- 1Department of General Microbiology, Institute of Microbiology and Genetics, Georg-August-Universität Göttingen, Göttingen, Germany
- 2Institut für Molekulare Physiologie, Mikrobiologie und Weinforschung, Johannes Gutenberg-Universität Mainz, Mainz, Germany
- 3Department for Plant Biochemistry, Albrecht-von-Haller-Institute for Plant Sciences, Georg-August-Universität Göttingen, Göttingen, Germany
The Gram-positive soil bacterium Bacillus subtilis relies on the glutamine synthetase and the glutamate synthase for glutamate biosynthesis from ammonium and 2-oxoglutarate. During growth with the carbon source glucose, the LysR-type transcriptional regulator GltC activates the expression of the gltAB glutamate synthase genes. With excess of intracellular glutamate, the gltAB genes are not transcribed because the glutamate-degrading glutamate dehydrogenases (GDHs) inhibit GltC. Previous in vitro studies revealed that 2-oxoglutarate and glutamate stimulate the activator and repressor function, respectively, of GltC. Here, we have isolated GltC variants with enhanced activator or repressor function. The majority of the GltC variants with enhanced activator function differentially responded to the GDHs and to glutamate. The GltC variants with enhanced repressor function were still capable of activating the PgltA promoter in the absence of a GDH. Using PgltA promoter variants (PgltA∗) that are active independent of GltC, we show that the wild type GltC and the GltC variants with enhanced repressor function inactivate PgltA∗ promoters in the presence of the native GDHs. These findings suggest that GltC may also act as a repressor of the gltAB genes in vivo. We discuss a model combining previous models that were derived from in vivo and in vitro experiments.
Introduction
Glutamate is the most abundant cellular metabolite that serves as an amino group donor in many anabolic reactions (Gunka and Commichau, 2012; Park et al., 2016). The enzymatic reactions involved in the synthesis and degradation of glutamate represent a central metabolic node, linking carbon to nitrogen metabolism (Figure 1A) (Commichau et al., 2006; Sonenshein, 2007). The Gram-positive soil bacterium Bacillus subtilis relies on the glutamine synthetase (GS) and the glutamate synthase (GltAB) for biosynthesis of glutamate from ammonium and 2-oxoglutarate (2OG) (Bohannon and Sonenshein, 1989). The glutamate dehydrogenases (GDHs) of B. subtilis are strictly catabolically active (Belitsky and Sonenshein, 1998; Commichau et al., 2008). B. subtilis can also take up glutamate from the environment via the high-affinity and low-affinity glutamate transporters GltT and GltP, respectively (Tolner et al., 1995; Zaprasis et al., 2015). Recently, it has been shown that the substrate specificity of GltT is relaxed because the transporter can mediate the uptake of aspartate as well as of the herbicides glyphosate and glufosinate (Zhao et al., 2018; Wicke et al., 2019).
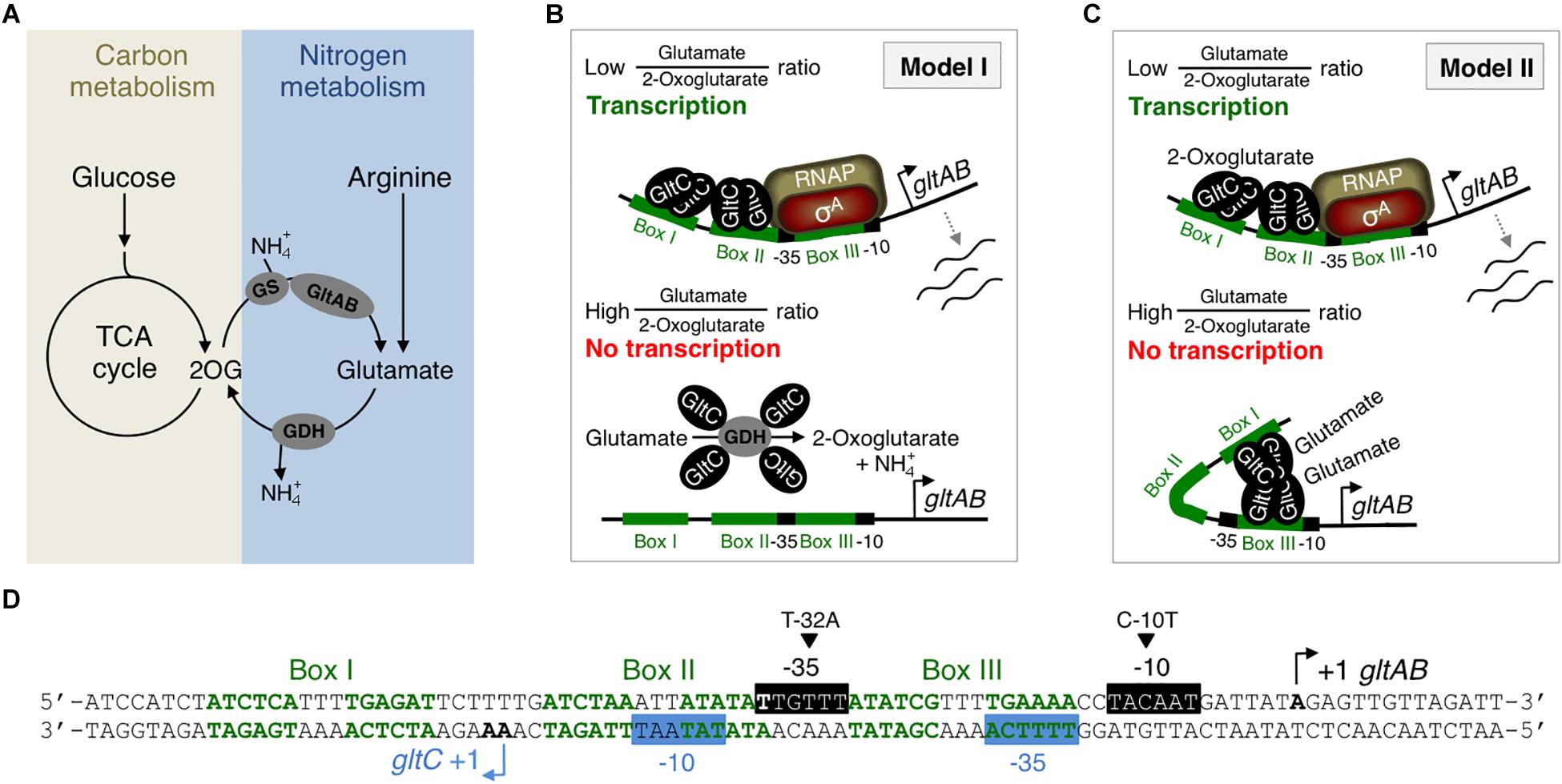
Figure 1. (A) Reactions connecting carbon with nitrogen metabolism in B. subtilis. (B,C) Models for the regulation of gltAB expression based on in vivo and in vitro studies, respectively (Commichau et al., 2007a; Picossi et al., 2007). (D) Part of the gltC-gltAB intergenic region showing the –10 and –35 elements of the PgltC (blue) and PgltA (black) promoters, as well as boxes I, II, and III that are bound by GltC. Bent arrows indicate transcription start sites. Point mutations affecting the activity of the PgltA promoter are indicated by triangles. GS, glutamine synthetase; GltAB, glutamate synthase; Glu, glutamate; 2-OG, 2-oxoglutarate; RNAP, RNA polymerase; RocG and GudB1, paralogous GDHs; σA, housekeeping sigma factor A; TCA, tricarboxylic acid.
Due to the importance of glutamate it is crucial to maintain its cellular concentration high (Yan, 2007; Commichau et al., 2008; Gunka and Commichau, 2012). This is achieved by complex regulatory systems in B. subtilis that sense the availability of carbon and nitrogen sources to adjust glutamate homeostasis accordingly (Gunka and Commichau, 2012). During growth with glucose and ammonium the LysR-type transcriptional activator GltC binds to the PgltA promoter and activates the transcription of the GltAB encoding gltAB genes (Figure 1B) (Bohannon and Sonenshein, 1989; Belitsky et al., 1995; Faires et al., 1999; Wacker et al., 2003; Picossi et al., 2007; Maddocks and Oyston, 2008). Under these growth conditions, the GDH RocG is not active since the carbon catabolite control protein CcpA prevents expression of the rocG gene (Belitsky et al., 2004; Choi and Saier, 2005; Gunka et al., 2012). During growth with nitrogen sources like arginine that is converted to glutamate and induces the expression of the rocG gene, the glutamate pool raises and the gltAB genes are not transcribed (Figure 1B) (Gardan et al., 1997; Belitsky et al., 2004; Commichau et al., 2007b; Stannek et al., 2015). The GDH RocG degrades glutamate to ammonium and 2OG, and prevents the transcription factor GltC from activating transcription of the gltAB genes (Figure 1B) (Commichau et al., 2007a; Stannek et al., 2015). So far, the interaction between GltC and the GDH RocG could only be demonstrated by in vivo-crosslinking using the membrane-permeable crosslinker formaldehyde (Commichau et al., 2007a). This suggests that the enzyme forms a transient complex with the transcription factor. However, RocG is a so-called “trigger enzyme” that are active in metabolism and in controlling gene expression (Commichau and Stülke, 2008).
Laboratory strains of B. subtilis like the strain 168 contain the cryptic gudB gene, which is constitutively transcribed and codes for an inactive GDH (Belitsky and Sonenshein, 1998; Zeigler et al., 2008; Gunka et al., 2012). GudB is inactive because it contains a perfect 18 bp-long direct repeat causing a duplication of three amino acids in the active center of the protein (Belitsky and Sonenshein, 1998). Strains synthesizing the functional GudB1 variant lacking the additional three amino acids in the active center can be isolated on glutamate-containing minimal medium (Belitsky and Sonenshein, 1998; Gunka et al., 2013). Like RocG, the active GudB1 variant can directly bind to the transcription factor GltC, thereby controlling de novo glutamate synthesis (Stannek et al., 2015). Also the interaction between GltC and the GDH GudB1 could only be demonstrated by in vivo-crosslinking experiments (Stannek et al., 2015). Recently, it has been shown that the GDH GudB1 requires glutamate for allosteric activation (Noda-Garcia et al., 2017). It is tempting to speculate that the allosteric activation of the GDH by glutamate is involved in the formation of the GudB1-GltC complex. Since non-domesticated isolates of B. subtilis and their derivatives can produce two catalytically active GDHs, the genetic makeup of the laboratory B. subtilis strain 168 does not reflect the situation in nature. In fact, the bacteria possess two GDHs that can control the DNA-binding activity of GltC (Stannek et al., 2015). To conclude, the tight control of glutamate metabolism by the GDHs ensures maintenance of the intracellular concentration of the metabolite over a wide range of nutritional conditions.
In addition to the GDH-dependent control of gltAB expression, it has been demonstrated that the metabolites 2OG and glutamate modulate the activity of GltC (Figure 1C) (Belitsky and Sonenshein, 2004; Picossi et al., 2007). In vitro transcription and DNAse I footprinting studies revealed that 2OG stimulates the binding of GltC to the boxes I and II in the PgltA promoter, thereby allowing transcription of the gltAB genes (Figures 1C,D). By contrast, glutamate enhances binding of GltC to boxes I and III, and the RNA polymerase (RNAP) can not access the −35 and −10 regions of the PgltA promoter (Figures 1C,D) (Belitsky et al., 1995; Picossi et al., 2007). Thus, GltC acts as an activator and as a repressor in vitro. The regulation of the DNA-binding activity of GltC by 2OG and glutamate, and thus gltAB expression seem to be physiologically meaningful because the bacteria require the glutamate synthase GltAB to synthesize glutamate if its cellular concentration drops and if 2OG and ammonium are available. By contrast, GltAB is not needed if glutamate or amino acids of the glutamate family (e.g., arginine) are available. Bioinformatic analyses of LysR-type transcription factors have revealed that the structural regions (domains) are highly conserved. The DNA-binding HTH motif and the cofactor-binding domain are always located at the N terminus and at the C terminus of the LysR-type regulators, respectively (Maddocks and Oyston, 2008). However, in case of GltC it remains to be elucidated where and how the metabolites and the GDHs bind to the effector domain of the regulator. In fact, the GDHs RocG and GudB1 seem to be the major factors modulating the DNA-binding activity of GltC in vivo (Figures 1B,C). First, GltC is active in strains lacking a functional GDH and it only weakly responds to glutamate, which stimulates the repressor function of GltC in vitro (Belitsky and Sonenshein, 2004; Commichau et al., 2007a; Stannek et al., 2015). Second, GltC is inactive when a GDH degrades glutamate to ammonium and 2OG, of which the latter stimulates the activator function of GltC in vitro (Commichau et al., 2007a; Picossi et al., 2007; Stannek et al., 2015). Third, both GDHs directly interact with and probably hinder GltC from binding to the PgltA promoter (Figure 1B) (Commichau et al., 2007a; Stannek et al., 2015). Thus, the DNA-binding activity of GltC seems to be in fact mainly regulated by a catalytically active GDH that degrades glutamate to 2OG and ammonium.
