- 1Institute of Food Science, Faculty of Agricultural and Food Science and Environmental Management, University of Debrecen, Debrecen, Hungary
- 2Department of Biochemical Engineering, Faculty of Science and Technology, University of Debrecen, Debrecen, Hungary
- 3Doctoral School of Chemistry, University of Debrecen, Debrecen, Hungary
- 4Juhász-Nagy Pál Doctoral School of Biology and Environmental Sciences, University of Debrecen, Debrecen, Hungary
- 5Institute of Chemical, Environmental & Bioscience Engineering, TU Wien, Vienna, Austria
The effects of the interplay of copper(II) and manganese(II) ions on growth, morphology and itaconic acid formation was investigated in a high-producing strain of Aspergillus terreus (NRRL1960), using carbon sources metabolized either mainly via glycolysis (D-glucose, D-fructose) or primarily via the pentose phosphate shunt (D-xylose, L-arabinose). Limiting Mn2+ concentration in the culture broth is indispensable to obtain high itaconic acid yields, while in the presence of higher Mn2+ concentrations yield decreases and biomass formation is favored. However, this low yield in the presence of high Mn2+ ion concentrations can be mitigated by increasing the Cu2+ concentration in the medium when D-glucose or D-fructose is the growth substrate, whereas this effect was at best modest during growth on D-xylose or L-arabinose. A. terreus displays a high tolerance to Cu2+ which decreased when Mn2+ availability became increasingly limiting. Under such conditions biomass formation on D-glucose or D-fructose could be sustained at concentrations up to 250 mg L–1 Cu2+, while on D-xylose- or L-arabinose biomass formation was completely inhibited at 100 mg L–1. High (>75%) specific molar itaconic acid yields always coincided with an “overflow-associated” morphology, characterized by small compact pellets (<250 μm diameter) and short chains of “yeast-like” cells that exhibit increased diameters relative to the elongated cells in growing filamentous hyphae. At low concentrations (≤1 mg L–1) of Cu2+ ions, manganese deficiency did not prevent filamentous growth. Mycelial- and cellular morphology progressively transformed into the typical overflow-associated one when external Cu2+ concentrations increased, irrespective of the available Mn2+. Our results indicate that copper ions are relevant for overflow metabolism and should be considered when optimizing itaconic acid fermentation in A. terreus.
Introduction
Itaconic acid (2-methylenesuccinic acid, 1-propene-2,3-dicarboxylic acid) is an unsaturated, weak diprotic acid. Its distinguished chemical properties are related to the conjugated double bond of the methylene group that allows polymerization both by addition and condensation, as well as the esterification of the carboxylic groups with different co-monomers (Kuenz et al., 2012). Itaconic acid is used as a building block for a wide variety of industrial products of added value such as resins, paints, synthetic fibers, plasticizers, and detergents (Okabe et al., 2009). Itaconic acid applications have also penetrated the dental, ophthalmic and drug delivery fields (Hajian and Yusoff, 2015). A promising chemical for the bio-based economy, itaconic acid polymers may even substitute petroleum-based polyacrylic acid, presenting a potential multi-billion dollar market (Cunha da Cruz et al., 2018).
On technical scale, itaconic acid is produced by batch fermentation in a process largely similar to that of citric acid, employing the ascomycete filamentous fungus Aspergillus terreus. The highest published specific molar yield of itaconic acid is about 85% of the theoretical value, accounting to a volumetric yield of ∼150 g L–1 (Karaffa et al., 2015). Itaconic acid overflow requires an excess of rapidly metabolizable carbon source, high dissolved oxygen levels, an initial medium pH between 3 and 5, a phosphate concentration low enough to limit fungal growth, and a paucity of manganese ions (Mn2+) (Hevekerl et al., 2014; Molnár et al., 2018; Karaffa and Kubicek, 2019; Saha and Kennedy, 2019b). In Aspergilli, manganese deficiency transforms filamentous hyphal morphology to one dominated by “yeast-like cells” (branches of short, swollen forms) on the micro-morphology-, and small compact (<0.5 mm diameter) pellets on the macro-morphology level (Detroy and Ciegler, 1971; Gyamerah, 1995). Progression of this transformation is accompanied by increased cell/hyphae diameter and reduced pellet size (Paul et al., 1994; Karaffa et al., 1997; El-Sabbagh et al., 2008). High (>75%) specific molar itaconic acid yields (Yp/s) occur only when cultures overwhelmingly comprise such morphology (henceforth referred to as “overflow-associated morphology”), improving the rheology of the culture broth, which in turn results in increased oxygen transfer throughout the fermentation (Kuenz et al., 2012; Karaffa et al., 2015).
Excess of copper(II) (Cu2+) ions results in mitigation of the deleterious effect that Mn2+ ions exert on the formation of citric acid in A. niger, possibly by interfering with cellular Mn2+ ion uptake and -homeostasis (Hockertz et al., 1987; Netik et al., 1997). It has recently been demonstrated that the Cu2+ ion concentration is a significant process variable for A. terreus itaconic acid fermentations, an attribute to further product yield in the presence of inhibitory concentrations of Mn2+ ions (Saha and Kennedy, 2019b). These antagonistic properties allowed the formulation of synthetic media for improved production of itaconic acid without the necessity to pretreat the carbon source with cation exchange resins to remove associated Mn2+. Industrial itaconic acid fermentations mostly utilize molasses or starch hydrolysates as the source of carbon (for a review, see Karaffa and Kubicek, 2019). However, competition with food applications increasingly impels the industry to utilize sugars released from non-food, lignocellulosic plant biomass (Cunha da Cruz et al., 2018; Kuenz and Krull, 2018). Lignocellulose is a complex network of carbohydrate polymers of predominantly hexose or pentose monomers crosslinked by lignin, highly inert polymers containing phenolic and other aromatic subunits (Saha, 2003; Saha et al., 2017). However, release, biodegradation, uptake and metabolic conversion of the different polysaccharide components and their constituent monosaccharides cross interact and interfere with one another. It is thus crucial to understand their individual metabolism and bioconversion first to appreciate their individual contributions to sustainable production of itaconic acid from cheap raw vegetative material (Kolláth et al., 2019).
The ability of Cu2+ ions to alleviate the negative effect of Mn2+ ions on citric acid and itaconic acid fermentations have almost exclusively been investigated on hexoses. The objective of this study was to investigate whether itaconic acid production on other monosaccharide carbon sources that constitute lignocellulose shows similar responses to the co-presence of Cu2+ and Mn2+. We investigated the influence of Cu2+ ions on the growth, morphology and itaconic acid formation in the high-titer itaconic acid producer Aspergillus terreus NRRL1960 on D-glucose, D-xylose, L-arabinose and an alternative glycolytic carbon source, D-fructose. We show that on D-glucose and D-fructose, the ratio of Cu2+ to Mn2+ – rather than their respective absolute concentrations – co-determines micro- and macromorphology of the biomass and modulates the itaconic acid production yield in batch cultures. However, on D-xylose and L-arabinose the collaborative antagonism between the two divalent cations is less straightforward, taking into account the increased sensitivity of A. terreus to Cu2+ of when growing on either of these two pentose sugars.
Materials and Methods
Fungal Strain and Cultivation Conditions
Aspergillus terreus NRRL 1960 (CBS 116.46; ATCC 10020), a standard high-producer strain, was maintained on agar plates (Kuenz et al., 2012). Per liter of distilled water, the chemically defined minimal medium used throughout the experiments contained 0.1 g KH2PO4, 3 g NH4NO3, 1 g MgSO4 × 7 H2O, 5 g CaCl2 × 2 H2O, 1.67 mg FeCl3 × 6 H2O, 8 mg ZnSO4 × 7 H2O. The default concentration for Cu2+ ions – in the form of CuSO4 × 7 H2O – was 3.3 mg L–1 (cf. Kuenz et al., 2012), but it was set between 0.01 and 1000 mg L–1 in the diverse experiments. Hexoses D-glucose and D-fructose and pentoses D-xylose and L-arabinose were used as sole carbon sources. Initial concentrations were 50 g L–1 for D-xylose (Kolláth et al., 2019), 80 g L–1 for L-arabinose (Saha et al., 2017), and 120 g L–1 for D-glucose (Karaffa et al., 2015) and D-fructose (data not shown; concentration optimized during preliminary experiments). Fermentations were carried out either under manganese(II) ion limitation (=1.5 μg L–1) or sufficiency (=300 μg L–1). To control the concentration of Mn2+ ions in the growth medium, all carbon sources were dissolved in distilled water and passed through a column (440 × 45 mm) of Dowex 50 W-X8 (100/200) cation exchange resin. All other medium components were added to these sugar solutions from sterile stock solutions made up with Dowex 50 W-X8 treated water. The final Mn2+ concentration in the medium was adjusted by the addition of appropriate volumes of a stock solution of MnCl2 × 4H2O.
