- 1UCIBIO, Departamento Ciências da Vida, Faculdade de Ciências e Tecnologia, Universidade Nova de Lisboa, Campus de Caparica, Caparica, Portugal
- 2UCIBIO, Departamento de Química, Faculdade de Ciências e Tecnologia, Universidade Nova de Lisboa, Campus de Caparica, Caparica, Portugal
Biofilms are generally defined as communities of cells involved in a self-produced extracellular matrix adhered to a surface. In biofilms, the bacteria are less sensitive to host defense mechanisms and antimicrobial agents, due to multiple strategies, that involve modulation of gene expression, controlled metabolic rate, intercellular communication, composition, and 3D architecture of the extracellular matrix. These factors play a key role in streptococci pathogenesis, contributing to therapy failure and promoting persistent infections. The species of the pyogenic group together with Streptococcus pneumoniae are the major pathogens belonging the genus Streptococcus, and its biofilm growth has been investigated, but insights in the genetic origin of biofilm formation are limited. This review summarizes pyogenic streptococci biofilms with details on constitution, formation, and virulence factors associated with formation.
Introduction
In nature, bacteria can exist in the planktonic form, where cells live freely in solution, but the sessile form, where a community grows enclosed in an extracellular polymer matrix while attached to a biotic or abiotic surface, is far more common (Donlan, 2002). This phenotype corresponds to a biofilm and can occur on virtually all types of surfaces (Garrett et al., 2008; Flemming and Wingender, 2010; Jamal et al., 2018). The biofilm consists of a tri-dimensional complex community of genetically similar or distinct cells that produce a matrix of extracellular polymeric substances (EPS), which accounts for 80% of the structures. The EPS contains a mixture of alginates, extracellular teichoic acid (TA), proteins, poly-N-acetyl glucosamine, lipids, phospholipids, polysaccharides, and extracellular DNA (e-DNA). About 97% of the EPS is composed of water, which is found as a solvent, dictating viscosity and mobility (Sutherland, 2001; Lu and Collins, 2007; Flemming and Wingender, 2010; Kumar et al., 2017; Jamal et al., 2018).
Biofilm formation is part of a defense mechanism that bacteria adopt to achieve a favorable environment, keep nutrients and increase the chances of survival (Chen and Wen, 2011; Young et al., 2016; Del Pozo, 2018; Jamal et al., 2018; Khatoon et al., 2018).
It is known that biofilms play an important role in streptococci pathogenesis, namely species that belong to the microbiota flora of animals and humans, but also species that are restricted human pathogens. These can cause opportunistic infections, that under appropriate conditions give rise to localized and systemic infections, with considerable implications on public health and veterinary industry (Pires et al., 2005; Rato et al., 2013; Barnett et al., 2015; Peters, 2017). Streptococci are divided into groups based on 16S rRNA gene sequence analysis: “Pyogenic,” “Sanguis,” “Bovis,” “Mutans,” “Mitis,” “Anginosus,” and “Salivarius” (Lal et al., 2011). Afterward, the “Downei” group was created to accommodate Streptococcus downei and Streptococcus criceti. However, some relationships between groups are not fully understood. This probably reflects the effect of horizontal gene transfer (HGT) during the early diversification of these clusters (Richards et al., 2014). In Table 1, a description of Streptococcus species with clinical and veterinary relevance is presented. The pyogenic Streptococci as Streptococcus pyogenes, Streptococcus agalactiae, Streptococcus dysgalactiae subsp. dysgalactiae (SDSD), and Streptococcus dysgalactiae subsp. equisimilis (SDSE), together with Streptococcus pneumoniae are the major pathogens belonging the genus Streptococcus (Parks et al., 2015).
Pyogenic bacteria are responsible for causing purulent respiratory tract and skin infections; among these are pharyngitis, septicemia, necrotizing fasciitis, bacteremia, meningitis, pneumonia, septic arthritis, rheumatic streptococcal toxic shock syndrome, acute rheumatic fever/rheumatic heart disease, scarlet fever, as described in Table 1 and references therein. The actual burden of pyogenic infections is difficult to trace due to the variety of settings and the level of severity of the manifestations, which can go from mild symptoms as sore throat, escalating to severe, life-threatening, conditions. According to Carapetis and coworkers 2005 estimation, “there are at least 517 000 deaths each year due to severe Streptococcus pyogens diseases” (Carapetis et al., 2005).
Biofilm growth of streptococci has been investigated, but insights in the genetic origin of biofilm formation are limited. Although most pyogenic streptococci are able to develop biofilms, there is substantial heterogeneity in biofilm formation among individual strains (Konto-Ghiorghi et al., 2009; Trappetti et al., 2011; Marks et al., 2014a; Genteluci et al., 2015; Rosini and Margarit, 2015; Young et al., 2016; Alves-Barroco et al., 2019). Avoiding failure of antimicrobial therapy in the pyogenic streptococcal group requires an in depth knowledge of these singularities and the integration of chemical and physical methods to prevent/control/eradicate the biofilm formation. This review summarizes pyogenic streptococci biofilms with details on the constitution, formation, and virulence factors associated with formation.
Biofilms Formation and Dispersal
Biofilm formation is a complex multi-step process, where adhesive and disruptive forces interplay (Jefferson, 2004; Chen and Wen, 2011; Young et al., 2016; Del Pozo, 2018). The formation of a biofilm is typically described in three stages: (a) initiation, where attachment occurs (which can be reversible and irreversible), (b) maturation, with microcolonies development, and (c) dispersal, where cells detach (Donlan, 2001; Garrett et al., 2008; Kumar et al., 2017; Jamal et al., 2018; Khatoon et al., 2018; Figure 1).
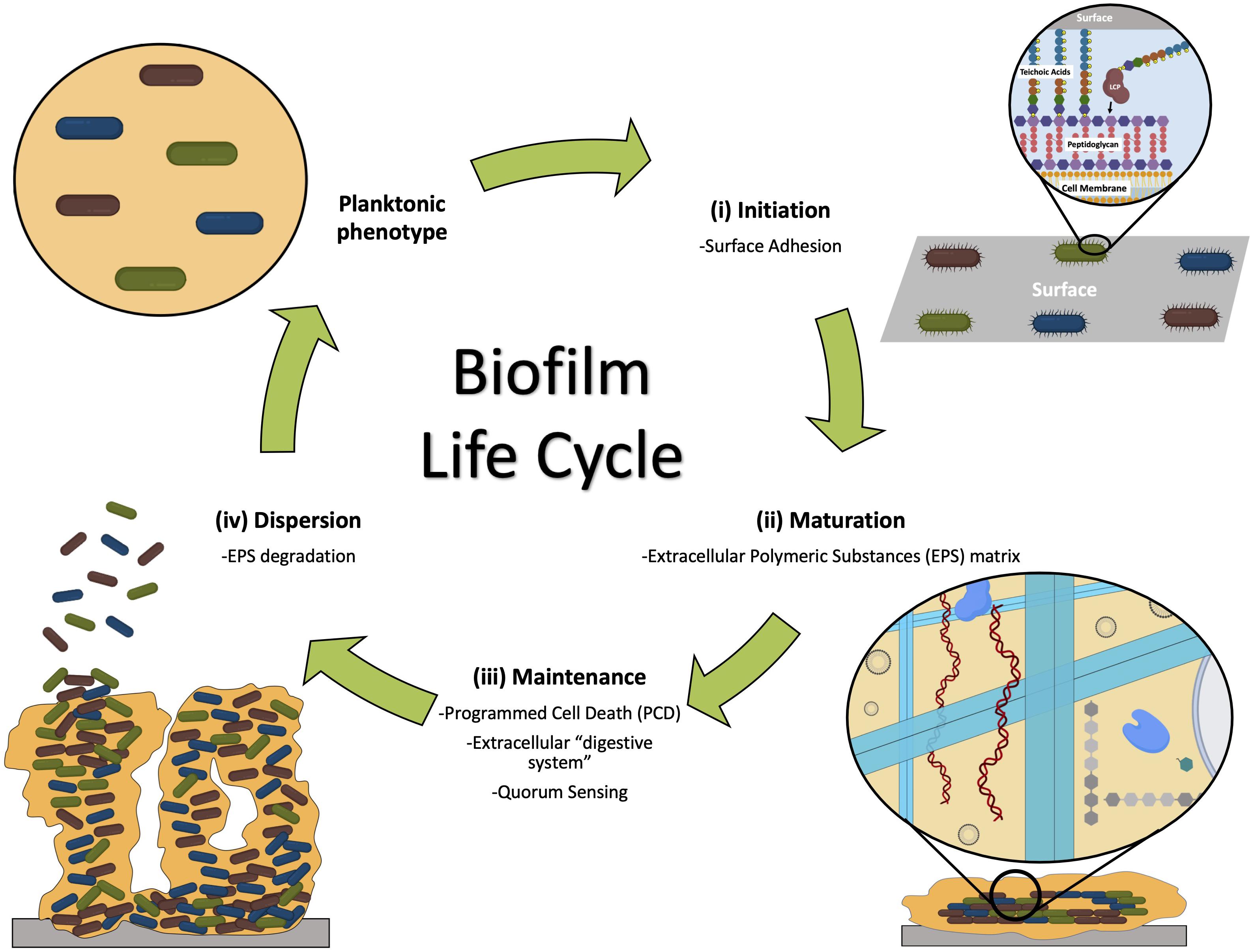
Figure 1. The three stages of biofilm formation and main events involved: (i) initiation, when bacteria attach to the surface; (ii) maturation, when microcolonies are formed, adhered to the surface and embedded by the EPS matrix; and (iii) dispersion, when some members of the community are released from the biofilm entering the planktonic phenotype. Maintenance of the biofilm ensures the community survival (Vasudevan, 2014).
Several streptococci virulence factors have been described as associated with biofilms formation (Table 2). In the next sections we will highlight some of them.
Regulatory Factors That Control Biofilm Development
Quorum-sensing (QS) is an intercellular communication system used by bacteria to control social behavior, intrinsically dependent on cell density. QS regulates both cooperation and competition within an interspecies bacterial community (Abisado et al., 2018). Several products, as proteases or toxins, are produced by individual cells but can be used by any member of the community, in a common good philosophy. QS has been implicated in all stages of biofilm development, namely by modulating initiation, maturation and dispersal (Parsek and Greenberg, 2005; Dickschat, 2010). Generally, QS relies on the production and sensing of extracellular signals. These systems are composed of two proteins and a signaling molecule; one of the proteins generates the signaling molecule, and the other protein acts as a sensor, activating the expression of other proteins. In the case of Gram-positive bacteria, the signal can be a linear or cyclic peptide, while the sensor is a two-component signal transduction system composed of a membrane-bound histidine kinase, and an intracellular response regulator (Kleerebezem et al., 1997).
In Streptococcus, the QS systems can be categorized into three main types: (i) Regulator of glucosyltransferase (Rgg), (ii) Streptococcal invasion locus (Sil), and (iii) LuxS/AI-2.
The Rgg family is composed of transcription regulators widely disseminated among Gram-positive bacteria, that respond to signaling peptides. These have been shown to modulate genetic determinants associated with virulence, competence, and biofilm establishment, maturation and dispersion in S. pyogenes, S. pneumonia, Streptococcus gordonii, Streptococcus mutans, and Streptococcus intermedius (Loo et al., 2000; Li et al., 2002; Petersen et al., 2004; Suntharalingam and Cvitkovitch, 2005; Mashburn-Warren et al., 2010; Junges et al., 2017). S. pyogenes contains four Rgg paralogs: RopB (Rgg1), Rgg2, Rgg3, and ComR (Rgg4) (Cook et al., 2013). RopB, the most studied Rgg, is required for transcription of the streptococcal pyrogenic exotoxin B (speB) gene that targets several different host proteins important for resistance (namely interleukins, antimicrobial peptides, and components of the extracellular matrix). The highest expression levels of speB are observed in the stationary phase, which suggests that gene activation by RopB requires high cell density (Cook et al., 2013). The role of the Rgg2 and Rgg3 systems to the S. pyogenes life cycle and pathogenesis has not been completely elucidated. Studies have demonstrated that these regulators respond to small hydrophobic peptides – SHP2 and SHP3, controlling differential gene expression and biofilm development. S. pyogenes Rgg2 is an activator of genes, while Rgg3 inactivate its expression; they compete for binding to the same regions on gene promoters, upstream of shp2 and shp3. In this way, Rgg2 and Rgg3 regulate the same function in antagonistic ways (Chang et al., 2011; Lasarre et al., 2012).
ComR-ComS (Rgg4) system has been reported to regulate streptococci competence genes in natural genetic transformation phenomenon. ComR – cytoplasmatic effector and ComS – the precursor of the competence pheromone, were first identified in Streptococcus thermophilus and Streptococcus salivarius (Gardan et al., 2013). The potential number of transformable Streptococcus was increased by the identification of orthologous in all available sequenced streptococci species (Fontaine et al., 2010).
It was experimentally proven that ComR-ComS system strongly induces ComX (also known as sigma factor σX or sigX) expression which leads to, consequently, natural DNA transformation in several species of the Streptococcus genus (Gardan et al., 2009; Fontaine et al., 2010; Khan et al., 2012; Marks et al., 2014b). In the early phase, comX rises in his net abundance inducing the X-state in cells, a transcriptional reprogramming (Claverys et al., 2006). When the cell reaches the late phase, σX associates itself with RNA polymerase core, making the target a specific target named σX-box, DNA-binding motif or Cin-box which regulates the expression of the regulon - typically consisting of fourteen critical late competence gene encoding the transformasome and many species-specific dispensable genes as the ones involved in the methylation of the exogenous ssDNA (Claverys and Martin, 2003; Petersen et al., 2004; Johnston et al., 2013).
Chemically defined medium with free amino acids and no complex oligopeptides seems optimal for the induction of comX by ComR-ComS. Some of the strains tested can be spontaneously transformable in similar conditions, but others being only transformable when growing as biofilm (Gardan et al., 2009; Fontaine et al., 2010; Marks et al., 2014a). In fact, in Streptococcus, the competence pheromones are produced in response (induced) to specific environmental stresses, including acidification of the medium, oxidative or temperature stress, mutagens, or nutrition stress (Fontaine et al., 2015).
In 2014, Marks et al. (2014a) reported the observation of S. pyogenes naturally competence in vitro in the form of biofilm structures, on epithelial cells and in biofilm colonization in vivo (BALB/cByJ mice). This was the first report of natural transformation in the pyogenic streptococcal group. ComR-ComS system orthologous genes are present in S. agalactiae and S. dysgalactiae subspecies, and it may, therefore, be a question of replicating the physiological model, like biofilm, to observe natural competence (Fontaine et al., 2015). ComR has dual functionality – in the growth of biofilms and natural transformation – which seems to point to a relation between these two processes (Marks et al., 2014a).
The Sil system is an important QS mechanism found in S. pyogenes that has been investigated for its potential role in biofilm formation through regulation of cell adhesion to surfaces (Young et al., 2016). The core sil system is encoded in a putative 15–17 kbps genomic island harboring six genes, silABCDE and silCR, and a much higher GC content compared to the rest of the genome. The locus includes a two-component system (silA and silB), putative ATP-binding cassette transporters (silD and silE), and silC that together with silC/R regulate the sil locus transcription (Jimenez and Federle, 2014). The silC/R encode a small 41-aa pro-peptide, and this pheromone has been identified to be signal peptide associated with modulation of expression of various uncharacterized genes in S. pyogenes (Jimenez and Federle, 2014).
LuxS protein is present in several Gram-negative and Gram-positive bacteria and is responsible for the production of the autoinducer-2 (AI-2), which has been identified as a universal signaling molecule for interspecies communication. Previous studies have demonstrated that the LuxS/AI-2 system influence the expression of various virulence determinants, and biofilm development in S. species, including S. gordonii, S. intermedius, and S. pyogenes (Galante et al., 2015). It was found to be necessary for the proper formation of biofilms in S. mutans, by regulating the expression of glucosyltransferases (Merritt et al., 2003). The same system in S. pneumoniae, regulates the initial stages of biofilm formation through regulation of the LytA (autolysin) expression and pneumolysin (Vidal et al., 2011).
Environmental Factors That Control Biofilm Initiation
Bacteria generally adopt the planktonic phenotype when nutrients are available namely, in a nutrient rich medium like the ones used in the laboratory. However, in nature, nutrients are not so abundant, and bacteria will then opt to grow in a phenotype where the growth rate is slower and less demanding metabolically, like the biofilm phenotype. This biofilm protects from dynamic environments and antimicrobial agents (Garrett et al., 2008; Olsen, 2015). The interplay between the planktonic phenotype and the biofilm state is regulated by several genetic determinants and transcription factors in response to environmental stimuli; these impact enzymatic and structural components of the cell that are required for biofilm development (Garrett et al., 2008; Kostakioti et al., 2013; Fiedler et al., 2015). Among the environmental factors, nutrient availability, temperature and pH variations, concentration of metals and osmolytes, redox potential, interaction with the host’s immune system are the most common. These factors affect bacterial cell properties, namely gene regulation and cell surface physicochemical characteristics, which may have a profound effect on cell–cell interaction and hence, on biofilm development (Garcia-Gonzalo and Pagán, 2015).
Several in vitro studies show that environmental factors influence the ability of streptococci pyogenic strains to form biofilms. Baldassarri et al. (2006) evaluated the influence of temperature and atmospheric conditions in the ability of S. pyogenes clinical strains to form biofilms. While temperature appeared to have no effect on the biofilm formation, there was a significant increase in biofilm formation under anaerobiosis (Baldassarri et al., 2006). An increased biofilm formation was also observed when S. pyogenes and S. agalactiae were grown in media supplemented with 1.5% (v/v) glucose as a result of medium acidification due to metabolism (Manetti et al., 2010; Rinaudo et al., 2010; Thenmozhi et al., 2011). D’Urzo et al. (2014) showed evidence that acidic pH and not glucose concentration is the environmental signal to S. agalactiae biofilm formation. However, some opposing results have been observed in several in vitro studies regarding the influence of pH in the biofilm formation by S. agalactiae. Some studies show an increased ability to form biofilms by S. agalactiae strains at pH 6.5 when compared to pH 4.2 (Kaur et al., 2009; Borges et al., 2012; Yang et al., 2012), by contrast, Ho et al. (2013) showed that low pH induced biofilm formation. These discrepancies can possibly be explained by the different strains intrinsic variability and ability to survive in an acidic environment. In vivo, exposure of S. agalactiae to the acidic environment of the vagina can be the signal sensed by the bacteria to grow in biofilm. From this perspective, changes in the growth of S. agalactiae strains were observed in a mouse model of vaginal colonization (Carey et al., 2014) and has also been reported in humans (Hansen et al., 2004). An increased ability to form biofilms in vivo was also observed by S. pyogenes and SDSE strains when compared to in vitro (Marks et al., 2014a; Genteluci et al., 2015). In this context, it is likely that apart from carbon sources, modified atmosphere, pH, and responses of the host’s immune system might trigger biofilm development in vivo.