In the present study, we have randomly mutagenized the gltC gene to introduce mutations enhancing either the transcriptional activator or the repressor function of GltC. The majority of the GltC variants with enhanced activator and repressor function did only weakly respond to the GDHs. The GltC variants with enhanced repressor function were still capable of activating the PgltA promoter in the absence of the GDH RocG. Using a PgltA promoter variant that is active independent of GltC, we have observed that the GltC variants with enhanced repressor function inactivate the promoter when the glutamate-degrading GDH RocG is synthesized. We also show that the wild type GltC protein can inactivate constitutively active PgltA promoter variants in the presence of the native GDHs and a source of glutamate. These findings suggest that GltC may also act as a repressor of the gltAB genes in vivo. We discuss a model combining previous models that were derived from in vivo and in vitro experiments.
Materials and Methods
Chemicals, Media and DNA Manipulation
Oligonucleotides purchased from Sigma-Aldrich (Taufkirchen, Germany) are listed in Table 1. B. subtilis chromosomal DNA was isolated using the DNeasy Blood & Tissue Kit (Qiagen, Hilden, Germany). Plasmid DNA was isolated from E. coli using the Nucleospin Extract Kit (Macherey-Nagel, Düren, Germany). DNA fragments that were generated by the PCR were purified using the PCR Purification Kit (Qiagen). Phusion DNA polymerase, restriction enzymes and T4 DNA ligase were purchased from Thermo Scientific (Schwerte, Germany) and used according to the manufacturer’s instructions. Chemicals and media were purchased from Sigma-Aldrich, Carl Roth (Karlsruhe, Germany) and Becton-Dickinson (Heidelberg, Germany). DNA sequencing was performed by Microsynth (Göttingen, Germany).
Bacterial Strains and Growth Conditions
The B. subtilis and E. coli strains are listed in Table 2. B. subtilis was grown in sporulation medium or in CSE minimal medium (Commichau et al., 2007a). CSE-Glc medium contains glucose (5 g l–1), sodium succinate (6 g l–1), potassium glutamate (8 g l–1) ammonium sulfate (3.3 g l–1) as sources of carbon and nitrogen. Arginine [5 g l–1 0.5% (w/v)] was added as an additional source of nitrogen as indicated. E. coli was grown in lysogeny broth (LB) and brain heart infusion (BHI) medium (37 g l–1). LB, SP and CSE plates were prepared with 17 g Bacto agar/l (Becton-Dickinson). 5-Bromo-4-chloro-3-indolyl β-D-galactopyranoside (X-gal) was added to a final concentration of 80 μg/ml to the media. β-Galactosidase activity assays were performed as described previously (Stannek et al., 2015). Briefly, cells were harvested during exponential growth (optical density OD600 of 0.6–0.8) and the cytoplasmic fraction was assayed for β-galactosidase activity.
DNA Manipulation, Transformation and Phenotypic Analysis
Escherichia coli DH5α was used for cloning experiments (Sambrook et al., 1989) and transformants were selected on LB plates containing ampicillin (100 μg l–1). B. subtilis was transformed as described previously (Kunst and Rapoport, 1995). Transformants were selected on SP plates containing kanamycin (10 μg l–1), chloramphenicol (5 μg l–1), spectinomycin (150 μg l–1), tetracycline (10 μg l–1), or erythromycin plus lincomycin (2 μg l–1 and 25 μg l–1, respectively). In B. subtilis, amylase activity was detected as described previously (Stannek et al., 2015).
Generation of Plasmids
All plasmids used in this study are listed in Table 2. To obtain high and constitutive expression of GudB1 in B. subtilis, we constructed the plasmid pBP482. For this purpose, the gudB1 gene lacking the 18 bp-long direct repeat that renders the encoded GDH cryptic was amplified with the primers MD246 and MD247 using chromosomal DNA of the B. subtilis strain BP848 as a template (Table 2). The PCR product was digested with BamHI and PstI and introduced into the overexpression vector pBQ200 that was cut with the same enzymes (Martin-Verstraete et al., 1994). The plasmid pGP948 for the generation of a B. subtilis rocG disruption mutant was constructed as follows. The rocG gene was amplified from plasmid pGP902 (Gunka et al., 2010) using the oligos PT12 and T7Prom. The PCR product was digested with XbaI and HindIII and ligated to the plasmid pBQ200 that was linearized with the same enzymes. The resulting plasmid was designated as pGP906. A fragment of the rocG gene that was cut out from plasmid pGP906 using the enzymes HindIII and SacI was introduced into the plasmid pBluescript SKII (+) that was digested with the same enzymes yielding in plasmid pGP946. Next, the aphA3 kanamycin resistance gene (Guérout-Fleury et al., 1995) was cut out from plasmid pDG792 using EcoRI and introduced into the plasmid pGP946 that was digested with the same enzyme yielding in plasmid pGP948. The correct insertion of the DNA fragments into the plasmids was confirmed by sequencing.
Random Mutagenesis of gltC
The plasmid pGP907 was mutagenized using the E. coli mutator strain XL1-Red as described previously (Greener et al., 1996; Gunka et al., 2010). For this purpose, pGP907 (wild type gltC) was used to transform E. coli XL1-Red, and the cells were plated on 9 LB plates resulting in approximately 100 colonies per plate. The colonies from each plate were resuspended in 1 ml of LB medium, and 100 μl of each suspension was used to inoculate 100 ml flasks containing 10 ml of LB medium. The cultures were grown for 48 h at 37°C to allow the emergence of mutations. Plasmid DNA from each culture was isolated individually and used to transform the indicator strain B. subtilis BP852 (gltC– rocG– gudB+ PgltA-lacZ). Transformants were selected on SP plates containing 2 (μg/ml erythromycin plus 25 (μg/ml lincomycin and X-gal. The mutant derivatives of plasmid pGP907 were digested with BamHI and PstI and the gltC alleles extracted from an agarose gel and ligated to a fresh backbone of plasmid pBQ200 (Martin-Verstraete et al., 1994) that was digested with the same enzymes. The correct insertion of the DNA fragments into the plasmids was confirmed by sequencing (Table 2). The plasmids carrying the gltC mutant alleles encoding GltC variants with enhanced activator function as well as the empty plasmid pBQ200 and the plasmid pGP907 were used for transformation of the indicator strains BP852 and BP881 (gltC– rocG+ gudB– PgltA-lacZ). The plasmids carrying the gltC mutant alleles inhibiting the PgltA promoter as well as the plasmids pBQ200 and pGP907 were used for transformation of the strains GP650 (PgltA-lacZ gltC– rocG+ gudB–) and GP692 (PgltA(C–10T)-lacZ gltC– rocG+ gudB–).
Results
Isolation of GltC Variants With Enhanced Activator and Repressor Function
Three variants of GltC (P88L, T99A and I160K) with enhanced activator function have been described previously (Belitsky and Sonenshein, 1995). Thus, single amino acid exchanges in the effector domain of GltC are sufficient to enhance the activator function of GltC. We were interested in isolating GltC variants with enhanced activator function activating the PgltA promoter at different levels. We were also wondering whether it is possible to obtain GltC variants showing enhanced repressor activity in vivo. For this purpose, we randomly mutagenized the gltC gene and screened for GltC variants that can be assigned to the different mutant classes. The random mutagenesis of the plasmid pGP907 carrying the wild type gltC gene was performed using the E. coli mutator strain XL1-Red (Figure 2) (see Experimental Procedures). The mutagenized plasmids were introduced into the indicator strain BP852 (PgltA-lacZ gltC– rocG– gudB1), which contains a translational PgltA-lacZ fusion to monitor the activity of GltC and synthesizes the active GDH GudB1. The 245 bp-long PgltA promoter fragment contains all GltC binding sites that have been described previously (Figure 1D) (Belitsky et al., 1995; Picossi et al., 2007). The transformed cells were propagated on SP rich medium plates containing 5-bromo-4-chloro-3-indolyl-(β-Dd-galactopyranoside (X-Gal) to visualize the activity of the PgltA promoter. As illustrated in Figure 2, the indicator strain forms light blue colonies on SP plates because GltC is overexpressed and the GDH GudB1 cannot fully inhibit the transcriptional regulator (Commichau et al., 2007a). We expected that the indicator strain synthesizing GltC variants with enhanced activator and repressor function would form dark blue and white colonies, respectively. By visual inspection of the agar plates, we could identify blue and white colonies. In total, we isolated 53 dark blue and 5 white colonies, isolated the plasmids and analyzed the DNA sequences of the gltC alleles. The majority of the plasmids obtained from the blue transformants carried gltC alleles with single point mutations yielding in GltC variants with single amino acid exchanges (Figure 3A). The amino acid exchanges increasing the activator function of GltC occurred in the linker and effector domains (Figure 3A). Only one gltC allele carried two point mutations (gltC G778A T895C) causing two amino acid exchanges in the encoded protein (GltC E260K Y299H) (Figure 3A). We also identified two gltC alleles having a point mutation (C898T) and a single-nucleotide insertion (879T) that likewise would truncate GltC after 299 (ΔQ300) and 294 (S294L ΔKLEQYQ) amino acids, respectively (Figure 3A). The insertion of T at position 879 also replaces serine by leucine at position 294. Moreover, we identified a gltC allele with a single-nucleotide deletion (T878) that would elongate GltC by 13 amino acids and replace the amino acids from position 293 to 300 (Figure 3A). Two out of five gltC alleles, probably encoding GltC variants with enhanced repressor function, had mutations in a region encoding the helix-turn-helix (HTH) motif (GltC H17Y and I32F), which is required for DNA binding (Figure 3A). Probably, these GltC variants are inactive because the amino acid exchanges affect the DNA-binding activity of the regulator (see below). The remaining three gltC alleles that were isolated from the white colonies had acquired single point mutations in the region encoding the effector domain of the regulator (L146S, A234T and P251S) (Figure 3A). Interestingly, several gltC alleles encoding GltC variants with enhanced activator function were isolated multiple times (11 × P196L, 9 × T99A, 6 × L106S, 4 × G222S) (Figure 3B). Probably, these variants of GltC strongly activate the PgltA promoter, which may have facilitated their identification in our genetic screen (see below). We have also isolated GltC variants with enhanced activator function, in which different amino acid replacements occurred at the same position (11 × P196L or 1 × P196S; 2 × P88L or 1 × P88S). Moreover, we could isolate the GltC T99A and GltC P88L variants with enhanced activator function that were described previously (Belitsky and Sonenshein, 1995). Based on a model of the tetramer structure of the CbnR LysR-type regulator from Cupriavidus necator (PDBid: 1lZ1) (Muraoka et al., 2003), we generated a model for a full-length GltC protomer using the SWISS-MODEL server for homology modeling of protein structures (Waterhouse et al., 2018). As shown in Figure 3C, many amino acid exchanges enhancing the activator function of GltC are located between the linker and the effector domain. Probably, the GltC variants with enhanced activator function are locked in the “activator” state. The remaining amino acid exchanges enhancing the activator and repressor function of GltC lie within the effector domain and at the C-terminus of the regulator. These amino acid exchanges might affect the dimerization/multimerization of the regulator, its interaction with the GDH, or both (Figure 3C). However, only the structural characterization of the GltC variants will help to elucidate how the mutations affect the DNA-binding activity and the interaction with the GDHs. To conclude, the genetic screen allowed us to isolate 19 GltC variants with enhanced activator function and 5 potential variants of GltC with enhanced repressor activity.
