- 1Departamento de Química Biológica, Facultad de Ciencias Exactas y Naturales, Universidad de Buenos Aires, Buenos Aires, Argentina
- 2Instituto de Química Biológica de la Facultad de Ciencias Exactas y Naturales, Universidad de Buenos Aires, Consejo Nacional de Investigaciones Científicas y Técnicas, Buenos Aires, Argentina
Probiotics have been shown to be effective against infectious diseases in clinical trials, with either intestinal or extraintestinal health benefits. Even though probiotic effects are strain-specific, some “widespread effects” include: pathogen inhibition, enhancement of barrier integrity and regulation of immune responses. The mechanisms involved in the health benefits of probiotics are not completely understood, but these effects can be mediated, at least in part, by probiotic-derived extracellular vesicles (EVs). However, to date, there are no clinical trials examining probiotic-derived EVs health benefits against infectious diseases. There is still a long way to go to bridge the gap between basic research and clinical practice. This review attempts to summarize the current knowledge about EVs released by probiotic bacteria to understand their possible role in the prevention and/or treatment of infectious diseases. A better understanding of the mechanisms whereby EVs package their cargo and the process involved in communication with host cells (inter-kingdom communication), would allow further advances in this field. In addition, we comment on the potential use and missing knowledge of EVs as therapeutic agents (postbiotics) against infectious diseases. Future research on probiotic-derived EVs is needed to open new avenues for the encapsulation of bioactives inside EVs from GRAS (Generally Regarded as Safe) bacteria. This could be a scientific novelty with applications in functional foods and pharmaceutical industries.
Introduction
Infectious diseases are disorders caused by organisms such as viruses, bacteria, fungi, or parasites. Could probiotics deal with infectious diseases? A lot of clinical trials have brought this question to the forefront with positive effects on prevention and/or treatment of infectious diseases. In this review, we conducted a search for probiotic bacteria utilized for treatment of infectious diseases and then discussed the current knowledge about extracellular vesicles (EVs) released by these probiotic species. It is important to highlight that according to the generally accepted definition of probiotic, probiotic effects are strain-specific. However, various effects of probiotics can be ascribed to the species level (Hill et al., 2014). Moreover, the study of EVs released by the probiotic strains is still in its infancy and has not been widely analyzed so far. For these two reasons, to collect the current evidence of probiotic-derived EVs we decided to extrapolate our search to the species level. In this line, EV-producing strains were shown to mediate beneficial effects both in vitro and in vivo models, but human trials (a requirement for probiotic claim) are still pending.
Probiotics
Definition
Probiotics are defined as “live microorganisms that, when administered in adequate amounts, confer a health benefit on the host” (Hill et al., 2014). In a position statement an expert panel of the International Scientific Association for Probiotics and Prebiotics (ISAPP) set four minimum criteria for probiotic claims (Binda et al., 2020). Probiotics must: (1) be identified to the genus, species and strain level, (2) be safe for the intended use, (3) have demonstrated health benefits in at least one clinical trial, and (4) have a suitable viable count at end of shelf life.
Before clinical trials are conducted, potential probiotics must be selected by a comprehensive approach including multiple steps. According to the “Guidelines for the Evaluation of Probiotics in Food” (FAO/WHO, 2002), candidate strains are suggested to be assessed for their stress tolerance, antimicrobial properties, epithelium adhesion ability, and safety. At the same time, in vitro and in vivo experiments should be performed to evaluate probiotic effects (de Melo Pereira et al., 2018; Santos et al., 2020).
As stated above, for validation of treatment safety and efficacy, probiotics must be subjected to at least one clinical trial, which must be conducted based on generally accepted scientific standards (Binda et al., 2020). In general, the weight ascribed to a trial result is higher when sources of bias are avoided (Higgins et al., 2019), and therefore randomized controlled trials are usually considered the most appropriate methodology for validating a probiotic health claim (Tamayo, 2008). In the last decades, there has been a rapid growth in the number of clinical trials for the use of probiotics for prophylactic and/or therapeutic applications in various fields: infectious diseases, cancer, depression and obesity (Zommiti et al., 2020).
Even though probiotics must be identified to the strain level, various meta-analyses indicate that “shared benefits” are achieved by many different strains of the same species, due to similar biological pathways (Sanders et al., 2018). In that regard, the ISAPP panel considered that well-studied beneficial species may be considered as “probiotics” even in the absence of randomized controlled trials that support this claim (Hill et al., 2014). Although clinical trials rarely compare different strains of the same species, certain health effects such as immunomodulation have been ascribed to many strains of the same species (Zhao et al., 2020, 2021).
Many probiotic lactic acid bacteria have long been used in dairy products, being awarded the status of GRAS (Ghosh et al., 2019). The projection of the global probiotics market is expected to grow at a compound annual growth rate of 7.2% from 2021 to 2028 (Grand View Research, 2021). The popularity of probiotic use has increased dramatically in the last decades, not only for their clinical use, but also in healthy individuals wishing to maintain a healthy gut microbiota (Fleming et al., 2016; Su et al., 2020).
Probiotics and Microbiota: Inter-Kingdom Communication
Our bodies are composed of human cells and microbiota, which is composed of viruses, bacteria, fungi and parasites (Cao and Mortha, 2020). These complex and dynamic populations of microorganisms are crucial for maintaining health and playing a decisive defensive role against pathogens (Sokol, 2019). Inside the human body there exist different microbiotas according to their localization: skin, lung, urethra, vagina, etc. In the last decade, organs that had been previously considered sterile today are hypothesized to have a microbiota. For example, despite it was always thought that the fetus was developed under sterile conditions, recent data suggested the presence of microorganisms in the uterus and placenta (Agostinis et al., 2019; Tang et al., 2020). Moreover, contrary to a long-held dogma, today we know that human milk is not sterile (McGuire and McGuire, 2017). The hypothesis of how bacteria from the maternal gastrointestinal tract (GIT) are translocated to human milk is that dendrites from dendritic cells (DCs) could cross the gut epithelium and transport gut lumen bacteria to the mammary gland through the lymphoid system (Olivares et al., 2015; Demmelmair et al., 2020).
Nowadays, the gut microbiota is considered a new “vital organ” of the human body and is connected with other organs through different axis via neural, endocrine and immune interactions (Ding et al., 2019; Ahlawat et al., 2021). In this line, the consumption of probiotics has been reported to have beneficial effects on the gut–brain axis, the gut–skin axis, etc. (Banfi et al., 2021; Park et al., 2021). In addition, it has recently been demonstrated that the consumption of probiotics can modulate other microbiotas too, e.g., vaginal microbiota (Silvia Ventimiglia et al., 2021).
Fermented foods and probiotics (these terms should not be confused) increase gut microbiota diversity with benefits on human health (Aljutaily et al., 2020; Vinderola and Pérez-Marc, 2021). Frequently, a disruption in the microbiota composition results in a less diverse or less “rich” microbiota, which is often linked to a leaky gut syndrome, higher gut inflammation and more oxidative stress. This microbiota imbalance is linked to various diseases including obesity, diabetes, irritable bowel syndrome, inflammatory bowel disease, depression, and cardiovascular disease (Hills et al., 2019).
It is important to emphasize that many probiotic strains do not colonize the gut and are no longer recoverable in stool 1–4 weeks after stopping their consumption. For example, the probiotic-containing fermented milk Activia did not change the bacterial composition in the gut, but instead altered gene expression patterns that are relevant to carbohydrate metabolism in the gut microbiota. These changes in the gut function were confined only to the time of probiotic consumption (Maguire and Maguire, 2019).
Probiotics for Infectious Diseases
In the context of probiotics against infectious diseases, widespread effects or “shared benefits” of probiotics include mechanisms that act directly by inhibiting pathogens and indirectly by reinforcing the host epithelial barrier function and immune responses (Lebeer et al., 2010; Sassone-Corsi and Raffatellu, 2016; Raheem et al., 2021). Even though probiotic effects are strain-specific, in this review we collected a series of clinical trials where probiotic species benefits were assessed against infectious diseases (Figure 1). According to our search, nearly 50% of these species were reported to release EVs (Table 1). Seven out of 24 strains released EVs that have had beneficial effects against pathogens in in vitro, ex vivo or in vivo models (Table 1, indicated by asterisks). In fact, some of these strains are well-known probiotics: i.e., Escherichia coli Nissle 1917 and Lacticaseibacillus rhamnosus GG. We limited our search to bacteria, although some fungi are also considered to be probiotics.
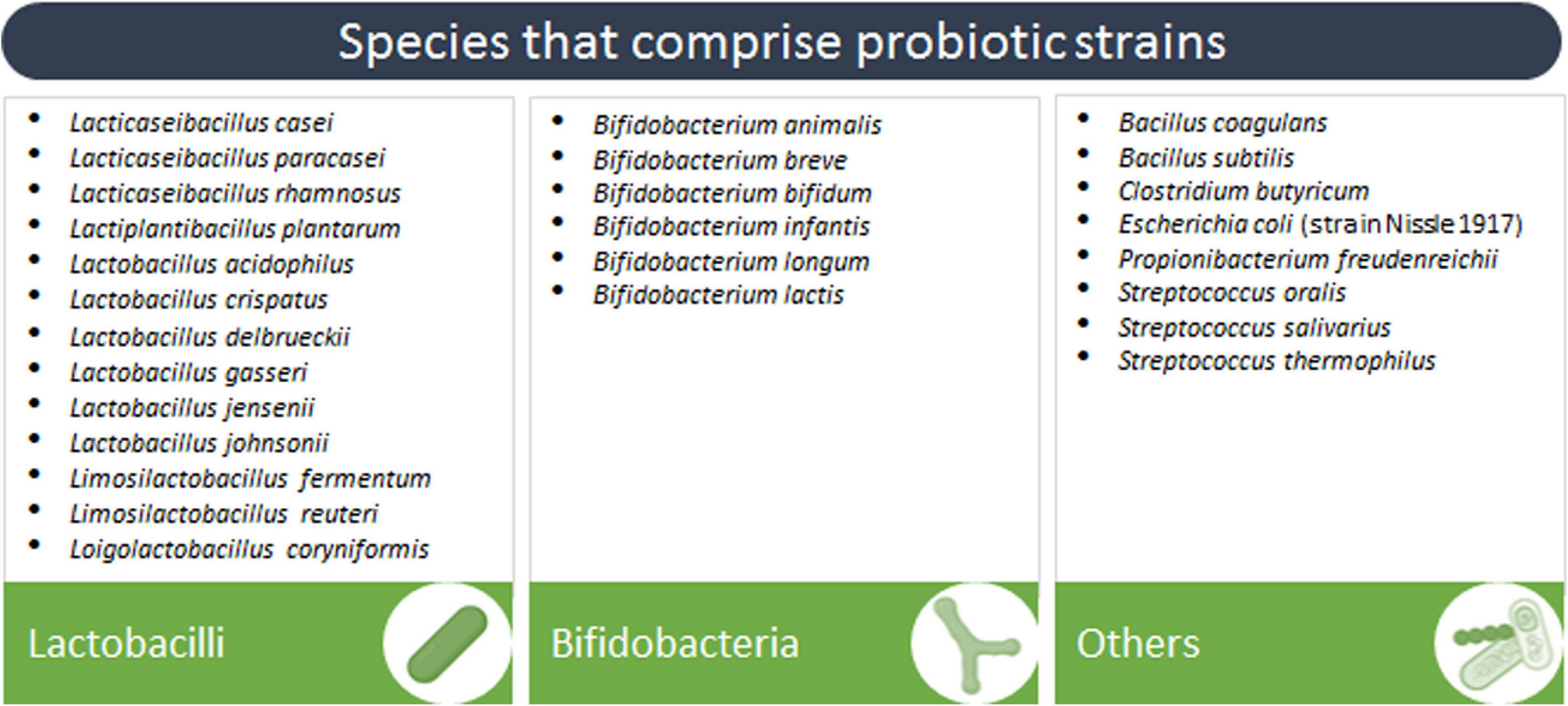
Figure 1. Species that comprise probiotic strains assessed for the prevention or treatment of infectious diseases in clinical trials.
Probiotic bacteria with successful results against infectious diseases mainly include bifidobacteria and lactobacilli, which represent the most studied probiotics (Stavropoulou and Bezirtzoglou, 2020), and other Gram (+) bacteria belonging to the genera Streptococcus, Bacillus, Propionibacterium and Clostridium. On the other hand, to our knowledge the only Gram (−) bacterial strain that was found to be effective in clinical trials is E. coli Nissle 1917. E. coli Nissle 1917 has been considered a probiotic for over a century and used to treat intestinal diseases. However, the strain contains a pathogenicity island (pks) that codes for colibactin, a genotoxin that mediates anti-inflammatory effects (Olier et al., 2012) and is now linked to causative mutations found in human colorectal cancer (Nougayrède et al., 2021).
Probiotics are commonly consumed in food or supplements (Hill et al., 2014; Figure 2). Oral administration, which is the most usual route of administration of probiotics, has resulted in satisfactory outcomes in clinical trials, even when the beneficial effect occurred in extraintestinal sites (Maldonado-Lobón et al., 2015; Panigrahi et al., 2017; Vladareanu et al., 2018; Lazou Ahrén et al., 2021). Possible mechanisms by which oral administration of probiotics may have extraintestinal and systemic effects on the host will be discussed in the following sections. However, there are many possible routes of administration of probiotics, such as mouth rinses and lozenges for periodontal disease (Tsubura et al., 2009; Invernici et al., 2018), vaginal suppositories for trichomoniasis, bacterial vaginosis and recurrent urinary tract infections (Stapleton et al., 2011; Sgibnev and Kremleva, 2020), intranasal administration for upper respiratory tract infections (Passali et al., 2019), and topical application for skin wounds (Peral et al., 2009). In the case of respiratory and skin infections, although topical administration could be advantageous (Lopes et al., 2017; Spacova et al., 2021), their study in clinical trials is currently underrepresented.
