- 1Department of Genetics and Genome Biology, University of Leicester, Leicester, United Kingdom
- 2School of Microbiology and APC Microbiome Ireland, University College Cork, Cork, Ireland
- 3King Faisal Specialist Hospital and Research Centre, Riyadh, Saudi Arabia
- 4International Centre for Diarrheal Diseases Research, Dhaka, Bangladesh
- 5Department of Chemical and Materials Engineering, University of Alberta, Edmonton, AB, Canada
- 6Section for Evolutionary Genomics, The GLOBE Institute, Faculty of Health and Medical Sciences, University of Copenhagen, Copenhagen, Denmark
- 7Centre of Excellence for Omics-Driven Computational Biodiscovery (COMBio), Faculty of Applied Sciences, AIMST University, Kedah, Malaysia
Antimicrobial resistance (AMR) is a major problem globally. The main bacterial organisms associated with urinary tract infection (UTI) associated sepsis are E. coli and Klebsiella along with Enterobacter species. These all have AMR strains known as ESBL (Extended Spectrum Beta-Lactamase), which are featured on the WHO priority pathogens list as “critical” for research. Bacteriophages (phages), as viruses that can infect and kill bacteria, could provide an effective tool to tackle these AMR strains. There is currently no “gold standard” for developing a phage cocktail. Here we describe a novel approach to develop an effective phage cocktail against a set of ESBL-producing E. coli and Klebsiella largely isolated from patients in United Kingdom hospitals. By comparing different measures of phage efficacy, we show which are the most robust, and suggest an efficient screening cascade that could be used to develop phage cocktails to target other AMR bacterial species. A target panel of 38 ESBL-producing clinical strains isolated from urine samples was collated and used to test phage efficacy. After an initial screening of 68 phages, six were identified and tested against these 38 strains to determine their clinical coverage and killing efficiency. To achieve this, we assessed four different methods to assess phage virulence across these bacterial isolates. These were the Direct Spot Test (DST), the Efficiency of Plating (EOP) assay, the planktonic killing assay (PKA) and the biofilm assay. The final ESBL cocktail of six phages could effectively kill 23/38 strains (61%), for Klebsiella 13/19 (68%) and for E. coli 10/19 (53%) based on the PKA data. The ESBL E. coli collection had six isolates from the prevalent UTI-associated ST131 sequence type, five of which were targeted effectively by the final cocktail. Of the four methods used to assess phage virulence, the data suggests that PKAs are as effective as the much more time-consuming EOPs and data for the two assays correlates well. This suggests that planktonic killing is a good proxy to determine which phages should be used in a cocktail. This assay when combined with the virulence index also allows “phage synergy” to inform cocktail design.
Introduction
Antimicrobial resistance (AMR) is a major global challenge. It is part of the key target priorities for several prominent organisations including the World Health Organisation (WHO), European Centre for Disease Prevention and Control (ECDC) and National Institute of Health Research (NIHR) (Tacconelli et al., 2018). It has been predicted that more people will die of AMR infections than cancer by 2050 and AMR associated deaths are estimated to be approximately 10 million people per year (O’Neill, 2014). AMR has been compounded by a reduction in novel antibiotic discovery, the persistent use of antibiotics and thus, the rapid emergence of bacterial strains that are resistant to both existing and new antibiotics (Tacconelli et al., 2018). The most clinically relevant group of multi-drug resistant (MDR) pathogens are referred to collectively as the ESPAKEE organisms (Gram-positive Enterococcus faecium and Staphylococcus aureus, as well as Gram-negative Pseudomonas aeruginosa, Acinetobacter baumannii, Klebsiella pneumoniae, Enterobacter species, and Escherichia coli), and are together responsible for the majority of hospital-acquired infections (Pendleton et al., 2013). Urinary tract infections (UTIs) are prevalent and can cause serious infections per se but can also act as infection sources for sepsis (urosepsis) and septicaemia. The majority of organisms associated with urosepsis are E. coli, which are responsible for 50% of cases, and Klebsiella along with other Enterobacter species, which total 15% of cases (Kalra and Raizada, 2009). Furthermore biofilm formation has been shown to be crucial in infections such as catheter-associated UTIs with both E. coli and Klebsiella (Hancock et al., 2010).
Extended Spectrum Beta Lactamases (ESBL) are plasmid-mediated enzymes that, if expressed by a bacterial strain, confer resistance to antibiotics containing a beta-lactam ring in their molecular structure such as penicillins, cephalosporins, and carbapenems (Sykes and Matthew, 1976; Livermore, 1987; van Duin and Doi, 2017). ESBL-producing strains of both E. coli and Klebsiella have been detected from a variety of sources worldwide (Bush, 2018). They pose a serious global public health threat due to the difficulties associated with treatment of infections with ESBL-producing bacteria. Although, Sakellariou et al. (2016) reported no difference in mortality rates of infections caused by either ESBL-producing E. coli (23.8%) or ESBL-producing Klebsiella (27.1%), they did report that septicaemia associated with ESBL-producing Klebsiella has a higher morbidity (sepsis with organ failure).
The decline in antibiotic discovery and emergence of resistance to last line antibiotics (Pendleton et al., 2013), motivates the need for alternative antimicrobials. A promising solution is the therapeutic application of lytic bacteriophages (phages), which are viruses that kill bacteria. Phage therapy has a long history of use in countries such as Georgia, Poland, and France (Kutateladze and Adamia, 2008; Ansaldi et al., 2018; Gorski et al., 2018) where it has been used alongside or instead of antibiotics to treat bacterial infections for more than 100 years. There is a critical need to widen access to this therapy, either as an alternative or supplement to antibiotic treatment. If phage therapy is to be developed in the Western world, it is advisable to focus on bacterial diseases for which no other treatments exist and those which have high levels of AMR (Tomas et al., 2018).
A phage cocktail is a mixture of several phages and has two potential clinical advantages (Chan and Abedon, 2012). One is to combine the individual phages to broaden the number of strains the phages are able to infect. The second is to combat phage resistance, which can occur with the use of single phages. By using a cocktail of phages, strains that become resistant to one phage can potentially be targeted by other phages within the cocktail. In the context of the current study, the primary goal for the phage cocktail was to provide a broader host range than any of the individual phages alone. Host range coverage was prioritised over efficiency of killing with regards to the phage cocktail selection. This is because in a clinical context, it would be beneficial to provide partial treatment to a wider number of patients, allowing synergy with the immune system and antibiotics, rather than treating only a select few patients (Chan et al., 2013; Mattila et al., 2015). The overall aim was to identify phages that individually have broad host ranges and collectively when combined would cover ∼90% of the either the ESBL-producing E. coli or Klebsiella collection.
Although phage cocktails have been designed and their efficacy reported in the literature previously, there are no current guidelines to standardise the development of an optimised cocktail for antibiotic resistant bacteria or indeed to predict the efficacy of phages at least under in vitro conditions. Through the development of the phage cocktail in the current study, we have generated a data set that allows comparison of four different methods of assessing phage virulence (the ability of the phage to kill bacteria) across a panel of 38 bacterial isolates. These tests are: Direct Spot Test (DST), Efficiency of Plating (EOP) assay, a planktonic killing assay (PKA) and a biofilm assay. Both the DST and EOP assay are frequently utilised in the determination of phage virulence (Mirzaei and Nilsson, 2015) and both tests use the double agar plate method. The DST is a reasonably good method for initial host range screening, but it does not provide a reliable indication that the phage can replicate on the host strain. The EOP assay indicates productive infection of the host strain by the phage from which the efficiency of infection of the host can be determined. The PKA was assessed as an alternative to the labour-intensive DST and EOP approaches. This method monitors the optical density of a liquid culture of bacteria to which a phage combination was added using a plate reader over 24 h. The previous three methods examine the virulence of phages based on killing bacteria under “normal” growth conditions in vitro and so the final method chosen was a biofilm assay. This assay provides an insight into phage virulence in an in vitro model of infection and biofilm formation. Genomic analysis was performed on all 38 ESBL-producing clinical isolates to determine the relationships between susceptibility to phage infection and genomic content. The genetic relationship between the most sensitive and most resistant clinical isolates was determined. The final six phages selected for the ESBL phage cocktail were also sequenced to confirm suitability for phage therapy by ensuring they did not encode for any known undesirable traits (toxins/lysogeny).
This article focuses on the development of a phage cocktail that is effective against ESBL-producing E. coli and Klebsiella that were largely isolated from patients in United Kingdom hospitals. In producing this data, we describe an efficient screening cascade to develop cocktails, which will be relevant for other target AMR bacterial species. This data shows a novel, direct comparison of results across the four phage virulence tests for individual clinical isolates and indicates that the PKAs are a reliable and time efficient way to assess phage efficacy.
Materials and Methods
Bacterial Strains
Thirty eighty strains of ESBL-producing bacteria were examined during this study; 19 E. coli and 19 Klebsiella. All strains were clinical isolates from UTIs; 14 of the E. coli isolates were from Leicester Royal Infirmary, United Kingdom; 5 from Huashan Hospital, Shanghai and 19 Klebsiella isolates from Leicester Royal Infirmary, United Kingdom (Supplementary Table S1). All bacteria were grown at 37°C in either Luria-Bertani Broth (LB—Thermo Fisher Scientific, United Kingdom) at 100 rpm or on LB 1% (w/v) agar plates. All strains were stored in 50% glycerol stocks at −80°C until required. The bacterial strains were sequenced by MicrobesNG with the Standard Whole Genome Service, Illumina Sequencing by sending the bacterial strains as samples.
Phage Collection, Isolation, Amplification, and Visualisation
Phages were collated from several sources with the majority coming from collaborations with other research projects (Supplementary Table S2). Phages were isolated using the method previously described by Kropinski et al. (2009). To identify phages, 100 μl enrichment, 100 μl culture and 3 ml LB 0.5% (w/v) agar were poured onto a LB 1% (w/v) agar plate and incubated overnight at 37°C. Single plaques were picked and transferred to 500 μl SM Buffer [100 mM NaCl (Sigma-Aldrich, United Kingdom), 8 mm MgSO4 7 H2O (Sigma-Aldrich, United Kingdom), 0.1% (w/v) gelatin (Sigma-Aldrich, United Kingdom), 50 mM Tris-HCl pH 7.5 (Sigma-Aldrich, United Kingdom)]. This process was repeated to give five rounds of single plaque purification and stored in SM buffer.
Phage stocks were made using the double layer agar method. Briefly, an overnight culture of the host strain was diluted 1:100 in LB and grown for 2 h to an ∼OD550 of 0.2 at 37°C, 100 rpm. 500 μl of the bacterial culture and 200 μl of phage stock were added to 8 ml of 0.5% (w/v) LB agar and poured onto 120 × 120 mm square LB 1% (w/v) agar plates. The plates were incubated overnight at 37°C. The plates were agitated for 2 h in 10 ml SM buffer. The top layer was removed and centrifuged at 4,000 × g for 15 min. The supernatant was filter-sterilised through 0.2 μm pore size filters and the resultant phage stock titre was determined using double agar overlay plaque assays (Kropinski et al., 2009). Stock was stored at 4°C. Phage UP17 (vB_EcoM_UP17) was propagated using E. coli EA2; phage JK08 (vB_SsoM_JK08)—E. coli MH10; phage 113 (vB_SsoM_113)—Shigella sonnei B31; phage 2811 (vB_KpnS_2811)—Klebsiella pneumoniae KR2811; phage 311F (vB_KpnM_311F)—K. pneumoniae KR311; phage 05F (vB_KpnM_05F)—K. pneumoniae MH05.
