- 1State Key Laboratory of Microbial Technology, Marine Biotechnology Research Center, Shandong University, Qingdao, China
- 2College of Marine Life Sciences & Frontiers Science Center for Deep Ocean Multispheres and Earth System, Ocean University of China, Qingdao, China
- 3Laboratory for Marine Biology and Biotechnology, Pilot National Laboratory for Marine Science and Technology, Qingdao, China
Bacterial leaf blight caused by Gram-negative pathogen Xanthomonas oryzae pv. oryzae (Xoo) is one of the most destructive bacterial diseases on rice. Due to the resistance, toxicity and environmental issues of chemical bactericides, new biological strategies are still in need. Although peptaibols produced by Trichoderma spp. can inhibit the growth of several Gram-positive bacteria and plant fungal pathogens, it still remains unclear whether peptaibols have anti-Xoo activity to control bacterial leaf blight on rice. In this study, we evaluated the antibacterial effects of Trichokonins A (TKA), peptaibols produced by Trichoderma longibrachiatum SMF2, against Xoo. The in vitro antibacterial activity analysis showed that the growth of Xoo was significantly inhibited by TKA, with a minimum inhibitory concentration of 54 μg/mL and that the three TKs in TKA all had remarkable anti-Xoo activity. Further inhibitory mechanism analyses revealed that TKA treatments resulted in the damage of Xoo cell morphology and the release of intracellular substances, such as proteins and nucleic acids, from Xoo cells, suggesting the damage of the permeability of Xoo cell membrane by TKA. Pathogenicity analyses showed that the lesion length on rice leaf was significantly reduced by 82.2% when treated with 27 μg/mL TKA. This study represents the first report of the antibacterial activity of peptaibols against a Gram-negative bacterium. Thus, TKA can be of a promising agent in controlling bacterial leaf blight on rice.
Introduction
Rice (Oryza sativa) is one of the most important staple food crops, serving for more than half of the population in the world (Hutin et al., 2016; Laborte et al., 2017). However, its production is severely affected by plant diseases caused by bacteria, fungi, viruses, nematodes and insects. Bacterial leaf blight caused by Xanthomonas oryzae pv. oryzae (Xoo) is one of the most destructive bacterial diseases on rice, which is prevalent in southeast Asia, west Africa, USA and northern Australia, resulting in up to 50% losses of rice production and representing a threat for food security (Niño-Liu et al., 2006; Quibod et al., 2020). Xoo, a rod-shaped Gram-negative bacterium, infects any growth stage of rice through hydathodes or wound sites on leaves and then colonizes in the space of epidermis to utilize the nutritional sources, leading to rice tissue necrosis and wilting (González et al., 2012; Ji et al., 2016; Timilsina et al., 2020).
Various management strategies have been used to minimize the loss of rice production caused by bacterial leaf blight. Chemical bactericides, including thiodiazole copper, thiazole zinc, phenazine-1-carboxamide, niclosamide 1,2,3,4-tetrahydro - β-carboline, S-thiazol-2-yl-furan-2-carbothioate, benzothiadiazole and bismerthiazol, were commonly used to control this disease (Shanmugaiah et al., 2010; Fan et al., 2017; Liang et al., 2018; Sahu et al., 2018; Jiang et al., 2019; Liu et al., 2020). In recent years, nano-technological products were considered as alternative strategies to control this disease, such as ZnO, MgO and MnO2 nanoparticles (Ogunyemi et al., 2020). Moreover, metal nanoparticles biosynthesized with chitosan, Trichoderma spp. or Bacillus cereus SZT1 also exhibited remarkable anti-Xoo activity (Abdallah et al., 2020; Ahmed et al., 2020; Shobha et al., 2020). However, the overuse of chemical bactericides and metal nanoparticles has resulted in environment pollution, increased resistance of pathogens and potential toxin to animals and humans. Therefore, the environmentally friendly and low-toxicity biological strategies have been contemplated as one of the most effective strategies to replace chemical bactericides and metal nanoparticles (Raaijmakers and Mazzola, 2012). To date, some bacterial strains, including Pesudomonas spp., Streptomyces spp. and Paenibacillus polymyxa, have been used as biological control agents to control this disease. Antibiotics produced by these bacterial strains played key roles in controlling this disease, such as phenazine-1-carboxamide from P. aeruginosa MML2212, pyoverdine from P. chlororaphis YL-1, carbazomycin B from S. roseoverticillatus 63 and fusaricidins P from P. polymyxa Sx3 (Shanmugaiah et al., 2010; Abdallah et al., 2019; Liu et al., 2021; Shi et al., 2021). However, effective strategies to control this disease by using biological control fungus is still lacking.
Trichoderma spp. are important fungal biological control agents, frequently living in root, soil, rotten wood and other environments with highly opportunistic potential and adaptability (Druzhinina et al., 2011). Many Trichoderma strains, including T. harzianum, T. atroviride and T. reesei, were effective to control soil-borne diseases caused by plant fungal pathogens (Green et al., 1999; Martinez et al., 2008; Seidl et al., 2009). The antimicrobial secondary metabolites (SMs) from Trichoderma spp., such as anthraquinones, stigmasterol, koninginins, harzianopyridone, pyrone and peptaibols, played key roles in controlling plant fungal diseases (Schirmböck et al., 1994; Vinale et al., 2006; Khan et al., 2020). Peptaibols are linear peptide antibiotics containing 5 to 20 amino acid residues with an acetylated N-terminus, a C-terminal amino alcohol and a high content of α-amino isobutyric acid (Aib) (Wiest et al., 2002). Antimicrobial activity analysis has revealed that peptaibols, including Trichorzianines, Trichorzins, Harzianines, Tricholongins and Trichotoxins, could effectively inhibit the growth of fungi, Gram-positive bacteria, viruses and nematodes (Bertelsen et al., 2007; Tamandegani et al., 2020). However, no peptaibol has been reported to inhibit Gram-negative bacteria till now.
Trichoderma longibrachiatum SMF2 (TlSMF2) has been reported to produce peptaibols designated as Trichokonins (TKs), including Trichokonins A (TKA) with 20 amino acid residues and Trichokonins B (TKB) with 12 amino acid residues (Xiao-Yan et al., 2006; Zhou et al., 2019). Genome sequencing and gene deletion analysis revealed that the two non-ribosomal peptide synthetase (NRPS) encoding genes, tlx1 and tlx2, are responsible for the biosynthesis of TKA and TKB, respectively (Xie et al., 2015; Zhou et al., 2019). TKs displayed broad-spectrum antimicrobial activity against several Gram-positive bacteria and plant fungal pathogens, but not against the analyzed Gram-negative bacteria, including P. aeruginosa, Ralstonia solanacearum, Erwinia carotovora and Escherichia coli (Xiao-Yan et al., 2006). In addition, although TKs could induce the resistance of Chinese cabbage against the infection caused by the Gram-negative bacterium Pectobacterium carotovorum subsp. carotovorum, TKs showed no antibacterial activity against this pathogen in vitro (Li et al., 2014).
In this study, we reported the antibacterial activity of TKA produced by TlSMF2 against the Gram-negative bacterium Xoo. We found that TlSMF2 could significantly inhibit the growth of Xoo, but the tlx1-deletion mutant strain could not. The purified TKA and its three components all showed remarkable anti-Xoo activity. Investigation of the inhibitory mechanism showed that TKA treatments led to the damage of the cell morphology of Xoo and the release of intracellular substances, such as proteins and nucleic acids, suggesting the damage of the permeability of cell membrane by TKA. Moreover, the pathogenicity analysis indicated that TKA had significant effect on controlling bacterial leaf blight caused by Xoo on rice, suggesting that TKA has the potential to be developed as an effective bio-bactericide to control this disease.
Materials and methods
Strains and culture conditions
The strains used in this study were listed in Table 1. Xoo and GFP tagged Xoo were grown on nutrient agar medium (Bacto™ Peptone 5 g/L, Yeast Extract 1 g/L, Sucrose 10 g/L, Beef extract 3 g/L and agar 15 g/L) at 28°C, or in the nutrient broth medium (Qian et al., 2013). The strains of WT, Δtlx1, Δtlx2 and Δtlx1&tlx2 were grown on potato dextrose agar medium (fresh potato 200 g/L, Glucose 20 g/L and 15 g/L) at 28°C, or in the potato dextrose broth medium (Xiao-Yan et al., 2006). The mutants of TlSMF2, Δtlx1, Δtlx2 and Δtlx1&tlx2 were previously constructed (Zhou et al., 2019). The GFP tagged Xoo was previously constructed (Zhang et al., 2019).
Extraction of secondary metabolites produced by TlSMF2 and purification of Trichokonins A and its components
In order to extract the SMs, 0.25 cm2 plate of the mycelium margin of the WT, Δtlx1, Δtlx2 or Δtlx1&tlx2 strain of TlSMF2 was grown on the plate containing 15 mL potato dextrose agar medium for 12 days, and then the potato dextrose agar medium was dipped into 200 mL ethanol for 24 h. The mixture was centrifuged at 10,000 g, and the supernatant was collected and was dried by using freeze-drying. The dried SMs were dissolved with 2 mL methanol, which were used for the analysis of the anti-Xoo activity.
TKA and Trichokonin VI (TK VI), Trichokonin VII (TK VII) and Trichokonin VIII (TK VIII) were purified and identified as previously described (Xiao-Yan et al., 2006; Zhou et al., 2019). Briefly, approximately 2 × 107 spores of the WT strain were inoculated into 100 mL potato dextrose broth medium in a 500 mL flask, which were cultured at 28°C with shaking at 180 rpm for 12 days. Then the potato dextrose broth culture was centrifuged at 10,000 g, and the collected supernatant (40 mL) was loaded on a Cleanert C18 SPE Cartridge, and the TKA was eluted by 2 mL methanol. The eluted TKA was furtuer purified by using HPLC on a reversed phase analytical column (Shimadzu, Japan) that were eluted with methanol/ddH2O (84:16, v/v) at a flow rate of 1.0 mL/min. The chromatogram was monitored at 203 nm. TKs VI, VII and VIII were collected together as purified TKA, or collected separately as purified TKs VI (retention time 17.35 min), VII (retention time 20 min) and VIII (retention time 22.5 min) according to previous identification (Xiao-Yan et al., 2006). The purified TKA and each TKA component (TKs VI, VII or VIII) were dried by using freeze-drying. The purified TKA and each component were dissolved in methanol at a concentration of 10 mg/mL as stock solution. The stock solution was filter-sterilized (0.22 μm) and then used for the analysis of their anti-Xoo activities.
