- 1Medicinal Chemistry Laboratory, Department of Biosciences, Jamia Millia Islamia, New Delhi, India
- 2Department of Food Science and Nutrition, College of Food and Agriculture Science, King Saud University, Riyadh, Saudi Arabia
- 3Department of Pharmacognosy, College of Pharmacy, King Saud University, Riyadh, Saudi Arabia
- 4Department of Tashreehul Badan, Faculty of Unani Medicine, Aligarh Muslim University, Aligarh, India
In recent years, the demand for novel antifungal therapies has increased several- folds due to its potential to treat severe biofilm-associated infections. Biofilms are made by the sessile microorganisms attached to the abiotic or biotic surfaces, enclosed in a matrix of exopolymeric substances. This results in new phenotypic characteristics and intrinsic resistance from both host immune response and antimicrobial drugs. Candida albicans biofilm is a complex association of hyphal cells that are associated with both abiotic and animal tissues. It is an invasive fungal infection and acts as an important virulent factor. The challenges linked with biofilm-associated diseases have urged scientists to uncover the factors responsible for the formation and maturation of biofilm. Several strategies have been developed that could be adopted to eradicate biofilm-associated infections. This article presents an overview of the role of C. albicans biofilm in its pathogenicity, challenges it poses and threats associated with its formation. Further, it discusses strategies that are currently available or under development targeting prostaglandins, quorum-sensing, changing surface properties of biomedical devices, natural scaffolds, and small molecule-based chemical approaches to combat the threat of C. albicans biofilm. This review also highlights the recent developments in finding ways to increase the penetration of drugs into the extracellular matrix of biofilm using different nanomaterials against C. albicans.
Introduction
Biofilms are structurally organized microbial communities attached to the surfaces of implanted devices encapsulated within a solid protective extracellular matrix (Spormann et al., 2004). Approximately, 65% of all human microbial infections embroil biofilm formation. Therefore, identifying its role, composition, and impact of microbial biofilms on human medication is an attractive proposition (Douglas, 2003). Biofilm “inhabitants,” fungi and bacteria, are less sensitive or insensitive to antimicrobial agents. The property of the microorganisms to adhere to different surfaces facilitates the formation of biofilm in clinical settings such as catheters, prosthetic heart valves, joints, and various tissues in the host leading to effective colonization and this results in persistent drug-resistant.
In recent years, the prevalence of fungal infection has increased. These mycotic diseases account for over 10 lakhs of human deaths annually, which has become a delinquent health issue across the world. In today’s scenario, these opportunistic fungal infections have affected immunosuppressed or immunocompromised patients, and folks proclaimed for administration in the intensive care unit. These mycotic infections are classified from non-life-threatening mucocutaneous illnesses to intrusive infections which can affect any organ (Pfaller and Diekema, 2007; Yapar, 2014). This kind of fungal infection is often connected with the other mild to lethal fungal or bacterial infections involving species such as Aspergillus (Williams et al., 2016; Richardson and Rautemaa-Richardson, 2019) different species of Candida, Staphylococcus aureus, and Pseudomonas aeruginosa (Kaplan, 2014; Pleszczyńska et al., 2015; Fleming et al., 2017) and Cryptococcus neofornams (Aslanyan et al., 2017). Of the several known and frequently studied Candida species, C. albicans is the one most commonly recorded and encountered fungal pathogen in the human race (Su et al., 2018). Systemic candidiasis, caused by C. albicans, is known to be the reason for death in nosocomial and opportunistically abysmal fungal infection in patients (Su et al., 2018). followed by Candida glabrata. Candida tropicalis is commonly found in urinary tract infections (UTI) whereas Candida parapsilosis is mostly located on the epidermis of a healthy individual. The latter is also the causative agent of catheter-related infections. Likewise, all Candida species depict differences in terms of their biofilm formation, structure, changes in the morphology extracellular matrix (ECM), and potential to resist antifungal drugs (Cavalheiro and Teixeira, 2018).
National Nosocomial Infection Survey (NNIS) indicated that Candida spp. were the fourth most common cause of nosocomial bloodstream infections during the 1990s. However, in more recent studies, it has been discovered that Candida spp. are the third most frequent nosocomial bloodstream isolates (Perlroth et al., 2007; Lohse et al., 2018). Additionally, Candida is the third mainstream infection of central-line-associated bloodstream infections (CLABSIs) and the second most prominent reason for catheter-associated urinary tract infections in the United States. Lockhart (2014) Subsequently, the incidence of disseminated candidiasis which broadly refers to the mucosal, cutaneous, and deep-seated organ infection caused by Candida genus has increased 15 to 20-fold in the last two decades (Lockhart, 2014; Pappas et al., 2018). It has been reported that systemic candidiasis, caused by C. albicans is one of the major reasons for death in nosocomial as well as opportunistically abysmal fungal infection in patients because of its ability to form a biofilm which decreases the susceptibility of fungal cells toward drug treatment. Consequently, it has been reported each year that up to 50% of systemic candidiasis adults patients and approximately 30% of the young population die due to candidiasis co-related with biofilms. Moreover, there is an estimation of 100 thousand deaths because of the infection initiated by biofilm formation. Approximately, $6500 millions are spent per year in the United States toward its treatment causing a high fiscal impact on the state (Gulati and Nobile, 2016; Silva et al., 2017). The five most widespread species, C. albicans, C. glabrata, C. parapsilosis, C. tropicalis, and C. krusei are the reason behind 92 to 95% of Intensive Care Unit (ICU) cases in the United States, although, distribution of all five species might vary (Kojic and Darouiche, 2004; Ten Cate et al., 2009). According to National Institute of Health (NIH) reports, pathogenic biofilms account for more than 80% of all microbial infections (Ramage et al., 2006; Perlroth et al., 2007; Tsui et al., 2016; Lohse et al., 2018), of which 75% cases are of vaginal yeast infection in women once or more than once in their lifetime (Perlroth et al., 2007; Yapar, 2014).
Candida albicans is a commensal fungi, asymptomatically associated with normal microflora of the host, but becomes invasive and virulent when converted to hyphal form covered by an extracellular polymeric substance (EPS), which is a dimorphic nature of C. albicans (Gulati and Nobile, 2016). It is the most stereotypical fungal pathogen in humans, causing disease ranging from apparent mucosal to lethal disseminated bloodstream infections which make a considerable contribution of more than 40% of mortality rates (Lohse et al., 2018).
The three stages of the development of C. albicans comprise adhesion of the yeast cells on the medical devices (early stage), differentiation of the yeast cells to hyphal cells (intermediate stage), and an increase in the matrix which is the maturation phase (Alim et al., 2018). The mature biphasic structure of C. albicans is promoted by the adhesive hydrophobic nature of indwelling devices with the proper growing environment. Biomedical devices inserted during transplantation catheter are the favorable ones as they provide nutrition like glucose from the excreted products (Ramage et al., 2006; Alim et al., 2018).
Candida albicans colonizes the skin, intestinal mucosa and/or genital mucosa of 30–70% of healthy individuals. Therefore, under normal circumstances, the fungus does not cause any significant disease (Ramage et al., 2014). Furthermore, about 15% of sepsis cases acquired in a hospital due to medical devices occur due to the predominant fungi Candida (Lohse et al., 2018). According to some recent reports, 63.5% of patients out of 224 patients suffering from septic shock showed a positive result for Candida and were found dead. Thus, it is the leading cause of death in hospital procured diseases (Ramage et al., 2014). In India, just 6-18% of cases of Candidemia are reported. A study exhibits 53 repeated episodes of Candidemia in 48 patients in 1.5 years. Different species made their contribution, like 45% of infections were due to C. tropicalis, while 23% of cases by C. albicans and the rest 32% by other Candida spp. (Kothari and Sagar, 2009). Western countries’ trend on candidemia differs from India as C. albicans is related to more than 50% of all candidemia incidences (Kaur et al., 2014).
Recent Challenge-Drug Resistance
The extensive use of antimicrobials medicaments in a well-connected global human population has increased the cases of antimicrobial resistance (Michael et al., 2014). According to the UN ad hoc Interagency Coordinating Group on Antimicrobial Resistance, drug-resistant diseases by 2050 can bring around 10 million deaths per year and cause an economic catastrophe similar to that of the 2008-2009 global financial recession. By 2030, antimicrobial resistance could affect approximately 24 million people plunging them into severe poverty (World Health Organisation [WHO], 2019). According to the Center for disease control and prevention, around 7% of all the blood samples from patients suffering from Candida is resistant to fluconazole. However, this resistance to azole is persistent for the last 20 years, but resistance toward echinocandins and other drugs is a huge concern. Thus, this situation will prove to be lethal because of the ever -growing human population.
Owing to its inappropriate use, antifungal drug resistance has emerged as a major challenge that needs immediate attention. This is further aggravated because of the overuse of antibiotics, which affects the normal microflora of humans, providing a favorable environment for C. albican to grow (Ben-Ami et al., 2012). Currently, available drugs are categorized into four major classes which include azoles, polyenes, pyrimidine analogs, and echinocandins. The primary targets of these antifungal drugs are the biosynthetic pathway of ergosterol, the cell wall of fungal cells, or the DNA/RNA of fungi (Borghi et al., 2016; Sagatova et al., 2016; Silva et al., 2017; Figure 1).
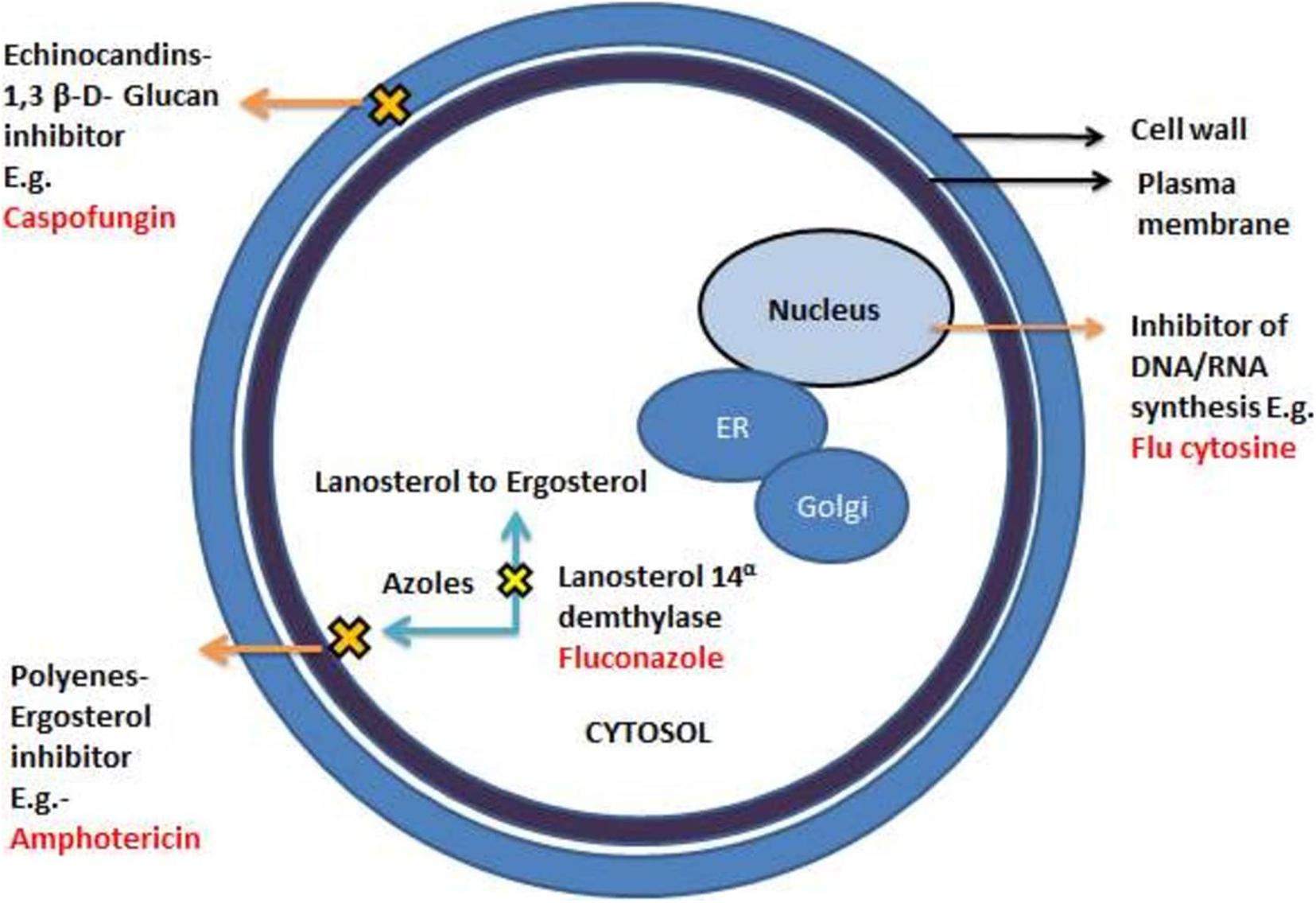
Figure 1. A different mechanism of actions of commonly known antifungal drugs adapted from Spampinato and Leonardi (2013).
Azoles are widely recommended antifungal drugs to treat superficial and invasive fungal infections. The first generation azoles include clotrimazole, bifonazole, econazole, and ketoconazole containing imidazole in their ring system. Fluconazole and itraconazole are some of the renowned examples of second- generation azoles., containing triazole moiety. Voriconazole and posaconazole are the FDA- approved broad -spectrum third generation azole- based antifungal drugs. They exhibit their antifungal effects by inhibiting cytochrome P450-dependent 14α-lanosterol demethylase (Cyp51) encrypted by the ERG11 gene which converts lanosterol to ergosterol (Taff et al., 2013; Silva et al., 2017). The ancient class of fungicidal drugs, polyenes, is used to treat severe infections. It intercalates with the steroids ergosterol, found in the cell membrane, creating pores that obliterate the proton gradient of the cell, destabilizing the cell membrane causing leakage of the ions in the process. Another fungicidal drug, Echinocandins (e.g., Caspofungin), is the newest class of antifungals that acts against Candida spp. and is administered intravenously. It interferes with the production of β-1,3- glucans, critical polysaccharides and the major constituent of fungal cell walls (Perfect, 2017; Pristov and Ghannoum, 2019).
Analogs of nucleosides like 5-flucytosine (5-FC) are antimetabolites that imitate nucleotide bases in the course of the synthesis of nucleosides. The 5-Flucytosine acts as a pyrimidine correspondent disturbing the synthesis of fungal RNA, DNA, and protein leading to cell cycle arrest. The 5-FC in itself does not act as an antifungal agent but becomes active when changed into 5-fluorouracil. This conversion of 5-FC into 5-FU is catalyzed by the enzyme cytosine deaminase, residing in fungal cells but not present inside the host cells. Even with a vast diversity of antifungal drugs, the problem of resistance persists against the drug sequestration, making it challenging to fight. The classic inventions, polyenes and azoles, are also ineffective against C. albicans biofilms, thereby limiting the range of treatment. Thus, the indispensable development of new antifungal therapies with high efficiency against the biofilm mode of growth is required. Biofilms are the major contributors and intensifiers of antifungal resilience. As yet, none of these factors works alone. Instead, this antifungal resilience is a multifactorial phenomenon, which is still unexplored. Various factors of intrinsic resilience which alter normal vegetative cell to more virulent form have been listed briefly. These include an increase in the density of cells within the biofilm, complex association extracellular matrix, the existence of persister cells, gene expression of the antifungal resistance genes, and the proliferation of sterols on the membrane of biofilm cells (Romo et al., 2017; Silva et al., 2017; Su et al., 2018).
Fungal Biofilms
Fungal biofilms are the complex association of hyphal cells which in turn are associated with both abiotic and animal tissues. They are important virulence factors and correlated with invasive fungal infection (Borghi et al., 2015). They are the sessile microorganisms that, when attached to the abiotic or biotic surfaces, lead to new phenotypic characteristic features (Hawser and Douglas, 1995; Lohse et al., 2018). Implantable medical devices are the favorable sites where C. albicans form a complex association forming the biofilms, thus becoming responsible for a proportion of clinical candidiasis (Douglas, 2002).
Furthermore, adherence of the fungal cell to the available biomaterial and its relatedness to bloodstream infections might be due to hematogenous spread. Medical devices provide a perfect niche to yeast cells because of their structure to chemical properties ranging from hydrophobicity to surface roughness. These devices are surrounded by body fluids like urine, blood, saliva, and synovial fluid, which condition them with glycoproteinaceous film (Gristina et al., 1988; Subbiahdoss et al., 2010). This acclimatizing film can accord chemical properties entirely different from its origin. The mature biphasic structure of C. albicans is promoted by non-specific factors (cell surface hydrophobicity and electrostatic forces) and specific adhesins on the fungal surface recognizing ligands in the conditioning films, such as serum proteins (fibrinogen and fibronectin) and salivary factors (Chaffin et al., 1998; Demuyser et al., 2014). Also, C. albicans cells can co-aggregate to interact with bacterial cells/colonies already vested in these devices (Chaffin et al., 1998; Ramage et al., 2006). Nevertheless, the preliminary focal attachment of the fungal cell to a substratum is accompanied by multiplication and propagation of cells followed by biofilm development (Soll and Daniels, 2016; Silva et al., 2017).
