- 1State Key Laboratory of Ecological Pest Control for Fujian and Taiwan Crops & Key Laboratory of Biopesticide and Chemical Biology of Ministry of Education & Ministerial and Provincial Joint Innovation Centre for Safety Production of Cross-Strait Crops, College of Life Sciences & College of Plant Protection, Fujian Agriculture and Forestry University, Fuzhou, China
- 2State Key Laboratory of Plant Diseases and Insect Pests Biology, Institute of Plant Protection, Chinese Academy of Agricultural Sciences, Beijing, China
After the biological pesticide Bacillus thuringiensis (Bt) is applied to the field, it has to remain on the surface of plants to have the insecticidal activities against insect pests. Bt can form biofilms on the surface of vegetable leaves, which were rich in polysaccharides. However, the relationship between polysaccharides of the leaves and the biofilm formation as well as the insecticidal activities of Bt is still unknown. Herein, this study focused on the effects of plant polysaccharides pectin and xylan on biofilm formation and the insecticidal activities of Bt strains. By adding pectin, there were 88 Bt strains with strong biofilm formation, 69 strains with weak biofilm formation, and 13 strains without biofilm formation. When xylan was added, 13 Bt strains formed strong biofilms, 98 strains formed weak biofilms, and 59 strains did not form biofilms. This indicated that two plant polysaccharides, especially pectin, modulate the biofilm formation of Bt strains. The ability of pectin to induce biofilm formation was not related to Bt serotypes. Pectin promoted the biofilms formed by Bt cells in the logarithmic growth phase and lysis phase at the air–liquid interface, while it inhibited the biofilms formed by Bt cells in the sporangial phase at the air–liquid interface. The dosage of pectin was positively correlated with the yield of biofilms formed by Bt cells in the logarithmic growth phase or lysis phase at the solid–liquid interfaces. Pectin did not change the free-living growth and the cell motility of Bt strains. Pectin can improve the biocontrol activities of the spore–insecticidal crystal protein mixture of Bt and BtK commercial insecticides, as well as the biofilms formed by the logarithmic growth phase or lysis phase of Bt cells. Our findings confirmed that plant polysaccharides modulate biofilm formation and insecticidal activities of Bt strains and built a foundation for the construction of biofilm-type Bt biopesticides.
Introduction
Biofilms are abundantly present in insect intestines, phyllospheres, rhizospheres, and soils. Bacterial biofilms are aggregates of bacteria embedded in extracellular polymeric substances (EPSs) produced by the bacteria themselves. Their EPSs mainly contain extracellular polysaccharides, proteins, lipids, and extracellular DNA, which can adhere to interfaces of solid–liquid, solid–gas, liquid–liquid, and liquid–gas (Flemming et al., 2016; Flemming and Wuertz, 2019). In fact, bacteria can colonize almost all kinds of natural or synthetic surfaces and form biofilms (Hall-Stoodley et al., 2004; Sweet et al., 2011; Flemming and Wuertz, 2019). Approximately 80% of the bacteria and archaea on the earth exist in the form of biofilms. The average numbers of bacteria in the biofilms locating at termite intestines, phyllospheres, and soil on the land surface are 6 × 1023, 2 × 1026, and 3 × 1029, and the average number of bacteria in the rhizosphere biofilms may be comparable to that of the rhizospheres (Flemming and Wuertz, 2019).
Compared with planktonic cells, the most important feature of bacterial biofilm is that it can help bacteria resist different environmental stresses such as ultraviolet (UV) radiation, antibiotic treatment, drying, temperature variation, oxidative stress, nutrient depletion, and pH change (Rinaudi and Giordano, 2010; Bernbom et al., 2011; Koerdt et al., 2011; Dang and Lovell, 2016).
Plant-derived substances are related to the formation of bacterial biofilms. Natural phenolic compounds have a significant inhibitory effect on the formation of Pseudomonas aeruginosa biofilm (Jagani et al., 2009). Laminaria polysaccharides, xylan, and k-carrageenan can promote the biofilm formation of Xylella fastidiosa in vitro, whereas lichen polysaccharides, oligosaccharides, k-carrageenan, laminarin, xylan, and β-D-glucan can significantly reduce its cell aggregation (Cheng et al., 2009). The fungal supernatants induced by arabinose and pectin can effectively remove the biofilm of Pseudomonas fluorescens (Orgaz et al., 2007). Pectin increased the cell viscosity of X. fastidiosa (Killiny and Almeida, 2009). Plant polysaccharides (xylan, pectin, arabinose) can stimulate the formation of Bacillus subtilis biofilm, which can be used to regulate the phosphorylation state of the main regulator Spo0A. In addition, plant polysaccharides can also be used to synthesize the carbon of the EPSs (Beauregard et al., 2013).
Bacillus thuringiensis (Bt) can produce proteinaceous crystalline inclusions (crystals) during sporulation, which are responsible for its toxicity toward a variety of invertebrates, especially insects (Sauka and Benintende, 2008). Its crystal proteins can be divided into different categories based on amino acid similarity. However, some Bt strains contain a complex family of cry genes. For example, HD1 expressed Cry1Aa, Cry1Ab, Cry1Ac, Cry1Ia, Cry2Aa, and Cry2Ab proteins. But HD73 had only one Cry protein (Cry1Ac) (Sauka et al., 2010). Bt is a widely used agricultural and forestry microbial pesticide (Zwick et al., 2012; Huang et al., 2016, 2018). As early as 2016, the global biocontrol market, including Bt and virus products, exceeded $1 billion1. In addition, HD1 and HD73 have been applied to produce mainstream Bt commercial insecticides. In 1969, HD1 was isolated by Dulmage HT, and its insecticidal ability was 20–200 times that of other Bt strains. In the following years, American manufacturers turned to produce HD1 preparations, becoming the main products of agriculture and forestry control. Hence, HD1 greatly promoted the commercialization of BtK insecticides. HD73 strain, isolated by Dulmage HT, was also extensively applied in Bt industry (Krywienczyk et al., 1978). The main active ingredients of Bt products are spores and insecticidal crystal proteins (ICPs). During the application process, Bt vegetative cells, spores, and their ICPs are sensitive to UV radiation, resulting in a relative short duration of Bt under field conditions. Researchers had tried UV radiation, addition of protective agents, construction of melanin gene mel genetic engineering bacteria, gene knockout of Bt mother cell lysis gene cwlC, and microcapsules to improve Bt’s UV resistance and thus enhance its toxicity to insect pests (Ruan et al., 2005; Chen et al., 2016; He et al., 2017; Zhang et al., 2018). Unfortunately, because of practicability, cost, difficulty in the approval of genetic engineering microbial pesticides, and docking of industry, academia, and research, these technologies are rarely used in Bt industrial production and field application. Herein, there is an urgent need to propose practical, high-quality, and inexpensive solutions to develop new Bt preparations with better insecticidal effects.
Bt can be colonized on the surface of cotton, cabbage, clover, and peas. The colonization time of Bt on the surface of cotton can be as long as 8 weeks, and the colonized leaves have certain insecticidal activity against Plutella xylostella and Spodoptera frugiperda (Normander et al., 1998; Bizzarri and Bishop, 2007, 2008; Bizzarri et al., 2008; Monnerat et al., 2009). P. xylostella (Lepidoptera: Plutellidae), the major insect pest of Brassica vegetable crops in southern China, occurs yearly and causes heavy damage (Wei et al., 2013). Because of the frequent use of biopesticides, P. xylostella is resistant to Bt preparations in some areas. The resistance of P. xylostella to Bt preparations was first discovered in a field trial in Hawaii. However, P. xylostella had low resistance to Bt or no resistance in most areas. A recent study had surveyed six representative populations of P. xylostella collected in Shanghai, Shandong, Hubei, Hunan, Zhejiang, Guangdong, and other places in China. The bioassay results showed that P. xylostella populations from different locations showed different resistance levels. The resistance of Guangdong field population to BtK preparation was 21.90 times. The populations of Shanghai, Hunan, Shandong, and Zhejiang had certain resistance to Cry1Ac, but the resistance to BtK preparation was not obvious. The Hubei population showed no resistance to Cry1Ac and BtK (Wang et al., 2007). However, the effects of plant polysaccharides on the formation and insecticidal activity of Bt biofilms are still unknown. Therefore, this article focuses on the effects of plant polysaccharides, especially pectin, on the regulation of the biofilm formation and insecticidal activity of Bt to build a foundation for the construction of biofilm-type Bt biopesticides.
Materials and Methods
Bt Strains
Most of Bt strains used in this study were obtained from the Bacillus Genetic Stock Center of Ohio State University as follows: 4A1, 4A2, 4A3, 4A4, 4A5, 4A6, 4A7, 4A8, 4A9, 4A10, 4A11T, 4B1, 4B2, 4B3, 4B4, 4B5, 4C1, 4C2, 4C3, 4D1, 4D2, 4D3, 4D4, 4D5, 4D12, 4D16, 4D17, 4D18, 4D19, 4E2, 4E6, 4E7, 4F1, 4F2, 4F3, 4F4, 4F5, 4G1, 4G2, 4G3, 4G4, 4G5, 4G6, 4G7, 4G8, 4H1, 4H2, 4H3, 4H4, 4H5, 4I1, 4I2, 4I3, 4I4, 4I5, 4I6, 4J1, 4J2, 4J3, 4J4, 4J5, 4J6, 4J7, 4J8, 4K1, 4K3, 4K5, 4K6, 4K7, 4K8, 4K9, 4L1, 4L2, 4L3, 4L4, 4L5, 4L6, 4M1, 4M2, 4M3, 4M4, 4M5, 4M6, 4N1, 4O1, 4P1, 4P2, 4Q1, 4Q3, 4Q4, 4Q12, 4R1, 4S2, 4S3, 4T1, 4U1, 4V1, 4V3, 4V6, 4W1, 4W2, 4X1, 4Y1, 4Z1, 4AA1, 4AC1, 4AE1, 4AF1, 4AG1, 4AH1, 4AK1, 4AL1, 4AM1, 4AN1, 4AO1, 4AP1, 4AQ1, 4AR1, 4AS1, 4AT1, 4AU1, 4AV1, 4AW1, 4AX1, 4AY1, 4AZ1, 4AZ2, 4AZ3, 4BA1, 4BB1, 4BC1, 4BD1, 4BE1, 4BF1, 4BG1, 4BH1, 4BJ1, 4BK1, 4BL1, 4BM1, 4BN1, 4BP1, 4BQ1, 4BR1, 4BS1, 4BT1, 4BU1, 4BV1, 4BW1, 4BX1, 4BY1, 4BZ1, 4CA1, 4CB1, 4CC1, 4CD1, 4CE1, 4CG1, 4XX1, 4XX2, 4XX3, 4XX4, and 4XX5. The rest of Bt strains deposited in the biotechnology group of the Institute of Plant Protection, Chinese Academy of Agricultural Sciences, China, were as follows: 261–1, JJX, G03, HD1, Bt185, 1126–1, HBF-18, HD73, G033A, and KN11.
