- 1College of Veterinary Medicine, South China Agricultural University, Guangzhou, China
- 2Guangdong Laboratory for Lingnan Modern Agriculture, Guangzhou, China
- 3Key Laboratory of Zoonosis Prevention and Control of Guangdong Province, Guangzhou, China
Foot-and-mouth disease virus (FMDV), Senecavirus A (SVA) and swine vesicular disease virus (SVDV) are members of the family Picornaviridae, which can cause similar symptoms - vesicular lesions in the tissues of the mouth, nose, feet, skin and mucous membrane of animals. Rapid and accurate diagnosis of these viruses allows for control measures to prevent the spread of these diseases. Reverse transcription-polymerase chain reaction (RT-PCR) and real-time RT-PCR are traditional and reliable methods for pathogen detection, while their amplification reaction requires a thermocycler. Isothermal amplification methods including loop-mediated isothermal amplification and recombinase polymerase amplification developed in recent years are simple, rapid and do not require specialized equipment, allowing for point of care diagnostics. Luminex technology allows for simultaneous detection of multiple pathogens. CRISPR-Cas diagnostic systems also emerging nucleic acid detection technologies which are very sensitivity and specificity. In this paper, various nucleic acid detection methods aimed at vesicular disease pathogens in swine (including FMDV, SVA and SVDV) are summarized.
Introduction
Picornaviruses are small non-enveloped viruses containing a single-stranded and positive-sense RNA protected by an icosahedral capsid (Holland et al., 1960; Kim et al., 2016). Picornaviruses seriously affect the respiratory system, digestive system and central nervous system, and cause a series of inflammation and lesions in organs and tissues such as heart, liver and skin (Zell, 2018). Picornaviruses are omnipresent and distributed worldwide. Studies have shown that the number of newly identified picornaviruses has increased dramatically over the past decade, and they become important pathogens that endanger human and animal health (Palmenberg et al., 2009; Lewis-Rogers and Crandall, 2010; Tapparel et al., 2013; Zell, 2018). Foot-and-mouth disease virus (FMDV), Senecavirus A (SVA) and swine vesicular disease virus (SVDV) are members of the family Picornaviridae, which can cause similar symptoms - vesicular lesions in the tissues of the mouth, nose, feet, skin and mucous membrane of animals. All three of them can threaten the development of animal industry and national economy.
FMDV, classified into the genus Aphthovirus in the family Picornaviridae, is still one of the important animal disease pathogens of economic concern (Rodriguez-Habibe et al., 2020; Brown et al., 2021). Based on genetic and antigenic analyzes, FMDVs throughout the world have been geographically divided into seven serotypes (O, A, Asia1, C, SAT1, SAT2, SAT3). FMDV has a broad host range to all cloven-hoofed animals including domestic and wild ruminants and pigs. (Grubman and Baxt, 2004; Rodriguez-Habibe et al., 2020). Animals infected with FMDV often present with symptoms of fever and blisters on the mouth and hooves, with low mortality but high morbidity, leading to production losses. FMDV can contaminate the environment through aerosols and cause long-distance transmission events, so that it complicates the control of Foot-and-mouth disease (FMD) outbreaks (Gloster et al., 2003; Brown et al., 2022).
SVA is the only member of the genus Senecavirus in the family Picornaviridae, which was discovered in 2002 and once named Seneca Valley virus 001 (SVV-001; Hales et al., 2008). Initially, it was used in different human cancer treatment research as its potent oncolytic activity (Reddy et al., 2007; Burke, 2016). Subsequently, pigs with idiopathic vesicular disease (IVD) like clinical symptom occurred in Canada (Pasma et al., 2008), America (Singh et al., 2012) and Brazil (Leme et al., 2015; Vannucci et al., 2015) were confirmed to be infected with SVA. In 2015, the pathogen of vesicular lesions occurred in a pig farm in Guangdong Province of China was identified as SVA (Wu Q. et al., 2017), and then SVA gradually spread to other provinces in the following years (Liu et al., 2020).
SVDV, the causative agent of Swine vesicular disease (SVD), was first diagnosed in Italy in 1966 (Nardelli et al., 1968). SVDV belongs to the Enterovirus genus in the family Picornaviridae and was considered to be a porcine variant of human coxsackievirus B5 due to their close serological relationship (Graves, 1973). The disease only affects pigs and does not have a serious impact on production. However, SVD has similar clinical symptoms to FMD, which can pose a threat to international trade (Lin and Kitching, 2000; Dibaba, 2019).
These pathogens are difficult to diagnose clinically due to the similar vesicular lesions observed on infected animals. With the development of biotechnology, molecular biology diagnostic technology is constantly innovating and plays an important role in the diagnosis and differentiation of different viruses. In this paper, we mainly describe available and novel emerging diagnostic methods and multiplex assays for the detection of FMDV, SVA and SVDV. Tables 1–3 summarizes some recent studies on molecular diagnostic assays for the detection of FMDV, SVA and SVDV, respectively.
The Vesicular exanthema of swine virus (VESV, Caliciviridae, Vesivirus) can also cause vesicular disease in pigs. The origin of VES was traced to feeding meat from sea mammals to pigs and was eradicated from California populations in 1956. We also briefly refer to the laboratory diagnosis of VESV in this article.
Diagnostic methods
Reverse transcription-polymerase chain reaction (RT-PCR) assay
RT-PCR, one of the most traditional and common nucleic acid detection methods in the laboratory, is used extensively in various fields such as biology and medicine, microbiology and food based on its characteristics of simple operation, high sensitivity and strong specificity. Currently, multiple RT-PCR assays aimed at different viral targets have been reported and used as diagnostic tests.
FMDV is divided into seven serotypes based on genetic and antigenic analyzes. Meyer et al. designed primers based on the highly conserved regions of the seven FMDV serotypes genome and reported the establishment of a rapid and sensitive FMDV RT-PCR detection method (Meyer et al., 1991). Nishi et al. designed the primer set FM8/9 to amplify 644 bases in the conserved 3D region of all seven FMDV serotypes using RT-PCR assay, which is suitable for FMD diagnosis (Nishi et al., 2019). The study found that the sensitivities of FM8/9 primers were 100.6- to 103.8-fold higher than 1F/R primers described in the OIE manual (Nishi et al., 2019). There is a lack of cross-immune protection between different FMDV serotypes, and even within the same serotype, there are differences in the antigenicity of different isolates. Thus, it is necessary to develop a rapid detection method for distinguishing FMDV serotypes. FMDV VP1 coding sequences vary considerably among different FMDV serotypes, so that it can be used to RT-PCR assay for FMDV serotyping (Callens and De Clercq, 1997; Le et al., 2011). Even so, important phenotypic traits of FMDV cannot be reflected by relying on the VP1 coding sequence, since the FMDV genome is prone to variation and recombination events can occurr in the non-structural genes of FMDV (Carrillo et al., 2005; Klein et al., 2007; Brito et al., 2018). Xu et al. developed a universal L-P1 RT-PCR for amplifying and sequencing a 3 kb fragment including FMDV leader and capsid-coding region, which can be used for rapid antigenic characterization of FMDV and phylogenetic analyzes, and is expected to be an instructive for vaccine selection during FMDV outbreaks (Xu et al., 2013). A simple, quick and cost-efficient RT-PCR assay suitable for generating genomic sequences of all FMDV serotypes was described, and this approach can amplify the sequence reaching from the IRES to the end of the open reading frame, which can assist in immediate virus genotyping, phylogenetic analysis, and epidemiological studies of FMDV (Dill et al., 2017).
Since 2015, pig-producing countries such as Brazil (Leme et al., 2015), China (Wu Q. et al., 2017), the United States (Canning et al., 2016), Colombia (Sun et al., 2017), Canada (Xu et al., 2017), and Thailand (Saeng-Chuto et al., 2018) have successively reported cases of SVA-VD. SVA seems to be becoming a globally prevalent virus. Several RT-PCR assays based on SVA conserved genome have been established for viral diagnosis and epidemiological investigation of SVA (Laguardia-Nascimento et al., 2016). Leme et al. designed a primer set which can amplify a 542 bp product size of the VP3/VP1 region of SVA genome in RT-PCR assay, and the primer set can be useful in molecular screening of SVA infection and for characterization of the virus (Leme et al., 2015). Wu et al. designed RT-PCR primers for amplification of the whole SVA genome, and firstly carried out complete genome identification, phylogenetic analysis and clinical characterization of SVA that infected pigs with Porcine Idiopathic Vesicular Disease (PIVD) in China (Wu Q. et al., 2017). Transient viremia can be caused in SVA infected animals. In the meanwhile, viral nucleic can be detected in almost all tissues through RT-PCR. However, after viremia disappeared in affected animals, the virus can only be detected in the tonsils and lymph nodes using this method (Houston et al., 2020). A specific nested-PCR assay based on VP1 fragments of SVA genome was established and the limit of detection was 0.0181 ng/μL for the cell-cultured SVA isolate, which is more sensitive than traditional RT-PCR (Feronato et al., 2018).
Italy was the first country to report the outbreak of SVD in1966 and began an eradication program in 1995 (Nardelli et al., 1968; Bellini et al., 2007). So far, SVD monitoring is still ongoing in Italy and no evidence of SVD activity has been found, which indicated the complete SVD virus eradication from the Italian pig industry (Tamba et al., 2020). Whatever, SVDV has clinically similar symptoms with FMDV and affects trade activities such as pork products, as a result, it is necessary yet for diagnosis and surveillance of SVDV. Several RT-PCR assays based on different targets including capsid, VP1, 3BC and 2A genes were reported for SVDV detection and molecular evolution analysis (Marquardt and Ohlinger, 1995; Lin et al., 1997; Zhang et al., 1999). Nielsen et al. depicted a method for rapid sequencing of the SVDV isolates genomes using the Roche GS FLX Sequencing Platform (Nielsen et al., 2014). Vázquez-Calvo et al. designed PCR Primer sets and reported the first complete coding sequence of a Spanish SVDV isolate (SPA/1/'93)(Vazquez-Calvo et al., 2016). A double PCR method was described for detecting ASFV and SVDV (Peng et al., 2015). Pigs infected with SVDV can shed large amounts of the virus through the nose, mouth and feces to contaminate the surrounding environment. Given the absence of clinical signs, feces are the sample of choice for viral testing to identify subclinical infections (Pezzoni et al., 2020). Sensitive detection methods are necessary due to the low viral content of stool samples. The diagnostic performance of six genomic amplification methods for SVDV detection including RT-PCR, real-time RT-PCR and RT-LAMP assay was evaluated and the result showed that the conventional 3D RT-PCR and the 3D rtRT-PCR using SYBR Green as a detector were still the most sensitive and efficient methods for detecting SVDV in fecal samples (Pezzoni et al., 2020). However, compared with RT-qPCR, the procedure of RT-PCR is more complicated, and agarose gel electrophoresis is required to determine the results of the corresponding samples.
Real-time PCR assay
As the gold standard of laboratory detection, the quantitative real-time reverse transcription PCR (qRT-PCR) assay has been successfully used in the diagnosis of various pathogenic pathogens (Munro et al., 2013; Shi et al., 2016; Fischer et al., 2017; Corman et al., 2020). qRT-PCR allows the quantification of molecular targets in templates by using standard substance. The sample content determination does not need to open the reaction tube, and can be analyzed by the fluorescence signal intensity generated during the qRT-PCR amplification process, avoiding cross-contamination between samples (Galluzzi et al., 2018). qRT-PCR has the advantages of rapidity and sensitivity, and is suitable for different clinical sample types including swabs, sera, vesicular fluid, milk and tissue samples (Burkhalter and Savage, 2017; Fontel et al., 2019; Yeo et al., 2020; Armson et al., 2020a). At present, Real-Time PCR is a commonly used method to detect the pathogens of animal diseases. Usually, the same diagnostic sample is repeated following a positive result to confirm the presence of the disease agent followed by secondary confirmatory testing if the disease is exotic. According to the OIE manual, qRT-PCR amplification of FMDV 3D genome sequence is recommended as a standard method for the viral diagnosis of all FMDV serotypes (Callahan et al., 2002). 5’UTR sequence is also the frequently-used target for FMDV detection (Reid et al., 2002). Both Reid et al. (2014) and Lim et al. (2022) established multiplex real-time RT-PCR based on FMDV VP1 coding region to detect and identify different FMDV serotypes (O, A and Asia). Normally, the FMDV RNA for diagnostic purposes can be harvested from infected animal samples containing blister fluid, blister tissue, serum, milk and swabs of lesion (Armson et al., 2019a; Wong et al., 2020). One study showed that EDTA-stabilized blood samples can be adapted to FMDV detection during FMD outbreak even though the sensitivity of it was 10-fold less than that of serum samples (Fontel et al., 2019). Armson et al. determined the utility of testing pooled milk by rRT-PCR as an alternative approach for FMD surveillance, and proved that pooled milk has potential value as a surveillance sample to reveal subclinical FMD infection (Armson et al., 2020a,b). Yeo et al. amplified the FMDV genomic sequence in meat juice using qRT-PCR and confirmed the presence of FMDV RNA (Yeo et al., 2020). The discovery indicated that meat juice can be used as a good sample type for FMDV detection and play a role in the import and export quarantine of meat products (Yeo et al., 2020). The application of qRT-PCR in low-and middle-income countries (LMICs) was limited because of the complex diagnostic procedures and expensive equipment (Vosloo et al., 2002; Namatovu et al., 2013). Recently, some of the filed-deployable molecular assays for FMD diagnosis were developed and proved the feasibility through research (Howson et al., 2017b, 2018; Hole and Nfon, 2019). Ambagala et al. described a potentially useful field-deployable reverse transcription-insulated isothermal PCR (RT-iiPCR) assay for rapid detection of FMDV in countries with poor equipment conditions (Ambagala et al., 2017). Moreover, a recent study described a portable, handheld real-time PCR platform that can be manipulated via a smartphone app, which has great potential for FMDV detection (Hole and Nfon, 2019).
qRT-PCR enables the fast and accurate diagnosis of any vesicular diseases including SVA, due to the characteristics of high efficiency and sensitivity (Leme et al., 2017). Different qRT-PCR targeting diverse SVA genomic conserved regions (including 3D, VP1 and 5’-UTR) were established for the SVA detection and quantification (Bracht et al., 2016; Gimenez-Lirola et al., 2016; Dall et al., 2017; Fowler et al., 2017; Zhang J. et al. 2019). Joshi et al. utilized real-time reverse transcriptase PCR and virus isolation to screen out the existence of SVA in pigs, mice and houseflies (Joshi et al., 2016). Several researchers detected the viral nucleic acid in tissue, serum, oral swabs and/or rectal swabs from pigs challenged with SVA by qRT-PCR, and found the low viral level in serum, which indicated that SVA can induce transient viremia (Fernandes et al., 2018; Buckley et al., 2021). Moreover, the study suggested that the tonsil may be the main site of SVA replication (Fernandes et al., 2018). Zhang et al. designed primers and probes based on conserved sequences of the viral genome, and established a SVV rRT-PCR targeting the conserved 5’-UTR and a SVV RT-iiPCR targeting the 3D gene, both of which have good consistency in the diagnosis process of clinical samples (Zhang J. et al., 2019).