Vajjala et al. (2019) characterized an in vitro model of S. pyogenes biofilm formation on mammalian cells that mimic a mouse model of human necrotizing fasciitis. The authors showed that the expression of secreted S. pyogenes streptolysins induced endoplasmic reticulum stress in the host. In vivo, the streptolysin null mutant is attenuated in biofilm formation and bacterial spread, revealing an important role of streptolysin in endoplasmic reticulum stress and the association with the formation of biofilms and necrotizing fasciitis disease progression (Vajjala et al., 2019).
Attachment Factors Involved in Initiation
Biofilm development starts when individual cells adhere/attach to abiotic or biotic surfaces. Attachment is mediated through several structures and molecules present at the surface of the bacterial cells, like motility elements as the flagellum or proteinaceous structures like the pili (type-IV pili), and other elements like lipopolysaccharides, TAs, surface proteins, and extracellular proteins (Schumacher-Perdreau et al., 1994; Hussain et al., 1997).
In S. pyogenes, attachment is suggested to occur in a two-step process with different molecules coming into play. The first step is dependent on lipoteichoic acids (LTA); in the second step bacteria cells adhere to specific receptors on the host cell (namely human) via bacterial surface components known as microbial surface components recognizing adhesive matrix molecules (MSCRAMMS), which include M protein, fibronectin-binding protein, serum opacity factor, etc. (Morath et al., 2005; Weidenmaier and Peschel, 2008; Rohde and Cleary, 2016).
While the first step is a very dynamic process, with an on/off kinetic effect, and relying on hydrophobic, ionic, and electrostatic forces responsible for an initial attachment, the second step of adhesion is more specific and complex since the strong affinity between several MSCRAMMS and host cells promote irreversible and species-specific interactions. S. pyogenes can adhere to human cells by interacting with different MSCRAMMS simultaneously, which strengthens biofilm initiation but hampers the detection of streptococcal adhesins (Šmitran et al., 2016).
Lipoteichoic Acids
Teichoic acids constitute the main class of anionic glycopolymers that are composed of phosphodiester-linked polyol units and are divided into two types: LTAs, and wall teichoic acids (WTAs; Armstrong et al., 1958; Ward, 1981; Brown et al., 2013). The difference between the two types is their connection to cell, with WTAs being covalently attached to the peptidoglycan, and LTAs are anchored in the cell membrane. LTA has been characterized in most Gram-positive bacteria including Staphylococcus aureus, S. agalactiae, S. pyogenes, and Lactobacillus plantarum (Kang et al., 2016). WTA has been less characterized in the pyogenic group and detailed studies revealing its importance are missing in the literature. With the growing number of pyogenic isolates available, future developments are expected in this topic. Teichoic acids biosynthesis is a complex multistep mechanism, and different WTAs/LTAs compositions and modifications are observed in different species (Poxton, 2014). The main chain of these biopolymers can be further modified by D-alanylation, by adding D-alanyl esters, and glycosylation, by adding mono- or oligosaccharides. These modifications impact several functions of the TAs.
Lipoteichoic acids are long, linear, anionic glycopolymers of phosphodiester-linked poly-glycerol phosphate (poly-GroP) repeating units, anchored to the plasma membrane of the cell through a glycolipid (Figure 2; Morath et al., 2005; Weidenmaier and Peschel, 2008; Shiraishi et al., 2016). LTAs are known to be major players in biofilm formation – studies estimate that 60% of initial adherence to epithelial cells is mediated by LTA (Šmitran et al., 2016) – however, other physiological roles are attributed to this amphipathic molecule: control of autolytic enzymes, maintain membrane integrity, cation homeostasis, and ions/nutrients trafficking (Fischer, 1988; Neuhaus and Baddiley, 2003; Poxton, 2014).
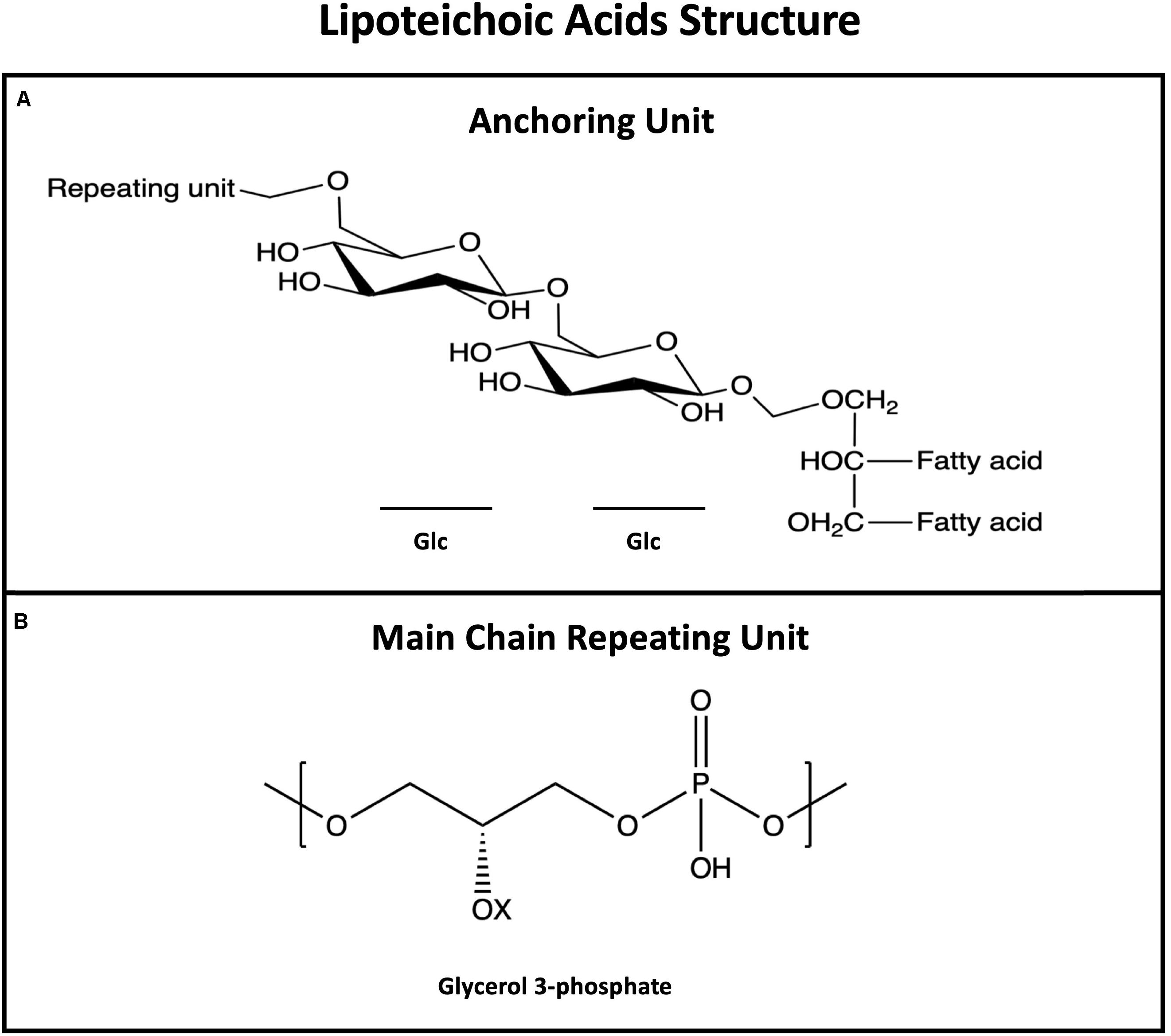
Figure 2. General structure of lipoteichoic acids (LTAs) showing the membrane anchoring moiety (A) and the most common repeating unit (B). LTAs repeating unit is more conserved than WTAs; Opposite to WTAs, LTAs are not bound to peptidoglycan but anchored to the membrane via the fatty acid.
The amphipathic nature of LTAs is crucial for pyogenic bacteria to surmount the repulsive electrostatic forces between bacteria and the attaching surface; LTA hydrophobic moieties are made accessible to the bacteria surface when LTA form stable complexes with surface proteins (see description of M proteins in the next section) while the anionic character achieved by the presence of phosphate and amino groups is important for interaction with cell receptors (as type I macrophage scavenger receptor) mediating bacteria-host adhesion (Dunne et al., 1994).
Several studies suggest that among the S. pyogenes strains the formation of complexes with M proteins provide anchoring LTA on the surface contributing to hydrophobicity and to biofilm formation (Courtney et al., 2009). Protein M is practically ubiquitous among S. pyogenes isolates, being expressed on the surface of the bacterial cell. Studies show that M proteins provide adhesion to different human cell lines, and the tropism for different cells demonstrates the heterogeneity of this protein (Tylewska et al., 1988; Caparon et al., 1991; Rohde and Cleary, 2016). It has also been demonstrated that M protein mediates initial surfaces interactions during biofilm formation (Cho and Caparon, 2005; Courtney et al., 2009). It was suggested that interactions between M protein of S. pyogenes and LTA expose the ester fatty acids of LTA, increasing bacterial hydrophobicity providing LTA–host cell interactions (Courtney et al., 2009). This structural conformation of the LTAs fosters biofilm development since it allows auto-aggregation and surface adhesion (Cho and Caparon, 2005; Lembke et al., 2006; Courtney et al., 2009; Young et al., 2016), which consequently influences the ability of some members of this species to form biofilms. This hypothesis is also supported by studies with M-protein defective mutants that showed a decrease in biofilm development regarding wild type strains (Cho and Caparon, 2005; Courtney et al., 2009). On the other hand, the studies by Courtney et al. (2009) suggest that the M-protein–LTA interaction may be specific to strains expressing only one M protein family member.
Protein M homologs (Mrp and Enn proteins) genetically and functionally related are present in most S. pyogenes strains. Despite considerable similarities among M, Mrp, and Enn proteins, the M-like proteins remain less studied. The current state of knowledge for M, Mrp and Enn proteins, is based on studies on function and immunogenicity, interactions between M-like proteins and host ligand proteins, and analysis of the genetic data supporting these interactions (reviewed in Frost et al., 2018). Overall, the similarity of protein M, the M-like proteins have been shown to be involved in the maintenance of LTA which may aid in the formation of biofilms and in colonization of the oropharynx (Beachey et al., 1983; Courtney et al., 2009). M-like proteins have been identified among SDSE strains (McNeilly and McMillan, 2014); however, their role in the formation of biofilms is not yet determined.
Streptococcal Pili
Pili (or fimbriae) are long filamentous structures that are extending from the surface of several bacterial cells, including SDSE (Genteluci et al., 2015; Ma et al., 2017), S. pyogenes (Manetti et al., 2007) and S. agalactiae (Konto-Ghiorghi et al., 2009; Rajagopal, 2009). Many functions can be attributed to pili; besides adhesive structures, they have been implicated in gene transfer, biofilm development, host cell invasion, twitching motility, and biofilm development, in the latter case by stimulating bacterial aggregation and attachment to the surfaces of host cells (Proft and Baker, 2009; Rohde and Cleary, 2016).
Streptococcus pyogenes pilus proteins have a C-terminal LPTXG-like motif attached to the host cell wall (Mora et al., 2005) and in pilus-defective mutants, a reduced biofilms formation ability was observed in different surfaces (Manetti et al., 2007). The role of pili in S. pyogenes virulence has also been evaluated in vivo in a humanized mouse by the deletion of AP1 gene, encoding the ancillary proteins (Lizano et al., 2007). This clearly shows the importance of pili in the initial stages of host colonization by S. pyogenes (Danne and Dramsi, 2012). In another study it was observed that pilus promote the recruitment of immune system cells, mainly neutrophils that provide neutrophil extracellular traps (NETs), which results in the decrease of S. pyogenes virulence in murine models (Crotty Alexander et al., 2010).
Streptococcus agalactiae strains encode two genomic islands, pilus islands-1 (PI-1) and -2 (PI-2), which includes the two variants PI-2a and PI-2b in separate loci and both flanked by direct repeats of conserved genes, where all genes responsible for pilus machinery are located. These genomic islands harbor three genes encoding protein essential for pilus assembly and accessory proteins (AP1 and AP2). Besides these, there are also two genes encoding sortase responsible for polymerizing the protein chains and cell wall attachment (Dramsi et al., 2006; Rosini et al., 2006). The importance of the genomic islands for bacteria–host cell interaction and biofilm formation in abiotic structures was revealed by previous studies with isogenic mutants lacking pilus 2a structures or the sortase enzymes demonstrated the role of pili in the interaction of bacteria with the host cell and biofilm establishment on abiotic surfaces (Rinaudo et al., 2010). Rinaudo et al. (2010) also used antibodies directed against the pilus 2a and its ancillary protein, or antibodies against small ancillary proteins present at the base of the pilus. The results showed that the first repressed biofilm development in a dose-dependent manner while the latter had no effect, suggesting that PI-2a pili are more relevant for biofilm adherence and formation in these bacteria then the other components (Rinaudo et al., 2010).
The presence of fibrillary-like structures was also observed on scanning electron microscopy in biofilm-grown SDSE strains. The increased expression of coding genes was observed in strong biofilm-producing strains (Genteluci et al., 2015). Oppegaard et al. (2017) showed that genetic alterations in the pilus-region (also known as the FCT-region – Fibronectin and Collagen binding, and T-antigen) were associated with bacteria’s propensity to adhere to different surfaces, which could also be related to the expression of a wide range of adhesins putatively encoded in this region, including proteins predicted to adhere to collagen and fibronectin. Interestingly, although they are genomic closely related, the presence of fibrillary-like structures was not observed in SDSD strains. Probably, the absence of fibrillary-like structures in this subspecies can be compensated by the expression of another protein with similar functions (Alves-Barroco et al., 2019).
Adhesins
The strategies for adhesion are complex and variable, and the expression of specific-adhesin is considerably modulated by microenvironment conditions (Rohde and Chhatwal, 2013). Several fibronectin-binding proteins are extensively expressed in streptococci, with different binding properties and affinities, giving rise to a great variety of protein–protein interactions; in some specific strains the fibronectin-binding proteins have high affinity to soluble fibronectin, whereas other strains require immobilized fibronectin for binding. Due to their importance, eleven different fibronectin-binding proteins are expressed in S. pyogenes, including SfbI/F1, FbaA, FbaB, FBP54, protein F2, serum opacity factor, and several M proteins (Rohde and Cleary, 2016). Streptococcal fibronectin-binding protein I (SfbI or protein F1) takes part in the adhesion to different surfaces and recently was found to bind to host collagen facilitating the bacterial aggregation, colonization, and evasion to the host immune system (Dinkla et al., 2003). Fibronectin-binding proteins have also been described in SDSE, including FnbA, FnbB, FnB, and fibronectin-binding protein A (GfbA). These proteins provide streptococcal adherence to human skin fibroblasts, consequently contributing to biofilm development in vivo and persistence of infection (Collin and Olsén, 2003; Brandt and Spellerberg, 2009).
Adhesion to different surfaces are largely conditioned by the physicochemical substratum characteristics, including surface charge and hydrophobicity (Donlan and Costerton, 2002). These characteristics could be further modified by other environmental factors, such as temperature variations, and sub-inhibitory concentrations of antimicrobials (Garcia-Gonzalo and Pagán, 2015; Ranieri et al., 2018; Šmitran et al., 2018). As expected, bacterial cells with hydrophobic characteristics adhere to hydrophobic surfaces, cells with hydrophilic properties adhere to hydrophilic surfaces. Additionally, hydrophobic cells are more able to attach to surfaces than hydrophilic (Garcia-Gonzalo and Pagán, 2015). During initiation, the attachment step is followed by a so-called “accumulative phase,” where the cells start to adhere to each other forming clusters (microcolonies). Cell–cell adhesion mechanisms mediate this multistep process through different factors. One of these factors is the glucosamine-based polysaccharide intercellular adhesin (PIA), or poly-N-succinylglucosamine (PNSG). The biosynthesis of PIA starts with the expression of the IcaA protein, the first product of the ica operon (Cue et al., 2012). Orthologous genes of the IcaA protein are found in two S. agalactiae strains (data from BioCyc database). The IcaA protein is a N-glycosyltransferase – glycosyltransferases are responsible for the production of exopolysaccharides necessary for the production of the EPS matrix and establishment of microcolonies, and are found in S. mutans (Koo et al., 2010). Recently, Matysik and Kline (2019) proposed a microcolony-independent mechanism for the S. pyogenes clinical strain, JS95. In this alternative mechanism, instead of forming microcolonies the cells will sediment and attach to the surface, with the capsule being of crucial importance. The role of the capsule in biofilm formation has been elusive in the past, with its presence being associated with biofilm inhibition. The same study suggests a dual role for the capsule that is growth stage dependent. At the early exponential growth, the capsule will mask the surfaces adhesins, inhibiting biofilm formation, while at later growth phases the capsule will promote biofilm formation (Matysik and Kline, 2019).
Disruptive Factors and Biofilm Dispersal
The presence of disruptive factors is of extreme importance for the proper development of the biofilm. These factors help the biofilm to reach its mature structure, with the creation of water channels and cavities; without these features the biofilm structure will be impaired at several levels, as the EPS matrix will not have the right properties. Also, factors with hydrolytic properties play important roles in the turn-over of the adhesive factors, allowing them to be replaced. This way the structure of the biofilm is maintained. Another very important function of the disruptive factors is their involvement in the return of the cells to the planktonic phenotype (dispersal stage). Proteases, together with DNases, are responsible for the degradation of the polymeric matrix of biofilms its tight regulation ensures the maintenance of the biofilm structure since high levels of these enzymes lead to disruption of the 3D architecture of the biofilm and ultimately, to its dispersion (Fiedler et al., 2015).