Shake-flask cultivations were performed in 500-mL Erlenmeyer flasks (VWR International Kft., Debrecen, Hungary) with 100 mL medium incubated at 33°C in a rotary shaker (Infors AG, Basel, Switzerland) operating at 300 revolutions per minute (rpm). This aeration regime is optimal for the production of itaconic acid in shake flasks, regardless of the growth substrate (Karaffa et al., 2015). The initial medium pH was set at 3.0 with 3 M HCl, and was not controlled during the fermentations (Karaffa et al., 2015).
Bioreactor cultivations were carried out in 2.5-L glass fermentors (Sartorius AG, Göttingen, Germany) with a culture (working) volume of 2 L, equipped with one six-blade Rushton disc turbine impeller. Operating conditions were 33°C, and 0.75 vessel volume per minute (vvm) of aeration. Before inoculation, the pH was adjusted to 3.0 with 3 M HCl but was not controlled during fermentation. Dissolved oxygen (DO) levels were maintained at 30% saturation by adjusting the impeller tip speed. DO, temperature, and impeller tip speed were controlled automatically by the regulatory units of the bioreactors. To minimize water loss, the waste gas (from the headspace) was cooled in a reflux condenser connected to an external cooling bath (4°C) before exiting the system. Both shake-flask and bioreactor cultures were inoculated with 1 × 106 A. terreus conidia per milliliter of medium from a freshly prepared, high-density spore suspension in a 1/10,000 Tween-20 solution.
All chemicals used were of analytical grade and purchased from Sigma-Aldrich.
Analytical Methods
Mycelial dry cell weight (DCW) was determined from 5-mL culture aliquots as described by Kozma and Karaffa (1996). The biomass was harvested on a pre-weighted glass wool filter and washed with cold tap water, after which the filter was dried at 70°C until constant weight. Biomass yields (Yx/s) were calculated by dividing the concentration of the highest biomass (DCW) value by that of the total supplied carbon source. Specific growth rates (μ, given as the reciprocal of time, h–1) were calculated from the DCW increase over the time elapsed between two consecutive sampling time points; the highest of the thus obtained values was taken as the maximal specific growth rate of the culture.
The concentrations of the sugar carbon sources and itaconic acid in the growth media were determined by high-pressure/performance liquid chromatography (HPLC; Agilent 1260 Infinity II with Quaternary pump and Vial sampler) with a proton exchange column (Bio-Rad Aminex HPX-87H+) at 55°C, using isocratic elution with 10 mM H2SO4 and refractive index detection as described by Fekete et al. (2002). The concentrations were calculated from two independent measurements, which never deviated more than 5%. Specific molar itaconic acid yield (Yp/s) is the ratio of the produced moles of itaconic acid and the consumed moles of carbon source. Biomass-specific itaconic acid yield (Yp/x) is the ratio of the volumetric yield of itaconic acid (g L–1) and the DCW measured at the same time-point.
Manganese- and copper ion concentrations in the growth media were determined by inductively coupled plasma quadrupole mass spectrometry (ICP-QMS; Thermo Fisher Scientific, Bremen, Germany) equipped with Hexapole Collision Cell Technology (CCT), as described in detail by Karaffa et al. (2015).
Copper toxicity was defined as the external Cu2+ concentration in the culture broth at which half-maximal specific growth rate values (LD50) were attained under the given growth conditions. The monitored effect of the toxic compound was the de-acceleration of growth during cultivation, assessed with successive DCW determinations. This approach is illustrated by Supplementary Figure 1, which shows the maximum specific growth rate versus the Cu2+ concentration on D-glucose both at low- and high manganese concentrations.
Fungal morphology was defined in three forms – (a) the swollen hyphal fragments called yeast-like forms, (b) the filamentous hyphae and (c) the mycelial pellets that are spherical colonies of highly entangled hyphal biomass (Bartoshevich et al., 1990; Paul and Thomas, 1998). Morphology was investigated microscopically with an Axio-Vision AC quantitative image analyzer system. To increase contrast and visibility, lactophenol cotton blue (Fluka Chemie, Buch, Switzerland) was added to the medium samples in a final concentration of 10 % (v/v), except when spore germination and agglutination was monitored. Stained samples were analyzed with a Zeiss AxioImager phase-contrast microscope, equipped with AxioCam MRc5 camera. Average cell- and average pellet diameters (also referred to as micro- and macro-morphology, respectively) were assessed with the AxioVision AC image analyzer system processing at least 50 cells or 10 pellets for each liquid culture sample studied.
Reproducibility
All presented data are the means of three to five independent experiments (biological replicates: starting with liquid cultures using different spore inocula). Data were analyzed and visualized with Sigmaplot software (Jandel Scientific), and for all datasets standard deviations were determined. Quantitative data (n ≥ 3) were compared using ANOVA (Analysis of Variance) with the Holm-Sidak-Test for pairwise comparisons. While probability (p) values were often <0.001, the criterion for significance was p < 0.05 in all cases.
Results
Verification of the Experimental System
A high number of growth conditions (cultures) was implicated to study the interplay of the two essential divalent cations and the consequences for itaconic acid overflow. Therefore shake-flasks were initially the cultivation vehicle of choice. To substantiate our findings toward application in scaled-up fermentations, we also performed controlled batch cultivations in 2-L scale bioreactors for those cultivation regimes that appeared crucial to verify and demonstrate the principles of our findings and their future exploitation. To ensure that the major physical parameters (pH, DO, temperature) of the two cultivation systems were essentially identical, and thus that fermentation kinetics would be independent of the vessel type, protocols for monitoring pH and DO were applied as previously described by us (Kolláth et al., 2019). Although either peak values of fungal biomass- and itaconic acid formation, as well as the residual carbon concentrations of the cultures grown in shake-flask or bioreactor deviated sometimes up to 20% at a given time point, the trends were always consistent. We thus considered our experimental setup appropriate for the purposes of this study.
Copper(II) Ion Tolerance of Aspergillus terreus Depends on the Carbon Source and the Concentration of Manganese Ions
A synthetic growth medium optimized for itaconic acid production (Kuenz et al., 2012) was used to test copper tolerance of A. terreus NRRL1960 using two hexoses (D-glucose, D-fructose) and two pentoses (D-xylose, L-arabinose) as carbon sources. Each carbon source was used at a concentration that allowed the highest itaconic acid yield, i.e., 120 g L–1 for D-glucose and D-fructose, 80 g L–1 for L-arabinose and 50 g L–1 for D-xylose (see “Materials and Methods” for details). The default Cu2+ concentration that allowed the highest growth rate was 3.3 mg L–1 for each carbon source tested. The Mn2+ ion concentration was set either at 1.5 μg L–1 – which is growth limiting but favors itaconic acid production – or 300 μg L–1, which is optimal for biomass formation (Saha and Kennedy, 2019a).
In the presence of 300 μg L–1 manganese ions, the addition of increased concentrations of copper ions started to decrease the biomass yield from the carbon source (Yx/s) at concentrations >50 mg L–1 (with the exception of L-arabinose where the decrease was only apparent at >100 mg L–1). Yet the half-maximal lethal concentrations (LD50) of copper ions on the two hexoses were higher (close to 1 g L–1) than on the two pentoses (around 0.75 g L–1) (Tables 1A, 2A). In the presence of 1.5 μg L–1 manganese ions, however, the decrease in biomass yield started already at copper concentrations of >75 mg L–1 on the two hexoses and at >25 mg L–1 on the two pentoses (Table 2B). In agreement with these findings, the half-maximal lethal concentration of copper ions was around 100 mg L–1 for the two hexoses, and 78 mg L–1 for D-xylose. The LD50 value of Cu2+ for L-arabinose was even only 48 mg L–1 (Table 1A). The sensitivity of A. terreus to Cu2+ ions thus appears to depend on the concentration of manganese ions as well as on the growth substrate, particularly at limiting concentrations of Mn2+.

Table 1A Copper(II) ion toxicity of A. terreus NRRL1960 grown on four different carbon sources in liquid minimal media either under manganese(II) ion limitation (1.5 μg L–1) or under manganese(II) ion sufficiency (300 μg L–1).
The germination of conidiospores tolerated much higher concentrations of copper – up to 3 g L–1 for hexoses in the presence of 300 μg L–1 manganese ions (Table 1B) – but otherwise showed the same trend as the biomass yield Yx/s, the sensitivity being higher at limiting manganese concentrations and during growth on pentoses (Table 1B). Interestingly, the determined LD50 values did not depend on the concentration of the carbon source as we obtained essentially the same threshold values for conidiospore germination on all four carbon sources tested across a range of 10–150 g L–1 initial carbon source concentration (data not shown).
