- 1Department of Conservative Dentistry and Periodontology, University Hospital Regensburg, Regensburg, Germany
- 2Institute of Clinical Microbiology and Hygiene, University Hospital Regensburg, Regensburg, Germany
- 3Department of Dermatology, University Hospital Regensburg, Regensburg, Germany
- 4Department of Operative Dentistry and Periodontology, Center for Dental Medicine, University of Freiburg, Freiburg im Breisgau, Germany
Antiseptics are widely used in dental practice and included in numerous over-the-counter oral care products. However, the effects of routine antiseptic use on microbial composition of oral biofilms and on the emergence of resistant phenotypes remain unclear. Microcosm biofilms were inoculated from saliva samples of four donors and cultured in the Amsterdam Active Attachment biofilm model for 3 days. Then, they were treated two times daily with chlorhexidine digluconate (CHX) or cetylpyridinium chloride (CPC) for a period of 7 days. Ecological changes upon these multiple antiseptic treatments were evaluated by semiconductor-based sequencing of bacterial 16S rRNA genes and identification of amplicon sequence variants (ASVs). Furthermore, culture-based approaches were used for colony-forming units (CFU) assay, identification of antiseptic-resistant phenotypes using an agar dilution method, and evaluation of their antibiotic susceptibilities. Both CHX and CPC showed only slight effects on CFU and could not inhibit biofilm growth despite the two times daily treatment for 7 days. Both antiseptics showed significant ecological effects on the microbial compositions of the surviving microbiota, whereby CHX led to enrichment of rather caries-associated saccharolytic taxa and CPC led to enrichment of rather gingivitis-associated proteolytic taxa. Antiseptic-resistant phenotypes were isolated on antiseptic-containing agar plates, which also exhibited phenotypic resistance to various antibiotics. Our results highlight the need for further research into potential detrimental effects of antiseptics on the microbial composition of oral biofilms and on the spread of antimicrobial resistance in the context of their frequent use in oral healthcare.
Introduction
At present, a wide variety of antiseptics are available as over-the-counter consumer products for daily use in oral care (van der Weijden et al., 2015; Figuero et al., 2019). The use of antiseptic mouthwashes as adjunct to mechanical removal of biofilm and use of fluorides has been recommended for certain high-risk patient populations, such as patients with intellectual disabilities (Waldron et al., 2019), patients following surgical procedures, such as periodontal or implant surgery (Solderer et al., 2019), with fixed orthodontic appliances (Pithon et al., 2015), or elderly persons who are restricted in performing tooth-brushing or other oral hygiene procedures themselves (Grönbeck Lindén et al., 2017). More recently, since the COVID-19 pandemic, antiseptic mouthwashes are also applied as preprocedural mouthrinses for potentially reducing the viral load and infectivity of SARS-CoV-2 in the oral cavity and dental aerosols (Gottsauner et al., 2020; Carrouel et al., 2021; Meister et al., 2022).
Chlorhexidine digluconate (CHX), a symmetric bis-biguanide molecule carrying two positive charges, and cetylpyridinium chloride (CPC), a monocationic quaternary ammonium compound (QAC), can be regarded as the most common antiseptics for dental professional use and as ingredients in oral care products (Jones, 1997; Haps et al., 2008; Sanz et al., 2013; van der Weijden et al., 2015; Cieplik et al., 2019a; Mao et al., 2020). While both CHX and CPC are highly effective against planktonic bacteria (Cieplik et al., 2019a; So Yeon and Si Young, 2019; Mao et al., 2020), it is well known that eradicating bacterial cells in biofilms is much more difficult than killing planktonic bacteria and usually requires the antiseptic concentrations of about 100–1,000 times higher than those required to eliminate planktonic bacteria (Ceri et al., 1999; Shani et al., 2000). Accordingly, in a classic study, Zaura-Arite et al. (2001) showed that treatment with 0.2% CHX for a clinically relevant treatment period of 1 min had some effects on the outer layers of biofilms formed in situ for 48 h, but did not affect their inner layers. Likewise, a previous study by our group showed that a single treatment with CHX (0.1 or 0.2%) or CPC (0.05 or 0.1%) on 72 h saliva-grown microcosm biofilms resulted in colony-forming unit (CFU) reductions of only less than 1 log10 step (Schwarz et al., 2021). The biofilm matrix may be the main cause for this low antibacterial efficacy acting as a diffusion barrier for positively charged antiseptics such as CHX or CPC (Jakubovics et al., 2021). Therefore, it seems reasonable that bacteria in deeper layers of biofilms will be exposed to subinhibitory antiseptic concentrations upon application of antiseptic-containing mouthwash (Cieplik et al., 2019a; Mao et al., 2020; Schwarz et al., 2021; Muehler et al., 2022). Previous studies have shown that repeated exposure to subinhibitory concentrations of CHX or CPC in vitro may lead to phenotypic adaptation of bacteria to these antiseptics (Kitagawa et al., 2016; Cieplik et al., 2019a; Verspecht et al., 2019; Mao et al., 2020; Schwarz et al., 2021; Auer et al., 2022). Furthermore, selection pressure due to antiseptic treatment may lead to selection of antibiotic-resistant strains (Wand et al., 2017; Verspecht et al., 2019). Accordingly, we recently analyzed the transcriptomic stress response following sublethal treatment of Streptococcus mutans with CHX by RNA-sequencing and found considerable numbers of genes and pathways significantly upregulated or downregulated (Muehler et al., 2022). Particularly, upregulation of pathways related to stress response, increased biofilm formation, and regulation of membrane-transporters such as ATP-binding cassettes (ABC) may be linked to development of (cross-)resistances (Muehler et al., 2022).
Despite those concerns about limited antibacterial efficacy and potential risks of resistance, it is also not entirely understood which ecological changes in microbial composition of oral biofilms are induced by the routine use of antiseptic mouthwashes (Al-Kamel et al., 2019; Bescos et al., 2020a; Chatzigiannidou et al., 2020; Brookes et al., 2021; Zayed et al., 2022). Two recent studies have shown that antiseptic treatment of in vitro biofilms affected their microbial composition and may potentially result in ecological shifts toward increased abundance of pathobionts (Chatzigiannidou et al., 2020; Zayed et al., 2022).
Therefore, the aim for this study was first, to investigate ecological changes in mature saliva-grown microcosm biofilms upon two times daily application of CHX and CPC for a period of 7 days, and second, to evaluate, whether suchlike multiple application of CHX or CPC selects for resistant phenotypes.
Materials and Methods
Test Substances
Chlorhexidine digluconate (CHX; Sigma C9394) and cetylpyridinium chloride (CPC; Merck 6,002,006; both: Merck, Darmstadt, Germany) were used as antiseptics in the present study. CHX and CPC were both dissolved in dH2O and diluted to the respective treatment concentrations (0.1% and 0.2% for CHX, 0.05% and 0.1% for CPC).
Saliva Collection
Four healthy volunteers (age range: 30–32 years) with no untreated dental caries, periodontitis, or other oral diseases, and no intake of antibiotics within the past 3 months volunteered for collection of saliva. Written informed consents were obtained after a detailed description of the study outline. The study protocol had been approved by the internal review board of the University of Regensburg (ref. 17-782_1-101).
The sampling was performed as described earlier (Schwarz et al., 2021). Unstimulated saliva was collected using the spitting method (Navazesh and Christensen, 1982) with the volunteers not having consumed anything except water on the respective day. The volunteers were asked to let saliva gather on the bottom of their mouth and spit into a tube every 30 s for a total period of 10 min. For separating aggregated bacteria, the collected saliva was vortexed (REAX top, Heidolph Instruments, Schwabach, Germany) for 10 s, placed in an ultrasonic water-bath chamber (Sonorex Super RK 102 H, Bandelin, Berlin, Germany; 35 kHz) for 2 min, and vortexed again for 10 s. Afterwards, saliva was divided into two aliquots, i.e., 2 ml was used for immediate biofilm inoculation and 50 μl was used as a baseline sample for 16S rRNA sequencing. For baseline samples, microbial nucleic acids were immediately stabilized by mixing 50 μl of the saliva 1:2 with magic PBI microbiome preservation buffer (microBIOMix GmbH, Regensburg, Germany). Stabilized samples were stored at –80°C until further processing.
Inoculation and Culture of Saliva-Derived Microcosm Biofilms
Biofilms were cultured using the so-called Amsterdam Active Attachment (AAA) biofilm model, which is based on active attachment of the bacteria to a substrate. The AAA model consists of a custom-made stainless-steel lid with 24 clamps, which contain the respective substrates, and fits on top of a 24-well polystyrene microtiter plate, thus allowing 24 individual biofilms to form (Exterkate et al., 2010; Cieplik et al., 2019b; Schwarz et al., 2021). For the present study, hydroxyapatite (HAP) disks (9.5 mm diameter, 2 mm thickness; Clarkson Chromatography Products, South Williamsport, PA, United States) were used as a substrate in the AAA model. As a basal nutrient broth, the complete saliva broth as described by Pratten et al. (1998) was modified by adding sucrose (final concentration: 0.1%) for mimicking caries-associated conditions (caries broth; CB), as described earlier (Schwarz et al., 2021). For inoculation of the biofilms, 800 μl of the collected saliva was mixed with 40 ml CB and vigorously vortexed. Subsequently, 1.5 ml was added per each well of a 24-well plate (Corning® Costar®, Corning, NY, United States). The steel lids containing HAP disks were placed upon, and the AAA models were incubated anaerobically (80% N2, 10% CO2, 10% H2) at 37°C in a microincubator (MI23NK, SCHOLZEN Microbiology Systems, St. Margrethen, Switzerland) for 8 h thus allowing initial attachment to the HAP disks. After this initial attachment period, the lids containing the HAP disks were carefully moved up and down to remove loosely bound bacteria and transferred to 24-well plates containing fresh CB. Medium was refreshed again in the same way after 24 and 48 h of culture.
Two Times Daily Treatment of Saliva-Grown Microcosm Biofilms
After 72 h of culture, the biofilms were treated by placing the steel lid containing the HAP disks in a new 24-well plate containing either 0.1% CHX, 0.05% CPC, or 0.9% NaCl as a negative control for a treatment period of 5 min. Subsequently, the steel lid was placed in a new 24-well plate containing 0.9% NaCl to carefully wash the biofilms. This washing procedure was performed two times. Then, the steel lid was finally placed back onto a new 24-well plate containing fresh CB and incubated again anaerobically at 37°C. The biofilms were treated daily at 8 am and 4 pm for a period of 7 days resulting in 14 treatments. For each of the four donors, eight biofilms formed on separate HAP disks were used for treatment with 0.1% CHX, 0.05% CPC, or 0.9% NaCl each.