Effects of Plant Polysaccharides Pectin and Xylan on the Biofilm Formation of Bt
MSN medium (5 mM K3PO4⋅3H2O, 100 mM MOPS, 50 μM MnCl2⋅4H2O, 700 μM CaCl2, 2 μM thiamine, 1 μM ZnCl2, 2 mM MgCl2⋅6H2O, 0.2% NH4Cl, pH 7.0) without plant polysaccharides pectin or xylan was used as the control group. One hundred milliliters of MSN medium were mixed with 50 mL of 15 mg/mL pectin or xylan. One milliliter of MSN medium with or without pectin or xylan was added to each well in the 24-well culture plates. Then, 13.5 μL of an overnight culture of Bt strains with OD600 ≈ 0.3 was added into each well and incubated at 30°C for 48 h. In this experiment, the method of qualitative analysis was used to analyze the effect of pectin on the formation of biofilm by Bt strains after cultured in a 24-well plate (Beauregard et al., 2013).
In order to explore whether the ability of pectin to induce biofilms was related to the serotypes of the Bt strains, the difference of biofilm formation induced by pectin in the Bt serotypes with more than five strains was analyzed.
Effect of Plant Polysaccharide Pectin on the Biofilms Formed by Bt Cells in Different Growth Phases at Solid–Liquid Interfaces
The biofilm induction effect of plant polysaccharide pectin was significantly higher than that of plant polysaccharide xylan. Therefore, the follow-up researches were focused only on plant polysaccharide pectin. Bt strains HD1, HD73, Bt185, HBF18, Bt185, G033A, and KN11 that are currently used for industrial production were selected to test the effect of pectin on the biofilm formation of Bt logarithmic growth phase cells. Briefly, the overnight cultured Bt strains were incubated to their logarithmic growth phases. Then, they were 1% transferred to 200 mL LB liquid medium (10 g/L tryptone, 5 g/L yeast extract, 10 g/L NaCl, pH 7.4) with or without 5 mg/mL pectin in a 250-mL flask and incubated at 30°C for 72 h. Then, the biofilms were harvested, dried, and weighed for quantitative analysis. The Bt strains that can form stronger biofilms induced by pectin were selected to further test the influence of pectin in the biofilm formation of Bt sporangial phase cells.
The above method was referred to in order to determine the effect of pectin on the biofilm formation of Bt spore–ICP mixture. Briefly, the mixture was prepared by liquid fermentation. The overnight cultured Bt strains were 2% transferred to 50 mL 1/2 LB liquid medium in a 250-mL flask containing and cultured with shaking at 220 revolutions/min (rpm) at 30°C until 50% of the spores were released. The Bt fermentation broth was centrifuged at 5,000 rpm for 5 min and washed three times with 0.5 M NaCl and sterile ddH2O. And the pellets were diluted with LB medium to 108 colony-forming units (CFUs)/mL to prepare the Bt spore–ICP mixture.
Effect of the Dosage of Plant Polysaccharide Pectin on the Biofilm Formed by Bt Cells in Different Growth Phases at Solid–Liquid Interfaces
Overnight cultured Bt strains were 1% transferred to LB medium and cultured at 30°C with shaking at 220 rpm to an OD600 ≈ 1.00. Then, the culture was 1% transferred to LB medium with 20, 10, 5, 2.5, 1.25, 0.625, and 0 mg/mL pectin. Two milliliters of the mixture was transferred into each well of 24-well plates. After sealing, the plates were treated at 30°C in a moist environment. After 72 h, the OD600 values of each well were determined in a microplate reader. Then, a pipette was used to carefully discard the culture medium in the wells, which were rinsed with 2 mL ddH2O three times to remove the non-adherent planktonic bacteria in the wells. The wells were allowed for air to dry at room temperature and then stained with 2 mL 0.1% crystal violet for 20 min. The wells were rinsed with 2 mL ddH2O three times. After the 24-well plates were air-dried, the dye was dissolved in the wells with 2 mL of absolute ethanol, and the plates were placed on a low-speed shaker to shake for 20 min. Then, the OD595 value of each well was measured with a microplate reader to quantify the biofilm formation.
Effect of Plant Polysaccharide Pectin on the Growth of Bt
Overnight culture of Bt strain was 1% transferred to LB medium with shaking at 220 rpm at 30°C until the OD600 was ∼1.00. The culture was 1% transferred to 200 mL LB medium with or without 5 mg/mL pectin in a 250-mL flask and cultured at 30°C with shaking at 220 rpm. The OD600 values were measured with a spectrophotometer (Cuevas and Edwards, 2017).
Effects of Plant Polysaccharide Pectin on the Cell Motility of Bt
The cell motility was tested by the semisolid plate method (Gnasekaran and Subramaniam, 2015). Overnight cultures of Bt strains were 1% transferred to LB medium and cultured at 30°C with shaking at 220 rpm until the OD600 values were ∼1.00. One microliter of the Bt culture was mixed with 1 μL of LB medium and 1 μL 10 mg/mL pectin (or LB medium). Then, 2 μL mixture was dropped onto a 5-mm round filter paper in the center of a 9-cm LB plate with 0.3, 0.5, and 0.7% agar. After incubation at 30°C for 24 h, the diameter of the culture was measured.
Effect of Plant Polysaccharide Pectin on the Toxicities of Spore–ICP Mixtures of Bt Against P. xylostella
The spore–crystal mixtures of Bt HD1 and HD73 were prepared according to the method described in the section Effect of Plant Polysaccharide Pectin on the Biofilms Formed by Bt Cells in Different Growth Phases at Solid–Liquid Interfaces. The mixtures were resuspended in sterile ddH2O, and their spore numbers were determined by the viable counting. The mixtures were diluted with sterile ddH2O to prepare 1.7 × 1013 and 0.85 × 1013 CFU/mL of Bt HD1, and 2.65 × 1015 and 1.325 × 1015 CFU/mL of Bt HD73. Then, 0, 5, and 25 mg/mL of pectin were added to them. The bioassay of P. xylostella of the spore–ICP mixtures was performed as follows: uniformly sized leaves that were not sprayed with pesticides were rinsed with water or the spore-ICP mixtures for 10 s and dried in the air; the leaves were soaked for 5 min in the mixtures with 0.1% detergent and air-dried. Then, the leaves were placed in 90-mm plates with absorbent papers; 30 3rd-instar larvae were transferred to each treatment, and the plates were strictly sealed with two-layer absorbent papers; after incubation in a light incubator for 2 days at 25°C, the number of dead and alive larvae was used for the calculation of corrected mortality.
Effect of Plant Polysaccharide Pectin on the Toxicity of the Cry1Ac Protein of Bt Against P. xylostella
In order to explore whether the promotion of plant polysaccharide pectin on the bioassay of the spore–ICP mixture of Bt HD73 with one ICP (Cry1Ac) against P. xylostella was related to Cry1Ac protein, the effect of 25 mg/mL pectin on the toxicity of the Cry1Ac extracted from Bt HD73 against P. xylostella was investigated.
Overnight cultured Bt HD73 was 1% transferred to a 1-L flask containing 300 mL of 1/2 LB medium and incubated at 30°C with shaking at 220 rpm. When 50% of spores were released, the fermentation product was centrifuged at 8,000 rpm, 4°C, for 15 min. Then, the pellets were washed with 1 M NaCl and centrifuged at 9,000 rpm, 4°C, for 15 min. After washed with sterile ddH2O, 3% β-mercaptoethanol was used to resuspend the pellets (50 mL β-mercaptoethanol/1-L culture), pH ∼9.5–10. After the samples were placed on ice with shaking at 110 rpm for 4–10 h, they were centrifuged at 8,000 rpm and 4°C for 15 min. Then, the supernatants were mixed with 4 M NaAc-HAc (pH 4.5) in a ratio of 7:1, and the pH values were adjusted to ∼4.5–5.0. After 4 h at 4°C, the samples were centrifuged at 8,000 rpm, 4°C, for 15 min, and the pellets were washed three times with precooled sterile ddH2O. Then, the pellets, Bt HD73 Cry1Ac protein, were completely dissolved in 50 mM Na2CO3 (pH 9.5) with a stir.
Sodium dodecyl sulfate–polyacrylamide gel electrophoresis (Hofte and Whiteley, 1989; Laemmli, 1970) was used to detect the Cry1Ac protein. After filtration with a 0.22-μm filter membrane, the protein was quantified with the Bradford method; 100, 33.33, 11.11, 3.703, and 1.345 μg/mL of Cry1Ac protein was prepared with phosphate-buffered saline buffer for bioassay. The treatment group was added with 25 mg/mL pectin. The bioassay of P. xylostella with Bt HD73 Cry1Ac was carried out as the aforementioned method.
Effect of Plant Polysaccharide Pectin on the Toxicity of Commercial BtK Preparation Against P. xylostella
Commercial BtK preparation 1.6 × 102 and 1.6 × 103 IU/mL with and without 25 mg/mL pectin was prepared. P. xylostella bioassay was carried out according to the above method, and the LC50 values, which cause 50% corrected mortality of the insect pest, were calculated with or without the induction of 25 mg/mL pectin.
Effect of Plant Polysaccharide Pectin on the Toxicities of Bt Biofilms Against P. xylostella
The biofilms formed by Bt cells in the logarithmic growth phase, sporangial phase, and lysis phase were prepared according to the method described in the section of Effect of Plant Polysaccharide Pectin on the Biofilms Formed by Bt Cells in Different Growth Phases at Solid–Liquid Interfaces. Ten milliliters of sterile ddH2O was added to each biofilm sample. The treatment group was added with 25 mg/mL pectin. P. xylostella bioassays of Bt biofilms were carried out with reference to the aforementioned method.
Statistical Analysis
The above treatment was set up with three replicates of three samples. Analysis of variance with Duncan test of the differences between groups was carried out using SPSS 25.0. And the data were expressed as mean ± standard deviation. P < 0.05 and P < 0.01 represented statistically significant differences and statistically extremely significant differences, respectively.
Results
Plant Polysaccharides Modulate the Biofilm Formation of Bt Strains
Combining with the scanned images for qualitative analysis of biofilms induced by pectin, there were 88 strains with strong biofilm formation, 69 strains with weak biofilm formation, and 13 strains without biofilm formation (Figure 1). When xylan was added, 13 Bt strains formed strong biofilms, 98 strains formed weak biofilms, and 59 strains did not form biofilms (Figure 1). This indicated that plant polysaccharides pectin and xylan can induce Bt strains to produce biofilms, and pectin can induce 92.4% of the tested Bt strains to form biofilms. Compared with xylan, pectin had a much better ability to induce Bt biofilm formation. Therefore, pectin was chosen for subsequent experiments.