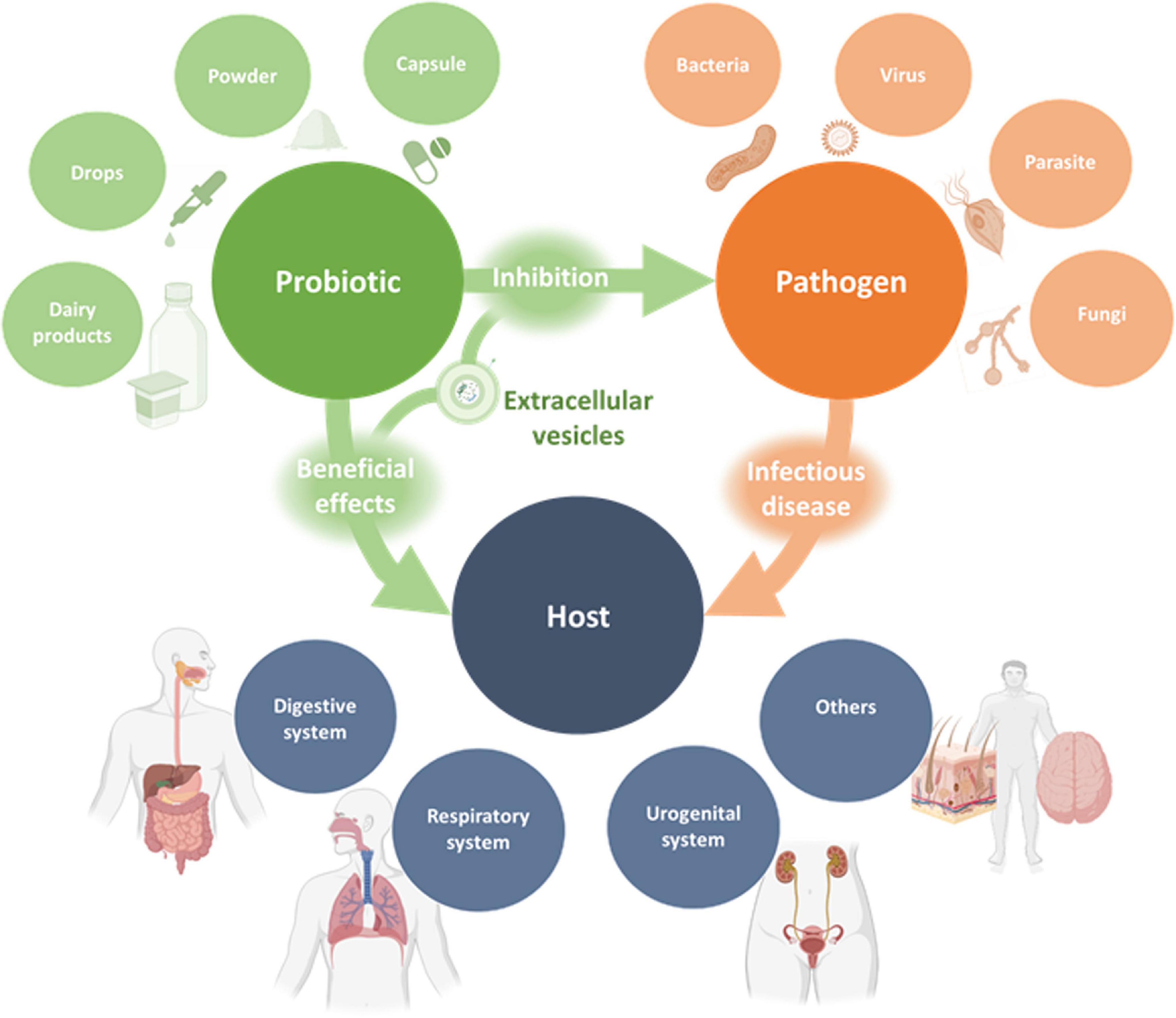
Figure 2. Schematic representation of the interactions between probiotics, pathogens and the host. Probiotics in various dosage forms were shown to exert beneficial effects on different human organ systems for the prevention or treatment of infectious diseases. These effects are exerted indirectly or directly through pathogen inhibition and may be mediated, at least in part, by probiotic-derived EVs.
It is important to note that many clinical trials examine the use of probiotics as a supplementation to conventional therapy against infectious diseases, such as antibiotic and antifungal agents (Shi et al., 2019; Joseph et al., 2021). In general terms, probiotics have shown effectiveness in preventing infectious diseases in different organ systems, from the respiratory and gastrointestinal tracts to the female urogenital system, among others. As regards gastrointestinal diseases, probiotics were effective in reducing frequency and duration of diarrhea (Francavilla et al., 2012; Park et al., 2017; Sharifi-Rad et al., 2020), reducing symptoms of gastroenteritis and H. pylori gastritis (Shafaghi et al., 2016; Shin et al., 2020), and preventing necrotizing enterocolitis (Chang et al., 2017). In respect of respiratory diseases, the benefit of probiotics has been mostly associated with prevention of infections, especially in the upper respiratory tract (Aryayev et al., 2018; Anaya-Loyola et al., 2019; Lazou Ahrén et al., 2021). Finally, certain probiotics were successful in reducing symptoms and frequency of recurrent vulvovaginal candidiasis, bacterial vaginosis and urinary tract infections (Laue et al., 2018; Russo et al., 2019; Sgibnev and Kremleva, 2020), possibly mainly by restoring the normal vaginal microbiota. Other benefits of probiotics demonstrated in clinical trials involve other organ systems, such as skin and the nervous system (Kotzampassi et al., 2015; Xia et al., 2018). Further high-quality clinical trials and meta-analyses should be undertaken to provide stronger evidence for the therapeutic use of probiotics (Stavropoulou and Bezirtzoglou, 2020).
Extracellular Vesicles
Bacterial Extracellular Vesicles
Probiotics seem to act through a wide repertoire of mechanisms but the specific pathways and key regulatory molecules underlying their beneficial effects are largely unknown (Plaza-Diaz et al., 2019). In this line, EVs have been associated with diverse functions in cell-to-cell communication and appear to be a common language between kingdoms (i.e., bacteria and eukaryotic cells) (Ñahui Palomino et al., 2021).
Extracellular vesicles are produced by all domains of life: archaea, bacteria and eukarya. To this day it has been seen that EVs appear to be produced by all cell types of all studied organisms. All EVs are composed of a lipid bilayer with membrane proteins and contain DNA, RNA and proteins (Théry et al., 2018). The level of knowledge about bacterial EVs is lower than eukaryotic EVs, but the number of studies is continuously increasing (Ñahui Palomino et al., 2021). In particular, EVs from Gram (+) bacteria have been less studied, and our understanding of their biogenesis and interaction with host cells is just being started (Briaud and Carroll, 2020).
The size of bacterial EVs is in the nanoscale (below 500 nm), and has been related to bacterial physiology including probiotic and pathogenic effects. In the case of Gram (+) bacteria, EVs are called membrane vesicles (MVs) and the lipid bilayer encloses cytosolic material. In contrast, in the case of Gram (−) bacteria, EVs are called outer-membrane vesicles (OMVs) and the lipid bilayer encloses periplasmic material. Gram (+) and Gram (−) bacterial EVs are also different in their surface composition for example the presence of lipopolysaccharide (LPS). The diversity in cargo molecules contained in EVs might explain the variety of described roles ranging from decoys for viral and antibiotic attack, quorum sensing as well as regulation of host immune defense (Kaparakis-Liaskos and Kufer, 2020).
Postbiotics, a New Concept
As mentioned before, probiotics comprise live microorganisms that confer a health benefit on the host when administered in adequate amounts. At the same time, there is increasing evidence of the health effects of non-viable microorganisms and their bioactive compounds (metabolites that they can produce by fermentation or by their action on food components) (Collado et al., 2019). An expert panel of ISAPP defined a postbiotic as a “preparation of inanimate microorganisms and/or their components that confers a health benefit on the host” (Salminen et al., 2021). In this line, EVs are secretory components associated with probiotic bacteria health benefits and consequently could be considered postbiotics (Wegh et al., 2019).
Extracellular vesicles play a central role in many physiological and pathological processes due to their capacity to transport biologically active macromolecules that can effectively alter the biological properties of target cells. Due to this property, they can be considered novel agents with different therapeutic applications. There are many clinical trials investigating the use of human EVs for various therapeutic approaches, including pathogen vaccination, anti-tumor therapy, regenerative therapies and drug delivery (Lener et al., 2015; Théry et al., 2018). In the case of EVs against infectious diseases there exist two different strategies: evaluation of EVs released naturally by the pathogen or infected cells, and EVs from in vitro antigen-pulsed DCs (Wahlund et al., 2017; Riley and Blanton, 2018; Santos and Almeida, 2021). However, to our knowledge there are no clinical trials related to the use of EVs from probiotic bacteria for the prevention and/or treatment of any infectious disease.
Extracellular Vesicles From Probiotic Bacteria and Infectious Diseases
In order to organize the information, we divided the current evidence of the knowledge about the role of EVs as mediators of probiotic beneficial effects into six categories. The first category addresses the role of EVs against pathogens. The second and third categories are related to their function in the host immune system that can also be divided into three lines of defense: physical and chemical barriers, innate immunity and adaptive immunity. The last categories describe EVs composition, how EVs are uptaken and transported across human cells and other functions.
Pathogen Inhibition
Probiotics can inhibit pathogens through production of antimicrobial agents and through competitive exclusion of pathogens by competing for adhesion or nutrients in the GIT (Surendran Nair et al., 2017; van Zyl et al., 2020; Raheem et al., 2021).
Antimicrobial agents mainly include reactive oxygen species, lactic acid, and bacteriocins (Rajilić-Stojanović, 2013). Bacteriocins are peptides with antimicrobial activity that have shown to inhibit not only bacteria, but also viruses, fungi and parasites (Dicks and Grobbelaar, 2021; Huang et al., 2021). Furthermore, bacteriocins might be an interesting alternative to the use of antibiotics for infectious diseases caused by antibiotic-resistant bacteria due to their high potency and low toxicity (Cui et al., 2021; Gradisteanu Pircalabioru et al., 2021).
Recent studies show that EVs released by L. acidophilus ATCC 53544 could deliver bacteriocins and thus kill other bacteria (Dean et al., 2019, 2020). Proteomic analyses revealed bacteriocins are enriched in EVs. Even though bacteriocins investigated by these authors are directed against a L. delbrueckii strain (Dean et al., 2020), other bacteriocins synthesized by probiotics are able to inhibit or kill pathogens, such as Listeria monocytogenes, Staphylococcus aureus, Acinetobacter baumannii, Gardnerella vaginalis, Streptococcus agalactiae, and Pseudomonas aeruginosa, in both in vitro and in vivo models (Gaspar et al., 2018; van Zyl et al., 2019; Hassan et al., 2020). It is noteworthy that EVs may protect bacteriocins from proteases and inactivation molecules that are normally present in the intestine. Whether EVs from probiotics can deliver bacteriocins to pathogens is still unknown and holds great potential for future research.
Several clinical trials have shown that probiotics improved vaginal microbiota composition (Ho et al., 2016; Laue et al., 2018; Vladareanu et al., 2018) and it has been demonstrated that a vaginal microbiota dominated by lactobacilli prevents infections caused by various pathogens, including HIV-1 (Chee et al., 2020). A possible relevant mechanism of EVs related to pathogen inhibition is their ability to prevent pathogen interaction with host cells. It has been demonstrated that some L. crispatus and L. gasseri EVs reduced HIV-1 attachment to host cells and in this way prevented infection in human cell lines and tissues (Ñahui Palomino et al., 2019). This effect was associated with the reduced accessibility of gp120 (a viral envelope protein) to host target cells after incubating HIV-1 virions with EVs.
Regarding competitive exclusion, probiotics can compete with enteric pathogens for adhesion sites on the mucus layer or on intestinal epithelial cells, and hence prevent pathogen colonization and infection (van Zyl et al., 2020). Competitive exclusion of pathogens has been demonstrated in in vitro models (Singh et al., 2017; Tuo et al., 2018), and possibly takes place not only in the GIT but also in the oral cavity and urogenital tract. Numerous authors have investigated the role of pathogenic bacteria EVs in transporting virulence factors and toxins into host cells (Macion et al., 2021). On the other hand, to the best of our knowledge, the only existing report of EVs from probiotics mediating the competition between pathogenic and probiotic bacteria was published by Kim et al. (2018). In this study, it was shown that EVs from L. plantarum prevented skin inflammation in a murine model of S. aureus EV-induced atopic dermatitis. Concerning the GIT, EVs from probiotics expose adhesion proteins that may interact with the mucus layer and human cells. Although it is likely that this interaction may affect viral and bacterial attachment, there remains a need for in vitro and in vivo studies addressing this question.
Barrier Function: Physical and Chemical Defense
The intestinal epithelial barrier acts as the first line of defense by avoiding the entrance of antigens and pathogens (Barbara et al., 2021). The alteration of the gut microbiota is the most important factor that disrupts the integrity of the intestinal epithelial barrier, leading to intestinal inflammation and diseases (Gareau et al., 2010). Probiotics, in this context, as transient constituents of the microbiota, are able to improve barrier function by surface components and secreted factors (postbiotics), among them, EVs (Liu et al., 2020). Since the exposure to infection can lead to the loss of epithelial integrity (König et al., 2016; Invernizzi et al., 2020), probiotic EVs participation in the improvement of barrier function could be an important point to regard them as potential prophylactic or therapeutic agents against infections.
As for the physical barrier, in vitro and in vivo experiments have demonstrated that EVs released by E. coli Nissle 1917 can mediate anti-inflammatory effects and protect the intestinal epithelial barrier function (Alvarez et al., 2016; Fábrega et al., 2016, 2017). A key role in the maintenance of intestinal epithelial barrier integrity is played by tight junctions, which are composed of a network of proteins that regulate paracellular permeability, such as claudins, zonula occludens (ZO) and occludin (Barbara et al., 2021). EVs released by E. coli Nissle 1917 have been shown to upregulate ZO-1 and claudin-14, downregulate claudin-2 (a gene that codes for a leaky protein), and in turn improve epithelial barrier function in an in vitro intestinal epithelium models (T-84 and Caco-2 cell lines) (Alvarez et al., 2016). This function of EVs has also been reported in these same cell lines infected with enteropathogenic E. coli (EPEC), an enteric pathogen that disrupts tight junctions as a way to increase invasion. In this work, EVs released by E. coli Nissle 1917 were able to counteract EPEC-altered mRNA levels of claudin-14 and occludin, preserve subcellular localization of ZO-1 and occludin, and maintain F-actin at the intercellular junctions. Barrier integrity restoration was further confirmed by measuring transepithelial electrical resistance (TEER) and the flux of FITC-dextran (Alvarez et al., 2019). In addition, restoration of epithelial integrity by EVs has been observed in an in vivo model of experimental colitis (Fábrega et al., 2017). In this regard, these authors demonstrated that oral administration of EVs from E. coli Nissle 1917 increased: trefoil factor 3 (TFF-3) mRNA levels, a marker of intestinal barrier function; and decreased MMP-9 mRNA levels, a protein involved in tissue injury.
Regarding the intestinal chemical defense, antimicrobial peptides and the mucus layer (mainly produced by the goblet cells) are further key factors that maintain intestinal barrier integrity by protecting epithelial cells from bacteria and other challenges (Hansson, 2020; Yong et al., 2020; Barbara et al., 2021; Fusco et al., 2021). In an in vivo model of experimental colitis, treatment with EVs from E. coli Nissle 1917 resulted in the restoration of the mucin content in goblet cells and in a smaller ulceration surface, as evidence of barrier integrity (Fábrega et al., 2017). On the other hand, a recent study conducted by Gu and colleagues showed that EVs from L. rhamnosus GG increased nuclear factor erythroid 2-related factor 2 (Nrf2) expression and, in turn, increased tight junction proteins and antimicrobial peptide Reg3 levels, which is involved in the prevention of Listeria monocytogenes and Salmonella enteritidis infections (Loonen et al., 2014; Gu et al., 2021). Furthermore, mRNA Reg3 levels increased after incubation of Caco-2 cells with EVs from L. plantarum WCFS1 (Li et al., 2017). In an in vivo model, the administration of these EVs prolonged the survival of Caenorhabditis elegans infected with vancomycin-resistant enterococci. In this line, EV-mediated protection against antimicrobial resistant pathogens could be useful to limit the development of antibiotic resistance that results from the widespread use of antibiotics.
Innate and Adaptive Immunity
As mentioned before, intestinal epithelial cells provide a physical barrier that separates the host from the external environment and form not merely static physical barriers: on the contrary, intestinal epithelial cells engage in a complex dynamic crosstalk between the microbiota and the intestinal immune system (Takiishi et al., 2017). Both bacteria and host-derived EVs are key players of such inter-kingdom crosstalk. There is now an accumulating body of evidence that bacterial EVs regulate the innate and adaptive immune system of the host. Consequently, EVs released by the gut microbiota may have great influence on human health and disease. EVs also carry a set of molecules known as microbe-associated molecular patterns (MAMPs) that are recognized by specific receptors expressed by host epithelial and immune cells. These pattern recognition receptors (PRRs), such as TLR2 and NOD1, are key components of innate immunity and mediate host responses (Lebeer et al., 2010; Díaz-Garrido et al., 2021).