Harvesting of the Biofilms
After 7 days of treatment with CHX, CPC, or NaCl two times daily, all biofilms were harvested by carefully removing the HAP disks from the lids using sterile forceps and transferring them to 5 ml Eppendorf tubes containing 1 ml of phosphate-buffered saline (PBS; Biochrom, Berlin, Germany). Biofilm dispersal was ensured by vortexing for 10 s, placing in an ultrasonic water-bath chamber (35 kHz) for 10 min, and vortexing again for 10 s, and confirmed visually. From those harvested samples, 50 μl was immediately stabilized by mixing with 250 μl magic PBI microbiome preservation buffer and stored at –80°C until further processing. The remaining 750 μl was immediately used for culture-based analysis.
Extraction of Nucleic Acids and Semiconductor-Based Sequencing of Bacterial 16S rRNA Genes
Extraction of nucleic acids and semiconductor-based sequencing of bacterial 16S rRNA genes was performed, as described previously (Schwarz et al., 2021). First, pre-lysis of microbial cells was performed by mechanical cell disruption using repeated bead beating. Therefore, the total volumes of 150 μl (inoculum samples) or 300 μl (biofilm samples) stabilized sample material, respectively, were added into lysing matrix Y tubes (MP Biomedicals, Eschwege, Germany) and further processed in the TissueLyser II instrument (Qiagen, Hilden, Germany) at 60 Hz for 3 × 1 min. Nucleic acids were purified from total crude cell extracts using the MagNA Pure 96 instrument (Roche Diagnostics, Mannheim, Germany). Quantification of total nucleic acids was carried out by means of the NanoDrop™ 1000 spectrophotometer (Thermo Fisher Scientific, Darmstadt, Germany).
Copy numbers of bacterial 16S rRNA genes were quantified in nucleic acid extracts by qRT-PCR, as described earlier (Hiergeist et al., 2016). Subsequently, V1–V3 hypervariable regions of bacterial 16S rRNA genes were amplified from a total of 1e + 7 bacterial 16S rDNA copies for each sample using primer S-D-Bact-0008-c-S-20 containing a 10-bp barcode sequence and IonTorrent-specific sequencing adaptor A, and S-D-Bact-0517-a-A-18 containing a 3’-P1 adapter sequence using the Platinum II Taq Hot-Start DNA Polymerase (Thermo Fisher Scientific). After 30 PCR cycles, amplicons were purified two times with a 0.8 bead to DNA ratio using MagSi-NGSPREP Plus beads (Steinbrenner Laborsysteme, Wiesenbach, Germany). Copy numbers of amplicons containing sequencing-adaptors were determined using the KAPA Library Quantification IonTorrent Kit (Roche Diagnostics) and pooled to equimolar amplicon concentrations of each sample. A total of 120 attomol of the final library pool was subjected to isothermal amplification with the IonChef instrument before running 1350 flow cycles during high-throughput sequencing on an Ion Torrent™ S5 Plus machine (Thermo Fisher Scientific).
Sequence Processing and Identification of Amplicon Sequence Variants
First, amplification primer and adapter sequences and low-quality bases were removed using cutadapt 3.5 and Trimmomatic 0.39. Cutadapt was also used for demultiplexing of filtered reads allowing no errors. All subsequent analyses were conducted with R 4.1.2. Here, the resulting reads (19,573 ± 7,048) were subjected to denoising sequencing data and generation of Amplicon Sequence Variants (ASVs) using dada2 (version 1.16). An unrooted phylogenetic tree was calculated with FastTree 2.1 after sequence alignment with DECIPHER 2.20 for later calculation of UniFrac distances with the phyloseq package. The IDTAXA algorithm and the All-Species Living Tree Project (LTP) reference database 12.2021 release was used for taxonomic classification of ASVs. Significantly altered taxa between groups were assessed with the linear discriminant analysis (LDA) effect size (LEfSe) method which is included within the microbiomeMarker R package (Cao, 2021). All plots were generated using the ggpubr 0.4 package.
Colony-Forming Units Assay, Identification of Antiseptic-Resistant Phenotypes, and Evaluation of Antibiotic Susceptibility
Immediately after harvesting the biofilms, 10-fold serial dilutions (10–1 to 10–7) were prepared in PBS and aliquots (180 μl) were plated on Schaedler blood agar plates for determination of total CFU following the two times daily treatment with CHX, CPC, or NaCl over 7 days, and incubated anaerobically at 37°C for 72 h. Afterward, CFU were evaluated.
Furthermore, aliquots (180 μl) from the lowest dilution steps (10–1 to 10–4) were plated on Schaedler blood agar plates containing 0.1 or 0.2% CHX for CHX-treated and NaCl-treated biofilms or containing 0.05 or 0.1% CPC for CPC-treated and NaCl-treated biofilms in order to investigate for antiseptic-resistant phenotypes. After anaerobic incubation at 37°C for 72 h, the plates were evaluated for growth of antiseptic-resistant phenotypes. Those colonies were first discriminated according to their respective colony morphology and separated by sub-culturing on fresh agar plates. These colonies were identified at the species level by means of matrix-assisted laser desorption/ionization time-of-flight mass spectrometry (MALDI-TOF MS) employing a Microflex mass spectrometer and BioTyper analysis software (both from Bruker, Billerica, MA, United States), as described earlier (Cieplik et al., 2020).
The antiseptic-resistant isolates (despite Enterobacteriaceae) were analyzed for their antibiotic susceptibilities by means of the ETEST® method (bioMérieux, Marcy l’Etoile, France). In brief, suspensions (McFarland 1.0) of Capnocytophaga, Fusobacterium, and Veillonella spp. were inoculated on Brucella blood agar (bioMérieux), Campylobacter spp. on Mueller-Hinton (MH) agar with horse blood (Oxoid, Wesel, Germany), and Neisseria spp. on MH blood agar (Oxoid), and the ETEST® strips were placed on the agar plates. Results were evaluated following incubation for 48 h at 37°C under microaerophilic (Campylobacter spp.) or anaerobic (Capnocytophaga, Fusobacterium, Veillonella, and Neisseria spp.) conditions. Using ETEST®, the following antibiotics were investigated: penicillin G, ampicillin, amoxicillin/clavulanic acid, piperacillin/tazobactam, imipenem, ceftriaxone/cefotaxime, ceftazidime, ciprofloxacin, erythromycin, clindamycin, tetracycline, and metronidazole.
Antibiotic susceptibilities of Enterobacteriaceae were tested using the BD Phoenix NMIC panel (Becton Dickinson, Sparks, MD, United States) according to the instructions of the manufacturer for the following antibiotics: ampicillin/amoxicillin, amoxicillin/clavulanic acid, piperacillin, piperacillin/tazobactam, imipenem, meropenem, ertapenem, aztreonam, cefuroxime, cefoxitin, ceftriaxone/cefotaxime, ceftazidime, cefepime, ciprofloxacin, levofloxacin, gentamicin, amikacin, tobramycin, fosfomycin, and trimethoprim/sulfamethoxazole. Interpretation of the results of both methods was done according to the EUCAST (European Committee on Antimicrobial Susceptibility Testing) 12.0 guidelines, and susceptibility was determined as susceptible (S), intermediate (I), or resistant (R), whereby non-species related breakpoints were used if no species-specific breakpoints were available.
Results
Microbial Composition of Saliva-Grown Microcosm Biofilms and Ecological Effects of Daily Treatment With Chlorhexidine Digluconate or Cetylpyridinium Chloride
A total of 4,036 (mean 294 ± 115 per sample) amplicon sequence variants (ASVs) were detected by high-throughput sequencing of V1–V3 variable regions of bacterial 16S rRNA genes. Alpha-diversity represented by the number of detected ASVs (Figure 1A) and the Effective Shannon Index (Figure 1B) was significantly lower in CHX-treated biofilms (mean 203 ± 72 ASVs; mean 19 ± 11 Shannon) as compared to NaCl-treated (mean 344 ± 84 ASVs; mean 63 ± 16 Shannon) or CPC-treated biofilms (mean 335 ± 125 ASVs; mean 59 ± 25 Shannon) with no significant differences between the latter.
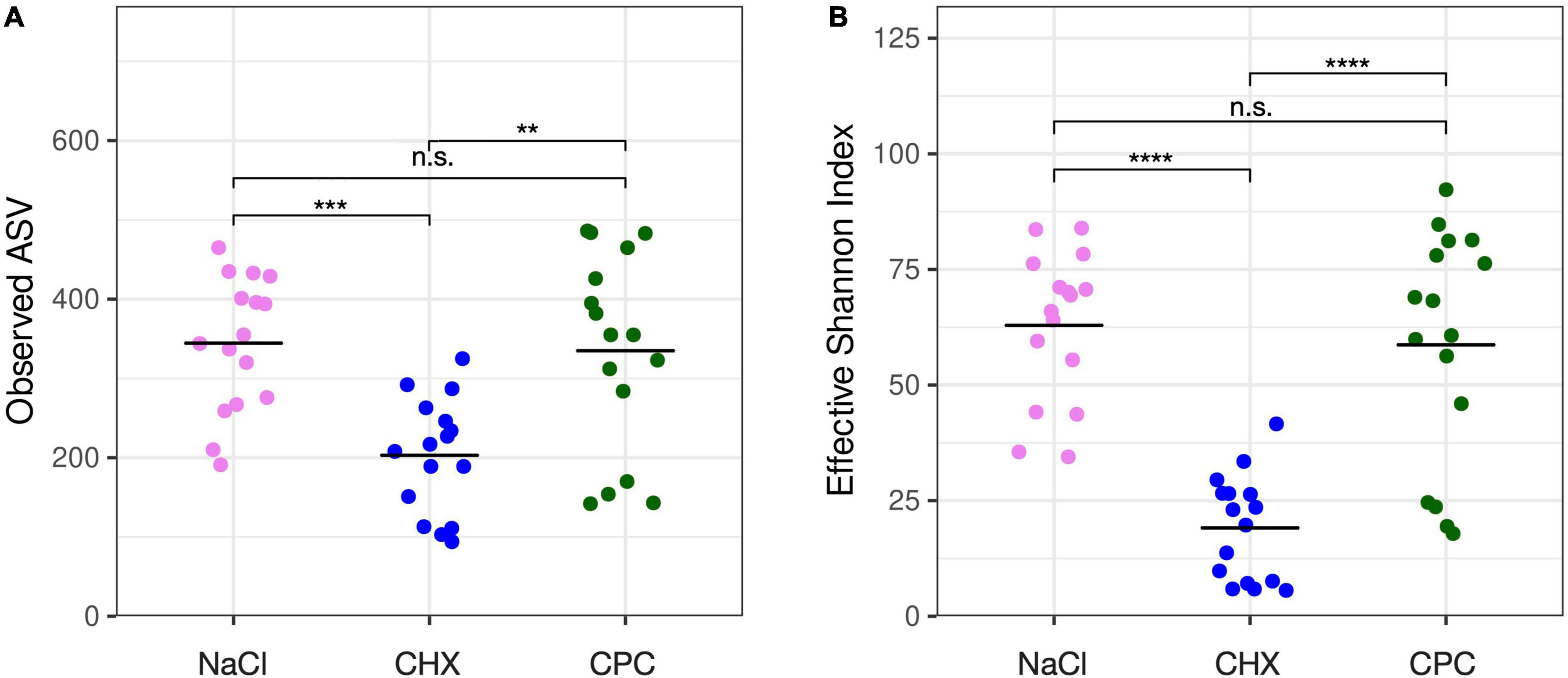
Figure 1. Alpha-diversity of the biofilms as shown by observed ASVs (A) and effective Shannon index (B). CHX-treated biofilms showed significantly lower alpha-diversity as compared to NaCl-treated or CPC-treated biofilms with no significant differences between the latter. Significance levels are indicated by asterisks: **p ≤ 0.01, ***p ≤ 0.001, ****p ≤ 0.0001.
