- Laboratory for Viral Immune Pathogenesis, Department of Experimental Immunology, Amsterdam UMC, Amsterdam Infection & Immunity Institute, University of Amsterdam, Amsterdam, Netherlands
Current antiretroviral therapy (ART) effectively suppresses Human Immunodeficiency Virus type 1 (HIV-1) in infected individuals. However, even long term ART does not eradicate HIV-1 infected cells and the virus persists in cellular reservoirs. Beside memory CD4+ T cells, cells of the myeloid lineage, especially macrophages, are believed to be an important sanctuary for HIV-1. Monocytes and macrophages are key players in the innate immune response to pathogens and are recruited to sites of infection and inflammation. Due to their long life span and ability to reside in virtually every tissue, macrophages have been proposed to play a critical role in the establishment and persistence of the HIV-1 reservoir. Current HIV-1 cure strategies mainly focus on the concept of “shock and kill” to purge the viral reservoir. This approach aims to reactivate viral protein production in latently infected cells, which subsequently are eliminated as a consequence of viral replication, or recognized and killed by the immune system. Macrophage susceptibility to HIV-1 infection is dependent on the local microenvironment, suggesting that molecular pathways directing differentiation and polarization are involved. Current latency reversing agents (LRA) are mainly designed to reactivate the HIV-1 provirus in CD4+ T cells, while their ability to abolish viral latency in macrophages is largely unknown. Moreover, the resistance of macrophages to HIV-1 mediated kill and the presence of infected macrophages in immune privileged regions including the central nervous system (CNS), may pose a barrier to elimination of infected cells by current “shock and kill” strategies. This review focusses on the role of monocytes/macrophages in HIV-1 persistence. We will discuss mechanisms of viral latency and persistence in monocytes/macrophages. Furthermore, the role of these cells in HIV-1 tissue distribution and pathogenesis will be discussed.
Introduction
Antiretroviral therapy (ART) has dramatically improved the clinical outcome of Human Immunodeficiency Virus type 1 (HIV-1) infection. However, eradication of HIV-1 is not achieved due to persistence of a viral reservoir that harbors latent provirus that is reactivated upon discontinuation of ART. This latent viral reservoir is a major hurdle in curative treatment of HIV-1 infection and therefore new therapeutic approaches that aim to eliminate or reduce the viral reservoir are explored.
CD4+ T cells and cells from the monocyte/macrophage lineage are considered as the most important target cells for HIV-1, and play an important role in viral persistence and the formation of the viral reservoir. While the major viral reservoir in treated HIV-1 infection is comprised of CD4+ T cells, the distribution and characteristics of the monocyte/macrophage reservoir remain largely unknown.
Monocytes and macrophages are part of the innate immune system and respond to different signals which will direct macrophage differentiation, polarization and function. Monocytes/macrophages sense and clear pathogens, serve as antigen presenting cells priming the adaptive immune response, are involved in both pro- and anti-inflammatory responses, and tissue repair. Macrophages are found in all lymphoid as well as non-lymphoid tissues and their origin was originally described to be dependent on circulating monocytes, which would replenish the tissue-resident macrophage pool if needed. Recent studies in mice showed that the majority of tissue-resident macrophages are embryonically derived and have been implicated to be self-renewing, with the exception of the mucosal/border tissues like the intestine, the dermis and the heart, where bone marrow-derived circulating monocytes constantly replenish the aging tissue-resident macrophage pool (Ginhoux et al., 2010; Schulz et al., 2012; Hashimoto et al., 2013; Yona et al., 2013; Bain et al., 2014; Hoeffel et al., 2015; Mass et al., 2016). The proportion between the pool of tissue-resident macrophages and the pool of monocytes-derived macrophages vary during a state of homeostasis or inflammation (Wang and Kubes, 2016).
Upon differentiation of monocytes into macrophages, these cells become susceptible to HIV-1 infection and due to their ability to migrate into tissues, they contribute to the spread of viral infection to nearly every tissue of the body including gut, semen, lung, gut-associated lymphoid tissue, brain, liver, urethra, and lymph nodes (Zhang et al., 1998; Mayer et al., 1999; Lambotte et al., 2005; Guadalupe et al., 2006; Poles et al., 2006; Chun et al., 2008; Zalar et al., 2010; Deleage et al., 2011; Abbas et al., 2014; Yukl et al., 2014; Cribbs et al., 2015; Hansen et al., 2016; Rose et al., 2016; Kandathil et al., 2018; Tso et al., 2018; Ganor et al., 2019; Ko et al., 2019). Furthermore, macrophages are considered long lived, resistant to virally induced cytopathic effects, and can reside in anatomical sanctuaries with restricted penetration of ART, which will support viral persistent even during ART (Ho et al., 1986; Nicholson et al., 1986; Lafeuillade et al., 1998; Solas et al., 2003; Estes et al., 2017; Clayton et al., 2018).
In this review we will discuss the role of monocytes/macrophages in HIV-1 pathogenesis, HIV-1 persistence and viral reservoir.
Monocytes and Macrophages in HIV-1 Pathogenesis
HIV-1 Transmission
Human Immunodeficiency Virus type 1 enters the body mostly through mucosal surfaces of the genital or gastrointestinal tract. The underlying lamina propria is populated with high populations of lymphocytes and macrophages, which express CD4 in combination with the coreceptor C-C chemokine receptor type 5 (CCR5) and serve as targets for HIV-1 infection. Although the CD4+ T cells in the lamina propria are considered the major target for HIV-1 (Zhang et al., 1999; Greenhead et al., 2000; Gupta et al., 2002; Guadalupe et al., 2003; Brenchley et al., 2004; Hladik et al., 2007), infection of macrophages located in the lamina propria of the intestinal, penile urethral and vaginal mucosa has been described (Shen et al., 2009; Zalar et al., 2010; Ganor et al., 2013; Josefsson et al., 2013; Yukl et al., 2014). This confirms a role for macrophages during the early phases of infection, however, it remains under debate whether these mucosal macrophages support HIV-1 replication and spread the infection. Indeed, HIV-1 RNA has been demonstrated to be present in macrophages located in the vagina (Shen et al., 2009), but not in the gut, indicating that local environmental signals required for efficient viral replication in macrophages can be lacking in local mucosal tissues under homeostatic conditions.
Monocytes in HIV-1 Infection
Human Immunodeficiency Virus type 1 infection is characterized by high immune activation and inflammation, caused by high levels of HIV-1 replication, bacterial translocation, coinfection with other viruses (e.g., CMV, HCV, HBV) and, immune dysregulation (Brenchley and Douek, 2008; Deeks, 2011). ART strongly reduces HIV-1 associated inflammation and immune activation, however, residual immune activation and inflammation is still observed even in effectively treated individuals (Brenchley and Douek, 2008; Deeks, 2011). During HIV-1 infection, increased activation and differentiation of peripheral blood monocytes is also observed (Allen et al., 1990; Thieblemont et al., 1995; Pulliam et al., 1997; Ancuta et al., 2008; Hearps et al., 2012; Williams et al., 2012; Westhorpe et al., 2014; Booiman et al., 2017), which may affect their susceptibility to HIV-1 infection and their ability to migrate into the tissues, thus contributing to HIV-1 pathogenesis.
In the peripheral blood, three monocyte subpopulations can be distinguished based on the expression of the LPS receptor CD14 and the FcγIII receptor CD16. The majority of the peripheral blood monocytes (>85%) are classical monocytes that express high levels of CD14 (CD14++CD16-), whereas CD16 is co-expressed on the intermediate (CD14++CD16+) and non-classical monocytes (CD14+CD16+) (Passlick et al., 1989; Ziegler-Heitbrock et al., 2010; Wong et al., 2011). Murine studies and more recently a humanized mouse study showed that monocyte precursors differentiate first into classical monocytes in bone marrow for a maturation phase of ∼ 38 h. This classical monocyte population is retained in the bone marrow and can respond to acute systemic inflammation. Most classical monocytes remain in the circulation for approximately 1–3 day before migration into the tissues. However, a small proportion matures further into intermediate monocytes within the circulation and most of these cells finally mature into non-classical monocytes before leaving the circulation (Sunderkotter et al., 2004; Varol et al., 2007; Yona et al., 2013; Gamrekelashvili et al., 2016; Patel et al., 2017). In HIV-1 infected individuals, an expansion of CD16 expressing monocytes or intermediate monocytes (CD14+CD16+) is evident during all phases of infection, especially in viremic individuals, which is normalized after initiation of ART in at least part of the patients (Allen et al., 1990; Thieblemont et al., 1995; Pulliam et al., 1997; Ancuta et al., 2008; Hearps et al., 2012; Williams et al., 2012; Westhorpe et al., 2014; Booiman et al., 2017). Moreover, monocytes express increased levels of activation markers (e.g., CD163, HLA-DR, CD69), T-cell costimulatory molecules (e.g., CD40 and CD86), adhesion molecules (e.g., CD11b, CD11c, CD91, CX3CR1), and chemokine receptors (e.g., C-C chemokine receptor type 2 (CCR2), CCR5), which is also observed during ART (Pulliam et al., 1997; Ancuta et al., 2008; Hearps et al., 2012; Westhorpe et al., 2014; Booiman et al., 2017).
In the peripheral blood, a small population (<0.1%) of the monocytes harbor replication competent HIV-1 provirus (Furtado et al., 1999; Crowe and Sonza, 2000; Sonza et al., 2001; Shiramizu et al., 2005; Ellery et al., 2007). While classical monocytes are relatively resistant to HIV-1 infection most likely due to low CCR5 expression, CD16+ or intermediate monocytes express higher levels of CCR5 and can be infected by HIV-1 (Ellery et al., 2007; Ancuta et al., 2008). Indeed, CD16+ or intermediate monocytes have been demonstrated to harbor proviral DNA in untreated as well as treated HIV-1 infection (Shiramizu et al., 2005; Jaworowski et al., 2006, 2007; Ellery et al., 2007).
Tissue Macrophages as HIV-1 Reservoirs
Macrophages can be found in all lymphoid as well as non-lymphoid tissues and could therefore serve as a tissue reservoir for HIV-1 (Figure 1). In the lamina propria of mucosal tissues like the gastro intestinal tract, penile urethra and vagina, HIV-1 infection of local macrophages has been demonstrated in tissues from healthy individuals in vitro (Shen et al., 2009; Ganor et al., 2013), and in vivo in HIV-1 infected untreated (Josefsson et al., 2013) and ART treated individuals (Zalar et al., 2010; Josefsson et al., 2013; Yukl et al., 2014; Ganor et al., 2019). Although, HIV-1 DNA has been readily detected in these mucosal tissues, viral RNA has been demonstrated only in vaginal and urethral macrophages (Shen et al., 2009; Ganor et al., 2019). Moreover, replication competent HIV-1 could be isolated from urethral macrophages (Ganor et al., 2019). In contrast, HIV-1 proviral integration could not be detected in macrophages from colon of ART treated HIV-1 infected individuals (Cattin et al., 2019), and therefore it seems unlikely that these cells contribute to the replication competent HIV-1 reservoir (Shen et al., 2011).
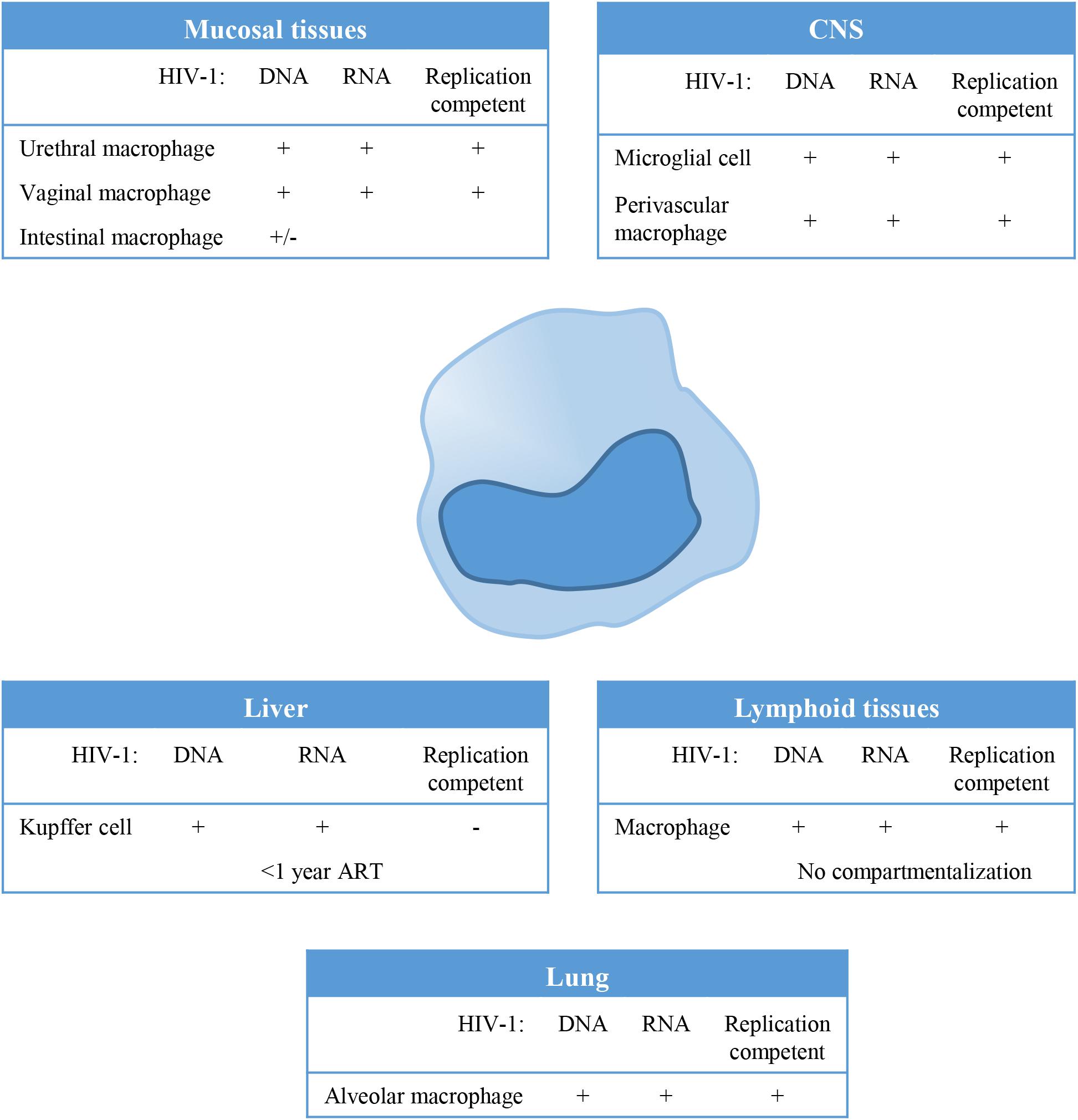
Figure 1. Tissue distribution and anatomical sanctuaries of latently infected macrophages. Macrophages can be found in all lymphoid as well as non-lymphoid tissues and could therefore serve as a tissue reservoir for Human Immunodeficiency Virus type 1(HIV-1). This figure summarizes findings on HIV-1 latency in monocytes/macrophages in vivo, showing anatomical sanctuaries, the presence of HV-1 DNA and RNA and whether the virus was replication competent or not.
In lymphoid tissues like spleen and lymph node, CD4+ T cells (resting memory cells) are the predominant reservoir, however, latently infected resident macrophages have been detected at low frequencies in lymph nodes (Embretson et al., 1993; Orenstein et al., 1997). HIV-1 residing in lymphoid tissues does not genetically differ from variants circulating in the blood, which suggest that there is no compartmentalization of HIV-1 in lymphoid tissue (Ball et al., 1994; van’t Wout et al., 1998). However, genetic diversity of HIV-1 and independent viral evolution in monocytes and CD4+ T cells during the course of infection in treated and untreated patients suggests cellular compartmentalization (Fulcher et al., 2004; Llewellyn et al., 2006).
