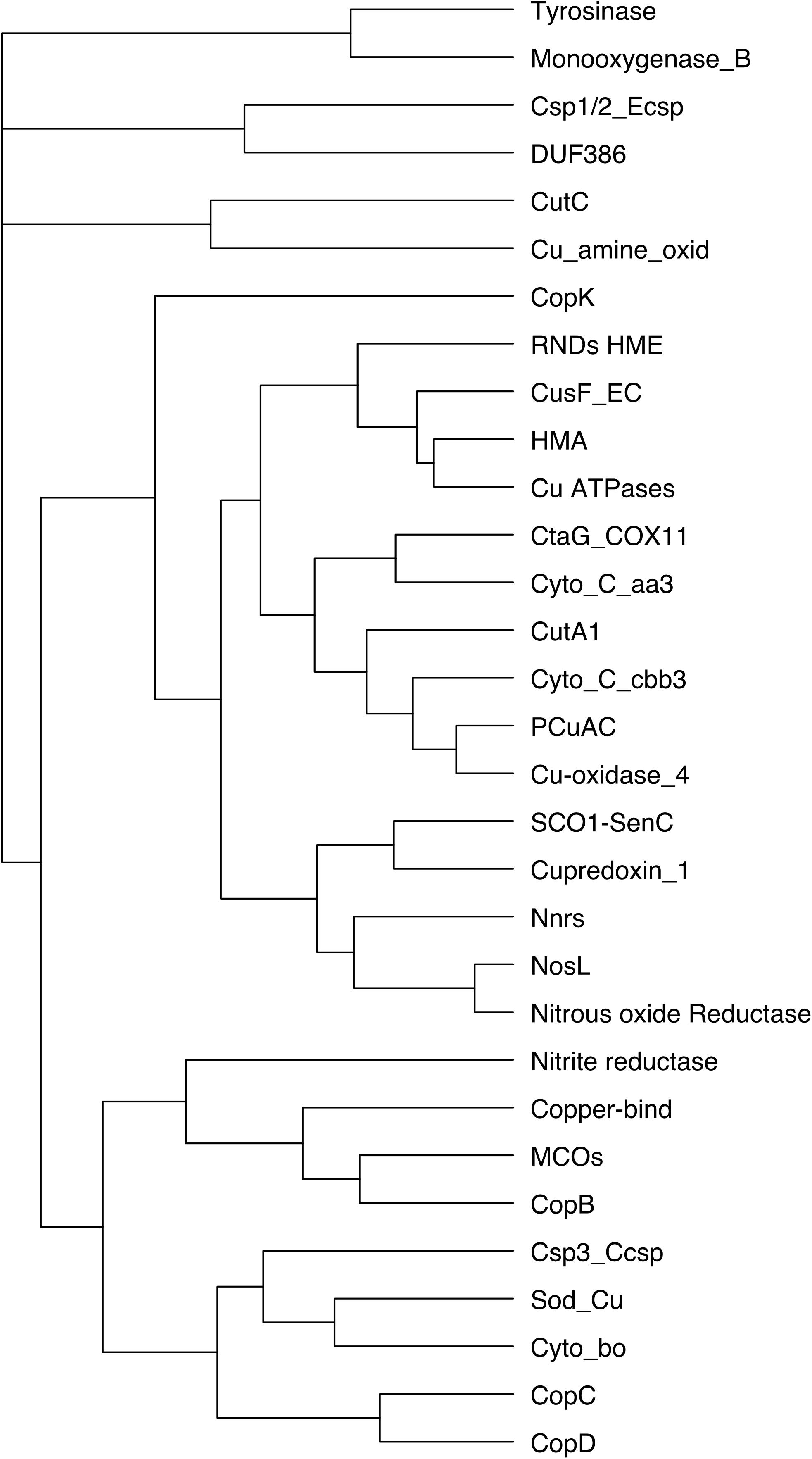
Figure 4. Clustering of copper-related proteins in β proteobacteria. Hierarchical clustering was performed for the 31 protein types and 86 species (including one species of each of the Neisseria, Burkholderia, and Bordetella genera) to identify co-occurrences of proteins in β proteobacteria. FigTree was used to visualize the results. The number of nodes between two proteins is negatively correlated with their co-occurrence.
Classification of β Proteobacteria According to Their Copper-Related Profiles
We also performed clustering of the bacterial species based on their respective copper-related proteomes and compared this classification with a 16S-RNA-based phylogenetic tree (Figures 5A,B). This analysis was performed on 86 species as above to avoid overrepresentation of the Burkholderia, Neisseria and Bordetella genera. As our results have indicated that the copper homeostasis protein complements found in β proteobacteria appear to correlate with lifestyles better than cuproproteins or chaperones, we first used the homeostasis subset of copper-related proteins to perform hierarchical clustering. Interestingly, those analyses yielded a tree in which bacteria with a host-associated lifestyle form a separate cluster (top branch in Figure 5B) from environmental bacteria, which form several other large clusters, most likely related to their niches. Outliers include O. formigenes, a commensal with a very different set of copper-related proteins than other commensals, and two other bacteria. Hierarchical clustering gives rather different results than the 16S-RNA-based phylogenetic tree with the same 86 species (Figure 5A).