Transmission Electron Microscopy imaging for the phages UP17, 113, 2811, 311F and 05F was performed at University of Leicester, United Kingdom. The phages were negatively stained with 1% (w/v) uranyl acetate on 3 mm carbon-coated copper grids and visualised with a JEM-1400 transmission electron microscope (JEOL UK Ltd., United Kingdom) with an accelerating voltage of 120 kV. Digital images were collected with an Xarosa digital camera (EMSIS, Germany) with Radius software for phage 113; all other phages were imaged using a Megaview III digital camera (EMSIS, Germany) instead. Imaging for phage JK08 was performed at the Max Rubner-Institut, Germany with a Tecnai 10 transmission electron microscope (FEI, Eindhoven, The Netherlands) operated at an acceleration voltage of 80 kV.
Direct Spot Testing (DST)
Bacterial cultures were grown overnight, then diluted 1/100 in LB and grown for 2 h to ∼OD550 of 0.2. 500 μL of the culture was added to 8 ml 0.5% (w/v) LB agar kept molten at 55°C and poured onto LB 1% (w/v) agar square 120 × 120 mm plates. 20 μl of phage stock (109/1010 pfu/ml) was spotted onto the plate, left to dry and then incubated overnight at 37°C. The appearance of the spot was graded: ++++ complete lysis; +++ lysis with resistant colonies; ++ hazy lysis; + visible plaques; 0 no visible plaques (Supplementary Tables S3, S4).
Efficiency of Plating (EOP)
This method has been previously described by Kutter (2009); 5 mM calcium chloride was supplemented to the 0.5% (w/v) LB agar for the E. coli and Shigella phages. Plaques on each plate were counted and the relative EOP was given as the ratio between the phage titre in pfu/ml (plaque forming units/ml) for the test host strain and the titre of the propagating host strain. Propagating host for phages UP17, JK08 and 113 was E. coli MH10. The propagating host for 2811—K. pneumoniae KR2811; for 311F—K. pneumoniae KR311; and for 05F—K. pneumoniae MH05.
Planktonic Killing Assay (PKA)
Experiments were carried out using the BMG Labtech SPECTROstar Omega, using a flat bottom 96 well plate (Sarstedt, Germany). 100 μl of a 1:100 dilution of overnight cultures was added to the 96 well plate, grown to A600 OD 0.15 (1 × 108 CFU/ml), then 100 μl of phage cocktail (containing 1 × 108 PFU/ml of each individual phage) was added. Working with a MOI of 1:1; throughout all the experiments. Final concentrations were achieved using LB as a diluent. The microtiter plates were securely sealed using gas-permeable parafilm M (Amcor, United States). OD readings (A600) were taken every 5 min for a total of 24 h with shaking 10 s prior to each reading. The microtiter plate had a positive control for every individual clinical strain for comparison (bacteria only), as well as a negative control (LB only) and 3 blanks (LB and gentamicin 10 μg/ml). Each cocktail was repeated in triplicate for each ESBL-producing clinical isolate and the data was merged to give a single killing assay curve.
The killing assay curves were analysed by a modified objective method (Storms et al., 2019) which was devised using the generated curve to give a “virulence index.” The virulence index score was calculated comparing the area under the curve of the individual phage or cocktail against the positive control whilst in log phase. This virulence index was normalised to a figure between 0 and 1, 0 = not effective and 1 = highly effective.
Biofilm Assay
Bacterial cultures were grown overnight at 37°C, 100 rpm. 100 μl of 1:100 dilution in LB of each bacterial strain was added to 96 well flat bottom microtiter plate in triplicate for both controls and phage cocktail treated. The whole experiment was also repeated in triplicate for all bacterial strains. After 24 h at 37°C, the LB was removed, and each well was washed with PBS. For the controls, 100 μl of fresh LB instead of 100 μl of the final ESBL cocktail was added (108 PFU/ml of each individual phage). After an additional 24 h of incubation, 20μl of resazurin (0.15 mg/ml—Sigma-Aldrich) was added and incubated at 37°C. OD readings were taken at A595 with Labtech.com LT-4500 at 4 and 24 h post incubation.
Phage DNA Extraction
Phage lysate at titres of 1011 PFU/ml was used to extract DNA using a modified phenol-chloroform-isoamyl method as previously described (Nale et al., 2015). The final DNA pellet was dissolved in 5 mM Tris HCl. This method only applies to phages UP17, 113, 2811, 311F, and 05F. For phage JK08, DNA isolation was performed using the Norgen Phage DNA isolation Kit (Norgen Biotek, ON, Canada) according to the manufacturer’s instructions.
Sequencing and Bioinformatic Analysis
Genome sequencing was conducted by MicrobesNG1, which was supported by the BBSRC (grant no. BB/L024209/1) for phages UP17, 311F and 05F as well as all the bacterial genomes. De novo assembly of the trimmed reads using Trimmomatic 0.30 (Bolger et al., 2014) from MicrobesNG was carried out using SPAdes genome assembler 3.12.0 (Bankevich et al., 2012) with default settings.
For the bacterial genomes, contigs were annotated using Prokka v1.12 (Seemann, 2014) and the assembly metrics were calculated using QUAST 5.0.2 (Gurevich et al., 2013). MLST 2.16.2 was used for characterisation of the bacterial strains (Seemann, 20202). ABRicate with Resfinder database was used with default settings to screen the genome of each strain for the presence of antimicrobial resistance and virulence genes (Zankari et al., 2012; Feldgarden et al., 2019).
Sequence data for the bacterial genomes was also used to create phylogenetic trees (Figure 4) using MEGA7 v7180411 (Kumar et al., 2018) and visualised using iTOL v5.5 (Letunic and Bork, 2007) based on the core genome SNPs. For phages 113 and 2811, the genomes were sequenced using an Illumina MiSeq, with a v3 kit (600 cycles). Genomic libraries were prepared using the Illumina Truseq Nano DNA library Preparation Kit as per the manufacturer’s instructions. The genomes were assembled using MEGAHIT (Li et al., 2015); phage 2811 (version 1.2.1) and phage 113 (version 1.1.4). Phage termini were identified using PhageTerm v1.0.11 (Garneau et al., 2017). Phage JK08 was sequenced using an Illumina MiSeq using a v2 kit (2 × 250). Illumina Truseq PCR-free library preparation kit was used as per manufacturer’s instructions for genomic library preparation. Genome assembly was performed with MIRA v4.0.2 (Chevreux et al., 1999).
The genomes of phage UP17, 311F, and 05F were assembled by subsampling reads to an approximate coverage of 100× with seqtk3 and assembled with SPAdes v3.12.0 with only assembler option (Bankevich et al., 2012). Phage genomes were annotated as previously described (Michniewski et al., 2019). To check for antibiotic resistance and virulence genes within the phage genomes, ABRicate was used with the card and vfdb databases, respectively.
Accession Numbers
All bacterial and phage genomes were submitted to the European Nucleotide Archive (ENA) under project accession number PRJEB34549. Individual accession numbers are provided in Supplementary Tables S1, S2.
Statistical Analysis
GraphPad Prism 7.04 (La Jolla, CA, United States) was used for statistical analysis for the biofilm assays. The results were expressed as mean ± SEM after analysis with 2-way ANOVA. A p < 0.05 was considered significant.
Results
Comparison of Phage Virulence Methods—DST, EOP, and PKA
The three methods used to assess phage virulence: DST, EOP, and PKA were compared. These three tests form the basis for the initial screening of a phage library to identify phages with the broadest host range. The data generated also allowed direct comparison of DST versus EOP, as these two assays are commonly used to characterise phages (Sybesma et al., 2016; Montso et al., 2019; Rivera et al., 2019; Supplementary Tables S5, S6).
The final three phages selected for the final ESBL cocktail based on their effectiveness against the ESBL-producing E. coli strains were UP17, JK08, and 113. With phages 2811, 311F, and 05F selected to target ESBL-producing Klebsiella. Phages were selected based on the results of the DST, EOP and PKA data (Figure 1). The selection of the final three phages was based on combining the minimum number of phages to have the maximal effect. For example, with the E. coli phages using four phages resulted in the same percentage coverage of using only three (Supplementary Table S5).
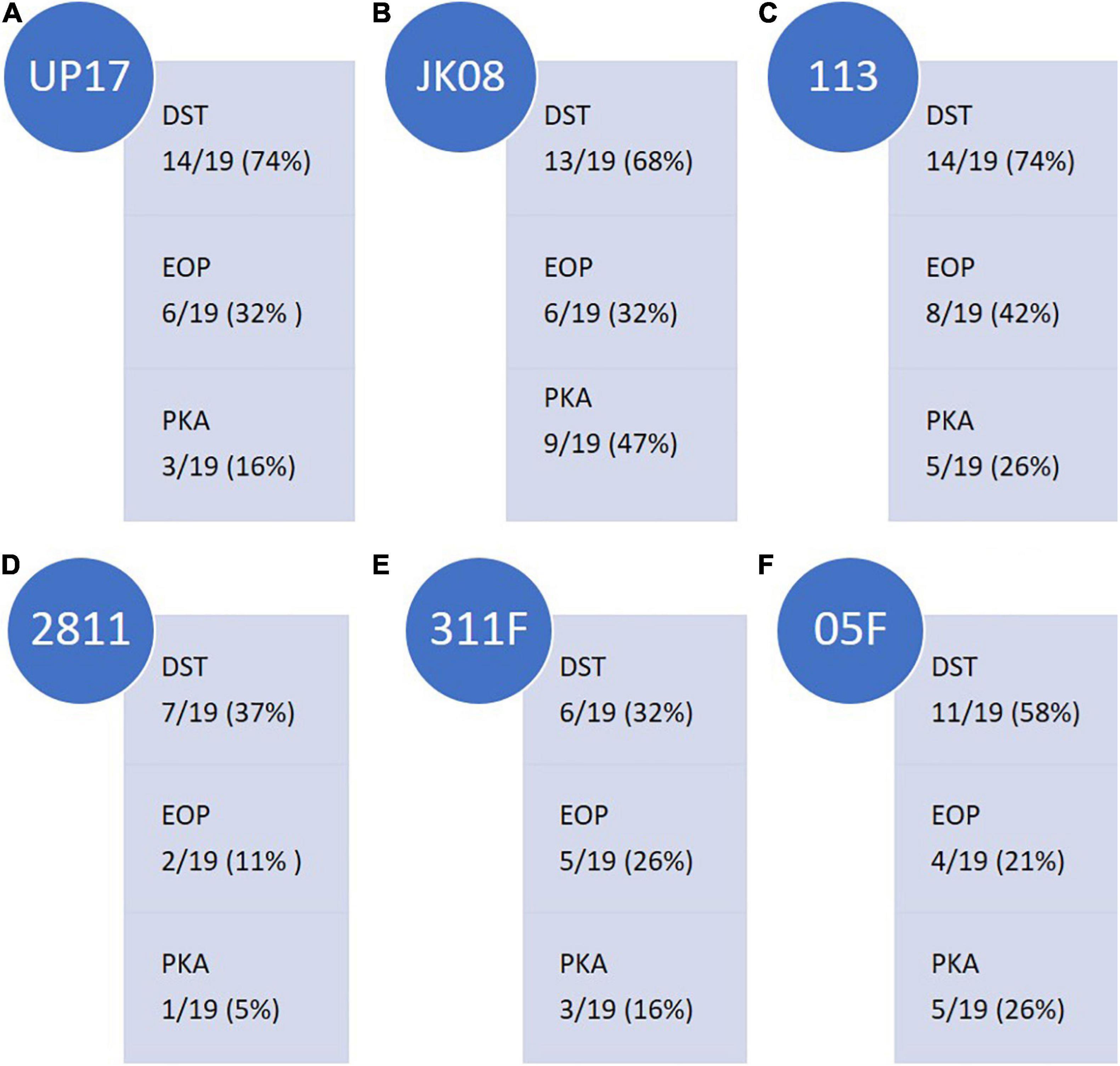
Figure 1. Summary of the ESBL-producing E.coli clinical isolate (n = 19) coverage of final E.coli phages (A) UP17, (B) JK08, and (C) 113 and ESBL-producing Klebsiella clinical isolate (n = 19) coverage of final Klebsiella phages (D) 2811, (E) 311F, and (F) 05F across the three selection tests [Direct Spot Test (DST), Efficiency of Plating (EOP) and Killing (Planktonic Killing Assay)]. Isolate coverage was determined by the following parameters: DST ≥ + appearance score; EOP > 0.01; Killing ≥0.2 virulence index score.
