- 1Division of Soil and Environmental Biotechnology, National Institute for Biotechnology and Genetic Engineering-Campus-Pakistan Institute of Engineering and Applied Sciences (NIBGE-C-PIEAS), Faisalabad, Pakistan
- 2Department of Bioinformatics and Biotechnology, Government College University, Faisalabad, Pakistan
- 3Department of Botany, Government College University, Faisalabad, Pakistan
- 4Department of Botany, University of Bagh, Kotli, Pakistan
- 5Department of Biotechnology, Institute of Molecular Biology and Biotechnology, University of Lahore, Sargodha, Pakistan
During and after the green revolution in the last century, agrochemicals especially nitrogen (N) were extensively used. However, it resulted in a remarkable increase in crop yield but drastically reduced soil fertility; increased the production cost, food prices, and carbon footprints; and depleted the fossil reserves with huge penalties to the environment and ecological sustainability. The groundwater, rivers, and oceans are loaded with N excess which is an environmental catastrophe. Nitrogen emissions (e.g., ammonia, nitrogen oxide, nitrous oxide) play an important role in global climate change and contribute to particulate matter and acid rain causing respiratory problems, cancers, and damage to forests and buildings. Therefore, the nitrogen-polluted planet Earth needs concerted global efforts to avoid the disaster. Improved agricultural N management focuses on the synchronization of crop N demand and N supply along with improving the N-use efficiency of the crops. However, there is very little focus on the natural sources of N available for plants in the form of diazotrophic bacteria present inside or on the root surface and the rhizosphere. These diazotrophs are the mini-nitrogen factories that convert available (78%) atmospheric N2 to ammonia through a process known as “biological nitrogen fixation” which is then taken up by the plants for its metabolic functioning. Diazotrophs also stimulate root architecture by producing plant hormones and hence improve the plant’s overall ability to uptake nutrients and water. In recent years, nanotechnology has revolutionized the whole agri-industry by introducing nano-fertilizers and coated/slow-releasing fertilizers. With this in mind, we tried to explore the following questions: To what extent can the crop N requirements be met by diazotroph inoculation? Can N input to agriculture be managed in a way leading to environmental benefits and farmers saving money? Can nanotechnology help in technological advancement of diazotroph application? The review suggests that an integrated technology based on slow-releasing nano-fertilizer combined with diazotrophs should be adopted to decrease nitrogen inputs to the agricultural system. This integrated technology would minimize N pollution and N losses to much extent.
Nitrogen Fertilizer: Trends, Use Efficiency, and Problems of Excessive Usage
Nitrogen (N) is a vital element for life. Apart from being an integral component of DNA, it is part of amino acid, NAD, and ATP in the cells of living organisms. The nitrogen available for plant uptake is the key determining factor for plant yield as it plays an important role in plant metabolism and food quality (Massignam et al., 2009). N deficiency in plants leads to pale yellowish-green plants with slow growth, smaller flowers and dormant buds, and reduced tiller and fruit development. The N moves from organic form (plant materials) to inorganic form in soil upon decomposition, and in this way, soil fertility is also maintained (Dong et al., 2015).
The global N cycle has been altered more than that of any other basic element intentionally by using it as a fertilizer or unintentionally as a by-product of fossil fuel combustion. The global population directly or indirectly relies on N fertilizer for food supply. The fertilizer trends (Figure 1A) show a synchronized growth of the population and N usage. The 2nd half of the 20th century showed an 80% increase in N usage from 11 million tons in 1961 to 119 million metric tons in 2018 (Liu et al., 2015; FAO, 2017). The Asia-Pacific region is the largest consumer of the total N-fertilizer market (Figure 1B), accounting for 60% of the total global N-fertilizer usage. Although N fertilizer is necessary for most of the crops, yields have nearly reached their biological maxima whereas farmers keep on adding more and more fertilizers. Overall, N added to the field is about 10-fold higher than it is consumed (Erisman et al., 2013) because crops have low N uptake (30–40%) and thus low N-use efficiency (20–40%; Zhu et al., 2016; Omara et al., 2019). Excess fertilizer does not become part of the plant, but it leaches down and becomes part of the soil or is emitted to the atmosphere (Sharma and Bali, 2018). This shows that agriculture is the primary source of about two-thirds of global N pollution.
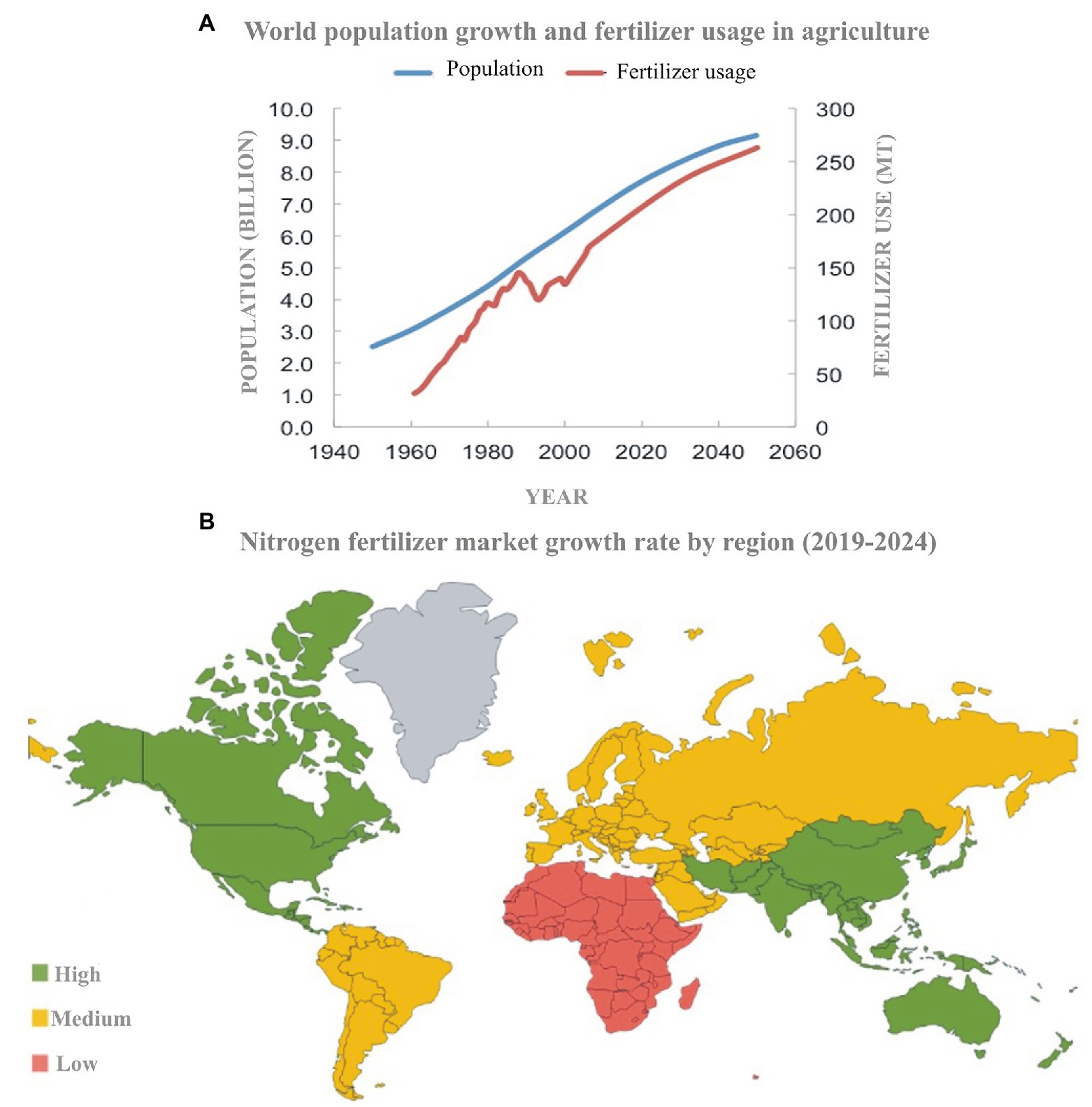
Figure 1. Fertilizer usage and population growth trends in the last 100 years and future prediction (source: Meer, 2016; A). Map showing the region-wise N-fertilizer input into the agricultural system (source: Mordor Intelligence; B).
Nitrogen emissions (e.g., ammonia, nitrogen oxide, nitrous oxide) play an important role in global climate change and contribute to particulate matter and acid rain causing respiratory problems, cancers, and damage to forests and buildings. Nitrous oxide (N2O) is a potent greenhouse gas, over 300 times more effective in trapping heat than carbon dioxide, and remains in the atmosphere for ≈114 years (Good and Beatty, 2011). Excessive N accumulation alters the atmospheric concentration of three anthropogenic greenhouse gases CO2, CH4, and N2O, and plays a crucial role in climate change. Mitigation of N use can lower the atmospheric burden of these greenhouse gases, thereby slowing the rate of climate change over the coming years.
Apart from the climate change, excessive use of N has led to increased pest infestation, e.g., aphids (Nevo and Coll, 2001), and accumulation of heavy metals (e.g., lead, chromium, and cadmium), radionuclides, and carcinogenic compounds (e.g., nitrosamine) as well as accumulation of NO2 and NO3 (Savci, 2012). Excessive use of N causes serious health concerns related to respiratory ailment, cardiac arrest, and several vector-borne diseases like malaria and cholera in cattle and humans (Ward et al., 2018). In Europe alone, the environmental and human health costs of N pollution are estimated to be 70–320 billion euros per year (Sutton et al., 2011). The continuous enrichment of N in the water leads to excessive growth of algae and other plants, a phenomenon known as “eutrophication” leading to the development of “dead zones.” These dead zones do not support any life forms due to the lack of oxygen and are found in any lake or coastal area. Eutrophication mediates the growth of harmful algal blooms (HABs; Paerl et al., 2011), and water becomes contagious for fisheries and drinking due to the increased growth of algae and oxygen shortage caused by their decomposition. The overall processes of HABs lead to global warming, salinization (drought), and longer seasons affecting plant growths as well. Excessive use of N also disrupts the growth and development of agronomic plants, especially affecting the phenolic, flavonoid, oil, and sugar contents in oil crops (Sesamum indicum) as well as antioxidant activity (Elhanafi et al., 2019). The ecological response to elevated nitrogen may increase allergenic pollen production in plants (Reinmuth-Selzle et al., 2017). Moreover, processes like nitrification, mineralization, and ammonia volatilization in air, water, and soil leachate leads to high nitrite nitrogen in drinking water, rivers, and crops, posing potential health hazards to ecosystems (Jia et al., 2015).
Management of N is a challenging goal and needs combined efforts to improve its efficiency. To avoid the problem from worsening, scientists warn that the global N use must cut back and N efficiency be increased in agriculture farms. European countries, being the leaders in “green policies” along with UN Environment Programme through the combination of the best nutrient management practices (BNMPs), International Nitrogen Management System (INMS), advances in fertilizer technology, and plant genetics, collectively aim to reduce worldwide N applications by 20–30% till 2050, saving US$ 150B annually.
Biological Nitrogen Fixation to Replace Fertilizer N
The process through which atmospheric nitrogen (N2) is converted to ammonia (NH3) is referred to as the biological nitrogen fixation (BNF), and the nitrogen-fixing bacteria are known as “Diazotrophs” (Shin et al., 2016). The BNF in legumes was described by Hellriegel and Wilfarth (1888) and Franche et al. (2009), and in cyanobacteria by Stewart (1969). The BNF is a highly energy-expensive process that is undertaken by symbiotic bacteria inside the root nodules and in soil or on plant roots by nonsymbiotic bacteria. This process is the natural and major source of nitrogen for plants within a range of ecosystems (Angus and Grace, 2017) and the key contributor to the N economy in the biosphere providing ≈128 Tg of N per annum globally (Vitousek et al., 2013), which is equal to 60% of total fixed N. Under field, diazotrophs fix ≈30–50% of N (Ormeño-Orrillo et al., 2013) contributing up to 15% of the total plant’s nitrogen. A low level of combined nitrogen and a high level of carbon compounds trigger the nitrogen-fixing activity of microorganisms. The process requires 1 mol of N2, a supply of electrons and protons, and 16 moles of ATP to produce 2 mol of ammonia as shown in the following equation:
The formation of ammonia from molecular hydrogen and nitrogen has an overall negative enthalpy of reaction (ΔH°= −45.2 KJ mol−1 NH3); the energy barrier to activation is generally insurmountable (EA°= 420 KJ mol−1) without the assistance of catalysis. The enzyme, therefore, requires a great deal of chemical energy, released from the hydrolysis of ATP (16 mol of ATP for each mole of N2 reduced), and reducing agents, such as dithionite in vitro or ferredoxin in vivo.
Plant-Microbe Interaction Concerning BNF and Suitable Modifications to Harness the Potential of BNF
The diazotrophs are phylogenetically diverse (Figure 2) comprising of organisms of varying physiological properties and belong to taxonomically diverse groups of bacteria from alphaprotobacteria, betaprotobacteria, gammaprotobacteria (Shin et al., 2016). Major genera include Azospirillum, Azorhizobium, Pseudomonas, Rhizobium, and phyla cyanobacteria, and Firmicutes. The three major types of bacteria that fix the nitrogen are rhizospheric, endophytic, and nodulating bacteria (Brusamarello-Santos et al., 2012) with a varying range of nitrogen fixation (Table 1). The associative and symbiotic diazotrophs are detailed below.
