- Unité des Virus Émergents (UVE: Aix-Marseille Univ-IRD 190-Inserm 1207), Marseille, France
Jingmenviruses are a group of viruses identified recently, in 2014, and currently classified by the International Committee on Taxonomy of Viruses as unclassified Flaviviridae. These viruses closely related to flaviviruses are unique due to the segmented nature of their genome. The prototype jingmenvirus, Jingmen tick virus (JMTV), was discovered in Rhipicephalus microplus ticks collected from China in 2010. Jingmenviruses genomes are composed of four to five segments, encoding for up to seven structural proteins and two non-structural proteins, both of which display strong similarities with flaviviral non-structural proteins (NS2B/NS3 and NS5). Jingmenviruses are currently separated into two phylogenetic clades. One clade includes tick- and vertebrate-associated jingmenviruses, which have been detected in ticks and mosquitoes, as well as in humans, cattle, monkeys, bats, rodents, sheep, and tortoises. In addition to these molecular and serological detections, over a hundred human patients tested positive for jingmenviruses after developing febrile illness and flu-like symptoms in China and Serbia. The second phylogenetic clade includes insect-associated jingmenvirus sequences, which have been detected in a wide range of insect species, as well as in crustaceans, plants, and fungi. In addition to being found in various types of hosts, jingmenviruses are endemic, as they have been detected in a wide range of environments, all over the world. Taken together, all of these elements show that jingmenviruses correspond exactly to the definition of emerging viruses at risk of causing a pandemic, since they are already endemic, have a close association with arthropods, are found in animals in close contact with humans, and have caused sporadic cases of febrile illness in multiple patients. Despite these arguments, the vast majority of published data is from metagenomics studies and many aspects of jingmenvirus replication remain to be elucidated, such as their tropism, cycle of transmission, structure, and mechanisms of replication and restriction or epidemiology. It is therefore crucial to prioritize jingmenvirus research in the years to come, to be prepared for their emergence as human or veterinary pathogens.
Introduction
According to the International Committee on Taxonomy of Viruses, the genus Flavivirus, family Flaviviridae, includes 53 officially identified species and 38 related unclassified viruses (mammalian tick-borne, mosquito-borne, insect-specific flaviviruses, viruses with no known arthropod vector, segmented flavi-like viruses) (International Committee on Taxonomy of Viruses [ICTV], 2022). Flaviviruses are found worldwide and can be responsible for significant human and veterinary diseases (Pierson and Diamond, 2020). Examples include the mosquito-transmitted dengue, West Nile, Japanese encephalitis, yellow fever, Zika viruses, or the tick-transmitted tick-borne encephalitis virus, which infect hundreds of millions of individuals each year (Pierson and Diamond, 2020). Most of the identified flaviviruses to date are either mosquito- or tick-borne in which case their replication cycle alternates between an arthropod vector and a vertebrate host (International Committee on Taxonomy of Viruses [ICTV], 2022). However, some flaviviruses have been found to be insect-specific, while others have no known vector associations (International Committee on Taxonomy of Viruses [ICTV], 2022).
The genome of flaviviruses is composed of an 11 kilobase-long single molecule of positive single-stranded RNA (+ssRNA), with no polyadenylation at the 3 prime end, and is encapsidated in an enveloped ∼45 nm icosahedral virus particle (Westaway et al., 1985). The genome contains an open reading frame encoding for a single polyprotein co- and post-translationally cleaved into three structural and seven non-structural proteins (Westaway et al., 1985). Non-structural proteins of interest here are NS5, the most conserved, which contains the RNA-dependent RNA polymerase (RdRp) and methyltransferase (MTase) domains, and NS2B/NS3 which forms the viral protease while NS3 also has a helicase domain (Westaway et al., 1985).
Qin et al. (2014) published the discovery and identification of a flavi-like virus with a segmented genome. Jingmen tick virus (JMTV) was identified as a flavi-like virus due to the high level of similarity of two of its segment sequences with the NS5 and NS2B/NS3 proteins of flaviviruses. Since this discovery, and due to the development and more affordable access to high throughput next-generation sequencing, JMTV sequences have been detected all over the world, alongside multiple other species of segmented flavi-like viruses. This review details what has been found on segmented flavi-like viruses, and why research on these viruses should be a high priority, in particular due to their high risk of emergence as pathogens.
Tick- and vertebrate-associated jingmenviruses
Jingmen tick virus
Segmented flaviviruses, i.e., flavi-like viruses with segmented genomes, were first described by Qin et al. (2014) as Jingmen tick virus (JMTV) was identified in a pool of Rhipicephalus microplus ticks collected in the Jingmen region of Hubei province in China in 2010 (Qin et al., 2014). The newly identified virus was shown to have a (+ssRNA) genome composed of four 3 prime polyadenylated segments, one bicistronic and three monocistronic, with 5 prime (GCAAGUGCA) and 3 prime (GGCAAGUGC) termini conserved sequences across segments (Qin et al., 2014). To date, no study has addressed the existence or nature of an RNA cap in 5 prime as in flaviviruses, but the genome contains an MTase domain, which is known to be involved in the cap synthesis for flaviviruses (Liu et al., 2010). Indeed, the first segment encodes for NSP1, a flavivirus NS5-like protein with putative RNA-dependent RNA polymerase (RdRp) and methyltransferase domains (Figures 1, 2; Qin et al., 2014). Segment 3 encodes for NSP2, which shares similarities with the NS2B/NS3 flavivirus protein complex, namely, transmembrane regions, serine protease, and helicase domains (Qin et al., 2014). Cartoon representations of the structure of functional domains of both non-structural proteins are shown in Figure 2 for three representative viruses, including JMTV. The other two segments encode for VP1 (segment 2) and VP2-3 (segment 4) which are thought to be structural proteins, likely to be the putative envelope, capsid, and membrane proteins, respectively (Qin et al., 2014). An additional open reading frame (ORF) overlapping with VP1 coding sequence at the start of segment 2 has been proposed for JMTV and related viruses (nuORF, or VP4), which would encode for a small membrane protein (Kholodilov et al., 2020). The overall length of the JMTV genome is 11,401 nucleotides, similar to that of flaviviruses (Qin et al., 2014).
