- Sorbonne Université, CNRS, Laboratoire d’Ecogéochimie des Environnements Benthiques (LECOB), Banyuls-sur-Mer, France
Cold-water coral (CWC) ecosystems provide niches and nurseries for many deep-sea species. Lophelia pertusa and Madrepora oculata, two cosmopolitan species forming three dimensional structures, are found in cold waters under specific hydrological regimes that provide food and reoxygenation. There is now more information about their feeding, their growth and their associated microbiome, however, little is known about the influence of their habitat on their physiology, or on the composition of their bacterial community. The goal of this study was to test if the habitat of L. pertusa and M. oculata influenced the hosts associated bacterial communities, the corals’ survival and their skeletal growth along the slope of a submarine canyon. A transplant experiment was used, based on sampling and cross-redeployment of coral fragments at two contrasted sites, one deeper and one shallower. Our results show that M. oculata had significantly higher skeletal growth rates in the shallower site and that it had a specific microbiome that did not change between sites. Inversely, L. pertusa had the same growth rates at both sites, but its bacterial community compositions differed between locations. Additionally, transplanted L. pertusa acquired the microbial signature of the local corals. Thus, our results suggest that M. oculata prefer the shallower habitat.
Introduction
Scleractinian cold-water corals (CWC) such as Lophelia pertusa and Madrepora oculata, iconic engineer species of submarine canyons, are important frame-builders that provide shelter for a large diversity of associated fauna (Buhl-Mortensen et al., 2010). During the last decades, significant efforts have been dedicated to characterize CWC’s feeding, reproduction, growth, and their associated coral microbiome (Waller and Tyler, 2005; Tsounis et al., 2010; Lartaud et al., 2014; Meistertzheim et al., 2016; Galand et al., 2020). However, due to the difficulty of sampling in the deep sea, a detailed knowledge of their ecology is still lacking when compared to their shallow water counterparts. Such knowledge is important as these animals with a great ecological value are threatened by trawling, pollution and climate change (Freiwald et al., 2004; Foley and Armstrong, 2010).
Lophelia pertusa (synonymized recently as Desmophyllum pertusum (Addamo et al., 2016) and M. oculata are the most abundant species forming reef frameworks in the North Atlantic, the Gulf of Mexico, and the Mediterranean Sea (Freiwald et al., 2004, 2009; Roberts et al., 2006). While L. pertusa dominates in many regions, M. oculata is often found as a secondary species together with L. pertusa. M. oculata have fragile branches and it is therefore thought that their framework building capacity is limited (Freiwald, 2002). The relative abundance and/or spatial distribution of L. pertusa and M. oculata change following geographical locations, which suggests different living strategies (Freiwald et al., 2004; Schröder-Ritzrau et al., 2005; Lartaud et al., 2019). The two species exhibit different responses to environmental changes (Naumann et al., 2014), different skeletal growth rates (Lartaud et al., 2014), reproductive cycles (Waller and Tyler, 2005), feeding strategies (Tsounis et al., 2010; Gori et al., 2014; Galand et al., 2020), and bacterial community associations (Hansson et al., 2009; Meistertzheim et al., 2016; Galand et al., 2018, 2020), with a more stable microbiome for M. oculata.
Corals live in very close association with communities of microbes forming together a holobiont. Studies of shallow coral species suggest that the coral microbiome, dominated by photosynthetic zooxanthellate algae, is involved in a variety of physiological functions (Bourne et al., 2016). For CWCs living in a cold and deep environment, without symbiotic zooxanthellae, the role of the associated bacterial community may be different. Recent efforts have been dedicated to characterize the bacterial communities associated to CWCs, to identify their potential role in the nutrition and health of the holobiont, and to assess their responses to environmental changes (Neulinger et al., 2008; Jensen et al., 2019; Kellogg, 2019). Specific bacteria have already been identified such as Spirochetes or Endozoicomonas, which are observed in L. pertusa or M. oculata (Kellogg et al., 2009; Meistertzheim et al., 2016), and which may be crucial to the host physiology (Neave et al., 2016). It has also been demonstrated that L. pertusa and M. oculata have different species-specific microbial communities that can be maintained across different locations (Schöttner et al., 2012; Kellogg et al., 2017; Jensen et al., 2019). In addition, the bacterial community composition of L. pertusa can exhibit a strong temporal variability, but also differences within colonies, and between polyps from the same colony, contrary to the M. oculata microbiome, which appears much more stable (Meistertzheim et al., 2016; Galand et al., 2018). However, it is still unclear how those corals acquired their microbiome (e.g., vertical or horizontal transfer) and what is the role of the local environmental conditions in the bacterial community dynamic. Studies in aquaria are difficult to conduct to treat this subject as CWCs in captivity change their bacterial community composition with different dynamics seen between species (Galand et al., 2018); analyses in situ remain thus fundamental, but transplant experiments have never been conducted for cold water coral microbiomes.
The general aim of this study was to test how the local habitat of L. pertusa and M. oculata impact their bacterial associations, together with other integrative parameters such as coral calcification and mortality. The study was based on in situ transplant experiments with sampling and redeployment of coral fragments at two contrasted sites, one deeper and one shallower, in the Lacaze-Duthiers canyon (LDC) where L. pertusa and M. oculata reefs are naturally abundant (Fiala et al., 2010; Gori et al., 2013; Lartaud et al., 2014). This multidisciplinary approach ultimately aimed at better understanding the adequacy between CWC and their surrounding environmental conditions, and thus to better assess their resilience to a changing ocean.
Materials and Methods
Study Sites
The Lacaze-Duthiers canyon, part of the Gulf of Lion Nature Marine Park, is located in the Gulf of Lion, in the northwestern part of the Mediterranean Sea. The present study focused on two different morpho-bathymetric sites of the canyon: the deeper “A” site (42°32.43N, 03°25.17E), at 530 m depth, is characterized by large colonies of L. pertusa and some smaller M. oculata colonies that grow on hard substrates outcropping the floor (Lartaud et al., 2017). The shallower “B” site (42°33.47N, 03°24.29E), is located closer to the head of the canyon, where numerous M. oculata colonies, together with L. pertusa, grow on vertical cliffs that extend from 300 to 480 m depth. This submarine canyon, is characterized by the presence of episodic dense water shelf cascade events driven by wind conditions (Canals et al., 2009). Three different types of events are described, corresponding to autumnal storm events (stratified water masses with low intensity), winter storm events (large amount of particles), and winter cascading events (non-stratified water masses with high intensity) (Canals et al., 2006). These events lead to ventilation, changes in the temperature, current speed and transport of material such as organic matter and sediments. These events do not have the same strength at the deeper site compared to the shallow site.
Coral Sampling and Transplant Procedure
Lophelia pertusa and M. oculata were sampled from the deeper sites “A” at 530 m and the shallower site “B” at 380 m using a remotely operated vehicle (ROV) deployed form the R/V Minibex Vessel (COMEX SA). Coral fragments were collected in thermally insulated polypropylene boxes to maintain ambient seawater temperature (13°C) during transport to the surface. On board, colony fragments were transferred into aerated 30 L seawater tanks maintained at 13°C using a chiller. The same day, once in the laboratory, the apical parts of corals from both sites were cut with sharp pliers into different nubbins (nubbins with ∼5 polyps for L. pertusa and ∼10 polyps for M. oculata) and glued on cobblestones with an aquatic epoxy resin to form different transplants units (see Lartaud et al., 2014). Gloves were always used to avoid contamination and pliers were rinsed with alcohol and distilled water between each nubbin handling. Nubbins were kept in aquarium over the night until redeployment the following day. Two in situ experiments were performed but all samples were treated the same way. The first focused on coral colonies from both species collected at site B and redeployed on site B (control transplant unit called BB) or on site A (called BA) for 9 months. The second experiment was conducted with corals collected on site A and redeployed on site A (control called AA) or on site B (called AB) for 3 months. A total of twenty-three transplant units were used for L. pertusa (BB = 8, BA = 4, AA = 6, and AB = 5) and forty-three for M. oculata (BB = 10, BA = 4, AA = 18, and AB = 11). Corals from the transplant unit BA were not collected for the coral microbiome characterization due to the low number of polyps available. When transplant units were retrieved, all nubbins were flash frozen in liquid nitrogen after macroscopic analyses (for mortality and growth rate measures).
Mortality and Growth Rates
Each coral fragment was photographed on the cobblestones before and after deployment with the same orientation for macroscopic comparisons. Living, dead and newly formed polyps were identified and measured with a caliper following the protocols described in Lartaud et al. (2017) to determine the mortality, budding rates and linear extensions.