Figure 2 depicts a heatmap of ASV abundance on genus level for NaCl-treated, CHX-treated, and CPC-treated biofilms from all four donors. The biofilms show a diverse microbial composition with Streptococcus, Veillonella, Fusobacterium, Haemophilus, and Granulicatella spp. being the most abundant. The heatmap clearly depicts the differences in microbial composition between the biofilms from different donors as well depending on the respective treatment. Accordingly, beta-diversity based on weighted UniFrac distances showed clear clustering depending on the donor (Figure 3A; Adonis R2 = 0.25, p.adj = 0.001) and also regarding their respective treatment (Figure 3B; Adonis R2 = 0.41, p.adj = 0.001).
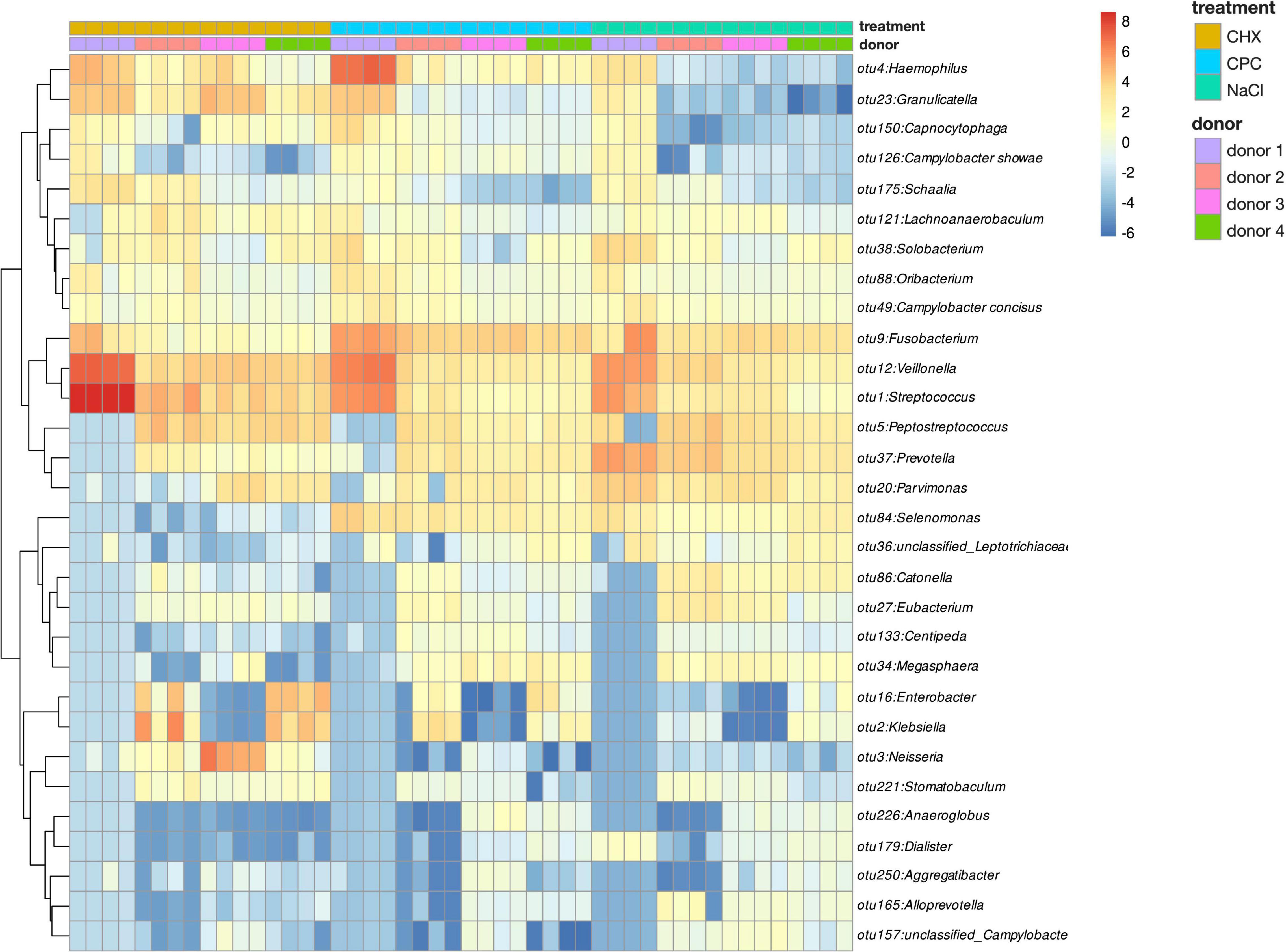
Figure 2. Heatmap of ASV abundance on genus level for NaCl-, CHX-, and CPC-treated biofilms from all four donors.
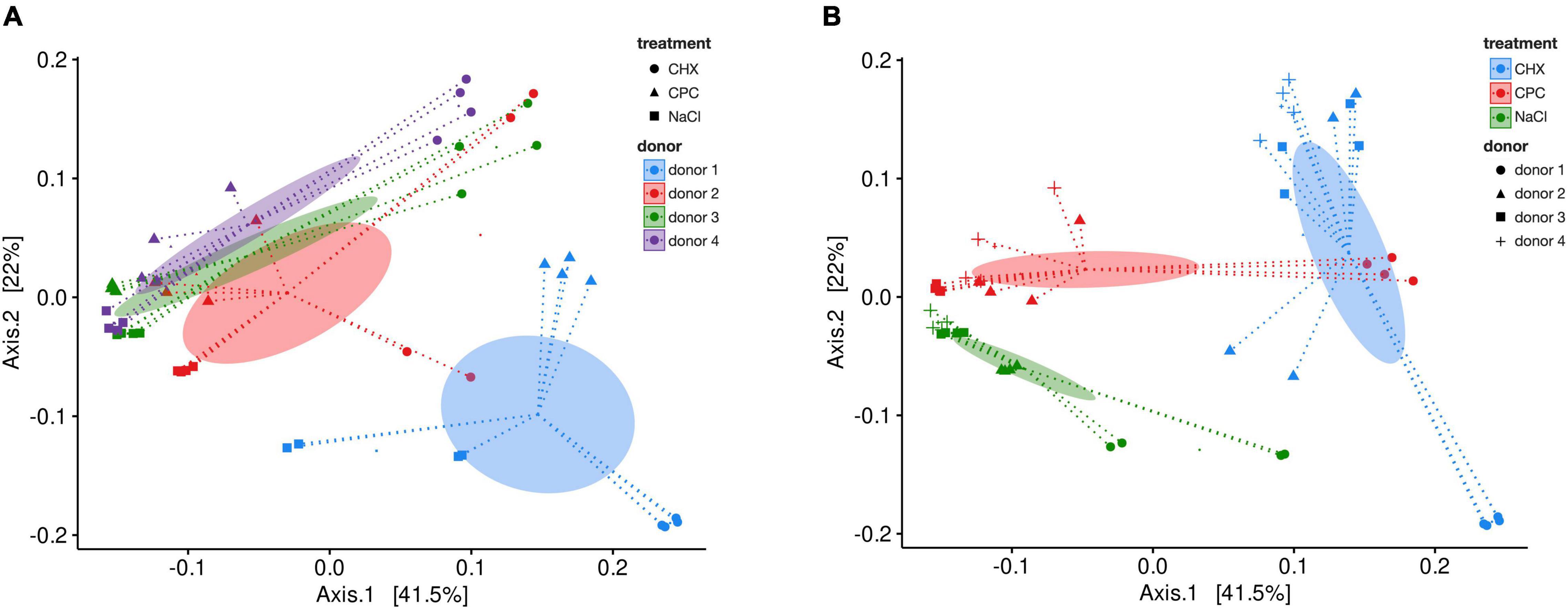
Figure 3. Beta-diversity analysis by principal coordinate analysis (PCoA) of weighted UniFrac distances for NaCl-, CHX-, and CPC-treated biofilms from all four donors. Ellipses indicate the 95% confidence interval of group centroids summarized for donors (A) or treatments (B). Depicted are coordinates 1 and 2, which explained 63.5% of the total variance. (Adonis NaCl vs. CHX: R2 = 0.42, p.adj = 0.003, NaCl vs. CPC: R2 = 0.23, p.adj = 0.003, CHX vs. CPC: R2 = 0.36, p.adj = 0.003).
Several ASVs were found to be discriminatory between the biofilms treated by CHX, CPC, or NaCl, as revealed by LefSE (Figure 4). Accordingly, the CHX-treated biofilms were characterized by the enrichment of several ASVs within the orders Lactobacillales (mainly Streptococcus and Granulicatella spp.), Neisseriales (mainly Neisseria spp.), and Actinomycetales (mainly Schaalia spp.). In contrast, CPC-treated biofilms were enriched by several ASVs within the orders Fusobacteriales (mainly Fusobacterium and Leptotrichia spp.), Selenomonadales (mainly Selenomonas spp.), Pasteurellales (mainly Haemophilus spp.), and Campylobacterales, as well as Oribacterium and Prevotella loescheii. Furthermore, both CHX- and CPC-treated biofilms were characterized by a loss of several Prevotella, Catonella, and Parvimonas spp. as compared to the NaCl-treated biofilms.