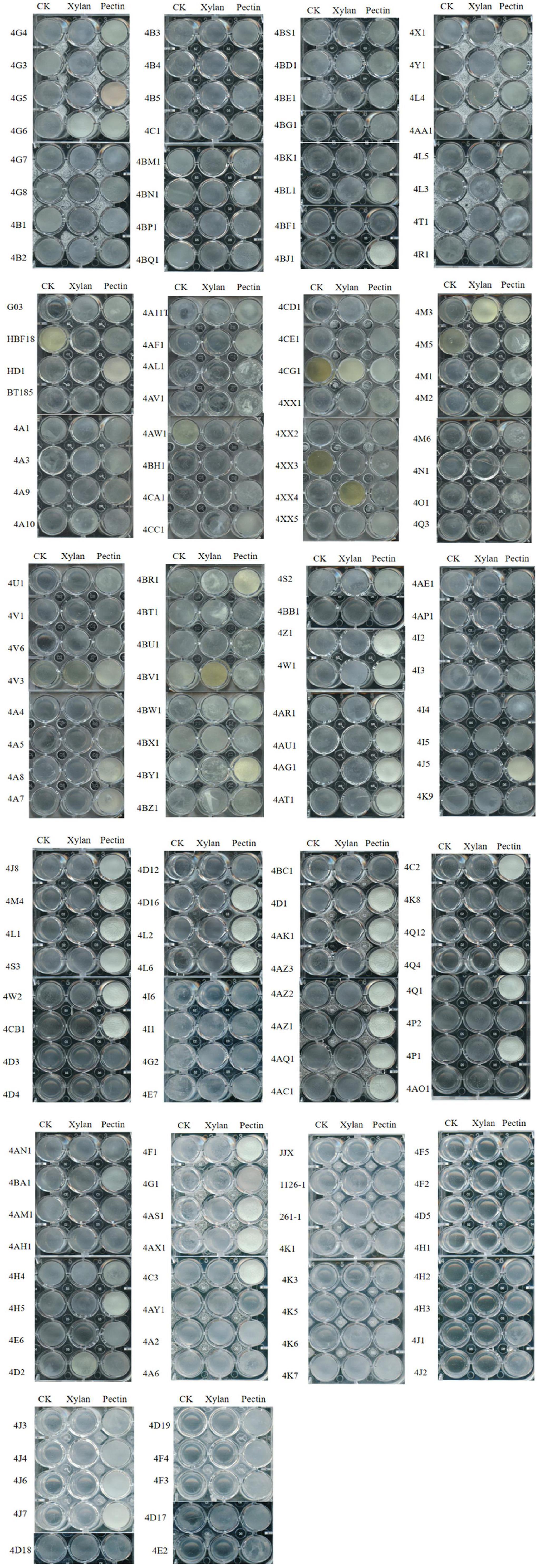
Figure 1. Effects of plant polysaccharides xylan and pectin on the biofilms formed by 170 Bt strains.
The Ability of Pectin to Induce Biofilm Formation Was Not Related to Bt Serotypes
Pectin can induce all tested strains from the serotype subspecies aizawai/pacificus, galleriae, morrisoni, tolworthi, and kenyae of Bt to form biofilms (Table 1). Pectin can also induce the biofilm formation of most Bt strains from the serotypes kurstaki and thuringiensis. This indicated that the Bt biofilm induction by pectin was related to the genetic characteristics of the Bt strains, but not the serotype subspecies (Figure 2).
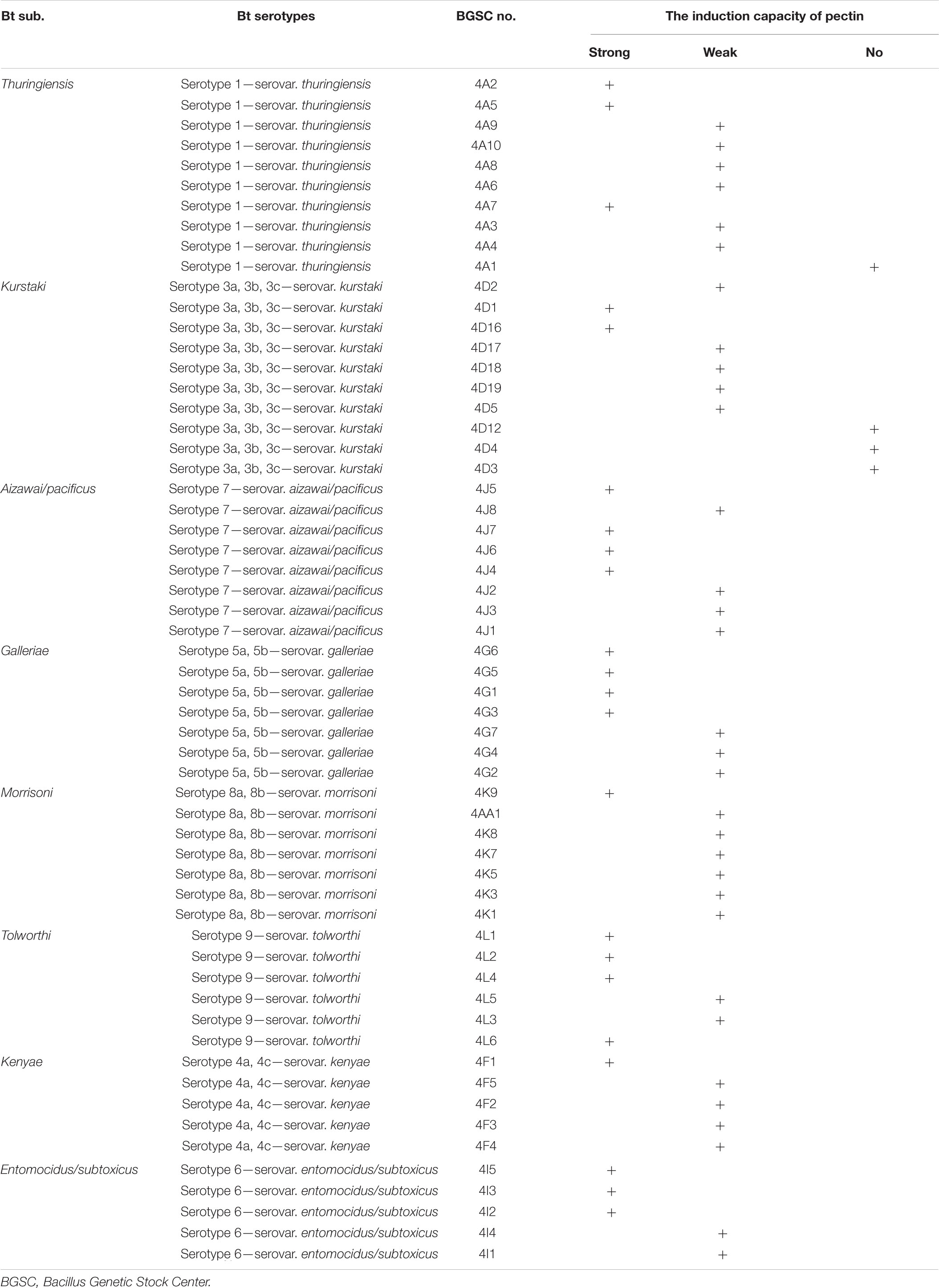
Table 1. Comparison of induction ability of pectin on the biofilms formed by different Bt serotypes.
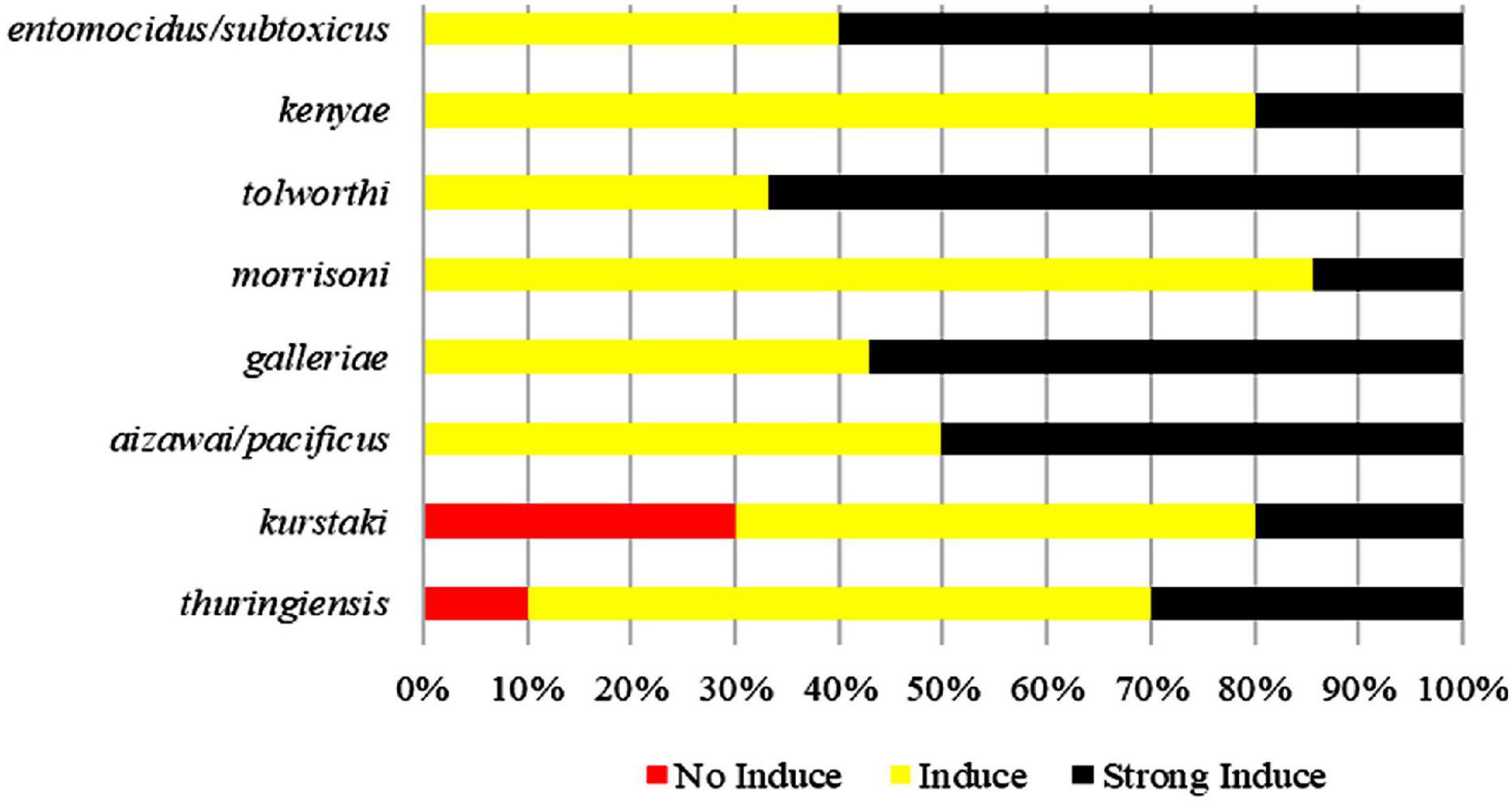
Figure 2. Comparison of induction ability of pectin on the biofilms formed by different Bt serotypes.