Associative Diazotrophs
Diazotrophs present in the soil mostly belong to this group and form a subset of the plant growth-promoting rhizobacteria (PGPR) which are directly beneficial for plant growth and yield. They reside on the surface of roots specifically in the zone of elongation or in root hairs. The bacteria found inside the shoot/root of the phyllosphere of the plants are classified as endophytic diazotrophs, and those found on the root surface are associative diazotrophs (Bashan et al., 2004). In a relative comparison of plant growth promotion and nitrogen assimilation by the plants, endophytic diazotrophs have an advantage over associative diazotrophs due to the establishment of better and stable niche within the plant tissues for nitrogen fixation and further assimilation of fixed nitrogen compounds by the plants (Moyes et al., 2016). The presence of these diazotrophs in the proximity to roots helps in the nutrient acquisition of plants, ultimately influencing the growth and yield in various crops (Wani et al., 2013).
They are omnipresent and belong to different genera, such as Cupriavidus, Frankia, sulfate-reducing bacteria, Pseudomonas, Azoarcus, Azospirillum, Burkholderia, Azorhizobium, Gluconacetobacter, Citrobacter, Klebsiella, Enterobacter, and Herbaspirillum (Santi et al., 2013). Examples are Herbaspirillum and Burkholderia which fix nitrogen in vesicular and intercellular spaces of sugarcane (Van Deynze et al., 2018), and Azospirillum, which is a facultative endophytic diazotroph of cereals. They move with the help of flagella to migrate to a micro-aerophilic condition where the nitrogenase enzyme is protected from oxygen (Steenhoudt and Vanderleyden, 2000). Azospirillum frequently exists in different regions of the world and develops an association with a wide-ranging species of plants (Pereg et al., 2016).
Symbiotic Diazotrophs
One of the best-studied symbioses is the symbiotic nitrogen fixation that involves plants (both legumes and non-legumes) and specific diazotrophs (rhizobia and Frankia). During this symbiotic relationship, a niche and carbon molecules are provided to the microorganisms by the plant in exchange for nitrogen fixation (Schwember et al., 2019). The symbiotic nitrogen fixation is the most efficient fixing system which approximately fixes 170–300 kg of nitrogen per hectare per year. Symbiotic diazotrophs are dominant in the soil environment, where they arrive inside the root of the plant and form a nodule on the plant where the symbiotic dialogue takes place (Tu et al., 2016). This process is controlled by several genes like nod, nif, and fix genes. The main function of the nodule is to produce an environment that restrains oxygen-free flow and triggers the nitrogen fixation process (Oldroyd, 2013).
Rhizobium-Legume Symbiosis
Rhizobia are classical legume symbionts belonging to Betaproteobacteria and Alphaproteobacteria. They form an effective nodule mainly on the root and in a few cases on the stem of the legume host with few exceptions of non-legumes (i.e., Parasponia). Parasponia nodules have a tissue organization and ontogeny different from that of legumes but very similar to that of actinorhizal nodules (Pawlowski and Bisseling, 1996). Some legumes belonging to the genera Neptunia, Aeschynomene, and Sesbania bear nodules on both roots and stems. The rhizobial species are distributed among 18 genera of different families including Methylobacteriaceae, Rhizobiaceae, Bradyrhizobiaceae, Phyllobacteriaceae, Brucellaceae, Hyphomicrobiaceae, Burkholderiaceae, and Xanthobacteraceae (Mousavi, 2016; De Lajudie et al., 2019). Some genera (e.g., Azorhizobium, Pararhizobium Allorhizobium, Neorhizobium, Sinorhizobium, and Mesorhizobium) are known as promiscuous hosts while others (e.g., Rhizobium) are highly specific.
Frankia-Non-legume Symbiosis
Nitrogen fixation in the nodules of non-legumes usually takes place by the symbiotic association with Frankia. Frankia is a filamentous, Gram-positive actinomycete that forms a symbiotic relationship with plant species of 25 genera belonging to eight different families (220 species) of dicotyledonous plants including Betulaceae, Casuarinaceae, Myricaceae, Elaeagnaceae, Rhamnaceae, Rosaceae, Coriariaceae, and Datisticaceae (Normand et al., 2014). Most of these nitrogen-fixing non-legumes are found in poor, sandy soils low in nitrogen. The common examples are alder, bayberry, sweet fern, sweet gale, buffalo berry, bitterbrush, pine, and olives. Their ability to fix nitrogen is a significant factor in their survival under conditions that would be inhospitable to ordinary plants. There are two ways through which Frankia forms an association with actinorhizal plants; i.e., the intracellular and intercellular penetration (Carvalho et al., 2014). It has different types of cells, e.g., vesicles and spores, located on the sporangia and the vegetative hyphae. Vesicles are the actual cells that are formed under limited N conditions and contain enzymes responsible for N fixation.
Cyanobacteria
Nonsymbiotic fixation of nitrogen involves fixation through heterotrophic or autotrophic organisms or by free-living diazotrophs called cyanobacteria. Cyanobacteria are the Gram-negative oxygenic photo-autotrophs that developed during the Precambrian period and reformed the anoxic earth’s atmosphere into oxic, which is appropriate for the process of oxygenic metabolism (Kaushik et al., 2017). Cyanobacteria have great agricultural importance. In freshwater and marine systems, they are the key N2 fixers which provide a great source of fixed nitrogen in the marine ecosystem in the world’s oceans. In terrestrial environments including rainforests and deserts, they abundantly grow and are involved in nitrogen fixation. Because of their special adaptations like nitrogen fixation and desiccation resistance ability, they can survive in harsh conditions. In modern agriculture, cyanobacterial mats have been used as biofertilizers as they provide more than 70% of the nitrogen fixed to the agricultural systems (Benavides et al., 2013; Berthelot et al., 2015). In the Baltic Sea, A. flos aquae fixes 75 percent nitrogen (Klawonn et al., 2016).
Under aerobic and anaerobic conditions, they can fix nitrogen in structures called cyanobacterial mats besides the progression of molecular H2. Cyanobacteria especially blue-green algae are the key players in the regulation of nitrogen and carbon cycling. These also have a positive effect on the inhibition of different diseases of plants through the population of host-pathogen and herbicides (Smith and Crews, 2014). Heterocystous cyanobacteria (e.g., Nostoc, Anabaena, and Aulosira) develop associations with roots (Prasanna et al., 2013). The nitrogen fixation capability is restricted not only to heterocystous cyanobacteria, but there are many non-heterocystous cyanobacteria which are filamentous and unicellular (e.g., Aphanothece, Oscillatoria, Gloeothece, Gloeocapsa, and Plectonema) which also fix nitrogen significantly.
The Detail of Deep-Down Symbiotic BNF Dialogue
The overall symbiotic process is a highly specific and sophisticated exchange of signals (Figure 3A) between both the partners involved; “the macrosymbiont and the microsymbiont.” The first symbiotic signal comes from the host in the form of “Flavonoids” which induce the expression of different genes (nod, noe, nol, and others) in the rhizobia within the vicinity of the root. The nod genes encode the synthesis of Nod factors decorated with host-specific modifications which act as the second symbiotic signals. Nod factors are released in the rhizosphere where they serve as the first rhizobial determinant of host specificity (Spaink, 2002). The Nod factor triggers several responses such as ion changes, calcium oscillations, and gene expression. Rhizobia are attracted to host plants by chemotaxis and attach themselves to the root hair with the help of exo/lipopolysaccharides. This attachment leads to root hair deformation and curling which provides a site for infection thread (IT) initiation (Nanda et al., 2010). Curling of the root hairs occurs due to the localized presence of Nod factor molecules. Simultaneously, root cortex cells are stimulated to reinitiate mitosis, leading to the formation of nodule primordia and the formation of a functional nodule starts.
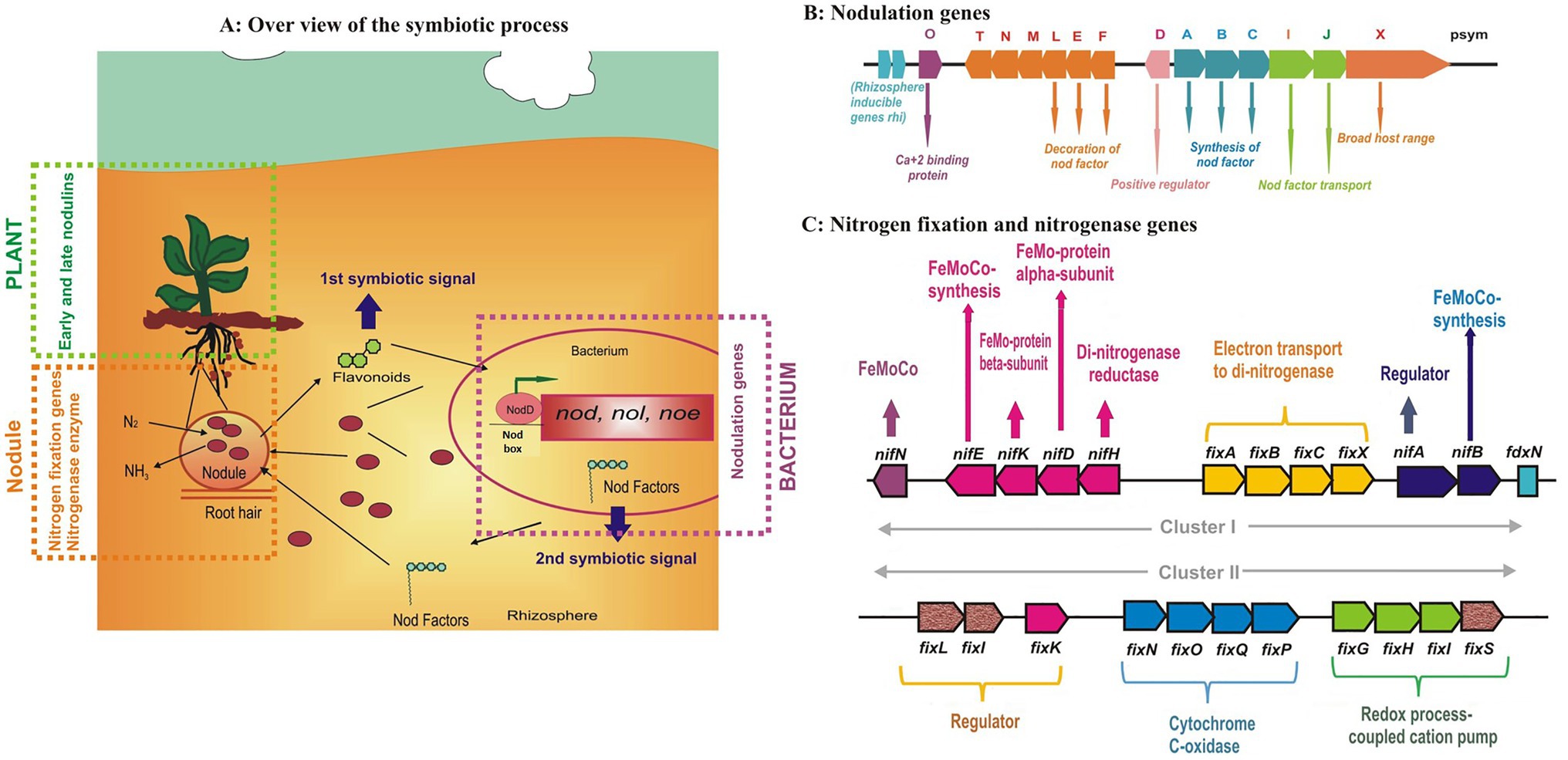
Figure 3. The overall process of Rhizobium-legume symbiosis showing the locks and keys of the symbiotic specificity of (A) nodulation genes (B) and details of nitrogen fixation genes (C) present in the rhizobia (figures drawn from the available literature from different sources).
Root hair curling results in the entrapping of attached bacteria within the deformation. Local lysis of the root hair cell wall is followed by invagination of the plant cell membrane. The process starts with the curling of root hairs, and a tube-like structure called an infection thread (IT) is formed through which the bacteria enter the plant. Infection threads are plant-derived structures originating from plasma membrane invagination accompanied by external deposition of the cell wall material. The IT grows inwardly toward the base of the root hair and the dividing cells in the nodule primordium. Intracellular infection is facilitated by the cell division in cortical cells. During IT development, rhizobial surface polysaccharides (lipopolysaccharides, exopolysaccharides, capsular polysaccharides, and cyclic glucans) interact with the host plant. Successful symbiosis depends on their correct composition, making them the second rhizobial determinant of host specificity (Perret et al., 2000). Bacteria reach the nodule primordium infecting several primordium cells and are released into the host cytoplasm by a process resembling endocytosis. During this release, the bacteria become surrounded by a plant membrane, and a symbiosome is formed. When the symbiosome divides, the infected cells become fully occupied with the microsymbiont. In actinorhizal symbioses and also in some legumes, bacteria are not released from the infection threads; rather, infected cells are filled with branching infection threads containing the microsymbionts, which is now called bacteroid. These bacteroids are surrounded by the plant membrane “peribacteriodal membrane” which forms a symbiotic interface with the host.
After infection, nodules are developed from the root cortical cells which are mitotically reactivated to form the nodule primordium (Mus et al., 2016) where thousands of bacteroids are present. Nodules are mainly of two types: “indeterminate” and “determinate.” The indeterminate nodules originate from cell divisions in the inner cortex and have a persistent meristem at their apex, and a clear “spatial developmental gradient” is formed from the distal meristem to the proximal root attachment site where these developmental zones can be characterized by the expression of specific plant genes. The determinate nodules originate from cell divisions in the outer cortex and do not have a persistent meristem, because the meristem of the nodule ceases to divide at an early stage of development and undergoes “temporal developmental stages” which are similar in the whole nodule. Legume nodules have a stem-like anatomy with peripheral vascular bundles and infected cells in the central tissue, while actinorhizal nodules have a root-like anatomy and develop from primordia formed in the pericycle.
The early stages of the symbiosis are controlled by highly specific chemical signals; the later stages by the expression of certain bacterial genes. During nodule morphogenesis, decreasing oxygen concentrations are maintained by the combined effects of specialized plant cells acting as an oxygen diffusion barrier and an abundant nodulin: the leghemoglobin. During this dramatic physiological switch, rhizobia initiate the expression of both nitrogen fixation genes and genes encoding a high-affinity terminal oxidase.
