- Institut de Ciències del Mar, CSIC, Barcelona, Spain
Proper thermal adaptation is key to understanding how species respond to long-term changes in temperature. However, this is seldom considered in protozooplankton and mixoplankton experiments. In this work, we studied how two heterotrophic dinoflagellates (Gyrodinium dominans and Oxyrrhis marina), one heterotrophic ciliate (Strombidium arenicola), and one mixotrophic dinoflagellate (Karlodinium armiger) responded to warming. To do so, we compared strains adapted at 16, 19, and 22°C and those adapted at 16°C and exposed for 3 days to temperature increases of 3 and 6°C (acclimated treatments). Neither their carbon, nitrogen or phosphorus (CNP) contents nor their corresponding elemental ratios showed straightforward changes with temperature, except for a modest increase in P contents with temperature in some grazers. In general, the performance of both acclimated and adapted grazers increased from 16 to 19°C and then dropped at 22°C, with a few exceptions. Therefore, our organisms followed the “hotter is better” hypothesis for a temperature rise of 3°C; an increase of >6°C, however, resulted in variable outcomes. Despite the disparity in responses among species and physiological rates, 19°C-adapted organisms, in general, performed better than acclimated-only (16°C-adapted organisms incubated at +3°C). However, at 22°C, most species were at the limit of their metabolic equilibrium and were unable to fully adapt. Nevertheless, adaptation to higher temperatures allowed strains to maintain physiological activities when exposed to sudden increases in temperature (up to 25°C). In summary, adaptation to temperature seems to confer a selective advantage to protistan grazers within a narrow range (i.e., ca. 3°C). Adaptation to much higher increases of temperatures (i.e., +6°C) does not confer any clear physiological advantage (with few exceptions; e.g., the mixotroph K. armiger), at least within the time frame of our experiments.
Introduction
The progressive increase of temperature due to anthropogenic sources (Intergovernmental Panel on Climate Change [IPCC], 2014) will certainly affect planktonic communities in the coming future. This is because temperature is a major factor driving biological activity; the resulting changes in the fitness of a species in response to temperature may provide a selective advantage/disadvantage in comparison to other coexisting species (Halsband-Lenk et al., 2002). This rule applies to all marine organisms, including protistan grazers, such as microzooplankton (pure heterotrophic protists) and mixoplankton (autotrophic protists with phagotrophic capability). Both groups of protistan grazers, which encompass many ciliates and dinoflagellates, are key components of marine pelagic food webs because of their functions as major grazers of phytoplanktonic primary production and as very important prey for larger zooplankton (Calbet, 2001; Calbet and Saiz, 2005; Flynn et al., 2019; Traboni et al., 2021).
Despite the relevance of temperature, most ecophysiological studies addressing its direct effects on protistan grazers do not include multigenerational effects and typically only address short-term responses to variations in environmental temperature [see review by Montagnes et al. (2003)]. Therefore, the actual response of protist grazers to gradual and longer-term temperature changes in marine systems remains essentially unexplored. Conversely, long-term adaptations to temperature have been studied in planktonic algae, and the results have been very revealing and occasionally opposite to those that were expected. For instance, the accepted faster thermal response of respiration vs. photosynthesis, predicted by the metabolic theory of ecology (López-Urrutia et al., 2006; Rose and Caron, 2007), may not be the same after proper adaptation (>100 generations) to environmental conditions (Padfield et al., 2016; Barton et al., 2020). This is because basal metabolism diminishes after genetic adaptation to temperature (Padfield et al., 2016; Barton et al., 2020). Other observed changes in temperature-adapted species are stoichiometric (lower C:N ratios) and related to more efficient use of carbon (Padfield et al., 2016; Aranguren-Gassis et al., 2019). The elemental composition of an organisms is the result of the balance between its metabolic demands and the relative supply of elements in the environment (Sterner and Elser, 2002). It is assumed that phytoplankton exhibit wide variations in their elemental composition and protozoans are more homoeostatic, showing a narrower range of variation (Sterner and Elser, 2002; Klausmeier et al., 2004). However, several studies have shown that the elemental stoichiometry of protozoans may vary significantly in response to the environment and prey composition (Hantzsche and Boersma, 2010; Malzahn et al., 2010; Meunier et al., 2012), and may affect the energy transfer to upper trophic levels (Traboni et al., 2021). The incorporation of elemental ratios and absolute elemental contents (dependent on cellular volume) of different planktonic groups into ecosystem models could improve our ability to predict the response of planktonic communities to environmental threats, and help to understand their influence on the biogeochemistry of the ocean (Litchman et al., 2013; Meunier et al., 2017). Little is known, however, about protistan grazers, both mixotrophic and heterotrophic, in this respect.
We hypothesize that because of the different activation energies between physiological processes, a rise in temperature will favor phagotrophy over photosynthetic carbon acquisition in mixotrophs (Wilken et al., 2013; Lin et al., 2018). We could also hypothesize that following the von Bertalanffy-Perrin model, which states that catabolism is more affected by temperature than anabolism (Perrin, 1995; Li et al., 2011), higher temperatures will enhance respiratory losses to a larger extent than ingestion in protozooplankton (at least for short-term responses). This imbalance should result in a reduction of gross growth efficiency (GGE; Straile, 1997; Li et al., 2011) and perhaps in lower cellular C:N and C:P ratios, depending on the temperature-sensitivity of the excretion response (Alcaraz et al., 2013). We cannot discard, however, that fully temperature-adapted species may not show such differences, as previously reported for algae (Padfield et al., 2016; Barton et al., 2020). This hypothesis, if true, could have very relevant consequences for understanding the functioning of the marine food web and for biochemical modeling ecosystems.
In this study, we aim to explore the responses (volume, stoichiometry growth, and grazing) of different protistan grazers to temperature rise after a short-term exposure (acclimation) and compare them to the performances of long-term adapted organisms. By working with temperature-adapted species, we will also be able to test whether protistan grazers follow the “hotter is better hypothesis” (Bennett, 1987), which predicts that organisms adapted to lower temperatures will have lower maximum performances than those adapted to higher temperatures (Knies et al., 2009). Finally, we also aim to investigate the response of the strains adapted to different temperatures to an extreme temperature episode, here represented by a sudden increase in temperature to 25°C. Heatwaves may become a relevant instrument of species selection in a future scenario of a warmer ocean, where many species will likely be at the edge of their physiological limits. The reported increases of temperature during heatwaves do not reach >6.5°C (Hobday et al., 2016). However, we wanted to go one step further and expose the experimental organisms to relatively extremer temperatures (up to +9°C), to evaluate their survival and performance under critical conditions, and how temperature-adaptation would modify this response. Overall, understanding the processes involved in the thermal adaptation of protozooplankton and mixoplankton and their response to warming will improve the accuracy of climate change models in predicting ecological or biogeochemical effects of temperature projections in the near future.
Materials and Methods
Experimental Organisms
For the experiments, we used cultures of two heterotrophic dinoflagellates (Gyrodinium dominans and Oxyrrhis marina), one heterotrophic ciliate (Strombidium arenicola), and one mixotrophic dinoflagellate (Karlodinium armiger), all of which originated from the NW Mediterranean. G. dominans (ICM-ZOO-GD001) was isolated in February 2011, then kept at 19°C, and transferred to 16°C in June 2019 and to 22°C in November 2020. O. marina (ICM-ZOO-OM001) was isolated in 1995 and kept at 19°C; then, it was transferred to 16°C in June 2019 and from 19 to 22°C in November 2020. S. arenicola (ICM-ZOO-SA001) was isolated in April 2017, kept at 19°C, and transferred to 16°C in July 2020 and from 19 to 22°C in November 2020. Finally, K. armiger (ICM-ZOO-KA001) was isolated in winter 2013, kept at 19°C, and transferred to 16°C in June 2019 and from 19 to 22°C in November 2020. The cultures were kept in temperature-controlled incubators in 260 mL untreated tissue culture PTE flasks with autoclaved 0.1-μm filtered seawater, at a salinity of 38, ca. 35 μmol photons m–2 s–1, and they were fed exponentially growing Rhodomonas salina (K-0294) every 1–2 weeks. We made sure that all the species were kept at the selected temperatures for at least 7 months before conducting the experiments.
Elemental Analysis
We prepared stock cultures of the adapted strains of all species and starved them. Once no prey was detected both visually and with the aid of a Multisizer IV Coulter Counter, we waited for an additional 24 h to ensure digestion of any remaining prey in the food vacuoles. To evaluate the changes in the stoichiometry of prey during the incubations, we also incubated cultures of R. salina (grown at 19°C) at 16, 19, 22, and 25°C for 24 and 48 h. Then, we filtered aliquots of known grazer and prey concentrations onto pre-combusted (450°C, 5 h) GF/F filters (Whatman, 25 mm) for determination of the carbon (C), nitrogen (N), and phosphorus (P) elemental compositions. The filters for CN analysis were dried at 60°C for 48–72 h and then stored in a desiccator until processing with a Flash EA1112 (Thermo Finnigan, München, Germany) CHNS analyzer. The filters for P analysis were immediately frozen at −80°C until processing. We processed P samples with the acid persulfate digestion method and posterior conversion to dissolved inorganic P with a Seal Analytical AA3 (Bran + Luebbe) analyzer. We calculated stoichiometric ratios as molar ratios, and we considered error propagation (square root law) in the calculation of the CP and NP ratios (Saiz et al., 2020).
