- 1State Key Laboratory of North China Crop Improvement and Regulation, College of Life Sciences, Hebei Agricultural University, Baoding, China
- 2Center for Advanced Measurement Science, National Institute of Metrology, Beijing, China
- 3Biotechnology Research Institute, Chinese Academy of Agricultural Sciences, Beijing, China
- 4Excellence and Innovation Center, Jiangsu Academy of Agricultural Sciences, Nanjing, China
- 5Department of Botany and Applied Microbiology, Faculty of Science, Mansoura University, Mansoura, Egypt
Viral coat protein (CP) has numerous critical functions in plant infection, but little is known about p25, the CP of maize chlorotic mottle virus (MCMV; Machlomovirus), which causes severe yield losses in maize worldwide. Here, we investigated the roles of p25 in pathogenicity and systemic movement, as well as potential interactions with host plants, using a hybrid tobacco mosaic virus (TMV)-based expression system. Highly conserved protein p25 is predicted to contain a membrane-anchored nuclear localization signal (NLS) sequence and an extracellular sequence. In transgenic Nicotiana benthamiana plants containing the movement protein (MP) of TMV (TMV-MP), p25 induced severe symptoms, including dwarf and foliar necrosis, and was detected in inoculated and non-inoculated leaves. After the deletion of NLS from nuclear-located p25, the protein was found throughout the host cell, and plant stunting and starch granule deformity were reduced. Systemic movement and pathogenicity were significantly impaired when the C-terminal regions of p25 were absent. Using virus-induced gene silencing (VIGS), the transcript level of heat shock protein HSP90 was distinctly lower in host plants in association with the absence of leaf necrosis induced by TMV-p25. Our results revealed crucial roles for MCMV p25 in viral pathogenicity, long-distance movement, and interactions with N. benthamiana.
Introduction
Maize chlorotic mottle virus (MCMV; genus Machlomovirus, family Tombusviridae), a positive-sense, single-stranded RNA maize virus (Nutter et al., 1989), is an exclusive pathogen of maize around the world. First reported in Peru in 1974, then in the United States in 1976, this virus is now widespread (Niblett and Claflin, 1978; Lommel et al., 1991; Mahuku et al., 2015). It was first detected in the Yunnan province of China in 2009 (Xie et al., 2011). The only natural host of MCMV is maize, and its host range is limited to several gramineous plants. Transmitted primarily by seed, corn residues, thrips, and leaf beetles, the virus is listed for import inspection and quarantine because of the tremendous threat it poses to maize-based food security worldwide (Bockelman, 1982; Jensen, 1991; Cabanas et al., 2013; Wang et al., 2014).
In general, MCMV infection can cause slight chlorosis and mottling, yellow striping, and dwarfing. It causes severe corn lethal necrosis disease and serious yield losses when present in maize in a mixed infection with various maize-infecting potyviruses and even in single infection (Goldberg and Brakke, 1987; Wamaitha et al., 2018; Mwatuni et al., 2020). Synergistic interactions with wheat streak mosaic virus, maize dwarf mosaic virus, or sugarcane mosaic virus can result in 3.3–11.2-fold higher levels of virions (Scheets, 1998; Adams et al., 2013; Xia et al., 2016). The economic losses for maize in a heavily affected region can reach up to 100% (Rao et al., 2010; Hilker et al., 2017).
The icosahedral virion of MCMV is 30 nm in diameter with 180 copies of chemically identical coat protein (CP). The viral genomic RNA (gRNA, 4,436 or 4,437 nt) produces two sub-genomic RNA (sgRNA) in host cells, has a capped structure at the 5′ end and lacks a poly(A) tail at the 3′ end. It comprises seven open reading frames (ORFs), encoding p7a, p7b, p25, p32, p50, p111, and p31 (Nutter et al., 1989; Scheets, 2000; Stenger and French, 2008). Protein p32 is directly translated from the gRNA, although the start codon sequences are not the optimal sequences for plant ribosomes. P50 is located downstream of p32 in the genome but is only 19 bases away from the start codon of p32. The termination codon of p50 is read through to synthesize p111. Proteins p7a, p7b, p31, and p25 are synthesized by the generated sgRNA (Nutter et al., 1989; Lommel et al., 1991). Among the encoded proteins, p50 and p111 function in MCMV replication in plant protoplasts. The absence of p32 results in a two-thirds decrease of transcriptional capacity compared with the wild type; p31 participates in viral systemic infection of the plant host. Coat protein p25 cooperates with two movement proteins (MPs), p7a and p7b, in the intercellular movement of the virus in the host (Scheets, 2016).
Increasing evidence has demonstrated that the CP of plant viruses has vital multifunctional roles in viral RNA-binding activity, systemic movement, host symptom formation, gene silencing suppression, and induction of the host hypersensitive response (Azevedo et al., 2010; Park et al., 2013; Kang et al., 2015; Rehman et al., 2019). The CP of another member of the family Tombusviridae, olive latent virus 1 (OLV-1), loses the ability to systemically infect if the 49 amino acids (aa) of the C-terminal region are replaced with 39 aa (Pantaleo et al., 2006). The N-terminal region of the CP of the beet black scorch virus is essential for nuclear localization, binding of viral RNA, virion assembly, and systemic infection (Zhang et al., 2011, 2013). The CP of turnip crinkle virus competitively combines with AGO1 in plant cells and reduces the ability of AGO to bind siRNA and miRNA, leading to a decrease in defensive RNA silencing by the host, thus promoting virus infection (Jin and Zhu, 2010).
Although the functions of other MCMV proteins have been studied intensively and significant functional diversity has been shown for MCMV CP, p25 has not been characterized in depth. Thus, our main objectives were to (1) perform bioinformatic analysis of p25; (2) identify the function of p25 in symptom development; (3) analyze the nuclear localization signal (NLS) of p25 and its role in viral pathogenicity; (4) explore the C domain of p25 in viral movement; and (5) study the host factors participated in TMV-p25 infection.
Materials and methods
In silico analysis of the maize chlorotic mottle virus p25 protein
The nucleic acid and aa sequences of MCMV Yunnan2 p25 were downloaded from the NCBI database1 (JQ943666.1; AFM31229.1). The nucleic acid sequences of MCMV p25 isolated in different parts of the world were also obtained from the NCBI non-redundant database. The NLS of the MCMV p25 protein was predicted by PredictNLS2. Transmembrane helices were analyzed with TMHMM-2.03. The putative three-dimensional structure of p25 was analyzed using the SWISS-MODEL tool4. A phylogenetic tree was constructed by MEGA5.22 software with the neighbor-joining method (Su et al., 2018).
Construction of plasmids
The MCMV p25 gene was cloned from an MCMV infectious clone vector using PCR and primer pair tobacco mosaic virus (TMV)-p25F/TMV-p25R. The amplified fragments were digested with restriction enzymes EcoR V and Xho I and inserted into the EcoR V/Xho I-digested vector pTMVcpGFP to generate pTMV-p25 (Lewandowski and Adkins, 2005). Deletion mutant HA tag-infused plasmids pTMV-p25N, pTMV-p25C1, pTMV-p25C2, pTMV-p25-HA, pTMV-p25N-HA, and pTMV-p32 were constructed with the same strategy.
For subcellular localization assays in Nicotiana benthamiana, the coding sequences of P25/P25N were amplified and inserted into Xba I/BamH I sites in pYBA1152, thus forming pP25-GFP and pP25N-GFP, respectively.
To construct TRV-virus-induced gene silencing (VIGS) vectors, the partial coding sequence of NbNPR1, NbCOI1, or NbHSP90 was ligated into EcoR I/Sma I-treated pTRV2 to generate the recombinant plasmids pTRV2-NPR1, pTRV2-COI1, and pTRV2-HSP90, respectively.
The primers are listed in Supplementary Table 1.
Plant growth conditions and inoculation
Wild type and TMV MP-transgenic N. benthamiana plants were grown in growth chambers (25°C day/23°C night, 16 h light/8 h dark). At the 4–6-leaf stage, leaves of the TMV MP-transgenic N. benthamiana plants were rub-inoculated with infectious RNA, as previously described (Dawson et al., 1986). TRV VIGS analysis was performed as described previously (Tang et al., 2010). The brief steps are as follows: The Agrobacterium GV3101 harboring pTRV1, pTRV2, and their derivatives were cultivated overnight and resuspended in agroinfiltration buffer (10 mm MES, 10 mm MgCl2 and 100 μM acetosyringone). After incubation at 28°C for 3–5 h, the Agrobacterium harboring TRV RNA1 and RNA2 (their derivative) were mixed at 1:1 ratio and infiltrated into N. benthamiana leaves. All the experiment was repeated three times, six plants per treatment were used for every experiment. Confocal laser scanning microscopy and transmission electron microscopy analysis.