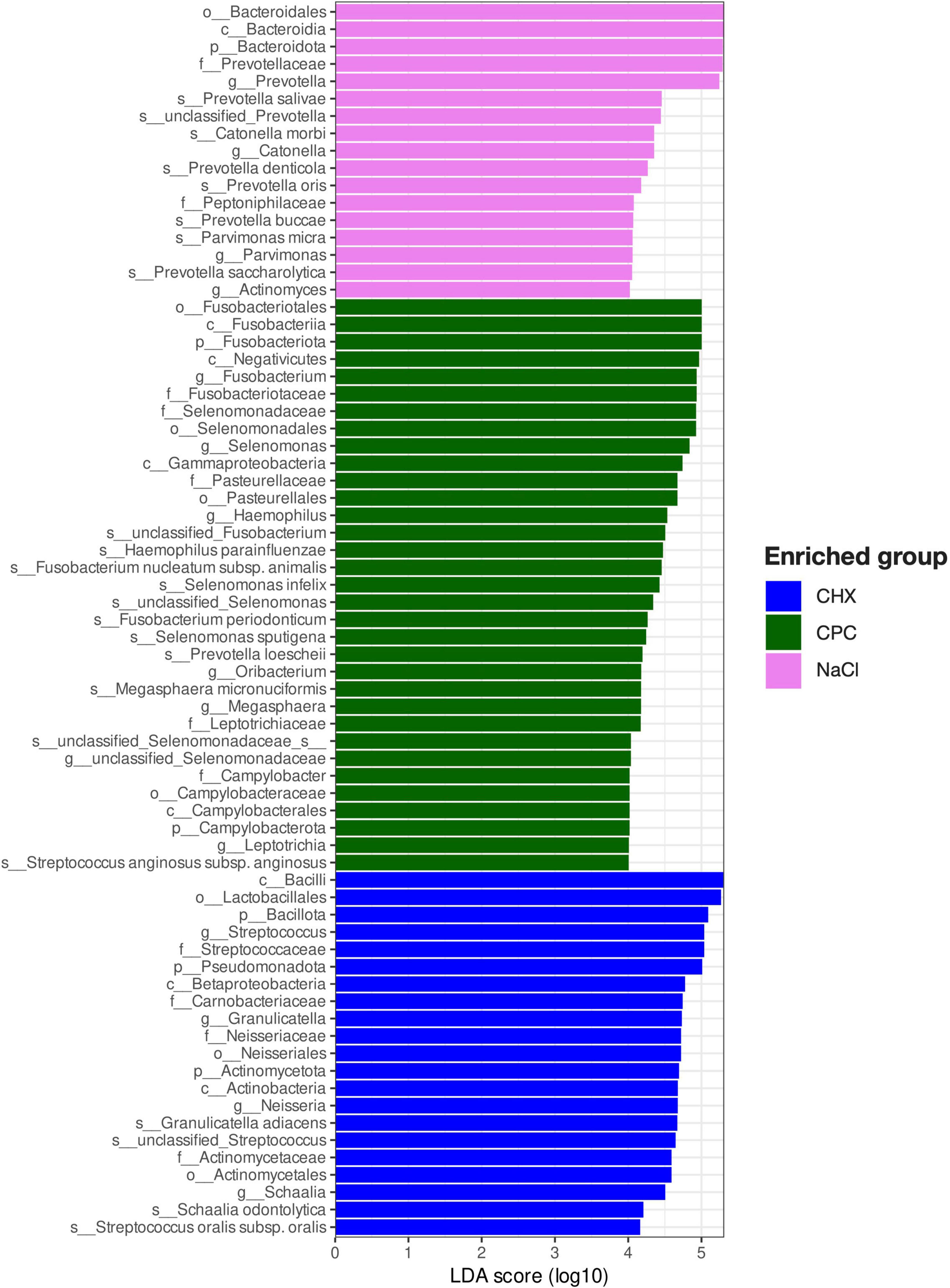
Figure 4. Discriminatory ASVs for biofilms treated with NaCl, CPC, or CHX, respectively, as identified by linear discriminant analysis (LDA) effect size (LEfSe). ASVs exhibiting LDA-score ≥ 4 and adjusted p-values < 0.01 are shown.
Colony-Forming Units Assay and Identification of Antiseptic-Resistant Phenotypes
Figure 5 shows the CFU assay results. Biofilms treated with NaCl exhibited median CFU numbers of 9.3 × 107 CFU, while CHX- or CPC-treated biofilms showed 3.3 × 106 or 2.5 × 107 CFU, respectively, resulting in CFU-reductions of 1.5 log10 steps for CHX and 0.6 log10 steps for CPC as compared to the biofilms treated with NaCl.
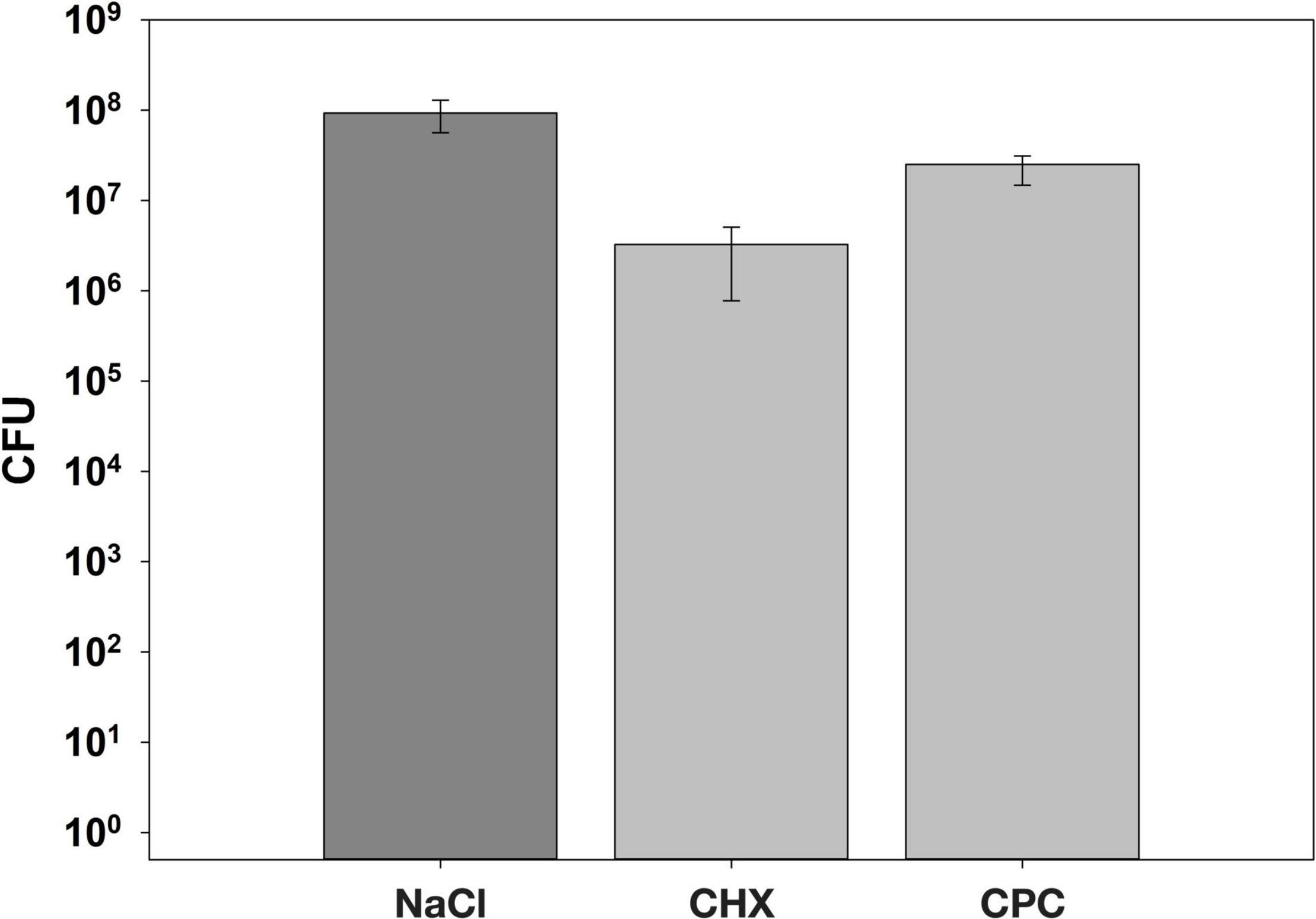
Figure 5. The CFU results following two times daily treatment with either 0.9% NaCl, 0.1% CHX, or 0.05% CPC for a period of 7 days. All results are depicted as medians, 1st and 3rd quartiles from eight individual biological replicates on a log10-scaled ordinate.
Table 1 summarizes the antiseptic-resistant phenotypes as identified by MALDI-TOF MS along with their respective antibiotic susceptibilities as tested using ETEST® or by means of the BD Phoenix NMIC panel. In each donor, at least one taxon could be isolated that was able to grow on the antiseptic-containing agar plates. In donor 1, Capnocytophaga sputigena was found on the CHX-containing agar plate from the NaCl-treated biofilm and was susceptible to all tested antibiotics, while Campylobacter showae, which was isolated on the CPC-containing agar plate from both NaCl- and CPC-treated biofilms presented resistance to ciprofloxacin and piperacillin/tazobactam. Moreover, Campylobacter showae, which was isolated on the CPC-containing agar plate from CPC-treated biofilms, showed additional resistance to Penicillin G. In donor 2, only taxa identified as Klebsiella oxytoca or Raoultella sp. were isolated on both CHX- and CPC-containing agar from the corresponding antiseptic-treated biofilms. Both Klebsiella oxytoca and Raoultella sp. isolates showed resistance to ampicillin/amoxicillin, piperacillin, and fosfomycin. The highest number of antiseptic-resistant phenotypes was isolated from donor 3. Capnocytophaga sputigena (susceptible to all tested antibiotics) and Capnocytophaga gingivalis (susceptible to all tested antibiotics) were isolated on the CHX-containing agar plate from the NaCl-treated biofilms, while Fusobacterium sp. (resistant to penicillin G, ampicillin and ciprofloxacin), Veillonella rogosae (resistant to clindamycin and intermediate resistant to ceftazidime), and Campylobacter curvus (intermediate resistant to ciprofloxacin) were isolated on the CPC-containing agar plate from the NaCl-treated biofilm. Additionally, Neisseria perflava (resistant to penicillin G, ampicillin and ceftriaxone/cefotaxime) was isolated from the CHX-treated biofilm and Veillonella rogosae (resistant to clindamycin) was isolated from the CPC-treated biofilm. In donor 4, two strains identified as Klebsiella oxytoca or Raoultella sp. were collected from NaCl- or CPC-treated biofilms and isolated from CHX- or CPC-containing agar. Both showed resistance to ampicillin/amoxicillin, piperacillin, and fosfomycin. One taxon identified to be from the Enterobacter cloacae-complex was isolated on the CHX-containing agar originating from the CHX-treated biofilms growing and exhibited resistance to ampicillin/amoxicillin, amoxicillin/clavulanic acid, piperacillin, piperacillin/tazobactam, and cefazolin.