Acclimation vs. Adaptation Responses Experimental Setup
We compared strains grown for multiple generations (>7 months) at 16, 19, and 22°C (adapted treatments) with those from 16°C exposed for a short period (2 days preconditioning and 1 day experiment) to a temperature rise of either 3°C (19°C) or 6°C (22°C) (acclimated treatments). These temperatures are within the range of annual oscillation in the area of study and isolation of the microbial grazers used (Calbet et al., 2001). The protocol used was the same for all species and temperatures; i.e., adapted strains incubated at their respective long-term temperature and those incubated at a different temperature followed the same 2d + 1d protocol. The 2-days acclimation took place in 620 mL Pyrex glass bottles submerged in a temperature-controlled bath at the previously noted temperatures. Light conditions were a 10:14 light/dark cycle at 15–20 μmol photons m–2 s–1. We used saturating prey concentrations for the experiments. Thus, G. dominans and K. armiger were incubated with 50,000 R. salina mL–1 (Calbet et al., 2013; Martínez and Calbet, unpublished), whereas O. marina and S. arenicola were supplied with 100,000–120,000 R. salina mL–1 (Calbet et al., 2013; Arias and Calbet, unpublished). We added 20 mL of f/2 medium per liter of suspension in all treatments to avoid nutrient deficiency. After the 2 days of acclimation grazers usually had grown and prey were partially depleted. Therefore, we readjusted the prey and grazer concentrations to the ones indicated before, added nutrients again (20 mL f/2 L–1), and sequentially transferred the organisms to triplicate 75 mL untreated tissue culture flasks (Falcon), where they were incubated for another 24 h (experiment). The grazer concentrations were chosen to allow a decrease in prey between 10–20%. We also set triplicate control bottles with only R. salina at each temperature. Initial and final samples were quantified using a Multisizer IV Coulter Counter, which provided data on abundance and cell volume (Multisizer™ user’s manual, 2010). Predator growth rates were calculated in cell numbers assuming exponential growth. To obtain grazing rates and average prey concentrations during the incubations, we used Frost’s (1972) equations; we calculated per capita values using the average concentration of grazers in each replicate according to Heinbokel (1978). GGEs were calculated as the quotient between carbon-based specific growth and ingestion rates x 100.
Extreme Thermal Exposure Experimental Setup
We used the same experimental setup described before. However, here, we exposed all the temperature-adapted strains to 25°C for 3 days (two pre-conditioning and one experiment). This temperature is around the maximum average summer temperatures experienced in the area of origin of the species (Calbet et al., 2001). Then, we compared the growth, grazing, and GGEs of the adapted strains at their normal temperature with those after exposure to 25°C. Similar to the previous experiment, we used Frost’s (1972) equations to obtain ingestion rates.
Statistical Analysis
We used GraphPad Prism 7.0 software to conduct the statistical analysis. For stoichiometric effects of temperature, we sought for significant linear responses in the relationship between temperature and elemental composition or elemental ratio. Regarding experiments, our main objective was to investigate whether the physiological response of temperature-adapted organisms was different from that of only acclimated ones. To do such comparisons, we conducted ANOVA tests, with Tukey’s test to compare the treatments at each temperature, and assuming a p < 0.05 for significant differences. Our experimental design rendered more information, such as the different response of both adapted and acclimated organisms to temperature; the effects of temperature on each physiological activity were evaluated by ANOVA, with Tukey’s test to compare the response of each temperature. The differences in the slope of the relationship between temperature (16–19°C) and growth rates (d–1) for the different species were evaluated with ANCOVA tests (see Section Acclimation vs. Adaptation). Error propagation, when necessary, was calculated using the square root law (Saiz et al., 2020).
Results
Elemental Composition
Table 1 presents the elemental compositions of the temperature-adapted predators. No statistically significant relationship between temperature and either elemental composition or elemental molar ratios was observed in any of the grazers (simple linear regression analysis, P > 0.05), with only two exceptions. The P contents of K. armiger slightly increased with temperature (from 0.0030 to 0.0039 pgP μm–3), and the C:P ratio of O. marina diminished with temperature to some degree (from 71 to 64). Table 2 presents the volume and elemental composition of R. salina after exposure for 24 and 48 h to the experimental temperatures. This information is relevant for detecting any change in the nutritional composition that the temperature may have produced during the incubations. The volume and CNP composition of R. salina were relatively stable and unaffected by exposure at the different temperatures within the incubation periods (variations always <20% with respect to 16°C, and no statistically significant pattern with temperature).
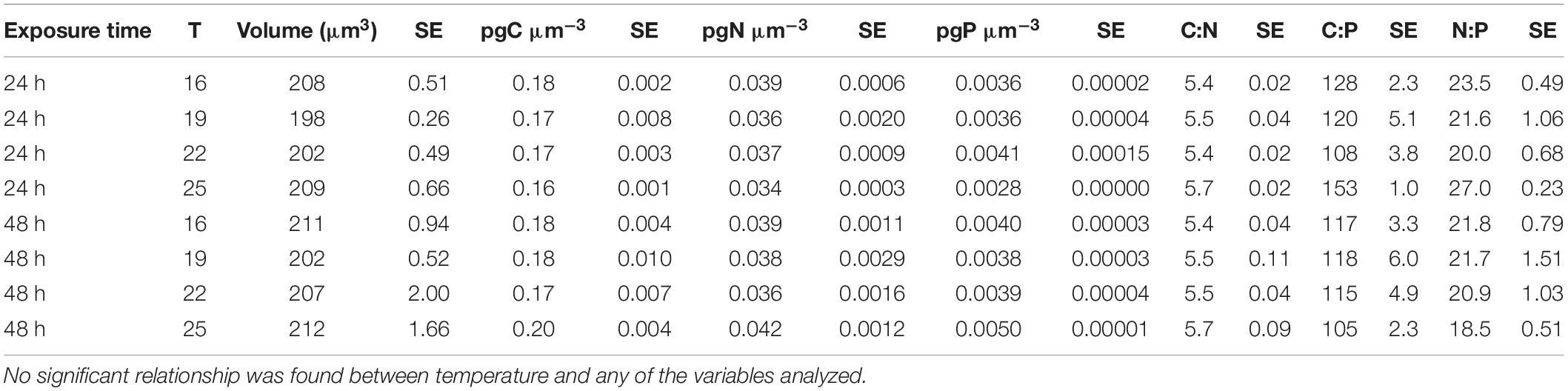
Table 2. Cell volume, elemental contents and molar stoichiometric ratios of Rhodomonas salina after 24 and 48 of exposure to the experimental temperatures.
Acclimation vs. Adaptation
Our goal was to compare the physiologies and behaviors of the thermal-adapted strains with those of the parental 16°C strains when exposed to warmer temperatures (acclimated treatment). The focus was on cell volume, growth rates (d–1), ingestion rates (in cells ind–1 d–1), and GGE. For simplicity, we present the significance of the comparison between temperature-adapted strains with their acclimated counterparts in the figures (asterisks indicating p < 0.05), whereas the significance of the differences between the response to the different temperatures for adapted and acclimated strains is shown in Table 3. Compared to the 16°C strain, G. dominans and O. marina cell volumes slightly (although not significantly) augmented with a 3°C temperature increase (19°C), and warmer conditions (+6°C) resulted in a decrease in volume (not statistically significant either), both in adapted and acclimated strains (Figures 1A,B and Table 3). The effect of temperature on S. arenicola cell volume was weak for both acclimated and adapted strains (Figure 1C and Table 3). The treatments of 16°C-adapted S. arenicola incubated at +3°C (19°C-acclimated treatment) was the only one significantly different from its adapted counterpart (Figure 1C). Finally, the acclimated strains of K. armiger decreased in volume at higher temperatures (19 and 22°C) compared to the adapted counterparts (Figure 1D and Table 3). Among the adapted strains of this species, the ones at warmer temperatures showed higher cell volumes than those at 16°C (Table 3), and their volumes were larger than their temperature-acclimated counterparts (Figure 1D; p < 0.001).
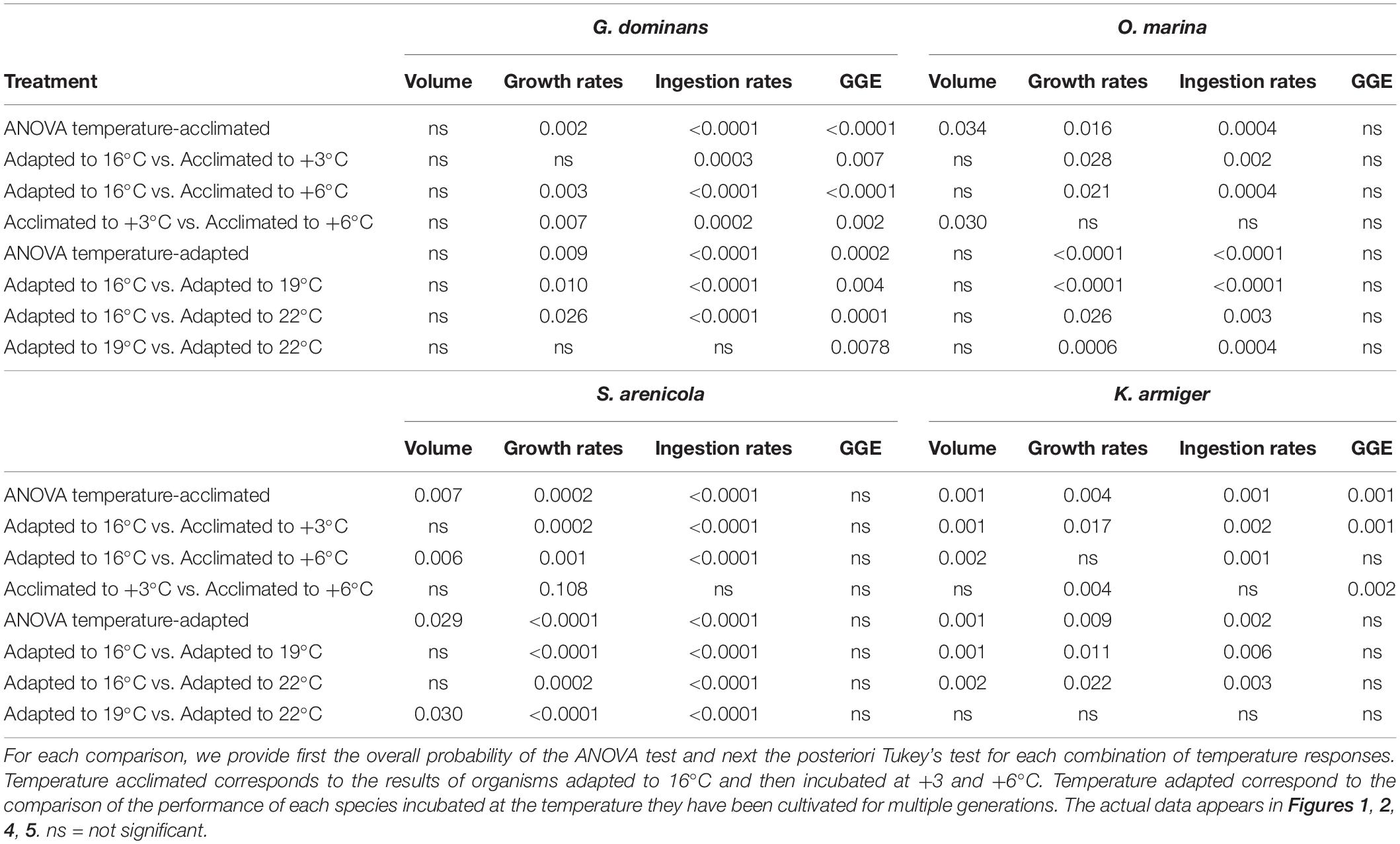
Table 3. Probability values of the ANOVA tests comparing the response to temperature in Volume (μm3), Growth rates (d–1), Ingestion rates (cells grazer–1 d–1), and GGE (gross-growth efficiency) of the four species of protistan grazers studied.