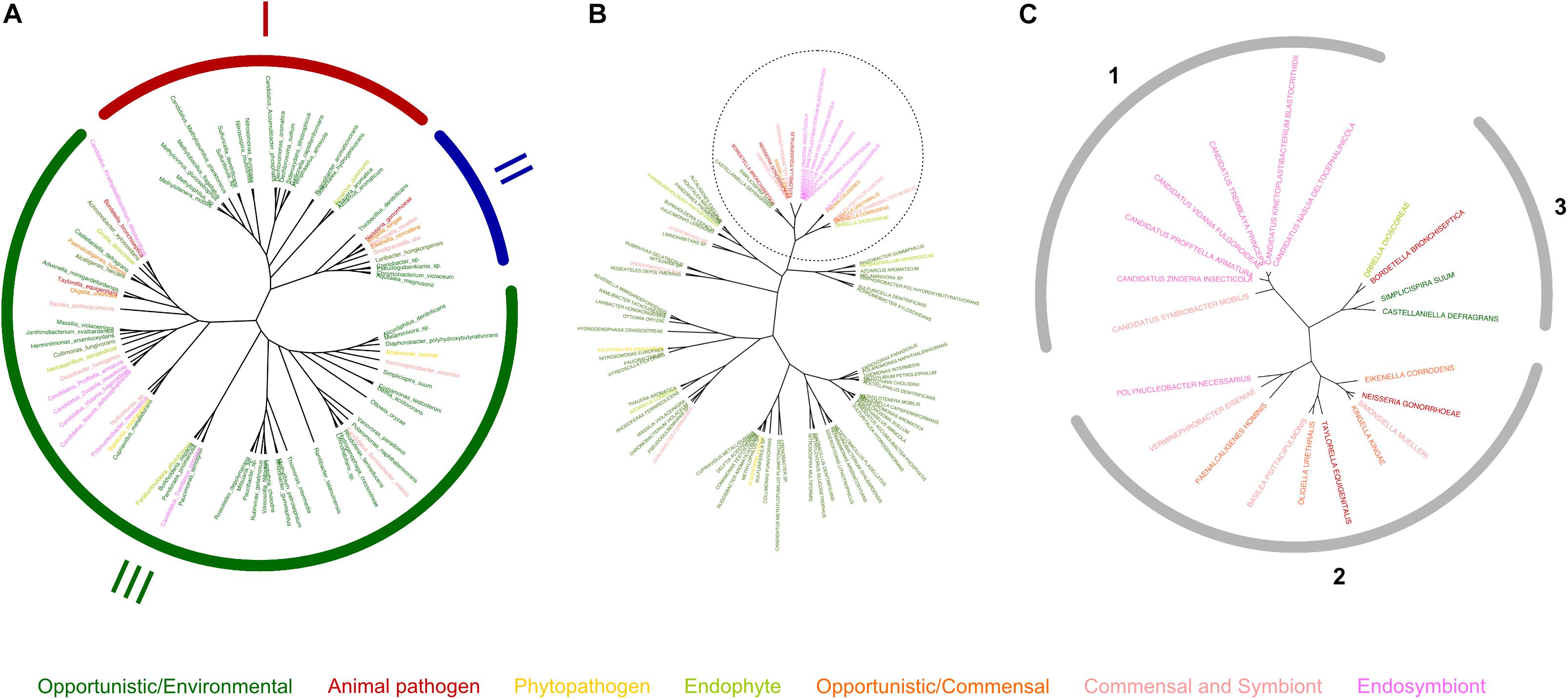
Figure 5. Hierarchical clustering of β proteobacteria based on their copper-related proteomes. (A) Phylogenetic tree based on the 16S RNA sequences. For readability, the branches of the tree do not represent true phylogenetic distances, and only one species of Burkholderia (B. cepacia), Bordetella (B. bronchiseptica), and Neisseria (N. gonorrhoeae) were used in these analyses. The tree thus comprises 86 species that form three large phylogenetic groups, namely Rhodocyclales and Nitrosomonadales (I), Nesseriales (II), and Burkholderiales (III). (B) Tree representation of the hierarchical clustering of the 86 bacterial species based on their sets of copper-homeostasis proteins (composition and numbers). The color code is as follows: dark green, environmental and opportunistic/environmental bacteria; pale green, endophytes; yellow, phytopathogens; salmon pink, commensal bacteria and symbionts; pink, endosymbionts; orange, opportunistic/commensal bacteria; red, animal pathogens. (C) The upper branch of the tree shown in (B) (21 species) was further analyzed by hierarchical clustering based on the complete sets of copper-related proteins in the bacteria present in that branch. The results are represented as a tree. The three groups that result from this analysis are (1) bacteria fully dependent on host cells (endosymbionts); (2) extracellular bacteria that depend on hosts; and (3), environmental bacteria. See text for details.
In an attempt to refine the sorting of bacteria with host-associated lifestyles, we performed a second round of hierarchical clustering with the subset of bacteria (21 species) found in the upper branch of the tree shown in Figure 5B, this time based on their entire complements of copper-related proteins. This analysis revealed distinct groups (Figure 5C). Those found in group 1 are totally dependent on a host cell. They are all endosymbionts, except for Candidatus S. mobilis, which forms a consortium by maintaining cell-to-cell contact with Chlorobium chlorochromatii, a non-motile photolithoautotrophic green sulfur bacterium (see Supplementary Table S1). Unlike C. chlorochromatii, Candidatus S. mobilis fully depends on this symbiosis. Bacteria found in group 2 are extracellular but host-dependent bacteria, i.e., symbionts, commensals, and pathogens. One exception is Polynucleobacter necessarius, an endosymbiont of a protist. Its large proteome and its free living Polynucleobacter asymbioticus relative suggest that this symbiosis evolved recently. Bacteria found in group 3 live in the environment, even if one of them, B. bronchiseptica, is also an animal pathogen. Altogether, thus, the known copper-related proteomes of β proteobacteria correlate reasonably well with their lifestyles and niches.
Copper-Related Proteins in Specific β Proteobacterial Genera
We took advantage of the availability of the full genomic sequences of large numbers of species of Bordetella (15), Burkholderia (11), and Neisseria (10) to perform more detailed analyses of the relationship between lifestyle and copper-related proteomes. All Neisseriae have small-size proteomes and are mostly commensals (Liu et al., 2015). However, N. meningitidis, a commensal of the human nasopharynx, is responsible for life-threatening meningitis or sepsis when it breaches the epithelial barrier, and N. gonorrhoeae, an obligate human-restricted pathogen, infects the genital tract. In contrast, the 11 species of Burkholderia all have large proteomes. They are environmental species, phytopathogens, or patho-opportunists that can cause serious infections (Mahenthiralingam et al., 2005; Cui et al., 2016). With 15 representatives, the genus Bordetella displays more varied lifestyles, including obligate host-restricted pathogens, environmental species, wide-host-range pathogens, commensals, opportunists, and uncharacterized species (Linz et al., 2019) (Supplementary Table S1).
The proportions of copper-related proteins relative to the total proteomes vary more widely among Burkholderiae and Bordetellae than among Neisseriae (Figure 6). Environmental and opportunistic species of the three genera generally have more cuproenzymes, in particular involved in aerobic respiration in most Bordetella and Burkholderia species, than obligate pathogens or commensals. In contrast, Neisseriae have few Cu-containing subunits of aerobic respiratory chains, but they harbor other cuproenzymes, i.e., nitrite reductase and/or N2OR, that are absent from most Bordetellae and Burkholderiae. Neisseriae are devoid of MCOs, unlike the other two genera. Interestingly, plastocyanin-like proteins are found in all Neisseriae and a majority of Bordetellae, but they are absent from all but one Burkholderia.