Tissue resident macrophages like Kupffer cells in the liver have been demonstrated to be susceptible to HIV-1 infection, and persistence of HIV-1 in Kupffer cells has been demonstrated in HIV-1 infected individuals on ART (Schmitt et al., 1990; Hufert et al., 1993; Mosoian et al., 2017; Kandathil et al., 2018). However, virus particles produced by Kupffer cells could not be propagated in vitro using CEMx174 cells (Kandathil et al., 2018). Phylogenetic analysis of the viral quasi-species showed distinct clustering of viral variants in the liver, evident of compartmentalization of HIV-1 in the liver (Penton and Blackard, 2014). Also alveolar macrophages in the lung have been identified as HIV-1 targets, and HIV-1 persistence as determined by the presence of HIV-1 DNA and HIV-1 RNA has been demonstrated in patients, even during effective ART (Sierra-Madero et al., 1994; Jambo et al., 2014; Cribbs et al., 2015). Analysis of the HIV quasi-species also suggested compartmentalization of macrophage tropic HIV-1 variants in the lung (van’t Wout et al., 1998).
The central nervous system (CNS) has been identified as an important viral reservoir. HIV-1 enters the CNS through trafficking of infected cells across the blood brain barrier (BBB) which occurs already shortly after primary infection. Indeed, HIV-1 associated monocyte activation increases migratory abilities of peripheral monocytes by the upregulation of adhesion molecules and chemokine receptors, in response to for instance the monocyte chemoattractant protein 1 (MCP1) (Ancuta et al., 2004; Williams et al., 2013, 2014). In the CNS, HIV-1 persists predominantly in resident macrophages, like microglial cells and perivascular macrophages (Stoler et al., 1986; Wiley et al., 1986; Neuen-Jacob et al., 1993; Fischer-Smith et al., 2001; Cosenza et al., 2002; Ko et al., 2019). Genetic analysis of the viral quasi-species revealed compartmentalized viral replication in the CNS (Korber et al., 1994; van’t Wout et al., 1998; Schnell et al., 2009).
Although HIV-1 infection of macrophages has been demonstrated in different tissue, their role as a replication-competent reservoir remains under debate (Figure 1). Macrophages are terminally differentiated and their contribution to the viral reservoir is mainly dependent on their lifespan, whereas latently infected resting CD4+ memory T cells which are also long lived cells, contribute to the viral reservoir through homeostatic or antigen specific proliferation. However, recent reports have clearly demonstrated that macrophages can be infected in vivo and contribute to the viral reservoir (Figure 1; Micci et al., 2014; Clayton et al., 2017; DiNapoli et al., 2017). In SHIV infected rhesus macaques, in vivo viral replication was sustained by tissue macrophages upon CD4 T cell depletion (Igarashi et al., 2001). Moreover, HIV-1 persistence in macrophages was confirmed in HIV-1 infected humanized myeloid only mice in which viral rebound was observed in 33% of the animals following treatment interruption (Honeycutt et al., 2017).
Macrophages in HIV-1 Related Comorbidities
Monocytes and macrophages play a crucial role in several HIV-1 related comorbidities including neurological disorders, atherosclerosis and cardiovascular disease (CVD). HIV-1 associated neurocognitive disorders (HAND) describe a broad range of neurocognitive disorders from asymptomatic neurocognitive impairment, mild neurocognitive disorders (MND), and HIV-1 associated dementia (HAD). Although the use of ART has dramatically reduced development of HAD, milder forms of HAND are still highly prevalent amongst effectively treated HIV-1 infected individuals (Robertson et al., 2007; Heaton et al., 2010; Simioni et al., 2010). In HIV-1 infection, activated peripheral blood monocytes have increased migratory ability (Ancuta et al., 2004; Williams et al., 2013, 2014) and can cross the BBB, and therefore it is generally believed that HIV-1 infected peripheral monocytes are the main source of HIV-1 infection in the brain. Increased monocyte activation, expansion of the activated monocyte population as well as the level of HIV-1 DNA positive monocytes (CD14+/CD16+) have been associated with HIV-1 associated neuroinflammation and HAND (Pulliam et al., 1997, 2004; Shiramizu et al., 2005; Williams et al., 2014; Booiman et al., 2017). The ongoing neuroinflammation caused by monocyte activation and migration, is considered a strong mediator of neuronal damage in HAND. Moreover, persistent HIV-1 replication in resident macrophages and microglial cells of the brain, contributes to the ongoing neuroinflammation and neuronal damage. In particular the viral envelope protein gp120 (Giulian et al., 1993; Meucci et al., 1998; Kaul and Lipton, 1999; Ohagen et al., 1999), the HIV-1 accessory protein Vpr (Piller et al., 1998; Patel et al., 2000) and the HIV-1 transcriptional activator Tat (Liu et al., 2000; Park et al., 2001; Andras et al., 2003; Song et al., 2003) have been identified to contribute directly or indirectly to neurotoxicity.
HIV-1 associated inflammation and immune activation, both risk factors in the onset of atherosclerosis and CVD, likely contribute to the increased risk of CVD in the HIV-1 infected population (Nou et al., 2016; Triant and Grinspoon, 2017). In early stages of atherosclerosis development, hyperlipidemia [(cholesterol, triglyceride, and low-density lipoprotein (LDL)] promotes the retention and uptake of LDL in the sub-endothelial space (Fogelstrand and Boren, 2012), which results in activation of endothelial cells and smooth muscle cells by the upregulation of adhesion molecules [intercellular adhesion molecule 1 (ICAM-1) and vascular cell adhesion protein 1 (VCAM-1)] and secretion of chemokines [MCP-1 and macrophage colony-stimulating factor (M-CSF)] in the arterial wall (Frostegard et al., 1991). Cells of the immune system expressing the MCP-1 receptor CCR2, are recruited and can infiltrate the lesion (Frostegard et al., 1991). During HIV-1 infection, circulating activated monocytes express increased levels of CCR2, and have an increased ability to migrate in response to MCP1 into the arterial wall (Ancuta et al., 2004; Williams et al., 2013, 2014). These infiltrating monocytes further increase arterial inflammation by cytokine production [interleukin 1ß (IL-1ß) and IL-6] and uptake of (modified) lipoproteins, thereby accelerating atherosclerosis and CVD progression (Hansson, 2005). Indeed, it has been shown that aortic inflammation as measured by 18fluorodeoxyglucose uptake (18F-fluorodexoyglucose Positron Emission Tomography – Computed Tomography: 18F-FDG PET-CT) was increased in effectively treated HIV-1 infected patients to a degree resembling that of uninfected individuals with known CVD. Furthermore, 18F-FDG PET-CT activity was associated with serum levels of soluble CD163 (sCD163), a marker of monocyte/macrophage activation (Subramanian et al., 2012; Tawakol et al., 2017), clearly indicating a role of these cells in accelerated onset of atherosclerosis and CVD in HIV-1 infection.
Mechanisms of HIV-1 Latency
Macrophage Differentiation and Polarization
Human Immunodeficiency Virus type 1 infection in macrophages and their role in the viral reservoir are difficult to study in vivo and therefore in vitro culture models have been developed. In these models, monocytes are isolated from peripheral blood and differentiated in an in vitro culture system toward monocyte derived macrophages. In addition, different stimuli or cytokines can be added to the cultures in order to obtain phenotypically and functionally distinct macrophages. An advantage of this in vitro culture system is that large number of cells are available for analysis and macrophage activation, differentiation, polarization and function can easily be directed. A major disadvantage is that conditions in the culture system are not representative of the local environment in the different tissues, as tissue resident macrophages continuously respond to local signals influencing their function and phenotype resulting in a heterogeneous resident macrophage population. Recently, macrophages isolated from tissues of HIV-1 infected individuals have provided a better insight in the in vivo relevance and the state of the viral reservoir (latent, activated) (Zalar et al., 2010; Yukl et al., 2014; Cribbs et al., 2015; Kandathil et al., 2018; Ganor et al., 2019; Ko et al., 2019). However, these materials are difficult to obtain and invasive for the patient.
In vitro, monocytes become highly susceptible to HIV-1 infection upon differentiation into macrophages (Rich et al., 1992; Schuitemaker et al., 1992; Sonza et al., 1996; Peng et al., 2007; Wang et al., 2009; Cobos Jimenez et al., 2012). Macrophages respond to different stimuli in their environment which will shape their physiology and function. Interferon-γ (IFN-γ) and tumor necrosis factor-α (TNF-α) polarize macrophages into an M1 or pro-inflammatory phenotype (Mosser, 2003). M2 or alternatively activated macrophages are generated upon exposure to IL-4 or IL-13 (M2a), immunoglobulin G (IgG) immune complexes and toll-like receptor (TLR) agonists (M2b) or IL-10 or glucocorticoids (M2c) (Mosser, 2003; Mantovani et al., 2004; Mosser and Edwards, 2008; Martinez et al., 2009). Polarization toward a M2a phenotype results in resistance to HIV-1 infection due to restrictions at the level of reverse transcription (Schuitemaker et al., 1992; Montaner et al., 1997; Wang et al., 1998; Cobos Jimenez et al., 2012), whereas polarization toward M1 or M2c inhibits viral replication at a transcriptional or post-transcriptional level (Kootstra et al., 1994; Montaner et al., 1994; Naif et al., 1996; Perez-Bercoff et al., 2003; David et al., 2006; Cassol et al., 2009, 2010; Cobos Jimenez et al., 2012). This data suggests that polarizing signals can induce viral latency in macrophages at both a pre- or post-integration level (Table 1).
Mechanisms of Pre-integration Latency
Integration of HIV-1 proviral DNA into the host genome is essential for viral replication. Upon entry into the cell, HIV-1 RNA is reverse transcribed, and subsequently the pre-integration complex (PIC), consisting of viral proteins (integrase, matrix, Vpr, capsid) and double stranded DNA (dsDNA) is assembled (Greene and Peterlin, 2002). The PIC is transported into the nucleus and where the proviral DNA integrates into the host cell genome. Pre-integration latency results when the viral replication cycle is partially or completely blocked at steps prior to integration (Table 1). Pre-integration latency is frequently observed in CD4+ T cells but was assumed not to be clinically relevant, because in vitro studies demonstrated that the unintegrated proviral DNA only persists for 1 day in these cells (Pierson et al., 2002; Zhou et al., 2005). However, in patients treated with ART, including an integrase inhibitor, unintegrated HIV-1 DNA subspecies where shown to persist in CD4+ T cells and can potentially integrate when integrase inhibitor medication is discontinued (Murray et al., 2014). Moreover, metabolically active macrophages can contain large quantities of unintegrated viral DNA, which remains stable and biologically active in non-dividing macrophages for up to 2 months (Gillim-Ross et al., 2005; Kelly et al., 2008). Therefore, unintegrated HIV-1 DNA in macrophages may contribute to the viral reservoir in HIV-1 pathogenesis. Indeed, some studies have reported detection of unintegrated HIV-1 DNA in macrophages from patients, mostly in the brain (Pang et al., 1990; Teo et al., 1997). In monocytes/macrophages pre-integration latency could be a consequence of poor reverse transcription efficiency due to a reduced deoxynucleotide triphosphates (dNTP) pool or inhibition of nuclear transport of the PIC as a consequence of low adenosine triphosphate (ATP) levels (Bukrinsky et al., 1992; Kootstra et al., 2000; Kennedy et al., 2010; Lahouassa et al., 2012). Several cellular factors have been implicated to play a role in the observed restriction in monocytes/macrophages: apolipoprotein B mRNA editing enzyme catalytic subunit 3G (APOBEC3G) and subunit 3A (APOBEC3A), a cellular deoxycytidine deaminases which induces G to A hypermutation and degradation of the HIV-1 genome (Peng et al., 2007; Miyagi et al., 2008; Berger G. et al., 2011); Sterile α-motif/histidine-aspartate domain-containing protein 1 (SAMHD1) which hydrolyses dNTPs into their precursors (nucleosides and triphosphates), in turn reducing the dNTPs pool and thereby limiting the reverse transcriptase activity (Berger A. et al., 2011; Hrecka et al., 2011; Laguette et al., 2011; Lahouassa et al., 2012); Myxovirus-resistance protein 2 (MX2) which inhibits HIV-1 replication post entry by hindering the nuclear accumulation and integration of proviral DNA into host chromatin (Goujon et al., 2013; Kane et al., 2013; Wang et al., 2017; Buffone et al., 2019); and members of the RNA polymerase II-associated factor 1 (PAF1) family, which are expressed in monocytes/macrophages and repress HIV-1 reverse transcription and proviral DNA integration (Liu et al., 2011; Tyagi and Kashanchi, 2012).
Mechanisms of Post-integration Latency
Post-integration latency, which is established after integration of the HIV-1 proviral DNA into the host chromatin, is in contrast to pre-integration latency very stable and only limited by the lifespan of the infected cell. Mechanisms underlying HIV-1 latency in vivo are incompletely understood, however, it is a multifactorial phenomenon. Post-integration viral latency may be maintained at a transcriptional or a post-transcriptional level inhibiting HIV-1 protein production and virus formation. Several mechanisms acting at transcriptional and post-transcriptional level inducing HIV-1 latency in CD4+ T cells are well described. However, it is unknown whether these mechanism are also effective in cells of the monocyte/macrophage lineage. Nevertheless, several mechanisms inducing HIV-1 post-integration latency have been described in monocytes/macrophages, including chromatin environment, the absence of transcriptional activation, presence of transcriptional repressors and host antiviral processes. However, not all of these mechanisms are specific for post-integration latency in monocytes/macrophages (Table 1).
Site of Integration and Chromatin Remodeling
The host chromatin is organized into heterochromatin which is densely packed and transcriptionally silent and euchromatin which is loosely packed and transcriptionally active (Demeret et al., 2001). After nuclear import a transcriptional co-activator, lens epithelium-derived growth factor (LEDGF)/p75, which interacts with HIV-1 integrase (Vandegraaff et al., 2006; Meehan et al., 2009), targets the PIC mainly to intronic regions of actively transcribed genes (Schroder et al., 2002; Lewinski et al., 2006). The majority of silent HIV-1 provirus is integrated into the euchromatin, which seems paradoxical considering the fact that euchromatin is transcriptionally active (Han et al., 2004). Different mechanisms of transcriptional interference (e.g., steric hindrance, promotor occlusion and enhancer trapping) have been suggested as possible explanation for integrated proviral DNA expression suppression (Lassen et al., 2004; Bisgrove et al., 2005; Taube and Peterlin, 2013). Most of the data on HIV-1 integration and latency has been derived from CD4+ T cells. However, the integration of HIV-1 proviruses has also been studied in primary macrophages. Barr et al., for instance, studied HIV-1 DNA integration sites in primary macrophages by sequencing 754 unique integration sites and found that, similar to CD4+ T cells, HIV-1 also integrates preferentially into the transcriptionally active regions in macrophages (Mack et al., 2003; Barr et al., 2006; Wellensiek et al., 2009). In CD4+ T cells transcription gene silencing is the most favored mechanism for the establishment and maintenance of HIV-1 latency, whether this is also the case in monocytes/macrophages still has to be determined. However, it is well established that chromatin organization and reorganization influences the gene expression and that HIV-1 proviral DNA follows the same rules that apply to host genes. The role of histone H3 lysine 9 trimethylation (H3K9me3) in heterochromatin formation and the transcriptional silencing of integrated HIV-1 has been described (Grewal and Moazed, 2003; Stewart et al., 2005; du Chene et al., 2007; Marban et al., 2007). In addition, there are two nucleosomes, nuc-0 and nuc-1, that interact with the HIV-1 promoter irrespective of the HIV-1 integration site in the host genome. HIV-1 gene transcription from proviral DNA is only possible with the displacement of nuc-1 (Verdin et al., 1993). These studies were performed in the monocytic U1 cell line and collectively suggest that chromatin remodeling is an essential mechanism of HIV-1 latency establishment and regulation in monocytes/macrophages, however, these observations have not yet been confirmed in primary monocytes/macrophages.
