- 1School of Light Industry, Beijing Technology and Business University, Beijing, China
- 2Key Laboratory of Cleaner Production and Integrated Resource Utilization of China National Light Industry, Beijing Technology and Business University, Beijing, China
- 3College of Life Sciences and Bioengineering, School of Science, Beijing Jiaotong University, Beijing, China
- 4Key Laboratory of Feed Biotechnology, Ministry of Agriculture and Rural Affairs, Beijing, China
- 5Feed Research Institute, Chinese Academy of Agricultural Sciences, Beijing, China
The overuse of antibiotics in food animals has led to the development of bacterial resistance and the widespread of resistant bacteria in the world. Antibiotic-resistant bacteria (ARB) and antibiotic-resistant genes (ARGs) in food animals are currently considered emerging contaminants, which are a serious threat to public health globally. The current situation of ARB and ARGs from food animal farms, manure, and the wastewater was firstly covered in this review. Potential risks to public health were also highlighted, as well as strategies (including novel technologies, alternatives, and administration) to fight against bacterial resistance. This review can provide an avenue for further research, development, and application of novel antibacterial agents to reduce the adverse effects of antibiotic resistance in food animal farms.
Introduction
Antibiotics have been used extensively in animal feeds for nearly 70 years and approximately 11,000 tons of antibiotics were applied to farm animals in 2019 (National Research Council [NRC], 1980; Food and Drug Administration [FDA], 2019). In Africa, the European Union, and United States, an estimated 50∼80% of all antibiotics are applied to animals, primarily to promote the growth of animals and to prevent bacterial infection (Martin et al., 2015; Van et al., 2020). Antibiotics used in food animals are predicted to increase by 11.5% (up to 200,235 tons) in 2030 (Tiseo et al., 2020). Approximately 75% of antibiotics are not absorbed by the animals and are excreted from the body via feces and urine, which can directly contaminate and harm the surrounding environment (Mackie et al., 2006; Zhou et al., 2022). Meanwhile, the misuse or overuse of antibiotics in animal production has led to diverse antibiotic-resistant bacteria (ARB) and antibiotic resistance genes (ARGs), which can be transferred in animals and humans (Martin et al., 2015; Zhang et al., 2015; He et al., 2020; Liu et al., 2022; Yang et al., 2022). There is increasing evidence that antibiotic resistance in humans is mainly related to the wide application of nontherapeutic antibiotics in animals (Martin et al., 2015).
Animal farms are a tremendous reservoir of ARB and ARGs, which is one of the emerging issues globally for human and animal health threats (He et al., 2020; Bai et al., 2021; Wang Y. et al., 2021; Gwenzi et al., 2022; Larsson and Flach, 2022). These ARB and their carried mobile ARGs confer resistance to nine major antibiotic classes, including β-lactams (bla), aminoglycosides (aac), tetracyclines (tet), sulfonamides (sul), macrolide-lincosamide-streptogramin B (MLSB; erm), FCA (fluoroquinolone, quinolone, florfenicol, chloramphenicol, and amphenicol; fca), vancomycin (van), colistin (mcr), and multidrug (mdr), respectively, (He et al., 2020; Larsson and Flach, 2022). Hu et al. (2016b) found that the spread of antibiotic resistance between farm animals and humans can mostly be attributed to the transfer of mobile ARGs, significantly enriched in Proteobacteria (mainly including Klebsiella pneumoniae and Pseudomonas aeruginosa), Bacteroidetes, Actinobacteria, and Firmicutes. Meanwhile, these shared ARGs between animals and humans confer resistance to bla, aac, tet, chloramphenicol, MLSB, and sul; humans shared more ARGs with the chicken than other animals (such as pigs and cattle) from different countries (Hu et al., 2016b).
Various exposure routes of ARB and ARGs originated in farms to humans, including direct or indirect contact with animals, manure or products, and inhalation of bioaerosol that harbors them, may be contributing to increased infections that are hardly treated with antibiotics (He et al., 2020; Bai et al., 2021; Founou et al., 2021; Sirichokchatchawan et al., 2021). Some ARBs (such as Staphylococcus spp., Escherichia coli, Salmonella, etc.) and ARGs can be transferred to humans by direct contact with animals, exposure to animal feces or wastewater, and consumption of contaminated animal food products (including meat, eggs, milk, etc.; Founou et al., 2016, 2021; Khan et al., 2020; Bai et al., 2021; Sazykin et al., 2021). Additionally, airborne ARB and ARGs have also been frequently detected in the farms, and the abundance of some ARGs (such as tet, sul, erm, bla, mec, etc.) in farm bioaerosols are up to 2.3∼10.6 log copies/m3 (Sancheza et al., 2016; Song et al., 2021; Gwenzi et al., 2022). Recent work by Bai et al. (2021) showed that Staphylococcus, Acinetobacter, and Sphingomonas were identified in farm airborne; numerous ARGs (including mec, tet, bla, aad, efr, flor, sul, etc.) were dispersed from the animal farms to a distance of 10 km. Multidrug-resistant (MDR) bacteria (including Staphylococcus spp., Enterococcus spp., Salmonella spp., E. coli, etc.) and ARGs were transferred to workers and residents in the surrounding environment via inhalation, thus, posing the risk of diseases (Bai et al., 2021; Jeżak and Kozajda, 2022; Yang et al., 2022).
This review introduces a brief outline of the current situations of ARB and ARGs from food animal farms, manure, and wastewater, as well as risks and strategies in the battle against bacterial resistance (Figure 1). By studying current antibiotic resistance in food animal farms, we hope that more people can clearly understand the adverse effects of antibiotic resistance, human exposure, and health risks associated with animal farming activities, which may further help develop more efficient alternatives to antibiotics.
Bacterial Resistance to Antibiotics in Food Animal Farms
The routine utilization of antibiotics in food animal farms can accelerate the development of bacterial resistance and dissemination of ARB and ARGs (Figure 2 and Table 1), which is an increasing threat to the health of humans and animals (Van et al., 2020).
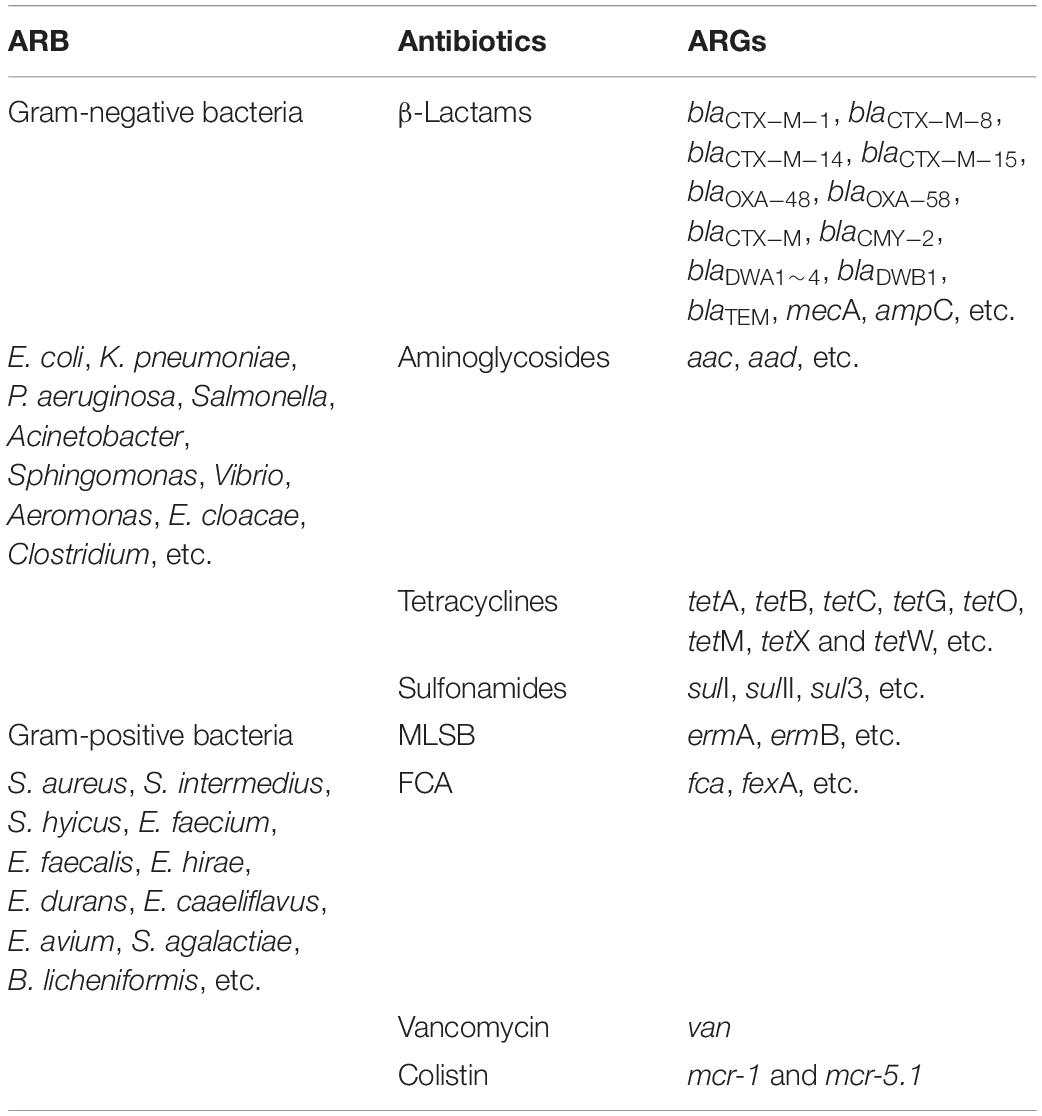
Table 1. Typical antibiotics, antibiotic-resistant genes (ARGs), and antibiotic -resistant bacteria (ARB) in food animal farms.
Farming animals are an important source of bacteria with resistance to antibiotics (Founou et al., 2021). A total of 1,739 samples of zoonotic and commensal bacteria were collected from food-producing animals (including cattle, pigs, and chickens) at slaughter in nine European Union countries and their susceptibility to medically important antibiotics was detected by minimum inhibitory concentration (MIC) testing according to Clinical and Laboratory Standards Institute recommendations (de Jong et al., 2018). It was found that Enterococcus faecium and E. faecalis had higher resistance to tetracycline and erythromycin (27.1∼78.3%) in pigs and chickens than that in cattle (5.2∼30.4%); bacterial resistance to van and tigecycline was absent or low; other strains of E. hirae, E. durans, and E. caaeliflavus had no or low resistance to daptomycin, linezolid, tigecycline, and vancomycin. This pan-European Union survey indicated that bacterial clinical resistance to critically important antibiotics commonly used in human medicine was absent or low, except for erythromycin (de Jong et al., 2018). Nesporova et al. (2021) collected 66 samples from 12 poultry farms and analyzed the linkage between antibiotic resistance and hatcheries from Paraguay and Brazil. After whole-genome sequencing, 62 E. coli and 22 K. pneumonia isolates were identified in these farms. It was observed a higher prevalence of isolates with mcr-5.1 and blaCTX–M–8 in three farms, in which chickens were obtained from a Paraguayan hatchery, than others from a Brazilian hatchery; none of the K. pneumoniae isolates were related to the Paraguayan hatchery. The results implied that antibiotic resistance may be relevant to the source of the chicken and the farms are considered a reservoir of antibiotic resistance (Nesporova et al., 2021).
Animal gut microbiota is a reservoir of ARB and ARGs, and antibiotic utilization is the main cause of ARG prevalence (Zhu et al., 2020). Zhu et al. collected 30 fecal samples from different bovine farms and explored the prevalence and distribution of ARGs using a metagenomic method. The results showed that the diversity and abundance of ARGs in dairy and beef cattle were similar and significantly higher than those in individual yaks, which may be associated with the long-term use of antibiotics. Additionally, insertions, integrations, and transposons were identified and responsible for the common existence of ARGs, leading to horizontal transfer among microbes, especially pathogenic bacteria (Zhu et al., 2020). Zhang J. et al. (2019) investigated the relationship between animal types and the ARGs emission into the environment in typical swine farms in China and found that approximately 4 ± 1.3 × 1017 gene copies of ARGs per day were released from typical swine farms with a stock of 10,000 pigs. Both Firmicutes and Bacteroidetes belong to the dominant phylum in swine manure, but Bacteroidetes, Proteobacteria, and Spirochaetes play a key role in determining the ARGs profiles, indicating that the microbial community in swine manure may mainly contribute to the transfer of ARGs (Zhang J. et al., 2019).
Utilization patterns, excretion, and contamination of 50 antibiotics were investigated in a typical swine farm by Zhou et al. (2013b). A total of 11, 17, and 15 target antibiotics (including tetracycline, bacitracin, chlortetracycline, and florfenicol) were found in feces, flush water, and suspended particles in this farm, which was mainly from the feeds; in comparison, most sul (such as oxytetracycline and doxycycline), macrolides, fluoroquinolones, and trimethoprim mainly originated from oral and injection. Moreover, the daily excretion of antibiotics was 1.47∼48.3 mg/d per pig, which is associated with the growth stage of pigs, and chlortetracycline and bacitracin are major contributors. However, simple waste treatments such as anaerobic digesters or lagoons did not effectively remove antibiotics (Zhou et al., 2013b). Novel treatment systems and improved methods are needed for animal wastewater to eliminate veterinary antibiotics.