The Concentrations of Cu2+ and Mn2+ Influence the Itaconic Acid Yield in a Carbon Source-Dependent Manner
Under fully optimized fermentation conditions (initial substrate concentration, culture broth pH, Mn2+ limitation and high DO) A. terreus NRRL1960 is capable of converting >80% of the available D-glucose, on a molar basis (Yp/s), into itaconic acid (Karaffa et al., 2015). Slightly lower specific yields were achieved on D-fructose, whereas significantly lower yields were attained on D-xylose and particularly on L-arabinose (Table 2A). Specific molar itaconic acid yields were always highest at a Cu2+ concentration of 3.3 mg L–1. On the two hexoses, the lowest yields were observed at the lowest of the Cu2+ concentrations tested (0.01 mg L–1; Figure 1B). On D-glucose, D-fructose and D-xylose, the molar itaconic acid yield Yp/s decreased only slightly at copper concentrations higher than 3.3 mg L–1, which was accompanied by an increased yield of itaconic acid per biomass unit (Yp/x). However, unlike hexose-grown cultures, the pentose-grown cultures did not form any itaconic acid at Cu2+ concentration of ≥75 mg L–1 (Table 2A). Finally, the generally low itaconic acid yields on L-arabinose did not seem to variate with the copper concentration in the growth medium.
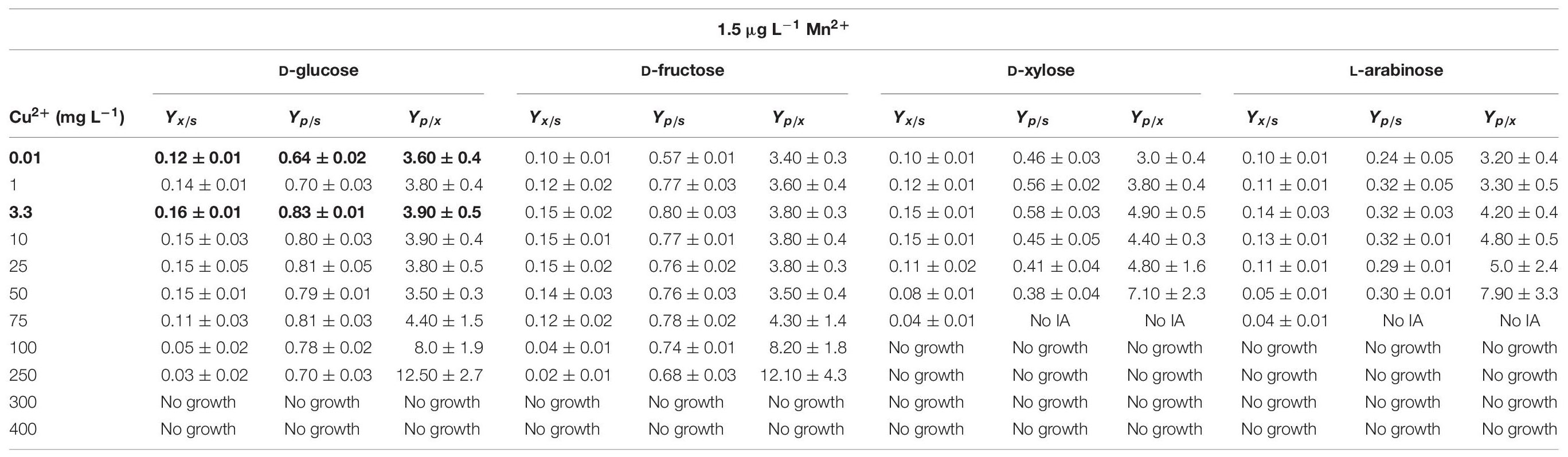
Table 2A Specific molar itaconic acid yield (Yp/s), biomass-specific itaconic acid yield (Yp/x) and biomass yield (Yx/s) of Aspergillus terreus NRRL1960 cultures as a function of the copper(II) ion concentration in the culture broth.
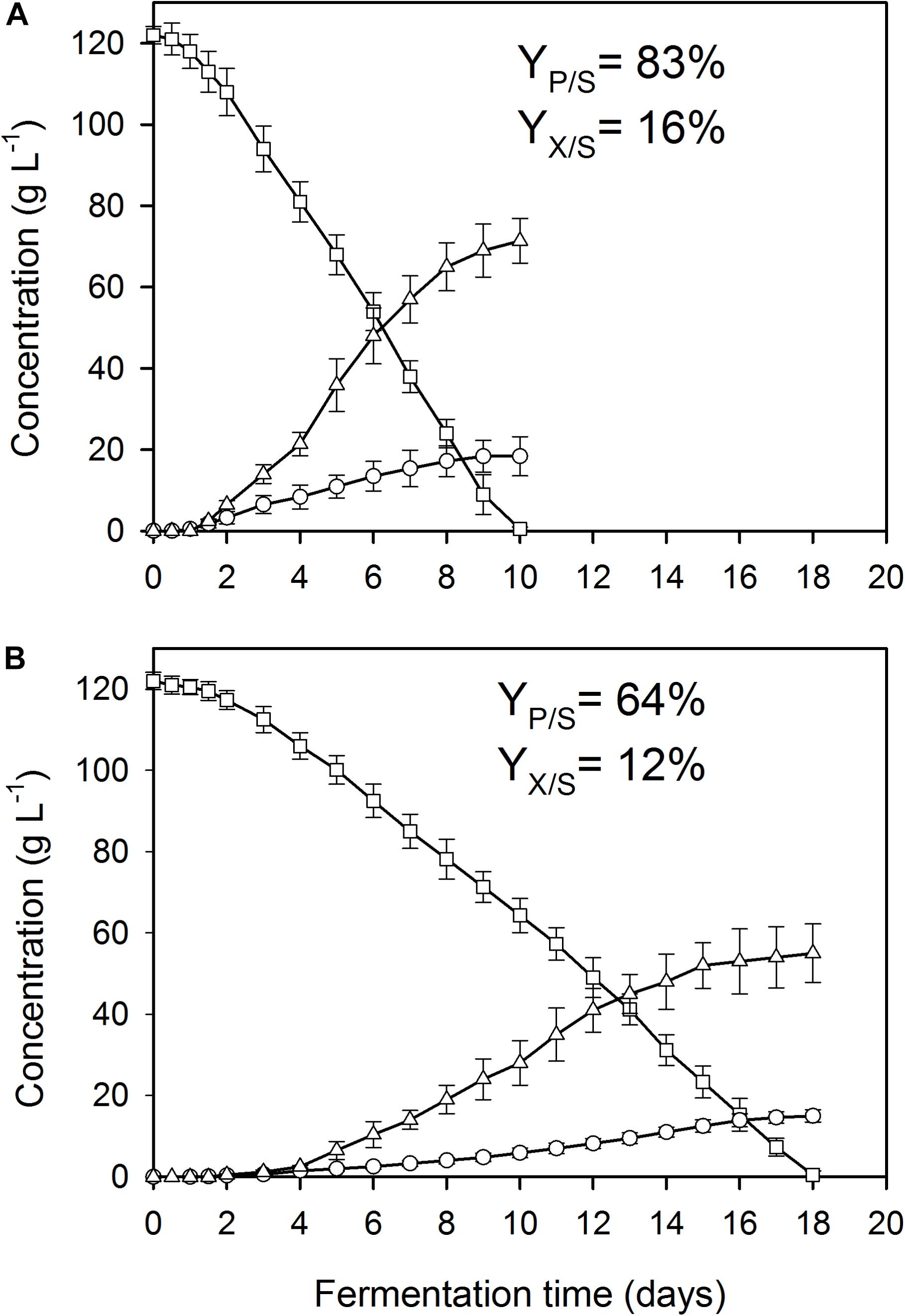
Figure 1. Kinetics of fungal biomass (O), itaconic acid (△), and residual D-glucose (□) concentrations in controlled batch fermentations of A. terreus NRRL 1960, grown in a 2-L scale bioreactor in the presence of 3 μg L–1 Mn2+ ions (manganese limitation). The variables share the same y-axis as they are expressed in the same unit (g L–1). Yp/s, molar itaconic acid yield; Yx/s, biomass (DCW) yield. (A) Culture supplemented with 3.3 mg L–1 Cu2+ ions. (B) Culture supplemented with 0.01 mg L–1 Cu2+ ions.