Transcriptional Regulation
The integrated HIV-1 proviral DNA is flanked by the long terminal repeats (LTR). The 5′LTR has binding sites for several transcription factors including specificity protein 1 (Sp1), activator protein 1 (AP1), nuclear factor of activated T-cells (NFAT), nuclear factor-κB (NF-κB), c-myc, chicken ovalbumin upstream promoter (COUP), upstream stimulatory factor (USF), CCAAT box transcription factor/nuclear factor 1 (CTF/NF1), T cell factor 1α (TCF-1α) and the glucocorticoid receptor (Verdin et al., 1993). These transcription factors act together to regulate HIV-1 proviral DNA expression (Table 1). Sp1, for instance, recruits c-myc to the 5′LTR of proviral DNA, which in turn recruits histone deacetylase 1 (HDAC1). HDAC1 then induces chromatin remodeling, which results in the suppression of HIV-1 gene expression (Jiang et al., 2007; Gray et al., 2016). In addition, HDACs were also shown to be recruited to the proviral promoter by COUP transcription factor interacting protein 2 (CTIP2), Ying Yang 1 (YY1), C-promoter binding factor-1 (CBF-1) and Late SV40 Factor (LSF), thereby promoting the establishment of latency (Coull et al., 2000; He and Margolis, 2002; Tyagi and Karn, 2007; Buescher et al., 2009; Le Douce et al., 2010). Similar results were observed in microglial cells, where CTIP2 has been shown to recruit both HDAC1 and HDAC2 to the 5′LTR of HIV-1 proviral DNA (Marban et al., 2007). Moreover, CTIP2 also interacts with suppressor of variegation 3–9 homolog 1 (SUV39H1), a methyl transferase for H3K9me3 which subsequently promotes the recruitment of heterochromatin protein 1 (HP1) leading to local heterochromatization and induction of viral latency (Rohr et al., 2003; Marban et al., 2005; du Chene et al., 2007). The role of CTIP2 was further confirmed by Desplats et al. (2013) who analyzed the expression of CTIP2 in postmortem brain tissue of HIV-1 infected patients with HIV-1 encephalitis (HIV-1 DNA and RNA positive) and HIV-1 positive patients with latent HIV-1 (HIV-1 DNA positive and HIV-1 RNA negative) and no detectable HIV-1 (HIV-1 DNA negative) in the CNS. Higher amounts of CTIP2 were observed in the HIV-1 patients with latent HIV-1 in the CNS as compared to the other groups. Notably, CTIP2 was detected in microglial cells of patients with latent HIV-1, indicating that CTIP2 may play an important role in the regulation of viral latency in microglial cells (Desplats et al., 2013). Furthermore, lysine-specific demethylase 1 (LSD1) was found to regulate HIV-1 gene expression in a synergistic way with CTIP2 in microglial cells (Le Douce et al., 2012). LSD1 also assists in the recruitment of CTIP2 and human SET1/WD40-repeat protein 5 (hSET1/WDR5) at the Sp-1 binding sites of the HIV-1 proximal promoter, resulting in increased H3K4 trimethylation (H3K4me3), which in turn represses viral gene expression (Le Douce et al., 2012). This property of LSD1 seems to be highly specific for cells of the monocyte/macrophage lineage.
Recently, the host factor dual specificity tyrosine-phosphorylation-regulated kinase 1A (DYRK1A) was shown to control HIV-1 replication by regulating HIV-1 provirus transcription in macrophages and CD4+ T cells (Bol et al., 2011; Booiman et al., 2015). DYRK1A was shown to regulate HIV-1 transcription via phosphorylation of the NFAT, thereby promoting NFAT translocation from the nucleus to the cytoplasm resulting in decreased viral transcription and latency. Chemical inhibition of DYRK1A resulted in an increased NFAT binding to the viral LTR and reactivating latent provirus (Bol et al., 2011; Booiman et al., 2015).
Furthermore, it has been reported the 5′LTR of the HIV-1 provirus contains multiple T cell factor 4 (TCF-4) binding sites. TCF-4 interacts with β-catenin and the scaffold matrix attachment region-binding protein 1 (SMAR1) at the 5′LTR and this complex has been shown to repress HIV-1 proviral gene expression in different cell types including lymphocytes and astrocytes (Kumar et al., 2008; Henderson et al., 2012). β-catenin/TCF4 signaling has also been demonstrated to regulate HIV-1 replication in cells of the monocyte/macrophage lineage (Aljawai et al., 2014), which makes it plausible that these proteins also play a role in viral latency in these cells (Kumar et al., 2008).
Apart from cellular factors, HIV-1 latency is also influenced by viral factors. HIV-1 replication requires the viral trans-activator protein Tat for efficient transcription and virus production. In the absence of Tat, only low level of HIV-1 transcription of mostly short abortive transcripts is observed (Karn, 2011). These short RNA transcripts contain a trans-activation response (TAR) element which is the binding site for the Tat protein (Berkhout et al., 1989). The positive transcription elongation factor (P-TEFb) is a critical cellular cofactor of Tat, that favors the generation of complete transcripts from HIV-1 proviral DNA (Price, 2000; Karn, 2011). P-TEFb is composed of a catalytic subunit, cyclin-dependent kinase 9 (CDK9), and a regulatory subunit, cyclin T1 (CycT1) (Herrmann et al., 1998; Rice and Herrmann, 2003; Bres et al., 2008). Tat binds to the TAR region of the RNA transcript and directs P-TEFb to the RNA polymerase II resulting in transcription of full-length HIV-1 RNA (Berkhout et al., 1989; Karn, 2011; Cherrier et al., 2013). The CDK2 can phosphorylate Ser90 on CDK9, thereby assisting in HIV-1 transcription (Ammosova et al., 2005; Breuer et al., 2012). Monocytes express very low levels of CycT1, which transiently increases during differentiation into macrophages. In contrast CDK9 expression levels remain constant in monocytes and macrophages (Liou et al., 2002). The low levels of CycT1 in monocytes result in low functional levels of P-TEFb, resulting in low HIV-1 transcription and thus viral latency in monocytes (Liou et al., 2002).
Post-transcriptional Regulation
Human Immunodeficiency Virus type 1 latency can also be regulated at a post-transcriptional level (Table 1). miRNAs are small single stranded non-coding RNAs that can regulate host gene expression at a post-transcriptional level. miRNAs have also been reported to influence HIV-1 gene expression (Huang et al., 2007; Sung and Rice, 2009; Haasnoot and Berkhout, 2011; Narayanan et al., 2011; Lodge et al., 2017). For instance, the miRNA cluster miR-17/92 is actively inhibited by HIV-1 to support efficient replication in different cell types including lymphocytes, monocytes and macrophages (Triboulet et al., 2007). Furthermore, miR-28; miR-150; miR-223; and miR-382 have been demonstrated to target HIV-1 and play a role in HIV-1 susceptibility of monocytes and macrophages. Inhibition of these miRNAs in monocytes results in increased HIV-1 replication, while increased expression of these miRNAs in macrophages decrease of HIV-1 replication (Huang et al., 2007; Wang et al., 2009). These data indicate that miRNAs can play an important role in viral latency in macrophages.
RNA binding host proteins like GTPase-activating protein-(SH3 domain)-binding protein 1 (G3BP1) and the matrix-associated RNA binding protein Matrin 3 (MATR3), have been shown to play a role in the post-transcriptional regulation of HIV-1 latency. G3BP1 is a single-strand-specific endonuclease that binds mRNA transcripts at the 3′UTR inside stress granules to control mRNA translation during cellular stress (Tourriere et al., 2001; Ortega et al., 2010). G3BP1 was shown to interact with HIV-1 mRNA transcripts thus preventing viral protein translation and restricting HIV-1 replication in T cells and macrophages (Cobos Jimenez et al., 2015). Resting T cells and IFNγ and TNFα polarized macrophages, express high levels of G3BP1 indicating a role of this host factor in HIV-1 latency (Cobos Jimenez et al., 2015). MATR3 is a nuclear matrix protein that has the ability to bind DNA and RNA, and is involved in the regulation of gene expression and controls mRNA export from the nucleus. MATR3 also plays an essential role in Rev-mediated export of HIV-1 mRNA transcript from the nucleus (Kula et al., 2011; Yedavalli and Jeang, 2011), through the interaction with the polypyrimidine tract binding protein and associated splicing factor (PSF) and the viral protein Rev (Zolotukhin et al., 2003). Depletion of MATR3 was demonstrated to inhibit nuclear export of Rev responsive element (RRE)-containing viral RNAs resulting in decreased HIV-1 replication (Sarracino et al., 2018). Resting T cells only express low levels of MATR3 which increases upon activation, indicating a role of this protein in the establishment of latency in resting cells (Sarracino et al., 2018). Since MATR3 is also expressed in monocytes (Ancuta et al., 2009), it can be expected that it is also involved in the posttranscriptional regulation of HIV-1 in these cells.
Eradication Strategies Targeting the Transcriptionally Latent Reservoir
Even though ART has significantly increased life expectancy of HIV-1 infected individuals, complete eradication of the virus is not achievable without targeting the HIV-1 latent reservoirs. Currently, several therapeutic strategies are explored and early findings suggest that different strategies may be required to eliminate the different cellular viral reservoirs: memory CD4+ T cells which importantly maintain the viral reservoir through homeostatic- or antigen specific proliferation, and long lived cells like macrophages residing in the tissue.
Latency Reversal
The latent viral reservoir is not recognized by the immune system and therefore these cells are not eliminated. The “shock and kill” strategy aims to induce viral protein production by reactivation of the latent HIV-1 provirus to prompt elimination of the infected cells through recognition by the immune system or cell death as a result of viral replication (Matalon et al., 2011; Badley et al., 2013). The “shock and kill” strategy has been investigated primarily using CD4+ T cells and T cell lines. Histone deacetylases (HDACs), for instance, are important for suppression of HIV-1 proviral DNA expression, and several HDAC inhibitors including SAHA, vorinostat, oxamflatin, metacept-1, and metacept-3 (Shehu-Xhilaga et al., 2009; Badley et al., 2013) have been studied for their ability to reactivate and eliminate latently infected cells. Although HDAC inhibitors have shown activity to reactivate HIV-1 from latent reservoirs in patients on ART, no decline in HIV-1 proviral DNA in CD4+ T cells was observed (Archin et al., 2009a, b, 2012, 2017). In contrast, in vitro studies in both the monocytic U1 cell line and monocyte-derived macrophages show that HDAC inhibitors not only reactivate and decrease HIV-1 release, but also degrades the viral particles through the canonical autophagy pathway (Shehu-Xhilaga et al., 2009; Rasmussen et al., 2013; Campbell et al., 2015). Moreover, several other compounds targeting different pathways have also been shown to effectively reactivate latent HIV-1, these include protein kinase C agonists (in vitro in both monocytic and T cell lines) (e.g., prostratin and ingenols) (Gulakowski et al., 1997; Korin and Zack, 1999; Warrilow et al., 2006; Colin and Van Lint, 2009; Abreu et al., 2014; Jiang et al., 2014, 2015; Darcis et al., 2015), histone methyltransferases inhibitors (in vitro in T cell lines and primary monocytes/macrophages and CD4+ T cells from HIV-1 infected ART treated patients) (Greiner et al., 2005; Miranda et al., 2009; Bernhard et al., 2011; Bouchat et al., 2012), anti-miRNA inhibitors (Zhang, 2009), NF-κB activators (Fernandez et al., 2013; Kumar et al., 2013), and cytokine therapy in vitro and in vivo in CD4+ T cells as well as in cells of the monocyte/macrophage lineage (Chun et al., 1999; Scripture-Adams et al., 2002; Oguariri et al., 2007). Although the efficacy of the majority of these latency reversing agents (LRAs) has been demonstrated, a major hurdle in this treatment approach is that HIV-1 reactivation only occurs in a small subset of the latently infected cells, which might be overcome at least in part by the use of combinations of these LRAs. Furthermore, targeting of cellular factors and pathways identified to be involved in the regulation of HIV-1 latency at a transcription or post-transcription level, such as DYRK1A, β-catenin/TCF4 and Tat/pTEFb (Kumar et al., 2008; Aljawai et al., 2014; Booiman et al., 2015; Wu et al., 2017; Abner et al., 2018; Table 1) may improve the efficacy of latency reversal. Moreover, LRAs alone are mostly not sufficient to induce cell death and therefore an effective immune response is essential for the elimination of the reactivated cells and this is lacking in the majority of HIV-1 infected patients. Furthermore, HIV-1 infected macrophages can reside in immune privileged compartments like the CNS and can therefore not be reached by cytotoxic T cells (Shan et al., 2012; Badley et al., 2013).
Apoptosis Inducing Agents
Recently more research is done into the “Prime, Shock, and Kill” method, where latent reservoirs are made sensitive to apoptosis (Prime), followed by reactivation of HIV-1 (Shock) and subsequently leading to cell death of the infected cell (Kill) (Badley et al., 2013). HIV-1 infection of CD4+ T cells and macrophages have been shown to induce an anti-apoptotic gene profile and resistance to apoptosis (Olivares et al., 2009). Several viral proteins have been associated with the resistance to apoptosis (Tanaka et al., 1999; Fernandez Larrosa et al., 2008): Vpr upregulates expression of anti-apoptotic proteins like B-cell lymphoma 2 (Bcl-2) in T cells only, thereby promoting cell survival (Conti et al., 1998). In monocytes/macrophages vpr facilitates HIV-1 replication (Connor et al., 1995). Tat, however, does upregulate Bcl-2 and X-linked inhibitor of apoptosis protein (XIAP) in both monocytes/macrophages and CD4+ T cells (Zhang et al., 2002; Lopez-Huertas et al., 2013); Nef upregulates the anti-apoptotic protein B-cell lymphoma-extra large (Bcl-Xl) in macrophages (Choi and Smithgall, 2004), while nef inactivates the Bcl-2-associated death promoter protein (BAD), a pro-apoptotic Bcl-2 family member, in a phosphoinositide 3-kinase (PI3K)– and p21-activated kinase (PAK)-dependent manner in T cells (Wolf et al., 2001); Furthermore, gp120 has been demonstrated to increase in expression of M-CSF in HIV-1 infected macrophages resulting in the upregulation of anti-apoptotic proteins [e.g., induced myeloid leukemia cell differentiation protein 1 (Mcl-1) and Bcl-2-related protein A1 (Bfl-1)] (Swingler et al., 2007). Therefore, targeting of the HIV-1 induced anti-apoptotic pathways during proviral reactivation strategies may promote cell death of the latently infected cells. Multiple agents targeting the anti-apoptotic pathways including PI3K/Protein kinase B (AKT) inhibitors, Bcl2 inhibitors and second mitochondria-derived activator of caspase (SMAC) mimetics/XIAP inhibitors, are explored for their ability to clear latently infected cells (Busca et al., 2012; Badley et al., 2013; Kim et al., 2018). SMAC mimetics/XIAP inhibitors, for instance, function by sensitizing macrophages to Vpr-induced cell death (Busca et al., 2012). In addition, SMAC mimetics/XIAP inhibitors can trigger transcription of latent proviruses via the non-canonical NF-κB pathway. Furthermore, in vitro studies showed that combinations of HDAC inhibitors and SMAC/XIAP mimetics have synergistic activities in T cells (Pache et al., 2015). However, all these compounds do not specifically target the viral reservoir, and targeting of non-HIV-1 infected bystander cells will likely occur especially in ART-treated individuals with increased levels of immune activation, inflammation, and high prevalence of coinfections.
Indeed, an apoptosis resistant gene expression signature was observed in monocytes from HIV-1 infected individuals (Giri et al., 2009). The circulating monocyte population is largely uninfected, which indicates that the anti-apoptotic expression profile may not be due to direct effects of viral infection. Moreover, in vitro infection of monocytes/macrophages demonstrated that HIV-1 infection, as well as exposure to HIV-1 or immune modulators increased apoptosis resistance (Giri et al., 2009).
Concluding Remarks
Despite effective treatment, HIV-1 infected patients are not cured because the virus is not eradicated and latently infected cells persist. The latent viral reservoir mainly resides in resting CD4+ T cells, however, latent HIV-1 proviruses have also been detected in cells of the monocyte/macrophage lineage. HIV-1 infected macrophages can be found in virtually every tissue including the CNS. Viral latency in cells of the monocyte/macrophage lineage can be regulated by diverse molecular mechanisms either at a transcriptional or post-transcriptional level and this is most likely dependent on the activation and differentiation state of these cells. Current eradication strategies rely on reactivation of the latent virus and subsequent kill of the infected cell. The majority of LRAs under study, have been shown to reactivate only small proportions of the latent HIV-1 reservoir, and therefore there is an urgent need for the development of new compounds that target different mechanisms of viral latency both in CD4+ T cells and monocytes/macrophages. Another hurdle is the lack of kill of latently infected cells upon reactivation, which is mainly due to an inefficient antiviral immune response and HIV-1 induced resistance to apoptosis. Apoptosis inducing compounds have been developed for cancer therapy, and their efficacy to specifically induce apoptosis in HIV-1 infected cells is currently under study.
