- 1Departmen of Studies in Microbiology, University of Mysore, Mysuru, India
- 2Department of Microbiology and Immunology, Faculty of Pharmacy, Mansoura University, Mansoura, Egypt
- 3School of Nutrition Sciences, Faculty of Health Sciences, University of Ottawa, Ottawa, ON, Canada
The use of probiotics and antifungal capabilities of the lactic acid bacteria (LAB) isolated from different niches is a strategy to prepare functional cultures and biopreservatives for food/feed industries. In the present study, LAB strains isolated from an Indian traditional fermented food, Pozha, were evaluated for their probiotic properties and biocontrol potential. A total of 20 LAB isolates were selected from Pozha samples collected aseptically and screened for their antagonistic activity against Fusarium verticillioides. Among the bioactive isolates, Lacticaseibacillus brevis MYSN105 showed the highest antifungal activity in vitro, causing some morphological alterations such as damaged mycelia and deformed conidia. Cell-free supernatant (CFS) from L. brevis MYSN105 at 16% concentration effectively reduced the mycelial biomass to 0.369 g compared to 1.938 g in control. Likewise, the conidial germination was inhibited to 20.12%, and the seed treatment using CFS induced a reduction of spore count to 4.1 × 106 spores/ml compared to 1.1 × 109 spores/ml for untreated seeds. The internal transcribed spacer (ITS) copy number of F. verticillioides decreased to 5.73 × 107 and 9.026 × 107 by L. brevis MYSN105 and CFS treatment, respectively, compared to 8.94 × 1010 in control. The L. brevis MYSN105 showed high tolerance to in vitro gastrointestinal conditions and exhibited high adhesive abilities to intestinal epithelial cell lines. The comparative genome analysis demonstrated specific secondary metabolite region coding for bacteriocin and T3PKS (type III polyketide synthase) possibly related to survival and antimicrobial activity in the gut environment. Our results suggest that L. brevis MYSN105 has promising probiotic features and could be potentially used for developing biological control formulations to minimize F. verticillioides contamination and improve food safety measures.
Introduction
Contamination of food/feed products by mycotoxigenic fungi and the presence of mycotoxins have attracted the attention of the scientific and economic world due to the major economic losses and reduction in food/feed quality. The main sources of fungal colonization in food/feed originate from plants, primarily cereals used to formulate food/feed (Lee and Ryu, 2017; Nematollahi et al., 2019). It has been estimated by Food and Agriculture Organizations (FAO) that 25% of the world’s food/feed commodities are contaminated with mycotoxigenic fungi (Fraeyman et al., 2017).
The fungal species more often encountered with intoxication and mycotoxicoses in cereal-based food/feed belong primarily to three genera Aspergillus, Fusarium, and Penicillium. Fusarium species are the most common fungi associated with cereal contamination worldwide, affecting the quality and shelf-life of cereal-based products. The nutritional characteristics of cereal grains make them susceptible to contamination by Fusarium species (Deepa et al., 2016). For instance, Fusarium verticillioides is considered the main cereal pathogen, with a wide range of plant hosts and fumonisin production (Deepa and Sreenivasa, 2017). Fumonisins traditionally associated with cereals and cereal-based products can induce both acute and chronic toxic effects in poultry and animals. Fumonisin-contaminated cereal-based products have increased the incidence of esophageal and liver cancer (Khan et al., 2018; Arumugam et al., 2019) while causing neural discomforts in humans. In animals, fumonisin is associated with several mycotoxicoses, pulmonary edema in pigs, and decreased bodyweight, immune disorders, and liver sclerosis in poultry (Pósa et al., 2014; Vendruscolo et al., 2016). Fumonisin B1, in particular, is classified as carcinogenic to poultry and animals (Coppock and Dziwenka, 2015).
Safe elimination of this mycotoxigenic fungus from food/feed is of paramount importance because of their health-related issues and significant economic losses. Most physical and chemical strategies followed to reduce fungal contamination have been shown to be relatively ineffective or difficult to implement into the production process. Many fungicides used to control F. verticillioides contamination in cereal grains are conventional chemical agents, which negatively impact the environment and human health (Algharibeh and AlFararjeh, 2019). Therefore, biological control is the best alternative to these chemical agents, as they do not cause adverse effects on the environment while not being harmful to humans and livestock. Among the biocontrol agents, probiotic lactic acid bacteria (LAB) represent a potent and interesting strategy, as they are widely used in food/feed and are part of beneficial gut microbiota capable of producing antimicrobial metabolites. There are different reports of using bacteria to control mycotoxigenic Fusarium species (Nagaraja et al., 2016; Deepthi et al., 2017). However, probiotic LAB has not been used so far to control F. verticillioides.
Probiotics are live microorganisms used in food supplements, which confer beneficial effects on the host by colonizing the gastrointestinal tract (GIT) (Gibson et al., 2004). These microorganisms have important features such as antiallergic, anticancer, and antimicrobial properties; enhancing host immune function; or reducing lipid and serum cholesterol (Elbanna et al., 2018). For many years, fermented foods have been considered for their beneficial properties while being enriched with beneficial LAB (Behera et al., 2020), such as Lactiplantibacillus plantarum, Lactobacillus acidophilus, Leuconostoc species, and Lacticaseibacillus casei (Deepthi et al., 2016; Yadav et al., 2016; Zommiti et al., 2017; Muhammad et al., 2019).
South Indian tribes have an ancient tradition in food technology, with fermented food being very popular across the country and consumed at breakfast, lunch, and dinner. One such fermented food is Pozha prepared at the household level in the Kerala region, composed of coconut milk, rice, and coconut water, which is not well characterized and varies with the region of production. In order to improve the safety and quality of this food internationally, there is a need to explore their microbiota, particularly LAB as biopreservatives.
Many food matrices similar to Pozha are used to isolate LAB that are promising biocontrol agents due to their antagonistic activity against fungal species (Kharazian et al., 2017; Siedler et al., 2019) and their ability to detoxify mycotoxins (Chlebicz and Śliżewska, 2020). Their antifungal activity has been associated with the production of several bioactive metabolites, including organic acids, fatty acids, and peptides (Luz et al., 2017; Kwak et al., 2018). Likewise, different studies have reported the capacity of LAB to degrade mycotoxins to less toxic intermediate compounds and are used as biopreservatives in food/feed industries (Martinez Tuppia et al., 2017). In this context, the present study aimed to isolate new LAB strains from an Indian traditional fermented food Pozha and evaluate their probiotic features and in vitro antifungal activity against F. verticillioides.
Materials and Methods
Sampling and Isolation of Lactic Acid Bacteria From Pozha
Five different samples of Indian traditional fermented food Pozha were collected aseptically from different regions of Kerala state of India. A total of 10 g from each sample was suspended aseptically in 90 ml de Man Rogosa Sharpe (MRS) broth (pH 6.5) and homogenized by vortexing. Each sample was enriched by incubating anaerobically at 37°C for 48 h. Each suspension was then serially diluted in phosphate-bufferred saline (PBS), plated on MRS agar plates, and incubated for 48 h at 37°C under anaerobic conditions. Single isolated colonies were selected and purified by repeated streaking, then were grown overnight in MRS broth at 37°C and stored at −20°C in 25% (w/v) glycerol.
Preliminary Screening and Characterization of LAB Isolates for Antifungal Activity
Antifungal activity of the LAB isolates against F. verticillioides MTCC 8792 was determined using the agar overlay method described by Magnusson and Schnürer (2001) with slight modifications. Briefly, the LAB isolates were inoculated in 3-cm lines on MRS agar plates and incubated at 37°C for 24 h in anaerobic jars. The plates were then overlaid with 10 ml of potato dextrose soft agar (PDA) containing 106 spores/ml of F. verticillioides. These plates were incubated at 28°C for 4 days, and the clear zone of inhibition was observed. The LAB isolates showing significant antifungal activity were selected for further studies.
The bioactive LAB isolates were presumptively identified by phenotypic analysis like colony morphology, Gram staining, and catalase assay, as previously described (Somashekaraiah et al., 2019). The Gram-positive and catalase-negative isolates were tested for acid–bile tolerance, autoaggregation, and cell-surface hydrophobicity as per Yadav et al. (2016). Furthermore, the isolates were tested for their antimicrobial activity against indicator microorganisms. The most promising antifusarial isolate MYSN105 was selected for further phenotypic and genotypic characterization and presented in this study.
Molecular Identification of LAB Isolate
The selected LAB isolate MYSN105 was characterized genotypically based on 16S ribosomal DNA (rDNA) sequencing (Belfiore et al., 2013). The genomic DNA isolated using Qiagen DNeasy blood and tissue kit (Qiagen, Hilden, Germany) was subjected to 16S rDNA amplification using the universal primers, Bact8F (AGAGTTTGATCCTGGCTCAG) (Edwards et al., 1989) and 1391R (GACGGGCGGTGTGTRCA) (Lane et al., 1985), and Sanger sequencing. The generated sequence was analyzed using the National Center for Biotechnology Information (NCBI) BLAST tool (Altschul et al., 1990). The sequences of L. brevis MYSN105 and other representative strains were aligned using the computer software MEGA X 10.1.8, while the phylogenetic tree was constructed using the neighbor-joining method (Kumar et al., 2018).
Probiotic Characterization of L. brevis MYSN105
Antibiotic Susceptibility
The antibiotic susceptibility of the selected L. brevis MYSN105 was assessed according to the European Food Safety Authority (EFSA, 2012) technical guidelines. The overnight grown culture was inoculated onto the surface of MRS agar plates by swabbing, and the antibiotic MIC strips (Hi Media, Mumbai, India) were applied. Plates were incubated anaerobically at 37°C for 24 h. After incubation, the plates were observed for inhibition zones, and the antibiotic sensitivity was expressed as sensitive or resistant (Noda et al., 2019).
Antibacterial Activity
The inhibitory effects of L. brevis MYSN105 against bacterial pathogens Escherichia coli ATCC 25922, Staphylococcus aureus ATCC 6538, and Salmonella Paratyphi ATCC 9150 were measured using the agar-well diffusion method as per Ridwan et al. (2008).
Tolerance to Gastrointestinal Conditions
The viability of the L. brevis MYSN105 exposed to simulated GIT conditions was determined, according to Soares et al. (2019), with some modifications. The overnight culture of L. brevis MYSN105 in MRS broth was centrifuged at 8,000 rpm for 10 min. The cell pellets were washed twice with PBS and resuspended in the same buffer and serially diluted to obtain a final concentrate of 107 CFU/ml. The cells were then exposed to the action of pepsin (3 g/L) and lipase (0.9 g/L), corrected to pH 2.0 using a 1 M solution of sterile hydrochloric acid (HCl) simulating gastric phase. To simulate enteric phase I, bile salts (10 g/L) and pancreatin (1 g/L) were added, and the pH was adjusted to 5 using a 1 M solution of sodium hydroxide (NaOH). Finally, to simulate enteric phase II, 10 g/L of bile salts and 1 g/L of pancreatin were added to the sample again, and the pH was adjusted to 7. The sample was maintained at 37°C on a shaker (200 rpm) for 3 h. Samples were collected at 0, 2, 4, and 6 h, subjected to serial dilution using PBS buffered at pH 6.5. Aliquots of 100 μl were plated onto the MRS agar and incubated anaerobically at 37°C for 24 h to determine the viable count. The experimental procedures were performed in triplicates. The survival rate of the L. brevis MYSN105 was expressed using the following formula:
Adhesion Assay on Caco-2 and HT-29 Cells
Caco-2 (ATCC HTB-37) and HT-29 (ATCC HTB-38) cells were cultured in Dulbecco’s modified Eagle’s medium (DMEM) at 37°C under a 5% CO2 atmosphere. Cells were seeded in 24-well plates and used at their confluence (>90% estimated by microscopic observation, reached after 3 days) to avoid association with the well. Adhesion assay was adopted from Moroni et al. (2006). The overnight grown cell cultures were washed twice (6,000 × g for 5 min) and resuspended at 6 × 108 CFU/ml in sterile PBS. Adhesion assay was carried out using 100 μl of L. brevis MYSN105 and 100 μl of DMEM without serum and added to each well to attain a 100:1 ratio of bacteria to cell lines. Plates were incubated for 2 h at 37°C under a 5% CO2 atmosphere, drained, and washed three times with sterile PBS. Cells with adherent bacteria were harvested using Trypsin–ethylenediaminetetraacetic acid (EDTA) for 10 min at 37°C, and 200 μl of DMEM media was then added. Adherent L. brevis MYSN105 was enumerated on MRS agar incubated anaerobically at 37°C for 24 h. The results were expressed as the adhesion index, which refers to the number of bacteria adhering to 100 Caco-2 and HT-29 cells.
Growth Kinetics of L. brevis MYSN105 and Acidification Profile
The growth kinetics and acidification profile of L. brevis MYSN105 were assessed as per Hammami et al. (2009) with modifications. Briefly, the strain was inoculated in MRS broth and incubated anaerobically at 37°C for 24 h, and absorbance at 600 nm and pH decrease were measured every 2 h using a spectrophotometer and pH meter. The values are represented as means of two independent experiments in triplicates.