Pectin Promoted the Biofilms Formed by Bt Cells in the Logarithmic Growth Phase at the Air–Liquid Interface
Bt strains HD1, HD73, HBF18, Bt 185, G033A, and KN11 with industrial production applications from the above strains were selected to test the abilities to form biofilms at the air–liquid interface. It turned out that only strains HD1, HD73, and Bt185 were able to form weak circular biofilms without pectin. When the pectin was added, Bt strains HD1 and HD73 were significantly enhanced. That is, they can form intact biofilms with severely wrinkled surface at the air–liquid interface, and the thickness was greater than 1 mm (Figure 3A). Therefore, we continued to determine the effects of pectin on the growth and motility capacity of HD1 and HD73, as well as the biofilm formed by the two Bt cells at different growth phases. The results further proved that the ability of pectin to induce Bt biofilm had individual strain differences.
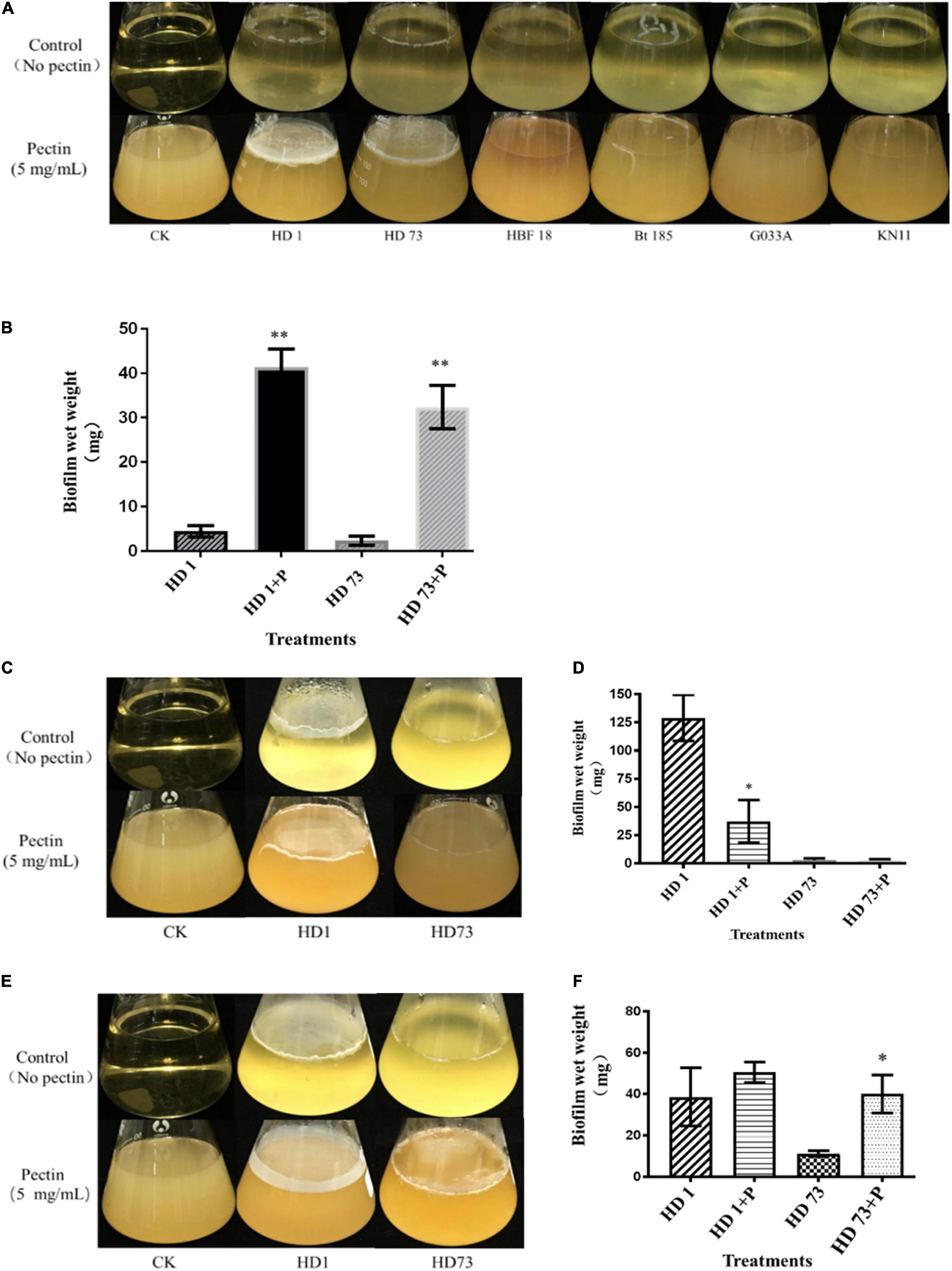
Figure 3. Effects of pectin on the biofilms formed by industrial Bt cellsat the air–liquid interfaces. (A) Bt strains HD1, HD73, Bt185, HBF18, Bt185, G033A, and KN11 that are currently used for industrial production were selected to test the effect of pectin on the biofilm formation of Bt logarithmic growth phase cells. (B) Quantification of the results of Bt HD1 and HD73 from (A). (C) Bt strains HD1 and HD73 that are currently used for industrial production were selected to test the effect of pectin on the biofilm formation of Bt sporangial phase cells. (D) Quantification of the results of Bt HD1 and HD73 from (C). (E) Bt strains HD1 and HD73 that are currently used for industrial production were selected to test the effect of pectin on the biofilm formation of Bt lysis phase cells. (F) Quantification of the results of Bt HD1 and HD73 from (E). P in the figures B–F represents pectin. * and ** represent a significant difference and an extremely significant difference between the biofilms formed by the Bt strains without or with pectin induction, respectively.
Pectin-induced Bt biofilms formed at the air–liquid interface of 200 mL LB liquid medium were harvested, dried, and quantified (Figure 3B). The results showed that pectin increased the yield of Bt HD1 biofilm by 9.27 times, from 4.46 ± 1.26 mg to 41.34 ± 4.10 mg (P < 0.01), whereas that of Bt HD73 increased 13.66-fold by shifting from 2.37 ± 1.03 to 32.38 ± 4.84 mg (P < 0.01).
Pectin Inhibited the Biofilms Formed by Bt Cells in the Sporangial Phase at the Air–Liquid Interface
Without pectin, Bt HD1 cells in the sporangial phase were able to form an intact biofilm at the gas–liquid interface, whereas Bt HD73 can only form a circular biofilm at the same condition. With the induction of pectin, Bt HD1 biofilm appeared as a thin film, whereas Bt HD73 biofilm formation did not change (Figure 3C). By adding pectin, the amount of biofilm formed by Bt HD1 cells in the sporangial phase was reduced from 128.99 ± 20.34 mg to 37.28 ± 18.89 mg (P > 0.05). And the yield of the biofilm formed by the sporangial phase of Bt HD73 was changed from 2.90 ± 1.64 mg and 2.67 ± 1.02 mg (P > 0.05) (Figure 3D). The results showed that pectin has an inhibitory effect on the biofilm formed by the sporangial cells of Bt HD1.
Pectin Enhanced the Biofilms Formed by Bt Cells in the Lysis Phase at the Air–Liquid Interface
The cells of Bt HD1 and HD73 in the lysis phase (spore–ICP mixture) formed circular biofilms at the contact position of the flask at the gas–liquid interface during static culture. Under the induction of pectin, the cells in the lysis phase of Bt HD1 also formed a film-like biofilm at the gas–liquid interface, whereas the cells in the lysis phase of Bt HD73 cannot form an intact biofilm at the gas–liquid interface (Figure 3E). With the addition of pectin, both of Bt HD1 and HD73 formed better biofilms, in that the yields of biofilms changed from 38.64 ± 14.06 mg to 50.54 ± 5.00 mg for HD1 (P > 0.05), and from 11.18 ± 1.39 mg to 40.08 ± 9.21 mg for HD73 (P > 0.05). The biofilm yield of HD73 increased 3.58 times (Figure 3F). Herein, there were certain differences in the ability of pectin to induce biofilms formed by Bt cells in the lysis phase.
The Dosage of Pectin Was Positively Correlated With the Yield of Biofilms Formed by Bt Cells in the Logarithmic Growth Phase or Lysis Phase at the Solid–Liquid Interfaces
With the increase of the pectin dosage, Bt HD1 cells from the logarithmic growth phase did not dramatically alter their growth, but it formed the strongest biofilm with the induction of 10 mg/mL pectin (Figure 4A). The OD600 values and biofilm formation of Bt HD73 cells from the logarithmic growth phase increased with the increase of pectin dosage, and 20 mg/mL pectin can induce the strongest biofilm (Figure 4B). Therefore, pectin can increase the biofilm formation of Bt cells in the logarithmic growth phase of strains HD1 and HD73.