Cure research has mainly focused on CD4+ T cells, because these cells contribute to maintenance pf the viral reservoir through their long lifespan as well as their ability to proliferate under homeostatic conditions or in response to antigens. In contrast, macrophages are terminally differentiated and their contribution to the viral reservoir is mainly dependent on their lifespan and distribution to immune privileged tissues like the CNS. Although, these terminally differentiated latently infected macrophages cannot proliferate, they could be able to spread HIV-1 infection to CD4+ T cells upon reactivation of the virus by LRAs. Therefore, development of eradication strategies combining both novel LRAs and apoptosis inducing agents, targeting either specific cell types or ideally target both latently infected CD4+ T cells and monocytes/macrophages are needed.
Author Contributions
ZK and NK wrote and edited the review.
Conflict of Interest
The authors declare that the research was conducted in the absence of any commercial or financial relationships that could be construed as a potential conflict of interest.
References
Abbas, W., Khan, K. A., Kumar, A., Tripathy, M. K., Dichamp, I., Keita, M., et al. (2014). Blockade of BFA-mediated apoptosis in macrophages by the HIV-1 Nef protein. Cell Death Dis. 5:e1080. doi: 10.1038/cddis.2014.16
Abner, E., Stoszko, M., Zeng, L., Chen, H. C., Izquierdo-Bouldstridge, A., Konuma, T., et al. (2018). A new quinoline BRD4 inhibitor targets a distinct latent HIV-1 reservoir for reactivation from other “Shock” drugs. J. Virol. 92:e2056-17. doi: 10.1128/JVI.02056-17
Abreu, C. M., Price, S. L., Shirk, E. N., Cunha, R. D., Pianowski, L. F., Clements, J. E., et al. (2014). Dual role of novel ingenol derivatives from Euphorbia tirucalli in HIV replication: inhibition of de novo infection and activation of viral LTR. PLoS One 9:e97257. doi: 10.1371/journal.pone.0097257
Aljawai, Y., Richards, M. H., Seaton, M. S., Narasipura, S. D., and Al-Harthi, L. (2014). beta-Catenin/TCF-4 signaling regulates susceptibility of macrophages and resistance of monocytes to HIV-1 productive infection. Curr. HIV Res. 12, 164–173. doi: 10.2174/1570162x12666140526122249
Allen, J. B., McCartney-Francis, N., Smith, P. D., Simon, G., Gartner, S., Wahl, L. M., et al. (1990). Expression of interleukin 2 receptors by monocytes from patients with acquired immunodeficiency syndrome and induction of monocyte interleukin 2 receptors by human immunodeficiency virus in vitro. J. Clin. Invest. 85, 192–199. doi: 10.1172/JCI114412
Ammosova, T., Berro, R., Kashanchi, F., and Nekhai, S. (2005). RNA interference directed to CDK2 inhibits HIV-1 transcription. Virology 341, 171–178. doi: 10.1016/j.virol.2005.06.041
Ancuta, P., Kamat, A., Kunstman, K. J., Kim, E. Y., Autissier, P., Wurcel, A., et al. (2008). Microbial translocation is associated with increased monocyte activation and dementia in AIDS patients. PLoS One 3:e2516. doi: 10.1371/journal.pone.0002516
Ancuta, P., Liu, K. Y., Misra, V., Wacleche, V. S., Gosselin, A., Zhou, X., et al. (2009). Transcriptional profiling reveals developmental relationship and distinct biological functions of CD16+ and CD16- monocyte subsets. BMC Genomics 10:403. doi: 10.1186/1471-2164-10-403
Ancuta, P., Moses, A., and Gabuzda, D. (2004). Transendothelial migration of CD16+ monocytes in response to fractalkine under constitutive and inflammatory conditions. Immunobiology 209, 11–20. doi: 10.1016/j.imbio.2004.04.001
Andras, I. E., Pu, H., Deli, M. A., Nath, A., Hennig, B., and Toborek, M. (2003). HIV-1 Tat protein alters tight junction protein expression and distribution in cultured brain endothelial cells. J. Neurosci. Res. 74, 255–265. doi: 10.1002/jnr.10762
Archin, N. M., Espeseth, A., Parker, D., Cheema, M., Hazuda, D., and Margolis, D. M. (2009a). Expression of latent HIV induced by the potent HDAC inhibitor suberoylanilide hydroxamic acid. AIDS Res. Hum. Retrovirus. 25, 207–212. doi: 10.1089/aid.2008.0191
Archin, N. M., Keedy, K. S., Espeseth, A., Dang, H., Hazuda, D. J., and Margolis, D. M. (2009b). Expression of latent human immunodeficiency type 1 is induced by novel and selective histone deacetylase inhibitors. AIDS 23, 1799–1806. doi: 10.1097/QAD.0b013e32832ec1dc
Archin, N. M., Kirchherr, J. L., Sung, J. A., Clutton, G., Sholtis, K., Xu, Y., et al. (2017). Interval dosing with the HDAC inhibitor vorinostat effectively reverses HIV latency. J. Clin. Invest. 127, 3126–3135. doi: 10.1172/JCI92684
Archin, N. M., Liberty, A. L., Kashuba, A. D., Choudhary, S. K., Kuruc, J. D., Crooks, A. M., et al. (2012). Administration of vorinostat disrupts HIV-1 latency in patients on antiretroviral therapy. Nature 487, 482–485. doi: 10.1038/nature11286
Badley, A. D., Sainski, A., Wightman, F., and Lewin, S. R. (2013). Altering cell death pathways as an approach to cure HIV infection. Cell Death Dis. 4:e718. doi: 10.1038/cddis.2013.248
Bain, C. C., Bravo-Blas, A., Scott, C. L., Perdiguero, E. G., Geissmann, F., Henri, S., et al. (2014). Constant replenishment from circulating monocytes maintains the macrophage pool in the intestine of adult mice. Nat. Immunol. 15, 929–937. doi: 10.1038/ni.2967
Baldauf, H. M., Pan, X., Erikson, E., Schmidt, S., Daddacha, W., Burggraf, M., et al. (2012). SAMHD1 restricts HIV-1 infection in resting CD4(+) T cells. Nat. Med. 18, 1682–1687. doi: 10.1038/nm.2964
Ball, J. K., Holmes, E. C., Whitwell, H., and Desselberger, U. (1994). Genomic variation of human immunodeficiency virus type 1 (HIV-1): molecular analyses of HIV-1 in sequential blood samples and various organs obtained at autopsy. J. Gen. Virol. 75(Pt 4), 67–79. doi: 10.1099/0022-1317-75-4-867
Barr, S. D., Ciuffi, A., Leipzig, J., Shinn, P., Ecker, J. R., and Bushman, F. D. (2006). HIV integration site selection: targeting in macrophages and the effects of different routes of viral entry. Mol. Ther. 14, 218–225. doi: 10.1016/j.ymthe.2006.03.012
Berger, A., Sommer, A. F., Zwarg, J., Hamdorf, M., Welzel, K., Esly, N., et al. (2011). SAMHD1-deficient CD14+ cells from individuals with Aicardi-Goutieres syndrome are highly susceptible to HIV-1 infection. PLoS Pathog. 7:e1002425. doi: 10.1371/journal.ppat.1002425
Berger, G., Durand, S., Fargier, G., Nguyen, X. N., Cordeil, S., Bouaziz, S., et al. (2011). APOBEC3A is a specific inhibitor of the early phases of HIV-1 infection in myeloid cells. PLoS Pathog. 7:e1002221. doi: 10.1371/journal.ppat.1002221
Berkhout, B., Silverman, R. H., and Jeang, K. T. (1989). Tat trans-activates the human immunodeficiency virus through a nascent RNA target. Cell 59, 273–282. doi: 10.1016/0092-8674(89)90289-4
Bernhard, W., Barreto, K., Saunders, A., Dahabieh, M. S., Johnson, P., and Sadowski, I. (2011). The Suv39H1 methyltransferase inhibitor chaetocin causes induction of integrated HIV-1 without producing a T cell response. FEBS Lett. 585, 3549–3554. doi: 10.1016/j.febslet.2011.10.018
Bisgrove, D., Lewinski, M., Bushman, F., and Verdin, E. (2005). Molecular mechanisms of HIV-1 proviral latency. Expert Rev. Anti Infect. Ther. 3, 805–814. doi: 10.1586/14787210.3.5.805
Bol, S. M., Moerland, P. D., Limou, S., van Remmerden, Y., Coulonges, C., van Manen, D., et al. (2011). Genome-wide association study identifies single nucleotide polymorphism in DYRK1A associated with replication of HIV-1 in monocyte-derived macrophages. PLoS One 6:e17190. doi: 10.1371/journal.pone.0017190
Booiman, T., Loukachov, V. V., van Dort, K. A., van’t Wout, A. B., and Kootstra, N. A. (2015). DYRK1A controls HIV-1 replication at a transcriptional level in an NFAT dependent manner. PLoS One 10:e0144229. doi: 10.1371/journal.pone.0144229
Booiman, T., Wit, F. W., Maurer, I., De Francesco, D., Sabin, C. A., Harskamp, A. M., et al. (2017). High cellular monocyte activation in people living with human immunodeficiency virus on combination antiretroviral therapy and lifestyle-matched controls is associated with greater inflammation in Cerebrospinal Fluid. Open Forum Infect. Dis. 4:ofx108. doi: 10.1093/ofid/ofx108
Bouchat, S., Gatot, J. S., Kabeya, K., Cardona, C., Colin, L., Herbein, G., et al. (2012). Histone methyltransferase inhibitors induce HIV-1 recovery in resting CD4(+) T cells from HIV-1-infected HAART-treated patients. AIDS 26, 1473–1482. doi: 10.1097/QAD.0b013e32835535f5
Brenchley, J. M., and Douek, D. C. (2008). The mucosal barrier and immune activation in HIV pathogenesis. Curr. Opin. HIV AIDS 3, 356–361. doi: 10.1097/COH.0b013e3282f9ae9c
Brenchley, J. M., Schacker, T. W., Ruff, L. E., Price, D. A., Taylor, J. H., Beilman, G. J., et al. (2004). CD4+ T cell depletion during all stages of HIV disease occurs predominantly in the gastrointestinal tract. J. Exp. Med. 200, 749–759. doi: 10.1084/jem.20040874
Bres, V., Yoh, S. M., and Jones, K. A. (2008). The multi-tasking P-TEFb complex. Curr. Opin. Cell Biol. 20, 334–340. doi: 10.1016/j.ceb.2008.04.008
Breuer, D., Kotelkin, A., Ammosova, T., Kumari, N., Ivanov, A., Ilatovskiy, A. V., et al. (2012). CDK2 regulates HIV-1 transcription by phosphorylation of CDK9 on serine 90. Retrovirology 9:94. doi: 10.1186/1742-4690-9-94
Buescher, J. L., Martinez, L. B., Sato, S., Okuyama, S., and Ikezu, T. (2009). YY1 and FoxD3 regulate antiretroviral zinc finger protein OTK18 promoter activation induced by HIV-1 infection. J. Neuroimmune Pharmacol. 4, 103–115. doi: 10.1007/s11481-008-9139-x
Buffone, C., Kutzner, J., Opp, S., Martinez-Lopez, A., Selyutina, A., Coggings, S. A., et al. (2019). The ability of SAMHD1 to block HIV-1 but not SIV requires expression of MxB. Virology 531, 260–268. doi: 10.1016/j.virol.2019.03.018
Bukrinsky, M. I., Sharova, N., Dempsey, M. P., Stanwick, T. L., Bukrinskaya, A. G., Haggerty, S., et al. (1992). Active nuclear import of human immunodeficiency virus type 1 preintegration complexes. Proc. Natl. Acad. Sci. U.S.A. 89, 6580–6584. doi: 10.1073/pnas.89.14.6580
Busca, A., Saxena, M., and Kumar, A. (2012). Critical role for antiapoptotic Bcl-xL and Mcl-1 in human macrophage survival and cellular IAP1/2 (cIAP1/2) in resistance to HIV-Vpr-induced apoptosis. J. Biol. Chem. 287, 15118–15133. doi: 10.1074/jbc.M111.312660
Campbell, G. R., Bruckman, R. S., Chu, Y. L., and Spector, S. A. (2015). Autophagy induction by histone deacetylase inhibitors inhibits HIV type 1. J. Biol. Chem. 290, 5028–5040. doi: 10.1074/jbc.M114.605428
Cassol, E., Cassetta, L., Alfano, M., and Poli, G. (2010). Macrophage polarization and HIV-1 infection. J. Leukoc. Biol. 87, 599–608. doi: 10.1189/jlb.1009673
Cassol, E., Cassetta, L., Rizzi, C., Alfano, M., and Poli, G. (2009). M1 and M2a polarization of human monocyte-derived macrophages inhibits HIV-1 replication by distinct mechanisms. J. Immunol. 182, 6237–6246. doi: 10.4049/jimmunol.0803447
Cattin, A., Wiche Salinas, T. R., Gosselin, A., Planas, D., Shacklett, B., Cohen, E. A., et al. (2019). HIV-1 is rarely detected in blood and colon myeloid cells during viral-suppressive antiretroviral therapy. AIDS 33, 1293–1306. doi: 10.1097/QAD.0000000000002195
Cherrier, T., Le Douce, V., Eilebrecht, S., Riclet, R., Marban, C., Dequiedt, F., et al. (2013). CTIP2 is a negative regulator of P-TEFb. Proc. Natl. Acad. Sci. U.S.A. 110, 12655–12660. doi: 10.1073/pnas.1220136110
Choi, H. J., and Smithgall, T. E. (2004). HIV-1 Nef promotes survival of TF-1 macrophages by inducing Bcl-XL expression in an extracellular signal-regulated kinase-dependent manner. J. Biol. Chem. 279, 51688–51696. doi: 10.1074/jbc.M410068200
Chun, T. W., Engel, D., Mizell, S. B., Hallahan, C. W., Fischette, M., Park, S., et al. (1999). Effect of interleukin-2 on the pool of latently infected, resting CD4+ T cells in HIV-1-infected patients receiving highly active anti-retroviral therapy. Nat. Med. 5, 651–655. doi: 10.1038/9498
Chun, T. W., Nickle, D. C., Justement, J. S., Meyers, J. H., Roby, G., Hallahan, C. W., et al. (2008). Persistence of HIV in gut-associated lymphoid tissue despite long-term antiretroviral therapy. J. Infect. Dis. 197, 714–720. doi: 10.1086/527324
Clayton, K. L., Collins, D. R., Lengieza, J., Ghebremichael, M., Dotiwala, F., Lieberman, J., et al. (2018). Resistance of HIV-infected macrophages to CD8(+) T lymphocyte-mediated killing drives activation of the immune system. Nat. Immunol. 19, 475–486. doi: 10.1038/s41590-018-0085-3
Clayton, K. L., Garcia, J. V., Clements, J. E., and Walker, B. D. (2017). HIV infection of macrophages: implications for pathogenesis and cure. Pathog. Immun. 2, 179–192. doi: 10.20411/pai.v2i2.204
Cobos Jimenez, V., Booiman, T., de Taeye, S. W., van Dort, K. A., Rits, M. A., Hamann, J., et al. (2012). Differential expression of HIV-1 interfering factors in monocyte-derived macrophages stimulated with polarizing cytokines or interferons. Sci. Rep. 2:763. doi: 10.1038/srep00763
Cobos Jimenez, V., Martinez, F. O., Booiman, T., van Dort, K. A., van de Klundert, M. A., Gordon, S., et al. (2015). G3BP1 restricts HIV-1 replication in macrophages and T-cells by sequestering viral RNA. Virology 486, 94–104. doi: 10.1016/j.virol.2015.09.007
Colin, L., and Van Lint, C. (2009). Molecular control of HIV-1 postintegration latency: implications for the development of new therapeutic strategies. Retrovirology 6:111. doi: 10.1186/1742-4690-6-111
Connor, R. I., Chen, B. K., Choe, S., and Landau, N. R. (1995). Vpr is required for efficient replication of human immunodeficiency virus type-1 in mononuclear phagocytes. Virology 206, 935–944. doi: 10.1006/viro.1995.1016
Conti, L., Rainaldi, G., Matarrese, P., Varano, B., Rivabene, R., Columba, S., et al. (1998). The HIV-1 vpr protein acts as a negative regulator of apoptosis in a human lymphoblastoid T cell line: possible implications for the pathogenesis of AIDS. J. Exp. Med. 187, 403–413. doi: 10.1084/jem.187.3.403
Cosenza, M. A., Zhao, M. L., Si, Q., and Lee, S. C. (2002). Human brain parenchymal microglia express CD14 and CD45 and are productively infected by HIV-1 in HIV-1 encephalitis. Brain Pathol. 12, 442–455. doi: 10.1111/j.1750-3639.2002.tb00461.x
Coull, J. J., Romerio, F., Sun, J. M., Volker, J. L., Galvin, K. M., Davie, J. R., et al. (2000). The human factors YY1 and LSF repress the human immunodeficiency virus type 1 long terminal repeat via recruitment of histone deacetylase 1. J. Virol. 74, 6790–6799. doi: 10.1128/jvi.74.15.6790-6799.2000
Cribbs, S. K., Lennox, J., Caliendo, A. M., Brown, L. A., and Guidot, D. M. (2015). Healthy HIV-1-infected individuals on highly active antiretroviral therapy harbor HIV-1 in their alveolar macrophages. AIDS Res. Hum. Retroviruses 31, 64–70. doi: 10.1089/AID.2014.0133
Crowe, S. M., and Sonza, S. (2000). HIV-1 can be recovered from a variety of cells including peripheral blood monocytes of patients receiving highly active antiretroviral therapy: a further obstacle to eradication. J. Leukoc. Biol. 68, 345–350.