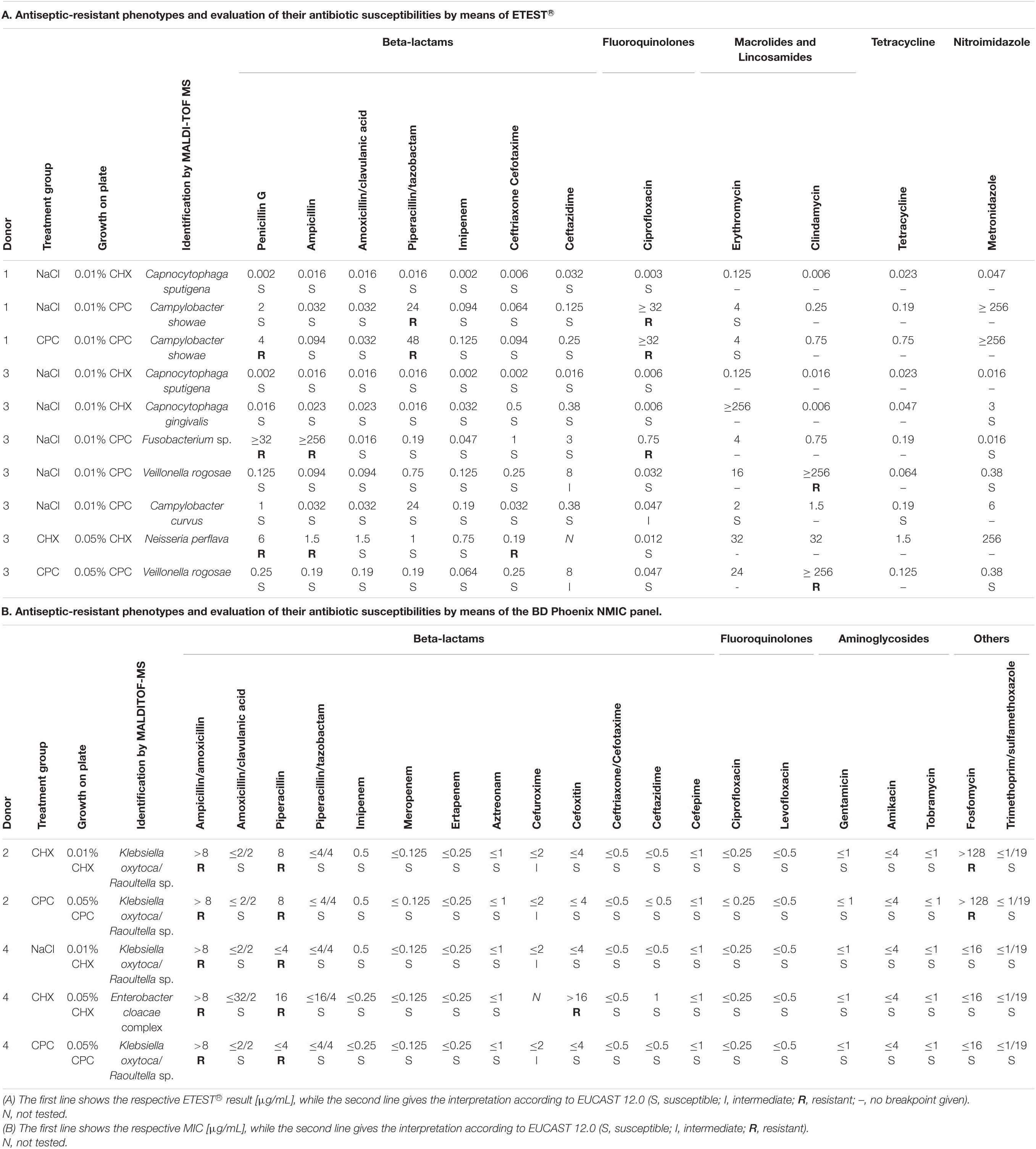
Table 1. Antiseptic-resistant phenotypes isolated from the biofilms and evaluation of antibiotic susceptibilities.
Discussion
Antiseptics are in widespread use in dental practice and also included in numerous over-the-counter oral care products (Haps et al., 2008; Sanz et al., 2013; van der Weijden et al., 2015), but the effects of routine antiseptic use on microbial composition of oral biofilms (Chatzigiannidou et al., 2020; Zayed et al., 2022) and on the emergence of resistant phenotypes are still unclear (Cieplik et al., 2019a; Mao et al., 2020). Therefore, this study aimed to investigate the ecological effects of daily treatment with CHX or CPC on mature saliva-grown biofilms and whether a suchlike treatment selects for resistant phenotypes.
For this purpose, microcosm biofilms were cultured from human saliva employing the so-called Amsterdam Active Attachment (AAA) biofilm model, as described previously (Schwarz et al., 2021). Sampling was performed from healthy donors, and a basal nutrient broth mimicking human saliva was modified by adding sucrose (Pratten et al., 1998; Cieplik et al., 2013; Schwarz et al., 2021) to provide environmental conditions leading to biofilms that resemble microbial communities in rather early stage of dysbiosis dominated by early colonizers of dental plaque (Schwarz et al., 2021). While the biofilms in this previous study showed rather low alpha-diversity and mostly growth of Streptococcus and Veillonella spp., a considerably higher alpha-diversity was found for the biofilms in the present study. This may be attributed to several factors: Once, HAP disks were used as a substrate for biofilm culture instead of glass disks as used previously (Schwarz et al., 2021). These HAP disks may better mimic dental hard tissues and might improve bacterial attachment toward the substrate (Hannig and Hannig, 2009), which is particularly crucial for the AAA model used in the present study, as it relies on the active attachment of bacteria (Exterkate et al., 2010; Cieplik et al., 2019b). Furthermore, another important aspect may be the different period for culture of the biofilms. While the biofilms were cultured only for 3 days in the previous study (Schwarz et al., 2021), here, the biofilms were cultured for 10 days in total (3 days of biofilm formation followed by 7 days of treatment with NaCl, CHX, or CPC), which may have given the more fastidious bacteria more time to establish themselves in the biofilms (Edlund et al., 2013; Kistler et al., 2015; Cieplik et al., 2019b). The microbial compositions of the biofilms were found dependent on the respective donor source, which is in line with the results from a previous study, where we found a much stronger clustering of microbial compositions of biofilms per each donor than per niche of each donor even after up to 28 days of in vitro culture, indicating a strong donor-driven “fingerprint” (Cieplik et al., 2019b). Similar results were reported by Chatzigiannidou et al. (2020) who also observed a strong donor-dependency regarding microbial composition of their tongue-swab-derived microcosm biofilms. Notably, here, the biofilms from one donor (donor 1) clustered particularly different from the other three donors. This may be explained by the different ethnicity of this donor (Asian, while the other three donors were Caucasian), as also shown in previous studies (Mason et al., 2013; Premaraj et al., 2020). For instance, microbial communities in saliva and subgingival biofilms were found to have distinct ethnicity profiles, and based on these results, it was even possible to identify the ethnicity of individuals from subgingival microbial signatures using a machine learning classifier (Mason et al., 2013). Furthermore, a recent metagenome-wide association study found that human genetics account for at least 10% of oral microbiome compositions between different individuals, which may also explain the stable microbial composition within one single individual over time (Liu et al., 2021).
After undisturbed culture of the biofilms for 3 days, a mouthwash was simulated two times daily using either of the tested compounds for 5 min each. The AAA biofilm model facilitates controlling treatment periods as opposed to other biofilms models, which are based on bacterial sedimentation rather than active bacterial attachment (Exterkate et al., 2010). Due to the well-known high substantivity of CHX and CPC (Elworthy et al., 1996), biofilms were washed after the 5 min treatment period to dilute potentially remaining CHX or CPC and limit potential prolonged effects of both antiseptics. The two times daily treatment with CHX or CPC reduced CFU in the biofilms only by 1.5 log10 or 0.6 log10 steps as compared to the NaCl group. These results clearly show that both antiseptics exhibited only temporary effects and could not inhibit bacterial regrowth, in line with several other studies indicating that antiseptic mouthwashes are not able to effectively limit microbial numbers, particularly when applied to mature biofilms (Cieplik et al., 2019a; Chatzigiannidou et al., 2020; Brookes et al., 2021; Schwarz et al., 2021). Therefore, it is crucial to investigate the ecological effects on the microbial composition of the surviving microbiota, which is still discussed controversially for CHX and has not been investigated so far for CPC (Al-Kamel et al., 2019; Bescos et al., 2020a; Chatzigiannidou et al., 2020; Brookes et al., 2021).
We found that treatment with CHX significantly reduced alpha-diversity in the biofilms as compared to treatment with CPC or NaCl (with no significant difference between the latter), in accordance with several other studies evaluating the effects of CHX on microbial communities in vitro and in vivo (Fernandez et al., 2017; Tribble et al., 2019; Brookes et al., 2020, 2021; Chatzigiannidou et al., 2020). Furthermore, beta-diversity showed a strong and significant ecological shift following treatment with CHX, resulting in enrichment of rather caries-associated taxa such as Streptococcus, Neisseria, Schaalia [genus recently created by subdivision from Actinomyces (Nouioui et al., 2018)], and Granulicatella spp. For instance, the significantly enriched species Streptococcus oralis subsp. oralis and Schaalia odontolytica (formerly classified as Actinomyces odontolyticus) have been associated with dental caries (ElSalhy et al., 2016; Da Costa Rosa et al., 2021). Bescos et al. (2020a) investigated the effects of 7-day use of a CHX mouthwash on the salivary microbiota in 36 healthy individuals. They observed an increase in the abundance of taxa from the genera Streptococcus, Neisseria, and Granulicatella, but a decrease of Actinomyces (Bescos et al., 2020a), and a significantly lower salivary pH and buffer capacity after using the CHX mouthwash for 7 days, concluding that CHX may have a significant impact on the oral microbiota, potentially favoring dental caries (Bescos et al., 2020a). Likewise, Chatzigiannidou et al. (2020) found an ecological shift toward a streptococci-dominated microbial community and increased lactate production after treating in vitro 14-species biofilms with CHX over 3 days for 5 min each, while they observed a contrary trend with increase in Granulicatella and Fusobacterium spp. after treating microcosm biofilms inoculated from tongue scrapings.
Interestingly, treatment with CPC had a different effect on the biofilms. Alpha-diversity was not significantly affected as compared to the NaCl-treated biofilms, but beta-diversity also revealed a significant ecological shift, resulting in enrichment of proteolytic and Gram-negative taxa such as Fusobacterium, Leptotrichia, and Selemonas spp. as well as Oribacterium, which are mainly associated with gingivitis (Diaz et al., 2016; Bryan et al., 2017; Nowicki et al., 2018).
Both antiseptic treatments led to a loss of Prevotella spp., which are known nitrite producers and associated with high nitrate-reduction capacity (Hyde et al., 2014; Rosier et al., 2022). Accordingly, clinical studies have shown that the use of CHX mouthwashes led to lower nitrite concentrations in saliva and plasma followed by slight increases in systolic blood pressure (Tribble et al., 2019; Bescos et al., 2020a). Although the oral microbiota is known to be highly resilient, particularly as compared to the intestinal microbiota (Wade, 2021), clinicians should be aware of potential detrimental effects of long-term use of antiseptic mouthwashes with regard to oral microbial ecology (Bescos et al., 2020b), which may potentially further perturb the commensal microbiota rather than shifting to a health-associated state (Chatzigiannidou et al., 2020). However, it needs to be considered that here the effects of “pure” antiseptics were investigated, whereas the effects of antiseptic mouthwashes seem to be strongly dependent on their respective compositions and formulations, as recently shown (Zayed et al., 2022). Also, a commercially available mouthwash comprising both CHX and CPC showed capability to even improve the microbial ecology of a 14-species biofilm in vitro reducing the level of pathobionts to less than 10% (Zayed et al., 2022), whereas in our study, CHX led to enrichment of rather caries-associated saccharolytic taxa and CPC led to enrichment of rather gingivitis-associated proteolytic taxa.