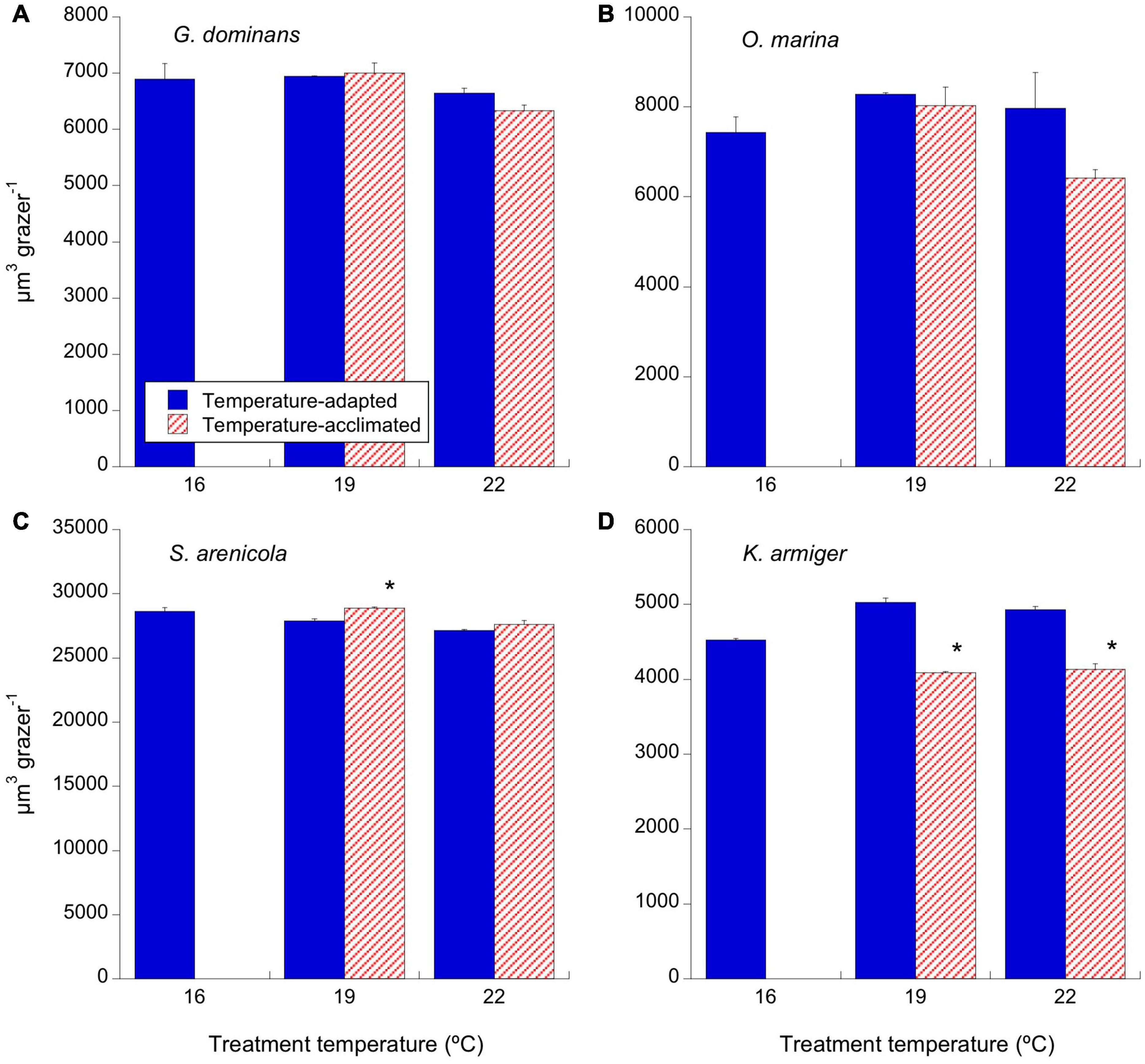
Figure 1. Effects of temperature on cell volume (μm3) of the different grazers. Solid blue bars correspond to the temperature-adapted organisms incubated at their standard temperature. Stripped red bars correspond to those organisms adapted at 16°C and exposed to either +3°C (19°C) or +6°C (22°C) temperature raise (2 days acclimation plus 1-day experimental incubation). (A) G. dominans, (B) O. marina, (C) S. arenicola, and (D) K. armiger. Asterisks denote differences (p < 0.05) between acclimated and adapted treatments. The statistics corresponding to differences between temperature treatments of adapted and acclimated organisms can be found in Table 3. The error bars are SE.
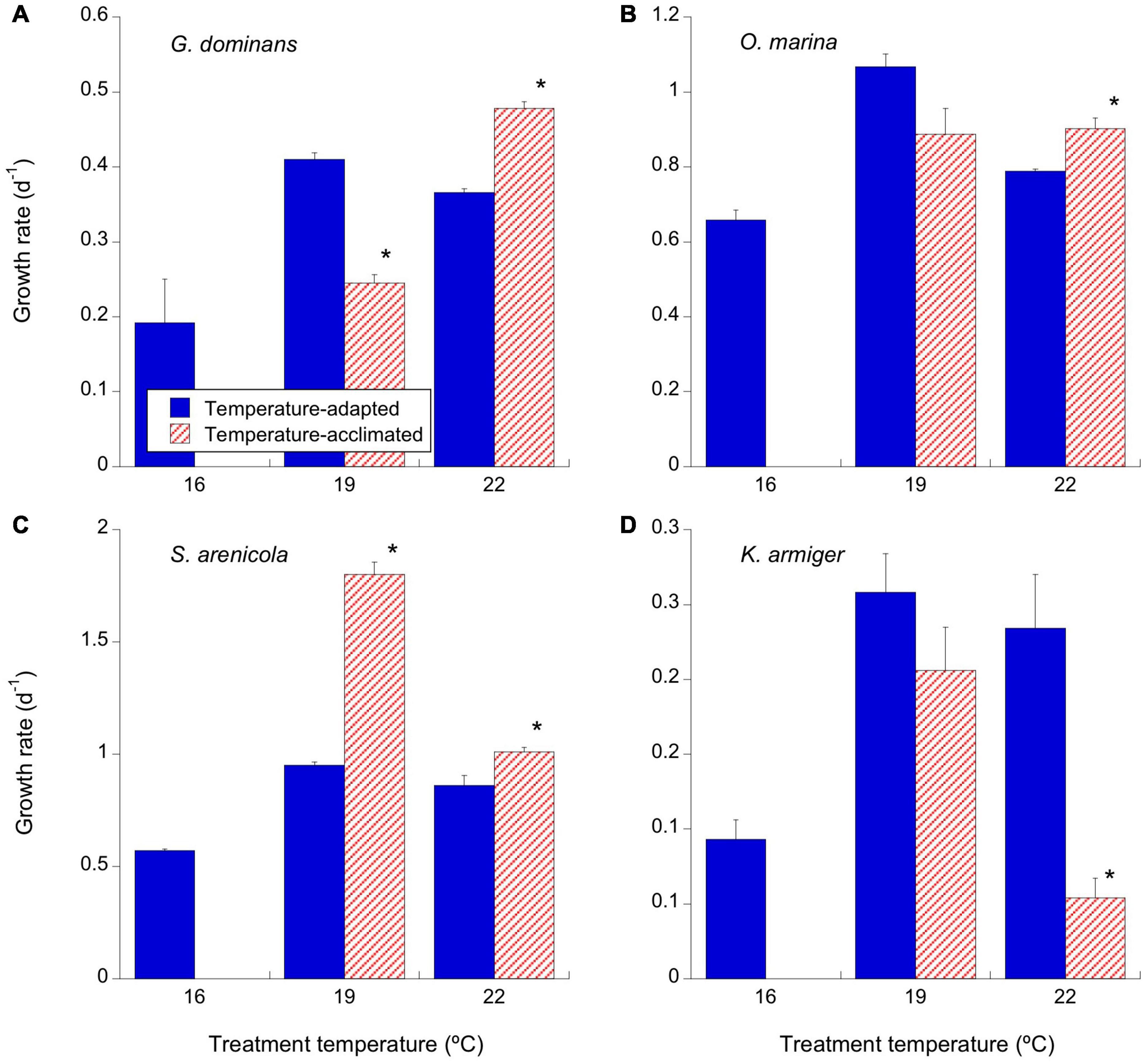
Figure 2. Effects of temperature on the growth rates (d–1) of the experimental protistan grazers. Solid blue bars correspond to the temperature-adapted organisms incubated at their standard temperature. Stripped red bars correspond to those organisms adapted at 16°C and exposed to either +3°C (19°C) or +6°C (22°C) temperature raise (2 days acclimation plus 1-day experimental incubation). (A) G. dominans, (B) O. marina, (C) S. arenicola, and (D) K. armiger. Asterisks denote differences (p < 0.05) between acclimated and adapted treatments. The statistics corresponding to differences between temperature treatments of adapted and acclimated organisms can be found in Table 3. The error bars are SE.