During nitrogen fixation, cyanobacteria usually differentiate into heterocyst which is the particular N2-fixing cells at consistent intervals of every 10–20 vegetative cells (Hilton et al., 2016). A nitrogen-fixing cyanobacterium (Cylindrospermopsis raciborskii) which lacks a patL ortholog at each end separates the trichome by almost a hundred vegetative cells and serves as a special site for the biosynthesis of nifH and activation of nitrogenase enzymes (Plominsky et al., 2013). A unicellular, photoheterotrophic bacteria (Candidatus Atelocyanobacterium thalassa) which lives with the genus Braarudosphaera (prymnesiophyte), the host usually attains fixed nitrogen from Candidatus Atelocyanobacterium thalassa. This heterotrophic nitrogen-fixing cyanobacterium has a Gamma A nifH phylotype, which is a dominant characteristic of a large aquatic proteobacterial nifH group.
Nitrogenase Enzyme Complex
Fixation is the process in which nitrogen from the atmosphere moves into the soil. The symbiotic and associative N2 bacteria reduce the dinitrogen to ammonia using the nitrogenase enzyme. There is sufficient evidence showing the horizontal transfer of genes among the different prokaryotic species, for example green sulfur bacteria, proteobacteria, methanobacteria, and cyanobacteria (Ivleva et al., 2016). The nitrogenase enzyme consists of two metalloproteins (Figures 3B,C), (i) molybdenum-iron (MoFe) and (ii) Fe protein, which are named according to their metal composition. The molybdenum-iron protein, encoded by nifD and nifK, is a heterotetramer (α2β2) where actual binding and reduction of N2 take place. The Fe protein is a homodimer (α2) that is encoded by the nifH gene and responsible for providing electrons to the MoFe protein. These genes are part of the same operon and frequently appear as nifHDK (Dixon and Kahn, 2004). Besides, several genes such as nifBEN genes play a role in the biosynthesis of the iron-molybdenum cofactor of dinitrogenase, nifA, which is a regulatory gene, and nifF and nifJ genes encoding electron transport proteins are also present in the core operons. Thus, nitrogen fixation is regulated by nif regulon which is a set of various operons comprising genes encoding nitrogenases, proteins involved in electron transfer, and regulatory genes (Shin et al., 2016).
In addition to the molybdenum-iron nitrogenase system, two alternative vanadium and iron-only nitrogenases have been identified in Azotobacter vinelandii and Rhodobacter capsulatus that contain vanadium and iron at their active sites (Mus et al., 2018). These enzymes comprise VnfHDK and AnfHDK subunits that are homologous to the NifHDK subunits of Mo nitrogenase but only expressed under Mo-depleting conditions. Moreover, these nitrogenases contain either vanadium-iron or iron-iron cofactors at their active sites as well as additional components like VnfG and AnfG subunits with unknown function (Dixon and Kahn, 2004).
The BNF requires a reductant (flavodoxin, ferredoxin, or sodium dithionite) which delivers electrons for reduction of N2. In principle, six electrons are required for the reduction of N2 to NH3, but this process is also coupled with the generation of 1 mol of H2 (Newton, 2007). Therefore, in most of the diazotrophs, eight high-potential electrons are provided by reduced ferredoxin. The transfer of electrons from reductase to nitrogenase is also coupled with ATP hydrolysis by reductase.
Nitrogen Assimilation and Metabolism
The prime assimilation of nitrogen through plants comprises of the utilization of a diverse kind of NO3− or NH4+ (inorganic N), based on the availability of nitrogen, adaptation, and species of plants. The accumulation and sufficient assimilation of the secondary ammonia group (NH4+) also take place in the plants toward re-assimilating the ammonium ion which usually produces endogenously for different processes such as biosynthesis of phenylpropanoid, photorespiration, and amino acid catabolism in the plants (Betti et al., 2014).
The ammonia produced in legume nodules crosses the bacteroid membranes via diffusion and is taken up by the plant cell as NH4+ and/or NH3. To ensure the loss of ammonia via recovery by a bacterium from a plant cell, the bacterial NH4+ transporter AmtB is repressed in bacteroids which transports NH4+ in the opposite direction (Mus et al., 2016). The ammonia is then protonated in the acidic environment of the symbiosome and crosses the membrane through an NH3 channel and/or cation channel for K, Na, and NH4+ transport and subsequently assimilated into organic form in the cytoplasm (Day et al., 2001). The ammonia is assimilated into amino acids glutamine and glutamate through the action of enzymes such as glutamine synthetase (GS) and glutamate synthase which are produced during nodule development. The enzymes interact with different membrane transporters for efficient assimilation of ammonia; for instance, the cytosolic GS involved in the assimilation of ammonia to glutamine physically interact with nodulin 26, which can transport NH3 (Masalkar et al., 2010). Glutamine and glutamate act as nitrogen donors for the biosynthesis of essential amino acids and serve to translocate organic nitrogen to various parts in legumes as well as non-legumes.
Initially, glutamine and glutamate are used for the biosynthesis of aspartate and asparagine. Aspartate is an important part of the malate-aspartate shuttle that is involved in the translocation of electrons from mitochondria and chloroplast into the cytoplasm. It is a precursor for two main pathways: (i) synthesis of asparagine and (ii) synthesis of aspartate-derived amino acids such as lysine, methionine, isoleucine, and threonine (Xu et al., 2012). Asparagine is an important nitrogen storage and transport compound in legumes and non-legume plants and synthesized through the transfer of the amide group of glutamine to aspartate via the action of asparagine synthetase (Lomelino et al., 2017). Asparagine is catabolized to form ammonia through the asparaginase enzyme, and the produced ammonia is subsequently re-assimilated through the glutamate synthase cycle. The essential amino acids of the aspartate family appear to be synthesized in the chloroplast; however, the methylation of homocysteine which converts it into methionine has been shown to take place in the cytoplasm. Different amino acids are used to produce different proteins which constitute about 80% of plant N. Moreover, the purines which are components of nucleic acids contain nitrogen and are derived from two amide-N of glutamine, one amino-N of aspartate, and one amino-N of glycine. Similarly, pyrimidines are derived from aspartate and carbamoyl phosphate. The N of carbamoyl phosphate comes from the amide-N of glutamine via a reaction by carbamoyl phosphate synthetase with 2 ATP.
Termination of the Symbiotic Dialogue (BNF)
Usually, the nodules have a limited lifespan and the symbiotic process is terminated gradually. Nodule senescence can occur at various developmental stages of the legume or may be triggered by some environmental stresses. It is commonly believed that after infection and differentiation, bacteroids of indeterminate nodules are terminally differentiated and are unable to return to a free-living state, while bacteroids of determinate nodules are thought to retain the capacity for free-living growth and can undergo a reverse differentiation process upon nodule senescence (Dixon and Kahn, 2004). It is, therefore, hypothesized that polyhydroxybutyrate (PHB) accumulation by bacteroids in the determinate nodules and by undifferentiated cells in the infection thread of indeterminate nodules may function to give the rhizobial cells a competitive advantage when released into the soil after nodule senescence.
Regulation and Plant Growth During BNF
BNF is an extremely complex biological process known to be very sensitive to environmental conditions. The development of advanced molecular biology and next-generation sequencing technologies has shown us details of basic machinery and regulatory networks during BNF. The basic mechanism and machinery are similar to those already described in rhizobia-legume symbiosis. Diazotrophs fix atmospheric nitrogen, which improves the nitrogen contents, and are found within the roots and in the phyllosphere of various C3 and C4 crops (Li et al., 2019). In addition to providing fixed-N, diazotrophs also improve the N uptake from the soil which may be due to a general increase in the root surface area (Islam et al., 2013) as a positive correlation has been demonstrated between N uptake and root architecture. Evidence shows that diazotrophic bacteria exhibit several other traits that are considered important for plant development and yield. Production of phytohormone, siderophores, HCN, and antibiotics; solubilization of phosphorus, zinc, and potassium; calcite degradation; and 1-aminocyclopropane-1-carboxylic acid (ACC) deaminase activity are some of the growth-promoting mechanisms exhibited by associative and symbiotic diazotrophs (Hakim et al., 2020).
Plant growth is a complex phenomenon. Plants themselves continuously produce and secrete compounds, including organic/amino acids, sugars, phenolics, and other secondary metabolites into their surroundings for the selective assemblage of those microbes which help out the plants through multiple ways on practical grounds (Ali et al., 2020). On the other side, there is always a competition between the soil microbes for the successful colonization of the roots, and in most cases, microbes exhibiting high affinity for the roots and having multiple traits are favored by the plants. The PGP activities of diazotrophs have a well-documented effect on overall plant growth and yield in natural environments as well as experimental settings (Dobbelaere et al., 2003). Studies also demonstrate their role in stress alleviation under drought, salt and heavy metal stress (Valentine et al., 2018), restriction of the phytopathogens (Rosenblueth and Martínez-Romero, 2006), and increased bioaccumulation of metals from the soils to the biomass of plants (Ullah et al., 2015). Moreover, cold-adapted diazotrophs have also shown their potential to alleviate cold stress (Suyal et al., 2014, 2019; Kumar et al., 2020). Compared to synthetic fertilizers (N) which provide a single source of nutrients, diazotrophs serve as a multipurpose fertilizer and plant growth stimulator. Important plant-beneficial traits of diazotrophs important for BNF are discussed in detail in the following section.
Role of Phytohormones
Phytohormone production by symbiotic and associative diazotrophs is the major mechanism promoting the growth of the host plant. Many plant hormones/growth regulators (auxins, gibberellin, cytokinin) are produced by these bacteria, and this ability is widespread (>80% inhabiting the rhizosphere) in the microbes. These hormones are important regulators of plant development, regulating different processes involved in root proliferation, early plant growth, root and shoot growth, plant elongation, etc. The secondary roots and root hairs are the preferred colonization sites for these diazotrophs. Rhizodeposition also takes place on the junctions of primary roots and rootlets for better and stable attachment to initiate nitrogen fixation and other cellular processes (Naqqash et al., 2020). Therefore, microbes act on the initiation of secondary roots and root hairs, cell division, and elongation in the roots. The bacterial association also regulates the plant’s inherent ability of hormone production, thus improving plant health (Carvalho et al., 2014). Microbial association and phytohormone production improve root growth and the root surface area, which facilitate the plant to harbor maximum sources of soil nutrients. A few examples of diazotrophs that produce phytohormones and their role in plant growth and development are described in Table 2.
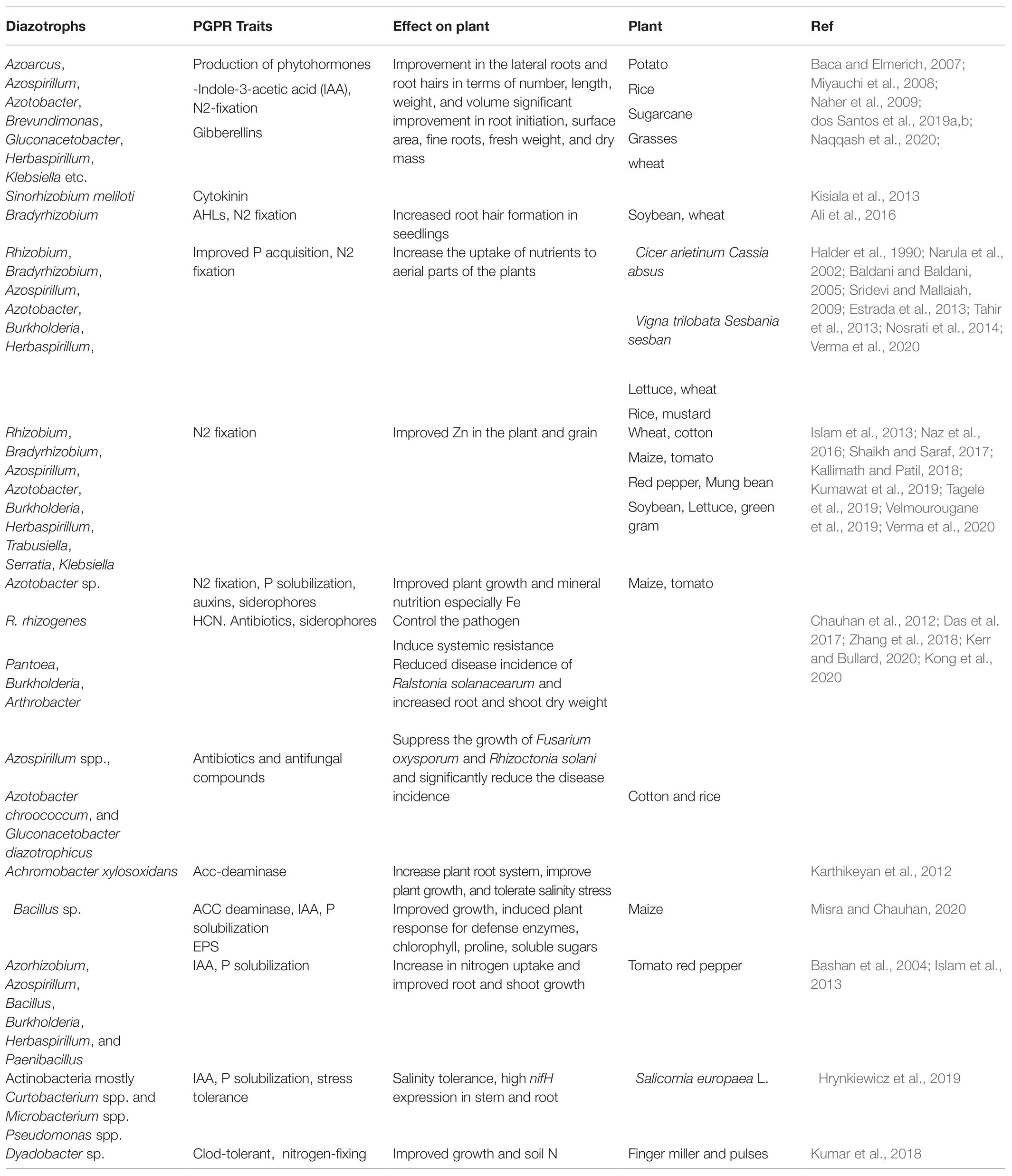
Table 2. List of few diazotrophs with multi-trait characters important for the regulation of BNF and plant growth during BNF.