Darcis, G., Kula, A., Bouchat, S., Fujinaga, K., Corazza, F., Ait-Ammar, A., et al. (2015). An in-depth comparison of latency-reversing agent combinations in various in vitro and ex vivo HIV-1 latency models identified Bryostatin-1+JQ1 and ingenol-B+JQ1 to potently reactivate viral gene expression. PLoS Pathog. 11:e1005063. doi: 10.1371/journal.ppat.1005063
David, A., Saez-Cirion, A., Versmisse, P., Malbec, O., Iannascoli, B., Herschke, F., et al. (2006). The engagement of activating FcgammaRs inhibits primate lentivirus replication in human macrophages. J. Immunol. 177, 6291–6300. doi: 10.4049/jimmunol.177.9.6291
Deeks, S. G. (2011). HIV infection, inflammation, immunosenescence, and aging. Annu. Rev. Med. 62, 141–155. doi: 10.1146/annurev-med-042909-093756
Deleage, C., Moreau, M., Rioux-Leclercq, N., Ruffault, A., Jegou, B., and Dejucq-Rainsford, N. (2011). Human immunodeficiency virus infects human seminal vesicles in vitro and in vivo. Am. J. Pathol. 179, 2397–2408. doi: 10.1016/j.ajpath.2011.08.005
Demeret, C., Vassetzky, Y., and Mechali, M. (2001). Chromatin remodelling and DNA replication: from nucleosomes to loop domains. Oncogene 20, 3086–3093. doi: 10.1038/sj.onc.1204333
Descours, B., Cribier, A., Chable-Bessia, C., Ayinde, D., Rice, G., Crow, Y., et al. (2012). SAMHD1 restricts HIV-1 reverse transcription in quiescent CD4(+) T-cells. Retrovirology 9:87. doi: 10.1186/1742-4690-9-87
Desplats, P., Dumaop, W., Smith, D., Adame, A., Everall, I., Letendre, S., et al. (2013). Molecular and pathologic insights from latent HIV-1 infection in the human brain. Neurology 80, 1415–1423. doi: 10.1212/WNL.0b013e31828c2e9e
DiNapoli, S. R., Ortiz, A. M., Wu, F., Matsuda, K., Twigg, H. L. III, Hirsch, V. M., et al. (2017). Tissue-resident macrophages can contain replication-competent virus in antiretroviral-naive, SIV-infected Asian macaques. JCI Insight 2:e91214. doi: 10.1172/jci.insight.91214
du Chene, I., Basyuk, E., Lin, Y. L., Triboulet, R., Knezevich, A., Chable-Bessia, C., et al. (2007). Suv39H1 and HP1gamma are responsible for chromatin-mediated HIV-1 transcriptional silencing and post-integration latency. EMBO J. 26, 424–435. doi: 10.1038/sj.emboj.7601517
Ellery, P. J., Tippett, E., Chiu, Y. L., Paukovics, G., Cameron, P. U., Solomon, A., et al. (2007). The CD16+ monocyte subset is more permissive to infection and preferentially harbors HIV-1 in vivo. J. Immunol. 178, 6581–6589. doi: 10.4049/jimmunol.178.10.6581
Embretson, J., Zupancic, M., Ribas, J. L., Burke, A., Racz, P., Tenner-Racz, K., et al. (1993). Massive covert infection of helper T lymphocytes and macrophages by HIV during the incubation period of AIDS. Nature 362, 359–362. doi: 10.1038/362359a0
Estes, J. D., Kityo, C., Ssali, F., Swainson, L., Makamdop, K. N., Del Prete, G. Q., et al. (2017). Defining total-body AIDS-virus burden with implications for curative strategies. Nat. Med. 23, 1271–1276. doi: 10.1038/nm.4411
Fernandez, G., Zaikos, T. D., Khan, S. Z., Jacobi, A. M., Behlke, M. A., and Zeichner, S. L. (2013). Targeting IkappaB proteins for HIV latency activation: the role of individual IkappaB and NF-kappaB proteins. J. Virol. 87, 3966–3978. doi: 10.1128/JVI.03251-12
Fernandez Larrosa, P. N., Croci, D. O., Riva, D. A., Bibini, M., Luzzi, R., Saracco, M., et al. (2008). Apoptosis resistance in HIV-1 persistently-infected cells is independent of active viral replication and involves modulation of the apoptotic mitochondrial pathway. Retrovirology 5:19. doi: 10.1186/1742-4690-5-19
Fischer-Smith, T., Croul, S., Sverstiuk, A. E., Capini, C., L’Heureux, D., Regulier, E. G., et al. (2001). CNS invasion by CD14+/CD16+ peripheral blood-derived monocytes in HIV dementia: perivascular accumulation and reservoir of HIV infection. J. Neurovirol. 7, 528–541. doi: 10.1080/135502801753248114
Fogelstrand, P., and Boren, J. (2012). Retention of atherogenic lipoproteins in the artery wall and its role in atherogenesis. Nutr. Metab. Cardiovasc. Dis. 22, 1–7. doi: 10.1016/j.numecd.2011.09.007
Frostegard, J., Haegerstrand, A., Gidlund, M., and Nilsson, J. (1991). Biologically modified LDL increases the adhesive properties of endothelial cells. Atherosclerosis 90, 119–126. doi: 10.1016/0021-9150(91)90106-d
Fulcher, J. A., Hwangbo, Y., Zioni, R., Nickle, D., Lin, X., Heath, L., et al. (2004). Compartmentalization of human immunodeficiency virus type 1 between blood monocytes and CD4+ T cells during infection. J. Virol. 78, 7883–7893. doi: 10.1128/JVI.78.15.7883-7893.2004
Furtado, M. R., Callaway, D. S., Phair, J. P., Kunstman, K. J., Stanton, J. L., Macken, C. A., et al. (1999). Persistence of HIV-1 transcription in peripheral-blood mononuclear cells in patients receiving potent antiretroviral therapy. N. Engl. J. Med. 340, 1614–1622. doi: 10.1056/NEJM199905273402102
Gamrekelashvili, J., Giagnorio, R., Jussofie, J., Soehnlein, O., Duchene, J., Briseno, C. G., et al. (2016). Regulation of monocyte cell fate by blood vessels mediated by Notch signalling. Nat. Commun. 7:12597. doi: 10.1038/ncomms12597
Ganor, Y., Real, F., Sennepin, A., Dutertre, C. A., Prevedel, L., Xu, L., et al. (2019). HIV-1 reservoirs in urethral macrophages of patients under suppressive antiretroviral therapy. Nat. Microbiol. 4, 633–644. doi: 10.1038/s41564-018-0335-z
Ganor, Y., Zhou, Z., Bodo, J., Tudor, D., Leibowitch, J., Mathez, D., et al. (2013). The adult penile urethra is a novel entry site for HIV-1 that preferentially targets resident urethral macrophages. Mucosal. Immunol. 6, 776–786. doi: 10.1038/mi.2012.116
Gillim-Ross, L., Cara, A., and Klotman, M. E. (2005). HIV-1 extrachromosomal 2-LTR circular DNA is long-lived in human macrophages. Viral Immunol. 18, 190–196. doi: 10.1089/vim.2005.18.190
Ginhoux, F., Greter, M., Leboeuf, M., Nandi, S., See, P., Gokhan, S., et al. (2010). Fate mapping analysis reveals that adult microglia derive from primitive macrophages. Science 330, 841–845. doi: 10.1126/science.1194637
Giri, M. S., Nebozyhn, M., Raymond, A., Gekonge, B., Hancock, A., Creer, S., et al. (2009). Circulating monocytes in HIV-1-infected viremic subjects exhibit an antiapoptosis gene signature and virus- and host-mediated apoptosis resistance. J. Immunol. 182, 4459–4470. doi: 10.4049/jimmunol.0801450
Giulian, D., Wendt, E., Vaca, K., and Noonan, C. A. (1993). The envelope glycoprotein of human immunodeficiency virus type 1 stimulates release of neurotoxins from monocytes. Proc. Natl. Acad. Sci. U.S.A. 90, 2769–2773. doi: 10.1073/pnas.90.7.2769
Goujon, C., Moncorge, O., Bauby, H., Doyle, T., Ward, C. C., Schaller, T., et al. (2013). Human MX2 is an interferon-induced post-entry inhibitor of HIV-1 infection. Nature 502, 559–562. doi: 10.1038/nature12542
Gray, L. R., Cowley, D., Welsh, C., Lu, H. K., Brew, B. J., Lewin, S. R., et al. (2016). CNS-specific regulatory elements in brain-derived HIV-1 strains affect responses to latency-reversing agents with implications for cure strategies. Mol. Psychiatry 21, 574–584. doi: 10.1038/mp.2015.111
Greene, W. C., and Peterlin, B. M. (2002). Charting HIV’s remarkable voyage through the cell: Basic science as a passport to future therapy. Nat. Med. 8, 673–680. doi: 10.1038/nm0702-673
Greenhead, P., Hayes, P., Watts, P. S., Laing, K. G., Griffin, G. E., and Shattock, R. J. (2000). Parameters of human immunodeficiency virus infection of human cervical tissue and inhibition by vaginal virucides. J. Virol. 74, 5577–5586. doi: 10.1128/jvi.74.12.5577-5586.2000
Greiner, D., Bonaldi, T., Eskeland, R., Roemer, E., and Imhof, A. (2005). Identification of a specific inhibitor of the histone methyltransferase SU(VAR)3-9. Nat. Chem. Biol. 1, 143–145. doi: 10.1038/nchembio721
Grewal, S. I., and Moazed, D. (2003). Heterochromatin and epigenetic control of gene expression. Science 301, 798–802. doi: 10.1126/science.1086887
Guadalupe, M., Reay, E., Sankaran, S., Prindiville, T., Flamm, J., McNeil, A., et al. (2003). Severe CD4+ T-cell depletion in gut lymphoid tissue during primary human immunodeficiency virus type 1 infection and substantial delay in restoration following highly active antiretroviral therapy. J. Virol. 77, 11708–11717. doi: 10.1128/jvi.77.21.11708-11717.2003
Guadalupe, M., Sankaran, S., George, M. D., Reay, E., Verhoeven, D., Shacklett, B. L., et al. (2006). Viral suppression and immune restoration in the gastrointestinal mucosa of human immunodeficiency virus type 1-infected patients initiating therapy during primary or chronic infection. J. Virol. 80, 8236–8247. doi: 10.1128/JVI.00120-06
Gulakowski, R. J., McMahon, J. B., Buckheit, R. W. Jr., Gustafson, K. R., and Boyd, M. R. (1997). Antireplicative and anticytopathic activities of prostratin, a non-tumor-promoting phorbol ester, against human immunodeficiency virus (HIV). Antiviral Res. 33, 87–97. doi: 10.1016/s0166-3542(96)01004-2
Gupta, P., Collins, K. B., Ratner, D., Watkins, S., Naus, G. J., Landers, D. V., et al. (2002). Memory CD4(+) T cells are the earliest detectable human immunodeficiency virus type 1 (HIV-1)-infected cells in the female genital mucosal tissue during HIV-1 transmission in an organ culture system. J. Virol. 76, 9868–9876. doi: 10.1128/jvi.76.19.9868-9876.2002
Haasnoot, J., and Berkhout, B. (2011). RNAi and cellular miRNAs in infections by mammalian viruses. Methods Mol. Biol. 721, 23–41. doi: 10.1007/978-1-61779-037-9_2
Han, Y., Lassen, K., Monie, D., Sedaghat, A. R., Shimoji, S., Liu, X., et al. (2004). Resting CD4+ T cells from human immunodeficiency virus type 1 (HIV-1)-infected individuals carry integrated HIV-1 genomes within actively transcribed host genes. J. Virol. 78, 6122–6133. doi: 10.1128/JVI.78.12.6122-6133.2004
Hansen, E. C., Ransom, M., Hesselberth, J. R., Hosmane, N. N., Capoferri, A. A., Bruner, K. M., et al. (2016). Diverse fates of uracilated HIV-1 DNA during infection of myeloid lineage cells. eLife 5:e18447. doi: 10.7554/eLife.18447
Hansson, G. K. (2005). Inflammation, atherosclerosis, and coronary artery disease. N. Engl. J. Med. 352, 1685–1695. doi: 10.1056/NEJMra043430
Hashimoto, D., Chow, A., Noizat, C., Teo, P., Beasley, M. B., Leboeuf, M., et al. (2013). Tissue-resident macrophages self-maintain locally throughout adult life with minimal contribution from circulating monocytes. Immunity 38, 792–804. doi: 10.1016/j.immuni.2013.04.004
He, G., and Margolis, D. M. (2002). Counterregulation of chromatin deacetylation and histone deacetylase occupancy at the integrated promoter of human immunodeficiency virus type 1 (HIV-1) by the HIV-1 repressor YY1 and HIV-1 activator Tat. Mol. Cell Biol. 22, 2965–2973. doi: 10.1128/mcb.22.9.2965-2973.2002
Hearps, A. C., Maisa, A., Cheng, W. J., Angelovich, T. A., Lichtfuss, G. F., Palmer, C. S., et al. (2012). HIV infection induces age-related changes to monocytes and innate immune activation in young men that persist despite combination antiretroviral therapy. AIDS 26, 843–853. doi: 10.1097/QAD.0b013e328351f756
Heaton, R. K., Clifford, D. B., Franklin, DR Jr., Woods, S. P., Ake, C., Vaida, F., et al. (2010). HIV-associated neurocognitive disorders persist in the era of potent antiretroviral therapy: CHARTER Study. Neurology 75, 2087–2096. doi: 10.1212/WNL.0b013e318200d727
Heffern, E. F., Ramani, R., Marshall, G., and Kyei, G. B. (2019). Identification of isoform-selective hydroxamic acid derivatives that potently reactivate HIV from latency. J. Virus Erad. 5, 84–91.
Henderson, L. J., Narasipura, S. D., Adarichev, V., Kashanchi, F., and Al-Harthi, L. (2012). Identification of novel T cell factor 4 (TCF-4) binding sites on the HIV long terminal repeat which associate with TCF-4, beta-catenin, and SMAR1 to repress HIV transcription. J. Virol. 86, 9495–9503. doi: 10.1128/JVI.00486-12
Herrmann, C. H., Carroll, R. G., Wei, P., Jones, K. A., and Rice, A. P. (1998). Tat-associated kinase, TAK, activity is regulated by distinct mechanisms in peripheral blood lymphocytes and promonocytic cell lines. J. Virol. 72, 9881–9888.