Despite analyzing effects on biofilm ecology, we also sought to investigate whether antiseptic treatment selects for resistant phenotypes. For this purpose, an agar dilution technique was employed, and the biofilms were plated on Schaedler agar plates containing 0.01 or 0.05% CHX or CPC, respectively. Although this method is in line with the guidelines of the Clinical Laboratory Standards Institute (CLSI) (CLSI, 2018a,b) and has also been used in earlier studies investigating antiseptics (Eick et al., 2011; Akca et al., 2016), it should be considered that the biologically available concentrations on the surface of the plates may not necessarily reflect the rather high concentrations mixed into the agar plates, due to potential interactions of the cationic antiseptics and constituents of the solid growth media (Akca et al., 2016). Thus, only qualitative, but no quantitative results (i.e., CFU numbers) are reported in this study. We found antiseptic-resistant phenotypes in biofilms from each donor, which also showed resistance to various antibiotics. As some of them were isolated from the NaCl-treated biofilms, these isolates originate from the inoculum source and could establish in the biofilms even without selection pressure due to the two times daily antiseptic treatment, supporting recent studies, which highlight the oral microbiota as a reservoir of antimicrobial resistance (AMR) genes (Jiang et al., 2018; Arredondo et al., 2020).
The isolated antiseptic-resistant phenotypes were found to be highly donor-dependent: In the biofilms from two donors (1 and 3), typical oral taxa could be isolated from the antiseptic-containing agar plates. Capnocytophaga spp. could be isolated from CHX-containing agar, in line with older studies reporting that Capnocytophaga spp. exhibited minimum inhibitory concentrations (MICs) for CHX of up to 250 μg/ml (0.025%) and were the least susceptible among all oral bacteria included in these experiments (Stanley et al., 1989; Wade and Addy, 1989). Furthermore, the earliest study probably reporting isolation of this genus was from a group of dental students following use of a 0.2% CHX mouthwash for 22 days (Davies et al., 1972; Leadbetter et al., 1979). Two V. rogosae strains, which were both isolated from CPC-containing agar, showed high-level resistance to clindamycin, which was also found in 55% of Veillonella isolates in a previous study (Teng et al., 2002). N. perflava isolated from CHX-treated biofilms on CHX-containing agar was found resistant to penicillin G, ampicillin, and ceftriaxone/cefotaxime, which is in line with a recent systematic review stating that antimicrobial susceptibilities in commensal as well as pathogenic Neisseria spp. have been increasing considerably following decades of antibiotic exposure (Vanbaelen et al., 2022). Remarkably, the MIC of 6 μg/ml found here for penicillin G is higher than all reported for commensal Neisseria spp. in this systematic review (Vanbaelen et al., 2022). Campylobacter spp. could be isolated from CPC-containing agar. The two C. showae isolates showed high-level resistance to ciprofloxacin and piperacillin/tazobactam, while they were sensitive to amoxicillin/clavulanic acid. Resistance to piperacillin has previously been described in poultry isolates of Campylobacter spp., although MICs of piperacillin/tazobactam were 16- to 32-fold lower than for piperacillin alone, they still were found quite high (around 32 μg/ml) (Griggs et al., 2009), in line with the MICs found here. In Campylobacter spp., resistance to fluoroquinolones like ciprofloxacin is mainly due to single point mutation(s) in the gyrA gene (Wieczorek and Osek, 2013; Sproston et al., 2018). An isolate of the genus Fusobacterium, which was obtained from CPC-containing agar, exhibited high-level resistance to penicillin G and ampicillin, which was also found previously and attributed to be due to expression of a class D beta-lactamase (Al-Haroni et al., 2008).
Apart from these typical oral taxa, Enterobacteriaceae could be isolated from the biofilms from donors 2 and 4. Although members of Enterobacteriaceae are usually considered as transient components of the oral microbiota, they have consistently been detected at low numbers in subgingival biofilm samples (Espíndola et al., 2022; Jepsen et al., 2022) and also on toothbrushes (Zinn et al., 2020). For instance, Jepsen et al. (2022) recently analyzed biofilm samples collected between 2008 and 2015 from deep periodontal pockets in 16,612 German adults diagnosed with periodontitis and found mean annual prevalence rates of 3.6% for K. oxytoca and 2.5% for E. cloacae. The fosfomycin resistance detected in two K. oxytoca/Raoultella sp. isolates may be attributable to the chromosomal fosA gene, which is present in the majority of genomes in Klebsiella spp. (Ito et al., 2017). Likewise, the resistance of all Enterobacteriaceae isolated in the present study to aminopenicillins such as ampicillin, amoxicillin, and piperacillin and, in part, to second-generation cephalosporins like cefuroxime or cefoxitin may be mainly attributed to their production of AmpC-type beta-lactamases (Meini et al., 2019). Thus, adjunctive prescription of amoxicillin, e.g., in the course of periodontal treatment, may pose the risk of overgrowth of such taxa in oral biofilms (Jepsen et al., 2022). Accordingly, Baker at al. (2019) reported recently that K. oxytoca, K. pneumoniae, and Providencia alcalifaciens were the only taxa found to be transcriptionally active and recoverable after long-term starvation of a saliva-derived microbial community over 100 days in vitro, which may explain that hospital surfaces contaminated with saliva can serve as source of outbreaks of drug-resistant Enterobacteriaceae. Although such long-term starvation does not reflect the environmental conditions in the oral cavity (Baker et al., 2019), it may be similar to long-term selective pressure due to extensive use of antiseptic mouthwashes (Cieplik et al., 2019a; Mao et al., 2020). Besides selection of intrinsically less susceptible phenotypes, adaptation to antiseptics such as CHX may also occur, which was recently linked to development of antibiotic cross-resistance to colistin in K. pneumoniae (Wand et al., 2017).
Conclusion
This study shows that both CHX and CPC exhibited significant ecological effects on the microbial compositions of microcosm biofilms upon two times daily treatment for a period of 7 days. CHX led to enrichment of rather caries-associated saccharolytic taxa as compared to NaCl-treated biofilms, while CPC led to enrichment of rather gingivitis-associated proteolytic taxa. Antiseptic-resistant phenotypes could be isolated from all biofilms regardless which treatment group they belonged to. These isolates exhibited resistance to various antibiotics. Therefore, further studies are needed to elucidate implications of the widespread use of antiseptics in oral healthcare with regard to their ecological effects on oral biofilms as well as on the spread of antimicrobial resistance. Clinicians should be aware of the potential risks associated with the widespread and indiscriminate use of antiseptics and apply or prescribe them only for appropriate indications and preferably only for short periods of time.
Data Availability Statement
The datasets presented in this study can be found in online repositories. The names of the repository/repositories and accession number(s) can be found below: NCBI – PRJEB52604.
Ethics Statement
The studies involving human participants were reviewed and approved by the Internal Review Board of the University of Regensburg (ref.: 17-782_1-101). The participants provided their written informed consent to participate in this study.
Author Contributions
FC, AA-A, AH, and AG conceived and designed the experiments. XM, AH, DA, KS, and DM performed the experiments. XM, AH, FC, AG, KS, K-AH, TM, WB, and EH analyzed and interpreted the data. FC and AA-A acquired the funding and supervised the study. FC, XM, and AH wrote the manuscript with input from all authors. All authors reviewed and approved the manuscript.
Funding
This study was funded by the Deutsche Forschungsgemeinschaft (DFG, German Research Foundation; grants CI 263/3-1 and AL 1179/4-1) and by the Bavarian Ministry of Science and the Arts in the framework of the Bavarian Research Network ‘New Strategies Against Multi-Resistant Pathogens by Means of Digital Net-working – bayresq.net, project “IRIS”’. XM and DA thank for funding by doctoral scholarships from the Affiliated Stomatology Hospital of Tongji University (Shanghai, China) or the Medical Faculty of the University of Regensburg (Regensburg, Germany), respectively.
Conflict of Interest
The authors declare that the research was conducted in the absence of any commercial or financial relationships that could be construed as a potential conflict of interest.
Publisher’s Note
All claims expressed in this article are solely those of the authors and do not necessarily represent those of their affiliated organizations, or those of the publisher, the editors and the reviewers. Any product that may be evaluated in this article, or claim that may be made by its manufacturer, is not guaranteed or endorsed by the publisher.
Acknowledgments
Heike Preuschl and Stefan Lukas are gratefully acknowledged for their excellent technical support.