Role of Soil Nutrients
The BNF is a complex exchange of nutrients between the partners. Both organisms change their metabolic routine to fine-tune and accommodate the BNF which is monitored and regulated by both partners. Apart from C and N, several other compounds are made available to symbiotic microbes especially in the case of symbiotic (endophytic) diazotrophs which rely on the host for their functioning. Nutrient application (e.g., P) improves the overall soil microbial N and soil N fixation rates (Reed et al., 2007) because P is essential for the metabolism of both the host and the microsymbiont. Nodules are strong sinks for P, and limited P decreases the BNF (Yelenik et al., 2013). The studies also support the fact that high plant P contents are required for the development of symbiotic BNF in legumes (Divito and Sadras, 2014). Iron is an essential component of nitrogenases as well as leghemoglobin and is usually transferred across the symbiotic membrane. Likewise, sulfur is also an essential component of the nitrogenase enzyme and transported through the symbiotic membrane (Krusell et al., 2005). Besides, diazotrophs required cobalt, molybdenum, and vanadium (Bellenger et al., 2008) and deficiency of any of these elements result in the development of nonfunctional nodules.
Multi-trait associative or symbiotic diazotrophs (enlisted in Table 2) solubilize the unavailable compounds of different nutrients (e.g., P, Zn, Ca, Fe, etc.) and convert them into plant-available forms, thus increasing their uptake to aerial parts of the plants (Dobbelaere et al., 2003) and having an overall positive impact on plant growth and BNF (Piceno and Lovell, 2000). Moreover, the stimulation of BNF in legumes has been reported by the addition of P-solubilizing arbuscular mycorrhiza (Pueschel et al., 2017).
Oxygen Regulation
The BNF enzyme “nitrogenase” is extremely sensitive to oxygen and rapidly inactivated in the presence of oxygen. Legumes, as well as non-legumes like Parasponia and actinorhizal plants, maintain microaerobic conditions through various mechanisms using various physiological strategies: (i) anaerobic growth to avoid high oxygen level, (ii) increased rate of respiration involving a specialized cytochrome, (iii) conformational protection under oxygen stress conditions which involve the association of a FeSII protein with nitrogenase thus temporarily inactivating the enzyme (Maier and Moshiri, 2000), and (iv) oxygen diffusion barriers through the production of an alginate capsule (Sabra et al., 2000). The microaerobic conditions trigger a signaling cascade in rhizobia which involves the activation of FixL which is an oxygen sensor protein. FixL induces the expression of transcriptional activator FixJ, which in turn activates nifA and fixK, subsequently inducing the expression of various proteins involved in BNF. Furthermore, the rhizobia express a heme-copper cbb3-type oxidase with high oxygen affinity. The nitrogenase enzyme of Streptomyces thermoautotrophicus is unaffected by the presence of oxygen. The enzyme contains manganese superoxide dismutase as nitrogenase reductase which shows no homology with nifH (Ribbe et al., 1997). Moreover, this Mo nitrogenase requires significantly lower energy as compared to standard nitrogenases.
In nitrogen-fixing cyanobacteria, different ecological, biochemical, and morphological adaptations are established to reduce the oxygen-associated complications. The paradox of oxygenic photosynthesis diazotrophs is resolved by the segregation of oxygen-sensitive machinery for the fixation of nitrogen in heterocysts. Oxygenic photosynthesis is separated from nitrogen fixation spatially or temporally in non-heterocystous cyanobacteria. In temporal separation, BNF happens during the shadowy period and photosynthesis during the light period, while in the spatial separation, the dominant non-photosynthetic cells develop and are involved in BNF and the upper green cells become photosynthetic (Issa et al., 2014).
BNF Under Stress
Several of the environmental conditions limit the growth and activity of the N2-fixing plant as well as the diazotrophs. BNF is mostly suppressed under different biotic and abiotic factors, e.g., pathogen, salt, drought, acidity, alkalinity, heavy metals, fertilizers, etc. (Zahran, 1999). These stress factors suppress the growth and BNF potential of most of the diazotrophs. Salt and drought stress inhibit the initial steps of BNF, i.e., root hair curling and infection thread development. A high stress level reduces the nodule number and size, or complete inhibition of nodulation, and nitrogen fixation is reduced due to a reduction in nodule respiration. Similarly, high temperature (above 35–40°C) affects root hair infection, bacteroid differentiation, nodule structure, and functioning (reviewed by Zahran 1999). Diazotrophs are highly sensitive to fertilizer-managing practices which reduce the diversity, abundance, and the structure of diazotroph community (Reardon et al., 2014; Wang et al., 2017; Xiao et al., 2020). T-RFLP profiling of N2-fixing bacterial communities in the rhizosphere was found decreased at higher N-fertilization rates, showing a negative influence of fertilizer on the abundance of diazotrophs (Meng et al., 2012). Metatranscriptomic analysis revealed a diverse set of diazotrophs associated with switchgrass roots with enrichment of a subset of diazotrophs in roots of N-deficient plants that exhibited the highest nitrogen fixation rates. Higher levels of N fertilization resulted in lower levels of associative nitrogen fixation (ANF) in the roots where at the maximum level (180 kg N/Ha) it essentially abolished the ANF (Bahulikar et al., 2020).
Many of the diazotrophs have ecologically evolved to manage these stresses by different mechanisms. Some of the diazotrophs are enlisted in Table 2. For adaptation under salinity, two distinct classes of osmoprotectants exist in diazotrophs: one such as proline, glycine betaine, and glutamate, and another which acts as a chemical mediator such as ectoine (Zahran, 1999). Drought-tolerant and heat-tolerant diazotrophs produce different enzymes and proteins and produce 1-aminocyclopropane-1-carboxylate (ACC) deaminase, which lowers the concentration of ethylene in plant roots by catabolizing ACC, a precursor of ethylene. Consequently, the plant can cope with a variety of ethylene-inducing stresses and proliferate its root system. Diazotrophs can inhibit the growth of a diverse range of plant pathogens causing various diseases by producing antibiotics, hydrogen cyanide (HCN), and siderophores or inducing systemic resistance in the plants (Das et al., 2017; Ali et al., 2020). So, the development of diazotrophic inoculants with dual attributes of nitrogen fixation and antagonism against phytopathogens can contribute to increased plant growth and productivity.
Other diazotrophs, e.g., Cyanobacteria, are usually recognized from an extended evolutionary past due to their distinctive traits such as high yield of biomass, production of beneficial biofuels and by-products, and oxygenic photosynthesis, improving the fertility of the soil and saline soil reclamation.
Cropping System Modifications to Upscale BNF for an Ecosystem Benefit
The review and potentials, opportunities, and limiting factors of BNF suggest that many priority issues be addressed for researchers and policymakers. The involvement of diazotrophic microorganisms in the BNF process is one of the important agricultural practices to improve crop yield as well as environmental quality. Diazotrophs and host plants positively interact with each other, which help in reducing the demand of N fertilizers (Ai’shah et al., 2009). The total annual terrestrial inputs of N from BNF have been estimated from 139 to 175 million tons of N where symbiotic associations account for 25–30% (35–44 million tons of N) and permanent pasture accounts for the other 30% (45 million tons of N; Paul, 2000). Although the accuracy of the estimates of N inputs through BNF may be open to question, its relative importance in cropping and pasture system can be improved to substitute a part of the million tons of N fertilizer applied to the agriculture system annually (dos Santos et al., 2017).
Bioinoculants
BNF is no doubt an environment-friendly approach for the improvement of soil fertility and the production of crops (Bashan et al., 2004). Maximum benefits from BNF can be obtained by diazotrophic inoculation to different cropping systems. Several reports illustrate (few enlisted in Table 3) that the application of diazotrophs completely or partially eliminates the need for chemical N fertilizers. Inoculation of different rhizobia on respective hosts increases plant growth comparable to N fertilizer treatment (Jensen et al., 2020). An experiment with a combined application of N-15-labeled nitrogen and diazotrophs on pine reveals that 30% less nitrogen fertilizer is required when diazotrophic bacteria were applied (Bal and Chanway, 2012). In some cases, inoculated plants are even better as the bacteria contain multiple properties that stimulate a plethora of plant growth parameters rather than the sole N nutrition. These results and many more signify the fact that the diazotrophs partially fulfill the N needs of the plants and their inoculation at reduced N fertilizer gives similar growth and yield responses as those grown with full N fertilizers. This natural and eco-friendly crop fertilization will reduce the input of synthetic fertilizers to agriculture and reduce overall GHG emissions in the atmosphere.
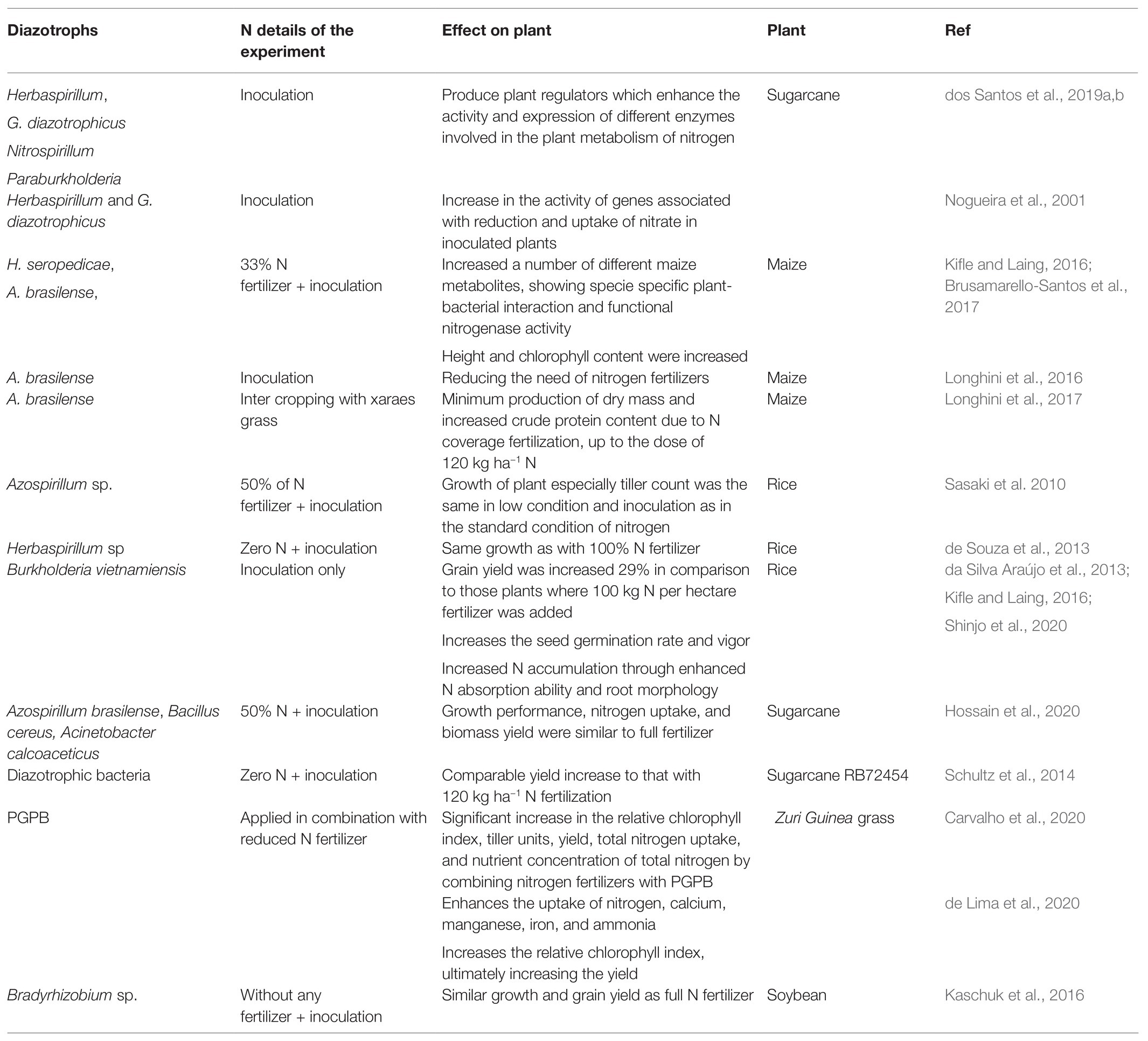
Table 3. List of few diazotrophs’ inoculation under field conditions for BNF under reduced application of N fertilizer.
The main thing to consider is to have a clear understanding of the factors affecting BNF under field conditions for improving inoculum quality and ultimately strengthening the capacities of farmers. The need for improvising the BNF, particularly by fertilizers or in the symbiosis of N-fixing microbes, must be addressed internationally. The best tool for reducing the yield gap is the integration of BNF to plant-breeding programs and to develop better formulation technologies for bioinoculants. Furthermore, a significant percentage of the bioinoculants should be subsidized for small farmers like the chemical fertilizers.