Whole-Genome Sequencing of L. brevis MYSN105
DNA was extracted from an overnight culture of L. brevis MYSN105 in MRS broth using NucleoSpin Microbial DNA kit (Macherey-Nagel, Duren, Germany) as per the manufacturer. The extracted DNA was quantified with a Qubit fluorometer (Invitrogen, Carlsbad, CA, United States) and stored at −20°C till use. The whole-genome sequencing library was prepared using NexteraTM DNA Flex Library Prep (Illumina; San Diego, CA, United States) as per its protocol. The prepared library was paired end sequenced (2 × 151 bp) in a 1/20 MiSeq run with Illumina MiSeq platform (NuGut Research Platform, University of Ottawa, Ottawa, ON, Canada) using 300 bp MiSeq Reagent Kit v2 (Illumina).
Genome Assembly and Analyses of L. brevis MYSN105
The generated reads were de novo assembled using the Velvet Assembler V1.0.0 incorporated in Illumina BaseSpace Sequence Hub (Illumina). Kraken (Wood and Salzberg, 2014) was used to assign the taxonomy to the generated reads. The assembled contigs were annotated using the Prokka V2.4.1 (Seemann, 2014) and Rapid Annotation using Subsystem Technology (RAST) server (Aziz et al., 2008; Overbeek et al., 2014; Brettin et al., 2015). Mining for secondary metabolite genes in the assembled genome was conducted via antiSMASH 5.0 (Blin et al., 2019). The assembled contigs were submitted to the Phage Search Tool Enhanced Release (PHASTER) (Arndt et al., 2016) to identify and annotate the prophage sequences L. brevis MYSN105 genome.
Antifungal Activity of L. brevis MYSN105 and Its Cell-Free Supernatant
Agar-Overlay Assay
The antifungal activity of L. brevis MYSN105 was determined using the agar overlay method, as described above in preliminary screening.
Antifungal Activity by Microdilution Method
The antifungal assay was done using 96-well microtiter plates described by Hammami et al. (2009) with some modifications. The L. brevis MYSN105-CFS at different concentrations was diluted using PD broth and transferred to wells, which were subsequently seeded with 106 spores/ml of F. verticillioides per well. The microplates were incubated at 28°C for 24 h, and the absorbance at 600 nm was measured every 20 min using The SPARK® multimode microplate reader (Tecan, Männedorf, Switzerland). The antifungal activity was plotted in comparison to the positive control.
Minimum fungicidal concentration (MFC) values of L. brevis MYSN105-CFS was expressed as the percentage of the CFS used for dilution in PD broth corresponding to the lowest concentration that inhibited the growth of F. verticillioides (OD600 nm) after 24 h of incubation. The MFC values were reported as means of two independent experiments in triplicates.
Residual Bioactivity of MYSN105-CFS After Treatment With Heat, Proteinase K, or pH Neutralization
The residual bioactivity of MYSN105-CFS was assessed following treatments with protease, pH neutralization, and high temperature, as described by Boubezari et al. (2018). The CFS was tested for its susceptibility to proteinase K (1 mg/ml) treatment incubated at 37°C for 2 h, followed by enzyme inactivation at 80°C for 10 min. To assess the thermal stability, CFS was heated at 100°C for 15 min and then cooled to room temperature. The effect of pH on CFS was tested by adjusting the pH to 7 using sterile 1 M NaOH. After each treatment, the residual antifungal activity was determined in triplicates by the microdilution method, as described above. The untreated CFS was used as a control.
Fungal Biomass Inhibition Assay
The fungal biomass inhibition was carried out using L. brevis MYSN105 and its CFS, as described by Valan Arasu et al. (2013). To prepare CFS, L. brevis MYSN105 was inoculated in MRS broth and incubated at 37°C for 24 h, then fermented broth centrifuged (8,000 rpm for 10 min) and filter sterilized (0.22 μm syringe filter, Millipore, New York, NY, United States). One hundred-milliliter potato dextrose broth (PDB) flasks preinoculated with the strain at 106 CFU/ml or CFS at different dilutions (4, 8, 12, and 16%) were inoculated with a 7-mm diameter fungal disk of F. verticillioides. The flasks were then incubated at 28°C for a week. The flask without strain or CFS was used as control. The fungal mycelial mat was harvested and dried in a hot air oven at 50°C for 2 h. The dry weight of the fungal biomass of each treatment was measured and compared to the control. The experiment was carried out in triplicates.
Conidia Germination Inhibition Assay
The 24-well microtiter plate was used to evaluate the inhibitory effects of L. brevis MYSN105 and its CFS on the conidial germination of F. verticillioides (Deepthi et al., 2016). To 1.0 ml 0.1 M PBS in the well, 100 μl of L. brevis MYSN105 (106 CFU/ml) and 106 spores/ml of F. verticillioides were added. Another reaction mixture of 16% CFS in 1.0 ml of 0.1 M PBS, along with 106 spores/ml of F. verticillioides, was added into the well. The reaction mixture with 106 spores/ml of F. verticillioides and 1.0 ml of 0.1 M PBS was considered a control. The microtiter plate was incubated at 28°C for 24 h. The conidial germination was observed periodically at 2, 4, 8, 16, and 24-h time intervals, and the germinated conidia were counted using a hemocytometer. The conidia germination percentage was calculated using the formula: (number of conidia germinated/total conidia counted) × 100.
In vitro qPCR-Based Antifungal Activity
The quantification of fungal growth was performed by quantitative PCR (qPCR). The F. verticillioides samples treated with L. brevis MYSN105 or its CFS were collected at different times for 14 days. The qPCR amplification was performed using 20 ng of DNA with 0.5 μM primers ITS1-F 5′-CTTGGTCATTTAGAGGAAGTAA-3′ (Gardes and Bruns, 1993) and ITS2-R 5′-GCTGCGTTCTTCATCGATGC-3′ (White et al., 1990) and 1 × SsoAdvanced Universal SYBR Green Supermix (Bio-Rad, Hercules, CA, United States) in a total volume of 25 μl. The reaction mixture contained 1x SYBR Green Mastermix, 0.5 μM of forward and reverse primer, 20 ng of template DNA, and ddH2O to the 25 μl reaction volume. Amplification conditions consisted of a preliminary step of 5 min at 95°C followed by 40 cycles at 95°C for 15 s and 60°C for 30 s. A no-template control for primer pair was included in the run. The results were analyzed using Bio-Rad CFX Maestro software and Microsoft Office Excel based on Cq values observed. The Cq values generated by qPCR were compared with the standard curve to quantify the internal transcribed spacer (ITS) copy number of F. verticillioides in different treatments.
In situ Biocontrol of F. verticillioides in Maize Seeds
Biocontrol assay of F. verticillioides in maize seeds was performed as per Yang and Chang (2010) with slight modifications. The maize seeds were surface sterilized using 2% sodium hypochlorite solution for 10 min and later soaked in sterile distilled water for 3 h. The maize seeds were further soaked in CFS for about 4 h at room temperature. The seeds (approximately 10 g) were then placed in sterile Petri dishes. The seeds were then spiked with 50 μl of F. verticillioides (106 spores/ml), and the maize seeds without CFS treatment were considered as control. The plates were incubated at 28°C for 7 days, and the growth of F. verticillioides was observed.
Statistical Analysis
The results are expressed as mean ± standard error (SE) of triplicate values of independent experiments. Analysis of statistical significance was performed with Student’s t-test using GraphPad Prism8, where appropriate p < 0.05 is considered significant.
Results
Isolation, Screening, and Molecular Characterization of Bioactive LAB Isolates
After enrichment of microbes from Pozha, we obtained around 20 different isolates based on their colony morphology and catalase test. These isolates were subjected to screening for their antifungal activity (preliminary results), and the most potent isolate was selected for further characterization. The results confirmed that the MYSN105 isolate was a Gram-positive, rod-shaped, and catalase-negative bacterium. The MYSN105 isolate was non-hemolytic and was characterized as a hetero-fermentative LAB. The isolated MYSN105 was able to ferment glucose, xylose, arabinose, mannose, galactose, cellobiose, xylitol, arabitol, and lactose while producing organic acids (Table 1). The MYSN105 isolate was identified as Lactobacillus brevis (newly named as Levilactobacillus brevis) through Sanger sequencing of its 16S ribosomal RNA (rRNA) amplicon and sequence alignment (99.77% identity). The phylogenetic tree analysis was carried out to verify the isolate’s evolutionary position, which was constructed based on 16S rDNA sequences retrieved from the GenBank database (Figure 1). The 16S rRNA sequence of MYSN105 was submitted to GenBank under the accession number of MN911442 and designated as L. brevis MYSN105 MN911442.
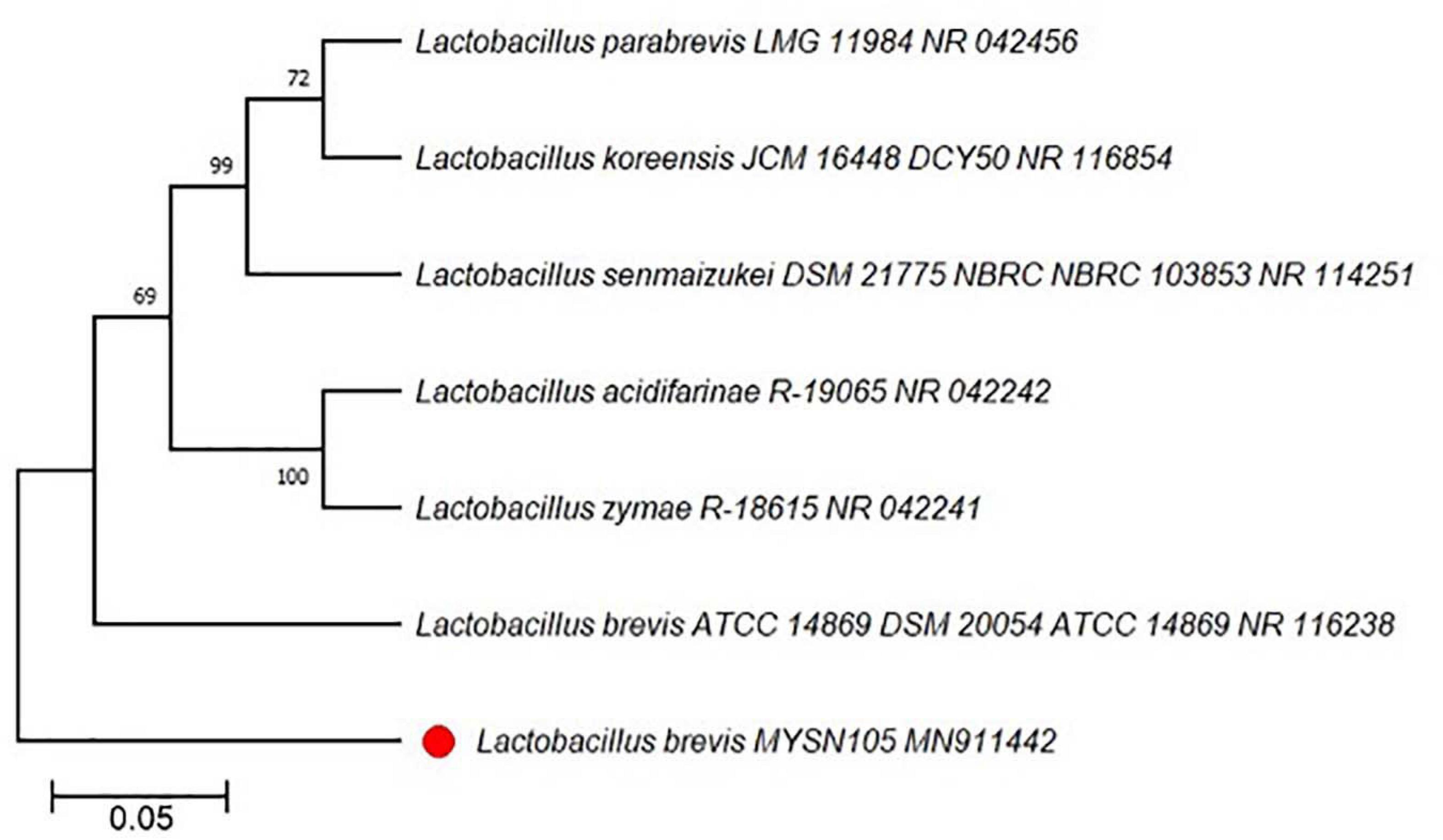
Figure 1. Phylogenetic tree showing the relative position of Lactobacillus brevis MYSN105 MN911442 marked with red taxon marker, using the neighbor-joining method of partial 16S rDNA sequences. Reference strains used for the comparison are labeled with their accession numbers of the 16SrDNA sequences. Bootstrap values for 100 replicates are shown at the nodes of the tree, using MEGA X 10.1.8. The scale bar corresponds to 0.05 U of the number of base substitutions per site.