A variety of real-time quantitative PCR methods have been described for the detection of SVDV (Reid et al., 2004a; Hakhverdyan et al., 2006; Niedbalski, 2009; McMenamy et al., 2011). The use of oral fluid (OF) samples to assess animal group health status has been proposed as an attractive and cost-effective method for disease surveillance, since this approach has no complex collection process and can be operated easily for anyone (Mur et al., 2013; Senthilkumaran et al., 2017). Senthilkumaran et al. utilized a qRT-PCR assay based on SVDV 3D target and an adapted competitive ELISA to detect the levels of viral nucleic acid and antibodies in OF samples (Senthilkumaran et al., 2017). The result showed that the high levels of SVDV RNA could be detected from 1 day post-infection (DPI) to 21 DPI and the antibodies to SVDV could be discovered starting at DPI 6, which indicated the potential value of OF for SVD surveillance (Senthilkumaran et al., 2017). Pezzoni et al. compared the sensitivity of the two real-time RT-PCRs targeted on the 3D region (based on SYBR Green and TaqMan probe detection), and they showed an equivalent analytical sensitivity, while the diagnostic sensitivity of the 3D rtRT-PCR using SYBR Green as a detector was high than the assay based on TaqMan probe detection (Pezzoni et al., 2020).
Droplet digital PCR
ddPCR is considered a graceful adaptation of the current qPCR format and has the potential application value on virus diagnosis (Jones et al., 2014). Its principle is shown in Figure 1. In ddPCR, the clinical sample is divided into thousands of droplets, each of which contains zero or several copies of the target nucleic acids. Molecular targets of each droplet are amplified by PCR and the fluorescence intensity produced by the reaction products is read after amplification, so as to achieve accurate and absolute quantification of target nucleic acid (Taylor et al., 2017). ddPCR does not depend on the standard calibration curve, and has a strong tolerance to PCR inhibitors so that it can realize the direct quantification of clinical samples including stool, blood, and tissue without nucleic acid purification (Taylor et al., 2017; Sahore et al., 2018). The rise of ddPCR is of great significance for the development of precision virus detection platform and viral diagnosis in the early period of infection. At present, dd-PCR has been widely used in the detection of various pathogens such as Epstein-Barr virus; human cytomegalovirus, human T-cell leukemia virus type 1, respiratory syncytial virus (RSV) and Japanese encephalitis virus (JEV) (Lin et al., 2016; Nicot et al., 2016; Wu X. et al., 2017; Persson et al., 2019). A study reported a RT-ddPCR method for the quantification of foot-and-mouth virus RNA, which is based on an OIE-recognized real-time RT-PCR that detects the 3D-encoding region of FMDV (Pinheiro-de-Oliveira et al., 2018). The assay can detect and quantify three serotypes of FMDV (O, A and C) with high specificity, and can detect at least 101.4 TCID50/mL of FMDV and 2 fg of the circularized or linearized plasmid with the same analytical sensitivity of RT-qPCR (Pinheiro-de-Oliveira et al., 2018). Besides, T F Pinheiro-de-Oliveira et al. developed a one-step RT-ddPCR method with superior performance to identify SVA in biological samples (Pinheiro-de-Oliveira et al., 2019). Based on the high sensitivity and specificity, the one-step RT-ddPCR can be a useful tool for the prevention and control of vesicular diseases such as SVA (Pinheiro-de-Oliveira et al., 2019). Zhang et al. also established a novel RT-ddPCR assay targeting the conserved SVA polymerase 3D gene, and the detection limit of this assay was 10-fold more sensitive than the RT-rPCR assay (Zhang Z. et al. 2019). Currently, there is no digital PCR reported for SVDV.
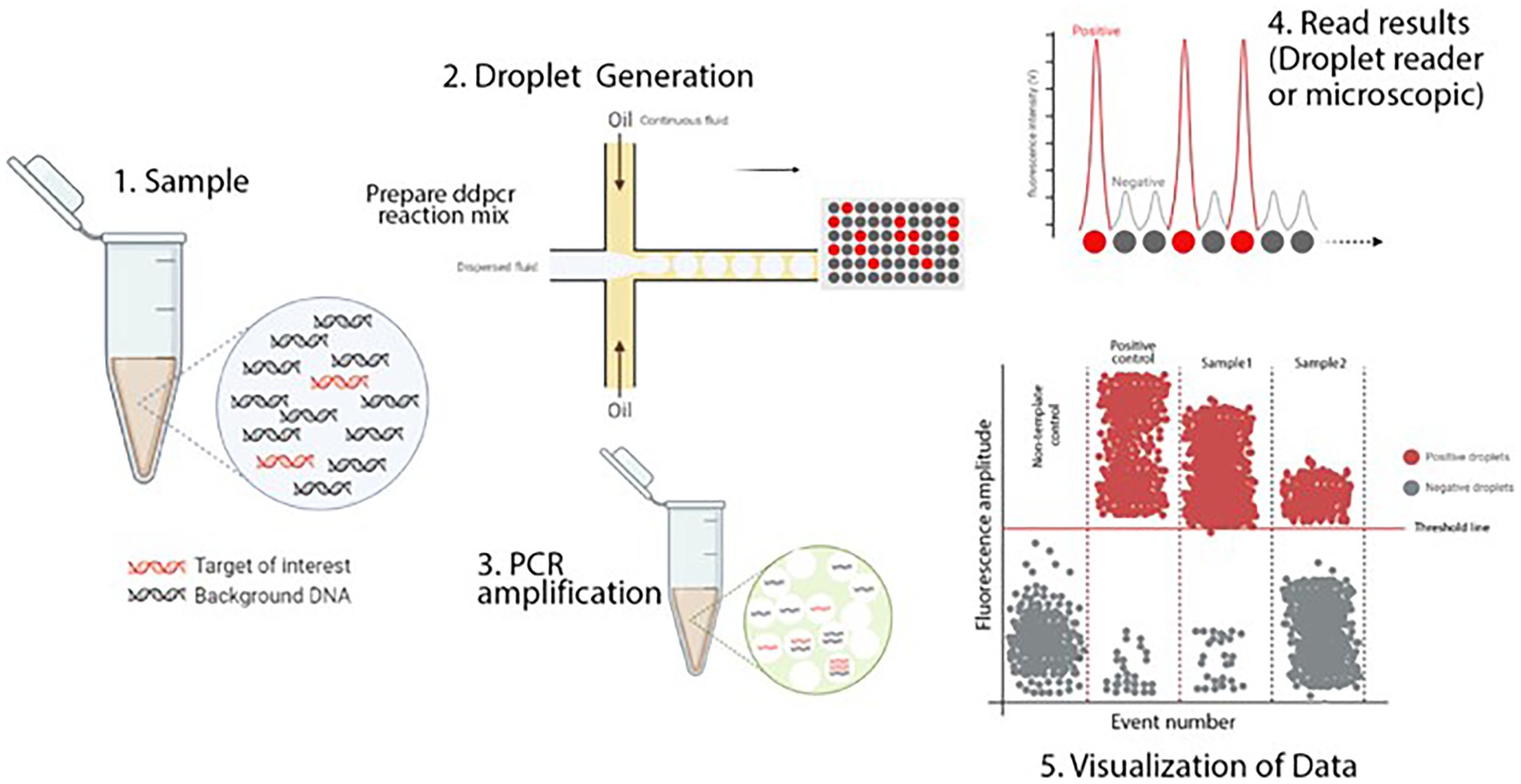
Figure 1. Schematic illustration of ddPCR. This figure illustrates the digital PCR droplet principle and how a single sample containing the target sequence is partitioned. PCR amplified produces thousands of copies that can be detected and interpreted by a detection system. In a typical ddPCR workflow, a single sample includes target and nonspecific sequences (DNA or RNA), real-time PCR primers and fluorescent-labeled probes, and standard real-time PCR master mixes. (1) A sample partitioned into thousands of single nanoliter droplets with the generation of water-in-oil emulsions. A proportion of droplets contain no template molecules, while others contain one or more targets. The generation of droplets from the sample is achieved in several ways. Standard methods include T-junction and flow-focusing geometry. Only the flow-focusing geometry is shown here. For more details, see the text. (2) Traditional end-point PCR is then performed to amplify the target sequence. (3) Target sequence droplets exhibit higher fluorescence intensity and are known to be positive droplets. Empty or no targets show low and negative fluorescent intensity. This fluorescence intensity versus time is plotted on a graph. The number of targets per partition will follow the normal Poisson distribution encapsulation of the DNA or RNA that occurs randomly. Various methods can interpret the fluorescent intensity of droplets. The most popular of these is a fluorescent microscope or a droplet reader. (4) The acquired data is visualized in a graph by different software. The threshold value indicates the intensity of fluorescence as the positive particles are separated from the negative. Although this value is set automatically by the software, it can be adjusted manually (Kojabad et al., 2021).
ddPCR has the characteristic of accurate quantification and can quantify the mutated target genes by using a small amount of sample, which is important in the detection of rare mutation targets (Huggett and Whale, 2013; Milbury et al., 2014). In addition, it gives great benefits in fast and easy diagnosis for some diseases difficulted to identify accurately due to the high sensitivity and specificity of ddPCR (Li et al., 2018). However, ddPCR is still limited in use even in many modern diagnostic laboratories for routine testing (Liao and Huang, 2017). In terms of technology use, professional personnel is required for ddPCR operations and results reading. Anyhow, as a novel, sensitive, accurate and promising quantitative technique, ddPCR plays an important role in the monitoring of cancer, pathogens, water environment and so on (Li et al., 2018; Postel et al., 2018; Kojabad et al., 2021; Tiwari et al., 2022).
Loop-mediated isothermal amplification
Loop-mediated isothermal amplification (LAMP) is an isothermal amplification approach developed in 2000, and has become the focus of point-of-care (POC) diagnostics due to its simple operation, rapid measurement and high sensitivity of this assay (Notomi et al., 2000). As shown in Figure 2 (Bhat et al., 2022), under the strand displacement activity of the Bst DNA polymerase from Bacillus stearothermophilus in 60-65oC, the target nucleotide was transformed into a stem-loop structure through the exploit of four pairs of primers (outward forward (F3), outward backward (B3), forward inner (FIP), and backward inner (BIP)). Then the stem-loop structure containing multiple initiation sites was used as the template of LAMP reaction for cyclic amplification, and many nucleotide chains with different lengths were generated finally. The results of LAMP assay can be visualized through the following ways (Nie, 2005; Almasi et al., 2014; Mansour et al., 2015; Peng et al., 2021; Bhat et al., 2022), (1) by agarose gel electrophoresis; (2) measuring the fluorescence emitted by SYBR Green or other dyes on a real-time basis; (3) the turbidity of LAMP reaction based on magnesium pyrophosphate; (4) the green fluorescence intensity based on calcein-manganese or fluorexon under UV light; (5) be visualized with a lateral flow device (LFD).