Ecological Intensification and Crop Diversification
With ecological intensification (EI), it is possible to increase food production by redesigning agricultural lands in such a way that both biodiversity and ecological processes are not hampered. EI increases crop production per area by exploiting ecological processes that assist beneficial organisms by diversifying croplands and enhances profitability by minimizing the need for costly processes. The agricultural matrix can be made both more permeable and habitable to biodiversity by planting a variety of crops into landscapes which will help in conserving biodiversity gradually. EI practices facilitate the habitation and resources for colonizing beneficial organisms (e.g., microorganisms or soil invertebrates) that promote crop growth. Beneficial soil microorganisms cycle and/or supply nutrients to plants, while the invertebrates decompose organic matter and aerate soils and prey on crop pests (Kremen, 2020). Analysis of the effect of management (ecological intensive vs. conventional intensive) and comparison of the diazotrophic communities in both systems show that ecological intensive management promoted a beneficial N-related microbial community composition involved in N-cycling processes (Lori et al., 2020).
Crop diversification (CD) has proved to be beneficial to improve crop production as well as to reduce market and production risks which are caused by tremendous price fluctuations and uncertain water availability, respectively. These practices have reduced the risk of pests, drought, and diseases, hence increasing the overall productivity of the system without compromising the yield (Manjunatha et al., 2013). Many models and meta-analyses have reported that crop diversification is positively correlated with increased profitability and productivity (Letourneau et al., 2011; Davis et al., 2012; Avasthe et al., 2020). The CD practices include cultivation of diverse crops (Renard and Tilman, 2019), mixed cropping (Bedoussac et al., 2015), rotation of diverse crops (Reckling et al., 2016), and cultivation of region-adapted crop varieties (Yang et al., 2019). Crop rotations and cropping sequence diversity are essential factors influencing bacterial assemblages and species diversity because different crops produce different root exudates which attract different bacterial population. Soil fertility management, crop protection, and crop rotation have all been shown to exert significant effects on the soil microbial communities present in the agricultural soils. The effect of fertilization rate and crop rotation analysis on cyanobacterial diazotrophs community structure showed that rice-mustard-moong (RMM) crop rotation is more suitable for cyanobacterial nitrogen fixation than rice-wheat-maize rotation. Furthermore, nitrogenase activity was found higher in the cropped plots than fallow plots. The low fertility coupled with RMM rotation was found to be best suited for promoting nitrogen fixation by cyanobacteria to supply the rice plants (Jha et al., 2001).
Legume Integration
Legumes are regarded as agricultural and ecological wonders because they utilize the normal sources of nitrogen available in the air through BNF and improve soil fertility. The BNF ability of different legumes is variable; e.g., Leucaena 325, Lucerne 250, pigeonpea 220, cowpea 210, mung bean 200, soybean 110, groundnut 100, chickpea 102, and common bean 50 kg N Ha−1 (Serraj, 2004). The introduction of legumes into the pastures has been seen as the best strategy to improve nitrogen nutrition of grasses (between 75 and 97 kg N ha−1 in 97 days growth of Stylosanthes guianensis; Viera-Vargas et al., 1995). It was observed that the denitrification rate was lower in the unfertilized non-legume-cultivated areas in contrast to the legume-cultivated areas. This is an indication that by growing legumes and mineralization of the lower carbon-nitrogen ratio of its residues, the availability of soil nitrogen will increase (Zilli et al., 2020).
The addition of any legume crops in the current cropping system will have lasting benefits along with the enhanced crop productivity and profitability (Choudhury and Kennedy, 2004). The legumes in the cropping system will improve the abundance of diazotrophs (Joshi et al., 2007; Zou et al., 2020). Similarly, the addition of Pisum sativum in the agriculture system enhanced the abundance of free-living diazotrophs in Eastern Oregon, USA (Reardon et al., 2014). Rahman and Chima (2016) established a diagnostic model for crop diversification and revealed a significant correlation between diversifying a system into multiple crops and profit generation. So, crop diversity magnanimously contributes to overall sustainable agriculture.
Technological Development
Available information indicates that the current application of BNF at a large scale is generally constrained by the poor effectiveness and competitiveness of the technology compared to other alternatives for soil fertility management. This requires a concerted effort for improving the efficiency and stability of the technology, especially in a stressful environment. Different organic fertilizers, e.g., livestock manure and crop residues, contribute to the reestablishment of the diazotroph population (Liao et al., 2018). These properties of fertilization on rhizospheric diazotroph may be direct, due to the increased availability of several nutrients, for example phosphorus, carbon-nitrogen ratio, availability of nitrogen, and soil organic carbon, and indirect by different soil features, such as pH of the soil (Wang et al., 2016, 2017). Furthermore, the diazotrophs in the environment are prone to different harsh conditions of weather which may affect their stability and population. Investment in the BNF will ensure economic profitability, energy efficiency, environmental quality, and agricultural sustainability.
Nanotechnology holds great promise for upgrading the conventional fertilizer industry. Many nano-formulations of chemical fertilizers are being tested in the agriculture system that have low leaching losses, are not easily volatilized, and do not cause any deterioration to land quality. Nano-based products, e.g., nano-sensors, nano-pesticides, nano-fertilizers, nano-films, nano-magnets, and nano-filters, are available in the market which will change the agricultural production if applied on larger scale. Nanotechnology has materialized the concept of bacterial encapsulation where single and composite polymers are used as carriers of beneficial plant microorganisms to increase the performance and consistency of bioinoculants and minimize costs and the effect on the environment (Pacheco-Aguirre et al., 2017). Due to their smaller size, nanoparticles provide a large surface area for the diazotrophs to grow and enable plants to uptake nutrients slowly and sustainably as needed by plants. The coating shields diazotrophs from unfavorable environments and control a gradual release in the soil.
A new frontier would be to combine the benefits of nano-formulations of chemical fertilizers with the diazotrophs to formulate hybrid fertilizers. These fertilizers may be coated with biodegradable polymers that degrade slowly in the rhizosphere. It will function as a single multipurpose fertilizer that serves the purpose of biological nitrogen fixation and as a slow-releasing chemical fertilizer. It will minimize the chemical fertilizer input into the agriculture system, thus saving billions of dollars to the economy and the ecosystem. On the other hand, it will boost the fertilizer industry to a new hybrid fertilizer industry that will have more acceptability at the farmer level and national level and in the scientific community because it will be safer for future generations and the environment. This approach has been explained well in the Graphical Abstract.
Summary and Implications
Nitrogen is the major element required by the crops and is critical for growth yield. It has played a phenomenal role in the success of the Green Revolution but at the cost of adverse impacts on the quality of the overall environment. Chemical fertilizers are not available to everyone everywhere, and they cannot completely maintain the equilibrium of the ecosystem. The potential economic and environmental benefits of BNF favor investing in the diazotroph technology. Although BNF is already making a large contribution in total N-fixed globally, in agriculture, its use and benefits can be maximized by adding legumes in the cropping systems. Legumes have a large scope (species >3,000) with open prospects for developing ecologically sustainable and economically viable agricultural cropping systems. Field data of diazotroph inoculation to legumes and non-legumes presented in this review show that BNF can substitute 30–50% of the fertilizer-N demand of different crops. This not only saves the farmer input (by saving fertilizer) but also improves overall returns (by decreasing pollution and increased sustainability). Despite a lot of research and investment in BNF, problems like old-fashioned technology, inadequate availability, nonuniformity of product and results, and climate issues render the wide-scale adaptation by the farmers. To harness the maximum potential of BNF, technology should be improved and refined using the advancements made in the 21st century. Developing nano-hybrid formulations will improve not only efficacy, application, stability, and shelf life but also product uniformity. It will revolutionize the whole biofertilizer industry. Governments should encourage the private sector to come forward and develop a nano-hybrid inoculant industry. Farmer participation should be increased in technology development and evaluation. A holistic approach and better consideration of the market, agricultural policies, legislation, and investment of private sector will support the BNF to maximize the benefits for the ecosystem and beyond.
Author Contributions
AI conceived the review, formulated the layout, and edited the final version. SH collected and reviewed the literature regarding nitrogenase enzyme. MT collected and reviewed the literature regarding legumes. MN collected and reviewed the literature regarding symbiotic specificity. IL collected and reviewed the literature regarding nitrogen usage in agriculture. UG collected and reviewed the literature regarding rhizobium-legume symbiosis. MKH collected and reviewed the literature regarding symbiotic diazotrophs. MS collected and reviewed the literature regarding multiple diazotrophic properties. MH collected and reviewed the literature regarding health concerns of excessive N-application. MA and AF formulated the tables, made the figures and formatted the reference list. All authors contributed to the article and approved the submitted version.
Conflict of Interest
The authors declare that the research was conducted in the absence of any commercial or financial relationships that could be construed as a potential conflict of interest.
References
Ai’shah, O. N., Amir, H., Keng, C. L., and Othman, A. (2009). Influence of various combinations of diazotrophs and chemical fertilizer on plant growth and N2 fixation capacity of oil palm seedlings (Elaeis guineensis jacq.). Thai J. Agric. Sci. 42, 139–149.
Ali, J. G., Casteel, C., Mauck, K., and Trase, O. (2020). Chemical ecology of multitrophic microbial interactions: plants, insects, microbes and the metabolites that connect them. J. Chem. Ecol. 46, 645–648. doi: 10.1007/s10886-020-01209-y
Ali, A., Hameed, S., Imran, A., Iqbal, M., Iqbal, J., and Oresnik, I. J. (2016). Functional characterization of a soybean growth stimulator Bradyrhizobium sp. strain SR-6 showing acylhomoserine lactone production. FEMS Microbiol. Ecol. 92:fiw115. doi: 10.1093/femsec/fiw115
Angus, J., and Grace, P. (2017). Nitrogen balance in Australia and nitrogen use efficiency on Australian farms. Soil Res. 55, 435–450. doi: 10.1071/SR16325
Avasthe, R., Babu, S., Singh, R., Yadav, G., and Kumar, A. (2020). Productivity and profitability assessment of organically grown vegetables embedded in rice based cropping sequences in Sikkim himalayas, north East India. J. Environ. Biol. 41, 111–117. doi: 10.22438/jeb/41/1/MRN-1146
Baca, B., and Elmerich, C. (2007). “Microbial production of plant hormones,” in Associative and Endophytic Nitrogen-Fixing Bacteria and Cyanobacterial Associations. ed. W. E. N. Claudine Elmerich (Netherlands: Springer), 113–143.