Probiotic Features of L. brevis MYSN105
Resistance to GIT Conditions
As shown in Table 1, the L. brevis MYSN105 strain survived at pH 2, 3, and 6 for 3 h of incubation with cell count (log CFU/ml–1) of 3.22 ± 1.20, 4.94 ± 1.01, and 5.71 ± 1.91, respectively. The strain also exhibited a strong tolerance to bile salts for 4 h of incubation with cell count (log CFU/ml–1) of 5.29 ± 2.11 and 6.17 ± 1.26 at 2% and 4% bile salt concentration, respectively. Likewise, in the presence of GIT digestive enzymes, the survival rate of the strain after 6 h of incubation was 64.7% (Table 1).
Antibiotic Susceptibility of L. brevis MYSN105
The antibiotic susceptibility of L. brevis MYSN105 was tested against various antibiotics generally recommended by EFSA guidelines. The results were obtained by measuring the inhibition zone, indicating the minimum inhibitory concentration (MIC) at the intersection point between the inhibition zone and the strip edge. Compared to the MIC breakpoints recommended by EFSA, the MYSN105 strain was sensitive to ampicillin, gentamicin, erythromycin, clindamycin, tetracycline, and chloramphenicol while being resistant to kanamycin and streptomycin (Table 2).
Adhesion to Intestinal Cell Lines
The adhesion of L. brevis MYSN105 to Caco-2 and HT-29 cells was evaluated along with autoaggregation and cell surface hydrophobicity. The adhesion index of L. brevis MYSN105 to Caco-2 cells was estimated as 659.66 and to HT-29 cells as 1,066. Besides, the autoaggregation capability of L. brevis MYSN105 was 58.99%, while the cell surface hydrophobicity of MYSN105 was 65.90%.
Growth Kinetics and Acidification Profile
Figure 2 illustrates the growth kinetics of L. brevis MYSN105 with the pH variation for 24 h. The stationary phase was attained after 20 h of incubation, reaching the maximum growth, and OD remained stable up to 24 h. The acidification profile was assessed using pH measurement, which decreased from 6.7 to 4.79 as growth reached the stationary phase and 4.4 at 24 h.
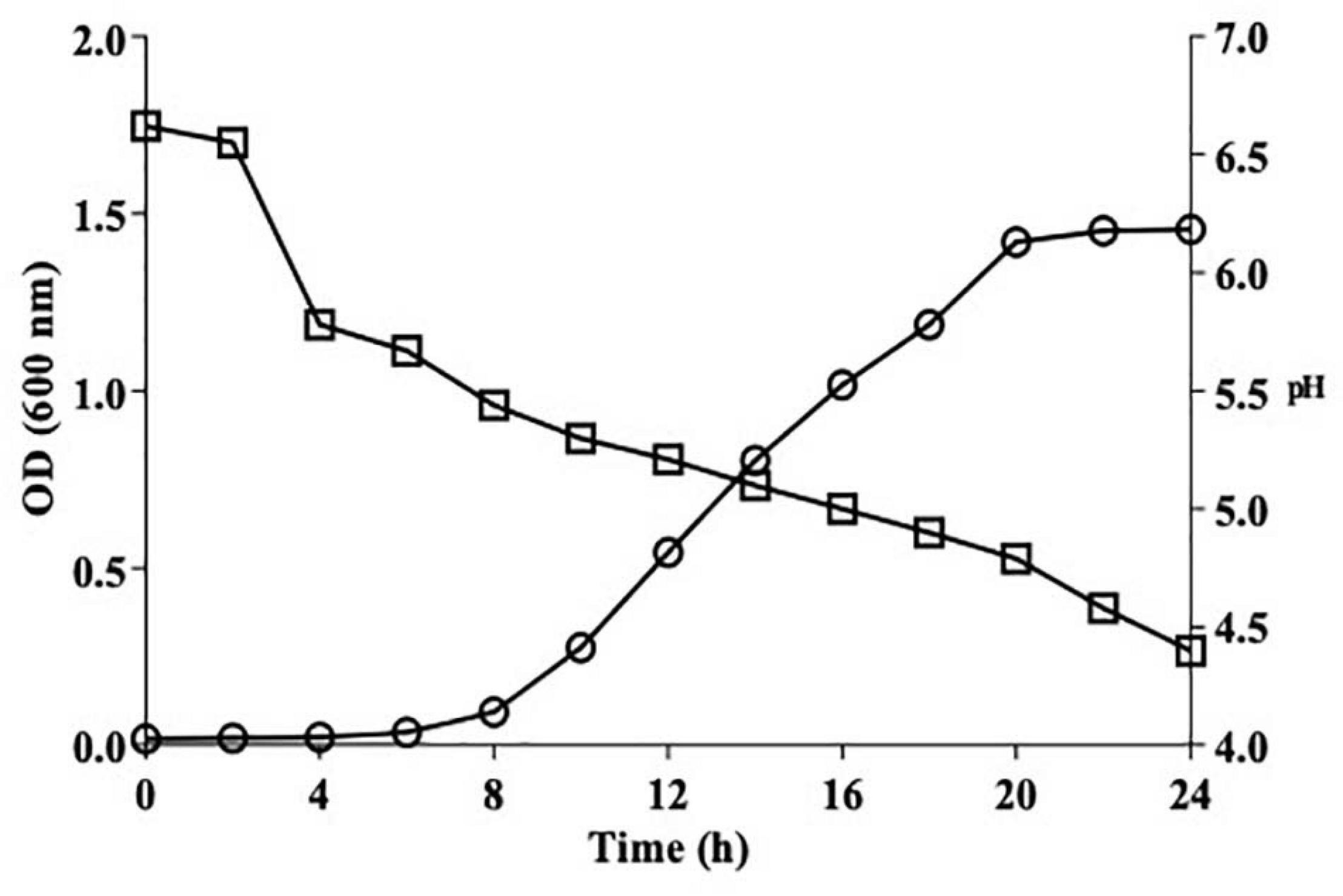
Figure 2. Growth kinetics (circle) and acidification profile (square) of L. brevis MYSN105 in MRS broth at 37°C for 24 h.
Genome Characteristics of L. brevis MYSN105
While Kraken assigned 95% of the reads to Lactobacillus, 84% of the generated reads were assigned to L. brevis. Those reads were assembled in a draft genome of size 2,097,265 base with 46.27% GC content. Prokka annotation predicted 1,849 genes, 1,834 of which are protein-coding genes. Of the genes, 1,096 have a non-hypothetical function, and 491 with seed subsystem ontology. On the other hand, RAST annotation identified 2,926 CDs, with 1,315 assigned a functional category (Figure 3). AntiSMASH database identified two secondary metabolite regions, one for bacteriocin and the second for T3PKS (type III polyketide synthase). Four incomplete prophage sequence regions (3.8–7.6 kb) were identified in the genome, all belong to the PHAGE_Lactob_LBR48_NC_027990. The assembled genome was deposited in GenBank under bioproject accession number PRJNA679667.
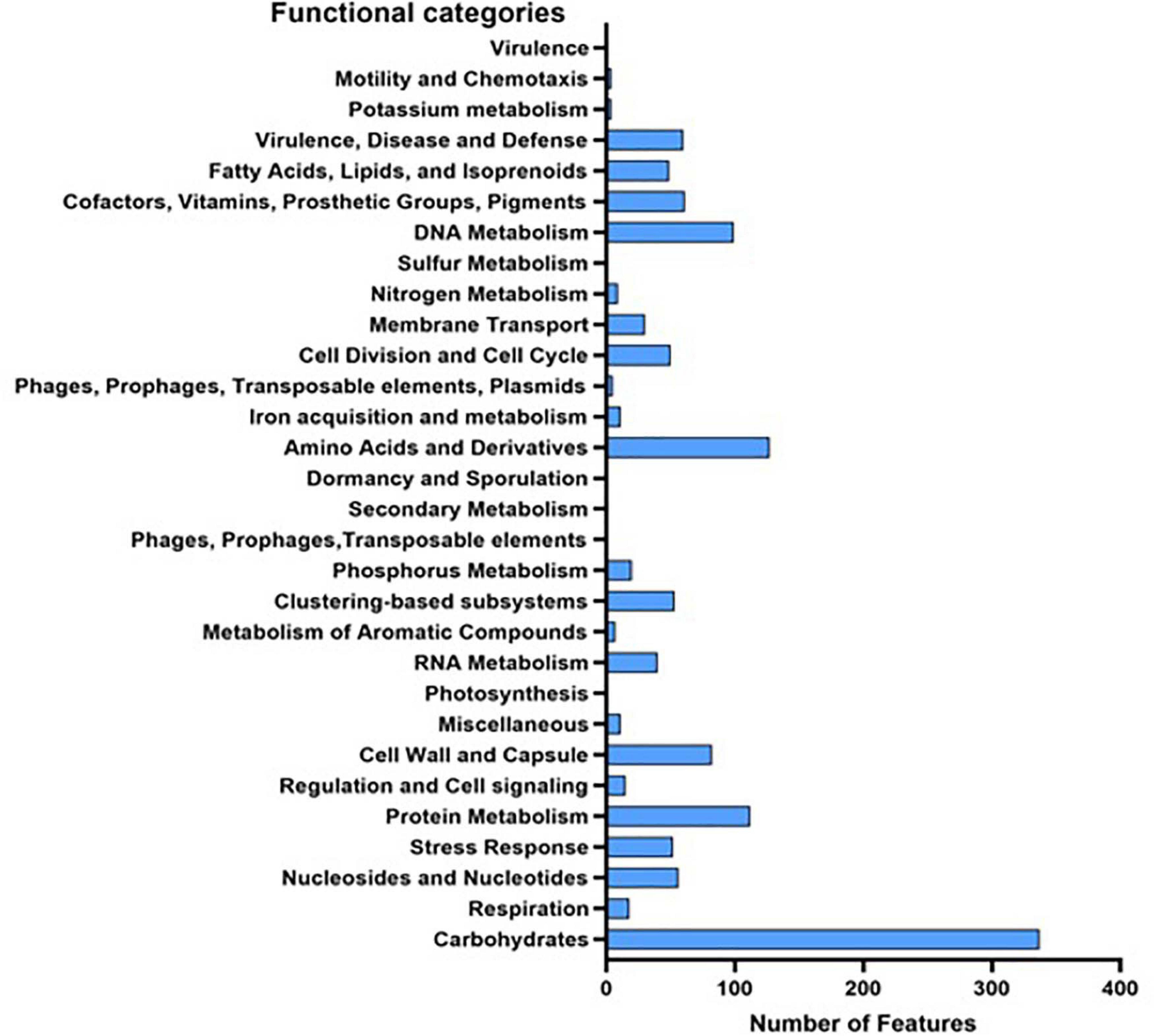
Figure 3. Functional categories of all predicted genes in L. brevis MYSN105 genome based on RAST and Prokka annotation.
Antimicrobial Activity of L. brevis MYSN105 and Its CFS
Antibacterial Activity
The antibacterial effect of L. brevis MYSN105 was also tested on different foodborne bacteria, and the effective inhibition is summarized in Table 1.
Antifungal Activity on F. verticillioides
The strain L. brevis MYSN105 exhibited potent inhibitory activity against F. verticillioides, as shown in Figure 4. The strain also reduced the mycelial biomass of the target fungus, with biomass reduced to 0.679 ± 0.20 g after 14 days of incubation compared to the control (1.938 ± 0.15 g) (Figure 5A). In addition, the CFS from L. brevis MYSN105 strongly inhibited the F. verticillioides spore formation (Figure 6). The CFS showed a dose-dependent inhibition of F. verticillioides (Figure 7). The MFC was found to be 16% of CFS, inhibiting 85.71% of the spores compared to control. Likewise, after 14 days of treatment with 16% CFS, the biomass of F. verticillioides was highly decreased (0.369 ± 0.21 g) compared to control (1.938 ± 0.15 g) (Figure 5A). The absolute quantification of fungal growth in the presence of L. brevis MYSN105 and its CFS was also performed by qPCR. The results indicated that the fungal ITS copy number was decreased to 5.73 × 107 and 9.026 × 107 in the presence of L. brevis MYSN105 or CFS, respectively, in comparison to 8.94 × 1010 copies of the positive control (Figure 8).