Mn2+ Inhibition of Itaconic Acid Formation Is Mitigated by Cu2+ Ions in a Carbon Source-Dependent Manner
As reported previously for D-glucose and D-xylose (Karaffa et al., 2015; Kolláth et al., 2019), increasing the extracellular Mn2+ ion concentrations in the medium (to 300 μg L–1 in this study) significantly lowered the maximal Yp/s of itaconic acid on all four carbon sources. At 3.3 mg L–1 Cu2+, i.e., the concentration conducing optimal itaconic acid production under conditions of Mn2+ paucity, and 300 μg L–1 Mn2+, the molar yield decreased by 21% on D-glucose (Figure 2A) and by 16% on D-fructose relative to the best conditions for itaconic acid production (Table 2A). Lowering the Cu2+ ion concentration down to 0.01 mg L–1 did not change biomass production of the cultures but significantly decreased molar itaconic acid yield (Figure 2B). However, increasing the copper(II) ion concentration within the same range of concentrations as in the earlier experiments with Mn2+ ion limited cultivations described above, gradually increased the specific molar itaconic acid yield. On D-glucose, essentially the same itaconic acid yields could be reached at 1.5 μg L–1 Mn2+/3.3 mg L–1 Cu2+ (Figure 1A) and at 300 μg L–1 Mn2+/300 mg L–1 Cu2+ (Figure 3). Similarly, molar yields could be restored to over 90% of the optimal yield on D-fructose by increasing the amount of Cu2+ ions by two orders of magnitude to counteract the 200-fold excess of Mn2+. However, contrary to the situation on the two glycolytic hexoses, the inhibitory effect of manganese(II) ion sufficiency on itaconic acid production was not fully alleviated by an excess of copper(II) ions in the case of either of the pentoses as the growth substrate. On D-xylose, Yp/s did increase with rising copper ion concentrations, but only to half of the maximal yield obtained at 1.5 μg L–1 Mn2+, whereas no attenuation could be observed on L-arabinose as the carbon source (Tables 2A,B).
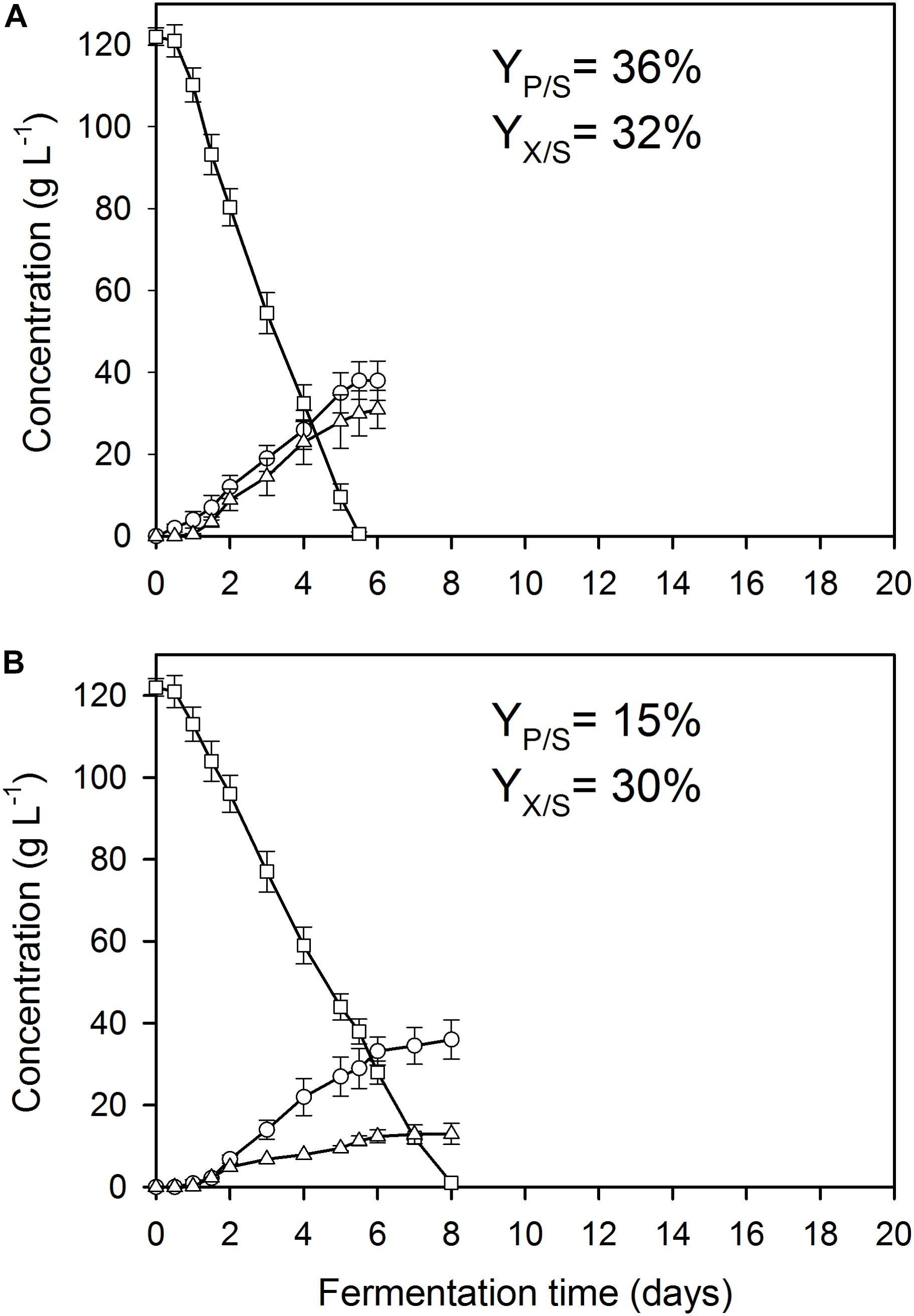
Figure 2. Kinetics of fungal biomass (O), itaconic acid (△), and residual D-glucose (□) concentrations in controlled batch fermentations of A. terreus NRRL 1960, grown in a 2-L scale bioreactor in the presence of 300 μg L–1 Mn2+ ions. The variables share the same y-axis as they are expressed in the same unit (g L–1). (A) Culture supplemented with 3.3 mg L–1 Cu2+ ions. (B) Culture supplemented with 0.01 mg L–1 Cu2+ ions.
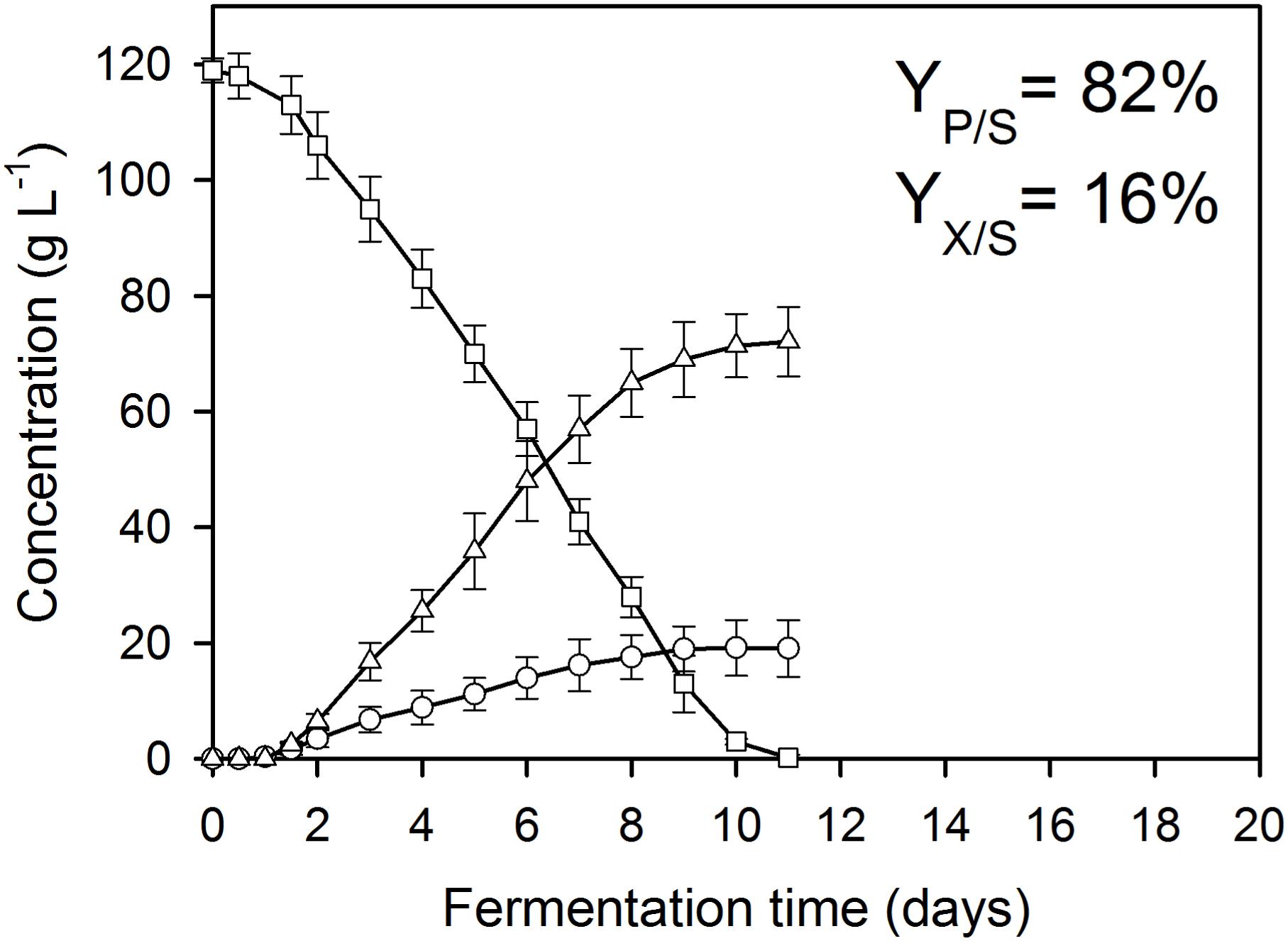
Figure 3. Kinetics of fungal biomass (O), itaconic acid (△), and residual D-glucose (□) concentrations in controlled batch fermentations of A. terreus NRRL 1960, grown in a 2-L scale bioreactor in the presence of 300 μg L–1 Mn2+ and 300 mg L–1 Cu2+ ions. The variables share the same y-axis as they are expressed in the same unit (g L–1).