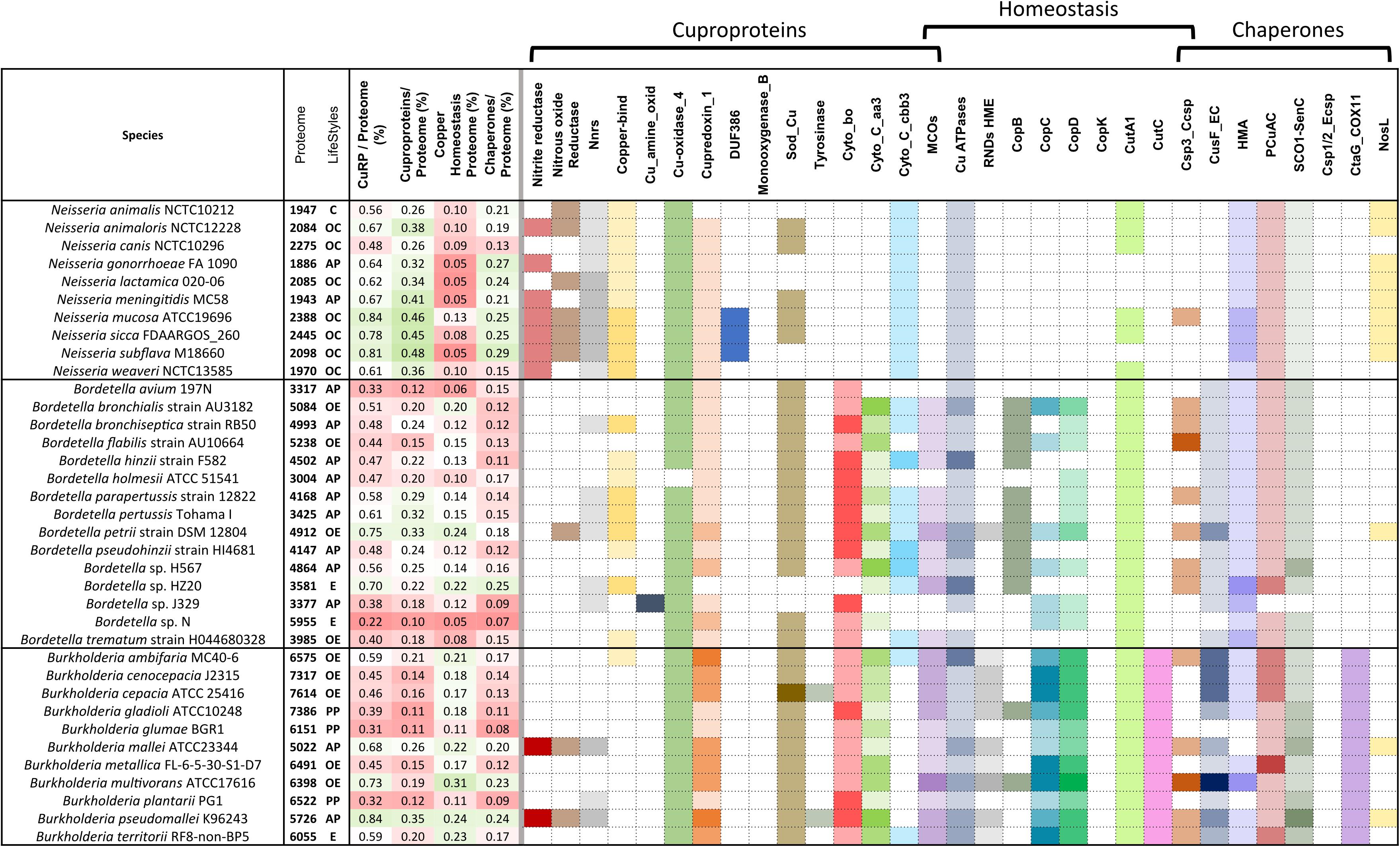
Figure 6. Copper-related proteomes of various Bordetella, Burkholderia, and Neisseria species. See the legend to Figure 2 for details.
Major differences between lifestyles are again reflected in the sets of copper homeostasis proteins. Neisseriae have shed most of their Cu homeostasis systems, which represent only between 7 and 18% of their copper-related proteomes, much lower than the β–proteobacterial median (27%). This contrasts starkly with Burkholderiae, that are replete with copper homeostasis proteins and chaperones. In that group, Burkholderia multivorans has the largest proportions of total copper-related proteins and of copper homeostasis systems, while phytopathogens, Burkholderia glumae and Burkholderia plantarii, have fewer such proteins.
Bordetellae present more varied patterns of copper-related proteins than the other two genera. Obligate, host-restricted pathogens Bordetella holmesii and Bordetella avium have the smallest proteomes and very few defense systems, in contrast with environmental Bordetella petrii, which has the largest proportions of cuproproteins, copper homeostasis systems, and copper chaperones. Interestingly, differences are conspicuous among environmental species in their complements and copy numbers of copper-homeostasis proteins, suggesting specific adaptations to distinct niches by horizontal gene transfers or gene duplications. For instance, Bordetella flabilis has one of the largest proteomes among environmental Bordetellae but fewer copper-homeostasis systems than B. petrii or Bordetella bronchialis, possibly indicating that it is in the process of adaption to a more restricted niche. Bordetella sp. N has the largest proteome of Bordetellae so far, which predicts an environmental niche. However, it has hardly any homeostasis systems or chaperones, at odds with a bona fide environmental lifestyle. Another intriguing isolate is Bordetella sp. J329 isolated from a patient. It is devoid of Cyt C oxidases and SodC, which are rare features among Bordetellae.
Copper-Related Proteomes in Other Proteobacteria
Finally, to determine whether our findings with β Proteobacteria could be generalized to other phylogenetic groups, we selected 30 species of α and γ Proteobacteria of various lifestyles (pathogens, symbionts, and environmental species), with fully sequenced and assembled genomes, and we analyzed their copper-related proteomes as above (Supplementary Table S3). Similar to β Proteobacteria, environmental species have larger proportions of their copper-related proteomes dedicated to homeostasis than the other groups, and symbionts have hardly any copper homeostasis systems and chaperones (Figure 7). However, differences between pathogens and environmental species are less marked in this analysis, most likely because of the limited sample size and because several species defined as pathogens are also environmental, such as Legionella pneumophila and Vibrio cholerae. Altogether, thus, the trends observed in this smaller set confirm the correlation between lifestyles and copper homeostasis.
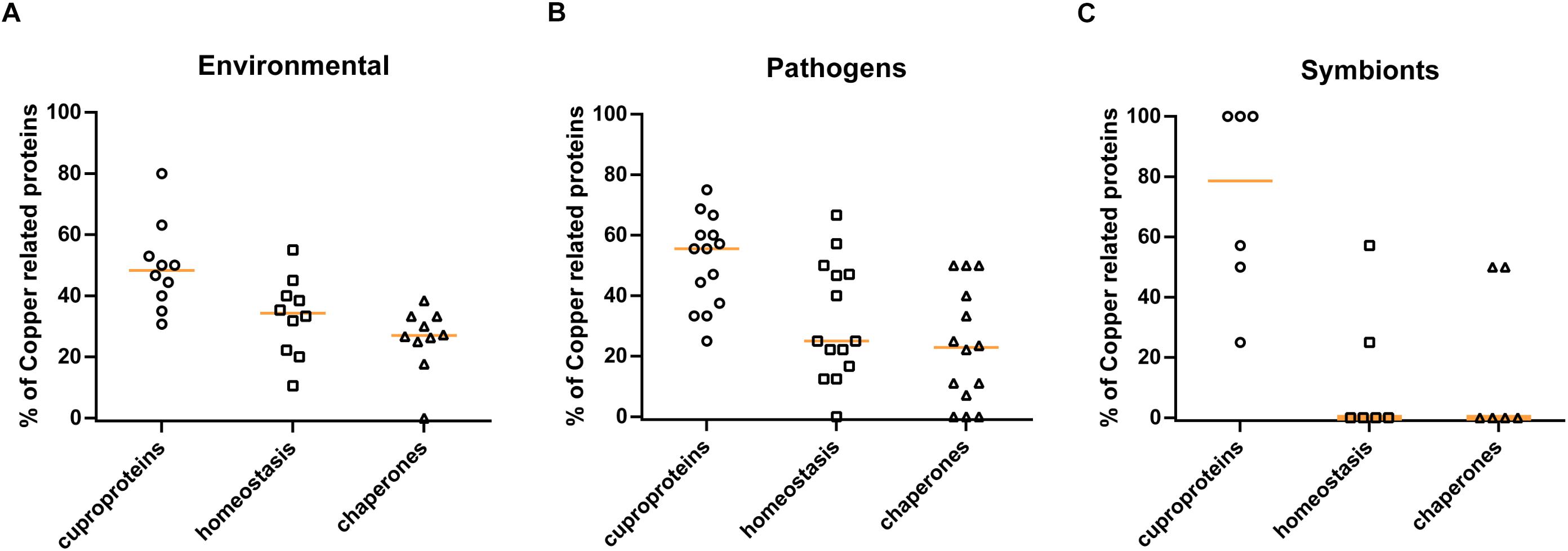
Figure 7. Profiles of copper-related proteomes as a function of the lifestyles of other proteobacteria. The predicted copper-related proteomes of a sample of α and γ proteobacteria were analyzed. The 30 species used in this analysis and their full sets of data are shown in Supplementary Table S3. Three groups (panel A: environmental species, panel B: pathogens, and panel C: symbionts) were made according to lifestyles, and the proportions (in%) of cuproproteins, copper-homeostasis proteins, and copper chaperones relative to the complete copper-related proteomes were determined for each species. The orange horizontal lines represent the medians of the values for the various groups.