Proteases
The streptococcal pyogenic exotin B (SpeB), a cysteine protease, is known to play a substantial role in S. pyogenes virulence. Studies show that SpeB can destabilize the extracellular matrix of the host cell, complement system molecules, immunoglobulins, interleukin, antimicrobial peptides, and serum protease inhibitors (Hytönen et al., 2001). SpeB is also responsible for the ability of S. pyogenes to colonize host cells since it is involved in adhesion to glycoproteins. However, with high levels of active SpeB the biofilm enters the dispersion phase and its development is prevented (Doern et al., 2009). Several transcriptional regulators associated to the transcription of speB have also been found to regulate the formation and maturation of biofilms in S. pyogenes. RopB (Rgg family) and the sugar metabolism regulator CcpA are positive regulators that directly interact with promoter of the speB gene (Chang et al., 2011), while the transcriptional regulator Srv down-regulates this gene. When Srv is inactivated, an increase of the expression of SpeB is observed and the consequence is biofilm dispersal (Doern et al., 2009; Roberts et al., 2010a; Connolly et al., 2011). Roberts et al. (2010a) demonstrated that Srv regulates SpeB transcription in the initial step of the adhesion, that is, Srv represses SpeB, which allows for biofilm development. In response to external environmental signals, Srv decreases this repression, thereby providing SpeB production and consequent biofilm dispersion with the colonization of new sites. The response regulator CovR of the CovRS two-component system interacts with speB promoter, acting as a transcriptional repressor.; again, repression of speB by CovR allows the biofilm development (Doern et al., 2009; Roberts et al., 2010b; Connolly et al., 2011). CodY is another regulator associated with biofilm formation, involved in response to nutrient deprivation. in vitro studies with CodY deletion mutants demonstrated a reduction in the ability of biofilm formation by S. pyogenes (Fiedler et al., 2015).
The high-temperature requirement protein A (HtrA) is a protease widely distributed among streptococci that contains a transmembrane domain responsible for anchoring the enzyme to the cell surface. As SpeB, HtrA degrades other proteins in response to stress. Homologs of the HtrA identified in other Gram-positive bacteria degrade abnormal proteins in response to adverse environmental conditions (Kim and Kim, 2005). An equivalent role of this protein suggested for streptococci since the deletion of htrA in S. mutans decreased its ability to tolerate environmental stress (Diaz-Torres and Russell, 2001), and altered biofilm appearance, becoming more granular than wild type (Biswas and Biswas, 2005). Additionally, in S. pyogenes, the expression of virulence determinants was affected (Lyon and Caparon, 2004). In addition to the proteolytic properties of these enzymes, they share an ability to adhere to the extracellular matrix that enable adhesion to host tissues (Lyon and Caparon, 2004; Kim and Kim, 2005).
Extracellular DNases
Despite its abundance among Streptococcus species, the biologic role of e-DNA in biofilm development is not completely understood. The DNases of the S. pyogenes are the best characterized so far; six of the genes encoding DNases in this species were found in prophage regions (sda1, sda2, spd1, spd3, spd4, and sdn) and two other genes were found in the chromosome (spnA and spdB). Homologs of S. pyogenes DNases associated with prophage and chromosomally encoded have been found in other streptococcal species, as SDSD and SDSE (Rato et al., 2010, 2011; Remmington and Turner, 2018). The existence of extracellular nucleases in S. agalactiae has been proposed since the 1980’s. The major DNase in this species is encoded by the gene gbs0661, and the protein is named Nuclease A (NucA; Derré-Bobillot et al., 2013).
The focus in studying streptococcal DNases is the degradation of extracellular niches that are produced by the host’s immune system cells (like neutrophils), to capture and eliminate bacteria. Neutrophils secrete long, entangled, e-DNA chains called Neutrophil extracellular traps (NETs) that are enriched with antimicrobial molecules, small peptides and proteases. These traps imprison and degrade invasive cells. The DNA-based structure of the NTEs is not affected by the host proteolytic activity but can be hydrolyzed by the bacterial eDNases, releasing bacteria from the trap (Brinkmann and Zychlinsky, 2012; Remmington and Turner, 2018).
Streptococci DNases may also be involved in managing the biofilm since eDNA is one of the important components of the EPS matrix (Sharma and Pagedar Singh, 2018). By cleaving eDNA chains, DNases are involved in QS, regulation of biofilm formation, disruption of competitive biofilms and bacterial clearance (Sharma and Pagedar Singh, 2018).
Studies show that DNase is effective in removing Streptococcal biofilms on several substrates (Genteluci et al., 2015). The regulatory mechanism that control the protease SpeB, CovR/CovS, Rgg, and CodY, together with the systems PerR and Ihk/Irr, regulate the expression of DNases (Sumby et al., 2006; Anbalagan and Chaussee, 2013; Wang et al., 2013). Depending on the DNase the system might up- or down-regulate its expression. Yet, further research is required to fully elucidate the mechanism of regulation of DNases (Remmington and Turner, 2018).
The same disruptive forces necessary for the proper maturation of the biofilm are crucial for the detachment of bacterial cells from an established biofilm. This dispersal step usually leads to a modification in gene expression and allows the colonization of bacterial pathogens in other infection spots, and for this reason, the development of new biofilms, leading to the systemic diffusion of infection (Donlan, 2001; Kaplan, 2010; Kostakioti et al., 2013; Jamal et al., 2018).
The biofilm dispersion can be initiated by the bacterial community, called active dispersal, or by external forces – fluid shear, bacteriocin, and human intervention – called passive dispersal (Kaplan, 2010; Kostakioti et al., 2013). The mechanisms in which pyogenic streptococcal actively regulates biofilm dispersal is not yet completely understood. Overall, the presence of DNA and protein in the matrix of pyogenic streptococcal biofilms suggest that uncharacterized DNase or protease may participate in the regulation of biofilm dispersal (Doern et al., 2009; Genteluci et al., 2015; Young et al., 2016; Guilhen et al., 2017; Alves-Barroco et al., 2019).
Studies suggest that the streptococcal regulator of virulence (Srv) and the streptococcal pyrogenic exotin B (SpeB) play a significant role in the dispersion of S. pyogenes biofilm. in vivo and in vitro studies demonstrated that the deletion of srv coupled with an increase of SpeB results in decreased biofilm production. When the srv is active, SpeB levels increase, promoting degradation of components in the biofilm matrix allow biofilm cells to return to a planktonic form (Doern et al., 2009; Roberts et al., 2010a, b; Connolly et al., 2011).
EPS Matrix
The biofilm is a dynamic and heterogeneous environment that allows bacterial cells to reach homeostasis, and to use all available nutrients (Sutherland, 2001; Flemming and Wingender, 2010). The EPS matrix composition is extremely important for the properties of the biofilm since it offers cohesion and a three-dimensional scaffolding structure that holds the microbial cells close and provides mechanical integrity to the biofilm (Figure 3) (Wilking et al., 2011). Overall, the nature of the biofilm matrix will depend on the microbial cells present, their physiological status, the nutrients available, and the environmental/physical conditions (Costa et al., 2018; Florez Salamanca and Klein, 2018).
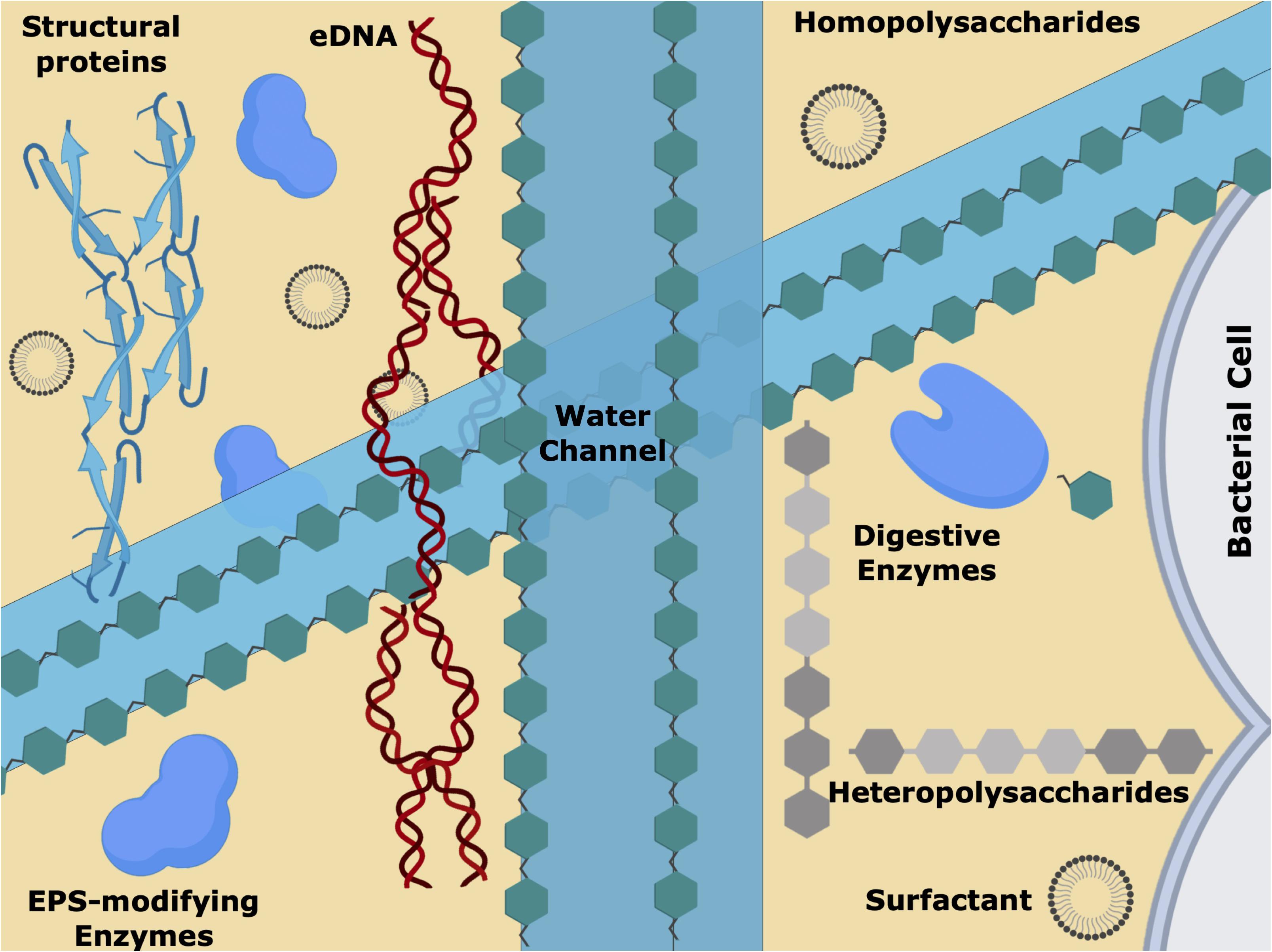
Figure 3. Schematic representation of the extracellular polymeric substances (EPS) matrix, showing the biofilm water channels, surfactants, the short and long sugar chains, the different proteins/enzymes and extracellular DNA (eDNA). This intricate 3D net of biomolecules is responsible for the chemical/physical and mechanical characteristics of the biofilm, allowing cell–cell communication, nutrients/gas diffusion and antimicrobial tolerance.
Whether a microbial biofilm will form on biotic/abiotic surfaces or not, is a consequence of the EPS matrix formed and how it responds to stress. To ensure spreading, survival, and adaptation to changing environments, the biofilm creates spatial heterogeneity of extracellular components and generates different types of forces: (i) elastic tension, due to polymeric chains with weak hydrogen bonding; (ii) viscous damping due to friction of polymeric compounds and hydrogen bond breakage, and (iii) alignment of the polymers in the shear direction. Since biofilms are composed by cells and a polymeric gel, they have features of both solids and liquids and hence are considered an active viscoelastic material, exhibiting time-dependent response to mechanical stress (Wilking et al., 2011). Such properties change with increasing temperatures (Sutherland, 2001; Garrett et al., 2008; Flemming and Wingender, 2010).
The main component of biofilm is water. Representing 97% of the EPS matrix (although, as with all aspects of biofilms, this depends on the system under examination), the water establishes a hydrated environment that protects the bacterial community from desiccation. The EPS is an intricate 3-D network of long polymeric chains of sugars and e-DNA, decorated with enzymes and structural proteins, and filled with water. Acting as solvent, the water content dictates viscosity, mobility, and mechanical response; water is organized within the fine structure of the biofilm, and while filling the internal channels of the biofilm, mediates nutrients transport throughout the colonies, potentially mitigating starvation (Sutherland, 2001; Lu and Collins, 2007; Flemming and Wingender, 2010; Kumar et al., 2017; Jamal et al., 2018). Increasing evidence suggests that the extracellular matrix of pyogenic streptococcal biofilms is rich in proteins and, as in other organisms, these play an essential role in biofilm development, maintenance, and spreading (Genteluci et al., 2015; Rosini and Margarit, 2015; Young et al., 2016; Alves-Barroco et al., 2019). Within the proteinaceous group are the structural proteins and the enzymes, which can be further divided into (digestive) enzymes and EPS modifying enzymes (Flemming and Wingender, 2010).
Enzymes present in the EPS matrix can degrade it; a biofilm is a microconsortium of microorganisms and enzymes participate in the extracellular digestive system, by supplying low molecular mass products arising from the breakdown of biopolymers. This metabolic activity contributes decisively for the maturation of the biofilm by forming channels and pores that allow diffusion, but also for the detachment of bacteria from the biofilm during the dispersal step. Usually, these enzymes are maintained close to the cells through interactions with the exopolysaccharide chains, enhancing nutrients uptake, and protecting the enzymes from denaturation and proteolysis (Schumacher-Perdreau et al., 1994; Wingender et al., 1999).
The EPS modifying enzymes help in the maintenance of the EPS matrix but also in mechanisms of proliferation and adaptation, by targeting different elements of the matrix to be modified. Epimerases modify the structural conformation of the polysaccharides, influencing the intermolecular interactions within the biofilm and its 3D architecture (Whitfield et al., 2015). Hydrolases and transferases and add or remove functional groups as acetyls, glyceryls, pyruvyls, lactyls to the exopolysaccharide chains, controlling the polymer’s electrostatic potential and solubility. These modifications protect bacteria cells against ROS produced by host immune cells, antimicrobial peptides, bacteriocins produced by competing bacterial, ultimately contributing to fixation, and establishment of colonization and infection. Acetylation has been shown to play a critical role in S. pneumoniae – the presence of acetyl-decorated polysaccharides in S. pneumoniae clinical isolates seemed to be advantageous for the bacteria to resist recognition by innate immune mechanisms, making them more virulent than modification-free biofilms (Vuong et al., 2004; Rajam et al., 2007; Melin et al., 2010).
Structural proteins have a pivotal role in keeping the EPS matrix intact (Lasa and Penadés, 2006). Extracellular lectins, which are carbohydrate-binding proteins, are bridging the polysaccharide chains and cell surface-associated proteins, acting as cross-linking elements. Amyloid fibbers are also extensively formed in biofilms; long, ordered, and H-bonded beta-sheets of proteins or peptides self-assemble to form functional fibbers, that contribute to biofilm architecture and integrity (Fowler et al., 2007; Besingi et al., 2017). Furthermore, these low-energy protein structures, with a tensile strength comparable to steel, are resistant to degradation by detergents and proteases (Higgins and Novak, 1997; Van Schaik et al., 2005; Otzen and Nielsen, 2008). S. mutans is responsible for biofilm formation in the oral cavity, and amyloid fibbers are detectable in dental plaque. Inhibition of the biofilm is obtained using inhibitors of the amyloid-fibril forming proteins as adhesin P1 (Ag I/II, PAc) or wall-associated protein A (WapA; Oli et al., 2012; Besingi et al., 2017).
The EPS matrix is also composed of long chains of homo/heteropolysaccharides. The type of sugar residues, the net charge, the presence of organic functional groups or inorganic substituents (Ca2+ and other ions), and the internal glycosidic bonds present, affect the physical and, as a consequence, the biological properties of the matrix. Bacteria produce different polysaccharides along the “life-cycle” of the biofilm, and these polysaccharides have competent ability to interact with themselves, the biotic/abiotic substratum, and the other players of the biofilm arena, gaining an important role regarding function and structure. This might explain why many bacteria require exopolysaccharides to form a mature biofilm. Aggregative polysaccharides are essential for initial attachment, microcolony and macrocolony formation, and detachment or disassembly. Protective polysaccharides create a barrier that obstructs the entrance of antimicrobial agents, giving microcolony time to respond to the stimuli by upregulating protective genes. Capsular polysaccharides are covalently bound to the bacteria cell surface and grant chemical and structural variability to the cell and is one of the most effective strategies to deceive the host immune system (Wessels, 2016).
Polysaccharides of the EPS are often covalently bound to lipids. These lipopolysaccharides are slightly negatively charged, shield off the hydrophobic antimicrobial agents, and form a protective gelatinous layer at the bacteria surface.
Extracellular DNA is a structural element of the EPS and a source of genetic material. It arises from cell lysis, but also from specific mechanisms (autolysis, active secretion mechanisms, or formation of membrane vesicles) that allow DNA damage repair and HGT, and provide symbiotic competitive advantages to the community (Ibáñez de Aldecoa et al., 2017). The eDNA releasing mechanisms are modulated by quorum sensing – a cell-to-cell communication system that allows a coordinated response of the microorganisms. This is the case for S. pneumoniae, where a certain level of cell density triggers the lysis of a subpopulation, allowing the release of genomic DNA (for details, see Ibáñez de Aldecoa et al., 2017 and references therein). Genetically encoded systems have been found to control programmed cell death for the release of bacterial DNA (Bayles, 2007). In 2007, Bayles (2007) described a system that works based on a holin-like (CidA) and antiholin-like (LrgA) proteins, responsible for regulating programmed cell death (PCD). Holins are membrane proteins that promote lysis and antiholins counteract holins. PCD is extremely important for biofilm formation, and this regulatory system is virtually ubiquitous to all bacteria. It is proposed to be associated with antibiotic resistance: like what happens in drug-resistant cancers when Bcl-2 is overexpressed, it seems that in biofilms, cells will resist to death because the system will be preventing cell death. Studies where cidA or lrgA genes were deleted or mutated strongly suggest this point.
Recently, it was demonstrated, the capacity of Streptococcus dysgalactiae subs. dysgalactiae to produce biofilms and the multifactorial nature of their composition (Alves-Barroco et al., 2018). Confocal laser scanning, fluorescence microscopy and scanning electron microscopy were used to analyze the SDSD biofilm structure. The results achieved demonstrated that there are differences in biofilms produced among different SDSD strains, and in some cases, the presence of mucus-like extracellular material covering the cell surface was observed in the biofilms. This material corresponded to eDNA and proteins since in the presence of proteinase K, biofilm production by SDSD strains is inhibited (Alves-Barroco et al., 2019).
Doern et al. (2009) examined S. pyogenes strains from different clinical origins and showed that DNA and proteins are the major structural components of the biofilm, while carbohydrates had a modest role (Doern et al., 2009). This is in contrast to SDSE, which requires the presence of several different polysaccharides (Genteluci et al., 2015). Also, adding a carbohydrate oxidant as sodium metaperiodate to SDSE biofilm indicated the presence of an exopolysaccharide, similarly to what was observed for different biofilms of S. mutans (Liao et al., 2014) and S. intermedius (Nur et al., 2013).