Maintaining the proper balance of immune responses at mucosal surfaces is critical for maintaining homeostasis and successfully clearing pathogens. Epithelial cells have been identified as key players in the development of elaborate immune responses that discriminate between non-pathogenic and pathogenic microorganisms. In this regard, intestinal epithelial cells contribute to delaying and dampening infections by initiating the development of an immune response and attracting immune cells to the infectious site (Pellon et al., 2020). Among intestinal epithelial cells, enterocytes are the most abundant cells and represent approximately 90% of the total number. To study absorption and immune responses there are different in vitro models of cell lines: Caco-2, HT-29, and T-84. The remaining 10% of the cells consist of mucus-producing goblet cells, enteroendocrine cells, antimicrobial peptide-producing Paneth cells and others (Jochems et al., 2018).
In vitro and in vivo experiments with intestinal epithelial cells have demonstrated that EVs released by E. coli, L. casei, L. paracasei, P. freudenreichii, and L. rhamnosus can modulate NFκB levels (Cañas et al., 2018; Bäuerl et al., 2020; Choi et al., 2020; Vargoorani et al., 2020; Tong et al., 2021). NF-κB is a family of transcription factors and has an essential role in a variety of aspects related with human health including the development of both innate and adaptive immunity. EVs from L. paracasei and P. freudenreichii decreased NF-κB levels in LPS-induced inflammation in HT-29 cell line (Choi et al., 2020; Rodovalho et al., 2020). At the same time, L. rhamnosus EVs had the same effect in an in vivo model of dextran sulfate sodium-induced colitis in mice (Tong et al., 2021). On the other hand, EVs from E. coli and L. casei increased NF-κB levels per se in Caco-2 and HT-29 cell lines (Cañas et al., 2018; Bäuerl et al., 2020). This opposite modulation of NFκB levels, in the presence or absence of LPS, was also observed for pro-inflammatory cytokines like IL-8 in both Caco-2 and HT-29 cell lines, and in ex vivo human colonic explant (Fábrega et al., 2016; Choi et al., 2020; Vargoorani et al., 2020).
On the contrary, in the presence or absence of LPS, L. casei and L. paracasei EVs always increase the levels of anti-inflammatory cytokines like IL-10. The inhibition of the NF-κB pathway and the increase of IL-10 by EVs have been extensively reported for probiotic bacteria in both in vitro and in vivo models of infection and/or inflammation (Liu et al., 2017; Bhardwaj et al., 2020). Moreover, Fábrega and colleagues demonstrated in an in vivo model of dextran sulfate sodium-induced colitis in mice that E. coli Nissle 1917 EVs decreased COX-2 and iNOS mRNA levels that encode important inducible enzymes for the synthesis of prostaglandins and nitric oxide, respectively. This decrease in COX-2 and iNOS levels leads to inflammation and tissue damage, and correlated with the reduced expression of the pro-inflammatory cytokines TNF-and IFN-γ, lower colon inflammation and tissue damage in EV-treated mice (Fábrega et al., 2016, 2017). This evidence suggests that EVs could mediate, at least in part, the beneficial effect of probiotics against infectious diseases.
With regard to immune cells, EVs from different species increase per se the levels of pro-inflammatory cytokines like TNF-alpha and IL-6 (Fábrega et al., 2016; Hu et al., 2020; Gu et al., 2021; Morishita et al., 2021) and, at the same time, increase the level of anti-inflammatory cytokines like IL-10 and IL-22 produced by macrophages, DCs and peripheral blood mononuclear cells (PBMC) (López et al., 2012; Al-Nedawi et al., 2015; Fábrega et al., 2016; Hu et al., 2020). In agreement with this effect, it has been reported that different probiotic bacteria stimulate pro-inflammatory and/or anti-inflammatory cytokines in different immune cells (Ren et al., 2016; Cristofori et al., 2021).
Modulation of the immune system by bacterial EVs has also been studied against pathogens in in vitro models. EVs from L. rhamnosus GG and L. reuteri DSM 17938 decreased inflammatory mediators like IFN-γ and IL-17A in S. aureus-stimulated human PBMC (Mata Forsberg et al., 2019), while EVs from the probiotic strain E. coli Nissle 1917 improved the antibacterial activity of macrophages against three bacterial pathogenic strains of E. coli, S. typhimurium, and S. aureus (Hu et al., 2020).
Regarding macrophage differentiation, L. plantarum APsulloc 331261 EVs induced monocyte-to-macrophage transition and polarization to M2b in human THP-1 (Kim et al., 2020). M2b, a subtype of M2 macrophages, has attracted increasing attention due to its strong immunoregulatory and anti-inflammatory effect (Wang et al., 2019). Probiotic bacteria are reported to have a beneficial effect on the host immune status through their ability to modulate macrophage polarization. Some probiotic strains are reported to activate macrophages to M1 phenotype to kill intracellular pathogens, while some other probiotics can induce M2 macrophages to exert an anti-inflammatory effect. Similarly, another strain of the same species (L. plantarum CLP-0611) also ameliorated colitis in mice by polarizing M1 to M2-like mouse peritoneal macrophages (Jang et al., 2014).
In line with the anti-inflammatory effects of bacterial EVs, L. paracasei and L. reuteri BBC3 EVs increased mRNA levels of TGF-β in a model of LPS-induced inflammation in human intestinal epithelial cells (HT-29) and jejunum tissues from chicken (Choi et al., 2020; Hu et al., 2020). TGF-β plays a critical role in the development of Treg cells (Zhao et al., 2017). At the same time, B. bifidum LMG13195 and L. rhamnosus JB-1 EVs incubation with human DCs induced differentiation to Treg cells and increased IL-10 levels (López et al., 2012), and L. rhamnosus EVs increased the number of Treg cells in Peyer’s patch from mice (Al-Nedawi et al., 2015). While in some instances Treg cells appear to limit the efficiency of antiviral protective immunity, in other cases they reduce the level of tissue damage caused by a virus infection (Veiga-Parga et al., 2013).
Regarding adaptive immunity, vaccination with engineered EVs from the probiotic strain E. coli Nissle 1917 in mice increased the levels of IgG against a recombinant antigen comparable to the “gold standard” adjuvant (alum) (Rosenthal et al., 2014). This strong adjuvant capability of EVs from probiotic strains provides evidence that engineered EVs could be a useful platform for vaccines in humans. On the other hand, it is interesting to note that L. johnsonii N6.2 EVs are recognized by IgA and IgG from the plasma of individuals who had consumed the probiotic. In particular, the increase of IgA occurs as a result of a specific response to EV components: Sdp_SH3b2 and Sdp_SH3b6 (Harrison et al., 2021). Although the function of bacterial SH3b domains is not completely known, they are proposed to be cell wall binding domains in prokaryotes. In a previous work, the authors had shown that L. johnsonii N6.2 increased circulating levels of IgA (Marcial et al., 2017). Moreover, it has been demonstrated that L. sakei EVs enhanced IgA production by murine Peyer’s patch cells (Yamasaki-Yashiki et al., 2019). A similar study found that commensal bacteria increase the serum levels of IgA, providing a protective effect against polymicrobial sepsis (Wilmore et al., 2018). Therefore, serum IgA concentrations depend on the interaction with the gut microbiota and these effects could be mediated, at least in part, by EVs.
It is interesting to note that L. plantarum KCTC 11401BP EVs decreased IL-6 levels, protected cell viability of human epidermal keratinocytes (HaCaT) incubated with S. aureus EVs and reduced skin inflammation in S. aureus EV-induced atopic dermatitis in mice (Kim et al., 2018). Moreover, L. plantarum EVs increased IL-10 and granulocyte Macrophage Colony-Stimulating Factor (GM-CSF) levels in ex vivo human skin cultures (Kim et al., 2020). These findings suggest that oral administration of bacteria could have a preventive effect on skin inflammation and these effects could be mediated by EVs. As mentioned below in Section “Uptake and Transport,” L. rhamnosus JB-1 EVs appeared in blood after oral consumption and consequently the presence of EVs in the bloodstream could in part explain the benefit of probiotics in extraintestinal tissues and organs (Stentz et al., 2018; Champagne-Jorgensen et al., 2021a).
Composition
Throughout the years, it has been shown that the supernatant from probiotic bacteria exert beneficial effects in both in vitro and in vivo models (De Marco et al., 2018; Mantziari et al., 2020). For instance, the culture supernatant from L. rhamnosus GG induces resistance to Escherichia coli K1 infection by enhancing intestinal defense in neonatal rats (He et al., 2017). In recent years, with the discovery of EVs from probiotics, we can speculate that at least part of these beneficial effects could be mediated by EV components.
As far as we know, there are differences in EV metabolite, nucleic acid and protein content compared with that of the bacterial cell (Briaud and Carroll, 2020). The relative abundance of certain components suggests not only a possible sorting mechanism to package EV cargo, but also a special biological role for EVs (Kim et al., 2018; Huang et al., 2021). For example, EVs from L. rhamnosus GG contain high levels of tryptophan metabolites that lead to an improved barrier function (Gu et al., 2021).
In the last few years, “omics” approaches, such as proteomics, transcriptomics and metabolomics, have enabled a comprehensive characterization of probiotics and their EVs, allowing us to gain a deeper understanding of their mechanisms of action (Cunningham et al., 2021). Proteomic analyses showed that EVs from Lacticaseibacillus genus (including L. casei and L. rhamnosus species) contain p40 and p75, two proteins associated with probiotic effects (Domínguez Rubio et al., 2017; Dean et al., 2019; Gu et al., 2021). In particular, p40, when administered in early life, increased TGF-β levels in mice and consequently prevented intestinal inflammation in adulthood (Gu et al., 2021). These proteins, p40 and p75, are able to induce the phosphorylation of the epidermal growth factor receptor (EGFR), and thus have anti-apoptotic effects, as demonstrated in intestinal epithelial cells (Zhang et al., 2020). For p40, this effect was also observed in a murine model of colitis (Yan et al., 2011). EGFR activation can also be triggered by L. casei EVs, which expose p40 and p75 at the surface (Bäuerl et al., 2020). Intriguingly, EVs from L. rhamnosus GG were shown to have apoptotic effects in hepatic cancer cells by the intrinsic pathway of apoptosis (Behzadi et al., 2017). Therefore, apoptotic effects seem to depend on the dose of EVs and on the model used. On the other hand, p40 and p75 were able to prevent the disruption of tight junctions by protein kinase C (PKC)-dependent mechanisms in Caco-2 cell monolayers (Seth et al., 2008). Anti-apoptotic effects and protection of tight junctions in intestinal epithelial cells are related to an enhancement of intestinal epithelial integrity, a key factor in the maintenance of barrier function, the first line of defense. On the other hand, p40 was proven to increase IgA levels. As mentioned in Section “Innate and Adaptive Immunity,” IgA further contributes to the protection of the host against infections (Ho et al., 2016; Wang and Jeffery, 2016).
It has been shown that EVs from probiotics contain proteins that could mediate pathogen inhibition, and in this way could possibly compete with pathogens for colonization in the intestine (Domínguez Rubio et al., 2017; Bajic et al., 2020; Bäuerl et al., 2020; Nishiyama et al., 2020). Proteomic analyses of EVs from three different lactobacilli strains showed that protein composition of EVs can be very different among species (Dean et al., 2019). Interestingly, antimicrobial bacteriocins are enriched in EVs from L. acidophilus ATCC 53544. These EVs can fuse with other bacteria and thus may constitute a useful platform for the delivery of antimicrobial compounds (Dean et al., 2020). On the other hand, it would be interesting to investigate the occurrence of moonlighting proteins in EVs. Moonlighting proteins are proteins that have different functions according to their cellular location (Wang and Jeffery, 2016; Jeffery, 2018). For example, glyceraldehyde-3-phosphate dehydrogenase (GAPDH), a well-known cytoplasmic metabolic protein, is exposed at the surface of the bacterial cell and performs adhesion functions. Further analyses are necessary to confirm the localization of these proteins within EVs to better understand their multiple functions.
Indeed, EV composition is relevant to understand their biological function, even in the context of infections. EVs from L. crispatus BC3 and L. gasseri BC12, but not EVs from other strains, were capable of protecting vaginal tissues from HIV-1 infection ex vivo, suggesting virus inhibition was due to the presence of specific components of EVs (Ñahui Palomino et al., 2019). Regarding immunomodulatory effects of EVs from probiotics, EVs from Propionibacterium freudenreichii contain surface-layer protein B (SlpB), which effectively mitigated NF-κB activation (Rodovalho et al., 2020).
Lipoteichoic acid (LTA) has been found on the surface of EVs from L. gasseri JCM 1131, L. casei BL23 and L. rhamnosus JB-1 (Shiraishi et al., 2018; Champagne-Jorgensen et al., 2021b). LTA is a ligand for TLR2 in a heterodimer with TLR6, and it seems to induce immune tolerance in intestinal epithelial cells (Lebeer et al., 2010). In agreement with this, EVs from L. rhamnosus JB-1 expose LTA, which was responsible for TLR-2 activation and increase of IL-10 production by bone marrow-derived DCs (Champagne-Jorgensen et al., 2021b). LTA from probiotics could play a role in attenuating infections. In this regard, it has been shown that L. plantarum LTA inhibits virus-induced inflammatory responses in porcine intestinal epithelial cells and reduced Enterococcus faecalis biofilm in vitro (Kim et al., 2017, 2020).
As mentioned before, peptidoglycan contained in EVs from Gram (+) and Gram (−) probiotics is also an important factor in the enhancement of innate immunity and the maintenance of intestinal homeostasis (Cañas et al., 2018; Morishita et al., 2021). In fact, EVs from Bifidobacterium longum, Clostridium butyricum, and L. plantarum WCFS1 have been proposed as a novel immunotherapy formulation that would be advantageous over bacterial lysates due to protection from degradation of bioactives within EVs (Morishita et al., 2021).
As aforesaid, EVs from the Gram (−) probiotic strain E. coli Nissle 1917 were shown to have a strong adjuvant capability. The authors ascribed this result to LPS, proteins and glycosyl composition (Rosenthal et al., 2014). The presence of LPS and other MAMPs, such as flagellin and mannose, may be responsible for the strong immune response when applying these EVs as vaccine platforms.
Previous work has established that bacterial EVs contain DNA and RNA (Koeppen et al., 2016; Bitto et al., 2017; Li and Stanton, 2021). Regarding EVs from probiotics, little is known about their nucleic acid cargo. Even though DNA and RNA were found in EVs from L. reuteri BBC3 and L. casei BL23 (Domínguez Rubio et al., 2017; Hu et al., 2021), the characterization of nucleic acids from probiotics remains to be studied. Small RNA contained in EVs from probiotics might possibly regulate gene expression in host cells, as it is the case for EVs from pathogenic bacteria, and this interaction could have implications in preventing and treating infections (Lee, 2019; Munhoz da Rocha et al., 2020).
Extracellular vesicles from probiotics have shown to contain phage nucleic acids (Domínguez Rubio et al., 2017; Champagne-Jorgensen et al., 2021b; Gu et al., 2021) and phage proteins (Domínguez Rubio et al., 2017; Gu et al., 2021). EVs can even transmit phage receptors to phage-resistant bacteria, which in turn become phage-sensitive (Tzipilevich et al., 2017). Both phage nucleic acid and phage-receptors transmission would lead to a broadened phage host range with potential applications in the treatment of infections (Liu et al., 2018).