Hladik, F., Sakchalathorn, P., Ballweber, L., Lentz, G., Fialkow, M., Eschenbach, D., et al. (2007). Initial events in establishing vaginal entry and infection by human immunodeficiency virus type-1. Immunity 26, 257–270. doi: 10.1016/j.immuni.2007.01.007
Ho, D. D., Rota, T. R., and Hirsch, M. S. (1986). Infection of monocyte/macrophages by human T lymphotropic virus type III. J. Clin. Invest. 77, 1712–1715. doi: 10.1172/JCI112491
Hoeffel, G., Chen, J., Lavin, Y., Low, D., Almeida, F. F., See, P., et al. (2015). C-Myb(+) erythro-myeloid progenitor-derived fetal monocytes give rise to adult tissue-resident macrophages. Immunity 42, 665–678. doi: 10.1016/j.immuni.2015.03.011
Honeycutt, J. B., Thayer, W. O., Baker, C. E., Ribeiro, R. M., Lada, S. M., Cao, Y., et al. (2017). HIV persistence in tissue macrophages of humanized myeloid-only mice during antiretroviral therapy. Nat. Med. 23, 638–643. doi: 10.1038/nm.4319
Hrecka, K., Hao, C., Gierszewska, M., Swanson, S. K., Kesik-Brodacka, M., Srivastava, S., et al. (2011). Vpx relieves inhibition of HIV-1 infection of macrophages mediated by the SAMHD1 protein. Nature 474, 658–661. doi: 10.1038/nature10195
Huang, J., Wang, F., Argyris, E., Chen, K., Liang, Z., Tian, H., et al. (2007). Cellular microRNAs contribute to HIV-1 latency in resting primary CD4+ T lymphocytes. Nat. Med. 13, 1241–1247. doi: 10.1038/nm1639
Hufert, F. T., Schmitz, J., Schreiber, M., Schmitz, H., Racz, P., and von Laer, D. D. (1993). Human Kupffer cells infected with HIV-1 in vivo. J. Acquir. Immune Defic. Syndr. 6, 772–777.
Igarashi, T., Brown, C. R., Endo, Y., Buckler-White, A., Plishka, R., Bischofberger, N., et al. (2001). Macrophage are the principal reservoir and sustain high virus loads in rhesus macaques after the depletion of CD4+ T cells by a highly pathogenic simian immunodeficiency virus/HIV type 1 chimera (SHIV): Implications for HIV-1 infections of humans. Proc. Natl. Acad. Sci. U.S.A. 98, 658–663. doi: 10.1073/pnas.021551798
Jambo, K. C., Banda, D. H., Kankwatira, A. M., Sukumar, N., Allain, T. J., Heyderman, R. S., et al. (2014). Small alveolar macrophages are infected preferentially by HIV and exhibit impaired phagocytic function. Mucosal. Immunol. 7, 1116–1126. doi: 10.1038/mi.2013.127
Jaworowski, A., Ellery, P., Maslin, C. L., Naim, E., Heinlein, A. C., Ryan, C. E., et al. (2006). Normal CD16 expression and phagocytosis of Mycobacterium avium complex by monocytes from a current cohort of HIV-1-infected patients. J. Infect. Dis. 193, 693–697. doi: 10.1086/500367
Jaworowski, A., Kamwendo, D. D., Ellery, P., Sonza, S., Mwapasa, V., Tadesse, E., et al. (2007). CD16+ monocyte subset preferentially harbors HIV-1 and is expanded in pregnant Malawian women with Plasmodium falciparum malaria and HIV-1 infection. J. Infect. Dis. 196, 38–42. doi: 10.1086/518443
Jiang, G., Espeseth, A., Hazuda, D. J., and Margolis, D. M. (2007). c-Myc and Sp1 contribute to proviral latency by recruiting histone deacetylase 1 to the human immunodeficiency virus type 1 promoter. J. Virol. 81, 10914–10923. doi: 10.1128/JVI.01208-07
Jiang, G., Mendes, E. A., Kaiser, P., Sankaran-Walters, S., Tang, Y., Weber, M. G., et al. (2014). Reactivation of HIV latency by a newly modified Ingenol derivative via protein kinase Cdelta-NF-kappaB signaling. AIDS 28, 1555–1566. doi: 10.1097/QAD.0000000000000289
Jiang, G., Mendes, E. A., Kaiser, P., Wong, D. P., Tang, Y., Cai, I., et al. (2015). Synergistic Reactivation of Latent HIV Expression by Ingenol-3-Angelate, PEP005, Targeted NF-kB Signaling in Combination with JQ1 Induced p-TEFb Activation. PLoS Pathog. 11:e1005066. doi: 10.1371/journal.ppat.1005066
Josefsson, L., von Stockenstrom, S., Faria, N. R., Sinclair, E., Bacchetti, P., Killian, M., et al. (2013). The HIV-1 reservoir in eight patients on long-term suppressive antiretroviral therapy is stable with few genetic changes over time. Proc. Natl. Acad. Sci. U.S.A. 110, E4987–E4996. doi: 10.1073/pnas.1308313110
Kandathil, A. J., Sugawara, S., Goyal, A., Durand, C. M., Quinn, J., Sachithanandham, J., et al. (2018). No recovery of replication-competent HIV-1 from human liver macrophages. J. Clin. Invest. 128, 4501–4509. doi: 10.1172/JCI121678
Kane, M., Yadav, S. S., Bitzegeio, J., Kutluay, S. B., Zang, T., Wilson, S. J., et al. (2013). MX2 is an interferon-induced inhibitor of HIV-1 infection. Nature 502, 563–566. doi: 10.1038/nature12653
Karn, J. (2011). The molecular biology of HIV latency: breaking and restoring the Tat-dependent transcriptional circuit. Curr. Opin. HIV AIDS 6, 4–11. doi: 10.1097/COH.0b013e328340ffbb
Kaul, M., and Lipton, S. A. (1999). Chemokines and activated macrophages in HIV gp120-induced neuronal apoptosis. Proc. Natl. Acad. Sci. U.S.A. 96, 8212–8216. doi: 10.1073/pnas.96.14.8212
Kelly, J., Beddall, M. H., Yu, D., Iyer, S. R., Marsh, J. W., and Wu, Y. (2008). Human macrophages support persistent transcription from unintegrated HIV-1 DNA. Virology 372, 300–312. doi: 10.1016/j.virol.2007.11.007
Kennedy, E. M., Gavegnano, C., Nguyen, L., Slater, R., Lucas, A., Fromentin, E., et al. (2010). Ribonucleoside triphosphates as substrate of human immunodeficiency virus type 1 reverse transcriptase in human macrophages. J. Biol. Chem. 285, 39380–39391. doi: 10.1074/jbc.M110.178582
Kim, Y., Anderson, J. L., and Lewin, S. R. (2018). Getting the “Kill” into “Shock and Kill”: Strategies to Eliminate Latent HIV. Cell Host Microbe 23, 14–26. doi: 10.1016/j.chom.2017.12.004
Ko, A., Kang, G., Hattler, J. B., Galadima, H. I., Zhang, J., Li, Q., et al. (2019). Macrophages but not astrocytes harbor HIV DNA in the brains of HIV-1-infected aviremic individuals on suppressive antiretroviral therapy. J. Neuroimmune Pharmacol. 14, 110–119. doi: 10.1007/s11481-018-9809-2
Kootstra, N. A., van’t Wout, A., Huisman, H. G., Miedema, F., and Schuitemaker, H. (1994). Interference of interleukin-10 with human immunodeficiency virus type 1 replication in primary monocyte-derived macrophages. J. Virol. 68, 6967–6975.
Kootstra, N. A., Zwart, B. M., and Schuitemaker, H. (2000). Diminished human immunodeficiency virus type 1 reverse transcription and nuclear transport in primary macrophages arrested in early G(1) phase of the cell cycle. J. Virol. 74, 1712–1717. doi: 10.1128/jvi.74.4.1712-1717.2000
Korber, B. T., Kunstman, K. J., Patterson, B. K., Furtado, M., McEvilly, M. M., Levy, R., et al. (1994). Genetic differences between blood- and brain-derived viral sequences from human immunodeficiency virus type 1-infected patients: evidence of conserved elements in the V3 region of the envelope protein of brain-derived sequences. J. Virol. 68, 7467–7481.
Korin, Y. D., and Zack, J. A. (1999). Nonproductive human immunodeficiency virus type 1 infection in nucleoside-treated G0 lymphocytes. J. Virol. 73, 6526–6532.
Kula, A., Guerra, J., Knezevich, A., Kleva, D., Myers, M. P., and Marcello, A. (2011). Characterization of the HIV-1 RNA associated proteome identifies Matrin 3 as a nuclear cofactor of Rev function. Retrovirology 8:60. doi: 10.1186/1742-4690-8-60
Kumar, A., Abbas, W., and Herbein, G. (2013). TNF and TNF receptor superfamily members in HIV infection: new cellular targets for therapy? Mediators Inflamm. 2013:484378. doi: 10.1155/2013/484378
Kumar, A., Zloza, A., Moon, R. T., Watts, J., Tenorio, A. R., and Al-Harthi, L. (2008). Active beta-catenin signaling is an inhibitory pathway for human immunodeficiency virus replication in peripheral blood mononuclear cells. J. Virol. 82, 2813–2820. doi: 10.1128/JVI.02498-07
Lafeuillade, A., Chollet, L., Hittinger, G., Profizi, N., Costes, O., and Poggi, C. (1998). Residual human immunodeficiency virus type 1 RNA in lymphoid tissue of patients with sustained plasma RNA of <200 copies/mL. J. Infect. Dis. 177, 235–238. doi: 10.1086/517362
Laguette, N., Sobhian, B., Casartelli, N., Ringeard, M., Chable-Bessia, C., Segeral, E., et al. (2011). SAMHD1 is the dendritic- and myeloid-cell-specific HIV-1 restriction factor counteracted by Vpx. Nature 474, 654–657. doi: 10.1038/nature10117
Lahouassa, H., Daddacha, W., Hofmann, H., Ayinde, D., Logue, E. C., Dragin, L., et al. (2012). SAMHD1 restricts the replication of human immunodeficiency virus type 1 by depleting the intracellular pool of deoxynucleoside triphosphates. Nat. Immunol. 13, 223–228. doi: 10.1038/ni.2236
Lambotte, O., Chaix, M. L., Gasnault, J., Goujard, C., Lebras, P., Delfraissy, J. F., et al. (2005). Persistence of replication-competent HIV in the central nervous system despite long-term effective highly active antiretroviral therapy. AIDS 19, 217–218. doi: 10.1097/00002030-200501280-00018
Lassen, K., Han, Y., Zhou, Y., Siliciano, J., and Siliciano, R. F. (2004). The multifactorial nature of HIV-1 latency. Trends Mol. Med. 10, 525–531. doi: 10.1016/j.molmed.2004.09.006
Le Douce, V., Colin, L., Redel, L., Cherrier, T., Herbein, G., Aunis, D., et al. (2012). LSD1 cooperates with CTIP2 to promote HIV-1 transcriptional silencing. Nucleic Acids Res. 40, 1904–1915. doi: 10.1093/nar/gkr857
Le Douce, V., Herbein, G., Rohr, O., and Schwartz, C. (2010). Molecular mechanisms of HIV-1 persistence in the monocyte-macrophage lineage. Retrovirology 7:32. doi: 10.1186/1742-4690-7-32
Lewinski, M. K., Yamashita, M., Emerman, M., Ciuffi, A., Marshall, H., Crawford, G., et al. (2006). Retroviral DNA integration: viral and cellular determinants of target-site selection. PLoS Pathog. 2:e60. doi: 10.1371/journal.ppat.0020060
Liou, L. Y., Herrmann, C. H., and Rice, A. P. (2002). Transient induction of cyclin T1 during human macrophage differentiation regulates human immunodeficiency virus type 1 Tat transactivation function. J. Virol. 76, 10579–10587. doi: 10.1128/jvi.76.21.10579-10587.2002
Liu, L., Oliveira, N. M., Cheney, K. M., Pade, C., Dreja, H., Bergin, A. M., et al. (2011). A whole genome screen for HIV restriction factors. Retrovirology 8:94. doi: 10.1186/1742-4690-8-94
Liu, Y., Jones, M., Hingtgen, C. M., Bu, G., Laribee, N., Tanzi, R. E., et al. (2000). Uptake of HIV-1 tat protein mediated by low-density lipoprotein receptor-related protein disrupts the neuronal metabolic balance of the receptor ligands. Nat. Med. 6, 1380–1387. doi: 10.1038/82199
Llewellyn, N., Zioni, R., Zhu, H., Andrus, T., Xu, Y., Corey, L., et al. (2006). Continued evolution of HIV-1 circulating in blood monocytes with antiretroviral therapy: genetic analysis of HIV-1 in monocytes and CD4+ T cells of patients with discontinued therapy. J. Leukoc. Biol. 80, 1118–1126. doi: 10.1189/jlb.0306144
Lodge, R., Ferreira Barbosa, J. A., Lombard-Vadnais, F., Gilmore, J. C., Deshiere, A., Gosselin, A., et al. (2017). Host MicroRNAs-221 and -222 inhibit HIV-1 entry in macrophages by targeting the CD4 viral receptor. Cell Rep. 21, 141–153. doi: 10.1016/j.celrep.2017.09.030
Lopez-Huertas, M. R., Mateos, E., Sanchez Del Cojo, M., Gomez-Esquer, F., Diaz-Gil, G., Rodriguez-Mora, S., et al. (2013). The presence of HIV-1 Tat protein second exon delays fas protein-mediated apoptosis in CD4+ T lymphocytes: a potential mechanism for persistent viral production. J. Biol. Chem. 288, 7626–7644. doi: 10.1074/jbc.M112.408294
Mack, K. D., Jin, X., Yu, S., Wei, R., Kapp, L., Green, C., et al. (2003). HIV insertions within and proximal to host cell genes are a common finding in tissues containing high levels of HIV DNA and macrophage-associated p24 antigen expression. J. Acquir. Immune Defic. Syndr. 33, 308–320. doi: 10.1097/00126334-200307010-00004
Mantovani, A., Sica, A., Sozzani, S., Allavena, P., Vecchi, A., and Locati, M. (2004). The chemokine system in diverse forms of macrophage activation and polarization. Trends Immunol. 25, 677–686. doi: 10.1016/j.it.2004.09.015
Marban, C., Redel, L., Suzanne, S., Van Lint, C., Lecestre, D., Chasserot-Golaz, S., et al. (2005). COUP-TF interacting protein 2 represses the initial phase of HIV-1 gene transcription in human microglial cells. Nucleic Acids Res. 33, 2318–2331. doi: 10.1093/nar/gki529
Marban, C., Suzanne, S., Dequiedt, F., de Walque, S., Redel, L., Van Lint, C., et al. (2007). Recruitment of chromatin-modifying enzymes by CTIP2 promotes HIV-1 transcriptional silencing. EMBO J. 26, 412–423. doi: 10.1038/sj.emboj.7601516
Margolis, D. M., Somasundaran, M., and Green, M. R. (1994). Human transcription factor YY1 represses human immunodeficiency virus type 1 transcription and virion production. J. Virol. 68, 905–910.