References
Akca, A. E., Akca, G., Topçu, F. T., Macit, E., Pikdöken, L., and Özgen, I. Ş. (2016). The Comparative Evaluation of the Antimicrobial Effect of Propolis with Chlorhexidine against Oral Pathogens: an In Vitro Study. Biomed Res. Int. 2016:3627463. doi: 10.1155/2016/3627463
Al-Haroni, M., Skaug, N., Bakken, V., and Cash, P. (2008). Proteomic analysis of ampicillin-resistant oral Fusobacterium nucleatum. Oral Microbiol. Immunol. 23, 36–42. doi: 10.1111/j.1399-302X.2007.00387.x
Al-Kamel, A., Baraniya, D., Al-Hajj, W. A., Halboub, E., Abdulrab, S., Chen, T., et al. (2019). Subgingival microbiome of experimental gingivitis: shifts associated with the use of chlorhexidine and N-acetyl cysteine mouthwashes. J. Oral Microbiol. 11:1608141. doi: 10.1080/20002297.2019.1608141
Arredondo, A., Blanc, V., Mor, C., Nart, J., and León, R. (2020). Tetracycline and multidrug resistance in the oral microbiota: differences between healthy subjects and patients with periodontitis in Spain. J. Oral Microbiol. 13:1847431. doi: 10.1080/20002297.2020.1847431
Auer, D. L., Mao, X., Anderson, A. C., Muehler, D., Wittmer, A., von Ohle, C., et al. (2022). Phenotypic Adaptation to Antiseptics and Effects on Biofilm Formation Capacity and Antibiotic Resistance in Clinical Isolates of Early Colonizers in Dental Plaque. Antibiotics 11:688. doi: 10.3390/antibiotics11050688
Baker, J. L., Hendrickson, E. L., Tang, X., Lux, R., He, X., Edlund, A., et al. (2019). Klebsiella and Providencia emerge as lone survivors following long-term starvation of oral microbiota. Proc. Natl. Acad. Sci. U. S. A. 116, 8499–8504. doi: 10.1073/pnas.1820594116
Bescos, R., Ashworth, A., Cutler, C., Brookes, Z. L., Belfield, L., Rodiles, A., et al. (2020a). Effects of Chlorhexidine mouthwash on the oral microbiome. Sci. Rep. 10:5254. doi: 10.1038/s41598-020-61912-4
Bescos, R., Casas-Agustench, P., Belfield, L., Brookes, Z., and Gabaldón, T. (2020b). Coronavirus Disease 2019 (COVID-19): emerging and Future Challenges for Dental and Oral Medicine. J. Dent. Res. 99:1113. doi: 10.1177/0022034520932149
Brookes, Z. L. S., Belfield, L. A., Ashworth, A., Casas-Agustench, P., Raja, M., Pollard, A. J., et al. (2021). Effects of chlorhexidine mouthwash on the oral microbiome. J. Dent. 113:103768. doi: 10.1016/j.jdent.2021.103768
Brookes, Z. L. S., Bescos, R., Belfield, L. A., Ali, K., and Roberts, A. (2020). Current uses of chlorhexidine for management of oral disease: a narrative review. J. Dent. 103:103497. doi: 10.1016/j.jdent.2020.103497
Bryan, N. S., Tribble, G., and Angelov, N. (2017). Oral Microbiome and Nitric Oxide: the Missing Link in the Management of Blood Pressure. Curr. Hypertens. Rep. 19:33. doi: 10.1007/s11906-017-0725-2
Carrouel, F., Gonçalves, L. S., Conte, M. P., Campus, G., Fisher, J., Fraticelli, L., et al. (2021). Antiviral Activity of Reagents in Mouth Rinses against SARS-CoV-2. J. Dent. Res. 100, 124–132. doi: 10.1177/0022034520967933
Ceri, H., Olson, M. E., Stremick, C., Read, R. R., Morck, D., and Buret, A. (1999). The Calgary Biofilm Device: new technology for rapid determination of antibiotic susceptibilities of bacterial biofilms. J. Clin. Microbiol. 37, 1771–1776. doi: 10.1128/JCM.37.6.1771-1776.1999
Chatzigiannidou, I., Teughels, W., van de Wiele, T., and Boon, N. (2020). Oral biofilms exposure to chlorhexidine results in altered microbial composition and metabolic profile. NPJ Biofilms Microbiomes 6:13. doi: 10.1038/s41522-020-0124-3
Cieplik, F., Jakubovics, N. S., Buchalla, W., Maisch, T., Hellwig, E., and Al-Ahmad, A. (2019a). Resistance Toward Chlorhexidine in Oral Bacteria - Is There Cause for Concern? Front. Microbiol. 10:587. doi: 10.3389/fmicb.2019.00587
Cieplik, F., Zaura, E., Brandt, B. W., Buijs, M. J., Buchalla, W., Crielaard, W., et al. (2019b). Microcosm biofilms cultured from different oral niches in periodontitis patients. J. Oral Microbiol. 11:1551596. doi: 10.1080/20022727.2018.1551596
Cieplik, F., Späth, A., Regensburger, J., Gollmer, A., Tabenski, L., Hiller, K.-A., et al. (2013). Photodynamic biofilm inactivation by SAPYR–an exclusive singlet oxygen photosensitizer. Free Radic Biol. Med. 65, 477–487. doi: 10.1016/j.freeradbiomed.2013.07.031
Cieplik, F., Wiedenhofer, A. M., Pietsch, V., Hiller, K.-A., Hiergeist, A., Wagner, A., et al. (2020). Oral Health, Oral Microbiota, and Incidence of Stroke-Associated Pneumonia-A Prospective Observational Study. Front. Neurol. 11:528056. doi: 10.3389/fneur.2020.528056
CLSI (2018a). M07: Methods For Dilution Antimicrobial Susceptibility Tests For Bacteria That Grow Aerobically,: 11th Edition. Pennsylvania, USA: Clinical and Laboratory Standards Institute.
CLSI (2018b). M11: Methods For Antimicrobial Susceptibility Testing Of Anaerobic Bacteria: 9th Edition. Pennsylvania, USA: Clinical and Laboratory Standards Institute.
Da Costa Rosa, T., de Almeida Neves, A., Azcarate-Peril, M. A., Divaris, K., Wu, D., Cho, H., et al. (2021). The bacterial microbiome and metabolome in caries progression and arrest. J. Oral Microbiol. 13:1886748. doi: 10.1080/20002297.2021.1886748
Davies, R. M., Jensen, S. B., Rindom Schiott, C., Löe, H., and Theilade, J. (1972). Anaerobic gliding bacteria isolated from the oral cavity. Acta. Pathol. Microbiol. Scand. B Microbiol. Immunol. 80, 397–402. doi: 10.1111/j.1699-0463.1972.tb00052.x
Diaz, P. I., Hoare, A., and Hong, B.-Y. (2016). Subgingival Microbiome Shifts and Community Dynamics in Periodontal Diseases. J. Calif. Dent. Assoc. 44, 421–435.
Edlund, A., Yang, Y., Hall, A. P., Guo, L., Lux, R., He, X., et al. (2013). An in vitro biofilm model system maintaining a highly reproducible species and metabolic diversity approaching that of the human oral microbiome. Microbiome 1:25. doi: 10.1186/2049-2618-1-25
Eick, S., Goltz, S., Nietzsche, S., Jentsch, H., and Pfister, W. (2011). Efficacy of chlorhexidine digluconate-containing formulations and other mouthrinses against periodontopathogenic microorganisms. Quintessence Int. 42, 687–700.
ElSalhy, M., Söderling, E., Honkala, E., Fontana, M., Flannagan, S., Kokaras, A., et al. (2016). Salivary microbiota and caries occurrence in Mutans Streptococci-positive school children. Eur. J. Paediatr. Dent. 17, 188–192.
Elworthy, A., Greenman, J., Doherty, F. M., Newcombe, R. G., and Addy, M. (1996). The substantivity of a number of oral hygiene products determined by the duration of effects on salivary bacteria. J. Periodontol. 67, 572–576. doi: 10.1902/jop.1996.67.6.572
Espíndola, L. C. P., Picão, R. C., Mançano, S. M. C. N., Martins do Souto, R., and Colombo, A. P. V. (2022). Prevalence and antimicrobial susceptibility of Gram-negative bacilli in subgingival biofilm associated with periodontal diseases. J. Periodontol. 93, 69–79. doi: 10.1002/JPER.20-0829
Exterkate, R. A. M., Crielaard, W., and Ten Cate, J. M. (2010). Different response to amine fluoride by Streptococcus mutans and polymicrobial biofilms in a novel high-throughput active attachment model. Caries Res. 44, 372–379. doi: 10.1159/000316541
Fernandez, Y., Mostajo, M., Exterkate, R. A. M., Buijs, M. J., Crielaard, W., and Zaura, E. (2017). Effect of mouthwashes on the composition and metabolic activity of oral biofilms grown in vitro. Clin. Oral Investig. 21, 1221–1230. doi: 10.1007/s00784-016-1876-2
Figuero, E., Herrera, D., Tobías, A., Serrano, J., Roldán, S., Escribano, M., et al. (2019). Efficacy of adjunctive anti-plaque chemical agents in managing gingivitis: a systematic review and network meta-analyses. J. Clin. Periodontol. 46, 723–739. doi: 10.1111/jcpe.13127
Gottsauner, M. J., Michaelides, I., Schmidt, B., Scholz, K. J., Buchalla, W., Widbiller, M., et al. (2020). A prospective clinical pilot study on the effects of a hydrogen peroxide mouthrinse on the intraoral viral load of SARS-CoV-2. Clin. Oral Investig. 24, 3707–3713. doi: 10.1007/s00784-020-03549-1
Griggs, D. J., Peake, L., Johnson, M. M., Ghori, S., Mott, A., and Piddock, L. J. V. (2009). Beta-lactamase-mediated beta-lactam resistance in Campylobacter species: prevalence of Cj0299 (bla OXA-61) and evidence for a novel beta-Lactamase in C. jejuni. Antimicrob. Agents Chemother. 53, 3357–3364. doi: 10.1128/AAC.01655-08
Grönbeck Lindén, I., Hägglin, C., Gahnberg, L., and Andersson, P. (2017). Factors Affecting Older Persons’ Ability to Manage Oral Hygiene: a Qualitative Study. JDR Clin. Trans. Res. 2, 223–232. doi: 10.1177/2380084417709267
Hannig, C., and Hannig, M. (2009). The oral cavity–a key system to understand substratum-dependent bioadhesion on solid surfaces in man. Clin. Oral Investig. 13, 123–139. doi: 10.1007/s00784-008-0243-3
Haps, S., Slot, D. E., Berchier, C. E., and van der Weijden, G. A. (2008). The effect of cetylpyridinium chloride-containing mouth rinses as adjuncts to toothbrushing on plaque and parameters of gingival inflammation: a systematic review. Int. J. Dent. Hyg. 6, 290–303. doi: 10.1111/j.1601-5037.2008.00344.x
Hiergeist, A., Reischl, U., and Gessner, A. (2016). Multicenter quality assessment of 16S ribosomal DNA-sequencing for microbiome analyses reveals high inter-center variability. Int. J. Med. Microbiol. 306, 334–342. doi: 10.1016/j.ijmm.2016.03.005
Hyde, E. R., Andrade, F., Vaksman, Z., Parthasarathy, K., Jiang, H., Parthasarathy, D. K., et al. (2014). Metagenomic analysis of nitrate-reducing bacteria in the oral cavity: implications for nitric oxide homeostasis. PLoS One 9:e88645. doi: 10.1371/journal.pone.0088645
Ito, R., Mustapha, M. M., Tomich, A. D., Callaghan, J. D., McElheny, C. L., Mettus, R. T., et al. (2017). Widespread Fosfomycin Resistance in Gram-Negative Bacteria Attributable to the Chromosomal fosA Gene. mBio 8, e749–e717. doi: 10.1128/mBio.00749-17
Jakubovics, N. S., Goodman, S. D., Mashburn-Warren, L., Stafford, G. P., and Cieplik, F. (2021). The dental plaque biofilm matrix. Periodontol. 2000 86, 32–56. doi: 10.1111/prd.12361