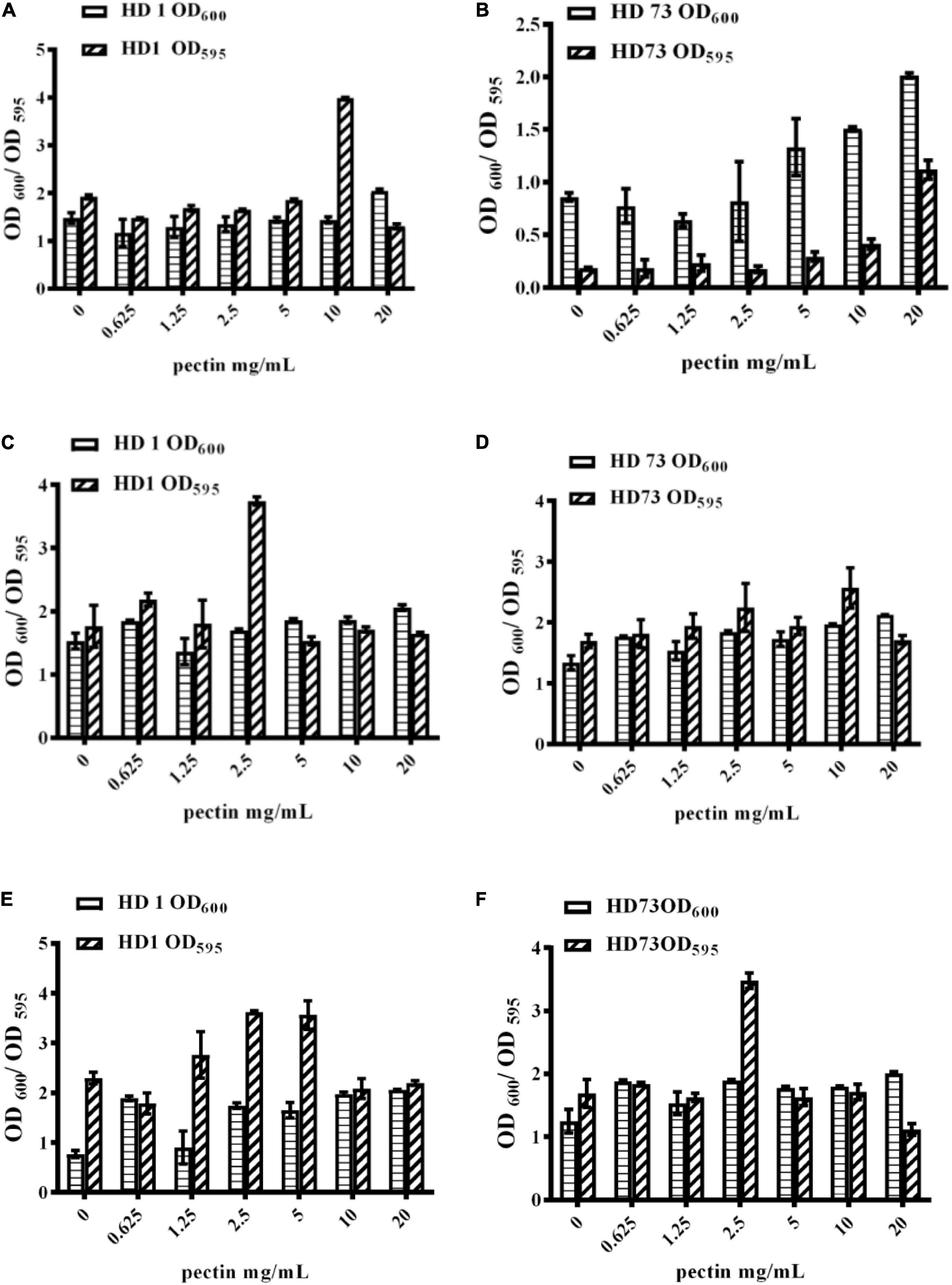
Figure 4. Effects of pectin dosage on the biofilms formed by Bt cells in different growth phases at the solid–liquid interfaces. (A,B) The logarithmic growth phase cells of Bt HD1 and HD73, respectively. (C,D) The sporangial phase cells of Bt HD1 and HD73, respectively. (E,F) The lysis phase cells of Bt HD1 and HD73, respectively.
With the increase of pectin dosage, the OD600 values of Bt HD1 and HD73 cells from the sporangial phase did not change significantly. Bt HD1 and HD73 reached the maximum biofilm formation by adding 2.5 and 10 mg/mL pectin, respectively (Figures 4C,D).
With the increase of the pectin dosage, the growth of Bt HD1 and HD73 in the lysis phase increased at most of the pectin dosages with an exception of 1.25 mg/mL for Bt HD1. The biofilm formation of HD1 cells from the lysis phase reached higher yields with the induction of 1.25, 2.5, or 5 mg/mL pectin, whereas pectin only promoted the biofilm formation of Bt HD73 cells from the lysis phase at 2.5 mg/mL (Figures 4E,F).
Pectin Did Not Dramatically Change the Free-Living Growth of Bt HD1 and HD73
The number of Bt HD1 free-living cells induced by pectin exceeded that of Bt cells without pectin after culture for 12–23 and 28–48 h (Figure 5A). At most of the growth phases, the free-living growth of Bt HD73 was not promoted by pectin (Figure 5B).
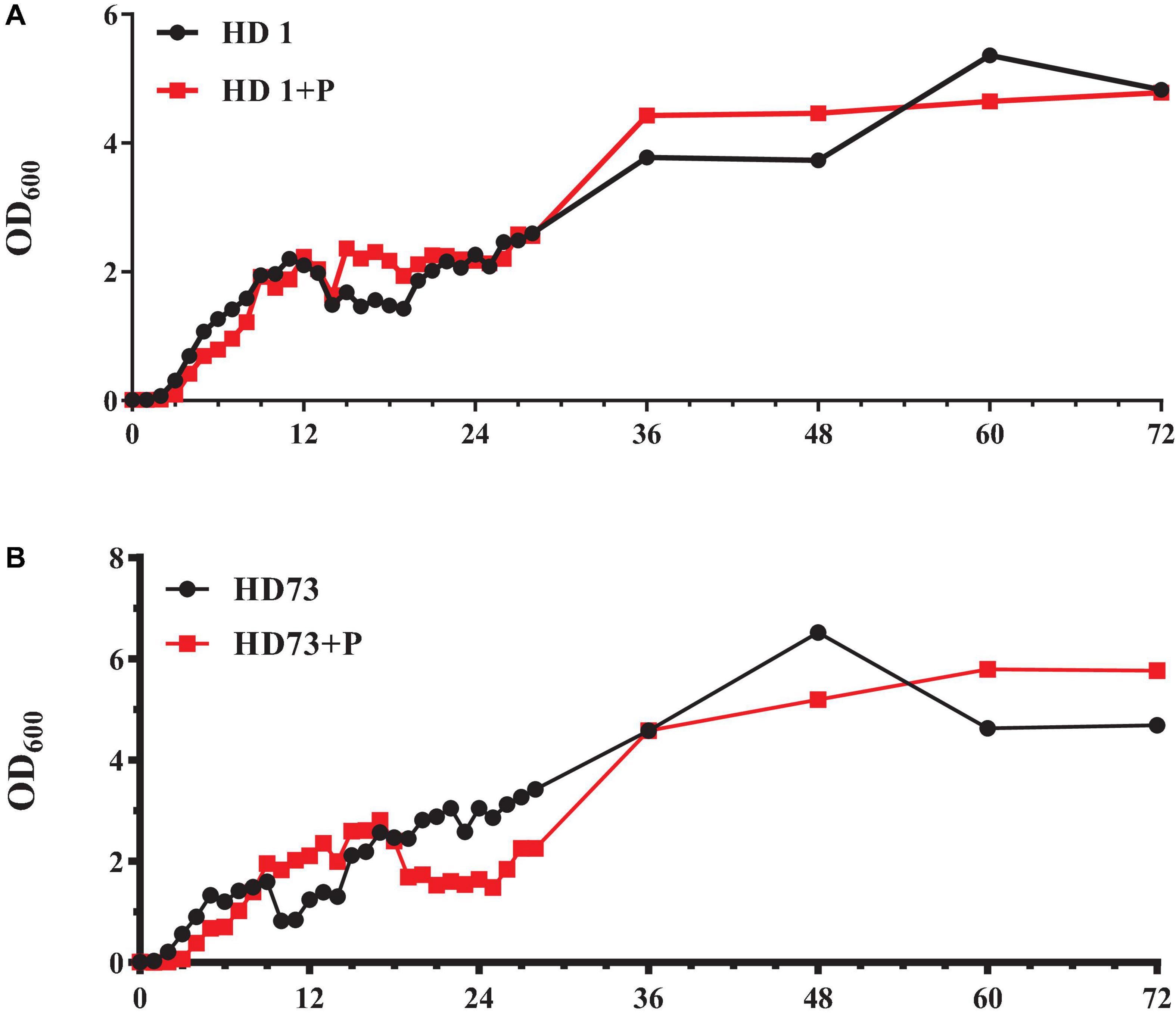
Figure 5. Growth curves of free-living Bt cells with or without the induction of pectin. (A) Bt HD1. (B) Bt HD73.
Pectin Did Not Affect the Cell Motility of Bt Strains
In order to explore the effect of pectin on the cell motility of Bt strains, their swimming abilities and swarming abilities were measured on 90-mm semisolid plates with 0.3, 0.5, or 0.7% agar. The results showed that pectin did not affect the cell motility of Bt HD1 and HD73 (Figure 6).
Pectin Can Improve the Biocontrol Activity of the Spore–ICP Mixture of Bt
Pectin was explored for its effect on the biocontrol activity of Bt. The results showed that 25 mg/mL pectin significantly increased the mortalities of the spore–ICP mixtures of Bt HD1 (0.85 × 1013 CFU/mL) or HD73 (1.325 × 1015 and 2.65 × 1015 CFU/mL) against P. xylostella (Figures 7A,B). However, 5 mg/mL pectin had negative or no effect on the biocontrol activity of the strains against P. xylostella (Figures 7A,B), indicating that the promotion of pectin on biocontrol activity of the spore–ICP mixture of Bt HD1 was dosage-dependent. Pectin 25 mg/mL was used for subsequent experiments. The LC50 of HD1 for the spore–ICP mixture with 25 mg/mL pectin was 0.469 × 1013 CFU/mL. As a result, the HD1 treated with 25 mg/mL pectin was more toxic than HD1 strain without pectin. And the LC50 of HD73 with 5 mg/mL pectin was 0.332 × 1015 CFU/mL; it turned out that HD73 treated with 5 mg/mL pectin is more toxic than the spore–ICPs without pectin (Table 2).