The final three E. coli phages were selected on the basis of having the broadest clinical isolate coverage. The following coverage was observed: phages UP17, JK08, and 113 could lyse 14/19 (74%), 13/19 (68%), and 14/19 (74%) of E. coli clinical isolates, respectively (Figure 1). When the phages were combined based on DST data, they provided coverage of 18/19 clinical isolates (95%) (Supplementary Table S5). The final three phages selected to be effective against the ESBL-producing Klebsiella clinical isolates gave overall coverage of 17/19 (89%) based on DST data (Supplementary Table S6). In comparison, the individual phages gave the following coverage: phage 2811 lysed 7/19 (37%), phage 311F lysed 6/19 (32%), and phage 05F lysed 11/19 (58%) (Figure 1).
The DST data highlighted which clinical isolates were lysed by the phages. To determine if the phages could efficiently replicate on the clinical isolates they infected, EOP studies were conducted. A detectable EOP was defined as the ratio compared to the control stain was > 0.01. Across all the phages, the number of isolates on which they could replicate within (EOP) were lower than those lysed (DST) as would be expected (Figure 1). Collectively for the three E. coli infecting phages, EOP data showed 13/19 strains (68%) compared to 18/19 (95%) predicted by the DST. DST overestimates the efficiency of killing compared to EOP and PKA. For example, UP17 only effectively replicates (EOP score of ≥ +) in 6/14 of the clinical isolates identified by DST.
There is a closer relationship between the PKA and EOP data; but the trend appears to be that PKA is slightly lower than EOP isolate coverage. For example, the PKA showed that phage 05F was effective (virulence index ≥ 0.2) for 5/19 (26%) clinical isolates compared with EOP 4/19 (21%) (Figure 1). Based on EOP data for phage 2811, it suggests that the phage could only replicate on 2/19 (11%) clinical isolates compared with 1/19 (5%) on the PKA (Figure 1).
Characterisation of the Final Six Phages Selected for the ESBL Phage Cocktail
The final phages selected to target ESBL-producing E. coli were UP17, JK08, 113 and for Klebsiella the final phages were 2811, 311F, and 05F, totalling 6 phages in the final cocktail. There was no lytic activity of the Klebsiella phages against the E. coli clinical isolates or vice-versa based on DST (Supplementary Tables S3, S4). The phage genomes were analysed to ensure that they did not carry genes known to allow a lysogenic lifestyle and did not contain any genes encoding for known toxins. A summary of the characteristics of the final six phages are shown in Figure 2.
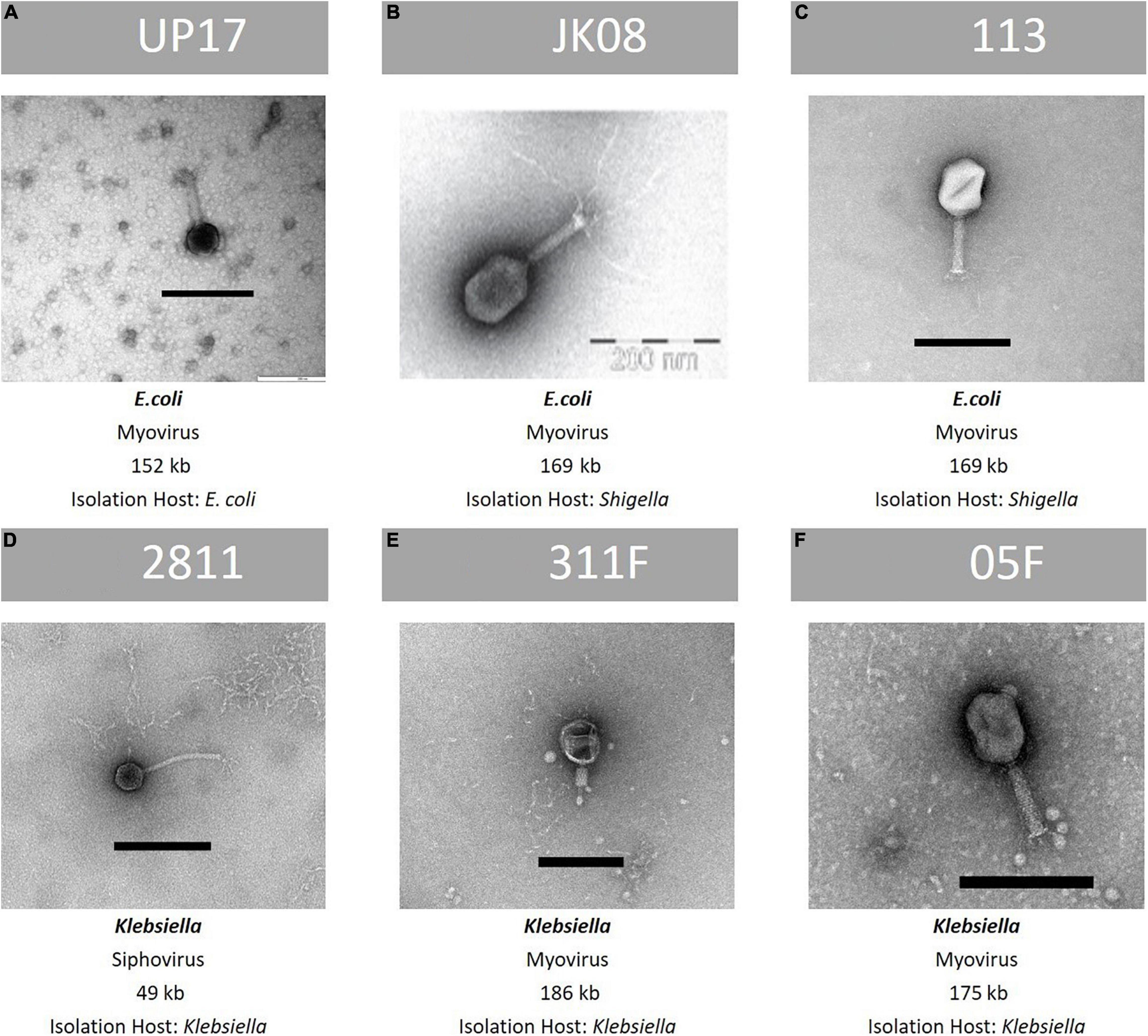
Figure 2. Summary of key features of the final six phages within the ESBL cocktail—TEM image, family classification, genome size and species of propagation host. From top row left to right, (A): phage UP17, (B): phage JK08, (C): phage 113. From bottom left to right: (D): phage 2811, (E): 311F, and (F): phage 05F. Black bar represents 200 nm.
Use of Virulence Index Score Demonstrates Synergy Within Phage Combinations
Analysis of the Combinations of ESBL E. coli Phages Using Virulence Index Scores
Phages UP17, JK08, and 113 used in various combinations of doublets, triplets and also in the final ESBL six-phage cocktail were tested using the PKA. Using the quantitative virulence index scores, all data was compared (Table 1). Data was compared on two scales; the macroscale to analyse only the number of clinical isolates within each virulence index category and the microscale to analyse individual clinical isolate virulence index scores for each phage combination.
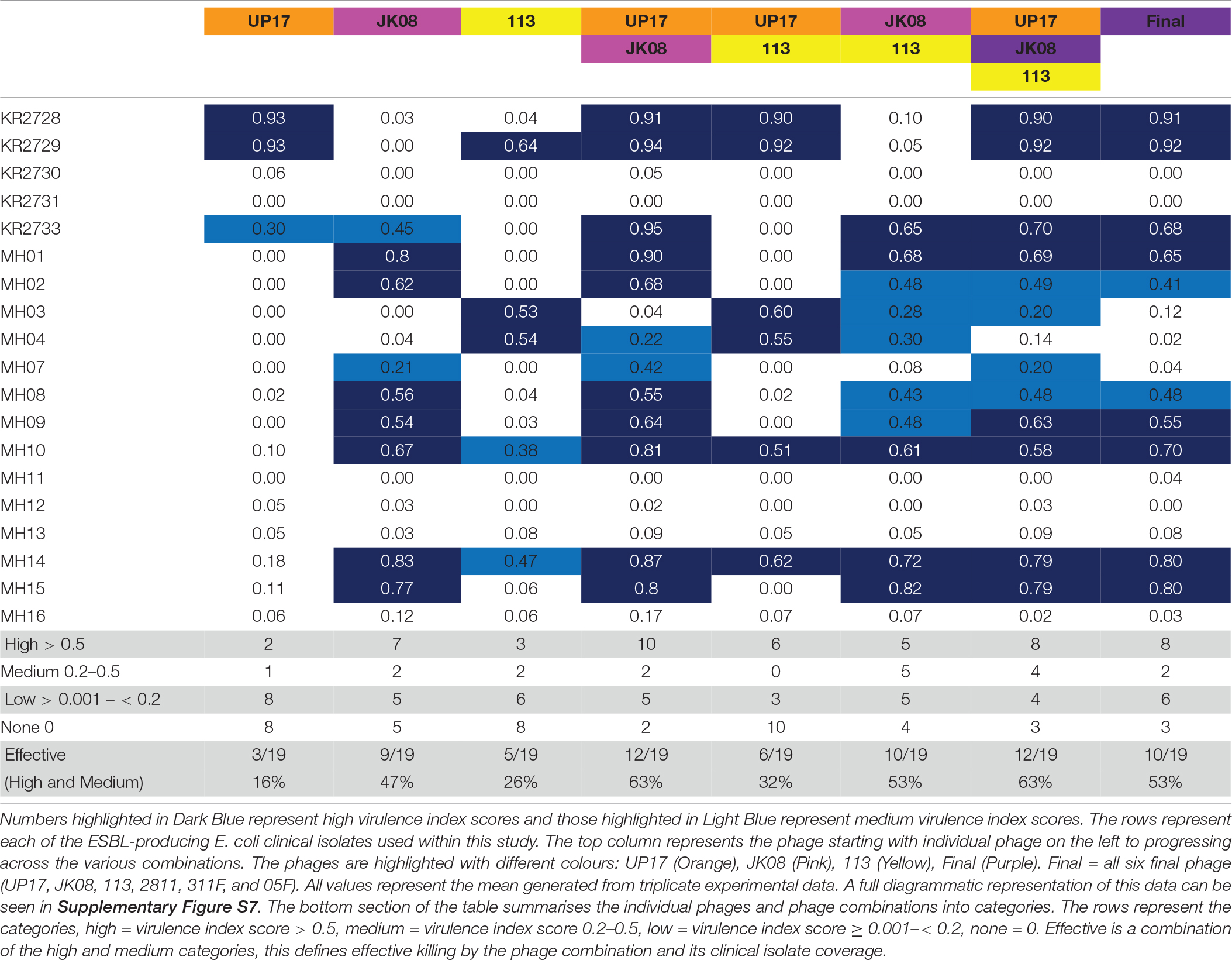
Table 1. The virulence index scores of individual phage and various phage combinations across all the 19 ESBL-producing E. coli clinical isolates.