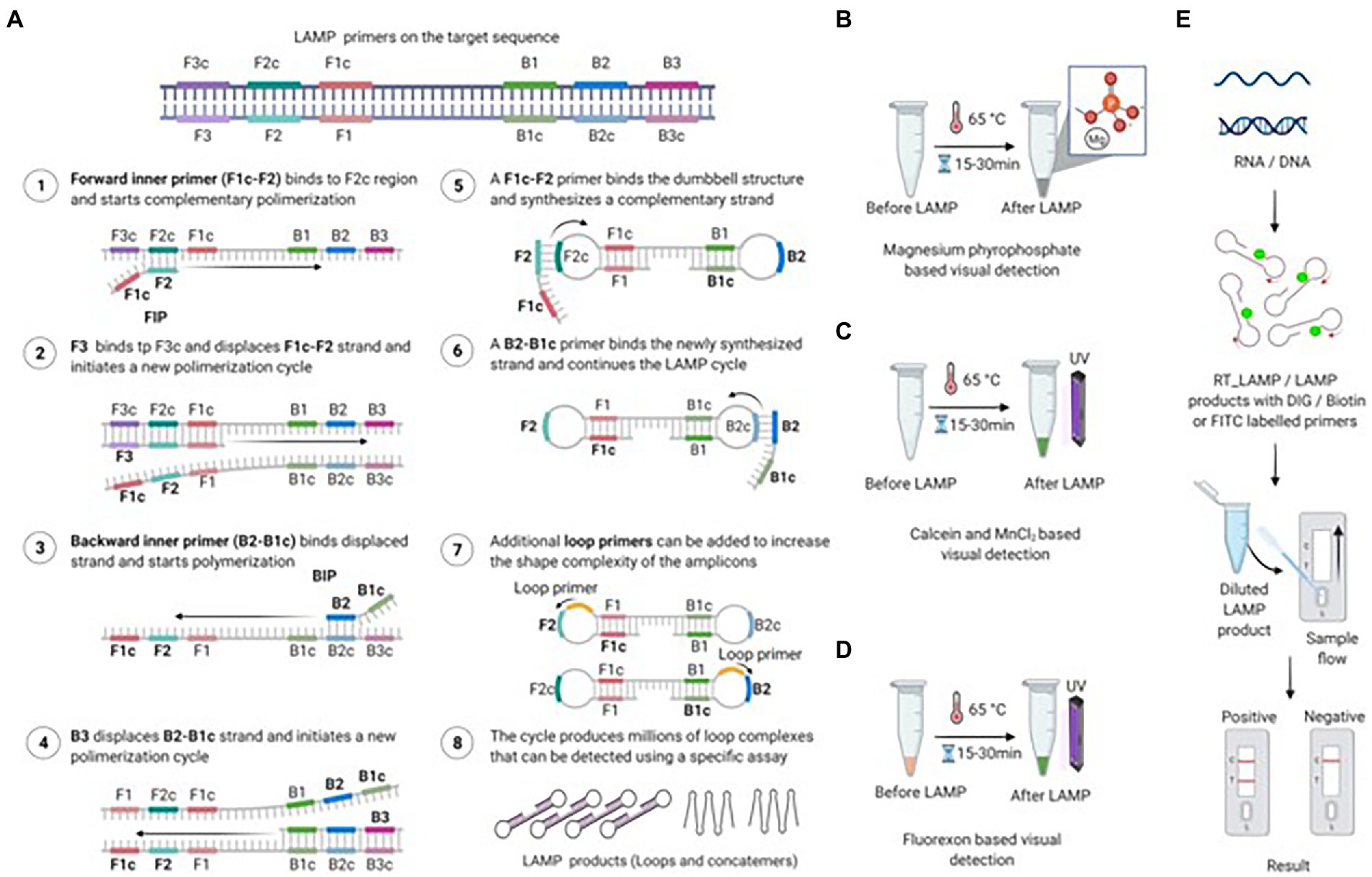
Figure 2. Amplification via loop-mediated isothermal amplification (LAMP) and various detection methods. (A) Diagram of LAMP primers located on the target sequence and the amplification process. The FIP primer binds to its complementary sequence and begins amplifying the first strand, followed by binding and amplification of the second strand by the F3 primer. The following steps involve the amplification of the FIP-amplified strand by the B3 primer. Finally, FIP and BIP continue the LAMP cycle, resulting in dumbbell-shaped amplicons. A full stepwise description of the individual steps is provided in the figure. (B) Magnesium pyrophosphate-based visual detection of the LAMP product. During LAMP, a large amount of pyrophosphate ion is produced as a by-product, which reacts with magnesium provided in the reaction mixture. The resulting product, magnesium pyrophosphate, forms a white precipitate that allows easy visual detection. The turbidity of the final LAMP reaction confirms the presence or absence of the targeted nucleic acid. (C) Calcein-based fluorescence detection of the LAMP product. The reaction of the by-product pyrophosphate with magnesium or calcein-manganese aids in the visualization of the LAMP product by producing a precipitate or emitting bright green fluorescence under UV light. Calcein-based fluorescence is enhanced by the presence of magnesium in the reaction mixture. (D) Fluorexon-based visual detection of the LAMP product. Upon completion of the LAMP reaction, fluorexon-MnCl turns from orange to green. The resulting green fluorescence is visualized with the naked eye under UV light. (E) Lateral flow-based detection of the LAMP product. To visualize LAMP with LFA, the target nucleic acid (RNA/DNA) is amplified via RT-LAMP or LAMP with DIG, biotin, or FITC-labeled primers. The labeled LAMP product is diluted and applied to the lateral flow strip. After 5 to 15 min of incubation, the appearance of bands on the test and control lines indicates the presence of the target nucleic acid (Bhat et al., 2022).
To date, several reverse transcription LAMP (RT-LAMP) assays have been developed that are capable of detecting all seven FMDV serotypes and serotype-specific detection of O, A, C and Asia 1 FMDVs (Lim et al., 2020). Timely diagnosis is essential for the control, monitoring and eradication of FMD. Compared with PCR, LAMP does not require complex temperature cycling conditions and requires less time to perform allowing it to be used for FMDV rapid detection in the field. The use of lyophilized reagents allows for pathogen detection in field environments where equipment conditions are insufficient. Howson et al. described the performance of lyophilized rRT-PCR and RT-LAMP assays to detect FMDV (Howson et al., 2017a). They found that lyophilization greatly improved the storage stability of test reagents without jeopardizing the assays' performance, which further supported the potential on field diagnosis of RT-LAMP assay (Howson et al., 2017a). Recently, Lim et al. developed a probe-based real-time reverse transcription loop-mediated isothermal amplification (RRT-LAMP) assay for rapid and specific detection of FMDV, and the detection limit of the assay was 102 copies/μL which is comparable to that achieved by qRT-PCR (Lim et al., 2020). Rapid and accurate detection of SVA is necessary to confirm the disease causing agent, and to initiate the implementation of control processes. Zeng et al. described a real time RT-LAMP targeting the VP1 and VP2 regions of SVA and this assay can detect at least 1 TCID50/mL of virus titers (Zeng et al., 2018). Li et al. combined RT-LAMP with a lateral flow dipstick (LFD) for spot rapid diagnosis of SVA (Li et al., 2019). Armson et al. established SVV-1 RT-LAMP assay using lyophilized reagents, which negated the requirement for RNA extraction and the time of sample amplification was reduced within 12 min (Armson et al., 2019b). RT-LAMP technology is also used in the rapid detection of SVDV. Blomström et al. developed a one-step RT-LAMP assay based on the highly conserved 3D polymerase gene to test viral nucleic acid easily and quickly (Blomström et al., 2008).
Unlike PCR assays, LAMP does not require expensive equipment and complex cycling-temperature conditions, and can be easily performed by an untrained person. The designing principle of LAMP primers is a bit complicated. LAMP primers can be designed using the free online software package Primer Explorer version 51 or paid software2 (Bhat et al., 2022). On the whole, LAMP is a potential tool for use of pathogen detection in resource-poor environments.
Recombinase polymerase amplification
RPA was originally developed by Piepenburg and collaborators for the use of human pathogen detections, and was later applied in the diagnosis of different RNA targets (Piepenburg et al., 2006; Silva et al., 2018). Generally speaking, RPA reaction contains 3 main protein components: uvsX recombinase from T4 phage, DNA polymerase, and single-stranded DNA binding protein (SSB). As shown in Figure 3, the recombinase protein can bind with primers to form a complex that can recognize and pair complementary sequences of the template under constant temperature. Then the target nucleic acid is amplified in large quantities under the action of polymerase and binding enzyme. Recombinase-aided amplification (RAA) developed by Qitian (Wuxi, China) has similar amplification rules with RPA while the recombinant enzyme is obtained from Escherichia coli (Shen et al., 2019). Similarly, the results of RPA can be visualized by agarose gel electrophoresis while it is easy to appear smeared bands. To avoid this phenomenon, the RPA product can be purified to remove proteins and crowding agents prior to gel electrophoresis (Bhat et al., 2022). In addition, real-time fluorimeter and lateral flow assay can also be used to detect RPA products when using intercalating dyes and specific probes (Daher et al., 2016; Lobato and O'Sullivan, 2018; Powell et al., 2018). A RPA reaction is incubated at a single temperature of 25–42oC without the need for thermocycling, and the time of DNA amplification is usually between 5 and 30 min, which is faster than that of other nucleic acid amplification techniques such as PCR assay (Piepenburg et al., 2006; LaBarre et al., 2011).
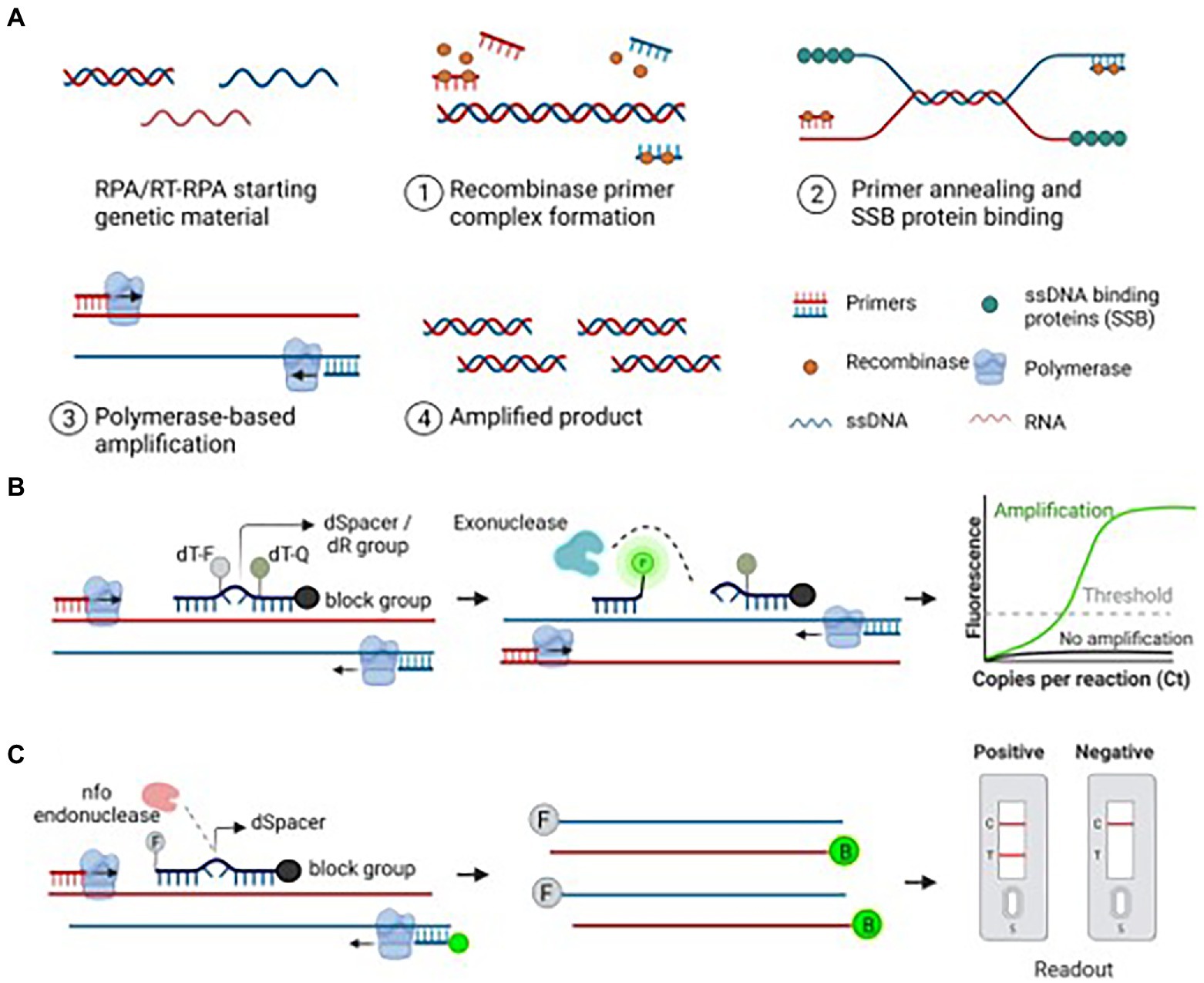
Figure 3. Recombinase polymerase amplification (RPA)-based amplification and its detection methods. (A) Schematic diagram of the RPA assay. An ideal RPA assay consists of forward and reverse primers, a recombinase protein that helps bind primers to the target nucleic acid, ssDNA-binding proteins to stabilize the ssDNA, and a polymerase to amplify the primer-bound strands. The stepwise amplification process is shown in the figure. (B) Exonuclease-based detection of the RPA product. A 46–52 bp long Exo probe flanked by a quencher and fluorophore binds to the amplified product. Exo probe contains a THF (tetrahydrofuran) residue known as dSpacer, which is cleaved by the exonuclease, thus releasing the fluorophore from the quencher. The observed fluorescent signal indicates the presence of the target nucleic acid. (C) Endonuclease-based detection of the RPA product. A 46–52 bp long oligonucleotide probe labeled with FAM or Alexa fluor binds to the target strand. The annealed probe is cleaved by the nfo enzyme, freeing the 3′-OH group of the probe and is used as a primer in the subsequent reactions. The resulting amplicons are produced with FAM and biotin using a biotin-labeled reverse primer. The final RPA product is applied to the LFA strip, and the results are visualized after the appearance of matching lanes (Bhat et al., 2022).