Analysis of the anti-Xoo activity
The anti-Xoo activity of TlSMF2 was analyzed by using the method described previously by Dos et al. with some modification (Dos Santos et al., 2015). Briefly, 0.25 cm2 plate of the mycelium margin of the WT, Δtlx1, Δtlx2 or Δtlx1&tlx2 was transferred to the center of a test plate containing 15 mL nutrient agar medium or to a 250 mL flask containing 50 mL nutrient broth medium, both of which contained Xoo at 1 × 107 CFU/mL. The co-cultures were incubated at 28°C with (for nutrient broth medium) or without (for nutrient agar medium) shaking at 180 rpm for 2 to 6 days. The antagonistic circle around the colony of TlSMF2 or its mutants on the test plate was observed, and the OD600 of the lens cleaning tissue filtered liquid culture was recorded.
The anti-Xoo activities of SMs, TKA and TKs VI, VII and VIII were analyzed using agar well-diffusion assay as described previously with some modification (Nanda and Saravanan, 2009). Briefly, Xoo was cultured in nutrient broth medium to 1 × 107 CFU/mL, and 200 μL suspension of Xoo was spread on nutrient agar medium in a test plate containing 15 mL nutrient agar medium by using a sterile triangular glass coating rod. Then, the wells of 5 mm diameter were loaded on the surface of the test plate and the extracted SMs (20 μL, 40 μL, 60 μL, and 80 μL), 80 μg of TKA and TKs VI, VII and VIII were poured into the wells. The test plate was incubated at 28°C for 3 days, and the diameter of the inhibition zone was recorded. Moreover, 5 × 108 Xoo cells were inoculated into 250 mL flask containing 50 mL nutrient broth medium containing the extracted SMs (0.2%, 0.4%, 0.6%, and 0.8%, v/v), and were incubated with shaking (180 rpm) at 28°C for 48 h, and the OD600 of the culture was recorded every 2 h. Methanol (0.8%, v/v) was used as the negative control. Three replicates were performed in each treatment, and the experiment was repeated three times.
Determination of minimum inhibitory concentration
The MICs of TKA and TKs VI, VII and VIII against Xoo were determined using the method as described previously with some modification (Du et al., 2020). Briefly, 2 × 106 Xoo cells were inoculated into the column of a 96-well plate containing 200 μL nutrient broth medium, and different concentration (0 to 100 μg/mL) of TKA or TKs VI, VII or VIII was added to the 200 μL nutrient broth medium. The 96-well plate was incubated at 28°C for 2 days. The OD600 of the culture was recorded every 2 h using Bioscreen C optical growth analyzer (Bioscreen, Finland) to assess the growth of Xoo. The lowest concentration of TKA or TKs VI, VII or VIII that completely inhibited the growth of Xoo was regarded as the MIC. The results were further confirmed by repeating the experiment in 30 mL bottles containing with 8 × 107 Xoo cells in 8 mL nutrient broth medium with different concentrations (0 to 100 μg/mL) of TKA or TKs VI, VII or VIII at 28°C for 2 days. Methanol (1.2%, v/v) was used as the negative control. Three replicates were performed in each treatment, and the experiment was repeated three times.
Transmission electron microscopy observation of Xoo
The cell morphology of Xoo was observed using transmission electron microscopy as described previously with some modification (Erdmann et al., 2017). Xoo was cultured in nutrient broth medium to 1 × 109 CFU/mL. The cells were collected by centrifugation at 6,000 g for 5 min, and then were washed with sterile ddH2O for three times. The cells were suspended in sterile ddH2O, and were treated with 54 μg/mL TKA at 28°C for 24 h. After treatment, 5 μL cell preparation was adsorbed onto the carbon-coated copper grids for 1.5 min, and then the cells were stained with 2% uranyl acetate for 30 s. Images were pictured by using transmission electron microscopy (JEOL, Japan). Methanol (0.3%, v/v) treatment was used as the negative control. At least 50 Xoo cells were detected in each treatment. Three replicates were performed in each treatment, and the experiment was repeated three times.
Atomic force microscopy observation of Xoo
The cell morphology of Xoo was observed using atomic force microscopy with a previously described method with some modification (Tang et al., 2020). Cell preparations with 1 × 109 CFU/mL were obtained as described in the method of TEM observation. After treated with 54 μg/mL TKA at 28°C for 24 h, 2.5 μL cell preparation was deposited onto freshly cleaved mica and was dried in a chamber at room temperature. Atomic force microscopy was performed using a Multimode VIII AFM with Nanoscope V controller (Bruker AXS, Germany), and images were pictured in the scanasyst mode under air condition. Methanol (0.3%, v/v) treatment was used as the negative control. At least 50 Xoo cells were detected in each treatment. Three replicates were performed in each treatment, and the experiment was repeated three times.
Detection of the release of intracellular substances from Xoo cells treated by Trichokonins A
The method to detect the release of intracellular substances from cells was carried out as previously described with some modification (Liang et al., 2020). Cell preparations with 1 × 109 CFU/mL were obtained as described in the method of TEM observation. After treated with 54 μg/mL TKA at 28°C for 24 h, the OD600 of the culture was recorded, and the supernatant was collected by centrifugation at 8,000 g for 5 min. The concentration of nucleic acids in the supernatant was measured by recording the absorbance of the supernatant at 260 nm using NanoDrop™ One (Thermo Scientific, USA). The supernatant was concentrated (1:20) by using a 3, 000 Da ultrafilter tube (Merck Millipore, USA), and then the concentration of protein in the concentrated supernatant was measured by using Pierce BCA Protein Assay Kit (Thermo Scientific, USA) with bovine serum albumin as the standard.
The GFP tagged Xoo cells were prepared and treated with TKA using the same method as WT Xoo. The concentration of released GFP protein in the supernatant of GFP tagged Xoo cells was detected by Western blot using the method described previously with some modification (Shi et al., 2019). Proteins were separated by SDS-PAGE at 90 V for 30 min, and then at 120 V for 90 min. Proteins in the gel were transferred onto PVDF membrane at 120 mA for 60 min. The PVDF membrane was incubated in the blocking buffer for 1 h, and then in a new blocking buffer containing the primary antibody (GFP-Tag Mouse mAb, 1: 5000, Abmart, China) for 2 h, followed by an incubation in a new blocking buffer containing the secondary antibody (Goat Anti-Rabbit$Mouse IgG-HRP, 1: 5000, Abmart, China) for 1 h. After that, the PVDF membrane was stained with the Super ECL Plus kit and imaged using FluorChem M (Alpha Innotech, USA). Methanol (0.3%, v/v) treatment was used as the negative control. Three replicates were performed in each treatment, and the experiment was repeated three times.
Pathogenicity analysis
The pathogenicity of Xoo on rice was analyzed with the method as described previously with some modification (Zhang et al., 2019). Briefly, the susceptible rice cultivar IR24 was planted in greenhouse under a cycle of light at 28°C for16 h and dark at 25°C for 8 h. The 4 weeks old rice seedlings were dipped into water containing TKA at the concentration of 13.5 μg/mL, 27 μg/mL or 54 μg/mL for 2 days. After that, the rice leaves were inoculated with Xoo cells suspension in sterile distilled water (OD600 = 0.5) by the method of leaf-clipping. Moreover, TKA at the concentration of 13.5 μg/mL, 27 μg/mL or 54 μg/mL also was sprayed to the Xoo-inoculated rice leaves on the 5th and the 10th day. Lesion lengths on the rice leaves were measured after 14 days from the inoculation. Methanol (0.3%, v/v) was used as the negative control. At least 50 rice leaves were inoculated in each treatment. Three replicates were performed in each treatment, and the experiment was repeated three times.
Data analysis
All analysis was conducted by using SPSS 14.0 (SPSS Inc., Chicago, IL, USA). Significant differences were determined via the hypothesis test of percentages (t-test) (** p < 0.01).
Results
TlSMF2 and its secondary metabolites exhibited anti-Xoo bacterial activity
To investigate whether TlSMF2 has anti-Xoo activity, they were co-cultured on nutrient agar medium. An antagonistic circle could be clearly observed around the margin of TlSMF2 after co-cultured for 2 to 6 days (Figure 1A), indicating that TlSMF2 likely produced SMs to inhibit the growth of Xoo. Then, the SMs produced by TlSMF2 were extracted and the anti-Xoo activity of the SMs extract was analyzed. Compared with the negative control of methanol, inhibition zones could be clearly seen on the test plate and their diameter ranged from 2.19 to 2.99 cm when Xoo was treated with the SMs extract from 20 to 80 μL (Figure 1B). Correspondingly, the growth of Xoo in nutrient broth liquid medium was severely inhibited by the addition of 0.2 to 0.6% (v/v) SMs extract, and almost complete inhibited by the addition of 0.8% (v/v) (Figure 1C). These results indicated that one or more SMs produced by TlSMF2 had anti-Xoo activity.