The importance of eDNA was also demonstrated by disruption of the biofilm structure of SDSE strains after treating with DNase I. The authors suggest that eDNA is essential for the initial stage of adhesion and the biofilm development. The secondary role of proteins in the biofilm structure was proposed by the low protein content, 12.14%, and its disruption upon treatment with proteases (Genteluci et al., 2015).
Clinical and Veterinary Relevance of Pyogenic Biofilm
The significance of this bacterial phenotype in clinical settings is often underestimated (Hall-Stoodley et al., 2004; Chen and Wen, 2011; Kumar et al., 2017). Several different surfaces in clinical environments are prone to develop biofilms, consequently increasing the risk of infection (Garnett and Matthews, 2012; Garnett et al., 2012). Some characteristics of the human body as shear forces caused by teeth, blood pressure, or the innate immune system, actually trigger the bacterial cells to adopt the biofilm phenotype, as they resemble some of the stimuli that induce biofilm formation in challenging environments (Stewart and Costerton, 2001; Jefferson, 2004; Gupta et al., 2016).
The main requirements for successful infection of different tissues is the ability of bacteria to adhere and to remain attached to the host cells. In these environments, the development of biofilm enhances resistance to host defenses and to nutrient privation, improving bacterial survival (Hall-Stoodley et al., 2004; Melchior et al., 2006; Kumar et al., 2017). From a clinical point of view, there is a considerable relationship between the ability to form biofilms and resistance to conventional antibiotics (Sharma et al., 2019). According to the National Institute of Health, in humans, biofilms account for up to 80% of the total bacterial infections, including endocarditis, periodontitis, sinusitis, meningitis, osteomyelitis, chronic wounds, and prosthesis and implantable devices related infections (Khatoon et al., 2018). In many of these cases, infection arises from implantable medical devices, such as catheters, implants, and implantable electronic devices (Khatoon et al., 2018; Narayana and Srihari, 2019; Pelling et al., 2019) that become contaminated with bacteria, usually biofilms of staphylococci, streptococci, Gram-negative bacteria, and fungi (Kokare et al., 2009; Marks et al., 2014b; Rosini and Margarit, 2015; Gomes et al., 2016; Young et al., 2016; Castilho et al., 2017; Stewart and Bjarnsholt, 2020).
Streptococcus pyogenes biofilm was first detected in the skin (Akiyama et al., 2003) and accepted to occur in other sites of infection (Lembke et al., 2006; Swidsinski et al., 2007). Pyogenic bacteria can integrate the host natural microbiome or form its own biofilm during infection. Apart from person to person contact, contaminated airborne droplets are the most common way of transmitting the pathogen within humans. Bacterial colonization occurs in mucosal membranes of the oropharynx and non-intact skin; for disease to develop, bacterial cells first adhere and later internalize the host cells (Fiedler et al., 2015). Very recently, Siggins showed that in severe invasive infections, S. pyogenes reach the bloodstream using efferent postnodal lymphatic vessels through sequential draining lymph nodes (Siggins et al., 2020). The authors also showed that while traveling form the primary site of infection, the bacteria remains extracellular. Self-healing or severe invasive infections are dependent on several different factors, namely the formation of biofilm. In 2013, Fiedler et al. (2013) demonstrated that S. salivarius and Streptococcus oralis – abundant species in the oral cavity – can form mixed-species biofilm with S. pyogenes; in this case, S. pyogenes is in the upper layer. The biofilm phenotype can be correlated with asymptomatic carrier persons, recurrent infections, systemic dissemination of the infection and antibiotic failure (Fiedler et al., 2013, 2015).
In veterinary settings, the production of biofilms can significantly affect the effectiveness of the treatment of bovine mastitis. Besides increased resistance to different antibiotics, the biofilm promotes adherence and colonization of mammary tissue (Wallis et al., 2018). In bovine mastitis, the most prevalent species of the pyogenic group are S. agalactiae, Streptococcus uberis, and SDSD (Rato et al., 2013; Rosini and Margarit, 2015; Pang et al., 2017; Klaas and Zadoks, 2018; Kaczorek et al., 2017; Tomazi et al., 2018).
Streptococcus uberis and SDSD were initially classified as environmental, meaning that infection occurs mainly from environmental sources. However, molecular epidemiology studies suggested that infections occur predominantly in a cow-to-cow fashion. S. agalactiae has been considered an extremely contagious mastitis pathogen for several years, and an important human pathogen. It was reported that some clonal complexes of S. agalactiae were shared between cows and farm personnel, indicating the zoonotic potential of this species (Cobo-Angel et al., 2019). These pathogens are also producers of biofilms (Gomes et al., 2016; Reinoso, 2017; Alves-Barroco et al., 2019; Horiuk et al., 2019), and this virulence characteristic has been associated with persistent infections and development of antibiotic resistance (Olson et al., 2002; Ruppen et al., 2017).
Increased Tolerance and Resistance Against Antimicrobial Agents
Overall, upon biofilm formation, there is a delayed internalization of the antimicrobial through the biofilm matrix, as the primary physical and/or chemical diffusion barrier prevents the entrance of polar and charged antibiotics (Mah and O’Toole, 2001; Patel, 2005; Høiby et al., 2010). Additionally, the heterogeneous growth of the biofilm cells and activation of the stress response genes contribute to the resistance phenotype (Bjarnsholt et al., 2013a, b; Macià et al., 2014). Besides, studies show that a biofilm-specific phenotype is induced in a subpopulation, and it results in the differential expression of active mechanisms to combat the effects of antimicrobial agents (Konto-Ghiorghi et al., 2009; Genteluci et al., 2015).
The role of biofilm in evading conventional antimicrobials for the treatment against streptococcal infections is not yet entirely understood, although several studies have shown that streptococcal biofilms survive after treatments with high concentrations of antibiotics (Ogawa et al., 2011; Horiuk et al., 2019). Overall, this resistance mechanism is the consequence of the multicellular and matrix nature of biofilms, which leads to the antibiotic resistance of biofilm communities, along with the known conventional resistance mechanisms, such as, efflux pumps, modifications of the antimicrobial target, and enzyme inactivation (Rosini and Margarit, 2015; Young et al., 2016). The main mechanisms of resistance in pyogenic streptococci are revised in Alves-Barroco et al., 2020. Several in vitro studies demonstrated that when enclosed and protected by a biofilm, bacteria are 10–1000 times more resistant to antimicrobial drugs when compared to the planktonic counterpart (Olson et al., 2002; Baldassarri et al., 2006; Shen et al., 2013; Chadha, 2014; Wu et al., 2015; Boonyayatra and Pata, 2016; Singh et al., 2017). Macià et al. (2014) proposed a method for selecting antibiotics against biofilm bacteria which can also be used for developing new antimicrobial agents. This strategy is based on assessing six pharmacodynamic parameters:
i minimal inhibitory concentration (MIC), can be defined as the lowest concentration of an antimicrobial that inhibits the growth of planktonic cells;
ii minimal biofilm inhibitory concentrations (MBIC), which is the lowest concentration of an antimicrobial that results in a difference of 1 log in growth after six h of incubation;
iii minimal bactericidal concentration (MBC) is the lowest concentration of an antimicrobial that killing 99.9% of the colony-forming units (CFUs);
iv biofilm bactericidal concentration (BBC) is the lowest concentration of an antimicrobial producing a 99.9% reduction of the CFUs from a biofilm culture when compared to the control;
v minimum biofilm eradication concentration (MBEC) is the lowest concentration of an antimicrobial that inhibits visible after to collect biofilm cells;
vi biofilm-prevention concentration (BPC) is the similar as the MBIC, but bacterial inoculation and antimicrobial exposure co-occur (Macià et al., 2014).
To the best of our knowledge, the information available regarding the MBEC of pyogenic streptococcal is scarce. However, some studies indicate that biofilms produced by pyogenic streptococci are resistant to various antibiotics (Conley et al., 2003; Baldassarri et al., 2006; Pichichero and Casey, 2007; Horiuk et al., 2019), suggesting that most of the antibiotics evaluated would be ineffective as antimicrobial agents.
The β-lactams (mainly penicillin) have been universally accepted as the antibiotics of choice for pyogenic streptococci infections; however, therapeutic failures have been reported and attributable to different causes, including biofilm formation (Pichichero and Casey, 2007; Bonofiglio et al., 2018; Moroi et al., 2019). Conley and coworkers reported penicillin treatment failure in 32% of case-patients with S. pyogenes infection. The authors first reported in vitro S. pyogenes insensitivity to penicillin by MBEC assay (Conley et al., 2003). Torretta et al. (2012) reported pharyngitis treatment failure 37% of children to have biofilm producer S. pyogenes and with increased MBEC.
Macrolides are considered antibiotics of choice in human therapy for the treatment of pneumonia, sinusitis, and otitis in cases where patients are allergic to β-lactams (Kanoh and Rubin, 2010), and lincosamides are used as alternative to penicillin G against anaerobic bacteria and streptococci strains (Greenwood and Irving, 2012). However, therapeutic failures of macrolide have been suggested as a consequence of the formation of biofilms by S. pyogenes, resulting in clonal spread (Baldassarri et al., 2006). Some antibiotics, such as fluoroquinolones, and aminoglycosides, are not active in anaerobic conditions, affecting only the outer part of the biofilm (Borriello et al., 2004).
Ruppen and coworker compared MICs and MBECs using penicillin, gentamicin, and a combination of both among S. agalactiae biofilm-forming strains. The results showed reduced susceptibility to penicillin, and that the concentration of gentamicin against S. agalactiae biofilm cannot be achieved in bone with systemic administration, only administered locally (Ruppen et al., 2017).
Horiuk and coworkers analyzed resistance to antimicrobials among bovine mastitis pathogens biofilm producers. The results showed resistance to penicillin, aminoglycosides, and macrolides, emphasizing the necessity of alternative approaches and develop new antibiotics to effectively treat bovine mastitis (Horiuk et al., 2019). In addition, the indiscriminate administration of antibiotics in dairy cows various for the promotion of growth and treatment provides substantial residues of antibiotics that are released through milk of dairy and significant effects on human health (Sachi et al., 2019).
Horizontal Gene Transfer is Increased in Biofilms
Biofilms increase HGT rates among community cells, by 16,000 times, due to high cell density and/or accumulation of genetic elements, either by transformation, conjugation or transduction, (Savage et al., 2013; Kragh et al., 2016; Emamalipour et al., 2020). Besides the genes involved in mobility, regulation or maintenance, mobile genetic elements (MGEs) provide the conditions for the uptake of antibiotic-resistant genes and virulence factors (Haenni et al., 2010). Thus, HGT has been found to be a major mechanism in generating gene diversity through intragenic and intergenic recombination, which can modulate host-pathogen interactions and extending the host range. Such gene transfer events frequently occur within the pyogenic group, particularly in S. pyogenes, S. agalactiae, Streptococcus canis, SDSD, SDSE, Streptococcus equi subsp. equi, Streptococcus equi subsp. zooepidemicus, and S. uberis (Baiano and Barnes, 2009; Haenni et al., 2010; Rato et al., 2010; Wong and Yuen, 2012; Richards et al., 2014; Rohde and Cleary, 2016).
In S. pyogenes the lateral exchange of virulence genes, mediated by bacteriophage infection, is a very important factor in the diversification of the species (McShan and Nguyen, 2016). Bacteriophages may convey genes that are advantageous to the hosts, thus fostering their own dissemination (Von Wintersdorff et al., 2016). An extreme case is presented by S. pyogenes in which almost all major gaps in the alignment of different M serotypes could be traced to prophage integration events (Canchaya et al., 2004). Marks and coworkers demonstrated that the biofilm microenvironment of S. pyogenes populations results in the induction of competence genes; therefore, it is more conducive to HGT. This study shows for the first time that S. pyogenes can be naturally transformed in the presence of exogenous DNA when grown as biofilms both in vitro and in vivo (Marks et al., 2014a).
Horizontal gene transfer also appears to play a role in the evolution and population structure of SDSE. Studies have revealed that HGT and recombination occur between S. pyogenes and SDSE, indeed, the two share several virulence factors and are coexist in the human host (McNeilly and McMillan, 2014).
We reported in previous studies for the first time the ability of milk udder SDSD isolates containing phage-encoded S. pyogenes genes to adhere and internalize primary human keratinocytes (Roma-Rodrigues et al., 2016) and niches of colonization/infection of S. pyogenes from the respiratory track: Detroit 562, a cell line derived from the metastatic site of pharynx carcinoma, primary Bronchial/Tracheal Epithelial Cells (BTEC), and A549, a cell line derived from a human adenocarcinoma of the alveolar basal epithelial cells (Alves-Barroco et al., 2018). Several genes are responsible for the increased virulence of these subspecies, providing the dissemination to a different host and propagating the infection persistent (Rato et al., 2011; Alves-Barroco et al., 2018). This subspecies has been considered by some authors as an emerging zoonotic pathogen (Chennapragada et al., 2018). Moreover, their biofilm-producing ability (Alves-Barroco et al., 2019) can make them highly difficult to eradicate.
The successful treatment of biofilm-associated streptococcal infections is troubled due to high antibiotic resistance. Conventional antibacterial therapy is unable to fully eradicate biofilms cells. Therefore, to overcome the resistance of biofilm, alternative strategies and antibiofilm agents have been studied.
Emergent Alternatives to Fight Biofilm Infection
Despite the increasing recognition of the impact of pyogenic infections, namely when adopting the biofilm phenotype, recurrent infections, uncontrolled contagion, therapeutic failure and morbidity, motivated scientists to find new solutions for this old problem. Some alternatives of antibiotics to combat biofilm-based infections are reviewed in Alves-Barroco et al. (2020). In the next paragraphs we want to give special attention to the protective effect of probiotic bacteria against pyogenic bacteria.
The use of live organisms as therapeutic agents has been accepted for many years, with more emphasis on the gut and oral cavity pathologies (Hoare et al., 2017). Since the last decade, studies have emerged describing how streptococcal infection can be overwhelmed by non-pathogenic bacteria, namely the resident bacterial species that form the microbiome. In 2013, the in vitro studies of Fiedler et al. (2013) showed that in direct contact experiments, S. salivarius and S. oralis in the planktonic phenotype eliminated the growth of S. pyogenes, and reduced its cell adhesion to eukaryotic laryngeal epithelial cell line (Hep2). In fact, the authors suggest that it is the structure of the S. salivarius biofilm covering the eukaryotic cells that protects them from the pathogen through steric hindrance. Recently, Lactobacillus plantarum, Lactobacillus acidophilus, and S. salivarius were tested for inhibition toward S. pyogenes cultures in deferred antagonism agar assays. The results show that these strains have anti-biofilm effects, especially S. salivarius (Humphreys and McBain, 2019). The antagonistic effect was not dependent on cell–cell contact since the pathogen and the probiotic were not in direct contact, but separated by a semi-permeable membrane that allowed diffusion of bacteriocins as salivaricin A2 (SalA2) and salivaricin B (SboB) in the case of S. salivarius K12 (Hyink et al., 2007), acidocins (Acedo et al., 2015) and plantaricins (Lages et al., 2015), in the case of of L. acidophilus and L. plantarum, respectively (in all cases, the acidification of the media was not observed). Effectiveness of the probiotic Streptococcus salivarius K12 for the treatment and/or prevention of sore throat was recently reviewed due to the large number of studies reporting the use of this agent in the treatment/prevention of upper respiratory tract infections (Wilcox et al., 2019). Despite describing S. salivarius K12 as being safe and well tolerated, the main conclusion of this systematic review of the literature is that this organism might have a prophylactic/therapeutic effect on sore throat in adults and children (namely derived from streptococcal infections), but more reliable and unbiased trials are required to establish this relation and boot this probiotic for regular use.
New targets for the development of new drugs are also gaining scientists attention. The LytR-A-Psr (LCP) family of proteins, are responsible for the insertion of WTAs and other anionic polymers into the peptidoglycan and have been associated with host cell adhesion and biofilm development (Chatfield et al., 2005). The brpA-like gene encoding the biofilm regulatory proteins A – homologous of the LytR protein – is a member of the LCP family (Shemesh et al., 2007). The brpA gene encodes a membrane associated protein (BrpA – biofilm regulator protein A) that has been implicated in biofilm formation, autolysis, and cell division in S. mutans. The deficiency of BrpA decreases the ability of the response to oxidative stress, cell envelope stress, and pH alteration. In vitro, the BrpA-deficient mutant can adhere to the surface, but its ability to form mature biofilm decreases considerably (Bitoun et al., 2012, 2014). Recently, we have reported that brpA-like gene is harbored by SDSD biofilm-producing strains, and its expression levels are associated with the biofilm-forming ability (Alves-Barroco et al., 2019). Structural and functional data on these and similar proteins will be an asset for the development of new antimicrobials with high specificity toward pyogenic infection, decreasing biofilm formation, affecting bacteria viability and decreasing antibiotic tolerance.
In addition, many pyogenic streptoccocci adhesins have been studied as antigens to vaccine potential, the most advanced candidates, having entered clinical trials, are based on the M and M-like proteins, while fibronectin-binding proteins and components of the pilus are in pre-clinical development (Frost et al., 2018; Raynes et al., 2018).
Conclusion
Biofilms play an essential role in streptococci pathogenesis, contributing to therapy failure and promoting persistent infections. Although the influence of variations in environmental conditions has been extensively studied on planktonic phenotype, further information about how these variations modulate streptococci biofilm development is needed. It is known that multiple virulence factors modulate biofilm formation by pyogenic streptococci; however, there are missing pieces about biofilm formation and its impact on streptococcal disease. Details on mechanical, chemical, physical, genetic, and structural properties of pyogenic streptococci biofilm will allow envisioning new therapeutic strategies to prevent biofilms formation or to eradicate mature biofilm.
Author Contributions
AF and TS-S conceived the idea. CA-B and JP-F wrote the draft. Revision by AF and TS-S. All authors contributed to the article and approved the submitted version.
Conflict of Interest
The authors declare that the research was conducted in the absence of any commercial or financial relationships that could be construed as a potential conflict of interest.