RPA has been successfully used to detect several infectious agents, including viruses. Howson et al. defined the relative performance of RT-RPA and RT-LAMP for the detection of FMDV, benchmarked against rRT-PCR (Howson et al., 2017b). They found that RT-LAMP can detect FMDV RNA without RNA extraction, while accurate results by RT-RPA only obtained when using RNA extraction (Howson et al., 2017b). A visible and equipment-free RT-RPA combined with lateral flow strip (LFS RT-RPA) was developed to detect FMDV 3D gene (Liu et al., 2018). The FMDV LFS RT-RPA assay was performed successfully in a closed fist using body heat for 15 min, and the products were visible on the LFS inspected by the naked eyes within 2 min (Liu et al., 2018). Wang et al. established a series of serotype-specific RT-RPA assays combined with LFD to differentiate FMDV serotypes A, O or Asia 1, respectively, and the detection limits of these assays were 3 copies of plasmid DNA or 50 copies of viral RNA per reaction (Wang H. et al., 2018). Wang et al. developed a VP2-based real-time fluorescent reverse transcription RPA (rRT-RPA) assay to rapidly detect SVA, and the detection limit of this assay is 1.185 TCID50, which is comparable to that of a previously published rRT-PCR assay (Wang et al., 2021). A simple, rapid and accurate (RPA-LF) diagnostic assay for SVA detection was developed, and the optimal reaction conditions were incubated at 35 °C for 25 min, and the result was visualized directly on the dipstrip (Wang et al., 2022).
Luminex (beads-based technology)
In 2001, Luminex technology, a bead-based detection platform, became the first multi-target detection technology approved by FDA for clinical diagnosis. Luminex has two core technologies including xMAP and xTAG. xMAP, a solution-phase array composed of spectrally distinct microspheres (“beads”), could detect up to 100 targets in a reaction by coupling probes of different targets with microspheres. The TAG sequence is a generic TAG unique to Luminex and consists of 24 bases. The primers with TAG sequence were mixed with nucleic acids for PCR amplification to obtain the target products containing TAG sequence. The TAG sequence specifically combined with the anti-TAG sequence on the beads to acquire a “beads-detector.” Then the fluorescence of each microsphere was detected and analyzed by flow cytometry. With the advantages of high throughput, rapidity, sensitivity and accuracy, Luminex technology has been widely used in many fields such as drug development, disease diagnosis and food safety. Wu et al. developed a PCR-based fluorescent Luminex assay for human papillomavirus (HPV) genotyping, and the assay may be used to provide critical clinical information for the early detection of HPV (Oh et al., 2007). A Luminex xTAG multiplex detection method with high sensitivity and specificity, was developed for the simultaneous detection of 11 porcine viral diarrhea pathogens (Shi et al., 2021). A total of 753 porcine stool specimens from five districts in Shanghai were detected using this assay and the surveillance confirmed that PEDV is still the main pathogen of porcine diarrhea (Shi et al., 2021). Wang et al. reported a multiplex Luminex assay for rapid detection and differentiation of the PRRSV field strains and vaccine strains (Wang et al., 2020b). Compared to RT-qPCR method, Luminex assay can achieve higher strain coverage since only two oligos may be required in the detection system (Wang et al., 2020b). A rapid, highly multiplexed nucleic acid assay employing Luminex™ liquid array technology was developed early for the differential diagnosis of FMDV, SVDV and other vesicular diseases pathogens (Lenhoff et al., 2008). Due to its high-throughput characteristics, Luminex technology enables multiplex detection of pathogens. A variety of commercial kits based on Luminex technology for different pathogens have also been developed, which provides convenience for the rapid diagnosis of different pathogens, especially those with similar symptoms (Krunic et al., 2011; Gray and Coupland, 2014; Glushakova et al., 2019).
CRISPR-Cas technology
In order to deal with the threat of emerging and re-emerging infectious diseases, rapid detection of pathogen specific nucleic acid is essential. As a novel biosensing platform, CRISPR-Cas technology plays a key role in gene editing and molecular diagnosis. The system was originally discovered to resist the invasion of foreign DNA and viruses in bacteria and archaea (Wiedenheft et al., 2012). Subsequently, CRISPR-Cas9 system was developed as a powerful genome engineering tool and applied in various fields, especially in the treatment of genetic diseases, screening and detection of disease-related genes, cancer treatment, transformation of animals and plants, and prevention of pathogenic microorganisms (Yin et al., 2021). Cas12a is a single RNA-guided endonuclease lacking trans-activating crRNA (tracrRNA), which could recognize a T-rich PAM and exert the DNA cleavage activity (Zetsche et al., 2015). Unlike Cas12a, Cas13a targets and cleaves RNA at multiple locations without recognizing PAM (Yin et al., 2021). Intriguingly, both of the two nucleases exhibit collateral, nonspecific activities on random ssDNA or ssRNA respectively upon target recognition, so that they are respectively explored for nucleic acid detection (Yin et al., 2021). Diagnostic tool DETECTOR based on Cas12a (Chen et al., 2018) and SHERLOCK based on Cas13a (Gootenberg et al., 2017) were successively developed for DNA or RNA diagnosis (Figure 4). When the two nucleases recognized the corresponding targets, the trans-cleavage activity of Cas12a and Cas13a was activated to degrade the doubly labeled fluorescent reporter, which could produce fluorescence. CRISPR-Cas systems are developed as novel and powerful diagnostic techniques, and could use different strategies for virus detection including different signal readouts and lateral flow assays (LFA) (Yin et al., 2021). In general, good real-time PCR assays can detect 10 or less genome copies in a sample and the CRISPR-Cas assays can detect at similar levels. Meanwhile, combined with LFA, CRISPR-Cas technology is being developed as a sensitive, specific, rapid molecular diagnostic tool with potential, and shows great advantages in field-deployable detection. Multiple detection methods based on CRISPR-Cas12a/Cas13a were developed for different virus diagnosis such as influenza A viruses (Liu et al., 2019), SARS-CoV-2(Broughton et al., 2020), ASFV (Yang et al., 2022), PRRSV (Chang et al., 2020) and JEV (Xu et al., 2022). Multiplex detection of viruses
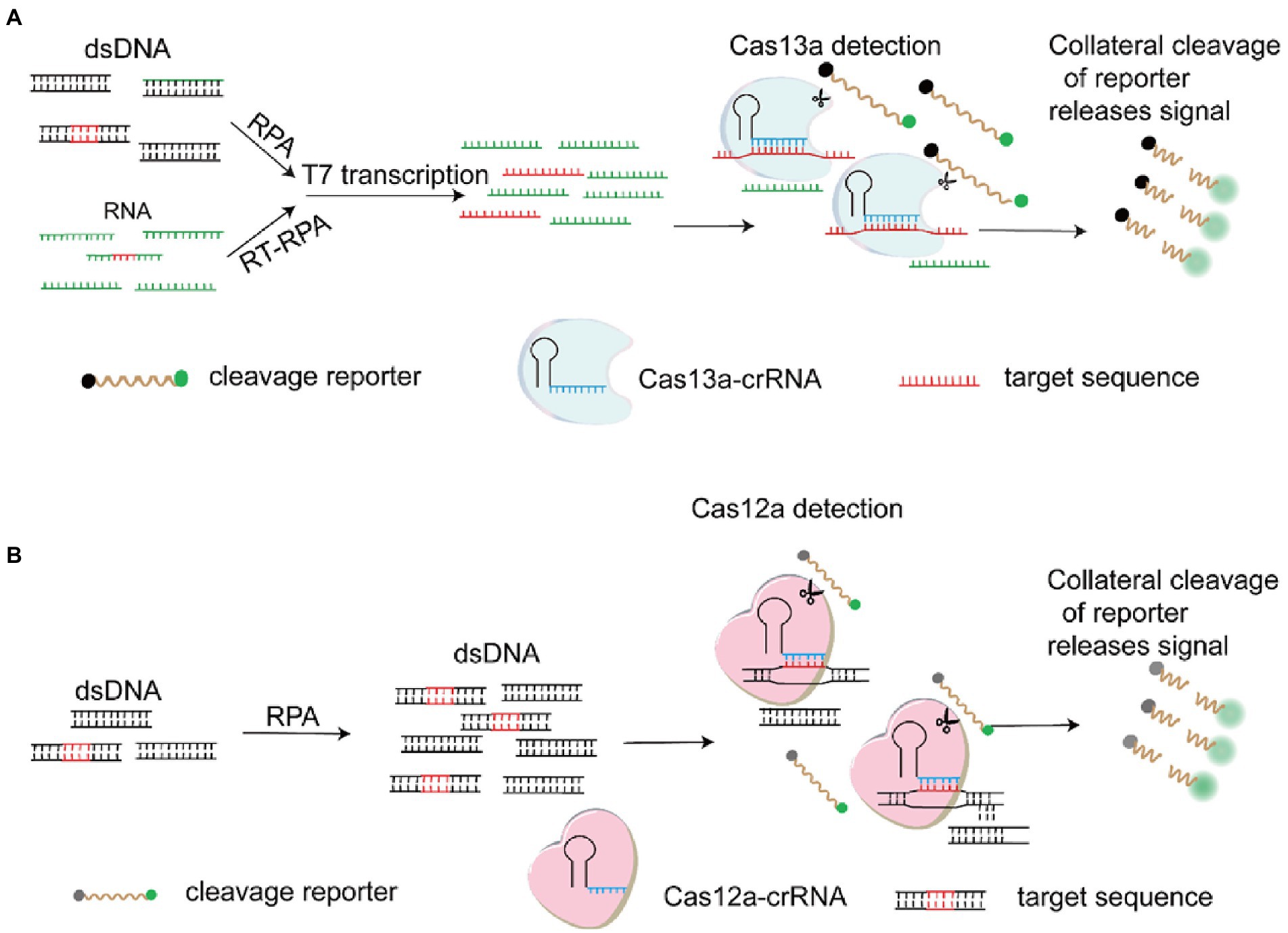
Figure 4. Schematic of the SHERLOCK and DETECTR. (A) CRISPR-Cas13a based detection (SHERLOCK). Following the RPA (Recombinase Polymerase Amplification) or RT (Reverse Transcription)-RPA, the RNA target recognized by Cas13a-crRNA complex is produced by in vitro transcription of the DNA amplicons. On recognition of the RNA target, the trans-cleavage activity of Cas13a is activated, resulting in the degradation of the doubly labeled fluorescent reporter. (B) CRISPR-Cas12a based detection (DETECTR). The RPA amplicon is used directly as the target for the Cas12a-crRNA complex. On the recognition of the DNA target, the trans-cleavage activity of Cas12a is activated, resulting in the degradation of the doubly labeled fluorescent reporter (Yin et al., 2021).
Different molecular biological detection methods including those mentioned earlier have played an important role in disease diagnosis and pathogen monitoring. PCR and other amplification reactions using different primer pairs simultaneously can quickly confirm the exact causative agent. Multiple specific primers pairs (including SVA, FMDV, SVDV and VSV) were used to identify SVA as the pathogen of vesicular disease in PIVD piglets from Guangdong and Hubei province, China (Qian et al., 2016; Zhang et al., 2018). However, these primers are reacted in separate tubes, so that it is time consuming, labor intensive and not cost-effective. The advantage of multiplex tests is that they increase the chance of identifying the microbial causes of viral infections and can detect more than one pathogen at a single time point when there are co-infections. Multiplex PCR (mPCR) is a validated strategy for the rapid detection and precise identification of a large number of viruses by incorporating several primers within one reaction tube to amplify genomic fragments of many pathogens. Fernandez et al. established a highly sensitive and specific one-step multiplex RT-PCR assay for the simultaneous and differential detection of FMDV, SVDV and vesicular stomatitis virus (VSV) (Fernandez et al., 2008). Lung et al. developed a multiplex RT-PCR and microarray for the detection/typing of 4 vesicular disease viruses (FMDV, VSV, SVDV, VESV) (Lung et al., 2011). In this study, multiple virus-specific probes for each of the four target viruses and serotype-specific probes for all VSV and FMDV serotypes were identified. Probe redundancy is an important advantage of microarrays over real-time RT-PCR in allowing broader coverage of diverse strains (Erickson et al., 2018). The use of a large number of probes increases the confidence of positive results and also decreases the likelihood of generating false negative results that may occur due to genetic changes resulting from virus evolution. Several other microarray assays have been described recently for the detection of vesicular disease viruses, but only two of these serotyped any viruses: FMDV (Baxi et al., 2006) and VSV (Baner et al., 2007). The assay used padlock probe methodology and both rolling circle amplification and PCR amplification. This assay targeted three vesicular disease viruses (FMDV, VSV and SVDV), but not VESV. Padlock probe technology has the capacity for highly multiplex assays and is promising for the detection and typing of genetically variable RNA viruses because the number of conserved regions on the target needed for amplification and detection is reduced when compared with conventional PCR amplification. The assay as described by Banér et al. had a run time that was comparable to real-time RT-PCR, but required separate addition of several reagents and the assay did not serotype FMDV. Furthermore, the sensitivity of the assay presented here appears to be higher for all three vesicular disease viruses targeted by Baner et al. (2007), although viral strains used for evaluation of the diagnostic sensitivity were different between the two studies. The single virus FMDV serotyping assay developed by Baxi et al. (2006) was expanded in this current work by including a multiplex RT-PCR for four vesicular disease viruses and a microarray that serotypes VSV in addition to FMDV. The additional information obtained regarding the presence or absence of differential pathogens may make the assay an attractive screening tool for vesicular viruses for use on a herd basis. Erickson and the colleagues combined PCR assay with microarray technology to develop a multiplex reverse transcription PCR and automated electronic microarray assay for detection and differentiation of FMDV, SVDV, VESV, CSFV, and ASFV (Erickson et al., 2018). Even though these assays with microarrays have been demonstrated to be effective, these microarrays are not used for diagnostics due to the cost and complexity of them. Shi et al. developed and validated a panel of multiplex real-time assays for simultaneous detection and differentiation of 12 important viruses and viral serotypes (Shi et al., 2016). The newly developed panel of multiplex real-time PCR assays offers a rapid, high-throughput, and reliable screening system for the 12 major viruses including FMDV and SVDV in swine (Shi et al., 2016). Recently, multiplex real-time RT-PCR assays for the detection and differentiation of FMDV and SVA was also developed and evaluated (Wang et al., 2020a).