Pathogenesis of Biofilm
Stages of Biofilm Development
It has been observed that biofilm development follows sequential steps over a period of 24–48 h (Mathé and Van Dijck, 2013; Taff et al., 2013; Figure 2). Initially, a single yeast cell adheres to the substratum making a foundation for the layer of a yeast cell (adherence step) (Finkel and Mitchell, 2011; Mathé and Van Dijck, 2013; Williams et al., 2013). Following this initial phase is the phase of cell proliferation where cells project out and continue to grow into the filamentous structure of hyphal cells through the surface (initiation step). The assembly of hyphae marks the beginning of biofilm formation accompanied by the accretion of an extracellular matrix (ECM) on maturation biofilm (maturation step). Lastly, non-adhering yeast cells detach themselves from the biofilm into the environment to find a favorable site of attachment (dispersal step).
Spreading of biofilm-associated yeast cells has tremendous clinical significance as they can start the formation of new biofilms or circulate throughout the host cell and tissues leading to disseminated invasive diseases or candidemia. Various factors promoting the pathogenesis of C. albicans biofilm (Figure 3) have been reported, which are discussed in the following section.
Extracellular Matrix Formation (ECM)
The extracellular matrix is a critically important feature of biofilms that guards the adherent cells against the host immune system and antifungal agents by forming an extensive structure of the matrix (Borghi et al., 2016). In some of the pioneer works, it was shown that Candida species biofilm’s matrices increase when highly dynamic flow environments influence biofilm, and its quantity extensively depends upon strain and the species. Moreover, the chemical composition of C. albicans ECM suggests that the extracellular matrix is composed of approximately 55% of a combination of glycoproteins with carbohydrates contributing to only 25% of the total composition. Carbohydrates consist largely of α-mannan and β-1, 6-glucan polysaccharides with β-1, 3-glucans making a very little contribution. ECM also consists of 15% of lipids and only 5% of nucleic acids. β- 1, 3- glucan plays a major role in azole resilience by specific binding. Additionally, the biofilm is two times thicker than planktonic cells. Upon comparison of the chemical composition of planktonic cells with biofilm cells, it was observed that there is a difference in carbohydrate and β 1, 3 glucan composition (Mah and O’Toole, 2001; Nett et al., 2010; Silva et al., 2017; Lohse et al., 2018; Pappas et al., 2018).
Extracellular DNA and Genetic Factors
Extracellular DNA (e-DNA) present inside the extracellular matrix is a major contributor to the stability of the C. albicans biofilm. The e-DNA is present both in bacterial and fungal biofilms; hence, when these biofilm-forming microorganisms are treated with DNAase enzyme in combination with the respective drugs, they exhibit a decrease in the biofilm matrix (Martins et al., 2014). Various genetic factors like Bcr1 (a transcription factor required for the attachment of fungal cells to the abiotic surfaces), Rlmp, Brg1, Efg1, Ndt80, Rob1, and Tec1, Fsk1p, Smi1p (which works in unification with Fsk1p and Rlmp), Gcr1, Mnn4 are under study. All these factors work together and interact with different genes to regulate and generate biofilms, thus, has given new insight to biofilm formation (Finkel and Mitchell, 2011; Nett et al., 2011; Nobile et al., 2011).
Quorum Sensing (QS)
Another mechanism related to extracellular properties of the matrix of the C. albicans is quorum sensing, which plays a significant role in the growth of biofilms. It is a density-dependent cell-cell communication mechanism in which autoinducers (signal molecules) are released in response to the increasing density, which enhances or represses the activation of certain genes or factors. This density-dependent mechanism affects different aspects of microorganisms like pathogenesis, morphology, competence, etc. Importantly, it also makes its contribution to biofilm formation. Earlier, QS was thought to be an exclusive feature of a certain bacterial system, but the recent discovery of farnesol, a quorum-sensing molecule inhibiting C. albicans biofilms, has publicized QS (Ramage et al., 2002). Genetic regulation of virulent genes in pathogenic microorganisms by QS has shown an indirect connection with the emergence of multi-drug resistant pathogens. Thus, it necessitates the finding of alternative strategies to target QS and restrain the same (Finkel and Mitchell, 2011; Taff et al., 2013).
Evasion of Host Immune Responses
The immune system plays a crucial role in the recognition and elimination of the C. albicans. The innate immune system, which is the first line of defense, recognizes pathogen-associated molecular patterns of a pathogenic strain of C. albicans leading to the activation signaling channels of a host organism resulting in the extermination of the C. albicans cells.
According to recent studies, ten diverse surface receptors participate in this recognition pattern. They are Toll-like receptors -TLR4 and TLR2, TLR9, and NLR Family Pyrin Domain Containing 3 (NLRP3) the internal receptors, C-type lectin receptors, Dectin-1and 2, Dendritic Cell-Specific Intercellular adhesion molecule-3-Grabbing Non-integrin (DCSIGN), Mincle, and Mannose-binding lectin. Usually, these receptors recognize and bind to sugar moieties (β-1, 3-glucans, and mannose derivatives) present on the cell surface of C. albicans. Due to this binding, the cytokine complement system gets activated, phagocytizing the fungal cells. Internal uptake of these fungal cells by APCs (antigen-presenting cells) expedites the activation of internal receptors; thus, resultant activation of TLR9 or NLRP3 inflammasome occurs. This non-specific immune response (innate response) has a significant role in preventing C. albicans infection. Besides, the adaptive immune response also makes its contribution by producing antibodies against some specific extracellular proteins, obstructing the C. albicans growth.
On the other hand, there are many strategies employed by C. albicans to evade the immune response. Mature biofilms escape immune responses owing to the presence of a top layer of biofilm composed of hyphal cells which mask the β-glucans component of biofilm. Subsequently, these hyphal cells escape the neutrophil killing either by penetrating the epithelial cell layers in the course of invasive growth or by escaping immune response by physical penetrating inside the cell. The variance in the degree of expression of genes in planktonic cells concerning biofilms cells has a connection with a mechanism of immune evasion. There are several proteins that are highly expressed and they also restrict the stimulation of the host complement system. For example, Zinc binding cell surface protein Pra1, a cell surface glycerol-3-phosphate dehydrogenase protein (Gpd2), and all the secretory proteins of aspartyl protease (Sap) family. Another well-known protein that functions as a sensor for cell wall damage with high expression in biofilms is Msb2. This protein secretes and blocks the antimicrobial peptides preventing complement activation.
Neutrophils are the first foot soldiers of the immune system which are altered during biofilm formation. Neutrophils work with different modes of action like phagocytosis, oxidative response, and non-oxidative response against the emerging pathogen. Another known novel mechanism that was discovered in 2004, is the Neutrophil extracellular trap “NETosis.” It is a complex structure that comprises neutrophil chromatin, DNA, and protein. NETosis degranulates neutrophils and releases a lytic enzyme, which is different from necrosis and apoptosis. It has killing properties against planktonic yeast cells; however, it is seemingly ineffective against C. albicans biofilms (Branzk and Papayannopoulos, 2013; Johnson et al., 2016, 2017; Tsui et al., 2016). Some studies also suggest that the peripheral mononuclear cells (PBMCs) -due to some unknown factors- thicken the C. albicans biofilms rather than phagocytizing them. Furthermore, mature biofilms do not evoke a vigorous oxidative response, a primary mechanism of neutrophil killing microbes (Lohse et al., 2018; Pappas et al., 2018).
Polymicrobial Species
Humans provide an immediate small- scale environment to a varied population of microbial species. The human microbiota embraces members from three kingdoms of life: Archaea, Bacteria, and fungi. They usually exist in a symbiotic relationship leading to a complex association of ecosystems. Imbalance in this symbiotic association due to genetic or environmental factors of the host - like changes in pH, shifts in host immunity, and transient viscosity of mucosal layers, and indiscriminate usage of broad-spectrum antimicrobial agents- lead to the disproportionate infection by the overgrowth of specific microbial species over others.
Candida albicans is the most frequent fungal pathogen present in commensalism with bacteria. Furthermore, there is incremental progress in the dual-species biofilms formation between common bacterial species and C. albicans that usually interact in human beings to cause infection. These kinds of interaction which lead to diseases are studied and concluded based on observational studies, arising mainly in immunocompromised patients. It is difficult to establish a clinical prognosis of polymicrobial interaction from immunocompromised individuals as they are highly vulnerable to other infections. Hence, it is tough to evaluate the degree of polymicrobial interactions and molecular mechanisms from such studies. In vitro studies suggest that bacterial species and Candida spp. isolated from different parts, like the vagina, oral cavity, etc., interact with each other in different ways. This includes exudation of signaling molecules which influences species behavior toward each other, physical interaction between microbial cells (e.g., hyphal cells provides an attachment site to bacterial cells contained within polymicrobial biofilms), biochemical alterations of the local environment like change in oxygen content and pH. For instance, in the case of the vaginal microbiota, C. albicans interacts with Lactobacillus species which produces lactic acid that changes local pH, thus hindering C. albicans growth on the mucosal surface of the vagina. Some hyphal cell wall proteins (E.g., Hwp1), transcription regulators (Tec1 and Bcr1, Efg1, Cph1, etc.) and adhesive proteins (E.g., Als1, Als2, and Als3) show a pivotal role in enhancing the expression of virulent genes and hence the resistance in bacterial species such as Staphylococcus aureus, Streptococcus gordonii and Staphylococcus epidermidis (Harriott and Noverr, 2010; Pammi et al., 2013; Chinnici et al., 2019). However, deletion or mutation of these proteins results in a decrease in the number of bacterial cells and weakens their interaction with C. albicans. Although biofilms are formed in an oxygen-rich environment, some anaerobic bacteria can easily grow under C. albicans biofilms. These biofilms provide bacteria with a positive anaerobic environment to grow. In return, the bacteria augments the formation of C. albicans “mini biofilms” which can easily float and grow under the toxic condition (Douglas, 2003; Finkel and Mitchell, 2011; Gulati and Nobile, 2016; Alim et al., 2018).
Efflux Pumps
Overexpression of efflux pumps is one of the major contributors to antifungal drug resistance in C. albicans as they lead to drug sequestration by pumping out the antifungal drugs given as a treatment. Subsequently, these are primarily concerned with first-generation azoles but ineffective toward Echinocandins resistance. During usual antifungal treatment toward planktonic cells, efflux pumps prevent the intracellular accumulation of antifungal drugs by up-regulating their expression (Borghi et al., 2016; Soll and Daniels, 2016; Tsui et al., 2016). The Cdr1, 2 (ATP binding cassette transporter superfamily), and Mdr1 (Major facilitator transporter superfamily) are the two major classes of efflux pumps that control drug exportation in C. albicans. Moreover, initially, after a few hours of up-regulation in biofilms, they persist in being up-regulated all through the biofilm growth even in the absence of the antifungal drug. Rapid upregulation of efflux pumps happens during the primary stage of biofilm formation.
Persister Cell and Stress Response
Another major contributing element toward resistance is persister cells, which are the inconsequential subset of yeast cells with minimum metabolic activity assumed to be raised as a phenotypic variant but not a mutant type. They are highly resistant cells within biofilms. Amphotericin B treatment upon C. albicans led to the discovery of persister cells. These cells are acknowledged to be persister as they maintain themselves in a dormant stage. Still, in a stressful situation, they reactivate themselves to a state of active metabolism and reinstate as biofilms. Subsequently, drug sequestration by persister cells occurs due to some virulent traits like hyphal growth rather than an expression of efflux pumps and cell membrane structure (Mah and O’Toole, 2001; Tsui et al., 2016; Silva et al., 2017; Lohse et al., 2018).
Antibiofilm Strategies
The social burden of fungal disease is massive with approximately more than 1.5 billion people affected by the fungal disease worldwide per annum. However, these invasive fungal infections are estimated to be the cause of about 1.5 million deaths per year, making this a noteworthy health problem (Bongomin et al., 2017). While flourishing in its most usual mode of growth, a biofilm of C. albicans biofilm displays increased resistance to the available antifungal drugs. These persistent groups of cells are difficult to eradicate and, ever so often, accountable for treatment failures. There is a necessity to develop new strategies to exterminate and treat these emerging C. albicans biofilm infections in the medical setting.
Different targets have gained attention aiming to tackle this growing antifungal resistance problem. Treating these biofilms is a big hurdle in medical mycology; thus, there is a vast exigency for the development of new antifungal agents and identification of novel targets.
Considering the efforts undertaken to find solutions to the fungal biofilm infections, the reader may acknowledge the complicated mechanisms that impede the path to reach the panacea. The research was done while managing the patients so far has led to the conclusion that the study of biofilm phenotype is inevitable to restrict fungal biofilms. Besides, the counter effect of fungus on the drug should be examined not just against the planktonic cells but also against the biofilm. Since fully established mature biofilms are much more challenging to treat and eradicate, preemptive practices targeting planktonic cells further inhibiting the early development of biofilms are the need of the hour. Nonetheless, strategies to combat mature biofilms should also be kept in mind. We discuss specific strategies that are under scrutiny, globally, against the problem of drug resistance (Pierce et al., 2015b; Romo et al., 2017).
Prostaglandins
Molecular studies on biofilms have revealed the important role of lipids on antifungal resistance. The lipid profile of planktonic cells differs from that of biofilms, making lipids a censorious modulator of resistance. This change in the sphingolipids and sterol profile promotes biofilm formation affects cells morphology and physiology altering the adhesion properties in C. albicans (Alim et al., 2018; Su et al., 2018). Thus exploiting these prostaglandins adaptation and their properties could be targeted for treatment. The signaling cascade of Arachidonic acid (AA) is pivotal for both biology of humans and fungi morphology and growth. Very nearly each of the following enzymes of the AA cascade can be subjected to the pharmacological investigation: Cyclooxygenase (COX), Cytochrome P 450 (CYP), and Lipoxygenase (LOX) (Liu et al., 2016). Arachidonic acid could be processed into different types of eicosanoids.
Prostaglandins are synthesized by cyclooxygenase enzymes in human cells as well as pathogenic fungi. Prostaglandin E2 is the profusely synthesized prostaglandin that transfers immune responses of humans in the direction which endorses fungal establishment and chronic illness (Erb-Downward and Noverr, 2007; Alim et al., 2018). PGE2 inhibits T- helper cells (Type-1) –Th-1 and stimulates the progression of T-helper cells (Type II)-Th-2. These cells are responsible for maintaining the inflammatory responses as Th-1 is accountable for pro-inflammatory responses, while Th-2 for producing anti-inflammatory responses (Berger, 2000). PGE2 also down-regulates the production of chemokine and TNFα, thus augmenting the fungal colonization and germ tube formation.
Arachidonic acid, when used along with antifungal drugs, affects the level of prostaglandin E2 in a variety of Candida spp. The above-mentioned study suggests that lipid plays a specific role in the structural aspects, signaling cascade, fungal pathogenicity, and metabolic activity of biofilm development in C. albicans. However, the defined part of lipid-dependent processes in the development of C. albicans biofilm is still not known. Genetic modulation and pharmacological inflection of biosynthetic pathways of lipids are the two stratagems that have been browbeaten in linking lipid alterations with C. albicans biofilm morphology- its formation and function in this fungi. Modulating the lipid composition of the cell membrane by comprehensively targeting genes that encode enzymes for lipid biosynthesis regulates endogenic lipid levels (Noverr and Huffnagle, 2004; Erb-Downward and Noverr, 2007; Sherry et al., 2012; Fourie et al., 2016; Liu et al., 2016).
Modification of the Surface of Biomedical Devices
Biomaterial-associated-infection (BAI) is the major cause of dereliction of biomaterial implants. Microbial contamination of biomaterial devices during implant surgery (peri-operative contamination) or hospitalization (Gristina et al., 1988), causes the onset of BAI. Microorganisms present in BAI are resistant due to the biofilm mode of growth. The extensive use of indwelling catheters in recent medicine, particularly central venous and hemodialysis catheters, has contributed significantly to the increasing incidence of fungal bloodstream infections, in particular, candidiasis (Pierce et al., 2015b).
Traditionally, to decrease the cases of nosocomial infection, which occurs due to central line-associated bloodstream infections (CLABSIs), removal of the devices and providing systemic antimicrobial therapy was performed to eradicate these microorganisms.