References
Abisado, R. G., Benomar, S., Klaus, J. R., Dandekar, A. A., and Chandler, J. R. (2018). Bacterial quorum sensing and microbial community interactions. mBio 9:e02331-17. doi: 10.1128/mBio.02331-17
Acedo, J. Z., van Belkum, M. J., Lohans, C. T., McKay, R. T., Miskolzie, M., and Vederas, J. C. (2015). Solution structure of acidocin B, a circular bacteriocin produced by lactobacillus acidophilus M46. Appl. Environ. Microbiol. 81, 2910–2918. doi: 10.1128/AEM.04265-14
Akiyama, H., Morizane, S., Yamasaki, O., Oono, T., and Iwatsuki, K. (2003). Assessment of Streptococcus pyogenes microcolony formation in infected skin by confocal laser scanning microscopy. J. Dermatol. Sci. 32, 193–199. doi: 10.1016/s0923-1811(03)00096-3
Al Safadi, R., Amor, S., Hery-Arnaud, G., Spellerberg, B., Lanotte, P., Mereghetti, L., et al. (2010). Enhanced expression of lmb gene encoding laminin-binding protein in Streptococcus agalactiae strains harboring IS1548 in scpB-lmb intergenic region. PLoS One 5:e10794. doi: 10.1371/journal.pone.0010794
Alves-Barroco, C., Rivas-García, L., Fernandes, A. R., and Baptista, P. V. (2020). Tackling multidrug resistance in Streptococci – From novel biotherapeutic strategies to nanomedicines. Front. Microbiol. 11:579916. doi: 10.3389/fmicb.2020.579916
Alves-Barroco, C., Roma-Rodrigues, C., Balasubramanian, N., Guimarães, M. A., Ferreira-Carvalho, B. T., Muthukumaran, J., et al. (2019). Biofilm development and computational screening for new putative inhibitors of a homolog of the regulatory protein BrpA in Streptococcus dysgalactiae subsp. dysgalactiae. Int. J. Med. Microbiol. 309, 169–181. doi: 10.1016/j.ijmm.2019.02.001
Alves-Barroco, C., Roma-Rodrigues, C., Raposo, L. R., Brás, C., Diniz, M., Caço, J., et al. (2018). Streptococcus dysgalactiae subsp. dysgalactiae isolated from milk of the bovine udder as emerging pathogens: in vitro and in vivo infection of human cells and zebrafish as biological models. MicrobiologyOpen 8:e00623. doi: 10.1002/mbo3.623
Anbalagan, S., and Chaussee, M. S. (2013). Transcriptional regulation of a bacteriophage encoded extracellular DNase (Spd-3) by Rgg in Streptococcus pyogenes. PLoS One 8:e61312. doi: 10.1371/journal.pone.0061312
Armstrong, J. J., Baddiley, J., Buchanan, J. G., Carss, B., and Greenberg, G. R. (1958). Isolation and structure of ribitol phosphate derivatives (teichoic acids) from bacterial cell walls. J. Chem. Soc. 1985, 4344–4354. doi: 10.1039/JR9580004344
Baiano, J. C., and Barnes, A. C. (2009). Towards control of Streptococcus iniae. Emerg. Infect. Dis. 15, 1891–1896. doi: 10.3201/eid1512.090232
Baldassarri, L., Creti, R., Recchia, S., Imperi, M., Facinelli, B., Giovanetti, E., et al. (2006). Therapeutic failures of antibiotics used to treat macrolide-susceptible Streptococcus pyogenes infections may be due to biofilm formation. J. Clin. Microbiol. 44, 2721–2727. doi: 10.1128/JCM.00512-06
Barnett, T. C., Cole, J. N., Rivera-Hernandez, T., Henningham, A., Paton, J. C., Nizet, V., et al. (2015). Streptococcal toxins: role in pathogenesis and disease. Cell. Microbiol. 17, 1721–1741. doi: 10.1111/cmi.12531
Basaranoglu, S. T., Ozsurekci, Y., Aykac, K., Aycan, A. E., Bıcakcigil, A., Altun, B., et al. (2019). Streptococcus mitis/oralis causing blood stream infections in pediatric patients. Jpn. J. Infect. Dis. 72, 1–6. doi: 10.7883/yoken.JJID.2018.074
Bayles, K. W. (2007). The biological role of death and lysis in biofilm development. Nat. Revi.Microbiol. 5, 721–726. doi: 10.1038/nrmicro1743
Beachey, E. H., Simpson, W. A., Ofek, I., Hasty, D. L., Dale, J. B., and Whitnack, E. (1983). Attachment of Streptococcus pyogenes to mammalian cells. Rev. Infect. Dis. 5(Suppl. 4), S670–S677. doi: 10.1093/clinids/5.supplement_4.s670
Berlutti, F., Catizone, A., Ricci, G., Frioni, A., Natalizi, T., Valenti, P., et al. (2010). Streptococcus mutans and Streptococcus sobrinus are able to adhere and invade human gingival fibroblast cell line. Int. J. Immunopathol. Pharmacol. 23, 1253–1260. doi: 10.1177/039463201002300430
Besingi, R. N., Wenderska, I. B., Senadheera, D. B., Cvitkovitch, D. G., Long, J. R., Wen, Z. T., et al. (2017). Functional amyloids in Streptococcus mutans, their use as targets of biofilm inhibition and initial characterization of SMU_63c. Microbiology 163, 488–501. doi: 10.1099/mic.0.000443
Biswas, S., and Biswas, I. (2005). Role of HtrA in surface protein expression and biofilm formation by Streptococcus mutans. Infect. Immun. 73, 6923–6934. doi: 10.1128/IAI.73.10.6923-6934.2005
Bitoun, J. P., Liao, S., Xie, G. G., Beatty, W. L., and Wen, Z. T. (2014). Deficiency of BrpB causes major defects in cell division, stress responses and biofilm formation by Streptococcus mutans. Microbiology 160(Pt 1), 67–78. doi: 10.1099/mic.0.072884-0
Bitoun, J. P., Liao, S., Yao, X., Ahn, S. J., Isoda, R., Nguyen, A. H., et al. (2012). BrpA is involved in regulation of cell envelope stress responses in Streptococcus mutans. Appl. Environ. Microbiol. 78, 2914–2922. doi: 10.1128/AEM.07823-11
Bjarnsholt, T., Alhede, M., Alhede, M., Eickhardt-Sørensen, S. R., Moser, C., Kühl, M., et al. (2013a). The in vivo biofilm. Trends Microbiol. 21, 466–474. doi: 10.1016/j.tim.2013.06.002
Bjarnsholt, T., Ciofu, O., Molin, S., Givskov, M., and Høiby, N. (2013b). Applying insights from biofilm biology to drug development - can a new approach be developed? Nat. Rev.Drug Discov. 12, 791–808. doi: 10.1038/nrd4000
Bonofiglio, L., Gagetti, P., García Gabarrot, G., Kaufman, S., Mollerach, M., Toresani, I., et al. (2018). Susceptibility to β-lactams in β-hemolytic streptococci. Rev. Argent. Microbiol. 50, 431–435. doi: 10.1016/j.ram.2017.11.002
Boonyayatra, S., and Pata, P. (2016). Antimicrobial resistance of biofilm-forming Streptococcus agalactiae Isolated from Bovine Mastitis. J. Vet. Sci. Technol. 7:5. doi: 10.4172/2157-7579.1000374
Borges, S., Silva, J., and Teixeira, P. (2012). Survival and biofilm formation by Group B streptococci in simulated vaginal fluid at different pHs. Antonie Van Leeuwenhoek 101, 677–682. doi: 10.1007/s10482-011-9666-y
Borriello, G., Werner, E., Roe, F., Kim, A. M., Ehrlich, G. D., and Stewart, P. S. (2004). Oxygen limitation contributes to antibiotic tolerance of Pseudomonas aeruginosa in biofilms. Antimicrob. Agents Chemother. 48, 2659–2664. doi: 10.1128/AAC.48.7.2659-2664.2004
Brandt, C. M., and Spellerberg, B. (2009). Human infections due to Streptococcus dysgalactiae subspecies equisimilis. Clin. Infect. Dis. 49, 766–772. doi: 10.1086/605085
Brinkmann, V., and Zychlinsky, A. (2012). Neutrophil extracellular traps: is immunity the second function of chromatin? J. Cell Biol. 198, 773–783. doi: 10.1083/jcb.201203170
Brouwer, S., Barnett, T. C., Rivera-Hernandez, T., Rohde, M., and Walker, M. J. (2016). Streptococcus pyogenes adhesion and colonization. FEBS Lett. 590, 3739–3757. doi: 10.1002/1873-3468.12254
Brown, S., Santa Maria, J. P. Jr., and Walker, S. (2013). Wall teichoic acids of gram-positive bacteria. Annu. Rev. Microbiol. 67, 313–336. doi: 10.1146/annurev-micro-092412-155620
Canchaya, C., Fournous, G., and Brüssow, H. (2004). The impact of prophages on bacterial chromosomes. Mol. Microbiol. 53, 9–18. doi: 10.1111/j.1365-2958.2004.04113.x
Cao, K., Li, N., Wang, H., Cao, X., He, J., Zhang, B., et al. (2018). Two zinc-binding domains in the transporter AdcA from Streptococcus pyogenes facilitate high-affinity binding and fast transport of zinc. J. Biol. Chem. 293, 6075–6089. doi: 10.1074/jbc.M117.818997
Caparon, M. G., Stephens, D. S., Olsén, A., and Scott, J. R. (1991). Role of M protein in adherence of group A streptococci. Infect. Immun. 59, 1811–1817. doi: 10.1128/IAI.59.5.1811-1817.1991
Carapetis, J. R., Steer, A. C., Mulholland, E. K., and Weber, M. (2005). The global burden of group A streptococcal diseases. Lancet Infect. Dis. 5, 685–694. doi: 10.1016/S1473-3099(05)70267-X
Carey, A. J., Tan, C. K., Mirza, S., Irving-Rodgers, H., Webb, R. I., Lam, A., et al. (2014). Infection and cellular defense dynamics in a novel 17β-estradiol murine model of chronic human group B Streptococcus genital tract colonization reveal a role for hemolysin in persistence and neutrophil accumulation. J. Immunol. 192, 1718–1731. doi: 10.4049/jimmunol.1202811
Carothers, K. E., Liang, Z., Mayfield, J., Donahue, D. L., Lee, M., Boggess, B., et al. (2020). The streptococcal protease SpeB antagonizes the biofilms of the human pathogen Staphylococcus aureus USA300 through cleavage of the Staphylococcal SdrC Protein. J. Bacteriol. 202:e00008-20. doi: 10.1128/JB.00008-20
Castilho, I. G., Dantas, S., Langoni, H., Araújo, J. P. Jr., Fernandes, A. Jr., Alvarenga, F., et al. (2017). Host-pathogen interactions in bovine mammary epithelial cells and HeLa cells by Staphylococcus aureus isolated from subclinical bovine mastitis. J. Dairy Sci. 100, 6414–6421. doi: 10.3168/jds.2017-12700
Chadha, T. (2014). Bacterial biofilms: survival mechanisms and antibiotic resistance. J. Bacteriol. Parasitol. 05, 5–8. doi: 10.4172/2155-9597.1000190
Chang, J. C., LaSarre, B., Jimenez, J. C., Aggarwal, C., and Federle, M. J. (2011). Two group A streptococcal peptide pheromones act through opposing Rgg regulators to control biofilm development. PLoS Pathog. 7:e1002190. doi: 10.1371/journal.ppat.1002190
Chatfield, C. H., Koo, H., and Quivey, R. G. (2005). The putative autolysin regulator LytR in Streptococcus mutans plays a role in cell division and is growth-phase regulated. Microbiology 151(Pt 2), 625–631. doi: 10.1099/mic.0.27604-0
Chen, L., and Wen, Y. M. (2011). The role of bacterial biofilm in persistent infections and control strategies. Int. J. Oral Sci. 3, 66–73. doi: 10.4248/IJOS11022
Chen, Y. M., Lu, P. S., Tsai, P. H., and Chiu, C. H. (2020). Functional analysis of a fibronectin binding protein of Streptococcus parasanguinis FW213. Curr. Microbiol. 77, 3430–3440. doi: 10.1007/s00284-020-02152-7
Chennapragada, S. S., Ramphul, K., Barnett, B. J., Mejias, S. G., and Lohana, P. (2018). A rare case of Streptococcus dysgalactiae Subsp. Dysgalactiae Human Zoonotic Infection. Cureus 10:e2901. doi: 10.7759/cureus.2901
Cho, K. H., and Caparon, M. G. (2005). Patterns of virulence gene expression differ between biofilm and tissue communities of Streptococcus pyogenes. Mol. Microbiol. 57, 1545–1556. doi: 10.1111/j.1365-2958.2005.04786.x
Claverys, J. P., and Martin, B. (2003). Bacterial “competence” genes: signatures of active transformation, or only remnants? Trends Microbiol. 11, 161–165. doi: 10.1016/s0966-842x(03)00064-7
Claverys, J. P., Prudhomme, M., and Martin, B. (2006). Induction of competence regulons as a general response to stress in gram-positive bacteria. Annu. Rev. Microbiol. 60, 451–475. doi: 10.1146/annurev.micro.60.080805.142139
Cobo-Angel, C. G., Jaramillo-Jaramillo, A. S., Palacio-Aguilera, M., Jurado-Vargas, L., Calvo-Villegas, E. A., Ospina-Loaiza, D. A., et al. (2019). Potential group B Streptococcus interspecies transmission between cattle and people in Colombian dairy farms. Sci. Rep. 9:14025. doi: 10.1038/s41598-019-50225-w
Cole, J. N., Barnett, T. C., Nizet, V., and Walker, M. J. (2011). Molecular insight into invasive group A streptococcal disease. Nat. Rev.Microbiol. 9, 724–736. doi: 10.1038/nrmicro2648
Collin, M., and Olsén, A. (2003). Extracellular enzymes with immunomodulating activities: variations on a theme in Streptococcus pyogenes. Infect. Immun. 71, 2983–2992. doi: 10.1128/iai.71.6.2983-2992.2003
Conley, J., Olson, M. E., Cook, L. S., Ceri, H., Phan, V., and Davies, H. D. (2003). Biofilm formation by group a streptococci: is there a relationship with treatment failure? J. Clin. Microbiol. 41, 4043–4048. doi: 10.1128/jcm.41.9.4043-4048.2003
Connolly, K. L., Roberts, A. L., Holder, R. C., and Reid, S. D. (2011). Dispersal of Group A streptococcal biofilms by the cysteine protease SpeB leads to increased disease severity in a murine model. PLoS One 6:e18984. doi: 10.1371/journal.pone.0018984
Cook, L. C., LaSarre, B., and Federle, M. J. (2013). Interspecies communication among commensal and pathogenic streptococci. mBio 4:e00382-13. doi: 10.1128/mBio.00382-13
Costa, O., Raaijmakers, J. M., and Kuramae, E. E. (2018). Microbial extracellular polymeric substances: ecological function and impact on soil aggregation. Front. Microbiol. 9:1636. doi: 10.3389/fmicb.2018.01636
Courtney, H. S., Ofek, I., Penfound, T., Nizet, V., Pence, M. A., Kreikemeyer, B., et al. (2009). Relationship between expression of the family of M proteins and lipoteichoic acid to hydrophobicity and biofilm formation in Streptococcus pyogenes. PLoS One 4:e4166. doi: 10.1371/journal.pone.0004166
Crotty Alexander, L. E., Maisey, H. C., Timmer, A. M., Rooijakkers, S. H., Gallo, R. L., von Köckritz-Blickwede, M., et al. (2010). M1T1 group A streptococcal pili promote epithelial colonization but diminish systemic virulence through neutrophil extracellular entrapment. J. Mol. Med. 88, 371–381. doi: 10.1007/s00109-009-0566-9
Cue, D., Lei, M. G., and Lee, C. Y. (2012). Genetic regulation of the intercellular adhesion locus in staphylococci. Front. Cell. Infect. Microbiol. 2:38. doi: 10.3389/fcimb.2012.00038
Danne, C., and Dramsi, S. (2012). Pili of gram-positive bacteria: roles in host colonization. Res. Microbiol. 163, 645–658. doi: 10.1016/j.resmic.2012.10.012
Dekker, A. F. L. (2016). An Update on the Streptococcus bovis group: classification, identification, and disease associations. J. Clin. Microbiol. 54, 1694–1699. doi: 10.1128/JCM.02977-15
Del Pozo, J. L. (2018). Biofilm-related disease. Expert Rev. Anti Infect. Ther. 16, 51–65. doi: 10.1080/14787210.2018.1417036
Derré-Bobillot, A., Cortes-Perez, N. G., Yamamoto, Y., Kharrat, P., Couvé, E., Da Cunha, V., et al. (2013). Nuclease A (Gbs0661), an extracellular nuclease of Streptococcus agalactiae, attacks the neutrophil extracellular traps and is needed for full virulence. Mol. Microbiol. 89, 518–531. doi: 10.1111/mmi.12295
Diaz-Torres, M. L., and Russell, R. R. (2001). HtrA protease and processing of extracellular proteins of Streptococcus mutans. FEMS Microbiol. Lett. 204, 23–28. doi: 10.1111/j.1574-6968.2001.tb10856.x
Dickschat, J. S. (2010). Quorum sensing and bacterial biofilms. Nat. Prod. Rep. 27, 343–369. doi: 10.1039/b804469b
Dinkla, K., Rohde, M., Jansen, W. T., Carapetis, J. R., Chhatwal, G. S., and Talay, S. R. (2003). Streptococcus pyogenes recruits collagen via surface-bound fibronectin: a novel colonization and immune evasion mechanism. Mol. Microbiol. 47, 861–869. doi: 10.1046/j.1365-2958.2003.03352.x