Concluding remarks
In recent years, with the growth of international trade, the risk of cross-border transmission of certain diseases has increased significantly. In order to prevent the spread of the vital pathogens that could harm economic animals, accurate diagnosis is essential. The possible disease agent can be determined through the observation of clinical symptoms and pathological changes of sick animals. However, the viruses with similar symptoms could not be distinguished such as FMDV, SVA and SVDV. Virus isolation is time-consuming and requires multiple blind passes of samples to produce usable amounts of infectious material. Further accurate and rapid confirmation of pathogens is required by serological and molecular biological methods. With the development of biotechnology, different molecular diagnostic techniques are constantly developing, which play a significant effect in the pathogen detection of swine vesicular diseases. RT-PCR and real-time RT-PCR are still the most reliable methods for detecting the viruses including FMDV, SVA and SVDV, among which real-time RT-PCR with high sensitivity is regarded as the gold standard. The development of multiplex detection provides a powerful technical means for the diagnosis of pathogens with similar clinical symptoms and the detection of co-infections. The combination of isothermal nucleic acid amplification method and lateral flow test can get rid of the limitation of instruments, save time and effort, and be helpful for rapid and accurate diagnosis on-site. How to improve sensitivity is the key to the development of this technology. Luminex technology has the advantages of high throughput, wide detection range and small sample size. In addition, it is suitable for large-scale screening of clinical samples, especially for the detection of multiple infections. The technology provides a new platform for high-throughput nucleic acid detection, and is widely used in the detection of various infectious diseases. CRISPR-Cas, characterized by sensitivity, specificity and programmability for nucleic acid recognition, has been repurposed for molecular diagnostics, thereby advancing a new path in biosensing. Disease diagnosis is an important part of epidemic prevention and control. New rapid and sensitive detection platforms applicable to the field are required to provide more time-saving and effective means in the outbreak of infectious diseases and minimize economic losses. Surveillance for FMDV and other viruses with similar symptoms including SVA, SVDV and VESV is necessary. The key to field diagnosis is the development of simple, low cost, user friendly, highly sensitive and specific rapid tests. This can be potentially accomplished using inexpensive, portable fluorescent instruments and test strips.
Author contributions
WC: writing—original draft preparation. WW and XW: writing—review and editing. ZL, XL, and KW: conceptualization, supervision, and project administration. YL, LY, MZ, HD, SF, and JC: contributed to both the conception and design of the work. All authors have read and agreed to the published version of the manuscript.
Funding
This work was supported by grants from the National Key R&D Program of China (2021YFD1800300); the Program of National Natural Science Foundation of China (No. 32172824); the Key Research Projects of Universities in Guangdong Province (No. 2019KZDXM026).
Conflict of interest
The authors declare that the research was conducted in the absence of any commercial or financial relationships that could be construed as a potential conflict of interest.
Publisher’s note
All claims expressed in this article are solely those of the authors and do not necessarily represent those of their affiliated organizations, or those of the publisher, the editors and the reviewers. Any product that may be evaluated in this article, or claim that may be made by its manufacturer, is not guaranteed or endorsed by the publisher.
Footnotes
References
Abd, E. W. A., El-Deeb, A., El-Tholoth, M., Abd, E. K. H., Ahmed, A., Hassan, S., et al. (2013). A portable reverse transcription recombinase polymerase amplification assay for rapid detection of foot-and-mouth disease virus. Plos One. 8:e71642. doi: 10.1371/journal.pone.0071642
Almasi, M. A., Hosseyni-Dehabadi, S. M., and Aghapour-ojaghkandi, M. (2014). Comparison and evaluation of three diagnostic methods for detection of beet curly top virus in sugar beet using different visualizing systems. Appl. Biochem. Biotechnol. 173, 1836–1848. doi: 10.1007/s12010-014-0970-7
Ambagala, A., Fisher, M., Goolia, M., Nfon, C., Furukawa-Stoffer, T., Ortega, P. R., et al. (2017). Field-deployable reverse transcription-insulated isothermal pcr (rt-iipcr) assay for rapid and sensitive detection of foot-and-mouth disease virus. Transbound. Emerg. Dis. 64, 1610–1623. doi: 10.1111/tbed.12554
Armson, B., Di Nardo, A., Nyaguthii, D. M., Sanz-Bernardo, B., Kitala, P. M., Chepkwony, E., et al. (2020a). Utilizing milk from pooling facilities as a novel approach for foot-and-mouth disease surveillance. Transbound. Emerg. Dis. 67, 1532–1542. doi: 10.1111/tbed.13487
Armson, B., Gubbins, S., Mioulet, V., Qasim, I. A., King, D. P., and Lyons, N. A. (2020b). Foot-and-mouth disease surveillance using pooled milk on a large-scale dairy farm in an endemic setting. Front. Vet. Sci. 7:264. doi: 10.3389/fvets.2020.00264
Armson, B., Wadsworth, J., Kibona, T., Mshanga, D., Fowler, V. L., Knowles, N. J., et al. (2019a). Opportunities for enhanced surveillance of foot-and-mouth disease in endemic settings using milk samples. Transbound. Emerg. Dis. 66, 1405–1410. doi: 10.1111/tbed.13146
Armson, B., Walsh, C., Morant, N., Fowler, V. L., Knowles, N. J., and Clark, D. (2019b). The development of two field-ready reverse transcription loop-mediated isothermal amplification assays for the rapid detection of seneca valley virus 1. Transbound. Emerg. Dis. 66, 497–504. doi: 10.1111/tbed.13051
Baner, J., Gyarmati, P., Yacoub, A., Hakhverdyan, M., Stenberg, J., Ericsson, O., et al. (2007). Microarray-based molecular detection of foot-and-mouth disease, vesicular stomatitis and swine vesicular disease viruses, using padlock probes. J. Virol. Methods. 143, 200–206. doi: 10.1016/j.jviromet.2007.03.004
Baxi, M. K., Baxi, S., Clavijo, A., Burton, K. M., and Deregt, D. (2006). Microarray-based detection and typing of foot-and-mouth disease virus. Vet. J. 172, 473–481. doi: 10.1016/j.tvjl.2005.07.007
Bellini, S., Santucci, U., Zanardi, G., Brocchi, E., and Marabelli, R. (2007). Swine vesicular disease surveillance and eradication activities in Italy. Rev Sci Tech. 26, 585–593.
Bhat, A. I., Aman, R., and Mahfouz, M. (2022). Onsite detection of plant viruses using isothermal amplification assays. Plant Biotechnol. J. 20, 1859–1873. doi: 10.1111/pbi.13871
Blomström, A., Hakhverdyan, M., Reid, S. M., Dukes, J. P., King, D. P., Belák, S., et al. (2008). A one-step reverse transcriptase loop-mediated isothermal amplification assay for simple and rapid detection of swine vesicular disease virus. J. Virol. Methods. 147, 188–193. doi: 10.1016/j.jviromet.2007.08.023
Bracht, A. J., O'Hearn, E. S., Fabian, A. W., Barrette, R. W., and Sayed, A. (2016). Real-time reverse transcription PCR assay for detection of Senecavirus A in swine vesicular diagnostic specimens. PLoS One. 11:e146211. doi: 10.1371/journal.pone.0146211
Brito, B., Pauszek, S. J., Hartwig, E. J., Smoliga, G. R., Vu, L. T., Dong, P. V., et al. (2018). A traditional evolutionary history of foot-and-mouth disease viruses in southeast Asia challenged by analyses of non-structural protein coding sequences. Sci Rep. 8:6472. doi: 10.1038/s41598-018-24870-6
Broughton, J. P., Deng, X., Yu, G., Fasching, C. L., Servellita, V., Singh, J., et al. (2020). Crispr-cas 12-based detection of SARS-cov-2. Nat. Biotechnol. 38, 870–874. doi: 10.1038/s41587-020-0513-4
Brown, V. R., Miller, R. S., McKee, S. C., Ernst, K. H., Didero, N. M., Maison, R. M., et al. (2021). Risks of introduction and economic consequences associated with African swine fever, classical swine fever and foot-and-mouth disease: a review of the literature. Transbound. Emerg. Dis. 68, 1910–1965. doi: 10.1111/tbed.13919
Brown, E., Nelson, N., Gubbins, S., and Colenutt, C. (2022). Airborne transmission of foot-and-mouth disease virus: a review of past and present perspectives. Viruses. 14. doi: 10.3390/v14051009
Buckley, A. C., Michael, D. D., Faaberg, K. S., Guo, B., Yoon, K., and Lager, K. M. (2021). Comparison of historical and contemporary isolates of Senecavirus A. Vet. Microbiol. 253:108946. doi: 10.1016/j.vetmic.2020.108946
Burke, M. J. (2016). Oncolytic Seneca valley virus: past perspectives and future directions. Oncolytic Virother. 5, 81–89. doi: 10.2147/OV.S96915
Burkhalter, K. L., and Savage, H. M. (2017). Detection of zika virus in desiccated mosquitoes by real-time reverse transcription PCR and plaque assay. Emerg. Infect. Dis. 23, 680–681. doi: 10.3201/eid2304.161772
Callahan, J. D., Brown, F., Osorio, F. A., Sur, J. H., Kramer, E., Long, G. W., et al. (2002). Use of a portable real-time reverse transcriptase-polymerase chain reaction assay for rapid detection of foot-and-mouth disease virus. J. Am. Vet. Med. Assoc. 220, 1636–1642. doi: 10.2460/javma.2002.220.1636
Callens, M., and De Clercq, K. (1997). Differentiation of the seven serotypes of foot-and-mouth disease virus by reverse transcriptase polymerase chain reaction. J. Virol. Methods. 67, 35–44. doi: 10.1016/s0166-0934(97)00074-8
Canning, P., Canon, A., Bates, J. L., Gerardy, K., Linhares, D. C. L., Piñeyro, P. E., et al. (2016). Neonatal mortality, vesicular lesions and lameness associated with Senecavirus A in a U.S. Sow farm. Transbound. Emerg. Dis. 63, 373–378. doi: 10.1111/tbed.12516
Carrillo, C., Tulman, E. R., Delhon, G., Lu, Z., Carreno, A., Vagnozzi, A., et al. (2005). Comparative genomics of foot-and-mouth disease virus. J. Virol. 79, 6487–6504. doi: 10.1128/JVI.79.10.6487-6504.2005
Chang, Y., Deng, Y., Li, T., Wang, J., Wang, T., Tan, F., et al. (2020). Visual detection of porcine reproductive and respiratory syndrome virus using CRISPR-Cas 13a. Transbound. Emerg. Dis. 67, 564–571. doi: 10.1111/tbed.13368