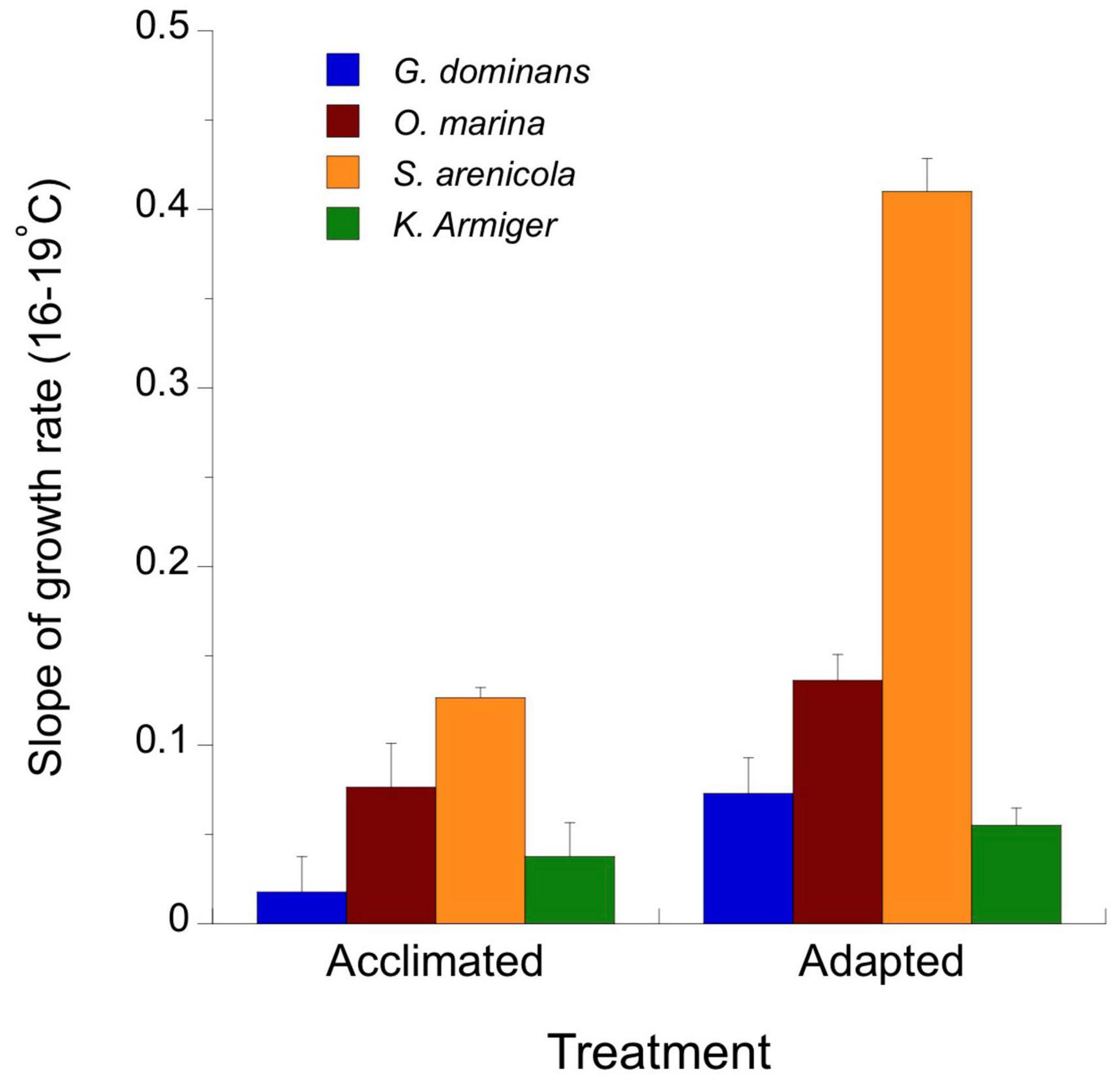
Figure 3. Slopes of the simple linear regression fit between growth rate and temperature for temperature-acclimated and temperature-adapted grazers. The slopes were calculated using the data showed in Figure 2 between 16 and 19°C.
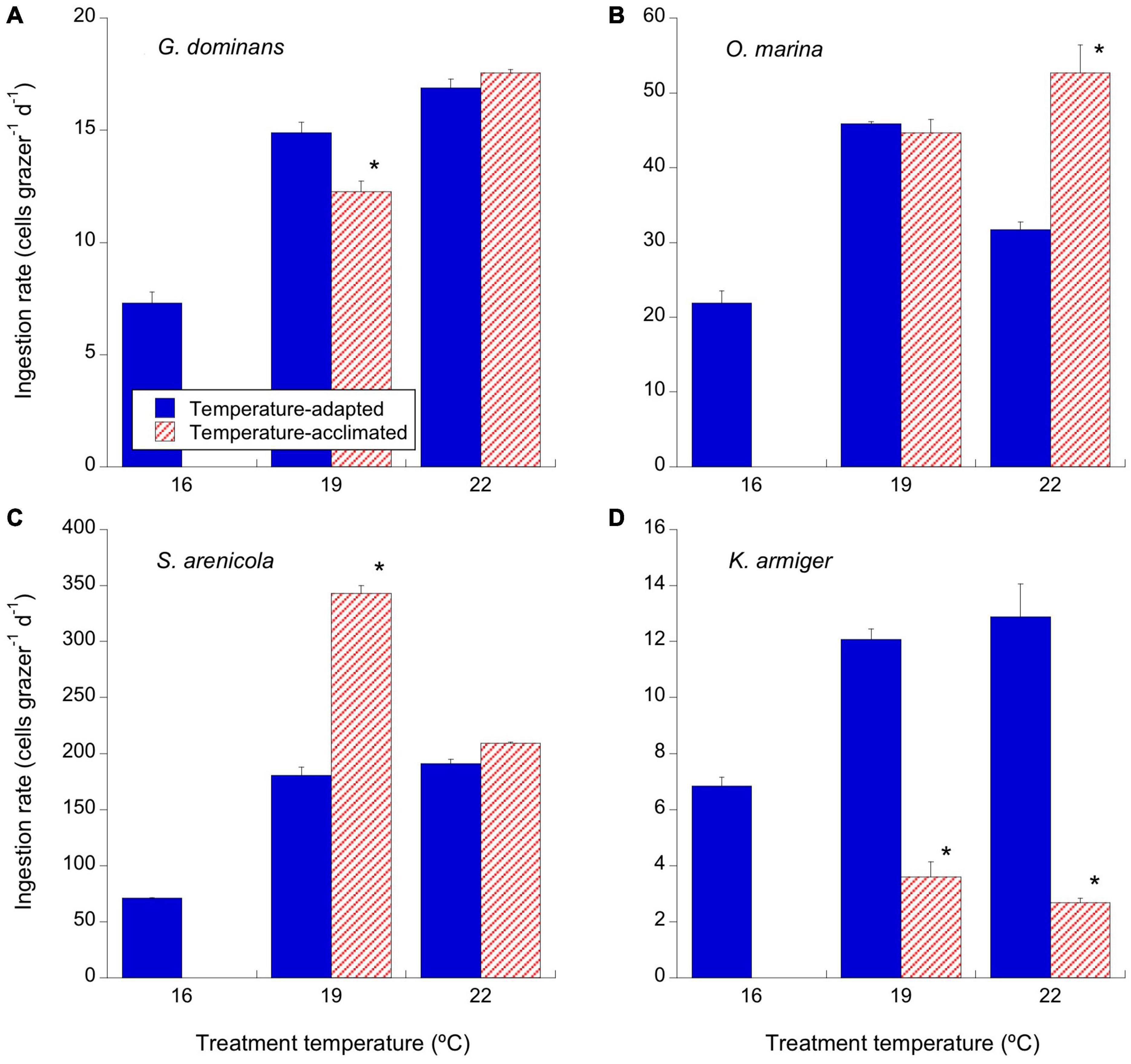
Figure 4. Effects of temperature on the ingestion rates (cells consumed per grazer and day) of the experimental protistan grazers. Solid blue bars correspond to the temperature-adapted organisms incubated at their standard temperature. Stripped red bars correspond to those organisms adapted at 16°C and exposed to either +3°C (19°C) or +6°C (22°C) temperature raise (2 days acclimation plus 1 day experimental incubation). Asterisks denote differences (p < 0.05) between acclimated and adapted treatments. (A) G. dominans, (B) O. marina, (C) S. arenicola, and (D) K. armiger. The statistics corresponding to differences between temperature treatments of adapted and acclimated organisms can be found in Table 3. The error bars are SE.