Discussion
In the evolution of life on Earth, the use of copper has been linked to the appearance of molecular oxygen, as oxidation of insoluble Cu(I) to soluble Cu(II) made copper bio-available for enzymatic oxido-reductions, hydrolysis reactions, and electron transfer chains (Solioz, 2018). Most aerobic or facultative aerobic bacteria use copper, as several protein complexes involved in aerobic respiration, i.e., cytochrome bo ubiquinol oxidases, type cbb3 cytochrome C oxidases and type aa3 cytochrome C oxidases, include copper-containing subunits. In contrast, many anaerobes do not use copper at all and thus in general, aerobic bacteria have larger cuproproteomes than anaerobic ones (Ridge et al., 2008). The link between oxygen and copper utilization is a reason why the overwhelming majority of β proteobacteria have cuproproteins, with the exception of endosymbionts that have shed most of their metabolic capacities. Indeed, most β proteobacteria in our set are aerobes or facultative aerobes. Median numbers show that the proportions of cuproproteins relative to the sizes of the predicted proteomes are rather similar between pathogens, commensals, and environmental bacteria, but smaller in symbionts and endosymbionts. This is also broadly true for copper chaperones, many of which are involved in assembly of energy-generating complexes.
In β Proteobacteria, two factors appear to determine the proportions of proteins involved in copper homeostasis: the size of the global proteome of each species and its lifestyle. Unsurprisingly, bacteria with larger proteomes generally have more defense systems than smaller-proteome species, as they probably live in less constant environments and thus need to adapt to a variety of stressful conditions, possibly including high concentrations of toxic metals. The sizes of copper-homeostasis proteomes are mainly determined by the lifestyles of the species. Thus, as a general rule, environmental bacteria have relatively large proportions of their proteomes dedicated to copper homeostasis, irrespective of the sizes of their total proteomes. Copper-homeostasis proteins are more abundant relative to the total proteomes, by a factor of three, in environmental bacteria than in the other bacterial groups. The evolution from environmental bacteria to pathogens, commensals, and symbionts in β proteobacteria is globally characterized by the shedding of copper homeostasis systems. In other words, natural selection specifically favors the elimination of copper defense genes from bacteria in the course of their adaptation to eukaryotic hosts niches. This appears to be the case in other Proteobacteria as well.
However, absence of known copper homeostasis genes does not preclude the existence of other, yet unidentified homeostasis systems. Non-specific systems might also be involved, as exemplified by yersiniabactin in E. coli, a siderophore that also participates in copper homeostasis (Chaturvedi et al., 2012; Koh et al., 2017). Other small molecules such as glutathione, bacillithiol, and mycothiol may also play important roles in metal tolerance (Helbig et al., 2008).
Among β Proteobacteria, two groups of bacteria harbor greater than average numbers of copper-homeostasis genes: those that live in environments polluted by metals, and Nitrosomonadales. The first group is exemplified by extremely resistant organisms such as C. metallidurans or H. arsenicoxidans, which thrive in soils heavily contaminated with transition metals thanks to large numbers of export, storage, and detoxification systems. Overrepresentation of copper-related proteins involved in homeostasis relative to cuproproteins and copper chaperones in environmental bacteria corroborates the idea that the use of copper as a cofactor and the defense against copper are not correlated but evolved independently, as in other phylogenetic groups (Solioz, 2018). Note that C. metallidurans and H. arsenicoxydans also have larger proportions of cuproproteins than most β proteobacteria, in part because they have four or five MCOs, cuproproteins that also contribute to protection against copper.
Another factor that is likely to strongly contribute to the abundance of copper defense systems in environmental bacteria is the necessity to survive predation by protozoa in soils and water. The relationship between protozoa and bacteria is a long-standing one, and copper is part of the arsenal used by the former to poison and kill their bacterial preys (Hao et al., 2016). In consequence, there is a strong correlation between the presence of copper efflux systems in bacteria and their ability to survive in amoeba and other protozoa. Those systems in turn may contribute to the ability of environmental bacteria to cause opportunistic infections in specific settings such as in immunocompromised hosts. Indeed phagocytes, which use transition metals to kill invading microbes as part of the innate immune response (Fu et al., 2014; Djoko et al., 2015), share pathways of intracellular copper trafficking with protozoa (Hao et al., 2016). It is thus very likely that the abundance of copper homeostasis systems in many environmental β proteobacteria has been selected for by the need to resist killing by protozoa, and those defenses then enabled opportunists to resist killing by phagocytic cells in their occasional mammalian hosts.
Pathogenic bacteria of mammals represent a special case with respect to copper. Copper is sequestrated away from the pathogens by host proteins in mucosa or body fluids, and therefore bacteria need to specifically acquire it for assembly of their own cuproproteins. On the other hand, as outlined above, the innate immune system uses copper as a line of defense, with macrophages importing copper into the phagolysosome compartment as a means to destroy bacteria, together with oxidative and nitrosative stress and antimicrobial peptides. Animal pathogens must thus strike a fine balance to deal with copper starvation or excess depending on the specific environments encountered in their hosts. We have started to address this issue with the host-restricted, obligate pathogen B. pertussis and discovered that it has lost a number of genes coding for defense systems present in model bacterial pathogens. According to phylogenetic classifications, Bordetellae are close to an environmental/opportunist species, Achromobacter xylosoxidans, and to an endosymbiont, Candidatus Kinetoplastibacterium blastocrithidii. Comparisons between A. xylosoxidans (genome: 7.3 Mb), K. blastocrithidii (0.86 Mb), B. pertussis (4.086 Mb), and B. bronchiseptica (5.34 Mb), the latter of which can both infect mammals and survive in the environment, show that A. xylosoxidans has considerably more Cu-ATPases, HME transporters, MCO and other homeostasis systems than the two Bordetella species, but only slightly more cuproproteins and chaperones. B. bronchiseptica is in an intermediate situation regarding its defenses against copper (see below). Unlike B. pertussis, B. bronchiseptica has two distinct but interconnected cycles, in mammalian hosts and in amoeba (Taylor-Mulneix et al., 2017). Protozoan predation may thus have positively selected for the copper homeostasis systems that remain in B. bronchiseptica but are no longer functional in B. pertussis. At the other end of this spectrum, the endosymbiont K. blastocrithidii has no homeostasis systems. Such a scenario fully supports the global trends observed on our large sample of β proteobacteria.