Based on the virulence index data from the three individual phages (UP17, JK08, and 113), 13/19 (68%) of E. coli isolates should be targeted. However, only 12/19 (63%) were actually targeted (Table 1). There was an unexpected reduction in the number of isolates killed by the triplet phage combination (63%) when compared with the final six phage combination (53%) (Table 1).
When comparing virulence index scores, there were no substantial differences between the triplet cocktail (UP17, JK08, and 113) and the final six phage cocktail for the majority of the individual clinical isolates (Table 1). However, the virulence index identified inhibitory combinations. For example, when KR2729 was treated with phage 113 alone a high virulence index score of 0.64 is obtained (Table 1). But when used in combination with phage JK08 (JK08 and 113), its virulence index score dropped to almost zero (0.05) (Table 1). When all three phages were used in combination, the high virulence index score is restored to 0.92, which could be due to phage UP17 alone (Table 1). This effect is only noted where phage 113 is the only phage to have a high virulence index score, but with no noticeable effect from phage JK08 (Table 1). The effect was not noted in combinations where both phages JK08 and 113 had medium or high virulence index scores. This was exemplified by clinical isolates MH10 and MH14 (Table 1).
Conversely synergistic interactions were also observed. Treating KR2733 with phage UP17 or JK08 results in virulence index scores of 0.3 and 0.45, respectively. However, when used in combination the virulence index increases to 0.95 (Table 1). A similar pattern can be seen for clinical isolates, MH01, MH10, and MH07 (Table 1) with this phage combination.
There is an example within the virulence index data of diminished returns with E. coli clinical isolate MH03. When the clinical isolate is infected with 113 alone (0.53), JK08 and 113 (0.28), all 3 E. coli phages (0.20), and the final 6-phage cocktail (0.12). This demonstrates that increasing the number of phages within a cocktail is not necessarily beneficial.
Analysis of the Combinations of ESBL Klebsiella Phages Using Virulence Index Scores
The same selection process was carried out for comparison of Klebsiella phages. The effectiveness of different combinations of phages 2811, 311F, and 05F was compared using the virulence index scores to assess the efficacy (Table 2). The most effective doublet combination was 311F and 05F, which targets 53% of isolates. The addition of a further phage had a detrimental effect, reducing the number of isolates killed to 37% (Table 2).
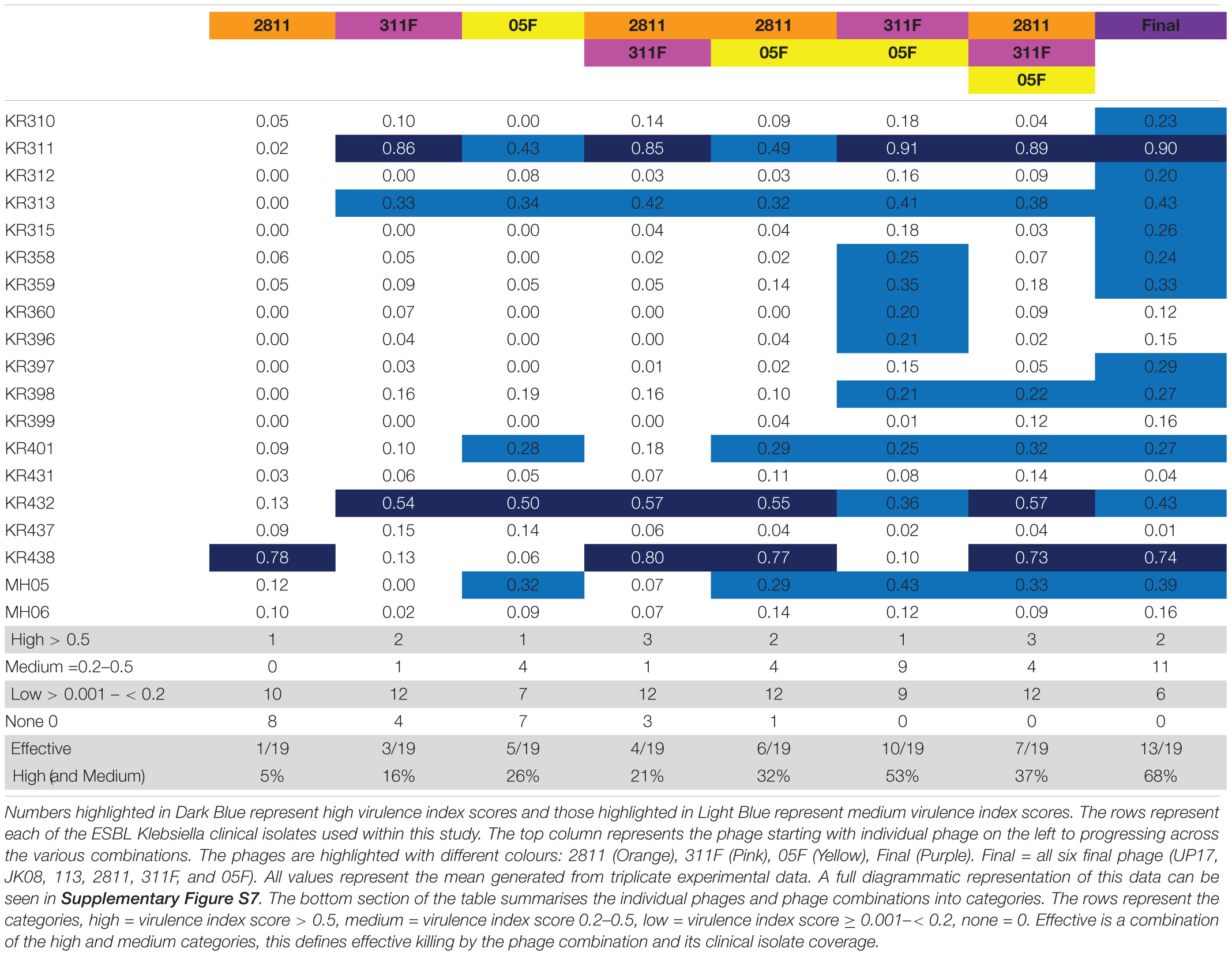
Table 2. The virulence index scores of individual phage and various phage combinations across all the 19 ESBL-producing Klebsiella clinical isolates.
Seven clinical isolates are targeted by the triplet cocktail compared to the six isolates covered based on the individual phage data (Table 2). The additional clinical isolate targeted by the triplet, KR398, showed a virulence index score (0.22) just above the threshold (Table 2). This suggests that for the Klebsiella phages, the killing seen with the individual phages translates directly to the triplet combination of phages. Additionally, the virulence index scores of the individual phages and of the triplet suggesting no synergy or competitive inhibition across all the clinical isolates. For example, clinical isolate KR438, phage 2811 only (0.78), triplet (0.73) or clinical isolate MH05 phage 05F only (0.32), triplet (0.33) (Table 2).
Analysis of the doublet (311F and 05F) showed unexpected synergistic combination. For five clinical isolates (KR358, KR359, KR360, KR396, and KR398), individually phages 311F and 05F had an almost negligible effect, but when combined (311F and 05F) they demonstrated medium virulence index scores for all strains (Table 2). For the triplet cocktail (2811, 311F, and 05F), five clinical isolates (KR310, KR312, KR315, KR358, KR359, KR397) again had negligible virulence index scores (Table 2). But when exposed to the final cocktail (UP17, JK08, 113, 2811, 311F, and 05F), all five clinical isolates had a medium virulence index score (Table 2). This demonstrated a further unexpected synergy when added with the ESBL E. coli phages.
Effectiveness of the Final ESBL Phage Cocktail
The final ESBL cocktail was effective against 23/38 clinical isolates (61%) based on the virulence index data (any clinical isolates with a medium or high virulence index score > 0.2). The final ESBL cocktail was then tested in a 24 h biofilm assay, to test the cocktail in a bacterial virulence model.
The final ESBL cocktail was most effective against the E. coli clinical isolates. There was a significant (p < 0.05) decrease in bacterial cell viability in 11 (58%) and 13 (68%) isolates after 4 and 24 h of resazurin incubation, respectively (Figure 3).