Agroinfiltrated leaf tissues were imaged using an LSM700 confocal laser scanning microscope (Zeiss, Oberkochen, Germany). GFP was excited at 488 nm, and the emissions were captured between 497 and 510 nm. The dsRed signal was excited at 557 nm, and emissions were captured from 566 to 600 nm. Images were captured digitally and analyzed using ZEN 2010 software (Zeiss, Oberkochen, Germany).
Inoculated leaves were harvested and cut into 1 mm × 1 mm sections and then fixed in 2.5% v/v glutaraldehyde and 1% w/v osmium tetroxide sequentially. The fixed tissues were dehydrated using an ethanol series and embedded in Lowicryl K4M resin as instructed by the manufacturer (Electron Microscopy Sciences, Fort Washington, PA, United States). Ultrathin sections were cut and examined using a Hitachi-7650 TEM (Tokyo, Japan).
RT-polymerase chain reaction and RT-qPCR
Total RNA from N. benthamiana plants was extracted using the RNeasy Plant Mini Kit (Qiagen, Valencia, CA, United States). Immediately, RNA was equalized, and 1 μg was used in each 20 μL reverse transcription reaction using the PrimeScript™ RT Reagent Kit (TaKaRa, Kusatsu, Japan) as instructed by the manufacturer. A total of 500 ng of each cDNA sample served as a template in the polymerase chain reaction (PCR) assay using specific primers. As a control for equal loading, primers specific to the endogenous gene Nbactin were used. qPCR was performed using a 2 × SYBR Premix Ex Taq™ (TaKaRa, Kusatsu, Japan) following the manufacturer’s instructions. Each construct was evaluated in three independent experiments. The primers used in RT-PCR are listed in Supplementary Table 1.
Protein extraction and western blot
Protein extraction and western blotting were carried out as described previously (Zhan et al., 2021). Approximately 0.1 g of leaf tissue from the inoculated plants was ground in 0.3 mL protein extraction buffer (6 M urea, 1 mm EDTA, 50 mm, 124 Tris-HCl, 1% SDS, pH 7.5). The crude extracts were boiled for 5 min, then centrifuged at 12,000 × g for 10 min, and the resulting supernatant (12 μL per sample plus 3 μL 5 × loading buffer) was boiled for 5 min, then chilled on ice for 5 min. Protein samples were separated in 12.5% SDS-PAGE gels and transferred to nitrocellulose membranes. The membranes were then probed with the MCMV-p25, HA or GFP monoclonal antibody, followed by a secondary antibody conjugated to AP (Abcam, Cambridge, MA, United States). The experiment was repeated three times.
Results
Bioinformatic analysis of p25
As shown in Figure 1A, the CP of MCMV, p25, is a 236 aa protein and contains the viral coat domain (20–204). According to the cNLS Mapper prediction analysis, position 6–38 is a putative NLS sequences. In the C-terminal of p25, aa 198–213 and 214–236 were predicted to be the membrane-anchored sequence and the extracellular sequence, respectively. The structural model analysis showed that p25 consists of 8 alpha helices and 11 beta folds (Figure 1B). In the phylogenetic analysis, to better understand the relationships among the nucleotide sequences of MCMV CP worldwide (Figure 1C), MCMV CP clustered into two distinct branches, which was further divided into three sub-branches. In China, where MCMV is mainly distributed in Yunnan Province, the sequences had high identity with that of the Thailand isolate in this study. Thus, MCMV are highly conserved worldwide.
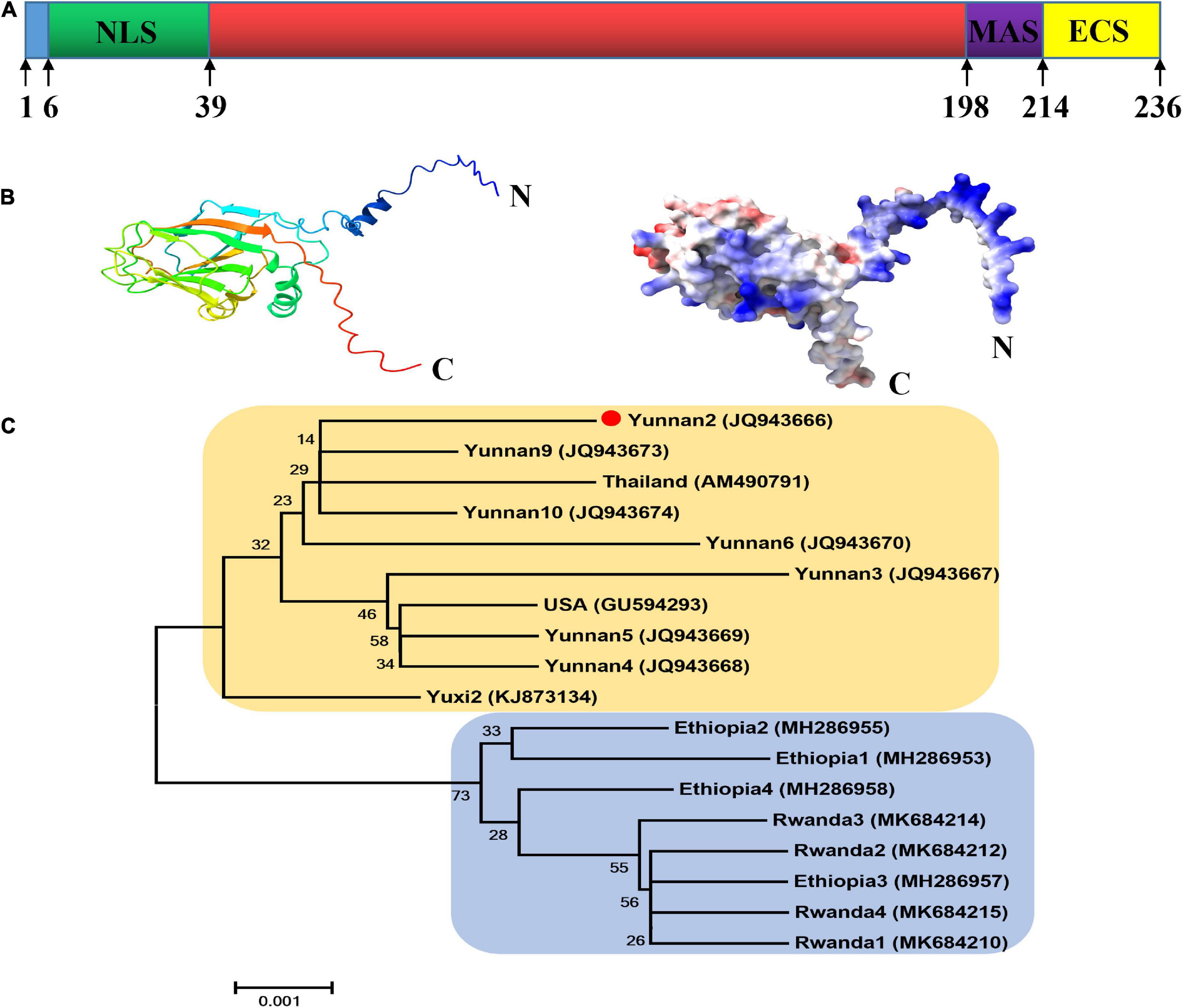
Figure 1. Bioinformatic analysis of the p25 of maize chlorotic mottle virus (MCMV). (A) Domain arrangement of p25. NLS, nuclear localization sequence; MAS, membrane-anchored sequence; ECS, extracellular sequence. Special function domains are represented with different colors. (B) Structural model analysis using SWISS-MODEL software. (C) Phylogenetic tree of CP nucleotide sequences in MCMV. The phylogenetic tree was constructed with MEGA5.22 software using the neighbor-joining method.