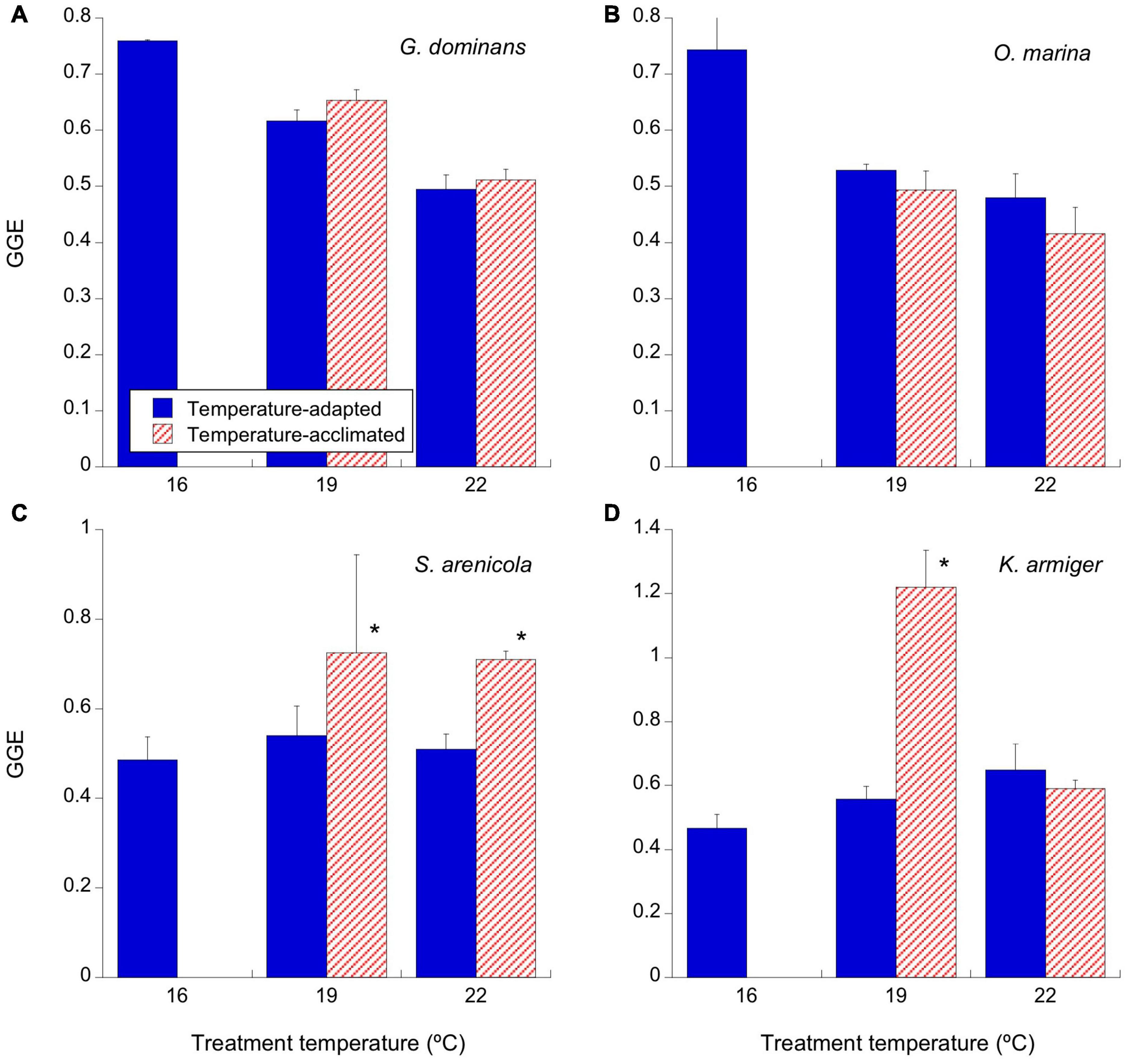
Figure 5. Effects of temperature on the gross growth efficiency (GGE; as the percentage of carbon produced out the one ingested) of the experimental protistan grazers (d–1). Given we did not have carbon data for S. sulcatum at 16°C, we used the values at the closest temperature (19°C). Solid blue bars correspond to the temperature-adapted organisms incubated at their standard temperature. (A) G. dominans, (B) O. marina, (C) S. arenicola, and (D) K. armiger. Stripped red bars correspond to those organisms adapted at 16°C and exposed to either +3°C (19°C) or +6°C (22°C) temperature raise (2 days acclimation plus 1-day experimental incubation). Asterisks denote differences (p < 0.05) between acclimated and adapted treatments. The statistics corresponding to differences between temperature treatments of adapted and acclimated organisms can be found in Table 3. The error bars are SE.
Gyrodinium dominans cell-based growth rates increased exponentially with temperature from 0.19 d–1 at 16°C to 0.48 d–1 at 22°C for only the acclimated strains (Figure 2A and Table 3). Adapted strains showed higher growth rates than acclimated strains at 19°C (p < 0.001), but it was the opposite at 22°C (p < 0.001; Figure 2A). The growth rates of O. marina increased from 0.66 d–1 at 16°C to 0.89 d–1 and 1.07 d–1 at 19°C for the acclimated and adapted organisms, respectively (Figure 2B and Table 3). At 22°C, the rates either remained the same (acclimated) or dropped to 0.8 d–1 in the adapted O. marina (Figure 2B and Table 3). There were differences in the growth rates between the acclimated and adapted O. marina, but they were statistically significant only at 22°C (Figure 2C, p < 0.05). S. arenicola showed a similar pattern to that of O. marina, with clear enhancements in growth rates at 19°C, acutely in the acclimated strains (up to 1.8 d–1), compared to a drop in growth rates at 22°C (Figure 2C and Table 3). Overall, the growth rates of S. arenicola were consistently higher for the temperature-acclimated strains than for the adapted ones (Figure 2C). The K. armiger thermal response was also similar to the previously described ones; however, the drop in growth rates at 22°C for the acclimated organisms was more severe than that for the adapted organisms (Figure 2D). Conversely, the growth rates for the 22°C-adapted strains of K. armiger were similar to those at 19°C (Table 3).
Because most species responded negatively to 22°C in both the adapted and acclimated strains, we restricted the calculation of thermal-driven enhancement of the grazer growth rates to the 16–19°C interval. Given the expected linear (not exponential) relationship between temperature (°C) and growth rates (d–1) in protozoans shown by Montagnes et al. (2003), in Figure 3, we present the slope of this relationship for acclimated and adapted organisms. Temperature-adapted organisms always showed higher slopes than those of their interacting counterparts; however, the differences between slopes were only statistically significant for S. arenicola (ANCOVA, p < 0.0001).
There was a consistent increase in the number of cells consumed daily by G. dominans and O. marina in their acclimated strains, from 16 to 22°C (Figures 4A,B and Table 3). This pattern was mirrored by the adapted organisms at 19°C in both species, with only significantly higher rates for the adapted vs. the acclimated strains in G. dominans (Figures 4A,B and Table 3). The ingestion rates dropped for the 22°C-adapted O. marina strain, while they remained high for G. dominans (Figures 4A,B and Table 3). The ciliate S. arenicola ingested R. salina at increasing rates with temperature up to 19°C (Figure 4C and Table 3); above this temperature, the rates were steady for acclimated organisms and dropped for the adapted organisms (Figure 4C and Table 3). In contrast, K. armiger-acclimated strains reduced their ingestion performance at increasing temperatures (Figure 4D and Table 3). This trend did not occur in the adapted strains, which showed higher rates at 19 and 22°C (Table 3).
There was an overall tendency to decrease GGE at increasing temperatures for both adapted and acclimated G. dominans and O. marina (Figures 5A,B and Table 3). However, S. arenicola and K. armiger showed slightly increasing tendencies in GGEs with increasing temperature, and those were significant only in few occasions (Figures 5C,D and Table 3). Both species responded positively to a short term increase of 3°C (acclimation experiment), and in the case of the S. arenicola, also to an increase of 6°C (Figures 5C,D and Table 3).
Response to an Extreme Temperature Episode
We present data about the response to a sudden temperature increase (Figure 6) only for G. dominans, O. marina, and K. armiger because the 16°C-adapted S. arenicola strains quickly died (within 24 h) when exposed to 25°C, and the remaining strains of this species (those adapted to higher temperatures) died after 48 h of incubation. The growth rates of G. dominans exposed to 25°C were very similar irrespective of the origin of the strain, although the growth rate of the strain adapted to 16°C was 26% higher than those of the other 19 and 22°C adapted ones (ANOVA and Tukey’s test, p < 0.001; Figure 6A). The opposite was apparent for O. marina adapted to 16°C, which at 25°C exhibited the lowest growth rates followed by the 22 and 19°C adapted strains (ANOVA p < 0.001; Tukey’s test: 16°C was different from 19°C, p < 0.01; 19°C different from 22°C, p < 0.05, and 16°C was different from 22°C, p < 0.05; Figure 6A). Similarly, when exposed to 25°C, the K. armiger 16°C-adapted strain also showed the lowest (even negative) growth rates compared to the other two strains that grew at higher and rather similar rates (ANOVA and Tukey’s test p < 0.01; Figure 6A). Overall, the growth rates of K. armiger at 25°C were low compared to the strains adapted and incubated at 19 and 22°C (Figure 2D).
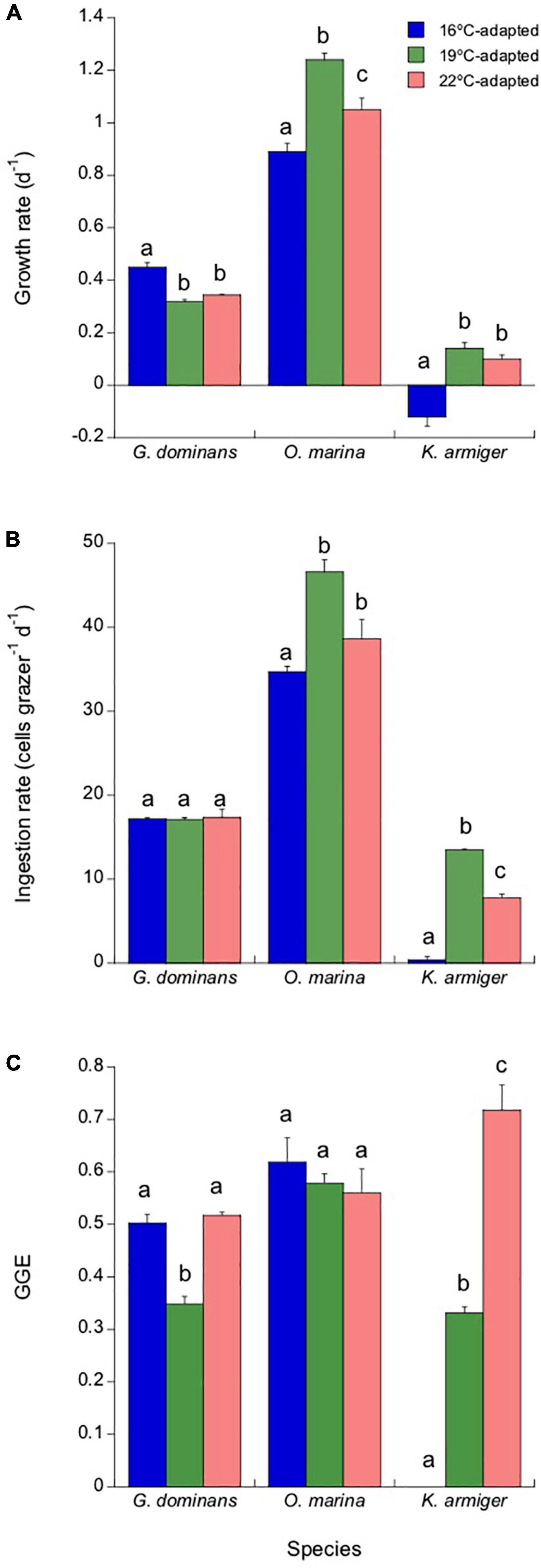
Figure 6. Response of the different adapted grazers (16, 19, and 22°C) to a sudden exposure to 25°C (2 days acclimation plus 1-day experimental incubation), simulating a heatwave episode. Growth rates (A), ingestion rates (B), and GGEs (C) are presented. Statistical differences (p < 0.05) within each species are indicated by different letters. The error bars are SE.