Chen, J. S., Ma, E., Harrington, L. B., Da, C. M., Tian, X., Palefsky, J. M., et al. (2018). CRISPR-cas12a target binding unleashes indiscriminate single-stranded DNase activity. Science. 360, 436–439. doi: 10.1126/science.aar6245
Chen, H., Zhang, J., Liu, Y., and Liu, X. (2011). Detection of foot-and-mouth disease virus RNA by reverse transcription loop-mediated isothermal amplification. Virol. J. 8:510. doi: 10.1186/1743-422X-8-510
Corman, V. M., Landt, O., Kaiser, M., Molenkamp, R., Meijer, A., Chu, D. K., et al. (2020). Detection of 2019 novel coronavirus (2019-ncov) by real-time RT-PCR. Euro Surveill. 25:2000045. doi: 10.2807/1560-7917.ES.2020.25.3.2000045
Daher, R. K., Stewart, G., Boissinot, M., and Bergeron, M. G. (2016). Recombinase polymerase amplification for diagnostic applications. Clin. Chem. 62, 947–958. doi: 10.1373/clinchem.2015.245829
Dall, A. A., Otonel, R., Leme, R. A., Alfieri, A. A., and Alfieri, A. F. (2017). A taqman-based qRT-PCR assay for Senecavirus A detection in tissue samples of neonatal piglets. Mol Cell Probes. 33, 28–31. doi: 10.1016/j.mcp.2017.03.002
Dibaba, A. B. (2019). The risk of introduction of swine vesicular disease virus into Kenya via natural sausage casings imported from Italy. Prev. Vet. Med. 169:104703. doi: 10.1016/j.prevetmed.2019.104703
Dill, V., Beer, M., and Hoffmann, B. (2017). Simple, quick and cost-efficient: a universal RT-PCR and sequencing strategy for genomic characterisation of foot-and-mouth disease viruses. J. Virol. Methods. 246, 58–64. doi: 10.1016/j.jviromet.2017.04.007
Erickson, A., Fisher, M., Furukawa-Stoffer, T., Ambagala, A., Hodko, D., Pasick, J., et al. (2018). A multiplex reverse transcription PCR and automated electronic microarray assay for detection and differentiation of seven viruses affecting swine. Transbound. Emerg. Dis. 65, e272–e283. doi: 10.1111/tbed.12749
Fernandes, M., Maggioli, M. F., Joshi, L. R., Clement, T., Faccin, T. C., Rauh, R., et al. (2018). Pathogenicity and cross-reactive immune responses of a historical and a contemporary Senecavirus A strains in pigs. Virology 522, 147–157. doi: 10.1016/j.virol.2018.06.003
Fernandez, J., Aguero, M., Romero, L., Sanchez, C., Belak, S., Arias, M., et al. (2008). Rapid and differential diagnosis of foot-and-mouth disease, swine vesicular disease, and vesicular stomatitis by a new multiplex RT-PCR assay. J. Virol. Methods. 147, 301–311. doi: 10.1016/j.jviromet.2007.09.010
Feronato, C., Leme, R. A., Diniz, J. A., Agnol, A. M. D., Alfieri, A. F., and Alfieri, A. A. (2018). Development and evaluation of a nested-PCR assay for senecavirus a diagnosis. Trop. Anim. Health Pro. 50, 337–344. doi: 10.1007/s11250-017-1436-z
Fischer, C., Torres, M. C., Patel, P., Moreira-Soto, A., Gould, E. A., Charrel, R. N., et al. (2017). Lineage-specific real-time rt-pcr for yellow fever virus outbreak surveillance, Brazil. Emerg. Infect. Dis. 23. doi: 10.3201/eid2311.171131
Fontel, K. S., Botner, A., Belsham, G. J., and Lohse, L. (2019). Diagnostic comparison of serum and EDTA-stabilized blood samples for the detection of foot-and-mouth disease virus RNA by RT-qPCR. J. Virol. Methods. 270, 120–125. doi: 10.1016/j.jviromet.2019.05.003
Fowler, V. L., Ransburgh, R. H., Poulsen, E. G., Wadsworth, J., King, D. P., Mioulet, V., et al. (2017). Development of a novel real-time RT-PCR assay to detect Seneca valley virus-1 associated with emerging cases of vesicular disease in pigs. J. Virol. Methods. 239, 34–37. doi: 10.1016/j.jviromet.2016.10.012
Galluzzi, L., Ceccarelli, M., Diotallevi, A., Menotta, M., and Magnani, M. (2018). Real-time PCR applications for diagnosis of leishmaniasis. Parasite. Vector. 11:273. doi: 10.1186/s13071-018-2859-8
Gimenez-Lirola, L. G., Rademacher, C., Linhares, D., Harmon, K., Rotolo, M., Sun, Y., et al. (2016). Serological and molecular detection of senecavirus a associated with an outbreak of swine idiopathic vesicular disease and neonatal mortality. J. Clin. Microbiol. 54, 2082–2089. doi: 10.1128/JCM.00710-16
Gloster, J., Champion, H. J., Sorensen, J. H., Mikkelsen, T., Ryall, D. B., Astrup, P., et al. (2003). Airborne transmission of foot-and-mouth disease virus from burnside farm, heddon-on-the-wall, northumberland, during the 2001 epidemic in the United Kingdom. Vet. Rec. 152, 525–533. doi: 10.1136/vr.152.17.525
Glushakova, L. G., Alto, B. W., Kim, M. S., Hutter, D., Bradley, A., Bradley, K. M., et al. (2019). Multiplexed kit based on luminex technology and achievements in synthetic biology discriminates zika, chikungunya, and dengue viruses in mosquitoes. Bmc Infect. Dis. 19:418. doi: 10.1186/s12879-019-3998-z
Goller, K. V., Dill, V., Madi, M., Martin, P., Van der Stede, Y., Vandenberge, V., et al. (2018). Rapid and simple detection of foot-and-mouth disease virus: evaluation of a cartridge-based molecular detection system for use in basic laboratories. Transbound. Emerg. Dis. 65, 578–584. doi: 10.1111/tbed.12744
Gootenberg, J. S., Abudayyeh, O. O., Lee, J. W., Essletzbichler, P., Dy, A. J., Joung, J., et al. (2017). Nucleic acid detection with CRISPR-cas13a/c2c2. Science. 356, 438–442. doi: 10.1126/science.aam9321
Graves, J. H. (1973). Serological relationship of swine vesicular disease virus and coxsackie b5 virus. Nature. 245, 314–315. doi: 10.1038/245314a0
Gray, J., and Coupland, L. J. (2014). The increasing application of multiplex nucleic acid detection tests to the diagnosis of syndromic infections. Epidemiol. Infect. 142, 1–11. doi: 10.1017/S0950268813002367
Grubman, M. J., and Baxt, B. (2004). Foot-and-mouth disease. Clin. Microbiol. Rev. 17, 465–493. doi: 10.1128/CMR.17.2.465-493.2004
Hakhverdyan, M., Rasmussen, T. B., Thorén, P., Uttenthal, A., and Belák, S. (2006). Development of a real-time PCR assay based on primer-probe energy transfer for the detection of swine vesicular disease virus. Arch. Virol. 151, 2365–2376. doi: 10.1007/s00705-006-0817-9
Hales, L. M., Knowles, N. J., Reddy, P. S., Xu, L., Hay, C., and Hallenbeck, P. L. (2008). Complete genome sequence analysis of seneca valley virus-001, a novel oncolytic picornavirus. J. Gen. Virol. 89, 1265–1275. doi: 10.1099/vir.0.83570-0
Hole, K., and Nfon, C. (2019). Foot-and-mouth disease virus detection on a handheld real-time polymerase chain reaction platform. Transbound. Emerg. Dis. 66, 1789–1795. doi: 10.1111/tbed.13227
Holland, J. J., Mclaren, L. C., Hoyer, B. H., and Syverton, J. T. (1960). Enteroviral ribonucleic acid. Ii. Biological, physical, and chemical studies. J. Exp. Med. 112, 841–864. doi: 10.1084/jem.112.5.841
Houston, E., Temeeyasen, G., and Pineyro, P. E. (2020). Comprehensive review on immunopathogenesis, diagnostic and epidemiology of senecavirus a. Virus Res. 286:198038. doi: 10.1016/j.virusres.2020.198038
Howson, E., Armson, B., Lyons, N. A., Chepkwony, E., Kasanga, C. J., Kandusi, S., et al. (2018). Direct detection and characterization of foot-and-mouth disease virus in east Africa using a field-ready real-time PCR platform. Transbound. Emerg. Dis. 65, 221–231. doi: 10.1111/tbed.12684
Howson, E., Armson, B., Madi, M., Kasanga, C. J., Kandusi, S., Sallu, R., et al. (2017a). Evaluation of two lyophilized molecular assays to rapidly detect foot-and-mouth disease virus directly from clinical samples in field settings. Transbound. Emerg. Dis. 64, 861–871. doi: 10.1111/tbed.12451
Howson, E., Kurosaki, Y., Yasuda, J., Takahashi, M., Goto, H., Gray, A. R., et al. (2017b). Defining the relative performance of isothermal assays that can be used for rapid and sensitive detection of foot-and-mouth disease virus. J. Virol. Methods. 249, 102–110. doi: 10.1016/j.jviromet.2017.08.013
Huggett, J. F., and Whale, A. (2013). Digital pcr as a novel technology and its potential implications for molecular diagnostics. Clin. Chem. 59, 1691–1693. doi: 10.1373/clinchem.2013.214742
Jones, M., Williams, J., Gartner, K., Phillips, R., Hurst, J., and Frater, J. (2014). Low copy target detection by droplet digital pcr through application of a novel open access bioinformatic pipeline, 'definetherain'. J. Virol. Methods. 202, 46–53. doi: 10.1016/j.jviromet.2014.02.020
Joshi, L. R., Mohr, K. A., Clement, T., Hain, K. S., Myers, B., Yaros, J., et al. (2016). Detection of the emerging picornavirus senecavirus a in pigs, mice, and houseflies. J. Clin. Microbiol. 54, 1536–1545. doi: 10.1128/JCM.03390-15
Kim, D. S., Son, K. Y., Koo, K. M., Kim, J. Y., Alfajaro, M. M., Park, J. G., et al. (2016). Porcine sapelovirus uses alpha 2, 3-linked sialic acid on GD1a ganglioside as a receptor. J. Virol. 90, 4067–4077. doi: 10.1128/JVI.02449-15
Klein, J., Hussain, M., Ahmad, M., Normann, P., Afzal, M., and Alexandersen, S. (2007). Genetic characterisation of the recent foot-and-mouth disease virus subtype A/IRN/2005. Virol. J. 4:122. doi: 10.1186/1743-422X-4-122
Kojabad, A. A., Farzanehpour, M., Galeh, H., Dorostkar, R., Jafarpour, A., Bolandian, M., et al. (2021). Droplet digital pcr of viral DNA/RNA, current progress, challenges, and future perspectives. J. Med. Virol. 93, 4182–4197. doi: 10.1002/jmv.26846
Krunic, N., Merante, F., Yaghoubian, S., Himsworth, D., and Janeczko, R. (2011). Advances in the diagnosis of respiratory tract infections: role of the luminex xtag respiratory viral panel. Ann N Y Acad Sci. 1222, 6–13. doi: 10.1111/j.1749-6632.2011.05964.x
LaBarre, P., Hawkins, K. R., Gerlach, J., Wilmoth, J., Beddoe, A., Singleton, J., et al. (2011). A simple, inexpensive device for nucleic acid amplification without electricity-toward instrument-free molecular diagnostics in low-resource settings. Plos One. 6:e19738. doi: 10.1371/journal.pone.0019738
Laguardia-Nascimento, M., Gasparini, M. R., Sales, E. B., Rivetti, A. J., Sousa, N. M., Oliveira, A. M., et al. (2016). Molecular epidemiology of senecavirus a associated with vesicular disease in pigs in Brazil. Vet. J. 216, 207–209. doi: 10.1016/j.tvjl.2016.08.013