Uptake and Transport
The communication between bacteria and the host could in part occur through bacterial EVs and other soluble factors (postbiotics). EVs are able to transport diverse bioactive molecules to host cells and trigger different effects such as the modulation of immune responses. It is generally accepted that, due to their nanosize, bacterial EVs can overcome epithelial barriers and migrate long distances in the human body (Macion et al., 2021). In fact, bacterial EVs have been demonstrated to enter host cells by several routes, including clathrin, caveolin or lipid raft mediated endocytosis, and membrane fusion (O’Donoghue and Krachler, 2016). Even though much research in the last decades has focused on the study of uptake and transport of EVs released by pathogenic bacteria (Bielaszewska et al., 2017; Bitto et al., 2017), few researchers have addressed these issues for EVs released by non-pathogenic bacteria. However, in the last years there has been an increase in research trying to understand the way that EVs from probiotics are internalized by host cells, or even more, transported through the intestinal barrier and delivered to different tissues and organs.
Before being uptaken by intestinal cells, EVs must also diffuse through the mucus layer. In this regard, EVs from E. coli Nissle 1917 were able to diffuse through the mucus layer in the mucin-producer HT29-MTX cell line (Cañas et al., 2016). Although there is no direct evidence of EV diffusion through the mucus layer in vivo, this event can be assumed from the fact that EVs can reach the bloodstream after oral administration (Champagne-Jorgensen et al., 2021a).
Extracellular vesicles from probiotics were proven to be internalized by intestinal epithelial cells in several studies (Fábrega et al., 2016; Bajic et al., 2020; Domínguez Rubio et al., 2020; Champagne-Jorgensen et al., 2021b). Although there are several routes of entry for EVs from pathogens into epithelial cells, clathrin-mediated endocytosis has been the most widely reported route among EVs from probiotics so far. Inhibitors of clathrin-mediated endocytosis, such as chlorpromazine and the dynamin dynasore, blocked the uptake of EVs by intestinal cells cultivated in vitro (Cañas et al., 2016; Bajic et al., 2020; Champagne-Jorgensen et al., 2021b). Additionally, EVs from L. rhamnosus JB-1 were shown to be internalized by intestinal epithelial cells in an in vivo model within 2 h after oral consumption (Champagne-Jorgensen et al., 2021a). It is likely that EVs are internalized simultaneously by different endocytic pathways depending on their size (El-Sayed and Harashima, 2013).
With respect to intracellular trafficking, colocalization analyses showed that EVs from E. coli Nissle 1917 are present in early endosomes and, once inside the cell, EV peptidoglycan interacts with NOD1 that leads to the activation of the immune system (Cañas et al., 2016, 2018; Fernández-García et al., 2021). Moreover, EVs can also fuse with lysosomes (Cañas et al., 2016). On the other hand, it was demonstrated that EVs from a pathogenic E. coli strain can deliver toxins to other subcellular compartments including the cytosol, nucleus and mitochondria (Bielaszewska et al., 2017). Bacterial EVs can deliver DNA or RNA to host cells (Bitto et al., 2017; Lécrivain and Beckmann, 2020), and there is evidence that nucleic acid cargo of EVs from pathogens may enter the nucleus of eukaryotic host cells (Blenkiron et al., 2016; Bitto et al., 2017). Furthermore, EVs from pathogens contain small RNA that might regulate gene expression in host cells (Koeppen et al., 2016). Although little studied to date, these mechanisms may be also applicable to EVs from probiotics.
While a portion of EVs may act in intestinal cells, another portion is possibly transported through the intestinal epithelium, either by paracellular or transcellular transport, to finally reach extraintestinal tissues and organs (Jang et al., 2015; Stentz et al., 2018; Jones et al., 2020). Park et al. (2017) revealed EVs from intestinal bacteria reach the bloodstream in a mouse model, where blood EV diversity was directly linked to intestinal microbiota diversity. Regarding probiotics, a proportion of CFSE-labeled EVs from Bacillus subtilis were transported through a monolayer of polarized epithelial cells in a transwell system. Transcellular transport resulted in the detection of intact EVs in the lower chamber in 60–120 min (Domínguez Rubio et al., 2020). Alternatively, EVs could possibly be transported through the intestinal epithelium via DCs, goblet cells or M-cells. On the other hand, microbiota EV transport through the epithelium can occur by paracellular transport when the intestinal epithelial barrier integrity is compromised (Chronopoulos and Kalluri, 2020).
The transport of EVs across the intestinal epithelium implies that EVs could reach the lamina propria, where they are able to interact with immune cells. EV uptake by immune cells has been described in a few studies. In vivo studies showed that EVs from L. rhamnosus JB-1 were uptaken by DCs in the lamina propria (Champagne-Jorgensen et al., 2021b). This internalization was thought to occur via clathrin-mediated endocytosis, as it was prevented by dynasore, even though phagocytosis cannot be ruled out since dynamin is required for this process. In another study, probiotic-derived EVs were uptaken by mouse macrophage-like and DCs via clathrin-mediated endocytosis and macropinocytosis, as demonstrated in the presence of endocytosis inhibitors (Morishita et al., 2021).
Different studies support EVs distribution and delivery to distal body sites. For example, EVs from L. rhamnosus JB-1 were present in the bloodstream of mice fed with the bacteria (Champagne-Jorgensen et al., 2021a), as demonstrated by the detection of DNA from prophages in EVs. What is more, oral administration of EVs from L. plantarum reduced skin inflammation in mice with S. aureus EV-induced atopic dermatitis (Kim et al., 2018). In humans, microbiota-derived EVs were able to reach urine. In fact, urine-EVs were proposed as a useful assessment method of microbiota profiles (Li et al., 2017). In another study, intraperitoneally injected EVs from L. plantarum increased brain-derived neurotrophic factor (BDNF) mRNA levels in the hippocampus of mice and produced antidepressant effects (Choi et al., 2019). This increase in gene expression in the brain suggests that EVs might possibly cross the brain blood barrier. Indeed, EV transport could be one of the reasons why probiotics consumption exerts not only local but also systemic effects, since it is likely that EVs are released by probiotics in the GIT after the consumption of these bacteria.
Other Biological Effects
As it can be inferred from probiotic beneficial effects, modulation of symptoms is one important factor that explains the clinical efficacy of probiotics in the treatment of infectious diseases. It is often observed that EVs mimic the effect of the parent bacteria. For example, L. reuteri DSM 17938 clinical efficacy has been demonstrated for the treatment of colic, diarrhea and constipation (Coccorullo et al., 2010; Chau et al., 2015; Dinleyici et al., 2015). Accordingly, EVs from this strain could reproduce the bacteria beneficial effects on gut motility in jejunum and colon explants from mice (West et al., 2020). Therefore, EV release could be one mechanism whereby probiotics mediate their beneficial effects.
In relation to stress and immunity, chronic stress leads to constantly high corticosteroid levels in blood, an impaired immune function, and an increased susceptibility to infections and other health disorders (Bae et al., 2019). At the same time, exposure to stress can cause a decrease in the expression of BDNF in humans, a molecule with antidepressant-like effects (Yang et al., 2015). Some probiotics were shown to be antidepressants in patients and animal models, and even though the gut–brain axis is involved in this effect, the mechanisms of action are not completely understood (Yong et al., 2020). EVs might come into play here. In this regard, EVs from L. plantarum KCTC 11401BP counteracted the decreased levels of BDNF mRNA in the hippocampus of corticosteroid treated mice and also blocked the decrease in the levels of BDNF mRNA in corticosteroid post-treated mice, which was further evidenced in mice antidepressant behavior (Choi et al., 2019). If the anti-depressant effects of EVs are proven, they could possibly participate in preventing and/or treating infections given that immune function may be impaired in patients with depression (Andersson et al., 2016).
Discussion
Potential Use of Extracellular Vesicles
The use of EVs as delivery systems could provide several advantages including their nanosize, their biocompatibility in comparison to synthetic drug delivery systems (low toxicity), the ability to cross biological barriers, their ability to protect their cargo from unfavorable environmental conditions (pH, enzymes, oxidative stress) and the possibility of engineering parent cells to modify EV composition (Figure 3). There is still a huge gap between basic research and clinical trials as far as bacterial EVs are concerned.
Postbiotics are a novel clinical strategy to consider for the treatment of infections in absence of cells. For example, in diabetic foot ulcers, skin barrier is impaired and thus administration of live bacteria is not a safe approach (Nam et al., 2021). Here is where probiotic-derived EV administration could fall into place and replace probiotic beneficial effects like pathogen inhibition and immunomodulation.
To prevent infectious diseases, only Gram (−) pathogenic bacterial EVs have been used as vaccines up to now, showing to be safe and efficacious on several occasions, while others are under evaluation (Behrens et al., 2021). For example, there are clinically available EV-based vaccines against Neisseria meningitidis, a causative agent of meningitis. The development of EV-based vaccines is a promising field for the prevention of infections. However, the isolation of EVs from several pathogenic microorganisms for vaccine design may have limitations. For example, many pathogens like bacteria, fungi and parasites cannot be cultured in the laboratory (Li et al., 2014; Roig et al., 2018). In the case of viruses, which do not produce EVs, cell cultures are necessary for the design of EV-based vaccines (Shehata et al., 2019; Yang et al., 2021). In this line, vaccination with engineered EVs from probiotic bacteria could be a useful platform to express pathogen antigens to be used as vaccines without toxicity in humans. To our knowledge, E. coli Nissle 1917 was the only strain assessed for this application in an animal model (Rosenthal et al., 2014). Further studies comparing Gram (−) and Gram (+) probiotic EVs would be necessary to elucidate whether the presence of certain components like LPS or LTA on the surface is important for the enhancement of the immune response. It is important to highlight that different chemical composition of LPS and LTA induce differential inflammatory responses and this must be taken into account to enhance EV immunogenicity (Migale et al., 2015; Jastrząb et al., 2021).
On the other hand, to treat infectious diseases, genetic engineering could be exploited for pathogen inhibition by increasing the expression of antimicrobial peptides and further encapsulation in EVs (Dean et al., 2020). Bacteriocins are potent small antimicrobial peptides synthesized by certain bacteria that may be appointed as alternatives to traditional antibiotics (Gradisteanu Pircalabioru et al., 2021). Bacteriocins within EVs turn them into potential candidates against infections, including those caused by antimicrobial resistant pathogens. According to WHO, antimicrobial resistance continues to be a global health and development threat (World Health Organization, 2021). Indeed, an important advantage of probiotic administration is the reduction in the use of strong anti-inflammatory agents and/or antibiotics that can be unfavorable in the long term (Kasatpibal et al., 2017; Guo and Leung, 2020; Raheem et al., 2021). In this regard, the indiscriminate use of antimicrobials leads not only to the development of antimicrobial resistance in pathogens, but also to the loss of our microbiota. The latter increases the susceptibility to infections such as vaginal candidiasis (Xu et al., 2008). Administration of probiotic EVs could be used not only to treat and/or prevent infections, but also would decrease antimicrobial use.
By taking advantage of EV versatility, other genetic engineering approaches can be applied to modify EV cargo or surface for the delivery of drugs to target cells. Genetic engineering enables the overexpression of proteins or the synthesis of small RNA that could silence target host genes (Fantappiè et al., 2014; Koeppen et al., 2016). EV cargo could be protected from harsh environmental conditions and additionally surface molecules could direct EVs to target host cells. This strategy could be relevant for the delivery of two or more synergistic drugs and/or the delivery of compounds that have difficulties in crossing the cell membrane (Liu et al., 2018).
Missing Knowledge and Challenges
As documented in several studies, probiotic-derived EVs could be involved in the prevention and treatment of infectious diseases. However, the protective capacity of probiotic bacteria EVs against pathogen infections was only studied against one virus (HIV-1) and a few bacteria (S. aureus, S. typhimurium and E. coli) (Mata Forsberg et al., 2019; Ñahui Palomino et al., 2019; Hu et al., 2020). Therefore, there is still no information on its beneficial effect against fungal and parasitic infections.
To date, there are many unknowns regarding the use of probiotics EVs as pharmaceutical agents. Current challenges are the lack of standardized and cost-effective methods for EV isolation, purification, characterization and upscale processing (Gurunathan et al., 2021). Unlike human EV markers, specific bacterial EV markers remain mostly unidentified (Ñahui Palomino et al., 2021). Identifying these molecular markers could not only optimize current characterization techniques, but also improve our understanding about EV physiology and future possible biomedical applications. For example, the probiotic B. subtilis produced S. aureus intestinal decolonization by inhibiting the pathogen quorum-sensing, and thereby produced a general decolonization (including the nose) (Piewngam and Otto, 2020). It would be interesting to study if probiotic EV components can mediate the inhibition of quorum-sensing among pathogenic bacteria. Even more, advances in the understanding of the role of EVs in inter-kingdom communication will almost certainly provide valuable insights into the development of novel therapies against pathogens.
Regarding the use of probiotic bacteria to create engineered EVs with vaccination purposes, the expression of antigenic proteins from non-culturable eukaryotic pathogens (fungi and parasites) has some limitations related to bacterial ability to make post-translational modifications. In this case, expression of antigens in eukaryotic probiotic organisms like yeasts would be a better and low cost option.
One alternative to administering isolated EVs that remains to be evaluated is whether it would be more advantageous to administer functional food with probiotics as a platform for EV delivery. As far as we know, EVs are constantly secreted by metabolically active bacteria (Brown et al., 2015; Liu et al., 2018). In a bacterial culture, EV release can vary depending on the growth conditions, including pH, oxygen presence, and agitation rate (Müller et al., 2021). For example, at pH 5 L. plantarum released a smaller number of EVs than at pH 7. On the other hand, there is recent evidence that L. rhamnosus JB-1 EVs can reach the bloodstream of mice after oral administration of the probiotic (Champagne-Jorgensen et al., 2021a). This outcome strongly suggests in situ EV release in the GIT. Whole cells would resist better than EVs to conditions during storage and transit through the GIT. In the case of spore-producing probiotics (e.g., B. subtilis), spore administration would be a cost-effective option. In this way, problems concerning EV stability would be avoided. Another strategy to consider is the microencapsulation of probiotics contained in food matrices to improve their viability during storage and in the GIT (Qi et al., 2020). Besides, if the encapsulating agent is mucoadhesive, a longer residence time in the GIT may allow a sustained release of EVs over time (Yao et al., 2020). Another microparticle-based delivery system could be a particle with coupled EVs on the surface to achieve high concentrations of EVs, maximizing EV effects as demonstrated in in vitro models (Kuhn et al., 2020).
Conclusion
The new era of postbiotics has brought a new point of view on the beneficial effects of probiotics. Probiotic-derived EVs could be mediating, at least in part, the beneficial effects of probiotics against infectious diseases via: inhibition of pathogens, enhancement of epithelial barrier function and modulation of the immune system. Remarkably, EVs can reach the bloodstream and consequently be delivered to extraintestinal organs, where probiotics were shown to have beneficial effects. Future studies should be focused on the characterization of EV active components and their interaction with the host. Novel EV-based technologies are promising for the design of therapies and/or vaccines against infections. Moreover, probiotics contained in food matrices could be used as EV-releasing devices in the GIT with potential applications in the functional food industry.
Author Contributions
APDR and CLD designed the idea, collected literature data, created the tables and figures, and wrote the manuscript. MP and OP reviewed and approved the final version of the manuscript. All authors contributed to the article and approved the submitted version.
Funding
This research was supported by CONICET, Agencia Nacional de Promoción Científica y Tecnológica (PICT 2017-1683) and Universidad de Buenos Aires (UBACyT20020150100079BA to OP and UBACYT 20020170100591BA to MP).
Conflict of Interest
The authors declare that the research was conducted in the absence of any commercial or financial relationships that could be construed as a potential conflict of interest.