Doern, C. D., Roberts, A. L., Hong, W., Nelson, J., Lukomski, S., Swords, W. E., et al. (2009). Biofilm formation by group A Streptococcus: a role for the streptococcal regulator of virulence (Srv) and streptococcal cysteine protease (SpeB). Microbiology 155(Pt 1), 46–52. doi: 10.1099/mic.0.021048-0
Döhrmann, S., Anik, S., Olson, J., Anderson, E. L., Etesami, N., No, H., et al. (2014). Role for streptococcal collagen-like protein 1 in M1T1 group A Streptococcus resistance to neutrophil extracellular traps. Infect. Immun. 82, 4011–4020. doi: 10.1128/IAI.01921-14
Donlan, R. M. (2001). Biofilm formation: a clinically relevant microbiological process. Clin. Infect. Dis. 33, 1387–1392. doi: 10.1086/322972
Donlan, R. M. (2002). Biofilms: microbial life on surfaces. Emerg. Infect. Dis. 8, 881–890. doi: 10.3201/eid0809.020063
Donlan, R. M., and Costerton, J. W. (2002). Biofilms: survival mechanisms of clinically relevant microorganisms. Clin. Microbiol. Rev. 15, 167–193. doi: 10.1128/cmr.15.2.167-193.2002
Dramsi, S., Caliot, E., Bonne, I., Guadagnini, S., Prévost, M. C., Kojadinovic, M., et al. (2006). Assembly and role of pili in group B streptococci. Mol. Microbiol. 60, 1401–1413. doi: 10.1111/j.1365-2958.2006.05190.x
Dunne, D. W., Resnick, D., Greenberg, J., Krieger, M., and Joiner, K. A. (1994). The type I macrophage scavenger receptor binds to gram-positive bacteria and recognizes lipoteichoic acid. Proc. Nat. Acad. Sci. U.S.A. 91, 1863–1867. doi: 10.1073/pnas.91.5.1863
D’Urzo, N., Martinelli, M., Pezzicoli, A., De Cesare, V., Pinto, V., Margarit, I., et al. (2014). Acidic pH strongly enhances in vitro biofilm formation by a subset of hypervirulent ST-17 Streptococcus agalactiae strains. Appl. Environ. Microbiol. 80, 2176–2185. doi: 10.1128/AEM.03627-13
Emamalipour, M., Seidi, K., Zununi Vahed, S., Jahanban-Esfahlan, A., Jaymand, M., Majdi, H., et al. (2020). Horizontal gene transfer: from evolutionary flexibility to disease progression. Front. Cell Dev. Biol. 8:229. doi: 10.3389/fcell.2020.00229
Eraso, J. M., Kachroo, P., Olsen, R. J., Beres, S. B., Zhu, L., Badu, T., et al. (2020). Genetic heterogeneity of the Spy1336/R28-Spy1337 virulence axis in Streptococcus pyogenes and effect on gene transcript levels and pathogenesis. PLoS One 15:e0229064. doi: 10.1371/journal.pone.0229064
Falaleeva, M., Zurek, O. W., Watkins, R. L., Reed, R. W., Ali, H., Sumby, P., et al. (2014). Transcription of the Streptococcus pyogenes hyaluronic acid capsule biosynthesis operon is regulated by previously unknown upstream elements. Infect. Immun. 82, 5293–5307. doi: 10.1128/IAI.02035-14
Fiedler, T., Köller, T., and Kreikemeyer, B. (2015). Streptococcus pyogenes biofilms-formation, biology, and clinical relevance. Front. Cell. Infect. Microbiol, 5:15. doi: 10.3389/fcimb.2015.00015
Fiedler, T., Riani, C., Koczan, D., Standar, K., Kreikemeyer, B., and Podbielski, A. (2013). Protective mechanisms of respiratory tract Streptococci against Streptococcus pyogenes biofilm formation and epithelial cell infection. Appl. Environ. Microbiol. 79, 1265–1276. doi: 10.1128/AEM.03350-12
Fischer, W. (1988). Physiology of lipoteichoic acids in bacteria. Adv. Microb. Physiol. 29, 233–302. doi: 10.1016/S0065-2911(08)60349-5
Flemming, H. C., and Wingender, J. (2010). The biofilm matrix. Nat. Rev.Microbiol. 8, 623–633. doi: 10.1038/nrmicro2415
Florez Salamanca, E. J., and Klein, M. I. (2018). Extracellular matrix influence in Streptococcus mutans gene expression in a cariogenic biofilm. Mol. Oral Microbiol. 33, 181–193. doi: 10.1111/omi.12212
Fontaine, L., Boutry, C., de Frahan, M. H., Delplace, B., Fremaux, C., Horvath, P., et al. (2010). A novel pheromone quorum-sensing system controls the development of natural competence in Streptococcus thermophilus and Streptococcus salivarius. J. Bacteriol. 192, 1444–1454. doi: 10.1128/JB.01251-09
Fontaine, L., Wahl, A., Fléchard, M., Mignolet, J., and Hols, P. (2015). Regulation of competence for natural transformation in streptococci. Infect.Genet. Evol. 33, 343–360. doi: 10.1016/j.meegid.2014.09.010
Fowler, D. M., Koulov, A. V., Balch, W. E., and Kelly, J. W. (2007). Functional amyloid–from bacteria to humans. Trends Biochem. Sci. 32, 217–224. doi: 10.1016/j.tibs.2007.03.003
Frost, H. R., Sanderson-Smith, M., Walker, M., Botteaux, A., and Smeesters, P. R. (2018). Group A streptococcal M-like proteins: from pathogenesis to vaccine potential. FEMS Microbiol. Rev. 42, 193–204. doi: 10.1093/femsre/fux057
Galante, J., Ho, A. C., Tingey, S., and Charalambous, B. M. (2015). Quorum sensing and biofilms in the pathogen, Streptococcus pneumoniae. Curr. Pharm. Des. 21, 25–30. doi: 10.2174/1381612820666140905113336
Garcia-Gonzalo, D., and Pagán, R. (2015). Influence of environmental factors on bacterial biofilm formation in the food industry: a review. Postdoc J. 3, 3–13. doi: 10.14304/SURYA.JPR.V3N6.2
Gardan, R., Besset, C., Gitton, C., Guillot, A., Fontaine, L., Hols, P., et al. (2013). Extracellular life cycle of ComS, the competence-stimulating peptide of Streptococcus thermophilus. J. Bacteriol. 195, 1845–1855. doi: 10.1128/JB.02196-12
Gardan, R., Besset, C., Guillot, A., Gitton, C., and Monnet, V. (2009). The oligopeptide transport system is essential for the development of natural competence in Streptococcus thermophilus strain LMD-9. J. Bacteriol. 191, 4647–4655. doi: 10.1128/JB.00257-09
Garnett, J. A., Martínez-Santos, V. I., Saldaña, Z., Pape, T., Hawthorne, W., Chan, J., et al. (2012). Structural insights into the biogenesis and biofilm formation by the Escherichia coli common pilus. Proc. Natl. Acad. Sci. U.S.A. 109, 3950–3955. doi: 10.1073/pnas.1106733109
Garnett, J. A., and Matthews, S. (2012). Interactions in bacterial biofilm development: a structural perspective. Curr. Protein Peptide Sci. 13, 739–755. doi: 10.2174/138920312804871166
Garrett, T. R., Bhakoo, M., and Zhang, Z. (2008). Bacterial adhesion and biofilms on surfaces. Prog. Nat. Sci. 18, 1049–1056. doi: 10.1016/j.pnsc.2008.04.001
Genteluci, G. L., Silva, L. G., Souza, M. C., Glatthardt, T., de Mattos, M. C., Ejzemberg, R., et al. (2015). Assessment and characterization of biofilm formation among human isolates of Streptococcus dysgalactiae subsp. equisimilis. Int. J. Med. Microbiol. 305, 937–947. doi: 10.1016/j.ijmm.2015.10.004
Giuliano, S., Rubini, G., Conte, A., Goldoni, P., Falcone, M., Vena, A., et al. (2012). Streptococcus anginosus group disseminated infection: case report and review of literature. Infez. Med. 20, 145–154.
Gomes, F., Saavedra, M. J., and Henriques, M. (2016). Bovine mastitis disease/pathogenicity: evidence of the potential role of microbial biofilms. Pathog. Dis. 74:ftw006. doi: 10.1093/femspd/ftw006
Guilhen, C., Forestier, C., and Balestrino, D. (2017). Biofilm dispersal: multiple elaborate strategies for dissemination of bacteria with unique properties. Mol. Microbiol. 105, 188–210. doi: 10.1111/mmi.13698
Gupta, P., Sarkar, S., Das, B., Bhattacharjee, S., and Tribedi, P. (2016). Biofilm, pathogenesis and prevention–a journey to break the wall: a review. Arch. Microbiol. 198, 1–15. doi: 10.1007/s00203-015-1148-6
Haenni, M., Saras, E., Bertin, S., Leblond, P., Madec, J. Y., and Payot, S. (2010). Diversity and mobility of integrative and conjugative elements in bovine isolates of Streptococcus agalactiae, S. dysgalactiae subsp. dysgalactiae, and S. uberis. Appl. Environ. Microbiol. 76, 7957–7965. doi: 10.1128/AEM.00805-10
Hall-Stoodley, L., Costerton, J. W., and Stoodley, P. (2004). Bacterial biofilms: from the natural environment to infectious diseases. Nat. Rev.Microbiol. 2, 95–108. doi: 10.1038/nrmicro821
Hansen, S. M., Uldbjerg, N., Kilian, M., and Sørensen, U. B. (2004). Dynamics of Streptococcus agalactiae colonization in women during and after pregnancy and in their infants. J. Clin. Microbiol. 42, 83–89. doi: 10.1128/jcm.42.1.83-89.2004
Henriques-Normark, B., and Tuomanen, E. I. (2013). The pneumococcus: epidemiology, microbiology, and pathogenesis. Cold Spring Harbor Perspec. Med. 3:a010215. doi: 10.1101/cshperspect.a010215
Higgins, M. J., and Novak, J. T. (1997). Characterization of exocellular protein and its role in bioflocculation. J. Environ. Eng. 123, 479–485. doi: 10.1061/(ASCE)073393721997123:5(479)
Ho, Y. R., Li, C. M., Yu, C. H., Lin, Y. J., Wu, C. M., Harn, I. C., et al. (2013). The enhancement of biofilm formation in Group B streptococcal isolates at vaginal pH. Med. Microbiol. Immunol. 202, 105–115. doi: 10.1007/s00430-012-0255-0
Hoare, A., Marsh, P. D., and Diaz, P. I. (2017). Ecological therapeutic opportunities for oral diseases. Microbiol. Spectr. 5:10.1128/microbiolsec.BAD–0006–2016. doi: 10.1128/microbiolspec.BAD-0006-2016
Høiby, N., Bjarnsholt, T., Givskov, M., Molin, S., and Ciofu, O. (2010). Antibiotic resistance of bacterial biofilms. Int. J. Antimicrob. Agents 35, 322–332. doi: 10.1016/j.ijantimicag.2009.12.011
Horiuk, Y., Kukhtyn, M., Kovalenko, V., Kornienko, L., Horiuk, V., and Liniichuk, N. (2019). Biofilm formation in bovine mastitis pathogens and the effect on them of antimicrobial drugs. May Indep. J. Manag. Prod. 10:897. doi: 10.14807/ijmp.v10i7.1012
Humphreys, G. J., and McBain, A. J. (2019). Antagonistic effects of Streptococcus and Lactobacillus probiotics in pharyngeal biofilms. Lett. Appl. Microbiol. 68, 303–312. doi: 10.1111/lam.13133
Hussain, M., Herrmann, M., von Eiff, C., Perdreau-Remington, F., and Peters, G. (1997). A 140-kilodalton extracellular protein is essential for the accumulation of Staphylococcus epidermidis strains on surfaces. Infect. Immun. 65, 519–524. doi: 10.1128/IAI.65.2.519-524.1997
Hyink, O., Wescombe, P. A., Upton, M., Ragland, N., Burton, J. P., and Tagg, J. R. (2007). Salivaricin A2 and the novel lantibiotic salivaricin B are encoded at adjacent loci on a 190-kilobase transmissible megaplasmid in the oral probiotic strain Streptococcus salivanus K12. Appl. Environ. Microbiol. 73, 1107–1113. doi: 10.1128/AEM.02265-06
Hytönen, J., Haataja, S., Gerlach, D., Podbielski, A., and Finne, J. (2001). The SpeB virulence factor of Streptococcus pyogenes, a multifunctional secreted and cell surface molecule with strepadhesin, laminin-binding and cysteine protease activity. Mol. Microbiol. 39, 512–519. doi: 10.1046/j.1365-2958.2001.02269.x
Ibáñez de Aldecoa, A. L., Zafra, O., and González-Pastor, J. E. (2017). Mechanisms and regulation of extracellular DNA Release and its biological roles in microbial communities. Front. Microbiol. 8:1390. doi: 10.3389/fmicb.2017.01390
Isaacs, D., and Dobson, S. R. M. (2016). Severe Group A streptococcal infections. Curr. Opin. Infect. Dis. 2, 453–456. doi: 10.1097/00001432-198906000-00022
Jamal, M., Ahmad, W., Andleeb, S., Jalil, F., Imran, M., Nawaz, M. A., et al. (2018). Bacterial biofilm and associated infections. J. Chin. Med. Assoc. 81, 7–11. doi: 10.1016/j.jcma.2017.07.012
Jefferson, K. K. (2004). What drives bacteria to produce a biofilm? FEMS Microbiol. Lett. 236, 163–173. doi: 10.1016/j.femsle.2004.06.005
Jimenez, J. C., and Federle, M. J. (2014). Quorum sensing in group A Streptococcus. Front. Cell. Infect. Microbiol. 4:127. doi: 10.3389/fcimb.2014.00127
Johnston, C., Polard, P., and Claverys, J. P. (2013). The DpnI/DpnII pneumococcal system, defense against foreign attack without compromising genetic exchange. Mobile Genet. Elements 3:e25582. doi: 10.4161/mge.25582
Jordal, S., Glambek, M., Oppegaard, O., and Kittang, B. R. (2015). New tricks from an old cow: infective endocarditis caused by Streptococcus dysgalactiae subsp. dysgalactiae. J. Clin. Microbiol. 53, 731–734. doi: 10.1128/JCM.02437-14
Junges, R., Salvadori, G., Shekhar, S., Åmdal, H. A., Periselneris, J. N., Chen, T., et al. (2017). A quorum-sensing system that Regulates Streptococcus pneumoniae biofilm formation and surface polysaccharide production. mSphere 2:e00324-17. doi: 10.1128/mSphere.00324-17
Kaczorek, E., Małaczewska, J., Wójcik, R., and Siwicki, A. K. (2017). Biofilm production and other virulence factors in Streptococcus spp. isolated from clinical cases of bovine mastitis in Poland. BMC Vet. Res. 13:398. doi: 10.1186/s12917-017-1322-y
Kang, S. S., Sim, J. R., Yun, C. H., and Han, S. H. (2016). Lipoteichoic acids as a major virulence factor causing inflammatory responses via Toll-like receptor 2. Arch. Pharm. Res. 39, 1519–1529. doi: 10.1007/s12272-016-0804-y
Kanoh, S., and Rubin, B. K. (2010). Mechanisms of action and clinical application of macrolides as immunomodulatory medications. Clin. Microbiol. Rev. 23, 590–615. doi: 10.1128/CMR.00078-09
Kaplan, J. B. (2010). Biofilm dispersal: mechanisms, clinical implications, and potential therapeutic uses. J. Dent. Res. 89, 205–218. doi: 10.1177/0022034509359403
Kaur, H., Kumar, P., Ray, P., Kaur, J., and Chakraborti, A. (2009). Biofilm formation in clinical isolates of group B streptococci from north India. Microb. Pathog. 46, 321–327. doi: 10.1016/j.micpath.2009.04.004
Khan, R., Rukke, H. V., Ricomini Filho, A. P., Fimland, G., Arntzen, M. Ø, Thiede, B., et al. (2012). Extracellular identification of a processed type II ComR/ComS pheromone of Streptococcus mutans. J. Bacteriol. 194, 3781–3788. doi: 10.1128/JB.00624-12
Khatoon, Z., McTiernan, C. D., Suuronen, E. J., Mah, T. F., and Alarcon, E. I. (2018). Bacterial biofilm formation on implantable devices and approaches to its treatment and prevention. Heliyon 4:e01067. doi: 10.1016/j.heliyon.2018.e01067
Kim, D. Y., and Kim, K. K. (2005). Structure and function of HtrA family proteins, the key players in protein quality control. J. Biochem. Mol. Biol. 38, 266–274. doi: 10.5483/bmbrep.2005.38.3.266
Klaas, I. C., and Zadoks, R. N. (2018). An update on environmental mastitis: challenging perceptions. Transbound. Emerg. Dis. 65(Suppl. 1), 166–185. doi: 10.1111/tbed.12704
Kleerebezem, M., Quadri, L. E., Kuipers, O. P., and de Vos, W. M. (1997). Quorum sensing by peptide pheromones and two-component signal-transduction systems in Gram-positive bacteria. Mol. Microbiol. 24, 895–904. doi: 10.1046/j.1365-2958.1997.4251782.x
Koh, T. H., Sng, L. H., Yuen, S. M., Thomas, C. K., Tan, P. L., Tan, S. H., et al. (2009). Streptococcal cellulitis following preparation of fresh raw seafood. Zoonoses Public Health 56, 206–208. doi: 10.1111/j.1863-2378.2008.01213.x
Kojima, A., Nakano, K., Wada, K., Takahashi, H., Katayama, K., Yoneda, M., et al. (2012). Infection of specific strains of Streptococcus mutans, oral bacteria, confers a risk of ulcerative colitis. Sci. Rep. 2:332. doi: 10.1038/srep00332
Kokare, C. R., Chakraborty, S., Khopade, A. N., and Mahadik, K. R. (2009). Biofilm: importance and applications. Indian J. Biotechnol. 8, 159–168.