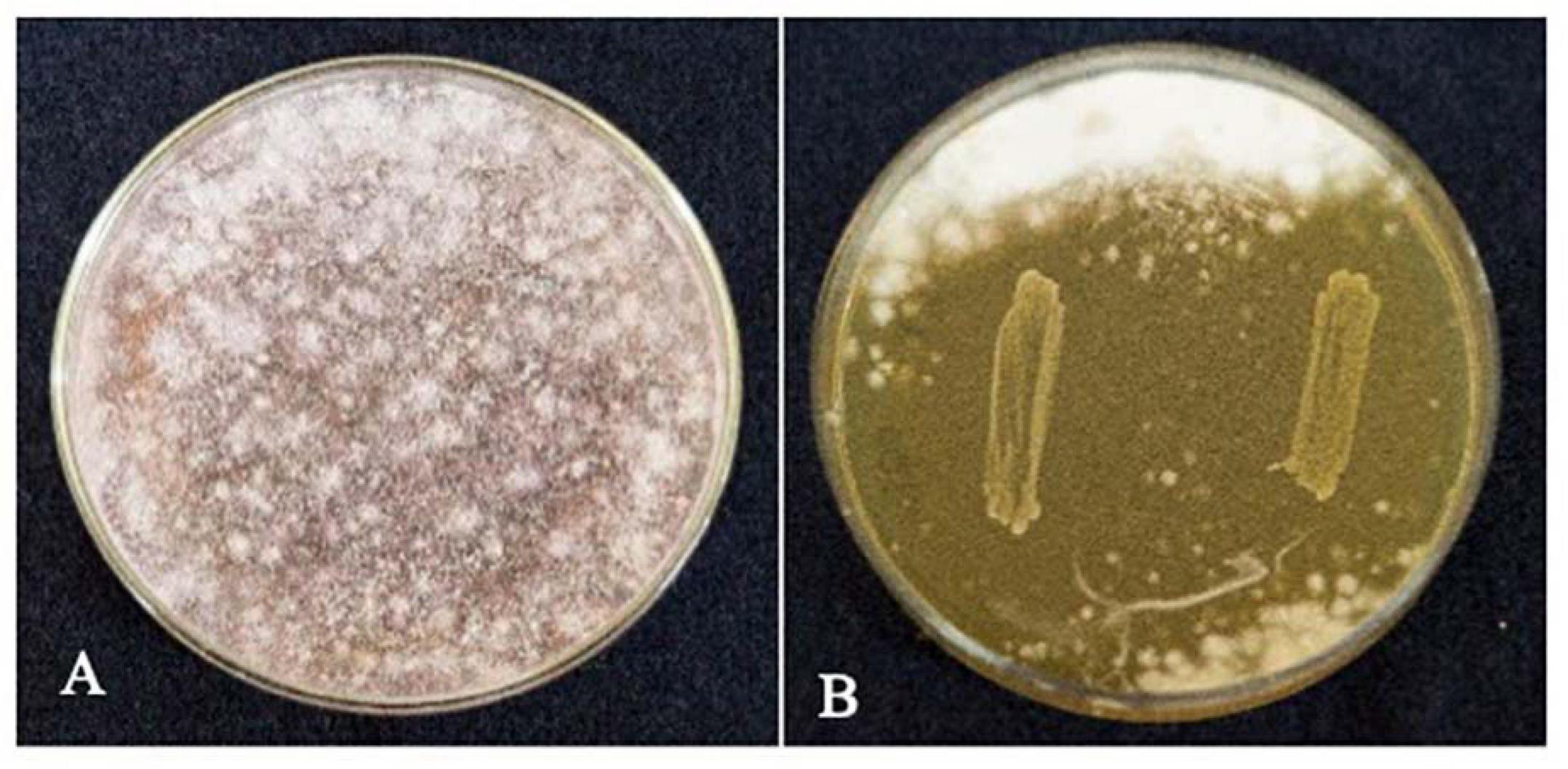
Figure 4. Inhibition of F. verticillioides by L. brevis MYSN105 by agar overlay method after 4 days incubation at 26°C. (A) Control F. verticillioides. (B) L. brevis MYSN105 overlaid by F. verticillioides.
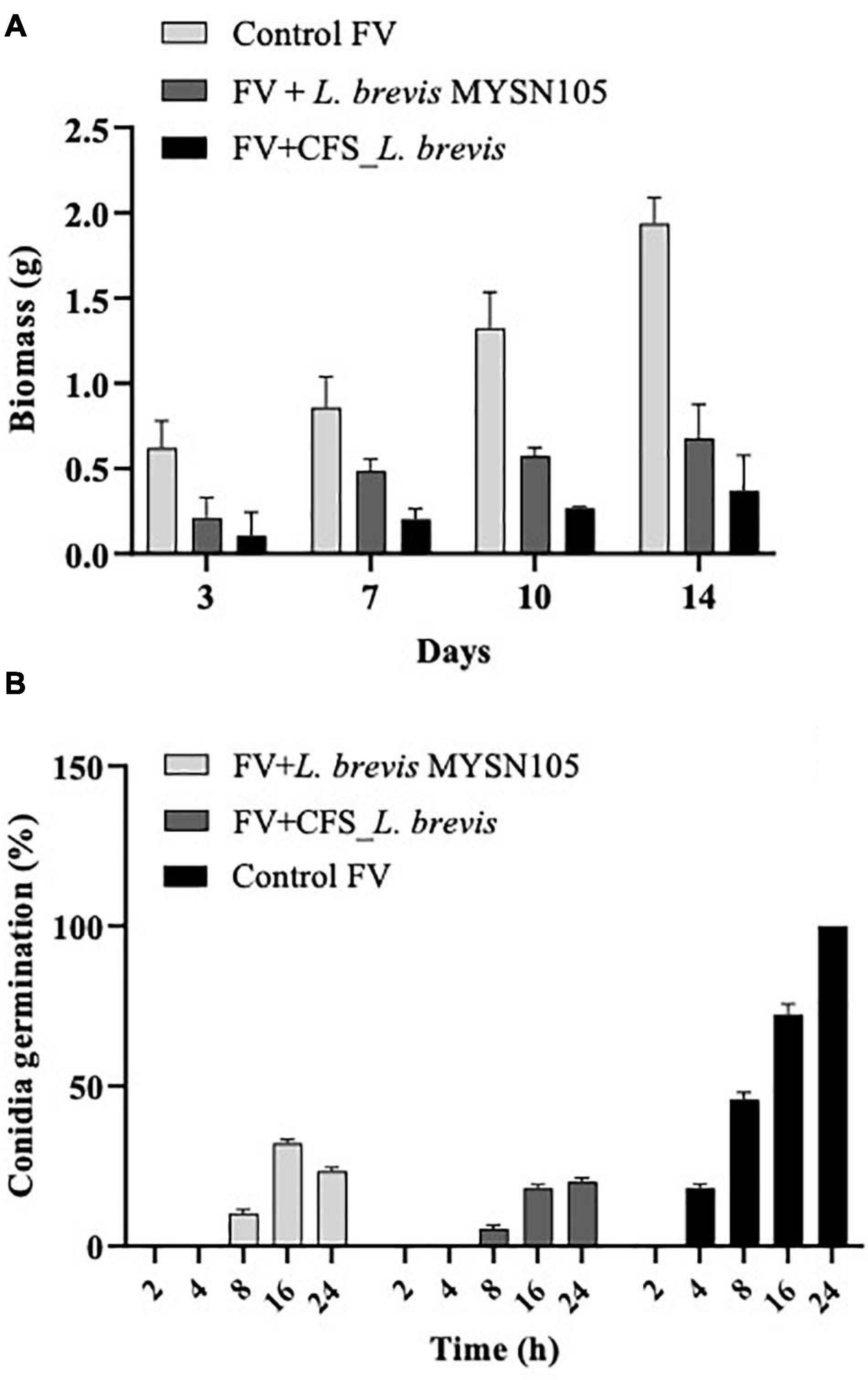
Figure 5. Effect of L. brevis MYSN105 and its CFS on fungal biomass and conidia germination. (A) Fungal biomass reduction by L. brevis MYSN105. (B) Conidia germination inhibition by L. brevis MYSN105. Data shown are mean ± SE of triplicate values of three independent experiments.
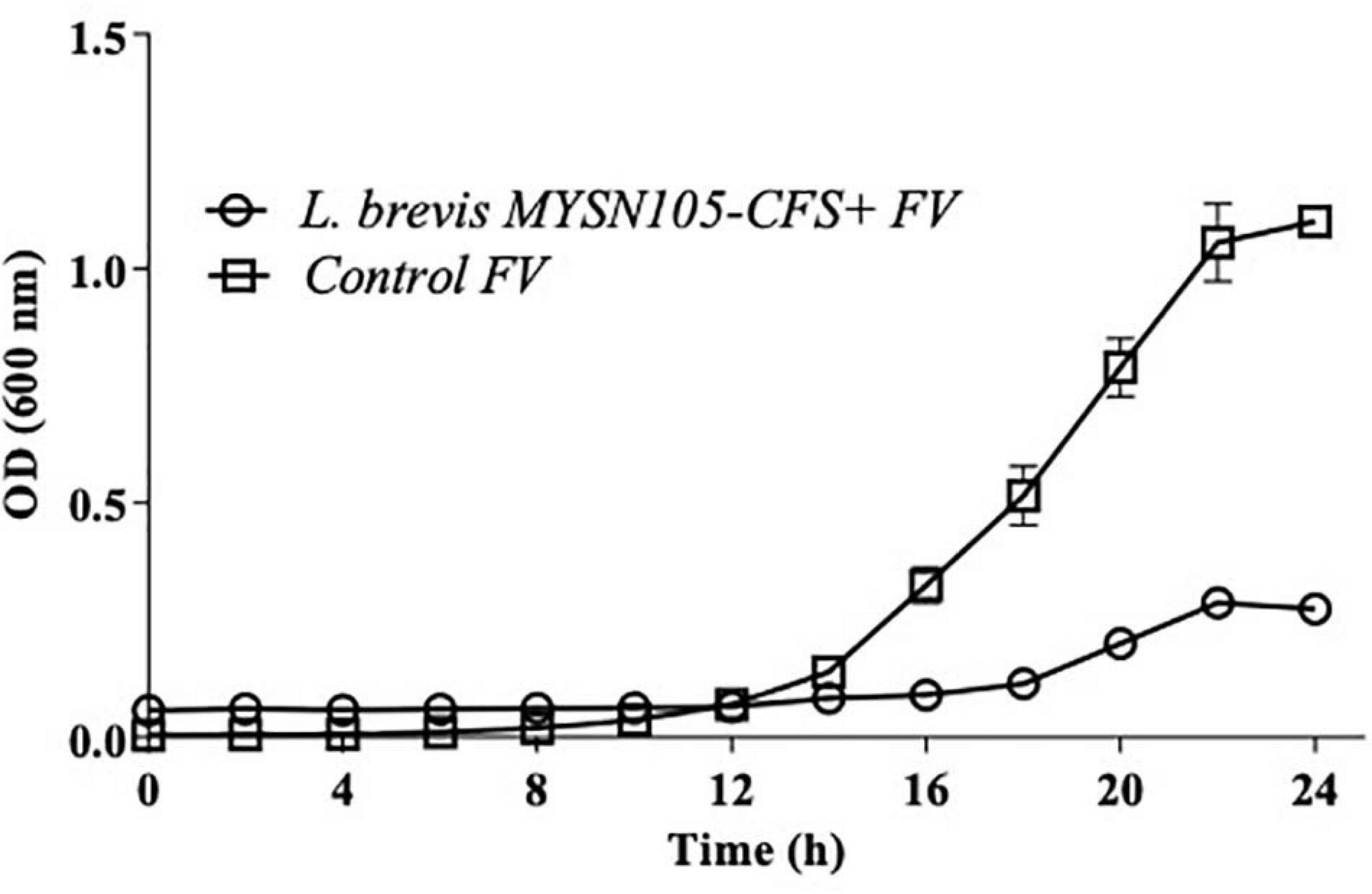
Figure 6. Antifungal activity of L. brevis MYSN105 against F. verticillioides in a microtiter plate for 24 h. Data shown are mean ± SE of triplicate values of three independent experiments.