Publisher’s Note
All claims expressed in this article are solely those of the authors and do not necessarily represent those of their affiliated organizations, or those of the publisher, the editors and the reviewers. Any product that may be evaluated in this article, or claim that may be made by its manufacturer, is not guaranteed or endorsed by the publisher.
Acknowledgments
We are grateful to Sergio I. Nemirovsky for his collaboration. All figures were partially created with BioRender.com.
References
Agostinis, C., Mangogna, A., Bossi, F., Ricci, G., Kishore, U., and Bulla, R. (2019). Uterine immunity and microbiota: a shifting paradigm. Front. Immunol. 10:2387. doi: 10.3389/fimmu.2019.02387
Ahlawat, S., and Asha Sharma, K. K. (2021). Gut–organ axis: a microbial outreach and networking. Lett. Appl. Microbiol. 72, 636–668. doi: 10.1111/lam.13333
Aljutaily, T., Huarte, E., Martinez-Monteagudo, S., Gonzalez-Hernandez, J. L., Rovai, M., and Sergeev, I. N. (2020). Probiotic-enriched milk and dairy products increase gut microbiota diversity: a comparative study. Nutr. Res. 82, 25–33. doi: 10.1016/j.nutres.2020.06.017
Al-Nedawi, K., Mian, M. F., Hossain, N., Karimi, K., Mao, Y. K., Forsythe, P., et al. (2015). Gut commensal microvesicles reproduce parent bacterial signals to host immune and enteric nervous systems. FASEB J. 29, 684–695. doi: 10.1096/fj.14-259721
Alvarez, C. S., Badia, J., Bosch, M., Giménez, R., and Baldomà, L. (2016). Outer membrane vesicles and soluble factors released by probiotic Escherichia coli Nissle 1917 and commensal ECOR63 enhance barrier function by regulating expression of tight junction proteins in intestinal epithelial cells. Front. Microbiol. 7:1981. doi: 10.3389/fmicb.2016.01981
Alvarez, C. S., Giménez, R., Cañas, M. A., Vera, R., Díaz-Garrido, N., Badia, J., et al. (2019). Extracellular vesicles and soluble factors secreted by Escherichia coli Nissle 1917 and ECOR63 protect against enteropathogenic E. coli-induced intestinal epithelial barrier dysfunction. BMC Microbiol. 19:166. doi: 10.1186/s12866-019-1534-3
Anaya-Loyola, M. A., Enciso-Moreno, J. A., López-Ramos, J. E., García-Marín, G., Orozco Álvarez, M. Y., Vega-García, A. M., et al. (2019). Bacillus coagulans GBI-30, 6068 decreases upper respiratory and gastrointestinal tract symptoms in healthy Mexican scholar-aged children by modulating immune-related proteins. Food Res. Int. 125:108567. doi: 10.1016/j.foodres.2019.108567
Andersson, N. W., Goodwin, R. D., Okkels, N., Gustafsson, L. N., Taha, F., Cole, S. W., et al. (2016). Depression and the risk of severe infections: prospective analyses on a nationwide representative sample. Int. J. Epidemiol. 45, 131–139. doi: 10.1093/ije/dyv333
Aryayev, M. L., Senkivska, L. I., Bredeleva, N. K., and Talashova, I. V. (2018). Prophylaxis of acute respiratory infections via improving the immune system in late preterm newborns with E. coli strain Nissle 1917: a controlled pilot trial. Pilot Feasibility Stud. 4:79. doi: 10.1186/s40814-018-0271-y
Bae, Y. S., Shin, E. C., Bae, Y. S., and Van Eden, W. (2019). Editorial: stress and immunity. Front. Immunol. 10:245. doi: 10.3389/fimmu.2019.00245
Bajic, S. S., Cañas, M. A., Tolinacki, M., Badia, J., Sánchez, B., Golic, N., et al. (2020). Proteomic profile of extracellular vesicles released by Lactiplantibacillus plantarum BGAN8 and their internalization by non-polarized HT29 cell line. Sci. Rep. 10:21829. doi: 10.1038/s41598-020-78920-z
Banfi, D., Moro, E., Bosi, A., Bistoletti, M., Cerantola, S., Crema, F., et al. (2021). Impact of microbial metabolites on microbiota–gut–brain axis in inflammatory bowel disease. Int. J. Mol. Sci. 22:1623. doi: 10.3390/ijms22041623
Barbara, G., Barbaro, M. R., Fuschi, D., Palombo, M., Falangone, F., Cremon, C., et al. (2021). Inflammatory and microbiota-related regulation of the intestinal epithelial barrier. Front. Nutr. 8:718356. doi: 10.3389/fnut.2021.718356
Bäuerl, C., Coll-Marqués, J. M., Tarazona-González, C., and Pérez-Martínez, G. (2020). Lactobacillus casei extracellular vesicles stimulate EGFR pathway likely due to the presence of proteins P40 and P75 bound to their surface. Sci. Rep. 10:19237. doi: 10.1038/s41598-020-75930-9
Behrens, F., Funk-Hilsdorf, T. C., Kuebler, W. M., and Simmons, S. (2021). Bacterial membrane vesicles in pneumonia: from mediators of virulence to innovative vaccine candidates. Int. J. Mol. Sci. 22:3858. doi: 10.3390/ijms22083858
Behzadi, E., Mahmoodzadeh Hosseini, H., and Imani Fooladi, A. A. (2017). The inhibitory impacts of Lactobacillus rhamnosus GG-derived extracellular vesicles on the growth of hepatic cancer cells. Microb. Pathog. 110, 1–6. doi: 10.1016/j.micpath.2017.06.016
Bhardwaj, R., Singh, B. P., Sandhu, N., Singh, N., Kaur, R., Rokana, N., et al. (2020). Probiotic mediated NF-κB regulation for prospective management of type 2 diabetes. Mol. Biol. Rep. 47, 2301–2313. doi: 10.1007/s11033-020-05254-4
Bielaszewska, M., Rüter, C., Bauwens, A., Greune, L., Jarosch, K. A., Steil, D., et al. (2017). Host cell interactions of outer membrane vesicle-associated virulence factors of enterohemorrhagic Escherichia coli O157: intracellular delivery, trafficking and mechanisms of cell injury. PLoS Pathog. 13:e1006159. doi: 10.1371/journal.ppat.1006159
Binda, S., Hill, C., Johansen, E., Obis, D., Pot, B., Sanders, M. E., et al. (2020). Criteria to qualify microorganisms as “probiotic” in foods and dietary supplements. Front. Microbiol. 11:1662. doi: 10.3389/fmicb.2020.01662
Bitto, N. J., Chapman, R., Pidot, S., Costin, A., Lo, C., Choi, J., et al. (2017). Bacterial membrane vesicles transport their DNA cargo into host cells. Sci. Rep. 7:7072. doi: 10.1038/s41598-017-07288-4
Blenkiron, C., Simonov, D., Muthukaruppan, A., Tsai, P., Dauros, P., Green, S., et al. (2016). Uropathogenic Escherichia coli releases extracellular vesicles that are associated with RNA. PLoS One 11:e0160440. doi: 10.1371/journal.pone.0160440
Briaud, P., and Carroll, R. K. (2020). Extracellular vesicle biogenesis and functions in gram-positive bacteria. Infect. Immun. 88:e00433-20. doi: 10.1128/IAI.00433-20
Brown, L., Wolf, J. M., Prados-Rosales, R., and Casadevall, A. (2015). Through the wall: extracellular vesicles in Gram-positive bacteria, mycobacteria and fungi. Nat. Rev. Microbiol. 13, 620–630. doi: 10.1038/nrmicro3480
Cañas, M. A., Fábrega, M. J., Giménez, R., Badia, J., and Baldomà, L. (2018). Outer membrane vesicles from probiotic and commensal escherichia coli activate NOD1-mediated immune responses in intestinal epithelial cells. Front. Microbiol. 9:498. doi: 10.3389/fmicb.2018.00498
Cañas, M. A., Giménez, R., Fábrega, M. J., Toloza, L., Baldomà, L., and Badia, J. (2016). Outer membrane vesicles from the probiotic Escherichia coli Nissle 1917 and the commensal ECOR12 enter intestinal epithelial cells via clathrin-dependent endocytosis and elicit differential effects on DNA damage. PLoS One 11:e0160374. doi: 10.1371/journal.pone.0160374
Cao, E. Y., and Mortha, A. (2020). “Eukaryome: emerging field with profound translational potential,” in Eukaryome Impact on Human Intestine Homeostasis and Mucosal Immunology, ed. N. Gullen (Cham: Springer). doi: 10.1007/978-3-030-44826-4
Champagne-Jorgensen, K., Jose, T. A., Stanisz, A. M., Mian, M. F., Hynes, A. P., and Bienenstock, J. (2021a). Bacterial membrane vesicles and phages in blood after consumption of Lacticaseibacillus rhamnosus JB-1. Gut Microbes 13:1993583. doi: 10.1080/19490976.2021.1993583
Champagne-Jorgensen, K., Mian, M. F., McVey Neufeld, K. A., Stanisz, A. M., and Bienenstock, J. (2021b). Membrane vesicles of Lacticaseibacillus rhamnosus JB-1 contain immunomodulatory lipoteichoic acid and are endocytosed by intestinal epithelial cells. Sci. Rep. 11:13756. doi: 10.1038/s41598-021-93311-8
Chang, H. Y., Chen, J. H., Chang, J. H., Lin, H. C., Lin, C. Y., and Peng, C. C. (2017). Multiple strains probiotics appear to be the most effective probiotics in the prevention of necrotizing Enterocolitis and mortality: an updated meta-analysis. PLoS One 12:e0171579. doi: 10.1371/journal.pone.0171579
Chau, K., Lau, E., Greenberg, S., Jacobson, S., Yazdani-Brojeni, P., Verma, N., et al. (2015). Probiotics for infantile colic: a randomized, double-blind, placebo-controlled trial investigating Lactobacillus reuteri DSM 17938. J. Pediatr. 166, 74–78.e1. doi: 10.1016/j.jpeds.2014.09.020
Chee, W. J. Y., Chew, S. Y., and Than, L. T. L. (2020). Vaginal microbiota and the potential of Lactobacillus derivatives in maintaining vaginal health. Microb. Cell Fact. 19, 1–24. doi: 10.1186/s12934-020-01464-4
Choi, J. H., Mo Moon, C., Shin, T.-S., Kim, E. K., Mcdowell, A., Jo, M.-K., et al. (2020). Experimental & Molecular Medicine Lactobacillus paracasei-derived extracellular vesicles attenuate the intestinal inflammatory response by augmenting the endoplasmic reticulum stress pathway. Exp. Mol. Med. 52, 423–437. doi: 10.1038/s12276-019-0359-3
Choi, J., Kim, Y. K., and Han, P. L. (2019). Extracellular vesicles derived from Lactobacillus plantarum increase BDNF expression in cultured hippocampal neurons and produce antidepressant-like effects in mice. Exp. Neurobiol. 28, 158–171. doi: 10.5607/en.2019.28.2.158
Chronopoulos, A., and Kalluri, R. (2020). Emerging role of bacterial extracellular vesicles in cancer. Oncogene 39, 6951–6960. doi: 10.1038/s41388-020-01509-3
Coccorullo, P., Strisciuglio, C., Martinelli, M., Miele, E., Greco, L., and Staiano, A. (2010). Lactobacillus reuteri (DSM 17938) in infants with functional chronic constipation: a double-blind, randomized, placebo-controlled study. J. Pediatr. 157, 598–602. doi: 10.1016/j.jpeds.2010.04.066
Collado, M. C., Vinderola, G., and Salminen, S. (2019). Postbiotics: facts and open questions. A position paper on the need for a consensus definition. Benef. Microbes 10, 711–719. doi: 10.3920/BM2019.0015
Cristofori, F., Dargenio, V. N., Dargenio, C., Miniello, V. L., Barone, M., and Francavilla, R. (2021). Anti-inflammatory and immunomodulatory effects of probiotics in gut inflammation: a door to the body. Front. Immunol. 12:578386. doi: 10.3389/fimmu.2021.578386
Cui, Y., Luo, L., Wang, X., Lu, Y., Yi, Y., Shan, Y., et al. (2021). Mining, heterologous expression, purification, antibactericidal mechanism, and application of bacteriocins: a review. Compr. Rev. Food Sci. Food Saf. 20, 863–899. doi: 10.1111/1541-4337.12658
Cunningham, M., Azcarate-Peril, M. A., Barnard, A., Benoit, V., Grimaldi, R., Guyonnet, D., et al. (2021). Shaping the future of probiotics and prebiotics. Trends Microbiol. 29, 667–685. doi: 10.1016/j.tim.2021.01.003
De Marco, S., Sichetti, M., Muradyan, D., Piccioni, M., Traina, G., Pagiotti, R., et al. (2018). Probiotic cell-free supernatants exhibited anti-inflammatory and antioxidant activity on human gut epithelial cells and macrophages stimulated with LPS. Evid. Based Complement. Alternat. Med. 2018:1756308. doi: 10.1155/2018/1756308
de Melo Pereira, G. V., de Oliveira Coelho, B., Magalhães Júnior, A. I., Thomaz-Soccol, V., and Soccol, C. R. (2018). How to select a probiotic? A review and update of methods and criteria. Biotechnol. Adv. 36, 2060–2076. doi: 10.1016/j.biotechadv.2018.09.003
Dean, S. N., Leary, D. H., Sullivan, C. J., Oh, E., and Walper, S. A. (2019). Isolation and characterization of Lactobacillus-derived membrane vesicles. Sci. Rep. 9:877. doi: 10.1038/s41598-018-37120-6
Dean, S. N., Rimmer, M. A., Turner, K. B., Phillips, D. A., Caruana, J. C., Hervey, W. J., et al. (2020). Lactobacillus acidophilus membrane vesicles as a vehicle of bacteriocin delivery. Front. Microbiol. 11:710. doi: 10.3389/fmicb.2020.00710
Demmelmair, H., Jiménez, E., Collado, M. C., Salminen, S., and McGuire, M. K. (2020). Maternal and perinatal factors associated with the human milk microbiome. Curr. Dev. Nutr. 4, 1–14. doi: 10.1093/cdn/nzaa027
Díaz-Garrido, N., Badia, J., and Baldomà, L. (2021). Microbiota-derived extracellular vesicles in interkingdom communication in the gut. J. Extracell. Vesicles 10:e12161. doi: 10.1002/jev2.12161
Dicks, L. M. T., and Grobbelaar, M. J. (2021). Double-barrel shotgun: probiotic lactic acid bacteria with antiviral properties modified to serve as vaccines. Microorganisms 9:1565. doi: 10.3390/microorganisms9081565
Ding, R., Goh, W. R., Wu, R., Yue, X., Luo, X., Khine, W. W. T., et al. (2019). Revisit gut microbiota and its impact on human health and disease. J. Food Drug Anal. 27, 623–631. doi: 10.1016/j.jfda.2018.12.012
Dinleyici, E. C., Dalgic, N., Guven, S., Metin, O., Yasa, O., Kurugol, Z., et al. (2015). Lactobacillus reuteri DSM 17938 shortens acute infectious diarrhea in a pediatric outpatient setting. J. Pediatr. (Rio. J.) 91, 392–396. doi: 10.1016/j.jped.2014.10.009
Domínguez Rubio, A. P., Martínez, J. H., Casillas, D. C. M., Leskow, F. C., Piuri, M., and Pérez, O. E. (2017). Lactobacillus casei BL23 produces microvesicles carrying proteins that have been associated with its probiotic effect. Front. Microbiol. 8:1783. doi: 10.3389/fmicb.2017.01783
Domínguez Rubio, A. P., Martínez, J., Palavecino, M., Fuentes, F., López, C. M. S., Marcilla, A., et al. (2020). Transcytosis of Bacillus subtilis extracellular vesicles through an in vitro intestinal epithelial cell model. Sci. Rep. 10:3120. doi: 10.1038/s41598-020-60077-4
El-Sayed, A., and Harashima, H. (2013). Endocytosis of gene delivery vectors: from clathrin-dependent to lipid raft-mediated endocytosis. Mol. Ther. 21, 1118–1130. doi: 10.1038/mt.2013.54
Fábrega, M. J., Aguilera, L., Giménez, R., Varela, E., Cañas, M. A., Antolín, M., et al. (2016). Activation of immune and defense responses in the intestinal mucosa by outer membrane vesicles of commensal and probiotic Escherichia coli strains. Front. Microbiol. 7:705. doi: 10.3389/fmicb.2016.00705
Fábrega, M. J., Rodríguez-Nogales, A., Garrido-Mesa, J., Algieri, F., Badía, J., Giménez, R., et al. (2017). Intestinal anti-inflammatory effects of outer membrane vesicles from Escherichia coli Nissle 1917 in DSS-experimental colitis in mice. Front. Microbiol. 8:1274. doi: 10.3389/fmicb.2017.01274
Fantappiè, L., de Santis, M., Chiarot, E., Carboni, F., Bensi, G., Jousson, O., et al. (2014). Antibody-mediated immunity induced by engineered Escherichia coli OMVs carrying heterologous antigens in their lumen. J. Extracell. Vesicles 3, 24015. doi: 10.3402/jev.v3.24015
FAO/WHO (2002). Guidelines for the Evaluation of Probiotics in Food. Working Gr, 1–11. Working Group Report on Drafting Guidelines for the Evaluation of Probiotics in Food. Geneva: World Health Organization.