The Ratio of Manganese and Copper Ions Concentrations Affects Fungal Morphology
Under conditions conductive to itaconic acid production, the morphology of D-glucose-grown A. terreus cultures is characterized by small, compact pellets and yeast-like cells (Supplementary Figure 2), rather than elongated hyphae (Karaffa et al., 2015). They are characterized by increases in cell diameter and decreases in pellet diameters. These two measurable parameters were therefore assessed for variation during the course of the fermentations. On D-glucose at 1.5 μg L–1 Mn2+ ions, the average cell diameter in the 24-h old cultures was 2.41 ± 0.58 μm when 0.01 mg L–1 Cu2+ ions were present in the culture broth, and displayed a continuous increase up until a maximum diameter was observed at 25 mg L–1 (Table 3A). Above 25–50 mg L–1 Cu2+ the trend reversed, and the average cell diameter were not significantly lower (3.57 ± 1.04) at 250 mg L–1 Cu2+ than they were at 0.01 mg L–1. The difference between the most extreme cell diameters observed was over four-fold, and the largest cell diameters were observed in the cultures with the highest molar itaconic acid yields. These trends were similar at each time-point tested, from a day after inoculation until carbon source exhaustion, although the span between the two most extreme cell diameter values decreased with the culture age. Pellet sizes followed an opposite pattern in that the pellets were at their largest at 0.01 mg L–1 Cu2+, and sharply decreased in diameter with increasing Cu2+ concentrations. The pellets were at their smallest at 50 mg L–1 Cu2+ (Table 4A).

Table 3A Average cell diameter in A. terreus NRRL1960 D-glucose-grown mycelia as a function of the copper(II) ion concentration in the culture broth at Mn(II) limitation and sufficiency.
Fungal morphology was fundamentally different in the presence of sufficient Mn2+ ions in the growth medium, 300 μg L–1. Maximal average cell diameters from early time-point samples were either smaller or similar than the later ones, and the diameters were generally lower than those measured under Mn2+ limitation (Table 3B). Cultures with Cu2+ ion concentrations in the range of 0.01 and 100 mg L–1 displayed mostly filamentous morphology with average cell diameters less than 2.5 μm. Cultures with copper concentrations above 100 mg L–1 had increasingly higher cell diameters, particularly in the later stages of cultivation (72, 96, 168 h). At 300 and 400 mg L–1 Cu2+ ions, average cell diameters were 60–70% of the values measured in cultures grown under itaconic acid production conditions (Table 3B). Our data suggest that variation in average cell diameter is correlated with the ratio of manganese(II) and copper(II) ions in the fermentation rather than with the concentration of either of the two cations. Hyphal diameter remained ∼ 2 μm during the course of the fermentation as long as the Mn:Cu ratio was higher than 1.2 × 10–3. However, average diameter of cells – as well as specific molar itaconic acid yields – significantly increased when the Mn:Cu ratio fell between 1 × 10–3 and 0.75 × 10–3. No such correlation was found in the cultures grown at low Mn2+ ion concentrations.
Macro-morphology at 300 μg L–1 Mn2+ ion and low-to-medium Cu2+ ion concentrations was constituted by overwhelmingly loose (so-called periferal hairy) regions where pellet diameters were larger than 350 μm (Table 4B). However, in the presence of more than 250 mg L–1 Cu2+, the size of these pellets decreased to diameters of 250 μm as an average, more resembling cultures with low Mn2+ and Cu2+ (Table 4A). Essentially identical results were obtained on D-fructose as the sole carbon source at both the low and the high Mn2+ concentration (data not shown).

Table 4A Average pellet size in A. terreus NRRL1960 D-glucose-grown cultures as a function of the copper(II) ion concentration in the culture broth at Mn(II) limitation and sufficiency.
D-Xylose-grown cultures likewise displayed the typical overflow-associated morphology under manganese limitation, with average cell diameters increasing with the Cu2+ concentration and with the cultivation time (Table 5A). However, in contrast to what was observed on D-glucose, formation of pellets with characteristic core region was observed at lower Cu2+ ion concentrations tested: >350 μm pellets at 0.01 mg L–1 and >250 μm pellets at 1 mg L–1, as opposed to the morphology seen at the standard Cu2+ ion concentration of 3.3 mg L–1, optimal for itaconic acid yield also on D-xylose (Table 6A). Under Mn2+ ion sufficient conditions, fungal morphology on D-xylose was generally similar to that in D-glucose fermentations (Tables 5B, 6B). However, no correlation could be observed between any of the morphological parameters investigated and the itaconic acid production measured either on this pentose or on L-arabinose.

Table 5A Average cell diameter in A. terreus NRRL1960 D-xylose-grown mycelia as a function of the copper(II) ion concentration in the culture broth at Mn(II) limitation and sufficiency.
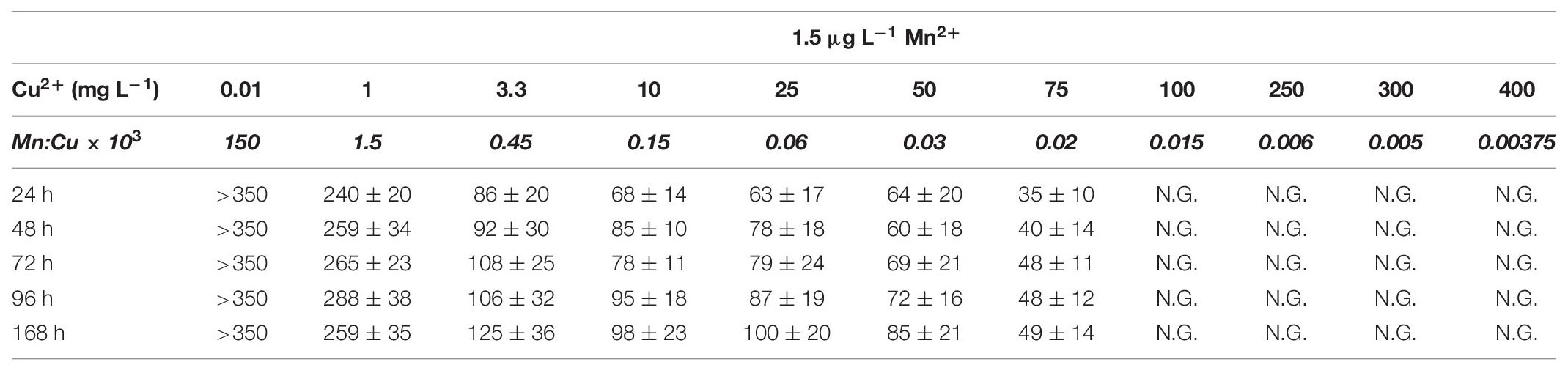
Table 6A Average pellet size in A. terreus NRRL1960 D-xylose-grown cultures as a function of the copper(II) ion concentration in the medium at Mn2+ limitation and sufficiency.