These biomedical-assisted devices with a wide range of biomaterials are utilized by a wide range of pathogenic fungi like C. albicans to support adhesion, colonization, and subsequent biofilm formation (Ramage et al., 2006; Talsma, 2007). Thus, to preclude these contagious microorganisms on the surface of implanted devices with biofilms, there is an inquisitiveness in the development and improvement of unconventional biomaterials which are unfavorable to microbial (both fungal and bacterial) adhesion and colonization. Another modification strategy from some studies suggests that modifying the surface chemistry of the biomaterials could be an approach to prevent or reduce biofilm formation. This could be achieved by adding surface-modifying end groups (SMEs) or by altering the chemical composition of substrates. For example, SME Polyetherurethane when added to Elasthane 80A, a biomaterial, decreased the C. albicans ability to form biofilm significantly (Chandra et al., 2005).
Antibiotic Lock Therapy (ALT)
Antibiotic lock therapy is, in general, a combination of an antibacterial solution that has minimum inhibitory concentrations (MIC) 100-1000 times greater than the antibiotic used for planktonic cells in combination with the anticoagulant instilled into the lumen of a catheter. The solution is allowed to dwell or is “locked” while the Central Venous Catheter (CVC) is not used to prevent colonization or sterilize a previously infected catheter (Bookstaver et al., 2013; Justo and Bookstaver, 2014; Norris et al., 2017). The study suggests that only 10% of the patients subjected to ALT needed conventional replacement therapy compared to 33% of the patients treated with systemic antimicrobial drugs (O’Horo et al., 2011). Ethanol Lock solution at more than 40% dwelled onto catheter with C. albicans biofilm cleansed the catheter within 30 minutes (Öncü, 2014). Interestingly, an in vitro study of 40% ethanol lock solution in combination with 60IU heparin showed a significant decrease in ≥90% of C. albicans biofilm metabolism (Alonso et al., 2018).
Small Molecular Inhibitors and Natural Products
Microbiological resistance depends on various fungal factors that have been established due to genetic alteration in the fungi. Clinical resistance is due to host- or drug-related factors. All these factors may cause fungal resistance individually or in tandem. In addition to standardized susceptibility testing and appropriate drug dosing, one way to avoid resistance is the use of combinational antifungal therapy. Combination therapy also offers advantages in increased synergistic action with enhanced spectrum activity. Newer insights into mechanisms of drug resistance will help in the development of appropriate antifungal therapy.
For dealing with this problem of resistance, there are two ways: (i) finding of the novel anti-biofilm molecules and (ii) repurposing of the known drug to increase the activity of antifungal agents by combinational therapy. Due to the scarcity of known molecular scaffolds that inhibit/disperse fungal biofilms, high throughput screening (HTS) has been employed in an attempt to discover leads for new anti-biofilm modulators. Intending to identify novel small molecules that possessed anti-biofilm activity, screening of an extensive chemical library of compounds took a new start. It was observed that clinical isolates of C. albicans have varied abilities to respond to different growth media to form hyphae and also biofilms, and this variability is not associated with specific host conditions or characteristics. A high-content screen has identified many new structural series of antifungal compounds with the mode of action, namely inhibition of biofilm formation and filamentation. Many compounds among them represent a promising candidate for the development of novel anti-virulence approaches against C. albicans infections, aimed at minimizing the development of resistance.
Different Targets of Small Molecule Inhibitors
Small molecular inhibitors have been identified specifically for various targets along with their MIC/IC50 values which are summarized in the tables mentioned below.
Inhibitors of budded to hyphal transition
The pathogenic yeast C. albicans can exist in multiple morphological states, including budded, pseudohyphal, and true hyphal forms. The ability to change between the budded and hyphal forms, termed as the budded-to-hyphal-form transition, is important for virulence and is regulated by multiple environmental and cellular signals (Toenjes et al., 2005).
Filastatin 1, inhibits yeast to hyphal transition, impedes the adhesion of fungal cells to different biomaterials by inhibiting the hyphal specific HWP1 promoter. Interestingly, in the in vivo studies, filastatin in the presence of fluconazole protected C. elegans against C. albicans infection. Moreover, it also inhibited biofilm formation in a mouse model of vulvovaginal candidal infection (Fazly et al., 2013). Similarly, Johnson and the group identified several small molecular inhibitors. This includes Buhytrin A 2, CGP-37157 3 (affecting the calcium metabolism of bud to hyphal transition), and ETYA 4 (inhibiting multiple signaling pathways) and Clozapine 5 (FDA approved antipsychotic drug inhibiting Efg1 pathway at Gpr1 G-protein-coupled receptor level). These molecules restrict the bud to hyphal growth in C. albicans and affect multiple signaling pathways that are intricate in the filamentation process which regulates the biofilm formation (Midkiff et al., 2011; Grald et al., 2012; Pierce et al., 2015b). By screening a library of 50,240 small molecules of inhibitors for yeast-to-hypha transition, Sm21 6 was identified as a novel small antifungal molecule that showed its efficacy in both in vivo oral candidiasis mouse model and in vitro system. The study also revealed that compound 6 when given to C. albicans strain led to reactive oxygen species (ROS) accumulation and mitochondrial dysfunction (Wong et al., 2014; Truong et al., 2018). In another study, Chrysazin 7 (1, 8-dihydroxyanthraquinone) and Alizarin 8 (1, 2-dihydroxyanthraquinone) an anthraquinone derivative, effectively inhibits biofilm formation in C. albicans. Their anti-biofilm activity was mainly due to a hydroxyl group (-OH) at C1 position. Unlike other commercially available drugs, alizarin was found to be a non-toxic compound. It downregulates the expression of various hyphal-specific and biofilm related genes like ALS3, ECE1, ECE2, and RBT1. Additionally, Chrysazin and Alizarin at 2 μg/ml adequately inhibited yeast to hyphal formation and increased the survivability of C. albicans infected C. elegans, thus, proving to be a strong candidate for future investigation as an antifungal agent (Manoharan et al., 2017b).
While performing high throughput screening in a chemical library (NOVACoreTM), about 20,000 small molecules were identified as a novel series of a Diazaspiro-decane and its structural analogs (9a-d). These molecules inhibit progressions of the virulence-associated with C. albicans, especially biofilm formation and filamentation, without affecting the overall growth or prompting the resistance in C. albicans. These compounds displayed a potent inhibitory activity when tested against murine models of oral candidiasis (Pierce et al., 2015a). In addition to all these, when 678 small molecules were screened from the chemical library by the group scientist against the invasive hyphal growth of the opportunistic human yeast C. albicans, it led to the discovery of two halogenated compounds (N1-(3,5-dichlorophenyl)-5-chloro-2-hydroxybenzamide) 10 and Niclosamide 11 which is the analog of salicylanilide, an FDA-approved anthelmintic in humans, both exhibiting an anti-filamentation and anti-biofilm activities against C. albicans (Garcia et al., 2018).
Another compound N-[3-(allyloxy)-phenyl]-4-methoxyben zamide 12 was recognized as the lead one showing inhibition against C. albicans filamentation and was found effective in both in vitro and in vivo study of C. albicans infected murine model. The whole transcriptomic analysis revealed a total of 618 genes that were up-regulated and 702 were down-regulated. It was observed that most of the down-regulated genes such as SAP5, ECE1, and ALS3 already well-characterized were associated with filamentation and virulency. In addition, some genes affect metal chelation and utilization (Romo et al., 2019).
Homology studies using Candida Genome Database showed that Candida accounts for type III 5-α-reductase, Dfg10p, and shares a 27% sequence identity and 41% similarity to the human type III 5-α-reductase, which were identified as a target for benign prostatic hyperplasia. Finasteride 13 was recognized as one of the potent inhibitors against type III 5-α-reductase. Studying its activity showed some promising results at dose ≥16 mg/liter. Finasteride alone was highly efficient in preventing filamentation of C. albicans and demonstrated synergy with FLC against in vitro urinary biofilm (Chavez-Dozal et al., 2014).
LaFleur group, while filtering through 120,000 small compounds from the NIH Molecular Libraries Small Molecule Repository, identified 1,3-benzothiazole 14, 14(a-c) and its scaffolds as an anti-filamentation drug which acts as an potentiate a known antifungal drug Clotrimazole by increasing its activity to >100 fold against C. albican biofilm isolates (LaFleur et al., 2011).
Enzymes
Potent inhibitors of essential microbial enzymes are significant growth inhibitors of C. albicans, a pathogenic fungi. The enzyme aspartate semialdehyde dehydrogenase (ASADH) is critical for the functioning of the biosynthetic pathway of aspartate in microbes and plants, an important step for the biosynthesis of other essential amino acids. Because the aspartate pathway is absent in humans, ASADH can be a promising new target for antifungal research. Deleting the ASD gene encoding for ASADH significantly decreases the survival of C. albicans, establishing this enzyme as essential for this organism (Dahal and Viola, 2018; Dahal et al., 2020).
The 1,4-Napthoquinone and its derivatives 15, 15 (c-e) did possess activity against clinical isolates of C. albicans derived from gynecological patients by inhibiting phenotypic changes in C. albicans. Additionally, some other studies also establish that dichloro-derivatives of 1,4 naphthoquinone i.e., 2-chloro 1,4-Naphthoquinone 15a and 2,3-dichloro naphthoquinone 15b are the inhibitors of ASADH (aspartate dehydrogenase) (Janeczko et al., 2018).
Secreted Aspartic Protease-2 enzymes (SAP2) of the C. albicans are one of the known classes of the virulent factor for localized and systemic infection. In C. albicans, ten distinct SAP genes (SAP1-10) were expressed in vitro and in vivo. The SAPs are essential for the fungal nutrition process and contribute to the fungal pathogenicity due to their critical participation in several stages of the infective process, including adhesion, invasion, and tissue damage and so on. Pepstatin and peptidomimetic are the known peptide inhibitors, but it is challenging to clinically synthesize them due to pharmacokinetic characteristics. So from the studies, compound 16, one of the derivatives of pepstatin, came out to be a potent inhibitor against 3 of the SAP2 present in fungi. Moreover, compound 16 and fluconazole when administered to FLC resistant mouse model infected with C. albicans showed synergistic effect by increasing the survival rate of the mouse by 50% (Cadicamo et al., 2013).
Targeting proteinases and phospholipases, which are vital to fungal invasion of host tissues and immunosuppression, Aneja et al. (2016) developed an s triazole series–amino acid hybrids. It was found that compounds 17 and 18 significantly reduce the secretion of proteinases and phospholipases in Candida spp. which are vital to fungal invasion of host tissues and immunosuppression. The study showed that on treatment with compound 17, proteinase secretion was decreased by 29, 22 and 23.5% in standard, FLC-sensitive and resistant strains of C. albicans, respectively, while at the same concentration, compound 18 decreased the proteinase secretion by 30, 33 and 17% against the respective strains. At similar concentrations in the same strains, compound 17 caused 40, 38, and 38% inhibition in phopholipase secretion whereas compound 18 decreased the secretions by 36, 27 and 38% respectively.
Efflux pump
One of the structural derivatives of cyclobutene-dione [3-(phenylamino)-4-{[1,3,3-trimethyl-2,3-dihydro-1H-indol-2-ylidene]methyl}-cyclobut-3-ene-1,2-dione] 19 was chemically synthesized and was identified as the puissant inhibitor of the efflux pumps residing in plasma membrane - Major Facilitator Superfamily (MFS) and ATP-binding cassette (ABC) transporters accountable for efflux pump-mediated drug resistance in the fungal pathogen C. albicans (Keniya et al., 2015).
Quorum Sensing Molecules
As already mentioned, quorum sensing plays an important role in mediating the formation of biofilm of C. albicans. One of the known quorum-sensing molecules released from Candida itself, “Farnesol” 20, is known to inhibit filamentation in C. albicans, which is a known virulence factor enhancing biofilm formation. From the previous studies it is known that the upregulation of the sterol biosynthetic pathway gene ERG and the efflux pump genes CDR and MDR are one of the major contributors to the azole tolerance in C. albicans. Therefore, through northern blot studies, it was examined that Farnesol down-regulates the expression of partial gene expression in ergosterol biosynthesis, Ras1-cAMP-Efg1 signaling cascade, HWP1 promoter, which encodes for hyphal –specific cell wall protein (Ramage et al., 2002; Yu et al., 2012; Dižová and Bujdáková, 2017). Further, it was observed that compound 20 is in synergism with already known antifungal drugs augmenting the accumulation of ROS, leading to early apoptosis in fungal cells (Sharma and Prasad, 2011). In a recent study, a bacterial quorum sensing quencher S8- Thiazolidinedione-8 21 has been identified as an effective small molecular inhibitor which significantly decreases biofilm formation by C. albicans. Additionally, it shows, anti-filamentation, as well as anti-adhesion activity at four to eight fold decreased MIC concentrations. Nevertheless, it down-regulates HWP1, ALS3, EAP1, HST7 and CPH transcription factors that play a key role in biofilm formation (Feldman et al., 2014). Another newly discovered Thiazolidinedione molecule- N-(oxazolylmethyl)-thiazolidinedione 22 scaffolds has been identified as a novel compound that inhibits C. albicans Als surface protein (Marc et al., 2018; Table 1).
High Throughput Screening of the Libraries
Around 400 heterogeneous drugs like molecules assembled by Medicines for Malaria Venture [MMV], Switzerland was screened with the motto to hasten the identification of medicinal molecules C. albicans biofilm. Three confirmed hits were obtained- MMV688768, MMV687807, and MMV687273, which exhibited activity against pre- biofilms. However, by additional exploration, it was observed that compound MMV688768- 2-methyl-3-[(4-methylpiperazin-1-yl)-thiophen-2-ylmethyl]-1H- indole 23 an anti-Schistosoma drugs are the best showing anti-biofilm activity against C. albicans, with concentrations as low as 3.12 μM (Vila and Lopez-Ribot, 2017).
Ebselen 24 an organoselenium compound, was screened out from the off-patent drugs using the Prestwick Chemical Library, a repurposing library of about 1,280 small molecules. Out of nine molecules, Ebselen illustrated 100% inhibition against multi-drug resistant species, Candida auris at concentration ≤ 2.5 μM (Wall et al., 2018). Furthermore, it elucidates its antifungal activity by accumulating reactive oxygen species (ROS) inside the fungal cells. Further, in vivo study of C. elegan model infected with C. albicans showed complete eradication of fungal load when treated with 8 μg/ml of ebselen (Thangamani et al., 2017). Additional screening of New Prestwick Chemical Library comprised of FDA-approved 1,200 off-patent drugs. Alexidine dihydrochloride 25 (AXD) and Thimerosal 26 showed more than 80% of inhibition mature biofilms. Subsequently, AXD showed antibiofilm potency C. albicans mouse model disseminated with fungal infection (Mamouei et al., 2018; Table 2).
Phenotypic Screening
Phenotypic screening refers to the nascent methodology for the biological screening of the chemical entities to assess their therapeutic effects. It is not a target- based strategy but involves detailed cell-based studies (Aulner et al., 2019). The phenotypic screening was done to identify different small molecules which depict antibiofilm and antifilamentation activity against C. albicans. This study leads to the identification of about 2293 compounds from the chemical library of the National Cancer Institute which were categorized into three sets- (i) NCI Natural set, Out of all the compounds present in this set six hits were confirmed against C. albicans biofilm formation. These include -Trichoderonin 27; Nanaomycin 28; Rapamycin 29; Anisomycin 30; Valinomycin 31 and Bacitracin 32. Three of these molecules (Trichoderonin, Nanaomycin, Rapamycin) showed inhibition of both filamentation and biofilm formation while the rest three showed inhibition against biofilm formation.
Furthermore in the next (ii)-NCI-Structural diversity set, in total, there were 12 hits out of which eight were identified as biofilm inhibitor. These compounds were-Phenanthroline Hydrochloride 33; 2-isoquinolin-2-ium-2-yl-1-phenanthren-3- ylethanone, iodide 34;Metanilamide (3-aminobenzenesul fonamide) 35; Mercury, (4-amino phenyl)(6-thioguano sinato- N7,S6)- 36; 2-[7-[3-(carboxymethyl)-5,10-dihydroxy-1-methyl- 6,9-dioxo-3,4-dihydro-1H-benzo[g] isochromen-7-yl]-5,10-dihy droxy-1-methyl-6,9-dioxo-3,4-dihydro-1H-benzo [g]isochro men-3-yl]acetic acid 37 are only biofilm inhibitors while Mercury,(2-aminio-1,9-dihydro-6H-purine-6-thionato-N7,S6) hexyl-,2-benzo[a]phenothiazin-12-yl-N,N-diethylethanamine 38; 17-[1-[2(dimethylamino)ethylamino]ethyl]-13-methyl-6,7,8, 9,11,12,14,15,16,17 decahydrocyclopenta[a]phenanthren-3-ol 39. Three of these compounds inhibited both filamentation and biofilm formation. From all these compounds related to 17- aminoestradiols, a Mercury containing organometallic was the most potent compound with IC50 value in the range of nanomolar concentration.