Martinez, F. O., Helming, L., and Gordon, S. (2009). Alternative activation of macrophages: an immunologic functional perspective. Annu. Rev. Immunol. 27, 451–483. doi: 10.1146/annurev.immunol.021908.132532
Mass, E., Ballesteros, I., Farlik, M., Halbritter, F., Gunther, P., Crozet, L., et al. (2016). Specification of tissue-resident macrophages during organogenesis. Science 353:aaf4238. doi: 10.1126/science.aaf4238
Matalon, S., Rasmussen, T. A., and Dinarello, C. A. (2011). Histone deacetylase inhibitors for purging HIV-1 from the latent reservoir. Mol. Med. 17, 466–472. doi: 10.2119/molmed.2011.00076
Mayer, K. H., Boswell, S., Goldstein, R., Lo, W., Xu, C., Tucker, L., et al. (1999). Persistence of human immunodeficiency virus in semen after adding indinavir to combination antiretroviral therapy. Clin. Infect. Dis. 28, 1252–1259. doi: 10.1086/514775
Meehan, A. M., Saenz, D. T., Morrison, J. H., Garcia-Rivera, J. A., Peretz, M., Llano, M., et al. (2009). LEDGF/p75 proteins with alternative chromatin tethers are functional HIV-1 cofactors. PLoS Pathog. 5:e1000522. doi: 10.1371/journal.ppat.1000522
Meucci, O., Fatatis, A., Simen, A. A., Bushell, T. J., Gray, P. W., and Miller, R. J. (1998). Chemokines regulate hippocampal neuronal signaling and gp120 neurotoxicity. Proc. Natl. Acad. Sci. U.S.A. 95, 14500–14505. doi: 10.1073/pnas.95.24.14500
Micci, L., Alvarez, X., Iriele, R. I., Ortiz, A. M., Ryan, E. S., McGary, C. S., et al. (2014). CD4 depletion in SIV-infected macaques results in macrophage and microglia infection with rapid turnover of infected cells. PLoS Pathog. 10:e1004467. doi: 10.1371/journal.ppat.1004467
Miranda, T. B., Cortez, C. C., Yoo, C. B., Liang, G., Abe, M., Kelly, T. K., et al. (2009). DZNep is a global histone methylation inhibitor that reactivates developmental genes not silenced by DNA methylation. Mol. Cancer Ther. 8, 1579–1588. doi: 10.1158/1535-7163.MCT-09-0013
Miyagi, E., Schwartzkopff, F., Plishka, R., Buckler-White, A., Clouse, K. A., and Strebel, K. (2008). APOBEC3G-independent reduction in virion infectivity during long-term HIV-1 replication in terminally differentiated macrophages. Virology 379, 266–274. doi: 10.1016/j.virol.2008.06.033
Montaner, L. J., Bailer, R. T., and Gordon, S. (1997). IL-13 acts on macrophages to block the completion of reverse transcription, inhibit virus production, and reduce virus infectivity. J. Leukoc. Biol. 62, 126–132. doi: 10.1002/jlb.62.1.126
Montaner, L. J., Griffin, P., and Gordon, S. (1994). Interleukin-10 inhibits initial reverse transcription of human immunodeficiency virus type 1 and mediates a virostatic latent state in primary blood-derived human macrophages in vitro. J. Gen. Virol. 75(Pt 12), 3393–3400. doi: 10.1099/0022-1317-75-12-3393
Mosoian, A., Zhang, L., Hong, F., Cunyat, F., Rahman, A., Bhalla, R., et al. (2017). Frontline science: HIV infection of Kupffer cells results in an amplified proinflammatory response to LPS. J. Leukoc. Biol. 101, 1083–1090. doi: 10.1189/jlb.3HI0516-242R
Mosser, D. M. (2003). The many faces of macrophage activation. J. Leukoc. Biol. 73, 209–212. doi: 10.1189/jlb.0602325
Mosser, D. M., and Edwards, J. P. (2008). Exploring the full spectrum of macrophage activation. Nat. Rev. Immunol. 8, 958–969. doi: 10.1038/nri2448
Murray, J. M., Zaunders, J. J., McBride, K. L., Xu, Y., Bailey, M., Suzuki, K., et al. (2014). HIV DNA subspecies persist in both activated and resting memory CD4+ T cells during antiretroviral therapy. J. Virol. 88, 3516–3526. doi: 10.1128/JVI.03331-13
Naif, H. M., Chang, J., Ho-Shon, M., Li, S., and Cunningham, A. L. (1996). Inhibition of human immunodeficiency virus replication in differentiating monocytes by interleukin 10 occurs in parallel with inhibition of cellular RNA expression. AIDS Res. Hum. Retroviruses 12, 1237–1245. doi: 10.1089/aid.1996.12.1237
Narayanan, A., Kehn-Hall, K., Bailey, C., and Kashanchi, F. (2011). Analysis of the roles of HIV-derived microRNAs. Expert Opin. Biol. Ther. 11, 17–29. doi: 10.1517/14712598.2011.540564
Neuen-Jacob, E., Arendt, G., Wendtland, B., Jacob, B., Schneeweis, M., and Wechsler, W. (1993). Frequency and topographical distribution of CD68-positive macrophages and HIV-1 core proteins in HIV-associated brain lesions. Clin. Neuropathol. 12, 315–324.
Nicholson, J. K., Cross, G. D., Callaway, C. S., and McDougal, J. S. (1986). In vitro infection of human monocytes with human T lymphotropic virus type III/lymphadenopathy-associated virus (HTLV-III/LAV). J. Immunol. 137, 323–329.
Nou, E., Lo, J., and Grinspoon, S. K. (2016). Inflammation, immune activation, and cardiovascular disease in HIV. AIDS 30, 1495–1509. doi: 10.1097/QAD.0000000000001109
Oguariri, R. M., Brann, T. W., and Imamichi, T. (2007). Hydroxyurea and interleukin-6 synergistically reactivate HIV-1 replication in a latently infected promonocytic cell line via SP1/SP3 transcription factors. J. Biol. Chem. 282, 3594–3604. doi: 10.1074/jbc.M608150200
Ohagen, A., Ghosh, S., He, J., Huang, K., Chen, Y., Yuan, M., et al. (1999). Apoptosis induced by infection of primary brain cultures with diverse human immunodeficiency virus type 1 isolates: evidence for a role of the envelope. J. Virol. 73, 897–906.
Olivares, I., Ballester, A., Lombardia, L., Dominguez, O., and Lopez-Galindez, C. (2009). Human immunodeficiency virus type 1 chronic infection is associated with different gene expression in MT-4, H9 and U937 cell lines. Virus Res. 139, 22–31. doi: 10.1016/j.virusres.2008.09.010
Orenstein, J. M., Fox, C., and Wahl, S. M. (1997). Macrophages as a source of HIV during opportunistic infections. Science 276, 1857–1861. doi: 10.1126/science.276.5320.1857
Ortega, A. D., Willers, I. M., Sala, S., and Cuezva, J. M. (2010). Human G3BP1 interacts with beta-F1-ATPase mRNA and inhibits its translation. J. Cell Sci. 123(Pt 16), 2685–2696. doi: 10.1242/jcs.065920
Pache, L., Dutra, M. S., Spivak, A. M., Marlett, J. M., Murry, J. P., Hwang, Y., et al. (2015). BIRC2/cIAP1 is a negative regulator of HIV-1 transcription and can be targeted by smac mimetics to promote reversal of viral latency. Cell Host Microbe 18, 345–353. doi: 10.1016/j.chom.2015.08.009
Pang, S., Koyanagi, Y., Miles, S., Wiley, C., Vinters, H. V., and Chen, I. S. (1990). High levels of unintegrated HIV-1 DNA in brain tissue of AIDS dementia patients. Nature 343, 85–89. doi: 10.1038/343085a0
Park, I. W., Wang, J. F., and Groopman, J. E. (2001). HIV-1 Tat promotes monocyte chemoattractant protein-1 secretion followed by transmigration of monocytes. Blood 97, 352–358. doi: 10.1182/blood.v97.2.352
Passlick, B., Flieger, D., and Ziegler-Heitbrock, H. W. (1989). Identification and characterization of a novel monocyte subpopulation in human peripheral blood. Blood 74, 2527–2534. doi: 10.1182/blood.v74.7.2527.2527
Patel, A. A., Zhang, Y., Fullerton, J. N., Boelen, L., Rongvaux, A., Maini, A. A., et al. (2017). The fate and lifespan of human monocyte subsets in steady state and systemic inflammation. J. Exp. Med. 214, 1913–1923. doi: 10.1084/jem.20170355
Patel, C. A., Mukhtar, M., and Pomerantz, R. J. (2000). Human immunodeficiency virus type 1 Vpr induces apoptosis in human neuronal cells. J. Virol. 74, 9717–9726. doi: 10.1128/jvi.74.20.9717-9726.2000
Peng, G., Greenwell-Wild, T., Nares, S., Jin, W., Lei, K. J., Rangel, Z. G., et al. (2007). Myeloid differentiation and susceptibility to HIV-1 are linked to APOBEC3 expression. Blood 110, 393–400. doi: 10.1182/blood-2006-10-051763
Penton, P. K., and Blackard, J. T. (2014). Analysis of HIV quasispecies suggests compartmentalization in the liver. AIDS Res. Hum. Retroviruses 30, 394–402. doi: 10.1089/AID.2013.0146
Perez-Bercoff, D., David, A., Sudry, H., Barre-Sinoussi, F., and Pancino, G. (2003). Fcgamma receptor-mediated suppression of human immunodeficiency virus type 1 replication in primary human macrophages. J. Virol. 77, 4081–4094. doi: 10.1128/jvi.77.7.4081-4094.2003
Pierson, T. C., Zhou, Y., Kieffer, T. L., Ruff, C. T., Buck, C., and Siliciano, R. F. (2002). Molecular characterization of preintegration latency in human immunodeficiency virus type 1 infection. J. Virol. 76, 8518–8531. doi: 10.1128/jvi.76.17.8518-8513.2002
Piller, S. C., Jans, P., Gage, P. W., and Jans, D. A. (1998). Extracellular HIV-1 virus protein R causes a large inward current and cell death in cultured hippocampal neurons: implications for AIDS pathology. Proc. Natl. Acad. Sci. U.S.A. 95, 4595–4600. doi: 10.1073/pnas.95.8.4595
Poles, M. A., Boscardin, W. J., Elliott, J., Taing, P., Fuerst, M. M., McGowan, I., et al. (2006). Lack of decay of HIV-1 in gut-associated lymphoid tissue reservoirs in maximally suppressed individuals. J. Acquir. Immune Defic. Syndr. 43, 65–68. doi: 10.1097/01.qai.0000230524.71717.14
Price, D. H. (2000). P-TEFb, a cyclin-dependent kinase controlling elongation by RNA polymerase II. Mol. Cell Biol. 20, 2629–2634. doi: 10.1128/mcb.20.8.2629-2634.2000
Pulliam, L., Gascon, R., Stubblebine, M., McGuire, D., and McGrath, M. S. (1997). Unique monocyte subset in patients with AIDS dementia. Lancet 349, 692–695. doi: 10.1016/S0140-6736(96)10178-1
Pulliam, L., Sun, B., and Rempel, H. (2004). Invasive chronic inflammatory monocyte phenotype in subjects with high HIV-1 viral load. J. Neuroimmunol. 157, 93–98. doi: 10.1016/j.jneuroim.2004.08.039
Rasmussen, T. A., Schmeltz Sogaard, O., Brinkmann, C., Wightman, F., Lewin, S. R., Melchjorsen, J., et al. (2013). Comparison of HDAC inhibitors in clinical development: effect on HIV production in latently infected cells and T-cell activation. Hum. Vaccin. Immunother. 9, 993–1001. doi: 10.4161/hv.23800
Rice, A. P., and Herrmann, C. H. (2003). Regulation of TAK/P-TEFb in CD4+ T lymphocytes and macrophages. Curr. HIV Res. 1, 395–404. doi: 10.2174/1570162033485159
Rich, E. A., Chen, I. S., Zack, J. A., Leonard, M. L., and O’Brien, W. A. (1992). Increased susceptibility of differentiated mononuclear phagocytes to productive infection with human immunodeficiency virus-1 (HIV-1). J. Clin. Invest. 89, 176–183. doi: 10.1172/JCI115559
Robertson, K. R., Smurzynski, M., Parsons, T. D., Wu, K., Bosch, R. J., Wu, J., et al. (2007). The prevalence and incidence of neurocognitive impairment in the HAART era. AIDS 21, 1915–1921. doi: 10.1097/QAD.0b013e32828e4e27
Rohr, O., Lecestre, D., Chasserot-Golaz, S., Marban, C., Avram, D., Aunis, D., et al. (2003). Recruitment of Tat to heterochromatin protein HP1 via interaction with CTIP2 inhibits human immunodeficiency virus type 1 replication in microglial cells. J. Virol. 77, 5415–5427. doi: 10.1128/jvi.77.9.5415-5427.2003
Rose, R., Lamers, S. L., Nolan, D. J., Maidji, E., Faria, N. R., Pybus, O. G., et al. (2016). HIV maintains an evolving and dispersed population in multiple tissues during suppressive combined antiretroviral therapy in individuals with cancer. J. Virol. 90, 8984–8993. doi: 10.1128/JVI.00684-16
Sarracino, A., Gharu, L., Kula, A., Pasternak, A. O., Avettand-Fenoel, V., Rouzioux, C., et al. (2018). Posttranscriptional Regulation of HIV-1 Gene Expression during Replication and Reactivation from Latency by Nuclear Matrix Protein MATR3. mBio 9:e2158-18. doi: 10.1128/mBio.02158-18
Schmitt, M. P., Gendrault, J. L., Schweitzer, C., Steffan, A. M., Beyer, C., Royer, C., et al. (1990). Permissivity of primary cultures of human Kupffer cells for HIV-1. AIDS Res. Hum. Retroviruses 6, 987–991. doi: 10.1089/aid.1990.6.987
Schnell, G., Spudich, S., Harrington, P., Price, R. W., and Swanstrom, R. (2009). Compartmentalized human immunodeficiency virus type 1 originates from long-lived cells in some subjects with HIV-1-associated dementia. PLoS Pathog. 5:e1000395. doi: 10.1371/journal.ppat.1000395
Schroder, A. R., Shinn, P., Chen, H., Berry, C., Ecker, J. R., and Bushman, F. (2002). HIV-1 integration in the human genome favors active genes and local hotspots. Cell 110, 521–529. doi: 10.1016/s0092-8674(02)00864-4
Schuitemaker, H., Kootstra, N. A., Koppelman, M. H., Bruisten, S. M., Huisman, H. G., Tersmette, M., et al. (1992). Proliferation-dependent HIV-1 infection of monocytes occurs during differentiation into macrophages. J. Clin. Invest. 89, 1154–1160. doi: 10.1172/JCI115697
Schulz, C., Gomez Perdiguero, E., Chorro, L., Szabo-Rogers, H., Cagnard, N., Kierdorf, K., et al. (2012). A lineage of myeloid cells independent of Myb and hematopoietic stem cells. Science 336, 86–90. doi: 10.1126/science.1219179
Scripture-Adams, D. D., Brooks, D. G., Korin, Y. D., and Zack, J. A. (2002). Interleukin-7 induces expression of latent human immunodeficiency virus type 1 with minimal effects on T-cell phenotype. J. Virol. 76, 13077–13082. doi: 10.1128/jvi.76.24.13077-13082.2002
Shan, L., Deng, K., Shroff, N. S., Durand, C. M., Rabi, S. A., Yang, H. C., et al. (2012). Stimulation of HIV-1-specific cytolytic T lymphocytes facilitates elimination of latent viral reservoir after virus reactivation. Immunity 36, 491–501. doi: 10.1016/j.immuni.2012.01.014
Shehu-Xhilaga, M., Rhodes, D., Wightman, F., Liu, H. B., Solomon, A., Saleh, S., et al. (2009). The novel histone deacetylase inhibitors metacept-1 and metacept-3 potently increase HIV-1 transcription in latently infected cells. AIDS 23, 2047–2050. doi: 10.1097/QAD.0b013e328330342c
Shen, R., Meng, G., Ochsenbauer, C., Clapham, P. R., Grams, J., Novak, L., et al. (2011). Stromal down-regulation of macrophage CD4/CCR5 expression and NF-kappaB activation mediates HIV-1 non-permissiveness in intestinal macrophages. PLoS Pathog. 7:e1002060. doi: 10.1371/journal.ppat.1002060
Shen, R., Richter, H. E., Clements, R. H., Novak, L., Huff, K., Bimczok, D., et al. (2009). Macrophages in vaginal but not intestinal mucosa are monocyte-like and permissive to human immunodeficiency virus type 1 infection. J. Virol. 83, 3258–3267. doi: 10.1128/JVI.01796-08
Shiramizu, B., Gartner, S., Williams, A., Shikuma, C., Ratto-Kim, S., Watters, M., et al. (2005). Circulating proviral HIV DNA and HIV-associated dementia. AIDS 19, 45–52. doi: 10.1097/00002030-200501030-00005
Sierra-Madero, J. G., Toossi, Z., Hom, D. L., Finegan, C. K., Hoenig, E., and Rich, E. A. (1994). Relationship between load of virus in alveolar macrophages from human immunodeficiency virus type 1-infected persons, production of cytokines, and clinical status. J. Infect. Dis. 169, 18–27. doi: 10.1093/infdis/169.1.18
Simioni, S., Cavassini, M., Annoni, J. M., Rimbault Abraham, A., Bourquin, I., Schiffer, V., et al. (2010). Cognitive dysfunction in HIV patients despite long-standing suppression of viremia. AIDS 24, 1243–1250. doi: 10.1097/QAD.0b013e3283354a7b
Solas, C., Lafeuillade, A., Halfon, P., Chadapaud, S., Hittinger, G., and Lacarelle, B. (2003). Discrepancies between protease inhibitor concentrations and viral load in reservoirs and sanctuary sites in human immunodeficiency virus-infected patients. Antimicrob. Agents Chemother. 47, 238–243. doi: 10.1128/aac.47.1.238-243.2003
Song, L., Nath, A., Geiger, J. D., Moore, A., and Hochman, S. (2003). Human immunodeficiency virus type 1 Tat protein directly activates neuronal N-methyl-D-aspartate receptors at an allosteric zinc-sensitive site. J. Neurovirol. 9, 399–403. doi: 10.1080/13550280390201704
Sonza, S., Maerz, A., Deacon, N., Meanger, J., Mills, J., and Crowe, S. (1996). Human immunodeficiency virus type 1 replication is blocked prior to reverse transcription and integration in freshly isolated peripheral blood monocytes. J. Virol. 70, 3863–3869.