Le, V. P., Lee, K. N., Nguyen, T., Kim, S. M., Cho, I. S., Van Quyen, D., et al. (2011). Development of one-step multiplex RT-PCR method for simultaneous detection and differentiation of foot-and-mouth disease virus serotypes o, a, and Asia 1 circulating in Vietnam. J. Virol. Methods. 175, 101–108. doi: 10.1016/j.jviromet.2011.04.027
Leme, R. A., Alfieri, A. F., and Alfieri, A. A. (2017). Update on senecavirus infection in pigs. Viruses. 9. doi: 10.3390/v9070170
Leme, R. A., Zotti, E., Alcântara, B. K., Oliveira, M. V., Freitas, L. A., Alfieri, A. F., et al. (2015). Senecavirus a: an emerging vesicular infection in Brazilian pig herds. Transbound. Emerg. Dis. 62, 603–611. doi: 10.1111/tbed.12430
Lenhoff, R. J., Naraghi-Arani, P., Thissen, J. B., Olivas, J., Carillo, A. C., Chinn, C., et al. (2008). Multiplexed molecular assay for rapid exclusion of foot-and-mouth disease. J. Virol. Methods. 153, 61–69. doi: 10.1016/j.jviromet.2008.06.015
Lewis-Rogers, N., and Crandall, K. A. (2010). Evolution of Picornaviridae: an examination of phylogenetic relationships and cophylogeny. Mol. Phylogenet. Evol. 54, 995–1005. doi: 10.1016/j.ympev.2009.10.015
Li, H., Bai, R., Zhao, Z., Tao, L., Ma, M., Ji, Z., et al. (2018). Application of droplet digital PCR to detect the pathogens of infectious diseases. Biosci Rep. 38. doi: 10.1042/BSR20181170
Li, J., Liang, W., Xu, S., Shi, J., Zhou, X., Liu, B., et al. (2019). Rapid and sensitive detection of senecavirus a by reverse transcription loop-mediated isothermal amplification combined with a lateral flow dipstick method. Plos One. 14:e216245. doi: 10.1371/journal.pone.0216245
Liao, P., and Huang, Y. (2017). Digital PCR: endless frontier of “divide and conquer”. Micromachines (Basel). 8. doi: 10.3390/mi8080231
Lim, D., Kim, H., Chae, H., Ku, B., Nah, J., Ryoo, S., et al. (2020). Probe-based real-time reverse transcription loop-mediated isothermal amplification (RRT-LAMP) assay for rapid and specific detection of foot-and-mouth disease virus. Transbound. Emerg. Dis. 67, 2936–2945. doi: 10.1111/tbed.13669
Lim, D. R., Kim, H. R., Park, M. J., Chae, H. G., Ku, B. K., Nah, J. J., et al. (2018). An improved reverse transcription loop-mediated isothermal amplification assay for sensitive and specific detection of serotype o foot-and-mouth disease virus. J. Virol. Methods. 260, 6–13. doi: 10.1016/j.jviromet.2018.06.017
Lim, D., Kim, H., Park, M., Chae, H., Ku, B., Nah, J., et al. (2018). A tailored reverse transcription loop-mediated isothermal amplification for sensitive and specific detection of serotype a foot-and-mouth disease virus circulating in pool 1 region countries. Transbound. Emerg. Dis. 65, 1898–1908. doi: 10.1111/tbed.12971
Lim, D., Ryoo, S., Kang, H., Park, H., Hwang, H., Kim, J., et al. (2022). Enhanced detection and serotyping of foot-and-mouth disease virus serotype o, a, and Asia 1 using a novel multiplex real-time RT-PCR. Transbound. Emerg. Dis. doi: 10.1111/tbed.14603
Lin, F., and Kitching, R. P. (2000). Swine vesicular disease: an overview. Vet. J. 160, 192–201. doi: 10.1053/tvjl.2000.0505
Lin, F., Mackay, D. K., and Knowles, N. J. (1997). Detection of swine vesicular disease virus RNA by reverse transcription-polymerase chain reaction. J. Virol. Methods. 65, 111–121. doi: 10.1016/s0166-0934(96)02174-x
Lin, H. T., Okumura, T., Yatsuda, Y., Ito, S., Nakauchi, H., and Otsu, M. (2016). Application of droplet digital pcr for estimating vector copy number states in stem cell gene therapy. Hum Gene Ther Methods. 27, 197–208. doi: 10.1089/hgtb.2016.059
Liu, F., Wang, Q., Huang, Y., Wang, N., and Shan, H. (2020). A 5-year review of senecavirus a in china since its emergence in 2015. Front Vet Sci. 7:567792. doi: 10.3389/fvets.2020.567792
Liu, L., Wang, J., Zhang, R., Lin, M., Shi, R., Han, Q., et al. (2018). Visual and equipment-free reverse transcription recombinase polymerase amplification method for rapid detection of foot-and-mouth disease virus. Bmc Vet. Res. 14:263. doi: 10.1186/s12917-018-1594-x
Liu, Y., Xu, H., Liu, C., Peng, L., Khan, H., Cui, L., et al. (2019). Crispr-cas 13a nanomachine based simple technology for avian influenza a (h7n9) virus on-site detection. J. Biomed. Nanotechnol. 15, 790–798. doi: 10.1166/jbn.2019.2742
Lobato, I. M., and O'Sullivan, C. K. (2018). Recombinase polymerase amplification: basics, applications and recent advances. Trends Analyt Chem. 98, 19–35. doi: 10.1016/j.trac.2017.10.015
Lung, O., Fisher, M., Beeston, A., Hughes, K. B., Clavijo, A., Goolia, M., et al. (2011). Multiplex RT-PCR detection and microarray typing of vesicular disease viruses. J. Virol. Methods. 175, 236–245. doi: 10.1016/j.jviromet.2011.05.023
Madhanmohan, M., Nagendrakumar, S. B., Manikumar, K., Yuvaraj, S., Parida, S., and Srinivasan, V. A. (2013). Development and evaluation of a real-time reverse transcription-loop-mediated isothermal amplification assay for rapid serotyping of foot-and-mouth disease virus. J. Virol. Methods. 187, 195–202. doi: 10.1016/j.jviromet.2012.08.015
Mansour, S. M., Ali, H., Chase, C. C., and Cepica, A. (2015). Loop-mediated isothermal amplification for diagnosis of 18 world organization for animal health (oie) notifiable viral diseases of ruminants, swine and poultry. Anim Health Res Rev. 16, 89–106. doi: 10.1017/S1466252315000018
Marquardt, O., and Ohlinger, V. F. (1995). Differential diagnosis and genetic analysis of the antigenically related swine vesicular disease virus and coxsackie viruses. J. Virol. Methods. 53, 189–199. doi: 10.1016/0166-0934(95)00014-l
McMenamy, M. J., McKillen, J., Reid, S. M., Hjertner, B., King, D. P., Adair, B., et al. (2011). Development of a minor groove binder assay for real-time one-step rt-pcr detection of swine vesicular disease virus. J. Virol. Methods. 171, 219–224. doi: 10.1016/j.jviromet.2010.11.001
Meyer, R. F., Brown, C. C., House, C., House, J. A., and Molitor, T. W. (1991). Rapid and sensitive detection of foot-and-mouth disease virus in tissues by enzymatic rna amplification of the polymerase gene. J. Virol. Methods. 34, 161–172. doi: 10.1016/0166-0934(91)90096-i
Milbury, C. A., Zhong, Q., Lin, J., Williams, M., Olson, J., Link, D. R., et al. (2014). Determining lower limits of detection of digital PCR assays for cancer-related gene mutations. Biomol Detect Quantif. 1, 8–22. doi: 10.1016/j.bdq.2014.08.001
Munro, S. B., Kuypers, J., and Jerome, K. R. (2013). Comparison of a multiplex real-time pcr assay with a multiplex luminex assay for influenza virus detection. J. Clin. Microbiol. 51, 1124–1129. doi: 10.1128/JCM.03113-12
Mur, L., Gallardo, C., Soler, A., Zimmermman, J., Pelayo, V., Nieto, R., et al. (2013). Potential use of oral fluid samples for serological diagnosis of African swine fever. Vet. Microbiol. 165, 135–139. doi: 10.1016/j.vetmic.2012.12.034
Namatovu, A., Wekesa, S. N., Tjornehoj, K., Dhikusooka, M. T., Muwanika, V. B., Siegsmund, H. R., et al. (2013). Laboratory capacity for diagnosis of foot-and-mouth disease in eastern Africa: implications for the progressive control pathway. Bmc Vet. Res. 9:19. doi: 10.1186/1746-6148-9-19
Nardelli, L., Lodetti, E., Gualandi, G. L., Burrows, R., Goodridge, D., Brown, F., et al. (1968). A foot and mouth disease syndrome in pigs caused by an Enterovirus. Nature. 219, 1275–1276. doi: 10.1038/2191275a0
Nicot, F., Cazabat, M., Lhomme, S., Marion, O., Saune, K., Chiabrando, J., et al. (2016). Quantification of HEV RNA by droplet digital PCR. Viruses. 8. doi: 10.3390/v8080233
Nie, X. (2005). Reverse transcription loop-mediated isothermal amplification of DNA for detection of potato virus y. Plant Dis. 89, 605–610. doi: 10.1094/PD-89-0605
Niedbalski, W. (2009). Application of real-time reverse transcription polymerase chain reaction for the detection of SVDV. Pol. J. Vet. Sci. 12, 119–121.
Nielsen, S. C., Bruhn, C. A., Samaniego, J. A., Wadsworth, J., Knowles, N. J., and Gilbert, M. T. (2014). Near-complete genome sequencing of swine vesicular disease virus using the roche gs flx sequencing platform. Plos One. 9:e97180. doi: 10.1371/journal.pone.0097180
Nishi, T., Kanno, T., Shimada, N., Morioka, K., Yamakawa, M., and Fukai, K. (2019). Reverse transcription-PCR using a primer set targeting the 3d region detects foot-and-mouth disease virus with high sensitivity. Transbound. Emerg. Dis. 66, 1776–1783. doi: 10.1111/tbed.13202
Notomi, T., Okayama, H., Masubuchi, H., Yonekawa, T., Watanabe, K., Amino, N., et al. (2000). Loop-mediated isothermal amplification of DNA. Nucleic Acids Res. 28:E63. doi: 10.1093/nar/28.12.e63
Oh, Y., Bae, S. M., Kim, Y. W., Choi, H. S., Nam, G. H., Han, S. J., et al. (2007). Polymerase chain reaction-based fluorescent Luminex assay to detect the presence of human papillomavirus types. Cancer Sci. 98, 549–554. doi: 10.1111/j.1349-7006.2007.00427.x
Palmenberg, A. C., Spiro, D., Kuzmickas, R., Wang, S., Djikeng, A., Rathe, J. A., et al. (2009). Sequencing and analyses of all known human rhinovirus genomes reveal structure and evolution. Science. 324, 55–59. doi: 10.1126/science.1165557
Pasma, T., Davidson, S., and Shaw, S. L. (2008). Idiopathic vesicular disease in swine in Manitoba. Can. Vet. J. 49, 84–85.
Peng, Q., Ning, J., Xu, Q., Yang, T., Wang, Y., Zheng, T., et al. (2021). Development and application of a reverse transcription loop-mediated isothermal amplification combined with lateral flow dipstick for rapid and visual detection of citrus leaf blotch virus in kiwifruit. Crop Prot. 143
Peng, S., Wang, Y., Yang, Z., Yao, X., Hu, L., Chen, P., et al. (2015). A double polymerase chain reaction method for detecting African swine fever and swine vesicular disease virus. Trop. J. Pharm. Res. 14
Persson, S., Karlsson, M., Borsch-Reniers, H., Ellstrom, P., Eriksson, R., and Simonsson, M. (2019). Missing the match might not cost you the game: primer-template mismatches studied in different hepatitis a virus variants. Food Environ. Virol. 11, 297–308. doi: 10.1007/s12560-019-09387-z
Pezzoni, G., Benedetti, D., Bregoli, A., Barbieri, I., Foglia, E. A., Grazioli, S., et al. (2020). Diagnostic performances of different genome amplification assays for the detection of swine vesicular disease virus in relation to genomic lineages that circulated in Italy. Viruses. 12:doi:10.3390/v12111336.