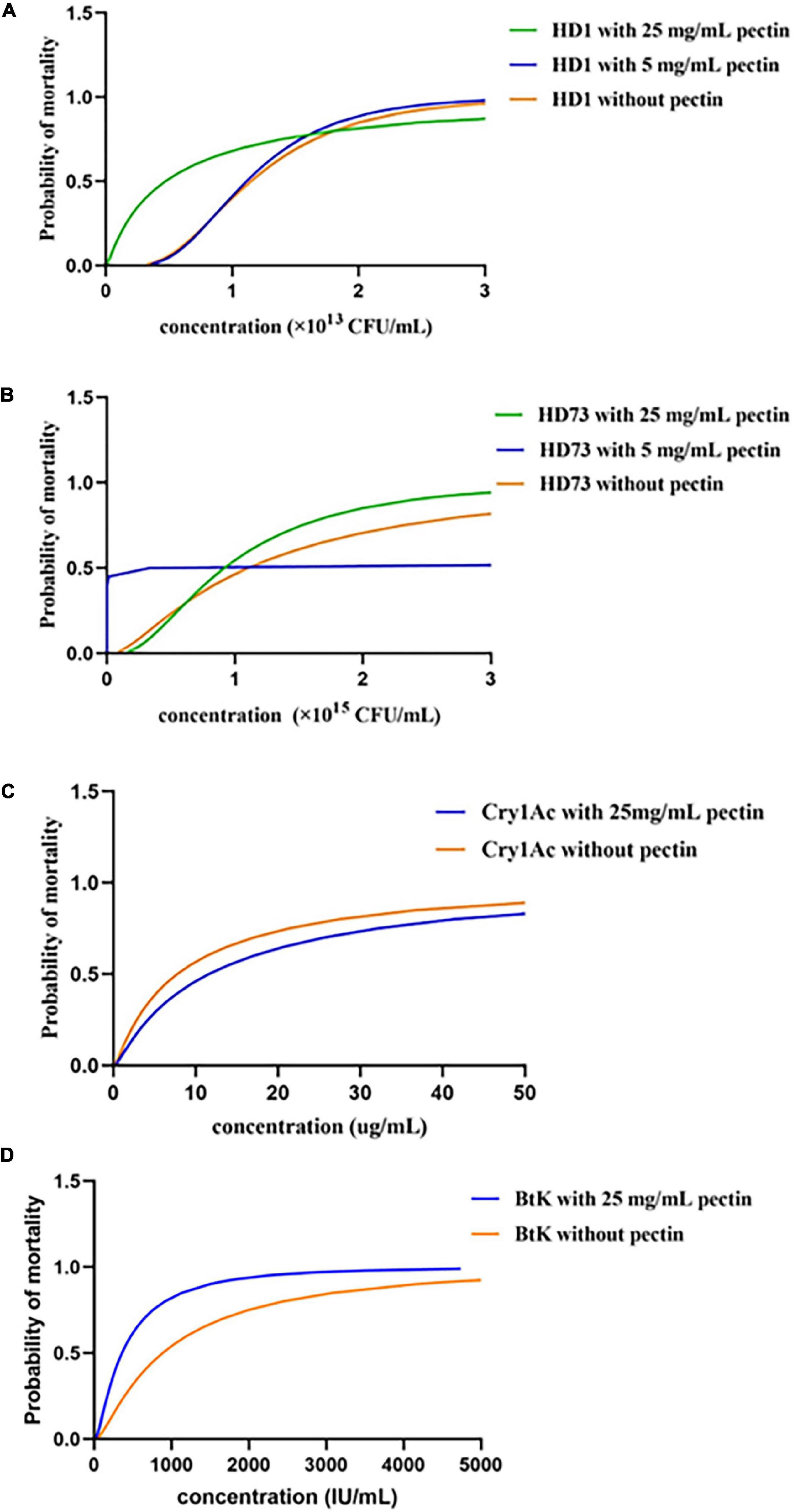
Figure 7. Effects of pectin on the biocontrol activities of Bt strains and preparations. (A,B) Effect of pectin on the biocontrol activities of the spore–ICP mixtures of Bt strains HD1 and HD73 against P. xylostella, respectively. (C) Effect of pectin on the biocontrol activity of Cry1Ac extracted from Bt HD73 against P. xylostella; the value of LC50 of Cry1Ac from HD73. (D) Effect of pectin on the biocontrol activity of BtK preparations against P. xylostella.
Pectin Did Not Affect the Biological Activity of Cry1Ac Protein
Our data showed that pectin can improve the biocontrol activity of the spore–ICP mixture of Bt. In order to clarify whether this activity promotion was due to the effect of pectin on ICPs, the effect of pectin on the biocontrol activity of Cry1Ac protein was investigated by extraction of Cry1Ac from Bt HD73 with one ICP (Cry1Ac). The results showed that 25 mg/mL pectin can inhibit the toxicity of 33.33 μg/mL Cry1Ac against P. xylostella, but it had no effect on the toxicities of Cry1Ac at 100, 11.11, 3.07, and 1.234 μg/mL (Figure 7C), indicating that pectin did not promote the insecticidal activity of the Cry1Ac protein alone, and it may promote the production of other virulence factors in Bt. The LC50 of the Cry1Ac extracted from HD73 strains was 7.738 μg/mL without pectin. And with 25 mg/mL pectin, the LC50 was 11.583 μg/mL (Table 3).
Pectin Improved the Biocontrol Activity of BtK Commercial Insecticides
Pectin 25 mg/mL can increase the mortality of 1.6 × 103 and 1.6 × 102 IU/mL BtK preparation against P. xylostella (Figure 7D). At 1.6 × 103 and 1.6 × 102 IU/mL, BtK preparation caused higher mortality in P. xylostella. The LC50 value for BtK preparation against P. xylostella was 884.197 IU/mL. And with the induction of 25 mg/mL pectin, the LC50 value for BtK preparation against P. xylostella was 358.781 IU/mL (Table 4).
Pectin Can Promote the Biocontrol Activities of Biofilms Formed by the Logarithmic Growth Phase or Lysis Phase of Bt Cells
By inducing with 25 mg/mL pectin, the toxicities of biofilms formed by the logarithmic growth phase or lysis phase of Bt HD1 and HD73 against P. xylostella were significantly higher than the controls. However, pectin inhibited the insecticidal activity of the biofilm formed by Bt HD73 in the sporangial phase against P. xylostella (Figure 8).
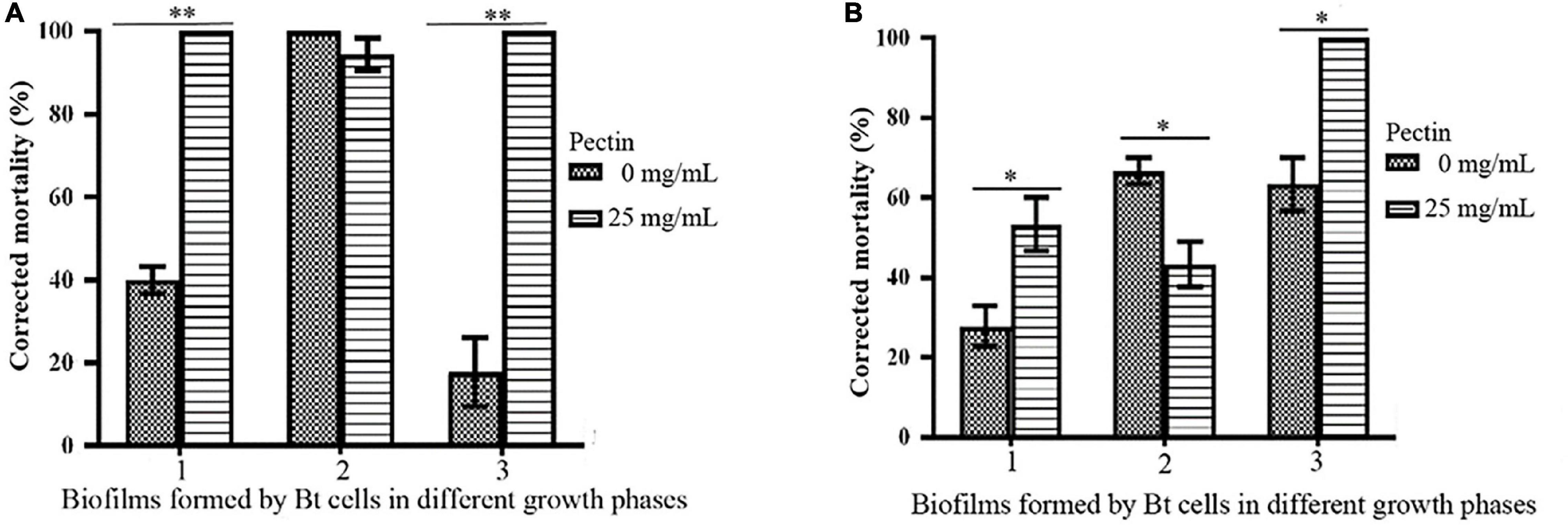
Figure 8. Effects of pectin on the toxicities of biofilms formed by Bt cells in different growth phases. (A) HD1. (B) HD73. 1, 2, 3: Biofilms formed by Bt cells in the logarithmic growth phase, the sporangial phase, and the lysis phase, respectively. * and ** represent a significant difference and an extremely significant difference between the biofilms formed by the Bt strains without or with pectin induction, respectively.
Discussion
Although bacterial biofilms are harmful to human society in many cases, people have gradually realized the beneficial effects of bacterial biofilms in agriculture and industry (Bogino et al., 2013; Berlanga and Guerrero, 2016). They can be used for plant protection, bioremediation, wastewater treatment, and corrosion prevention (Morikawa, 2006; Singh et al., 2006, 2019; Edwards and Kjellerup, 2013; Naidoo and Olaniran, 2013). For example, biofilms of rhizosphere bacteria such as B. subtilis, Pseudomonas, Streptomyces, and Serratia marcescens can be used as biological control agents (Bais et al., 2004; Vejan et al., 2016; Arrebola et al., 2019). Bacterial biofilms such as Aspergillus and Enterobacter can be used as biological fertilizers (Bais et al., 2004; Khan et al., 2018; Singhalage et al., 2019). Compared with the researches of harmful bacterial biofilms, beneficial bacterial biofilms are still in their infant.
Plant polysaccharides are important biological macromolecules in plants, as well as one of the four basic substances that maintain and ensure normal biological activities. They had biological activities, including regulating immunity, antiradiation, antibacteria, and antivirus (Chen et al., 2016). Plant polysaccharides can induce the formation of biofilms by serving as an initial carbon source during the formation of biofilms or being degraded into galactose to be bound to the extracellular matrix (Beauregard et al., 2013). Plant polysaccharides are signal factors for the formation of B. subtilis biofilm (Beauregard et al., 2013). Pectin is a complex plant polysaccharide, constituting of the main component of the plant cell wall. It can be used as a carbon source to promote the formation of the extracellular matrix of strains and induce the formation of biofilm as environmental cues, whereas xylan is a polymer of xylose, a pentose. It is present mostly in fiber cells, contrary to pectin. However, the relationship between plant polysaccharides and the beneficial biofilms formed by microbial pesticide Bt, as well as its insecticidal activity, is still an unsolved mystery. In this study, a 24-well plate method was used to determine the polysaccharide-induced biofilms formed by 170 Bt strains, combining with the scanned images for qualitative analysis of the biofilms. The results showed that pectin can induce much better biofilms formed by Bt than that of xylan. Hence, we chose pectin to study its modulation on biofilm formation and insecticidal activities of Bt strains. And there was no difference in the abilities of pectin and xylan to induce biofilms among Bt serotypes. We further selected six Bt strains with industrial production applications to test their abilities to form biofilms at the air–liquid interface. It turned out that only strains HD1, HD73, and Bt185 can form weak biofilms without pectin. When the pectin was added, biofilm formation of Bt strains HD1 and HD73 was significantly enhanced. Therefore, we continued to determine the effects of pectin on the growth and motility capacity of HD1 and HD73, as well as the biofilm formed by the two Bt cells at different growth phases. In PubMed, there were 2,915 articles related to Bt strains searched using the keyword “thuringiensis strains,” among which there were 200 articles about HD1 strain (6.9%) and 117 articles about HD73 strain (4.0%). Both strains accounted for more than 10%. According to statistics, there were more than thousands of Bt strains stocked in the world. Therefore, the selection of HD1 and HD73 strains as the key research objects was widely representative for 170 tested Bt strains.