Interestingly, however, most differences between B. pertussis and B. bronchiseptica with respect to copper-related systems are not found in their genomes but at the level of transcription (our unpublished observations). Thus, B. pertussis possesses genes coding for a Cu-ATPAse and an MCO that are no longer expressed and regulated by copper, while in B. bronchiseptica both are strongly upregulated. B. pertussis has thought to have derived by genomic reduction from a common ancestor close to current-day B. bronchiseptica (Diavatopoulos et al., 2005), and our observations support the idea that streamlining of the B. pertussis genome is an on-going process. Thus, a caveat of in silico genomic analyses is that they can only reveal which genes are present or absent, but not whether they are functional.
With very small total proteomes and few copper-related proteins, endosymbionts represent one extreme of our bacterial set. The proportions of cuproproteins relative to their total proteomes are hardly lower than in other β proteobacterial groups, and thus those genes appear to follow the general genomic reduction toward a symbiotic lifestyle. The proportions of their copper-homeostasis proteins relative to their total proteomes are markedly lower than in other bacterial groups, indicating that those genes are shed faster than others. Genes coding for chaperones tend to follow the same route. If chaperones were only providing copper for cuproenzyme assembly, one would expect them to decrease in similar proportions as genes for cuproproteins. That they are lost in greater proportions suggests that some of them also served defense purposes, likely by sequestration, in the bacterial ancestors of those symbionts.
Copper is increasingly used as an antibacterial agent, notably in hospitals, in agriculture, and in animal breeding (Grass et al., 2011; Lemire et al., 2013; Schmidt et al., 2016). As shown here, environmental bacteria are well-equipped to deal with such aggressions. Alarmingly, such bacteria cause a growing number of opportunistic infections, including C. metallidurans and other β proteobacteria that we have classified as “environmental opportunists” based on our review of the literature (Langevin et al., 2011; Bayhan et al., 2013; Bilgin et al., 2015; Jardine et al., 2017). Finding ways to limit the emergence of new opportunists favored by widespread use of copper as an antibacterial agent will thus become increasingly important. Finally, our study includes a number of bacterial species with bioremediation potential. Deciphering the interplay between metallic stress and stress caused by toxic chemicals might help make the best use of such organisms.
Conclusion
In silico analyses of the predicted copper-related proteomes of a large panel of β proteobacteria have indicated that lifestyle shapes the copper-related proteome, and this is particularly reflected in systems involved in copper homeostasis. Evolution from environmental niches toward commensalism, obligate pathogenesis or symbiosis parallels the loss of copper-homeostasis systems. Endosymbionts represent an extreme situation with respect to copper, having lost almost all copper-related genes with the exception of few cuproproteins involved in electron transfer. The correlation between lifestyle and copper homeostasis appears to hold true in other groups of Proteobacteria as well.
Data Availability Statement
All datasets generated for this study are included in the manuscript/Supplementary Files.
Author Contributions
RA and FJ-D conceived the study. RA, AR-M, and GR gathered the data and prepared the figures and tables. FJ-D wrote the manuscript. All authors analyzed the data and reviewed the manuscript.
Funding
This work was funded by the Institut National de la Santé et de la Recherche Médicale (INSERM) and the University of Lille.
Conflict of Interest
The authors declare that the research was conducted in the absence of any commercial or financial relationships that could be construed as a potential conflict of interest.
Acknowledgments
AR-M and GR acknowledge the support of doctoral fellowships from the University of Lille-Région Nord-Pas-de Calais and from the University of Lille, respectively.
Supplementary Material
The Supplementary Material for this article can be found online at: https://www.frontiersin.org/articles/10.3389/fmicb.2019.02217/full#supplementary-material
FIGURE S1 | Full complements of copper-related proteins (cuproproteins, copper-homeostasis proteins, and copper chaperones) in the entire set of β proteobacteria analyzed in this study. The sizes of the proteomes (numbers of proteins) and the lifestyles are given in the second vertical panel. E, OE, AP, PP, OC, C, S, EnP, and EnS represent environmental, opportunistic/environmental, animal pathogen, phytopathogen, opportunistic/commensal, commensal, symbiotic, endophytic, and endosymbiotic bacteria, respectively. In the third vertical panel are shown the proportions (in %) of the copper-related proteomes (CuRP) relative to the full proteomes for each species, and the proportions (in %) of the proteins in the three functional categories (cuproproteins, copper-homeostasis proteins, and copper chaperones) relative to the complete proteomes. In that panel, the white, green, and red background colors represent average values, above-average values and below-average values, respectively, relative to the 119 species, with the intensities of the colors relating to the distances to the average values. The abundances of each type of proteins in each bacterial species are represented in the last panel. Note that MCOs are both cuproproteins and copper- homeostasis proteins, and Csp3s (cytoplasmic copper storage proteins) are both copper-homeostasis proteins and copper chaperones. The intensities of the colors increase with the numbers of paralogs in each bacterial species, with white indicating the absence of the protein. The absolute numbers of proteins of each type in each bacterial species can be found in Supplementary Table S2.
TABLE S1 | β proteobacterial species analyzed in this study. The species are sorted according to phylogenetic analyses, and the references used to determine their lifestyles are indicated along with the links to the corresponding publications. The different lifestyles are represented by colors: dark green for environmental species (E), green for opportunist/environmental species (OE), red for animal pathogens (AP), yellow for phytopathogens (PP), light beige for commensal species (C), orange for opportunist/commensal species (OC), beige for symbionts (S), light green for endophytes (EnP) and pink for endosymbionts (EnS). The sizes of the predicted proteomes are also provided.
TABLE S2 | Copper-related proteomes of β proteobacteria. The list of the 119 species analyzed in this study is shown. The species are sorted according to phylogenetic analyses. The GenBank accession numbers of the replicons are given as well as their total sizes in megabases (Mb). The GC content (% GC) and the sizes of the proteomes (numbers of predicted proteins) are also reported. For each Pfam domain, the number of occurrences in each species is given as well as the locus_tag name (ID xxxx) found in the corresponding GenBank file. For respiratory components no locus_tag is given but only the number of occurrences of these multi-component systems.
TABLE S3 | Copper-related proteomes of α and γ proteobacteria. The list of the 30 species analyzed in this study is shown. The GenBank accession numbers of the replicons are given as well as their total sizes in megabases (Mb). The GC content (% GC) and the sizes of the proteomes (numbers of predicted proteins) are also reported. For each Pfam domain, the number of occurrences in each species is given as well as the locus_tag name (ID xxxx) found in the corresponding GenBank file. For respiratory components no locus_tag is given but only the number of occurrences of these multi-component systems.
FILE S1 | HMM profile for the copper storage protein domain, CSP.hmm.