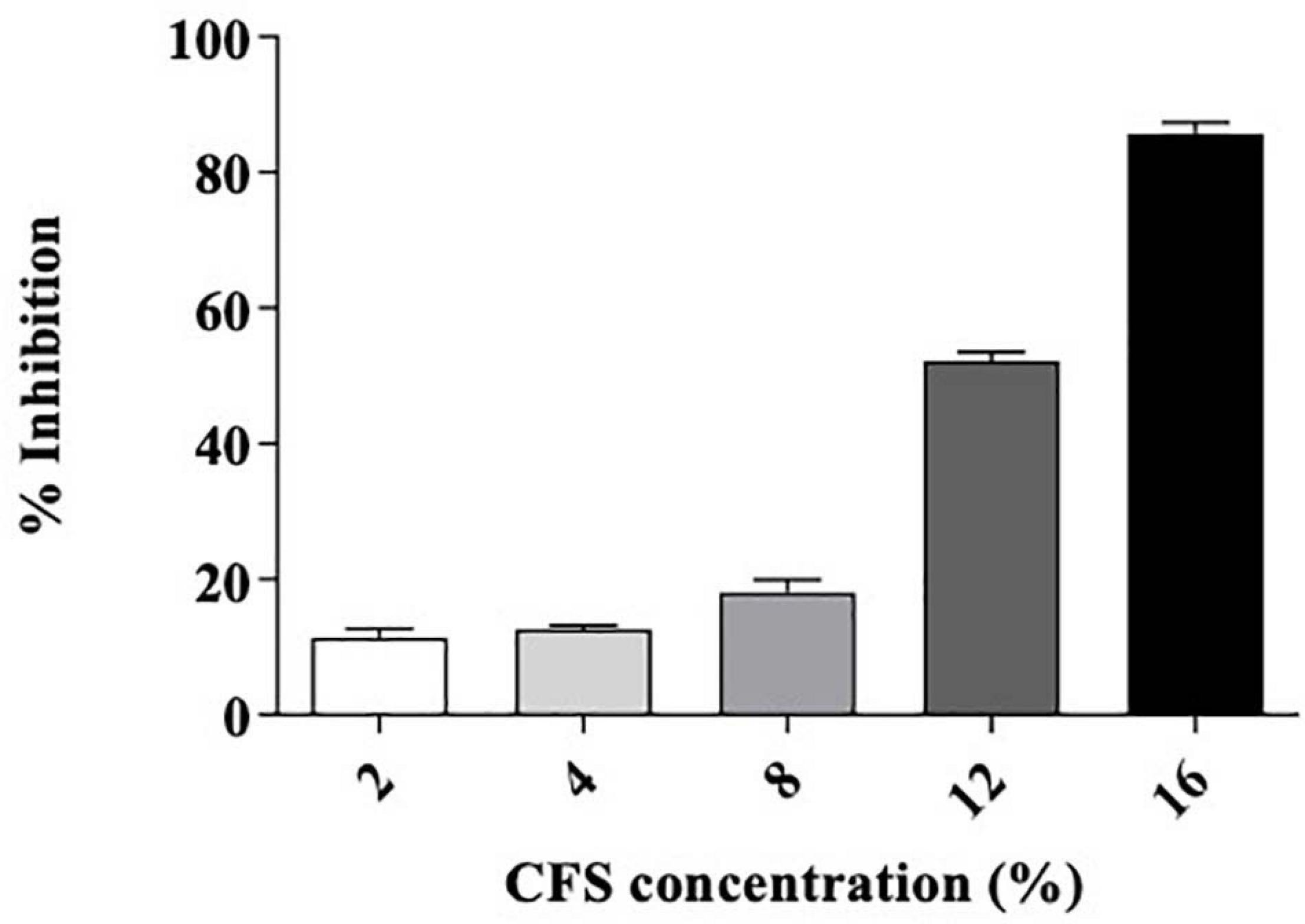
Figure 7. Minimum fungicidal concentration of L. brevis MYSN105-CFS against F. verticillioides by microdilution method. Data shown are mean ± SE of triplicate values of three independent experiments.
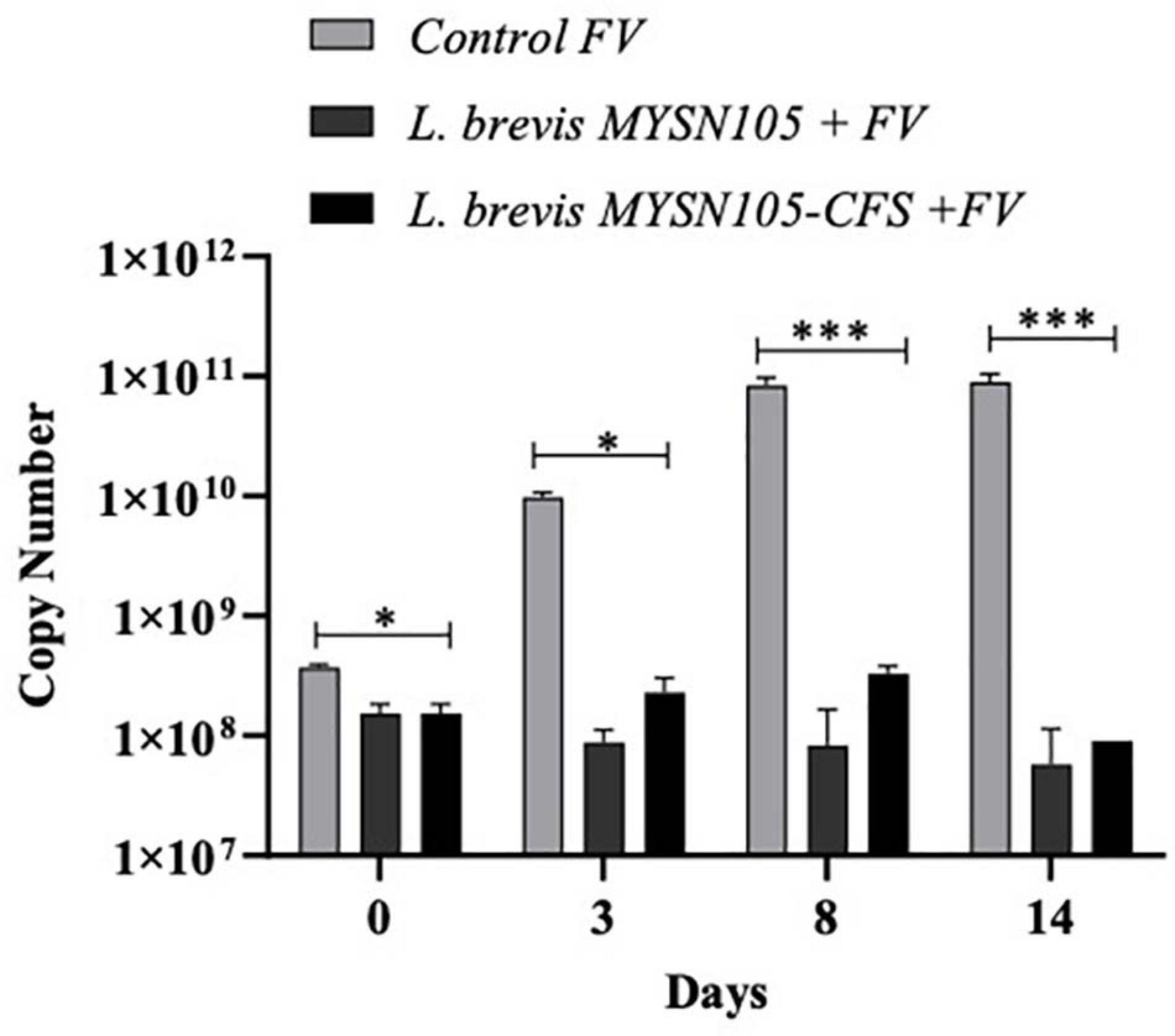
Figure 8. Quantitative PCR amplification of F. verticillioides using ITS primer. ITS copy number quantified using Cq values. Data shown are mean ± SE of triplicate values of two independent experiments and differ significantly (∗p < 0.05, ∗∗∗p < 0.001).
Conidia Germination Inhibition Assay
The L. brevis MYSN105 and its CFS caused irreversible damage to conidia, resulting in cell mortality and hyphal growth inhibition (Figure 5B). After 24 h incubation, conidia showed deformation and were reduced to 23.41% ± 1.12 and 20.12% ± 1.15 in the presence of the strain or its CFS, respectively.
Sensitivity of the CFS From L. brevis MYSN105 to Proteinase K, pH Neutralization, and Heat Treatment
The effect of proteinase K, pH neutralization, and heat treatment on the antifungal activity of L. brevis MYSN105-CFS is presented in Figure 9. The antimicrobial activity was moderately sensitive to proteinase K. The antifungal activity of CFS decreased at neutral pH 7, while a partial inactivation of the CFS was observed in heat treatment at 80°C for 20 min.
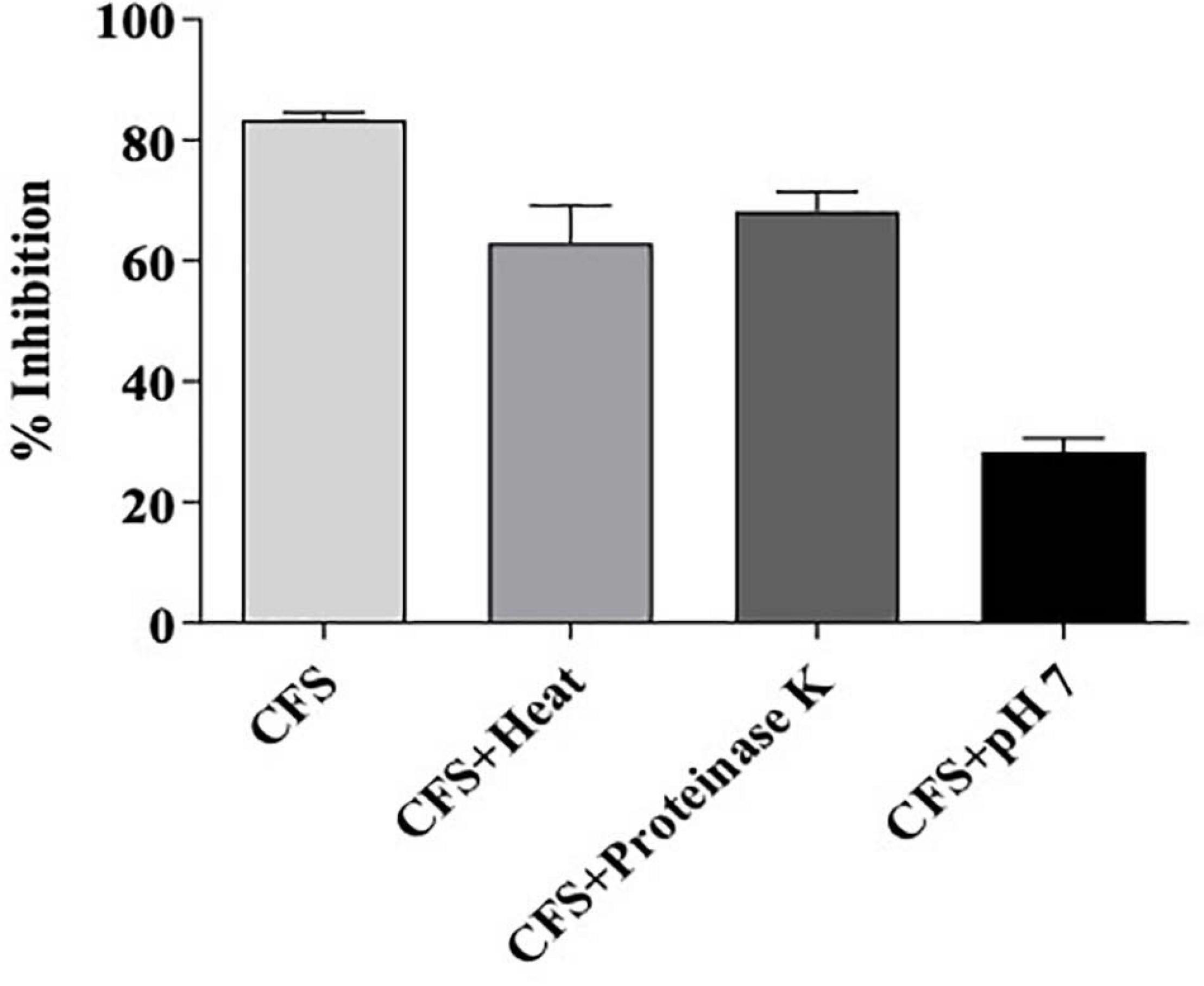
Figure 9. Residual bioactivity of L. brevis MYSN105-CFS against F. verticillioides after treatment with heat, proteinase K, or pH neutralization. Data shown are mean ± SE of triplicate values of three independent experiments.
Biocontrol of F. verticillioides in Maize Seeds
The treatment of maize seeds using the CFS of L. brevis MYSN105 highly inhibited the F. verticillioides growth, with no mycelium formation after 7 days of ambient incubation conditions compared to control (Figure 10). The spore count in the control maize samples treated with fresh MRS broth reached 4.1 × 102 spores/ml.