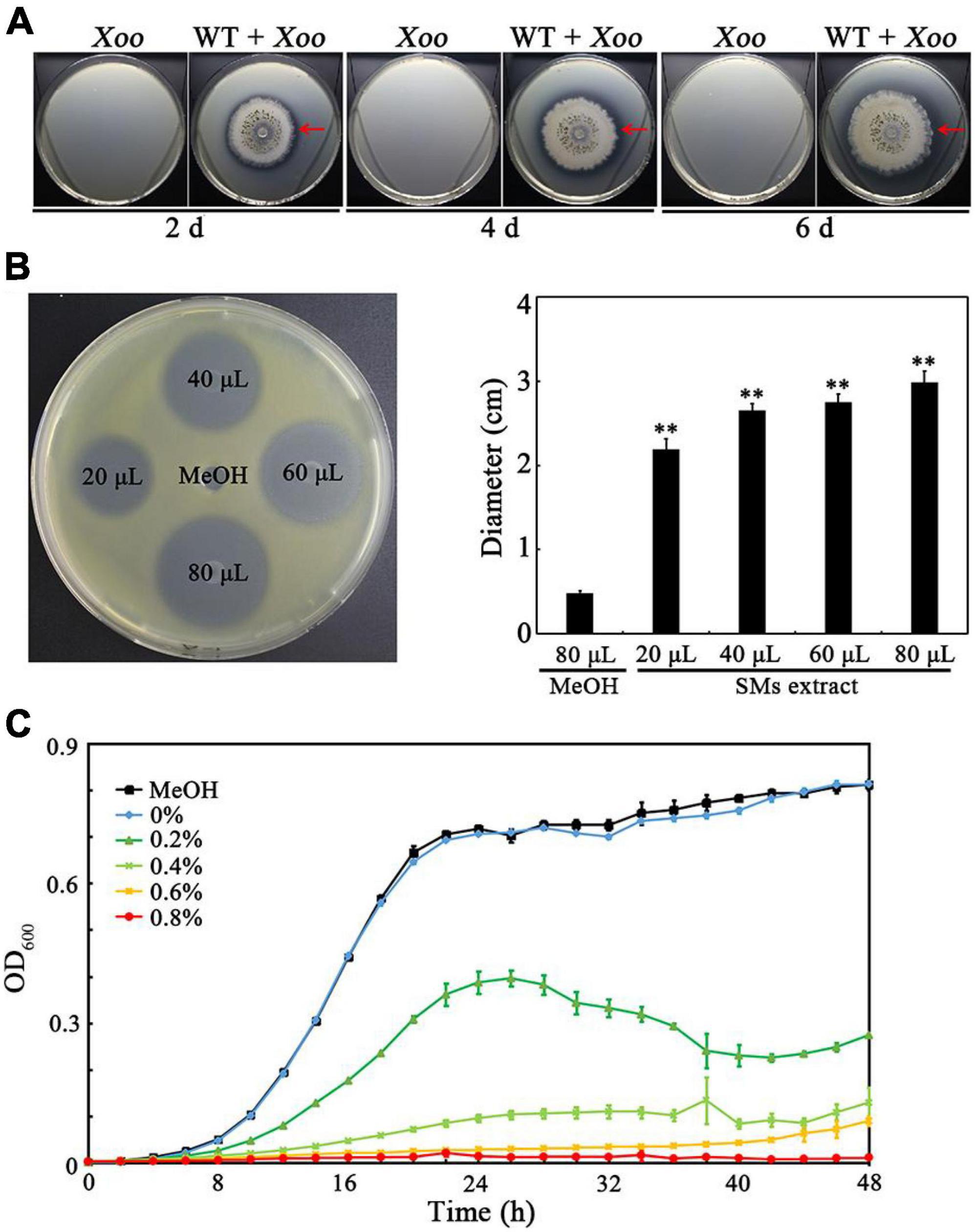
Figure 1. Determination of the anti-Xoo activities of TlSMF2 and its SMs. (A) The representative anti-Xoo activity of TlSMF2 on the test plates containing nutrient agar medium after co-cultured for 2, 4 and 6 days. (B) The representative Xoo-inhibition zones of the SMs extracted from TlSMF2 on the test plate containing nutrient agar medium (left) and the diameters of the zones (right). The diameters were data from three repeats (mean ± S.D.). Asterisk indicates significant difference compared with the control of MeOH (** means P < 0.01). (C) The growth of Xoo in nutrient broth medium containing different volume of the SMs (0%, 0.2%, 0.4%, 0.6%, and 0.8%, v/v) extracted from TlSMF2. MeOH, the nutrient broth medium containing methanol (0.8%, v/v). The graph shows data from triplicate experiments.
The anti-Xoo activity of TlSMF2 was attributed to Trichokonins A
Because TKs have been shown to be a kind of antimicrobial peptides in the SMs produced by TlSMF2 (Xiao-Yan et al., 2006), we speculated that the TKs produced by TlSMF2, TKA and/or TKB, may have anti-Xoo activity. To test this hypothesis, we analyzed the anti-Xoo activities of the wild-type strain of TlSMF2 and its gene-deletion strains Δtlx1, Δtlx2, and Δ tlx1&tlx2 previously constructed (Zhou et al., 2019). On potato dextrose agar plates, the three mutant strains displayed similar growth rate to wild-type TlSMF2 (Figure 2A), suggesting that gene deletion had little impact on the growth of these mutants. On the co-culture plates containing nutrient agar medium, Δtlx2 formed an antagonistic circle with a size similar to that of WT, but Δtlx1 and Δtlx1& tlx2 both formed a negligible one, indicating that Δtlx1 and Δtlx1& tlx2 almost completely lost the anti-Xoo activity, but Δtlx2 still retained this activity (Figure 2B). This was further supported by co-culture in nutrient broth liquid medium. After 48 h co-culture of Xoo with WT or its mutants, the OD600 of the control (containing only Xoo) and the co-cultures of Xoo with Δtlx1 or Δtlx1&tlx2 all reached to approximately 2.5, but those of the co-cultures of Xoo with WT and Δtlx2 were only 0.18 and 0.32, respectively (Figure 2C), indicating that the growth of Xoo was significantly inhibited by WT or Δtlx2, but not by Δtlx1 or Δtlx1&tlx2. Because gene tlx1 encodes TKA and gene tlx2 encodes TKB in TlSMF2 (Zhou et al., 2019), these results suggested that the anti-Xoo activity of TlSMF2 was mainly attributed to the production of TKA in its SMs. This was also supported by analyzing the anti-Xoo activities of the SMs from WT and its mutants in solid and liquid culture. On the test plate containing nutrient agar medium, the SMs from WT and Δtlx2 showed noticeable inhibition zones, but those from Δtlx1 or Δtlx1&tlx2 did not (Figure 2D). In nutrient broth liquid culture, the SMs from WT and Δtlx2 both showed a noticeable inhibitory effect on the growth of Xoo, but those from Δtlx1 or Δtlx1&tlx2 did not (Figure 2E).
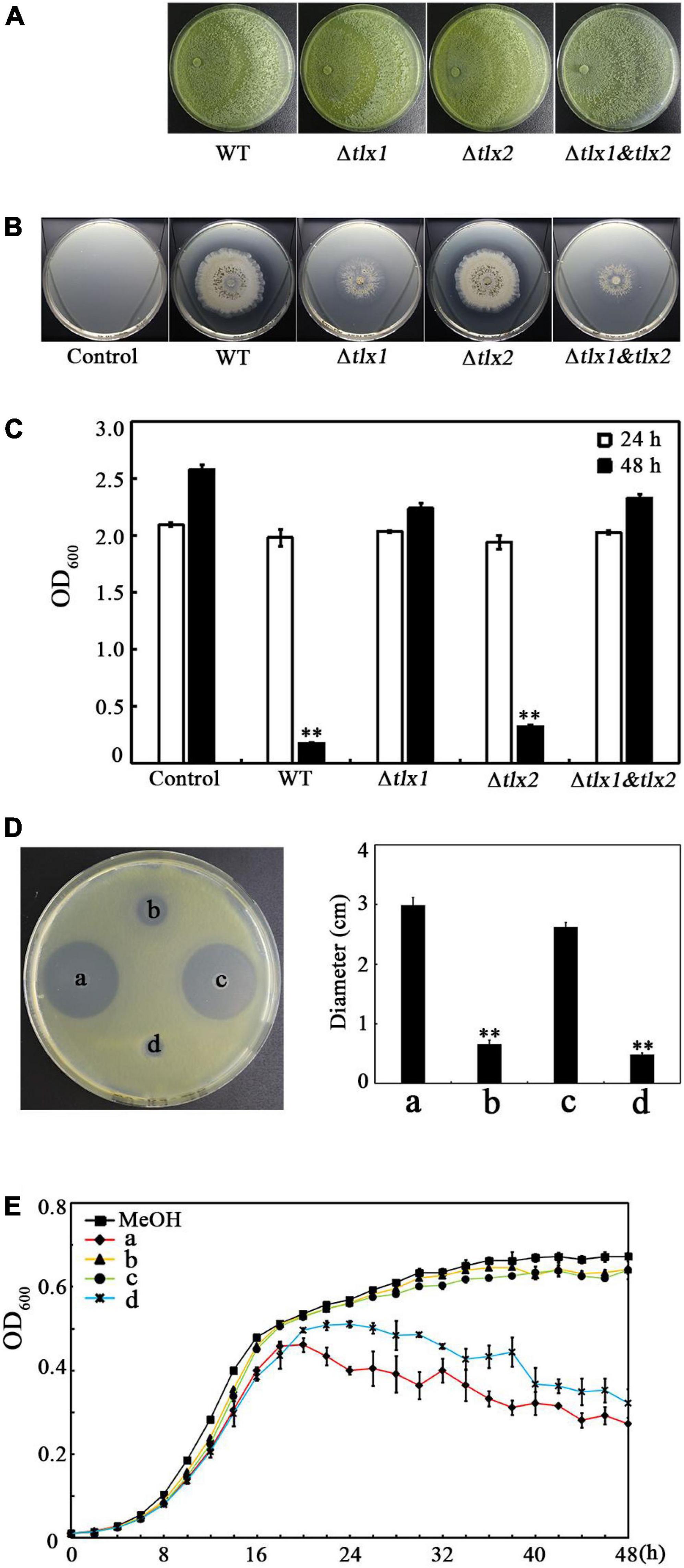
Figure 2. Effect of the gene tlx1 and tlx2 deletion on the anti-Xoo activity of TlSMF2. (A) Growth of the WT TlSMF2 and its mutants on the test plates containing potato dextrose agar medium after cultured for 4 days. The picture shows a representative of three repeats. (B) The representative anti-Xoo activities of the WT TlSMF2 and its mutants on the test plates containing nutrient agar medium after co-cultured for 6 days. (C) The OD600 of the co-cultures with the WT TlSMF2 and its mutants after 24 and 48 h. Control: Xoo cultured without WT TlSMF2 or its mutants. WT, the co-culture of Xoo with WT TlSMF2. Δtlx1, Δtlx2 and Δtlx1&tlx2, the co-cultures of Xoo with the mutant strains Δtlx1, Δtlx2 and Δtlx1& tlx2, respectively. (D) The representative Xoo-inhibition zones of the SMs extracted from WT TlSMF2 and its mutants on the test plate containing nutrient agar medium (left) and the diameters of the zones (right). a, b, c and d represent the SMs produced by strains WT TlSMF2 (a), Δtlx1(b), Δtlx2 (c) and Δtlx1&tlx2 (d), respectively. The diameters were data from three repeats (mean ± S.D.). Asterisk indicates significant difference compared with the control of MeOH (** means P < 0.01). (E) The growth of Xoo in nutrient broth medium containing the SMs (0.8%, v/v) extracted from the WT TlSMF2 and its mutants. MeOH, the nutrient broth medium containing methanol (0.8%, v/v). The graphs show data from triplicate experiments.