Fernández-García, V., González-Ramos, S., Martín-Sanz, P., del Portillo, F. G., Laparra, J. M., and Boscá, L. (2021). NOD1 in the interplay between microbiota and gastrointestinal immune adaptations. Pharmacol. Res. 171:105775. doi: 10.1016/j.phrs.2021.105775
Fleming, D., Kesey, J., Rumbaugh, K., and Dissanaike, S. (2016). Comparing the survivability of Lactobacillus species in various probiotic delivery vehicles. JPEN J. Parenter. Enteral Nutr. 41, 1411–1413. doi: 10.1177/0148607116672266
Francavilla, R., Lionetti, E., Castellaneta, S., Ciruzzi, F., Indrio, F., Masciale, A., et al. (2012). Randomised clinical trial: Lactobacillus reuteri DSM 17938 vs. placebo in children with acute diarrhoea-a double-blind study. Aliment. Pharmacol. Ther. Randomised 36, 363–369. doi: 10.1111/j.1365-2036.2012.05180.x
Fusco, A., Savio, V., Donniacuo, M., Perfetto, B., and Donnarumma, G. (2021). Antimicrobial peptides human beta-defensin-2 and -3 protect the gut during Candida albicans infections enhancing the intestinal barrier integrity: in vitro study. Front. Cell. Infect. Microbiol. 11:666900. doi: 10.3389/fcimb.2021.666900
Gareau, M. G., Sherman, P. M., and Walker, W. A. (2010). Probiotics and the gut microbiota in intestinal health and disease. Nat. Rev. Gastroenterol. Hepatol. 7, 503–514. doi: 10.1038/nrgastro.2010.117
Gaspar, C., Donders, G. G., Palmeira-de-Oliveira, R., Queiroz, J. A., Tomaz, C., Martinez-de-Oliveira, J., et al. (2018). Bacteriocin production of the probiotic Lactobacillus acidophilus KS400. AMB Express 8:153. doi: 10.1186/s13568-018-0679-z
Ghosh, T., Beniwal, A., Semwal, A., and Navani, N. K. (2019). Mechanistic insights into probiotic properties of lactic acid bacteria associated with ethnic fermented dairy products. Front. Microbiol. 10:502. doi: 10.3389/fmicb.2019.00502
Gradisteanu Pircalabioru, G., Popa, L. I., Marutescu, L., Gheorghe, I., Popa, M., Czobor Barbu, I., et al. (2021). Bacteriocins in the era of antibiotic resistance: rising to the challenge. Pharmaceutics 13, 1–15. doi: 10.3390/pharmaceutics13020196
Grand View Research (2021). Probiotics Dietary Supplements Market Size, Share & Trends Analysis Report. Available online at: https://www.researchandmarkets.com/reports/3972861/probiotics-market-size-share-and-trends-analysis (accessed February 2, 2022).
Gu, Z., Li, F., Liu, Y., Jiang, M., Zhang, L., He, L., et al. (2021). Exosome- like nanoparticles from Lactobacillus rhamnosus GG protect against alcohol- associated liver disease through intestinal aryl hydrocarbon receptor in mice. Hepatol. Commun. 5, 846–864. doi: 10.1002/hep4.1679
Guo, C. G., and Leung, W. K. (2020). Potential strategies in the prevention of nonsteroidal anti-inflammatory drugs-associated adverse effects in the lower gastrointestinal tract. Gut Liver 14, 179–187. doi: 10.5009/gnl19201
Gurunathan, S., Kang, M. H., Qasim, M., Khan, K., and Kim, J. H. (2021). Biogenesis, membrane trafficking, functions, and next generation nanotherapeutics medicine of extracellular vesicles. Int. J. Nanomedicine 16, 3357–3383. doi: 10.2147/IJN.S310357
Hansson, G. C. (2020). Mucins and the microbiome. Annu. Rev. Biochem. 89, 769–793. doi: 10.1146/annurev-biochem-011520-105053
Harrison, N. A., Gardner, C. L., da Silva, D. R., Gonzalez, C. F., and Lorca, G. L. (2021). Identification of biomarkers for systemic distribution of nanovesicles from Lactobacillus johnsonii N6.2. Front. Immunol. 12:723433. doi: 10.3389/fimmu.2021.723433
Hassan, M. U., Nayab, H., Rehman, T. U., Williamson, M. P., Haq, K. U., Shafi, N., et al. (2020). Characterisation of bacteriocins produced by Lactobacillus spp. isolated from the traditional Pakistani yoghurt and their antimicrobial activity against common foodborne pathogens. Biomed Res. Int. 2020:8281623. doi: 10.1155/2020/8281623
He, X., Zeng, Q., Puthiyakunnon, S., Zeng, Z., Yang, W., Qiu, J., et al. (2017). Lactobacillus rhamnosus GG supernatant enhance neonatal resistance to systemic Escherichia coli K1 infection by accelerating development of intestinal defense. Sci. Rep. 7:43305. doi: 10.1038/srep43305
Higgins, J., Thomas, J., Chandler, J., Cumpston, M., Li, T., Page, M., et al. (2019). Cochrane Handbook for Systematic Reviews of Interventions, Second Edn. Hoboken, NJ: John Wiley & Sons.
Hill, C., Guarner, F., Reid, G., Gibson, G. R., Merenstein, D. J., Pot, B., et al. (2014). The International Scientific Association for Probiotics and Prebiotics consensus statement on the scope and appropriate use of the term probiotic. Nat. Rev. Gastroenterol. Hepatol. 11, 506–514. doi: 10.1038/nrgastro.2014.66
Hills, R. D., Pontefract, B. A., Mishcon, H. R., Black, C. A., Sutton, S. C., and Theberge, C. R. (2019). Gut microbiome: profound implications for diet and disease. Nutrients 11, 1–40. doi: 10.3390/nu11071613
Ho, M., Chang, Y. Y., Chang, W. C., Lin, H. C., Wang, M. H., Lin, W. C., et al. (2016). Oral Lactobacillus rhamnosus GR-1 and Lactobacillus reuteri RC-14 to reduce Group B Streptococcus colonization in pregnant women: a randomized controlled trial. Taiwan. J. Obstet. Gynecol. 55, 515–518. doi: 10.1016/j.tjog.2016.06.003
Hu, R., Lin, H., Li, J., Zhao, Y., Wang, M., Sun, X., et al. (2020). Probiotic Escherichia coli Nissle 1917-derived outer membrane vesicles enhance immunomodulation and antimicrobial activity in RAW264.7 macrophages. BMC Microbiol. 20:268. doi: 10.1186/s12866-020-01953-x
Hu, R., Lin, H., Wang, M., Zhao, Y., Liu, H., Min, Y., et al. (2021). Lactobacillus reuteri-derived extracellular vesicles maintain intestinal immune homeostasis against lipopolysaccharide-induced inflammatory responses in broilers. J. Anim. Sci. Biotechnol. 12, 1–18. doi: 10.1186/s40104-020-00532-4
Huang, F., Teng, K., Liu, Y., Cao, Y., Wang, T., Ma, C., et al. (2021). Bacteriocins: potential for human health. Oxid. Med. Cell. Longev. 2021:5518825. doi: 10.1155/2021/5518825
Invernici, M. M., Salvador, S. L., Silva, P. H. F., Soares, M. S. M., Casarin, R., Palioto, D. B., et al. (2018). Effects of Bifidobacterium probiotic on the treatment of chronic periodontitis: a randomized clinical trial. J. Clin. Periodontol. 45, 1198–1210. doi: 10.1111/jcpe.12995
Invernizzi, R., Lloyd, C. M., and Molyneaux, P. L. (2020). Respiratory microbiome and epithelial interactions shape immunity in the lungs. Immunology 160, 171–182. doi: 10.1111/imm.13195
Jang, S. C., Kim, S. R., Yoon, Y. J., Park, K. S., Kim, J. H., Lee, J., et al. (2015). In vivo kinetic biodistribution of nano-sized outer membrane vesicles derived from bacteria. Small 11, 456–461. doi: 10.1002/smll.201401803
Jang, S. E., Han, M. J., Kim, S. Y., and Kim, D. H. (2014). Lactobacillus plantarum CLP-0611 ameliorates colitis in mice by polarizing M1 to M2-like macrophages. Int. Immunopharmacol. 21, 186–192. doi: 10.1016/j.intimp.2014.04.021
Jastrząb, R., Graczyk, D., and Siedlecki, P. (2021). Molecular and cellular mechanisms influenced by postbiotics. Int. J. Mol. Sci. 22:13475. doi: 10.3390/ijms222413475
Jeffery, C. (2018). Intracellular proteins moonlighting as bacterial adhesion factors. AIMS Microbiol. 4, 362–376. doi: 10.3934/microbiol.2018.2.362
Jochems, P. G. M., Garssen, J., Van Keulen, A. M., Masereeuw, R., and Jeurink, P. V. (2018). Evaluating human intestinal cell lines for studying dietary protein absorption. Nutrients 10, 1–15. doi: 10.3390/nu10030322
Jones, E. J., Booth, C., Fonseca, S., Parker, A., Cross, K., Miquel-Clopés, A., et al. (2020). The uptake, trafficking, and Biodistribution of Bacteroides thetaiotaomicron generated outer membrane vesicles. Front. Microbiol. 11:57. doi: 10.3389/fmicb.2020.00057
Joseph, R. J., Ser, H. L., Kuai, Y. H., Tan, L. T. H., Arasoo, V. J. T., Letchumanan, V., et al. (2021). Finding a balance in the vaginal microbiome: how do we treat and prevent the occurrence of bacterial vaginosis? Antibiotics 10, 1–39. doi: 10.3390/antibiotics10060719
Kaparakis-Liaskos, M., and Kufer, T. A. (2020). in Bacterial Membrane Vesicles Biogenesis, Functions and Applications, eds M. Kaparakis-Liaskos and T. A. Kufer, Cham: Springer. doi: 10.1007/978-3-030-36331-4_4
Kasatpibal, N., Whitney, J. D., Saokaew, S., Kengkla, K., Heitkemper, M. M., and Apisarnthanarak, A. (2017). Effectiveness of probiotic, prebiotic, and synbiotic therapies in reducing postoperative complications: a systematic review and network meta-analysis. Clin. Infect. Dis. 64, S153–S160. doi: 10.1093/cid/cix114
Kim, K. W., Kang, S. S., Woo, S. J., Park, O. J., Ahn, K. B., Song, K. D., et al. (2017). Lipoteichoic acid of probiotic Lactobacillus plantarum attenuates poly I: C-induced IL-8 production in porcine intestinal epithelial cells. Front. Microbiol. 8:1827. doi: 10.3389/fmicb.2017.01827
Kim, M. H., Choi, S. J., Choi, H. I., Choi, J. P., Park, H. K., Kim, E. K., et al. (2018). Lactobacillus plantarum-derived extracellular vesicles protect atopic dermatitis induced by Staphylococcus aureus-derived Extracellular Vesicles. Allergy Asthma Immunol. Res. 10, 516–532. doi: 10.4168/aair.2018.10.5.516
Kim, W., Lee, E. J., Bae, I. H., Myoung, K., Kim, S. T., Park, P. J., et al. (2020). Lactobacillus plantarum-derived extracellular vesicles induce anti-inflammatory M2 macrophage polarization in vitro. J. Extracell. Vesicles 9, 314–319. doi: 10.1080/20013078.2020.1793514
Koeppen, K., Hampton, T. H., Jarek, M., Scharfe, M., Gerber, S. A., Mielcarz, D. W., et al. (2016). A novel mechanism of host-pathogen interaction through sRNA in bacterial outer membrane vesicles. PLoS Pathog. 12:e1005672. doi: 10.1371/journal.ppat.1005672
König, J., Wells, J., Cani, P. D., García-Ródenas, C. L., MacDonald, T., Mercenier, A., et al. (2016). Human intestinal barrier function in health and disease. Clin. Transl. Gastroenterol. 7:e196. doi: 10.1038/ctg.2016.54
Kotzampassi, K., Stavrou, G., Damoraki, G., Georgitsi, M., Basdanis, G., Tsaousi, G., et al. (2015). A four-probiotics regimen reduces postoperative complications after colorectal surgery: a randomized, double-blind, placebo-controlled study. World J. Surg. 39, 2776–2783. doi: 10.1007/s00268-015-3071-z
Kuhn, T., Koch, M., and Fuhrmann, G. (2020). Probiomimetics—novel Lactobacillus -mimicking microparticles show anti-inflammatory and barrier-protecting effects in gastrointestinal models. Small 16:2003158. doi: 10.1002/smll.202003158
Laue, C., Papazova, E., Liesegang, A., Pannenbeckers, A., Arendarski, P., Linnerth, B., et al. (2018). Effect of a yoghurt drink containing Lactobacillus strains on bacterial vaginosis in women - a double-blind, randomised, controlled clinical pilot trial. Benef. Microbes 9, 35–50. doi: 10.3920/BM2017.0018
Lazou Ahrén, I., Hillman, M., Arvidsson Nordström, E., Larsson, N., and Martinsson Niskanen, T. (2021). Fewer community-acquired colds with daily consumption of Lactiplantibacillus plantarum HEAL9 and Lacticaseibacillus paracasei 8700:2. A randomized, placebo-controlled clinical trial. J. Nutr. Nutr. Immunol. 151, 214–222. doi: 10.1093/jn/nxaa353
Lebeer, S., Vanderleyden, J., and De Keersmaecker, S. C. J. (2010). Host interactions of probiotic bacterial surface molecules: comparison with commensals and pathogens. Nat. Rev. Microbiol. 8, 171–184. doi: 10.1038/nrmicro2297
Lécrivain, A. L., and Beckmann, B. M. (2020). Bacterial RNA in extracellular vesicles: a new regulator of host-pathogen interactions? Biochim. Biophys. Acta Gene Regul. Mech. 1863:194519. doi: 10.1016/j.bbagrm.2020.194519
Lee, H. J. (2019). Microbe-host communication by small RNAs in extracellular vesicles: vehicles for transkingdom RNA transportation. Int. J. Mol. Sci. 20:1487. doi: 10.3390/ijms20061487
Lener, T., Gimona, M., Aigner, L., Börger, V., Buzas, E., Camussi, G., et al. (2015). Applying extracellular vesicles based therapeutics in clinical trials – an ISEV position paper. J. Extracell. Vesicles 4:30087. doi: 10.3402/jev.v4.30087
Li, L., Mendis, N., Trigui, H., Oliver, J. D., and Faucher, S. P. (2014). The importance of the viable but non-culturable state in human bacterial pathogens. Front. Microbiol. 5:258. doi: 10.3389/fmicb.2014.00258
Li, M., Lee, K., Hsu, M., Nau, G., Mylonakis, E., and Ramratnam, B. (2017). Lactobacillus-derived extracellular vesicles enhance host immune responses against vancomycin-resistant enterococci. BMC Microbiol. 17:66. doi: 10.1186/s12866-017-0977-7
Li, Z., and Stanton, B. A. (2021). Transfer RNA-derived fragments, the underappreciated regulatory small RNAs in microbial pathogenesis. Front. Microbiol. 12:687632. doi: 10.3389/fmicb.2021.687632