The effects of incubation at 25°C on ingestion rates are presented in Figure 6B. G. dominans did not present any difference between the ingestion rates at 25°C, irrespective of the strain. In contrast, in O. marina and K. armiger, the strains adapted to 19°C (Tukey’s test for both p < 0.05) always showed the highest feeding rates, followed by those adapted to 22°C, and finally, the lowest rates were exhibited for the strains adapted to 16°C. K. armiger ingestion rates at 25°C were near zero for the strain adapted to 16°C (Figure 6B). The combination of ingestion and growth rates determine the values of GGE (Figure 6C). G. dominans grown at 19°C showed a lower GGE than that of the remaining strains of this species. All O. marina strains, on the other hand, showed similar GGEs at 25°C, irrespective of the temperature of adaptation. Finally, the K. armiger GGE was a direct function of adaptation to temperature, with the strains grown at higher temperatures showing more elevated GGEs (Figure 6C).
Discussion
We evaluated the adaptive responses of four species of protistan grazers, including both heterotrophs and mixotrophs, to temperature. We started with several hypotheses that proved to be partially wrong. For instance, we did not find any robust evidence of lower C:N or C:P ratios resulting from temperature adaptation in either heterotrophs or mixotrophs. On the other hand, we found lower GGEs with increasing temperatures for some species, but others showed the opposite pattern. We also discovered evidence partially supporting the “hotter is better hypothesis” (Bennett, 1987), but only for a range of temperatures.
Temperature Effects on Stoichiometry
For autotrophs, one would expect that at short time intervals (acclimation) and given the higher Q10 of respiration than photosynthesis predicted by the metabolic theory of ecology (López-Urrutia et al., 2006; Rose and Caron, 2007), the C:N and C:P ratios would diminish with warming. The elemental ratio decrease is explained because increased respiration over photosynthesis would make C use proportionally more favored by warming than C assimilation, reducing the overall C pool over other elements. In contrast to expectations, this pattern in elemental ratios is not commonly found for well-adapted species to temperature, particularly in terms of C:P (Yvon-Durocher et al., 2017). For heterotrophs, the stoichiometric response to temperature is not straightforward because it is impossible to separate the direct effects of temperature from those of their prey. In their meta-analysis, Woods et al. (2003) concluded that P and N contents were higher in cold vs. warm environments across various animal groups, except for prokaryotes. However, generalizations are not always corroborated by individual experiments. For instance, copepod C:P ratios decreased with temperature in nauplii and copepodites under no limitation of this element in their diets; nevertheless, when P is limiting, copepodites increased their C:P with temperature, whereas nauplii kept it low (Mathews et al., 2018). For Daphnia spp., the P content is negatively related to temperature, as predicted, although this relationship is species-specific and dependent on the P contents of the prey (McFeeters and Frost, 2011). For protistan grazers, unfortunately, there is an obvious need for data. Our results show that the variations in the elemental composition per unit of cell volume in adapted protistan grazers due to temperature are minor. We should consider that equal stoichiometry combined with a hypothetic reduction in volume due to temperature may nevertheless result in a lower CNP transfer (via mesozooplankton grazing) to higher trophic levels. Therefore, the overall effects of temperature on nutrient transfer through the food web should be considered, even if the stoichiometry of the protistan grazers does not change much.
Does Size Matter?
Some organisms used in this study have been shown to present high body plasticity, modifying their cellular volume depending on the amount of ingested prey (Calbet et al., 2013). This fact could have masked the patterns of cell volume variations related to temperature. This does not seem to be the case because the experiments were conducted under satiating food conditions. Therefore, any change in volume during the incubations was because of a direct effect of temperature. It is surprising, then, that we did not observe a decrease in size between organisms adapted to 16°C and those adapted to 19°C. On the other hand, most of the strains adapted to 22°C, appeared smaller than those adapted at 19°C, although the differences were not significant in most of the occasions.
A decrease in the volume of autotrophs at higher temperatures, with its consequent increase in the surface/volume relationship, may favor the acquisition of dissolved inorganic nutrients. For heterotrophs, a size reduction does not seem to provide any advantage in terms of the acquisition of prey. A higher surface/volume relationship may also help obtain more oxygen, which availability is reduced by increased temperatures (Atkinson et al., 2003). Another advantage associated with the size decrease that accompanies temperature for unicellular organisms is to reduce settling rates (Atkinson, 1994; Atkinson et al., 2003). In our case, all the species considered swim, and size reduction would have only offered limited advantages. It has also been suggested that because an increased temperature will favor higher growth rates, a reduction in cell size at higher temperatures may be a response to increased population growth rates because cells that divide early will make up a more significant fraction of the total population (Atkinson et al., 2003). However, the only decrease in size we observed in our experiments (even though not significant) occurred at 22°C, and at that temperature, only G. dominans showed increased growth rates. In summary, our results do not clearly support the hypothesis of consistent decrease in size motivated by temperature in protozoans (Atkinson et al., 2003).
Acclimation vs. Adaptation
Most global theories about temperature effects on plankton are mainly based on acclimated-only organisms [e.g., Rose and Caron (2007)]. Therefore, it is critical to assess whether temperature-adapted organisms show the same response than acclimated organisms. Our data showed that this is not the case for many of the variables tested. Based on growth rates, we could conclude that our organisms adapted at 16°C followed the hypothesis “hotter is better” (Bennett, 1987; Knies et al., 2009) when exposed to a moderate temperature rise (+3°C). Increases of 6°C, however, compromised the physiological performance of most of the species, except for G. dominans, which continued to show an enhancement in growth rates.
When considering the GGE, however, we observed that this species showed a decrease in this variable with temperature, indicating a lower efficiency of converting food into growth at high temperatures. This is not unexpected because for zooplankton, the GGE at saturated prey concentrations usually decreases with increasing temperatures (Straile, 1997; Li et al., 2011), probably due to an imbalance between physiological rates. Surprisingly, the ciliate S. arenicola showed higher GGEs when exposed for a short period of time to a 3 and 6°C increase. This result could suggest that this species might be favored under a future climate change scenario. However, the lack of response to temperature in the adapted strains, and the collapse of the population when exposed to 25°C, indicate that 22°C was close to its physiological thermal limit, at least for the given temporal exposure.
Finally, we want to call attention to the fact that some GGEs found in this study were at the higher limit of those reported previously (Straile, 1997). Regarding K. armiger, it is important to note that the very high GGE found at 19°C for the acclimated organisms may have been a combination of its autotrophic and mixotrophic metabolism. Nevertheless, for the remaining grazers, this explanation does not apply. We believe the reason for such elevated GGEs may be a consequence of the body plasticity of protozoans, in many instances a direct function of the prey ingested (Calbet et al., 2013). When considering the specific growth used to calculate GGE, we could not discern between the actual increase in biomass due to cell growth and that derived from an accumulation of prey inside the grazer’s cell. Nevertheless, even though the absolute values may be somewhat high, we feel that the comparison between treatments should be correct.
Response to a Sudden Extreme Rise in Temperature: 25°C Experiment
The Intergovernmental Panel on Climate Change [IPCC] (2014) predicts an increase of ocean surface temperature from 0.3 to 4.8°C by the end of the twenty first century relative to 1986–2005. We have seen that many species will tolerate this increase, if gradual. However, climate change is also associated with abrupt temperature rises, known as heatwaves (Müren et al., 2005; Hayashida et al., 2020). The increase in temperature experienced by the 19 and 22°C adapted strains in the 25°C treatment was within the maximum range expected to occur in nature [up to 6.5°C, Hobday et al. (2016)], but maybe was unrealistically high for the 16°C adapted strains (+9°C). Despite this fact, however, the 16°C-adapted strains of G. dominans and O. marina showed higher growth and ingestion rates when incubated at 25°C than at 16°C (Figures 2, 4, and 6). Nevertheless, it is worth mentioning that the GGEs of the 16°C-adapted strains of all the species investigated were lower at 25°C than at 16°C, and that the maximum growth and ingestion rates of O. marina and K. armiger at 25°C were found for the 19°C-adapted strains (Figure 6). Particularly, the mixotrophic dinoflagellate seemed quite susceptible to abrupt exposure to high temperatures, dying at 25°C if adapted to 16°C, but thriving relatively well when adapted to higher temperatures. For this species, adaptation conferred clear resistance to sudden increases of temperature because the performance under 25°C was greatly improved when adapted to warmer temperatures. S. arenicola was the most extreme case of weak resistance to high temperature not surviving the acclimation period, and with even the 16°C adapted strain dying in less than 24 h. In summary, adaptation to warmer temperatures, even if not always fully developing the potential of the species, helps overcome the effects of short-term abrupt increases in temperature in some cases.
Were the Strains Fully Adapted to the Experimental Temperatures?