P25 contributes to viral pathogenicity and tobacco mosaic virus hybrid systemic movement
The ORF of p25 was introduced into the pTMVcp-GFP plasmid to generate the hybrid virus TMV-p25. Then, the RNA transcripts of TMV-p25 were used to inoculate TMV MP-transgenic N. benthamiana plants, and plants were inoculated with TMVcpGFP as a negative control (Zhang et al., 2012). Thereafter, the symptoms were observed and the transcript and expression level of p25 were further detected (Figure 2). The inoculated plants displayed severe symptoms (Figure 2A). The infected plants were severely stunted to half the height of the TMVcpGFP inoculated plants (Supplementary Figure 1). The inoculated leaves exhibited typical chlorosis and necrosis. Similarly, leaves that were systemically infected (leaves that were not inoculated; hereafter, systemic leaves) also had strong symptoms induced by this chimera virus.
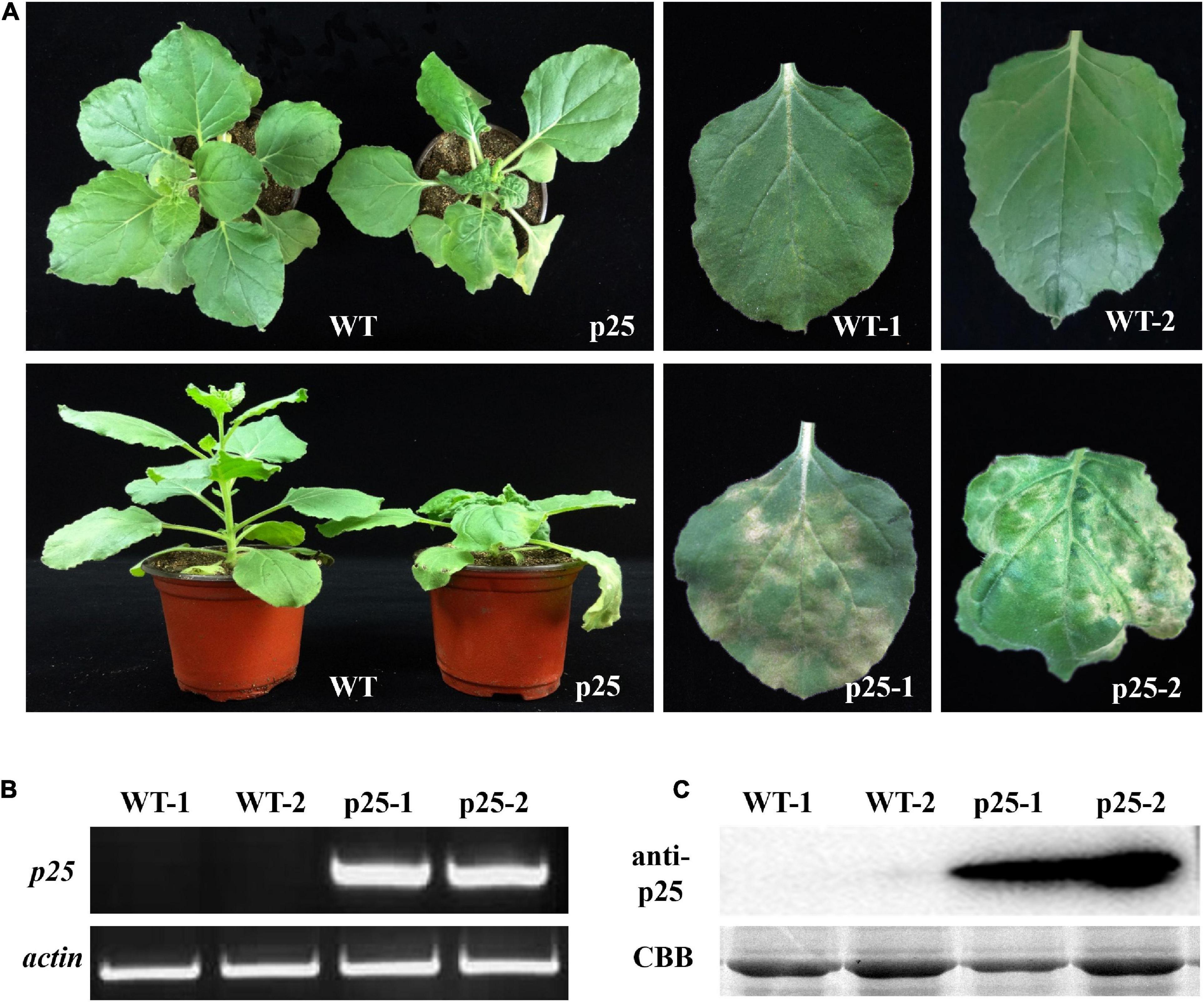
Figure 2. Maize chlorotic mottle virus (MCMV) p25 is required for viral pathogenicity and systemic movement in TMV MP-transgenic plants of Nicotiana benthamiana. (A) Phenotypes of transgenic plants after inoculation with TMV-p25 and TMVcp-GFP (WT) at 15 days post-inoculation. (B) RT-PCR analysis of RNA from leaves of plants after the two treatments. Actin from N. benthamiana was used as an internal standard. (C) Western blot detection of p25 from the leaves of plants in the two treatments. An anti-p25 antibody was used. Coomassie brilliant blue-stained Rubisco protein (CBB) was used as a total protein loading control. WT, TMV MP-transgenic plants inoculated with TMVcp-GFP; p25, TMV MP-transgenic plants inoculated with TMV-p25.1, inoculated leaves (WT or p25); 2, non-inoculated leaves on the same plant.
To confirm that symptoms were induced by the presence of p25, we used RT-PCR and western blot analysis to detect the p25 transcript and protein levels, respectively (Figures 2B,C). Intense bands for p25 appeared for inoculated and systemic leaves but not for the TMVcpGFP-inoculated leaves. These results indicate that p25 is required for viral pathogenicity and systemic movement in N. benthamiana.
Maize chlorotic mottle virus p25 is located in the nucleus
A stretch of basic residues in the N-terminal region of MCMV p25 resembled those of NLS. Leaves of N. benthamiana were examined with confocal laser scanning microscopy after the ORF of p25, and p25 lacking the NLS (p25N) were inserted into vector pYBA1152 to transiently express an eGFP-fused protein, driven by the 35S promoter (Figure 3A). As shown in Figure 3B, RFP-H2B was detected in the nucleus, and eGFP was detected in the nucleus and cytoplasm. As expected, p25-eGFP was uniquely located in the nucleus, whereas p25N-eGFP was found in the nucleus and cytoplasm, which is consistent with free eGFP localization. The use of p25-eGFP and p25N-eGFP with RFP-H2B/eGFP shows that p25 is located in the nucleus, and the NLS of p25 is needed for transport and restriction to the nucleus. Moreover, the western blot result indicates that the expressions of eGFP, p25-eGFP and p25N-eGFP proteins were correct and stabilized in N. benthamiana leaves (Figure 3C).