Liu, Q., Yu, Z., Tian, F., Zhao, J., Zhang, H., Zhai, Q., et al. (2020). Surface components and metabolites of probiotics for regulation of intestinal epithelial barrier. Microb. Cell Fact. 19:23. doi: 10.1186/s12934-020-1289-4
Liu, T., Zhang, L., Joo, D., and Sun, S. C. (2017). NF-κB signaling in inflammation. Signal Transduct. Target. Ther. 2:17023. doi: 10.1038/sigtrans.2017.23
Liu, Y., Defourny, K. A. Y., Smid, E. J., and Abee, T. (2018). Gram-positive bacterial extracellular vesicles and their impact on health and disease. Front. Microbiol. 9:1502. doi: 10.3389/fmicb.2018.01502
Loonen, L. M., Stolte, E. H., Jaklofsky, M. T., Meijerink, M., Dekker, J., van Baarlen, P., et al. (2014). REG3γ-deficient mice have altered mucus distribution and increased mucosal inflammatory responses to the microbiota and enteric pathogens in the ileum. Mucosal Immunol. 7, 939–947. doi: 10.1038/mi.2013.109
Lopes, E. G., Moreira, D. A., Gullón, P., Gullón, B., Cardelle-Cobas, A., and Tavaria, F. K. (2017). Topical application of probiotics in skin: adhesion, antimicrobial and antibiofilm in vitro assays. J. Appl. Microbiol. 122, 450–461. doi: 10.1111/jam.13349
López, P., González-Rodríguez, I., Sánchez, B., Gueimonde, M., Margolles, A., and Suárez, A. (2012). Treg-inducing membrane vesicles from Bifidobacterium bifidum LMG13195 as potential adjuvants in immunotherapy. Vaccine 30, 825–829. doi: 10.1016/j.vaccine.2011.11.115
Macion, A., Wyszyńska, A., and Godlewska, R. (2021). Delivery of toxins and effectors by bacterial membrane vesicles. Toxins (Basel) 13:845. doi: 10.3390/toxins13120845
Maguire, M., and Maguire, G. (2019). Gut dysbiosis, leaky gut, and intestinal epithelial proliferation in neurological disorders: towards the development of a new therapeutic using amino acids, prebiotics, probiotics, and postbiotics. Rev. Neurosci. 30, 179–201. doi: 10.1515/revneuro-2018-0024
Maldonado-Lobón, J. A., Díaz-López, M. A., Carputo, R., Duarte, P., Díaz-Ropero, M. P., Valero, A. D., et al. (2015). Lactobacillus fermentum CECT 5716 reduces Staphylococcus load in the breastmilk of lactating mothers suffering breast pain: a randomized controlled trial. Breastfeed. Med. 10, 425–432. doi: 10.1089/bfm.2015.0070
Mantziari, A., Salminen, S., Szajewska, H., and Malagón-Rojas, J. N. (2020). Postbiotics against pathogens commonly involved in pediatric infectious diseases. Microorganisms 8, 1–22. doi: 10.3390/microorganisms8101510
Marcial, G. E., Ford, A. L., Haller, M. J., Gezan, S. A., Harrison, N. A., Cai, D., et al. (2017). Lactobacillus johnsonii N6.2 modulates the host immune responses: a double-blind, randomized trial in healthy adults. Front. Immunol. 8:655. doi: 10.3389/fimmu.2017.00655
Mata Forsberg, M., Björkander, S., Pang, Y., Lundqvist, L., Ndi, M., Ott, M., et al. (2019). Extracellular membrane vesicles from Lactobacilli dampen IFN-γ responses in a monocyte-dependent manner. Sci. Rep. 9:17109. doi: 10.1038/s41598-019-53576-6
McGuire, M. A., and McGuire, M. K. (2017). “Prebiotics and probiotics in human milk,” in Prebiotics and Probiotics in Human Milk, eds M. K. Mcguire, M. A. McGuire, and L. Bode (Amsterdam: Elsevier), 463–468. doi: 10.1016/B978-0-12-802725-7.00017-8
Migale, R., Herbert, B. R., Lee, Y. S., Sykes, L., Waddington, S. N., Peebles, D., et al. (2015). Specific lipopolysaccharide serotypes induce differential maternal and neonatal inflammatory responses in a murine model of preterm labor. Am. J. Pathol. 185, 2390–2401. doi: 10.1016/j.ajpath.2015.05.015
Morishita, M., Horita, M., Higuchi, A., Marui, M., Katsumi, H., and Yamamoto, A. (2021). Characterizing different probiotic-derived extracellular vesicles as a novel adjuvant for immunotherapy. Mol. Pharm. 18, 1080–1092. doi: 10.1021/acs.molpharmaceut.0c01011
Müller, L., Kuhn, T., Koch, M., and Fuhrmann, G. (2021). Stimulation of probiotic bacteria induces release of membrane vesicles with augmented anti-inflammatory activity. ACS Appl. Bio Mater. 4, 3739–3748. doi: 10.1021/acsabm.0c01136
Munhoz da Rocha, I. F., Amatuzzi, R. F., Lucena, A. C. R., Faoro, H., and Alves, L. R. (2020). Cross-Kingdom extracellular vesicles EV-RNA communication as a mechanism for host–pathogen interaction. Front. Cell. Infect. Microbiol. 10:593160. doi: 10.3389/fcimb.2020.593160
Ñahui Palomino, R. A., Vanpouille, C., Costantini, P. E., and Margolis, L. (2021). Microbiota–host communications: bacterial extracellular vesicles as a common language. PLoS Pathog. 17:e1009508. doi: 10.1371/journal.ppat.1009508
Ñahui Palomino, R. A., Vanpouille, C., Laghi, L., Parolin, C., Melikov, K., Backlund, P., et al. (2019). Extracellular vesicles from symbiotic vaginal lactobacilli inhibit HIV-1 infection of human tissues. Nat. Commun. 10:5656. doi: 10.1038/s41467-019-13468-9
Nam, Y., Kim, J., Baek, J., and Kim, W. (2021). Improvement of cutaneous wound healing via topical application of heat-killed Lactococcus chungangensis CAU 1447 on diabetic mice. Nutrients 13:2666. doi: 10.3390/nu13082666
Nishiyama, K., Takaki, T., Sugiyama, M., Fukuda, I., Aiso, M., Mukai, T., et al. (2020). Extracellular vesicles produced by Bifidobacterium longum export mucin-binding proteins. Appl. Environ. Microbiol. 86:e01464-20. doi: 10.1128/AEM.01464-20
Nougayrède, J.-P., Chagneau, C., Motta, J.-P., Bossuet-Greif, N., Belloy, M., Taieb, F., et al. (2021). A toxic friend: genotoxic and mutagenic activity of the probiotic strain Escherichia coli Nissle 1917. mSphere 6:e0062421. doi: 10.1128/mSphere.00624-21
O’Donoghue, E. J., and Krachler, A. M. (2016). Mechanisms of outer membrane vesicle entry into host cells. Cell. Microbiol. 18, 1508–1517. doi: 10.1111/cmi.12655
Olier, M., Marcq, I., Salvador-Cartier, C., Secher, T., Dobrindt, U., Boury, M., et al. (2012). Genotoxicity of escherichia coli nissle 1917 strain cannot be dissociated from its probiotic activity. Gut Microbes 3, 501–509. doi: 10.4161/gmic.21737
Olivares, M., Albrecht, S., De Palma, G., Ferrer, M. D., Castillejo, G., Schols, H. A., et al. (2015). Human milk composition differs in healthy mothers and mothers with celiac disease. Eur. J. Nutr. 54, 119–128. doi: 10.1007/s00394-014-0692-1
Panigrahi, P., Parida, S., Nanda, N. C., Satpathy, R., Pradhan, L., Chandel, D. S., et al. (2017). A randomized synbiotic trial to prevent sepsis among infants in rural India. Nature 548, 407–412. doi: 10.1038/nature23480
Park, D. H., Kim, J. W., Park, H.-J., and Hahm, D.-H. (2021). Comparative analysis of the microbiome across the gut–skin axis in atopic dermatitis. Int. J. Mol. Sci. 22:4228. doi: 10.3390/ijms22084228
Park, J. Y., Choi, J., Lee, Y., Lee, J. E., Lee, E. H., Kwon, H. J., et al. (2017). Metagenome analysis of bodily microbiota in a mouse model of Alzheimer disease using bacteria-derived membrane vesicles in blood. Exp. Neurobiol. 26, 369–379. doi: 10.5607/en.2017.26.6.369
Passali, D., Passali, G. C., Vesperini, E., Cocca, S., Visconti, I. C., Ralli, M., et al. (2019). The efficacy and tolerability of Streptococcus salivarius 24SMB and Streptococcus oralis 89a administered as nasal spray in the treatment of recurrent upper respiratory tract infections in children. Eur. Rev. Med. Pharmacol. Sci. 23, 67–72. doi: 10.26355/eurrev_201903_17352
Pellon, A., Sadeghi Nasab, S. D., and Moyes, D. L. (2020). New insights in Candida albicans innate immunity at the mucosa: toxins, epithelium, metabolism, and beyond. Front. Cell. Infect. Microbiol. 10:81. doi: 10.3389/fcimb.2020.00081
Peral, M. C., Huaman Martinez, M. A., and Valdez, J. C. (2009). Bacteriotherapy with Lactobacillus plantarum in burns. Int. Wound J. 6, 73–81. doi: 10.1111/j.1742-481X.2008.00577.x
Piewngam, P., and Otto, M. (2020). Probiotics to prevent Staphylococcus aureus disease? Gut Microbes 11, 94–101. doi: 10.1080/19490976.2019.1591137
Plaza-Diaz, J., Ruiz-Ojeda, F. J., Gil-Campos, M., and Gil, A. (2019). Mechanisms of action of probiotics. Adv. Nutr. 10, S49–S66. doi: 10.1093/advances/nmy063
Qi, X., Simsek, S., Ohm, J. B., Chen, B., and Rao, J. (2020). Viability of: Lactobacillus rhamnosus GG microencapsulated in alginate/chitosan hydrogel particles during storage and simulated gastrointestinal digestion: role of chitosan molecular weight. Soft Matter 16, 1877–1887. doi: 10.1039/c9sm02387a
Raheem, A., Liang, L., Zhang, G., and Cui, S. (2021). Modulatory effects of probiotics during pathogenic infections with emphasis on immune regulation. Front. Immunol. 12:616713. doi: 10.3389/fimmu.2021.616713
Rajilić-Stojanović, M. (2013). Function of the microbiota. Best Pract. Res. Clin. Gastroenterol. 27, 5–16. doi: 10.1016/j.bpg.2013.03.006
Ren, C., Zhang, Q., de Haan, B. J., Zhang, H., Faas, M. M., and de Vos, P. (2016). Identification of TLR2/TLR6 signalling lactic acid bacteria for supporting immune regulation. Sci. Rep. 6:34561. doi: 10.1038/srep34561
Riley, L. W., and Blanton, R. E. (2018). Advances in molecular epidemiology of infectious diseases: definitions, approaches, and scope of the field. Microbiol. Spectr. 6, 1–12. doi: 10.1128/microbiolspec.ame-0001-2018
Rodovalho, V. D. R., da Luz, B. S. R., Rabah, H., do Carmo, F. L. R., Folador, E. L., Nicolas, A., et al. (2020). Extracellular vesicles produced by the probiotic Propionibacterium freudenreichii CIRM-BIA 129 mitigate inflammation by modulating the NF-κB pathway. Front. Microbiol. 11:1544. doi: 10.3389/fmicb.2020.01544
Roig, J., Saiz, M. L., Galiano, A., Trelis, M., Cantalapiedra, F., Monteagudo, C., et al. (2018). Extracellular vesicles from the helminth Fasciola hepatica prevent DSS-induced acute ulcerative colitis in a T-lymphocyte independent mode. Front. Microbiol. 9:1036. doi: 10.3389/fmicb.2018.01036
Rosenthal, J. A., Huang, C., Doody, A. M., Leung, T., Mineta, K., Feng, D. D., et al. (2014). Mechanistic insight into the Th1-biased immune response to recombinant subunit vaccines delivered by probiotic bacteria-derived outer membrane vesicles. PLoS One 9:e112802. doi: 10.1371/journal.pone.0112802
Russo, R., Superti, F., Karadja, E., and De Seta, F. (2019). Randomised clinical trial in women with recurrent vulvovaginal Candidiasis: efficacy of probiotics and lactoferrin as maintenance treatment. Mycoses 62, 328–335. doi: 10.1111/myc.12883
Salminen, S., Collado, M. C., Endo, A., Hill, C., Lebeer, S., Quigley, E. M. M., et al. (2021). The international scientific association of probiotics and prebiotics (ISAPP) consensus statement on the definition and scope of postbiotics. Nat. Rev. Gastroenterol. Hepatol. 18, 649–667. doi: 10.1038/s41575-021-00440-6
Sanders, M. E., Benson, A., Lebeer, S., Merenstein, D. J., and Klaenhammer, T. R. (2018). Shared mechanisms among probiotic taxa: implications for general probiotic claims. Curr. Opin. Biotechnol. 49, 207–216. doi: 10.1016/j.copbio.2017.09.007
Santos, P., and Almeida, F. (2021). Exosome-based vaccines: history, current state, and clinical trials. Front. Immunol. 12:711565. doi: 10.3389/fimmu.2021.711565
Santos, T. T., Maria, R., Santos, D., Acurcio, L. B., Henrique, S., Sandes, C., et al. (2020). Differential immune response of Lactobacillus plantarum 286 against Salmonella typhimurium infection in conventional and germ-free mice. Adv. Exp. Med. Biol. 1323, 1–17. doi: 10.1007/5584_2020_544
Sassone-Corsi, M., and Raffatellu, M. (2016). No vacancy: how beneficial microbes cooperate with immunity to provide colonization resistance to pathogens. J. Immunol. 176, 139–148. doi: 10.1016/j.physbeh.2017.03.040
Seth, A., Yan, F., Polk, D. B., and Rao, R. K. (2008). Probiotics ameliorate the hydrogen peroxide-induced epithelial barrier disruption by a PKC- And MAP kinase-dependent mechanism. Am. J. Physiol. Gastrointest. Liver Physiol. 294, 1060–1069. doi: 10.1152/ajpgi.00202.2007
Sgibnev, A., and Kremleva, E. (2020). Probiotics in addition to metronidazole for treatment Trichomonas vaginalis in the presence of BV: a randomized, placebo-controlled, double-blind study. Eur. J. Clin. Microbiol. Infect. Dis. 39, 345–351. doi: 10.1007/s10096-019-03731-8
Shafaghi, A., Pourkazemi, A., Khosravani, M., Fakhrie Asl, S., Amir Maafi, A., Atrkar Roshan, Z., et al. (2016). The effect of probiotic plus prebiotic supplementation on the tolerance and efficacy of Helicobacter pylori eradication quadruple therapy: a randomized prospective double blind controlled trial. Middle East J. Dig. Dis. 8, 179–188. doi: 10.15171/mejdd.2016.30