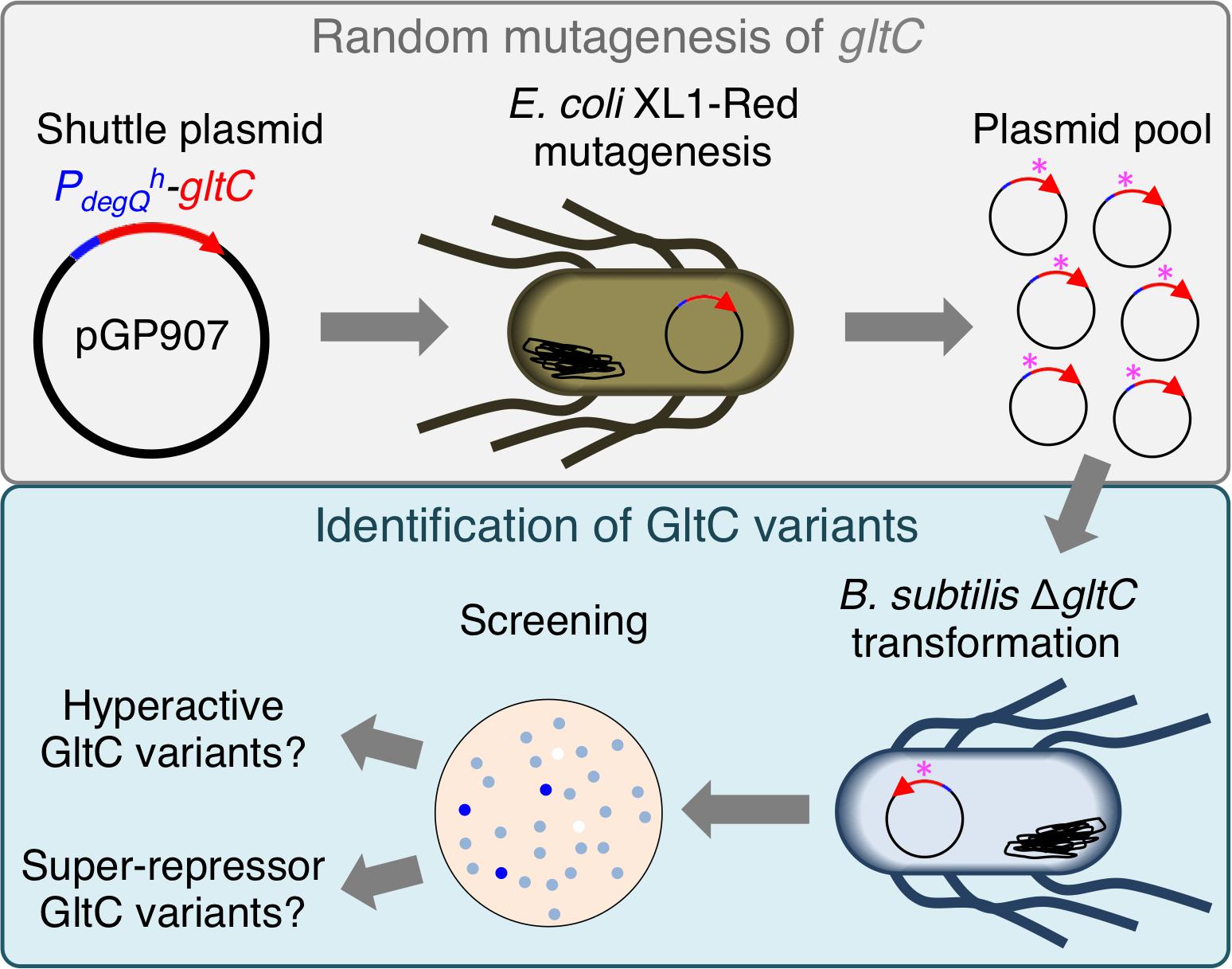
Figure 2. Working flow describing the random mutagenesis procedure for isolating GltC variants with enhanced activator and repressor function.
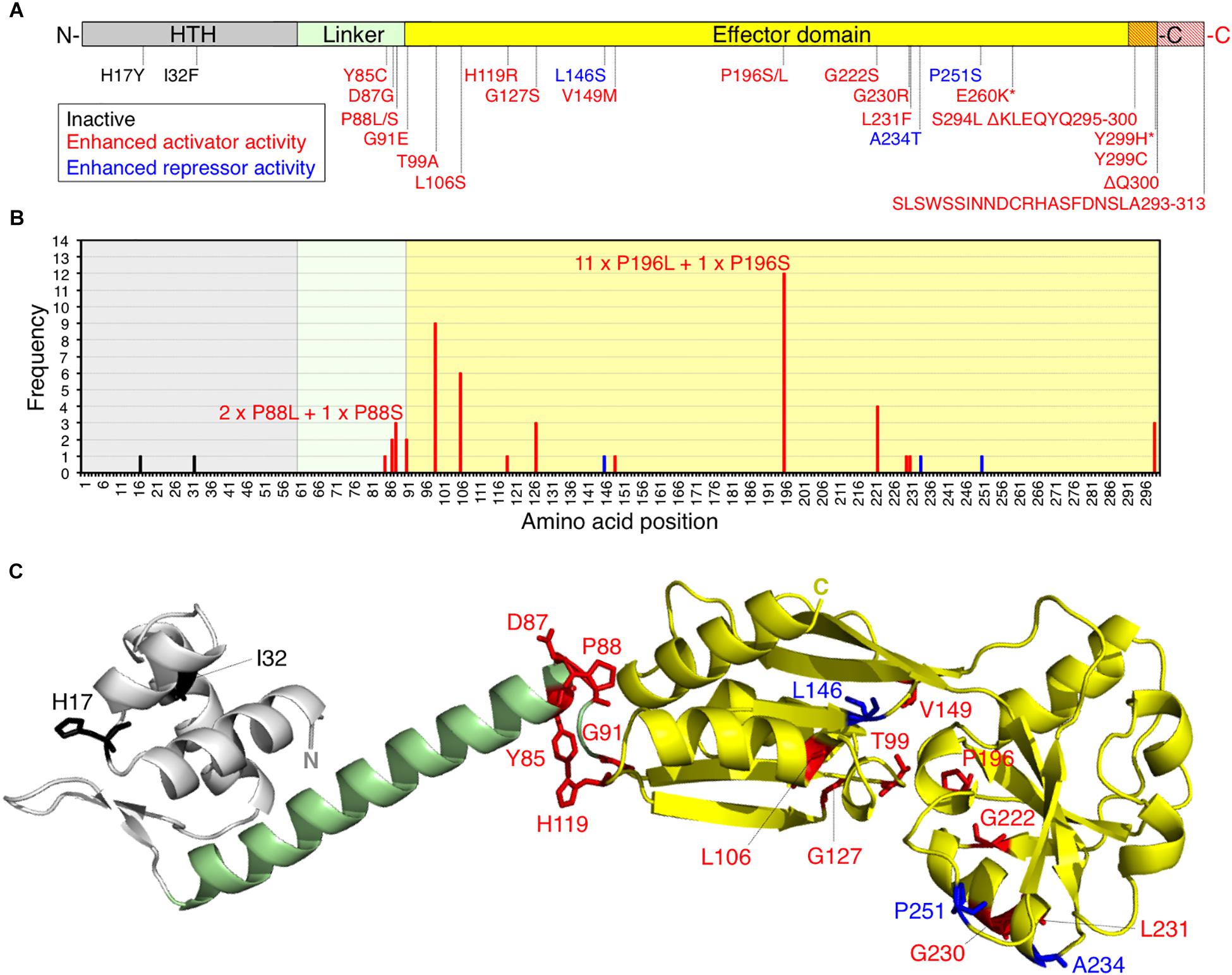
Figure 3. (A) Amino acid replacements in GltC that affect the DNA-binding activity of the regulator. The asterisks indicate that the amino acid replacements E260K and Y299H occurred simultaneously in one GltC variant. (B) Frequency of the amino acid replacements in GltC. The GltC E260K Y299H variant, the truncated as well as the elongated GltC variants appeared only once and are not included in the graph. (C) Localization of the amino acid exchanges that affect the DNA-binding of GltC in a full-length model of the protein. Coloring of the GltC monomer model corresponds to that used for illustrating the domain organization of the regulator in (A). The model was generated using the SWISS-MODEL server for homology modeling of protein structures (Waterhouse et al., 2018) and a model of the tetramer structure of the CbnR LysR-type regulator from Cupriavidus necator (PDBid: 1lZ1) (Muraoka et al., 2003). The overall amino acid sequence identity between GltC and CbnR is 28%. HTH, helix-turn-helix motiv.
Characterization of GltC Variants With Enhanced Activator Activity
Next, we evaluated the ability of the GltC variants with enhanced activator function to activate PgltA promoter. We also aimed to elucidate whether they are still responsive to either RocG or GudB1. To exclude the possibility that the random mutagenesis of the plasmid pGP907 led to the accumulation of mutations affecting the plasmid copy number and thus the cellular levels of GltC, we re-introduced all identified gltC alleles into plasmid pBQ200 carrying a constitutively active promoter. The resulting plasmids (see Table 2) as well as the empty plasmid pBQ200 and the plasmid pGP907 carrying the wild type gltC allele were used to transform the strains BP881 (PgltA-lacZ gltC– rocG+ gudB–) and BP852 (PgltA-lacZ gltC– rocG– gudB1) synthesizing RocG and GudB1, respectively, and carrying a translational PgltA-lacZ fusion. To monitor the ability of GltC to activate the PgltA promoter, the strains were cultivated in CSE-Glc minimal medium containing glucose and succinate as carbon sources and ammonium and glutamate as nitrogen sources. Ammonium was added to the medium to relieve the repression of the PgltA promoter by TnrA, which is a global regulator of nitrogen metabolism in B. subtilis (Belitsky et al., 2000). As expected, the PgltA promoter was only active in the presence of GltC (Figure 4A). As reported previously, due to the overexpression of the gltC gene, the activity of the PgltA promoter was about 2-fold enhanced in the strains BP881 and BP852 carrying the plasmid pGP907 (gltC) as compared to the strains GP342 (PgltA-lacZ gltC+ rocG+ gudB–) and BP817 (PgltA-lacZ gltC+ rocG– gudB+) carrying the gltC gene at the native locus (β-galactosidase activities of 258 ± 79 and 185 ± 15 for the strains GP342 and BP817, respectively) (Figure 6B) (Commichau et al., 2007a). Twelve of the 19 GltC variants with enhanced activator function did activate the PgltA promoter 2- to 6.4-fold stronger than the wild type GltC protein in the strain BP881 (PgltA-lacZ gltC– rocG+ gudB–). The strain BP881 produces only little GDH levels because the rocG gene is repressed by glucose CSE-Glc medium and the gudB gene is deleted (Belitsky and Sonenshein, 2004; Choi and Saier, 2005; Gunka et al., 2012). In the strain BP852 (PgltA-lacZ gltC– rocG– gudB1) synthesizing the active GDH GudB1, 17 of the 19 GltC variants with enhanced activator function did activate the PgltA promoter 1.5- to 10.3-fold stronger than the wild type GltC protein (Figure 4A). Interestingly, the GltC variants S294L ΔKLEQYQ295-300, SLSWSSINNDCRHASFDNSLA293-313 (FS) and H119R were less active in the strain BP881 lacking the GDH GudB1 (Figure 4A). By contrast, the GltC variants Y85C and D87G were less active when the GDH GudB1 was synthesized. Thus, the amino acid replacements in the GltC variants differentially affect the interaction with the RocG and GudB1. To conclude, the diverse set of isolated GltC variants activate the PgltA promoter at different levels during growth in CSE-Glc medium depending on the presence of either RocG or GudB1.