To confirm the anti-Xoo activity of TKA, we purified TKA (Figure 3A) and analyzed its anti-Xoo activity. The purified TKA showed remarkable anti-Xoo activity on the test plate (Figure 3B). It has been reported the TKA produced by TlSMF2 contained three TKs, that is, TKs VI, VII and VIII (Xiao-Yan et al., 2006). We then purified the three TKs of TKA separately (Figure 3A) and tested their anti-Xoo activities. As shown in Figure 3B, the three TKs all showed remarkable anti-Xoo activity. To further compare their anti-Xoo activities, we determined the MIC of TKA and TKs VI, VII and VIII against Xoo by monitoring their inhibitory effects on the growth of Xoo under different concentrations. The MIC of TKA against Xoo was 54 μg/mL. Compared with TKA, both TKs VI and VIII showed a stronger anti-Xoo activity, with the MICs at 38 μg/mL and 42 μg/mL, respectively, but the anti-Xoo activity of TK VII was much weaker, with the MIC at 100 μg/mL (Figure 3C). Thus, TK VI had the strongest anti-Xoo activity among the three components of TKA.
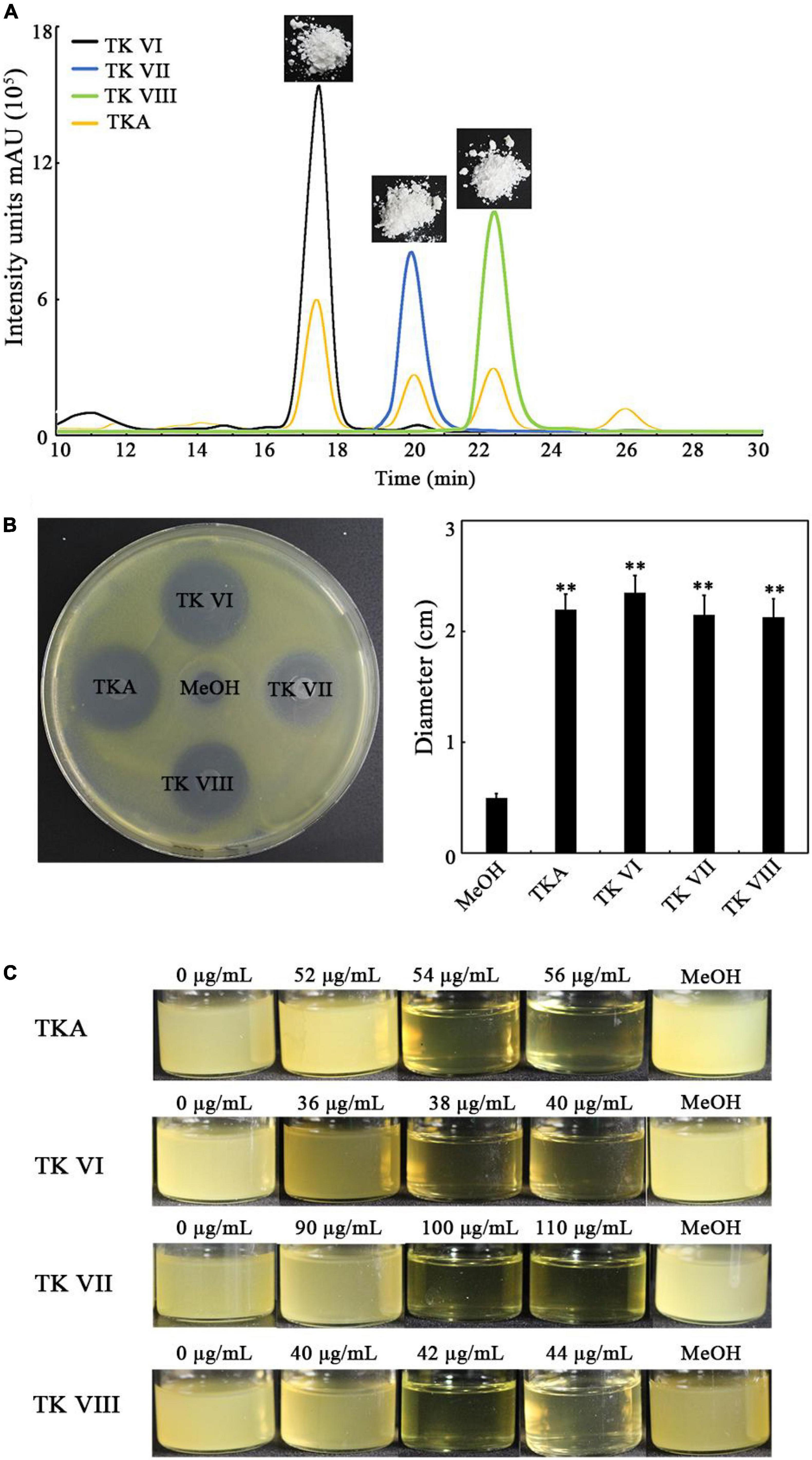
Figure 3. Determination of the anti-Xoo activities of TKA and TKs VI, VII and VIII. (A) The representative purified TKA and TKs VI, VII and VIII detected by using HPLC. (B) The representative Xoo-inhibition zones of 80 μg TKA and TKs VI, VII and VIII on the test plate containing nutrient agar medium (left) and the diameters of the zones (right). The diameters were data from triplicate experiments (mean ± S.D.). Asterisk indicates significant difference compared with the control of MeOH (** means P < 0.01). (C) The growth of Xoo in 8 mL nutrient broth medium containing different concentrations (0 to 110 μg/mL) of TKA or TKs VI, VII or VIII, respectively. MeOH, the nutrient broth medium containing methanol (1.2%, v/v).
Trichokonins A treatment led to the damage of the Xoo cell morphology and the release of intracellular substances
Cell morphology is fundamental for the cellular functions (Fukuda et al., 2021). To investigate whether TKA affects the cell morphology of Xoo, Xoo cells treated with TKA or methanol (as a negative control) was observed by using transmission electron microscopy and atomic force microscopy. The result of transmission electron microscopy observation showed that, after treated with 54 μg/mL TKA for 24 h, 96% Xoo cells displayed distorted and irregular morphology, with separated cell membrane from the cell envelop and intracellular permeated uranyl acetate, suggesting the damage of the cell membrane permeability. In contrast, the cells treated with methanol all displayed intact, normal morphology, with uranyl acetate being attached on the cell surface (Figure 4A). Correspondingly, the atomic force microscopy observation showed that the Xoo cells untreated and those treated with methanol displayed similar intact and smooth surfaces and height, but those treated with 54 μg/mL TKA for 24 h were clearly destroyed, with roughed surfaces and significantly decreased height (Figure 4B). In addition, it seemed that intracellular substances were released from the Xoo cells treated with 54 μg/mL TKA based on the atomic force microscopy observation (Figure 4B).
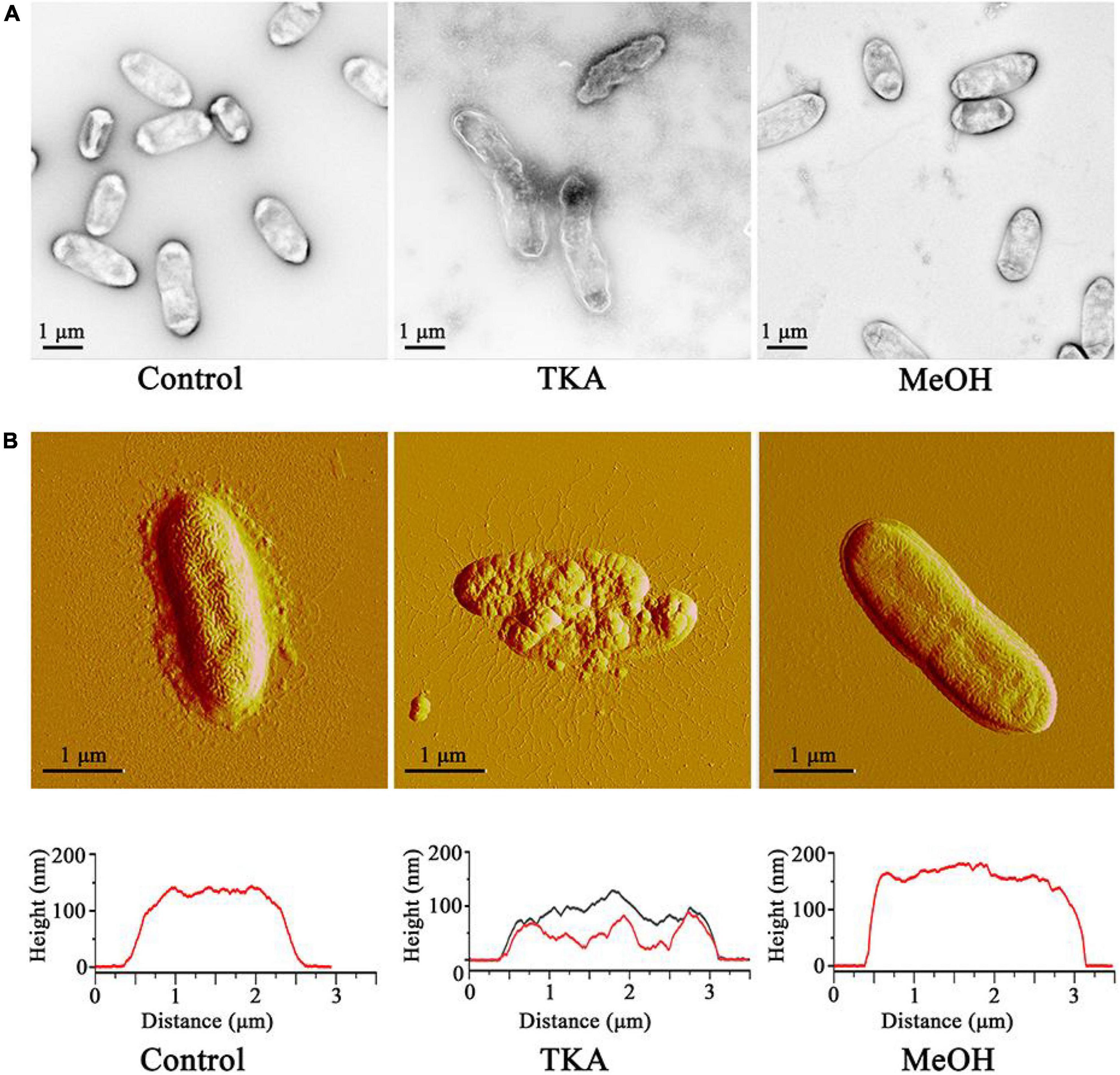
Figure 4. Effect of TKA on the cell morphology of Xoo. (A) Morphology of representative Xoo cells observed by using transmission electron microscopy. (B) Morphology (up) and height (down) of representative Xoo cells observed by using atomic force microscopy. Control, a representative of Xoo cells untreated with methanol or TKA. TKA, two representative of Xoo cells treated with 54 μg/mL TKA for 24 h. MeOH, a representative of Xoo cells treated with methanol (0.3%, v/v) for 24 h. Fifty Xoo cells were observed in each treatment. Each treatment was repeated three times.