Bioaerosols from swine confinement buildings (SCBs) are a reservoir of zoonotic pathogens and ARGs from feces, which may cause a high incidence of respiratory infections in pig farmers (Radon et al., 2001; Hoppin et al., 2014; Sigsgaard et al., 2020). Meanwhile, SCB bioaerosols are comprised of various pathogens, such as Staphylococcus, Acinetobacter, Pseudomonas, and E. avium, and they can spread over long distances to the external environment, posing a tremendous risk to public health. To determine the bacterial microbiome and resistome profiles of SCB bioaerosols and their correlation with the growth stages of pigs, Yan et al. (2021) collected 24 samples and analyzed the bacterial microbiome and resistome profiles by using the next-generation high-throughput sequencing method. The results showed that the predominant phyla were Proteobacteria and the most abundant ARGs were aminoglycoside resistance genes; bioaerosols from farrowing sows exhibited higher abundances of aminoglycoside, MLSB, bleomycin, multidrug, and trimethoprim resistance genes, but lower abundances of polymyxin and fosmidomycin resistance genes than other stage samples (Yan et al., 2021). It suggested there is a strong relationship between the bacterial microbiome and the resistome in SCB bioaerosols, as well as abundant opportunistic pathogens and potential ARG hosts in the indoor air from pig farms.
Additionally, ARB and ARGs are widespread in aquaculture farms, highlighting a great threat to humans and aquatic organisms (Harnisz et al., 2015; Ranjan and Thatikonda, 2021). The diversity of ARGs in the intestinal bacteria of shrimp was investigated by polymerase chain reaction (PCR) and metagenomic analysis (Liu et al., 2019). Tetracycline, quinolone, sulfadiazine, and erythromycin resistance genes were detected in Penaeus vannamei by PCR; 62 different ARGs, which were classified into 21 types (such as aac, macrolides, quinolones, tet, bla, peptide antibiotics, etc.), were identified in the shrimp gut by the plasmid metagenomic method. These ARGs are from Vibrio (accounted for 2.8∼51%) and Aeromonas (16∼55%) plasmids; especially Vibrio may be major bacterial pathogens in shrimp. Notably, the plasmid metagenomic focusing on the mobile genetic elements (MGEs) may have great potential to identify ARGs in complex environments (Liu et al., 2019). Meanwhile, Oviedo-Bolaños collected 450 individuals from tilapia ponds and organ pools in the Northern Pacific region, Costa Rica, and identified ARGs (including tetM, tetO, fexA, and ermB) by the PCR method (Oviedo-Bolaños et al., 2021). The results showed that S. iniae was not detected, and 60% of the ponds and 46% of the organ pools were positive for Streptococcus agalactiae with tetO (29.1%), and tetM (12.7%) and ermB (1.8%), respectively. It is favorable to improve the knowledge of the diseases infected with Streptococcus sp. and to efficiently prevent and control these pathogens in tilapia farming worldwide (Oviedo-Bolaños et al., 2021).
Sharma et al. (2020) investigated antibiotic use and animal health management in smallholder dairy farms in four urban and peri-urban areas of India and found that the knowledge level and practices related to antibiotic use and antimicrobial resistance can potentially increase the risk of resistance antibiotic development and its transfer in the community, which is a major global public health threat. Unregulated or inappropriate antibiotic use in animal farms and using different types of antibiotics at the same time are still common practices in developing countries (Gebeyehu et al., 2015; Chowdhury et al., 2021; Geta and Kibret, 2021). In a survey of small-scale farms in Thailand, 57.1% of farmers reported the use of antibiotics for prevention (Lekagul et al., 2020). Some farmers believed that antibiotics could be used to treat all animal diseases, although, 42.9∼72% understood that antibiotic misuse in animals caused bacterial resistance and the spread of ARB (Nuangmek et al., 2018; Ozturk et al., 2019).
Bacterial Resistance to Antibiotics in Food Animal Manure
Manure, an important fertilizer, contains residual antibiotics, ARB, and diverse ARGs, and its utilization in agricultural fields can affect the structure and function of microflora, leading to an increased abundance of resistant bacteria and resistance genes in the environment (Kim et al., 2010; Wichmann et al., 2014; Van Epps and Blaney, 2016; Xie et al., 2017; Menz et al., 2019).
The vast majority of antibiotics (70%) used in the animals are not absorbed by them and are excreted from their bodies (Mackie et al., 2006). A total of 71 concentrated animal feedlots were collected from Northern China and 24 antibiotics were detected by Li C. et al. (2021). The amounts of antibiotics variated in different animal manures; antibiotic residues in swine manure were 83.18 mg/kg, higher than those of chicken (52.93 mg/kg), beef (37.12 mg/kg), and dairy manures (305 μg/kg), posing serious threats to the terrestrial environment (Li C. et al., 2021). Patyra et al. (2020) detected antibiotics in livestock feces, liquid manure, and digestate from biogas factories located in Poland and Spain by using the liquid chromatography-mass spectrometry (LC-MS) method. The result showed that after administration, active antibiotics were excreted, in some cases 90% of the consumed dose were present in the feces or urine as parent molecules; 18 out of 70 samples were positive for the presence of tetracycline, doxycycline, oxytetracycline, enrofloxacin, chlortetracycline, lincomycin, and tiamulin (Patyra et al., 2020). It suggested that livestock farms may be an important pollution source to transmit antibiotics in feces or manure into the environment. Zhou et al. investigated the excretion masses and environmental occurrence of 50 antibiotics of 11 types in different livestock farms in southern China. A total of 28 antibiotics (such as macrolides, tetracvclines, sulfonamides, fluoroquinolones, bacitracin, lincomycin, etc.) were present in the feeds, wastes, and receiving environments. Antibiotics excreted by swine were mainly from the feeds and 20 times larger than those of dairy cattle, which were from the injection route. The normalized daily excretion masses of chlortetracycline, bacitracin, lincomycin, and tetracycline were 11.6, 3.81, 1.19, and 1.04 mg/day per swine, respectively; chlortetracycline (3.66 mg/day per cattle) contributed to 86% of the antibiotic excretion. Moreover, macrolides, tetracvclines, sulfonamides, fluoroquinolones, etc. were also found in well water, stream, and soil, implying that animal farms may be a vital pollution source of various antibiotics to the receiving environments (Zhou et al., 2013a).
It has demonstrated that a variety of ARB and ARGs in animal manure can confer resistance to aac, macrolides, fluoroquinolones, tetracvclines, sulfonamides, phenicols, and glycopeptides, respectively, (Wichmann et al., 2014; Yang F. et al., 2019; Furlan et al., 2020; Huang J. et al., 2021; Li C. et al., 2021). Fournier et al. (2021) collected 81 fecal samples from a pig farm and detected resistance in Enterobacterales to colistin, β-lactam, and aminoglycoside. There were 38 β-lactam-resistant E. coli isolates and one Enterobacter cloacae, which carry plasmids with blaCTX–M–1, sul2, and tetA genes, respectively, conferring resistance to β-lactam, sul, and tetracycline; it was also identified two colistin-resistant K. pneumoniae isolates and a single E. cloacae isolate with a truncated mgrB, encoding resistance to colistin. The results indicated that a prevalence of β-lactam-resistant E. coli can increase the risk of resistance distribution in the environment (Fournier et al., 2021). A total of 40 feces were collected from yaks, beef, and dairy cattle by Wang Y. et al. (2021), and bacterial antibiotic resistance was analyzed by the metagenomic method (Wang W. et al., 2021). It was found that 734 ARG subtypes of 1,688 annotated genes, were associated with β-lactam, tetracyclines, aminoglycosides, and quinolones. Meanwhile, it was found higher abundance of ARGs and a lower level of integron in beef and dairy cattle than in yak, which may be related to the antibiotic selective pressure of their different density feeding patterns. Yaks raised under a low-density feeding pattern may use fewer or no antibiotics, leading to less emergence of resistance. For beef and dairy cattle under high-density feeding patterns, ARGs may be transmitted horizontally from the environment by integron (Wang W. et al., 2021).
Wang X.R. et al. (2021) determined the diversity of ARGs from duck wastes by using the metagenomic method. Among 76 samples, there were 61 variants or novel ARGs, which included five novel β-lactam ARGs (such as blaDWA1∼4 and blaDWB1) and two fosfomycin-resistant genes (such as fosA-like1 and fosA-like2). These novel ARGs were related to either hydrolyzation of both penicillins and cephalosporins or MGEs. Noticeably, the MICs of E. coli carrying fosA-like genes increased by 128-fold, indicating a key resistome reservoir in duck farms and posing a high spread risk (Wang X.R. et al., 2021). Ruuskanen et al. (2016) collected manure samples for storage for 8 months and then investigated the effects of manure on the abundance of resistance genes in the farm environment in southern Finland by PCR and quantitative PCR (qPCR) methods. It was found that the relative abundance of AGRs increased by 4-fold in the soil after manure application; carbapenemase encoding blaOXA–58 gene was found in four dairy cattle and swine farms, indicating dissemination in the farm environment. In addition, the relative abundance of ARGs in stored manure increased by 5-fold compared with fresh manure roughly, indicating that ARGs are proliferated and dispersed in the farm environment (Ruuskanen et al., 2016).
Antibiotic residues, ARB, and ARGs in manure are considered a growing threat to the human, animal, and environmental health (Lima et al., 2020). Ma et al. (2021) investigated the influence of chlortetracycline on the gut microbiota in pigs and ARGs by 16S rDNA sequencing and metabolomics analysis. The result showed that after the addition of 75 mg/kg of chlortetracycline into the basal diet, the diversity of the gut microbiota in feces and manure dramatically reduced and affected its structure; regulating metabolic pathways mainly involved in the tricarboxylic acid cycle, either propionate or pyruvate metabolism. For 30∼120 day-old pigs, the levels of chlortetracycline residue in feces and the abundance of three tetracycline-resistant genes (including tetC, tetG, and tetW) markedly increased. Additionally, chlortetracycline residues and ARGs abundance in feces and manure gradually decreased with fermentation time, and aerobic composting more potently reduced ARGs abundance (by 84.4%) than anaerobic digestion (79.4%), indicating that fermentation could remove most of the antibiotic residues. The results suggest that although chlortetracycline can reduce the diversity of the gut microbiota in pigs and increase the abundance of ARGs in feces, fermentation may be a common effective method of waste recycling (Ma et al., 2021). Huang J. et al. (2021) investigated the effects of animal manure application on ARG abundance and bacterial distribution in the soil-plant system by qPCR and 16S rRNA sequence analysis. It was found that the utilization of poultry or swine manure remarkably increased ARG abundance in the soil, especially tetG and tetC; animal manure significantly enhanced ARG abundance in the lettuce endosphere, especially tetG in poultry or swine manure and tetX in chemical fertilizer, but not in the lettuce phyllosphere. Sphingomonadaceae, Flavobacteriaceae, Comamonadaceae, Flavobacteriaceae, Hyphomicrobiaceae, and others were shared in the soil, lettuce endosphere, and phyllosphere. A significant increase in ARG abundance in the soil-lettuce system caused by animal manure application may be related to the shared bacterial distribution (Huang J. et al., 2021). Therefore, it is crucial to reduce the number of antibiotics and a load of resistant bacteria/genes that end up in the surrounding environment.
Bacterial Resistance to Antibiotics in Animal Farming Wastewater
Antibiotic-resistant bacteria and ARGs, conferring resistance to antibiotics, have all been found in animal farming wastewater, which is currently considered a serious public health issue (Al Salah et al., 2019; Yang Y. et al., 2019). In recent years, increasing attention is drawn to antibiotic resistance in animal wastewater, especially in developing countries (Van Epps and Blaney, 2016).
Brooks et al. (2014) analyzed the constituents of bacterial pathogens and several ARGs (such as tet, erm, mec, int, etc.) in swine manure wastewater by using the qPCR method. Nearly all tested ARGs were found in sow, nursery, and finisher farms; the highest levels of tetracycline- and macrolide-resistant tetA and ermF genes were approximately 109 and 6 × 108 GU/100 mL in nursery farm lagoon effluent. Other ARGs, including ermA, tetB, and intI, ranged from 106 to 107 GU/100 mL. However, finisher farms had less diversity of microbes and fewer ARGs than others, which may be related to the continuous application of antibiotics in feed. Noticeably, it was found to reduce the methicillin-resistant mecA gene as the pigs aged. This result implied that microbial populations and ARGs in swine farm wastewater may be influenced by farm management systems (Brooks et al., 2014).