Sonza, S., Mutimer, H. P., Oelrichs, R., Jardine, D., Harvey, K., Dunne, A., et al. (2001). Monocytes harbour replication-competent, non-latent HIV-1 in patients on highly active antiretroviral therapy. AIDS 15, 17–22. doi: 10.1097/00002030-200101050-00005
Stewart, M. D., Li, J., and Wong, J. (2005). Relationship between histone H3 lysine 9 methylation, transcription repression, and heterochromatin protein 1 recruitment. Mol. Cell Biol. 25, 2525–2538. doi: 10.1128/MCB.25.7.2525-2538.2005
Stoler, M. H., Eskin, T. A., Benn, S., Angerer, R. C., and Angerer, L. M. (1986). Human T-cell lymphotropic virus type III infection of the central nervous system. A preliminary in situ analysis. JAMA 256, 2360–2364. doi: 10.1001/jama.256.17.2360
Subramanian, S., Tawakol, A., Burdo, T. H., Abbara, S., Wei, J., Vijayakumar, J., et al. (2012). Arterial inflammation in patients with HIV. JAMA 308, 379–386. doi: 10.1001/jama.2012.6698
Sunderkotter, C., Nikolic, T., Dillon, M. J., Van Rooijen, N., Stehling, M., Drevets, D. A., et al. (2004). Subpopulations of mouse blood monocytes differ in maturation stage and inflammatory response. J. Immunol. 172, 4410–4417. doi: 10.4049/jimmunol.172.7.4410
Sung, T. L., and Rice, A. P. (2009). miR-198 inhibits HIV-1 gene expression and replication in monocytes and its mechanism of action appears to involve repression of cyclin T1. PLoS Pathog. 5:e1000263. doi: 10.1371/journal.ppat.1000263
Swingler, S., Mann, A. M., Zhou, J., Swingler, C., and Stevenson, M. (2007). Apoptotic killing of HIV-1-infected macrophages is subverted by the viral envelope glycoprotein. PLoS Pathog. 3:e0030134. doi: 10.1371/journal.ppat.0030134
Tanaka, Y., Kameoka, M., Ota, K., Itaya, A., Ikuta, K., and Yoshihara, K. (1999). Establishment of persistent infection with HIV-1 abrogates the caspase-3-dependent apoptotic signaling pathway in U937 cells. Exp. Cell Res. 247, 514–524. doi: 10.1006/excr.1998.4376
Taube, R., and Peterlin, M. (2013). Lost in transcription: molecular mechanisms that control HIV latency. Viruses 5, 902–927. doi: 10.3390/v5030902
Tawakol, A., Ishai, A., Li, D., Takx, R. A., Hur, S., Kaiser, Y., et al. (2017). Association of arterial and lymph node inflammation with distinct inflammatory pathways in human immunodeficiency virus infection. JAMA Cardiol. 2, 163–171. doi: 10.1001/jamacardio.2016.4728
Teo, I., Veryard, C., Barnes, H., An, S. F., Jones, M., Lantos, P. L., et al. (1997). Circular forms of unintegrated human immunodeficiency virus type 1 DNA and high levels of viral protein expression: association with dementia and multinucleated giant cells in the brains of patients with AIDS. J. Virol. 71, 2928–2933.
Thieblemont, N., Weiss, L., Sadeghi, H. M., Estcourt, C., and Haeffner-Cavaillon, N. (1995). CD14lowCD16high: a cytokine-producing monocyte subset which expands during human immunodeficiency virus infection. Eur. J. Immunol. 25, 3418–3424. doi: 10.1002/eji.1830251232
Tourriere, H., Gallouzi, I. E., Chebli, K., Capony, J. P., Mouaikel, J., van der Geer, P., et al. (2001). RasGAP-associated endoribonuclease G3Bp: selective RNA degradation and phosphorylation-dependent localization. Mol. Cell Biol. 21, 7747–7760. doi: 10.1128/MCB.21.22.7747-7760.2001
Triant, V. A., and Grinspoon, S. K. (2017). Epidemiology of ischemic heart disease in HIV. Curr. Opin. HIV AIDS 12, 540–547. doi: 10.1097/COH.0000000000000410
Triboulet, R., Mari, B., Lin, Y. L., Chable-Bessia, C., Bennasser, Y., Lebrigand, K., et al. (2007). Suppression of microRNA-silencing pathway by HIV-1 during virus replication. Science 315, 1579–1582. doi: 10.1126/science.1136319
Tso, F. Y., Kang, G., Kwon, E. H., Julius, P., Li, Q., West, J. T., et al. (2018). Brain is a potential sanctuary for subtype C HIV-1 irrespective of ART treatment outcome. PLoS One 13:e0201325. doi: 10.1371/journal.pone.0201325
Tyagi, M., and Karn, J. (2007). CBF-1 promotes transcriptional silencing during the establishment of HIV-1 latency. EMBO J. 26, 4985–4995. doi: 10.1038/sj.emboj.7601928
Tyagi, M., and Kashanchi, F. (2012). New and novel intrinsic host repressive factors against HIV-1: PAF1 complex, HERC5 and others. Retrovirology 9:19. doi: 10.1186/1742-4690-9-19
Vandegraaff, N., Devroe, E., Turlure, F., Silver, P. A., and Engelman, A. (2006). Biochemical and genetic analyses of integrase-interacting proteins lens epithelium-derived growth factor (LEDGF)/p75 and hepatoma-derived growth factor related protein 2 (HRP2) in preintegration complex function and HIV-1 replication. Virology 346, 415–426. doi: 10.1016/j.virol.2005.11.022
van’t Wout, A. B., Ran, L. J., Kuiken, C. L., Kootstra, N. A., Pals, S. T., and Schuitemaker, H. (1998). Analysis of the temporal relationship between human immunodeficiency virus type 1 quasispecies in sequential blood samples and various organs obtained at autopsy. J. Virol. 72, 488–496.
Varol, C., Landsman, L., Fogg, D. K., Greenshtein, L., Gildor, B., Margalit, R., et al. (2007). Monocytes give rise to mucosal, but not splenic, conventional dendritic cells. J. Exp. Med. 204, 171–180. doi: 10.1084/jem.20061011
Verdin, E., Paras, P. Jr., and Van Lint, C. (1993). Chromatin disruption in the promoter of human immunodeficiency virus type 1 during transcriptional activation. EMBO J. 12, 3249–3259. doi: 10.1002/j.1460-2075.1993.tb05994.x
Wang, J., and Kubes, P. (2016). A reservoir of mature cavity macrophages that can rapidly invade visceral organs to affect tissue repair. Cell 165, 668–678. doi: 10.1016/j.cell.2016.03.009
Wang, J., Roderiquez, G., Oravecz, T., and Norcross, M. A. (1998). Cytokine regulation of human immunodeficiency virus type 1 entry and replication in human monocytes/macrophages through modulation of CCR5 expression. J. Virol. 72, 7642–7647.
Wang, X., Wang, H., Liu, M. Q., Li, J. L., Zhou, R. H., Zhou, Y., et al. (2017). IFN-lambda inhibits drug-resistant HIV infection of macrophages. Front. Immunol. 8:210. doi: 10.3389/fimmu.2017.00210
Wang, X., Ye, L., Hou, W., Zhou, Y., Wang, Y. J., Metzger, D. S., et al. (2009). Cellular microRNA expression correlates with susceptibility of monocytes/macrophages to HIV-1 infection. Blood 113, 671–674. doi: 10.1182/blood-2008-09-175000
Warrilow, D., Gardner, J., Darnell, G. A., Suhrbier, A., and Harrich, D. (2006). HIV type 1 inhibition by protein kinase C modulatory compounds. AIDS Res. Hum. Retroviruses 22, 854–864. doi: 10.1089/aid.2006.22.854
Wellensiek, B. P., Ramakrishnan, R., Sundaravaradan, V., Mehta, R., Harris, D. T., and Ahmad, N. (2009). Differential HIV-1 integration targets more actively transcribed host genes in neonatal than adult blood mononuclear cells. Virology 385, 28–38. doi: 10.1016/j.virol.2008.10.052
Westhorpe, C. L., Maisa, A., Spelman, T., Hoy, J. F., Dewar, E. M., Karapanagiotidis, S., et al. (2014). Associations between surface markers on blood monocytes and carotid atherosclerosis in HIV-positive individuals. Immunol. Cell Biol. 92, 133–138. doi: 10.1038/icb.2013.84
Wiley, C. A., Schrier, R. D., Nelson, J. A., Lampert, P. W., and Oldstone, M. B. (1986). Cellular localization of human immunodeficiency virus infection within the brains of acquired immune deficiency syndrome patients. Proc. Natl. Acad. Sci. U.S.A. 83, 7089–7093. doi: 10.1073/pnas.83.18.7089
Williams, D. W., Byrd, D., Rubin, L. H., Anastos, K., Morgello, S., and Berman, J. W. (2014). CCR2 on CD14(+)CD16(+) monocytes is a biomarker of HIV-associated neurocognitive disorders. Neurol. Neuroimmunol. Neuroinflamm. 1:e36. doi: 10.1212/NXI.0000000000000036
Williams, D. W., Calderon, T. M., Lopez, L., Carvallo-Torres, L., Gaskill, P. J., Eugenin, E. A., et al. (2013). Mechanisms of HIV entry into the CNS: increased sensitivity of HIV infected CD14+CD16+ monocytes to CCL2 and key roles of CCR2, JAM-A, and ALCAM in diapedesis. PLoS One 8:e69270. doi: 10.1371/journal.pone.0069270
Williams, D. W., Eugenin, E. A., Calderon, T. M., and Berman, J. W. (2012). Monocyte maturation, HIV susceptibility, and transmigration across the blood brain barrier are critical in HIV neuropathogenesis. J. Leukoc. Biol. 91, 401–415. doi: 10.1189/jlb.0811394
Wolf, D., Witte, V., Laffert, B., Blume, K., Stromer, E., Trapp, S., et al. (2001). HIV-1 Nef associated PAK and PI3-kinases stimulate Akt-independent Bad-phosphorylation to induce anti-apoptotic signals. Nat. Med. 7, 1217–1224. doi: 10.1038/nm1101-1217
Wong, K. L., Tai, J. J., Wong, W. C., Han, H., Sem, X., Yeap, W. H., et al. (2011). Gene expression profiling reveals the defining features of the classical, intermediate, and nonclassical human monocyte subsets. Blood 118, e16–e31. doi: 10.1182/blood-2010-12-326355
Wu, J., Ao, M. T., Shao, R., Wang, H. R., Yu, D., Fang, M. J., et al. (2017). A chalcone derivative reactivates latent HIV-1 transcription through activating P-TEFb and promoting Tat-SEC interaction on viral promoter. Sci. Rep. 7:10657. doi: 10.1038/s41598-017-10728-w
Yedavalli, V. S., and Jeang, K. T. (2011). Matrin 3 is a co-factor for HIV-1 Rev in regulating post-transcriptional viral gene expression. Retrovirology 8:61. doi: 10.1186/1742-4690-8-61
Yona, S., Kim, K. W., Wolf, Y., Mildner, A., Varol, D., Breker, M., et al. (2013). Fate mapping reveals origins and dynamics of monocytes and tissue macrophages under homeostasis. Immunity 38, 79–91. doi: 10.1016/j.immuni.2012.12.001
Yukl, S. A., Sinclair, E., Somsouk, M., Hunt, P. W., Epling, L., Killian, M., et al. (2014). A comparison of methods for measuring rectal HIV levels suggests that HIV DNA resides in cells other than CD4+ T cells, including myeloid cells. AIDS 28, 439–442. doi: 10.1097/QAD.0000000000000166
Zalar, A., Figueroa, M. I., Ruibal-Ares, B., Bare, P., Cahn, P., de Bracco, M. M., et al. (2010). Macrophage HIV-1 infection in duodenal tissue of patients on long term HAART. Antiviral Res. 87, 269–271. doi: 10.1016/j.antiviral.2010.05.005
Zhang, H. (2009). Reversal of HIV-1 latency with anti-microRNA inhibitors. Int. J. Biochem. Cell Biol. 41, 451–454. doi: 10.1016/j.biocel.2008.07.016
Zhang, H., Dornadula, G., Beumont, M., Livornese, L. Jr., Van Uitert, B., Henning, K., et al. (1998). Human immunodeficiency virus type 1 in the semen of men receiving highly active antiretroviral therapy. N. Engl. J. Med. 339, 1803–1809. doi: 10.1056/NEJM199812173392502
Zhang, M., Li, X., Pang, X., Ding, L., Wood, O., Clouse, K. A., et al. (2002). Bcl-2 upregulation by HIV-1 Tat during infection of primary human macrophages in culture. J. Biomed. Sci. 9, 133–139. doi: 10.1007/bf02256024
Zhang, Z., Schuler, T., Zupancic, M., Wietgrefe, S., Staskus, K. A., Reimann, K. A., et al. (1999). Sexual transmission and propagation of SIV and HIV in resting and activated CD4+ T cells. Science 286, 1353–1357. doi: 10.1126/science.286.5443.1353
Zhou, Y., Zhang, H., Siliciano, J. D., and Siliciano, R. F. (2005). Kinetics of human immunodeficiency virus type 1 decay following entry into resting CD4+ T cells. J. Virol. 79, 2199–2210. doi: 10.1128/JVI.79.4.2199-2210.2005
Ziegler-Heitbrock, L., Ancuta, P., Crowe, S., Dalod, M., Grau, V., Hart, D. N., et al. (2010). Nomenclature of monocytes and dendritic cells in blood. Blood 116, e74–e80. doi: 10.1182/blood-2010-02-258558
Keywords: HIV-1, monocytes/macrophages, latency, pathogenesis, reservoir
Citation: Kruize Z and Kootstra NA (2019) The Role of Macrophages in HIV-1 Persistence and Pathogenesis. Front. Microbiol. 10:2828. doi: 10.3389/fmicb.2019.02828
Received: 10 July 2019; Accepted: 21 November 2019;
Published: 05 December 2019.
Edited by:
Maria J. Buzon, Vall d’Hebron Research Institute (VHIR), SpainReviewed by:
Guochun Jiang, The University of North Carolina at Chapel Hill, United StatesShannon Marie Murray, Fred Hutchinson Cancer Research Center, United States
Copyright © 2019 Kruize and Kootstra. This is an open-access article distributed under the terms of the Creative Commons Attribution License (CC BY). The use, distribution or reproduction in other forums is permitted, provided the original author(s) and the copyright owner(s) are credited and that the original publication in this journal is cited, in accordance with accepted academic practice. No use, distribution or reproduction is permitted which does not comply with these terms.
*Correspondence: Neeltje A. Kootstra, bi5hLmtvb3RzdHJhQGFtc3RlcmRhbXVtYy5ubA==; bi5hLmtvb3RzdHJhQGFtYy51dmEubmw=