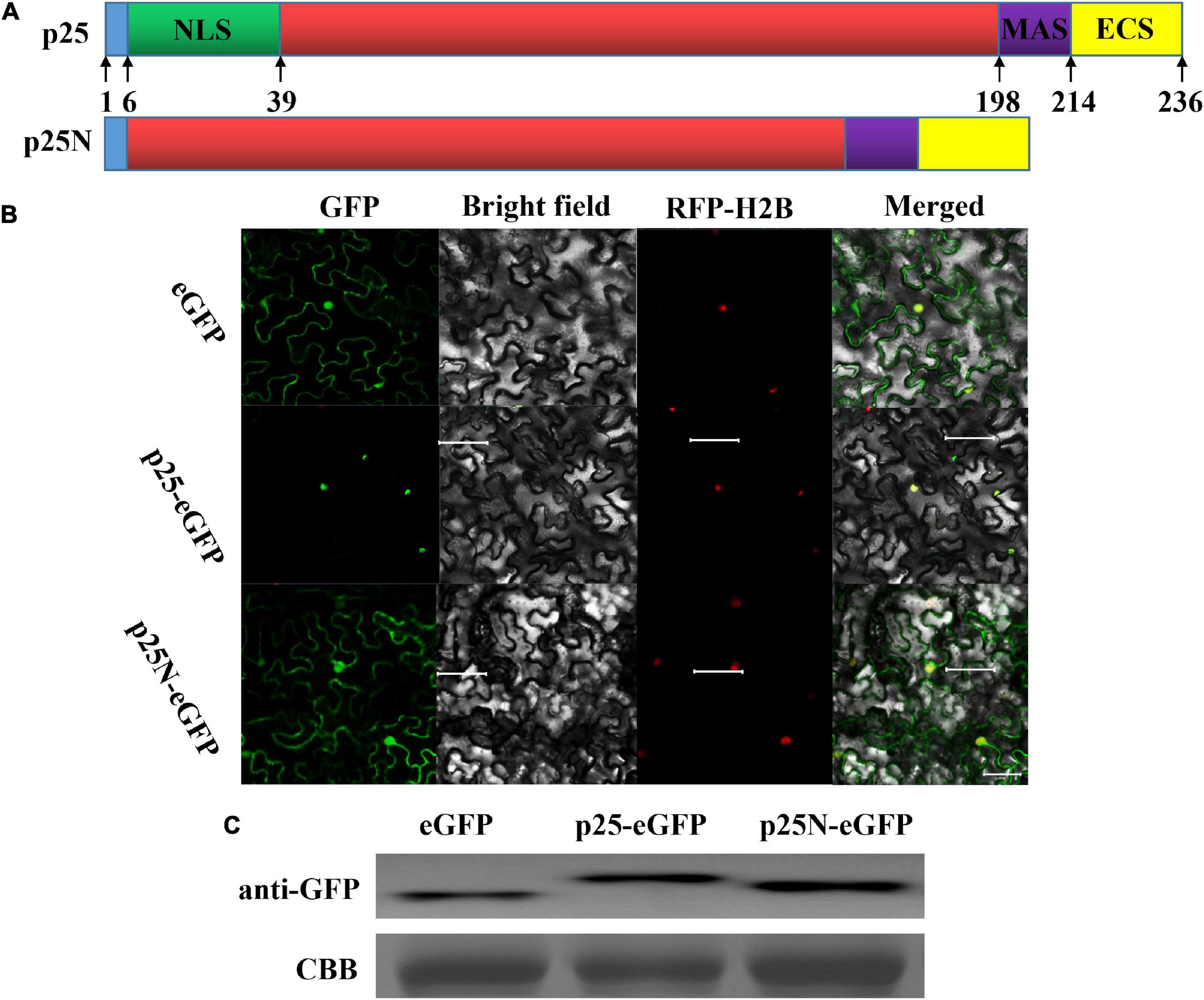
Figure 3. Subcellular location of p25. (A) Schematic sketch of the construction strategies of p25 and p25N. p25, the whole open reading frame (ORF) of p25; p25N, the truncated sequence lacking the nuclear localization signal (NLS) (in green) of p25. (B) Confocal laser scanning micrographs of the location of p25 in Nicotiana benthamiana. The co-localization signal of RFP-H2B is shown as a positive nuclear control, and eGFP was used as a control located in the nucleus and cytoplasm. Scale bars: 50 μm. (C) Western blot of eGFP, p25-eGFP, and p25N-eGFP using anti-GFP antibody. Coomassie brilliant blue-stained Rubisco protein (blue bands) was used as a total protein loading control.
The nuclear localization signal of p25 is required for pathogenicity
To further determine whether the NLS of p25 is involved in pathogenicity, TMV MP-transgenic plants were inoculated with a hybrid virus, TMV-p25 or TMV-p25N, then examined for symptoms and analyzed for p25 transcript and protein levels (Figure 4). In Figure 4A, although the plants inoculated with TMV-p25N had viral symptoms, the plants were distinctly taller than those inoculated with TMV-p25 (Supplementary Figure 1). As viewed by transmission electron microscopy observation, starch grains in the chloroplasts of TMV-p25 inoculated leaves were significantly larger than in those inoculated with TMV-p25N (Figure 4B). However, the transcription and expression levels of p25 and p25N were not affected between TMV-p25 and TMV-p25N in leaves (Figures 4C,D). These results suggest that the NLS of p25 is important for full pathogenicity.
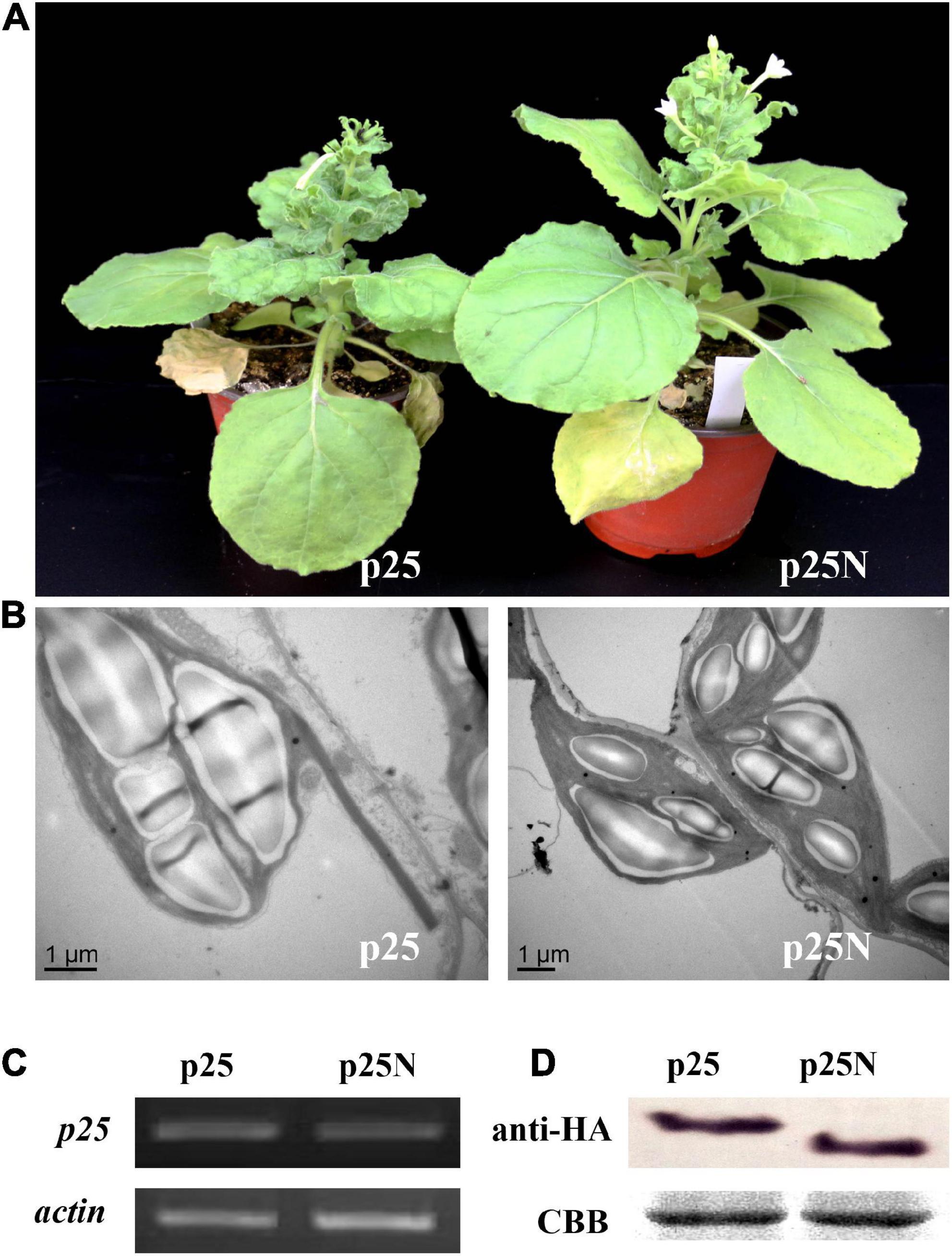
Figure 4. Pathogenicity analysis of the nuclear localization signal (NLS) in p25 using Nicotiana benthamiana plants. p25, plants inoculated with hybrid virus TMV-p25; p25N, plants inoculated with hybrid virus TMV-p25N lacking NLS. (A) Phenotype of plants inoculated with either the hybrid virus TMV-p25 or TMV-p25N. (B) Transmission electron micrographs of infected leaves. Scale bars: 1 μm. (C) Transcripts were detected by RT-PCR using extracted leaf RNA that was reverse transcribed. The actin of N. benthamiana was used as the internal control. (D) Western blot of p25 using an anti-HA antibody. Coomassie brilliant blue-stained Rubisco protein (blue bands) is shown as a total protein loading control.