West et al. (2010) detected bacterial antibiotic resistance and gene transfer in waterways near confined animal feeding operation (CAFO) farms. The results showed that among 830 bacterial isolates, ampicillin-resistant bacteria accounted for 77.1%, and others were resistant to many antibiotics, including kanamycin, ampicillin, streptomycin, oxytetracycline, and chlortetracycline, respectively, which can be affected by CAFO farms. Additionally, 83.3% of the bacterial isolates could transfer resistance genes-tetB and tetC to Salmonella enterica Typhimurium by conjugation, indicating a high risk for pollution of MDR bacteria from animal waste (West et al., 2010).
Al Salah et al. (2019) detected the bacterial abundance and ARGs in Sub-Saharan rivers receiving animal farming wastewaters in Kinshasa, Congo by the qPCR method. It was found that the two rivers are a reservoir of resistant bacteria (such as E. coli, Pseudomonas, and Enterococcus), and β-lactam, sulfonamide, and tetracycline resistance genes (including blaOXA–48, blaCTX–M, sul1, sul2, sul3, and tetB, respectively); both sul1and sul2 genes were the most abundant and the most detected ARGs. Contamination sources mainly originated from pigs and anthropogenic activities, and animal farming wastewaters did not exclusively lead to antibiotic resistance profiles. This serves as a very valuable tool to evaluate antibiotic resistance contaminants in aquatic ecosystems (Al Salah et al., 2019).
Zhao et al. (2022) identified ARGs in decentralized sewage treatment facilities from both livestock and households in developed rural areas in eastern China using metagenomics. A total of 825 bacterial-related and 19 bacteriophage-related ARGs in the rural wastewater were identified; the dominant ARGs were resistant to bacitracin, indicating the difference between rural and municipal wastewater. Bacteriophage ARGs (including gyrA, drfE, rpoB, and parC) mainly confer resistance to rifampin, trimethoprim, aminocoumarin, lipopeptide, fluoroquinolone, and pyrazinamide. Acidovorax and Prymnesiovirus are the dominant hosts of ARGs. Noticeably, bacteriophages, as a reservoir of ARGs, are also involved in the dissemination of ARGs (Zhao et al., 2022). Therefore, we should pay attention to the transmission of ARGs by bacteria and phages in wastewater treatment facilities in the future.
Antibiotic residues and ARGs in farm wastewater can dramatically destroy the micro-ecological balance and enhance the prevalence of resistant bacteria in the environment, which urgently need environmental stewardship in some farm operations (Van Epps and Blaney, 2016; Goulas et al., 2018).
Potential Risks of Antibiotic Resistance in Food Animal Farms
Overuse or misuse of antibiotics to treat infectious diseases in animals can lead to the residues of antibiotics and ARGs, which is an increasingly serious threat to human health worldwide and food security (Martin et al., 2015; Founou et al., 2021). As shown in Figure 3, ARB is transferred to humans by direct contact with farm animals, through exposure to animal manure, wastewater, or aerosol, and by consumption of uncooked animal products (such as meat, eggs, milk, etc.; Li et al., 2016; Koch et al., 2017; Deng et al., 2018; Li C. et al., 2021; Liao et al., 2021).
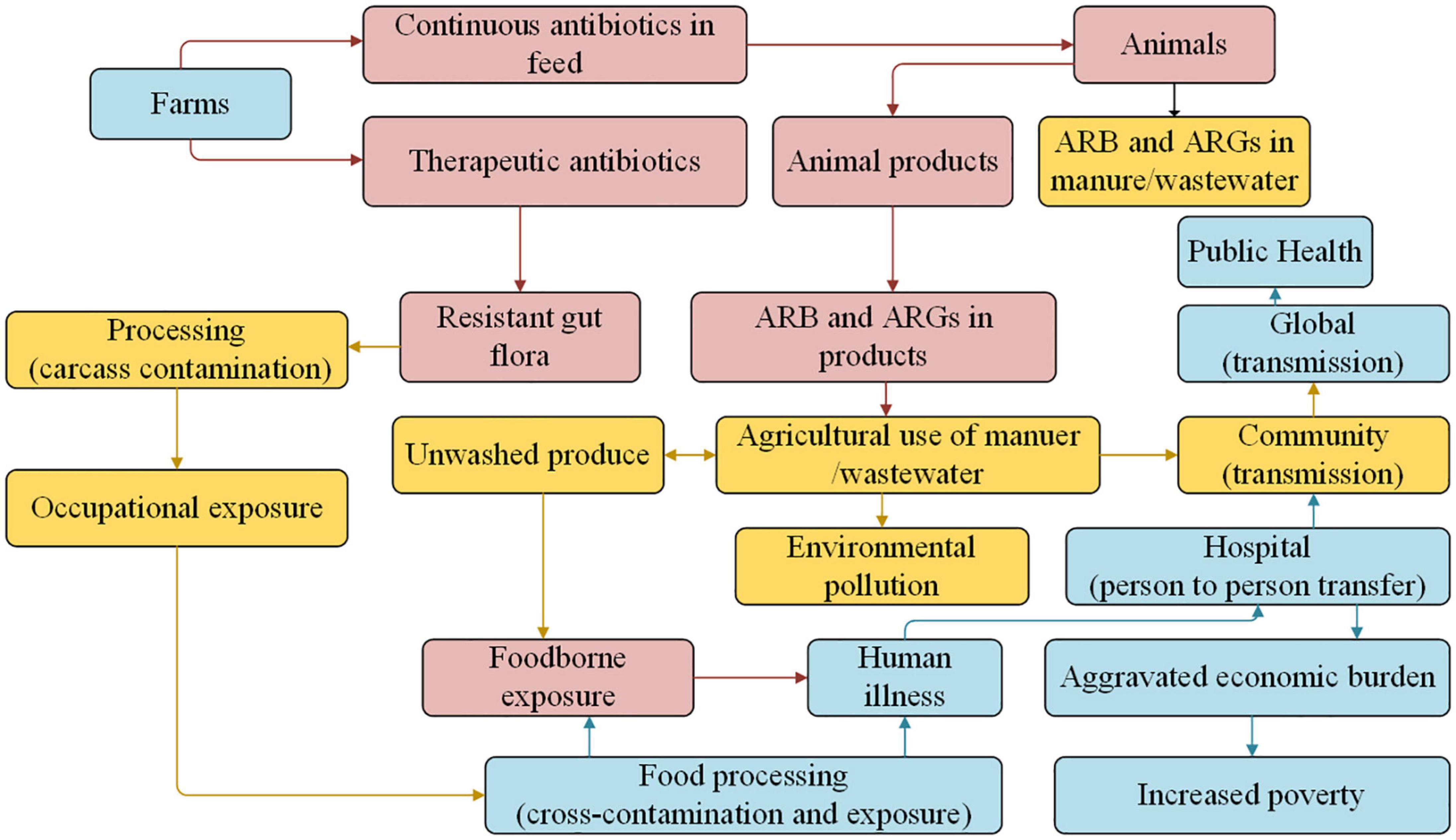
Figure 3. Multiple pathways involved in ARB and ARGs in the food animal and human health (Koch et al., 2017).
Direct Contact With Animals
Several studies have demonstrated that farm animals are a reservoir of ARB (such as E. coli, K. pneumonia, Salmonella, S. aureus, etc.) and ARGs (Table 1), and people can be infected with ARB through close contact with farm animals (Tomley and Shirley, 2009; Dierikx et al., 2013; McDaniel et al., 2014; Saliu et al., 2017; Founou et al., 2021; Meijs et al., 2021).
The occurrence and prevalence of extended-spectrum β-lactamase (ESBL)- and AmpC β-lactamase (AmpC)-producing E. coli and K. pneumoniae in farmworkers and household members in Netherlands were investigated by Meijs et al. (2021). PCR and sequencing results showed that ESBL-producing E. coli and K. pneumoniae in workers accounted for 9.8%, higher than that in the Dutch population (5%), which may be related to direct contact with animals. The resistant genes-blaCTX–M–15 (48.5∼64.3%), blaCTX–M–14 (7.1∼18.2%), and blaDHA–1 (12.1%) were the most prevalent ESBL ones in workers. Additionally, 17.4% of household members had ESBL-producing E. coli and K. pneumonia and 13% of members carried the same ESBL gene as workers. The data indicated that direct or indirect contact with animals is a potential source of ARB and ARGs (Meijs et al., 2021).
Huijbers et al. (2014) investigated whether resistant E. coli can be transferred from broilers to farmers. The result showed that the prevalence of ESBL- and AmpC-producing E. coli among people on farms was up to 14.3∼27.1% after contact with infectious live broilers, higher than those among partners and family members who are not living on farms (11.4∼15.7%). This result was consistent with that of previous studies, in which direct contact with broilers may have a high risk for ESBL- and AmpC-producing E. coli among humans (Huijbers et al., 2013, 2014). Subsequently, Huijbers et al. (2015) evaluated the prevalence of methicillin-resistant S. aureus (MRSA) and AmpC-producing E. coli among broilers at different ages and workers. No MRSA isolates were found in broilers and workers; the prevalence of ESBL- and AmpC-producing E. coli in farms was 87.5 (34 day-age) and 100% (68 day-age), higher than that of workers (18.5%). Meanwhile, both blaCTX–M–1 and blaCMY–2 resistant genes were detected in broilers and workers, which may be associated with contact with live animals. This result stated that direct contact with animals is a high-risk factor for the carriage of ARB and ARGs (Huijbers et al., 2015).
Vines et al. (2021) collected 210 fecal samples from farm animals and the farmer and analyzed the spread of mcr-associated with plasmids among E. coli by multiplex PCR. It was identified 18 colistin-resistant E. coli isolates carrying mcr-1 (including 13 from calves, four from pigs, and one from the farmer, respectively) and 33 virulence factors. Moreover, the mcr-1 gene was transferred from the calf to the farmer, posing the risk of a farm as a reservoir of ARB with zoonotic potential (Vines et al., 2021).
Workers on farms may have an increased risk of acquiring ARB and ARGs due to their direct contact with animals and transferring between persons, except for exposure to antibiotics in their daily practice environment (Meijs et al., 2021).
Exposure to Animal Manure, Wastewater, or Bioaerosols
Animal manure and wastewater have been regarded as the hotspots for the transmission of ARB and ARG from animals to humans (Sirichokchatchawan et al., 2021). A large amount of evidence has demonstrated that animal waste and slaughterhouses are a revisor of MDR bacteria, indicating an occupational risk to workers (Aworh et al., 2021).
Aworh et al. (2021) analyzed the prevalence of fecal carriage of MDR E. coli among 118 healthy workers in a slaughterhouse by using the Kirby-Bauer disk diffusion method. It was found that the prevalence of MDR E. coli among butchers was up to 50%, higher than that among cleaners at the slaughterhouse. This result may be related to keeping animals at home and eating or collecting waste at the slaughterhouse (Aworh et al., 2021). These data provide further evidence demonstrating the occupational hazards for people working in slaughterhouses.
Farm bioaerosols generated during farming practices have also been regarded as a reservoir of ARB and ARGs and may pose a high risk to animal and human health (McEachran et al., 2015; Van Leuken et al., 2016; Anand et al., 2021; Gwenzi et al., 2022). Bai et al. (2021) investigated the spread of ARB from animal bioaerosols and found that ARB and ARGs in bioaerosols could easily be transmitted from animal farms to the environmental dust through the natural wind. Guo et al. analyzed the abundance and transmission route of airborne ARGs in pig farms (Song et al., 2021). The result showed that both Clostridium and Streptococcus were dominant ARBs, and the abundance of ARGs and MGEs in bioaerosols during winter was higher than that during the summer. The concentration of dominant tetM in bioaerosols was 6.3 log copies/m3, higher than that in feces (10.6 copies/m3), and the transposon IS613 had the highest abundance of 4.9 log copies/m3. Additionally, the wind speed and high temperature could increase the spread of ARGs in bioaerosols. Noticeably, fecal contribution to ARB in bioaerosols accounted for 59.4% in winter, higher than that in summer (19.9%). Horizontal gene transfer of ARGs in bioaerosol exhibited a higher possibility in winter (77.8%) than that in summer (12.0%; Song et al., 2021). These ARB and ARGs in bioaerosols can be inhaled into the respiratory tract of animals and humans, causing negative health effects such as allergies or asthma (Gao et al., 2018).
Therefore, frequent exposure to animal manure, waste, or bioaerosol, which contain a large number of ARB and ARGs, is considered one of the potential human health threats.
Consumption of Food Animal Products
The threat of antibiotic over-usage in food-production animals and the associated emergence of antibiotic resistance in zoonotic bacterial pathogens have been recognized for decades (Overdevest et al., 2011). Animal products including meat, eggs, and milk have been proven to be a key route of extraintestinal MDR pathogens, especially for E. coli and Salmonella, posing a potential health risk to consumers (Borzi et al., 2018; Yamaji et al., 2018; Diaz-Jimenez et al., 2020). Previous studies have demonstrated the contamination rate of animal meat carried ARB reached up to 100% (Geser and Hächler, 2012; Saliu et al., 2017).