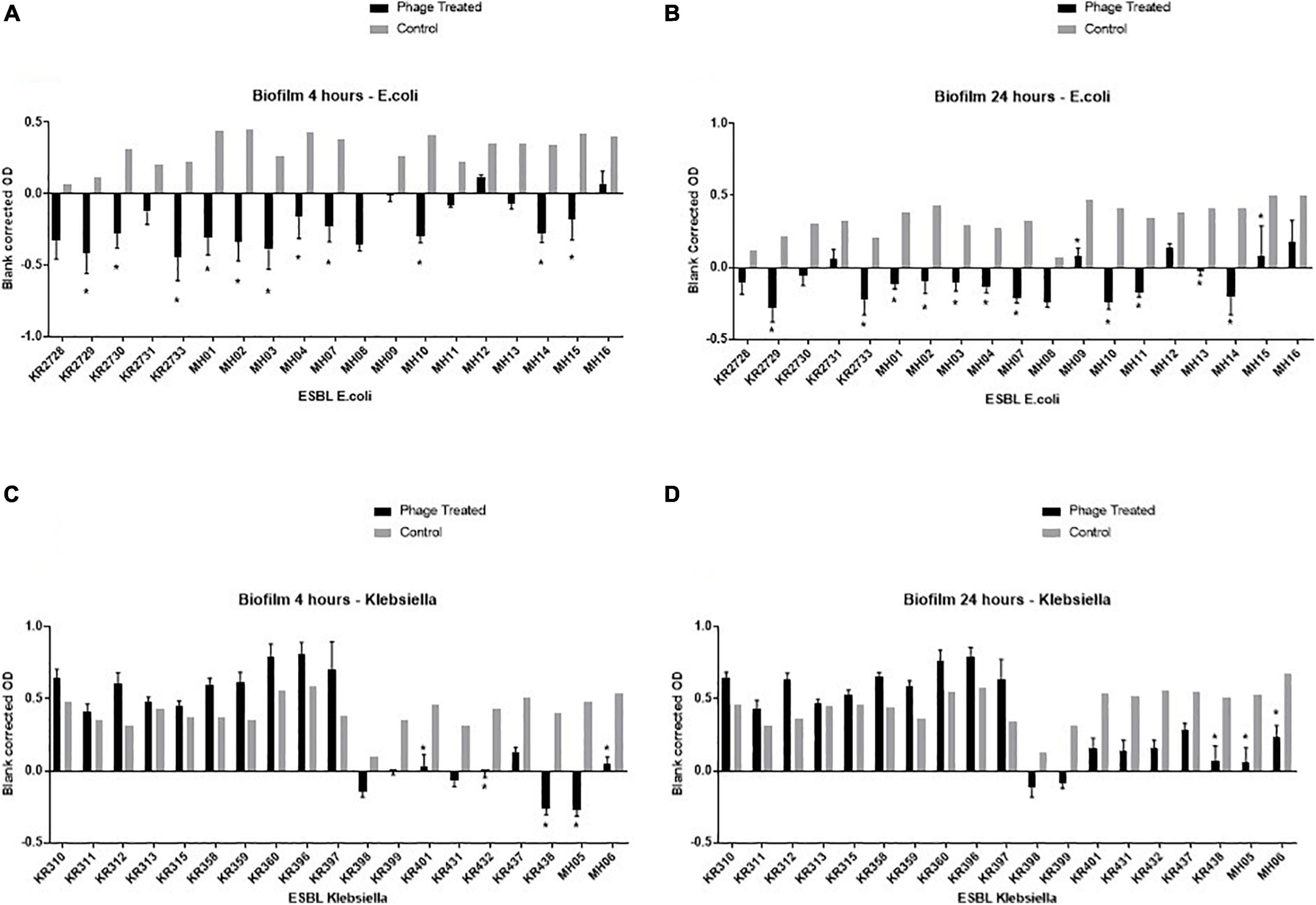
Figure 3. Graphical representation of the biofilm assay data—resazurin cell viability-based model on a 96 well plate. Clinical isolates were grown for 24 h on a flat bottom 96 well plate, then incubated for an additional 24 h with either LB (control) or the final phage cocktail (phage treated). Resazurin was then added, OD readings were taken at 4 and 24 h post adding resazurin. Each ESBL-producing clinical isolate has two bars: the black bar represents the phage treated blank corrected OD and the grey bar represents the control blank corrected OD. OD taken at A595, experiments repeated in triplicate for all clinical isolates, columns represents mean with standard error of the mean. * = significance difference between those treated with final phage cocktail and the control, p < 0.05. The top left graph (A) depicts the all ESBL producing E.coli clinical isolates 4 h post incubation with resazurin, top right (B) depicts ESBL producing E.coli clinical isolates 24 h post incubation with resazurin, the bottom left (C) depicts all ESBL-producing Klebsiella clinical isolates 4 h post incubation with resazurin and bottom right (D) depicts all ESBL-producing Klebsiella clinical isolates 24 h post incubation with resazurin.
For Klebsiella, at 4 h the cocktail only killed 5/19 (26%) of isolates and at 24 h 3/19 (16%) (Figure 3). This is in stark contrast to the high clinical isolate killing observed by the PKA of 13/19 (68%) (Table 2). An example of the disparity of results between the two tests is clinical isolate KR311. It had the highest virulence index score of 0.9 (Table 2), when using the final ESBL cocktail in the PKA but had no significant (p < 0.05) decrease in bacterial cell viability (Figure 3). However, the second highest virulence index score of 0.74 on isolate KR438 (Table 2) correlated with a significant (p < 0.05) reduction in the biofilm assay (Figure 3).
Genomic Analysis of the ESBL-Producing Clinical Isolates
Core genome SNP analysis was used to compare the clinical isolates (Figure 4). Ten different ST types of E. coli were identified, with the cocktail being able to target 10 strains across three ST types. The cocktail could target 5/6 of the ST131 clinical isolates, which is the most prevalent multidrug resistant uropathogen (Johnson et al., 2010; Kudinha et al., 2013). The core-genome SNP analysis of Klebsiella clearly separated the isolates into two different species (Figure 4). Three isolates were Klebsiella oxytoca and the remainder Klebsiella pneumoniae. There was a broad diversity of ST types present with 12 different types detected. There are representatives of the global endemic carbapenem-resistant associated ST258 as well as high risk AMR type ST147 (Bowers et al., 2015; Dhar et al., 2016; Peirano et al., 2020). The cocktail of phages was able to target a broad diversity of ST types across the three different bacterial species.
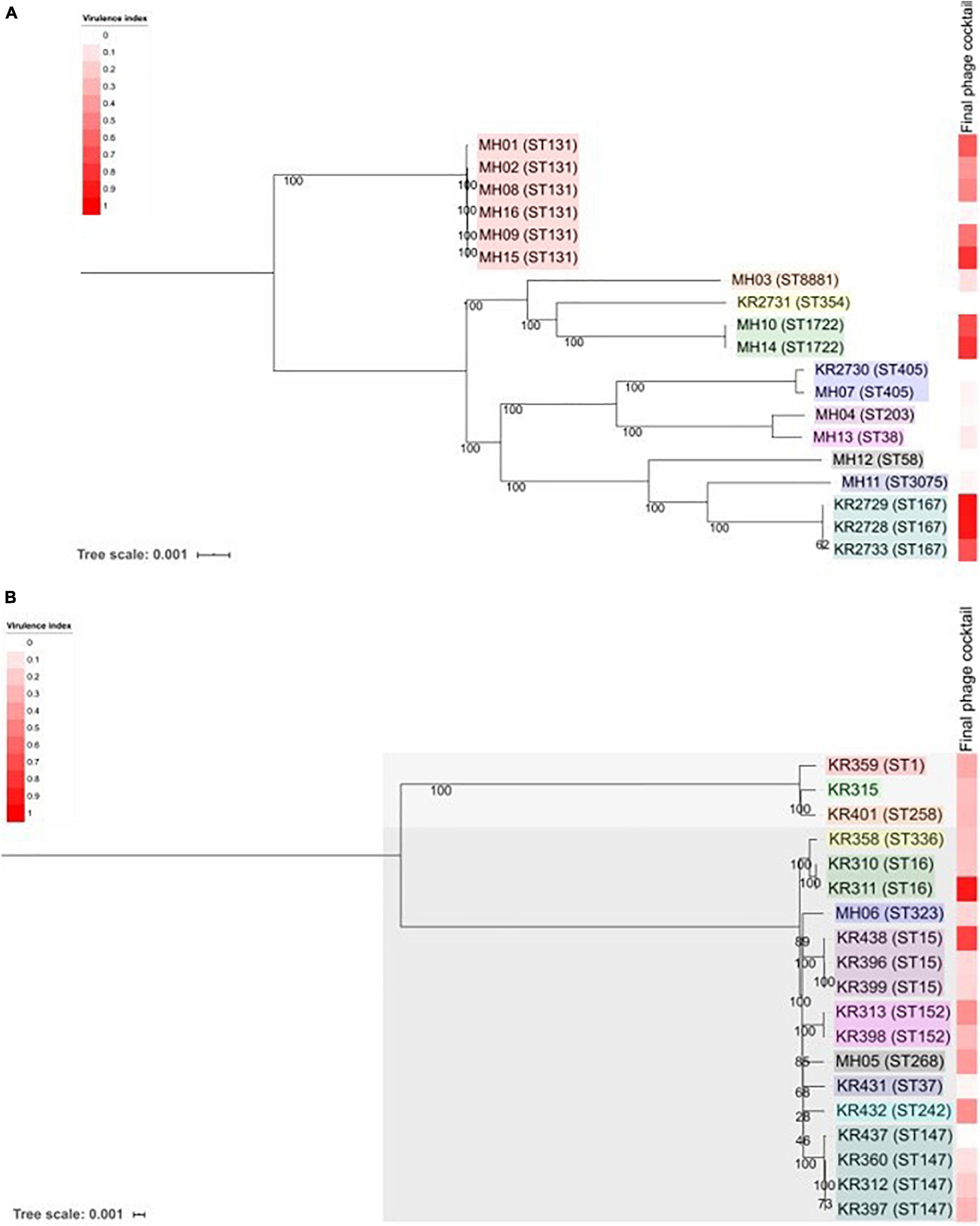
Figure 4. Phylogenetic analysis of the 38 ESBL-producing clinical isolates used in this study. (A) Shows ESBL-producing E. coli and (B) shows ESBL-producing Klebsiella spp. Trees were produced using MEGA7 to assess the core genome SNPs. Core-genome SNP analysis revealed that there were two species of Klebsiella. KR315, KR359 and KR401 are Klebsiella oxytoca and all others are Klebsiella pneumoniae. Each clinical isolate name is followed by its MLST (Achtman—E. coli)—please note KR315 was unable to be assigned. Tree scale noted and bootstrap values are labelled on branches. Coloured boxes within each tree represent groups of sequence types. The heat map on each tree represents the virulence index score assigned to the final phage cocktail for each strain.
Figure 5 allows an overview of the PKA virulence index scores taking into consideration all phage combinations including individual, doublets, triplets and the six-phage final cocktail that were used during this work. It also includes combinations using phages that were screened but not selected as the final six phages.