Bahulikar, R. A., Chaluvadi, S. R., Torres-Jerez, I., Mosali, J., Bennetzen, J. L., and Udvardi, M. (2020). Nitrogen fertilization reduces nitrogen fixation activity of diverse diazotrophs in switchgrass roots. Phytobiome J. 9–19. doi: 10.1094/PBIOMES-09-19-0050-FI
Bal, A., and Chanway, C. P. (2012). Evidence of nitrogen fixation in lodgepole pine inoculated with diazotrophic Paenibacillus polymyxa. J. Bot. 90, 891–896. doi: 10.1139/b2012-044
Baldani, J. I., and Baldani, V. L. (2005). History on the biological nitrogen fixation research in graminaceous plants: special emphasis on the brazilian experience. Acad. Bras. Cienc. 77, 549–579. doi: 10.1590/S0001-37652005000300014
Bashan, Y., Holguin, G., and De-Bashan, L. E. (2004). Azospirillum-plant relationships: physiological, molecular, agricultural, and environmental advances (1997-2003). Can. J. Microbiol. 50, 521–577. doi: 10.1139/w04-035
Bedoussac, L., Journet, E.-P., Hauggaard-Nielsen, H., Naudin, C., Corre-Hellou, G., Jensen, E. S., et al. (2015). Ecological principles underlying the increase of productivity achieved by cereal-grain legume intercrops in organic farming. A review. Agron. Sustain. Dev. 35, 911–935. doi: 10.1007/s13593-014-0277-7
Bellenger, J. P., Wichard, T., Kustka, A. B., and Kraepiel, A. M. L. (2008). Uptake of molybdenum and vanadium by a nitrogen-fixing soil bacterium using siderophores. Nat. Geosci. 1, 243–246. doi: 10.1038/ngeo161
Benavides, M., Agawin, N. S., Arístegui, J., Peene, J., and Stal, L. J. (2013). Dissolved organic nitrogen and carbon release by a marine unicellular diazotrophic cyanobacterium. Aquat. Microb. Ecol. 69, 69–80. doi: 10.3354/ame01621
Berthelot, H., Bonnet, S., Camps, M., Grosso, O., and Moutin, T. (2015). Assessment of the dinitrogen released as ammonium and dissolved organic nitrogen by unicellular and filamentous marine diazotrophic cyanobacteria grown in culture. Front. Mar. Sci. 2:80. doi: 10.3389/fmars.2015.00080
Betti, M., García-Calderón, M., Pérez-Delgado, C. M., Credali, A., Pal'ove-Balang, P., Estivill, G., et al. (2014). Reassimilation of ammonium in Lotus japonicus. J. Exp. Bot. 65, 5557–5566. doi: 10.1093/jxb/eru260
Brusamarello-Santos, L. C., Gilard, F., Brulé, L., Quilleré, I., Gourion, B., Ratet, P., et al. (2017). Metabolic profiling of two maize (Zea mays L.) inbred lines inoculated with the nitrogen fixing plant-interacting bacteria Herbaspirillum seropedicae and Azospirillum brasilense. PLoS One 12:e0174576. doi: 10.1371/journal.pone.0174576
Brusamarello-Santos, L., Pacheco, F., Aljanabi, S., Monteiro, R., Cruz, L., Baura, V., et al. (2012). Differential gene expression of rice roots inoculated with the diazotroph Herbaspirillum seropedicae. Plant Soil 356, 113–125. doi: 10.1007/s11104-011-1044-z
Carvalho, T., Balsemão-Pires, E., Saraiva, R., Ferreira, P., and Hemerly, A. (2014). Nitrogen signalling in plant interactions with associative and endophytic diazotrophic bacteria. J. Exp. Bot. 65, 5631–5642. doi: 10.1093/jxb/eru319
Carvalho, J. M., Barreto, R. F., Prado, R. D. M., Habermann, E., Martinez, C. A., and Branco, R. B. F. (2020). Elevated [CO2] and warming increase the macronutrient use efficiency and biomass of Stylosanthes capitata vogel under field conditions. J. Agron. Crop Sci. 206, 597–606. doi: 10.1111/jac.12398
Chauhan, S., Wadhwa, K., Vasudeva, M., and Narula, N. (2012). Potential of Azotobacter spp. as biocontrol agents against Rhizoctonia solani and Fusarium oxysporum in cotton (Gossypium hirsutum), guar (Cyamopsis tetragonoloba) and tomato (Lycopersicum esculentum). Arch. Agron. Soil Sci. 58, 1365–1385. doi: 10.1080/03650340.2011.590134
Choudhury, A., and Kennedy, I. (2004). Prospects and potentials for systems of biological nitrogen fixation in sustainable rice production. Biol. Fertil. Soils 39, 219–227. doi: 10.1007/s00374-003-0706-2
da Silva Araújo, A. E., Baldani, V. L. D., de Souza Galisa, P., Pereira, J. A., and Baldani, J. I. (2013). Response of traditional upland rice varieties to inoculation with selected diazotrophic bacteria isolated from rice cropped at the northeast region of Brazil. Appl. Soil Ecol. 64, 49–55. doi: 10.1016/j.apsoil.2012.10.004
Das, K., Prasanna, R., and Saxena, A. K. (2017). Rhizobia: a potential biocontrol agent for soilborne fungal pathogens. Folia Microbiol. 62, 425–435. doi: 10.1007/s12223-017-0513-z
Davis, A. S., Hill, J. D., Chase, C. A., Johanns, A. M., and Liebman, M. (2012). Increasing cropping system diversity balances productivity, profitability and environmental health. PLoS One 7:e47149. doi: 10.1371/journal.pone.0047149
Day, D. A., Kaiser, B. N., Thomson, R., Udvardi, M. K., Moreau, S., and Puppo, A. (2001). Nutrient transport across symbiotic membranes from legume nodules. Funct. Plant Biol. 28, 669–676. doi: 10.1071/PP01028
De Lajudie, P. M., Andrews, M., Ardley, J., Eardly, B., Jumas-Bilak, E., Kuzmanović, N., et al. (2019). Minimal standards for the description of new genera and species of rhizobia and agrobacteria. Int. J. Syst. Evol. Microbiol. 69, 1852–1863. doi: 10.1099/ijsem.0.003426
de Lima, G. C., Hungria, M., Nogueira, M. A., Teixeira Filho, M. C. M., Moreira, A., Heinrichs, R., et al. (2020). Yield, Yield Components and Nutrients Uptake in Zuri guinea Grass Inoculated With Plant Growth-Promoting bacteria. Int. J. Innov. Educ. Res. 8, 103–124. doi: 10.31686/ijier.vol8.iss4.2268
de Souza, R., Beneduzi, A., Ambrosini, A., Da Costa, P. B., Meyer, J., Vargas, L. K., et al. (2013). The effect of plant growth-promoting rhizobacteria on the growth of rice (Oryza sativa L.) cropped in southern brazilian fields. Plant Soil 366, 585–603. doi: 10.1007/s11104-012-1430-1
Divito, G. A., and Sadras, V. O. (2014). How do phosphorus, potassium and sulphur affect plant growth and biological nitrogen fixation in crop and pasture legumes? A meta-analysis. Field Crop Res. 156, 161–171. doi: 10.1016/j.fcr.2013.11.004
Dixon, R., and Kahn, D. (2004). Genetic regulation of biological nitrogen fixation. Nat. Rev. Microbiol. 2, 621–631. doi: 10.1038/nrmicro954
Dobbelaere, S., Vanderleyden, J., and Okon, Y. (2003). Plant growth-promoting effects of diazotrophs in the rhizosphere. CRC. Crit. Rev. Plant Sci. 22, 107–149. doi: 10.1080/713610853
Dong, T., Li, J., Zhang, Y., Korpelainen, H., Niinemets, Ü., and Li, C. (2015). Partial shading of lateral branches affects growth, and foliage nitrogen-and water-use efficiencies in the conifer Cunninghamia lanceolata growing in a warm monsoon climate. Tree Physiol. 35, 632–643. doi: 10.1093/treephys/tpv036
dos Santos, S. G., Chaves, V. A., da Silva Ribeiro, F., Alves, G. C., and Reis, V. M. (2019a). Rooting and growth of pre-germinated sugarcane seedlings inoculated with diazotrophic bacteria. Appl. Soil Ecol. 133, 12–23. doi: 10.1016/j.apsoil.2018.08.015
dos Santos, S. G., da Silva Ribeiro, F., Alves, G. C., Santos, L. A., and Reis, V. M. (2019b). Inoculation with five diazotrophs alters nitrogen metabolism during the initial growth of sugarcane varieties with contrasting responses to added nitrogen. Plant Soil 451, 25–24. doi: 10.1007/s11104-019-04101-1
dos Santos, S. G., da Silva Ribeiro, F., Da Fonseca, C. S., Pereira, W., Santos, L. A., and Reis, V. M. (2017). Development and nitrate reductase activity of sugarcane inoculated with five diazotrophic strains. Arch. Microbiol. 199, 863–873. doi: 10.1007/s00203-017-1357-2
Elhanafi, L., Houhou, M., Rais, C., Mansouri, I., Elghadraoui, L., and Greche, H. (2019). Impact of excessive nitrogen fertilization on the biochemical quality, phenolic compounds, and antioxidant power of Sesamum indicum L. seeds. J. Food Qual. 2019, 1–6. doi: 10.1155/2019/9428092
Erisman, J. W., Galloway, J. N., Seitzinger, S., Bleeker, A., Dise, N. B., Petrescu, A. R., et al. (2013). Consequences of human modification of the global nitrogen cycle. Philos. Trans. R. Soc. 368:20130116. doi: 10.1098/rstb.2013.0116
Estrada, G. A., Baldani, V. L. D., de Oliveira, D. M., Urquiaga, S., and Baldani, J. I. (2013). Selection of phosphate-solubilizing diazotrophic Herbaspirillum and Burkholderia strains and their effect on rice crop yield and nutrient uptake. Plant Soil 369, 115–129. doi: 10.1007/s11104-012-1550-7
Fao (2017). World Fertiliser Trends and Outlook to 2020. Rome: Food and Agricultural Organisation of the United Nations, 1–38.
Franche, C., Lindström, K., and Elmerich, C. (2009). Nitrogen-fixing bacteria associated with leguminous and non-leguminous plants. Plant Soil 321, 35–59. doi: 10.1007/s11104-008-9833-8
Good, A. G., and Beatty, P. H. (2011). Fertilizing nature: a tragedy of excess in the commons. PLoS Biol. 9:e1001124. doi: 10.1371/journal.pbio.1001124
Hakim, S., Mirza, B. S., Imran, A., Zaheer, A., Yasmin, S., Mubeen, F., et al. (2020). Illumina sequencing of 16S rRNS tag shows disparity in rhizobial and non-rhizobial diversity associated with root nodules of mung bean (Vigna radiata L.) growing in different habitats in Pakistan. Microbiol. Res. 231:126356. doi: 10.1016/j.micres.2019.126356
Halder, A., Mishra, A., Bhattacharyya, P., and Chakrabartty, P. (1990). Solubilization of rock phosphate by Rhizobium and Bradyrhizobium. J. Gen. Appl. Microbiol. 36, 81–92. doi: 10.2323/jgam.36.81
Hellriegel, H., and Wilfarth, H. (1888). Untersuchungen uber die Stickstoffnahrung der Gramineen und Leguminosen, Beilageheft zu der Ztschr. Ver. Rubenzucker-Industrie Deutschen Reich. 234.
Hilton, J. A., Meeks, J. C., and Zehr, J. P. (2016). Surveying DNA elements within functional genes of heterocyst-forming cyanobacteria. PLoS One 11:e0156034. doi: 10.1371/journal.pone.0156034
Hossain, G. M. A., Solaiman, A. R. M., Karim, A. J. M. S., Rahman, G. K. M. M., and Mia, M. A. B. (2020). Influence of diazotrophic bacteria on growth and biomass production of sugarcane in vitro. Int. J. Curr. Microbiol. App. Sci. 9, 3077–3088. doi: 10.20546/ijcmas.2020.903.353
Hrynkiewicz, K., Patz, S., and Ruppel, S. (2019). Salicornia europaea L. as an underutilized saline-tolerant plant inhabited by endophytic diazotrophs. J. Adv. Res. 19, 49–56. doi: 10.1016/j.jare.2019.05.002
Islam, M. R., Sultana, T., Joe, M. M., Yim, W., Cho, J. C., and Sa, T. (2013). Nitrogen-fixing bacteria with multiple plant growth-promoting activities enhance growth of tomato and red pepper. J. Basic Microbiol. 53, 1004–1015. doi: 10.1002/jobm.201200141
Issa, A. A., Abd-Alla, M. H., and Ohyama, T. (2014). “Nitrogen fixing cyanobacteria: future prospect,” in Advances in Biology and Ecology of Nitrogen Fixation. Vol. 2. ed. T. Ohyama (Croatia: InTech), 23–48.
Ivleva, N. B., Groat, J., Staub, J. M., and Stephens, M. (2016). Expression of active subunit of nitrogenase via integration into plant organelle genome. PLoS One 11:e0160951. doi: 10.1371/journal.pone.0160951
Jensen, E. S., Carlsson, G., and Hauggaard-Nielsen, H. (2020). Intercropping of grain legumes and cereals improves the use of soil N resources and reduces the requirement for synthetic fertilizer n: a global-scale analysis. Agron. Sustain. Dev. 40:5. doi: 10.1007/s13593-020-0607-x
Jha, M. N., Prasad, A. N., Sharma, S. G., and Bharati, R. C. (2001). Effects of fertilization rate and crop rotation on diazotrophic cyanobacteria in paddy field. World J. Microbiol. Biotechnol. 17, 463–468. doi: 10.1023/A:1011913316988
Jia, X., Huang, J., Xiang, C., and Powlson, D. (2015). Reducing excessive nitrogen use in chinese wheat production through knowledge training: what are the implications for the public extension system? Agroecol. Sust. Food. 39, 189–208. doi: 10.1080/21683565.2014.967436
Joshi, P., Gulati, A., and Cummings, R. W. (2007). Agricultural Diversification and Smallholders in South Asia. eds. P. K. Joshi, A. Joshi, and R. W. Cummings (New Dehli: Academic Foundation).
Kallimath, G., and Patil, C. (2018). An exploration of rhizobium from green gram root nodules in the three agroclimatic zones of Karnataka, India. Int. J. Curr. Microbiol. App. Sci. 7, 2118–2130. doi: 10.20546/ijcmas.2018.703.249
Karthikeyan, B., Joe, M. M., Islam, M. R., and Sa, T. (2012). ACC deaminase containing diazotrophic endophytic bacteria ameliorate salt stress in Catharanthus roseus through reduced ethylene levels and induction of antioxidative defense systems. Symbiosis 56, 77–86. doi: 10.1007/s13199-012-0162-6
Kaschuk, G., Nogueira, M. A., De Luca, M. J., and Hungria, M. (2016). Response of determinate and indeterminate soybean cultivars to basal and topdressing n fertilization compared to sole inoculation with Bradyrhizobium. Field Crop Res. 195, 21–27. doi: 10.1016/j.fcr.2016.05.010
Kaushik, M. S., Srivastava, M., Singh, A., and Mishra, A. K. (2017). “NTCA transcriptional factor: a global nitrogen regulator and connecting link between nitrogen metabolism and other crucial metabolisms,” in Plants and Microbes in Ever Changing Environment. ed. S. S. Singh (India: NOVA Science Publisher), 101–127.