To confirm the effects of TKA treatment on the intracellular substances, Xoo cells were treated with 54 μg/mL TKA for 24 h, and then the cell density (OD600) and the concentrations of nucleic acids and proteins in the supernatant were determined and compared to those untreated or treated with methanol. After TKA treatment, the OD600 of the cell suspension reduced 84.4%, but that treated with methanol reduced only 19.2%, similar to that untreated with TKA or methanol (Figure 5A), which indicated the damage of Xoo cells by TKA. The concentration of both nucleic acids and proteins in the supernatant of Xoo cells treated with TKA were significantly higher (approximately 3 folds and 5 folds, respectively) than those in the supernatant of Xoo cells untreated or treated with methanol (Figures 5B,C), suggesting that TKA treatment led to much more release of intracellular nucleic acids and proteins from Xoo cells. To further confirm the effects of TKA, the endogenously expressed GFP protein was used as an indicator to investigate the leakage of intracellular substances. Similar to the case in WT Xoo, TKA treatment led to significant decrease in the cell number of GFP tagged Xoo and significant increase in the release of intracellular nucleic acids and proteins (Figures 5A–C). Moreover, a large concentration of GFP protein was detected by western blot in the supernatant of the GFP tagged Xoo cells treated with TKA for 24 h, but only trace concentration of GFP protein was detected in the supernatant of the GFP tagged Xoo cells untreated or treated with methanol (Figure 5D), indicating the severe leakage of intracellular GFP protein from Xoo cells treated with TKA. Altogether, these results demonstrated that TKA treatment led to the severe release of intracellular substances from Xoo cells, suggesting that the permeability of the cell membrane of Xoo was likely destroyed by TKA.
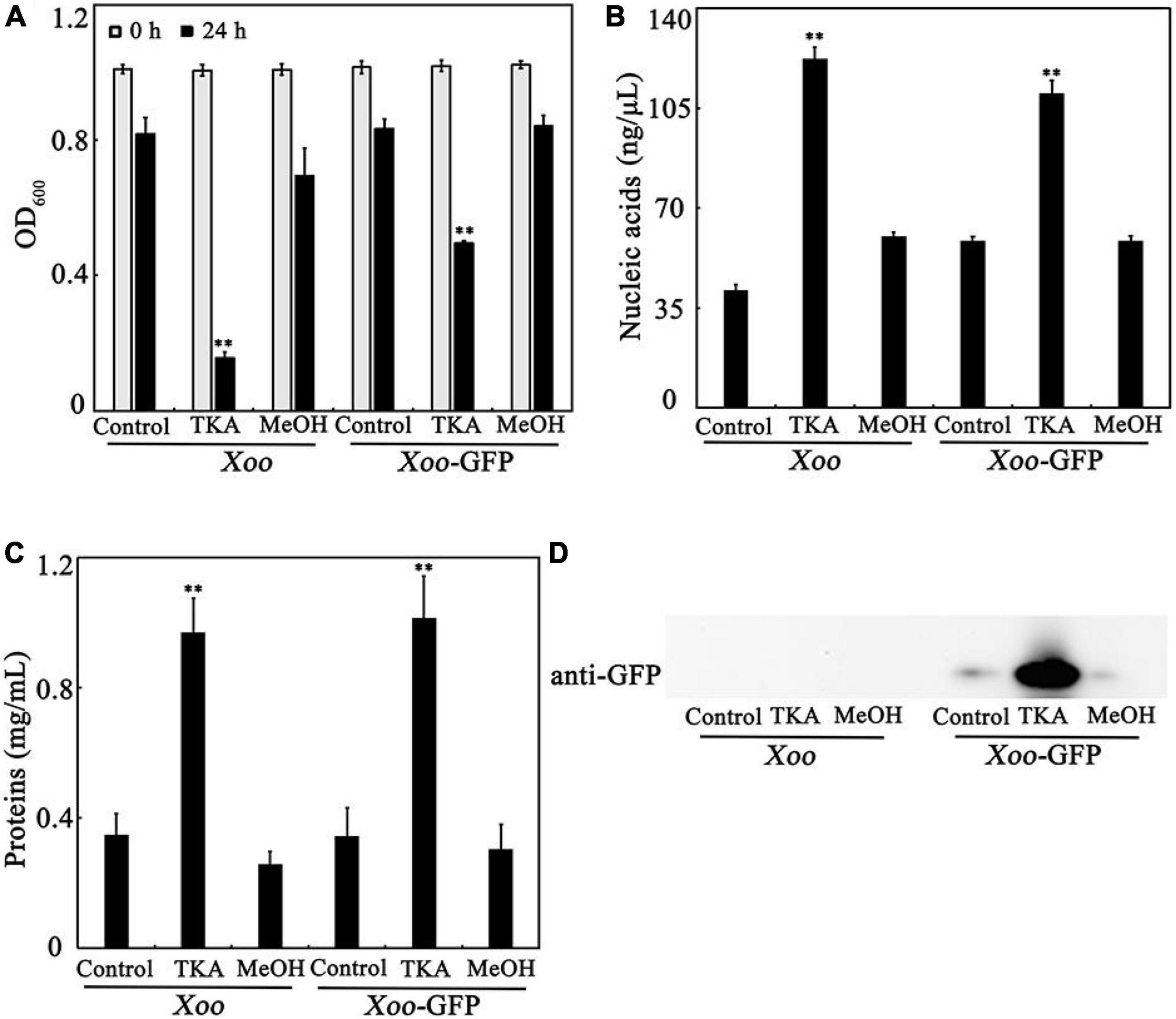
Figure 5. Effect of TKA on the release of intracellular substances from Xoo cells. (A) The OD600 of the cultures of WT Xoo and GFP tagged Xoo (Xoo-GFP) in ddH2O incubated at 28°C with or without TKA for 0 and 24 h. (B) The concentration of nucleic acids in the supernatants of the cultures of WT Xoo and GFP tagged Xoo incubated with or without TKA for 24 h. (C) The concentration of total proteins in the supernatants of the cultures of WT Xoo and GFP tagged Xoo incubated with or without TKA for 24 h. (D) Western blot detection of the GFP protein in the supernatants of the cultures of WT Xoo and GFP tagged Xoo incubated with or without TKA for 24 h. Control, WT Xoo and GFP tagged Xoo incubated without methanol or TKA. TKA, WT Xoo and GFP tagged Xoo incubated with 54 μg/mL TKA. MeOH, WT Xoo and GFP tagged Xoo incubated with methanol (0.3%, v/v). The graphs show data from triplicate experiments (mean ± S.D.). The picture in D is a representative of three repeats. Asterisk indicates significant difference compared with the control (** means P < 0.01).
Trichokonins A reduced the pathogenicity of Xoo on rice
Because TKA showed remarkable anti-Xoo activity, the role of TKA in controlling bacterial leaf blight on rice caused by Xoo was further evaluated by pathogenicity analysis. The lesion length on the leaf of rice untreated with TKA or methanol was approximately 21.2 cm, which was approximately 20.3 cm on that treated with methanol. In contrast, when the rice was treated with 13.5 μg/mL, 27 μg/mL and 54 μg/mL TKA, the lesion length on rice leaf was reduced to approximately 17.2 cm, 3.8 cm and 5.4 cm, respectively. The calculated protective efficiency was approximately 18.8%, 82.2% and 74.3% when the rice was treated with 13.5 μg/mL, 27 μg/mL and 54 μg/mL TKA, respectively (Figure 6). This result showed that TKA could reduce the pathogenicity of Xoo on rice, and that 27 μg/mL TKA had the best protective efficiency.