DNA Extractions and Sequencing
For Lophelia pertusa, DNA was extracted from the polyps of eight different colonies, and for M. oculata, from seven different colonies. In total, the study is based on 23 DNA samples for L. pertusa and 25 for M. oculata. For the DNA extraction, individual polyps were crushed using a hammer and the tissues were homogenized in tubes containing a garnet matrix without the large ball (Lysing Matrix A) and lysed mechanically using a FastPrep Instrument (MP, Biomedical, Santa Ana, CA, United States). DNA was extracted using the Maxwell Blood DNA Purification Kit LEV and the Maxwell 16 MDx Instrument (Promega, Madison, WI, United States). DNA concentrations were measured by spectrophotometry directly after extractions (NanoDrop ND-1000, Thermo Fisher Scientific, Waltham, MA, United States).
The V1–V3 region of the bacterial 16S rRNA genes were amplified using the primers 27F AGRGTTTGATCMTGGCTCAG modified from Lane (1991) and 519R GTNTTACNGCGGCKGCTG modified from Lane et al. (1985) with a single step and 28 cycles of polymerase chain reaction (PCR), annealing at 53°C, using the HotStarTaq Plus Master Mix Kit (Qiagen, Valencia, CA, United States). Following the PCR, all the amplicon products from the different samples were mixed in equal concentrations and purified using Agencourt AMPure beads (Agencourt Bioscience Corporation, MA, United States). The purified PCR products were used to prepare a DNA library by following the Illumina TruSeq DNA library preparation protocol. All the samples were sequenced on the same MiSeq Illumina sequencer run (Illumina, San Diego, CA, United States) using MiSeq reagent kit V3 (Illumina) producing 2 × 300-bp long reads. PCR and sequencing were conducted in a commercial laboratory (MR DNA, Shallowater, TX, United States). All sequences were deposited in GenBank under SRA accession number PRJNA563840.
Sequence Analysis
Sequence analysis were performed with the open-source software package DADA2 (v. 1.12) in “R” to model and correct Illumina-sequenced amplicon errors (Callahan et al., 2016). We applied the standard pipeline with the following parameters: trimLeft = 20, truncLen = c(290,250), maxN = 0, maxEE = c(2,2), truncQ = 2. Thus, the sequences were filtered, dereplicated, denoised by removing sample inference and chimeras, and merged. Representative sequences were classified against the SILVA v.128 database (Quast et al., 2012) for the taxonomy assignment and to obtain amplicon sequence variants (ASVs). To produce a finer taxonomical resolution, an additional BLAST search (Altschul et al., 1990) was performed on the ASVs that were the most abundant under each transplant condition. We obtained a total of 843,483 sequences for the entire datasets, which is an average of 17,000 sequences per sample.
Statistical Analysis
Tests for normality of variance were performed using the Shapiro–Wilk test on R software (v3.4.3), which revealed that the data distribution was not normal for mortality and skeletal growth rates (p < 0.05). A multiple-comparison non-parametric Kruskal–Wallis test was used to analyze differences between the sites for each parameter investigated.
To compare the bacterial community composition and diversity, the sequence data were normalized by dividing counts by sample size to obtain a relative abundance. Possible differences in bacterial community composition were assessed by correspondence analysis (CA) with the vegan package in R (Oksanen et al., 2013). The Bray Curtis index (Bray and Curtis, 1957) and the Shannon diversity index (Shannon, 1948) were computed for all samples with the vegan package in R. To identify the ASVs that characterized the different samples, a simper test was applied with the plyr package in R.
Results
Coral Mortality
For Madrepora oculata, polyp mortality was low when redeployed at their site of origin (Figure 1). For L. pertusa redeployed at their site of origin, there was no polyp mortality at site A, but some mortality at site B. There was no significant difference in mortality between species (n = 66, p > 0.05).
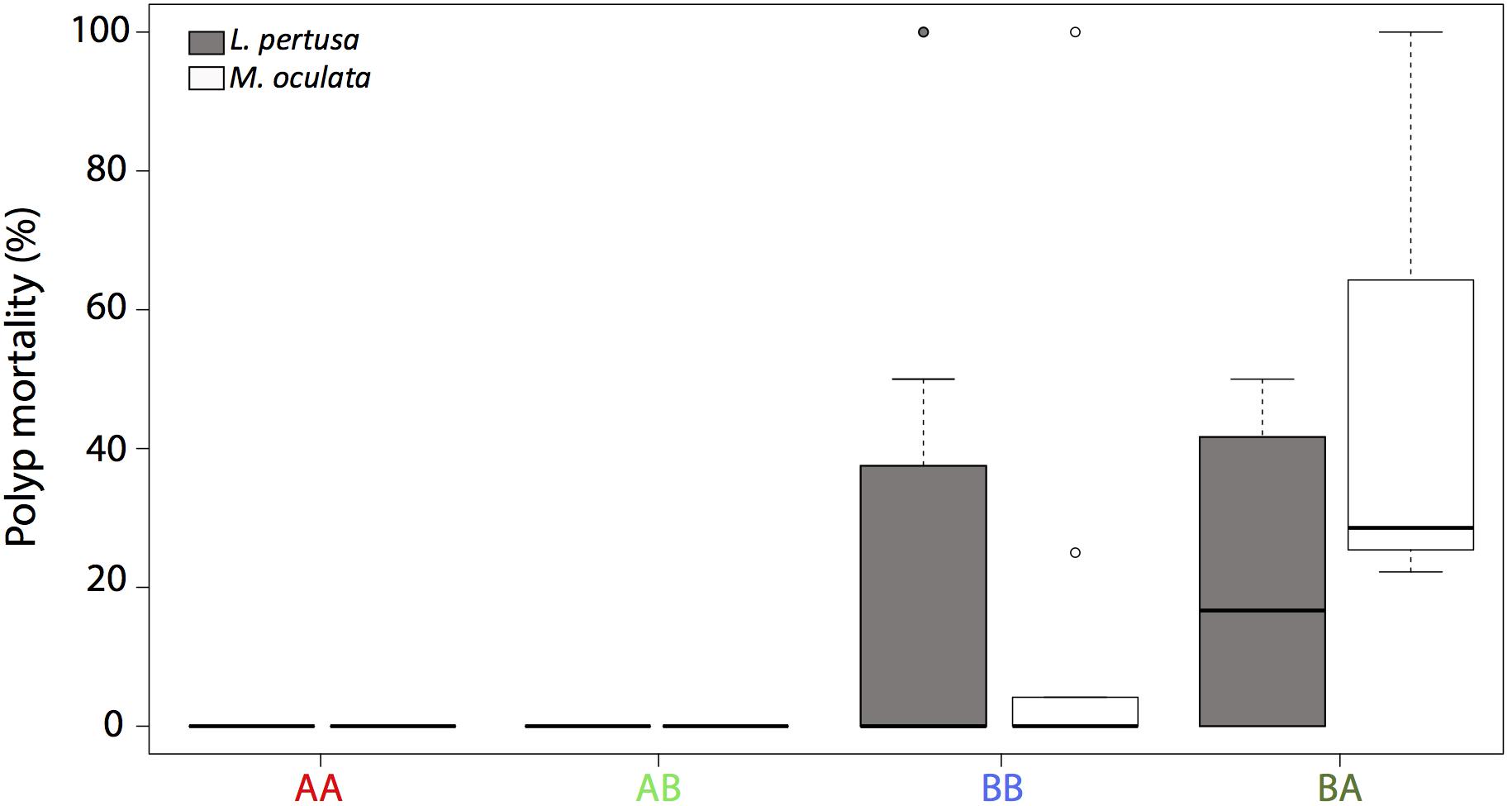
Figure 1. Polyp mortality of L. pertusa (gray) and M. oculata (white) at the deeper site A and the shallower site B. AA, coral originating from site A and redeployed at site A; BA, coral from site B and redeployed to A; AB, coral from site A and redeployed to B; BB, coral from site B and redeployed to B. The boxplots represent the median values, the quartiles, and the minimum and maximum.
For Lophelia pertusa, there was no difference in mortality (average < 10%) between individuals that were cross transplanted from one site to another and those that were not (n = 23, p > 0.05). For M. oculata, there was a difference in mortality between the cross transplanted and non-cross transplanted corals (n = 43, p < 0.001) with a higher mortality (average 50%) for the corals that were moved from site B to A (Figure 1).
Coral Growth Rates
For each coral fragment, the budding rates and the linear extension were measured (Figure 2). Overall, M. oculata had a significantly higher budding rate than L. pertusa (p < 0.001).