Next and last was (iii)-NCI-Challenge Set. In this, there were total 11 hits, of which 10 showed inhibition against C. albicans biofilm formation whereas only one compound inhibited filamentation transformation. Ten hits which were identified from these compounds displayed common inhibition against both biofilm and filament formation. These include Biofilm Inhibitor- Trichopolyn-B40, Vengicide (Unamycin B, Toyocamycin)41, 4Z-4-[[4-(dimethylamino)phenyl]methyli dene]-1-methyl-2-phenylpyrazolo[1,5-a]indol-1-ium-6-ol;trifluo romethanesulfonate42, Anisomycin43, Azetidinecarbo thioic acid, [1-(2-pyridinyl) ethylidene] hydrazide4. Additionally, compounds with both antifilamentation and antibiofilm activity are.- 6-Hydroxy-3-((methanesulfonyloxy) Methyl)-1- ((5,6,7-tri methoxyindol-2-yl) carbonyl)indoline45, Hydrazineca rbothioamide, N, N-dipropyl-2-(2-pyridinemethylene)-,(N,N,S) copper(II)chloridecomplex(SP-4-3)3;3-Azabicyclo[3.22]nonane-3-carboselenoicacid,[1-(2pyridinyl)ethyidene] hydrazide46, 2- hydroxyethyl-[(2R)-2-hydroxyheptadecyl]-dimethylazanium iodide 47, 1H-Azepine-1-carbothioic acid, hexa hydro-, [1-(2-pyridinyl) ethylidene]hydrazide48 (Pierce et al., 2014; Table 3).
Repurposing FDA Approved Drug Showing Synergistic Effect
Haloperidol 49 and its derivative (Benzocyclane derivative B10 49a) (Ji et al., 2020) and Bromoperidol 50 and its derivative are some of the known antipsychotic drugs that were repurposed and were utilized as anti-biofilm molecules. Haloperidol’s derivative exhibited inhibition of yeast to hyphal transition in resistant strain of C. albicans in synergism with FLC while it also down-regulated the expression of ERG11, and MDR1 genes are leading to cell membrane damage. Moreover, compound 49a showed significant decrease in the CFU/g from 7.63 to 7.29 in murine model of invasive candidiasis. Similarly, Bromoperidol and its derivative showed a synergistic effect in combination with different azoles. The mechanism of action is unknown but it does decrease the amount of azole administered at the time of treatment (Holbrook et al., 2017).
While looking for different antibiofilm drug groups, scientists looked for the Hsp 90 and Calcineruin inhibitors as it chemo sensitizes the fungal strains toward azole- as the first line of antifungal drugs. Piperazinyl quinolone 51 screened from MLPCN library had no antifungal activity alone, but, in combination with Azoles, it selectively inversed the fluconazole resistance in the clinical isolates of C. albicans (Youngsaye et al., 2011). Similarly, FK506 52 and Cyclosporine A 53, the other potential calcineruin inhibitors also illustrated the increase in the susceptibility of C. albicans toward antifungal drugs (Uppuluri et al., 2008; Cordeiro et al., 2014; Jia et al., 2016). Ribavirin 54, already known antiviral drug, was screened through Prestwick Chemical Library against FLC-resistant strains. It illustrated a fungistatic effect against MDR species of C. albicans and acted synergistically with MIC < 24.4 μg/mL (Yousfi et al., 2019).
While going through 1,600 compounds present in the drug-repositioning library, 8 hits were obtained. These are Hexachlorophene 55 (anti- topical anti-infective drug) (Siles et al., 2013), Pyrvinium pamoate 56 (an anti-helminthic drug), Artesunate 57 (antimalarial drug), Broxyquinoline 58 (antiseptic drug), Dihydroartemisinin 59 (antimalarial drug), Gentian violet 60 (antibacterial and antihelminthic drug), Bithionate disodium 61 (antiseptic), and Nitroxoline (Antibacterial). Out of these 8 hits, the first seven hits showed inhibition of mature C. albicans biofilm in combination with miconazole at sub-inhibitory concentrations. Further, studies were performed on the best-acknowledged potentiators, Hexachlorophene, pyrvinium pamoate, and artesunate. Mechanistic synergy was most pronounced for artesunate, an artemisinin homolog, which prompted the study of different structural homologs of Artemisinia. Thus, it was observed that biofilm inhibition in combination with Micanazole was not specific only to artesunate but also applicable to dihydroartemisinin and other artemisinin derivatives, indicating artemisinin as a potential antifungal molecule that can be further investigated to establish its overall potentials in the human health care system (De Cremer et al., 2015).
In another study, the library comprehending 1600 off-patent drugs was sifted for the compound that enhances in vitro activity of amphotericin B when used in combination against C. albicans biofilms. Out of 1600 compounds, the team found 50 hits, and from these 50 hits, only seven of them illustrated BIC50 < 100 μM for biofilm inhibition. Those compounds are as follow: Prochlorperazine edisylate 5.2 μM (62), Danthron 12 μM (63), Chlorprothixene hydrochloride 17 μM (64), Toremifene citrate 19.5 μM (65), Clorgiline hydrochloride 24 μM (66); Perhexiline maleate 39 μM (67) Dicyclomine hydrochloride 60 μM(68). Subsequently, all of these compounds showed a synergistic effect with caspofungin. Additionally, while studying tormifene citrate (65) for its synergistic effect on C. elegans infection model, it showed strong antifungal potency (Delattin et al., 2014). Diclofenac 69 a non -steroidal anti-inflammatory drug, increased the susceptibility of caspofungin toward the in vitro C. albicans biofilm. Furthermore, catheter retrieved from the animal model-rat when treated with combination therapy of caspofungin and diclofenac showed a significant reduction in the number of biofilm cells (Bink et al., 2012). Further, an off-patent antipsychotic drug Aripiprazole 70 was identified as a potent inhibitor of C. albicans which inhibited the early formation of pseudohyphal cells and mimicked standard azoles at higher concentrations showing different mechanisms of action (Rajasekharan et al., 2019; Table 4).
Natural Products
Arising fungal resistance ascribed to mutational changes has diversified the complexity fueling the thought processes along this line. To overcome this issue, we need to find some substantial solutions. For the past few decades, natural products have emerged as an essential source of antibacterial, antimalarial, and chemotherapeutic agents. Presently, around 60% of used drugs for cancer treatment are procured from natural products. Furthermore, the modification of natural products is one of the most common and productive methodologies to obtain novel therapeutic agents using medicinal chemistry (Zaki et al., 2019). Thus, targeting fungal biofilms by either natural derivatives or synthetic analogs could be a novel approach. Natural product screening has proved to be one of the promising strategies. Although anti-biofilm agents themselves might not kill the bacteria, they can make them more susceptible to conventional antibiotics and the action of the host immune system. The search for biofilm inhibitors has led to identifying a significant number of compounds of potential therapeutic use as biofilm inhibitors. A literature survey reveals that some of the most active anti-biofilm compounds discovered to date have been based upon the molecular scaffolds of natural products isolated from marine natural products and plant products (Fazly et al., 2013). As compiled by the World Health Organization, more than 21,000 plant species containing a vast array of biologically active compounds have been used worldwide in herbal medicines (Midkiff et al., 2011). Naturally occurring alcohols magnolol 71 and its isomer honokiol 72, carvacrol 73, thymol 74, and geraniol 75 have been identified as a potent inhibitor of both bacterial and C. albicans biofilms (de Castro et al., 2015; Magi et al., 2015; Sun et al., 2015; Sharma et al., 2016; Behbehani et al., 2017). Furthermore, when C. elegan was treated with 16 μg/ml of magnolol and honokiol, there was a decrease in the number of colony forming units (CFU) of C. albicans in the nematode which enhances its survival. Additionally, antibiofilm activity of these compounds 69 and 70 were related to Ras1-cAMP-Efg1 pathway of C. albicans (Sun et al., 2015). A Recent study shows that compound 70 and 71 are antifungal and induce ROS mediated programmed cell death in C. albicans (Sun et al., 2017; Niu et al., 2020). Saponins are one of the known natural products that bind to the ergosterol rather than cholesterol of C. albicans. This increases the susceptibility to photodynamic inactivation due to an increase in the permeability of photosensitizers by sapnonin. In one study, a total of 12 different saponins were identified as potential antifungal agents, but out of those 12, only two were selected. One was 76, which belongs to the aginoside family of saponins and the secone one 77 to the barrgeniol family of the saponins. Both of these compounds were potent antifungal agents with MIC 16 and 32 μg/ml, respectively. Furthermore, to study toxicity and efficacy of the compound, C. elegans and C. albicans models were used. In an in vivo study, compound 76 and 77 showed no sign of toxicity and conferred high percentage of nematode survival from upto 73% to 80%, respectively (Coleman et al., 2010).
Occasionally, other known naturally occurring compounds are found to be useful. The Carvone 78 and perillaledhyde 79 (McGeady et al., 2002; Tian et al., 2017; Moro et al., 2018) at very low concentration inhibit the filamentous formation of C. albicans. Furthermore, it was observed that at MIC of 4 μl/mL compound 77 increased the level of ROS, which activated the programmed cell death in C. albicans. Additionally, it equalizes the level of E-cadherins the epithelial barriers preventing which decreases in number due to invasion of pathogenic fungi (Tian et al., 2017; Qu et al., 2019). The Riccardin D80, 80a - macrocyclic bisbibenzyl isolated from Dumortiera hirsute chinese liverwort, exhibited an inhibitory effect on the biofilm formation of C. albicans by downregulating HWP1, ASL3, and EFG1 genes thus, inhibiting hyphal formation. Additionally, one of the semi-synthetic derivative of Riccardin D with a bromine atom attached to the arene ring showed better antifungal activity with MIC-2 μg/ml in comparison to Riccardin with MIC-16 μg/ml (Li et al., 2012; Sun et al., 2016). Another known compound is Emodin 81 (1, 3, 8−trihydroxy−6−methyl−anthraquinone) which is a natural secondary plant product, originally isolated from the rhizomes of Rheum palmatum. This compound suppressed the growth of the cells of reference and clinical C. albicans strains with minimal inhibitory and minimal fungicidal concentration values between 12.5 and 200 μg/mL, respectively, showing anti-virulent potential. Research showed that emodin added to C. albicans culture inhibited the phosphorylation of many cellular proteins, presumably, owing to the inhibition of protein kinase CK2. Notably, the enzyme isolated from the C. albicans cells was found to be susceptible to emodin with IC50 of 2.8 μg/mL, revealing that emodin was able to occupy the ATP−binding pocket of CK2 (Janeczko et al., 2017). In another finding, M. sylvestris root was also shown to inhibit C. albicans biofilm formation (Alizadeh et al., 2017). Studies on quorum sensing inhibition and the quest for QS inhibitors have shown plants to produce anti-QS substances. Several anti-QS methods have been used, including natural products from plant-based secondary metabolites (Berde et al., 2019). Carbohydrate derived fulvic acid (CHD-FA) 82 from pure form of fulvic acid is obtained from humic substances, also exhibits anti-biofilm activity against C. albicans (Sherry et al., 2012; Borghi et al., 2015). Plant alkaloid berberine 83 showed 91% biofilm inhibition against C. albicans in combination with Miconazole. Furthermore, it reduces the metabolic activity of early- stage biofilm formation in C. albicans. Individually, Berberine inactivates fungal biofilm at MIC range 0.98-31.25mg/mL (Wei et al., 2011). Resveratrol (3, 4, 5 -trihydroxystilbene) 84 is a phytoalexin, a known antimicrobial agent present in a wide range of dietary sources, including peanuts, plums, grapes and in red wines. A semisynthetic compound -EB487 84a was synthesized from resveratrol, which showed an antibiofilm and anti-preformed biofilm activity against C. albicans strain. Subsequently, the study revealed that resveratrol regulates its antifungal activity from early to late apoptosis stage. Furthermore, it increases the ROS concentration in fungal cells of C. albicans and modulates the activation of metacaspase release in response to mitochondrial dysfunction (Lee and Lee, 2015; Juin et al., 2019; Table 5).
Natural Extracts and Essential Oils
Ethanol extract of Tovomita krukovii led to the identification of few new xanthones like 1,3,5-trihydroxy-8-isoprenylxanthone 85a, 1,5,7-trihydroxy-8-isoprenylxanthone 85b, betulinic acid 86, and 3,4-dihydroxybenzoic acid 87 targeting secreted aspartic protease SAP2 of C. albicans with IC50 values 15 μg/ml, 25 μg/ml, 40 μg/ml, and 6.5 μg/ml, respectively (Zhang et al., 2002). Crude hydro alcoholic extract prepared through peel extract of Punica grantum presented strong inhibitory activity against C. albicans at MIC 3.9 μg/mL and led to the two-fold decrease in the concentration of FLC when used in combination (Endo et al., 2010). In certain studies, essential oils from plants and their compounds have been scrutinized as antifungal agents. The essential oil of Coriandrum sativum delineates fungicidal activity in a combination of amphotericin B, mainly targeting germ tube formation in C. albicans (Silva et al., 2011). According to the studies, Rumex root extract and Nepodin 88, a compound extracted from Rumex roots, were defined as anti-biofilm agents against C. albicans. It also increased the survivability in nematode infection model. Further, Nepodin also showed repressed expression of several genes related to bud to hyphal transition Like ECE1, UME6, HWP1, and HGT10, while the increase in the expression of quite a few transport genes (CDR4, TPO2, and CDR11) essential for phenotypic expression (Lee et al., 2019). Additionally while screening compounds from the crude extract of aerial parts of Waltheria indica, 10 quinoline alkaloids were identified as a potential molecule which inhibit biofilm formation in C. albicans: These include Waltherione N 89, (R)-Vanessine 90, Waltherione Q 91, 8-deoxoantidesmone 92, Antidesmone 93, Waltherione E 94, Waltherione G 95, Waltherione I 96, Waltherione J 97, Waltherione F 98 (Cretton et al., 2016; Table 6).
The Unexplored Brazilian Organic Propolis –BOP6 crude extract was characterized as an anti-inflammatory and anti-biofilm agent. It reduces the mortality rate by 30% in C. albicans infected sample with MIC ranging from 50 to 100 μg/mL while in combination with Amphotericin B, it decreases to 0.25 to 1.0 μg/mL (Nani et al., 2019). Through library screening with the information regarding fungal extract, it was revealed that Bionectria ochroleuca, an endophytic fungus. when cultivated on cereals, lead to the production of certain secondary metabolites-Polyketide glycosides 99. These Polyketide glycosides exhibited anti-biofilm activity against C. albicans and correspondingly showed a synergistic effect with Amphotericin B (Wang et al., 2014).
From HPLC, a different set of compounds were extracted from the crude dichloromethane extract from the roots of Swartzia simplex. In total 14 compounds were identified out of which only six compounds such as Simplexene A 100, (5S,10S)-11,15(R)-Dihydroxy,12 methoxyswartziarboreol G 101, Simplexene B 102, Simplexene D 103, 11,12-Dihydroxy-15, 16-dihydroswartziarboreol C104,11,12-Dihydroxy-8,11,13,15- cassatetraen-17,16-olide(11,12 Dihydroxyswartziarboreol C) 105 depicted strong biofilm inhibition against C. albicans (Favre-Godal et al., 2015; Table 7).
The crude extract of eucalyptus oil and its component 1, 8- cineole 106 showed antimicrobial activity against different microbes including C. albicans biofilm. It showed inhibitory effect alone and also in combination with chlorhexidine (Hendry et al., 2009; Simsek and Duman, 2017). While studying different components of essential oils as a treatment against invasive candidiasis, some terpenic derivatives showed promising results. These include carvacrol 73, geraniol 75, carvone 78, terpinen-4-ol107, linalool 108, menthol 109, α-terpineol 110, nerol 111, isopulegol 112, menthone 113, and α-thujone 114. On further study, tyrosol 115 – (a phenethyl alcohol), tyrosine derivative and eugenol- phenylpropanoid 116 both showed a strong inhibitory effect against C. albicans biofilm (Marcos-Arias et al., 2011; Raut et al., 2013). Furthermore, tyrosol 115 showed antibiofilm activity when applied exogenously at millimolar concentration. However, at micromolar concentration this compound stimulates hyphal production during the intial stage of C. albicans biofilm formation. This inhibition of biofilm formation by the exogenous addition of quorum sensing molecule usually occurs due to disruption in the qurom sensing mechanisms or due to the limitation of yeast adhesion on medical devices (Monteiro et al., 2015; Sebaa et al., 2019).