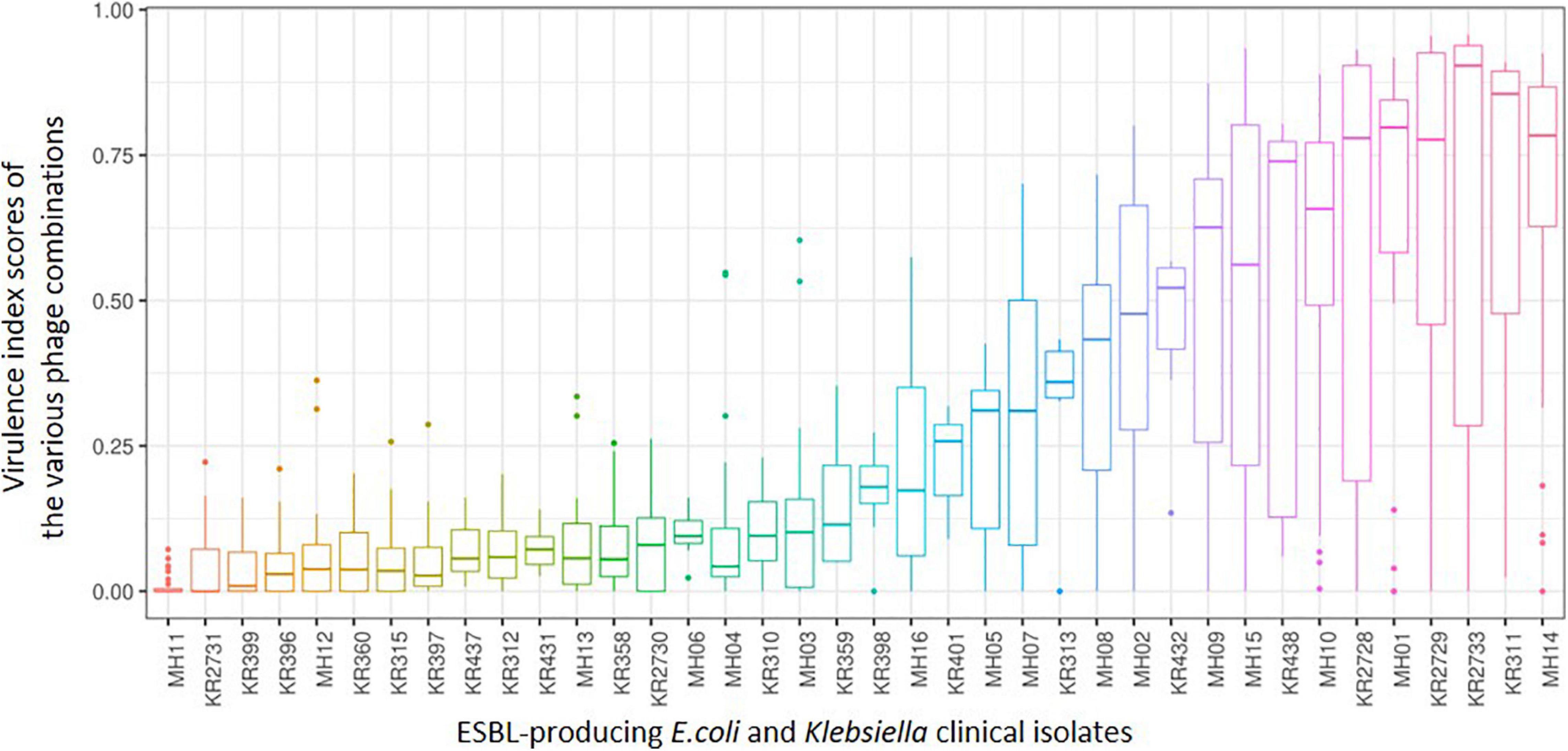
Figure 5. A box and whisker plot depicting the range of killing assay virulence index scores from all phage combinations explored during this work for each of the 38 ESBL-producing clinical isolates. From left to right shows the ESBL-producing clinical isolates that are most resistant to the combinations attempted to those that are most sensitive. Please note not all combinations were completed in triplicate for those combinations that were not part of the final ESBL cocktail (all six phages).
When comparing this data with the two phylogenetic trees (Figure 4) of all 38 clinical isolates, there is no clear pattern of genomic similarities to phage susceptibility. The most sensitive E. coli clinical isolates (MH14, KR2733, KR2729, MH01, KR2728) are spread across three different clades. In contrast the most resistant clinical isolates were spread across five different clades (MH11, KR2731, MH12, MH13, and KR2730). With regards to Klebsiella, the most sensitive strains were spread across five clades (KR311, KR438, KR432, KR313, MH05). The most resistant strains were distributed across three different clades (KR399, KR396, KR360, KR315, KR397).
Discussion
Antimicrobial resistance is an urgent issue that needs to be addressed. Phage therapy could be part of the solution. This work focuses on the development of an effective phage cocktail in response to this need. The aim of this work was to assess phage selection methods to streamline the development of a phage cocktail. This was achieved using an example of a phage cocktail against ESBL-producing clinical isolates of E. coli and Klebsiella.
DST is commonly used in the literature to assess the host range of phage (Sybesma et al., 2016; Hyman, 2019; Montso et al., 2019). The data demonstrated that the DST overestimates the host range or clinical isolate coverage of the individual phage by approximately 50% compared with the EOP and the PKA (Figure 1). The discrepancy between DST and EOP is in keeping with previous publications relating to Enterobacteriaceae species (Mirzaei and Nilsson, 2015; Manohar et al., 2019). It is considered to be due to other mechanisms of killing noted with DST, such as “lysis from without,” that are not a result of phage replication (Hyman and Abedon, 2010). But when comparing EOP with PKA data, there is less disparity in the numbers of clinical isolates and specific individual clinical isolates covered. There is also a trade-off in experimental time between the methods described in terms of labour-intensity and actual experimental time under our laboratory conditions. For example, set-up time for DST ∼5 h, EOP ∼6 h versus PKA ∼2 h. In addition, EOP being the most labour intensive and requiring the most materials. This is compared to PKA which due to the 96-well plate format uses small volume of materials and allows the use of labour-saving device such as a multi-channel pipettor. So as EOP and PKA give similar results, the savings in labour and material would favour PKA.
Use of the virulence index score for analysis across a large dataset allowed direct comparison of individual phages and phage combinations, which would be a powerful tool for cocktail design. Overall, based on the dataset generated by this work, there is not a clear formula for the expected outcome when combining phages. This is due to either synergy or inhibition, which cannot be easily predicted. The ability of the virulence index to detect these interactions is a clear advantage over the use of DST or EOP as a selection method. The concepts of viral interference and augmentation have previously been discussed in the literature (Casey et al., 2018). Synergistic enhancement could be due to an effect on one or more of the three properties: (1) rate of infection, (2) production of progeny, or (3) the time window between infection and progeny release (Schmerer et al., 2014). Therefore, synergy of phage infection is an additional advantage for the creation of a successful phage cocktail (Schmerer et al., 2014). The PKA method alongside analysis using the virulence index score could make this a realistic research aim during future cocktail design. UP17 was interesting in that it also appeared to be resistant to interference from the other phages within the cocktail. This is shown with Table 1, where UP17 had a high virulence score against a particular clinical isolate (KR2728 and KR2729) this score is maintained throughout all the other phage combinations with UP17 (UP17 and JK08, UP17 and 113, UP17 and JK08 and 113, all 6).
Overall, it would be worth investigating further, why UP17 is resistant to interference from the other phages as well as to why its effectiveness increases when combined with JK08. In addition to this, also consider why JK08 and 113 had an antagonistic relationship. This could be due to the phages having similar receptor sites and one being more likely to lead to an abortive infection, or superinfection resulting in an unsuccessful infection for both (Abedon, 2015). Answering all of these questions, may help determine effective future cocktail design. The strength of this work is the use of the virulence index score to be able to support the combination of phages together in a cocktail by providing clear evidence of synergy. This synergy would not be apparent from other commonly used selection methods such as DST and EOP. In addition, this method also outperforms the previous PKA methods, with the use of time course measurements in a 96-well plate format, as it allows high throughput of a large number of individual phage/phage combinations and clinical isolate panels.
The data suggests that other factors may come into play for Klebsiella clinical isolates. When comparing the phage virulence assays of the biofilm assay and PKA, there appears to be no correlation for Klebsiella clinical isolates. For example, when using the final ESBL cocktail the clinical isolate KR311 has the highest virulence index score of 0.9 in the PKA (Table 2) and yet there was no significant (p < 0.05) decrease in the biofilm assay (Figure 3). In contrast, the second highest virulence index score of 0.74 against KR438 correlated with a significant (p < 0.05) reduction in cell viability in the biofilm assay (Table 2 and Figure 3). Overall, when assessing the clinical isolates that demonstrated a significant (p < 0.05) reduction in cell viability for at least one of the timepoints during the biofilm assay, there appears to be no correlation with the PKA virulence index. This is a disappointing result, at least for this biofilm model, as the ideal case would be for the high-throughput method to determine virulence such as the PKA to translate to effectiveness in an in vitro bacterial model of virulence. The final cocktail covered 13 isolates (68%) in the PKA (Table 2) in comparison to 5/19 (26%) clinical isolates at 4 h and 3/19 (16%) clinical isolates at 24 h within the biofilm assay (Figure 3). It has been demonstrated in the literature that the use of phage can cause a significant reduction in biofilm production in Klebsiella spp. (Tabassum et al., 2018; Taha et al., 2018). This is in conflict to data demonstrated in this work. The results with E. coli were more promising as the clinical isolate coverage was similar 10/19 (53%) for PKA and in the biofilm assay 11/19 (58%) at 4 h and 13/19 (68%) at 24 h. The overall aim of this work was to develop a phage cocktail that was effective against 90% of the ESBL-producing clinical isolate collection. This was not achieved, and the result may influence the clinical application of the final cocktail. The data presented here demonstrated it was highly effective against the global prominent AMR UTI-associated E. coli ST131 isolates with 5/6 (83%) isolates killed. The data in this paper would need to be reconciled with prevalence data of the different sequence types within the general population to be able to draw conclusions with regards to the true clinical application.
In this study, we also performed basic bacterial genetic analysis identifying those clinical isolates that were most resistant to phage infection and those that were most sensitive. This highlighted clinical isolates that were on the same clade on the phylogenetic tree (Figure 4) but have polar opposite phage sensitivity. An example, Klebsiella KR396 and KR399 are both resistant isolates against sensitive isolate Klebsiella KR438 (Figure 4). Further genetic analysis of those with polar opposite phage sensitivity could provide further insight into mechanisms of resistance. It could also provide an opportunity to assess the individual clinical isolates susceptibility across three different screening methods and biofilm assay to see if there were any markers that predicted the outcome. These markers could help in the design of cocktails. In the future, a more detailed genomic analysis of the clinical isolates will be reported.
This paper is intended to outline the initial selection of phages for a final cocktail formulation. It will provide a basis for further building toward a “gold” standard within the community. This data could be used alongside other publications that have generated comparative data from the phage virulence methods described—DST, EOP, PKA and biofilm assay (Chen et al., 2018; Forti et al., 2018; Yang et al., 2020). There are two alternative routes that could be considered for use (Figure 6). Both commence with DST as the initial screening test. This would be used to eliminate phage candidates with low coverage of the clinical isolate collection. The refined list of phage candidates will either undergo EOP or PKA as individual phages against the clinical isolate collection to refine the list further. This will be achieved by providing a more accurate host range. In turn, this will allow selection of phages that have broad and complementary host ranges to ensure the widest coverage of the clinical isolate collection. Those phages selected will be used in various combinations within the PKA. When the optimal combination is elicited, it will be tested in the biofilm assay.