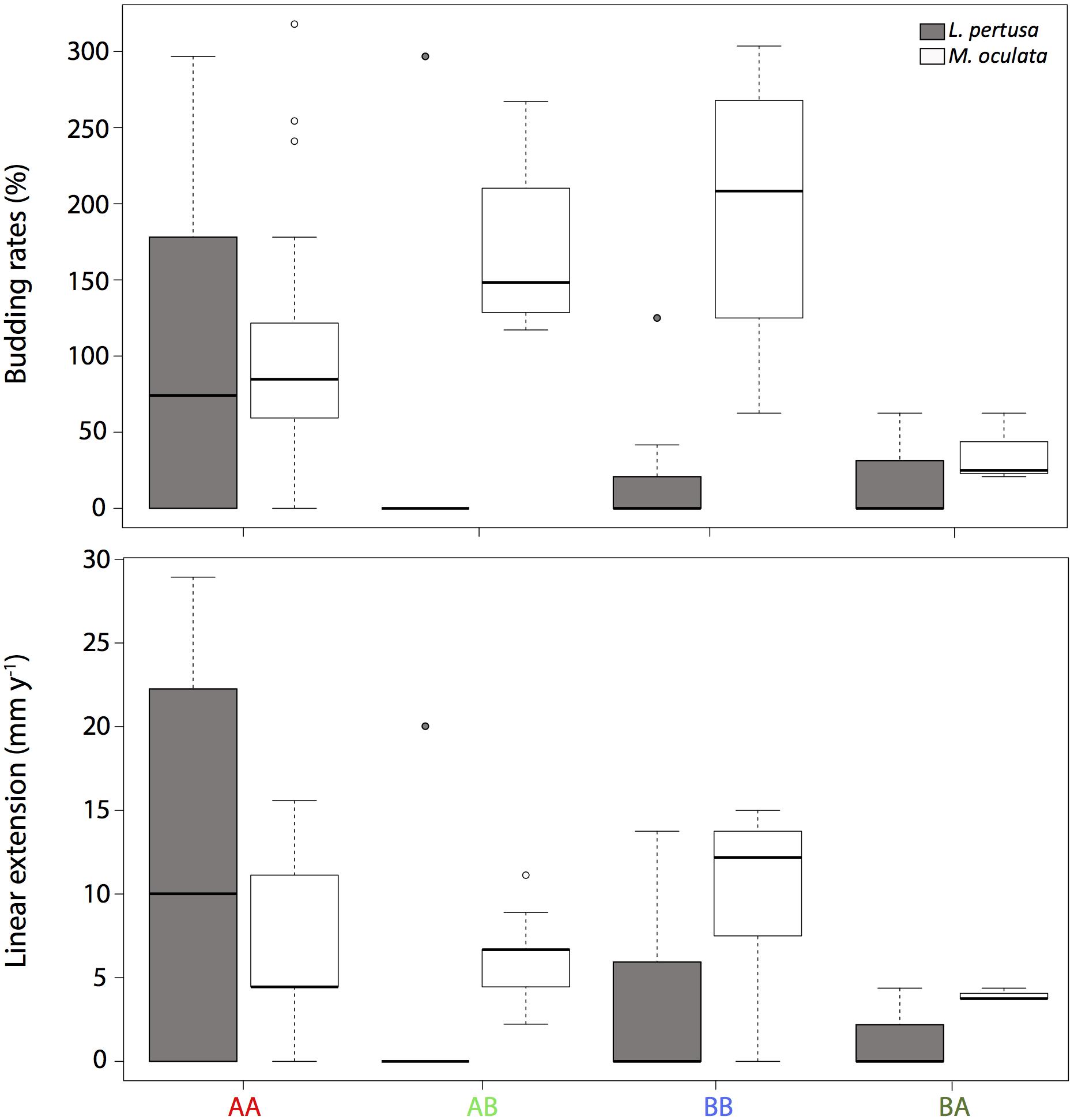
Figure 2. A Budding rates and B linear extension of L. pertusa (gray) and M. oculata (white) at the deeper site A and the shallower site B. AA, coral originating from site A and redeployed at site A; BA, coral from site B and redeployed to A; AB, coral from site A and redeployed to B; BB, coral from site B and redeployed to B. The boxplots represent the median values, the quartiles, and the minimum and maximum.
For Lophelia pertusa, there was no significant difference between corals growing at the two sites, although the highest budding rate values (average of 104 ± 124%) were observed for corals that originated and remained at the deeper site A (Figure 2). For M. oculata, the corals that grew on the shallower site B (averages of 200 ± 80% and 170 ± 50% for BB and AB, respectively) had significantly higher budding rates than corals at site A (averages of 100 ± 90% and 36 ± 23% for AA and BA, respectively, p < 0,001) (Figure 2).
For the linear extensions, there was overall no significant differences between species. For L. pertusa, there was no significant difference between sites but the highest values (12 ± 13 mm y–1) were found at AA. For M. oculata, the non-cross transplanted corals at site B (mean of 10 ± 5 mm y–1 at BB) had significantly higher linear extension compared to corals from site A (mean of 6 ± 5 mm y–1 at AA, p < 0.05). However, there were no differences between cross transplanted and non-cross transplanted corals (Figure 2).
Coral Bacterial Communities
The in situ experiment showed that L. pertusa and M. oculata had different bacterial community composition (ANOSIM p < 0.001) (Figure 3). M. oculata’s bacterial communities did not differ between the two sites A and B (ANOSIM p > 0.05). The M. oculata transferred from site A to site B seemed to have a different microbiome composition, but as only two samples were available, it could not be conclusive (Figure 3). Inversely, the bacterial communities associated to L. pertusa differed between the sites A and B (ANOSIM p < 0.05). The coral microbiome composition of L. pertusa transferred from site A to site B (AB) was similar to the bacterial community composition of the corals growing at site B (ANOSIM p > 0.05) (Figure 3).
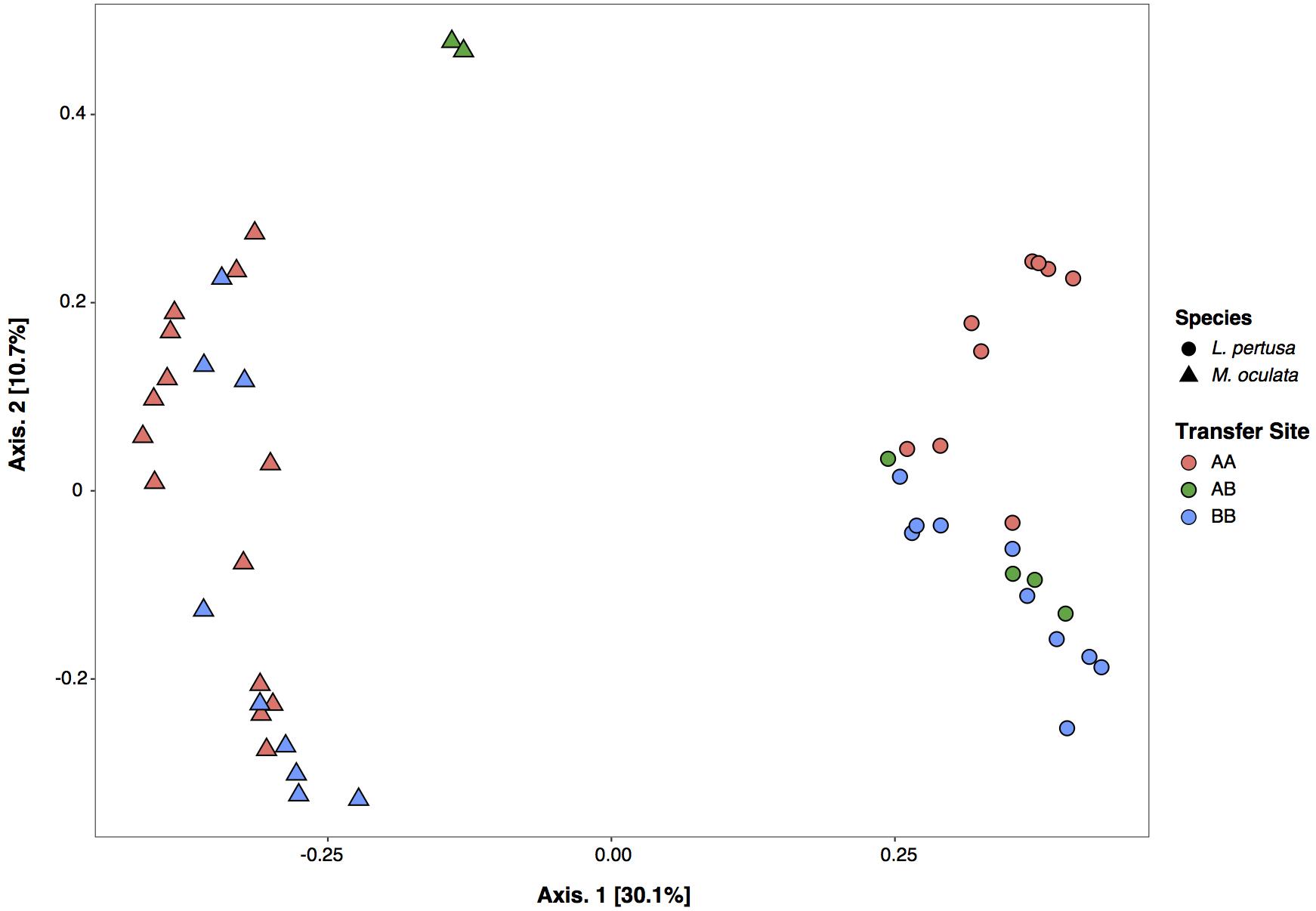
Figure 3. Correspondence analysis (CA) of bacterial community composition based on 16S rRNA gene for L. pertusa and M. oculata. AA, coral originating from site A and redeployed at site A; AB, coral from site A and redeployed to B; BB, coral from site B and redeployed to B.