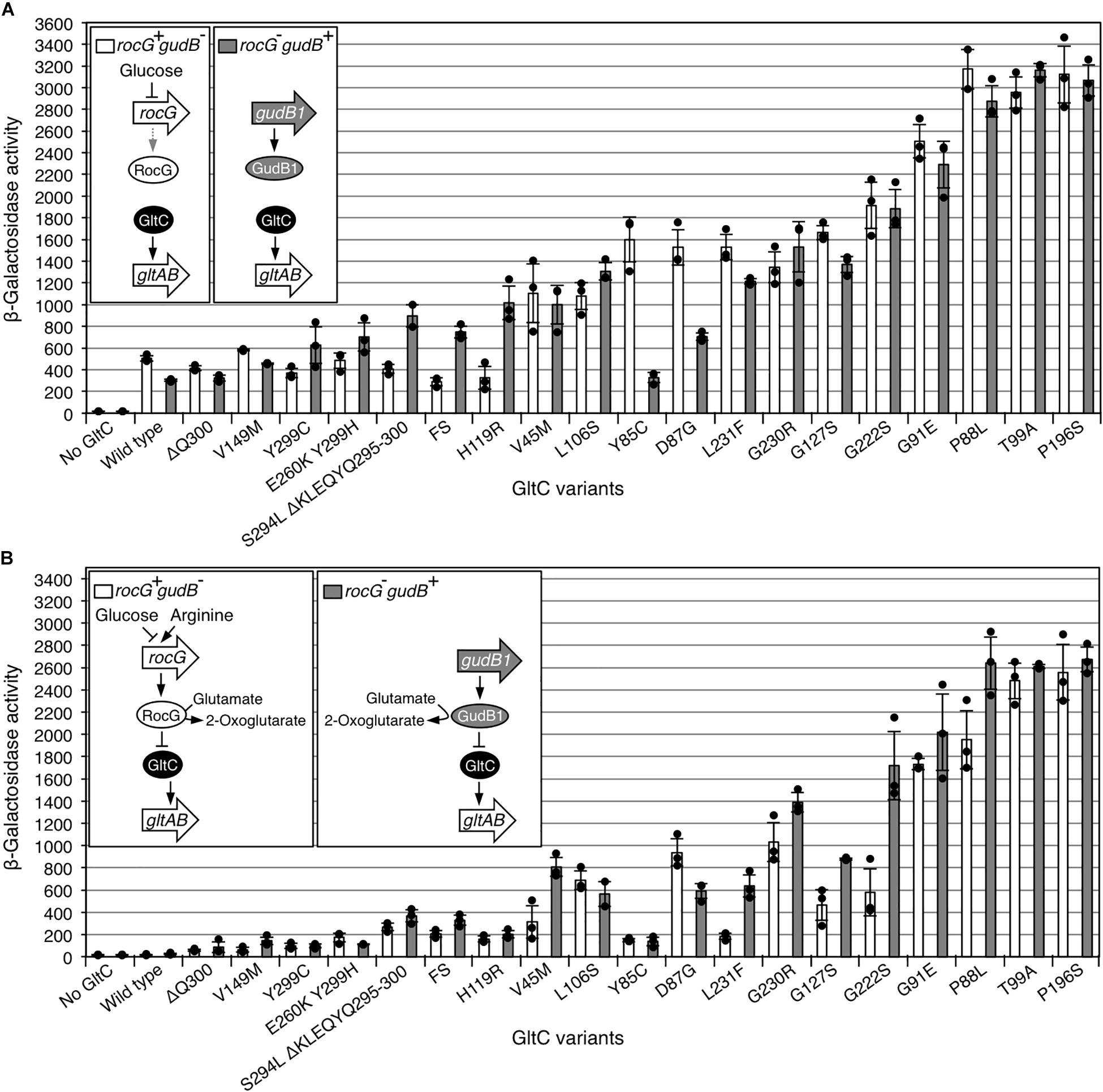
Figure 4. Regulation of the PgltA promoter by the GltC variants with enhanced activator function in the strains BP881 (PgltA-lacZ gltC– rocG+ gudB–) and BP852 (PgltA-lacZ gltC– rocG– gudB1) synthesizing RocG and GudB1, respectively, and carrying a translational PgltA-lacZ fusion during growth in CSE-Glc minimal medium without (A) and with arginine (B). Arginine was added to a final concentration of 0.5% (w/v). Data points represent biologically independent replicates. Bars indicate means of replicates and the standard deviations are shown. β-Galactosidase activities are given as units per milligram of protein. The plasmids carrying the gltC alleles are listed in Table 2. FS, frame shift GltC mutant (SLSWSSINNDCRHASFDNSLA293-313).
Next, we evaluated the ability of the GltC variants with enhanced activator function to activate PgltA promoter in the strains BP881 (PgltA-lacZ gltC– rocG+ gudB–) and BP852 (PgltA-lacZ gltC– rocG– gudB1) during growth in CSE-Glc minimal medium that was supplemented with arginine. Previously, it has been shown that arginine, which is converted to glutamate, strongly reduces the activity of GltC in a GDH-dependent manner (Belitsky et al., 2004; Commichau et al., 2007a; Stannek et al., 2015) (Figure 1A). It has been suggested that the GDHs and glutamate synergistically inhibit the DNA-binding activity of GltC to prevent de novo glutamate biosynthesis (Stannek et al., 2015). As shown in Figure 4B, the ability of the GltC variants with enhanced activator function to activate transcription at the PgltA promoter was indeed reduced in the strains BP881 and BP852 synthesizing RocG and GudB1, respectively. Moreover, the GltC variants V45M, G127S, G222S, G230R and L231F were less active when the GDH RocG was produced. By contrast, the GDH GudB1 stronger inhibited the GltC variant D87G than the GDH RocG. To conclude, albeit to a different extent, all GltC variants with enhanced activator function still respond to a glutamate-degrading active GDH. The differential responses of some of the GltC variants to RocG and GudB1 may indicate that the GDHs interact with regulator at different sites due to regulator-enzyme coevolution. However, the molecular details of the GDH-GltC interaction may only be elucidated by co-crystallization attempts, which could be difficult due to the transient nature of the protein complex (Commichau et al., 2007a; Stannek et al., 2015).
Characterization of GltC Variants With Enhanced Repressor Function
Two gltC alleles that were identified in the five mutants forming white colonies had mutations in a region encoding the HTH motif (GltC H17Y and I32F), which is required for DNA binding (Figures 3A,B). As expected, the GltC H17Y and GltC I32F variants did not sustain growth of a gltC mutant strain on minimal medium plates in the absence of exogenous glutamate, indicating that these variants had lost ability to activate the transcription of the gltAB genes (data not shown). Therefore, these mutants were excluded from further experiments. The remaining three gltC alleles, probably encoding GltC variants with enhanced repressor function, had acquired single point mutations in the region encoding the effector domain of the regulator (L146S, A234T and P251S) (Figures 3A,B). To initially characterize the GltC variants, the plasmids pBP727, pBP738, and pBP726, encoding GltC L146S, A234T and P251S, respectively, were introduced into the strain BP881 (PgltA-lacZ gltC– rocG+ gudB–). The empty plasmid and the plasmids pGP907 (wild type GltC) and pBP718 (GltC T99A variant with enhanced activator function) served as controls, respectively. The generated strains were propagated on SP rich medium, glucose-ammonium-glutamate and glucose-ammonium minimal medium agar plates. As expected, the strain BP881 formed white and light blue colonies on SP plates, depending on the absence and presence of GltC, respectively (Figure 5). Derivatives of BP881 expressing the GltC variants with enhanced activator and repressor function formed dark blue and white colonies on SP rich medium plates, respectively. Thus, the L146S, A234T and P251S exchanges seem to indeed enhance the repressor activity of GltC. By contrast, with the strain BP881 carrying the empty vector formed slight blue colonies on glucose-glutamate-containing minimal medium plates due to the basal activity of the PgltA promoter (Figure 5). The derivatives of the strain BP881 synthesizing the wild type and the GltC variants with enhanced activator and repressor function formed blue colonies on this plate. This indicates that all GltC variants activate the PgltA promoter with low amounts of the GDH RocG because the rocG gene is repressed by glucose present in glucose-ammonium-glutamate medium (Belitsky and Sonenshein, 1998; Choi and Saier, 2005; Commichau et al., 2007b). With the exception of the strain carrying the empty vector, all strains synthesizing a GltC variant grew in the absence of glutamate (Figure 5). Thus, the GltC variants with enhanced repressor function are still able to activate transcription of the gltAB glutamate synthase genes (Figure 5).
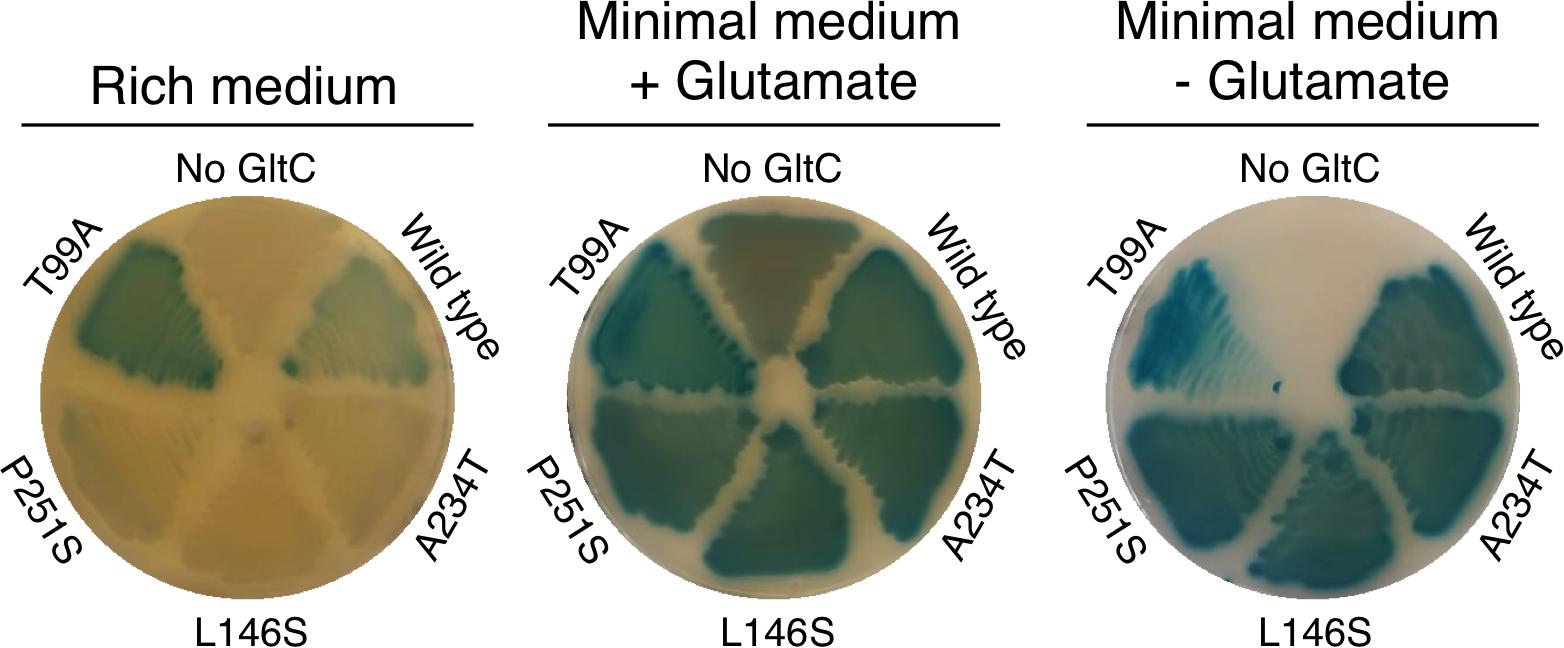
Figure 5. Activity of the PgltA promoter in the strains BP881 (PgltA-lacZ gltC– rocG+ gudB–) + pBQ200 (no GltC), BP881 + pGP907 (Wild type GltC), BP881 + pBP735 (GltC A234T), BP881 + pBP727 (GltC L146S), BP881 + pBP726 (GltC P251S), and BP881 + pBP718 (GltC T99A) during growth on SP agar plates (rich medium) and on CS-Glc minimal medium agar plates with glutamate (+) and without glutamate (–). The agar plates were supplemented with X-gal to monitor the activity of the PgltA promoter. The plates were incubated for 24 h at 37°C.
Next, we assessed the activities of the GltC variants in the strains GP650 (PgltA-lacZ gltC– rocG+ gudB–) and GP692 (PgltA(C–10T)-lacZ gltC– rocG+ gudB–) harboring translational promoter lacZ fusions containing the PgltA wild type and PgltA(C–10T) promoters, respectively. The strains only encode the GDH RocG. The PgltA(C–10T) promoter was included to evaluate the potential of the GltC variants L146S, A234T and P251S with enhanced repressor function to inhibit a derivative of the PgltA promoter, which is also active independent of GltC (Figure 1D) (Belitsky et al., 1995; Belitsky and Sonenshein, 2004; Commichau et al., 2007a). The bacteria were cultivated in SP rich medium and in glucose-ammonium-glutamate minimal medium without and with arginine, conditions that are known to increase and to reduce the activity of the PgltA promoter, respectively (Figure 6A) (Belitsky and Sonenshein, 2004; Commichau et al., 2007a, b; Stannek et al., 2015). As shown in Figure 6B, in SP rich medium the PgltA promoter was only slightly active with the GltC wild type protein and almost completely inactive when the GltC variants L146S, A234T and P251S were synthesized. As expected, the PgltA(C–10T) promoter was active in the absence and in the presence of GltC during growth in SP rich medium (Figure 6B). However, the activity of the PgltA(C–10T) promoter was slightly reduced and significantly lower when the GltC variants L146S, A234T and P251S were synthesized. Thus, the GltC variants with enhanced repressor function are able to inhibit the PgltA(C–10T) promoter, probably in a GDH-dependent manner because SP medium contains arginine and other sources of glutamate. When the strains that contain the translational promoter lacZ fusions containing the PgltA wild type and PgltA(C–10T) promoters were cultivated in glucose-ammonium-glutamate minimal medium, the GltC wild type protein and to a lesser extent also the GltC variants L146S, A234T and P251S activated the promoter-lacZ fusions (Figure 6B). Thus, albeit affected, the GltC variants with enhanced repressor function did not loose their ability to activate the transcription at the PgltA promoter derivatives. Moreover, the PgltA(C–10T) promoter is still responsive to GltC because transcription was enhanced when the GltC wild type protein and the GltC variants L146S, A234T and P251S were synthesized. When the strain GP650 carrying the wild type PgltA-lacZ fusion was cultivated in glucose-ammonium-glutamate minimal medium with arginine, transcription at the PgltA promoter was strongly reduced (Figure 6B). The GDH RocG, which is synthesized in the presence of arginine, probably binds to the GltC variants to prevent transcription activation at the PgltA promoter (Figures 1B, 6A). As expected, the PgltA(C–10T) promoter was active in the absence of GltC in medium containing arginine. By contrast, under the same conditions the activity of the promoter was slightly reduced when the GltC wild type protein was synthesized. Moreover, the activity of the PgltA(C–10T) promoter was 8-fold reduced in the strains synthesizing the GltC variants L146S, A234T and P251S. To conclude, the single amino acid exchanges in the GltC variants L146S, A234T and P251S indeed increase the repressor function of the DNA-binding protein and the repressor function of GltC seems to depend on a GDH that degrades glutamate to 2-oxoglutarate.