Footnotes
- ^ hmmer.org
- ^ http://www.tcdb.org/download.php
- ^ https://www.jcvi.org/tigrfams
- ^ http://signalfind.org/tatfind.html
- ^ http://bonsai.hgc.jp/~mdehoon/software/cluster/software.htm
- ^ tree.bio.ed.ac.uk/software/figtree/
- ^ eddylab.org/infernal/
- ^ https://www.megasoftware.net
References
Azzouzi, A., Steunou, A. S., Durand, A., Khalfaoui-Hassani, B., Bourbon, M. L., Astier, C., et al. (2013). Coproporphyrin III excretion identifies the anaerobic coproporphyrinogen III oxidase HemN as a copper target in the Cu(+)-ATPase mutant copA(-) of Rubrivivax gelatinosus. Mol. Microbiol. 88, 339–351. doi: 10.1111/mmi.12188
Battistoni, A. (2003). Role of prokaryotic Cu,Zn superoxide dismutase in pathogenesis. Biochem. Soc. Trans. 31, 1326–1329. doi: 10.1042/bst0311326
Bayhan, G. I., Tanir, G., Karaman, I., and Ozkan, S. (2013). Comamonas testosteroni: an unusual bacteria associated with acute appendicitis. Balkan Med. J. 30, 447–448. doi: 10.5152/balkanmedj.2013.9135
Bilgin, H., Sarmis, A., Tigen, E., Soyletir, G., and Mulazimoglu, L. (2015). Delftia acidovorans: a rare pathogen in immunocompetent and immunocompromised patients. Can. J. Infect. Dis. Med. Microbiol. 26, 277–279. doi: 10.1155/2015/973284
Broxton, C. N., and Culotta, V. C. (2016). SOD enzymes and microbial pathogens: surviving the oxidative storm of infection. PLoS Pathog. 12:e1005295. doi: 10.1371/journal.ppat.1005295
Capdevila, D. A., Edmonds, K. A., and Giedroc, D. P. (2017). Metallochaperones and metalloregulation in bacteria. Essays Biochem. 61, 177–200. doi: 10.1042/EBC20160076
Casciotti, K. L., and Ward, B. B. (2001). Dissimilatory nitrite reductase genes from autotrophic ammonia-oxidizing bacteria. Appl. Environ. Microbiol. 67, 2213–2221. doi: 10.1128/aem.67.5.2213-2221.2001
Chandrangsu, P., Rensing, C., and Helmann, J. D. (2017). Metal homeostasis and resistance in bacteria. Nat. Rev. Microbiol. 15, 338–350. doi: 10.1038/nrmicro.2017.15
Chaturvedi, K. S., Hung, C. S., Crowley, J. R., Stapleton, A. E., and Henderson, J. P. (2012). The siderophore yersiniabactin binds copper to protect pathogens during infection. Nat. Chem. Biol. 8, 731–736. doi: 10.1038/nchembio.1020
Cooksey, D. A. (1994). Molecular mechanisms of copper resistance and accumulation in bacteria. FEMS Microbiol. Rev. 14, 381–386. doi: 10.1016/0168-6445(94)90056-6
Corbett, D., Schuler, S., Glenn, S., Andrew, P. W., Cavet, J. S., and Roberts, I. S. (2011). The combined actions of the copper-responsive repressor CsoR and copper-metallochaperone CopZ modulate CopZ-mediated copper efflux in the intracellular pathogen Listeria monocytogenes. Mol. Microbiol. 81, 457–472. doi: 10.1111/j.1365-2958.2011.07705.x
Cui, Z., Zhu, B., Xie, G., Li, B., and Huang, S. (2016). Research status and prospect of burkholderia glumae, the pathogen causing bacterial panicle blight. Rice Sci. 23, 111–118. doi: 10.1016/j.rsci.2016.01.007
Dennison, C., David, S., and Lee, J. (2018). Bacterial copper storage proteins. J. Biol. Chem. 293, 4616–4627. doi: 10.1074/jbc.TM117.000180
Diavatopoulos, D. A., Cummings, C. A., Schouls, L. M., Brinig, M. M., Relman, D. A., and Mooi, F. R. (2005). Bordetella pertussis, the causative agent of whooping cough, evolved from a distinct, human-associated lineage of B. bronchiseptica. PLoS Pathog. 1:e45. doi: 10.1371/journal.ppat.0010045
Djoko, K. Y., and McEwan, A. G. (2013). Antimicrobial action of copper is amplified via inhibition of heme biosynthesis. ACS Chem. Biol. 8, 2217–2223. doi: 10.1021/cb4002443
Djoko, K. Y., Ong, C. L., Walker, M. J., and Mcewan, A. G. (2015). The role of copper and zinc toxicity in innate immune defense against bacterial pathogens. J. Biol. Chem. 290, 18954–18961. doi: 10.1074/jbc.R115.647099
Ekici, S., Yang, H., Koch, H. G., and Daldal, F. (2012). Novel transporter required for biogenesis of cbb3-type cytochrome c oxidase in Rhodobacter capsulatus. MBio 3:e00293-11. doi: 10.1128/mBio.00293-11
Felstenstein, J. (1985). Confidence limits on phylogenies: an approach using the bootstrap. Evolution 39, 783–791. doi: 10.1111/j.1558-5646.1985.tb00420.x
Fu, Y., Chang, F. M., and Giedroc, D. P. (2014). Copper transport and trafficking at the host-bacterial pathogen interface. Acc. Chem. Res. 47, 3605–3613. doi: 10.1021/ar500300n
Gonzalez-Guerrero, M., Raimunda, D., Cheng, X., and Arguello, J. M. (2010). Distinct functional roles of homologous Cu+ efflux ATPases in Pseudomonas aeruginosa. Mol. Microbiol. 78, 1246–1258. doi: 10.1111/j.1365-2958.2010.07402.x
Grass, G., Rensing, C., and Solioz, M. (2011). Metallic copper as an antimicrobial surface. Appl. Environ. Microbiol. 77, 1541–1547. doi: 10.1128/AEM.02766-10
Grosse, C., Herzberg, M., Schuttau, M., Wiesemann, N., Hause, G., and Nies, D. H. (2016). Characterization of the delta7 mutant of Cupriavidus metallidurans with deletions of seven secondary metal uptake systems. mSystems 1:e00004-16.