Konto-Ghiorghi, Y., Mairey, E., Mallet, A., Duménil, G., Caliot, E., Trieu-Cuot, P., et al. (2009). Dual role for pilus in adherence to epithelial cells and biofilm formation in Streptococcus agalactiae. PLoS Pathog. 5:e1000422. doi: 10.1371/journal.ppat.1000422
Koo, H., Xiao, J., Klein, M. I., and Jeon, J. G. (2010). Exopolysaccharides produced by Streptococcus mutans glucosyltransferases modulate the establishment of microcolonies within multispecies biofilms. J. Bacteriol. 192, 3024–3032. doi: 10.1128/JB.01649-09
Kostakioti, M., Hadjifrangiskou, M., and Hultgren, S. J. (2013). Bacterial biofilms: development, dispersal, and therapeutic strategies in the dawn of the postantibiotic era. Cold Spring Harbor Perspect. Med. 3:a010306. doi: 10.1101/cshperspect.a010306
Kragh, K. N., Hutchison, J. B., Melaugh, G., Rodesney, C., Roberts, A. E., Irie, Y., et al. (2016). Role of multicellular aggregates in biofilm formation. mBio 7:e00237. doi: 10.1128/mBio.00237-16
Krantz, A. M., Ratnaraj, F., Velagapudi, M., Krishnan, M., Gujjula, N. R., Foral, P. A., et al. (2017). Streptococcus gordonii empyema: a case report and review of empyema. Cureus 9:e1159. doi: 10.7759/cureus.1159
Kumar, A., Alam, A., Rani, M., Ehtesham, N. Z., and Hasnain, S. E. (2017). Biofilms: Survival and defense strategy for pathogens. Int. J. Med. Microbiol. 307, 481–489. doi: 10.1016/j.ijmm.2017.09.016
Lages, A. C., Mustopa, A. Z., Sukmarini, L., and Suharsono. (2015). Cloning and expression of plantaricin W Produced by Lactobacillus plantarum U10 Isolate from “Tempoyak” indonesian fermented food as immunity protein in Lactococcus lactis. Appl. Biochem. Biotechnol. 177, 909–922. doi: 10.1007/s12010-015-1786-9
Lal, D., Verma, M., and Lal, R. (2011). Exploring internal features of 16S rRNA gene for identification of clinically relevant species of the genus Streptococcus. Ann. Clin. Microbiol. Antimicrob. 10:28. doi: 10.1186/1476-0711-10-28
Lasa, I., and Penadés, J. R. (2006). Bap: a family of surface proteins involved in biofilm formation. Res. Microbiol. 157, 99–107. doi: 10.1016/j.resmic.2005.11.003
Lasarre, B., Aggarwal, C., and Federle, M. J. (2012). Antagonistic Rgg regulators mediate quorum sensing via competitive DNA binding in Streptococcus pyogenes. mBio 3:e00333-12. doi: 10.1128/mBio.00333-12
Lembke, C., Podbielski, A., Hidalgo-Grass, C., Jonas, L., Hanski, E., and Kreikemeyer, B. (2006). Characterization of biofilm formation by clinically relevant serotypes of group A streptococci. Appl. Environ. Microbiol. 72, 2864–2875. doi: 10.1128/AEM.72.4.2864-2875.2006
Li, Y. H., Tang, N., Aspiras, M. B., Lau, P. C., Lee, J. H., Ellen, R. P., et al. (2002). A quorum-sensing signaling system essential for genetic competence in Streptococcus mutans is involved in biofilm formation. J. Bacteriol. 184, 2699–2708. doi: 10.1128/jb.184.10.2699-2708.2002
Liao, S., Klein, M. I., Heim, K. P., Fan, Y., Bitoun, J. P., Ahn, S. J., et al. (2014). Streptococcus mutans extracellular DNA is upregulated during growth in biofilms, actively released via membrane vesicles, and influenced by components of the protein secretion machinery. J. Bacteriol. 196, 2355–2366. doi: 10.1128/JB.01493-14
Lima, B. P., Kho, K., Nairn, B. L., Davies, J. R., Svensäter, G., Chen, R., et al. (2019). Streptococcus gordonii Type I lipoteichoic acid contributes to surface protein biogenesis. mSphere 4:e00814-19. doi: 10.1128/mSphere.00814-19
Lindgren, P. E., Signäs, C., Rantamäki, L., and Lindberg, M. (1994). A fibronectin-binding protein from Streptococcus equisimilis: characterization of the gene and identification of the binding domain. Vet. Microbiol. 41, 235–247. doi: 10.1016/0378-1135(94)90104-x
Lizano, S., Luo, F., and Bessen, D. E. (2007). Role of streptococcal T antigens in superficial skin infection. J. Bacteriol. 189, 1426–1434. doi: 10.1128/JB.01179-06
Loo, C. Y., Corliss, D. A., and Ganeshkumar, N. (2000). Streptococcus gordonii biofilm formation: identification of genes that code for biofilm phenotypes. J. Bacteriol. 182, 1374–1382. doi: 10.1128/jb.182.5.1374-1382.2000
Lu, T. K., and Collins, J. J. (2007). Dispersing biofilms with engineered enzymatic bacteriophage. Proc. Natl. Acad. Sci. U S.A. 104, 11197–11202. doi: 10.1073/pnas.0704624104
Lukomski, S., Bachert, B. A., Squeglia, F., and Berisio, R. (2017). Collagen-like proteins of pathogenic streptococci. Mol. Microbiol. 103, 919–930. doi: 10.1111/mmi.13604
Lyon, W. R., and Caparon, M. G. (2004). Role for serine protease HtrA (DegP) of Streptococcus pyogenes in the biogenesis of virulence factors SpeB and the hemolysin streptolysin S. Infect. Immun. 72, 1618–1625. doi: 10.1128/iai.72.3.1618-1625.2004
Ma, J. S., Chen, S. Y., and Lo, H. H. (2017). Biofilm formation of beta-hemolytic group G Streptococcus dysgalactiae subspecies equisimilis isolates and its association with emm polymorphism. APMIS 125, 1027–1032. doi: 10.1111/apm.12746
Macià, M. D., Rojo-Molinero, E., and Oliver, A. (2014). Antimicrobial susceptibility testing in biofilm-growing bacteria. Clin. Microbiol. Infect. 20, 981–990. doi: 10.1111/1469-0691.12651
Mah, T. F., and O’Toole, G. A. (2001). Mechanisms of biofilm resistance to antimicrobial agents. Trends Microbiol. 9, 34–39. doi: 10.1016/s0966-842x(00)01913-2
Manetti, A. G., Köller, T., Becherelli, M., Buccato, S., Kreikemeyer, B., Podbielski, A., et al. (2010). Environmental acidification drives S. pyogenes pilus expression and microcolony formation on epithelial cells in a FCT-dependent manner. PLoS One 5:e13864. doi: 10.1371/journal.pone.0013864
Manetti, A. G., Zingaretti, C., Falugi, F., Capo, S., Bombaci, M., Bagnoli, F., et al. (2007). Streptococcus pyogenes pili promote pharyngeal cell adhesion and biofilm formation. Mol. Microbiol. 64, 968–983. doi: 10.1111/j.1365-2958.2007.05704.x
Marks, L. R., Mashburn-Warren, L., Federle, M. J., and Hakansson, A. P. (2014a). Streptococcus pyogenes biofilm growth in vitro and in vivo and its role in colonization, virulence, and genetic exchange. J. Infect. Dis. 210, 25–34. doi: 10.1093/infdis/jiu058
Marks, L. R., Reddinger, R. M., and Hakansson, A. P. (2014b). Biofilm formation enhances fomite survival of Streptococcus pneumoniae and Streptococcus pyogenes. Infect. Immun. 82, 1141–1146. doi: 10.1128/IAI.01310-13
Mashburn-Warren, L., Morrison, D. A., and Federle, M. J. (2010). A novel double-tryptophan peptide pheromone controls competence in Streptococcus spp. via an Rgg regulator. Mol. Microbiol. 78, 589–606. doi: 10.1111/j.1365-2958.2010.07361.x
Matysik, A., and Kline, K. A. (2019). Streptococcus pyogenes capsule promotes microcolony-independent biofilm formation. J. Bacteriol. 201:e00052-19. doi: 10.1128/JB.00052-19
McNeilly, C. L., and McMillan, D. J. (2014). Horizontal gene transfer and recombination in Streptococcus dysgalactiae subsp. equisimilis. Front. Microbiol. 5:676. doi: 10.3389/fmicb.2014.00676
McShan, W. M., and Nguyen, S. V. (2016). “The bacteriophages of Streptococcus pyogenes,” in Streptococcus pyogenes: Basic Biology to Clinical Manifestations, eds J. J. Ferretti, D. L. Stevens, and V. A. Fischetti (Oklahoma City, OK: University of Oklahoma Health Sciences Center).
Melchior, M. B., Vaarkamp, H., and Fink-Gremmels, J. (2006). Biofilms: a role in recurrent mastitis infections? Vet. J. 171, 398–407. doi: 10.1016/j.tvjl.2005.01.006
Melin, M., Trzciński, K., Antonio, M., Meri, S., Adegbola, R., Kaijalainen, T., et al. (2010). Serotype-related variation in susceptibility to complement deposition and opsonophagocytosis among clinical isolates of Streptococcus pneumoniae. Infect. Immun. 78, 5252–5261. doi: 10.1128/IAI.00739-10
Merritt, J., Qi, F., Goodman, S. D., Anderson, M. H., and Shi, W. (2003). Mutation of luxS affects biofilm formation in Streptococcus mutans. Infect. Immun. 71, 1972–1979. doi: 10.1128/iai.71.4.1972-1979.2003
Mora, M., Bensi, G., Capo, S., Falugi, F., Zingaretti, C., Manetti, A. G., et al. (2005). Group A Streptococcus produce pilus-like structures containing protective antigens and Lancefield T antigens. Proc. Natl. Acad. Sci. U.S.A. 102, 15641–15646. doi: 10.1073/pnas.0507808102
Morath, S., von Aulock, S., and Hartung, T. (2005). Structure/function relationships of lipoteichoic acids. J. Endotoxin Res. 11, 348–356. doi: 10.1179/096805105X67328
Moroi, H., Kimura, K., Kotani, T., Tsuda, H., Banno, H., Jin, W., et al. (2019). Isolation of group B Streptococcus with reduced β-lactam susceptibility from pregnant women. Emerg. Microb. Infect. 8, 2–7. doi: 10.1080/22221751.2018.1557987
Moulin, P., Patron, K., Cano, C., Zorgani, M. A., Camiade, E., Borezée-Durant, E., et al. (2016). The Adc/Lmb system mediates zinc acquisition in Streptococcus agalactiae and contributes to bacterial growth and survival. J. Bacteriol. 198, 3265–3277. doi: 10.1128/JB.00614-16
Narayana, S. V. V. S., and Srihari, S. V. V. (2019). A review on surface modifications and coatings on implants to prevent biofilm. Regen. Eng. Transl. Med. 6, 330–346. doi: 10.1007/s40883-019-00116-3
Neuhaus, F. C., and Baddiley, J. (2003). A continuum of anionic charge: structures and functions of d-alanyl-teichoic acids in gram-positive bacteria. Microbiol. Mol. Biol. Rev. 67, 686–723. doi: 10.1128/mmbr.67.4.686-723.2003
Noguchi, S., Yatera, K., Kawanami, T., Yamasaki, K., Naito, K., Akata, K., et al. (2015). The clinical features of respiratory infections caused by the Streptococcus anginosus group. BMC Pulm. Med. 15:133. doi: 10.1186/s12890-015-0128-6
Nur, A., Hirota, K., Yumoto, H., Hirao, K., Liu, D., Takahashi, K., et al. (2013). Effects of extracellular DNA and DNA-binding protein on the development of a Streptococcus intermedius biofilm. J. Appl. Microbiol. 115, 260–270. doi: 10.1111/jam.12202
Ogawa, T., Terao, Y., Okuni, H., Ninomiya, K., Sakata, H., Ikebe, K., et al. (2011). Biofilm formation or internalization into epithelial cells enable Streptococcus pyogenes to evade antibiotic eradication in patients with pharyngitis. Microb. Pathog. 51, 58–68. doi: 10.1016/j.micpath.2011.03.009
Oli, M. W., Otoo, H. N., Crowley, P. J., Heim, K. P., Nascimento, M. M., Ramsook, C. B., et al. (2012). Functional amyloid formation by Streptococcus mutans. Microbiology 158(Pt 12), 2903–2916. doi: 10.1099/mic.0.060855-0
Oliver-Kozup, H. A., Elliott, M., Bachert, B. A., Martin, K. H., Reid, S. D., Schwegler-Berry, D. E., et al. (2011). The streptococcal collagen-like protein-1 (Scl1) is a significant determinant for biofilm formation by group A Streptococcus. BMC Microbiol. 11:262. doi: 10.1186/1471-2180-11-262
Olsen, I. (2015). Biofilm-specific antibiotic tolerance and resistance. Eur. J. Clin. Microbiol. Infect. Dis. 34, 877–886. doi: 10.1007/s10096-015-2323-z
Olson, M. E., Ceri, H., Morck, D. W., Buret, A. G., and Read, R. R. (2002). Biofilm bacteria: formation and comparative susceptibility to antibiotics. Can. J. Vet. Res. 66, 86–92.
O’Neill, E., Humphreys, H., and O’Gara, J. P. (2009). Carriage of both the fnbA and fnbB genes and growth at 37 degrees C promote FnBP-mediated biofilm development in meticillin-resistant Staphylococcus aureus clinical isolates. J. Med. Microbiol. 58(Pt 4), 399–402. doi: 10.1099/jmm.0.005504-0
Oppegaard, O., Mylvaganam, H., Skrede, S., and Kittang, B. R. (2018). Exploring the arthritogenicity of Streptococcus dysgalactiae subspecies equisimilis. BMC Microbiol. 18:17. doi: 10.1186/s12866-018-1160-5
Oppegaard, O., Mylvaganam, H., Skrede, S., Lindemann, P. C., and Kittang, B. R. (2017). Emergence of a Streptococcus dysgalactiae subspecies equisimilis stG62647-lineage associated with severe clinical manifestations. Sci. Rep. 7:7589. doi: 10.1038/s41598-017-08162-z
Otzen, D., and Nielsen, P. H. (2008). We find them here, we find them there: functional bacterial amyloid. Cell. Mol. Life Sci. 65, 910–927. doi: 10.1007/s00018-007-7404-4
Pang, M., Sun, L., He, T., Bao, H., Zhang, L., Zhou, Y., et al. (2017). Molecular and virulence characterization of highly prevalent Streptococcus agalactiae circulated in bovine dairy herds. Vte. Res. 48:65. doi: 10.1186/s13567-017-0461-2
Park, M. J., Eun, I. S., Jung, C. Y., Ko, Y. C., Kim, Y. J., Kim, C. K., et al. (2012). Streptococcus dysgalactiae subspecies dysgalactiae infection after total knee arthroplasty: a case report. Knee Surg. Relat. Res. 24, 120–123. doi: 10.5792/ksrr.2012.24.2.120
Parks, T., Barrett, L., and Jones, N. (2015). Invasive streptococcal disease: a review for clinicians. Br. Med. Bull. 115, 77–89. doi: 10.1093/bmb/ldv027
Parsek, M. R., and Greenberg, E. P. (2005). Sociomicrobiology: the connections between quorum sensing and biofilms. Trends Microbiol. 13, 27–33. doi: 10.1016/j.tim.2004.11.007
Patel, R. (2005). Biofilms and antimicrobial resistance. Clin. Orthop. Relat. Res. 41–47. doi: 10.1097/01.blo.0000175714.68624.74
Patras, K. A., Derieux, J., Al-Bassam, M. M., Adiletta, N., Vrbanac, A., Lapek, J. D., et al. (2018). Group B Streptococcus Biofilm regulatory protein A contributes to bacterial physiology and innate immune resistance. J. Infect. Dis. 218, 1641–1652. doi: 10.1093/infdis/jiy341
Pelling, H., Nzakizwanayo, J., Milo, S., Denham, E. L., MacFarlane, W. M., Bock, L. J., et al. (2019). Bacterial biofilm formation on indwelling urethral catheters. Lett. Appl. Microbiol. 68, 277–293. doi: 10.1111/lam.13144
Peters, J. (2017). Staphylococcal and streptococcal infections. Medicine 45, 802–805. doi: 10.1016/j.mpmed.2017.09.010
Petersen, F. C., Pecharki, D., and Scheie, A. A. (2004). Biofilm mode of growth of Streptococcus intermedius favored by a competence-stimulating signaling peptide. J. Bacteriol. 186, 6327–6331. doi: 10.1128/JB.186.18.6327-6331.2004
Pichichero, M. E., and Casey, J. R. (2007). Systematic review of factors contributing to penicillin treatment failure in Streptococcus pyogenes pharyngitis. Otolaryngol. Head Neck Surg. 137, 851–857. doi: 10.1016/j.otohns.2007.07.033
Pires, R., Rolo, D., Gama-Norton, L., Morais, A., Lito, L., Salgado, M. J., et al. (2005). Group A Streptococci from carriage and disease in Portugal: evolution of antimicrobial resistance and T antigenic types during 2000-2002. Microb. Drug Resist. 11, 360–370. doi: 10.1089/mdr.2005.11.360
Poxton, I. R. (2014). Teichoic acids, lipoteichoic acids and other secondary cell wall and membrane polysaccharides of gram-positive bacteria. Mol. Med. Microbiol. Second Ed. 1– 3, 91–103. doi: 10.1016/B978-0-12-397169-2.00005-6
Proft, T., and Baker, E. N. (2009). Pili in Gram-negative and Gram-positive bacteria - structure, assembly and their role in disease. Cell. Mol. Life Sci. 66, 613–635. doi: 10.1007/s00018-008-8477-4
Rajagopal, L. (2009). Understanding the regulation of Group B Streptococcal virulence factors. Future Microbiol. 4, 201–221. doi: 10.2217/17460913.4.2.201
Rajam, G., Carlone, G. M., and Romero-Steiner, S. (2007). Functional antibodies to the O-acetylated pneumococcal serotype 15B capsular polysaccharide have low cross-reactivities with serotype 15C. Clin. Vaccine Immunol. 14, 1223–1227. doi: 10.1128/CVI.00184-07
Ranieri, M. R., Whitchurch, C. B., and Burrows, L. L. (2018). Mechanisms of biofilm stimulation by subinhibitory concentrations of antimicrobials. Curr. Opin. Microbiol. 45, 164–169. doi: 10.1016/j.mib.2018.07.006
Rato, M. G., Bexiga, R., Florindo, C., Cavaco, L. M., Vilela, C. L., and Santos-Sanches, I. (2013). Antimicrobial resistance and molecular epidemiology of streptococci from bovine mastitis. Vet. Microbiol. 161, 286–294. doi: 10.1016/j.vetmic.2012.07.043
Rato, M. G., Bexiga, R., Nunes, S. F., Vilela, C. L., and Santos-Sanches, I. (2010). Human group A streptococci virulence genes in bovine group C streptococci. Emerg. Infect. Dis. 16, 116–119. doi: 10.3201/eid1601.090632
Rato, M. G., Nerlich, A., Bergmann, R., Bexiga, R., Nunes, S. F., Vilela, C. L., et al. (2011). Virulence gene pool detected in bovine group C Streptococcus dysgalactiae subsp. dysgalactiae isolates by use of a group A S. pyogenes virulence microarray. J. Clin. Microbiol. 49, 2470–2479. doi: 10.1128/JCM.00008-11
Raynes, J. M., Young, P. G., Proft, T., Williamson, D. A., Baker, E. N., and Moreland, N. J. (2018). Protein adhesins as vaccine antigens for Group A Streptococcus. Pathog. Dis. 76:10.1093/femsd/fty016. doi: 10.1093/femspd/fty016
Reglinski, M., and Sriskandan, S. (2014). The contribution of group A streptococcal virulence determinants to the pathogenesis of sepsis. Virulence 5, 127–136. doi: 10.4161/viru.26400
Reinoso, J. (2017). Bovine mastitis caused by Streptococcus uberis: virulence factors and biofilm. Microb. Biochem. Technol. 9:5. doi: 10.4172/1948-5948.100037
Remmington, A., and Turner, C. E. (2018). The DNases of pathogenic Lancefield streptococci. Microbiology 164, 242–250. doi: 10.1099/mic.0.000612
Richards, V. P., Palmer, S. R., Pavinski Bitar, P. D., Qin, X., Weinstock, G. M., Highlander, S. K., et al. (2014). Phylogenomics and the dynamic genome evolution of the genus Streptococcus. Genome Biol. Evol. 6, 741–753. doi: 10.1093/gbe/evu048
Rinaudo, C. D., Rosini, R., Galeotti, C. L., Berti, F., Necchi, F., Reguzzi, V., et al. (2010). Specific involvement of pilus type 2a in biofilm formation in group B Streptococcus. PLoS One 5:e9216. doi: 10.1371/journal.pone.0009216
Roberts, A. L., Connolly, K. L., Doern, C. D., Holder, R. C., and Reid, S. D. (2010a). Loss of the group A Streptococcus regulator Srv decreases biofilm formation in vivo in an otitis media model of infection. Infect. Immun. 78, 4800–4808. doi: 10.1128/IAI.00255-10
Roberts, A. L., Holder, R. C., and Reid, S. D. (2010b). Allelic replacement of the streptococcal cysteine protease SpeB in a Δsrv mutant background restores biofilm formation. BMC Res. Notes 3:281. doi: 10.1186/1756-0500-3-281
Rohde, M., and Chhatwal, G. S. (2013). Adherence and invasion of streptococci to eukaryotic cells and their role in disease pathogenesis. Curr. Top. Microbiol. Immunol. 368, 83–110. doi: 10.1007/82_2012_281
Rohde, M., and Cleary, P. P. (2016). “Adhesion and invasion of Streptococcus pyogenes into host cells and clinical relevance of intracellular streptococci,” in Streptococcus pyogenes: Basic Biology to Clinical Manifestations, eds J. J. Ferreti, D. L. Stevens, and V. A. Fischetti (Oklahoma City, OK: University of Oklahoma Health Sciences Center).