Epigallocatechin-3-gallate 117-a Polyphenol compound from green tea extract and its analogs depicted a 75% reduction in the mature C. albicans biofilm (Evensen and Braun, 2009).
The α-Longipinene 118, a significant component of Helichrysum oil and Cascarilla bark oil, inhibited the C. albicans biofilm formation without affecting planktonic cell growth and also exhibited a decrease in the virulence in C. elegans model (Manoharan et al., 2017a). Further, the compounds Glabridin 119, Licochalcone A 120, and Glycyrrhizic acid 121 derived from Licorice- a natural compound extracted from the root of the plant Glycyrrhiza glabra showed biofilm inhibition against C. albicans. It was found that Glabridin, Licochalcone A showed 35-60% of biofilm inhibition while they inhabited more than 80% hyphal growth. Furthermore, they displayed a synergistic effect with Nystatin at sub-inhibitory concentration (Messier and Grenier, 2011). Another successful study on the essential oils obtained from Thyme species – T. camphoratus and T. carnosus, earlier used in Portuguese as an antimicrobial therapy, lead to the discovery of different molecules.
In Thymus carnosus oil, high aggregates of Camphene and Borneol were present while Thymus camphoratus oil was rich in 1,8-cineole and α-pinene. Both the oils were highly effective against C. albicans biofilm and germ tube formation with very little toxicity (Alves et al., 2019). Another category of essential oils Syzygium aromaticum and Cymbopogon citratus, largely used in Asia, especially in India, for the treatment of inflammation and skin infection, displayed a potent anti-biofilm activity against C. albicans strain (Sajjad et al., 2012). Furthermore, Safranal 122 and its thiosemicarbazone 122a derivative, the bioactive compounds obtained from Crocus sativa when added together in C. albicans culture, acted as a potent inhibitor of biofilm and germ tube formation at 32 times less concentration than MIC (Carradori et al., 2016; Table 8).
Work on small molecular inhibitors of plant origin, several molecules showed high to moderate activity toward C. albicans biofilm. These compounds like Cinnamaldehyde123, Piperidine 124, Citral 125, Furfuradehyde 126, and Indole 127 showed inhibitory activity toward ergosterol biosynthesis at varying concentrations (Rajput and Karuppayil, 2013). A recent study revealed that compound 123 and 124 regulated antifungal activity against C. albicans by ROS mediated apoptosis (Chen et al., 2019; Thakre et al., 2020). Similarly, another set of C. albicans biofilm inhibitor Shearinines D and E (128,129) derived from Penicillum species showed yeast to hyphal blockage. However, it was observed that these compounds are more potent against early biofilm formation than the mature biofilm (You et al., 2013). Gymnemic acid 130, a small molecular inhibitor obtained from the leaves of the plant Gymnema sylvestre, showed its activity against dual-species S. gordonii and C. albicans together, leading to biofilm formation in the mouth. It decreases the amount of e-DNA present in biofilm. It also inhibits the adhesive property of C. albicans by inhibiting an enzyme glyceraldehyde-3-phosphate dehydrogenase, which is thought to be responsible for the display of adhesion property in C. albicans (Veerapandian and Vediyappan, 2019). Similarly, Waikiloid A 131- a prenylated indole alkaloid and polyketide metabolite Waikialide A132 obtained from a Hawaiian Aspergillus spp. both acted as a potent biofilm inhibitor against C. albicans with a IC50 - 1.4 μM and 32.4 μM, respectively (Wang et al., 2012).
Myriocin 133 is a metabolite of the Isaria sinclairii - a pathogenic insect fungus. It disrupts the formation of sphingolipids as it is a structural analog of sphingosine precursors. It works very effectively in combination with known antifungal drugs like amphotericin and fluconazole, reducing the mortality rate from 26% to 0%, respectively. Additionally, G.mellonella larva infected with C. albicans showed a decrease in mortality rate when treated with myriocin. Furthermore, study of compound 133 indicated that it regulates C. albicans pathogenesis through NF-κB pathway, G protein coupled receptor and immunity (Melo et al., 2013). Acetylcholine 134, a known soluble protein that has a neuronal function, also displays strong anti-biofilm activity against C. albicans. Along with this, it also reduces the damage induced due to inflammation in the host. Additionally, in vivo study of acetylcholine on G. mellonella larvae showed robust antifungal potency with a decrease in mortality rate from 80 to 100% to 25%. Further, compound 134 acts by promoting the rapid cellular immune response in host cells (Rajendran et al., 2015).
Garcinol 135 and Xanthochymol 136, isoprenylated benzophenones obtained from Garcinia xanthochymus fruits, show antibiofilm activity against C. albicans by inducing programmed cell death in early biofilms without actually affecting the growth and viability of planktonic cells. Sampangine B 137, 137(a-b) a naturally derived azaoxoporphine alkaloid and its derivatives showed potent activity against C. albicans biofilms and yeast to hyphal formation. It was found to show antifungal activity by inducing the accumulation of ROS and subsequent heme dysfunction. Moreover, it showed strong in vivo antifungal activity with low cytotoxicity in nematode model of C. albicans (Wu et al., 2017; Table 9).
Recent Advances to Inhibit C. albicans Biofilm Formation
Throughout this article, we have seen different strategies and methods to combat antifungal drug resistance. However, after all the efforts and attempts we are still stuck at a point where biofilm formation is difficult to treat with both non-antifungal and antifungal drugs because of the poor penetration and non-specificity of the drugs. Therefore, to address this issue, researchers are working on finding ways to increase the penetration of drugs into the extracellular matrix of biofilm. Over the last few years, different metal nanoparticles have emerged as prospective candidates to treat microbial infections pertaining to their strong potential as antimicrobial agents. In this context, different research groups have initiated the exploration to identify the antibiofilm activity of different nanomaterials against C. albicans (Shukla, 2020).
The usage of silver as an antimicrobial agent spans a century. Silver was used as a disinfectant by Greeks and Romans to decontaminate their water and food supplies. Silver was also used in ancient times to treat burns and wounds (Alexander, 2009; Barillo and Marx, 2014; Adhya et al., 2015). Studying silver nanoparticles against biofilms has gained immense recognition. Silver nanoparticles exert an inhibitory effect against fungal biofilms by damaging cell wall mostly by distortion and disruption of the outer surface of the fungal cell wall (Lara et al., 2015). In subsequent experiments, AgNPs when used to functionalize medical and environmental surfaces, demonstrated biofilm inhibition (>50%) at relatively low concentrations (2.3 to 0.28 ppm) (Lara et al., 2020). Combination therapy of Fluconazole with silver nanoparticles has also shown a substantial decrease in the MIC (Longhi et al., 2016). The resistance to fluconazole (FLC) in C. albicans is strongly associated with overexpression of genes encoding efflux pumps or lanosterol 14α-demethylase (Sagatova et al., 2016). Subsequently, with the disruption of the cell wall and cytoplasmic membrane of C. albicans by AgNPs, these silver particles cause an increase in reactive oxygen species and hydroxyl radical production, which can also contribute to cell membrane damage (Hwang et al., 2012; Monteiro et al., 2012). Recently, researchers have identified bismuth oxide (BiO3) nanoparticles with potent antimycotic activity against C. albicans growth. Moreover, when these nanoparticles were compared to commercially available antifungal drugs like chlorhexidine, nystatin, they completely eradicated the biofilm formation (Hernandez-Delgadillo et al., 2013). In another study, magnesium oxide nanoparticles (MgO NP) were used to investigate their antifungal and antibiofilm property against C. albicans. The study revealed that MgO nanoparticles effectively inhibited C. albicans biofilm formation. Furthermore, adhesion experiments showed that MgO NPs repressed the preliminary adhesion of Candida albicans (Kong et al., 2020). In another study, when gold nanoparticles (Au-NP) were tested on the biofilms formed due to C. albicans, these particles showed robust inhibitory activity. Additionally, these Au-NPs increased the host immune response activity against these pathogenic organisms (Yu et al., 2016; Nani et al., 2019). From previous studies, chitostan and its nanoparticle (ChNPs) were already known for their antifungal property (Ing et al., 2012; Mohammed et al., 2017; Gondim et al., 2018). Therefore, to establish its antibiofilm activity, ChNPs were investigated, and it was found that ChNPs significantly inhibited the biofilm formation causing very less changes in the acrylic resin surface (Gondim et al., 2018). Likewise, another study conducted with selenium nanoparticles (Se-NP) showed strong inhibition on Candida biofilm formation as these particles out compete sulfur in biological process due to their similar chemical properties. Also, these nanoparticles enter into the cell to shrink and disrupt the outer membrane structure of C. albicans cell (Guisbiers et al., 2017).
The titanium oxide nanoparticles (Ti-NP) when scrutinized for their antifungal property showed strong antifungal activity against planktonic form of C. albicans. They also depicted robust potential against biofilm formation (Haghighi et al., 2013). In addition, zinc oxide nanoparticles (ZnO-NPs) used on Candida biofilm showed a decrease in the biofilm formation by abot 62%-85% at varied concentration of 125 ppm to 250 ppm respectively. The ZnO-NPs suggested to inhibit hyphae formation in C. albicans by production of reactive oxygen species in a dose-dependent manner (Hosseini et al., 2018; Golipour et al., 2019; Mahamuni-Badiger et al., 2020). Additionally, another study involving copper oxide nanoparticles (Cu2ONP and CuONP) showed potent inhibitory action against biofilm formation by C. albicans. The study revealed that copper oxide nanoparticles constrain the yeast for hypae transformation. Also CuO-NP elicited the reactive oxygen species while Cu2ONP pronounced the membrane damage in C. albicans. In comparison to both types of copper oxide nanoparticles, CuONP was more stable and depicted better antifungal activity in comparison to Cu2O-NP (Rasool, 2019; Padmavathi et al., 2020). In another study, iron-oxide nanoparticles (Fe3O4 NPs) were investigated on C. albicans biofilm which showed 87.2-100% inhibition based on their particle size which was 100 ppm to 200 pm respectively (Seddighi et al., 2017).
The nitric oxide nanoparticles (NO-NP) showed strong potential as an antifungal agent by hindering the extracellular matrix and biofilm formation on the surface of biomaterials. It was found that NO-NPs also decrease the metabolic activity of Candida cells both in vitro and in vivo (Ahmadi et al., 2016).
Concluding Remarks and Future Perspective
The high mortality and morbidity rate owing to C. albicans biofilm infection is a big challenge in medical mycology. Since the formation of biofilm and biomaterial infections is a progressively alarming problem, this warrants the development of new antifungal agents and the search for newer targets. This review elucidates the pathogenic foundation and molecular mechanism of C. albicans biofilms over antifungal drug resistance. A plethora of studies by several scientific groups and investigators have delivered essential knowledge regarding the pathogenesis associated with biofilm formation. These useful insights can represent an optimal starting point to find new therapeutic strategies related to drug resistance and mechanistic signals that govern C. albicans biofilm formation. Recent advances on different transcription factors, quorum sensing molecules, host response to adhesion, change in efflux pumps, enzymes, bud to hyphal transition, and change in lipid profile have broadened the knowledge of the complex mechanism underlying the biofilm resistance. Moreover, the development of new biomaterials with anti-adhesive properties, anti- infective lock therapies, high throughput phenotypic screening of small-molecule inhibitors, discovery and repurposing of naturally known compounds are under scrutiny. For medical instruments, a fine coating of nanomaterial may inhibit bacterial accumulation and biofilm formation. Recently, different metal nanoparticles have also emerged as antibiofilm agents against C. albicans and gaining momentum. Furthermore, different combinational therapies are harnessed for antibiofilm activity. Yet, the target-based approach is the need of the hour. For this multifaceted biofilm, the complex should be extensively studied to target different loops of phenotypic character change as they play a major role in biofilm formation. The detailed electron microscopic studies using both transmission electron microscopy (TEM) and scanning electron microscope (SEM) with much greater resolution may add additional repertoire to our knowledge. Yet another approach may be the use of some engineered enzymes that do not allow colony formation and inhibit in turn the biofilm formation. Any compound used for this purpose may be empirically optimized for its dose, sensitivity, and efficacy before a search for a target is mounted.
Given the diverse strategies to combat antifungal resistance, there is a hope that specific target-based drugs will be added to the arsenal in our fight against C. albicans biofilm formation in the not too distant future.
Author Contributions
All authors contributed equally and approved the final version of the manuscript.
Conflict of Interest
The authors declare that the research was conducted in the absence of any commercial or financial relationships that could be construed as a potential conflict of interest.
Acknowledgments
MFA and AH acknowledge the generous support from Research Supporting Project (No. RSP-2021-122) by King Saud University, Riyadh, Kingdom of Saudi Arabia.
References
Adhya, A., Bain, J., Dutta, G., Hazra, A., Majumdar, B., Ray, O., et al. (2015). Healing of burn wounds by topical treatment: a randomized controlled comparison between silver sulfadiazine and nano-crystalline silver. J. Basic Clin. Pharm. 6:29. doi: 10.4103/0976-0105.145776
Ahmadi, M. S., Lee, H. H., Sanchez, D. A., Friedman, A. J., Tar, M. T., Davies, K. P., et al. (2016). Sustained nitric oxide-releasing nanoparticles induce cell death in Candida albicans yeast and hyphal cells, preventing biofilm formation in vitro and in a rodent central venous catheter model. Antimicrob. Agents Chemother. 60, 2185–2194. doi: 10.1128/AAC.02659-15
Alexander, J. W. (2009). History of the medical use of silver. Surg. Infect. 10, 289–292. doi: 10.1089/sur.2008.9941
Alim, D., Sircaik, S., and Panwar, S. L. (2018). The significance of lipids to biofilm formation in Candida albicans: an emerging perspective. J. Fungi 4:140. doi: 10.3390/jof4040140
Alizadeh, F., Khodavandi, A., and Faraji, F. S. (2017). Malva sylvestris inhibits Candida albicans biofilm formation. J. Herbmed. Pharmacol. 6, 62–68.
Alonso, B., Pérez-Granda, M. J., Rodríguez-Huerta, A., Rodríguez, C., Bouza, E., and Guembe, M. (2018). The optimal ethanol lock therapy regimen for treatment of biofilm-associated catheter infections: an in-vitro study. J. Hosp. Infect. 100, e187–e195. doi: 10.1016/j.jhin.2018.04.007
Alves, M., Gonçalves, M. J., Zuzarte, M., Alves-Silva, J. M., Cavaleiro, C., Cruz, M. T., et al. (2019). Unveiling the antifungal potential of two Iberian thyme essential oils: effect on C. albicans germ tube and preformed biofilms. Front. Microbiol. 10:446. doi: 10.3389/fphar.2019.00446
Aneja, B., Irfan, M., Kapil, C., Jairajpuri, M. A., Maguire, R., Kavanagh, K., et al. (2016). Effect of novel triazole-amino acid hybrids on growth and virulence of Candida species: in vitro and in vivo studies. Org. Biomol. Chem. 14, 10599–10619. doi: 10.1039/c6ob01718e
Aslanyan, L., Sanchez, D., Valdebenito, S., Eugenin, E., Ramos, R., and Martinez, L. (2017). The crucial role of biofilms in Cryptococcus neoformans survival within macrophages and colonization of the central nervous system. J. Fungi 3:10. doi: 10.3390/jof3010010
Aulner, N., Danckaert, A., Ihm, J., Shum, D., and Shorte, S. L. (2019). Next-generation phenotypic screening in early drug discovery for infectious diseases. Trends Parasitol. 35, 559–570. doi: 10.1016/j.pt.2019.05.004
Barillo, D. J., and Marx, D. E. (2014). Silver in medicine: a brief history BC 335 to present. Burns 40, S3–S8. doi: 10.1016/j.burns.2014.09.009
Behbehani, J., Shreaz, S., Irshad, M., and Karched, M. (2017). The natural compound magnolol affects growth, biofilm formation, and ultrastructure of oral Candida isolates. Microb. Pathog. 113, 209–217. doi: 10.1016/j.micpath.2017.10.040
Ben-Ami, R., Olshtain-Pops, K., Krieger, M., Oren, I., Bishara, J., Dan, M., et al. (2012). Antibiotic exposure as a risk factor for fluconazole-resistant Candida bloodstream infection. Antimicrob. Agents Chemother. 56, 2518–2523. doi: 10.1128/AAC.05947-11
Berde, C. V., Salvi, S. P., Rawool, P. P., Prathyusha, A. M. V. N., and Berde, V. B. (2019). “Role of medicinal plants and endophytic bacteria of medicinal plants in inhibition of biofilm formation: interference in quorum sensing,” in Implication of Quorum Sensing and Biofilm Formation in Medicine, ed. P. Bramhachari (Singapore: Springer).