Discussion
In this paper, the effects of the interplay of Cu2+ and Mn2+ ions on growth, morphology and itaconic acid formation in A. terreus were analyzed and compared while growing on hexose (D-glucose, D-fructose) or pentose (D-xylose, L-arabinose) substrates, testing the hypothesis that the impact of excess Cu2+ ions on itaconic acid overflow is similar for all four monosaccharides. Copper is an essential transition metal ion. In fungi, it functions for instance, as a cofactor of enzymes of the respiratory chain, in free radical detoxification, pigmentation, and iron acquisition (Smith et al., 2017; Antsotegi-Uskola et al., 2020). An excess of copper ions, on the other hand, is toxic because it can inactivate other metalloenzymes by displacement of their functional divalent cation cofactor, and can catalyze the generation of radicals from hydrogen peroxide, a ubiquitous byproduct of oxidative respiration by the Fenton reaction (Smith et al., 2017; Antsotegi-Uskola et al., 2020). Cu2+ sensitivity is an interface between competing (micro)organisms. As an example, the innate phagocyte (alveolar macrophages and neutrophils) defense utilizes – in addition to other mechanisms – copper as a microbial toxin (Ding et al., 2014; García-Santamarina and Thiele, 2015). Consequently, all organisms have developed mechanisms to elude or alleviate Cu toxicity and intoxification (Garcia et al., 2014; Smith et al., 2017; Andrei et al., 2020; Linder, 2020). Some fungi – especially unicellular yeasts – produce copper-binding metallothioneins to modulate the concentration of the free cation (Mackie et al., 2016), whereas multicellular filamentous fungi (such as A. fumigatus and Fusarium oxysporum) make use of Cu2+-exporting ATPases (EC 7.2.2.9) to maintain intracellular copper homeostasis (Wiemann et al., 2017; Lorenzo-Gutiérrez et al., 2020). However, all organisms can only handle copper ions up to a certain threshold concentration, beyond which Cu-induced damage becomes irreversible.
We confirmed that A. terreus is sensitive to copper, and showed for four fermentable monosaccharides, that the actual toxicity threshold is dependent on the carbon growth substrate. This latter has, to the best of our knowledge, not been reported before and places a caveat on the comparisons of (heavy) metal sensitivity in the literature. Indeed, the copper tolerance is higher during growth on hexoses than on pentoses. Since the Cu2+-transporting ATPase is (in analogy to A. fumigatus; Wiemann et al., 2017) likely the major means to active copper disposal in A. terreus (the A. terreus ortholog of CrpA is encoded at locus ATET_04123; protein ID 76861), our observations suggest that copper export could be facilitated by an increased ATP pool. The generation of ATP from primary carbon source catabolism is considerably different between hexoses and pentoses: the catabolism of 1 mole of D-xylose or L-arabinose leads to the formation of only 0.83 mole ATP until the entry into the tricarboxylic acid cycle (Kolláth et al., 2019), whereas the catabolism of hexoses into acetyl-CoA (glycolysis) yields 2 ATP (Supplementary Figure 3). We may therefore assume that copper export is less efficient when growing on pentoses, which may lead to toxicity at lower copper ion concentrations.
An additional (or alternative) explanation for the difference in the copper sensitivity between growth on hexoses and pentoses could be the increased requirement for NADPH reduction equivalents: as explained above, excess of copper ions generates oxidative stress (hydroxyl radicals) by catalyzing a Fenton-like reaction. Studies in S. cerevisiae showed that oxidative stress triggers an up-regulation of genes coding for enzymes that use specifically NADPH as a cofactor, which function to revert glutathione and thioredoxin into their reduced state (Koerkamp et al., 2002). Since the total pool of NAD(P/H) is maintained in homeostasis (Croft et al., 2020), the availability of NADPH for glutathione and thioredoxin reducing enzymes is smaller during growth on pentoses than on hexoses. It would imply that the Cu2+ tolerance is lower when catabolizing L-arabinose than on D-xylose, because L-arabinose catabolism requires two NADPH whereas that of D-xylose requires one (Supplementary Figure 3). This is in fact what our data have shown.
The observation that A. terreus tolerates higher concentrations of copper ions during conidiospore germination – in a similar carbon source dependent manner – may simply be a consequence of the short time of the germination phase when compared to the whole growth process (until carbon source exhaustion). We assume that until the end of germination only a part of the supplied copper ions have entered the cell if any. During spore germination, the cell mobilizes intracellular deposits of metabolites, including trace elements like Mn2+ or Cu2+, as the necessary uptake capacity has to be developed first.
The addition of excess copper ions to the culture medium has been patented as a method to promote high yields of citric acid during fermentations of A. niger on molasses (Schweiger, 1958). Subsequently, Cu2+ supplementation was also reported to increase itaconic acid yields in A. terreus (Batti and Schweiger, 1963). In these two patents, addition of copper was shown to counteract the negative effect of ferrous iron ions (Fe2+) on the product titers obtained. However, it was not recognized that the addition of Fe2+ also introduced additional Mn2+ ions, which are present as impurities in the iron salts utilized in the medium formulations, in sufficient amounts to account for the negative effect of Mn2+ on citric acid production observed in A. niger (Kubicek and Röhr, 1985). Kisser et al. (1980) demonstrated that Cu2+ ions can mitigate the inhibition of citrate accumulation in the presence of Mn2+ ions.
Here, we have demonstrated that Cu2+ ions also alleviate the negative effect of Mn2+ ions on itaconic acid formation in A. terreus (Karaffa et al., 2015; Kolláth et al., 2019), especially on hexose carbon sources. By contrast, Cu2+ ions could only partially counteract the Mn2+ inhibition on D-xylose while no alleviation could be observed in L-arabinose cultures which accumulated little itaconic acid under any cultivation regime. These differences may be related with the increased sensitivity for Cu2+ when the fungus is grown on pentoses.
The mechanism by which copper ions counteract the negative effect of manganese ions on itaconic (or citric-) acid formation is unclear, although it has been suggested that copper ions may compete for the manganese uptake system (Netik et al., 1997). Hockertz et al. (1987) reported that copper(II) is a potent inhibitor of a high-affinity manganese transporter system in A. niger, and an excess of copper would thus prevent intracellular accumulation of Mn2+. However, if this were the sole mechanism by which Cu2+ actively counteracts Mn2+, why does not it work on xylose?
As explained above, copper catalyzes the accumulation of oxygen radicals from hydrogen peroxide in the mitochondria which are counteracted by the activity of a manganese-dependent superoxide dismutase (MnSOD). MnSOD is usually found in the mitochondrial matrix (Weisiger and Fridovich, 1973; Okado-Matsumoto and Fridovich, 2001) and exists as a homotetramer (Ravindranath and Fridovich, 1975; Wispe et al., 1989; Borgstahl et al., 1992). Under conditions of manganese deficiency, function of MnSOD is compromised leading to severe manifestations of oxidative stress (Borrello et al., 1992; Allen et al., 2007). All metabolic changes that have been reported in A. niger under manganese deficiency, such as increased protein turnover and changes in plasma membrane lipid composition (Ma et al., 1981; Meixner-Monori et al., 1984) are typical for cells under oxidative stress. It is possible that – besides inhibiting manganese uptake – excess copper ions can cause increased oxidative strain by catalyzing the Fenton-like conversion of hydrogen peroxide into the highly reactive hydroxyl radical, even in the presence of manganese in concentrations that normally provide adequate protection against ROS.
We also demonstrated that interplay of Mn2+ and Cu2+ ions strongly influence the morphology of A. terreus. In A. niger, Mn2+-deficient morphology coincides with a significant alteration in cell wall polymer composition (Kisser et al., 1980). Mn2+ ions in the Golgi apparatus can influence protein glycosylation, regulation of sorting and vesicular traffic, and removal of toxic levels of ions. Mannosyltransferases located in the Golgi require Mn2+ as a cofactor (Lobsanov et al., 2004). Crystal structures of several glycosyltransferases revealed that Mn2+ binds to a conserved DXD motif in the catalytic site (Gastinel et al., 2001; Persson et al., 2001; Lobsanov et al., 2004). In A. fumigatus, a galactofuranosyltransferase that requires Mn2+, and whose deletion cripples polarized growth and the ability to sporulate has been described (Komachi et al., 2013; Katafuchi et al., 2017). A very similar phenotype has also been observed in A. niger during growth under manganese deficiency: galactose-containing cell wall polysaccharides (including beta-galactofurans and galactomannans) were strongly reduced under these conditions (Kisser et al., 1980). In analogy, the morphological changes that occur in A. terreus as a consequence of the availability of Mn2+ ions in the culture broth could be due to a requirement of a manganese cofactor by glycosyltranferases, galactofuranosyltransferases and mannosyltransferases involved in protein glycosylation and the synthesis of cell wall polysaccharides. In A. niger, the addition of 1 mM (=63.5 mg L–1) Cu2+ ions to cultures pregrown under manganese sufficiency results in a transition into the overflow-associated morphology typical for manganese deficiency (Kisser et al., 1980). In A. terreus, we observed this shift upon the addition of >300 mg L–1 copper, i.e., at four to five times higher concentrations, when the Mn2+:Cu2+ ratio fell below 1.2 × 10–3. This quantitative difference regarding manganese-copper interplay in the two Aspergilli remains unexplained at this time.