Deletion of the C-terminal region of p25 impairs viral systemic movement and pathogenicity
To study the relevance of the C-terminal region of p25 in viral systemic movement and pathogenicity, we deleted the key domain and used the resultant constructs to inoculate TMV MP-transgenic N. benthamiana plants (Figure 5). As shown in Figure 5A, we deleted the 198–236 or 214–236 domain in the C-terminal region of p25 and inserted the respective constructs into the pTMVcpGFP plasmid to generate hybrid viruses TMV-p25C1 and TMV-p25C2. Plants inoculated with TMV-p25C1 developed mild symptoms and were taller than those inoculated with TMV-p25 or TMV-p25C2 (Supplementary Figure 1); plants inoculated with TMV-p25 or TMV-p25C2 exhibited very similar symptoms (Figure 5B). Similarly, p25 and p25C2 mRNA transcripts and protein were detected in systemic leaves but not in TMV-p25C1 inoculated leaves, which were defective for system infection (Figures 5C,D). These data demonstrate that the 198–236 residues in the C-terminal of p25 are not only involved in viral systemic movement but are also required for pathogenicity.
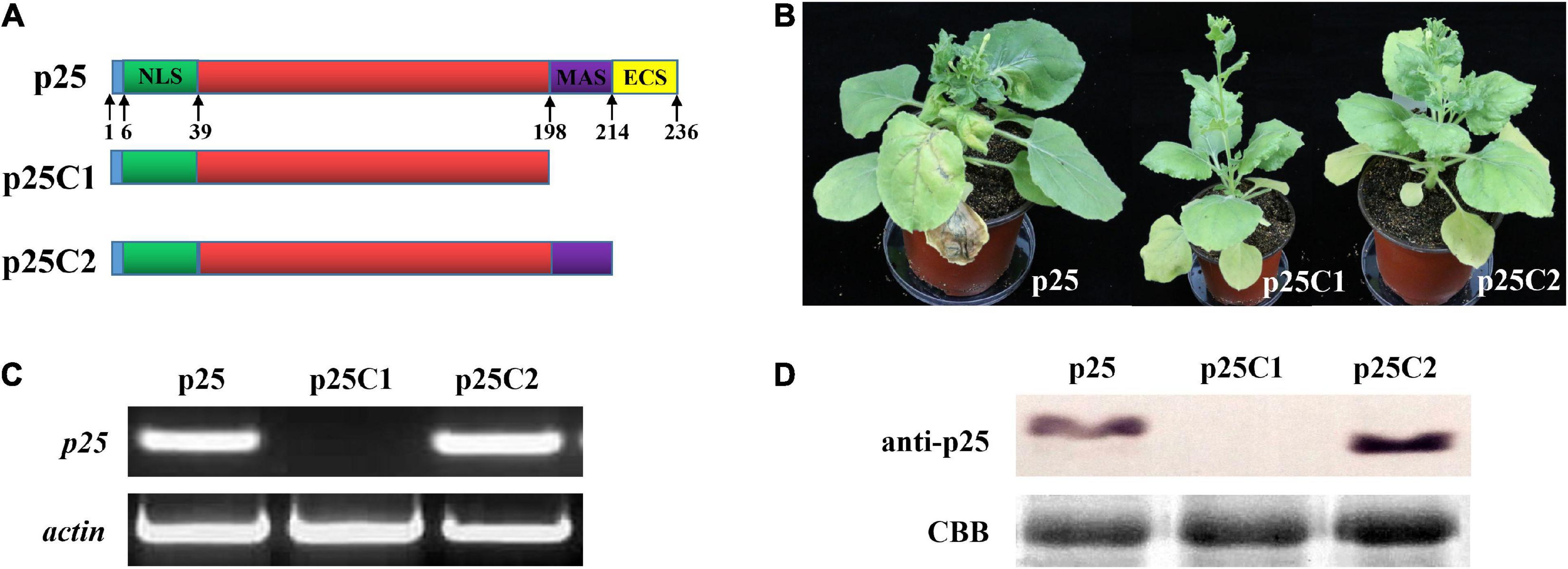
Figure 5. Functional analysis of the C-terminal region of p25. (A) The fused protein constructs. p25, plant inoculated with hybrid virus TMV-p25;. p25C1, plant inoculated with hybrid virus TMV-p25C1; p25C2, plant inoculated with hybrid virus TMV-p25C2. (B) Phenotype of Nicotiana benthamiana plants after inoculation with hybrid virus TMV-p25, TMV-p25C1, or TMV-p25C2. (C) The transcriptional level was detected by RT-PCR using extracted leaf RNA after reverse transcription. N. benthamiana actin was used as the internal control. (D) Western blot of p25 using anti-p25 antibody. Coomassie brilliant blue-stained Rubisco protein (blue bands) was used as a total protein loading control.
Nicotiana benthamiana HSP90 involved in p25 mediated viral pathogenicity
Previous studies have shown that host resistance genes participate in the interactions between viruses and their host plants (Liu et al., 2004; Tran et al., 2016; Zhao et al., 2019). Thus, we selected resistance genes COI1, NPR1, and HSP90 as potential candidates interacting with p25 in TMV MP-transgenic N. benthamiana plants after inoculation with hybrid virus TMV-p25 (Figure 6). The leaves with silenced COI1 and NPR1 were similar to the control, whereas silenced HSP90 resulted in tissue chlorosis rather than necrosis (Figure 6A). RT-qPCR analysis revealed that COI1, NPR1, and HSP90 were significantly downregulated by the TRV-VIGS system compared with the levels in the control (Figure 6B). Western blots showed typical p25 levels after all treatments (Figure 6C). These results suggest that host HSP90 is required for the p25 mediated viral pathogenicity and host resistance.
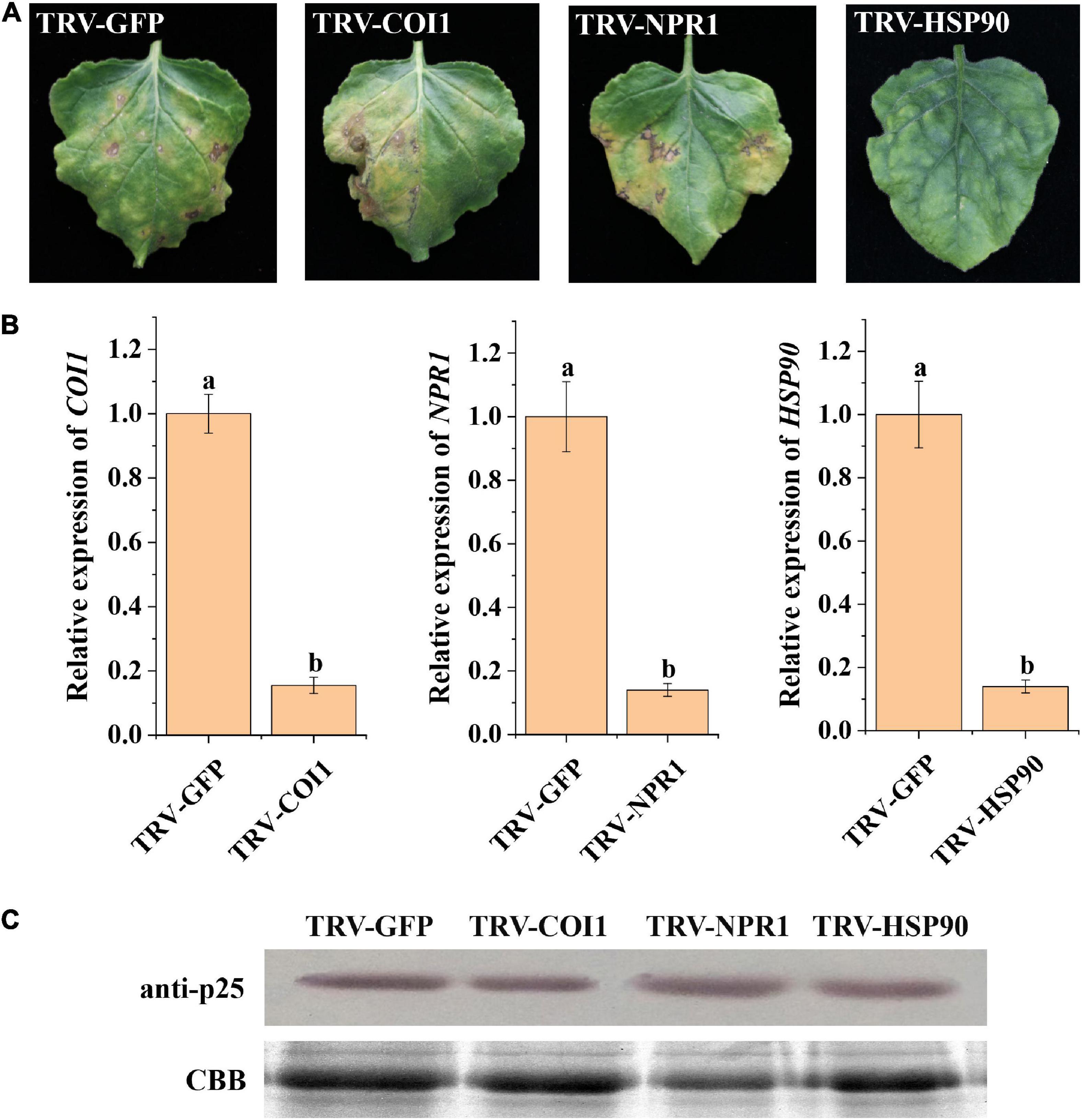
Figure 6. Analysis of plant resistance genes involved in TMV-p25 infection. (A) Leaves were infiltrated with Agrobacterium-containing pTRV1 or pTRV2-NbNPR1/NbCOI1/NbHSP90 plasmids. pTRV2-GFP was used as a control, and then plants were inoculated with hybrid virus TMV-p25. (B) RT-qPCR analysis of Nicotiana benthamiana leaves after VIGS. Error bars represent standard deviations. Different letters above the bars for each treatment indicate a significant difference (Student’s t-test, p < 0.05). (C) Western blot of p25 protein using the anti-p25 antibody. The Rubisco protein was stained with Coomassie brilliant blue (blue bands) as a total protein loading control.