At the phylum level, the M. oculata bacterial communities were mostly dominated by Spirochetae (ASV1) and Tenericutes (ASV2) with no significant differences observed between sites (ANOVA, p > 0.05) (Figure 4). Inversely, there was a difference between sites for L. pertusa. The deeper site A coral microbiome was characterized by the phylum Spirochetae (ASV4) and the shallower site B by Alphaproteobacteria (ASV3). Interestingly, the bacterial communities of L. pertusa transferred from site A to B (AB) were composed of the phylum characterizing site B (Figure 4).
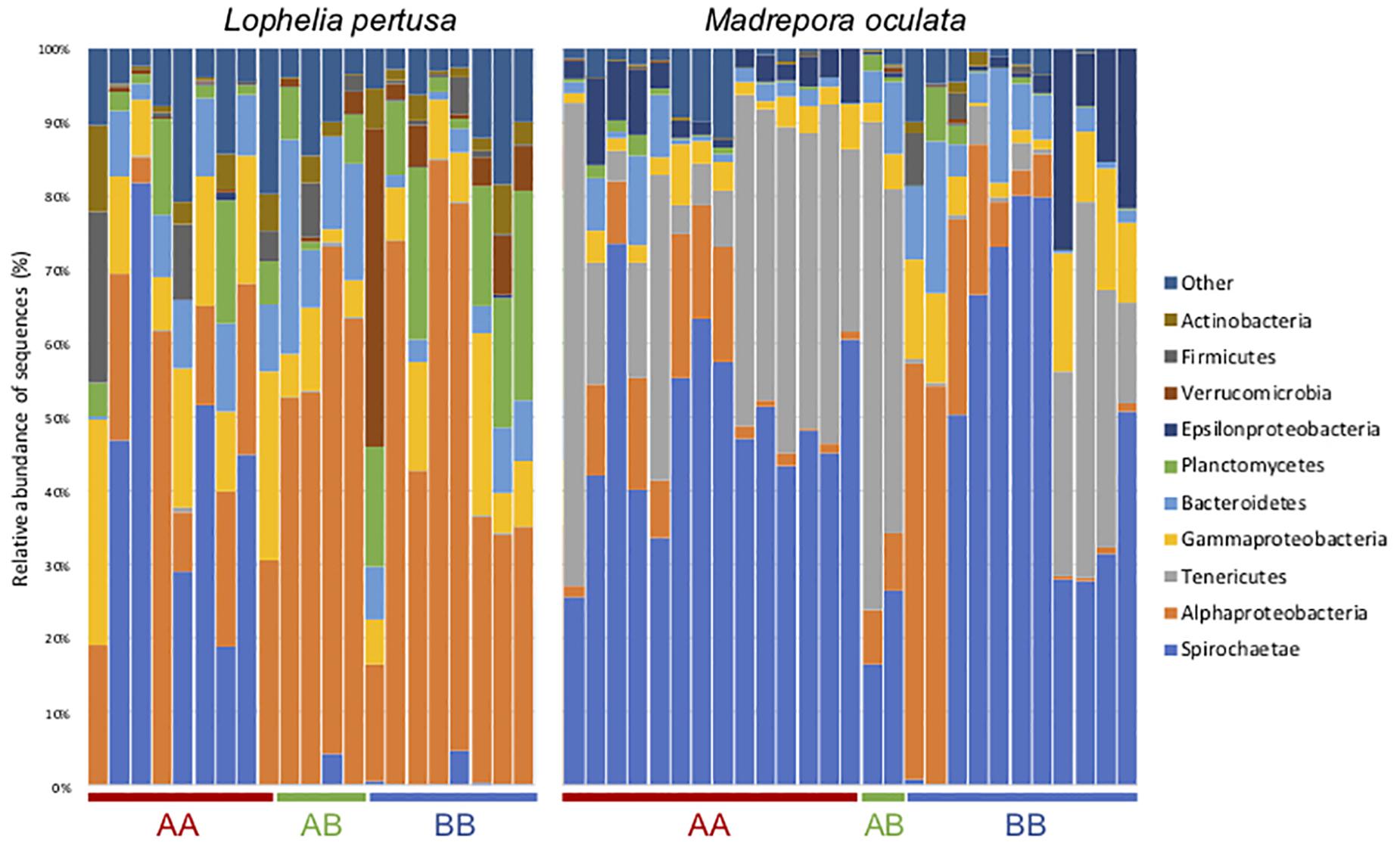
Figure 4. Bacterial community composition at the phylum/class level for L. pertusa and M. oculata from different transfer sites. AA, coral originating from site A and redeployed at site A; BA, coral from site B and redeployed to A; AB, coral from site A and redeployed to B; BB, coral from site B and redeployed to B. The 10 most abundant phyla are shown and the others are grouped as “Other.”
The Shannon diversity index (Shannon, 1948) showed no statistical differences between the deeper and the shallower sites, but it was significantly higher in L. pertusa compared to M. oculata (p < 0.001) (Figure 5).
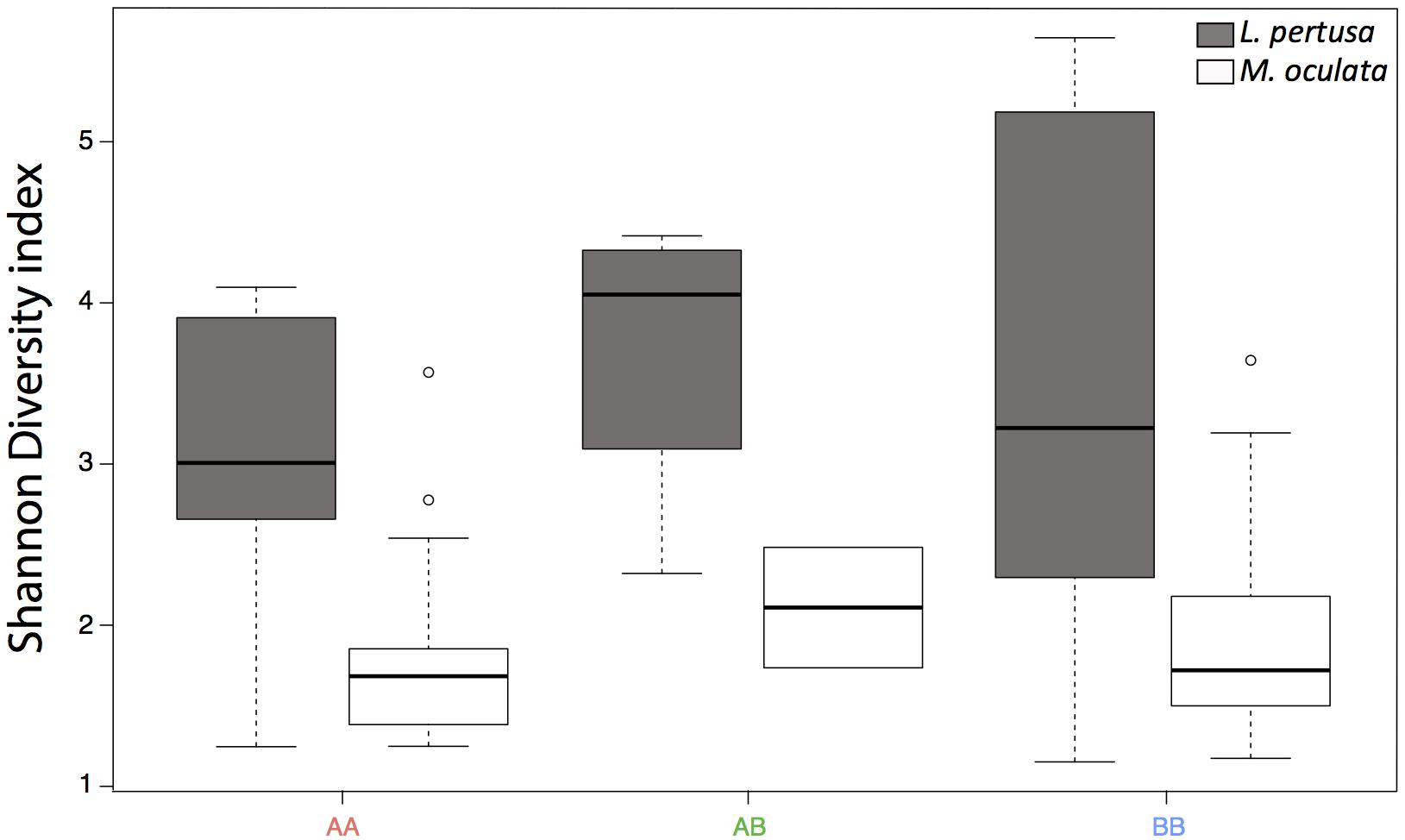
Figure 5. Shannon diversity index for L. pertusa (gray) and M. oculata (white) at the deeper A site and the shallower B site. AA, from site A and redeployed at site A; AB, from A and redeployed at B; BB, from B and redeployed at site B. The boxplots represent the median values, the quartiles, and the minimum and maximum.