Data Availability Statement
The original contributions presented in the study are included in the article/Supplementary Material, further inquiries can be directed to the corresponding author.
Author Contributions
ES, IK, and LK conceived this study. IK, ES, EF, and LK designed the experiments. IK, VB, and ES performed the experiments. All authors analyzed the data. BK contributed essential analysis tools. LK, EF, and ES supervised the experimental work and data analysis. LK and EF wrote grant proposals and obtained funding. ES, MF, CK, and LK wrote the manuscript. All authors read and approved the final manuscript.
Funding
This work was supported by the European Union and co-financed by the European Regional Development Fund, under grant GINOP-2.3.2-15-2016-00008; by the EFOP-3.6.1-16-2016-00022 project co-financed by the European Union and the European Social Fund; and by the Hungarian National Research, Development and Innovation Fund, grants KH 129602 and NN 128867, to LK. EF received a János Bolyai Research Scholarship from the Hungarian Academy of Science (BO/00093/18/8) and was supported by the ÚNKP-20-5-DE-13 New National Excellence Program of the Ministry for Innovation and Technology from the source of the National Research, Development and Innovation Fund. Funding for open access charge: Hungarian National Research, Development and Innovation Fund (NN 128867 to LK).
Conflict of Interest
The authors declare that the research was conducted in the absence of any commercial or financial relationships that could be construed as a potential conflict of interest.
Acknowledgments
The authors are grateful to Anett Gédl, Gabriella Győrfi, and Tamás Járvás for their contributions to the experimental work.
Supplementary Material
The Supplementary Material for this article can be found online at: https://www.frontiersin.org/articles/10.3389/fmicb.2021.680420/full#supplementary-material
Footnotes
References
Allen, M. D., Kropat, J., Tottey, S., Del Campo, J. A., and Merchant, S. S. (2007). Manganese deficiency in Chlamydomonas results in loss of photosystem II and MnSOD function, sensitivity to peroxides, and secondary phosphorus and iron deficiency. Plant Physiol. 143, 263–277. doi: 10.1104/pp.106.088609
Andrei, A., Öztürk, Y., Khalfaoui-Hassani, B., Rauch, J., Marckmann, D., Trasnea, P.-I., et al. (2020). Cu homeostasis in bacteria: the ins and outs. Membranes 10:242. doi: 10.3390/membranes10090242
Antsotegi-Uskola, M., Markina-Iñarrairaegui, A., and Ugalde, U. (2020). New insights into copper homeostasis in filamentous fungi. Int. Microbiol. 23, 65–73. doi: 10.1007/s10123-019-00081-5
Bartoshevich, Y. E., Zaslavskaya, P., Novak, M., and Yudina, O. (1990). Acremonium chrysogenum differentiation and biosynthesis of cephalosporin. J. Basic Microbiol. 30, 313–320. doi: 10.1002/jobm.3620300503
Batti, A. M., and Schweiger, L. B. (1963). Process for the Production of Itaconic Acid. US Patent 3078217A. Washington DC: U.S. Patent Office.
Borgstahl, G. E., Parge, H. E., Hickey, M. J., Beyer, W. F. Jr., Hallewell, R. A., and Tainer, J. A. (1992). The structure of human mitochondrial manganese superoxide dismutase reveals a novel tetrameric interface of two 4-helix bundles. Cell 71, 107–118. doi: 10.1016/0092-8674(92)90270-m
Borrello, S., De Leo, M., and Galeotti, T. (1992). Transcriptional regulation of MnSOD by manganese in the liver of manganese-deficient mice and during rat development. Biochem. Intern. 28, 595–601.
Croft, T., Venkatakrishnan, P., and Lin, S.-J. (2020). NAD+ metabolism and regulation: lessons from yeast. Biomolecules 10:330. doi: 10.3390/biom10020330
Cunha da Cruz, J., Machado, de Castro, A., and Camporese Sérvulo, E. F. (2018). World market and biotechnological production of itaconic acid. 3 Biotech. 8:138. doi: 10.1007/s13205-018-1151-0
Detroy, R. W., and Ciegler, A. (1971). Induction of yeastlike development in Aspergillus parasiticus. Microbiology 65, 259–264. doi: 10.1099/00221287-65-3-259
Ding, C., Festa, R. A., Sun, T. S., and Wang, Z. Y. (2014). Iron and copper as virulence modulators in human fungal pathogens. Mol. Microbiol. 93, 10–23. doi: 10.1111/mmi.12653
El-Sabbagh, N., Harvey, L., and McNeil, B. (2008). Effects of dissolved carbon dioxide on growth, nutrient consumption, cephalosporin C synthesis and morphology of Acremonium chrysogenum in batch cultures. Enzyme Microb. Technol. 42, 315–324. doi: 10.1016/j.enzmictec.2007.10.012
Fekete, E., Karaffa, L., Sándor, E., Seiboth, B., Bíró, S., Szentirmai, A., et al. (2002). Regulation of the intracellular beta-galactosidase activity of Aspergillus nidulans. Arch. Microbiol. 179, 7–14. doi: 10.1007/s00203-002-0491-496
Garcia, L., Welchen, E., and Gonzalez, D. H. (2014). Mitochondria and copper homeostasis in plants. Mitochondrion 19, 269–274. doi: 10.1016/j.mito.2014.02.011
García-Santamarina, S., and Thiele, D. J. (2015). Copper at the fungal pathogen-host Axis. J. Biol. Chem. 290, 18945–18953. doi: 10.1074/jbc.R115.649129
Gastinel, L. N., Bignon, C., Misra, A. K., Hindsgaul, O., Shaper, J. H., and Joziasse, D. H. (2001). Bovine α1, 3-galactosyltransferase catalytic domain structure and its relationship with ABO histo-blood group and glycosphingolipid glycosyltransferases. EMBO J. 20, 638–649. doi: 10.1093/emboj/20.4.638
Gyamerah, M. (1995). Factors affecting the growth form of Aspergillus terreus NRRL 1960 in relation to itaconic acid fermentation. Appl. Microbiol. Biotechnol. 44, 356–361. doi: 10.1007/BF00169929
Hajian, H., and Yusoff, W. M. W. (2015). Itaconic acid production by microorganisms: a review. Curr. Res. J. Biol. Sci. 7, 37–42. doi: 10.19026/crjbs.7.5205
Hevekerl, A., Kuenz, A., and Vorlop, K.-D. (2014). Influence of the pH on the itaconic acid production with Aspergillus terreus. Appl. Microbiol. Biotechnol. 98, 10005–10012. doi: 10.1007/s00253-014-6047-2
Hockertz, S., Schmid, J., and Auling, G. (1987). A specific transport system for manganese in the filamentous fungus Aspergillus niger. Microbiology 133, 3513–3519. doi: 10.1099/00221287-133-12-3513
Karaffa, L., Díaz, R., Papp, B., Fekete, E., Sándor, E., and Kubicek, C. P. (2015). A deficiency of manganese ions in the presence of high sugar concentrations is the critical parameter for achieving high yields of itaconic acid by Aspergillus terreus. Appl. Microbiol. Biotechnol. 99, 7937–7944. doi: 10.1007/s00253-015-6735-6
Karaffa, L., and Kubicek, C. P. (2019). Citric acid and itaconic acid accumulation: variations of the same story? Appl. Microbiol. Biotechnol. 103, 2889–2902. doi: 10.1007/s00253-018-09607-9
Karaffa, L., Sándor, E., Kozma, J., and Szentirmai, A. (1997). Methionine enhances sugar consumption, fragmentation, vacuolation and cephalosporin-C production in Acremonium chrysogenum. Process Biochem. 32, 495–499. doi: 10.1016/S0032-9592(97)00003-4
Katafuchi, Y., Li, Q., Tanaka, Y., Shinozuka, S., Kawamitsu, Y., Izumi, M., et al. (2017). GfsA is a β1,5-galactofuranosyltransferase involved in the biosynthesis of the galactofuran side chain of fungal-type galactomannan in Aspergillus fumigatus. Glycobiology 27, 568–581. doi: 10.1093/glycob/cwx028
Kisser, M., Kubicek, C. P., and Röhr, M. (1980). Influence of manganese on morphology and cell wall composition of Aspergillus niger during citric acid fermentation. Arch. Microbiol. 128, 26–33. doi: 10.1007/BF00422301
Koerkamp, M. G., Rep, M., Bussemaker, H. J., Hardy, G. P. M. A., Mul, A., Piekarska, K., et al. (2002). Dissection of transient oxidative stress response in Saccharomyces cerevisiae by using DNA microarrays. Mol. Biol. Cell 13, 2783–2794. doi: 10.1091/mbc.e02-02-0075
Kolláth, I. S., Molnár, ÁP., Soós, Á, Fekete, E., Sándor, E., Kovács, B., et al. (2019). Manganese deficiency is required for high itaconic acid production from D-xylose in Aspergillus terreus. Front. Microbiol. 10:1589. doi: 10.3389/fmicb.2019.01589
Komachi, Y., Hatakeyama, S., Motomatsu, H., Futagami, T., Kizjakina, K., Sobrado, P., et al. (2013). gfsA encodes a novel galactofuranosyltransferase involved in biosynthesis of galactofuranose antigen of O-glycan in Aspergillus nidulans and Aspergillus fumigatus. Mol. Microbiol. 90, 1054–1073. doi: 10.1111/mmi.12416
Kozma, J., and Karaffa, L. (1996). Effect of oxygen on the respiratory system and cephalosporin-C production in Acremonium chrysogenum. J. Biotechnol. 48, 59–66. doi: 10.1016/0168-1656(96)01400-9
Kubicek, C., and Röhr, M. (1985). Aconitase and citric acid fermentation by Aspergillus niger. Appl. Environ. Microbiol. 50, 1336–1338. doi: 10.1128/AEM.50.5.1336-1338.1985
Kuenz, A., Gallenmüller, Y., Willke, T., and Vorlop, K.-D. (2012). Microbial production of itaconic acid: developing a stable platform for high product concentrations. Appl. Microbiol. Biotechnol. 96, 1209–1216. doi: 10.1007/s00253-012-4221-y
Kuenz, A., and Krull, S. (2018). Biotechnological production of itaconic acid—things you have to know. Appl. Microbiol. Biotechnol. 102, 3901–3914. doi: 10.1007/s00253-018-8895-7
Linder, M. C. (2020). Copper homeostasis in mammals, with emphasis on secretion and excretion. A Review. Intern. J. Mol. Sci. 21:4932. doi: 10.3390/ijms21144932
Lobsanov, Y. D., Romero, P. A., Sleno, B., Yu, B., Yip, P., Herscovics, A., et al. (2004). Structure of Kre2p/Mnt1p: a yeast α1, 2-mannosyltransferase involved in mannoprotein biosynthesis. J. Biol. Chem. 279, 17921–17931. doi: 10.1074/jbc.M312720200
Lorenzo-Gutiérrez, D., Gómez-Gil, L., Guarro, J., Roncero, M. I. G., Capilla, J., and López-Fernández, L. (2020). Cu transporter protein CrpF protects against Cu-induced toxicity in Fusarium oxysporum. Virulence 11, 1108–1121. doi: 10.1080/21505594.2020.1809324
Ma, H., Kubicek, C. P., and Röhr, M. (1981). Malate dehydrogenase isoenzymes in Aspergillus niger. FEMS Microbiol. Lett. 12, 147–151.