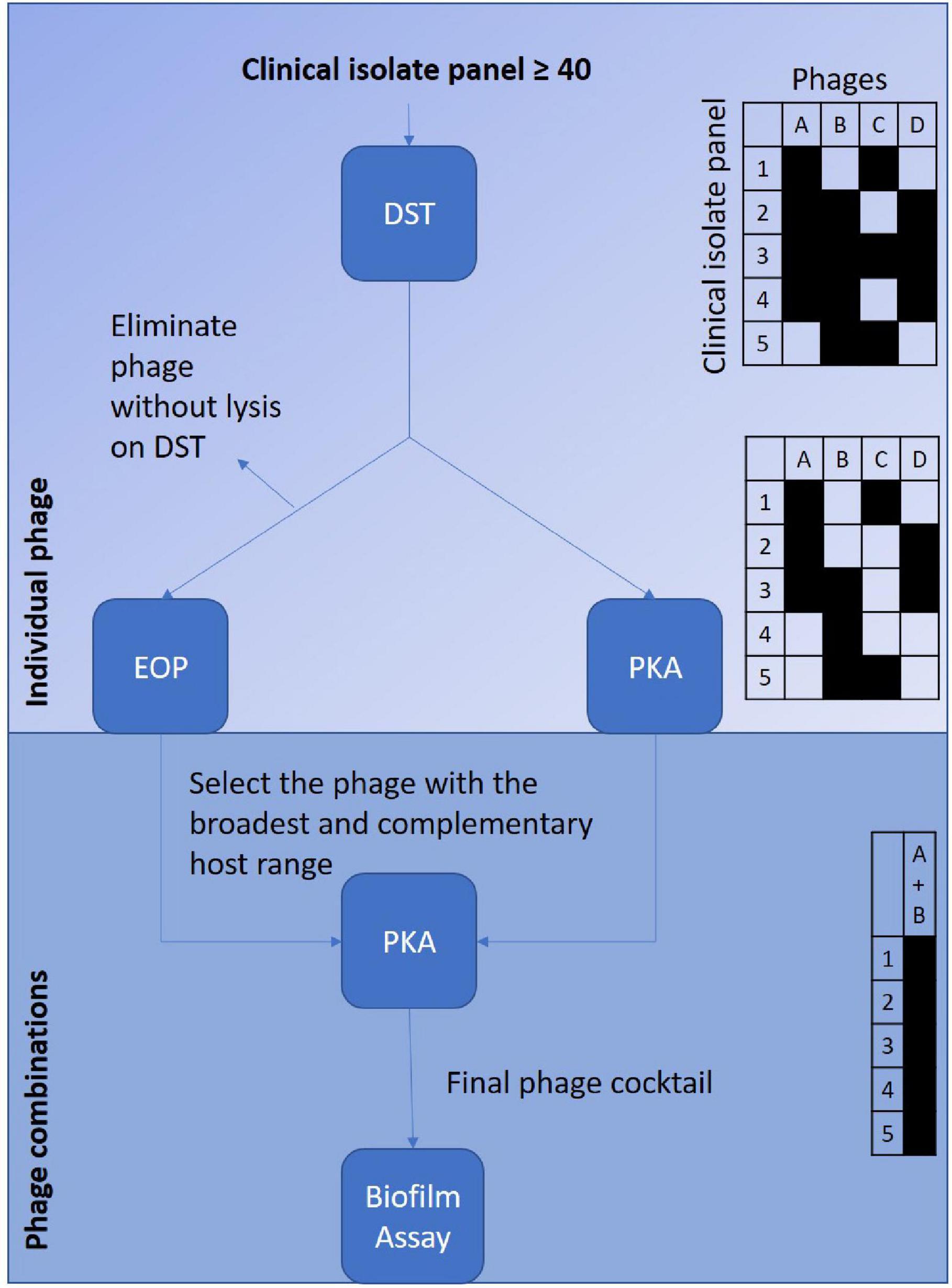
Figure 6. Depicts the suggested selection processes to screen a phage library against a clinical isolate collection to optimise development of a potentially therapeutic phage cocktail. The left sided route suggests starting with the screening of the individual phages via the DST followed by EOP to confirm host-range. The right sided route suggests starting initially screening the individual phages with the DST followed by the PKA to confirm host-range. Both routes are then followed by selection of the phages that have the broadest and complementary host range to combine to test in combinations against the clinical isolate collection with the PKA. The final phage combination will be then be tested in biofilm assay. The tables on the right hand of the figure are to depict examples of the host-range coverage provided by each of the virulence tests.
In conclusion, DST and EOP are not as useful as PKA as selection methods for designing phage cocktails. This is due to the inability of the DST and EOP to identify beneficial synergy as well as avoid inhibition. But DST and EOP are more easily accomplished and initially can add to the confidence of phage selection.
Data Availability Statement
The datasets presented in this study can be found in online repositories. The names of the repository/repositories and accession number(s) can be found in the article/Supplementary Material.
Author Contributions
MH and MC designed the experiments. MH, JN, JM, DvS, JK, BA, and MA provided the phage used in the experiments. MH performed the DST, planktonic killing assays, and biofilm assays. MH and FH performed the EOP assays. JK, NB, and AT prepared phage genomic DNA for sequencing. FH and AM performed the bioinformatic analysis of the phage and bacterial genomes. MH, FH, DS, and NB analysed the data. MH interpreted the results. TS-P for figure design and creation. MH drafted the manuscript. FH, JN, JM, DS, AT, AM, EG, and MC edited the manuscript. All authors contributed to the article and approved the submitted version.
Funding
MH was funded by a National Institute for Health Research (NIHR) Academic Clinical Fellowship for this research project. The views expressed in this publication are those of the author(s) and not necessarily those of the NIHR or the Department of Health and Social Care. BA was funded by the Ministry of Education in Saudi Arabia as a Ph.D. sponsorship to BA (KSU/1480/E). JM and DV were partly supported by a grant generously provided by the Bill and Melinda Gates Foundation (Ref. No. OPP1150567). JM was also supported by a Starting Investigator Research Grant (SIRG) (Ref. No. 15/SIRG/3430) funded by Science Foundation Ireland (SFI). DV was supported by a Principal Investigator award (Ref. No. 13/IA/1953) through SFI. FH was funded by the Biotechnology and Biological Sciences Research Council (BBSRC) Midlands Integrative Biosciences Training Partnership for this project. AM was funded by Natural Environment Research Council grants (NE/N019881/1 and NE/N003241/1) and MRC CLIMB (MR/L015080/1).
Conflict of Interest
The authors declare that the research was conducted in the absence of any commercial or financial relationships that could be construed as a potential conflict of interest.
Acknowledgments
Thanks to Mohammed Imam and Wafaa Alrashidi for access to their phages used during initial screening, but not used in final ESBL cocktail. Thanks to University of Leicester and Marialuisa Crosatti for access to the Kumar Rajakumar bacterial strain collection. Thanks to Shaun Livesey from University Hospitals of Leicester for support with completing the ESBL-producing clinical isolate collection. Thanks to Lucy Gannon and Christian Harrison for technical support. Thanks to Natalie Allcock from University of Leicester for producing Transmission Electron Microscopy Images for UP17, 113, 2811, 311F, and 05F. Thanks to Horst Neve at MRI, Kiel, Germany for producing Transmission Electron Microscopy Images for JK08. Thanks to Gurinder Vinner for support with laboratory skills and experiment planning. Thanks to Courtney Carter for creating the image composites. This publication made use of the PubMLST website (https://pubmlst.org/) developed by Keith Jolley (Jolley and Maiden, 2010; BMC Bioinformatics, 11:595) and sited at the University of Oxford. The development of that website was funded by the Wellcome Trust.
Supplementary Material
The Supplementary Material for this article can be found online at: https://www.frontiersin.org/articles/10.3389/fmicb.2021.613529/full#supplementary-material
Footnotes
References
Abedon, S. T. (2015). Bacteriophage secondary infection. Virol. Sin. 30, 3–10. doi: 10.1007/s12250-014-3547-2
Ansaldi, M., Debarbieux, L., Gandon, S., Petit, M. A., Tavares, P., and Boulanger, P. (2018). “French phage network”-third meeting report. Viruses 10:E123. doi: 10.3390/v10030123
Bankevich, A., Nurk, S., Antipov, D., Gurevich, A. A., Dvorkin, M., Kulikov, A. S., et al. (2012). SPAdes: a new genome assembly algorithm and its applications to single-cell sequencing. J. Comput. Biol. 19, 455–477. doi: 10.1089/cmb.2012.0021
Bolger, A. M., Lohse, M., and Usadel, B. (2014). Trimmomatic: a flexible trimmer for Illumina sequence data. Bioinformatics 30, 2114–2120. doi: 10.1093/bioinformatics/btu170
Bowers, J. R., Kitchel, B., Driebe, E. M., MacCannell, D. R., Roe, C., Lemmer, D., et al. (2015). Genomic analysis of the emergence and rapid global dissemination of the clonal group 258 Klebsiella pneumoniae pandemic. PLoS One 10:e0133727. doi: 10.1371/journal.pone.0133727
Bush, K. (2018). Past and present perspectives on β-lactamases. Antimicrob. Agents Chemother. 62:e01076-18. doi: 10.1128/AAC.01076-18
Casey, E., van Sinderen, D., and Mahony, J. (2018). In vitro characteristics of phages to guide ‘real life’ phage therapy suitability. Viruses 10:163. doi: 10.3390/v10040163
Chan, B. K., and Abedon, S. T. (2012). Phage therapy pharmacology phage cocktails. Adv. Appl. Microbiol. 78, 1–23. doi: 10.1016/B978-0-12-394805-2.00001-4
Chan, B. K., Abedon, S. T., and Loc-Carrillo, C. (2013). Phage cocktails and the future of phage therapy. Future Microbiol. 8, 769–783. doi: 10.2217/fmb.13.47
Chen, L., Yuan, S., Liu, Q., Mai, G., Yang, J., Deng, D., et al. (2018). In Vitro design and evaluation of phage cocktails against Aeromonas salmonicida. Front. Microbiol. 9:1476. doi: 10.3389/fmicb.2018.01476
Chevreux, B., Wetter, T., and Suhai, S. (1999). Genome sequence assembly using trace signals and additional sequence information. Comput. Sci. Biol. Proc. Ger. Conf. Bioinform. 99, 45–56.