Hao, X., Luthje, F., Ronn, R., German, N. A., Li, X., Huang, F., et al. (2016). A role for copper in protozoan grazing - two billion years selecting for bacterial copper resistance. Mol. Microbiol. 102, 628–641. doi: 10.1111/mmi.13483
Hassani, B. K., Astier, C., Nitschke, W., and Ouchane, S. (2010). CtpA, a copper-translocating P-type ATPase involved in the biogenesis of multiple copper-requiring enzymes. J. Biol. Chem. 285, 19330–19337. doi: 10.1074/jbc.M110.116020
Helbig, K., Bleuel, C., Krauss, G. J., and Nies, D. H. (2008). Glutathione and transition-metal homeostasis in Escherichia coli. J. Bacteriol. 190, 5431–5438. doi: 10.1128/JB.00271-08
Herzberg, M., Bauer, L., Kirsten, A., and Nies, D. H. (2016). Interplay between seven secondary metal uptake systems is required for full metal resistance of Cupriavidus metallidurans. Metallomics 8, 313–326. doi: 10.1039/c5mt00295h
Ibanez, M. M., Checa, S. K., and Soncini, F. C. (2015). A single serine residue determines selectivity to monovalent metal ions in metalloregulators of the MerR family. J. Bacteriol. 197, 1606–1613. doi: 10.1128/JB.02565-14
Jardine, J. L., Abia, A. L. K., Mavumengwana, V., and Ubomba-Jaswa, E. (2017). Phylogenetic analysis and antimicrobial profiles of cultured emerging opportunistic pathogens (Phyla Actinobacteria and Proteobacteria) identified in hot springs. Int. J. Environ. Res. Public Health 14:E1070. doi: 10.3390/ijerph14091070
Jen, F. E., Djoko, K. Y., Bent, S. J., Day, C. J., Mcewan, A. G., and Jennings, M. P. (2015). A genetic screen reveals a periplasmic copper chaperone required for nitrite reductase activity in pathogenic Neisseria. FASEB J. 29, 3828–3838. doi: 10.1096/fj.15-270751
Kaur, K., Sharma, A., Capalash, N., and Sharma, P. (2019). Multicopper oxidases: biocatalysts in microbial pathogenesis and stress management. Microbiol. Res. 222, 1–13. doi: 10.1016/j.micres.2019.02.007
Khalfaoui-Hassani, B., Wu, H., Blaby-Haas, C. E., Zhang, Y., Sandri, F., Verissimo, A. F., et al. (2018). Widespread distribution and functional specificity of the copper importer CcoA: distinct Cu uptake routes for bacterial cytochrome c oxidases. MBio 9:e00065-18. doi: 10.1128/mBio.00065-18
Kim, E. H., Nies, D. H., McEvoy, M. M., and Rensing, C. (2011). Switch or funnel: how RND-type transport systems control periplasmic metal homeostasis. J. Bacteriol. 193, 2381–2387. doi: 10.1128/JB.01323-10
Koh, E. I., Robinson, A. E., Bandara, N., Rogers, B. E., and Henderson, J. P. (2017). Copper import in Escherichia coli by the yersiniabactin metallophore system. Nat. Chem. Biol. 13, 1016–1021. doi: 10.1038/nchembio.2441
Kumar, S., Stecher, G., Li, M., Knyaz, C., and Tamura, K. (2018). MEGA X: molecular evolutionary genetics analysis across computing platforms. Mol. Biol. Evol. 35, 1547–1549. doi: 10.1093/molbev/msy096
Ladomersky, E., and Petris, M. J. (2015). Copper tolerance and virulence in bacteria. Metallomics 7, 957–964. doi: 10.1039/c4mt00327f
Langevin, S., Vincelette, J., Bekal, S., and Gaudreau, C. (2011). First case of invasive human infection caused by Cupriavidus metallidurans. J. Clin. Microbiol. 49, 744–745. doi: 10.1128/JCM.01947-10
Larosa, V., and Remacle, C. (2018). Insights into the respiratory chain and oxidative stress. Biosci. Rep. 38:BSR20171492. doi: 10.1042/BSR20171492
Lawaree, E., Gillet, S., Louis, G., Tilquin, F., Le Blastier, S., Cambier, P., et al. (2016). Caulobacter crescentus intrinsic dimorphism provides a prompt bimodal response to copper stress. Nat. Microbiol. 1:16098. doi: 10.1038/nmicrobiol.2016.98
Lemire, J. A., Harrison, J. J., and Turner, R. J. (2013). Antimicrobial activity of metals: mechanisms, molecular targets and applications. Nat. Rev. Microbiol. 11, 371–384. doi: 10.1038/nrmicro3028
Li, C., Li, Y., and Ding, C. (2019). The role of copper homeostasis at the host-pathogen axis: from bacteria to fungi. Int. J. Mol. Sci. 20:E175. doi: 10.3390/ijms20010175
Linz, B., Ma, L., Rivera, I., and Harvill, E. T. (2019). Genotypic and phenotypic adaptation of pathogens: lesson from the genus Bordetella. Curr. Opin. Infect. Dis. 32, 223–230. doi: 10.1097/QCO.0000000000000549
Liu, G., Tang, C. M., and Exley, R. M. (2015). Non-pathogenic Neisseria: members of an abundant, multi-habitat, diverse genus. Microbiology 161, 1297–1312. doi: 10.1099/mic.0.000086
Lund, M. B., Kjeldsen, K. U., and Schramm, A. (2014). The earthworm-Verminephrobacter symbiosis: an emerging experimental system to study extracellular symbiosis. Front. Microbiol. 5:128. doi: 10.3389/fmicb.2014.00128
Ma, Z., Jacobsen, F. E., and Giedroc, D. P. (2009). Coordination chemistry of bacterial metal transport and sensing. Chem. Rev. 109, 4644–4681. doi: 10.1021/cr900077w
Macomber, L., and Imlay, J. A. (2009). The iron-sulfur clusters of dehydratases are primary intracellular targets of copper toxicity. Proc. Natl. Acad. Sci. U.S.A. 106, 8344–8349. doi: 10.1073/pnas.0812808106
Mahenthiralingam, E., Urban, T. A., and Goldberg, J. B. (2005). The multifarious, multireplicon Burkholderia cepacia complex. Nat. Rev. Microbiol. 3, 144–156. doi: 10.1038/nrmicro1085
Mellies, J., Jose, J., and Meyer, T. F. (1997). The Neisseria gonorrhoeae gene aniA encodes an inducible nitrite reductase. Mol. Gen. Genet. 256, 525–532. doi: 10.1007/s004380050597
Melvin, J. A., Scheller, E. V., Miller, J. F., and Cotter, P. A. (2014). Bordetella pertussis pathogenesis: current and future challenges. Nat. Rev. Microbiol. 12, 274–288. doi: 10.1038/nrmicro3235
Mergeay, M., Nies, D., Schlegel, H. G., Gerits, J., Charles, P., and Van Gijsegem, F. (1985). Alcaligenes eutrophus CH34 is a facultative chemolithotroph with plasmid-bound resistance to heavy metals. J. Bacteriol. 162, 328–334.