We further identified at the ASV level the main bacteria present in each species and at each site by SIMPER analysis (Figure 6). The four most abundant ASVs represented more than 75% of the sequences in L. pertusa and M. oculata. The ASV1 (Order Spirochetales) and ASV2 (Order Entomoplasmatales) were significantly more abundant in M. oculata while ASV3 (Order Rickettsiales) and ASV4 (Order Spirochetales) were more abundant in L. pertusa microbiomes (Figure 6). Looking at sites, ASV4 appeared relatively more abundant at site A while ASV3 was dominant in corals growing at site B. Some of these ASVs were highly similar to sequences previously retrieved from CWCs and others were distantly related to sequences found in tropical corals (Supplementary Table S1). The ASV8 (Order Rickettsiales) was typical of the site B in both coral species (88% similarity to database sequences). The ASV19 (Order Spirochetales) was characteristic of M. oculata living at site A. ASV51 (Order Spirochetales) and ASV16 (Order Rickettsiales) were mostly present in the transplanted corals (AB) in both species. ASV16 was 100% similar to a sequence previously detected in L. pertusa (Supplementary Table S1). Finally, Endozoicomonas, a typical coral symbiont, was observed only in L. pertusa and M. oculata redeployed at their original site (Supplementary Figure S1).
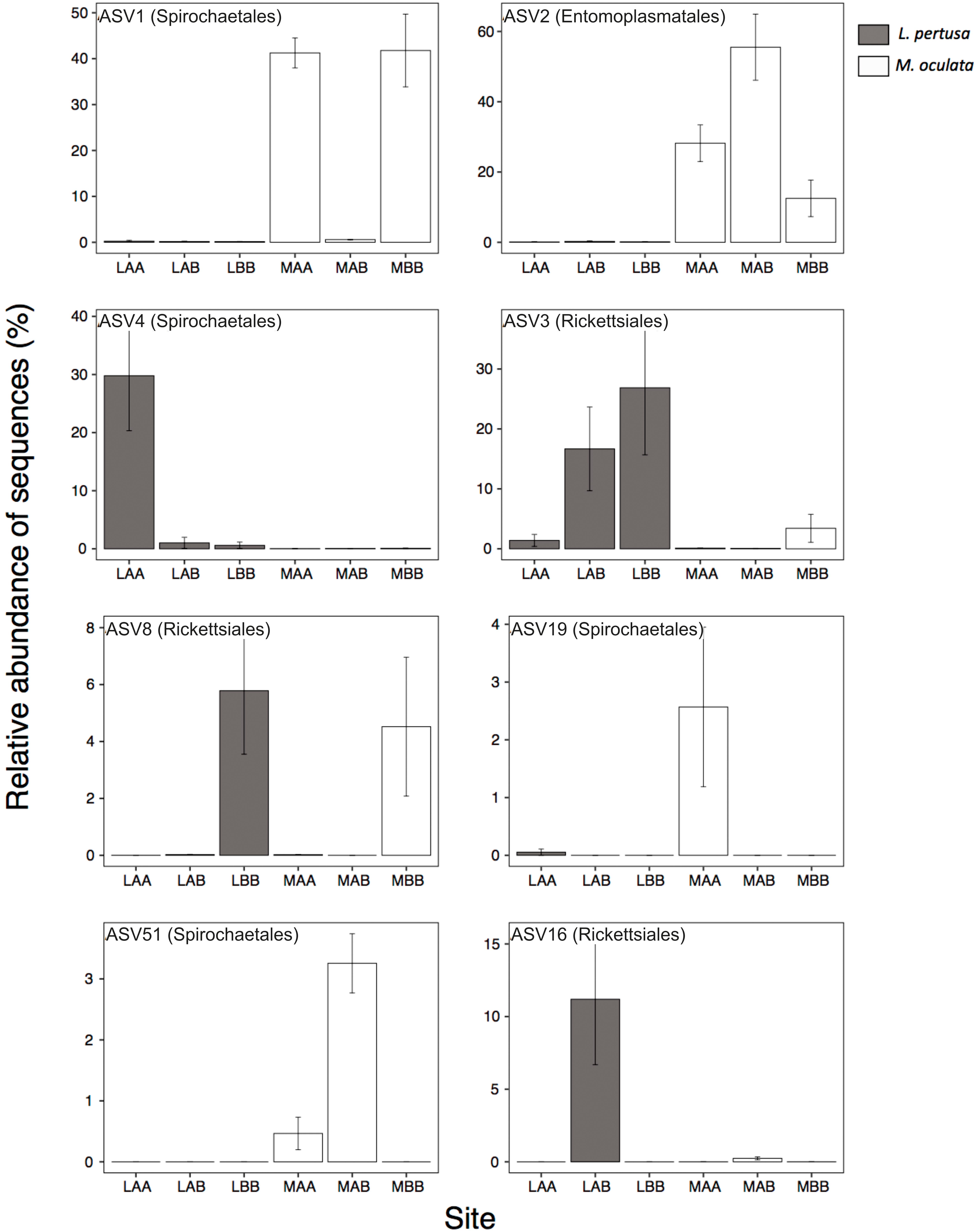
Figure 6. Relative proportion of the most abundant ASVs present in L. pertusa (gray) and M. oculata (white) for different transplant experiments (AA, AB, and BB). The affiliation of the ASVs at the order level is indicated in parentheses. A detailed taxonomic affiliation of each ASV is given in Supplementary Table S1. The mean ± SD is shown for each ASV.
Discussion
Our results cast a new light on the strength of the host-microbiome relationship in CWCs. Madrepora oculata, conserved overall its bacterial community composition at the ASV level across habitats, although some bacteria were only present at one of the sites. Inversely, L. pertusa had a more variable bacterial community composition that changed with habitat. Interestingly, cross transplanted L. pertusa microbiomes became similar in composition to the bacterial communities of the local L. pertusa corals. Differences in bacterial community composition have been observed earlier for L. pertusa and M. oculata at large geographical scale (Jensen et al., 2019) but also between L. pertusa from the Mediterranean Sea and a Norwegian fjord (Yakimov et al., 2006; Neulinger et al., 2008). At a smaller geographical scale, there were differences in bacterial community composition within the Trondheimsfjord, but they were not related to the distance between sites (Neulinger et al., 2008). The present observations of the acquisition of a local microbial signature by L. pertusa is new, and it opens new hypothesis about the corals’ acquisition of a microbiome. The geographical differences could result from a horizontal transfer of the bacterial community. Such transfer could give a plasticity to some coral microbiomes, which could change according to environmental conditions. This has been shown with the comparison of Eguchipsammia fistula microbiomes between in situ and aquarium samples (Röthig et al., 2017). Aquarium conditions were also recently shown to transform L. pertusa microbiome within a day (Galand et al., 2018). Inversely, M. oculata’s microbiome was maintained for at least 6 months in aquaria (Galand et al., 2018). Here we thus validate in situ the previous observations made in aquaria, showing that L. pertusa’s bacterial community can quickly change with a changing environment. The acquisition of new microbes by L. pertusa may also be due to differences in nutrition that may lead to biochemical and/or metabolic differences, which in turn influence the selection of different microbial communities (Dodds et al., 2007; Schöttner et al., 2012; Meistertzheim et al., 2016). The difference in bacterial community observed between L. pertusa and M. oculata sharing the same habitat agrees with former observations for these coral species (Hansson et al., 2009; Meistertzheim et al., 2016).