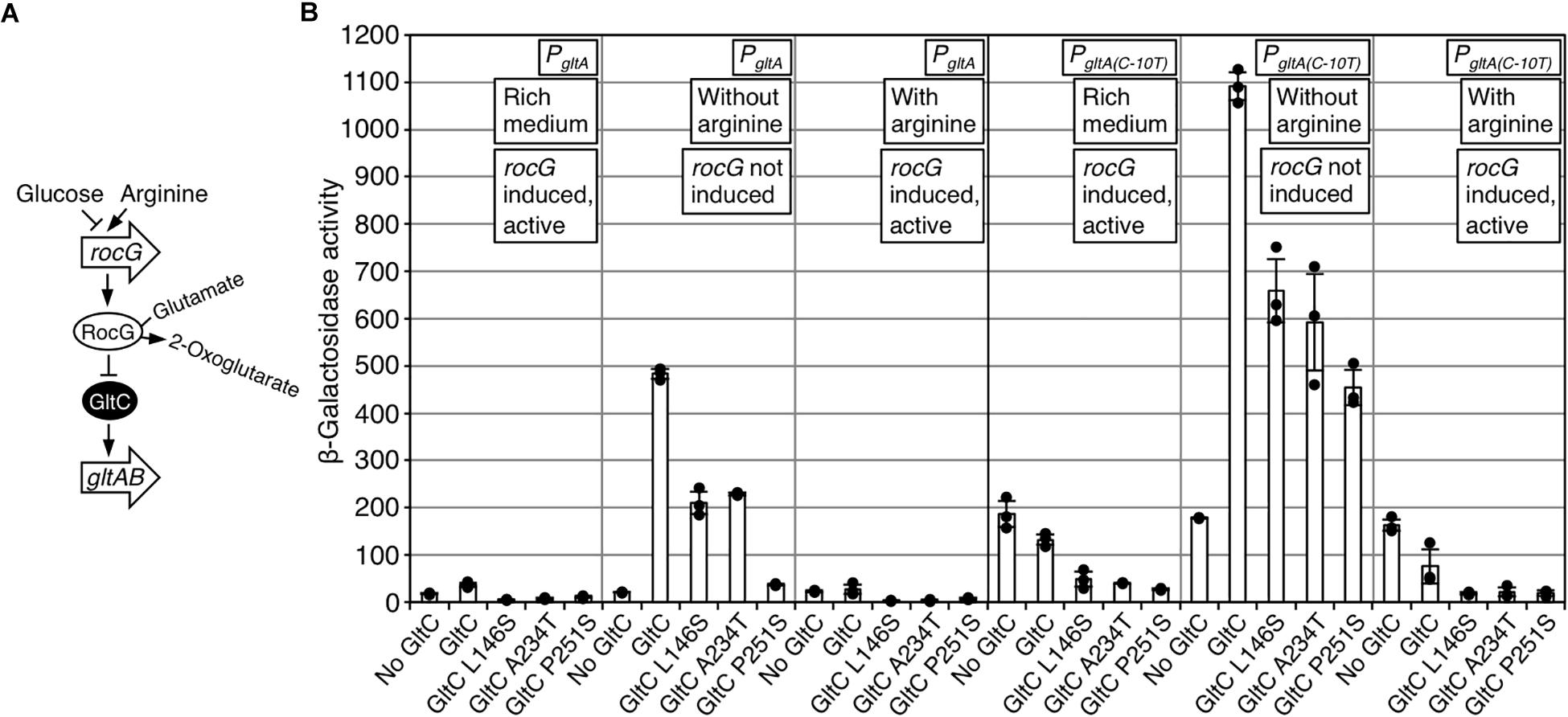
Figure 6. (A) Control of DNA-binding activity of GltC by RocG. (B) Regulation of the PgltA wild type and the PgltA(C–10T) promoter in the strains GP650 (PgltA-lacZ gltC– rocG+ gudB–) and GP692 (PgltA(C–10T)-lacZ gltC– rocG+ gudB–), respectively, synthesizing the GltC variants L146S, A234T, and P251S variants with enhanced repressor function during growth in SP rich medium, CSE-Glc minimal medium without and with arginine. Isogenic strains carrying the plasmids pBQ200 (empty plasmid) and pGP907 (GltC) served as controls. Arginine was added to a final concentration of 0.5% (w/v). Data points represent biologically independent replicates. Bars indicate means of replicates and the standard deviations are shown. β-Galactosidase activities are given as units per milligram of protein. The plasmids carrying the gltC alleles are listed in Table 2.
GltC- and GDH-Dependent Repression of Constitutively Active PgltA Promoters
The characterization of the GltC variants with enhanced repressor function revealed that single amino acid exchanges are sufficient to enhance the repressor function of the regulator (Figure 6B). However, the ability of the GltC variants to inactivate the PgltA wild type and the PgltA(C–10T) promoter seems to depend on a glutamate-degrading GDH (see above). To substantiate this finding, we assessed the activities of translational promoter lacZ fusions containing the PgltA wild type as well as the PgltA(T–32A) and PgltA(C–10T) promoters in strains lacking either GltC or the GDHs or both, GltC and GDH activity. Like the PgltA(C–10T) promoter, also the PgltA(T–32A) promoter is active independent of GltC (Figure 1D) (Commichau et al., 2007a). Thus, both promoters may be useful to unmask a repressor function of GltC. The bacteria were cultivated in glucose-ammonium-glutamate minimal medium without and with arginine, conditions that are known to increase and to reduce the activity of the PgltA promoter, respectively (Figure 7A) (Belitsky and Sonenshein, 2004; Commichau et al., 2007a, b; Stannek et al., 2015). While in the absence of arginine the wild type PgltA promoter was strictly dependent on GltC, the PgltA(T–32A) and PgltA(C–10T) promoters were active without the regulator (7-fold increased expression as compared to the wild type PgltA promoter) (Figure 7B). Moreover, in comparison to the PgltA wild type promoter, the PgltA(T–32A) and PgltA(C–10T) variants were 1.6 - to 3.5-fold more active, respectively, when GltC was synthesized (Figure 7B, compare panels 1–2 with 4–6 from the left). This suggests an additive effect of GltC-dependent and -independent transcription activation at the PgltA(T–32A) and PgltA(C–10T) promoters. Furthermore, the three promoters were slightly more active in a strain lacking both GDHs (Figure 7B, compare panel 4 with panels 5 and 6 from the left). Thus, the GltC and the PgltA promoter variants are still responsive to either RocG or GudB1. During growth with arginine, all promoters were inactive in strains synthesizing GltC and a functional GDH (Figure 7B, compare panel 4 with panels 5 and 6 from the left). The arginine-dependent inactivation of the PgltA(T–32A) and PgltA(C–10T) promoters was relieved in strains lacking GltC (Figure 7B, panels 1–3 from the left). The arginine-dependent inactivation of the promoters also did not occur in the absence of a GDH (Figure 7B, panel 4 from the left). We cannot fully exclude the possibility that the PgltA(T–32A) and PgltA(C–10T) promoters allow GltC to become a repressor of the gltAB genes. However, it is rather unlikely that the spatially separated mutations in the PgltA promoters serendipitously stimulate or cause the repressor function of GltC. Moreover, the wild type PgltA promoter is also inhibited in a GDH-dependent manner (Figure 7B, compare panel 4 with panels 5–6 from the left). The reduced activities of the PgltA, PgltA(T–32A), and PgltA(C–10T) promoters in the presence of arginine in strains lacking any GDH activity likely reflects the inhibitory effect of glutamate on GltC activity. To conclude, in the absence of arginine, GltC activates the transcription at the PgltA promoter and the partially constitutive PgltA(T–32A) and PgltA(C–10T) promoters variants. By contrast, during growth with arginine both GDHs inactivate the promoters in a GltC-dependent manner.
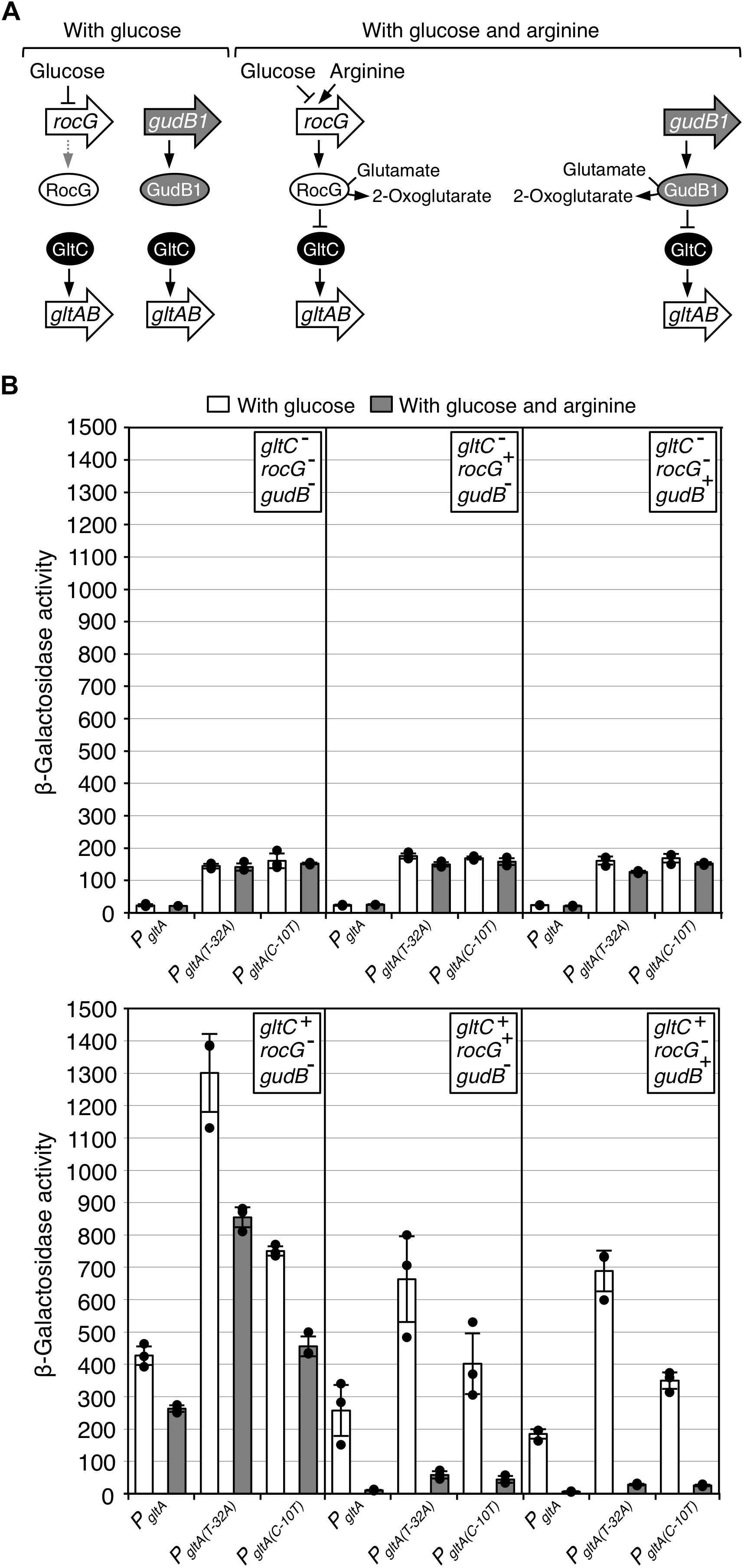
Figure 7. (A) Control of DNA-binding activity of GltC by RocG and GudB1. (B) Regulation of the PgltA wild type and the partially constitutively active PgltA(T–32A) and PgltA(C–10T) promoters by GltC, and the GDHs RocG and GudB1. The strains BP812 (PgltA-lacZ gltC– rocG– gudB–), BP813 (PgltA(T–32A)-lacZ gltC– rocG– gudB–), BP814 (PgltA(C–10T)-lacZ gltC– rocG– gudB–), GP650 (PgltA-lacZ gltC– rocG+ gudB–), GP689 (PgltA(T–32A)-lacZ gltC– rocG+ gudB–), GP692 (PgltA(C–10T)-lacZ gltC– rocG+ gudB–), BP818 (PgltA-lacZ gltC– rocG– gudB1), BP819 (PgltA(T–32A)-lacZ gltC– rocG– gudB1), BP820 (PgltA(C–10T)-lacZ gltC– rocG– gudB1), BP811 (PgltA-lacZ gltC+ rocG– gudB–), BP815 (PgltA(T–32A)-lacZ gltC+ rocG– gudB–), BP816 (PgltA(C–10T)-lacZ gltC+ rocG– gudB–), GP342 (PgltA-lacZ gltC+ rocG+ gudB–), BP809 (PgltA(T–32A)-lacZ gltC+ rocG+ gudB–), BP810 (PgltA(C–10T)-lacZ gltC+ rocG+ gudB–), BP817 (PgltA-lacZ gltC+ rocG– gudB1), BP821 (PgltA(T–32A)-lacZ gltC+ rocG– gudB1), and BP822 (PgltA(C–10T)-lacZ gltC+ rocG– gudB1) were cultivated in CSE-Glc minimal medium without and with arginine. Arginine was added to a final concentration of 0.5% (w/v). Data points represent biologically independent replicates. Bars indicate means of replicates and the standard deviations are shown. β-Galactosidase activities are given as units per milligram of protein.