Mackie, J., Szabo, E. K., Urgast, D. S., Ballou, E. R., Childers, D. S., MacCallum, D. M., et al. (2016). Host-imposed copper poisoning impacts fungal micronutrient acquisition during systemic Candida albicans infections. PLoS One 11:e0158683. doi: 10.1371/journal.pone.0158683
Meixner-Monori, B., Kubicek, C. P., and Röhr, M. (1984). Pyruvate kinase from Aspergillus niger: a regulatory enzyme in glycolysis? Can. J. Microbiol. 30, 16–22. doi: 10.1139/m84-003
Molnár, ÁP., Németh, Z., Kolláth, I. S., Fekete, E., Flipphi, M., Ág, N., et al. (2018). High oxygen tension increases itaconic acid accumulation, glucose consumption, and the expression and activity of alternative oxidase in Aspergillus terreus. Appl. Microbiol. Biotechnol. 102, 8799–8808. doi: 10.1007/s00253-018-9325-6
Netik, A., Torres, N. V., Riol, J.-M., and Kubicek, C. P. (1997). Uptake and export of citric acid by Aspergillus niger is reciprocally regulated by manganese ions. Biochim. Biophys. Acta Biomembr. 1326, 287–294. doi: 10.1016/S0005-2736(97)00032-1
Okabe, M., Lies, D., Kanamasa, S., and Park, E. Y. (2009). Biotechnological production of itaconic acid and its biosynthesis in Aspergillus terreus. Appl. Microbiol. Biotechnol. 84, 597–606. doi: 10.1007/s00253-009-2132-3 doi:
Okado-Matsumoto, A., and Fridovich, I. (2001). Subcellular distribution of superoxide dismutases (SOD) in rat liver: Cu, Zn-SOD in mitochondria. J. Biol. Chem. 276, 38388–38393. doi: 10.1074/jbc.M105395200
Paul, G., Kent, C., and Thomas, C. (1994). Hyphal vacuolation and fragmentation in Penicillium chrysogenum. Biotechnol. Bioeng. 44, 655–660. doi: 10.1002/bit.260440513
Paul, G. C., and Thomas, C. R. (1998). Characterisation of mycelial morphology using image analysis. Relat. Morphol. Process Perform. 60, 1–59. doi: 10.1007/BFb0102278
Persson, K., Ly, H. D., Dieckelmann, M., Wakarchuk, W. W., Withers, S. G., and Strynadka, N. C. (2001). Crystal structure of the retaining galactosyltransferase LgtC from Neisseria meningitidis in complex with donor and acceptor sugar analogs. Nat. Struct. Biol. 8, 166–175. doi: 10.1038/84168
Ravindranath, S., and Fridovich, I. (1975). Isolation and characterization of a manganese-containing superoxide dismutase from yeast. J. Biol. Chem. 250, 6107–6112.
Saha, B. C. (2003). Hemicellulose bioconversion. J. Industr. Microbiol. Biotechnol. 30, 279–291. doi: 10.1007/s10295-003-0049-x
Saha, B. C., and Kennedy, G. J. (2019a). Efficient itaconic acid production by Aspergillus terreus: overcoming the strong inhibitory effect of manganese. Biotechnol. Prog. 36:e2939. doi: 10.1002/btpr.2939
Saha, B. C., and Kennedy, G. J. (2019b). Phosphate limitation alleviates the inhibitory effect of manganese on itaconic acid production by Aspergillus terreus. Biocatalys. Agric. Biotechnol. 18:101016. doi: 10.1016/j.bcab.2019.01.054
Saha, B. C., Kennedy, G. J., Qureshi, N., and Bowman, M. J. (2017). Production of itaconic acid from pentose sugars by Aspergillus terreus. Biotechnol. Prog. 33, 1059–1067. doi: 10.1002/btpr.2485
Schweiger, L. B. (1958). Production of Citric Acid by Fermentation. US Patent US2970084A. Washington, DC: U.S. Patent Office.
Smith, A. D., Logeman, B. L., and Thiele, D. J. (2017). Copper acquisition and utilization in fungi. Annu. Rev. Microbiol. 71, 597–623. doi: 10.1146/annurev-micro-030117-020444
Weisiger, R. A., and Fridovich, I. (1973). Mitochondrial superoxide dismutase site of synthesis and intramitochondrial localization. J. Biol. Chem. 248, 4793–4796.
Wiemann, P., Perevitsky, A., Lim, F. Y., Shadkchan, Y., Knox, B. P., Landero Figueora, J. A., et al. (2017). Aspergillus fumigatus copper export machinery and reactive oxygen intermediate defense counter host copper-mediated oxidative antimicrobial offense. Cell Rep. 19, 2174–2176. doi: 10.1016/j.celrep.2017.05.075
Keywords: Aspergillus terreus, manganese(II) ions, copper(II) ions, itaconic acid overflow, pentose, hexose, mycelial pellets, divalent cation antagonism
Citation: Sándor E, Kolláth IS, Fekete E, Bíró V, Flipphi M, Kovács B, Kubicek CP and Karaffa L (2021) Carbon-Source Dependent Interplay of Copper and Manganese Ions Modulates the Morphology and Itaconic Acid Production in Aspergillus terreus. Front. Microbiol. 12:680420. doi: 10.3389/fmicb.2021.680420
Received: 14 March 2021; Accepted: 19 April 2021;
Published: 20 May 2021.
Edited by:
Saurabh Dhiman, South Dakota School of Mines and Technology, United StatesReviewed by:
Amit Ghosh, Indian Institute of Technology Kharagpur, IndiaShuji Tani, Osaka Prefecture University, Japan
Copyright © 2021 Sándor, Kolláth, Fekete, Bíró, Flipphi, Kovács, Kubicek and Karaffa. This is an open-access article distributed under the terms of the Creative Commons Attribution License (CC BY). The use, distribution or reproduction in other forums is permitted, provided the original author(s) and the copyright owner(s) are credited and that the original publication in this journal is cited, in accordance with accepted academic practice. No use, distribution or reproduction is permitted which does not comply with these terms.
*Correspondence: Levente Karaffa, bGV2ZW50ZS5rYXJhZmZhQHNjaWVuY2UudW5pZGViLmh1