Dhar, S., Martin, E. T., Lephart, P. R., McRoberts, J. P., Chopra, T., Burger, T. T., et al. (2016). Risk factors and outcomes for carbapenem-resistant Klebsiella pneumoniae isolation, stratified by its multilocus sequence typing: ST258 versus non-ST258. Open Forum Infect. Dis. 3:ofv213. doi: 10.1093/ofid/ofv213
Feldgarden, M., Brover, V., Haft, D. H., Prasad, A. B., Slotta, D. J., Tolstoy, I., et al. (2019). Validating the AMRFINder tool and resistance gene database by using antimicrobial resistance genotype-phenotype correlations in a collection of isolates. Antimicrob. Agents Chemother. 63:e00483-19. doi: 10.1128/AAC.00483-19
Forti, F., Roach, D. R., Cafora, M., Pasini, M. E., Horner, D. S., Fiscarelli, E. V., et al. (2018). Design of a broad-range bacteriophage cocktail that reduces Pseudomonas aeruginosa biofilms and treats acute infections in two animal models. Antimicrob. Agents Chemother. 62:e02573-17. doi: 10.1128/AAC.02573-17
Garneau, J. R., Depardieu, F., Fortier, L. C., Bikard, D., and Monot, M. (2017). PhageTerm: a tool for fast and accurate determination of phage termini and packaging mechanism using next-generation sequencing data. Sci. Rep. 7, 1–10. doi: 10.1038/s41598-017-07910-5
Gorski, A., Miedzybrodzki, R., Lobocka, M., Glowacka-Rutkowska, A., Bednarek, A., Borysowski, J., et al. (2018). Phage therapy: what have we learned? Viruses 10:E288. doi: 10.3390/v10060288
Gurevich, A., Saveliev, V., Vyahhi, N., and Tesler, G. (2013). QUAST: quality assessment tool for genome assemblies. Bioinformatics 29, 1072–1075. doi: 10.1093/bioinformatics/btt086
Hancock, V., Dahl, M., and Klemm, P. (2010). Abolition of biofilm formation in urinary tract Escherichia coli and klebsiella isolates by metal interference through competition for fur. Appl. Environ. Microbiol. 76, 3836–3841. doi: 10.1128/AEM.00241-10
Hyman, P. (2019). Phages for phage therapy: isolation, characterization, and host range breadth. Pharmaceuticals 12:35. doi: 10.3390/ph12010035
Hyman, P., and Abedon, S. T. (2010). Bacteriophage host range and bacterial resistance. Adv. Appl. Microbiol. 70, 217–248. doi: 10.1016/S0065-2164(10)70007-1
Johnson, J. R., Johnston, B., Clabots, C., Kuskowski, M. A., and Castanheira, M. (2010). Escherichia coli sequence type ST131 as the major cause of serious multidrug-resistant E. coli infections in the United States. Clin. Infect. Dis. 51, 286–294. doi: 10.1086/653932
Jolley, K. A., and Maiden, M. C. J. (2010). BIGSdb: Scalable analysis of bacterial genome variation at the population level. BMC Bioinformatics 11:595. doi: 10.1186/1471-2105-11-595
Kalra, O. P., and Raizada, A. (2009). Approach to a patient with urosepsis. J. Glob. Infect. Dis. 1, 57–63. doi: 10.4103/0974-777X.52984
Kropinski, A. M., Mazzocco, A., Waddell, T. E., Lingohr, E., and Johnson, R. P. (2009). Chapter 7: enumeration of bacteriophages by double agar overlay plaque assay. Methods Mol. Biol. 501, 69–76. doi: 10.1007/978-1-60327-164-6_7
Kudinha, T., Johnson, J. R., Andrew, S. D., Kong, F., Anderson, P., and Gilbert, G. L. (2013). Escherichia coli sequence type 131 as a prominent cause of antibiotic resistance among urinary Escherichia coli isolates from reproductive-age women. J. Clin. Microbiol. 51, 3270–3276. doi: 10.1128/JCM.01315-13
Kumar, S., Stecher, G., Li, M., Knyaz, C., and Tamura, K. (2018). MEGA X: molecular evolutionary genetics analysis across computing platforms. Mol. Biol. Evol. 35, 1547–1549. doi: 10.1093/molbev/msy096
Kutateladze, M., and Adamia, R. (2008). Phage therapy experience at the Eliava Institute. Med. Mal. Infect. 38, 426–430. doi: 10.1016/j.medmal.2008.06.023
Kutter, E. (2009). Chapter 14: phage host range and efficiency of plating. Methods Mol. Biol. 501, 141–149. doi: 10.1007/978-1-60327-164-6_14
Letunic, I., and Bork, P. (2007). Interactive Tree Of Life (iTOL): an online tool for phylogenetic tree display and annotation. Bioinformatics 23, 127–128. doi: 10.1093/bioinformatics/btl529
Li, D., Liu, C. M., Luo, R., Sadakane, K., and Lam, T. W. (2015). MEGAHIT: an ultra-fast single-node solution for large and complex metagenomics assembly via succinct de Bruijn graph. Bioinformatics 31, 1674–1676. doi: 10.1093/bioinformatics/btv033
Livermore, D. M. (1987). Clinical significance of beta-lactamase induction and stable derepression in gram-negative rods. Eur. J. Clin. Microbiol. 6, 439–445. doi: 10.1007/BF02013107
Manohar, P., Tamhankar, A. J., Lundborg, C. S., and Nachimuthu, R. (2019). Therapeutic characterization and efficacy of bacteriophage cocktails infecting Escherichia coli, Klebsiella pneumoniae, and Enterobacter Species. Front. Microbiol. 10:574. doi: 10.3389/fmicb.2019.00574
Mattila, S., Ruotsalainen, P., and Jalasvuori, M. (2015). On-demand isolation of bacteriophages against drug-resistant bacteria for personalized phage therapy. Front. Microbiol. 6:1271. doi: 10.3389/fmicb.2015.01271
Michniewski, S., Redgwell, T., Grigonyte, A., Rihtman, B., Aguilo-Ferretjans, M., Christie-Oleza, J., et al. (2019). Riding the wave of genomics to investigate aquatic coliphage diversity and activity. Environ. Microbiol. 21, 2112–2128. doi: 10.1111/1462-2920.14590
Mirzaei, M. K., and Nilsson, A. S. (2015). Isolation of phages for phage therapy: a comparison of spot tests and efficiency of plating analyses for determination of host range and efficacy. PLoS One 10:e0118557. doi: 10.1371/journal.pone.0118557
Montso, P. K., Mlambo, V., and Ateba, C. N. (2019). Characterization of lytic bacteriophages infecting multidrug-resistant shiga toxigenic atypical Escherichia coli O177 strains isolated from cattle feces. Front. Public Heal. 7:355. doi: 10.3389/fpubh.2019.00355
Nale, J. Y., Spencer, J., Hargreaves, K. R., Buckley, A. M., Trzepinski, P., Douce, G. R., et al. (2015). Bacteriophage combinations significantly reduce clostridium difficile growth in vitro and proliferation in vivo. Antimicrob. Agents Chemother. 60, 968–981. doi: 10.1128/AAC.01774-15
O’Neill, J. (2014). Antimicrobial resistance: tackling a crisis for the health and wealth of nations. Rev. Antimicrob. Resist. Available at: http://amrreview.org/ (accessed March 4, 2021).
Peirano, G., Chen, L., Kreiswirth, B. N., and Pitout, J. D. D. (2020). Emerging antimicrobial resistant high-risk clones among Klebsiella pneumoniae: ST307 and ST147. Antimicrob. Agents Chemother. 3:e01148-20. doi: 10.1128/aac.01148-20
Pendleton, J. N., Gorman, S. P., and Gilmore, B. F. (2013). Clinical relevance of the ESKAPE pathogens. Expert Rev. Anti. Infect. Ther. 11, 297–308. doi: 10.1586/eri.13.12
Rivera, D., Hudson, L. K., Denes, T. G., Hamilton-West, C., Pezoa, D., and Moreno-Switt, A. I. (2019). Two phages of the genera felixunavirus subjected to 12 hour challenge on Salmonella infantis showed distinct genotypic and phenotypic changes. Viruses 11:586. doi: 10.3390/v11070586
Sakellariou, C., Gurntke, S., Steinmetz, I., Kohler, C., Pfeifer, Y., Gastmeier, P., et al. (2016). Sepsis caused by extended-spectrum beta-lactamase (ESBL)-positive K. pneumoniae and E. coli: comparison of severity of sepsis, delay of anti-infective therapy and ESBL genotype. PLoS One 11:e0158039. doi: 10.1371/journal.pone.0158039
Schmerer, M., Molineux, I. J., and Bull, J. J. (2014). Synergy as a rationale for phage therapy using phage cocktails. PeerJ 2:e590. doi: 10.7717/peerj.590
Seemann, T. (2014). Prokka: rapid prokaryotic genome annotation. Bioinformatics 30, 2068–2069. doi: 10.1093/bioinformatics/btu153
Seemann, T. (2020). mlst, Github. Available online at: https://github.com/tseemann/mlst (accessed February 19, 2020).
Storms, Z. J., Teel, M. R., Mercurio, K., and Sauvageau, D. (2019). The virulence index: a metric for quantitative analysis of phage virulence. Phage 1, 17–26. doi: 10.1089/phage.2019.0001
Sybesma, W., Zbinden, R., Chanishvili, N., Kutateladze, M., Chkhotua, A., Ujmajuridze, A., et al. (2016). Bacteriophages as potential treatment for urinary tract infections. Front. Microbiol. 7:465. doi: 10.3389/fmicb.2016.00465
Sykes, R. B., and Matthew, M. (1976). The beta-lactamases of gram-negative bacteria and their role in resistance to beta-lactam antibiotics. J. Antimicrob. Chemother. 2, 115–157. doi: 10.1093/jac/2.2.115
Tabassum, R., Shafique, M., Khawaja, K. A., Alvi, I. A., Rehman, Y., Sheik, C. S., et al. (2018). Complete genome analysis of a Siphoviridae phage TSK1 showing biofilm removal potential against Klebsiella pneumoniae. Sci. Rep. 8, 17904–17911. doi: 10.1038/s41598-018-36229-y
Tacconelli, E., Carrara, E., Savoldi, A., Harbarth, S., Mendelson, M., Monnet, D. L., et al. (2018). Discovery, research, and development of new antibiotics: the WHO priority list of antibiotic-resistant bacteria and tuberculosis. Lancet Infect. Dis. 18, 318–327. doi: 10.1016/S1473-3099(17)30753-3
Taha, O. A., Connerton, P. L., Connerton, I. F., and El-Shibiny, A. (2018). Bacteriophage ZCKP1: a potential treatment for Klebsiella pneumoniae isolated from diabetic foot patients. Front. Microbiol. 9:2127. doi: 10.3389/fmicb.2018.02127
Tomas, M., Whiteson, K. L., Ramos-Vivas, J., Furfaro, L. L., Payne, M. S., and Chang, B. J. (2018). Bacteriophage therapy: clinical trials and regulatory hurdles the challenge of multi-drug resistant bacteria. Front. Cell. Infect. Microbiol. 8:376. doi: 10.3389/fcimb.2018.00376
van Duin, D., and Doi, Y. (2017). The global epidemiology of carbapenemase-producing Enterobacteriaceae. Virulence 8, 460–469. doi: 10.1080/21505594.2016.1222343
Yang, Y., Shen, W., Zhong, Q., Chen, Q., He, X., Baker, J. L., et al. (2020). Development of a bacteriophage cocktail to constrain the emergence of phage-resistant Pseudomonas aeruginosa. Front. Microbiol. 11:327. doi: 10.3389/fmicb.2020.00327
Keywords: antimicrobial resistance, antibiotic resistance, urinary tract infection, bacteriophage, phage therapy, ESBL, E. coli, Klebsiella
Citation: Haines MEK, Hodges FE, Nale JY, Mahony J, van Sinderen D, Kaczorowska J, Alrashid B, Akter M, Brown N, Sauvageau D, Sicheritz-Pontén T, Thanki AM, Millard AD, Galyov EE and Clokie MRJ (2021) Analysis of Selection Methods to Develop Novel Phage Therapy Cocktails Against Antimicrobial Resistant Clinical Isolates of Bacteria. Front. Microbiol. 12:613529. doi: 10.3389/fmicb.2021.613529
Received: 02 October 2020; Accepted: 23 February 2021;
Published: 29 March 2021.
Edited by:
Naomi Sulinger Hoyle, Eliava Phage Therapy Center (EPTC), GeorgiaReviewed by:
Dwayne R. Roach, San Diego State University, United StatesStephen Tobias Abedon, The Ohio State University, United States
Copyright © 2021 Haines, Hodges, Nale, Mahony, van Sinderen, Kaczorowska, Alrashid, Akter, Brown, Sauvageau, Sicheritz-Pontén, Thanki, Millard, Galyov and Clokie. This is an open-access article distributed under the terms of the Creative Commons Attribution License (CC BY). The use, distribution or reproduction in other forums is permitted, provided the original author(s) and the copyright owner(s) are credited and that the original publication in this journal is cited, in accordance with accepted academic practice. No use, distribution or reproduction is permitted which does not comply with these terms.
*Correspondence: Melissa E. K. Haines, mh508@leicester.ac.uk