Piepenburg, O., Williams, C. H., Stemple, D. L., and Armes, N. A. (2006). Dna detection using recombination proteins. Plos Biol. 4:e204. doi: 10.1371/journal.pbio.0040204
Pinheiro-de-Oliveira, T. F., Fonseca, A. J., Camargos, M. F., Laguardia-Nascimento, M., de Oliveira, A. M., Cottorello, A., et al. (2018). Development of a droplet digital RT-PCR for the quantification of foot-and-mouth virus RNA. J. Virol. Methods. 259, 129–134. doi: 10.1016/j.jviromet.2018.06.015
Pinheiro-de-Oliveira, T. F., Fonseca-Junior, A. A., Camargos, M. F., Laguardia-Nascimento, M., Giannattasio-Ferraz, S., Cottorello, A., et al. (2019). Reverse transcriptase droplet digital pcr to identify the emerging vesicular virus senecavirus a in biological samples. Transbound. Emerg. Dis. 66, 1360–1369. doi: 10.1111/tbed.13168
Postel, M., Roosen, A., Laurent-Puig, P., Taly, V., and Wang-Renault, S. F. (2018). Droplet-based digital PCR and next generation sequencing for monitoring circulating tumor DNA: a cancer diagnostic perspective. Expert Rev. Mol. Diagn. 18, 7–17. doi: 10.1080/14737159.2018.1400384
Powell, M. L., Bowler, F. R., Martinez, A. J., Greenwood, C. J., Armes, N., and Piepenburg, O. (2018). New FPG probe chemistry for direct detection of recombinase polymerase amplification on lateral flow strips. Anal. Biochem. 543, 108–115. doi: 10.1016/j.ab.2017.12.003
Qian, S., Fan, W., Qian, P., Chen, H., and Li, X. (2016). Isolation and full-genome sequencing of Seneca valley virus in piglets from China, 2016. Virol. J. 13:173. doi: 10.1186/s12985-016-0631-2
Reddy, P. S., Burroughs, K. D., Hales, L. M., Ganesh, S., Jones, B. H., Idamakanti, N., et al. (2007). Seneca valley virus, a systemically deliverable oncolytic picornavirus, and the treatment of neuroendocrine cancers. J Natl Cancer Inst. 99, 1623–1633. doi: 10.1093/jnci/djm198
Reid, S. M., Ferris, N. P., Hutchings, G. H., King, D. P., and Alexandersen, S. (2004a). Evaluation of real-time reverse transcription polymerase chain reaction assays for the detection of swine vesicular disease virus. J. Virol. Methods. 116, 169–176. doi: 10.1016/j.jviromet.2003.11.007
Reid, S. M., Ferris, N. P., Hutchings, G. H., Samuel, A. R., and Knowles, N. J. (2000). Primary diagnosis of foot-and-mouth disease by reverse transcription polymerase chain reaction. J. Virol. Methods. 89, 167–176. doi: 10.1016/s0166-0934(00)00213-5
Reid, S. M., Ferris, N. P., Hutchings, G. H., Zhang, Z., Belsham, G. J., and Alexandersen, S. (2002). Detection of all seven serotypes of foot-and-mouth disease virus by real-time, fluorogenic reverse transcription polymerase chain reaction assay. J. Virol. Methods. 105, 67–80. doi: 10.1016/s0166-0934(02)00081-2
Reid, S. M., Mioulet, V., Knowles, N. J., Shirazi, N., Belsham, G. J., and King, D. P. (2014). Development of tailored real-time rt-pcr assays for the detection and differentiation of serotype o, a and asia-1 foot-and-mouth disease virus lineages circulating in the middle east. J. Virol. Methods. 207, 146–153. doi: 10.1016/j.jviromet.2014.07.002
Reid, S. M., Paton, D. J., Wilsden, G., Hutchings, G. H., King, D. P., Ferris, N. P., et al. (2004b). Use of automated real-time reverse transcription-polymerase chain reaction (rt-pcr) to monitor experimental swine vesicular disease virus infection in pigs. J. Comp. Pathol. 131, 308–317. doi: 10.1016/j.jcpa.2004.05.003
Rodriguez-Habibe, I., Celis-Giraldo, C., Patarroyo, M. E., Avendano, C., and Patarroyo, M. A. (2020). A comprehensive review of the immunological response against foot-and-mouth disease virus infection and its evasion mechanisms. Vaccines (Basel). 8. doi: 10.3390/vaccines8040764
Saeng-Chuto, K., Rodtian, P., Temeeyasen, G., Wegner, M., and Nilubol, D. (2018). The first detection of senecavirus a in pigs in Thailand, 2016. Transbound. Emerg. Dis. 65, 285–288. doi: 10.1111/tbed.12654
Sahore, V., Doonan, S. R., and Bailey, R. C. (2018). Droplet microfluidics in thermoplastics: device fabrication, droplet generation, and content manipulation using integrated electric and magnetic fields. Anal Methods. 10, 4264–4274. doi: 10.1039/C8AY01474D
Senthilkumaran, C., Bittner, H., Ambagala, A., Lung, O., Babiuk, S., Yang, M., et al. (2017). Use of oral fluids for detection of virus and antibodies in pigs infected with swine vesicular disease virus. Transbound. Emerg. Dis. 64, 1762–1770. doi: 10.1111/tbed.12563
Shen, X., Qiu, F., Shen, L., Yan, T., Zhao, M., Qi, J., et al. (2019). A rapid and sensitive recombinase aided amplification assay to detect hepatitis b virus without DNA extraction. Bmc Infect. Dis. 19:229. doi: 10.1186/s12879-019-3814-9
Shi, Y., Li, B., Tao, J., Cheng, J., and Liu, H. (2021). The complex co-infections of multiple porcine diarrhea viruses in local area based on the Luminex xtag multiplex detection method. Front Vet Sci. 8:602866. doi: 10.3389/fvets.2021.602866
Shi, X., Liu, X., Wang, Q., Das, A., Ma, G., Xu, L., et al. (2016). A multiplex real-time pcr panel assay for simultaneous detection and differentiation of 12 common swine viruses. J. Virol. Methods. 236, 258–265. doi: 10.1016/j.jviromet.2016.08.005
Silva, G., Oyekanmi, J., Nkere, C. K., Bomer, M., Kumar, P. L., and Seal, S. E. (2018). Rapid detection of potyviruses from crude plant extracts. Anal. Biochem. 546, 17–22. doi: 10.1016/j.ab.2018.01.019
Singh, K., Corner, S., Clark, S. G., Scherba, G., and Fredrickson, R. (2012). Seneca valley virus and vesicular lesions in a pig with idiopathic vesicular disease. Journal of Veterinary Science & Technology. 3
Sun, D., Vannucci, F., Knutson, T. P., Corzo, C., and Marthaler, D. G. (2017). Emergence and whole-genome sequence of senecavirus a in Colombia. Transbound. Emerg. Dis. 64, 1346–1349. doi: 10.1111/tbed.12669
Tamba, M., Plasmati, F., Brocchi, E., and Ruocco, L. (2020). Eradication of swine vesicular disease in italy. Viruses. 12. doi: 10.3390/v12111269
Tapparel, C., Siegrist, F., Petty, T. J., and Kaiser, L. (2013). Picornavirus and Enterovirus diversity with associated human diseases. Infect. Genet. Evol. 14, 282–293. doi: 10.1016/j.meegid.2012.10.016
Taylor, S. C., Laperriere, G., and Germain, H. (2017). Droplet digital PCR versus qPCR for gene expression analysis with low abundant targets: from variable nonsense to publication quality data. Sci Rep. 7:2409. doi: 10.1038/s41598-017-02217-x
Tiwari, A., Ahmed, W., Oikarinen, S., Sherchan, S. P., Heikinheimo, A., Jiang, G., et al. (2022). Application of digital PCR for public health-related water quality monitoring. Sci. Total Environ. 837:155663. doi: 10.1016/j.scitotenv.2022.155663
Vandenbussche, F., Lefebvre, D. J., De Leeuw, I., Van Borm, S., and De Clercq, K. (2017). Laboratory validation of two real-time rt-pcr methods with 5'-tailed primers for an enhanced detection of foot-and-mouth disease virus. J. Virol. Methods. 246, 90–94. doi: 10.1016/j.jviromet.2017.04.014
Vannucci, F. A., Linhares, D. C. L., Barcellos, D. E. S. N., Lam, H. C., Collins, J., and Marthaler, D. (2015). Identification and complete genome of Seneca valley virus in vesicular fluid and sera of pigs affected with idiopathic vesicular disease, Brazil. Transbound. Emerg. Dis. 62, 589–593. doi: 10.1111/tbed.12410
Vazquez-Calvo, A., Saiz, J. C., Sobrino, F., and Martin-Acebes, M. A. (2016). First complete coding sequence of a Spanish isolate of swine vesicular disease virus. Genome Announc. 4. doi: 10.1128/genomeA.01742-15
Vosloo, W., Bastos, A. D., Sangare, O., Hargreaves, S. K., and Thomson, G. R. (2002). Review of the status and control of foot and mouth disease in sub-Saharan Africa. Rev Sci Tech. 21, 437–449. doi: 10.20506/rst.21.3.1349
Wang, Y., Das, A., Zheng, W., Porter, E., Xu, L., Noll, L., et al. (2020a). Development and evaluation of multiplex real-time rt-pcr assays for the detection and differentiation of foot-and-mouth disease virus and Seneca valley virus 1. Transbound. Emerg. Dis. 67, 604–616. doi: 10.1111/tbed.13373
Wang, H., Dong, J., Zhang, T., Wang, F., Yang, R., Zhang, Y., et al. (2022). A novel rapid detection of senecavirus a using recombinase polymerase amplification (RPA) coupled with lateral flow (LF) dipstrip. Anal. Biochem. 646:114627. doi: 10.1016/j.ab.2022.114627
Wang, H., Hou, P., Zhao, G., Yu, L., Gao, Y., and He, H. (2018). Development and evaluation of serotype-specific recombinase polymerase amplification combined with lateral flow dipstick assays for the diagnosis of foot-and-mouth disease virus serotype a, o and Asia 1. Bmc Vet. Res. 14:359. doi: 10.1186/s12917-018-1644-4
Wang, Y., Yim, I. W., Porter, E., Lu, N., Anderson, J., Noll, L., et al. (2020b). Development of a bead-based assay for detection and differentiation of field strains and four vaccine strains of type 2 porcine reproductive and respiratory syndrome virus (PRRSV-2) in the USA. Transbound. Emerg. Dis. 68, 1414–1423. doi: 10.1111/tbed.13808
Wang, H. M., Zhao, G. M., Hou, P. L., Yu, L., He, C. Q., and He, H. B. (2018). Rapid detection of foot-and-mouth disease virus using reverse transcription recombinase polymerase amplification combined with a lateral flow dipstick. J. Virol. Methods. 261, 46–50. doi: 10.1016/j.jviromet.2018.07.011
Wang, W., Zhou, L., Ge, X., Han, J., Guo, X., Chen, Y., et al. (2021). Development of a vp 2-based real-time fluorescent reverse transcription recombinase-aided amplification assay to rapidly detect senecavirus a. Transbound. Emerg. Dis. doi: 10.1111/tbed.14435
Wiedenheft, B., Sternberg, S. H., and Doudna, J. A. (2012). RNA-guided genetic silencing systems in bacteria and archaea. Nature 482, 331–338. doi: 10.1038/nature10886
Wong, C. L., Yong, C. Y., Ong, H. K., Ho, K. L., and Tan, W. S. (2020). Advances in the diagnosis of foot-and-mouth disease. Front Vet Sci. 7:477. doi: 10.3389/fvets.2020.00477
Wu, X., Lin, H., Chen, S., Xiao, L., Yang, M., An, W., et al. (2017). Development and application of a reverse transcriptase droplet digital pcr (rt-ddpcr) for sensitive and rapid detection of Japanese encephalitis virus. J. Virol. Methods. 248, 166–171. doi: 10.1016/j.jviromet.2017.06.015
Wu, Q., Zhao, X., Bai, Y., Sun, B., Xie, Q., and Ma, J. (2017). The first identification and complete genome of senecavirus a affecting pig with idiopathic vesicular disease in China. Transbound. Emerg. Dis. 64, 1633–1640. doi: 10.1111/tbed.12557
Xu, B., Gong, P., Zhang, Y., Wang, Y., Tao, D., Fu, L., et al. (2022). A one-tube rapid visual crispr assay for the field detection of Japanese encephalitis virus. Virus Res. 319:198869. doi: 10.1016/j.virusres.2022.198869
Xu, W., Hole, K., Goolia, M., Pickering, B., Salo, T., Lung, O., et al. (2017). Genome wide analysis of the evolution of senecavirus a from swine clinical material and assembly yard environmental samples. Plos One. 12:e176964. doi: 10.1371/journal.pone.0176964
Xu, L., Hurtle, W., Rowland, J. M., Casteran, K. A., Bucko, S. M., Grau, F. R., et al. (2013). Development of a universal rt-pcr for amplifying and sequencing the leader and capsid-coding region of foot-and-mouth disease virus. J. Virol. Methods. 189, 70–76. doi: 10.1016/j.jviromet.2013.01.009
Yang, B., Shi, Z., Ma, Y., Wang, L., Cao, L., Luo, J., et al. (2022). Lamp assay coupled with crispr/cas 12a system for portable detection of African swine fever virus. Transbound. Emerg. Dis. 69, e216–e223. doi: 10.1111/tbed.14285
Yeo, S., Yang, M., Nyachoti, M., Rauh, R., Callahan, J. D., and Nfon, C. (2020). Detection of foot-and-mouth disease virus in swine meat juice. Pathogens. 9. doi: 10.3390/pathogens9060424
Yin, L., Man, S., Ye, S., Liu, G., and Ma, L. (2021). CRISPR-Cas based virus detection: recent advances and perspectives. Biosens. Bioelectron. 193:113541. doi: 10.1016/j.bios.2021.113541
Zell, R. (2018). Picornaviridae-the ever-growing virus family. Arch. Virol. 163, 299–317. doi: 10.1007/s00705-017-3614-8
Zeng, F., Cong, F., Liu, X., Lian, Y., Wu, M., Xiao, L., et al. (2018). Development of a real time loop-mediated isothermal amplification method for detection of senecavirus a. J. Virol. Methods. 261, 98–103. doi: 10.1016/j.jviromet.2018.08.005
Zetsche, B., Gootenberg, J. S., Abudayyeh, O. O., Slaymaker, I. M., Makarova, K. S., Essletzbichler, P., et al. (2015). CPF 1 is a single rna-guided endonuclease of a class 2 CRISPR-cas system. Cell. 163, 759–771. doi: 10.1016/j.cell.2015.09.038
Zhang, G., Haydon, D. T., Knowles, N. J., and McCauley, J. W. (1999). Molecular evolution of swine vesicular disease virus. The Journal of general virology. 80, 639–651. doi: 10.1099/0022-1317-80-3-639
Zhang, J., Nfon, C., Tsai, C. F., Lee, C. H., Fredericks, L., Chen, Q., et al. (2019). Development and evaluation of a real-time rt-pcr and a field-deployable rt-insulated isothermal pcr for the detection of Seneca valley virus. Bmc Vet. Res. 15:168. doi: 10.1186/s12917-019-1927-4
Zhang, X., Xiao, J., Ba, L., Wang, F., Gao, D., Zhang, J., et al. (2018). Identification and genomic characterization of the emerging senecavirus a in southeast china, 2017. Transbound. Emerg. Dis. 65, 297–302. doi: 10.1111/tbed.12750
Keywords: FMDV, SVA, SVDV, PCR, isothermal amplification, Luminex, CRISPR-Cas, differential diagnosis
Citation: Chen W, Wang W, Wang X, Li Z, Wu K, Li X, Li Y, Yi L, Zhao M, Ding H, Fan S and Chen J (2022) Advances in the differential molecular diagnosis of vesicular disease pathogens in swine. Front. Microbiol. 13:1019876. doi: 10.3389/fmicb.2022.1019876
Edited by:
Kamal El Bissati, The University of Chicago, United StatesReviewed by:
Shawn Babiuk, National Centre for Foreign Animal Disease (NCFAD), CanadaFangfeng Yuan, University of Illinois at Urbana-Champaign, United States
Copyright © 2022 Chen, Wang, Wang, Li, Wu, Li, Li, Yi, Zhao, Ding, Fan and Chen. This is an open-access article distributed under the terms of the Creative Commons Attribution License (CC BY). The use, distribution or reproduction in other forums is permitted, provided the original author(s) and the copyright owner(s) are credited and that the original publication in this journal is cited, in accordance with accepted academic practice. No use, distribution or reproduction is permitted which does not comply with these terms.
*Correspondence: Shuangqi Fan, c2hxZmFuQHNjYXUuZWR1LmNu; Jinding Chen, amRjaGVuQHNjYXUuZWR1LmNu