The biofilms formed under high nutrient conditions were denser than low nutrient levels (Sutherland, 2001). Our results showed that Bt biofilms first formed ring-shaped bacterial aggregates at the gas–liquid interface. After culturing under pectin induction for 48 h, intact membrane biofilms of HD1 and HD73 can be observed at the air–liquid interface. After that, the structure of the biofilm became more complex, showing increased thickness and severe surface wrinkles. Bt had three growth phases (logarithmic growth phase, sporangial phase, and lysis phase) in planktonic state and the field. Hence, the effects of pectin on the biofilms formed by Bt cells at the three different growth phases were investigated. Pectin had differences in the biofilm formation of Bt cells in different growth phases. At the air–liquid interface, pectin induced Bt HD1 and HD73 cells in the logarithmic growth phase and Bt HD73 cells in the lysis phase to form stronger biofilms, but it inhibited Bt HD1 cells in the sporangial phase to form better biofilm.
Bacterial motility is a key factor in the formation of biofilms. Under static conditions, bacteria first reach a position suitable for biofilm formation and then begin the development of biofilm (Houry et al., 2010). As the biofilm ages, the transcription of flagellin continues to decline, and the bacterial population in the biofilm, including part of the motile cells at the edge of the colony, is heterogeneous (Houry et al., 2012). The increase in biofilm biomass when pectin was used to treat Bacillus amyloliquefaciens was mainly due to the production of surfactant and iturin (Wu et al., 2015). When pectin was used to induce biofilms formed by Bt cells in the growth phase, the biofilm production was also increased, but it did not affect the cell motility of Bt subsp. kurstaki HD1 and HD73. It is of great interest to elucidate what special substances contribute to this phenomenon shortly.
Pectin strongly induced the biofilm formation of Bt cells in the logarithmic growth phase, but exhibited different effects on Bt cells in the sporangial phase and lysis phase. This may be because spore differentiation and biofilm formation belong to different but related regulatory pathways. In Bt biofilm, the gene expressions of the four cell types—toxicity, necrosis, toxicity/necrosis, and necrosis/sporulation—were highly different (Verplaetse et al., 2015). The spore differentiation of Bt was mainly regulated by the phosphorylation pathway of Spo0A-P, whereas the pathways of Bt biofilm formation needs further studies (Verplaetse et al., 2015; Xu et al., 2017).
Pectin-rich amendment enhances soybean growth promotion and modulation mediated by Bacillus velezensis (Hassan et al., 2019). In this study, pectin can promote the biocontrol activities of biofilms formed by the logarithmic growth phase or lysis phase of Bt cells. It also found that 25 mg/mL pectin can improve the biocontrol activity of the spore–ICP mixtures of Bt HD1 and HD73, but the low one cannot promote the insecticidal activity. We speculated that pectin may provide rich nutrition as a carbon source to promote the biofilm formation and to improve the insecticidal activities. The reason why 25 mg/mL pectin cannot promote the insecticidal activity of the Cry1Ac extracted from Bt HD73 is that no living bacteria existed at this time and no production of biofilm. Thus, the insecticidal activity of Cry1Ac cannot be improved by pectin induction. When the BtK preparation was 1.6 × 103 and 1.6 × 102 IU/mL, 25 mg/mL pectin can increase its mortality of P. xylostella. And the LC50 values were 358.781 and 884.197 IU/mL with or without pectin, respectively. Herein, plant polysaccharides can promote the biocontrol activity of Bt against insect pests.
In summary, biological pesticide Bt can form biofilms on the surface of vegetable leaves rich in polysaccharides. The lifestyle of bacteria in the biofilm state is completely different from the planktonic lifestyle. Therefore, how to apply biofilm research finding to biological control of insect pests with Bt in the field has become the key concern of Bt colleagues. Main Bt formulations were wettable powders. When used in the field, they are dissolved in water and sprayed. Therefore, we directly add pectin and other plant polysaccharides to commercially produced Bt for biofilm and insecticidal activity determination, which was more conducive to the conversion of scientific research results into productivity. This study primarily elucidated the effects of plant polysaccharides on biofilm formation and insecticidal activities of Bt. Our findings confirmed that plant polysaccharides modulate biofilm formation and insecticidal activities of Bt. Indeed, the capacity of Bt to form biofilms when in contact with plant polysaccharides could be an advantage and thus build a foundation for the construction of biofilm-type Bt biopesticides with long-term field duration. Future research can be carried out in the mode of actions of pectin to further broaden the depth and breadth of bacterial biofilms.
Data Availability Statement
The original contributions presented in the study are included in the article/supplementary material, further inquiries can be directed to the corresponding author/s.
Author Contributions
TH conceived the research direction of this manuscript. ML and CS performed the experiments. ML, CS, XL, and YY analyzed the experimental results. ML and WK wrote the manuscript. XG, TH, and CS reviewed the manuscript. All authors contributed to the article and approved the submitted version.
Funding
This project was supported by the National Key R&D Project of China (Nos. 2017YFD0200400, 2017YFE0121700, and 2017YFE0122000), the Fujian Science and Technology Planning Project (No. 2020N5014), the Fujian Agriculture and Forestry University Construction Project for Technological Innovation and Service System of Tea Industry Chain (No. K1520007A03), and the National Natural Science Foundation of China (No. 31672084).
Conflict of Interest
The authors declare that the research was conducted in the absence of any commercial or financial relationships that could be construed as a potential conflict of interest.
Acknowledgments
We thank the teachers and students of the Biopesticide Research Center for their help. The content is solely the responsibility of the author and does not represent the opinions of the Ministry of Science and Technology, China, the National Natural Science Foundation of China, the Fujian Provincial Department of Science and Technology, and the Fujian Agriculture and Forestry University.
Footnotes
References
Arrebola, E., Tienda, S., Vida, C., de Vicente, A., and Cazorla, F. M. (2019). Fitness features involved in the biocontrol interaction of Pseudomonas chlororaphis with host plants: the case study of PcPCL1606. Front. Microbiol. 10:719. doi: 10.3389/fmicb.2019.00719
Bais, H. P., Fall, R., and Vivanco, J. M. (2004). Biocontrol of Bacillus subtilis against infection of Arabidopsis roots by Pseudomonas syringae is facilitated by biofilm formation and surfactin production. Plant Physiol. 134, 307–319. doi: 10.1104/pp.103.028712
Beauregard, P. B., Chai, Y., Vlamakis, H., Losick, R., and Kolter, R. (2013). Bacillus subtilis biofilm induction by plant polysaccharides. Proc. Natl. Acad. Sci. U.S. A. 110, E1621–E1630. doi: 10.1073/pnas.1218984110
Berlanga, M., and Guerrero, R. (2016). Living together in biofilms: the microbial cell factory and its biotechnological implications. Microb. Cell Fact. 15:165. doi: 10.1186/s12934-016-0569-5
Bernbom, N., Vogel, B. F., and Gram, L. (2011). Listeria monocytogenes survival of UV-C radiation is enhanced by presence of sodium chloride, organic food material and by bacterial biofilm formation. Int. J. Food Microbiol. 147, 69–73. doi: 10.1016/j.ijfoodmicro.2011.03.009
Bizzarri, M. F., and Bishop, A. H. (2007). Recovery of Bacillus thuringiensis in vegetative form from the phylloplane of clover (Trifolium hybridum) during a growing season. J. Invertebr. Pathol. 94, 38–47. doi: 10.1016/j.jip2006.08.007
Bizzarri, M. F., and Bishop, A. H. (2008). The ecology of Bacillus thuringiensis on the phylloplane: colonization from soil, plasmid transfer, and interaction with larvae of Pieris brassicae. Microb. Ecol. 56, 133–139. doi: 10.1007/s00248-007-9331-1
Bizzarri, M. F., Prabhakar, A., and Bishop, A. H. (2008). Multiple-locus sequence typing analysis of Bacillus thuringiensis recovered from the phylloplane of clover (Trifolium hybridum) in vegetative form. Microb. Ecol. 55, 619–625. doi: 10.1007/s00248-007-9305-3
Bogino, P. C., Oliva Mde, L., Sorroche, F. G., and Giordano, W. (2013). The role of bacterial biofilms and surface components in plant-bacterial associations. Int. J. Mol. Sci. 14, 15838–15859. doi: 10.3390/ijms140815838
Chen, Y., Yao, F., Ming, K., Wang, D., Hu, Y., and Liu, J. (2016). Polysaccharides from traditional Chinese medicines: extraction, purification, modification, and biological activity. Molecules 21:1705. doi: 10.3390/molecules21121705
Cheng, D. W., Lin, H., Walker, M. A., Stenger, D. C., and Civerolo, E. L. (2009). Effects of grape xylem sap and cell wall constituents on in vitro growth, biofilm formation and cellular aggregation of Xylella fastidiosa. Eur. J. Plant Pathol. 125, 213–222. doi: 10.1007/s10658-009-9473-8
Cuevas, D. A., and Edwards, R. A. (2017). PMA nalyzer: a new web interface for bacterial growth curve analysis. Bioinformatics 33, 1905–1906. doi: 10.1093/bioinformatics/btx084
Dang, H., and Lovell, C. R. (2016). Microbial surface colonization and biofilm development in marine environments. Microb. Mol. Biol. Rev. 80, 91–138. doi: 10.1128/mmbr.00037-15
Edwards, S. J., and Kjellerup, B. V. (2013). Applications of biofilms in bioremediation and biotransformation of persistent organic pollutants, pharmaceuticals/personal care products, and heavy metals. Appl. Microbiol. Biotechnol. 97, 9909–9921. doi: 10.1007/s00253-013-5216-z
Flemming, H. C., Wingender, J., Szewzyk, U., Steinberg, P., Rice, S. A., and Kjelleberg, S. (2016). Biofilms: an emergent form of bacterial life. Nat. Rev. Microbiol. 14, 563–575. doi: 10.1038/nrmicro.2016.94
Flemming, H. C., and Wuertz, S. (2019). Bacteria and archaea on Earth and their abundance in biofilms. Nat. Rev. Microbiol. 17, 247–260. doi: 10.1038/s41579-019-0158-9
Gnasekaran, P., and Subramaniam, S. (2015). Mapping of the interaction between Agrobacterium tumefaciens and Vanda Kasem’s delight orchid protocorm-like bodies. Indian J. Microbiol. 55, 285–291. doi: 10.1007/s12088-015-0519-7
Hall-Stoodley, L., Costerton, J. W., and Stoodley, P. (2004). Bacterial biofilms: from the natural environment to infectious diseases. Nat. Rev. Microbiol. 2, 95–108. doi: 10.1038/nrmicro821
Hassan, M. K., McInroy, J. A., Jones, J., Shantharaj, D., Liles, M. R., and Kloepper, J. W. (2019). Pectin-Rich amendment enhances soybean growth promotion and nodulation mediated by Bacillus velezensis Strains. Plants (Basel) 8:120. doi: 10.3390/plants8050120
He, X., Sun, Z., He, K., and Guo, S. (2017). Biopolymer microencapsulations of Bacillus thuringiensis crystal preparations for increased stability and resistance to environmental stress. Appl. Microbiol. Biotechnol. 101, 2779–2789.