Sharifi-Rad, J., Rodrigues, C. F., Stojanović, Z., Radić, S.-R., Dimitrijević, M. D., Aleksić, A. A., et al. (2020). Probiotics: versatile bioactive components in promoting human health. Medicina (B. Aires) 56, 433. doi: 10.3390/medicina56090433
Shehata, M. M., Mostafa, A., Teubner, L., Mahmoud, S. H., Kandeil, A., Elshesheny, R., et al. (2019). Bacterial outer membrane vesicles (OMVs)-based dual vaccine for influenza a H1N1 virus and MERS-CoV. Vaccines 7, 1–13. doi: 10.3390/vaccines7020046
Shi, X., Zhang, J., Mo, L., Shi, J., Qin, M., and Huang, X. (2019). Efficacy and safety of probiotics in eradicating Helicobacter pylori: a network meta-analysis. Medicine (Baltimore) 98:e15180. doi: 10.1097/MD.0000000000015180
Shin, D. Y., Yi, D. Y., Jo, S., Lee, Y. M., Kim, J. H., Kim, W., et al. (2020). Effect of a new Lactobacillus plantarum product, LRCC5310, on clinical symptoms and virus reduction in children with rotaviral enteritis. Medicine (Baltimore) 99:e22192. doi: 10.1097/MD.0000000000022192
Shiraishi, T., Yokota, S., Sato, Y., Ito, T., Fukiya, S., Yamamoto, S., et al. (2018). Lipoteichoic acids are embedded in cell walls during logarithmic phase, but exposed on membrane vesicles in Lactobacillus gasseri JCM 1131 T. Benef. Microbes 9, 653–662. doi: 10.3920/BM2017.0124
Silvia Ventimiglia, M., Jimena Valeff, N., Pozo Albán, M., Manuel Paturlanne, J., Juriol, L., Quadrana, F., et al. (2021). Probiotic Lactobacillus kefiri prevents endotoxin-induced preterm birth and stillbirth in mice. Reproduction 161, 657–667. doi: 10.1530/REP-20-0642
Singh, T. P., Kaur, G., Kapila, S., and Malik, R. K. (2017). Antagonistic activity of Lactobacillus reuteri strains on the adhesion characteristics of selected pathogens. Front. Microbiol. 8:486. doi: 10.3389/fmicb.2017.00486
Spacova, I., De Boeck, I., Bron, P. A., Delputte, P., and Lebeer, S. (2021). Topical microbial therapeutics against respiratory viral infections. Trends Mol. Med. 27, 538–553. doi: 10.1016/j.molmed.2021.03.009
Stapleton, A. E., Au-Yeung, M., Hooton, T. M., Fredricks, D. N., Roberts, P. L., Czaja, C. A., et al. (2011). Randomized, placebo-controlled phase 2 trial of a Lactobacillus crispatus probiotic given intravaginally for prevention of recurrent urinary tract infection. Clin. Infect. Dis. 52, 1212–1217. doi: 10.1093/cid/cir183
Stavropoulou, E., and Bezirtzoglou, E. (2020). Probiotics in medicine: a long debate. Front. Immunol. 11:2192. doi: 10.3389/fimmu.2020.02192
Stentz, R., Carvalho, A. L., Jones, E. J., and Carding, S. R. (2018). Fantastic voyage: the journey of intestinal microbiota-derived microvesicles through the body. Biochem. Soc. Trans. 46, 1021–1027. doi: 10.1042/BST20180114
Su, G. L., Ko, C. W., Bercik, P., Falck-Ytter, Y., Sultan, S., Weizman, A. V., et al. (2020). AGA clinical practice guidelines on the role of probiotics in the management of gastrointestinal disorders. Gastroenterology 159, 697–705. doi: 10.1053/j.gastro.2020.05.059
Surendran Nair, M., Amalaradjou, M. A., and Venkitanarayanan, K. (2017). Antivirulence Properties of Probiotics in Combating Microbial Pathogenesis. Amsterdam: Elsevier Ltd. doi: 10.1016/bs.aambs.2016.12.001
Takiishi, T., Fenero, C. I. M., and Câmara, N. O. S. (2017). Intestinal barrier and gut microbiota: shaping our immune responses throughout life. Tissue Barriers 5:e1373208. doi: 10.1080/21688370.2017.1373208
Tamayo, C. (2008). Clinical research on probiotics: the interface between science and regulation. Clin. Infect. Dis. 46, S101–S103. doi: 10.1086/523332
Tang, N., Luo, Z.-C., Zhang, L., Zheng, T., Fan, P., Tao, Y., et al. (2020). The association between gestational diabetes and microbiota in placenta and cord blood. Front. Endocrinol. (Lausanne) 11:550319. doi: 10.3389/fendo.2020.550319
Théry, C., Witwer, K. W., Aikawa, E., Alcaraz, M. J., Anderson, J. D., Andriantsitohaina, R., et al. (2018). Minimal information for studies of extracellular vesicles 2018 (MISEV2018): a position statement of the International Society for Extracellular Vesicles and update of the MISEV2014 guidelines. J. Extracell. Vesicles 7:1535750. doi: 10.1080/20013078.2018.1535750
Tong, L., Zhang, X., Hao, H., Liu, Q., Zhou, Z., Liang, X., et al. (2021). Lactobacillus rhamnosus GG derived extracellular vesicles modulate gut microbiota and attenuate inflammatory in DSS-induced colitis mice. Nutrients 13:3319. doi: 10.3390/nu13103319
Tsubura, S., Mizunuma, H., Ishikawa, S., Oyake, I., Okabayashi, M., Katoh, K., et al. (2009). The effect of Bacillus subtilis mouth rinsing in patients with periodontitis. Eur. J. Clin. Microbiol. Infect. Dis. 28, 1353–1356. doi: 10.1007/s10096-009-0790-9
Tuo, Y., Song, X., Song, Y., Liu, W., Tang, Y., Gao, Y., et al. (2018). Screening probiotics from Lactobacillus strains according to their abilities to inhibit pathogen adhesion and induction of pro-inflammatory cytokine IL-8. J. Dairy Sci. 101, 4822–4829. doi: 10.3168/jds.2017-13654
Tzipilevich, E., Habusha, M., and Ben-Yehuda, S. (2017). Acquisition of phage sensitivity by bacteria through exchange of phage receptors. Cell 168, 186–199.e12. doi: 10.1016/j.cell.2016.12.003
van Zyl, W. F., Deane, S. M., and Dicks, L. M. T. (2019). Bacteriocin production and adhesion properties as mechanisms for the anti-listerial activity of Lactobacillus plantarum 423 and Enterococcus mundtii ST4SA. Benef. Microbes 10, 329–349. doi: 10.3920/BM2018.0141
van Zyl, W. F., Deane, S. M., and Dicks, L. M. T. (2020). Molecular insights into probiotic mechanisms of action employed against intestinal pathogenic bacteria. Gut Microbes 12:1831339. doi: 10.1080/19490976.2020.1831339
Vargoorani, M. E., Modarressi, M. H., Vaziri, F., Motevaseli, E., and Siadat, S. D. (2020). Stimulatory effects of Lactobacillus casei derived extracellular vesicles on toll-like receptor 9 gene expression and cytokine profile in human intestinal epithelial cells. J. Diabetes Metab. Disord. 19, 223–231. doi: 10.1007/s40200-020-00495-3
Veiga-Parga, T., Sehrawat, S., and Rouse, B. T. (2013). Role of regulatory T cells during virus infection. Immunol. Rev. 255, 182–196. doi: 10.1111/imr.12085
Vinderola, G., and Pérez-Marc, G. (2021). Fermented foods and probiotics for children. The importance of knowing their microbiological differences. Arch. Argent. Pediatr. 119, 56–61. doi: 10.5546/AAP.2021.ENG.56
Vladareanu, R., Mihu, D., Mitran, M., Mehedintu, C., Boiangiu, A., Manolache, M., et al. (2018). Abstracts Lactobacillus plantarum new evidence on oral L. plantarum P17630 product in women with history of recurrent vulvovaginal Exopolysaccharide from Lactobacillus plantarum LRCC5310 offers protection against rotavirus-induced diarrhea and regulates. Eur. Rev. Med. Pharmacol. Sci. 22, 262–267. doi: 10.26355/eurrev_201801_14128
Wahlund, C. J. E., Güclüler, G., Hiltbrunner, S., Veerman, R. E., Näslund, T. I., and Gabrielsson, S. (2017). Exosomes from antigen-pulsed dendritic cells induce stronger antigen-specific immune responses than microvesicles in vivo. Sci. Rep. 7:17095. doi: 10.1038/s41598-017-16609-6
Wang, L., Zhang, S., Wu, H., Rong, X., and Guo, J. (2019). M2b macrophage polarization and its roles in diseases. J. Leukoc. Biol. 106, 345–358. doi: 10.1002/JLB.3RU1018-378RR
Wang, W., and Jeffery, C. J. (2016). An analysis of surface proteomics results reveals novel candidates for intracellular/surface moonlighting proteins in bacteria. Mol. Biosyst. 12, 1420–1431. doi: 10.1039/c5mb00550g
Wegh, C. A. M., Geerlings, S. Y., Knol, J., Roeselers, G., and Belzer, C. (2019). Postbiotics and their potential applications in early life nutrition and beyond. Int. J. Mol. Sci. 20:4673. doi: 10.3390/ijms20194673
West, C. L., Stanisz, A. M., Mao, Y. K., Champagne-Jorgensen, K., Bienenstock, J., and Kunze, W. A. (2020). Microvesicles from Lactobacillus reuteri (DSM-17938) completely reproduce modulation of gut motility by bacteria in mice. PLoS One 15:e0225481. doi: 10.1371/journal.pone.0225481
Wilmore, J. R., Gaudette, B. T., Gomez Atria, D., Hashemi, T., Jones, D. D., Gardner, C. A., et al. (2018). Commensal microbes induce serum IgA responses that protect against polymicrobial sepsis. Cell Host Microbe 23, 302–311.e3. doi: 10.1016/j.chom.2018.01.005
World Health Organization (2021). World Health Statistics 2021: Monitoring Health for the SDGs, Sustainable Development Goals. Available online at: https://reliefweb.int/report/world/world-health-statistics-2021-monitoring-health-sdgs (accessed December 30, 2021).
Xia, X., Chen, J., Xia, J., Wang, B., Liu, H., Yang, L., et al. (2018). Role of probiotics in the treatment of minimal hepatic encephalopathy in patients with HBV-induced liver cirrhosis. J. Int. Med. Res. 46, 3596–3604. doi: 10.1177/0300060518776064
Xu, J., Schwartz, K., Bartoces, M., Monsur, J., Severson, R. K., and Sobel, J. D. (2008). Effect of antibiotics on vulvovaginal Candidiasis: a MetroNet study. J. Am. Board Fam. Med. 21, 261–268. doi: 10.3122/jabfm.2008.04.070169
Yamasaki-Yashiki, S., Miyoshi, Y., Nakayama, T., Kunisawa, J., and Katakura, Y. (2019). IgA-enhancing effects of membrane vesicles derived from Lactobacillus sakei subsp. sakei NBRC15893. Biosci. Microbiota Food Heal. 38, 23–29. doi: 10.12938/bmfh.18-015
Yan, F., Cao, H., Cover, T. L., Washington, M. K., Shi, Y., Liu, L. S., et al. (2011). Colon-specific delivery of a probiotic-derived soluble protein ameliorates intestinal inflammation in mice through an EGFR-dependent mechanism. J. Clin. Invest. 121, 2242–2253. doi: 10.1172/JCI44031
Yang, L., Zhao, Y., Wang, Y., Liu, L., Zhang, X., Li, B., et al. (2015). The effects of psychological stress on depression. Curr. Neuropharmacol. 13, 494–504. doi: 10.2174/1570159x1304150831150507
Yang, Z., Hua, L., Yang, M., Liu, S. Q., Shen, J., Li, W., et al. (2021). RBD-modified bacterial vesicles elicited potential protective immunity against SARS-CoV-2. Nano Lett. 21, 5920–5930. doi: 10.1021/acs.nanolett.1c00680
Yao, M., Xie, J., Du, H., McClements, D. J., Xiao, H., and Li, L. (2020). Progress in microencapsulation of probiotics: a review. Compr. Rev. Food Sci. Food Saf. 19, 857–874. doi: 10.1111/1541-4337.12532
Yong, S. J., Tong, T., Chew, J., and Lim, W. L. (2020). Antidepressive mechanisms of probiotics and their therapeutic potential. Front. Neurosci. 13:1361. doi: 10.3389/fnins.2019.01361
Zhang, G., Huang, X., Xiu, H., Sun, Y., Chen, J., Cheng, G., et al. (2020). Extracellular vesicles: natural liver-accumulating drug delivery vehicles for the treatment of liver diseases. J. Extracell. Vesicles 10:e12030. doi: 10.1002/jev2.12030
Zhao, M., Zhang, H., Liu, X., Jiang, Y., Ren, L., and Hu, X. (2017). The effect of TGF-β on treg cells in adverse pregnancy outcome upon Toxoplasma gondii infection. Front. Microbiol. 8:901. doi: 10.3389/fmicb.2017.00901
Zhao, W., Liu, Y., Kwok, L. Y., Cai, T., and Zhang, W. (2020). The immune regulatory role of Lactobacillus acidophilus: an updated meta-analysis of randomized controlled trials. Food Biosci. 36:100656. doi: 10.1016/j.fbio.2020.100656
Zhao, W., Peng, C., Sakandar, H. A., Kwok, L. Y., and Zhang, W. (2021). Meta-analysis: randomized trials of Lactobacillus plantarum on immune regulation over the last decades. Front. Immunol. 12:643420. doi: 10.3389/fimmu.2021.643420
Keywords: probiotics, GRAS, postbiotics, extracellular vesicles, membrane vesicles, infectious diseases
Citation: Domínguez Rubio AP, D’Antoni CL, Piuri M and Pérez OE (2022) Probiotics, Their Extracellular Vesicles and Infectious Diseases. Front. Microbiol. 13:864720. doi: 10.3389/fmicb.2022.864720
Received: 28 January 2022; Accepted: 07 March 2022;
Published: 30 March 2022.
Edited by:
Martin Schwarzer, Institute of Microbiology, Academy of Sciences of the Czech Republic (ASCR), CzechiaReviewed by:
Maria de los Angeles Serradell, Universidad Nacional de La Plata, ArgentinaArthur C. Ouwehand, International Flavors and Fragrances, Finland
Copyright © 2022 Domínguez Rubio, D’Antoni, Piuri and Pérez. This is an open-access article distributed under the terms of the Creative Commons Attribution License (CC BY). The use, distribution or reproduction in other forums is permitted, provided the original author(s) and the copyright owner(s) are credited and that the original publication in this journal is cited, in accordance with accepted academic practice. No use, distribution or reproduction is permitted which does not comply with these terms.
*Correspondence: Oscar E. Pérez, oscarperez@qb.fcen.uba.ar