Among the bacterial taxa detected in the present study, the Entomoplasmatales (phylum Tenericutes), which are characterized by the lack of cell walls, were previously observed in association with gorgonian corals where they dominate the assemblage (Gray et al., 2011). These bacteria were also observed in Atlantic L. pertusa and are supposed to play a major role for the coral (Neulinger et al., 2008; Kellogg et al., 2009). For M. oculata, we detected Spirochetes, which have not been seen earlier in this coral species. This bacterial association has already been observed with L. pertusa (Kellogg et al., 2009; Meistertzheim et al., 2016), E. fistula (Röthig et al., 2017) and with gorgonian corals from the deep sea (Gray et al., 2011; Lawler et al., 2016; van de Water et al., 2016).
Here, we also report that L. pertusa acquired the coral-associated Rickettsiales when transferred to the shallower geographic location (B site). This bacterial order has already been observed in different gorgonian and coral species (Casas et al., 2004; Gray et al., 2011; Klinges et al., 2019). This microbe was also widespread in both healthy and diseased tropical corals and may induce white band disease (Gignoux-Wolfsohn and Vollmer, 2015). Moreover, this Rickettsiales-like prokaryote from the Alphaproteobacteria group, is a Gram-negative microbe and can cause disease in invertebrates (Antonio et al., 2000). Thus, we can make the hypothesis these Rickettsiales may be opportunistic bacteria, with potential pathogens, that affect the coral development. However, considering that we are at the taxonomic level of the Order, this hypothesis has to be carefully considered.
Our work based on an in situ transplant experiment revealed difference between species following habitats. Combining microbiome results with measures of coral mortality and skeletal growth, we can suggest that M. oculata has a habitat preference, the shallower B site. In contrast, the versatile microbiome and the stable skeletal growth suggested that L. pertusa may not have such a strict habitat preference. M. oculata showed higher growth rates (i.e., budding rate and linear extension) at site B compared to site A, and its transplantation to site A reduced its growth and increased mortality. This is in accordance with field observations showing that site A (deeper) is dominated by L. pertusa reefs whereas the site B (shallower) is dominated by M. oculata reefs (personal observations). Lophelia pertusa did not show significant difference in growth rate between sites, even though the highest values were always observed at site A.
The different habitat preferences may be associated to the different physiological characteristics of the two species coupled to the different hydrological features defining the two sites in the canyon. Lophelia pertusa and M. oculata exhibit distinct physiological functions, including skeletal growth rates (Lartaud et al., 2014), feeding behavior and preferences (Tsounis et al., 2010; Galand et al., 2020), reproduction cycles (Waller and Tyler, 2005), but also distinct associated microbial communities (Meistertzheim et al., 2016). Different patterns of distribution between L. pertusa and M. oculata have been reported in the Atlantic and Mediterranean regions where M. oculata is the dominant species in the mid slope while L. pertusa is more abundant in the down slope (Foubert et al., 2011; Fabri et al., 2017; Boolukos et al., 2019). Gori et al. (2013) also reported that M. oculata was more abundant at depths shallower than 500 m in the Mediterranean canyons Cap de Creus and Lacaze-Duthiers. These previous results together with our new data suggest that M. oculata has a strong preference for shallower habitats. This may be linked to different temperature range tolerance between species with a higher sensitivity of M. oculata to lower temperatures (Naumann et al., 2014).
Both Lophelia pertusa and Madrepora oculata feed mostly on zooplankton, but it has been demonstrated that they do not share the same metabolism (Gori et al., 2014). Analyses of lipid compositions, and their nitrogen isotopic signatures, suggest that the two species adopt different feeding strategies (Kiriakoulakis et al., 2005; Galand et al., 2020). Lophelia pertusa is a more opportunistic suspended feeder, so its capacity to catch enough prey to sustain growth may be higher compared to M. oculata (Mueller et al., 2014). This could explain the more widespread occurrence of L. pertusa reefs. In addition, it has been shown that CWC capture rates depend on current regimes (Purser et al., 2010). The hydrology of the Lacaze Duthiers canyon is driven by episodic dense water shelf cascades that overflow the slope and provide food to sessile organisms as the CWCs (Ulses et al., 2008; Canals et al., 2009). However, the different morpho-bathymetric sites of the canyon are not influenced by the same water currents, and thus, the availability of food transported from the surface varies following the slope (Sanchez-Vidal et al., 2008). The current regimes in the Lacaze Duthiers canyon also resuspend sediments that can cover the living polyps (Durrieu de Madron et al., 2005). Lophelia pertusa has a great tolerance to sediments and drill cuttings (Larsson et al., 2013), and may thus thrive in impacted sites. Inversely, M. oculata may be more sensitive to suspended materials and may better develop at the head of the canyon on vertical cliffs, where strong currents wash away sediments. This is in accordance with earlier reports suggesting that M. oculata settles preferentially on areas with high topography and strong but irregular water current (Fabri et al., 2017).
Conclusion
We identified, through a transplant experiment, changes in L. pertusa’s coral microbiome composition between two sites and the acquisition of a local coral microbial signature after transplant. This transplantation did not affect L. pertusa’s skeletal growth rates but the transfer induced the acquisition of bacteria in the shallower site. Inversely, M. oculata’s microbiome did not change with transplantation, but its skeletal growth was reduced. Our results suggest a higher plasticity of L. pertusa microbiome, which could explain its success across sites contrary to M. oculata which seem to harbor a habitat preference. Thus, in the context of ongoing climate change in Mediterranean Sea, both species may show different responses to changing environmental conditions.
Data Availability Statement
The datasets generated for this study can be found in the PRJNA563840.
Author Contributions
LC, NL, FL, and PG designed the study. LC, FL, and PG analyzed the data. LC, PG, NL, EP, and FL wrote the manuscript.
Funding
This work was supported by the experimentation program of the Chair “Biodiversity, Extreme Marine Environment and Global Change” supported by Foundation TOTAL, UPMC, and CNRS, Coord. NL (LECOB). LC’s Ph.D. grant was funded by the French Ministry of Higher Education, Research, and Innovation through the Doctoral School “Sciences de l’Environnement d’Ile de France – ED129.” PG research was funded by the Agence Nationale de la Recherche (ANR) through the project EUREKA (ANR-14-CE02-0004-01).
Conflict of Interest
The authors declare that the research was conducted in the absence of any commercial or financial relationships that could be construed as a potential conflict of interest.
Acknowledgments
We thank the crew RV “Minibex” (Comex) for their assistance in fieldwork. We acknowledge the technical support of the Marine Biodiversity and Biotechnology (Bio2Mar) platform from the Banyuls Oceanological Observatory for DNA extraction.
Supplementary Material
The Supplementary Material for this article can be found online at: https://www.frontiersin.org/articles/10.3389/fmicb..00275/full#supplementary-material
References
Addamo, A. M., Vertino, A., Stolarski, J., Garcìa-Jiménez, R., Taviani, M., and Machordom, A. (2016). Merging scleractinian genera: the overwhelming genetic similarity between solitary Desmophyllum and colonial Lophelia. BMC Evol. Biol. 16:108. doi: 10.1186/s12862-016-0654-8
Altschul, S. F., Gish, W., Miller, W., Myers, E. W., and Lipman, D. J. (1990). Basic local alignment search tool. J. Mol. Biol. 215, 403–410.