Inhibition of the Activator Function of GltC Depends on the Native GDHs
To assess whether the ability to modulate the DNA-binding function of GltC is specific for the GDHs from B. subtilis, we introduced the plasmid pGP934 (gdhA) encoding the anabolically active E. coli GDH GdhA into the B. subtilis strain BP220 (ΔgltAB PgltA-lacZ rocG– gudB–). The gltAB genes were deleted in the strain BP220 to prevent production and consumption of 2OG and glutamate by the glutamate synthase GltAB. As illustrated in Figure 8A, GdhA shows about 30% overall amino acid identity with RocG or GudB1. The derivatives of the strain BP220 carrying the plasmids pBQ200 (empty plasmid), pGP529 (rocG) and pGP482 (gudB1) served as controls. Next we propagated the bacteria on glucose-ammonium, glutamate-ammonium, and arginine-ammonium minimal medium agar plates. The growth experiments confirmed that the GdhA is anabolically active in B. subtilis because the enzyme relieves glutamate auxotrophy of the gltAB mutant strain on glucose-ammonium plates (Figure 8B) (Commichau et al., 2008). The E. coli GdhA synthesizes glutamate from ammonium and 2OG in the background of a B. subtilis cell because the affinity of the enzyme for ammonium exceeds that of RocG by a factor of 50 (Sakamoto et al., 1975; Khan et al., 2005; Gunka et al., 2010). As expected, overexpression of the rocG and gudB1 genes allowed the strain BP220 to utilize either glutamate or arginine as sole sources of carbon and nitrogen. By contrast, GdhA did not sustain growth of the bacteria with either glutamate or arginine. Thus, unlike the GDHs RocG and GudB1, the GDH from E. coli is strictly anabolically active in vivo.
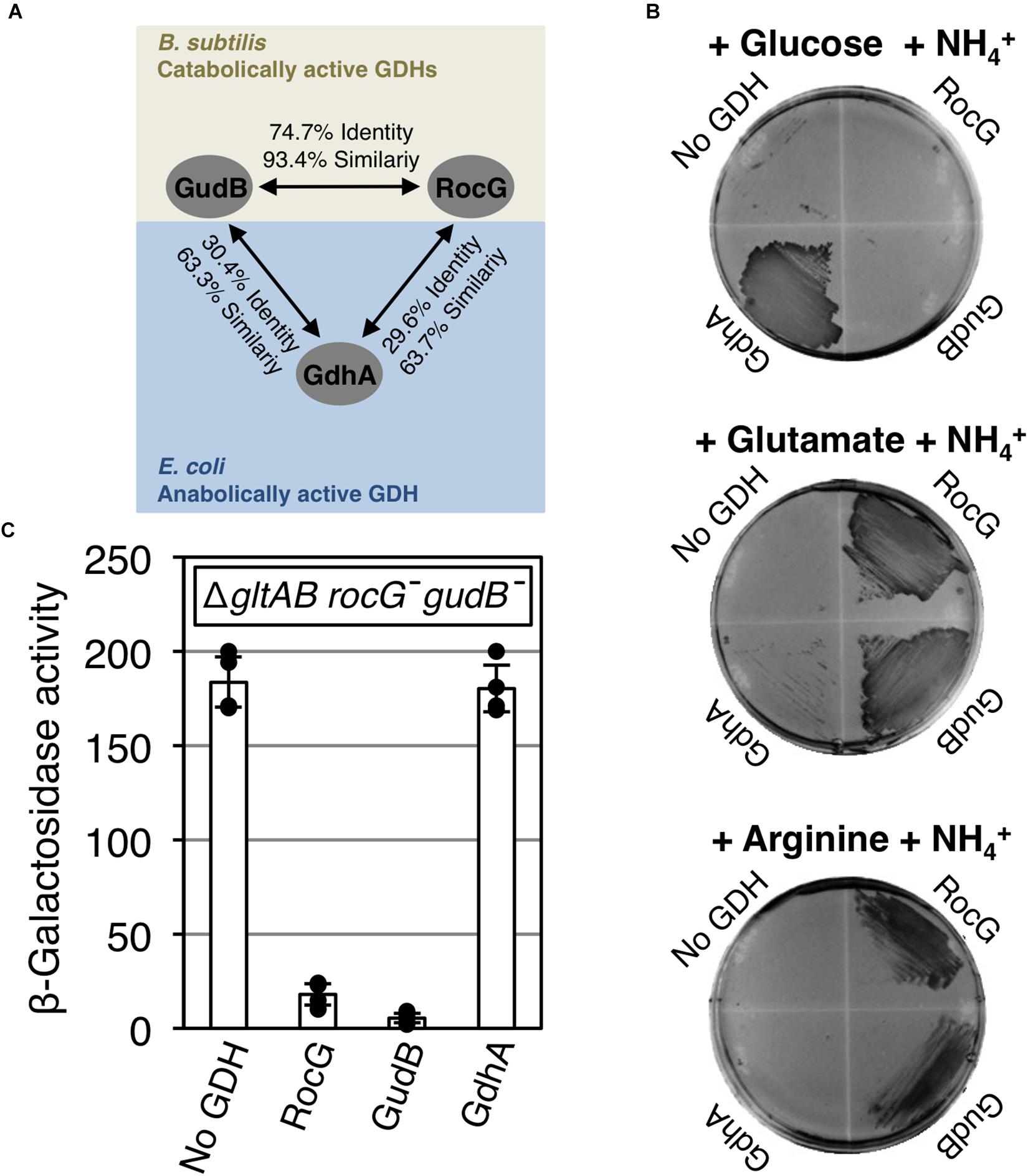
Figure 8. Inactivation of the PgltA promoter by GltC depends on the native GDHs RocG and GudB1. (A) Overall amino acid sequence identity and similarity between B. subtilis RocG and GudB1 and E. coli GdhA. (B) Growth experiments with the strains BP220 (ΔgltAB PgltA-lacZ rocG– gudB–) + pBQ200 (empty plasmid), BP220 + pGP529 (rocG), BP220 + pGP482 (gudB1) and BP220 + pGP934 (gdhA) on C minimal medium plates containing ammonium as a source of nitrogen and either 0.5% (w/v) glucose, 0.8% (w/v) glutamate or 0.5% (w/v) arginine as sources of carbon. (C) Arginine-dependent regulation of the PgltA promoter in the strains BP220 + pBQ200, BP220 + pGP529, BP220 + pGP482, and BP220 + pGP934 in CSE-Glc medium. Arginine was added to a final concentration of 0.5% (w/v). Data points represent biologically independent replicates. Bars indicate means of replicates and the standard deviations are shown. β-Galactosidase activities are given as units per milligram of protein.
Next, we determined the activity of the PgltA promoter lacZ fusion, which allows monitoring impact of the GDHs on the activity of GltC. The derivatives of the strain BP220 carrying the plasmids pBQ200 (empty plasmid), pGP529 (rocG) and pGP482 (gudB1) served as controls. The strains were cultivated in glucose-ammonium-glutamate minimal medium supplemented with arginine as additional nitrogen source, which is converted to glutamate (Figure 1A). As expected, the PgltA promoter was highly active in the absence of a GDH (Figure 8C). Moreover, the anabolically active GDH GdhA from E. coli was unable to inhibit GltC. Thus, glutamate, which accumulates under these growth conditions, is not sufficient to prevent activation of the PgltA promoter. By contrast, the expression of the GDHs RocG and GudB1 resulted in full inactivation of the PgltA promoter. To conclude, the metabolites 2OG and glutamate do not control the DNA-binding mode of GltC alone: repression of the gltAB genes depends on GltC as well as on a native and active GDH that converts glutamate to ammonium and 2OG.
Discussion
Here, we have identified 19 GltC variants that are more active than the wild type protein when the GDHs RocG or GudB1 are synthesized. Two of the GltC variants (P88L and T99A) variants with enhanced activator function have been described previously (Belitsky and Sonenshein, 1995). The amino acid exchanges enhancing the activator function of GltC are located between the linker and the effector domain and they probably facilitate the binding of the regulator to the boxes I and II of the PgltA promoter (Figures 3A,C). Both boxes were shown to be required for the GltC-dependent transcriptional activation of the gltAB glutamate synthase genes (Figure 1B) (Picossi et al., 2007). Alternatively, the amino acid exchanges increasing the activator function of GltC could affect the interaction with the GDHs RocG and GudB1, which were shown to bind to GltC, thereby preventing the transcriptional activation of the gltAB genes (Figure 1B) (Commichau et al., 2007a; Stannek et al., 2015). It has indeed been shown that a single amino acid exchange in the GltC T99A variant slightly weakens the interaction with the GDH RocG (Commichau et al., 2007a). However, only the further characterization of the remaining GltC variants with enhanced activator function will help to uncover how the amino acid exchanges affect the DNA-binding property of regulator and the interaction with the GDHs. We have also observed that some GltC variants (V45M, G127S, G222S, G230R, and L231F) were less active when RocG was produced in the presence of arginine that is converted the glutamate, which is the substrate of the GDH. By contrast, the GltC variant D87G was stronger inhibited by GudB1 than by RocG. The fact that RocG shows about 25% overall sequence divergence with GudB1 could explain why some of the GltC variants with enhanced activator function differentially respond to the GDHs. Probably, the coevolution of GltC and the GDHs is responsible for the emergence of enzyme-regulator interaction sites that slightly differ from each other. Therefore, it will be interesting to study to which extent the amino acid exchanges in the GltC variants with enhanced activator function affect the in vivo-complex formation with the GDHs.
We have also identified three GltC variants (L146S, A234T, and P251S), displaying enhanced repressor activity in vivo (Figures 3A,C). The GltC variants with enhanced repressor function are still capable of activating the transcription of the gltAB genes at the PgltA promoter (Figures 5, 6). Thus, the single amino acid exchanges in these GltC variants did not abolish the activator function of the regulator. Furthermore, we found that the GltC variants with enhanced repressor function and to a lesser extent also the GltC wild type protein were able to inactivate the PgltA(C–10T) promoter in the presence of arginine, which was previously shown to be active independent of GltC (Figures 1D, 6B, panels 5 and 6 from the left) (Commichau et al., 2007a). In addition to this, we show that the wild type GltC protein was capable of inactivating the PgltA(T–32A) promoter that is, like the PgltA(C–10T) promoter, active independent of GltC (Figures 1D, 7B) (Commichau et al., 2007a). Thus, the characterization of the PgltA(T–32A) and PgltA(C–10T) promoters and the GltC variants with enhanced repressor function revealed that GltC may indeed serve as a transcriptional activator and repressor of the gltAB genes in vivo. However, the ability of GltC to prevent transcription at the PgltA promoter was strictly dependent on the presence of a native and active GDH (Figures 7B, 8).