Discussion
Plant viral CP has multiple functions, not only as a structural protein that can encapsidate the viral DNA/RNA to form virions, it also plays an important role in viral infection, proliferation, cell to cell movement, pathogenicity, and vector transmission (Callaway et al., 2001). Here, we found that MCMV CP- p25, consists of 236 aa and is highly conserved in MCMV isolates from around the world. Bioinformatic analysis showed that p25 contains the typical viral CP domain, including the NLS (aa 6–38), membrane-anchored sequence (aa 198–213), and extracellular sequence (aa 214–236). We demonstrated that p25 caused the severe dwarfing and necrosis and was transported systemically in N. benthamiana by a TMV-based expression system. Subcellular localization analysis revealed that the NLS of p25 was exclusively localized in the host nucleus. This domain was also necessary for pathogenicity. Moreover, viral systemic movement in N. benthamiana was significantly weakened due to the deletion of the C-terminal region of p25, especially aa 198–213. Finally, the VIGS results showed that HSP90 may involve in TMV-p25 induced tissue necrosis. However, the mechanism of symptom development is undetermined.
Using chimeric viruses is a common strategy to study gene function in plant viruses, especially in negative-strand RNA viruses and some viruses that are difficult to manipulate (Yu et al., 2019; Mei et al., 2020). Thus, p25 is an important virulence factor in the TMV-p25 chimeric virus, consistent with the role of many viral CPs. The CP of TMV is broken down into monomers when it binds with the antimicrobial agent ningnanmycin, nearly resulting in the loss of pathogenicity (Li et al., 2017). The CP of cucumber mosaic virus (CMV) is also a determinant of chlorotic symptoms in infected hosts using chimeric ICs (Qiu et al., 2018). We also generated an NLS deletion mutant to examine subcellular localization and pathogenicity in plants and found that p25 is exclusively localized in the nucleus. At the N-terminal, the NLS is rich in basic aa that are essential for the nuclear location, consistent with the findings of a previous study (Zhan et al., 2016). However, Zhan et al. (2016) did not find that the p25 NLS was involved in pathogenicity. Here, we found that the deletion mutant protein did not affect viral accumulation but resulted in almost a total loss of pathogenicity. Growing evidence suggests that the location of the viral CP is responsible for symptom expression in the host (Liu et al., 2009). The N-terminal lysine-rich fragment induces the alfalfa mosaic virus CP to localize to the nucleus and accumulate in the nucleolus of infected plant cells (Herranz et al., 2012). The localization of pepper mild mottle virus changes from the chloroplast to the cell periphery when Asp is substituted for Asn at one location in the CP, which induces different symptoms (Han et al., 2020). The C-terminal region in viral CP has also been shown to be involved in viral cell-to-cell transport in plants (Fedorkin et al., 2001). In our current study, p25 mRNA transcripts or proteins were not detected in systemic leaves when the C-terminal was deleted from p25, which agrees with the significant reduction in symptom severity, including dwarfing and necrosis, in the whole plant. Thus, we consider C-terminal p25 to be indispensable for the long-distance movement and pathogenicity of the TMV-p25 chimeric virus. These findings encouraged us to further consider the subcellular localization of p25 and which domain plays a decisive role in pathogenicity.
Heat shock protein 90 (HSP90), a highly conserved protein in eukaryotes, participates in stimulating signal proteins, including protein kinases, hormone receptors, and transcription factors (Pearl and Prodromou, 2006). HSP90 also play an important role in the ubiquitination processes of defense response regulators in plants (Ishii et al., 2008; Chen et al., 2010; Kadota and Shirasu, 2012). HSP90-silenced plants do not develop the hypersensitive response (HR) elicited by INF1 and Pseudomonas cichorii, and the expression levels of pathogenesis-related protein genes decreased (Kanzaki et al., 2003). The activation of kinases NtMEK2, WIPK, and SIPK are also significantly inhibited when HSP90 is suppressed (Takabatake et al., 2007). In our study, necrosis did not develop in HSP90-silenced leaves, and p25 levels were almost the same in the different treatments. These results indicated that HSP90 is important for symptom development in the host plant rather than facilitating infection and replication, providing clues about the molecular mechanism of HSP90 essential in the pathway elicited by TMV-p25.
Other studies have suggested that CP interacts strongly with factors in host plants and other organisms. The long-distance movement of tomato mosaic virus is closely related to the interaction between viral CP and IP-L, which is located in the thylakoid membranes of tobacco (Zhang et al., 2008). Using the mutation of aa residues and a yeast two-hybrid system, soybean mosaic virus (SMV) CP was shown to interact with helper-component proteinase to enable the virus to be transmitted to plants by aphids (Seo et al., 2010). Moreover, the SMV CP interacts with soybean GmCPIP to facilitate viral infection and accumulation in soybean (Zong et al., 2020). Cucumber mosaic virus CP acts as a bridge between viral-encoded suppressors and host RNA silencing to facilitate viral self-attenuation and symptom recovery (Zhang et al., 2017). Resultantly, we considered that CP interactions with a host factor(s) were involved in viral invasion, symptom formation, and systemic movement; future studies will aim to determine more host factors by using a yeast two-hybrid system and bimolecular fluorescence complementation to better understand the involvement of MCMV p25 in symptom development and long-distance transport.
Data availability statement
The original contributions presented in this study are included in the article/Supplementary material, further inquiries can be directed to the corresponding authors.
Author contributions
XS and JL: conceptualization. WL: methodology and software. XS: validation. CZ and DW: formal analysis, data curation, and writing—original draft preparation. CZ, BZ, GA-F, and DW: investigation. CZ: resources and funding acquisition. XS and CZ: writing—review and editing. JL: visualization and supervision. All authors have read and agreed to the published version of the manuscript.
Funding
This research was funded by a grant from the National Natural Science Foundation of China (31800135).
Conflict of interest
The authors declare that the research was conducted in the absence of any commercial or financial relationships that could be construed as a potential conflict of interest.
The reviewer FL declared a shared affiliation with the authors, WL and XS to the handling editor at the time of review.
Publisher’s note
All claims expressed in this article are solely those of the authors and do not necessarily represent those of their affiliated organizations, or those of the publisher, the editors and the reviewers. Any product that may be evaluated in this article, or claim that may be made by its manufacturer, is not guaranteed or endorsed by the publisher.
Supplementary material
The Supplementary Material for this article can be found online at: https://www.frontiersin.org/articles/10.3389/fmicb.2022.951479/full#supplementary-material
Footnotes
- ^ https://www.ncbi.nlm.nih.gov/
- ^ https://rostlab.org/owiki/index.php/PredictNLS
- ^ https://services.healthtech.dtu.dk/service.php?TMHMM-2.0
- ^ http://www.swissmodel.expasy.org
References
Adams, I. P., Miano, D. W., Kinyua, Z. M., Wangai, A., Kimani, E., Phiri, N., et al. (2013). Use of next-generation sequencing for the identification and characterization of Maize chlorotic mottle virus and Sugarcane mosaic virus causing maize lethal necrosis in Kenya. Plant Pathol. 62, 741–749.