Antonio, D. B., Andree, K. B., Moore, J. D., Friedman, C. S., and Hedrick, R. P. (2000). Detection of rickettsiales-like prokaryotes by in situ hybridization in black abalone, Haliotis cracherodii, with withering syndrome. J. Invert. Pathol. 75, 180–182. doi: 10.1006/jipa.1999.4906
Boolukos, C. M., Lim, A., O’Riordan, R. M., and Wheeler, A. J. (2019). Cold-water corals in decline – A temporal (4 year) species abundance and biodiversity appraisal of complete photomosaiced cold-water coral reef on the Irish Margin. Deep Sea Res. I 146, 44–54. doi: 10.1016/j.dsr.2019.03.004
Bourne, D. G., Morrow, K. M., and Webster, N. S. (2016). Insights into the coral microbiome: underpinning the health and resilience of reef ecosystems. Ann. Rev. Microbiol. 70, 317–340. doi: 10.1146/annurev-micro-102215-095440
Bray, J. R., and Curtis, J. T. (1957). An ordination of upland forest communities of southern wisconsin.-ecological monographs. J. Ecol. Monogr. 27, 325–349. doi: 10.2307/1942268
Buhl-Mortensen, L., Vanreusel, A., Gooday, A. J., Levin, L. A., Priede, I. G., Buhl-Mortensen, P., et al. (2010). Biological structures as a source of habitat heterogeneity and biodiversity on the deep ocean margins: biological structures and biodiversity. Mar. Ecol. 31, 21–50. doi: 10.1111/j.1439-0485.2010.00359.x
Callahan, B. J., McMurdie, P. J., Rosen, M. J., Han, A. W., Johnson, A. J. A., and Holmes, S. P. (2016). DADA2: high-resolution sample inference from Illumina amplicon data. Nat. Methods 13, 581–583. doi: 10.1038/nmeth.3869
Canals, M., Danovaro, R., Heussner, S., Lykousis, V., Puig, P., Trincardi, F., et al. (2009). Cascades in mediterranean submarine grand canyons. Oceanography 22, 26–43. doi: 10.5670/oceanog.2009.03
Canals, M., Puig, P., de Madron, X. D., Heussner, S., Palanques, A., and Fabres, J. (2006). Flushing submarine canyons. Nature 444, 354–357. doi: 10.1038/nature05271
Casas, V., Kline, D. I., Wegley, L., Yu, Y., Breitbart, M., and Rohwer, F. (2004). Widespread association of a Rickettsiales-like bacterium with reef-building corals. Environ. Microbiol. 6, 1137–1148. doi: 10.1111/j.1462-2920.2004.00647.x
Dodds, L. A., Roberts, J. M., Taylor, A. C., and Marubini, F. (2007). Metabolic tolerance of the cold-water coral Lophelia pertusa (Scleractinia) to temperature and dissolved oxygen change. J. Exp. Mar. Biol. Ecol. 349, 205–214. doi: 10.1016/j.jembe.2007.05.013
Durrieu de Madron, X., Zervakis, V., Theocharis, A., and Georgopoulos, D. (2005). Comments on “Cascades of dense water around the world ocean.”. Prog. Oceanogr. 64, 83–90. doi: 10.1016/j.pocean.2004.08.004
Fabri, M.-C., Bargain, A., Pairaud, I., Pedel, L., and Taupier-Letage, I. (2017). Cold-water coral ecosystems in cassidaigne canyon: an assessment of their environmental living conditions. Deep Sea Res. II Top. Stud. Oceanogr. 137, 436–453. doi: 10.1016/j.dsr2.2016.06.006
Fiala, A., Reyss, D., Romans, P., Madurell, T., Pibot, A., Watremez, P., et al. (2010). Recent Observations Of Dense Living Cold-Water Coral Communities in the ≪Lacaze-Duthiers≫ Canyon (Western Mediterranean Sea). Available at: http://www.ciesm.org/online/archives/abstracts/pdf/39/PG_0516.pdf (accessed July 26, 2017).
Foley, N., and Armstrong, C. W. (2010). The Ecological and Economic Value of Cold-Water Coral Ecosystems. Available at: http://munin.uit.no/handle/10037/3813 (accessed July 26, 2017).
Foubert, A., Huvenne, V. A. I., Wheeler, A., Kozachenko, M., Opderbecke, J., and Henriet, J.-P. (2011). The moira mounds, small cold-water coral mounds in the porcupine Seabight, NE Atlantic: part B—evaluating the impact of sediment dynamics through high-resolution ROV-borne bathymetric mapping. Mar. Geol. 282, 65–78. doi: 10.1016/j.margeo.2011.02.008
Freiwald, A. (2002). “Reef-forming cold-water corals,” in Ocean Margin Systems, eds G. Wefer, D. Billett, D. Hebbeln, B. B. Jørgensen, M. Schlüter, and T. C. E. van Weering (Berlin: Springer), 365–385. doi: 10.1007/978-3-662-05127-6-23
Freiwald, A., Beuck, L., Rüggeberg, A., Taviani, M., and Hebbeln, D. (2009). The white coral community in the central Mediterranean Sea revealed by ROV surveys. Oceanography 22, 58–74. doi: 10.5670/oceanog.2009.06
Freiwald, A., Fossa, J. H., Grehan, A., Koslow, T., and Roberts, J. M. (2004). Cold Water Coral Reefs: Out of Sight-No Longer Out of Mind. Available at: http://wedocs.unep.org/handle/20.500.11822/8727 (accessed July 26, 2017).
Galand, P. E., Chapron, L., Meistertzheim, A.-L., Peru, E., and Lartaud, F. (2018). The effect of captivity on the dynamics of active bacterial communities differs between two deep-sea coral species. Front. Microbiol. 9:2565. doi: 10.3389/fmicb.2018.02565
Galand, P. E., Remize, M., Meistertzheim, A. L., Pruski, A. M., Peru, E., Suhrhoff, T. J., et al. (2020). Diet shapes cold-water corals bacterial communities. Environ. Microbiol. 22, 354–368. doi: 10.1111/1462-2920.14852
Gignoux-Wolfsohn, S. A., and Vollmer, S. V. (2015). Identification of candidate coral pathogens on white band disease-infected staghorn coral. PLoS One 10:e0134416. doi: 10.1371/journal.pone.0142760
Gori, A., Grover, R., Orejas, C., Sikorski, S., and Ferrier-Pagès, C. (2014). Uptake of dissolved free amino acids by four cold-water coral species from the Mediterranean Sea. Deep Sea Res. II Top. Stud. Oceanogr. 99, 42–50. doi: 10.1016/j.dsr2.2013.06.007
Gori, A., Orejas, C., Madurell, T., Bramanti, L., Martins, M., Quintanilla, E., et al. (2013). Bathymetrical distribution and size structure of cold-water coral populations in the Cap de Creus and Lacaze-duthiers canyons (northwestern Mediterranean). Biogeosciences 10, 2049–2060. doi: 10.5194/bg-10-2049-2013
Gray, M. A., Stone, R. P., McLaughlin, M. R., and Kellogg, C. A. (2011). Microbial consortia of gorgonian corals from the Aleutian islands: microbial consortia of aleutian gorgonians. FEMS Microbiol. Ecol. 76, 109–120. doi: 10.1111/j.1574-6941.2010.01033.x
Hansson, L., Agis, M., Maier, C., and Weinbauer, M. (2009). Community composition of bacteria associated with cold-water coral Madrepora oculata: within and between colony variability. Mar. Ecol. Prog. Ser. 397, 89–102. doi: 10.3354/meps08429
Jensen, S., Hovland, M., Lynch, M. D. J., and Bourne, D. G. (2019). Diversity of deep-water coral-associated bacteria and comparison across depth gradients. FEMS Microbiol. Ecol. 95:fiz091. doi: 10.1093/femsec/fiz091
Kellogg, C. A. (2019). Microbiomes of stony and soft deep-sea corals share rare core bacteria. Microbiome 7:90. doi: 10.1186/s40168-019-0697-3
Kellogg, C. A., Goldsmith, D. B., and Gray, M. A. (2017). Biogeographic comparison of Lophelia-associated bacterial communities in the Western Atlantic reveals conserved core microbiome. Front. Microbiol. 8:796. doi: 10.3389/fmicb.2017.00796
Kellogg, C. A., Lisle, J. T., and Galkiewicz, J. P. (2009). Culture-independent characterization of bacterial communities associated with the cold-water coral Lophelia pertusa in the Northeastern Gulf of Mexico. Appl. Environ. Microbiol. 75, 2294–2303. doi: 10.1128/AEM.02357-08
Kiriakoulakis, K., Fisher, E., Wolff, G. A., Freiwald, A., Grehan, A., and Roberts, J. M. (2005). “Lipids and nitrogen isotopes of two deep-water corals from the North-East Atlantic: initial results and implications for their nutrition,” in Cold-Water Corals and Ecosystems. Erlangen Earth Conference Series, eds A. Freiwald and J. M. Roberts (Berlin: Springer), 715–729. doi: 10.1007/3-540-27673-4_37
Klinges, J. G., Rosales, S. M., McMinds, R., Shaver, E. C., Shantz, A. A., Peters, E. C., et al. (2019). Phylogenetic, genomic, and biogeographic characterization of a novel and ubiquitous marine invertebrate-associated rickettsiales parasite, Candidatus Aquarickettsia rohweri, gen. nov., sp. nov. ISME J. 13, 2938–2953. doi: 10.1038/s41396-019-0482-0
Lane, D. J. (1991). “16S/23S rRNA sequencing,” in Nucleic Acid Techniques in Bacterial Systematics, eds E. Stackebrandt and M. Goodfellow (New York, NY: Wiley), 115–175.