As mentioned above, two models describe the metabolite- and the GDH-dependent regulation of the B. subtilis gltAB genes, which is mediated by GltC (Figures 1B,C). However, both models are incomplete. The model for the metabolite-dependent regulation of the gltAB genes does not include the role of the GDHs in modulating the DNA-binding activity of GltC (Figure 1C) (Belitsky and Sonenshein, 2004; Picossi et al., 2007). However, the GDHs were shown to be the major factors controlling the DNA-binding activity of GltC and thus de novo glutamate synthesis (Commichau et al., 2007a, b; Stannek et al., 2015). Based on the observations of the present study, we propose a model, which combines the metabolite- and the GDH-dependent transcriptional regulation of the gltAB genes (Figure 9). During growth with glucose and ammonium as a source of carbon and ammonium, respectively, GltC activates transcription of the gltAB genes by binding to the boxes I and II in the PgltA promoter in a 2OG-dependent manner and glutamate can be produced (Figure 9) (Picossi et al., 2007). If glutamate is provided to the bacteria, transcription of the gltAB genes is about 2- to 3-fold reduced indicating that GltC responds to glutamate in vivo, independent of a GDH (Commichau et al., 2007b). However, despite the fact that glutamate stimulates binding of GltC to the boxes I and III in the PgltA promoter, which abolishes transcription of the gltAB genes in vitro, glutamate alone does not lead to full repression of the gltAB genes in vivo (Belitsky et al., 2000; Picossi et al., 2007) (Figure 1C). We provide genetic evidence that the GDHs RocG and GudB1 may trigger the repressor function of GltC (Figure 9). Thus, GltC can be active as an activator or as a repressor of the gltAB genes, depending on the presence of an active GDH. In the light of the previous in vitro and in vivo studies it is conceivable that the repressor function of GltC is stimulated by glutamate, which in turn promotes the formation of a GDH-GltC-PgltA promoter complex in vivo (Picossi et al., 2007; Stannek et al., 2015). It has indeed been shown that the GDH-dependent inactivation of the PgltA promoter directly correlates with the glutamate pool (Stannek et al., 2015). However, it is difficult to detect variations in the intracellular 2-oxoglutarate and glutamate levels because the reactions involved in glutamate synthesis and degradation are part of a homeostatic system, enabling B. subtilis to maintain the cellular levels of 2-oxoglutarate and glutamate nearly constant over a wide range of nutritional conditions. Therefore, the direct correlation between the glutamate pool and the activity of the PgltA promoter could only be demonstrated in a strain lacking the gltAB glutamate synthase genes (Stannek et al., 2015). Moreover, it remains to be verified in vitro whether glutamate alone is sufficient to promote the GDH-dependent inhibition of the DNA-binding activity of GltC. Alternatively, in their catabolically active state the GDHs might serve as a “scaffold” facilitating the binding of GltC to the boxes I and III in the PgltA promoter, which leads to repression of the gltAB genes. However, biochemical studies have to be pursued (e.g., DNAse I footprinting and co-crystallization attempts) to understand the molecular details of the interaction between the GDH-GltC protein complex and the PgltA promoter.
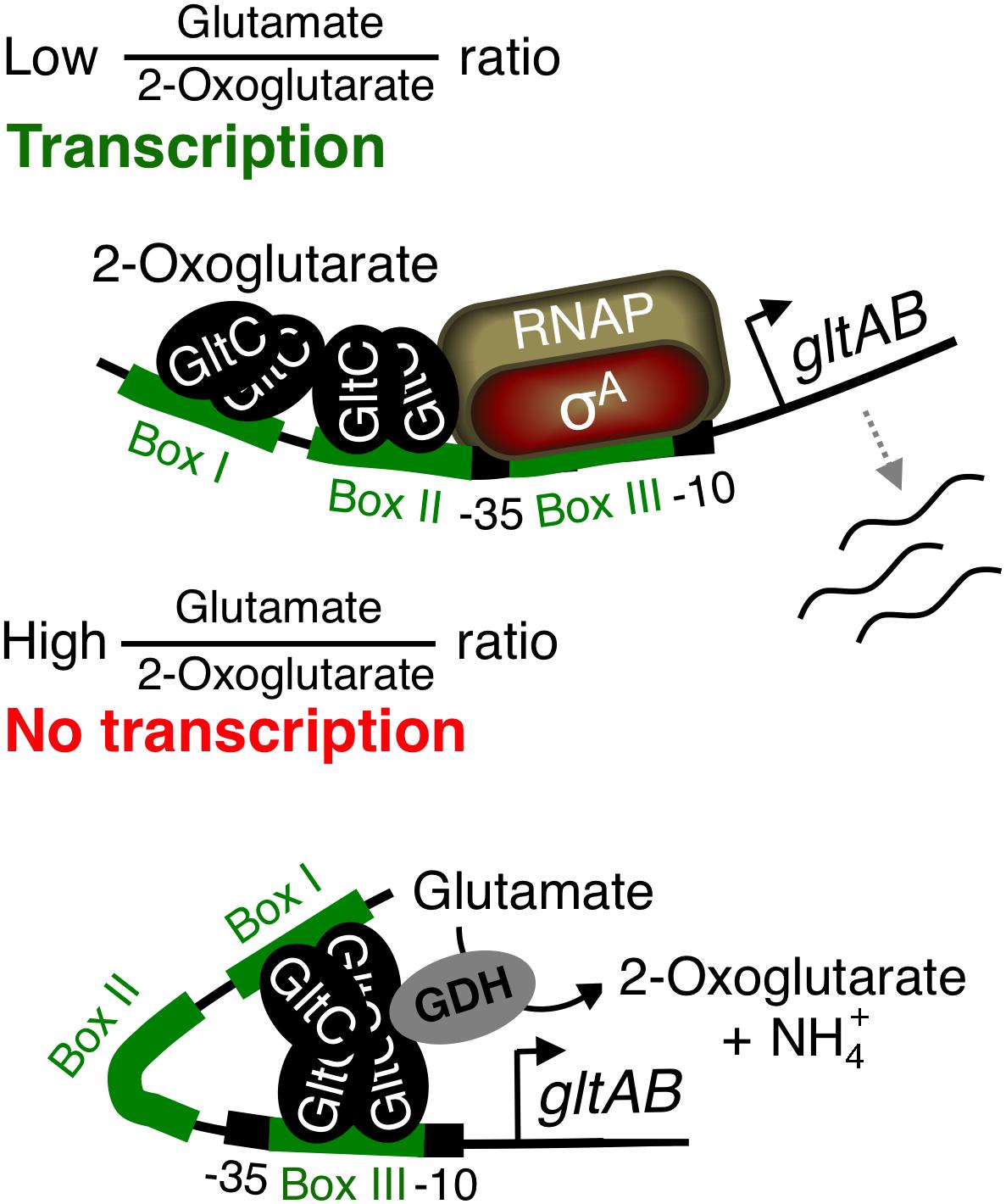
Figure 9. Model for the regulation of the PgltA promoter by GltC and the GDHs. During growth with glucose, GltC binds to boxes I and II of the PgltA promoter and stimulates gltAB transcription by RNA polymerase (RNAP). In the presence of glucose and high amounts of glutamate (e.g., growth with arginine), the glutamate-degrading GDHs RocG and GudB1 convert the activator GltC into a repressor. The binding mode of GltC and the stoichiometry of the protein complex remains to be defined. σA, housekeeping sigma factor A.
GltC is like other LysR-type regulators a dual regulator that can activate and inhibit the same promoter depending on the availability of small-molecule cofactors (Picossi et al., 2007; Mittal et al., 2013; Lerche et al., 2016). Similar to GltC, CcpC plays a dual role in the regulation of the citB aconitase gene in B. subtilis and Listeria monocytogenes (Mittal et al., 2013). At low citrate levels CcpC inhibits citB transcription by binding to two sites in the PcitB promoter, thereby blocking access of the RNAP. At high citrate levels the regulator binds only one site in the PcitB promoter and RNAP can transcribe the citB gene to prevent accumulation of citrate to toxic levels. However, in contrast to CcpC, GltC also depends on an active GDH to exert its repressor function in vivo. However, it remains to be elucidated where and how the metabolites and the GDHs bind to the effector domain of the GltC. Interestingly, many metabolic enzymes are involved in controlling gene expression by modulating the activity of DNA-binding transcription factors (Commichau and Stülke, 2008). For instance, the feedback-inhibited form of the B. subtilis glutamine synthetase (FBI-GS) controls the DNA-binding activities of the MerR-type transcription factors TnrA and GlnR (Wray et al., 2001; Fisher and Wray, 2008; Wray and Fisher, 2008; Murray et al., 2013; Schumacher et al., 2015). While the FBI-GS prevents TnrA from binding to DNA, the enzyme acts as a chaperone to stabilize the interaction between the repressor GlnR and its target promoters. So far only a few studies revealed that additional proteins modulate the DNA-binding activity of LysR-type regulators (Ghrist et al., 2001; Kovacikova et al., 2004; Commichau et al., 2007a; Dangel and Tabita, 2015; Stannek et al., 2015). In Vibrio cholerae the LysR-type regulator AphB requires the DNA-binding AphA protein to activate the tcpPH genes (Kovacikova et al., 2004; Taylor et al., 2012). While AphB seems to be the primary activator, both AphA and AphB are required for full expression of the tcpPH genes. In E. coli it has been demonstrated that the GcvR protein interacts with the DNA-binding regulator GcvA to prevent transcriptional activation of the gcvTHP operon encoding the glycine cleavage system (Ghrist et al., 2001). To conclude, here we provide genetic evidence that the repressor activity of GltC depends on the glutamate-degrading GDHs RocG and GudB1.
Data Availability Statement
All datasets generated for this study are included in the manuscript/supplementary files.
Author Contributions
MD, SL, BR, RH, and TI performed the experiments. FC analyzed the data and wrote the manuscript.
Conflict of Interest
The authors declare that the research was conducted in the absence of any commercial or financial relationships that could be construed as a potential conflict of interest.
Funding
This work was supported by grants from the DFG (Co 1139/1-2) and the Max-Buchner-Forschungsstiftung (http://www.dechema.de/mbf.html; MBFSt-Kennziffer 3381) to FC. The Göttingen Graduate School for Neuroscience, Biophysics and Molecular Biosciences (GGNB) supported MD.
Acknowledgments
We are grateful to Jörg Stülke for fruitful discussions, staff support, and providing plasmids and strains. We thank Philipp Tripal, Maurice Hädrich, Alexandra Berg, and Michael Schröder for the experimental support.
References
Belitsky, B. R., Janssen, P. J., and Sonenshein, A. L. (1995). Sites required for GltC-dependent regulation of Bacillus subtilis glutamate synthase expression. J. Bacteriol. 177, 5686–5695. doi: 10.1128/jb.177.19.5686-5695.1995
Belitsky, B. R., Kim, H. J., and Sonenshein, A. L. (2004). CcpA-dependent regulation of Bacillus subtilis glutamate dehydrogenase gene expression. J. Bacteriol. 186, 3392–3398. doi: 10.1128/jb.186.11.3392-3398.2004
Belitsky, B. R., and Sonenshein, A. L. (1995). Mutations in GltC that increase Bacillus subtilis gltA expression. J. Bacteriol. 177, 5696–5700. doi: 10.1128/jb.177.19.5696-5700.1995
Belitsky, B. R., and Sonenshein, A. L. (1998). Role and regulation of Bacillus subtilis glutamate dehydrogenase genes. J. Bacteriol. 180, 6298–6305.
Belitsky, B. R., and Sonenshein, A. L. (2004). Modulation of activity of Bacillus subtilis regulatory proteins GltC and TnrA by glutamate dehydrogenase. J. Bacteriol. 186, 3399–3407. doi: 10.1128/jb.186.11.3399-3407.2004
Belitsky, B. R., Wray, L. V. Jr., Fisher, S. H., Bohannon, D. E., and Sonenshein, A. L. (2000). Role of TnrA in nitrogen source-dependent repression of Bacillus subtilis glutamate synthase gene expression. J. Bacteriol. 182, 5939–5947. doi: 10.1128/jb.182.21.5939-5947.2000
Bohannon, D. E., and Sonenshein, A. L. (1989). Positive regulation of glutamate synthase in Bacillus subtilis. J. Bacteriol. 171, 4718–4727. doi: 10.1128/jb.171.9.4718-4727.1989
Choi, S. K. Jr., and Saier, M. H. (2005). Regulation of sigL expression by the catabolite control protein CcpA involves a roadblock mechanism in Bacillus subtilis: potential connection between carbon and nitrogen metabolism. J. Bacteriol. 187, 6856–6861. doi: 10.1128/jb.187.19.6856-6861.2005
Commichau, F. M., Forchhammer, K., and Stülke, J. (2006). Regulatory links between carbon and nitrogen metabolism. Curr. Opin. Microbiol. 9, 167–172. doi: 10.1016/j.mib.2006.01.001
Commichau, F. M., Gunka, K., Landmann, J. J., and Stülke, J. (2008). Glutamate metabolism in Bacillus subtilis: gene expression and enzyme activities evolved to avoid futile cycles and to allow rapid responses to perturbations of the system. J. Bacteriol. 190, 3557–3364. doi: 10.1128/JB.00099-08
Commichau, F. M., and Stülke, J. (2008). Trigger enzymes: bifunctional proteins active in metabolism and in controlling gene expression. Mol. Microbiol. 67, 692–702. doi: 10.1111/j.1365-2958.2007.06071.x
Commichau, F. M., Wacker, I., Schleider, J., Blencke, H. M., Reif, I., Tripal, P., et al. (2007b). Characterization of Bacillus subtilis mutants with carbon source-independent glutamate biosynthesis. J. Mol. Microbiol. Biotechnol. 12, 106–113. doi: 10.1159/000096465
Commichau, F. M., Herzberg, C., Tripal, P., Valerius, O., and Stülke, J. (2007a). A regulatory protein-protein interaction governs glutamate biosynthesis in Bacillus subtilis: the glutamate dehydrogenase RocG moonlights in controlling the transcription factor GltC. Mol. Microbiol. 65, 642–654. doi: 10.1111/j.1365-2958.2007.05816.x
Dangel, A. W., and Tabita, F. R. (2015). CbbR, the master regulator for microbial carbon dioxide fixation. J. Bacteriol. 197, 3488–3498. doi: 10.1128/JB.00442-15
Faires, N., Tobisch, S., Bachem, S., Martin-Verstraete, I., Hecker, M., and Stülke, J. (1999). The catabolite control protein CcpA controls ammonium assimilation in Bacillus subtilis. J. Mol. Microbiol. Biotechnol. 1, 141–148.