The prevalence of ESBL genes of Enterobacteriaceae and their relationship between animal meat and humans in Netherlands were determined by Overdevest et al. (2011). The prevalence of ESBL genes in chicken, mixed meat, beef, and pork was 79.8, 9.1, 4.7, and 1.8%, respectively, indicating higher ESBL contamination of chicken meat. Moreover, 6.7% of patients have been infected with ESBL-producing E. coli and 4.9% of samples carried ESBL genes, such as blaCTX–M–15, blaCTX–M–1, and blaCTX–M–14 (Overdevest et al., 2011). Deng et al. analyzed 152 Salmonella isolates from retail foods of animals by susceptibility testing and found that 92.8% of bacteria were resistant to over one antibiotic. A higher resistance to oxytetracycline and trimethoprim (80.9∼64.5%) was also observed than other antibiotics (28.9∼10.5%), including amoxicillin, ampicillin, levofloxacin, ciprofloxacin, and gentamicin, respectively, while both blaTEM and tetA genes occupied 44.7%. Additionally, tet and sul genes were closely related to disinfectant or heavy metal resistance genes (such as pcoC, pcoR, or qac). This result indicated that retail meat may be a reservoir for the transmission of antibiotic-resistant Salmonella (Deng et al., 2018), becoming a serious health threat to humans.
Zou et al. (2021) evaluated the prevalence of extraintestinal pathogenic E. coli (ExPEC) in healthy chickens. Among 926 E. coli isolates, there were 22 ExPEC ones (accounting for 2.4%), and most of them carried both blaCTX–M and fosA3 resistance genes, conferring resistance to β-lactam and fosfomycin, respectively. E. coli O78 was found to be the most predominant one of six serogroups, which commonly occurred in humans. It suggested that these ExPEC may be transmitted to humans by food supply (Zou et al., 2021).
Cornejo et al. (2020) collected chicken eggs from 83 backyard poultry production systems (BPS) in central Chile and detected antibiotic residues in eggs by using a Four-Plate Test screening assay. The results showed that eggs from 61, 53, and one BPS were positive for at least one antibiotic, more than one, and all four types of antibiotics; the positivity of BPS to bla, aac, tet, and macrolides was up to 59, 56.6, 20.5, and 13.3%, respectively. Antibiotic residues in eggs provide supporting information for non-addressed food safety and animal management, highlighting a potential health risk to consumers (Cornejo et al., 2020).
Balemi et al. (2021) detected the antibiotic resistance of 564 bacterial isolates from California Mastitis Test positive milk from dairy cows, camels, and goats against antibiotics commonly used in Southern Ethiopia. The results showed that coagulase-negative Staphylococcus species (accounted for 39.1%), S. aureus (17.2%), S. hyicus (14.1%), S. intermedius (9.4%), and E. coli (9.4%) were major pathogens; E. coli isolates from both cows and goats were resistant to vancomycin, ceftriaxone, spectinomycin, and doxycycline, and they were intrinsically resistant to penicillin; S. aureus isolates from all animals were resistant to spectinomycin, penicillin G, and clindamycin, indicating a public health risk of infection with MDR bacteria due to regular consumption of raw milk (Balemi et al., 2021).
These findings suggest that the food chain of animal products has been one of the important sources of ARB and ARGs transmission between animals and humans, posing a serious global public health threat. The abundant presence of ARB and ARGs transferring from animals to humans may have profound influences on future treatment, highlighting the importance of implementing hygiene measures to decrease the spread risk, thus, it urgently needs to be integrated, multi-sectoral, and global strategies (Overdevest et al., 2011; Stefanska et al., 2021).
Strategies to Reduce Antibiotic Resistance From Food Animal Farms
Vast amounts of antibiotics are introduced into animal farming and cause the growing prevalence of ARB and ARGs (Table 1), which is an increasing public health concern (Founou et al., 2021; Zalewska et al., 2021). More attention should be given to the antibiotic residues in animal waste to prevent its transfer to the environment, and effective strategies are required to minimize bacterial resistance in animal farms.
Novel Technologies
A few different methods, such as nanotechnology, anaerobic digestion, biochar composting, etc., have been developed to minimize ARB and ARGs in recent years (Figure 4; Yu et al., 2017; Goulas et al., 2018). Yu et al. (2017) collected water samples (including feedlots, fishponds, and wastewater treatment plants) and performed the removal test of ARGs for water by graphene oxide (GO) nanosheet. The results showed that GO nanosheet could nonspecifically bind to the ARGs (such as tetA, ermB, ampC, and sul2) by π-stacking interactions, and approximately 80% of ARGs can be removed from water by GO. The removal efficacy of GO nanosheet for ARGs reduced by <40% after 5 regeneration cycles, indicating excellent stability and reusability of GO nanosheet. GO nanosheet may be a desirable candidate for the efficient treatment of ARGs in animal wastewater or other water (Yu et al., 2017). Visca et al. (2021) firstly performed the effects of anaerobic digestion on the removal of ciprofloxacin, sulfamethoxazole, and enrofloxacin and their ARGs in a dairy farm. The result showed that degradation of sulfamethoxazole, ciprofloxacin, and enrofloxacin was 100, 92, and 84%, respectively; removal efficiency of their resistance genes was 78.3% (ciprofloxacin), 50.3% (enrofloxacin), and 37% (sulfamethoxazole), respectively. It noted that anaerobic digestion can be a promising practice for minimizing antibiotic residues and ARGs in animal waste (Visca et al., 2021). Additionally, both tetracycline and sulfadimethoxine were added into dairy manure and treated for 44 days; the effects of anaerobic digestion on the removal of antibiotics and ARGs were firstly investigated by Schueler et al. (2021). The results showed that tetracycline and sulfadimethoxine were reduced by 0∼96% and >99%, respectively, during the digestion processing, and were accompanied by a decline in tetM gene copies. However, the reduction in sulfadimethoxine was uncorrelated with a decline in sul1 gene copies. Additionally, after the addition of antibiotics at the concentration of 10 mg/L, methane production decreased by 7.8% when compared with the manure-only reactors (Schueler et al., 2021). It indicated that anaerobic digestion may offer the opportunity to remove some antibiotics and reduce antibiotic resistance in ecosystems.
Tao et al. (2014) determined ARGs in wastewater treatment plants of six large swine farms in Taiwan by qPCR. All resistant genes (including tetW, tetA, sulI, sulII, and blaTEM) were detected in six livestock farms, indicating that resistance to tetracyclines, sulfonamide, and β-lactams is prevalent in the wastewater treatment of livestock farming. The highest levels of five ARGs were found in the anaerobic treatment tank and the lowest in the activated sludge unit and the effluents. The removal efficiency of these ARGs ranged from 33.30 to 97.56% in influx and efflux samples. It suggested that anaerobic treatment of livestock farming wastewater may reduce the dissemination of ARB (Tao et al., 2014).
Liao et al. (2021) explored the effects of composting storage (4 weeks) on the abundance of residual ARGs and MGEs by using metagenomics, qPCR, and direct culturing methods. It was observed that 43.8% of ARGs and 39.9% of MEGs rapidly rebound during the 1st week of the composting phase, which was mainly caused by the regrowth of indigenous ARB; residual ARGs and MGEs also rebound at the end of the storage, which was associated with external airborne bacterial transmission (Liao et al., 2021). Moreover, hyperthermophilic composting with a relatively higher temperature (up to 90°C) was more efficient at reducing ARGs, MGEs, and their associated bacteria than that of conventional composting (Liao et al., 2018, 2021), indicating the importance of exploring more efficient compost strategies to inhibit the rebound of ARGs and MGEs. Zhang M. et al. (2019) detected the effects of manure composting on eliminating 41 veterinary antibiotics and found that 64.7% of antibiotics were removed after the 171 day-composting. The removal rates for macrolides, tet, and sul were 100, 73.4, and 45.1%, respectively, except for fluoroquinolones (negative), indicating their different removal rates. This may be associated with the compost’s physico-chemical parameters, such as temperature, moisture, and the ratio of total organic carbon and Kjeldahl nitrogen. During composting, low moisture and pile turning frequency could inhibit further dissipation of antibiotics (Zhang M. et al., 2019). This result is consistent with previous studies in which low moisture and high temperature could cause early dehydration and degradation (McCartney and Tingley, 1998; Ho et al., 2013; Mitchell et al., 2015). Utilization of compost manure with antibiotic residues can cause soil contamination, posing a risk of antibiotic resistance selection to the soil ecosystem (Zhang M. et al., 2019). Liu et al. (2021) explored the influences of co-composting on the removal of antibiotics and ARGs in pig manure by using different biomass ratios of microbial agents, including Phanerochaete chrysosporium (p), Bacillus licheniformis (b), and Aspergillus niger (a), respectively. There were four composting piles of A (p:b:a = 1:0:0), B (control), D (p:b:a = 1:5:5), and G (p:b:a = 1:4:0), respectively. The best removal effect of tetracycline (93.7%) and oxytetracycline (87.8%) was found in composting pile D, higher than that in pile B (75.9 and 58.6%, respectively); the highest removal of doxycycline (98.6%) and enrofloxacin (89%) existed in pile A, higher than that in pile B (64.2 and 65.4%, respectively). This result is superior to a previous study in which the removal rate of antibiotics ranged from 28.8 to 77.8% (Lu and Lu, 2019). Moreover, ARGs in pile D, except those for sul, were reduced by 1.059 × 10–3∼10–2 gene copies/16S rRNA copies compared with those in pile B. In pile A, the relative abundance of integrase genes-intI1 and intI2 effectively decreased (Liu et al., 2021).
Zhang et al. (2020) detected variations in AGRs of feces of the dairy cow during dairy manure piled up by metagenomic analysis. The results showed that the diversity of pathogens and abundance of ARGs had a significant increase in accumulated manure. Meanwhile, bacterial pathogens from manure were transferred to the environment when manure was applied as fertilizer (Zhang et al., 2020). Thus, effective management and proper measures should be taken in compost piles and manure application to reduce the dissemination of antibiotics in the environment.
Interestingly, Tian et al. (2021) collected 60 samples from 15 earthworm farms and investigated the fate of ARGs during earthworm conversion of cow dung in northern China. It was observed that the abundance of some ARGs sharply declined after composting with earthworms, but some ARGs remained in earthworms and vermicompost, with the level of 10–1 to 10–2 copies/16S copies. In 15 farms, the most abundant tetracycline-resistant tet gene was up to 10–6∼10–1 copies/16S copies and other high-risk β-lactam resistance bla genes were also prevalent, indicating the dispersion risk of ARGs during the earthworm conversion of cow dung. Additionally, other factors, such as heavy metals and total nitrogen content, may be linked to the abundance of some ARGs (Tian et al., 2021).
Liu et al. (2013) investigated the removal capability of some antibiotics (such as ciprofloxacin HCl, oxytetracycline HCl, and sulfamethazine) and ARGs (including tetO, tetM, and tetW) from swine wastewater by using volcanic- and zeolite-medium system treatment. The qPCR results showed that antibiotic concentrations significantly decreased in two vertical flow constructed wetlands, and oxytetracycline HCl had the highest removal rate, superior to ciprofloxacin HCl and sulfamethazine. The absolute abundance of tetO, tetM, and tetW declined by 50%, as well as one order of magnitude in volcanic and zeolite systems. Meanwhile, the zeolite-medium system more efficiently eliminated antibiotics from wastewater than the volcanic-medium one, which may be related to different pH and average pore sizes of media. More antibiotics were adsorbed in the soil, higher than that in the media and vegetation. This implied that soil is responsible for antibiotic removal from wastewater in constructed wetlands, which can adsorb all almost antibiotics (>90%) from the aqueous phase (Liu et al., 2013).
Except for the above, other methods (such as photocatalyst, biochar composting, nanotechnology, reactors, etc.) used in diverse environmental samples can be potentially applied for the degradation of antibiotics and ARGs in animal farms due to excellent stability and recyclability (Tables 2, 3; Chen et al., 2018; Peng et al., 2018; Shi et al., 2018; Srinivasa Raghavan et al., 2018; Guo et al., 2020; Hu et al., 2020; Meng et al., 2020, 2021; Huang B. et al., 2021; Li S. et al., 2021; Wang J. et al., 2021; Zhou et al., 2021).
Development of Alternatives to Antibiotics
Some effective or commercially viable alternatives have been implemented by animal farmers or are under development; various alternatives (such as herbal plant extracts, probiotics, vaccines, enzymes, antimicrobial peptides, phages, etc.) to antibiotics are available to reduce antibiotic use in food animals in farms (Figure 4; Cheng et al., 2014; Callaway et al., 2021; Sirichokchatchawan et al., 2021). Among them, some herbal plant extracts (such as tannins, saponins, essential oils, etc.) and probiotics are regarded as powerful natural alternatives to antibiotics due to their various functions including antimicrobial activity, antioxidant activity, anti-inflammatory, immunomodulation, etc. (Huang et al., 2018; Farha et al., 2020; Hernandez-Gonzalez et al., 2021).