Kerr, A., and Bullard, G. (2020). Biocontrol of crown gall by Rhizobium rhizogenes: challenges in biopesticide commercialisation. J. Agron. 10:1126. doi: 10.3390/agronomy10081126
Kifle, M. H., and Laing, M. D. (2016). Effects of selected diazotrophs on maize growth. Front. Plant Sci. 7:1429. doi: 10.3389/fpls.2016.01429
Kisiala, A., Laffont, C., Emery, R. N., and Frugier, F. (2013). Bioactive cytokinins are selectively secreted by Sinorhizobium meliloti nodulating and non-nodulating strains. Mol. Plant-Microbe Interact. 26, 1225–1231. doi: 10.1094/MPMI-02-13-0054-R
Klawonn, I., Nahar, N., Walve, J., Andersson, B., Olofsson, M., Svedén, J., et al. (2016). Cell-specific nitrogen-and carbon-fixation of cyanobacteria in a temperate marine system (Baltic Sea). Environ. Microbiol. 18, 4596–4609. doi: 10.1111/1462-2920.13557
Kong, P., Richardson, P., and Hong, C. (2020). Burkholderia sp. Ssg is a broad-spectrum antagonist against plant diseases caused by diverse pathogens. Biol. Control 151:104380. doi: 10.1016/j.biocontrol.2020.104380
Kremen, C. (2020). Ecological intensification and diversification approaches to maintain biodiversity, ecosystem services and food production in a changing world. Emerg. Top. Life Sci. 4, 229–240. doi: 10.1042/ETLS20190205
Krusell, L., Krause, K., Ott, T., Desbrosses, G., Krämer, U., Sato, S., et al. (2005). The sulfate transporters SST1 is crucial for symbiotic nitrogen fixation in Lotus japonicus root nodules. Plant Cell 17, 1625–1636. doi: 10.1105/tpc.104.030106
Kumar, S., Suyal, D. C., Bhoriyal, M., and Goel, R. (2018). Plant growth promoting potential of psychrotolerant Dyadobacter sp. for pulses and finger millet and impact of inoculation on soil chemical properties and diazotrophic abundance. J. Plant Nutr. 41, 1035–1046. doi: 10.1080/01904167.2018.1433211
Kumar, S., Suyal, D. C., Yadav, A., Shouche, Y., and Goel, R. (2020). Psychrophilic Pseudomonas helmanticensis proteome under simulated cold stress. Cell Stress Chaperones 25, 1025–1032. doi: 10.1007/s12192-020-01139-4
Kumawat, K., Sharma, P., Sirari, A., Singh, I., Gill, B., Singh, U., et al. (2019). Synergism of Pseudomonas aeruginosa (LSE-2) nodule endophyte with Bradyrhizobium sp. (LSBR-3) for improving plant growth, nutrient acquisition and soil health in soybean. World J. Microbiol. Biotechnol. 35:47. doi: 10.1007/s11274-019-2622-0
Letourneau, D. K., Armbrecht, I., Rivera, B. S., Lerma, J. M., Carmona, E. J., Daza, M. C., et al. (2011). Does plant diversity benefit agroecosystems? A synthetic review. Ecol. Appl. 21, 9–21. doi: 10.1890/09-2026.1
Li, Y., Li, Y., Zhang, H., Wang, M., and Chen, S. (2019). Diazotrophic Paenibacillus beijingensis BJ-18 provides nitrogen for plant and promotes plant growth, nitrogen uptake and metabolism. Front. Microbiol. 10:1119. doi: 10.3389/fmicb.2019.03037
Liao, H., Li, Y., and Yao, H. (2018). Fertilization with inorganic and organic nutrients changes diazotroph community composition and N2-fixation rates. J. Soils Sediments 18, 1076–1086. doi: 10.1007/s11368-017-1836-8
Liu, Y., Pan, X., and Li, J. (2015). A 1961-2010 record of fertilizer use, pesticide application and cereal yield: a review. Agron. Sustain. Dev. 35, 83–93. doi: 10.1007/s13593-014-0259-9
Lomelino, C. L., Andring, J. T., McKenna, R., and Kilberg, M. S. (2017). Asparagine synthetase: function, structure, and role in disease. J. Biol. Chem. 292, 19952–19958. doi: 10.1074/jbc.R117.819060
Longhini, V. Z., Andreotti, M., de Souza, W. C. R., Costa, N. R., Teixeira Filho, M. C. M., and Montanari, R. (2017). Nitrogen fertilization and inoculation with diazotrophic bacteria in corn intercropped with xaraés grass. Rev. Bras. Cienc. 12, 340–347. doi: 10.5039/agraria.v12i3a5462
Longhini, V. Z., Souza, W. C. R. D., Andreotti, M., Soares, N. D. A., and Costa, N. R. (2016). Inoculation of diazotrophic bacteria and nitrogen fertilization in topdressing in irrigated corn. Rev. Caatinga 29, 338–347. doi: 10.1590/1983-21252016v29n210rc
Lori, M., Piton, G., Symanczik, S., Legay, L., Brussaard, L., Jaenicke, S., et al. (2020). Compared to conventional, ecological intensive management promotes benefcial proteolytic soil microbial communities for agro-ecosystem functioning under climate change-induced rain regimes. Sci. Rep. 10:7296. doi: 10.1038/s41598-020-64279-8
Maier, R., and Moshiri, F. (2000). Role of the Azotobacter vinelandi initrogenase-protective shethna protein in preventing oxygen-mediated cell death. J. Bacteriol. Res. 182, 3854–3857. doi: 10.1128/JB.182.13.3854-3857.2000
Manjunatha, A., Anik, A. R., Speelman, S., and Nuppenau, E. (2013). Impact of land fragmentation, farm size, land ownership and crop diversity on profit and efficiency of irrigated farms in India. Land Use Policy 31, 397–405. doi: 10.1016/j.landusepol.2012.08.005
Masalkar, P., Wallace, I. S., Hwang, J. H., and Roberts, D. M. (2010). Interaction of cytosolic glutamine synthetase of soybean root nodules with the C-terminal domain of the symbiosome membrane nodulin 26 aquaglyceroporin. J. Biol. Chem. 285, 23880–23888. doi: 10.1074/jbc.M110.135657
Massignam, A., Chapman, S., Hammer, G., and Fukai, S. (2009). Physiological determinants of maize and sunflower grain yield as affected by nitrogen supply. Field Crop Res. 113, 256–267. doi: 10.1016/j.fcr.2009.06.001
Meer, E., (2016). EIP-AGRI focus group on nutrient recycling: Starting paper on how to improve the agronomic use of recycled nutrients (N and P) from livestock manure and other organic sources. Report No. EIP-AGRI FG19.
Meng, X., Wang, L., Long, X., Liu, Z., Zhang, Z., and Zed, R. (2012). Influence of nitrogen fertilization on diazotrophic communities in the rhizosphere of the Jerusalem artichoke (Helianthus tuberosus L.). Res. Microbiol. 163, 349–356. doi: 10.1016/j.resmic.2012.03.005
Misra, S., and Chauhan, P. S. (2020). ACC deaminase-producing rhizosphere competent Bacillus spp. mitigate salt stress and promote Zea mays growth by modulating ethylene metabolism. 3 Biotech 10:119. doi: 10.1007/s13205-020-2104-y
Miyauchi, M. Y. H., Lima, D. S., Nogueira, M. A., Lovato, G. M., Murate, L. S., Cruz, M. F., et al. (2008). Interactions between diazotrophic bacteria and mycorrhizal fungus in maize genotypes. Sci. Agric. 65, 525–531. doi: 10.1590/S0103-90162008000500012
Mousavi, S. A., (2016). Revised taxonomy of the family rhizobiaceae, and phylogeny of mesorhizobia nodulating glycyrrhiza spp. Dissertationes Schola Doctoralis Scientiae Circumiectalis, Alimentariae, Biologicae.
Moyes, A. B., Kueppers, L. M., Pett-Ridge, J., Carper, D. L., Vandehey, N., O’Neil, J., et al. (2016). Evidence for foliar endophytic nitrogen fixation in a widely distributed subalpine conifer. New Phytol. 210, 657–668. doi: 10.1111/nph.13850
Mus, F., Alleman, A. B., Pence, N., Seefeldt, L. C., and Peters, J. W. (2018). Exploring the alternatives of biological nitrogen fixation. Metallomics 10, 523–538. doi: 10.1039/C8MT00038G
Mus, F., Crook, M. B., Garcia, K., Costas, A. G., Geddes, B. A., Kouri, E. D., et al. (2016). Symbiotic nitrogen fixation and the challenges to its extension to nonlegumes. Appl. Environ. Microbiol. 82, 3698–3710. doi: 10.1128/AEM.01055-16
Naher, U. A., Othman, R., Shamsuddin, Z. H., Saud, H. M., and Ismail, M. R. (2009). Growth enhancement and root colonization of rice seedlings by Rhizobium and Corynebacterium spp. Int. J. Agric. Biol. 11, 586–590.
Nanda, A. K., Andrio, E., Marino, D., Pauly, N., and Dunand, C. (2010). Reactive oxygen species during plant-microorganism early interactions. J. Integr. Plant Biol. 52, 195–204. doi: 10.1111/j.1744-7909.2010.00933.x
Naqqash, T., Imran, A., Hameed, S., Shahid, M., Majeed, A., Iqbal, J., et al. (2020). First report of diazotrophic Brevundimonas spp. as growth enhancer and root colonizer of potato. Sci. Rep. 10:12893. doi: 10.1038/s41598-020-69782-6
Narula, N., Kukreja, K., Kumar, V., and Lakshminarayana, K. (2002). Phosphate solubilization by soil isolates of Azotobacter chroococcum and their survival at different temperatures. J. Agric. Trop. Subtrop. 103, 81–87.
Naz, I., Ahmad, H., Khokhar, S. N., Khan, K., and Shah, A. H. (2016). Impact of zinc solubilizing bacteria on zinc contents of wheat. Am. Eurasian J. Agric. Environ. Sci. 16, 449–454. doi: 10.5829/idosi.aejaes.2016.16.3.12886
Nevo, E., and Coll, M. (2001). Effect of nitrogen fertilization on Aphis gossypii (Homoptera: Aphididae): variation in size, color, and reproduction. J. Econ. Entomol. 94, 27–32. doi: 10.1603/0022-0493-94.1.27
Newton, W. E. (2007). “Physiology, biochemistry, and molecular biology of nitrogen fixation,” in Biology of the Nitrogen Cycle. eds. S. J. F. Hermann Bothe and W. E. Newton (Elsevier), 109–129.
Nogueira, E. D. M., Vinagre, F., Masuda, H. P., Vargas, C., Pádua, V. L. M. D., Silva, F. R. D., et al. (2001). Expression of sugarcane genes induced by inoculation with Gluconacetobacter diazotrophicus and Herbaspirillum rubrisubalbicans. Genet. Mol. Biol. 24, 199–206. doi: 10.1590/S1415-47572001000100027
Normand, P., Daffonchio, D., Gtari, M., and Rosenberg, E., (2014). The prokaryotes: Actinobacteria. The prokaryotes: Actinobacteria. Berlin, Heidelberg: Springer Berlin Heidelberg: 361–379.
Nosrati, R., Owlia, P., Saderi, H., Rasooli, I., and Malboobi, M. A. (2014). Phosphate solubilization characteristics of efficient nitrogen fixing soil azotobacter strains. Iran. J. Microbiol. 6:285.
Oldroyd, G. E. (2013). Speak, friend, and enter: Signalling systems that promote beneficial symbiotic associations in plants. Nat. Rev. Microbiol. 11, 252–263. doi: 10.1038/nrmicro2990
Omara, P., Aula, L., Oyebiyi, F., and Raun, W. R. (2019). World cereal nitrogen use efficiency trends: review and current knowledge. Agrosys. Geosci. Environ. 2, 1–8. doi: 10.2134/age2018.10.0045
Ormeño-Orrillo, E., Hungria, M., and Martinez-Romero, E. (2013). “Dinitrogen-fixing prokaryotes,” in The Prokaryotes. eds. E. Rosenberg, E. F. De Long, S. Lory, E. Stackebrandt, and F. Thompson (Springer, Berlin, Heidelberg).
Pacheco-Aguirre, A. J., Ruiz-Sanchez, E., Ballina-Gomex, H. S., and Alvarado-Lopez, C. J. (2017). Does polymer-based encapsulation enhances performance of plant growth promoting microorganisms? A meta-analysis view. Agrociencia 51, 173–187.
Paerl, H. W., Xu, H., McCarthy, M. J., Zhu, G., Qin, B., Li, Y., et al. (2011). Controlling harmful cyanobacterial blooms in a hyper-eutrophic lake (lake taihu, China): the need for a dual nutrient (n & p) management strategy. Water Res. 45, 1973–1983. doi: 10.1016/j.watres.2010.09.018
Paul, E. A. (2000). Towards the year (2000): directions for future nitrogen research Advances in nitrogen cycling in agricultural ecosystems. Wilson J. R. 1988 417 425 CAB International Wallingford, United Kingdom.
Pawlowski, K., and Bisseling, T. (1996). Rhizobial and actinorhizal symbioses: what are the shared features? Plant Cell 8:1899. doi: 10.2307/3870238
Pereg, L., De-Bashan, L. E., and Bashan, Y. (2016). Assessment of affinity and specificity of azospirillum for plants. Plant Soil 399, 389–414. doi: 10.1007/s11104-015-2778-9
Perret, X., Staehelin, C., and Broughton, W. J. (2000). Molecular basis of symbiotic promiscuity. Microbiol. Mol. Biol. Rev. 64, 180–201. doi: 10.1128/MMBR.64.1.180-201.2000
Piceno, Y., and Lovell, C. (2000). Stability in natural bacterial communities: I. nutrient addition effects on rhizosphere diazotroph assemblage composition. Microb. Ecol. 39, 32–40. doi: 10.1007/s002489900192
Plominsky, Á. M., Larsson, J., Bergman, B., Delherbe, N., Osses, I., and Vásquez, M. (2013). Dinitrogen fixation is restricted to the terminal heterocysts in the invasive cyanobacterium Cylindrospermopsis raciborskii CS-505. PLoS One 8:e51682. doi: 10.1371/journal.pone.0051682
Prasanna, R., Chaudhary, V., Gupta, V., Babu, S., Kumar, A., Singh, R., et al. (2013). Cyanobacteria mediated plant growth promotion and bioprotection against fusarium wilt in tomato. Eur. J. Plant Pathol. 136, 337–353. doi: 10.1007/s10658-013-0167-x
Pueschel, D., Janouskova, M., Voriskova, A., Gryndlerova, H., Vosatka, M., and Jansa, J. (2017). Arbuscular mycorrhiza stimulates biological nitrogen fixation in two Medicago spp. through improved phosphorus acquisition. Front. Plant Sci. 8:390. doi: 10.3389/fpls.2017.00390
Rahman, S., and Chima, C. D. (2016). Determinants of food crop diversity and profitability in southeastern Nigeria: a multivariate tobit approach. Agriculture 6:14. doi: 10.3390/agriculture6020014
Reardon, C., Gollany, H., and Wuest, S. (2014). Diazotroph community structure and abundance in wheat–fallow and wheat–pea crop rotations. Soil Biol. Biochem. 69, 406–412. doi: 10.1016/j.soilbio.2013.10.038
Reckling, M., Hecker, J.-M., Bergkvist, G., Watson, C. A., Zander, P., Schläfke, N., et al. (2016). A cropping system assessment framework—evaluating effects of introducing legumes into crop rotations. Eur. J. Agron. 76, 186–197. doi: 10.1016/j.eja.2015.11.005
Reed, S. C., Seastedt, T. R., Mann, C. M., Suding, K. N., Townsend, A. R., and Cherwin, K. L. (2007). Phosphorus fertilization stimulates nitrogen fixation and increases inorganic nitrogen concentrations in a restored prairie. Appl. Soil Ecol. 36, 238–242. doi: 10.1016/j.apsoil.2007.02.002
Reinmuth-Selzle, K., Kampf, C. J., Lucas, K., Lang-Yona, N., FröHlich-Nowoisky, J., Shiraiwa, M., et al. (2017). Air pollution and climate change effects on allergies in the anthropocene: abundance, interaction, and modification of allergens and adjuvants. Environ. Sci. Technol. 51, 4119–4141. doi: 10.1021/acs.est.6b04908
Renard, D., and Tilman, D. (2019). National food production stabilized by crop diversity. Nature 571, 257–260. doi: 10.1038/s41586-019-1316-y
Ribbe, M., Gadkari, D., and Meyer, O. (1997). N2 fixation by Streptomyces thermoautotrophicus involves a molybdenum-dinitrogenase and a manganese-superoxide oxidoreductase that couple N2-reduction to the oxidation of superoxide produced from O2 by a molybdenum-CO dehydrogenase. J. Biol. Chem. 272, 26627–26633. doi: 10.1074/jbc.272.42.26627
Rosenblueth, M., and Martínez-Romero, E. (2006). Bacterial endophytes and their interactions with hosts. Mol. Plant-Microbe Interact. 19, 827–837. doi: 10.1094/MPMI-19-0827
Sabra, W., Zeng, A.-P., Lünsdorf, H., and Deckwer, W.-D. (2000). Effect of oxygen on formation and structure of Azotobacter vinelandii alginate and its role in protecting nitrogenase. Appl. Environ. Microbiol. 66, 4037–4044. doi: 10.1128/AEM.66.9.4037-4044.2000
Santi, C., Bogusz, D., and Franche, C. (2013). Biological nitrogen fixation in non-legume plants. Ann. Bot. 111, 743–767. doi: 10.1093/aob/mct048
Sasaki, K., Ikeda, S., Eda, S., Mitsui, H., Hanzawa, E., Kisara, C., et al. (2010). Impact of plant genotype and nitrogen level on rice growth response to inoculation with Azospirillum sp. strain b510 under paddy field conditions. Soil Sci. Plant Nutr. 56, 636–644. doi: 10.1111/j.1747-0765.2010.00499.x
Savci, S. (2012). Investigation of effect of chemical fertilizers on environment. Apcbee Procedia 1, 287–292. doi: 10.1016/j.apcbee.2012.03.047
Schultz, N., Silva, J. A. D., Sousa, J. S., Monteiro, R. C., Oliveira, R. P., Chaves, V. A., et al. (2014). Inoculation of sugarcane with diazotrophic bacteria. Rev. Bras. Cienc. Solo. 38, 407–414. doi: 10.1590/S0100-06832014000200005
Schwember, A. R., Schulze, J., Del Pozo, A., and Cabeza, R. A. (2019). Regulation of symbiotic nitrogen fixation in legume root nodules. Plan. Theory 8:333. doi: 10.3390/plants8090333
Serraj, R. (2004). Symbiotic Nitrogen Fixation: Prospects for Enhanced Application in Tropical Agriculture. India: Oxford and IBH Publishing Co. Pvt. Ltd.