Fisher, S. H. Jr., and Wray, L. V. (2008). Bacillus subtilis glutamine synthetase regulates its own expression by acting as a chaperone to stabilize GlnR-DNA complexes. Proc. Natl. Acad. Sci. U.S.A. 105, 1014–1019. doi: 10.1073/pnas.0709949105
Gardan, R., Rapoport, G., and Débarbouillé, M. (1997). Role of the transcriptional activator RocR in the arginine-degradation pathway of Bacillus subtilis. Mol. Microbiol. 24, 825–837. doi: 10.1046/j.1365-2958.1997.3881754.x
Ghrist, A. C., Heil, G., and Stauffer, G. V. (2001). GcvR interacts with GcvA to inhibit activation of the Escherichia coli glycine cleavage operon. Microbiology 147, 2215–2221. doi: 10.1099/00221287-147-8-2215
Greener, A., Callahan, M., and Jerpseth, B. (1996). An efficient random mutagenesis technique using an E. coli mutator strain. Methods Mol. Biol. 57, 375–385.
Guérout-Fleury, A. M., Shazand, K., Frandsen, N., and Stragier, P. (1995). Antibiotic resistance cassettes for Bacillus subtilis. Gene 167, 335–336. doi: 10.1016/0378-1119(95)00652-4
Gunka, K., and Commichau, F. M. (2012). Control of glutamate homeostasis in Bacillus subtilis: a complex interplay between ammonium assimilation, glutamate biosynthesis and degradation. Mol. Microbiol. 85, 213–224. doi: 10.1111/j.1365-2958.2012.08105.x
Gunka, K., Newman, J. A., Commichau, F. M., Herzberg, C., Rodrigues, C., Hewitt, L., et al. (2010). Functional dissection of a trigger enzyme: mutations of the Bacillus subtilis glutamate dehydrogenase RocG that affect differentially its catalytic activity and regulatory properties. J. Mol. Biol. 400, 815–827. doi: 10.1016/j.jmb.2010.05.055
Gunka, K., Stannek, L., Care, R. A., and Commichau, F. M. (2013). Selection-driven accumulation of suppressor mutants in Bacillus subtilis: the apparent high mutation frequency of the cryptic gudB gene and the rapid clonal expansion of gudB(+) suppressors are due to growth under selection. PLoS One 8:e66120. doi: 10.1371/journal.pone.0066120
Gunka, K., Tholen, S., Gerwig, J., Herzberg, C., Stülke, J., and Commichau, F. M. (2012). A high-frequency mutation in Bacillus subtilis: requirements for the decryptification of the gudB glutamate dehydrogenase gene. J. Bacteriol. 194, 1036–1044. doi: 10.1128/JB.06470-11
Khan, M. I., Ito, K., Kim, H., Ashida, H., Ishikawa, T., Shibata, H., et al. (2005). Molecular properties and enhancement of thermostability by random mutagenesis glutamate dehydrogenases from Bacillus subtilis. Biosci. Biotechnol. Biochem. 69, 1861–1870. doi: 10.1271/bbb.69.1861
Kovacikova, G., Lin, W., and Skorupski, K. (2004). Vibrio cholerae AphA uses a novel mechanism for virulence gene activation that involves interaction with the LysR-type regulator AphB at the tcpPH promoter. Mol. Microbiol. 53, 129–142. doi: 10.1111/j.1365-2958.2004.04121.x
Kunst, F., and Rapoport, G. (1995). Salt stress is an environmental signal affecting degradative enzyme synthesis in Bacillus subtilis. J. Bacteriol. 177, 2403–2407. doi: 10.1128/jb.177.9.2403-2407.1995
Lerche, M., Dian, C., Round, A., Lönneborg, R., Brzezinski, P., and Leonard, G. A. (2016). The solution configurations of inactive and activated DntR have implications for the sliding dimer mechanism of LysR transcription factors. Sci. Rep. 6:19988. doi: 10.1038/srep19988
Maddocks, S. E., and Oyston, P. C. F. (2008). Structure and function of the LysR-type transcriptional regulator (LTTR) family proteins. Microbiology 154, 3609–3623. doi: 10.1099/mic.0.2008/022772-0
Martin-Verstraete, I., Débarbouillé, M., Klier, A., and Rapoport, G. (1994). Interactions of wild-type and truncated LevR of Bacillus subtilis with the upstream activating sequence of the levanase operon. J. Mol. Biol. 241, 178–192. doi: 10.1006/jmbi.1994.1487
Mittal, M., Pechter, K. B., Picossi, S., Kim, H. J., Kerstein, K. O., and Sonenshein, A. L. (2013). Dual role of CcpC protein in regulation of aconitase gene expression in Listeria monocytogenes and Bacillus subtilis. Microbiology 159, 68–76. doi: 10.1099/mic.0.063388-0
Muraoka, S., Okumura, R., Ogawa, N., Nonaka, T., Miyashita, K., and Senda, T. (2003). Crystal structure of a full-length LysR-type transcriptional regulator, CbnR: unusual combination of two subunit forms and molecular basis for causing a changing DNA bend. J. Mol. Biol. 328, 555–566. doi: 10.1016/s0022-2836(03)00312-7
Murray, D. S., Chinnam, N., Tonthat, N. K., Whitfill, T. Jr., Wray, L. V., Fisher, S. H., et al. (2013). Structures of the Bacillus subtilis glutamine synthetase dodecamer reveal large intersubunit catalytic conformational changes linked to a unique feedback inhibition mechanism. J. Biol. Chem. 288, 35801–35811. doi: 10.1074/jbc.M113.519496
Noda-Garcia, L., Romero Romero, M. L., Longo, L. M., Kolodkin-Gal, I., and Tawfik, D. S. (2017). Bacilli glutamate dehydrogenases diverged via coevolution of transcription and enzyme regulation. EMBO Rep. 18, 1139–1149. doi: 10.15252/embr.201743990
Park, J. O., Rubin, S. A., Xu, Y. F., Amador-Noguez, D., Fan, J., Shlomi, T., et al. (2016). Metabolic concentrations, fluxes and free energies imply efficient enzyme usage. Nat. Chem. Biol. 12, 482–489. doi: 10.1038/nchembio.2077
Picossi, S., Belitsky, B. R., and Sonenshein, A. L. (2007). Molecular mechanism of the regulation of Bacillus subtilis gltAB expression by GltC. J. Mol. Biol. 365, 1298–1313. doi: 10.1016/j.jmb.2006.10.100
Sakamoto, N., Kotre, A. M., and Savageau, M. A. (1975). Glutamate dehydrogenase from Escherichia coli: purification and propterties. J. Bacteriol. 124, 775–783.
Sambrook, J., Fritsch, E. F., and Maniatis, T. (1989). Molecular Cloning: a Laboratory Manual, 2nd Edn. Cold Spring Harbor, N.Y: Cold Spring Harbor Laboratory.
Schumacher, M. A., Chinnam, N. B., Cuthbert, B., Tonthat, N. K., and Whitfill, T. (2015). Structures of regulatory machinery reveal novel molecular mechanisms controlling B. subtilis nitrogen homeostasis. Genes Dev. 29, 451–464. doi: 10.1101/gad.254714.114
Sonenshein, A. L. (2007). Control of key metabolic intersections in Bacillus subtilis. Nat. Rev. Microbiol. 5, 917–927. doi: 10.1038/nrmicro1772
Stannek, L., Thiele, M. J., Ischebeck, T., Gunka, K., Hammer, E., Völker, U., et al. (2015). Evidence for synergistic control of glutamate biosynthesis by glutamate dehydrogenases and glutamate in Bacillus subtilis. Environ. Microbiol. 17, 3379–3390. doi: 10.1111/1462-2920.12813
Taylor, J. L., De Silva, S. R., Kovacikova, G., Lin, W., Taylor, R. K., Skorupski, K., et al. (2012). The crystal structure of AphB, a virulence gene activator from Vibrio cholerae, reveals residues that influence its response to oxygen and pH. Mol. Microbiol. 83, 457–470. doi: 10.1111/j.1365-2958.2011.07919.x
Tolner, B., Ubbink-Kok, T., Poolman, B., and Konings, W. N. (1995). Characterization of the protein/glutamate symport protein of Bacillus subtilis and its functional expression in Escherichia coli. J. Bacteriol. 177, 2863–2869. doi: 10.1128/jb.177.10.2863-2869.1995
Wacker, I., Ludwig, H., Reif, I., Blencke, H. M., Detsch, C., and Stülke, J. (2003). The regulatory link between carbon and nitrogen metabolism in Bacillus subtilis: regulation of the gltAB operon by the catabolite control protein CcpA. Microbiology 149, 3001–3009. doi: 10.1099/mic.0.26479-0
Waterhouse, A., Bertoni, M., Bienert, S., Studer, G., Tauriello, G., Gumienny, R., et al. (2018). SWISS-MODEL: homology modeling of protein structures and complexes. Nucleic Acids Res. 46, W296–W303. doi: 10.1016/j.apsb.2016.10.003
Wicke, D., Schulz, L. M., Lentes, S., Scholz, P., Poehlein, A., Gibhardt, J., et al. (2019). Identification of the first glyphosate transporter by genomic adaptation. Environ. Microbiol. 21, 1287–1305. doi: 10.1111/1462-2920.14534
Wray, L. V. Jr., and Fisher, S. H., (2008).Bacillus subtilis GlnR contains an autoinhibitory C-terminal domain required for the interaction with glutamine synthetase. Mol. Microbiol. 68, 277–285 doi: 10.1111/j.1365-2958.2008.06162.x
Wray, L. V. Jr., Zalieckas, J. M., and Fisher, S. H. (2001). Bacillus subtilis glutamine synthetase controls gene expression through a protein-protein interaction with transcription factor TnrA. Cell 107, 427–435. doi: 10.1016/s0092-8674(01)00572-4
Yan, D. (2007). Protection of the glutamate pool concentration in enteric bacteria. Proc. Natl. Acad. Sci. U.S.A. 104, 9475–9480. doi: 10.1073/pnas.0703360104
Zaprasis, A., Bleisteiner, M., Kerres, A., Hoffmann, T., and Bremer, E. (2015). Uptake of amino acids and their metabolic conversion into compatible solute proline confers osmoprotection to Bacillus subtilis. Appl. Environ. Microbiol. 81, 250–259. doi: 10.1128/AEM.02797-14
Zeigler, D., Prágai, Z., Rodrigues, S., Chevreux, B., Muffler, A., Albert, T., et al. (2008). The origins of 168, W23, and other Bacillus subtilis legacy strains. J. Bacteriol. 190, 6983–6995. doi: 10.1128/JB.00722-08
Keywords: glutamate biosynthesis, glutamate dehydrogenase, trigger enzyme, mutational analysis, promoter
Citation: Dormeyer M, Lentes S, Richts B, Heermann R, Ischebeck T and Commichau FM (2019) Variants of the Bacillus subtilis LysR-Type Regulator GltC With Altered Activator and Repressor Function. Front. Microbiol. 10:2321. doi: 10.3389/fmicb.2019.02321
Received: 01 August 2019; Accepted: 23 September 2019;
Published: 09 October 2019.
Edited by:
Patricia Coutinho Dos Santos, Wake Forest University, United StatesCopyright © 2019 Dormeyer, Lentes, Richts, Heermann, Ischebeck and Commichau. This is an open-access article distributed under the terms of the Creative Commons Attribution License (CC BY). The use, distribution or reproduction in other forums is permitted, provided the original author(s) and the copyright owner(s) are credited and that the original publication in this journal is cited, in accordance with accepted academic practice. No use, distribution or reproduction is permitted which does not comply with these terms.
*Correspondence: Fabian M. Commichau, ZmNvbW1pYzFAZ3dkZy5kZQ==