Tannins are a group of polyphenolic compounds with a molecular weight of 0.5∼30 kDa that are widely distributed in plants and have potent antibacterial activities against E. coli, MRSA, Shigella flexneri, and others (Si Heung et al., 2012; Huang et al., 2018; Girard and Bee, 2020). Similarly, plant saponins are comprised of sugar (glycon) and nonsugar ones (aglycon) connected by a glyosidic linkage and exhibit excellent activity against Salmonella, E. coli, and Streptococcus aureus in vitro and chickens (Khan et al., 2018). Garlic oils have long been known as the most effective plant extract to treat bacterial infections (Navidshad et al., 2018). Magrys et al. (2021) investigated the antibacterial activity of garlic extracts against Enterococcus faecalis, S. aureus, P. aeruginosa, and E. coli. It was found that garlic extracts had potent bactericidal activity against resistant S. aureus, E. coli, P. aeruginosa, and K. pneumonia; garlic extracts showed combinations with gentamycin and ciprofloxacin (Magrys et al., 2021). These functional secondary metabolites used in animals can improve gut health and digestive performance to animals, indicating their alternative treatment to improve the health of animals (Redondo et al., 2014).
Additionally, some probiotics used in animal production have increased significantly over 10 years. Particularly, both Bacillus and lactic acid bacteria (LAB) are considered effective and safe alternatives to antibiotics for animal production due to their high stability in vivo (Dowarah et al., 2017; Mingmongkolchai and Panbangred, 2018). Bacillus belongs to Gram-positive bacteria and can form spores, which are favorable for long-term storage; Bacillus can improve growth performance, immunity function, and gut health in animals (Grant et al., 2018; Hu et al., 2018; Ramlucken et al., 2020). LAB is a class of bacteria that produce bacteriocins and can prevent bacterial resistance, so LAB is a powerful alternative to antibiotics (Hernandez-Gonzalez et al., 2021). Musliu et al. (2021) investigated the antibiotic potential of LAB extract and supernatant on MDR pathogenic bacteria from animal farms. The result showed that both crude culture extract (CCE) and cell-free supernatant (CFS) of LAB exhibited significant inhibition zones (7∼25 mm) against E. coli, S. aureus, and S. agalactiae, respectively. Meanwhile, CFS of LAB was more efficient than CCE, which can contribute to declining the burden of infection caused by ARB in farms (Musliu et al., 2021).
The crude extract isolation of herbal plants and the cultivation of probiotics is a simple process that does not require the use of any sophisticated equipment and techniques, thus they may be recommended as ones of the most promising alternatives to antibiotics and used in the treatment of MDR pathogens in both local and modern animal farms to reduce antibiotic usage (Dowarah et al., 2017; Huang et al., 2018; Abdallah et al., 2019).
Stringent Administration of Antibiotic Application
To minimize antibiotic resistance, a reduction or limitation of antibiotics used in food animals is likewise a very important strategy (Lee et al., 2013). Firstly, many antibiotics cannot be used in food animals as growth promoters and prevention purposes. The European Union, the United States, China, and other countries have banned or restricted the use of some antibiotics as growth promoters and disease prevention in food animals (Table 4); however, use in healthy herds for disease prevention was not prohibited (Food and Drug Administration [FDA], 2017; Salim et al., 2018; Heffernan, 2022). Antibiotics are used only in food animals to treat clinical diseases in accordance with the instructions for use. Meanwhile, government officers should restrict or ban some important antibiotics such as colistin and van, which are last-hope or last-resort ones (Figure 4; Qiao et al., 2018). Early in 2012 and 2016, the plasmid-mediated van-resistance enterococci and colistin-resistance E. coli were found in broilers, pigs, pork products, and humans in Sweden and China, respectively, (Nilsson, 2012; Hu et al., 2016a; Qiao et al., 2018). Colistin-resistance gene (mcr) existed in more than 11 bacterial species and it has been disseminated to over 40 countries (Liu et al., 2020). It has demonstrated that interventions that restrict antibiotic application in food animals are closely related to a decrease in the presence of ARB and ARGs (Tang et al., 2017). Secondly, the authorities should publish the guidelines for the prudent application of antibiotics in food animals, strictly limiting the type, usage, amount of antibiotics used, and only allowing their use for therapeutic purposes, to reduce the lowest indispensable level and minimize the development of bacterial resistance (Ungemach et al., 2006). Of note, farmers do not use combinations of antibiotics in the same production cycle, which leads to a higher frequency of multi-drug resistance in pathogenic bacteria than single-drug treatment, especially on broiler farms (Jibril et al., 2021). Additionally, the licensing rules of antibiotics would be made more stringent and the authorities should impose penalties on defaulters (Figure 4; Lee et al., 2013).
Generally, concerted efforts are needed to minimize antibiotic resistance in animal farms, especially the development of novel technologies, alternatives, and appropriate use of antibiotics.
Conclusion
The increasing use of antibiotics in animals over the past century has led to the widespread transmission of ARB and AGRs between animals and humans. Here, we have mainly reviewed the current situation of ARB and ARGs from animal farms, animal manure, and wastewater, as well as potential risks of bacterial resistance in animal farms. We also proposed the development of novel technologies (such as nanotechnology, anaerobic digestion, and biochar composting), alternatives to antibiotics (including herbal plant extracts, probiotics, vaccines, etc.), and antibiotic administration to reduce ARB and ARGs in animal farms, which may help us address the issues of antibiotic resistance.
Author Contributions
CX helped to investigate, supervise, and wrote the manuscript. LK wrote the manuscript and prepared the figures. HG and XC helped to investigate and review the manuscript. XW conceptualized and wrote the manuscript. All authors read and approved the final manuscript.
Funding
The authors thank the Open Research Fund Program of the Key Laboratory of Cleaner Production and Integrated Resource Utilization of China National Light Industry (No. CP2021YB01) and the National Natural Science Foundation of China (Grant Nos. 32072770 and 31772640) for providing financial support.
Conflict of Interest
The authors declare that the research was conducted in the absence of any commercial or financial relationships that could be construed as a potential conflict of interest.
Publisher’s Note
All claims expressed in this article are solely those of the authors and do not necessarily represent those of their affiliated organizations, or those of the publisher, the editors and the reviewers. Any product that may be evaluated in this article, or claim that may be made by its manufacturer, is not guaranteed or endorsed by the publisher.
References
Abdallah, A., Zhang, P., Zhong, Q., and Sun, Z. (2019). Application of traditional Chinese herbal medicine by-products as dietary feed supplements and antibiotic replacements in animal production. Curr. Drug Metab. 20, 54–64. doi: 10.2174/1389200219666180523102920
Al Salah, D. M. M., Laffite, A., and Pote, J. (2019). Occurrence of bacterial markers and antibiotic resistance genes in Sub-Saharan rivers receiving animal farm wastewaters. Sci. Rep. 9:14847. doi: 10.1038/s41598-019-51421-4
Anand, U., Reddy, B., Singh, V. K., Singh, A. K., Kesari, K. K., Tripathi, P., et al. (2021). Potential environmental and human health risks are caused by antibiotic-resistant bacteria (ARB), antibiotic resistance genes (ARGs), and emerging contaminants (ECs) from municipal solid waste (MSW) Landfill. Antibiotics 10:374. doi: 10.3390/antibiotics10040374
Aworh, M. K., Abiodun-Adewusi, O., Mba, N., Helwigh, B., and Hendriksen, R. S. (2021). Prevalence and risk factors for faecal carriage of multidrug resistant Escherichia coli among slaughterhouse workers. Sci. Rep. 11:13362. doi: 10.1038/s41598-021-92819-3
Bai, H., He, L. Y., Wu, D. L., Gao, F. Z., Zhang, M., Zou, H. Y., et al. (2021). Spread of airborne antibiotic resistance from animal farms to the environment: dispersal pattern and exposure risk. Environ. Int. 158:106927. doi: 10.1016/j.envint.2021.106927
Balemi, A., Gumi, B., Amenu, K., Girma, S., Gebru, M., Tekle, M., et al. (2021). Prevalence of mastitis and antibiotic resistance of bacterial isolates from CMT positive milk samples obtained from dairy cows, camels, and goats in two pastoral districts in southern ethiopia. Animals (Basel). 11:1530. doi: 10.3390/ani11061530
Borzi, M. M., Cardozo, M. V., Oliveira, E. S., Pollo, A. S., Guastalli, E. A. L., Santos, L. F. D., et al. (2018). Characterization of avian pathogenic Escherichia coli isolated from free-range helmeted guineafowl. Braz J. Microbiol. 49(Suppl. 1), 107–112. doi: 10.1016/j.bjm.2018.04.011
Brooks, J. P., Adeli, A., and McLaughlin, M. R. (2014). Microbial ecology, bacterial pathogens, and antibiotic resistant genes in swine manure wastewater as influenced by three swine management systems. Water Res. 57, 96–103. doi: 10.1016/j.watres.2014.03.017
Callaway, T. R., Lillehoj, H., Chuanchuen, R., and Gay, C. G. (2021). Alternatives to antibiotics: a symposium on the challenges and solutions for animal health and production. Antibiotics (Basel) 10, 78–87. doi: 10.1017/s1466252313000030
Chen, Z., Xiao, T., Hu, D., Xu, J., Li, X., Jia, F., et al. (2018). The performance and membrane fouling rate of a pilot-scale anaerobic membrane bioreactor for treating antibiotic solvent wastewater under different cross flow velocity. Water Res. 135, 288–301. doi: 10.1016/j.watres.2018.02.027
Cheng, G., Hao, H., Xie, S., Wang, X., Dai, M., Huang, L., et al. (2014). Antibiotic alternatives: the substitution of antibiotics in animal husbandry? Front. Microbiol. 5:217. doi: 10.3389/fmicb.2014.00217
Chowdhury, S., Ghosh, S., Aleem, M. A., Parveen, S., Islam, M. A., Rashid, M. M., et al. (2021). Antibiotic usage and resistance in food animal production: what have we learned from Bangladesh? Antibiotics (Basel) 10:1032. doi: 10.3390/antibiotics10091032
Cornejo, J., Pokrant, E., Figueroa, F., Riquelme, R., Di Galdames, P., Pillo, F., et al. (2020). Assessing antibiotic residues in poultry eggs from backyard production systems in Chile, first approach to a non-addressed issue in farm animals. Animals (Basel) 10:1056. doi: 10.3390/ani10061056
de Jong, A., Simjee, S., Garch, F. E., Moyaert, H., Rose, M., Youala, M., et al. (2018). Antimicrobial susceptibility of enterococci recovered from healthy cattle, pigs and chickens in nine EU countries (EASSA study) to critically important antibiotics. Vet. Microbiol. 216, 168–175. doi: 10.1016/j.vetmic.2018.02.010
Deng, W., Quan, Y., Yang, S., Guo, L., Zhang, X., Liu, S., et al. (2018). Antibiotic resistance in Salmonella from retail foods of animal origin and its association with disinfectant and heavy metal resistance. Microb. Drug Resist. 24, 782–791. doi: 10.1089/mdr.2017.0127
Diaz-Jimenez, D., Garcia-Menino, I., Fernandez, J., Garcia, V., and Mora, A. (2020). Chicken and turkey meat: consumer exposure to multidrug-resistant Enterobacteriaceae including mcr-carriers, uropathogenic E. coli and high-risk lineages such as ST131. Int. J. Food Microbiol. 331:108750. doi: 10.1016/j.ijfoodmicro.2020.108750
Dierikx, C., van der Goot, J., Fabri, T., van Essen-Zandbergen, A., Smith, H., and Mevius, D. (2013). Extended-spectrum-β-lactamase- and Ampc-β-lactamase-producing Escherichia coli in dutch broilers and broiler farmers. J. Antimicrob. Chemother. 68, 60–67. doi: 10.1093/jac/dks349
Dowarah, R., Verma, A. K., and Agarwal, N. (2017). The use of Lactobacillus as an alternative of antibiotic growth promoters in pigs: a review. Anim. Nutr. 3, 1–6. doi: 10.1016/j.aninu.2016.11.002
Farha, A. K., Yang, Q.-Q., Kim, G., Li, H.-B., Zhu, F., Liu, H.-Y., et al. (2020). Tannins as an alternative to antibiotics. Food Biosci. 38:100751. doi: 10.1016/j.fbio.2020.100751
Food and Drug Administration [FDA] (2017). Drugs Prohibited for Extra-Label Use in Animals, 2017. Section 530.41 of Title 21 of the Code of Federal Regulations. Available online at: https://www.fda.gov/animal-veterinary/resources-you/ins-and-outs-extra-label-drug-use-animals-resource-veterinarians#prohibited
Food and Drug Administration [FDA] (2019). Summary Report on Antimicrobials Sold or Distributed for Use in Food-Producing Animals. Available online at: https://www.fda.gov/animal-veterinary/safety-health/antimicrobial-resistance. (accessed December 15, 2019)
Founou, L. L., Founou, R. C., and Essack, S. Y. (2016). Antibiotic resistance in the food chain: a developing country-perspective. Front. Microbiol. 7:1881. doi: 10.3389/fmicb.2016.01881
Founou, L. L., Founou, R. C., and Essack, S. Y. (2021). Antimicrobial resistance in the farm-to-plate continuum: more than a food safety issue. Futur. Sci. 2021:FSO692.