Shaikh, S., and Saraf, M. (2017). Biofortification of Triticum aestivum through the inoculation of zinc solubilizing plant growth promoting rhizobacteria in field experiment. Biocatal. Agric. Biotechnol. 9, 120–126. doi: 10.1016/j.bcab.2016.12.008
Sharma, L. K., and Bali, S. K. (2018). A review of methods to improve nitrogen use efficiency in agriculture. Sustainability 10:51. doi: 10.3390/su10010051
Shin, W., Islam, R., Benson, A., Joe, M. M., Kim, K., Gopal, S., et al. (2016). Role of diazotrophic bacteria in biological nitrogen fixation and plant growth improvement. Korean J. Soil. Sci. Fer. 49, 17–29. doi: 10.7745/KJSSF.2016.49.1.017
Shinjo, R., Tanaka, A., Sugiura, D., Suzuki, T., Uesaka, K., Takebayashi, Y., et al. (2020). Comprehensive analysis of the mechanisms underlying enhanced growth and root n acquisition in rice by the endophytic diazotroph, burkholderia vietnamiensis rs1. Plant Soil 450, 537–555. doi: 10.1007/s11104-020-04506-3
Smith, V. H., and Crews, T. (2014). Applying ecological principles of crop cultivation in large-scale algal biomass production. Algal Res. 4, 23–34. doi: 10.1016/j.algal.2013.11.005
Spaink, H. P. (2002). Plant-microbe interactions: a receptor in symbiotic dialogue. Nature 417, 910–911. doi: 10.1038/417910a
Sridevi, M., and Mallaiah, K. (2009). Phosphate solubilization by Rhizobium strains. Indian J. Microbiol. 49, 98–102. doi: 10.1007/s12088-009-0005-1
Steenhoudt, O., and Vanderleyden, J. (2000). Azospirillum, a free-living nitrogen-fixing bacterium closely associated with grasses: genetic, biochemical and ecological aspects. FEMS Microbiol. Rev. 24, 487–506. doi: 10.1111/j.1574-6976.2000.tb00552.x
Stewart, W. D. P. (1969). Biological and ecological aspects of nitrogen fixation by free-living microorganisms. Proc. R. Soc. B 172, 367–388.
Sutton, M. A., Oenema, O., Erisman, J. W., Leip, A., Van Grinsven, H., and Winiwarter, W. (2011). Too much of a good thing. Nature 472, 159–161. doi: 10.1038/472159a
Suyal, D. C., Joshi, D., Kumar, S., Soni, R., and Goel, R. (2019). Differential protein profiling of soil diazotroph Rhodococcus qingshengii S10107 towards low-temperature and nitrogen deficiency. Sci. Rep. 9, 1–9. doi: 10.1038/s41598-019-56592-8
Suyal, D. C., Shukla, A., and Goel, R. (2014). Growth promotory potential of the psychrophilic diazotroph Pseudmonas migulae S10724 against native Vigna radiata (L.) Wilczek. 3Biotech. 4, 665–668. doi: 10.1007/s13205-014-0259-0
Tagele, S. B., Kim, S. W., Lee, H. G., and Lee, Y. S. (2019). Potential of novel sequence type of burkholderia cenocepacia for biological control of root rot of maize (Zea mays L.) caused by Fusarium temperatum. Int. J. Mol. Sci. 20:1005. doi: 10.3390/ijms20051005
Tahir, M., Mirza, M. S., Zaheer, A., Dimitrov, M. R., Smidt, H., and Hameed, S. (2013). Isolation and identification of phosphate solubilizer Azospirillum, Bacillus and Enterobacter strains by 16S rRNA sequence analysis and their effect on growth of wheat (Triticum aestivum L.). Aust. J. Crop. Sci. 7, 1284–1292.
Tu, Q., Deng, Y., Yan, Q., Shen, L., Lin, L., He, Z., et al. (2016). Biogeographic patterns of soil diazotrophic communities across six forests in the north america. Mol. Ecol. 25, 2937–2948. doi: 10.1111/mec.13651
Ullah, A., Mushtaq, H., Ali, H., Munis, M. F. H., Javed, M. T., and Chaudhary, H. J. (2015). Diazotrophs-assisted phytoremediation of heavy metals: a novel approach. Environ. Sci. Pollut. Res. 22, 2505–2514. doi: 10.1007/s11356-014-3699-5
Valentine, A. J., Benedito, V. A., and Kang, Y. (2018). “Legume nitrogen fixation and soil abiotic stress: from physiology to genomics and beyond” in Nitrogen Metabolism in Plants in the Post-genomic Era. Annual Plant Reviews book series. ed. J. A. Roberts, 207–248.
Van Deynze, A., Zamora, P., Delaux, P.-M., Heitmann, C., Jayaraman, D., Rajasekar, S., et al. (2018). Nitrogen fixation in a landrace of maize is supported by a mucilage-associated diazotrophic microbiota. PLoS Biol. 16:e2006352. doi: 10.1371/journal.pbio.2006352
Velmourougane, K., Prasanna, R., Chawla, G., Nain, L., Kumar, A., and Saxena, A. K. (2019). Trichoderma–azotobacter biofilm inoculation improves soil nutrient availability and plant growth in wheat and cotton. J. Basic Microbiol. 59, 632–644. doi: 10.1002/jobm.201900009
Verma, M., Singh, A., Dwivedi, D. H., and Arora, N. K. (2020). Zinc and phosphate solubilizing Rhizobium radiobacter (LB2) for enhancing quality and yield of loose leaf lettuce in saline soil. J. Environ. Sustain. 3, 1–10. doi: 10.1007/s42398-020-00110-4
Viera-Vargas, M. S., Souto, C. M., Urquiaga, S., and Boddey, R. M. (1995). Quantification of the contribution of N2 fixation to tropical forage legumes and transfer to associated grass. Soil Biol. Biochem. 27, 1193–1200. doi: 10.1016/0038-0717(95)00022-7
Vitousek, P. M., Menge, D. N., Reed, S. C., and Cleveland, C. C. (2013). Biological nitrogen fixation: rates, patterns and ecological controls in terrestrial ecosystems. Philos. Trans. R. Soc. 368:20130119. doi: 10.1098/rstb.2013.0119
Wang, J., Zhang, D., Zhang, L., Li, J., Raza, W., Huang, Q., et al. (2016). Temporal variation of diazotrophic community abundance and structure in surface and subsoil under four fertilization regimes during a wheat growing season. Agric. Ecosyst. Environ. 216, 116–124. doi: 10.1016/j.agee.2015.09.039
Wang, C., Zheng, M., Song, W., Wen, S., Wang, B., Zhu, C., et al. (2017). Impact of 25 years of inorganic fertilization on diazotrophic abundance and community structure in an acidic soil in southern China. Soil Biol. Biochem. 113, 240–249. doi: 10.1016/j.soilbio.2017.06.019
Wani, S. A., Chand, S., and Ali, T. (2013). Potential use of Azotobacter chroococcum in crop production: an overview. Curr. agric. res. J. 1, 35–38. doi: 10.12944/CARJ.1.1.04
Ward, M. H., Jones, R. R., Brender, J. D., De Kok, T. M., Weyer, P. J., Nolan, B. T., et al. (2018). Drinking water nitrate and human health: an updated review. Int. J. Environ. Res. Public Health 15:1557. doi: 10.3390/ijerph15071557
Xiao, D., Xiao, L., Che, R., Tan, Y., Liu, X., Yang, R., et al. (2020). Phosphorus but not nitrogen addition significantly changes diazotroph diversity and community composition in typical karst grassland soil. Agric. Ecosyst. Environ. 301:106987. doi: 10.1016/j.agee.2020.106987
Xu, G., Fan, X., and Miller, A. J. (2012). Plant nitrogen assimilation and use efficiency. Annu. Rev. Plant Biol. 63, 153–182. doi: 10.1146/annurev-arplant-042811-105532
Yang, L.-N., Pan, Z.-C., Zhu, W., Wu, E.-J., He, D.-C., Yuan, X., et al. (2019). Enhanced agricultural sustainability through within-species diversification. Nat. Sustain. 2, 46–52. doi: 10.1038/s41893-018-0201-2
Yelenik, S., Perakis, S., and Hibbs, D. (2013). Regional constraints to biological nitrogen fixation in post-fire forest communities. Ecology 94, 739–750. doi: 10.1890/12-0278.1
Zahran, H. H. (1999). Rhizobium-legume symbiosis and nitrogen fixation under severe conditions and in an arid climate. Microbiol. Mol. Biol. Rev. 63, 968–989. doi: 10.1128/MMBR.63.4.968-989.1999
Zhang, J., Guo, T., Wang, P., Tian, H., Wang, Y., and Cheng, J. (2018). Characterization of diazotrophic growth-promoting rhizobacteria isolated from ginger root soil as antagonists against ralstonia solanacearum. Biotechnol. Biotechnol. Equip. 32, 1447–1454. doi: 10.1080/13102818.2018.1533431
Zhu, S., Vivanco, J. M., and Manter, D. K. (2016). Nitrogen fertilizer rate affects root exudation, the rhizosphere microbiome and nitrogen-use-efficiency of maize. Appl. Soil Ecol. 107, 324–333. doi: 10.1016/j.apsoil.2016.07.009
Zilli, J. É., Alves, B. J. R., Rouws, L. F. M., Simões-Araujo, J. L., de Barros Soares, L. H., Cassán, F., et al. (2020). The importance of denitrification performed by nitrogen-fixing bacteria used as inoculants in south america. Plant Soil 451, 5–24. doi: 10.1007/s11104-019-04187-7
Keywords: nitrogen fixation, rhizobia, slow-releasing fertilizers, nitrogen use efficiency, nitrogen pollution
Citation: Imran A, Hakim S, Tariq M, Nawaz MS, Laraib I, Gulzar U, Hanif MK, Siddique MJ, Hayat M, Fraz A and Ahmad M (2021) Diazotrophs for Lowering Nitrogen Pollution Crises: Looking Deep Into the Roots. Front. Microbiol. 12:637815. doi: 10.3389/fmicb.2021.637815
Edited by:
Alok Kumar Srivastava, National Bureau of Agriculturally Important Microorganisms (ICAR), IndiaReviewed by:
Tapan Kumar Adhya, KIIT University, IndiaDeep Chandra Suyal, Eternal University, India
Alan Bennett, University of California, Davis, United States
Copyright © 2021 Imran, Hakim, Tariq, Nawaz, Laraib, Gulzar, Hanif, Siddique, Hayat, Fraz and Ahmad. This is an open-access article distributed under the terms of the Creative Commons Attribution License (CC BY). The use, distribution or reproduction in other forums is permitted, provided the original author(s) and the copyright owner(s) are credited and that the original publication in this journal is cited, in accordance with accepted academic practice. No use, distribution or reproduction is permitted which does not comply with these terms.
*Correspondence: Asma Imran, YXNtYWFzbGFtMjAwMUB5YWhvby5jb20=