We define acclimation as the reversible physiological changes that improve an organism’s function in the environment, whereas adaptation involves more profound genetic changes (Bennett and Lenski, 1997). In phytoplankton, previous reports suggest that a few hundred generations are enough for thermal adaptation (Listmann et al., 2016; O’Donnell et al., 2018; Aranguren-Gassis et al., 2019). However, we are not aware of similar data for protistan grazers. As these organisms also have fast generation times, one could assume a similar rule may apply. The minimum time interval the adapted strains were reared at a given temperature was 7 months, and this occurred at the highest temperature tested (22°C), meaning shorter generation times. The growth rates found in our study at 22°C ranged from 0.23 d–1 in K. armiger to 1.0 d–1 in S. arenicola, which represents less than one doubling per day for the mixotrophs and over one doubling per ciliate. It can be argued that at least K. armiger at 22°C may not have gone through enough generations (one hundred at most, assuming food was not always in excess) to ensure a genotypic change.
However, it is precisely in this species where we found the highest performances (i.e., growth rates) at 22°C of the adapted strains compared to those of the merely acclimated strains (Figure 2). Thus, we can conclude that even if there is likely still room to adapt to warmer temperatures, the species showed clear evidence of adaptation to high temperatures. Similarly, Huertas et al. (2011) also concluded that not all phytoplankton adapt to the temperature at the same speed. Some species are much faster than others; i.e., some algae can adapt to tolerate high temperatures after only 45 days (Padfield et al., 2016). Perhaps, the changes shown in these responses to temperature did not involve mutation but are epigenetic, and therefore, reversible. In this respect, we should be aware that despite 16°C was closer to the temperature of origin of most of our strains, after so many years in the laboratory at 19°C under a controlled environment, different from what we find in nature (no predators, minor fluctuations in the physicochemical variables, etc.), our 16°C-adapted clones could be different from the wild specimens originating the cultures. Natural selection forces in nature are different than those in the laboratory. Therefore, we cannot expect wild protists to behave the same way as our cultures. Clear evidence of this is the results of Arias et al. (2021), who found the ciliate S. arenicola lost its diel feeding rhythm after prolonged periods of laboratory cultivation and partially recovered it when exposed to predators chemical cues.
It can also be questioned whether our food scenario during the cultivation of the organisms could have interfered with the adaptative process. The experiments presented here were conducted at saturating food concentrations to ease the comparison between treatments. However, the thermal adaptation of the strains took place in periods of variable food conditions. Routine maintenance of cultures is designed to feed the protistan grazers every 1 or 2 weeks, allowing them consume most of the available prey. Some researchers have suggested that nutrient limitation impairs the ability of algae to adapt to lethal temperatures because of a trade-off between increased temperature tolerance and higher N requirements (Aranguren-Gassis et al., 2019). Perhaps, this situation may also be similar for heterotrophs. In our study, the range of temperatures used was below those considered lethal for the selected species, except for 25°C, which was not used to adapt any strain. We cannot discount, however, that intermittent starvation could have affected our results, explaining why our 22°C-adapted strains did not perform better than those at 19°C. Given that constant food is seldom found in nature and that food fluctuations seem to be normal (Haury et al., 1978; Mackas et al., 1985), we believe our adaptative process mimics natural communities much better than constantly fed organisms in typical experimental setups.
Final Remarks
We can conclude that in protistan grazers, adaptation to temperature confers a selective advantage to warming within a reasonable limit (i.e., ca. +3°C), at least under food satiating conditions. Attempts to adapt to much higher temperatures (i.e., +6°C) do not confer any clear physiological advantage within the temporal framework of our experiments. Perhaps, the most remarkable exception to this was K. armiger, the only mixotroph that showed higher growth and grazing rates at 22°C in the adapted strains than in the acclimated strains. S. arenicola also showed this pattern in growth rates but not in ingestion rates. We cannot generalize with one single species that mixotrophs will thrive more than heterotrophs at higher temperatures. However, it is notable that the metabolic theory of ecology (López-Urrutia et al., 2006; Rose and Caron, 2007) predicts that heterotrophs may do better than autotrophs under a future global change scenario. Mixotrophs, showing a dual mode of nutrition, may then become more heterotrophic at higher temperatures and be favored. Interestingly, for short-term (24 h) responses to temperature, Ferreira et al. (submitted)1 found that in mixoplankton (including our strain of K. armiger), grazing was impaired at warmer temperatures, whereas photosynthesis increased. We can corroborate the results for grazing; our acclimated K. armiger did not respond well to warming in terms of ingestion rates. However, after proper adaptation, the ingestion rates were remarkably high (although we have no information on photosynthetic rates). Overall, this particular observation, even if not conclusive, calls for further research on the subject and a cautious interpretation of the interaction of marine protists and temperature when sufficient time to develop adaptive responses does not occur. Finally, we also want to emphasize that our experiments were conducted under excess of food and feeding on prey that were nutrient repleted. Other food scenarios would securely render different outcomes. This is particularly important because experimental assessments of temperature costs on fitness may be more evident when resources are limiting, and the other way around, food-limited organisms may have higher problems adapting to high temperatures (Aranguren-Gassis et al., 2019).
Data Availability Statement
The raw data supporting the conclusions of this article can be found at the open repository (DigitalCSIC, http://hdl.handle.net/10261/262244).
Author Contributions
AC wrote the manuscript and ES contributed to the manuscript revision. Both authors contributed to the conception and design of the study and approved the submitted version.
Funding
This research was funded by Grant CTM2017–84288-R by Fondo Europeo de Desarrollo Regional (FEDER)/Ministerio de Ciencia, Innovación y Universidades—Agencia Estatal de Investigacioìn (AEI), and by Grant PID2020-118645RB-I00 by Ministerio de Ciencia e innovación (MCIN)/AEI/10.13039/501100011033 and by ERDF A way of making Europe. It is a contribution of the Marine Zooplankton Ecology Group (2017 SGR 87). With the institutional support of the Severo Ochoa Centre of Excellence accreditation (CEX2019-000928-S). The open-access publication fee was partially covered by the CSIC Open Access Publication Support Initiative through its Unit of Information Resources for Research (URICI).
Conflict of Interest
The authors declare that the research was conducted in the absence of any commercial or financial relationships that could be construed as a potential conflict of interest.
Publisher’s Note
All claims expressed in this article are solely those of the authors and do not necessarily represent those of their affiliated organizations, or those of the publisher, the editors and the reviewers. Any product that may be evaluated in this article, or claim that may be made by its manufacturer, is not guaranteed or endorsed by the publisher.
Acknowledgments
We thank all the people from the lab involved in keeping the cultures alive during all the months the experiment lasted, particularly during the restrictive and dangerous confinement period of the pandemic COVID-19. We also thank C. Traboni for preparing the CNP samples for analysis.
Footnotes
- ^ Ferreira, G. D., Grigoropoulou, A., Saiz, E., and Calbet, A. (submitted). The effect of short-term temperature exposure on critical physiological processes of mixoplankton and protozooplankton.
References
Alcaraz, M., Almeda, R., Saiz, E., Calbet, A., Duarte, C. M., Agusti, S., et al. (2013). Effects of temperature on the metabolic stoichiometry of Arctic zooplankton. Biogeoscien 10, 689–697. doi: 10.5194/bg-10-689-2013
Aranguren-Gassis, M., Kremer, C. T., Klausmeier, C. A., Litchman, E., and Bates, A. (2019). Nitrogen limitation inhibits marine diatom adaptation to high temperatures. Ecol. Lett. 22, 1860–1869. doi: 10.1111/ele.13378
Arias, A., Selander, E., Saiz, E., and Calbet, A. (2021). Predator chemical cue effects on the diel feeding behaviour of marine protists. Microb. Ecol. 82, 356–364. doi: 10.1007/s00248-020-01665-9
Atkinson, A. (1994). Diets and feeding selectivity among the epipelagic copepod community near South Georgia in summer. Polar Biol. 14, 551–560.
Atkinson, D., Ciotti, B. J., and Montagnes, D. J. (2003). Protists decrease in size linearly with temperature: ca. 2.5% degrees C–1. Proc. Biol. Sci. 270, 2605–2611. doi: 10.1098/rspb.2003.2538
Barton, S., Jenkins, J., Buckling, A., Schaum, C. E., Smirnoff, N., Raven, J. A., et al. (2020). Evolutionary temperature compensation of carbon fixation in marine phytoplankton. Ecol. Lett. 23, 722–733. doi: 10.1111/ele.13469
Bennett, A. F. (1987). “Evolution of the control of body temperature: is warmer better?,” in Comparative Physiology: Life in Water and on Land, eds L. B. P. Dejours, C. R. Taylor, and E. R. Weibel (Padova: IX-Liviana Press).
Bennett, A. F., and Lenski, R. E. (1997). Evolutionary adaptation to temperature. VI. Phenotypic acclimation and its evolution in Escherichia Coli. Evolution 51, 36–46. doi: 10.1111/j.1558-5646.1997.tb02386.x
Calbet, A. (2001). Mesozooplankton grazing effect on primary production: a global comparative analysis in marine ecosystems. Limnol. Oceanogr. 46, 1824–1830.
Calbet, A., Garrido, S., Saiz, E., Alcaraz, M., and Duarte, C. M. (2001). Annual zooplankton succession in coastal NW Mediterranean waters: the importance of the smaller size fractions. J. Plankton Res. 23, 319–331.