Fournier, C., Nordmann, P., Pittet, O., and Poirel, L. (2021). Does an antibiotic stewardship applied in a pig farm lead to low ESBL prevalence? Antibiotics (Basel) 10:574. doi: 10.3390/antibiotics10050574
Furlan, J. P. R., Dos Santos, L. D. R., Ramos, M. S., Gallo, I. F. L., and Stehling, E. G. (2020). Fecal cultivable aerobic microbiota of dairy cows and calves acting as reservoir of clinically relevant antimicrobial resistance genes. Braz J. Microbiol. 51, 1377–1382. doi: 10.1007/s42770-020-00265-6
Gao, X. L., Shao, M. F., Wang, Q., Wang, L. T., Fang, W. Y., Ouyang, F., et al. (2018). Airborne microbial communities in the atmospheric environment of urban hospitals in China. J. Hazard. Mater. 349, 10–17. doi: 10.1016/j.jhazmat.2018.01.043
Gebeyehu, E., Bantie, L., and Azage, M. (2015). Inappropriate use of antibiotics and its associated factors among urban and rural communities of Bahir Dar city administration, northwest ethiopia. PLoS One 10:e0138179. doi: 10.1371/journal.pone.0138179
Geser, N., and Hächler, H. (2012). Occurrence and characteristics of extended-spectrum beta-lactamase (ESBL) producing Enterobacteriaceae in food producing animals, minced meat and raw milk. BMC Vet. Res. 8:21. doi: 10.1186/1746-6148-8-21
Geta, K., and Kibret, M. (2021). Knowledge, attitudes and practices of animal farm owners/workers on antibiotic use and resistance in Amhara region, north western Ethiopia. Sci. Rep. 11:21211. doi: 10.1038/s41598-021-00617-8
Girard, M., and Bee, G. (2020). Invited review: tannins as a potential alternative to antibiotics to prevent coliform diarrhea in weaned pigs. Animal 14, 95–107. doi: 10.1017/S1751731119002143
Goulas, A., Livoreil, B., Grall, N., Benoit, P., Couderc-Obert, C., Dagot, C., et al. (2018). What are the effective solutions to control the dissemination of antibiotic resistance in the environment? A systematic review protocol. Environ. Evid. 7:3. doi: 10.1186/s13054-016-1208-6
Grant, A., Gay, C. G., and Lillehoj, H. S. (2018). Bacillus spp. as direct-fed microbial antibiotic alternatives to enhance growth, immunity, and gut health in poultry. Avian Pathol. 47, 339–351. doi: 10.1080/03079457.2018.1464117
Guo, R., Wang, Y., Li, J., Cheng, X., and Dionysiou, D. D. (2020). Sulfamethoxazole degradation by visible light assisted peroxymonosulfate process based on nanohybrid manganese dioxide incorporating ferric oxide. Appl. Catal. B Environ. 278:119297. doi: 10.1016/j.apcatb.2020.119297
Gwenzi, W., Shamsizadeh, Z., Gholipour, S., and Nikaeen, M. (2022). The air-borne antibiotic resistome: occurrence, health risks, and future directions. Sci. Total Environ. 804:150154. doi: 10.1016/j.scitotenv.2021.150154
Harnisz, M., Korzeniewska, E., and Golas, I. (2015). The impact of a freshwater fish farm on the community of tetracycline-resistant bacteria and the structure of tetracycline resistance genes in river water. Chemosphere 128, 134–141. doi: 10.1016/j.chemosphere.2015.01.035
He, Y., Yuan, Q., Mathieu, J., Stadler, L., Senehi, N., Sun, R., et al. (2020). Antibiotic resistance genes from livestock waste: occurrence, dissemination, and treatment. NPJ Clean Water 4, 1–11.
Hernandez-Gonzalez, J. C., Martinez-Tapia, A., Lazcano-Hernandez, G., Garcia-Perez, B. E., and Castrejon-Jimenez, N. S. (2021). Bacteriocins from lactic acid bacteria. a powerful alternative as antimicrobials, probiotics, and immunomodulators in veterinary medicine. Animals (Basel) 11:979. doi: 10.3390/ani11040979
Ho, Y. B., Zakaria, M. P., Latif, P. A., and Saari, N. (2013). Degradation of veterinary antibiotics and hormone during broiler manure composting. Bioresour. Technol. 131, 476–484. doi: 10.1016/j.biortech.2012.12.194
Hoppin, J. A., Umbach, D. M., Long, S., Rinsky, J. L., Henneberger, P. K., Salo, P. M., et al. (2014). Respiratory disease in united states farmers. Occup. Environ. Med. 71, 484–491. doi: 10.1136/oemed-2013-101983
Hu, D., Min, H., Wang, H., Zhao, Y., Cui, Y., Wu, P., et al. (2020). Performance of an up-flow anaerobic bio-electrochemical system (UBES) for treating sulfamethoxazole (SMX) antibiotic wastewater. Bioresour. Technol. 305:123070. doi: 10.1016/j.biortech.2020.123070
Hu, S., Cao, X., Wu, Y., Mei, X., Xu, H., Wang, Y., et al. (2018). Effects of probiotic Bacillus as an alternative of antibiotics on digestive enzymes activity and intestinal integrity of piglets. Front. Microbiol. 9:2427. doi: 10.3389/fmicb.2018.02427
Hu, Y., Liu, F., Lin, I. Y. C., Gao, G. F., and Zhu, B. (2016a). Dissemination of the mcr-1 colistin resistance gene. Lancet Infect. Dis. 16, 146–147. doi: 10.1016/s1473-3099(15)00533-2
Hu, Y., Yang, X., Li, J., Lv, N., Liu, F., Wu, J., et al. (2016b). The bacterial mobile resistome transfer network connecting the animal and human microbiomes. Appl. Environ. Microbiol. 82, 6672–6681. doi: 10.1128/AEM.01802-16
Huang, B., Jia, H., Han, X., Gou, J., Huang, C., Wang, J., et al. (2021). Effects of biocontrol Bacillus and fermentation bacteria additions on the microbial community, functions and antibiotic resistance genes of prickly ash seed oil meal-biochar compost. Bioresour. Technol. 340:125668. doi: 10.1016/j.biortech.2021.125668
Huang, J., Mi, J., Yan, Q., Wen, X., Zhou, S., Wang, Y., et al. (2021). Animal manures application increases the abundances of antibiotic resistance genes in soil-lettuce system associated with shared bacterial distributions. Sci. Total Environ. 787:147667. doi: 10.1016/j.scitotenv.2021.147667
Huang, Q., Liu, X., Zhao, G., Hu, T., and Wang, Y. (2018). Potential and challenges of tannins as an alternative to in-feed antibiotics for farm animal production. Anim. Nutr. 4, 137–150. doi: 10.1016/j.aninu.2017.09.004
Huijbers, P. M., de Kraker, M., Graat, E. A., van Hoek, A. H., van Santen, M. G., de Jong, M. C., et al. (2013). Prevalence of extended-spectrum β-lactamase-producing Enterobacteriaceae in humans living in municipalities with high and low broiler density. Clin. Microbiol. Infect. 19, E256–E259. doi: 10.1111/1469-0691.12150
Huijbers, P. M., Graat, E. A., Haenen, A. P., van Santen, M. G., van Essen-Zandbergen, A., Mevius, D. J., et al. (2014). Extended-spectrum and Ampc β-lactamase-producing Escherichia coli in broilers and people living and/or working on broiler farms: prevalence, risk factors and molecular characteristics. J. Antimicrob. Chemother. 69, 2669–2675. doi: 10.1093/jac/dku178
Huijbers, P. M., van Hoek, A. H., Graat, E. A., Haenen, A. P., Florijn, A., Hengeveld, P. D., et al. (2015). Methicillin-resistant Staphylococcus aureus and extended-spectrum and Ampc β-lactamase-producing Escherichia coli in broilers and in people living and/or working on organic broiler farms. Vet. Microbiol. 176, 120–125. doi: 10.1016/j.vetmic.2014.12.010
Jeżak, K., and Kozajda, A. (2022). Occurrence and spread of antibiotic-resistant bacteria on animal farms and in their vicinity in Poland and Ukraine—review. Environ. Sci. Pollut. Res. Int. 29, 9533–9559. doi: 10.1007/s11356-021-17773-z
Jibril, A. H., Okeke, I. N., Dalsgaard, A., and Olsen, J. E. (2021). Association between antimicrobial usage and resistance in Salmonella from poultry farms in nigeria. BMC Vet. Res. 17:234. doi: 10.1186/s12917-021-02938-2
Khan, M. I., Ahhmed, A., Shin, J. H., Baek, J. S., Kim, M. Y., and Kim, J. D. (2018). Green tea seed isolated saponins exerts antibacterial effects against various strains of Gram positive and Gram negative bacteria, a comprehensive study in vitro and in vivo. Evid Based Compl. Alt. Med. 26:3486106. doi: 10.1155/2018/3486106
Khan, S. A., Imtiaz, M. A., Sayeed, M. A., Shaikat, A. H., and Hassan, M. M. (2020). Antimicrobial resistance pattern in domestic animal - wildlife - environmental niche via the food chain to humans with a bangladesh perspective; a systematic review. BMC Vet. Res. 16:302. doi: 10.1186/s12917-020-02519-9
Kim, K.-R., Owens, G., Kwon, S.-I., So, K.-H., Lee, D.-B., and Ok, Y. S. (2010). Occurrence and environmental fate of veterinary antibiotics in the terrestrial environment. Water Air Soil Poll. 214, 163–174. doi: 10.1007/s11270-010-0412-2
Koch, B. J., Hungate, B. A., and Price, L. B. (2017). Food-animal production and the spread of antibiotic resistance: the role of ecology. Front. Ecol. Environ. 15:309–318. doi: 10.1002/fee.1505
Larsson, D. G. J., and Flach, C.-F. (2022). Antibiotic resistance in the environment. Nat. Rev. Microbiol. 20, 257–269. doi: 10.1038/s41579-021-00649-x
Lee, C. R., Cho, I. H., Jeong, B. C., and Lee, S. H. (2013). Strategies to minimize antibiotic resistance. Int. J. Environ. Res. Public Health. 10, 4274–4305. doi: 10.3390/ijerph10094274
Lekagul, A., Tangcharoensathien, V., Mills, A., Rushton, J., and Yeung, S. (2020). How antibiotics are used in pig farming: a mixed-methods study of pig farmers, feed mills and veterinarians in thailand. BMJ Glob. Health. 5:e001918. doi: 10.1136/bmjgh-2019-001918
Li, C., Li, Y., Li, X., Ma, X., Ru, S., Qiu, T., et al. (2021). Veterinary antibiotics and estrogen hormones in manures from concentrated animal feedlots and their potential ecological risks. Environ. Res. 198:110463. doi: 10.1016/j.envres.2020.110463
Li, J., Zhou, L., Zhang, X., Xu, C., Dong, L., and Yao, M. (2016). Bioaerosol emissions and detection of airborne antibiotic resistance genes from a wastewater treatment plant. Atmos. Environ. 124, 404–412. doi: 10.1016/j.atmosenv.2015.06.030
Li, S., Jiang, J., Ho, S.-H., Li, F., and Zeng, W. (2021). Bimetallic nitrogen-doped porous carbon derived from ZIF-L&FeTPP@ZIF-8 as electrocatalysis and application for antibiotic wastewater treatment. Sep. Purif. Technol. 276:119259. doi: 10.1016/j.seppur.2021.119259
Liao, H., Bai, Y., Liu, C., Wen, C., Yang, Q., Chen, Z., et al. (2021). Airborne and indigenous microbiomes co-drive the rebound of antibiotic resistome during compost storage. Environ. Microbiol. 23, 7483–7496. doi: 10.1111/1462-2920.15672
Liao, H., Lu, X., Rensing, C., Friman, V. P., Geisen, S., Chen, Z., et al. (2018). Hyperthermophilic composting accelerates the removal of antibiotic resistance genes and mobile genetic elements in sewage sludge. Environ. Sci. Technol. 52, 266–276. doi: 10.1021/acs.est.7b04483
Lima, T., Domingues, S., and Da Silva, G. J. (2020). Manure as a potential hotspot for antibiotic resistance dissemination by horizontal gene transfer events. Vet. Sci. 7:110. doi: 10.3390/vetsci7030110
Liu, F., Zhang, R., Yang, Y., Li, H., Wang, J., Lan, J., et al. (2020). Occurrence and molecular characteristics of mcr-1-positive Escherichia coli from healthy meat ducks in shandong province of China. Animals (Basel) 10:1299. doi: 10.3390/ani10081299
Liu, K., Han, J., Li, S., Liu, L., Lin, W., and Luo, J. (2019). Insight into the diversity of antibiotic resistance genes in the intestinal bacteria of shrimp Penaeus vannamei by culture-dependent and independent approaches. Ecotox. Environ. Safe. 172, 451–459. doi: 10.1016/j.ecoenv.2019.01.109
Liu, L., Liu, C., Zheng, J., Huang, X., Wang, Z., Liu, Y., et al. (2013). Elimination of veterinary antibiotics and antibiotic resistance genes from swine wastewater in the vertical flow constructed wetlands. Chemosphere 91, 1088–1093. doi: 10.1016/j.chemosphere.2013.01.007