Hofte, H., and Whiteley, H. R. (1989). Insecticidal crystal proteins of Bacillus thuringiensis. Microbiol. Rev. 53, 242–255.
Houry, A., Briandet, R., Aymerich, S., and Gohar, M. (2010). Involvement of motility and flagella in Bacillus cereus biofilm formation. Microbiology 156, 1009–1018. doi: 10.1099/mic.0.034827-0
Houry, A., Gohar, M., Deschamps, J., Tischenko, E., Aymerich, S., and Gruss, A. (2012). Bacterial swimmers that infiltrate and take over the biofilm matrix. Proc. Natl. Acad. Sci. U.S.A. 109, 13088–13093. doi: 10.1073/pnas.1200791109
Huang, T., Lin, Q., Qian, X., Zheng, Y., Yao, J., and Wu, H. (2018). Nematicidal activity of Cry1Ea11 from Bacillus thuringiensis BRC-XQ12 against the pine wood nematode (Bursaphelenchus xylophilus). Phytopathology 108, 44–51. doi: 10.1094/phyto-05-17-0179-r
Huang, T., Zhang, X., Pan, J., Su, X., Jin, X., and Guan, X. (2016). Purification and characterization of a novel cold shock protein-like bacteriocin synthesized by Bacillus thuringiensis. Sci. Rep. 6:35560. doi: 10.1038/srep35560
Jagani, S., Chelikani, R., and Kim, D. S. (2009). Effects of phenol and natural phenolic compounds on biofilm formation by Pseudomonas aeruginosa. Biofouling 25, 321–324. doi: 10.1080/08927010802660854
Khan, N., Martinez-Hidalgo, P., Ice, T. A., Maymon, M., Humm, E. A., and Nejat, N. (2018). Antifungal activity of Bacillus species against fusarium and analysis of the potential mechanisms used in biocontrol. Front. Microbiol. 9:2363. doi: 10.3389/fmicb.2018.02363
Killiny, N., and Almeida, R. P. (2009). Host structural carbohydrate induces vector transmission of a bacterial plant pathogen. Proc. Natl. Acad. Sci. U.S.A. 106, 22416–22420. doi: 10.1073/pnas.0908562106
Koerdt, A., Orell, A., Trong Khoa, P., Mukherjee, J., Wlodkowski, A., and Karunakaran, E. (2011). Macromolecular fingerprinting of Sulfolobus species in biofilm: a transcriptomic and proteomic approach combined with spectroscopic analysis. J. Proteome Res. 10, 4105–4119. doi: 10.1021/pr2003006
Krywienczyk, J., Dulmage, H. T., and Fast, P. G. (1978). Occurrence of two serologically distinct groups within Bacillus thuringiensis serotype 3 ab var. kurstaki. J. Invertebr. Pathol. 31, 372–375.
Laemmli, U. K. (1970). Cleavage of structural proteins during the assembly of the head of bacteriophage T4. Nature 227, 680–685. doi: 10.1038/227680a0
Monnerat, R. G., Soares, C. M., Capdeville, G., Jones, G., Martins, E. S., and Praca, L. (2009). Translocation and insecticidal activity of Bacillus thuringiensis living inside of plants. Microb. Biotechnol. 2, 512–520.
Morikawa, M. (2006). Beneficial biofilm formation by industrial bacteria Bacillus subtilis and related species. J. Biosci. Bioeng. 101, 1–8. doi: 10.1263/jbb.101.1
Naidoo, S., and Olaniran, A. O. (2013). Treated wastewater effluent as a source of microbial pollution of surface water resources. Int. J. Environ. Res. Public Health 11, 249–270. doi: 10.3390/ijerph110100249
Normander, B., Christensen, B. B., Molin, S., and Kroer, N. (1998). Effect of bacterial distribution and activity on conjugal gene transfer on the phylloplane of the bush bean (Phaseolus vulgaris). Appl. Environ. Microbiol. 64, 1902–1909.
Orgaz, B., Kives, J., Pedregosa, A. M., Monistrol, I. F., Laborda, F., and Sanjosé, C. (2007). Bacterial biofilm removal using fungal enzymes. Enzyme Microbial. Technol. 40, 51–56.
Rinaudi, L. V., and Giordano, W. (2010). An integrated view of biofilm formation in rhizobia. FEMS Microbiol. Lett. 304, 1–11.
Ruan, L., He, W., He, J., Sun, M., and Yu, Z. (2005). Cloning and expression of mel gene from Bacillus thuringiensis in Escherichia coli. J Antonie Van Leeuwenhoek. 87, 283–288.
Sauka, D. H., Basurto-Ríos, R. E., Ibarra, J. E., and Benintende, G. B. (2010). Characterization of an Argentine isolate of Bacillus thuringiensis similar to the HD-1 strain. Neotrop. Entomol. 39, 767–773.
Sauka, D. H., and Benintende, G. B. (2008). Bacillus thuringiensis: general aspects. An approach to its use in the biological control of lepidopteran insects behaving as agricultural pests. Rev. Argent. Microbiol. 40, 124–140.
Singh, H. M., Pathak, A. K., Chopra, K., Tyagi, V. V., Anand, S., and Kothari, R. (2019). Microbial fuel cells: a sustainable solution for bioelectricity generation and wastewater treatment. Biofuels 10, 11–31. doi: 10.1080/17597269.2017.1413860
Singh, R., Paul, D., and Jain, R. K. (2006). Biofilms: implications in bioremediation. Trends Microbiol. 14, 389–397.
Singhalage, I. D., Seneviratne, G., Madawala, H., and Wijepalaa, P. C. (2019). Profitability of strawberry (Fragaria ananassa) production with biofilmed biofertilizer application. Sci. Hortic. 243, 411–413.
Sutherland, I. W. (2001). The biofilm matrix–an immobilized but dynamic microbial environment. Trends Microbiol. 9, 222–227.
Sweet, M. J., Croquer, A., and Bythell, J. C. (2011). Development of bacterial biofilms on artificial corals in comparison to surface-associated microbes of hard corals. PLoS One 6:e21195.
Vejan, P., Abdullah, R., Khadiran, T., Ismail, S., and Nasrulhaq Boyce, A. (2016). Role of plant growth promoting rhizobacteria in agricultural sustainability-a review. Molecules 21:573. doi: 10.3390/molecules21050573
Verplaetse, E., Slamti, L., Gohar, M., and Lereclus, D. (2015). Cell differentiation in a Bacillus thuringiensis population during planktonic growth, biofilm formation, and host infection. mBio 6:e00138-15. doi: 10.1128/mBio.00138-15
Wang, L., Li, X. F., Zhang, J., Zhao, J. Z., Wu, Q. J., and Xu, B. (2007). Monitoring of resistance for the diamondback moth to Bacillus thuringiensis Cry1Ac and Cry1Ba toxins and a Bt commercial formulation. J. Appl. Entomol. 131, 441–446. doi: 10.1111/j.1439-0418.2007.01187.x
Wei, S. J., Shi, B. C., Gong, Y. J., Jin, G. H., Chen, X. X., and Meng, X. F. (2013). Genetic structure and demographic history reveal migration of the diamondback moth Plutella xylostella (Lepidoptera: Plutellidae) from the southern to northern regions of China. PLoS One 8:e59654. doi: 10.1371/journal.pone.0059654
Wu, K., Fang, Z. Y., Guo, R., Pan, B., Shi, W., and Yuan, S. F. (2015). Pectin enhances bio-control efficacy by inducing colonization and secretion of secondary metabolites by Bacillus amyloliquefaciens SQY 162 in the rhizosphere of tobacco. PLoS One 10:17.
Xu, S., Yang, N., Zheng, S., Yan, F., Jiang, C., and Yu, Y. (2017). The spo0A-sinI-sinR regulatory circuit plays an essential role in biofilm formation, nematicidal activities, and plant protection in Bacillus cereus AR156. Mol. Plant Microbe Interact. 30, 603–619. doi: 10.1094/mpmi-02-17-0042-r
Zhang, L., Zhang, X., Batool, K., Hu, X., Chen, M., Xu, J., et al. (2018). Comparison and mechanism of the UV-resistant mosquitocidal Bt mutant LLP29-M19. J. Med. Entomol. 55, 210–216. doi: 10.1093/jme/tjx192
Keywords: Bacillus thuringiensis, biofilm, pectin, insecticidal activity, xylan, Plutella xylostella, plant polysaccharide
Citation: Li M, Shu C, Ke W, Li X, Yu Y, Guan X and Huang T (2021) Plant Polysaccharides Modulate Biofilm Formation and Insecticidal Activities of Bacillus thuringiensis Strains. Front. Microbiol. 12:676146. doi: 10.3389/fmicb.2021.676146
Received: 04 March 2021; Accepted: 14 May 2021;
Published: 28 June 2021.
Edited by:
Manoel V. F. Lemos, São Paulo State University, BrazilReviewed by:
Jin He, Huazhong Agricultural University, ChinaMa. Cristina Del Rincón-Castro, University of Guanajuato, Mexico
Copyright © 2021 Li, Shu, Ke, Li, Yu, Guan and Huang. This is an open-access article distributed under the terms of the Creative Commons Attribution License (CC BY). The use, distribution or reproduction in other forums is permitted, provided the original author(s) and the copyright owner(s) are credited and that the original publication in this journal is cited, in accordance with accepted academic practice. No use, distribution or reproduction is permitted which does not comply with these terms.
*Correspondence: Tianpei Huang, dGlhbnBlaWh1YW5nQGZhZnUuZWR1LmNu
†These authors have contributed equally to this work