Migocka, M. (2015). Copper-transporting ATPases: the evolutionarily conserved machineries for balancing copper in living systems. IUBMB Life 67, 737–745. doi: 10.1002/iub.1437
Monchy, S., Benotmane, M. A., Wattiez, R., Van Aelst, S., Auquier, V., Borremans, B., et al. (2006). Transcriptomic and proteomic analyses of the pMOL30-encoded copper resistance in Cupriavidus metallidurans strain CH34. Microbiology 152, 1765–1776. doi: 10.1099/mic.0.28593-0
Nies, D. H. (2003). Efflux-mediated heavy metal resistance in prokaryotes. FEMS Microbiol. Rev. 27, 313–339. doi: 10.1016/s0168-6445(03)00048-2
Novoa-Aponte, L., Ramirez, D., and Arguello, J. M. (2019). The interplay of the metallosensor CueR with two distinct CopZ chaperones defines copper homeostasis in Pseudomonas aeruginosa. J. Biol. Chem. 294, 4934–4945. doi: 10.1074/jbc.RA118.006316
Osman, D., Patterson, C. J., Bailey, K., Fisher, K., Robinson, N. J., Rigby, S. E., et al. (2013). The copper supply pathway to a Salmonella Cu,Zn-superoxide dismutase (SodCII) involves P(1B)-type ATPase copper efflux and periplasmic CueP. Mol. Microbiol. 87, 466–477. doi: 10.1111/mmi.12107
Padilla-Benavides, T., George Thompson, A. M., Mcevoy, M. M., and Arguello, J. M. (2014). Mechanism of ATPase-mediated Cu+ export and delivery to periplasmic chaperones: the interaction of Escherichia coli CopA and CusF. J. Biol. Chem. 289, 20492–20501. doi: 10.1074/jbc.M114.577668
Patra, S. K., Samaddar, S., Sinha, N., and Ghosh, S. (2019). Reactive nitrogen species induced catalases promote a novel nitrosative stress tolerance mechanism in Vibrio cholerae. Nitric Oxide 88, 35–44. doi: 10.1016/j.niox.2019.04.002
Pennella, M. A., Shokes, J. E., Cosper, N. J., Scott, R. A., and Giedroc, D. P. (2003). Structural elements of metal selectivity in metal sensor proteins. Proc. Natl. Acad. Sci. U.S.A. 100, 3713–3718. doi: 10.1073/pnas.0636943100
Pinel, N., Davidson, S. K., and Stahl, D. A. (2008). Verminephrobacter eiseniae gen. nov., sp. nov., a nephridial symbiont of the earthworm Eisenia foetida. Int. J. Syst. Evol. Microbiol. 58, 2147–2157. doi: 10.1099/ijs.0.65174-0
Putignano, V., Rosato, A., Banci, L., and Andreini, C. (2018). MetalPDB in 2018: a database of metal sites in biological macromolecular structures. Nucleic Acids Res. 46, D459–D464. doi: 10.1093/nar/gkx989
Ridge, P. G., Zhang, Y., and Gladyshev, V. N. (2008). Comparative genomic analyses of copper transporters and cuproproteomes reveal evolutionary dynamics of copper utilization and its link to oxygen. PLoS One 3:e1378. doi: 10.1371/journal.pone.0001378
Robinson, N. J., and Winge, D. R. (2010). Copper metallochaperones. Annu. Rev. Biochem. 79, 537–562. doi: 10.1146/annurev-biochem-030409-143539
Rubino, J. T., Chenkin, M. P., Keller, M., Riggs-Gelasco, P., and Franz, K. J. (2011). A comparison of methionine, histidine and cysteine in copper(I)-binding peptides reveals differences relevant to copper uptake by organisms in diverse environments. Metallomics 3, 61–73. doi: 10.1039/c0mt00044b
Schmidt, M. G., Von Dessauer, B., Benavente, C., Benadof, D., Cifuentes, P., Elgueta, A., et al. (2016). Copper surfaces are associated with significantly lower concentrations of bacteria on selected surfaces within a pediatric intensive care unit. Am. J. Infect. Control 44, 203–209. doi: 10.1016/j.ajic.2015.09.008
Sharma, A., Sharma, D., and Verma, S. K. (2018). In silico study of Iron, Zinc and Copper binding proteins of Pseudomonas syringae pv. lapsa: emphasis on secreted metalloproteins. Front. Microbiol. 9:1938. doi: 10.3389/fmicb.2018.01838
Silver, S., and Ji, G. (1994). Newer systems for bacterial resistances to toxic heavy metals. Environ. Health Perspect. 102(Suppl. 3), 107–113. doi: 10.1289/ehp.94102s3107
Singh, S. K., Grass, G., Rensing, C., and Montfort, W. R. (2004). Cuprous oxidase activity of CueO from Escherichia coli. J. Bacteriol. 186, 7815–7817. doi: 10.1128/jb.186.22.7815-7817.2004
Solioz, M. (2018). Copper and Bacteria. Evolution, Homeostasis and Toxicity. Cham: Springer Nature Switzerland AG.
Stewart, L. J., Thaqi, D., Kobe, B., Mcewan, A. G., Waldron, K. J., and Djoko, K. Y. (2019). Handling of nutrient copper in the bacterial envelope. Metallomics 11, 50–63. doi: 10.1039/c8mt00218e
Tamura, K., and Nei, M. (1993). Estimation of the number of nucleotide substitutions in the control region of mitochondrial DNA in humans and chimpanzees. Mol. Biol. Evol. 10, 512–526.
Taylor-Mulneix, D. L., Bendor, L., Linz, B., Rivera, I., Ryman, V. E., Dewan, K. K., et al. (2017). Bordetella bronchiseptica exploits the complex life cycle of Dictyostelium discoideum as an amplifying transmission vector. PLoS Biol. 15:e2000420. doi: 10.1371/journal.pbio.2000420
Trasnea, P. I., Utz, M., Khalfaoui-Hassani, B., Lagies, S., Daldal, F., and Koch, H. G. (2016). Cooperation between two periplasmic copper chaperones is required for full activity of the cbb3 -type cytochrome c oxidase and copper homeostasis in Rhodobacter capsulatus. Mol. Microbiol. 100, 345–361. doi: 10.1111/mmi.13321
Utz, M., Andrei, A., Milanov, M., Trasnea, P. I., Marckmann, D., Daldal, F., et al. (2019). The Cu chaperone CopZ is required for Cu homeostasis in Rhodobacter capsulatus and influences cytochrome cbb3 oxidase assembly. Mol. Microbiol. 111, 764–783. doi: 10.1111/mmi.14190
Vita, N., Landolfi, G., Basle, A., Platsaki, S., Lee, J., Waldron, K. J., et al. (2016). Bacterial cytosolic proteins with a high capacity for Cu(I) that protect against copper toxicity. Sci. Rep. 6:39065. doi: 10.1038/srep39065
Von Rozycki, T., and Nies, D. H. (2009). Cupriavidus metallidurans: evolution of a metal-resistant bacterium. Antonie Van Leeuwenhoek 96, 115–139. doi: 10.1007/s10482-008-9284-5
Wolschendorf, F., Ackart, D., Shrestha, T. B., Hascall-Dove, L., Nolan, S., Lamichhane, G., et al. (2011). Copper resistance is essential for virulence of mycobacterium tuberculosis. Proc. Natl. Acad. Sci. U.S.A. 108, 1621–1626. doi: 10.1073/pnas.1009261108
Wunsch, P., Herb, M., Wieland, H., Schiek, U. M., and Zumft, W. G. (2003). Requirements for Cu(A) and Cu-S center assembly of nitrous oxide reductase deduced from complete periplasmic enzyme maturation in the nondenitrifier Pseudomonas putida. J. Bacteriol. 185, 887–896. doi: 10.1128/jb.185.3.887-896.2003
Yoneyama, H., and Nakae, T. (1996). Protein C (OprC) of the outer membrane of Pseudomonas aeruginosa is a copper-regulated channel protein. Microbiology 142(Pt 8), 2137–2144. doi: 10.1099/13500872-142-8-2137
Keywords: copper homeostasis, metalloproteome, in silico analyses, β proteobacteria, lifestyles
Citation: Antoine R, Rivera-Millot A, Roy G and Jacob-Dubuisson F (2019) Relationships Between Copper-Related Proteomes and Lifestyles in β Proteobacteria. Front. Microbiol. 10:2217. doi: 10.3389/fmicb.2019.02217
Received: 04 June 2019; Accepted: 11 September 2019;
Published: 24 September 2019.
Edited by:
Marina G. Kalyuzhanaya, San Diego State University, United StatesReviewed by:
Soufian Ouchane, Centre National de la Recherche Scientifique (CNRS), FranceAngelica Reyes-Jara, University of Chile, Chile
Copyright © 2019 Antoine, Rivera-Millot, Roy and Jacob-Dubuisson. This is an open-access article distributed under the terms of the Creative Commons Attribution License (CC BY). The use, distribution or reproduction in other forums is permitted, provided the original author(s) and the copyright owner(s) are credited and that the original publication in this journal is cited, in accordance with accepted academic practice. No use, distribution or reproduction is permitted which does not comply with these terms.
*Correspondence: Françoise Jacob-Dubuisson, ZnJhbmNvaXNlLmphY29iQGlibC5jbnJzLmZy
†These authors have contributed equally to this work