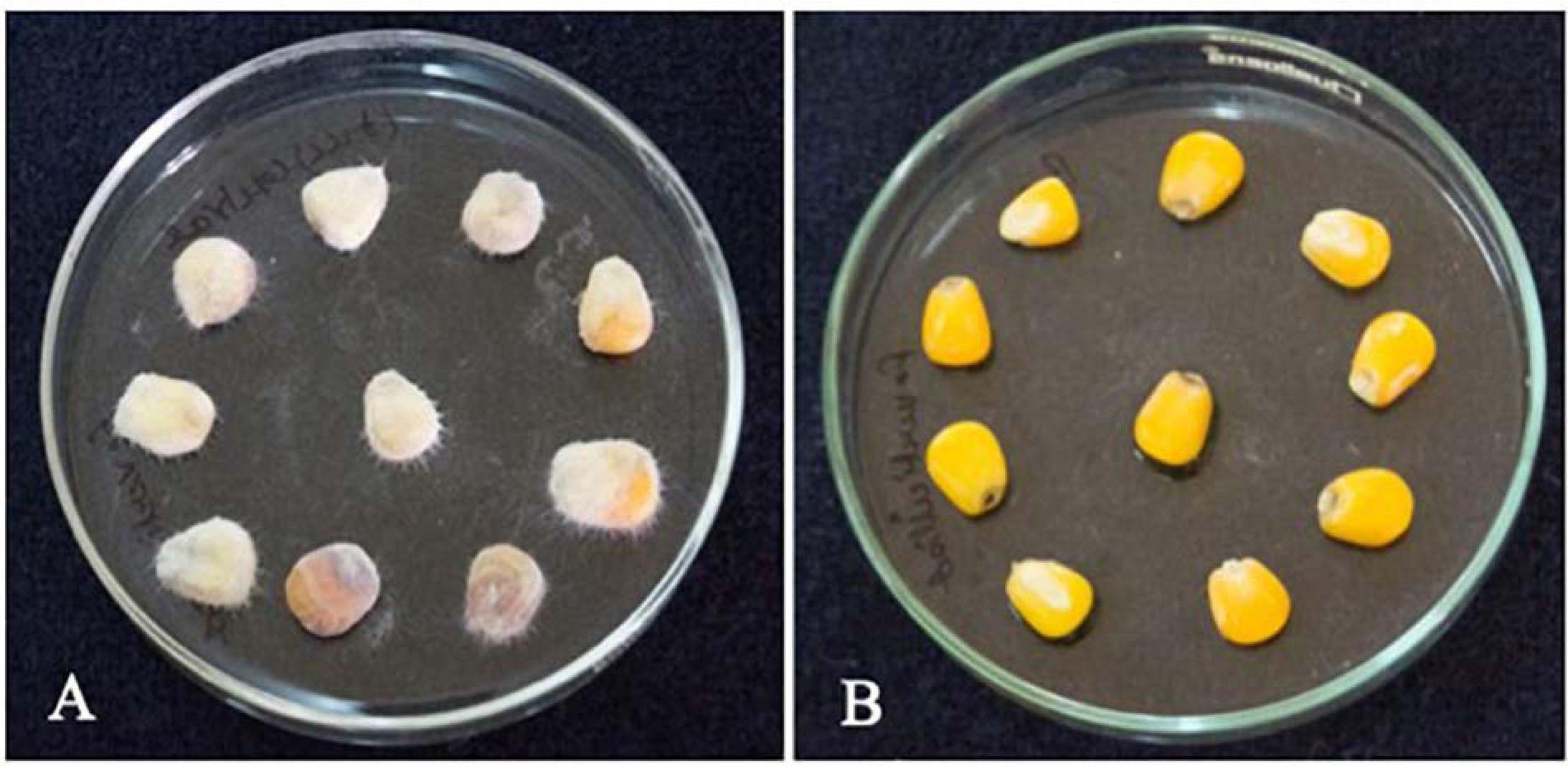
Figure 10. Effect of L. brevis MYSN105-CFS treatment on maize kernels inoculated with F. verticillioides after 7 days. (A) Control, maize kernel infected with F. verticillioides; (B) maize kernel treated with L. brevis MYSN105 CFS and infected with F. verticillioides.
Discussion
The current study investigated an unexplored niche Pozha for the presence of potent LAB having antifungal properties and desirable probiotic features. The Pozha samples were prepared using coconut milk, rice, and coconut water, fermented naturally, and later collected aseptically to isolate bioactive LAB. Among 20 isolates from different Pozha samples, a few were found to have antifungal activity against F. verticillioides. Of particular interest, L. brevis MYSN105 was highly potent against F. verticillioides while exhibiting attractive probiotic attributes. Taroub et al. (2019) and Judkins et al. (2020) showed that LABs are found in diverse ecological niches, including fermented food products, where they play an important role in nutrition and maintaining gut health. They are also promising biological control agents reported in several studies for their antifungal activity while being generally regarded as safe (GRAS). Our results are concomitant to other studies (Laitila et al., 2002; Oliveira et al., 2015; Deepthi et al., 2016), which demonstrated the efficiency of different LAB strains against some Fusarium species such as F. avenaceum, F. proliferatum, F. graminearum, and F. culmorum.
The candidate L. brevis MYSN105 exhibited interesting probiotic characteristics, including an excellent survival in acid and bile conditions in accordance with previous studies by Zommiti et al. (2017) and Soares et al. (2019). Besides, L. brevis MYSN105 was able to utilize a wide range of carbon sources, namely, glucose, xylose, arabinose, mannose, galactose, cellobiose, xylitol, arabitol, and lactose, with acid production, desirable features in food industries as probiotics (Ramesh et al., 2015; Lee et al., 2017; Kavitha et al., 2018). Probiotic features also include the ability to colonize and adhere to epithelial cells and mucosal surfaces (Lee and Salminen, 1995; Jacobsen et al., 1999); this helps them resist fluctuation of their intestinal level and inhibit the attachment of pathogenic bacteria via competitive adhesion all over the intestine nullifying inflammatory reactions (Guo et al., 2010; Sim et al., 2015). Therefore, autoaggregation and hydrophobicity determine the adhesion ability of probiotic bacteria (Collado et al., 2008; Meidong et al., 2017). In this study, L. brevis MYSN105 showed better hydrophobicity and strong autoaggregation, suggesting higher adhesion to epithelial cells. The adhesion results on Caco-2 and HT-29 cells demonstrated the potent adhesion capacity of L. brevis MYSN105 to the gastrointestinal tract. Previous reports have shown that adhesion of Bifidobacterium and Lactobacillus strains to intestinal mucus plays an important role in Enteropathogen exclusion (Collabo et al., 2005; Jung et al., 2019). Therefore, the antimicrobial activity exhibited by L. brevis MYSN105 provides a competitive inhibition of pathogenic microbes in the colonic environment. In addition, our probiotic candidate was non-hemolytic and sensitive to ampicillin, gentamicin, erythromycin, clindamycin, tetracycline, and chloramphenicol as per EFSA guidelines (EFSA, 2012). Hemolytic activity and antibiotic susceptibility are safety prerequisites for selecting probiotic strains (Argyri et al., 2013). Hemolysin is considered a virulence factor that can initiate infection and antibiotic resistance in bacterial strains having serious consequences on the host (Ramesh et al., 2015; Nandi et al., 2017). The genomic analysis is an important tool to help understand the functional properties of probiotic strains, including their safety, thus validating their efficacy (Qureshi et al., 2020). L. brevis MYSN105 was comprehensively studied for potential probiotic traits using whole-genome sequence analysis, which enabled identifying genes linked to safety and antimicrobial properties. Several studies have reported Lactobacillus species consisting of genes related to their stress resistance, antimicrobial properties, and other functional mechanisms (Chen et al., 2019; Toropov et al., 2020). The genomic finding suggests that understanding the functional and genetic characteristics could provide new insights into applications in gut health and animal feed additives (Heo et al., 2018). These attributes make L. brevis MYSN105 an attractive probiotic candidate (Jung et al., 2019) for industrial applications.
Food industries apply physical and chemical treatments to control mycotoxigenic fungi in food and feed (Faucet-Marquis et al., 2014). However, these treatments could reduce the nutritional value, safety, and sensory quality of food. Moreover, these methods require expensive equipment for the industry (Pierides et al., 2000; Kim et al., 2017). Many researchers have studied the management of mycotoxigenic fungi in food and feed using biological treatments such as probiotics microorganisms by assimilation or degradation approaches (Kim et al., 2017). Indeed, the biological approaches are increasingly explored due to their higher effectiveness and safety for humans (Jaibangyang et al., 2020; Rodríguez and Núñez, 2020). Our probiotic candidate L. brevis MYSN105 and its CFS both reduced significantly the growth of mycotoxigenic fungi, with findings from this study are in good accordance with previous investigations (Nagaraja et al., 2016; Deepthi et al., 2017; Plocková et al., 2018).
The neutralization of CFS from L. brevis MYSN105 reduced the antifungal activity of F. verticillioides, indicating a potential role for organic acids in the exhibited inhibition (Mauro and Garcia, 2019). Previously, the synergistic effects of different mixtures of identified compounds in L. plantarum, L. sanfrancisco, and Weissella cibaria were studied by Corsetti et al. (1998), Niku-Paavola et al. (1999), and Ndagano et al. (2011). The studies reveal that the combined effects of the compounds is much higher than the individual activities of the compounds (Lynch et al., 2014). The significant activity of L. brevis MYSN105 against F. verticillioides could be attributed to the combined effects of the organic acids. The CFS from L. brevis MYSN105 reduced the fungal growth and caused morphological alterations by inhibiting conidia, indicating that the antifungal activity is related to the bioactive compounds present in the CFS. Deepthi et al. (2016) also reported conidial and biomass inhibition of F. proliferatum after treatment with CFS from Lactobacillus plantarum MYS6. Likewise, Lactobacillus plantarum MYS44 suppressed the conidial germination and mycelial growth of Aspergillus parasiticus (Poornachandra Rao et al., 2019).
In the present study, L. brevis MYSN105 inhibited both mycelial biomass and conidial germination. This antifungal activity of LAB is a complex phenomenon mainly due to the high capacity of these bacteria to produce multiple antagonistic compounds that often act in synergy (Laitila et al., 2004). The CFS recovered from L. brevis MYSN105 had a potent inhibitory effect on F. verticillioides in maize seeds and in vitro cocultures, per in vitro reports on Lactobacillus species having antifungal activities (Muñoz et al., 2010; Voulgari et al., 2010; Gerbaldo et al., 2012). Finally, the strain L. brevis MYSN105 isolated from traditional fermented food Pozha showed antagonistic activity against F. verticillioides, suggesting a promising biocontrol potential.
Conclusion
The present study identifies L. brevis MYSN105 isolated from fermented food Pozha as an effective biocontrol of the mycotoxigenic F. verticillioides that are natural contaminants of raw materials used in the food and feed production. Future studies with L. brevis MYSN105 are required to test their ability to inhibit fumonisin biosynthesis and test their application in biocontrolling other fungal contaminants and extending the shelf life of food and feed.
Data Availability Statement
The datasets presented in this study can be found in online repositories. The names of the repository/repositories and accession number(s) can be found in the article/supplementary material.
Author Contributions
MYS, RH, and RS designed the research work. RS, AG, and UJ performed the research activities. RS and WM analyzed the data and wrote the manuscript. WM, RH, and MYS edited the manuscript submitted. All authors have given their approval for publication and have no conflict of interest.
Conflict of Interest
The authors declare that the research was conducted in the absence of any commercial or financial relationships that could be construed as a potential conflict of interest.
Acknowledgments
This work was supported by the Science and Engineering Research Board, New Delhi, India (REF. EEQ/2016/000273). MYS and RS thank Shastri Indo-Canadian Institute (SICI; New Delhi, India) for supporting to carry out the research in part at Ottawa, Canada. RS is grateful to the Indian Council for Medical Research (ICMR; New Delhi) for a doctoral fellowship (No. 3/1/2/105/2018-Nut).
References
Algharibeh, G. R., and AlFararjeh, M. S. (2019). Pesticide residues in fruits and vegetables in Jordan using liquid chromatography/tandem mass spectrometry. Food Addit. Contam. Part B Surveill. 12, 65–73. doi: 10.1080/19393210.2018.1548505
Altschul, S. F., Gish, W., Miller, W., Myers, E. W., and Lipman, D. J. (1990). Basic Local Alignment Search Tool. J. Mol. Biol. 215, 403–410.