Calbet, A., Isari, S., Martínez, R. A., Saiz, E., Garrido, S., Peters, J., et al. (2013). Adaptations to feast and famine in different strains of the marine heterotrophic dinoflagellates Gyrodinium dominans and Oxyrrhis marina. Mar. Ecol. Prog. Ser. 483, 67–84. doi: 10.3354/meps10291
Calbet, A., and Saiz, E. (2005). The ciliate-copepod link in marine ecosystems. Aquat. Microb. Ecol. 38, 157–167. doi: 10.3354/ame038157
Flynn, K. J., Mitra, A., Anestis, K., Anschütz, A. A., Calbet, A., Ferreira, G. D., et al. (2019). Mixotrophic protists and a new paradigm for marine ecology: where does plankton research go now? J. Plankton Res. 41, 375–391. doi: 10.1093/plankt/fbz026
Frost, B. W. (1972). Effects of size and concentration of food particles on the feeding behavior of the marine planktonic copepod Calanus pacificus. Limnol. Oceanogr. 17, 805–815. doi: 10.4319/lo.1972.17.6.0805
Halsband-Lenk, C., Hirche, H. J., and Carlotti, F. (2002). Temperature impact on reproduction and development of congener copepod populations. J. Exp. Mar. Biol. Ecol. 271, 121–153. doi: 10.1016/s0022-0981(02)00025-4
Hantzsche, F. M., and Boersma, M. (2010). Dietary-induced responses in the phagotrophic flagellate Oxyrrhis marina. Mar. Biol. 157, 1641–1651. doi: 10.1007/s00227-010-1437-1
Haury, L. R., Mcgowan, J. A., and Wiebe, P. H. (1978). “Patterns and processes in the time-scales of plankton distributions,” in Spatial Patterns in Plankton Communities. Plenum NATO Conference Series IV. Marine Sciences 3, ed. J. H. Steel (Berlin: Springer), 277–327. doi: 10.1007/978-1-4899-2195-6_12
Hayashida, H., Matear, R. J., and Strutton, P. G. (2020). Background nutrient concentration determines phytoplankton bloom response to marine heatwaves. Glob. Chang. Biol. 26, 4800–4811. doi: 10.1111/gcb.15255
Heinbokel, J. F. (1978). Studies on the functional role of tintinnids in the Southern California Bight. I. Grazing and growth rates in laboratory cultures. Mar. Biol. 47, 177–189. doi: 10.1007/bf00395638
Hobday, A. J., Alexander, L. V., Perkins, S. E., Smale, D. A., Straub, S. C., Oliver, E. C. J., et al. (2016). A hierarchical approach to defining marine heatwaves. Prog. Oceanogr. 141, 227–238. doi: 10.1016/j.pocean.2015.12.014
Huertas, I. E., Rouco, M., Lopez-Rodas, V., and Costas, E. (2011). Warming will affect phytoplankton differently: evidence through a mechanistic approach. Proc. Biol. Sci. 278, 3534–3543. doi: 10.1098/rspb.2011.0160
Intergovernmental Panel on Climate Change [IPCC] (2014). Climate Change 2014: Synthesis Report. Contribution of Working Groups I, II and III to the Fifth Assessment Report of the Intergovernmental Panel on Climate Change. Geneva: IPCC.
Klausmeier, C. A., Litchman, E., Daufresne, T., and Levin, S. A. (2004). Optimal nitrogen-to-phosphorus stoichiometry of phytoplankton. Nature 429, 171–174. doi: 10.1038/nature02454
Knies, J. L., Kingsolver, J. G., and Burch, C. L. (2009). Hotter is better and broader: thermal sensitivity of fitness in a population of bacteriophages. Am. Nat. 173, 419–430. doi: 10.1086/597224
Li, C. C., Xu, K. D., and Lei, Y. L. (2011). Growth and grazing responses to temperature and prey concentration of Condylostoma spatiosum, a large benthic ciliate, feeding on Oxyrrhis marina. Aquat. Microb. Ecol. 64, 97–104. doi: 10.3354/ame01521
Lin, C.-H., Flynn, K. J., Mitra, A., and Glibert, P. M. (2018). Simulating effects of variable stoichiometry and temperature on mixotrophy in the harmful dinoflagellate Karlodinium veneficum. Front. Mar. Sci. 5:320. doi: 10.3389/fmars.2018.00320
Listmann, L., Leroch, M., Schluter, L., Thomas, M. K., and Reusch, T. B. (2016). Swift thermal reaction norm evolution in a key marine phytoplankton species. Evol. Appl. 9, 1156–1164. doi: 10.1111/eva.12362
Litchman, E., Ohman, M. D., and Kiørboe, T. (2013). Trait-based approaches to zooplankton communities. J. Plankton Res. 35, 473–484. doi: 10.1093/plankt/fbt019
López-Urrutia, A., San Martin, E., Harris, R. P., and Irigoien, X. (2006). Scaling the metabolic balance of the oceans. Proc. Natl. Acad. Sci. U.S.A. 103, 8739–8744. doi: 10.1073/pnas.0601137103
Mackas, D. L., Denman, K. L., and Abbott, M. R. (1985). Plankton patchiness: biology in the physical vernacular. Bull. Mar. Sci. 37, 652–674.
Malzahn, A. M., Hantzsche, F., Schoo, K. L., Boersma, M., and Aberle, N. (2010). Differential effects of nutrient-limited primary production on primary, secondary or tertiary consumers. Oecologia 162, 35–48. doi: 10.1007/s00442-009-1458-y
Mathews, L., Faithfull, C. L., Lenz, P. H., and Nelson, C. E. (2018). The effects of food stoichiometry and temperature on copepods are mediated by ontogeny. Oecologia 188, 75–84. doi: 10.1007/s00442-018-4183-6
McFeeters, B. J., and Frost, P. C. (2011). Temperature and the effects of elemental food quality on Daphnia. Freshw. Biol. 56, 1447–1455. doi: 10.1007/s00442-009-1452-4
Meunier, C. L., Alguero-Muniz, M., Horn, H. G., Lange, J. A. F., and Boersma, M. (2017). Direct and indirect effects of near-future pCO(2) levels on zooplankton dynamics. Mar. Freshwater Res. 68, 373–380. doi: 10.1071/mf15296
Meunier, C. L., Haafke, J., Oppermann, B., Boersma, M., and Malzahn, A. M. (2012). Dynamic stoichiometric response to food quality fluctuations in the heterotrophic dinoflagellate Oxyrrhis marina. Mar. Biol. 159, 2241–2248. doi: 10.1007/s00227-012-2009-3
Montagnes, D. J. S., Kimmance, S. A., and Atkinson, D. (2003). Using Q10: can growth rates increase linearly with temperature? Aquat. Microb. Ecol. 32, 307–313. doi: 10.3354/ame032307
Multisizer™ user’s manual. (2010). MultisizerTM User’s Manual. Beckman Coulter, Inc. 250 Kraemer Blvd. Brea, CA 92821. Brea, CA: Beckman Coulter, Inc.
Müren, U., Berglund, J., Samuelsson, K., and Andersson, A. (2005). Potential effects of elevated sea-water temperature on pelagic food webs. Hydrobiologia 545, 153–166. doi: 10.1007/s10750-005-2742-4
O’Donnell, D. R., Hamman, C. R., Johnson, E. C., Kremer, C. T., Klausmeier, C. A., and Litchman, E. (2018). Rapid thermal adaptation in a marine diatom reveals constraints and trade-offs. Glob. Chang. Biol. 24, 4554–4565. doi: 10.1111/gcb.14360
Padfield, D., Yvon-Durocher, G., Buckling, A., Jennings, S., Yvon-Durocher, G., and Hillebrand, H. (2016). Rapid evolution of metabolic traits explains thermal adaptation in phytoplankton. Ecol. Lett. 19, 133–142. doi: 10.1111/ele.12545
Perrin, N. (1995). About Berrigan and Charnov’s life history puzzle. Oikos 73, 137–139. doi: 10.1111/1365-2656.12183
Rose, J. M., and Caron, D. A. (2007). Does low temperature constrain the growth rates of heterotrophic protists? Evidence and implications for algal blooms in cold waters. Limnol. Oceanogr. 52, 886–895. doi: 10.4319/lo.2007.52.2.0886
Saiz, E., Griffell, K., and Calbet, A. (2020). Ontogenetic changes in the elemental composition and stoichiometry of marine copepods with different life history strategies. J. Plankton Res. 42, 320–333. doi: 10.1093/plankt/fbaa018
Sterner, R., and Elser, J. (2002). Ecological Stoichiometry: the Biology of Elements From Molecules to the Biosphere. Princeton, NJ: Princeton University Press.
Straile, D. (1997). Gross growth efficiencies of protozoan and metazoan zooplankton and their dependence on food concentration, predator-prey weight ratio, and taxonomic group. Limnol. Oceanogr. 42, 1375–1385. doi: 10.4319/lo.1997.42.6.1375
Traboni, C., Calbet, A., and Saiz, E. (2021). Mixotrophy upgrades food quality for marine calanoid copepods. Limnol. Oceanogr. 66, 4125–4139. doi: 10.1002/lno.11948
Wilken, S., Huisman, J., Naus-Wiezer, S., and Van Donk, E. (2013). Mixotrophic organisms become more heterotrophic with rising temperature. Ecol. Lett. 16, 225–233. doi: 10.1111/ele.12033
Woods, H. A., Makino, W., Cotner, J. B., Hobbie, S. E., Harrison, J. F., Acharya, K., et al. (2003). Temperature and the chemical composition of poikilothermic organisms. Funct. Ecol. 17, 237–245.
Keywords: microzooplankton, protist, mixotroph, temperature, grazing, growth, climate change, adaptation
Citation: Calbet A and Saiz E (2022) Thermal Acclimation and Adaptation in Marine Protozooplankton and Mixoplankton. Front. Microbiol. 13:832810. doi: 10.3389/fmicb.2022.832810
Received: 10 December 2021; Accepted: 21 February 2022;
Published: 23 March 2022.
Edited by:
Jun Sun, China University of Geosciences Wuhan, ChinaReviewed by:
Casey Michael Godwin, University of Michigan, United StatesSophie Charvet, American Museum of Natural History, United States
Copyright © 2022 Calbet and Saiz. This is an open-access article distributed under the terms of the Creative Commons Attribution License (CC BY). The use, distribution or reproduction in other forums is permitted, provided the original author(s) and the copyright owner(s) are credited and that the original publication in this journal is cited, in accordance with accepted academic practice. No use, distribution or reproduction is permitted which does not comply with these terms.
*Correspondence: Albert Calbet, YWNhbGJldEBpY20uY3NpYy5lcw==
†These authors have contributed equally to this work