Berger, A. (2000). Science commentary: Th1 and Th2 responses: what are they? BMJ 321, 424–424. doi: 10.1136/bmj.321.7258.424
Bink, A., Kucharíková, S., Neirinck, B., Vleugels, J., Van Dijck, P., Cammue, B. P. A., et al. (2012). The nonsteroidal antiinflammatory drug diclofenac potentiates the in vivo activity of caspofungin against Candida albicans biofilms. J. Infect. Dis. 206, 1790–1797. doi: 10.1093/infdis/jis594
Bongomin, F., Gago, S., Oladele, R. O., and Denning, D. W. (2017). Global and multi-national prevalence of fungal diseases—estimate precision. J. Fungi 3:57. doi: 10.3390/jof3040057
Bookstaver, P. B., Rokas, K. E. E., Norris, L. B., Edwards, J. M., and Sherertz, R. J. (2013). Stability and compatibility of antimicrobial lock solutions. Am. J. Heal. Pharm. 70, 2185–2198. doi: 10.2146/ajhp120119
Borghi, E., Borgo, F., and Morace, G. (2016). Fungal biofilms: update on resistance. Adv Exp Med Biol. 931, 37–47. doi: 10.1007/5584_2016_7
Borghi, E., Morace, G., Borgo, F., Rajendran, R., Sherry, L., Nile, C., et al. (2015). New strategic insights into managing fungal biofilms. Front. Microbiol. 6:1077. doi: 10.3389/fmicb.2015.01077
Branzk, N., and Papayannopoulos, V. (2013). Molecular mechanisms regulating NETosis in infection and disease. Semin. Immunopathol. 35, 513–530. doi: 10.1007/s00281-013-0384-6
Cadicamo, C. D., Mortier, J., Wolber, G., Hell, M., Heinrich, I. E., Michel, D., et al. (2013). Design, synthesis, inhibition studies, and molecular modeling of pepstatin analogues addressing different secreted aspartic proteinases of Candida albicans. Biochem. Pharmacol. 85, 881–887. doi: 10.1016/j.bcp.2012.12.008
Carradori, S., Chimenti, P., Fazzari, M., Granese, A., and Angiolella, L. (2016). Antimicrobial activity, synergism and inhibition of germ tube formation by Crocus sativus-derived compounds against Candida spp. J. Enzyme Inhib. Med. Chem. 31, 189–193. doi: 10.1080/14756366.2016.1180596
Cavalheiro, M., and Teixeira, M. C. (2018). Candida biofilms: threats, challenges, and promising strategies. Front. Med. 5:28. doi: 10.3389/fmed.2018.00028
Chaffin, W. L., López-Ribot, J. L., Casanova, M., Gozalbo, D., and Martínez, J. P. (1998). Cell wall and secreted proteins of Candida albicans: identification, function, and expression. Microbiol. Mol. Biol. Rev. 62, 130–180. doi: 10.1128/mmbr.62.1.130-180.1998
Chandra, J., Patel, J. D., Li, J., Zhou, G., Mukherjee, P. K., Mccormick, T. S., et al. (2005). Modification of surface properties of biomaterials influences the ability of Candida albicans to form biofilms. Appl. Environ. Microbiol. 71, 8795–8801. doi: 10.1128/AEM.71.12.8795
Chavez-Dozal, A. A., Lown, L., Jahng, M., Walraven, C. J., and Lee, S. A. (2014). In vitro analysis of finasteride activity against candida albicans urinary biofilm formation and filamentation. Antimicrob. Agents Chemother. 58, 5855–5862. doi: 10.1128/AAC.03137-14
Chen, L., Wang, Z., Liu, L., Qu, S., Mao, Y., Peng, X., et al. (2019). Cinnamaldehyde inhibits Candida albicans growth by causing apoptosis and its treatment on vulvovaginal candidiasis and oropharyngeal candidiasis. Appl. Microbiol. Biotechnol. 103, 9037–9055. doi: 10.1007/s00253-019-10119-3
Chinnici, J., Yerke, L., Tsou, C., Busarajan, S., Mancuso, R., Sadhak, N. D., et al. (2019). Candida albicans cell wall integrity transcription factors regulate polymicrobial biofilm formation with Streptococcus gordonii. PeerJ 7:e7870. doi: 10.7717/peerj.7870
Coleman, J. J., Okoli, I., Tegos, G. P., Holson, E. B., Wagner, F. F., Hamblin, M. R., et al. (2010). Characterization of plant-derived saponin natural products against Candida albicans. ACS Chem. Biol. 5, 321–332. doi: 10.1021/cb900243b
Cordeiro, R. A., Macedo, R. B., Teixeira, C. E. C., Marques, F. J. F., Bandeira, T. J. P. G., Moreira, J. L. B., et al. (2014). The calcineurin inhibitor cyclosporin A exhibits synergism with antifungals against Candida parapsilosis species complex. J. Med. Microbiol. 63(Pt 7), 936–944. doi: 10.1099/jmm.0.073478-0
Cretton, S., Dorsaz, S., Azzollini, A., Favre-godal, Q., Marcourt, L., Ebrahimi, S. N., et al. (2016). Antifungal quinoline alkaloids from Waltheria indica. J. Nat. Prod. 79, 300–307. doi: 10.1021/acs.jnatprod.5b00896
Dahal, G. P., Launder, D., McKeone, K. M. M., Hunter, J. P., Conti, H. R., and Viola, R. E. (2020). Aspartate semialdehyde dehydrogenase inhibition suppresses the growth of the pathogenic fungus Candida albicans. Drug Dev. Res. 81, 736–744. doi: 10.1002/ddr.21682
Dahal, G. P., and Viola, R. E. (2018). A fragment library screening approach to identify selective inhibitors against an essential fungal enzyme. SLAS Discov. 23, 520–531. doi: 10.1177/2472555218767844
de Castro, R. D., de Souza, T. M., Bezerra, L. M., Ferreira, G. L., Costa, E. M., and Cavalcanti, A. L. (2015). Antifungal activity and mode of action of thymol and its synergism with nystatin against Candida species involved with infections in the oral cavity: an in vitro study. BMC Complement. Altern. Med. 15:417. doi: 10.1186/s12906-015-0947-2
De Cremer, K., Lanckacker, E., Cools, T. L., Bax, M., De Brucker, K., Cos, P., et al. (2015). Artemisinins, new miconazole potentiators resulting in increased activity against Candida albicans biofilms. Antimicrob. Agents Chemother. 59, 421–426. doi: 10.1128/AAC.04229-14
Delattin, N., De Brucker, K., Vandamme, K., Meert, E., Marchand, A., Chaltin, P., et al. (2014). Repurposing as a means to increase the activity of amphotericin B and caspofungin against Candida albicans biofilms. J. Antimicrob. Chemother. 69, 1035–1044. doi: 10.1093/jac/dkt449
Demuyser, L., Jabra-Rizk, M. A., and van Dijck, P. (2014). Microbial cell surface proteins and secreted metabolites involved in multispecies biofilms. Pathog. Dis. 70, 219–230. doi: 10.1111/2049-632X.12123
Dižová, S., and Bujdáková, H. (2017). Properties and role of the quorum sensing molecule farnesol in relation to the yeast Candida albicans. Pharmazie 72, 307–312. doi: 10.1691/ph.2017.6174
Douglas, L. J. (2002). Medical importance of biofilms in Candida infections. Rev. Iberoam. Micol. 19, 139−143.
Douglas, L. J. (2003). Candida biofilms and their role in infection. Trends Microbiol. 11, 30–36. doi: 10.1016/S0966-842X(02)00002-1
Endo, E. H., Cortez, D. A., Ueda-Nakamura, T., Nakamura, C. V., Prado, B., and Filho, D. (2010). Potent antifungal activity of extracts and pure compound isolated from pomegranate peels and synergism with fluconazole against Candida albicans. Res. Microbiol. 161, 534–540. doi: 10.1016/j.resmic.2010.05.002
Erb-Downward, J. R., and Noverr, M. C. (2007). Characterization of prostaglandin E2 production by Candida albicans. Infect. Immun. 75, 3498–3505. doi: 10.1128/IAI.00232-07
Evensen, N. A., and Braun, P. C. (2009). The effects of tea polyphenols on Candida albicans?: inhibition of biofilm formation and proteasome inactivation. Can. J. Microbiol. 55, 1033–1039. doi: 10.1139/W09-058
Favre-Godal, Q., Dorsaz, S., Queiroz, E. F., Marcourt, L., Ebrahimi, S. N., Allard, P-M., et al. (2015). Anti- candida cassane-type diterpenoids from the root bark of swartzia simplex. J. Nat. Prod. 78, 2994–3004. doi: 10.1021/acs.jnatprod.5b00744
Fazly, A., Jain, C., Dehner, A. C., Issi, L., Lilly, E. A., Ali, A., et al. (2013). Chemical screening identifies filastatin, a small molecule inhibitor of Candida albicans adhesion, morphogenesis, and pathogenesis. Proc. Natl. Acad. Sci. U.S.A. 110, 13594–13599. doi: 10.1073/pnas.1305982110
Feldman, M., Al-Quntar, A., Polacheck, I., Friedman, M., and Steinberg, D. (2014). Therapeutic potential of thiazolidinedione-8 as an antibiofilm agent against Candida albicans. PLoS One 9:e93225. doi: 10.1371/journal.pone.0093225
Finkel, J. S., and Mitchell, A. P. (2011). Genetic control of Candida Albicans biofilm development. Nat. Rev. Microbiol. 9, 109–118. doi: 10.1109/isdrs.2005.1596138
Fleming, D., Chahin, L., and Rumbaugh, K. (2017). Glycoside hydrolases degrade polymicrobial bacterial biofilms in wounds. Antimicrob. Agents Chemother. 61:e01998-16. doi: 10.1128/AAC.01998-16
Fourie, R., Ells, R., Swart, C. W., Sebolai, O. M., Albertyn, J., and Pohl, C. H. (2016). Candida albicans and Pseudomonas aeruginosa interaction, with focus on the role of eicosanoids. Front. Physiol. 7:64. doi: 10.3389/fphys.2016.00064
Garcia, C., Burgain, A., Chaillot, J., Pic, É, Khemiri, I., and Sellam, A. (2018). A phenotypic small-molecule screen identifies halogenated salicylanilides as inhibitors of fungal morphogenesis, biofilm formation and host cell invasion. Sci. Rep. 8:11559. doi: 10.1038/s41598-018-29973-8
Golipour, F., Habibipour, R., and Moradihaghgou, L. (2019). Investigating effects of superparamagnetic iron oxide nanoparticles on Candida albicans biofilm formation. Med. Lab. J. 13, 44–50. doi: 10.29252/mlj.13.6.44
Gondim, B. L. C., Castellano, L. R. C., de Castro, R. D., Machado, G., Carlo, H. L., Valença, A. M. G., et al. (2018). Effect of chitosan nanoparticles on the inhibition of Candida spp. biofilm on denture base surface. Arch. Oral Biol. 94, 99–107. doi: 10.1016/j.archoralbio.2018.07.004
Grald, A., Yargosz, P., Case, S., Shea, K., and Johnson, D. I. (2012). Small-molecule inhibitors of biofilm formation in laboratory and clinical isolates of Candida albicans. J. Med. Microbiol. 61, 109–114. doi: 10.1099/jmm.0.034124-0
Gristina, A. G., Naylor, P., and Myrvik, Q. (1988). Infections from biomaterials and implants: a race for the surface. Med. Prog. Technol. 14, 205–224.
Guisbiers, G., Lara, H. H., Mendoza-Cruz, R., Naranjo, G., Vincent, B. A., Peralta, X. G., et al. (2017). Inhibition of Candida albicans biofilm by pure selenium nanoparticles synthesized by pulsed laser ablation in liquids. Nanomed. Nanotechnol. Biol. Med. 13, 1095–1103. doi: 10.1016/j.nano.2016.10.011
Gulati, M., and Nobile, C. J. (2016). Candida albicans biofilms: development, regulation, and molecular mechanisms. Microbes Infect. 18, 310–321. doi: 10.1016/j.micinf.2016.01.002
Haghighi, F., Mohammadi, S. R., Mohammadi, P., Hosseinkhani, S., and Shidpour, R. (2013). Antifungal Activity of TiO 2 nanoparticles and EDTA on Candida albicans biofilms. Orig. Artic. Infect. Epidemiol. Med. 1, 33–38.
Harriott, M. M., and Noverr, M. C. (2010). Ability of Candida albicans mutants to induce Staphylococcus aureus vancomycin resistance during polymicrobial biofilm formation. Antimicrob. Agents Chemother. 54, 3746–3755. doi: 10.1128/AAC.00573-10
Hawser, S. P., and Douglas, L. J. (1995). Resistance of Candida albicans biofilms to antifungal agents in vitro. Antimicrob. Agents Chemother. 39, 2128–2131. doi: 10.1128/AAC.39.9.2128
Hendry, E. R., Worthington, T., Conway, B. R., and Lambert, P. A. (2009). Antimicrobial efficacy of eucalyptus oil and 1,8-cineole alone and in combination with chlorhexidine digluconate against microorganisms grown in planktonic and biofilm cultures. J. Antimicrob. Chemother. 64, 1219–1225. doi: 10.1093/jac/dkp362
Hernandez-Delgadillo, R., Velasco-Arias, Martinez-Sanmiguel, Diaz, D., Zumeta-Dubé, I., Cabral-Romero, C., et al. (2013). Bismuth oxide aqueous colloidal nanoparticles inhibit Candida albicans growth and biofilm formation. Int. J. Nanomed. 8, 1645–1652. doi: 10.2147/IJN.S38708
Holbrook, S. Y. L., Garzan, A., Dennis, E. K., Shrestha, S. K., and Garneau-Tsodikova, S. (2017). Repurposing antipsychotic drugs into antifungal agents: synergistic combinations of azoles and bromperidol derivatives in the treatment of various fungal infections. Eur. J. Med. Chem. 139, 12–21. doi: 10.1016/j.ejmech.2017.07.030
Hosseini, S. S., Ghaemi, E., and Koohsar, F. (2018). Influence of ZnO nanoparticles on Candida albicans isolates biofilm formed on the urinary catheter. Iran. J. Microbiol. 10, 424–432.
Hwang, I., Lee, J., Hwang, J. H., Kim, K., and Lee, D. G. (2012). Silver nanoparticles induce apoptotic cell death in Candida albicans through the increase of hydroxyl radicals. FEBS J. 6, 1327–1338. doi: 10.1111/j.1742-4658.2012.08527.x
Ing, L. Y., Zin, N. M., Sarwar, A., and Katas, H. (2012). Antifungal activity of chitosan nanoparticles and correlation with their physical properties. Int. J. Biomater. 2012, 1–9. doi: 10.1155/2012/632698
Janeczko, M., Kubiński, K., Martyna, A., Muzyczka, A., Boguszewska-Czubara, A., Czernik, S., et al. (2018). 1,4-Naphthoquinone derivatives potently suppress Candida albicans growth, inhibit formation of hyphae and show no toxicity toward zebrafish embryos. J. Med. Microbiol. 67, 598–609. doi: 10.1099/jmm.0.000700
Janeczko, M., Masłyk, M., Kubiński, K., and Golczyk, H. (2017). Emodin, a natural inhibitor of protein kinase CK2, suppresses growth, hyphal development, and biofilm formation of Candida albicans. Yeast 34, 253–265. doi: 10.1002/yea.3230
Ji, C., Liu, N., Tu, J., Li, Z., Han, G., Li, J., et al. (2020). Drug repurposing of haloperidol: discovery of new benzocyclane derivatives as potent antifungal agents against Cryptococcosis and Candidiasis. ACS Infect. Dis. 6, 768–786. doi: 10.1021/acsinfecdis.9b00197
Jia, W., Zhang, H., Li, C., Li, G., Liu, X., and Wei, J. (2016). The calcineruin inhibitor cyclosporine a synergistically enhances the susceptibility of Candida albicans biofilms to fluconazole by multiple mechanisms. BMC Microbiol. 16:113. doi: 10.1186/s12866-016-0728-1
Johnson, C. J., Cabezas-Olcoz, J., Kernien, J. F., Wang, S. X., Beebe, D. J., Huttenlocher, A., et al. (2016). The extracellular matrix of Candida albicans biofilms impairs formation of neutrophil extracellular traps. PLoS Pathog. 12:e1005884. doi: 10.1371/journal.ppat.1005884
Johnson, C. J., Kernien, J. F., Hoyer, A. R., and Nett, J. E. (2017). Mechanisms involved in the triggering of neutrophil extracellular traps (NETs) by Candida glabrata during planktonic and biofilm growth. Sci. Rep. 7:13065. doi: 10.1038/s41598-017-13588-6
Juin, C., Perrin, F., Puy, T., Mollichella, M. L., Girardot, M., Costa, D., et al. (2019). Anti-biofilm activity of a semi-synthetic molecule obtained from resveratrol against Candida albicans biofilm. Med. Mycol. 58, 530–542. doi: 10.1093/mmy/myz087
Justo, J. A., and Bookstaver, P. B. (2014). Antibiotic lock therapy: review of technique and logistical challenges. Infect. Drug Resist. 7, 343–363. doi: 10.2147/IDR.S51388
Kaplan, J. B. (2014). Biofilm matrix-degrading enzymes. Methods Mol. Biol. 1147, 203–213. doi: 10.1007/978-1-4939-0467-9_14
Kaur, R., Goyal, R., Dhakad, M. S., Bhalla, P., and Kumar, R. (2014). Epidemiology and virulence determinants including biofilm profile of candida infections in an ICU in a tertiary hospital in india. J. Mycol. 27, 171–172.