Argyri, A. A., Zoumpopoulou, G., Karatzas, K. A. G., Tsakalidou, E., Nychas, G. J. E., Panagou, E. Z., et al. (2013). Selection of potential probiotic lactic acid bacteria from fermented olives by in vitro tests. Food Microbiol. 33, 282–291. doi: 10.1016/j.fm.2012.10.005
Arndt, D., Grant, J. R., Marcu, A., Sajed, T., Pon, A., Liang, Y., et al. (2016). PHASTER: a better, faster version of the PHAST phage search tool. Nucleic Acids Res. 44, W16–W21. doi: 10.1093/nar/gkw387
Arumugam, T., Pillay, Y., Ghazi, T., Nagiah, S., Abdul, N. S., and Chuturgoon, A. A. (2019). Fumonisin B 1 -induced oxidative stress triggers Nrf2-mediated antioxidant response in human hepatocellular carcinoma (HepG2) cells. Mycotoxin Res. 35, 99–109. doi: 10.1007/s12550-018-0335-0
Aziz, R. K., Bartels, D., Best, A., DeJongh, M., Disz, T., Edwards, R. A., et al. (2008). The RAST Server: Rapid annotations using subsystems technology. BMC Genomics 9:1–15. doi: 10.1186/1471-2164-9-75
Behera, S. S., El Sheikha, A. F., Hammami, R., and Kumar, A. (2020). Traditionally fermented pickles: How the microbial diversity associated with their nutritional and health benefits? J. Funct. Foods 70:103971. doi: 10.1016/j.jff.2020.103971
Belfiore, C., Raya, R. R., and Vignolo, G. M. (2013). Identification, technological and safety characterization of Lactobacillus sakei and Lactobacillus curvatus isolated from Argentinean anchovies (Engraulis anchoita). Springerplus 2, 1–8. doi: 10.1186/2193-1801-2-257
Blin, K., Shaw, S., Steinke, K., Villebro, R., Ziemert, N., Lee, S. Y., et al. (2019). AntiSMASH 5.0: Updates to the secondary metabolite genome mining pipeline. Nucleic Acids Res. 47, W81–W87. doi: 10.1093/nar/gkz310
Boubezari, M. T., Idoui, T., Hammami, R., Fernandez, B., Gomaa, A., and Fliss, I. (2018). Bacteriocinogenic properties of Escherichia coli P2C isolated from pig gastrointestinal tract: purification and characterization of microcin V. Arch. Microbiol. 200, 771–782. doi: 10.1007/s00203-018-1482-6
Brettin, T., Davis, J. J., Disz, T., Edwards, R. A., Gerdes, S., Olsen, G. J., et al. (2015). RASTtk: A modular and extensible implementation of the RAST algorithm for building custom annotation pipelines and annotating batches of genomes. Sci. Rep. 5:sre08365. doi: 10.1038/srep08365
Chen, L., Gu, Q., Li, P., Chen, S., and Li, Y. (2019). Genomic analysis of Lactobacillus reuteri WHH1689 reveals its probiotic properties and stress resistance. Food Sci. Nutr. 7, 844–857. doi: 10.1002/fsn3.934
Chlebicz, A., and Śliżewska, K. (2020). In Vitro Detoxification of Aflatoxin B1, Deoxynivalenol, Fumonisins, T-2 Toxin and Zearalenone by Probiotic Bacteria from Genus Lactobacillus and Saccharomyces cerevisiae Yeast. Probiot. Antimicrob. Proteins 12, 289–301. doi: 10.1007/s12602-018-9512-x
Collabo, M. C., Gueimonde, M., Hernández, M., Sanz, Y., and Salminen, S. (2005). Adhesion of selected Bifidobacterium strains to human intestinal mucus and the role of adhesion in enteropathogen exclusion. J. Food Prot. 68, 2672–2678. doi: 10.4315/0362-028x-68.12.2672
Collado, M. C., Meriluoto, J., and Salminen, S. (2008). Adhesion and aggregation properties of probiotic and pathogen strains. Eur. Food Res. Technol. 226, 1065–1073. doi: 10.1007/s00217-007-0632-x
Coppock, R. W., and Dziwenka, M. (2015). Potential Agents That Can Cause Contamination of Animal Feedstuffs and Terror. Amsterdam: Elsevier Inc, doi: 10.1016/B978-0-12-800159-2.00053-1
Corsetti, A., Gobbetti, M., Rossi, J., and Damiani, P. (1998). Antimould activity of sourdough lactic acid bacteria: Identification of a mixture of organic acids produced by Lactobacillus sanfrancisco CB1. Appl. Microbiol. Biotechnol. 50, 253–256. doi: 10.1007/s002530051285
Deepa, N., and Sreenivasa, M. (2017). Fusarium verticillioides, a Globally Important Pathogen of Agriculture and Livestock: A Review. J. Vet. Med. Res. 4:1084.
Deepa, N., Nagaraja, H., and Sreenivasa, M. Y. (2016). Prevalence of fumonisin producing Fusarium verticillioides associated with cereals grown in Karnataka (India). Food Sci. Hum. Wellness 5, 156–162. doi: 10.1016/j.fshw.2016.07.001
Deepthi, B. V., Rao, K. P., Chennapa, G., Naik, M. K., Chandrashekara, K. T., and Sreenivasa, M. Y. (2016). Antifungal attributes of Lactobacillus plantarum MYS6 against fumonisin producing Fusarium proliferatum associated with poultry feeds. PLoS One 11:1–22. doi: 10.1371/journal.pone.0155122
Deepthi, B. V., Somashekaraiah, R., Poornachandra Rao, K., Deepa, N., Dharanesha, N. K., Girish, K. S., et al. (2017). Lactobacillus plantarum MYS6 ameliorates fumonisin B1-induced hepatorenal damage in broilers. Front. Microbiol. 8:1–14. doi: 10.3389/fmicb.2017.02317
Edwards, U., Rogall, T., Blöcker, H., Emde, M., and Böttger, E. C. (1989). Isolation and direct complete nucleotide determination of entire genes. Characterization of a gene coding for 16S ribosomal RNA. Nucleic Acids Res. 17, 7843–7853. doi: 10.1093/nar/17.19.7843
EFSA (2012). European Food Safety Authority, EFSA Panel on Additives and Products or Substances used in Animal Feed (FEEDAP). Scientific opinion Guidance on the assessment of bacterial susceptibility to antimicrobials of human and veterinary importance. EFSA J. 10:2740. doi: 10.2903/j.efsa.20YY.NNNN.Available
Elbanna, K., El Hadad, S., Assaeedi, A., Aldahlawi, A., Khider, M., and Alhebshi, A. (2018). In vitro and in vivo evidences for innate immune stimulators lactic acid bacterial starters isolated from fermented camel dairy products. Sci. Rep. 8, 1–15. doi: 10.1038/s41598-018-31006-3
Faucet-Marquis, V., Joannis-Cassan, C., Hadjeba-Medjdoub, K., Ballet, N., and Pfohl-Leszkowicz, A. (2014). Development of an in vitro method for the prediction of mycotoxin binding on yeast-based products: Case of aflatoxin B1, zearalenone and ochratoxin A. Appl. Microbiol. Biotechnol. 98, 7583–7596. doi: 10.1007/s00253-014-5917-y
Fraeyman, S., Croubels, S., Devreese, M., and Antonissen, G. (2017). Emerging fusarium and alternaria mycotoxins: Occurrence, toxicity and toxicokinetics. Toxins 9, 1–26. doi: 10.3390/toxins9070228
Gardes, M., and Bruns, T. D. (1993). ITS primers with enhanced specificity for basidiomycetes - application to the identification of mycorrhizae and rusts. Mol. Ecol. 2, 113–118. doi: 10.1111/j.1365-294X.1993.tb00005.x
Gerbaldo, G. A., Barberis, C., Pascual, L., Dalcero, A., and Barberis, L. (2012). Antifungal activity of two Lactobacillus strains with potential probiotic properties. FEMS Microbiol. Lett. 332, 27–33. doi: 10.1111/j.1574-6968.2012.02570.x
Gibson, G. R., Probert, H. M., Loo, J., Van, Rastall, R. A., and Roberfroid, M. B. (2004). Dietary modulation of the human colonic microbiota: updating the concept of prebiotics. Nutr. Res. Rev. 17, 259–275. doi: 10.1079/nrr200479
Guo, X. H., Kim, J. M., Nam, H. M., Park, S. Y., and Kim, J. M. (2010). Screening lactic acid bacteria from swine origins for multistrain probiotics based on in vitro functional properties. Anaerobe 16, 321–326. doi: 10.1016/j.anaerobe.2010.03.006
Hammami, R., Zouhir, A., Hamida, J., Ben, Neffati, M., Vergoten, G., et al. (2009). Antimicrobial properties of aqueous extracts from three medicinal plants growing wild in arid regions of Tunisia. Pharm. Biol. 47, 452–457. doi: 10.1080/13880200902822604
Heo, J., Shin, D., Chang, S. Y., Bogere, P., Park, M. R., Ryu, S., et al. (2018). Comparative Genome Analysis and Evaluation of Probiotic Characteristics of Lactobacillus plantarum Strain JDFM LP11. Korean J. Food Sci. Anim. Resour. 38, 878–888. doi: 10.5851/kosfa.2018.e21
Jacobsen, C. N., Nielsen, V. R., Hayford, A. E., Møller, P. L., Michaelsen, K. F., Pærregaard, A., et al. (1999). Screening of probiotic activities of forty-seven strains of Lactobacillus spp. by in vitro techniques and evaluation of the colonization ability of five selected strains in humans. Appl. Environ. Microbiol. 65, 4949–4956. doi: 10.1128/aem.65.11.4949-4956.1999
Jaibangyang, S., Nasanit, R., and Limtong, S. (2020). Biological control of aflatoxin-producing Aspergillus flavus by volatile organic compound-producing antagonistic yeasts. BioControl 65, 377–386. doi: 10.1007/s10526-020-09996-9
Judkins, T. C., Archer, D. L., Kramer, D. C., and Solch, R. J. (2020). Probiotics, Nutrition, and the Small Intestine. Curr. Gastroenterol. Rep. 22:2.
Jung, J. H., Kim, S. J., Lee, J. Y., Yoon, S. R., You, S. Y., and Kim, S. H. (2019). Multifunctional properties of Lactobacillus plantarum strains WiKim83 and WiKim87 as a starter culture for fermented food. Food Sci. Nutr. 7, 2505–2516. doi: 10.1002/fsn3.1075
Kavitha, M., Raja, M., and Perumal, P. (2018). Evaluation of probiotic potential of Bacillus spp. isolated from the digestive tract of freshwater fish Labeo calbasu (Hamilton, 1822). Aquac. Rep. 11, 59–69. doi: 10.1016/j.aqrep.2018.07.001
Khan, R. B., Phulukdaree, A., and Chuturgoon, A. A. (2018). Fumonisin B1 induces oxidative stress in oesophageal (SNO) cancer cells. Toxicon 141, 104–111. doi: 10.1016/j.toxicon.2017.12.041
Kharazian, Z. A., Salehi Jouzani, G., Aghdasi, M., Khorvash, M., Zamani, M., and Mohammadzadeh, H. (2017). Biocontrol potential of Lactobacillus strains isolated from corn silages against some plant pathogenic fungi. Biol. Control 110, 33–43. doi: 10.1016/j.biocontrol.2017.04.004
Kim, S., Lee, H., Lee, S., Lee, J., Ha, J., Choi, Y., et al. (2017). Invited review: Microbe-mediated aflatoxin decontamination of dairy products and feeds. J. Dairy Sci. 100, 871–880. doi: 10.3168/jds.2016-11264
Kumar, S., Stecher, G., Li, M., Knyaz, C., and Tamura, K. (2018). MEGA X: Molecular evolutionary genetics analysis across computing platforms. Mol. Biol. Evol. 35, 1547–1549. doi: 10.1093/molbev/msy096
Kwak, M. K., Liu, R., and Kang, S. O. (2018). Antimicrobial activity of cyclic dipeptides produced by Lactobacillus plantarum LBP-K10 against multidrug-resistant bacteria, pathogenic fungi, and influenza A virus. Food Control 85, 223–234. doi: 10.1016/j.foodcont.2017.10.001
Laitila, A., Alakomi, H. L., Raaska, L., Mattila-Sandholm, T., and Haikara, A. (2002). Antifungal activities of two Lactobacillus plantarum strains against Fusarium moulds in vitro and in malting of barley. J. Appl. Microbiol. 93, 566–576. doi: 10.1046/j.1365-2672.2002.01731.x
Laitila, A., Saarela, M., Kirk, L., Siika-Aho, M., Haikara, A., Mattila-Sandholm, T., et al. (2004). Malt sprout extract medium for cultivation of Lactobacillus plantarum protective cultures. Lett. Appl. Microbiol. 39, 336–340. doi: 10.1111/j.1472-765X.2004.01579.x
Lane, D. J., Pace, B., Olsen, G. J., Stahlt, D. A., Sogint, M. L., and Pace, N. R. (1985). Rapid determination of 16S ribosomal RNA sequences for phylogenetic analyses (reverse transcriptase/dideoxynudeotide). Evolution 82, 6955–6959. doi: 10.1073/pnas.82.20.6955
Lee, H. J., and Ryu, D. (2017). Worldwide Occurrence of Mycotoxins in Cereals and Cereal-Derived Food Products: Public Health Perspectives of Their Co-occurrence. J. Agric. Food Chem. 65, 7034–7051. doi: 10.1021/acs.jafc.6b04847
Lee, S., Lee, J., Jin, Y. I., Jeong, J. C., Chang, Y. H., Lee, Y., et al. (2017). Probiotic characteristics of Bacillus strains isolated from Korean traditional soy sauce. LWT Food Sci. Technol. 79, 518–524. doi: 10.1016/j.lwt.2016.08.040
Lee, Y. K., and Salminen, S. (1995). The coming of age of probiotics. Trends Food Sci. Technol. 6, 241–245. doi: 10.1016/S0924-2244(00)89085-8