Rohde, M., Graham, R. M., Branitzki-Heinemann, K., Borchers, P., Preuss, C., Schleicher, I., et al. (2011). Differences in the aromatic domain of homologous streptococcal fibronectin-binding proteins trigger different cell invasion mechanisms and survival rates. Cell. Microbiol. 13, 450–468. doi: 10.1111/j.1462-5822.2010.01547.x
Roma-Rodrigues, C., Alves-Barroco, C., Raposo, L. R., Costa, M. N., Fortunato, E., Baptista, P. V., et al. (2016). Infection of human keratinocytes by Streptococcus dysgalactiae subspecies dysgalactiae isolated from milk of the bovine udder. Microbes Infect. 18, 290–293. doi: 10.1016/j.micinf.2015.11.005
Rosenau, A., Martins, K., Amor, S., Gannier, F., Lanotte, P., van der Mee-Marquet, N., et al. (2007). Evaluation of the ability of Streptococcus agalactiae strains isolated from genital and neonatal specimens to bind to human fibrinogen and correlation with characteristics of the fbsA and fbsB genes. Infect. Immun. 75, 1310–1317. doi: 10.1128/IAI.00996-06
Rosini, R., and Margarit, I. (2015). Biofilm formation by Streptococcus agalactiae: influence of environmental conditions and implicated virulence factors. Front. Cell. Infect. Microbiol. 5:6. doi: 10.3389/fcimb.2015.00006
Rosini, R., Rinaudo, C. D., Soriani, M., Lauer, P., Mora, M., Maione, D., et al. (2006). Identification of novel genomic islands coding for antigenic pilus-like structures in Streptococcus agalactiae. Mol. Microbiol. 61, 126–141. doi: 10.1111/j.1365-2958.2006.05225.x
Ruppen, C., Hemphill, A., and Sendi, P. (2017). In vitro activity of gentamicin as an adjunct to penicillin against biofilm group B Streptococcus. J. Antimicrob. Chemother. 72, 444–447. doi: 10.1093/jac/dkw447
Sachi, S., Ferdous, J., Sikder, M. H., and Azizul Karim Hussani, S. M. (2019). Antibiotic residues in milk: past, present, and future. J. Adv. Vet. Anim. Res. 6, 315–332. doi: 10.5455/javar.2019.f350
Savage, V. J., Chopra, I., and O’Neill, A. J. (2013). Staphylococcus aureus biofilms promote horizontal transfer of antibiotic resistance. Antimicrob. Agents Chemother. 57, 1968–1970. doi: 10.1128/AAC.02008-12
Schumacher-Perdreau, F., Heilmann, C., Peters, G., Götz, F., and Pulverer, G. (1994). Comparative analysis of a biofilm-forming Staphylococcus epidermidis strain and its adhesion-positive, accumulation-negative mutant M7. FEMS Microbiol. Lett. 117, 71–78. doi: 10.1111/j.1574-6968.1994.tb06744.x
Seifert, K. N., McArthur, W. P., Bleiweis, A. S., and Brady, L. J. (2003). Characterization of group B streptococcal glyceraldehyde-3-phosphate dehydrogenase: surface localization, enzymatic activity, and protein-protein interactions. Can. J. Microbiol. 49, 350–356. doi: 10.1139/w03-042
Sharma, D., Misba, L., and Khan, A. U. (2019). Antibiotics versus biofilm: an emerging battleground in microbial communities. Antimicrob. Resist. Infect. Control 8:76. doi: 10.1186/s13756-019-0533-3
Sharma, K., and Pagedar Singh, A. (2018). Antibiofilm effect of DNase against single and mixed species biofilm. Foods 7:42. doi: 10.3390/foods7030042
Shelburne, S. A., Sahasrabhojane, P., Saldana, M., Yao, H., Su, X., Horstmann, N., et al. (2014). Streptococcus mitis strains causing severe clinical disease in cancer patients. Emerg. Infect. Dis. 20, 762–771. doi: 10.3201/eid2005.130953
Shemesh, M., Tam, A., and Steinberg, D. (2007). Expression of biofilm-associated genes of Streptococcus mutans in response to glucose and sucrose. J. Med. Microbiol. 56(Pt 11), 1528–1535. doi: 10.1099/jmm.0.47146-0
Shen, Y., Köller, T., Kreikemeyer, B., and Nelson, D. C. (2013). Rapid degradation of Streptococcus pyogenes biofilms by PlyC, a bacteriophage-encoded endolysin. J. Antimicrob. Chemother. 68, 1818–1824. doi: 10.1093/jac/dkt104
Shewmaker, P. L., Gertz, R. E. Jr., Kim, C. Y., de Fijter, S., DiOrio, M., Moore, M. R., et al. (2010). Streptococcus salivarius meningitis case strain traced to oral flora of anesthesiologist. J. Clin. Microbiol. 48, 2589–2591. doi: 10.1128/JCM.00426-10
Shiraishi, T., Yokota, S., Fukiya, S., and Yokota, A. (2016). Structural diversity and biological significance of lipoteichoic acid in Gram-positive bacteria: focusing on beneficial probiotic lactic acid bacteria. Biosci. Microb.Food Health 35, 147–161. doi: 10.12938/bmfh.2016-006
Siggins, M. K., Lynskey, N. N., Lamb, L. E., Johnson, L. A., Huse, K. K., Pearson, M., et al. (2020). Extracellular bacterial lymphatic metastasis drives Streptococcus pyogenes systemic infection. Nat. Commun. 11:4697. doi: 10.1038/s41467-020-18454-0
Singh, S., Singh, S. K., Chowdhury, I., and Singh, R. (2017). Understanding the mechanism of bacterial biofilms resistance to antimicrobial agents. Open Microbiol. J. 11, 53–62. doi: 10.2174/1874285801711010053
Šmitran, A., Vuković, D., Opavski, N., Gajić, I., Marinković, J., Božić, L., et al. (2018). Influence of subinhibitory antibiotic concentration on Streptococcus pyogenes adherence and biofilm production. Acta Microbiol. Immunol. Hung. 65, 229–240. doi: 10.1556/030.65.2018.026
Šmitran, A., Gajić, I., Božić, L., and Ranin, L. (2016). “Adherence and Biofilm Production of Streptococcus pyogenes,” in Microbial Biofilms - Importance and Applications, Dharumadurai Dhanasekaran and Nooruddin Thajuddin, eds D. Dhanasekaran and N. Thajuddin (London: IntechOpen), doi: 10.5772/63490
Stewart, P. S., and Bjarnsholt, T. (2020). Risk factors for chronic biofilm-related infection associated with implanted medical devices. Clin. Microbiol. Infect. 26, 1034–1038. doi: 10.1016/j.cmi.2020.02.027
Stewart, P. S., and Costerton, J. W. (2001). Antibiotic resistance of bacteria in biofilms. Lancet 358, 135–138. doi: 10.1016/s0140-6736(01)05321-1
Sumby, P., Whitney, A. R., Graviss, E. A., DeLeo, F. R., and Musser, J. M. (2006). Genome-wide analysis of group a streptococci reveals a mutation that modulates global phenotype and disease specificity. PLoS Pathog. 2:e5. doi: 10.1371/journal.ppat.0020005
Suntharalingam, P., and Cvitkovitch, D. G. (2005). Quorum sensing in streptococcal biofilm formation. Trends Microbiol. 13, 3–6. doi: 10.1016/j.tim.2004.11.009
Sutherland, I. W. (2001). The biofilm matrix–an immobilized but dynamic microbial environment. Trends Microbiol. 9, 222–227. doi: 10.1016/s0966-842x(01)02012-1
Swidsinski, A., Göktas, O., Bessler, C., Loening-Baucke, V., Hale, L. P., Andree, H., et al. (2007). Spatial organisation of microbiota in quiescent adenoiditis and tonsillitis. J. Clin. Pathol. 60, 253–260. doi: 10.1136/jcp.2006.037309
Thenmozhi, R., Balaji, K., Kumar, R., Rao, T. S., and Pandian, S. K. (2011). Characterization of biofilms in different clinical M serotypes of Streptococcus pyogenes. J. Basic Microbiol. 51, 196–204. doi: 10.1002/jobm.201000006
Tomazi, T., de Souza Filho, A. F., Heinemann, M. B., and Dos Santos, M. V. (2018). Molecular characterization and antimicrobial susceptibility pattern of Streptococcus agalactiae isolated from clinical mastitis in dairy cattle. PLoS One 13:e0199561. doi: 10.1371/journal.pone.0199561
Torretta, S., Marchisio, P., Drago, L., Baggi, E., De, V. E., and Garavello, W. (2012). Nasopharyngeal biofilm-producing otopathogens in children with nonsevere recurrent acute otitis media. Otolaryngol. Head Neck Surg. 146, 991–996. doi: 10.1177/0194599812438169
Tran, M. P., Caldwell-McMillan, M., Khalife, W., and Young, V. B. (2008). Streptococcus intermedius causing infective endocarditis and abscesses: a report of three cases and review of the literature. BMC Infect. Dis. 8:154. doi: 10.1186/1471-2334-8-154
Trappetti, C., Ogunniyi, A. D., Oggioni, M. R., and Paton, J. C. (2011). Extracellular matrix formation enhances the ability of Streptococcus pneumoniae to cause invasive disease. PLoS One 6:e19844. doi: 10.1371/journal.pone.0019844
Tylewska, S. K., Fischetti, V. A., and Gibbons, R. J. (1988). Binding selectivity of Streptococcus pyogenes and M-protein to epithelial cells differs from that of lipoteichoic acid. Curr. Microbiol. 16, 209–216. doi: 10.1007/BF01568531
Vajjala, A., Biswas, D., Tay, W. H., Hanski, E., and Kline, K. A. (2019). Streptolysin-induced endoplasmic reticulum stress promotes group A Streptococcal host-associated biofilm formation and necrotising fasciitis. Cell Microbiol. 21:e12956. doi: 10.1111/cmi.12956
Van Schaik, E. J., Giltner, C. L., Audette, G. F., Keizer, D. W., Bautista, D. L., Slupsky, C. M., et al. (2005). DNA binding: a novel function of Pseudomonas aeruginosa type IV pili. J. Bacteriol. 187, 1455–1464. doi: 10.1128/JB.187.4.1455-1464.2005
Vasudevan, R. (2014). Biofilms: microbial cities of scientific significance. J. Microbiol. Exp. 1, 84–98. doi: 10.15406/jmen.2014.01.00014
Vidal, J. E., Ludewick, H. P., Kunkel, R. M., Zähner, D., and Klugman, K. P. (2011). The LuxS-dependent quorum-sensing system regulates early biofilm formation by Streptococcus pneumoniae strain D39. Infect. Immun. 79, 4050–4060. doi: 10.1128/IAI.05186-11
Von Wintersdorff, C. J., Penders, J., van Niekerk, J. M., Mills, N. D., Majumder, S., van Alphen, L. B., et al. (2016). Dissemination of antimicrobial resistance in microbial ecosystems through horizontal gene transfer. Front. Microbiol. 7:173. doi: 10.3389/fmicb.2016.00173
Vuong, C., Kocianova, S., Voyich, J. M., Yao, Y., Fischer, E. R., DeLeo, F. R., et al. (2004). A crucial role for exopolysaccharide modification in bacterial biofilm formation, immune evasion, and virulence. J. Biol. Chem. 279, 54881–54886. doi: 10.1074/jbc.M411374200
Vyas, H., Proctor, E. J., McArthur, J., Gorman, J., and Sanderson-Smith, M. (2019). Current understanding of Group A streptococcal biofilms. Curr. Drug Targets 20, 982–993. doi: 10.2174/1389450120666190405095712
Wallis, J. K., Krömker, V., and Paduch, J. H. (2018). Biofilm formation and adhesion to bovine udder epithelium of potentially probiotic lactic acid bacteria. AIMS Microbiol. 4, 209–224. doi: 10.3934/microbiol.2018.2.209
Wang, C. H., Chiang-Ni, C., Kuo, H. T., Zheng, P. X., Tsou, C. C., Wang, S., et al. (2013). Peroxide responsive regulator PerR of group A Streptococcus is required for the expression of phage-associated DNase Sda1 under oxidative stress. PLoS One 8:e81882. doi: 10.1371/journal.pone.0081882
Ward, J. B. (1981). Teichoic and teichuronic acids: biosynthesis, assembly, and location. Microbiol Rev. 45, 211–243. doi: 10.1128/mmbr.45.2.211-243.1981
Weckel, A., Ahamada, D., Bellais, S., Méhats, C., Plainvert, C., Longo, M., et al. (2018). The N-terminal domain of the R28 protein promotes emm28 group A Streptococcus adhesion to host cells via direct binding to three integrins. J. Biol. Chem. 293, 16006–16018. doi: 10.1074/jbc.RA118.004134
Weidenmaier, C., and Peschel, A. (2008). Teichoic acids and related cell-wall glycopolymers in Gram-positive physiology and host interactions. Nat. Rev.Microbiol. 6, 276–287. doi: 10.1038/nrmicro1861
Wessels, M. R. (2016). “Cell wall and surface molecules: capsule,” in Streptococcus Pyogenes: Basic Biology to Clinical Manifestations, eds J. J. Ferreti, D. L. Stevens, and V. A. Fischetti (Oklahoma City, OK: University of Oklahoma Health Sciences Center), 53–63.
Whitfield, G. B., Marmont, L. S., and Howell, P. L. (2015). Enzymatic modifications of exopolysaccharides enhance bacterial persistence. Front. Microbiol. 6:471. doi: 10.3389/fmicb.2015.00471
Wilcox, C. R., Stuart, B., Leaver, H., Lown, M., Willcox, M., Moore, M., et al. (2019). Effectiveness of the probiotic Streptococcus salivarius K12 for the treatment and/or prevention of sore throat: a systematic review. Clin. Microbiol. Infect. 25, 673–680. doi: 10.1016/j.cmi.2018.12.031
Wilking, J. N., Angelini, T. E., Seminara, A., Brenner, M. P., and Weitz, D. A. (2011). Biofilms as complex fluids. MRS Bull. 36, 385–391. doi: 10.1557/mrs.2011.71
Wingender, J., Jaeger, K. E., and Flemming, H. C. (1999). “Interaction between extracellular polysaccharides and enzymes,” in Microbial Extracellular Polymeric Substances, eds J. Wingender, T. R. Neu, and H.-C. Flemming (Berlin: Springer Berlin Heidelberg), 231–251. doi: 10.1007/978-3-642-60147-7_13
Wong, S. S., and Yuen, K. Y. (2012). Streptococcus pyogenes and re-emergence of scarlet fever as a public health problem. Emerg. Microb. Infect. 1:e2. doi: 10.1038/emi.2012.9
Wu, H., Moser, C., Wang, H. Z., Høiby, N., and Song, Z. J. (2015). Strategies for combating bacterial biofilm infections. Int J. Oral Sci. 7, 1–7. doi: 10.1038/ijos.2014.65
Yang, Q., Porter, A. J., Zhang, M., Harrington, D. J., Black, G. W., and Sutcliffe, I. C. (2012). The impact of pH and nutrient stress on the growth and survival of Streptococcus agalactiae. Antonie Van Leeuwenhoek 102, 277–287. doi: 10.1007/s10482-012-9736-9
Young, C., Holder, R. C., and Reid, S. D. (2016). “Streptococcus pyogenes Biofilm,” in Streptococcus pyogenes: Basic Biology to Clinical Manifestations, eds J. J. Ferretti, D. L. Stevens, and V. A. Fischetti (Oklahoma City, OK: University of Oklahoma Health Sciences Center).
Zadoks, R., and Fitzpatrick, J. (2009). Changing trends in mastitis. Irish Vet. J. 62(Suppl. 4), S59–S70. doi: 10.1186/2046-0481-62-S4-S59
Keywords: biofilm, antibiotic resistance, virulence factors, quorum-sensing, streptococcal
Citation: Alves-Barroco C, Paquete-Ferreira J, Santos-Silva T and Fernandes AR (2020) Singularities of Pyogenic Streptococcal Biofilms – From Formation to Health Implication. Front. Microbiol. 11:584947. doi: 10.3389/fmicb.2020.584947
Received: 18 July 2020; Accepted: 20 November 2020;
Published: 23 December 2020.
Edited by:
Santi M. Mandal, Indian Institute of Technology Kharagpur, IndiaReviewed by:
Yang Wu, Shanghai Medical College, Fudan University, ChinaYuqing Li, West China Hospital of Stomatology, Sichuan University, China
Prosun Tribedi, The Neotia University, India
Ashwinkumar Subramenium Ganapathy, College of Medicine, Pennsylvania State University, United States
Copyright © 2020 Alves-Barroco, Paquete-Ferreira, Santos-Silva and Fernandes. This is an open-access article distributed under the terms of the Creative Commons Attribution License (CC BY). The use, distribution or reproduction in other forums is permitted, provided the original author(s) and the copyright owner(s) are credited and that the original publication in this journal is cited, in accordance with accepted academic practice. No use, distribution or reproduction is permitted which does not comply with these terms.
*Correspondence: Alexandra R. Fernandes, ma.fernandes@fct.unl.pt; Teresa Santos-Silva, tsss@fct.unl.pt