Keniya, M. V., Fleischer, E., Klinger, A., Cannon, R. D., and Monk, B. C. (2015). Inhibitors of the Candida albicans major facilitator superfamily transporter Mdr1p responsible for fluconazole resistance. PLoS One 10:e0126350. doi: 10.1371/journal.pone.0126350
Kojic, E. M., and Darouiche, R. O. (2004). Candida infections of medical devices. Clin. Microbiol. Rev. 17, 255–267. doi: 10.1128/CMR.17.2.255-267.2004
Kong, F., Wang, J., Han, R., Ji, S., Yue, J., Wang, Y., et al. (2020). Antifungal activity of magnesium oxide nanoparticles: effect on the growth and key virulence factors of Candida albicans. Mycopathologia 185, 485–494. doi: 10.1007/s11046-020-00446-9
Kothari, A., and Sagar, V. (2009). Epidemiology of candida bloodstream infections in a tertiary care institute in India. Indian J. Med. Microbiol. 27:171. doi: 10.4103/0255-0857.49440
LaFleur, M. D., Lucumi, E., Napper, A. D., Diamond, S. L., and Lewis, K. (2011). Novel high-throughput screen against Candida albicans identifies antifungal potentiators and agents effective against biofilms. J. Antimicrob. Chemother. 66, 820–826. doi: 10.1093/jac/dkq530
Lara, H. H., Ixtepan-Turrent, L., Yacaman, M. J., and Lopez-Ribot, J. (2020). Inhibition of Candida auris bio film formation on medical and environmental surfaces by silver nanoparticles. ACS Appl. Mater. Interfaces 12, 21183–21191. doi: 10.1021/acsami.9b20708
Lara, H. H., Romero-Urbina, D. G., Pierce, C., Lopez-Ribot, J. L., Arellano-Jiménez, M. J., and Jose-Yacaman, M. (2015). Effect of silver nanoparticles on Candida albicans biofilms?: an ultrastructural study. J. Nanobiotechnol. 13:91. doi: 10.1186/s12951-015-0147-8
Lee, J., and Lee, D. G. (2015). Novel antifungal mechanism of resveratrol: apoptosis inducer in Candida albicans. Curr. Microbiol. 70, 383–389. doi: 10.1007/s00284-014-0734-1
Lee, J.-H., Kim, Y. G., Khadke, S. K., Yamano, A., Watanabe, A., and Lee, J. (2019). Inhibition of biofilm formation by Candida albicans and polymicrobial microorganisms by nepodin via hyphal-growth suppression. ACS Infect. Dis. 5, 1177–1187. doi: 10.1021/acsinfecdis.9b00033
Li, Y., Ma, Y., Zhang, L., Guo, F., Ren, L., Yang, R., et al. (2012). In vivo inhibitory effect on the biofilm formation of Candida albicans by liverwort derived Riccardin D. PLoS One 7:e35543. doi: 10.1371/journal.pone.0035543
Liu, X., Wang, D., Yu, C., Li, T., Liu, J., and Sun, S. (2016). Potential antifungal targets against a candida biofilm based on an enzyme in the arachidonic acid cascade-a review. Front. Microbiol. 7:1925. doi: 10.3389/fmicb.2016.01925
Lockhart, S. R. (2014). Current epidemiology of candida infection. Clin. Microbiol. Newsl. 36, 131–136. doi: 10.1016/j.clinmicnews.2014.08.001
Lohse, M. B., Gulati, M., Johnson, A. D., and Nobile, C. J. (2018). Development and regulation of single-and multi-species Candida albicans biofilms. Nat. Rev. Microbiol. 16, 19–31. doi: 10.1038/nrmicro.2017.107
Longhi, C., Santos, J. P., Morey, A. T., Marcato, P. D., Duran, N., Pinge-filho, P., et al. (2016). Combination of fluconazole with silver nanoparticles produced by Fusarium oxysporum improves antifungal effect against planktonic cells and biofilm of drug-resistant Candida albicans. Med. Mycol. 54, 428–432. doi: 10.1093/mmy/myv036
Magi, G., Marini, E., and Facinelli, B. (2015). Antimicrobial activity of essential oils and carvacrol, and synergy of carvacrol and erythromycin, against clinical, erythromycin-resistant Group A Streptococci. Front. Microbiol. 6:165. doi: 10.3389/fmicb.2015.00165
Mah, T. F. C., and O’Toole, G. A. (2001). Mechanisms of biofilm resistance to antimicrobial agents. Trends Microbiol. 9, 34–39. doi: 10.1016/S0966-842X(00)01913-2
Mahamuni-Badiger, P. P., Patil, P. M., Badiger, M. V., Patel, P. R., Thorat- Gadgil, B. S., Pandit, A., et al. (2020). Biofilm formation to inhibition: role of zinc oxide-based nanoparticles. Mater. Sci. Eng. C 108:110319. doi: 10.1016/j.msec.2019.110319
Mamouei, Z., Alqarihi, A., Singh, S., Xu, S., Mansour, M. K., Ibrahim, A. S., et al. (2018). Alexidine dihydrochloride has broad-spectrum activities against diverse fungal pathogens. mSphere 3:e00539-18. doi: 10.1128/mSphere.00539-18
Manoharan, R. K., Lee, J.-H., Kim, Y.-G., Kim, S.-I., and Lee, J. (2017a). Inhibitory effects of the essential oils α-longipinene and linalool on biofilm formation and hyphal growth of Candida albicans. Biofouling 33, 143–155. doi: 10.1080/08927014.2017.1280731
Manoharan, R. K., Lee, J.-H., Kim, Y.-G., and Lee, J. (2017b). Alizarin and chrysazin inhibit biofilm and hyphal formation by Candida albicans. Front. Cell. Infect. Microbiol. 7:447. doi: 10.3389/fcimb.2017.00447
Marc, G., Araniciu, C., Oniga, S. D., Vlase, L., Pîrnau, A., Duma, M., et al. (2018). New N-(oxazolylmethyl)-thiazolidinedione active against candida albicans biofilm: potential Als proteins inhibitors. Molecules 23:2522. doi: 10.3390/molecules23102522
Marcos-Arias, C., Eraso, E., Madariaga, L., and Quindós, G. (2011). In vitro activities of natural products against oral Candida isolates from denture wearers. BMC Complement. Altern. Med. 11:119. doi: 10.1186/1472-6882-11-119
Martins, M., Uppuluri, P., Thomas, D. P., Cleary, I. A., Henriques, M., Lopez-Ribot, J. L., et al. (2014). Presence of extracellular DNA in the Candida albicans biofilm matrix and its contribution to biofilms. Mycopathologia 169, 323–331. doi: 10.1007/s11046-009-9264-y.Presence
Mathé, L., and Van Dijck, P. (2013). Recent insights into Candida albicans biofilm resistance mechanisms. Curr. Genet. 59, 251–264. doi: 10.1007/s00294-013-0400-3
McGeady, P., Wansley, D. L., and Logan, D. A. (2002). Carvone and perillaldehyde interfere with the serum-induced formation of filamentous structures in Candida albicans at substantially lower concentrations than those causing significant inhibition of growth. J. Nat. Prod. 65, 953–955. doi: 10.1021/np010621l
Melo, N. R., de, Abdrahman, A., Greig, C., Mukherjee, K., Thornton, C., et al. (2013). Myriocin significantly increases the mortality of a non-mammalian model host during Candida pathogenesis. PLoS One 8:e78905. doi: 10.1371/journal.pone.0078905
Messier, C., and Grenier, D. (2011). Effect of licorice compounds licochalcone A, glabridin and glycyrrhizic acid on growth and virulence properties of Candida albicans. Mycoses 54, e801–e806. doi: 10.1111/j.1439-0507.2011.02028.x
Michael, C. A., Dominey-howes, D., and Labbate, M. (2014). The antimicrobial resistance crisis?: causes, consequences, and management. Front. Public Health 2:145. doi: 10.3389/fpubh.2014.00145
Midkiff, J., Borochoff-Porte, N., White, D., and Johnson, D. I. (2011). Small molecule inhibitors of the Candida albicans budded-to-hyphal transition act through multiple signaling pathways. PLoS On e 6:e25395. doi: 10.1371/journal.pone.0025395
Mohammed, M., Syeda, J. T. M., Wasan, K. M., and Wasan, E. K. (2017). An overview of chitosan nanoparticles and its application in non-parenteral drug delivery. Pharmaceutics 9:53. doi: 10.3390/pharmaceutics9040053
Monteiro, D. R., Feresin, L. P., Arias, L. S., Barão, V. A. R., Barbosa, D. B., and Delbem, A. C. B. (2015). Effect of tyrosol on adhesion of Candida albicans and Candida glabrata to acrylic surfaces. Med. Mycol. 53, 656–665. doi: 10.1093/mmy/myv052
Monteiro, D. R., Silva, S., Negri, M., Gorup, L. F., de Camargo, E. R., Oliveira, R., et al. (2012). Silver nanoparticles: influence of stabilizing agent and diameter on antifungal activity against Candida albicans and Candida glabrata biofilms. Lett. Appl. Microbiol. 54, 383–391. doi: 10.1111/j.1472-765X.2012.03219.x
Moro, I. J., Gondo, G. D. G. A., Pierri, E. G., Rodrigues Pietro, C. L., Pienna Soares, C., Pergentino de Sousa, D., et al. (2018). Evaluation of antimicrobial, cytotoxic and chemopreventive activities of carvone and its derivatives. Braz. J. Pharm. Sci. 53, 1–8. doi: 10.1590/s2175-97902017000400076
Nani, B. D., Sardi, J. C. O., Lazarini, J. G., Silva, D. R., Massariolli, A. P., Cunha, T. M., et al. (2019). Anti-inflammatory and anti- candida effects of brazilian organic propolis, a promising source of bioactive molecules and functional food. J. Agric. Food Chem. 68, 2861–2871. doi: 10.1021/acs.jafc.8b07304
Nett, J. E., Crawford, K., Marchillo, K., and Andes, D. R. (2010). Role of Fks1p and matrix glucan in Candida albicans biofilm resistance to an echinocandin, pyrimidine, and polyene. Antimicrob. Agents Chemother. 54, 3505–3508. doi: 10.1128/AAC.00227-10
Nett, J. E., Sanchez, H., Cain, M. T., Ross, K. M., and Andes, D. R. (2011). Interface of Candida albicans biofilm matrix-associated drug resistance and cell wall integrity regulation. Eukaryot. Cell 10, 1660–1669. doi: 10.1128/EC.05126-11
Niu, C., Wang, C., Yang, Y., Chen, R., Zhang, J., Chen, H., et al. (2020). Carvacrol induces candida albicans apoptosis associated With Ca2+/Calcineurin pathway. Front. Cell. Infect. Microbiol. 10:192. doi: 10.3389/fcimb.2020.00192
Nobile, C. J., Fox, E. P., Nett, J. E., Sorrells, T. R., Mitrovich, Q. M., Hernday, A. D., et al. (2011). A recently evolved transcriptional network controls biofilm development in Candida albicans. Cell 148, 126–138. doi: 10.1016/j.cell.2011.10.048
Norris, L. A. B., Kablaoui, F., Brilhart, M. K., and Bookstaver, P. B. (2017). Systematic review of antimicrobial lock therapy for prevention of central-line-associated bloodstream infections in adult and pediatric cancer patients. Int. J. Antimicrob. Agents 50, 308–317. doi: 10.1016/j.ijantimicag.2017.06.013
Noverr, M. C., and Huffnagle, G. B. (2004). Regulation of Candida albicans morphogenesis by fatty acid metabolites. Infect. Immun. 72, 6206–6210. doi: 10.1128/IAI.72.11.6206-6210.2004
O’Horo, J. C., Silva, G. L. M., and Safdar, N. (2011). Anti-infective locks for treatment of central line-associated bloodstream infection: a systematic review and meta-analysis. Am. J. Nephrol. 34, 415–422. doi: 10.1159/000331262
Öncü, S. (2014). Optimal dosage and dwell time of ethanol lock therapy on catheters infected with Candida species. Clin. Nutr. 33, 360–362. doi: 10.1016/j.clnu.2013.04.014
Padmavathi, A. R., Das, A., Priya, A., Sushmitha, T. J., Pandian, S. K., et al. (2020). Impediment to growth and yeast-to-hyphae transition in Candida albicans by copper oxide nanoparticles. Biofouling 36, 56–72. doi: 10.1080/08927014.2020.1715371
Pammi, M., Liang, R., Hicks, J., Mistretta, T.-A., and Versalovic, J. (2013). Biofilm extracellular DNA enhances mixed species biofilms of Staphylococcus epidermidis and Candida albicans. BMC Microbiol. 13:257. doi: 10.1186/1471-2180-13-257
Pappas, P. G., Lionakis, M. S., Arendrup, M. C., Ostrosky-Zeichner, L., and Kullberg, B. J. (2018). Invasive candidiasis. Nat. Rev. Dis. Prim. 4:18026. doi: 10.1038/nrdp.2018.26
Perfect, J. R. (2017). The antifungal pipeline: a reality check. Nat. Rev. Drug Discov. 16, 603–616. doi: 10.1038/nrd.2017.46
Perlroth, J., Choi, B., and Spellberg, B. (2007). Nosocomial fungal infections: epidemiology, diagnosis, and treatment. Med. Mycol. 45, 321–346. doi: 10.1080/13693780701218689
Pfaller, M. A., and Diekema, D. J. (2007). Epidemiology of invasive candidiasis: a persistent public health problem. Clin. Microbiol. Rev. 20, 133–163. doi: 10.1128/CMR.00029-06
Pierce, C. G., Chaturvedi, A. K., Lazzell, A. L., Powell, A. T., Saville, S. P., McHardy, S. F., et al. (2015a). A novel small molecule inhibitor of Candida albicans biofilm formation, filamentation and virulence with low potential for the development of resistance. npj Biofilms Microbiomes 1:15012. doi: 10.1038/npjbiofilms.2015.12
Pierce, C. G., Saville, S. P., and Lopez-Ribot, J. L. (2014). High-content phenotypic screenings to identify inhibitors of Candida albicans biofilm formation and filamentation. Pathog. Dis. 70, 423–431. doi: 10.1111/2049-632X.12161
Pierce, C. G., Srinivasan, A., Ramasubramanian, A. K., and López-Ribot, J. L. (2015b). From biology to drug development: new approaches to combat the threat of fungal biofilms. Microbiol. Spectr. 3, 1–18. doi: 10.1128/microbiolspec.mb-0007-2014
Pleszczyńska, M., Wiater, A., Janczarek, M., and Szczodrak, J. (2015). (1→3)-α-d-Glucan hydrolases in dental biofilm prevention and control: a review. Int. J. Biol. Macromol. 79, 761–778. doi: 10.1016/j.ijbiomac.2015.05.052
Pristov, K. E., and Ghannoum, M. A. (2019). Resistance of Candida to azoles and echinocandins worldwide. Clin. Microbiol. Infect. 25, 792–798. doi: 10.1016/j.cmi.2019.03.028
Qu, S., Chen, L., Tian, H., Wang, Z., Wang, F., Wang, L., et al. (2019). Effect of perillaldehyde on prophylaxis and treatment of vaginal candidiasis in a murine model. Front. Microbiol. 10:1466. doi: 10.3389/fmicb.2019.01466
Rajasekharan, S. K., Lee, J.-H., and Lee, J. (2019). Aripiprazole repurposed as an inhibitor of biofilm formation and sterol biosynthesis in multidrug-resistant Candida albicans. Int. J. Antimicrob. Agents 54, 518–523. doi: 10.1016/j.ijantimicag.2019.05.016
Rajendran, R., Borghi, E., Falleni, M., Perdoni, F., Tosi, D., Lappin, D. F., et al. (2015). Acetylcholine protects against Candida albicans infection by inhibiting biofilm formation and promoting hemocyte function in a galleria mellonella infection model. Eukaryot. Cell 14, 834–844. doi: 10.1128/EC.00067-15
Rajput, S. B., and Karuppayil, S. M. (2013). Small molecules inhibit growth, viability and ergosterol biosynthesis in Candida albicans. Springerplus 2, 26.