Liu, Y., Zheng, L., Cai, Q., Xu, Y., Xie, Z., Liu, J., et al. (2021). Simultaneous reduction of antibiotics and antibiotic resistance genes in pig manure using a composting process with a novel microbial agent. Ecotox. Environ. Safe. 208:111724. doi: 10.1016/j.ecoenv.2020.111724
Liu, Z. H., Wang, K., Zhang, Y. R., Xia, L. N., Zhao, L., Guo, C. M., et al. (2022). High prevalence and diversity characteristics of blaNDM, mcr, and blaESBLs harboring multidrug-resistant Escherichia coli from chicken, pig, and cattle in China. Front. Cell. Infect. Microbiol. 11:755545. doi: 10.3389/fcimb.2021.755545
Lu, X. M., and Lu, P. Z. (2019). Synergistic effects of key parameters on the fate of antibiotic resistance genes during swine manure composting. Environ. Pollut. 252, 1277–1287. doi: 10.1016/j.envpol.2019.06.073
Ma, X., Yang, Z., Xu, T., Qian, M., Jiang, X., Zhan, X., et al. (2021). Chlortetracycline alters microbiota of gut or faeces in pigs and leads to accumulation and migration of antibiotic resistance genes. Sci. Total Environ. 796:148976. doi: 10.1016/j.scitotenv.2021.148976
Mackie, R. I., Koike, S., Krapac, I., Chee-Sanford, J., Maxwell, S., and Aminov, R. I. (2006). Tetracycline residues and tetracycline resistance genes in groundwater impacted by swine production facilities. Anim. Biotechnol. 17, 157–176. doi: 10.1080/10495390600956953
Magrys, A., Olender, A., and Tchorzewska, D. (2021). Antibacterial properties of Allium sativum L. against the most emerging multidrug-resistant bacteria and its synergy with antibiotics. Arch Microbiol. 203, 2257–2268. doi: 10.1007/s00203-021-02248-z
Martin, M. J., Thottathil, S. E., and Newman, T. B. (2015). Antibiotics overuse in animal agriculture: a call to action for health care providers. Am. J. Public Health. 105, 2409–2410. doi: 10.2105/AJPH.2015.302870
McCartney, D., and Tingley, J. (1998). Development of a rapid moisture content method for compost materials. Compost Sci. Util. 6, 14–25. doi: 10.1007/s10661-011-2423-x
McDaniel, C. J., Cardwell, D. M., Moeller, R. B. Jr., and Gray, G. C. (2014). Humans and cattle: a review of bovine zoonoses. Vector Borne Zoonot. Dis. 14, 1–19. doi: 10.1089/vbz.2012.1164
McEachran, A. D., Blackwell, B. R., Hanson, J. D., Wooten, K. J., Mayer, G. D., Cox, S. B., et al. (2015). Antibiotics, bacteria, and antibiotic resistance genes: aerial transport from cattle feed yards via particulate matter. Environ. Health Persp. 123, 337–343. doi: 10.1289/ehp.1408555
Meijs, A. P., Gijsbers, E. F., Hengeveld, P. D., Dierikx, C. M., de Greeff, S. C., and van Duijkeren, E. (2021). ESBL/pAmpc-producing Escherichia coli and Klebsiella pneumoniae carriage among veterinary healthcare workers in the Netherlands. Antimicrob. Resist. Infect Control. 10:147. doi: 10.1186/s13756-021-01012-8
Meng, L., Wang, J., Li, X., and Cui, F. (2020). Insight into effect of high-level cephalexin on fate and driver mechanism of antibiotics resistance genes in antibiotic wastewater treatment system. Ecotox. Environ. Safe. 201:110739. doi: 10.1016/j.ecoenv.2020.110739
Meng, L., Wang, J., Li, X., Yu, Y., and Zhu, Y. (2021). Microbial community and molecular ecological network in the EGSB reactor treating antibiotic wastewater: response to environmental factors. Ecotox. Environ. Safe. 208:111669. doi: 10.1016/j.ecoenv.2020.111669
Menz, J., Olsson, O., and Kummerer, K. (2019). Antibiotic residues in livestock manure: does the EU risk assessment sufficiently protect against microbial toxicity and selection of resistant bacteria in the environment? J. Hazard. Mater. 379:120807. doi: 10.1016/j.jhazmat.2019.120807
Mingmongkolchai, S., and Panbangred, W. (2018). Bacillus probiotics: an alternative to antibiotics for livestock production. J. Appl. Microbiol. 124, 1334–1346. doi: 10.1111/jam.13690
Mitchell, S. M., Ullman, J. L., Bary, A., Cogger, C. G., Teel, A. L., and Watts, R. J. (2015). Antibiotic degradation during thermophilic composting. Water Air Soil Pollut. 226:13.
Musliu, A., Sulaimon, A., and Arzika, S. (2021). Evaluation of antibiotic potential of crude culture extract of lactic acid bacteria on multi-drug resistance farm animal pathogens. Adv. Biosci. Bioengin. 9, 20–24. doi: 10.11648/j.abb.20210902.11
National Research Council [NRC] (1980). The Effects on Human Health of Subtherapeutic Use of Antimicrobials in Animal Feeds. Washington, DC: The National Academies Press (US).
Navidshad, B., Darabighane, B., and Malecky, M. (2018). Garlic: an alternative to antibiotics in poultry production, a review. Iran. J. Appl. Anim. Sci. 8, 9–17.
Nesporova, K., Valcek, A., Papagiannitsis, C., Kutilova, I., Jamborova, I., Davidova-Gerzova, L., et al. (2021). Multi-drug resistant plasmids with ESBL/Ampc and mcr-5.1 in paraguayan poultry farms: the linkage of antibiotic resistance and hatcheries. Microorganisms 9:866. doi: 10.3390/microorganisms9040866
Nilsson, O. (2012). Vancomycin resistant enterococci in farm animals - occurrence and importance. Infect. Ecol. Epidemiol. 2:16959. doi: 10.3402/iee.v2i0.16959
Nuangmek, A., Patchanee, P., Yano, T., Yamsakul, P., Chotinun, S., and Tadee, P. (2018). Knowledge, attitudes and practices toward antimicrobial usage: a cross-sectional study of layer and pig farm owners/managers in chiang mai, lamphun, and chonburi provinces, thailand, may 2014 to february 2016. Korean J. Vet. Res. 58, 17–25. doi: 10.14405/kjvr.2018.58.1.17
Overdevest, I., Willemsen, I., Rijnsburger, M., Eustace, A., Xu, L., Hawkey, P., et al. (2011). Extended-spectrum β-lactamase genes of Escherichia coli in chicken meat and humans, the netherlands. Emerg. Infect. Dis. 17, 1216–1222. doi: 10.3201/eid1707.110209
Oviedo-Bolaños, K., Rodríguez-Rodríguez, J. A., Sancho-Blanco, C., Barquero-Chanto, J. E., Peña-Navarro, N., Escobedo-Bonilla, C. M., et al. (2021). Molecular identification of Streptococcus sp. and antibiotic resistance genes present in Tilapia farms (Oreochromis niloticus) from the northern pacific region, costa rica. Aquacul. Int. 29, 2337–2355. doi: 10.1007/s10499-021-00751-0
Ozturk, Y., Celik, S., Sahin, E., Acik, M. N., and Cetinkaya, B. (2019). assessment of farmers’ knowledge, attitudes and practices on antibiotics and antimicrobial resistance. Animals 9:653. doi: 10.3390/ani9090653
Patyra, E., Kwiatek, K., Nebot, C., and Gavilan, R. E. (2020). Quantification of veterinary antibiotics in pig and poultry feces and liquid manure as a non-invasive method to monitor antibiotic usage in livestock by liquid chromatography mass-spectrometry. Molecules 25:3265. doi: 10.3390/molecules25143265
Peng, P., Huang, H., and Ren, H. (2018). Effect of adding low-concentration of rhamnolipid on reactor performances and microbial community evolution in MBBRs for low C/N ratio and antibiotic wastewater treatment. Bioresour. Technol. 256, 557–561. doi: 10.1016/j.biortech.2018.02.035
Qiao, M., Ying, G. G., Singer, A. C., and Zhu, Y. G. (2018). Review of antibiotic resistance in china and its environment. Environ. Int. 110, 160–172. doi: 10.1016/j.envint.2017.10.016
Radon, K., Danuser, B., Iversen, M., Jrres, R., and Nowak, D. (2001). Respiratory symptoms in european animal farmers. Eur. Respir. 17, 747–754. doi: 10.1183/09031936.01.17407470
Raghavan, D., Qiu, G., and Ting, Y. P. (2018). Fate and removal of selected antibiotics in an osmotic membrane bioreactor. Chem. Eng. J. 334, 198–205. doi: 10.1016/j.cej.2017.10.026
Ramlucken, U., Roets, Y., Ramchuran, S. O., Moonsamy, G., van Rensburg, C. J., Thantsha, M. S., et al. (2020). Isolation, selection and evaluation of bacillus spp. as potential multi-mode probiotics for poultry. J. Gen. Appl. Microbiol. 66, 228–238. doi: 10.2323/jgam.2019.11.002
Ranjan, R., and Thatikonda, S. (2021). β-Lactam resistance gene ndm-1 in the aquatic environment: a review. Curr. Microbiol. 78, 3634–3643. doi: 10.1007/s00284-021-02630-6
Redondo, L. M., Chacana, P. A., Dominguez, J. E., and Fernandez Miyakawa, M. E. (2014). Perspectives in the use of tannins as alternative to antimicrobial growth promoter factors in poultry. Front. Microbiol. 5:118. doi: 10.3389/fmicb.2014.00118
Ruuskanen, M., Muurinen, J., Meierjohan, A., Parnanen, K., Tamminen, M., Lyra, C., et al. (2016). Fertilizing with animal manure disseminates antibiotic resistance genes to the farm environment. J. Environ. Qual. 45, 488–493. doi: 10.2134/jeq2015.05.0250
Salim, H. M., Huque, K. S., and Kamaruddin, K. M. (2018). Global restriction of using antibiotic growth promoters and alternative strategies in poultry production. Sci. Prog. 101, 52–75. doi: 10.3184/003685018X15173975498947
Saliu, E. M., Vahjen, W., and Zentek, J. (2017). Types and prevalence of extended-spectrum beta-lactamase producing Enterobacteriaceae in poultry. Anim. Health Res. Rev. 18, 46–57. doi: 10.1017/s1466252317000020
Sancheza, H. M., Echeverria, C., Thulsiraj, V., Zimmer-Faust, A., Flores, A., Laitz, M., et al. (2016). Antibiotic resistance in airborne bacteria near conventional and organic beef cattle farms in california, USA. Water Air Soil Pollut. 227:280.
Sazykin, I. S., Khmelevtsova, L. E., Seliverstova, E. Y., and Sazykina, M. A. (2021). Effect of antibiotics used in animal husbandry on the distribution of bacterial drug resistance (review). Appl. Biochem. Microbiol. 57, 20–30. doi: 10.1134/s0003683821010166
Schueler, J., Lansing, S., Crossette, E., Naas, K., Hurst, J., Raskin, L., et al. (2021). Tetracycline, sulfadimethoxine, and antibiotic resistance gene dynamics during anaerobic digestion of dairy manure. J. Environ. Qual. 50, 694–705. doi: 10.1002/jeq2.20211
Sharma, G., Mutua, F., Deka, R. P., Shome, R., Bandyopadhyay, S., Shome, B. R., et al. (2020). A qualitative study on antibiotic use and animal health management in smallholder dairy farms of four regions of India. Infect. Ecol. Epidemiol. 10:1792033. doi: 10.1080/20008686.2020.1792033
Shi, X., Yeap, T. S., Huang, S., Chen, J., and Ng, H. Y. (2018). Pretreatment of saline antibiotic wastewater using marine microalga. Bioresour. Technol. 258, 240–246. doi: 10.1016/j.biortech.2018.02.110
Sigsgaard, T., Basinas, I., Doekes, G., de Blay, F., Folletti, I., Heederik, D., et al. (2020). Respiratory diseases and allergy in farmers working with livestock: a EAACI position paper. Clin. Transl. Allergy. 10:29. doi: 10.1186/s13601-020-00334-x
Sirichokchatchawan, W., Apiwatsiri, P., Pupa, P., Saenkankam, I., Khine, N. O., Lekagul, A., et al. (2021). Reducing the risk of transmission of critical antimicrobial resistance determinants from contaminated pork products to humans in south-east Asia. Front. Microbiol. 12:689015. doi: 10.3389/fmicb.2021.689015
Song, L., Wang, C., Jiang, G., Ma, J., Li, Y., Chen, H., et al. (2021). Bioaerosol is an important transmission route of antibiotic resistance genes in pig farms. Environ. Int. 154:106559. doi: 10.1016/j.envint.2021.106559
Stefanska, I., Kwiecien, E., Jozwiak-Piasecka, K., Garbowska, M., Binek, M., and Rzewuska, M. (2021). Antimicrobial susceptibility of lactic acid bacteria strains of potential use as feed additives - the basic safety and usefulness criterion. Front. Vet. Sci. 8:687071. doi: 10.3389/fvets.2021.687071
Si Heung, S., Kim, K. H., Jeon, B. T., Cheong, S. H., and Kim, D. H. (2012). Antibacterial and antioxidant activities of tannins extracted from agricultural by-products. J. Med. Plants Res. 6, 3072–3079.