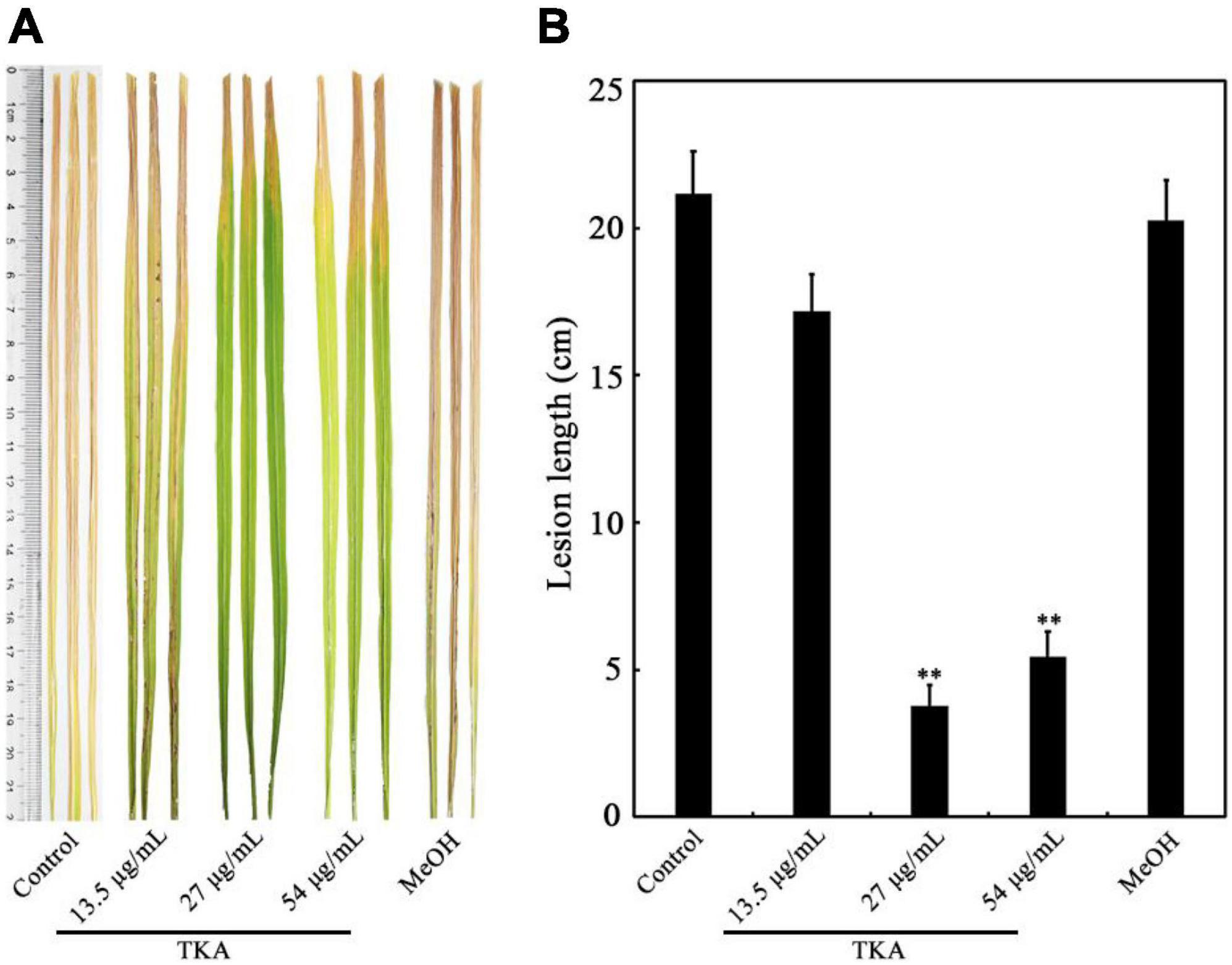
Figure 6. Effect of TKA on the pathogenicity of Xoo on rice. (A) The representative lesion lengths on the leaves of the Xoo-inoculated rice seedlings of cultivar IR24 treated with different concentration of TKA (13.5 μg/mL, 27 μg/mL and 54 μg/mL) in greenhouse for 14 days. At least 50 rice leaves were recorded in each treatment. Each treatment was repeated three times. (B) The lesion lengths on the leaves of the Xoo-inoculated rice seedlings of cultivar IR24 treated with different concentration of TKA (13.5 μg/mL, 27 μg/mL and 54 μg/mL) in greenhouse for 14 days. The graph shows data from triplicate experiments (mean ± S.D.). Asterisk indicates significant difference compared with the control of MeOH (** means P < 0.01). Control, the Xoo-inoculated rice seedling leaves of cultivar IR24 untreated with methanol or TKA. MeOH, the Xoo-inoculated rice seedling leaves of cultivar IR24 treated with methanol (0.3%, v/v).
Discussion
Peptaibols are a class of linear peptides mainly produced by Trichoderma and Emericellopsis (Daniel and Filho, 2007). Studies have shown that peptaibols exhibited broad-spectrum antimicrobial activity against several Gram-positive bacteria and plant fungal pathogens. For example, TKs exhibited broad-spectrum antimicrobial activity against B. subtilis, S. aureus and Fusarium oxysporum (Xiao-Yan et al., 2006). Trichotoxins showed antibacterial activity against B. stearothermophilus (Chutrakul et al., 2008). Emericellipsin A showed significant inhibitory activity against Aspergillus niger ATCC 16404 and Candida albicans ATCC 14053 (Kuvarina et al., 2021). A recent study found that water-soluble trichogin GA IV-derived peptaibols inhibited the growth of plant fungal pathogens, such as Botrytis cinerea, F. graminearum, Penicillium expansum and Pyricularia oryzae (Baccelli et al., 2022). However, there has been no peptaibol being shown to have antibacterial activity against Gram-negative bacteria. In this study, we found that TKA from TlSMF2 showed significant antibacterial activity against the Gram-negative bacterium Xoo. Moreover, the three Trichokonins in TKA, including TKs VI, VII and VIII, all showed remarkable anti-Xoo activity. Notably, the MIC of TK VII against Xoo was much higher than those of TKs VI and VIII, which may be caused by the amino acid difference in their sequences. The 17th amino acid residue is Iva in TK VII, but is Aib in TK VI and VIII. Further study needs to be conducted to decipher this difference. In addition, whether TKA has antibacterial activity against other Gram-negative bacterial pathogens awaits further investigation.
Most peptaibols are membrane-active compounds with the ability to form multimeric ion channels in lipid bilayer membranes (Chugh et al., 2002; Marik et al., 2019). This is considered to be the main antimicrobial mechanism of peptaibols. The pore formation in the membranes eventually results in the leakage of intracellular substances (Milov et al., 2016). For example, emericellipsin A disrupted the cells membrane of Staphylococcus aureus, resulting in the influx of propidium iodide into cells (Rogozhin et al., 2018). In our previous studies, TK VI could induce the autophagy through an influx of Ca2+ to inhibit the growth of HepG2 cancer cells (Shi et al., 2012). Moreover, TK VI could change the cell morphology of plant fungal pathogen F. oxysporum and induce the production of reactive oxygen species to inhibit its growth (Shi et al., 2010). In this study, consistent with the effect of emericellipsin A, we found the application of TKA led to clearly changed cell morphology of Xoo and significant release of intracellular substances from Xoo cells, such as nucleic acids and proteins. Thus, TKA likely adopts the similar antimicrobial strategy against Xoo as other peptaibols against Gram-positive bacteria, fungi and mammal cells, which rupture the integrity of Xoo cell membrane and promotes the cell leakage, thereby leading to the morphology change and the cell death.
In our previous study, we found that TKs from TlSMF2 controlled the tobacco mosaic virus by increasing the production of reactive oxygen species and phenolic compounds, as well as enhancing the expression of pathogenesis-related genes (Luo et al., 2010). Moreover, TKs from TlSMF2 also could enhance the resistance of Chinese cabbage against the infection of pathogen through inducing the production of reactive oxygen species and the expression of pathogenesis-related genes (Li et al., 2014). In addition, Viterbo et al. (2007) found that the application of two synthetic 18-amino-acid peptaibol isoforms (TvBI and TvBII) from T. virens strain Gv29-8 induced the expression of plant resistance related enzymes, including hydroxyperoxide lyase, phenylalanine ammonia lyase and peroxidase, to against the infection of P. syringae pv. lachrymans on cucumber seedlings. Therefore, the induced plant resistance against pathogens also plays an important role in the control of plant diseases by peptaibols. In this study, we found the pathogenicity of Xoo on rice significantly reduced after the application of TKA at the concentration of 27 μg/ml, only half of the MIC of TKA against Xoo. It would be an interesting topic for the future investigation to understand the underlined molecular mechanisms of TKA eliciting resistance response in rice. In recent years, some microbial SMs have been used to against Xoo and to decrease the incidence of bacterial leaf blight on rice. For example, staurosporine produced by Streptomyces sp. MJM4426 inhibited the growth of Xoo with the MIC at 200 μg/mL (Cheng et al., 2016). Difficidin and bacilysin produced by B. amyloliquefaciens FZB42 also exhibited anti-Xoo activity, and the protective rates to bacterial leaf blight reached to 58.82% and 72.31% with the concentration at 50 μg/mL, respectively (Wu et al., 2015). Decyl alcohol and 3,5,5-trimethylhexanol produced by Bacillus strain D13 inhibited the growth of Xoo with the MIC at 480 μg/mL and 2.4 mg/mL, respectively (Xie et al., 2018). In this study, we found the MIC of TKA produced by TlSMF2 against Xoo was 54 μg/mL, and that 82.2% protective rate could be achieved with TKA at the concentration of 27 μg/mL. However, the protective rate decreased to 74.3% at the concentration of 54 μg/mL, suggesting that the excess of TKA may be toxic to rice. The high protective rate indicates that TKA can be of a promising agent in controlling bacterial leaf blight on rice.
Data availability statement
The original contributions presented in this study are included in the article/supplementary material, further inquiries can be directed to the corresponding authors.
Author contributions
X-YS and H-YC: conceptualization. H-YL: extraction of SMs. Kun-Liu: purification of TKs. M-LS: TEM observation. H-NS: AEM observation. Y-QZ: analysis of anti-Xoo activity and pathogenicity and writing – original draft preparation. SZ, X-LC, and Y-ZZ: writing – review and editing. All authors have read and agreed to the published version of the manuscript.
Funding
This work was supported by grants from the National Natural Science Foundation of China (Grant Nos. 31971535 and 31961133016), and the China Postdoctoral Science Foundation (Grant No. 2021M691960).
Conflict of interest
The authors declare that the research was conducted in the absence of any commercial or financial relationships that could be construed as a potential conflict of interest.
Publisher’s note
All claims expressed in this article are solely those of the authors and do not necessarily represent those of their affiliated organizations, or those of the publisher, the editors and the reviewers. Any product that may be evaluated in this article, or claim that may be made by its manufacturer, is not guaranteed or endorsed by the publisher.
References
Abdallah, Y., Liu, M., Ogunyemi, S. O., Ahmed, T., Fouad, H., Abdelazez, A., et al. (2020). Bioinspired Green Synthesis of Chitosan and Zinc Oxide Nanoparticles with Strong Antibacterial Activity against Rice Pathogen Xanthomonas oryzae pv. oryzae. Molecules 25:4795. doi: 10.3390/molecules25204795
Abdallah, Y., Yang, M., Zhang, M., Masum, M., Ogunyemi, S. O., Hossain, A., et al. (2019). Plant growth promotion and suppression of bacterial leaf blight in rice by Paenibacillus polymyxa Sx3. Lett. Appl. Microbiol. 68, 423–429.