Ramage, G., Martínez, J. P., and López-Ribot, J. L. (2006). Candida biofilms on implanted biomaterials: a clinically significant problem. FEMS Yeast Res. 6, 979–986. doi: 10.1111/j.1567-1364.2006.00117.x
Ramage, G., Robertson, S. N., and Williams, C. (2014). Strength in numbers: antifungal strategies against fungal biofilms. Int. J. Antimicrob. Agents 43, 114–120. doi: 10.1016/j.ijantimicag.2013.10.023
Ramage, G., Saville, S. P., Wickes, B. L., and Loìpez-Ribot, J. L. (2002). Inhibition of Candida albicans biofilm formation by farnesol, a quorum-sensing molecule. Appl. Environ. Microbiol. 5459–5463. doi: 10.1128/AEM.68.11.5459-5463.2002
Rasool, U. (2019). Effect of biosynthesized copper nanoparticles (CUNPS) on the growth and biofilm formation of fluconazole-resistant Candida albicans. J. Microbiol. Biotechnol. Food Sci. 9, 21–24. doi: 10.15414/jmbfs.2019.9.1.21-24
Raut, J. S., Shinde, R. B., Chauhan, N. M., and Mohan Karuppayil, S. (2013). Terpenoids of plant origin inhibit morphogenesis, adhesion, and biofilm formation by Candida albicans. Biofouling 29, 87–96. doi: 10.1080/08927014.2012.749398
Richardson, M., and Rautemaa-Richardson, R. (2019). Exposure to aspergillus in home and healthcare facilities’ water environments: focus on biofilms. Microorganisms 7:7. doi: 10.3390/microorganisms7010007
Romo, J. A., Pierce, C. G., Chaturvedi, A. K., Lazzell, A. L., McHardy, S. F., Saville, S. P., et al. (2017). Development of anti-virulence approaches for candidiasis via a novel series of small-molecule inhibitors of Candida albicans filamentation. mBio 8:e01991-17. doi: 10.1128/mBio.01991-17
Romo, J. A., Zhang, H., Cai, H., Kadosh, D., Koehler, J. R., Saville, S. P., et al. (2019). Global transcriptomic analysis of the Candida albicans response to treatment with a novel inhibitor of filamentation. mSphere 4:e00620-19. doi: 10.1128/mSphere.00620-19
Sagatova, A. A., Keniya, M. V., Wilson, R. K., Sabherwal, M., Tyndall, J. D. A., and Monk, B. C. (2016). Triazole resistance mediated by mutations of a conserved active site tyrosine in fungal lanosterol 14α-demethylase. Sci. Rep. 6:26213. doi: 10.1038/srep26213
Sajjad, M., Khan, A., and Ahmad, I. (2012). Biofilm inhibition by Cymbopogon citratus and Syzygium aromaticum essential oils in the strains of Candida albicans. J. Ethnopharmacol. 140, 416–423. doi: 10.1016/j.jep.2012.01.045
Sebaa, S., Boucherit-Otmani, Z., and Courtois, P. (2019). Effects of tyrosol and farnesol on Candida albicans biofilm. Mol. Med. Rep. 19, 3201–3209. doi: 10.3892/mmr.2019.9981
Seddighi, N. S., Salari, S., and Izadi, A. R. (2017). Evaluation of antifungal effect of iron−oxide nanoparticles against different Candida species. IET Nanobiotechnol. 11, 883–888. doi: 10.1049/iet-nbt.2017.0025
Sharma, M., and Prasad, R. (2011). The quorum-sensing molecule farnesol is a modulator of drug efflux mediated by ABC multidrug transporters and synergizes with drugs in Candida albicans. Antimicrob. Agents Chemother. 55, 4834–4843. doi: 10.1128/AAC.00344-11
Sharma, Y., Khan, L. A., and Manzoor, N. (2016). Anti-Candida activity of geraniol involves disruption of cell membrane integrity and function. J. Mycol. Médicale 26, 244–254. doi: 10.1016/j.mycmed.2016.04.004
Sherry, L., Jose, A., Murray, C., Williams, C., Jones, B., Millington, O., et al. (2012). Carbohydrate derived fulvic acid: an in vitro investigation of a novel membrane active antiseptic agent against Candida albicans biofilms. Front. Microbiol. 3:116. doi: 10.3389/fmicb.2012.00116
Shukla, A. K. (2020). Nanoparticles and Their Biomedical Applications. Basingstoke: Springer Nature.
Siles, S. A., Srinivasan, A., Pierce, C. G., Lopez-Ribot, J. L., and Ramasubramanian, A. K. (2013). High-throughput screening of a collection of known pharmacologically active small compounds for identification of Candida albicans biofilm inhibitors. Antimicrob. Agents Chemother. 57, 3681–3687. doi: 10.1128/AAC.00680-13
Silva, F., Ferreira, S., Duarte, A., Mendonc, D. I., and Domingues, F. C. (2011). Phytomedicine antifungal activity of Coriandrum sativum essential oil, its mode of action against Candida species and potential synergism with amphotericin B. Phytomedicine 19, 42–47. doi: 10.1016/j.phymed.2011.06.033
Silva, S., Rodrigues, C. F., Araújo, D., Rodrigues, M. E., and Henriques, M. (2017). Candida species biofilms’ antifungal resistance. J. Fungi 3:8. doi: 10.3390/jof3010008
Simsek, M., and Duman, R. (2017). Investigation of effect of 1,8-cineole on antimicrobial activity of chlorhexidine gluconate. Pharmacognosy Res. 9:234. doi: 10.4103/0974-8490.210329
Soll, D. R., and Daniels, K. J. (2016). Plasticity of Candida albicans biofilms. Microbiol. Mol. Biol. Rev. 80, 565–595. doi: 10.1128/mmbr.00068-15
Spampinato, C., and Leonardi, D. (2013). Candida infections, causes, targets, and resistance mechanisms: traditional and alternative antifungal agents. Biomed Res. Int. 204237. doi: 10.1155/2013/204237
Spormann, A. M., Thormann, K., Saville, R., Shukla, S., and Entcheva, P. (2004). Microbial biofilms. Nanoscale Technol. Biol. Syst. 341–357. doi: 10.1201/9780203500224
Su, H., Han, L., and Huang, X. (2018). Potential targets for the development of new antifungal drugs. J. Antibiot. 71, 978–991. doi: 10.1038/s41429-018-0100-9
Subbiahdoss, G., Pidhatika, B., Coullerez, G., Charnley, M., Kuijer, R., van der Mei, H., et al. (2010). Bacterial biofilm formation versus mammalian cell growth on titanium-based mono- and bi-functional coating. Eur. Cells Mater. 19, 205–213. doi: 10.22203/ecm.v019a20
Sun, B., Zhang, M., Li, Y., Hu, Q., Zheng, H., Chang, W., et al. (2016). Synthesis of riccardin D derivatives as potent antimicrobial agents. Bioorg. Med. Chem. Lett. 26, 3617–3620. doi: 10.1016/j.bmcl.2016.06.006
Sun, L., Liao, K., and Wang, D. (2015). Effects of magnolol and honokiol on adhesion, yeast-hyphal transition, and formation of biofilm by Candida albicans. PLoS One 10:e0117695. doi: 10.1371/journal.pone.0117695
Sun, L., Liao, K., and Wang, D. (2017). Honokiol induces superoxide production by targeting mitochondrial respiratory chain complex I in Candida albicans. PLoS One 12:e0184003. doi: 10.1371/journal.pone.0184003
Taff, H. T., Mitchell, K. F., Edward, J. A., and Andes, D. R. (2013). Mechanisms of Candida biofilm drug resistance. Fut. Microbiol. 8, 1325–1337. doi: 10.2217/fmb.13.101
Talsma, S. S. (2007). Biofilms on medical devices. Home Healthc. Nurse 25, 589–594. doi: 10.1097/01.NHH.0000296117.87061.14
Ten Cate, J. M., Klis, F. M., Pereira-Cenci, T., Crielaard, W., and De Groot, P. W. J. (2009). Molecular and cellular mechanisms that lead to Candida biofilm formation. J. Dent. Res. 88, 105–115. doi: 10.1177/0022034508329273
Thakre, A., Jadhav, V., Kazi, R., Shelar, A., Patil, R., Kharat, K., et al. (2020). Oxidative stress induced by piperine leads to apoptosis in Candida albicans. Med. Mycol. 59:myaa058. doi: 10.1093/mmy/myaa058
Thangamani, S., Eldesouky, H. E., Mohammad, H., Pascuzzi, P. E., Avramova, L., Hazbun, T. R., et al. (2017). Ebselen exerts antifungal activity by regulating glutathione (GSH) and reactive oxygen species (ROS) production in fungal cells. Biochim. Biophys. Acta Gen. Subj. 1861, 3002–3010. doi: 10.1016/j.bbagen.2016.09.029
Tian, H., Qu, S., Wang, Y., Lu, Z., Zhang, M., Gan, Y., et al. (2017). Calcium and oxidative stress mediate perillaldehyde-induced apoptosis in Candida albicans. Appl. Microbiol. Biotechnol. 101, 3335–3345. doi: 10.1007/s00253-017-8146-3
Toenjes, K. A., Munsee, S. M., Ibrahim, A. S., Jeffrey, R., Edwards, J. E., and Johnson, D. I. (2005). Small-molecule inhibitors of the budded-to-hyphal-form transition in the pathogenic yeast Candida albicans. Antimicrob. Agents Chemother. 49, 963–972. doi: 10.1128/AAC.49.3.963-972.2005
Truong, T., Suriyanarayanan, T., Zeng, G., Le, T. D., Liu, L., and Wang, Y. (2018). Use of haploid model of Candida albicans to uncover mechanism of action of a novel antifungal agent. Front. Cell Infect. Microbiol. 8:164. doi: 10.3389/fcimb.2018.00164
Tsui, C., Kong, E. F., and Jabra-Rizk, M. A. (2016). Pathogenesis of Candida albicans biofilm. Pathog. Dis. 74:ftw018. doi: 10.1093/femspd/ftw018
Uppuluri, P., Nett, J., Heitman, J., and Andes, D. (2008). Synergistic effect of calcineurin inhibitors and fluconazole against Candida albicans biofilms. Antimicrob. Agents Chemother. 52, 1127–1132. doi: 10.1128/AAC.01397-07
Veerapandian, R., and Vediyappan, G. (2019). Gymnemic acids inhibit adhesive nanofibrillar mediated Streptococcus gordonii–Candida albicans mono-species and dual-species biofilms. Front. Microbiol. 10:2328. doi: 10.3389/fmicb.2019.02328
Vila, T., and Lopez-Ribot, J. L. (2017). Screening the pathogen box for identification of Candida albicans biofilm inhibitors. Antimicrob. Agents Chemother. 61:e02006-16. doi: 10.1128/AAC.02006-16
Wall, G., Chaturvedi, A. K., Wormley, F. L., Wiederhold, N. P., Patterson, H. P., Patterson, T. F., et al. (2018). Screening a repurposing library for inhibitors of multidrug-resistant Candida auris identifies ebselen as a repositionable candidate for antifungal drug development. Antimicrob. Agents Chemother. 62:e01084-18. doi: 10.1128/AAC.01084-18
Wang, B., You, J., King, J. B., Cai, S., Park, E., Powell, D. R., et al. (2014). Polyketide glycosides from Bionectria ochroleuca inhibit candida albicans bio film formation. J. Nat. Prod. 77, 2273–2279.
Wang, X., You, J., King, J. B., Powell, D. R., and Cichewicz, R. H. (2012). Waikialoid A suppresses hyphal morphogenesis and inhibits biofilm development in pathogenic Candida albicans. J. Nat. Prod. 75, 707–715. doi: 10.1021/np2009994
Wei, G., Xu, X., and Wu, C. D. (2011). In vitro synergism between berberine and miconazole against planktonic and biofilm Candida cultures. Arch. Oral Biol. 56, 565–572. doi: 10.1016/j.archoralbio.2010.11.021
Williams, C., Rajendran, R., and Ramage, G. (2016). Aspergillus biofilms in human disease. Adv. Exp. Med. Biol. 931, 1–11. doi: 10.1007/5584_2016_4
Williams, D. W., Jordan, R. P. C., Wei, X.-Q., Alves, C. T., Wise, M. P., Wilson, M. J., et al. (2013). Interactions of Candida albicans with host epithelial surfaces. J. Oral Microbiol. 5, 22434. doi: 10.3402/jom.v5i0.22434
Wong, S. S. W., Kao, R. Y. T., Yuen, K. Y., Wang, Y., Yang, D., Samaranayake, L. P., et al. (2014). In vitro and in vivo activity of a novel antifungal small molecule against Candida Infections. PLoS One 9:e85836. doi: 10.1371/journal.pone.0085836
World Health Organisation [WHO] (2019). New Report Calls for Urgent Action to Avert Antimicrobial Resistance Crisis. Available online at: https://www.who.int/news/item/29-04-2019-new-report-calls-for-urgent-action-to-avert-antimicrobial-resistance-crisis (accessed March 16, 2021).
Wu, S., Wang, Y., Liu, N., Dong, G., and Sheng, C. (2017). Tackling fungal resistance by biofilm inhibitors. J. Med. Chem. 60, 2193–2211. doi: 10.1021/acs.jmedchem.6b01203
Yapar, N. (2014). Epidemiology and risk factors for invasive candidiasis. Ther. Clin. Risk Manag. 10, 95–105. doi: 10.2147/TCRM.S40160
You, J., Du, L., King, J. B., Hall, B. E., and Cichewicz, R. H. (2013). Small-molecule suppressors of Candida albicans biofilm formation synergistically enhance the antifungal activity of Amphotericin B against clinical candida isolates. ACS Chem. Biol. 8, 840–848. doi: 10.1021/cb400009f
Youngsaye, W., Vincent, B., Hartland, C. L., Morgan, B. J., Buhrlage, S. J., Johnston, S., et al. (2011). Piperazinyl quinolines as chemosensitizers to increase fluconazole susceptibility of Candida albicans clinical isolates. Bioorg. Med. Chem. Lett. 21, 5502–5505. doi: 10.1016/j.bmcl.2011.06.105
Yousfi, H., Cassagne, C., Ranque, S., Rolain, J.-M., and Bittar, F. (2019). Repurposing of ribavirin as an adjunct therapy against invasive candida strains in an in vitro study. Antimicrob. Agents Chemother. 63:e00263-19. doi: 10.1128/AAC.00263-19
Yu, L.-H., Wei, X., Ma, M., Chen, X.-J., and Xu, S.-B. (2012). Possible inhibitory molecular mechanism of farnesol on the development of fluconazole resistance in Candida albicans biofilm. Antimicrob. Agents Chemother. 56, 770–775. doi: 10.1128/AAC.05290-11
Yu, Q., Li, J., Zhang, Y., Wang, Y., Liu, L., and Li, M. (2016). Inhibition of gold nanoparticles (AuNPs) on pathogenic biofilm formation and invasion to host cells. Sci. Rep. 6:26667. doi: 10.1038/srep26667
Zaki, M., Oukhrib, A., Hakmaoui, A., and El, and Hiebel, M. (2019). Synthesis of novel 1,2,3-triazole-substituted tomentosins. Zeitschrift für Naturforschung B 74, 273–281. doi: 10.1515/znb-2018-0225
Keywords: Candida albicans, biofilm, resistance, antifungal drugs, prostaglandins, small molecule inhibitors, naturally occurring compounds
Citation: Atriwal T, Azeem K, Husain FM, Hussain A, Khan MN, Alajmi MF and Abid M (2021) Mechanistic Understanding of Candida albicans Biofilm Formation and Approaches for Its Inhibition. Front. Microbiol. 12:638609. doi: 10.3389/fmicb.2021.638609
Received: 07 December 2020; Accepted: 30 March 2021;
Published: 30 April 2021.
Edited by:
Miguel Cacho Teixeira, University of Lisbon, PortugalReviewed by:
Suman Saha, Priyamvada Birla Aravind Eye Hospital, IndiaShanmugaraj Gowrishankar, Alagappa University, India
Copyright © 2021 Atriwal, Azeem, Husain, Hussain, Khan, Alajmi and Abid. This is an open-access article distributed under the terms of the Creative Commons Attribution License (CC BY). The use, distribution or reproduction in other forums is permitted, provided the original author(s) and the copyright owner(s) are credited and that the original publication in this journal is cited, in accordance with accepted academic practice. No use, distribution or reproduction is permitted which does not comply with these terms.
*Correspondence: Mohamed F. Alajmi, bWFsYWptaWlAa3N1LmVkdS5zYQ==; Mohammad Abid, bWFiaWRAam1pLmFjLmlu