Azevedo, J., Garcia, D., Pontier, D., Ohnesorge, S., Yu, A., Garcia, S., et al. (2010). Argonaute quenching and global changes in Dicer homeostasis caused by a pathogen-encoded GW repeat protein. Genes Dev. 24, 904–915. doi: 10.1101/gad.1908710
Bockelman, D. L. (1982). Host range and seed-transmission studies of maize chlorotic mottle virus in grasses and corn. Plant Dis. 66, 216–218.
Cabanas, D., Watanabe, S., Higashi, C. H., and Bressan, A. (2013). Dissecting the mode of maize chlorotic mottle virus transmission (Tombusviridae: Machlomovirus) by Frankliniella williamsi (Thysanoptera: Thripidae). J. Econ. Entomol. 106, 16–24. doi: 10.1603/ec12056
Callaway, A., Giesman-Cookmeyer, D., Gillock, E. T., Sit, T. L., and Lommel, S. A. (2001). The multifunctional capsid proteins of plant RNA viruses. Annu. Rev. Phytopathol. 39, 419–460. doi: 10.1146/annurev.phyto.39.1.419
Chen, L., Hamada, S., Fujiwara, M., Zhu, T., Thao, N. P., Wong, H. L., et al. (2010). The Hop/Sti1-Hsp90 chaperone complex facilitates the maturation and transport of a PAMP receptor in rice innate immunity. Cell Host Microbe 7, 185–196. doi: 10.1016/j.chom.2010.02.008
Dawson, W. O., Beck, D. L., Knorr, D. A., and Grantham, G. L. (1986). cDNA cloning of the complete genome of tobacco mosaic virus and production of infectious transcripts. Proc. Natl. Acad. Sci. U.S.A. 83, 1832–1836. doi: 10.1073/pnas.83.6.1832
Fedorkin, O. N., Solovyev, A. G., Yelina, N. E., Zamyatnin, A. A., Zinovkin, R. A., Makinen, K., et al. (2001). Cell-to-cell movement of potato virus X involves distinct functions of the coat protein. J. Gen. Virol. 82, 449–458. doi: 10.1099/0022-1317-82-2-449
Goldberg, K. A., and Brakke, M. K. (1987). Concentration of maize chlorotic mottle virus in mixed infections with maize dwarf mosaic virus, strain B. Phytopathology 77, 162–177.
Han, K., Zheng, H., Ji, M., Cui, W., Hu, S., Peng, J., et al. (2020). A single amino acid in coat protein of Pepper mild mottle virus determines its subcellular localization and the chlorosis symptom on leaves of pepper. J. Gen. Virol. 101, 565–570. doi: 10.1099/jgv.0.001398
Herranz, M. C., Pallas, V., and Aparicio, F. (2012). Multifunctional roles for the N-terminal basic motif of Alfalfa mosaic virus coat protein: Nucleolar/cytoplasmic shuttling, modulation of RNA-binding activity, and virion formation. Mol. Plant Microbe Interact. 25, 1093–1103. doi: 10.1094/MPMI-04-12-0079-R
Hilker, F. M., Allen, L. J. S., Bokil, V. A., Briggs, C. J., Feng, Z., Garrett, K. A., et al. (2017). Modeling virus coinfection to inform management of maize lethal necrosis in Kenya. Phytopathology 107, 1095–1108. doi: 10.1094/PHYTO-03-17-0080-FI
Ishii, K. J., Koyama, S., Nakagawa, A., Coban, C., and Akira, S. (2008). Host innate immune receptors and beyond: Making sense of microbial infections. Cell Host Microbe 3, 352–363. doi: 10.1016/j.chom.2008.05.003
Jin, H., and Zhu, J. K. (2010). A viral suppressor protein inhibits host RNA silencing by hooking up with Argonautes. Genes Dev. 24, 853–856. doi: 10.1101/gad.1927310
Kadota, Y., and Shirasu, K. (2012). The HSP90 complex of plants. Biochim. Biophys. Acta 1823, 689–697. doi: 10.1016/j.bbamcr.2011.09.016
Kang, S. H., Qu, F., and Morris, T. J. (2015). A nuclear fraction of turnip crinkle virus capsid protein is important for elicitation of the host resistance response. Virus Res. 210, 264–270. doi: 10.1016/j.virusres.2015.08.014
Kanzaki, H., Saitoh, H., Ito, A., Fujisawa, S., Kamoun, S., Katou, S., et al. (2003). Cytosolic HSP90 and HSP70 are essential components of INF1-mediated hypersensitive response and non-host resistance to Pseudomonas cichorii in Nicotiana benthamiana. Mol. Plant Pathol. 4, 383–391. doi: 10.1046/j.1364-3703.2003.00186.x
Lewandowski, D. J., and Adkins, S. (2005). The tubule-forming NSm protein from Tomato spotted wilt virus complements cell-to-cell and long-distance movement of Tobacco mosaic virus hybrids. Virology 342, 26–37. doi: 10.1016/j.virol.2005.06.050
Li, X., Hao, G., Wang, Q., Chen, Z., Ding, Y., Yu, L., et al. (2017). Ningnanmycin inhibits tobacco mosaic virus virulence by binding directly to its coat protein discs. Oncotarget 8, 82446–82458. doi: 10.18632/oncotarget.19401
Liu, C., Meng, C., Xie, L., Hong, J., and Zhou, X. (2009). Cell-to-cell trafficking, subcellular distribution, and binding to coat protein of Broad bean wilt virus 2 VP37 protein. Virus Res. 143, 86–93. doi: 10.1016/j.virusres.2009.03.009
Liu, Y., Burch-Smith, T., Schiff, M., Feng, S., and Dinesh-Kumar, S. P. (2004). Molecular chaperone Hsp90 associates with resistance protein N and its signaling proteins SGT1 and Rar1 to modulate an innate immune response in plants. J. Biol. Chem. 279, 2101–2108. doi: 10.1074/jbc.M310029200
Lommel, S. A., Kendall, T. L., Xiong, Z., and Nutter, R. C. (1991). Identification of the maize chlorotic mottle virus capsid protein cistron and characterization of its subgenomic messenger RNA. Virology 181, 382–385. doi: 10.1016/0042-6822(91)90509-a
Mahuku, G., Lockhart, B. E., Wanjala, B., Jones, M. W., Kimunye, J. N., Stewart, L. R., et al. (2015). Maize lethal necrosis (MLN), an emerging threat to maize-based food security in Sub-Saharan Africa. Phytopathology 105, 956–965. doi: 10.1094/PHYTO-12-14-0367-FI
Mei, Y., Zhang, F., Wang, M., Li, F., Wang, Y., Zhou, X., et al. (2020). Divergent symptoms caused by Geminivirus-encoded C4 proteins correlate with their ability to bind NbSKη. J. Virol. 94:e01307-20. doi: 10.1128/JVI.01307-20
Mwatuni, F. M., Nyende, A. B., Njuguna, J., Xiong, Z., Machuka, E., and Stomeo, F. (2020). Occurrence, genetic diversity, and recombination of maize lethal necrosis disease-causing viruses in Kenya. Virus Res. 286, 198081–198093. doi: 10.1016/j.virusres.2020.198081
Niblett, C. L., and Claflin, L. E. (1978). Corn lethal necrosis, a new virus disease of corn in Kansas. Plant Dis. Rep. 62, 15–19. doi: 10.1094/PDIS-07-20-1446-SR
Nutter, R. C., Scheets, K., Panganiban, L. C., and Lommel, S. A. (1989). The complete nucleotide sequence of the maize chlorotic mottle virus genome. Nucleic Acids Res. 17, 3163–3177. doi: 10.1093/nar/17.8.3163
Pantaleo, V., Grieco, F., Di Franco, A., and Martelli, G. P. (2006). The role of the C-terminal region of olive latent virus 1 coat protein in host systemic infection. Arch. Virol. 151, 1973–1983. doi: 10.1007/s00705-006-0767-2
Park, S. H., Sit, T. L., Kim, K. H., and Lommel, S. A. (2013). The red clover necrotic mosaic virus capsid protein N-terminal amino acids possess specific RNA binding activity and are required for stable virion assembly. Virus Res. 176, 107–118. doi: 10.1016/j.virusres.2013.05.014
Pearl, L. H., and Prodromou, C. (2006). Structure and mechanism of the Hsp90 molecular chaperone machinery. Annu. Rev. Biochem. 75, 271–294. doi: 10.1146/annurev.biochem.75.103004.142738
Qiu, Y., Zhang, Y., Wang, C., Lei, R., Wu, Y., Li, X., et al. (2018). Cucumber mosaic virus coat protein induces the development of chlorotic symptoms through interacting with the chloroplast ferredoxin I protein. Sci. Rep. 8, 1205–1215. doi: 10.1038/s41598-018-19525-5
Rao, Y., You, Y., Zhu, S., Yan, J., Huang, G., and Wei, G. (2010). The loss evaluation index system and the direct economic losses of Maize chlorotic mottle virus invading China. Plant Quar. 24, 5–8.