Ahmed, T., Shahid, M., Noman, M., Niazi, M., Mahmood, F., Manzoor, I., et al. (2020). Silver Nanoparticles Synthesized by Using Bacillus cereus SZT1 Ameliorated the Damage of Bacterial Leaf Blight Pathogen in Rice. Pathogens 9:160. doi: 10.3390/pathogens9030160
Baccelli, I., Luti, S., Bernardi, R., Favaron, F., De Zotti, M., and Sella, L. (2022). Water-Soluble Trichogin GA IV-Derived peptaibols protect tomato plants from Botrytis cinerea infection with limited impact on plant defenses. Front. Plant Sci. 13:881961. doi: 10.3389/fpls.2022.881961
Bertelsen, K., Pedersen, J. M., Rasmussen, B. S., Skrydstrup, T., Nielsen, N. C., and Vosegaard, T. (2007). Membrane-bound conformation of peptaibols with methyl-deuterated alpha-amino isobutyric acids by 2H magic angle spinning solid-state NMR spectroscopy. J. Am. Chem. Soc. 129, 14717–14723. doi: 10.1021/ja0749690
Cheng, J., Park, S. B., Kim, S. H., Yang, S. H., Suh, J. W., Lee, C. H., et al. (2016). Suppressing activity of staurosporine from Streptomyces sp. MJM4426 against rice bacterial blight disease. J. Appl. Microbiol. 120, 975–985. doi: 10.1111/jam.13034
Chugh, J. K., Brückner, H., and Wallace, B. A. (2002). Model for a helical bundle channel based on the high-resolution crystal structure of trichotoxin_A50E. Biochemistry 41, 12934–12941. doi: 10.1021/bi026150z
Chutrakul, C., Alcocer, M., Bailey, K., and Peberdy, J. F. (2008). The production and characterisation of trichotoxin peptaibols, by Trichoderma asperellum. Chem. Biodivers. 5, 1694–1706. doi: 10.1002/cbdv.200890158
Daniel, J. F., and Filho, E. R. (2007). Peptaibols of trichoderma. Nat. Prod. Rep. 24, 1128–1141. doi: 10.1039/b618086h
Dos Santos, I. P., da Silva, L. C., da Silva, M. V., de Araújo, J. M., Cavalcanti, M., and Lima, V. L. (2015). Antibacterial activity of endophytic fungi from leaves of Indigofera suffruticosa Miller (Fabaceae). Front. Microbiol. 6:350. doi: 10.3389/fmicb.2015.00350
Druzhinina, I. S., Seidl-Seiboth, V., Herrera-Estrella, A., Horwitz, B. A., Kenerley, C. M., and Monte, E. (2011). Trichoderma: The genomics of opportunistic success. Nat. Rev. Microbiol. 9, 749–759. doi: 10.1038/nrmicro2637
Du, F. Y., Li, X. M., Sun, Z. C., Meng, L. H., and Wang, B. G. (2020). Secondary metabolites with agricultural antagonistic potentials from Beauveria felina, a marine-derived entomopathogenic Fungus. J. Agric. Food Chem. 68, 14824–14831. doi: 10.1021/acs.jafc.0c05696
Erdmann, S., Tschitschko, B., Zhong, L., Raftery, M. J., and Cavicchioli, R. (2017). A plasmid from an Antarctic haloarchaeon uses specialized membrane vesicles to disseminate and infect plasmid-free cells. Nat. Microbiol. 2, 1446–1455. doi: 10.1038/s41564-017-0009-2
Fan, S., Tian, F., Li, J., Hutchins, W., Chen, H., Yang, F., et al. (2017). Identification of phenolic compounds that suppress the virulence of Xanthomonas oryzae on rice via the type III secretion system. Mol. Plant Pathol. 18, 555–568. doi: 10.1111/mpp.12415
Fukuda, S., Yamamoto, R., Yanagisawa, N., Takaya, N., Sato, Y., Riquelme, M., et al. (2021). Trade-off between plasticity and velocity in mycelial growth. mBio 12:e3196–e3120. doi: 10.1128/mBio.03196-20
González, J. F., Degrassi, G., Devescovi, G., De Vleesschauwer, D., Höfte, M., Myers, M. P., et al. (2012). A proteomic study of Xanthomonas oryzae pv. oryzae in rice xylem sap. J. Proteomics 75, 5911–5919. doi: 10.1016/j.jprot.2012.07.019
Green, H., Larsen, J., Olsson, P. A., Jensen, D. F., and Jakobsen, I. I. (1999). Suppression of the biocontrol agent Trichoderma harzianum by mycelium of the arbuscular mycorrhizal fungus glomus intraradices in root-free soil. Appl. Environ. Microbiol. 65, 1428–1434. doi: 10.1128/AEM.65.4.1428-1434.1999
Hutin, M., Césari, S., Chalvon, V., Michel, C., Tran, T. T., Boch, J., et al. (2016). Ectopic activation of the rice NLR heteropair RGA4/RGA5 confers resistance to bacterial blight and bacterial leaf streak diseases. Plant J. 88, 43–55. doi: 10.1111/tpj.13231
Ji, Z., Ji, C., Liu, B., Zou, L., Chen, G., and Yang, B. (2016). Interfering TAL effectors of Xanthomonas oryzae neutralize R-gene-mediated plant disease resistance. Nat. Commun. 7:13435. doi: 10.1038/ncomms13435
Jiang, S., He, M., Xiang, X. W., Adnan, M., and Cui, Z. N. (2019). Novel S-Thiazol-2-yl-furan-2-carbothioate derivatives as potential T3SS inhibitors against Xanthomonas oryzae on rice. J. Agric. Food Chem. 67, 11867–11876. doi: 10.1021/acs.jafc.9b04085
Khan, R., Najeeb, S., Hussain, S., Xie, B., and Li, Y. (2020). Bioactive secondary metabolites from Trichoderma spp. against phytopathogenic Fungi. Microorganisms 8:817. doi: 10.3390/microorganisms8060817
Kuvarina, A. E., Gavryushina, I. A., Kulko, A. B., Ivanov, I. A., Rogozhin, E. A., Georgieva, M. L., et al. (2021). The Emericellipsins A-E from an alkalophilic fungus Emericellopsis alkalina show potent activity against multidrug-resistant pathogenic fungi. J. Fungi 7:153. doi: 10.3390/jof7020153
Laborte, A. G., Gutierrez, M. A., Balanza, J. G., Saito, K., Zwart, S. J., Boschetti, M., et al. (2017). RiceAtlas, a spatial database of global rice calendars and production. Sci. Data 4:170074. doi: 10.1038/sdata.2017.74
Li, H. Y., Luo, Y., Zhang, X. S., Shi, W. L., Gong, Z. T., Shi, M., et al. (2014). Trichokonins from Trichoderma pseudokoningii SMF2 induce resistance against Gram-negative Pectobacterium carotovorum subsp. carotovorum in Chinese cabbage. FEMS Microbiol. Lett. 354, 75–82. doi: 10.1111/1574-6968.12427
Liang, H., He, K., Li, T., Cui, S., Tang, M., Kang, S., et al. (2020). Mechanism and antibacterial activity of vine tea extract and dihydromyricetin against Staphylococcus aureus. Sci. Rep. 10:21416. doi: 10.1038/s41598-020-78379-y
Liang, X., Yu, X., Pan, X., Wu, J., Duan, Y., Wang, J., et al. (2018). A thiadiazole reduces the virulence of Xanthomonas oryzae pv. oryzae by inhibiting the histidine utilization pathway and quorum sensing. Mol. Plant Pathol. 19, 116–128. doi: 10.1111/mpp.12503
Liu, H. W., Ji, Q. T., Ren, G. G., Wang, F., Su, F., Wang, P. Y., et al. (2020). Antibacterial functions and proposed modes of action of novel 1,2,3,4-Tetrahydro-β-carboline derivatives that possess an attractive 1,3-Diaminopropan-2-ol pattern against rice bacterial blight, kiwifruit bacterial canker, and citrus bacterial canker. J. Agric. Food Chem. 68, 12558–12568. doi: 10.1021/acs.jafc.0c02528
Liu, Y., Dai, C., Zhou, Y., Qiao, J., Tang, B., Yu, W., et al. (2021). Pyoverdines are essential for the antibacterial activity of Pseudomonas chlororaphis YL-1 under low-iron conditions. Appl. Environ. Microbiol. 87:e2840–e2820. doi: 10.1128/AEM.02840-20
Luo, Y., Zhang, D. D., Dong, X. W., Zhao, P. B., Chen, L. L., Song, X. Y., et al. (2010). Antimicrobial peptaibols induce defense responses and systemic resistance in tobacco against tobacco mosaic virus. FEMS Microbiol. Lett. 313, 120–126. doi: 10.1111/j.1574-6968.2010.02135.x
Marik, T., Tyagi, C., Balázs, D., Urbán, P., Szepesi, Á, Bakacsy, L., et al. (2019). Structural diversity and bioactivities of peptaibol compounds from the longibrachiatum clade of the filamentous fungal genus Trichoderma. Front. Microbiol. 10:1434. doi: 10.3389/fmicb.2019.01434
Martinez, D., Berka, R. M., Henrissat, B., Saloheimo, M., Arvas, M., Baker, S. E., et al. (2008). Genome sequencing and analysis of the biomass-degrading fungus Trichoderma reesei (syn. Hypocrea jecorina). Nat. Biotechnol. 26, 553–560. doi: 10.1038/nbt1403
Milov, A. D., Tsvetkov, Y. D., Raap, J., De Zotti, M., Formaggio, F., and Toniolo, C. (2016). Conformation, self-aggregation, and membrane interaction of peptaibols as studied by pulsed electron double resonance spectroscopy. Biopolymers 106, 6–24. doi: 10.1002/bip.22713
Nanda, A., and Saravanan, M. (2009). Biosynthesis of silver nanoparticles from Staphylococcus aureus and its antimicrobial activity against MRSA and MRSE. Nanomedicine 5, 452–456. doi: 10.1016/j.nano.2009.01.012
Niño-Liu, D. O., Ronald, P. C., and Bogdanove, A. J. (2006). Xanthomonas oryzae pathovars: Model pathogens of a model crop. Mol. Plant Pathol. 7, 303–324. doi: 10.1111/j.1364-3703.2006.00344.x