Luz, C., Saladino, F., Luciano, F. B., Mañes, J., and Meca, G. (2017). In vitro antifungal activity of bioactive peptides produced by Lactobacillus plantarum against Aspergillus parasiticus and Penicillium expansum. LWT Food Sci. Technol. 81, 128–135. doi: 10.1016/j.lwt.2017.03.053
Lynch, K. M., Pawlowska, A. M., Brosnan, B., Coffey, A., Zannini, E., Furey, A., et al. (2014). Application of Lactobacillus amylovorus as an antifungal adjunct toextend the shelf-life of Cheddar cheese. Int. Dairy J. 34, 167–173. doi: 10.1016/j.idairyj.2013.07.017
Magnusson, J., and Schnürer, J. (2001). Lactobacillus coryniformis subsp. coryniformis strain Si3 produces a broad-spectrum proteinaceous antifungal compound. Appl. Environ. Microbiol. 67, 1–5. doi: 10.1128/AEM.67.1.1-5.2001
Martinez Tuppia, C., Atanasova-Penichon, V., Chéreau, S., Ferrer, N., Marchegay, G., Savoie, J. M., et al. (2017). Yeast and bacteria from ensiled high moisture maize grains as potential mitigation agents of fumonisin B1. J. Sci. Food Agric. 97, 2443–2452. doi: 10.1002/jsfa.8058
Mauro, C. S. I., and Garcia, S. (2019). Coconut milk beverage fermented by Lactobacillus reuteri: optimization process and stability during refrigerated storage. J. Food Sci. Technol. 56, 854–864. doi: 10.1007/s13197-018-3545-8
Meidong, R., Doolgindachbaporn, S., Jamjan, W., Sakai, K., Tashiro, Y., Okugawa, Y., et al. (2017). A novel probiotic Bacillus siamensis B44v isolated from Thai pickled vegetables (Phak-dong) for potential use as a feed supplement in aquaculture. J. Gen. Appl. Microbiol. 63, 246–253. doi: 10.2323/jgam.2016.12.002
Moroni, O., Kheadr, E., Boutin, Y., Lacroix, C., and Fliss, I. (2006). Inactivation of adhesion and invasion of food-borne Listeria monocytogenes by bacteriocin-producing Bifidobacterium strains of human origin. Appl. Environ. Microbiol. 72, 6894–6901. doi: 10.1128/AEM.00928-06
Muhammad, Z., Ramzan, R., Abdelazez, A., Amjad, A., Afzaal, M., Zhang, S., et al. (2019). Assessment of the antimicrobial potentiality and functionality of Lactobacillus plantarum strains isolated from the conventional inner mongolian fermented cheese against foodborne pathogens. Pathogens 8, 1–20. doi: 10.3390/pathogens8020071
Muñoz, R., Arena, M. E., Silva, J., and González, S. N. (2010). Inhibition of mycotoxin-producing Aspergillus nomius VSC 23 by lactic acid bacteria and Saccharomyces cerevisiae. Braz. J. Microbiol. 41, 1019–1026. doi: 10.1590/S1517-83822010000400021
Nagaraja, H., Chennappa, G., Rakesh, S., Naik, M. K., Amaresh, Y. S., and Sreenivasa, M. Y. (2016). Antifungal activity of Azotobacter nigricans against trichothecene-producing Fusarium species associated with cereals. Food Sci. Biotechnol. 25, 1197–1204. doi: 10.1007/s10068-016-0190-8
Nandi, A., Dan, S. K., Banerjee, G., Ghosh, P., Ghosh, K., Ringø, E., et al. (2017). Probiotic Potential of Autochthonous Bacteria Isolated from the Gastrointestinal Tract of Four Freshwater Teleosts. Probiot. Antimicrob. Proteins 9, 12–21. doi: 10.1007/s12602-016-9228-8
Ndagano, D., Lamoureux, T., Dortu, C., Vandermoten, S., and Thonart, P. (2011). Antifungal Activity of 2 Lactic Acid Bacteria of the Weissella Genus Isolated from Food. J. Food Sci. 76, M305–M311. doi: 10.1111/j.1750-3841.2011.02257.x
Nematollahi, A., Kamankesh, M., Hosseini, H., Ghasemi, J., Hosseini-Esfahani, F., and Mohammadi, A. (2019). Investigation and determination of acrylamide in the main group of cereal products using advanced microextraction method coupled with gas chromatography-mass spectrometry. J. Cereal Sci. 87, 157–164. doi: 10.1016/j.jcs.2019.03.019
Niku-Paavola, M. L., Laitila, A., Mattila-Sandholm, T., and Haikara, A. (1999). New types of antimicrobial compounds produced by Lactobacillus plantarum. J. Appl. Microbiol. 86, 29–35. doi: 10.1046/j.1365-2672.1999.00632.x
Noda, M., Danshiitsoodol, N., Inoue, Y., Okamoto, T., Sultana, N., and Sugiyama, M. (2019). Antibiotic susceptibility of plant-derived lactic acid bacteria conferring health benefits to human. J. Antibiot. 72, 834–842. doi: 10.1038/s41429-019-0218-4
Oliveira, P. M., Brosnan, B., Furey, A., Coffey, A., Zannini, E., and Arendt, E. K. (2015). Lactic acid bacteria bioprotection applied to the malting process. Part I: Strain characterization and identification of antifungal compounds. Food Control 51, 433–443. doi: 10.1016/j.foodcont.2014.07.004
Overbeek, R., Olson, R., Pusch, G. D., Olsen, G. J., Davis, J. J., Disz, T., et al. (2014). The SEED and the Rapid Annotation of microbial genomes using Subsystems Technology (RAST). Nucleic Acids Res. 42, 206–214. doi: 10.1093/nar/gkt1226
Pierides, M., El-Nezami, H., Peltonen, K., Salminen, S., and Ahokas, J. (2000). Ability of dairy strains of lactic acid bacteria to bind aflatoxin M1 in a food model. J. Food Prot. 63, 645–650. doi: 10.4315/0362-028X-63.5.645
Plocková, M., Stiles, J., Chumchalová, J., and Halfarová, R. (2018). Control of mould growth by Lactobacillus rhamnosus VT1 and Lactobacillus reuteri CCM 3625 on milk agar plates. Czech J. Food Sci. 19, 46–50. doi: 10.17221/6574-cjfs
Poornachandra Rao, K., Deepthi, B. V., Rakesh, S., Ganesh, T., Achar, P., and Sreenivasa, M. Y. (2019). Antiaflatoxigenic Potential of Cell-Free Supernatant from Lactobacillus plantarum MYS44 Against Aspergillus parasiticus. Probiot. Antimicrob. Proteins 11, 55–64. doi: 10.1007/s12602-017-9338-y
Pósa, R., Stoev, S., Kovács, M., Donkó, T., Repa, I., and Magyar, T. (2014). A comparative pathological finding in pigs exposed to fumonisin B1 and/or Mycoplasma hyopneumoniae. Toxicol. Ind. Health 32, 998–1012. doi: 10.1177/0748233714543735
Qureshi, N., Gu, Q., and Li, P. (2020). Whole genome sequence analysis and in vitro probiotic characteristics of a Lactobacillus strain Lactobacillus paracasei ZFM54. J. Appl. Microbiol. 129, 422–433. doi: 10.1111/jam.14627
Ramesh, D., Vinothkanna, A., Rai, A. K., and Vignesh, V. S. (2015). Isolation of potential probiotic Bacillus spp. and assessment of their subcellular components to induce immune responses in Labeo rohita against Aeromonas hydrophila. Fish Shellfish Immunol. 45, 268–276. doi: 10.1016/j.fsi.2015.04.018
Ridwan, B. U., Koning, C. J. M., Besselink, M. G. H., Timmerman, H. M., Brouwer, E. C., Verhoef, J., et al. (2008). Antimicrobial activity of a multispecies probiotic (Ecologic 641) against pathogens isolated from infected pancreatic necrosis. Lett. Appl. Microbiol. 46, 61–67. doi: 10.1111/j.1472-765X.2007.02260.x
Rodríguez, M., and Núñez, F. (2020). Novel approaches to minimizing mycotoxin contamination. Toxins 12, 12–14. doi: 10.3390/toxins12040216
Seemann, T. (2014). Prokka: Rapid prokaryotic genome annotation. Bioinformatics 30, 2068–2069. doi: 10.1093/bioinformatics/btu153
Siedler, S., Balti, R., and Neves, A. R. (2019). Bioprotective mechanisms of lactic acid bacteria against fungal spoilage of food. Curr. Opin. Biotechnol. 56, 138–146. doi: 10.1016/j.copbio.2018.11.015
Sim, I., Koh, J. H., Kim, D. J., Gu, S. H., Park, A., and Lim, Y. H. (2015). In vitro assessment of the gastrointestinal tolerance and immunomodulatory function of Bacillus methylotrophicus isolated from a traditional Korean fermented soybean food. J. Appl. Microbiol. 118, 718–726. doi: 10.1111/jam.12719
Soares, M. B., Martinez, R. C. R., Pereira, E. P. R., Balthazar, C. F., Cruz, A. G., Ranadheera, C. S., et al. (2019). The resistance of Bacillus, Bifidobacterium, and Lactobacillus strains with claimed probiotic properties in different food matrices exposed to simulated gastrointestinal tract conditions. Food Res. Int. 125:108542. doi: 10.1016/j.foodres.2019.108542
Somashekaraiah, R., Shruthi, B., Deepthi, B. V., and Sreenivasa, M. Y. (2019). Probiotic properties of lactic acid bacteria isolated from neera: A naturally fermenting coconut palm nectar. Front. Microbiol. 10:1–11. doi: 10.3389/fmicb.2019.01382
Taroub, B., Salma, L., Manel, Z., Ouzari, H. I., Hamdi, Z., and Moktar, H. (2019). Isolation of lactic acid bacteria from grape fruit: antifungal activities, probiotic properties, and in vitro detoxification of ochratoxin A. Ann. Microbiol. 69, 17–27. doi: 10.1007/s13213-018-1359-6
Toropov, V., Demyanova, E., Shalaeva, O., Sitkin, S., and Vakhitov, T. (2020). Whole-genome sequencing of lactobacillus helveticus D75 and D76 confirms safety and probiotic potential. Microorganisms 8:8030329. doi: 10.3390/microorganisms8030329
Valan Arasu, M., Jung, M. W., Ilavenil, S., Jane, M., Kim, D. H., Lee, K. D., et al. (2013). Isolation and characterization of antifungal compound from Lactobacillus plantarum KCC-10 from forage silage with potential beneficial properties. J. Appl. Microbiol. 115, 1172–1185. doi: 10.1111/jam.12319
Vendruscolo, C. P., Frias, N. C., de Carvalho, C. B., de Sá, L. R. M., Belli, C. B., and Baccarin, R. Y. A. (2016). Leukoencephalomalacia Outbreak in Horses due to Consumption of Contaminated Hay. J. Vet. Intern. Med. 30, 1879–1881. doi: 10.1111/jvim.14588
Voulgari, K., Hatzikamari, M., Delepoglou, A., Georgakopoulos, P., Litopoulou-Tzanetaki, E., and Tzanetakis, N. (2010). Antifungal activity of non-starter lactic acid bacteria isolates from dairy products. Food Control 21, 136–142. doi: 10.1016/j.foodcont.2009.04.007
White, T. J., Bruns, T., Lee, S., and Taylor, J. (1990). Amplification and Direct Sequencing of Fungal Ribosomal Rna Genes for Phylogenetics. PCR Protoc. 1990, 315–322. doi: 10.1016/b978-0-12-372180-8.50042-1
Wood, D. E., and Salzberg, S. L. (2014). Kraken: Ultrafast metagenomic sequence classification using exact alignments. Genome Biol. 15:r46. doi: 10.1186/gb-2014-15-3-r46
Yadav, R., Puniya, A. K., and Shukla, P. (2016). Probiotic properties of Lactobacillus plantarum RYPR1 from an indigenous fermented beverage Raabadi. Front. Microbiol. 7:1–9. doi: 10.3389/fmicb.2016.01683
Yang, E. J., and Chang, H. C. (2010). Purification of a new antifungal compound produced by Lactobacillus plantarum AF1 isolated from kimchi. Int. J. Food Microbiol. 139, 56–63. doi: 10.1016/j.ijfoodmicro.2010.02.012
Keywords: probiotic, lactic acid bacteria, antifungal activity, fermented food, Fusarium
Citation: Somashekaraiah R, Mottawea W, Gunduraj A, Joshi U, Hammami R and Sreenivasa MY (2021) Probiotic and Antifungal Attributes of Levilactobacillus brevis MYSN105, Isolated From an Indian Traditional Fermented Food Pozha. Front. Microbiol. 12:696267. doi: 10.3389/fmicb.2021.696267
Received: 16 April 2021; Accepted: 03 June 2021;
Published: 05 July 2021.
Edited by:
Tongjie Liu, Ocean University of China, ChinaReviewed by:
Lukasz Stepien, Institute of Plant Genetics, Polish Academy of Sciences, PolandBijender Kumar Bajaj, University of Jammu, India
Copyright © 2021 Somashekaraiah, Mottawea, Gunduraj, Joshi, Hammami and Sreenivasa. This is an open-access article distributed under the terms of the Creative Commons Attribution License (CC BY). The use, distribution or reproduction in other forums is permitted, provided the original author(s) and the copyright owner(s) are credited and that the original publication in this journal is cited, in accordance with accepted academic practice. No use, distribution or reproduction is permitted which does not comply with these terms.
*Correspondence: M. Y. Sreenivasa, c3JlZW5pdmFzYW15QGdtYWlsLmNvbQ==; bXlzQG1pY3JvYmlvbG9neS51bmktbXlzb3JlLmFjLmlu