Tang, K. L., Caffrey, N. P., Nóbrega, D. B., Cork, S. C., Ronksley, P. E., Barkema, H. W., et al. (2017). Restricting the use of antibiotics in food-producing animals and its associations with antibiotic resistance in food-producing animals and human beings: a systematic review and meta-analysis. Lancet Planet. Health. 1, e316–e327. doi: 10.1016/S2542-5196(17)30141-9
Tao, C. W., Hsu, B. M., Ji, W. T., Hsu, T. K., Kao, P. M., Hsu, C. P., et al. (2014). Evaluation of five antibiotic resistance genes in wastewater treatment systems of swine farms by real-time PCR. Sci. Total Environ. 496, 116–121. doi: 10.1016/j.scitotenv.2014.07.024
Tian, X., Han, B., Liang, J., Yang, F., and Zhang, K. (2021). Tracking antibiotic resistance genes (ARGs) during earthworm conversion of cow dung in northern China. Ecotox. Environ. Safe. 222:112538. doi: 10.1016/j.ecoenv.2021.112538
Tiseo, K., Huber, L., Gilbert, M., Robinson, T. P., and Van Boeckel, T. P. (2020). Global trends in antimicrobial use in food animals from 2017 to 2030. Antibiotics (Basel) 9:918. doi: 10.3390/antibiotics9120918
Tomley, F. M., and Shirley, M. W. (2009). Livestock infectious diseases and zoonoses. Phil. Trans. R. Soc. B. 364, 2637–2642. doi: 10.1098/rstb.2009.0133
Ungemach, F. R., Muller-Bahrdt, D., and Abraham, G. (2006). Guidelines for prudent use of antimicrobials and their implications on antibiotic usage in veterinary medicine. Int. J. Med. Microbiol. 296(Suppl. 41), 33–38. doi: 10.1016/j.ijmm.2006.01.059
Van Epps, A., and Blaney, L. (2016). Antibiotic residues in animal waste: occurrence and degradation in conventional agricultural waste management practices. Curr. Pollut. Rep. 2, 135–155. doi: 10.1007/s40726-016-0037-1
Van Leuken, J. P. G., Swart, A. N., Havelaar, A. H., Van Pul, A., Van der Hoek, W., and Heederik, D. (2016). Atmospheric dispersion modelling of bioaerosols that are pathogenic to humans and livestock - a review to inform risk assessment studies. Microbiol. Risk Anal. 1, 19–39. doi: 10.1016/j.mran.2015.07.002
Van, T. T. H., Yidana, Z., Smooker, P. M., and Coloe, P. J. (2020). Antibiotic use in food animals worldwide, with a focus on africa: pluses and minuses. J. Glob. Antimicrob. Resist. 20, 170–177. doi: 10.1016/j.jgar.2019.07.031
Vines, J., Cusco, A., Napp, S., Alvarez, J., Saez-Llorente, J. L., Rosas-Rodoreda, M., et al. (2021). Transmission of similar mcr-1 carrying plasmids among different Escherichia coli lineages isolated from livestock and the farmer. Antibiotics (Basel) 10:313. doi: 10.3390/antibiotics10030313
Visca, A., Barra Caracciolo, A., Grenni, P., Patrolecco, L., Rauseo, J., Massini, G., et al. (2021). Anaerobic digestion and removal of sulfamethoxazole, enrofloxacin, ciprofloxacin and their antibiotic resistance genes in a full-scale biogas plant. Antibiotics (Basel) 10:502. doi: 10.3390/antibiotics10050502
Wang, J., Yang, Z., Wang, H., Wu, S., Lu, H., and Wang, X. (2021). Decomposition process of cefotaxime sodium from antibiotic wastewater by up-flow blanket filter (UBF) reactor: reactor performance, sludge characteristics and microbial community structure analysis. Sci. Total Environ. 758:143670. doi: 10.1016/j.scitotenv.2020.143670
Wang, W., Wei, X., Wu, L., Shang, X., Cheng, F., Li, B., et al. (2021). The occurrence of antibiotic resistance genes in the microbiota of yak, beef and dairy cattle characterized by a metagenomic approach. J. Antibiot. 74, 508–518. doi: 10.1038/s41429-021-00425-2
Wang, X. R., Lian, X. L., Su, T. T., Long, T. F., Li, M. Y., Feng, X. Y., et al. (2021). Duck wastes as a potential reservoir of novel antibiotic resistance genes. Sci. Total Environ. 771:144828. doi: 10.1016/j.scitotenv.2020.144828
Wang, Y., Lyu, N., Liu, F., Liu, W. J., Bi, Y., Zhang, Z., et al. (2021). More diversified antibiotic resistance genes in chickens and workers of the live poultry markets. Environ. Int. 153:106534. doi: 10.1016/j.envint.2021.106534
Wen, Q., Yang, L., Zhao, Y., Huang, L., and Chen, Z. (2018). Insight into effects of antibiotics on reactor performance and evolutions of antibiotic resistance genes and microbial community in a membrane reactor. Chemosphere 197, 420–429. doi: 10.1016/j.chemosphere.2018.01.067
West, B. M., Liggit, P., Clemans, D. L., and Francoeur, S. N. (2010). Antibiotic resistance, gene transfer, and water quality patterns observed in waterways near CAFO farms and wastewater treatment facilities. Water Air Soil Pollut. 217, 473–489. doi: 10.1007/s11270-010-0602-y
Wichmann, F., Udikovic-Kolic, N., Andrew, S., and Handelsman, J. (2014). Diverse antibiotic resistance genes in dairy cow manure. mBio 5:e01017. doi: 10.1128/mBio.01017-13
Xie, W. Y., Shen, Q., and Zhao, F. J. (2017). Antibiotics and antibiotic resistance from animal manures to soil: a review. Eur. J. Soil Sci. 69, 181–195. doi: 10.1111/ejss.12494
Yamaji, R., Friedman, C. R., Rubin, J., Suh, J., Thys, E., McDermott, P., et al. (2018). A population-based surveillance study of shared genotypes of Escherichia coli isolates from retail meat and suspected cases of urinary tract infections. mSphere 3, e179–e118. doi: 10.1128/mSphere.00179-18
Yan, H., Li, Y., Zhang, Y., Zhang, H., Guo, Z., and Liu, J. (2021). Deciphering of microbial diversity and antibiotic resistome of bioaerosols in swine confinement buildings. Sci. Total Environ. 781:147056. doi: 10.1016/j.scitotenv.2021.147056
Yang, F., Zhang, K., Zhi, S., Li, J., Tian, X., Gu, Y., et al. (2019). High prevalence and dissemination of β-lactamase genes in swine farms in northern China. Sci. Total Environ. 651, 2507–2513. doi: 10.1016/j.scitotenv.2018.10.144
Yang, L., Shen, Y. B., Jiang, J. Y., Wang, X. Y., Shao, D. Y., Lam, M. M. C., et al. (2022). Distinct increase in antimicrobial resistance genes among Escherichia coli during 50 years of antimicrobial use in livestock production in China. Nat. Food. 3, 197–205. doi: 10.1038/s43016-022-00470-6
Yang, Y., Liu, Z., Xing, S., and Liao, X. (2019). The correlation between antibiotic resistance gene abundance and microbial community resistance in pig farm wastewater and surrounding rivers. Ecotox. Environ. Safe. 182:109452. doi: 10.1016/j.ecoenv.2019.109452
Yu, W., Zhan, S., Shen, Z., Zhou, Q., and Yang, D. (2017). Efficient removal mechanism for antibiotic resistance genes from aquatic environments by graphene oxide nanosheet. Chem. Eng. J. 313, 836–846. doi: 10.1016/j.cej.2016.10.107
Zalewska, M., Blazejewska, A., Czapko, A., and Popowska, M. (2021). Antibiotics and antibiotic resistance genes in animal manure - consequences of its application in agriculture. Front. Microbiol. 12:610656. doi: 10.3389/fmicb.2021.610656
Zhang, J., Lu, T., Chai, Y., Sui, Q., Shen, P., and Wei, Y. (2019). Which animal type contributes the most to the emission of antibiotic resistance genes in large-scale swine farms in China? Sci. Total Environ. 658, 152–159. doi: 10.1016/j.scitotenv.2018.12.175
Zhang, M., He, L. Y., Liu, Y. S., Zhao, J. L., Liu, W. R., Zhang, J. N., et al. (2019). Fate of veterinary antibiotics during animal manure composting. Sci. Total Environ. 650, 1363–1370. doi: 10.1016/j.scitotenv.2018.09.147
Zhang, Q. Q., Ying, G. G., Pan, C. G., Liu, Y. S., and Zhao, J. L. (2015). Comprehensive evaluation of antibiotics emission and fate in the river basins of China: source analysis, multimedia modeling, and linkage to bacterial resistance. Environ. Sci. Technol. 49, 6772–6782. doi: 10.1021/acs.est.5b00729
Zhang, X., Ma, C., Zhang, W., Li, W., Yu, J., Xue, D., et al. (2020). Shifts in microbial community, pathogenicity-related genes and antibiotic resistance genes during dairy manure piled up. Microb. Biotechnol. 13, 1039–1053. doi: 10.1111/1751-7915.13551
Zhao, J., Li, B., Lv, P., Hou, J., Qiu, Y., and Huang, X. (2022). Distribution of antibiotic resistance genes and their association with bacteria and viruses in decentralized sewage treatment facilities. Front. Environ. Sci. Eng. 16:35. doi: 10.1007/s11783-021-1469-4
Zhou, J., Ding, J., Wan, H., and Guan, G. (2021). Boosting photocatalytic degradation of antibiotic wastewater by synergy effect of heterojunction and phosphorus doping. J. Colloid Interf. Sci. 582, 961–968. doi: 10.1016/j.jcis.2020.08.099
Zhou, J., Yun, X., Wang, J. T., Li, Q., and Wang, Y. L. (2022). A review on the ecotoxicological effect of sulphonamides on aquatic organisms. Toxicol. Rep. 9, 534–540. doi: 10.1016/j.toxrep.2022.03.034
Zhou, L. J., Ying, G. G., Liu, S., Zhang, R. Q., Lai, H. J., Chen, Z. F., et al. (2013a). Excretion masses and environmental occurrence of antibiotics in typical swine and dairy cattle farms in China. Sci. Total Environ. 444, 183–195. doi: 10.1016/j.scitotenv.2012.11.087
Zhou, L. J., Ying, G. G., Zhang, R. Q., Liu, S., Lai, H. J., Chen, Z. F., et al. (2013b). Use patterns, excretion masses and contamination profiles of antibiotics in a typical swine farm, south China. Environ. Sci. Proc. Imp. 15, 802–813. doi: 10.1039/c3em30682h
Zhu, Z., Cao, M., Wang, W., Zhang, L., Ma, T., Liu, G., et al. (2020). Exploring the prevalence and distribution patterns of antibiotic resistance genes in bovine gut microbiota using a metagenomic approach. Microb. Drug Resist. 27, 980–990. doi: 10.1089/mdr.2020.0271
Keywords: antibiotics, bacterial resistance, food animals, alternatives, strategies
Citation: Xu C, Kong L, Gao H, Cheng X and Wang X (2022) A Review of Current Bacterial Resistance to Antibiotics in Food Animals. Front. Microbiol. 13:822689. doi: 10.3389/fmicb.2022.822689
Received: 26 November 2021; Accepted: 04 April 2022;
Published: 12 May 2022.
Edited by:
András Fodor, University of Szeged, HungaryReviewed by:
Pardeep Singh, University of Delhi, IndiaEtih Sudarnika, Bogor Agricultural University, Indonesia
Copyright © 2022 Xu, Kong, Gao, Cheng and Wang. This is an open-access article distributed under the terms of the Creative Commons Attribution License (CC BY). The use, distribution or reproduction in other forums is permitted, provided the original author(s) and the copyright owner(s) are credited and that the original publication in this journal is cited, in accordance with accepted academic practice. No use, distribution or reproduction is permitted which does not comply with these terms.
*Correspondence: Xiumin Wang, wangxiumin@caas.cn
†These authors have contributed equally to this work