Rehman, A. U., Li, Z., Yang, Z., Waqas, M., Wang, G., Xu, W., et al. (2019). The coat protein of citrus yellow vein clearing virus interacts with viral movement proteins and serves as an RNA silencing suppressor. Viruses 11, 329–345. doi: 10.3390/v11040329
Scheets, K. (1998). Maize chlorotic mottle machlomovirus and wheat streak mosaic rymovirus concentrations increase in the synergistic disease corn lethal necrosis. Virology 242, 28–38. doi: 10.1006/viro.1997.8989
Scheets, K. (2000). Maize chlorotic mottle machlomovirus expresses its coat protein from a 1.47-kb subgenomic RNA and makes a 0.34-kb subgenomic RNA. Virology 267, 90–101. doi: 10.1006/viro.1999.0107
Scheets, K. (2016). Analysis of gene functions in Maize chlorotic mottle virus. Virus Res. 222, 71–79. doi: 10.1016/j.virusres.2016.04.024
Seo, J. K., Kang, S. H., Seo, B. Y., Jung, J. K., and Kim, K. H. (2010). Mutational analysis of interaction between coat protein and helper component-proteinase of Soybean mosaic virus involved in aphid transmission. Mol. Plant Pathol. 11, 265–276. doi: 10.1111/j.1364-3703.2009.00603.x
Stenger, D. C., and French, R. (2008). Complete nucleotide sequence of a maize chlorotic mottle virus isolate from Nebraska. Arch. Virol. 153, 995–997. doi: 10.1007/s00705-008-0069-y
Su, X., Rehman, L., Guo, H., Li, X., and Cheng, H. (2018). The oligosaccharyl transferase subunit STT3 mediates fungal development and is required for virulence in Verticillium dahliae. Curr. Genet. 64, 235–246. doi: 10.1007/s00294-017-0729-0
Takabatake, R., Ando, Y., Seo, S., Katou, S., Tsuda, S., Ohashi, Y., et al. (2007). MAP kinases function downstream of HSP90 and upstream of mitochondria in TMV resistance gene N-mediated hypersensitive cell death. Plant Cell Physiol. 48, 498–510. doi: 10.1093/pcp/pcm021
Tang, Y., Wang, F., Zhao, J., Xie, K., Hong, Y., and Liu, Y. (2010). Virus-based microRNA expression for gene functional analysis in plants. Plant Physiol. 153, 632–641. doi: 10.1104/pp.110.155796
Tran, P. T., Choi, H., Choi, D., and Kim, K. H. (2016). Virus-induced gene silencing reveals signal transduction components required for the Pvr9-mediated hypersensitive response in Nicotiana benthamiana. Virology 495, 167–172. doi: 10.1016/j.virol.2016.05.011
Wamaitha, M. J., Nigam, D., Maina, S., Stomeo, F., Wangai, A., Njuguna, J. N., et al. (2018). Metagenomic analysis of viruses associated with maize lethal necrosis in Kenya. Virol. J. 15, 90–108. doi: 10.1186/s12985-018-0999-2
Wang, Q., Zhou, X. P., and Wu, J. X. (2014). First report of maize chlorotic mottle virus infecting sugarcane (Saccharum officinarum). Plant Dis. 98:572. doi: 10.1094/PDIS-07-13-0727-PDN
Xia, Z., Zhao, Z., Chen, L., Li, M., Zhou, T., Deng, C., et al. (2016). Synergistic infection of two viruses MCMV and SCMV increases the accumulations of both MCMV and MCMV-derived siRNAs in maize. Sci. Rep. 6, 20520–20531. doi: 10.1038/srep20520
Xie, L., Zhang, J., Wang, Q., Meng, C., Hong, J., and Zhou, X. (2011). Characterization of maize chlorotic mottle virus associated with maize lethal necrosis disease in China. J. Phytopathol. 159, 191–193. doi: 10.1111/j.1439-0434.2010.01745.x
Yu, M., Liu, H., Zheng, H., Yan, F., Zhao, X., Xia, Z., et al. (2019). Viral sequences required for efficient viral infection differ between two Chinese pepper mild mottle virus isolates. Virus Res. 267, 9–15. doi: 10.1016/j.virusres.2019.04.007
Zhan, B., Fei, L., Zhou, T., and Fan, Z. (2016). Nuclear import of Maize chlorotic mottle virus capsid protein is mediated by importin-α. Eur. J. Plant Pathol. 146, 881–892. doi: 10.1007/s10658-016-0966-y
Zhan, J., Shi, H. P., Li, W. M., Zhang, C., and Zhang, Y. Q. (2021). NbTMP14 is involved in tomato spotted wilt virus infection and symptom development by interaction with the viral NSm protein. Viruses 13, 427–438. doi: 10.3390/V13030427
Zhang, C., Liu, Y., Sun, X., Qian, W., and Qiu, B. (2008). Characterization of a specific interaction between IP-L, a tobacco protein localized in the thylakoid membranes, and Tomato mosaic virus coat protein. Biochem. Biophys. Res. Commun. 374, 253–257. doi: 10.1016/j.bbrc.2008.07.010
Zhang, C., Pei, X., Wang, Z., Jia, S., Guo, S., Zhang, Y., et al. (2012). The Rice stripe virus pc4 functions in movement and foliar necrosis expression in Nicotiana benthamiana. Virology 425, 113–121. doi: 10.1016/j.virol.2012.01.007
Zhang, X. P., Liu, D. S., Yan, T., Fang, X. D., and Wang, X. B. (2017). Cucumber mosaic virus coat protein modulates the accumulation of 2b protein and antiviral silencing that causes symptom recovery in planta. PLoS Pathog. 13:e1006522. doi: 10.1371/journal.ppat.1006522
Zhang, X., Zhao, X., Zhang, Y., Niu, S., Qu, F., Zhang, Y., et al. (2013). N-terminal basic amino acid residues of Beet black scorch virus capsid protein play a critical role in virion assembly and systemic movement. Virol. J. 10, 200–208. doi: 10.1186/1743-422X-10-200
Zhang, Y., Zhao, W., Li, M., Chen, H., Zhu, S., and Fan, Z. (2011). Real-time TaqMan RT-PCR for detection of maize chlorotic mottle virus in maize seeds. J. Virol. Methods 171, 292–294. doi: 10.1016/j.jviromet.2010.11.002
Zhao, L., Hu, Z., Li, S., Zhou, X., Li, J., Su, X., et al. (2019). Diterpenoid compounds from Wedelia trilobata induce resistance to tomato spotted wilt virus via the JA signal pathway in tobacco plants. Sci. Rep. 9, 2763–2774. doi: 10.1038/s41598-019-39247-6
Keywords: MCMV, p25, pathogenicity, systemic movement, HSP90
Citation: Zhang C, Wang D, Li W, Zhang B, Abdel-Fattah Ouf GM, Su X and Li J (2022) The coat protein p25 from maize chlorotic mottle virus involved in symptom development and systemic movement of tobacco mosaic virus hybrids. Front. Microbiol. 13:951479. doi: 10.3389/fmicb.2022.951479
Received: 24 May 2022; Accepted: 14 July 2022;
Published: 05 August 2022.
Edited by:
Yong Wang, Guizhou University, ChinaReviewed by:
Gang Yu, Shanghai Jiao Tong University, ChinaFangfang Li, Institute of Plant Protection (CAAS), China
Copyright © 2022 Zhang, Wang, Li, Zhang, Abdel-Fattah Ouf, Su and Li. This is an open-access article distributed under the terms of the Creative Commons Attribution License (CC BY). The use, distribution or reproduction in other forums is permitted, provided the original author(s) and the copyright owner(s) are credited and that the original publication in this journal is cited, in accordance with accepted academic practice. No use, distribution or reproduction is permitted which does not comply with these terms.
*Correspondence: Xiaofeng Su, suxiaofeng@caas.cn; Jun Li, junli@hebau.edu.cn
†These authors have contributed equally to this work