Lane, D. J., Pace, B., Olsen, G. J., Stahl, D. A., Sogin, M. L., Pace, N. R., et al. (1985). Rapid determination of 16S ribosomal RNA sequences for phylogenetic analyses. Proc. Natl. Acad. Sci. U.S.A. 82, 6955–6959. doi: 10.1073/pnas.82.20.6955
Larsson, A. I., van Oevelen, D., Purser, A., and Thomsen, L. (2013). Tolerance to long-term exposure of suspended benthic sediments and drill cuttings in the cold-water coral Lophelia pertusa. Mar. Pollut. Bull. 70, 176–188. doi: 10.1016/j.marpolbul.2013.02.033
Lartaud, F., Meistertzheim, A. L., Peru, E., and Le Bris, N. (2017). In situ growth experiments of reef-building cold-water corals: the good, the bad and the ugly. Deep Sea Res. I Oceanogr. Res. Pap. 121, 70–78. doi: 10.1016/j.dsr.2017.01.004
Lartaud, F., Mouchi, V., Chapron, L., Meistertzheim, A. L., and Le Bris, N. (2019). “36 Growth patterns of mediterranean calcifying cold-water corals,” in Mediterranean Cold-Water Corals: Past, Present and Future. Coral Reefs of the World, Vol. 9, eds C. Orejas and C. Jiménez (Cham: Springer), doi: 10.1007/978-3-319-91608-8-36
Lartaud, F., Pareige, S., de Rafelis, M., Feuillassier, L., Bideau, M., Peru, E., et al. (2014). Temporal changes in the growth of two Mediterranean cold-water coral species, in situ and in aquaria. Deep Sea Res. II Top. Stud. Oceanogr. 99, 64–70. doi: 10.1016/j.dsr2.2013.06.024
Lawler, S. N., Kellogg, C. A., France, S. C., Clostio, R. W., Brooke, S. D., and Ross, S. W. (2016). Coral-associated bacterial diversity is conserved across two deep-sea Anthothela species. Front. Microbiol. 7:458. doi: 10.3389/fmicb.2016.00458
Meistertzheim, A.-L., Lartaud, F., Arnaud-Haond, S., Kalenitchenko, D., Bessalam, M., Le Bris, N., et al. (2016). Patterns of bacteria-host associations suggest different ecological strategies between two reef building cold-water coral species. Deep Sea Res. I Oceanogr. Res. Pap. 114, 12–22. doi: 10.1016/j.dsr.2016.04.013
Mueller, C. E., Larsson, A. I., Veuger, B., Middelburg, J. J., and van Oevelen, D. (2014). Opportunistic feeding on various organic food sources by the cold-water coral Lophelia pertusa. Biogeosciences 11, 123–133. doi: 10.5194/bg-11-123-2014
Naumann, M. S., Orejas, C., and Ferrier-Pagès, C. (2014). Species-specific physiological response by the cold-water corals Lophelia pertusa and Madrepora oculata to variations within their natural temperature range. Deep Sea Res. II Top. Stud. Oceanogr. 99, 36–41. doi: 10.1016/j.dsr2.2013.05.025
Neave, M. J., Apprill, A., Ferrier-Pagès, C., and Voolstra, C. R. (2016). Diversity and function of prevalent symbiotic marine bacteria in the genus Endozoicomonas. Appl. Microbiol. Biotechnol. 100, 8315–8324. doi: 10.1007/s00253-016-7777-0
Neulinger, S. C., Jarnegren, J., Ludvigsen, M., Lochte, K., and Dullo, W.-C. (2008). Phenotype-specific bacterial communities in the cold-water coral Lophelia pertusa (Scleractinia) and their implications for the coral’s nutrition, health, and distribution. Appl. Environ. Microbiol. 74, 7272–7285. doi: 10.1128/AEM.01777-08
Oksanen, J., Blanchet, F. G., Kindt, R., Legendre, P., Minchin, R. P., O’Hara, R., et al. (2013). Vegan: Community Ecology Package. R Package Version. 2.0-10.
Purser, A., Larsson, A. I., Thomsen, L., and van Oevelen, D. (2010). The influence of flow velocity and food concentration on Lophelia pertusa (Scleractinia) zooplankton capture rates. J. Exp. Mar. Biol. Ecol. 395, 55–62. doi: 10.1016/j.jembe.2010.08.013
Quast, C., Pruesse, E., Yilmaz, P., Gerken, J., Schweer, T., Yarza, P., et al. (2012). The SILVA ribosomal RNA gene database project: improved data processing and web-based tools. Nucleic Acids Res. 41, D590–D596. doi: 10.1093/nar/gks1219
Roberts, J. M., Wheeler, A. J., and Freiwald, A. (2006). Reefs of the deep: the biology and geology of cold-water coral ecosystems. Science 312, 543–547. doi: 10.1126/science.1119861
Röthig, T., Roik, A., Yum, L. K., and Voolstra, C. R. (2017). Distinct bacterial microbiomes associate with the deep-sea coral Eguchipsammia fistula from the Red Sea and from aquaria settings. Front. Mar. Sci. 4:259. doi: 10.3389/fmars.2017.00259
Sanchez-Vidal, A., Pasqual, C., Kerhervé, P., Calafat, A., Heussner, S., Palanques, A., et al. (2008). Impact of dense shelf water cascading on the transfer of organic matter to the deep western Mediterranean basin. Geophys. Res. Lett. 35:32825. doi: 10.1029/2007GL032825
Schöttner, S., Wild, C., Hoffmann, F., Boetius, A., and Ramette, A. (2012). Spatial scales of bacterial diversity in cold-water coral reef ecosystems. PLoS One 7:e32093. doi: 10.1371/journal.pone.0032093
Schröder-Ritzrau, A., Freiwald, A., and Mangini, A. (2005). “U/Th-dating of deep-water corals from the eastern north Atlantic and the Western Mediterranean Sea,” in Cold-water Corals and Ecosystems, eds A. Freiwald and J. M. Roberts (Berlin: Springer), 157–172. doi: 10.1007/3-540-27673-4_8
Tsounis, G., Orejas, C., Reynaud, S., Gili, J.-M., Allemand, D., and Ferrier-Pagès, C. (2010). Prey-capture rates in four Mediterranean cold water corals. Mar. Ecol. Prog. Ser. 398, 149–155. doi: 10.3354/meps08312
Ulses, C., Estournel, C., Bonnin, J., Durrieu de Madron, X., and Marsaleix, P. (2008). Impact of storms and dense water cascading on shelf-slope exchanges in the Gulf of Lion (NW Mediterranean). J. Geophys. Res. 113:C003795. doi: 10.1029/2006JC003795
van de Water, J., Melkonian, R., Junca, H., Voolstra, C. R., Reynaud, S., Allemand, D., et al. (2016). Spirochaetes dominate the microbial community associated with the red coral Corallium rubrum on a broad geographic scale. Sci. Rep. 6:27277. doi: 10.1038/srep27277
Waller, R. G., and Tyler, P. A. (2005). The reproductive biology of two deep-water, reef-building scleractinians from the NE Atlantic Ocean. Coral Reefs 24, 514–522. doi: 10.1007/s00338-005-0501-7
Yakimov, M. M., Cappello, S., Crisafi, E., Tursi, A., Savini, A., Corselli, C., et al. (2006). Phylogenetic survey of metabolically active microbial communities associated with the deep-sea coral Lophelia pertusa from the Apulian plateau, Central Mediterranean Sea. Deep Sea Res. I Oceanogr. Res. Pap. 53, 62–75. doi: 10.1016/j.dsr.2005.07.005
Keywords: Lophelia pertusa, Madrepora oculata, DNA, bacteria, skeletal growth rates, Mediterranean canyon
Citation: Chapron L, Lartaud F, Le Bris N, Peru E and Galand PE (2020) Local Variability in Microbiome Composition and Growth Suggests Habitat Preferences for Two Reef-Building Cold-Water Coral Species. Front. Microbiol. 11:275. doi: 10.3389/fmicb.2020.00275
Received: 16 October 2019; Accepted: 06 February 2020;
Published: 21 February 2020.
Edited by:
Sébastien Duperron, Muséum National d’Histoire Naturelle, FranceReviewed by:
Christina A. Kellogg, United States Geological Survey (USGS), United StatesSigmund Jensen, University of Bergen, Norway
Copyright © 2020 Chapron, Lartaud, Le Bris, Peru and Galand. This is an open-access article distributed under the terms of the Creative Commons Attribution License (CC BY). The use, distribution or reproduction in other forums is permitted, provided the original author(s) and the copyright owner(s) are credited and that the original publication in this journal is cited, in accordance with accepted academic practice. No use, distribution or reproduction is permitted which does not comply with these terms.
*Correspondence: Pierre E. Galand, cGllcnJlLmdhbGFuZEBvYnMtYmFueXVscy5mcg==