Jepsen, K., Falk, W., Brune, F., Cosgarea, R., Fimmers, R., Bekeredjian-Ding, I., et al. (2022). Prevalence and Antibiotic Susceptibility Trends of Selected Enterobacteriaceae, Enterococci, and Candida albicans in the Subgingival Microbiota of German Periodontitis Patients: a Retrospective Surveillance Study. Antibiotics 11:385. doi: 10.3390/antibiotics11030385
Jiang, S., Zeng, J., Zhou, X., and Li, Y. (2018). Drug Resistance and Gene Transfer Mechanisms in Respiratory/Oral Bacteria. J. Dent. Res. 97, 1092–1099. doi: 10.1177/0022034518782659
Jones, C. G. (1997). Chlorhexidine: is it still the gold standard? Periodontol. 2000 15, 55–62. doi: 10.1111/j.1600-0757.1997.tb00105.x
Kistler, J. O., Pesaro, M., and Wade, W. G. (2015). Development and pyrosequencing analysis of an in-vitro oral biofilm model. BMC Microbiol. 15:24. doi: 10.1186/s12866-015-0364-1
Kitagawa, H., Izutani, N., Kitagawa, R., Maezono, H., Yamaguchi, M., and Imazato, S. (2016). Evolution of resistance to cationic biocides in Streptococcus mutans and Enterococcus faecalis. J. Dent. 47, 18–22. doi: 10.1016/j.jdent.2016.02.008
Leadbetter, E. R., Holt, S. C., and Socransky, S. S. (1979). Capnocytophaga: new genus of gram-negative gliding bacteria. I. General characteristics, taxonomic considerations and significance. Arch. Microbiol. 122, 9–16. doi: 10.1007/BF00408040
Liu, X., Tong, X., Zhu, J., Tian, L., Jie, Z., Zou, Y., et al. (2021). Metagenome-genome-wide association studies reveal human genetic impact on the oral microbiome. Cell Discov. 7:117. doi: 10.1038/s41421-021-00356-0
Mao, X., Auer, D. L., Buchalla, W., Hiller, K.-A., Maisch, T., Hellwig, E., et al. (2020). Cetylpyridinium Chloride: mechanism of Action, Antimicrobial Efficacy in Biofilms, and Potential Risks of Resistance. Antimicrob. Agents Chemother. 64, e576–e520. doi: 10.1128/AAC.00576-20
Mason, M. R., Nagaraja, H. N., Camerlengo, T., Joshi, V., and Kumar, P. S. (2013). Deep sequencing identifies ethnicity-specific bacterial signatures in the oral microbiome. PLoS One 8:e77287. doi: 10.1371/journal.pone.0077287
Meini, S., Tascini, C., Cei, M., Sozio, E., and Rossolini, G. M. (2019). AmpC β-lactamase-producing Enterobacterales: what a clinician should know. Infection 47, 363–375. doi: 10.1007/s15010-019-01291-9
Meister, T. L., Gottsauner, J.-M., Schmidt, B., Heinen, N., Todt, D., Audebert, F., et al. (2022). Mouthrinses against SARS-CoV-2 - High antiviral effectivity by membrane disruption in vitro translates to mild effects in a randomized placebo-controlled clinical trial. Virus Res. 316:198791. doi: 10.1016/j.virusres.2022.198791
Muehler, D., Mao, X., Czemmel, S., Geißert, J., Engesser, C., Hiller, K.-A., et al. (2022). Transcriptomic Stress Response in Streptococcus mutans following Treatment with a Sublethal Concentration of Chlorhexidine Digluconate. Microorganisms 10:561. doi: 10.3390/microorganisms10030561
Navazesh, M., and Christensen, C. M. (1982). A comparison of whole mouth resting and stimulated salivary measurement procedures. J. Dent. Res. 61, 1158–1162. doi: 10.1177/00220345820610100901
Nouioui, I., Carro, L., García-López, M., Meier-Kolthoff, J. P., Woyke, T., Kyrpides, N. C., et al. (2018). Genome-Based Taxonomic Classification of the Phylum Actinobacteria. Front. Microbiol. 9:2007. doi: 10.3389/fmicb.2018.02007
Nowicki, E. M., Shroff, R., Singleton, J. A., Renaud, D. E., Wallace, D., Drury, J., et al. (2018). Microbiota and Metatranscriptome Changes Accompanying the Onset of Gingivitis. mBio 9, e575–e518. doi: 10.1128/mBio.00575-18
Pithon, M. M., Sant’Anna, L. I. D. A., Baião, F. C. S., dos Santos, R. L., Da Coqueiro, R. S., and Maia, L. C. (2015). Assessment of the effectiveness of mouthwashes in reducing cariogenic biofilm in orthodontic patients: a systematic review. J. Dent. 43, 297–308. doi: 10.1016/j.jdent.2014.12.010
Pratten, J., Wills, K., Barnett, P., and Wilson, M. (1998). In vitro studies of the effect of antiseptic-containing mouthwashes on the formation and viability of Streptococcus sanguis biofilms. J. Appl. Microbiol. 84, 1149–1155. doi: 10.1046/j.1365-2672.1998.00462.x
Premaraj, T. S., Vella, R., Chung, J., Lin, Q., Panier, H., Underwood, K., et al. (2020). Ethnic variation of oral microbiota in children. Sci. Rep. 10:14788. doi: 10.1038/s41598-020-71422-y
Rosier, B. T., Takahashi, N., Zaura, E., Krom, B. P., MartÍnez-Espinosa, R. M., van Breda, S. G. J., et al. (2022). The Importance of Nitrate Reduction for Oral Health. J. Dent. Res. 23:220345221080982. doi: 10.1177/00220345221080982
Sanz, M., Serrano, J., Iniesta, M., Santa Cruz, I., and Herrera, D. (2013). Antiplaque and antigingivitis toothpastes. Monogr. Oral Sci. 23, 27–44. doi: 10.1159/000350465
Schwarz, S. R., Hirsch, S., Hiergeist, A., Kirschneck, C., Muehler, D., Hiller, K.-A., et al. (2021). Limited antimicrobial efficacy of oral care antiseptics in microcosm biofilms and phenotypic adaptation of bacteria upon repeated exposure. Clin. Oral Investig. 25, 2939–2950. doi: 10.1007/s00784-020-03613-w
Shani, S., Friedman, M., and Steinberg, D. (2000). The anticariogenic effect of amine fluorides on Streptococcus sobrinus and glucosyltransferase in biofilms. Caries Res. 34, 260–267. doi: 10.1159/000016600
So Yeon, L., and Si Young, L. (2019). Susceptibility of Oral Streptococci to Chlorhexidine and Cetylpyridinium Chloride. Biocontrol. Sci. 24, 13–21. doi: 10.4265/bio.24.13
Solderer, A., Kaufmann, M., Hofer, D., Wiedemeier, D., Attin, T., and Schmidlin, P. R. (2019). Efficacy of chlorhexidine rinses after periodontal or implant surgery: a systematic review. Clin. Oral Investig. 23, 21–32. doi: 10.1007/s00784-018-2761-y
Sproston, E. L., Wimalarathna, H. M. L., and Sheppard, S. K. (2018). Trends in fluoroquinolone resistance in Campylobacter. Microb. Genom. 4:e000198. doi: 10.1099/mgen.0.000198
Stanley, A., Wilson, M., and Newman, H. N. (1989). The in vitro effects of chlorhexidine on subgingival plaque bacteria. J. Clin. Periodontol. 16, 259–264. doi: 10.1111/j.1600-051x.1989.tb01651.x
Teng, L.-J., Hsueh, P.-R., Tsai, J.-C., Liaw, S.-J., Ho, S.-W., and Luh, K.-T. (2002). High incidence of cefoxitin and clindamycin resistance among anaerobes in Taiwan. Antimicrob. Agents Chemother. 46, 2908–2913. doi: 10.1128/AAC.46.9.2908-2913.2002
Tribble, G. D., Angelov, N., Weltman, R., Wang, B.-Y., Eswaran, S. V., Gay, I. C., et al. (2019). Frequency of Tongue Cleaning Impacts the Human Tongue Microbiome Composition and Enterosalivary Circulation of Nitrate. Front. Cell Infect. Microbiol. 9:39. doi: 10.3389/fcimb.2019.00039
van der Weijden, F. A., van der Sluijs, E., Ciancio, S. G., and Slot, D. E. (2015). Can Chemical Mouthwash Agents Achieve Plaque/Gingivitis Control? Dent. Clin. North. Am. 59, 799–829. doi: 10.1016/j.cden.2015.06.002
Vanbaelen, T., van Dijck, C., Laumen, J., Gonzalez, N., De Baetselier, I., Manoharan-Basil, S. S., et al. (2022). Global epidemiology of antimicrobial resistance in commensal Neisseria species: a systematic review. Int. J. Med. Microbiol. 312:151551. doi: 10.1016/j.ijmm.2022.151551
Verspecht, T., Rodriguez Herrero, E., Khodaparast, L., Khodaparast, L., Boon, N., Bernaerts, K., et al. (2019). Development of antiseptic adaptation and cross-adapatation in selected oral pathogens in vitro. Sci. Rep. 9:8326. doi: 10.1038/s41598-019-44822-y
Wade, W. G. (2021). Resilience of the oral microbiome. Periodontol. 2000 86, 113–122. doi: 10.1111/prd.12365
Wade, W. G., and Addy, M. (1989). In vitro activity of a chlorhexidine-containing mouthwash against subgingival bacteria. J. Periodontol. 60, 521–525. doi: 10.1902/jop.1989.60.9.521
Waldron, C., Nunn, J., Mac Giolla Phadraig, C., Comiskey, C., Guerin, S., van Harten, M. T., et al. (2019). Oral hygiene interventions for people with intellectual disabilities. Cochrane Database Syst. Rev. 5, CD012628. doi: 10.1002/14651858.CD012628.pub2
Wand, M. E., Bock, L. J., Bonney, L. C., and Sutton, J. M. (2017). Mechanisms of Increased Resistance to Chlorhexidine and Cross-Resistance to Colistin following Exposure of Klebsiella pneumoniae Clinical Isolates to Chlorhexidine. Antimicrob. Agents Chemother. 61, e1162–e1116. doi: 10.1128/AAC.01162-16
Wieczorek, K., and Osek, J. (2013). Antimicrobial resistance mechanisms among Campylobacter. Biomed. Res. Int. 2013:340605. doi: 10.1155/2013/340605
Zaura-Arite, E., van Marle, J., and ten Cate, J. M. (2001). Conofocal microscopy study of undisturbed and chlorhexidine-treated dental biofilm. J. Dent. Res. 80, 1436–1440. doi: 10.1177/00220345010800051001
Zayed, N., Boon, N., Bernaerts, K., Chatzigiannidou, I., van Holm, W., Verspecht, T., et al. (2022). Differences in chlorhexidine mouthrinses formulations influence the quantitative and qualitative changes in in-vitro oral biofilms. J. Periodontal. Res. 57, 52–62. doi: 10.1111/jre.12937
Keywords: chlorhexidine, cetylpyridinium chloride, antiseptic, biofilm, resistance, biocide, antibiotic, dysbiosis
Citation: Mao X, Hiergeist A, Auer DL, Scholz KJ, Muehler D, Hiller K-A, Maisch T, Buchalla W, Hellwig E, Gessner A, Al-Ahmad A and Cieplik F (2022) Ecological Effects of Daily Antiseptic Treatment on Microbial Composition of Saliva-Grown Microcosm Biofilms and Selection of Resistant Phenotypes. Front. Microbiol. 13:934525. doi: 10.3389/fmicb.2022.934525
Received: 02 May 2022; Accepted: 25 May 2022;
Published: 30 June 2022.
Edited by:
Rustam Aminov, University of Aberdeen, United KingdomCopyright © 2022 Mao, Hiergeist, Auer, Scholz, Muehler, Hiller, Maisch, Buchalla, Hellwig, Gessner, Al-Ahmad and Cieplik. This is an open-access article distributed under the terms of the Creative Commons Attribution License (CC BY). The use, distribution or reproduction in other forums is permitted, provided the original author(s) and the copyright owner(s) are credited and that the original publication in this journal is cited, in accordance with accepted academic practice. No use, distribution or reproduction is permitted which does not comply with these terms.
*Correspondence: Fabian Cieplik, fabian.cieplik@ukr.de
†These authors have contributed equally to this work and share first authorship