Ogunyemi, S. O., Zhang, M., Abdallah, Y., Ahmed, T., Qiu, W., Ali, M. A., et al. (2020). The bio-synthesis of three metal oxide nanoparticles (ZnO, MnO2 and MgO) and their antibacterial activity against the bacterial leaf blight pathogen. Front. Microbiol. 11:588326. doi: 10.3389/fmicb.2020.588326
Qian, G., Liu, C., Wu, G., Yin, F., Zhao, Y., Zhou, Y., et al. (2013). AsnB, regulated by diffusible signal factor and global regulator Clp, is involved in aspartate metabolism, resistance to oxidative stress and virulence in Xanthomonas oryzae pv. oryzicola. Mol. Plant Pathol. 14, 145–157. doi: 10.1111/j.1364-3703.2012.00838.x
Quibod, I. L., Atieza-Grande, G., Oreiro, E. G., Palmos, D., Nguyen, M. H., Coronejo, S. T., et al. (2020). The green revolution shaped the population structure of the rice pathogen Xanthomonas oryzae pv. oryzae. ISME J. 14, 492–505. doi: 10.1038/s41396-019-0545-2
Raaijmakers, J. M., and Mazzola, M. (2012). Diversity and natural functions of antibiotics produced by beneficial and plant pathogenic bacteria. Annu. Rev. Phytopathol. 50, 403–424. doi: 10.1146/annurev-phyto-081211-172908
Rogozhin, E. A., Sadykova, V. S., Baranova, A. A., Vasilchenko, A. S., Lushpa, V. A., Mineev, K. S., et al. (2018). A novel lipopeptaibol emericellipsin A with antimicrobial and antitumor activity produced by the extremophilic fungus Emericellopsis alkalina. Molecules 23:2785. doi: 10.3390/molecules23112785
Sahu, S. K., Zheng, P., and Yao, N. (2018). Niclosamide blocks rice leaf blight by inhibiting biofilm formation of Xanthomonas oryzae. Front. Plant Sci. 9:408. doi: 10.3389/fpls.2018.00408
Salzberg, S. L., Sommer, D. D., Schatz, M. C., Phillippy, A. M., Rabinowicz, P. D., Tsuge, S., et al. (2008). Genome sequence and rapid evolution of the rice pathogen Xanthomonas oryzae pv. oryzae PXO99A. BMC Genomics 9:204. doi: 10.1186/1471-2164-9-204
Schirmböck, M., Lorito, M., Wang, Y. L., Hayes, C. K., Arisan-Atac, I., Scala, F., et al. (1994). Parallel formation and synergism of hydrolytic enzymes and peptaibol antibiotics, molecular mechanisms involved in the antagonistic action of Trichoderma harzianum against phytopathogenic fungi. Appl. Environ. Microbiol. 60, 4364–4370. doi: 10.1128/aem.60.12.4364-4370.1994
Seidl, V., Song, L., Lindquist, E., Gruber, S., Koptchinskiy, A., Zeilinger, S., et al. (2009). Transcriptomic response of the mycoparasitic fungus Trichoderma atroviride to the presence of a fungal prey. BMC Genomics 10:567. doi: 10.1186/1471-2164-10-567
Shanmugaiah, V., Mathivanan, N., and Varghese, B. (2010). Purification, crystal structure and antimicrobial activity of phenazine-1-carboxamide produced by a growth-promoting biocontrol bacterium, Pseudomonas aeruginosa MML2212. J. Appl. Microbiol. 108, 703–711. doi: 10.1111/j.1365-2672.2009.04466.x
Shi, D., Zhao, S., Jiang, W., Zhang, C., Liang, T., and Hou, G. (2019). TLR5: A prognostic and monitoring indicator for triple-negative breast cancer. Cell Death Dis. 10:954. doi: 10.1038/s41419-019-2187-8
Shi, M., Chen, L., Wang, X. W., Zhang, T., Zhao, P. B., Song, X. Y., et al. (2012). Antimicrobial peptaibols from Trichoderma pseudokoningii induce programmed cell death in plant fungal pathogens. Microbiology 158, 166–175. doi: 10.1099/mic.0.052670-0
Shi, M., Wang, H. N., Xie, S. T., Luo, Y., Sun, C. Y., Chen, X. L., et al. (2010). Antimicrobial peptaibols, novel suppressors of tumor cells, targeted calcium-mediated apoptosis and autophagy in human hepatocellular carcinoma cells. Mol. Cancer 9:26. doi: 10.1186/1476-4598-9-26
Shi, T., Guo, X., Zhu, J., Hu, L., He, Z., and Jiang, D. (2021). Inhibitory effects of Carbazomycin B produced by Streptomyces roseoverticillatus 63 against Xanthomonas oryzae pv. oryzae. Front. Microbiol. 12:616937. doi: 10.3389/fmicb.2021.616937
Shobha, B., Lakshmeesha, T. R., Ansari, M. A., Almatroudi, A., Alzohairy, M. A., Basavaraju, S., et al. (2020). Mycosynthesis of ZnO nanoparticles using Trichoderma spp. isolation from rhizosphere soils and its synergistic antibacterial effect against Xanthomonas oryzae pv. oryzae. J. Fungi 6:181. doi: 10.3390/jof6030181
Tamandegani, P. R., Marik, T., Zafari, D., Balázs, D., Vágvölgyi, C., Szekeres, A., et al. (2020). Changes in peptaibol production of Trichoderma species during in vitro antagonistic interactions with fungal plant pathogens. Biomolecules 10:730. doi: 10.3390/biom10050730
Tang, B. L., Yang, J., Chen, X. L., Wang, P., Zhao, H. L., Su, H. N., et al. (2020). A predator-prey interaction between a marine Pseudoalteromonas sp. and Gram-positive bacteria. Nat. Commun. 11:285. doi: 10.1038/s41467-019-14133-x
Timilsina, S., Potnis, N., Newberry, E. A., Liyanapathiranage, P., Iruegas-Bocardo, F., White, F. F., et al. (2020). Xanthomonas diversity, virulence and plant-pathogen interactions. Nat. Rev. Microbiol. 18, 415–427. doi: 10.1038/s41579-020-0361-8
Vinale, F., Marra, R., Scala, F., Ghisalberti, E. L., Lorito, M., and Sivasithamparam, K. (2006). Major secondary metabolites produced by two commercial Trichoderma strains active against different phytopathogens. Lett. Appl. Microbiol. 43, 143–148. doi: 10.1111/j.1472-765X.2006.01939.x
Viterbo, A., Wiest, A., Brotman, Y., Chet, I., and Kenerley, C. (2007). The 18mer peptaibols from Trichoderma virens elicit plant defence responses. Mol. Plant Pathol. 8, 737–746. doi: 10.1111/j.1364-3703.2007.00430.x
Wiest, A., Grzegorski, D., Xu, B. W., Goulard, C., Rebuffat, S., Ebbole, D. J., et al. (2002). Identification of peptaibols from Trichoderma virens and cloning of a peptaibol synthetase. J. Biol. Chem. 277, 20862–20868. doi: 10.1074/jbc.M201654200
Wu, L., Wu, H., Chen, L., Yu, X., Borriss, R., and Gao, X. (2015). Difficidin and bacilysin from Bacillus amyloliquefaciens FZB42 have antibacterial activity against Xanthomonas oryzae rice pathogens. Sci. Rep. 5:12975. doi: 10.1038/srep12975
Xiao-Yan, S., Qing-Tao, S., Shu-Tao, X., Xiu-Lan, C., Cai-Yun, S., and Yu-Zhong, Z. (2006). Broad-spectrum antimicrobial activity and high stability of Trichokonins from Trichoderma koningii SMF2 against plant pathogens. FEMS Microbiol. Lett. 260, 119–125. doi: 10.1111/j.1574-6968.2006.00316.x
Xie, B. B., Li, D., Shi, W. L., Qin, Q. L., Wang, X. W., Rong, J. C., et al. (2015). Deep RNA sequencing reveals a high frequency of alternative splicing events in the fungus Trichoderma longibrachiatum. BMC Genomics 16:54. doi: 10.1186/s12864-015-1251-8
Xie, S., Zang, H., Wu, H., Uddin Rajer, F., and Gao, X. (2018). Antibacterial effects of volatiles produced by Bacillus strain D13 against Xanthomonas oryzae pv. oryzae. Mol. Plant Pathol. 19, 49–58. doi: 10.1111/mpp.12494
Zhang, Y., Wu, G., Palmer, I., Wang, B., Qian, G., Fu, Z. Q., et al. (2019). The role of a host-induced Arginase of Xanthomonas oryzae pv. oryzae in promoting virulence on rice. Phytopathology 109, 1869–1877. doi: 10.1094/PHYTO-02-19-0058-R
Keywords: Trichoderma longibrachiatum SMF2, Trichokonins A, Xanthomonas oryzae pv. oryzae, biological control, bacterial leaf blight (BLB)
Citation: Zhang Y-Q, Zhang S, Sun M-L, Su H-N, Li H-Y, Kun-Liu, Zhang Y-Z, Chen X-L, Cao H-Y and Song X-Y (2022) Antibacterial activity of peptaibols from Trichoderma longibrachiatum SMF2 against gram-negative Xanthomonas oryzae pv. oryzae, the causal agent of bacterial leaf blight on rice. Front. Microbiol. 13:1034779. doi: 10.3389/fmicb.2022.1034779
Received: 02 September 2022; Accepted: 23 September 2022;
Published: 11 October 2022.
Edited by:
Peng Zhang, Tobacco Research Institute (CAAS), ChinaReviewed by:
Yin Chen, University of Warwick, United KingdomGuangyi Wang, Tianjin University, China
Copyright © 2022 Zhang, Zhang, Sun, Su, Li, Kun-Liu, Zhang, Chen, Cao and Song. This is an open-access article distributed under the terms of the Creative Commons Attribution License (CC BY). The use, distribution or reproduction in other forums is permitted, provided the original author(s) and the copyright owner(s) are credited and that the original publication in this journal is cited, in accordance with accepted academic practice. No use, distribution or reproduction is permitted which does not comply with these terms.
*Correspondence: Hai-Yan Cao, aGFpeWFuY2FvOTJAMTI2LmNvbQ==; Xiao-Yan Song, eHlzb25nQHNkdS5lZHUuY24=
†These authors have contributed equally to this work