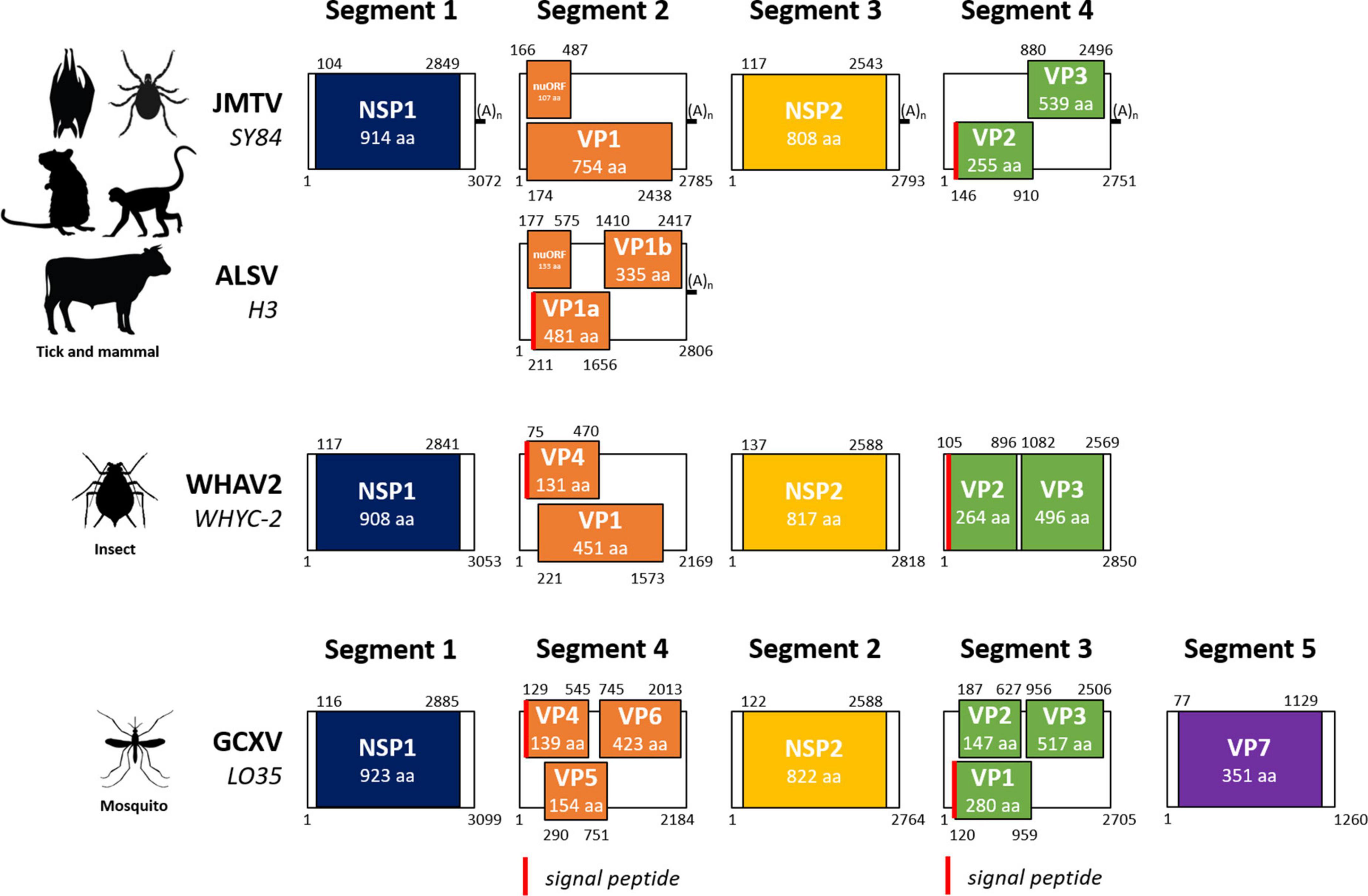
Figure 1. Genome organization of jingmenviruses. The layout of this figure is modified from Kobayashi et al. (2021). JMTV SY84, Jingmen tick virus strain SY84 (NC_024113-NC_024117); ALSV H3, Alongshan virus strain H3 (MH158415–MH158418); WHAV2 WHYC-2, Wuhan aphid virus 2 strain WHYC-2 (KR902725–KR902728); GCXV LO35, Guaico Culex virus strain LO35 (KM461666-KM461670). (A)n, polyadenylation. NSP1, non-structural protein 1, RNA-dependent RNA polymerase and methyltransferase domains; VP, virus (structural) protein; nuORF, open reading frame identified by Kholodilov et al. (2020); NSP2, non-structural protein 2, serine protease and helicase domains. Red bar: signal peptide. The genome organization of JMTV and ALSV are representative of tick- and mammal-associated jingmenviruses; WHAV2 represents insect-associated jingmenviruses; GCXV represents mosquito-associated jingmenviruses.
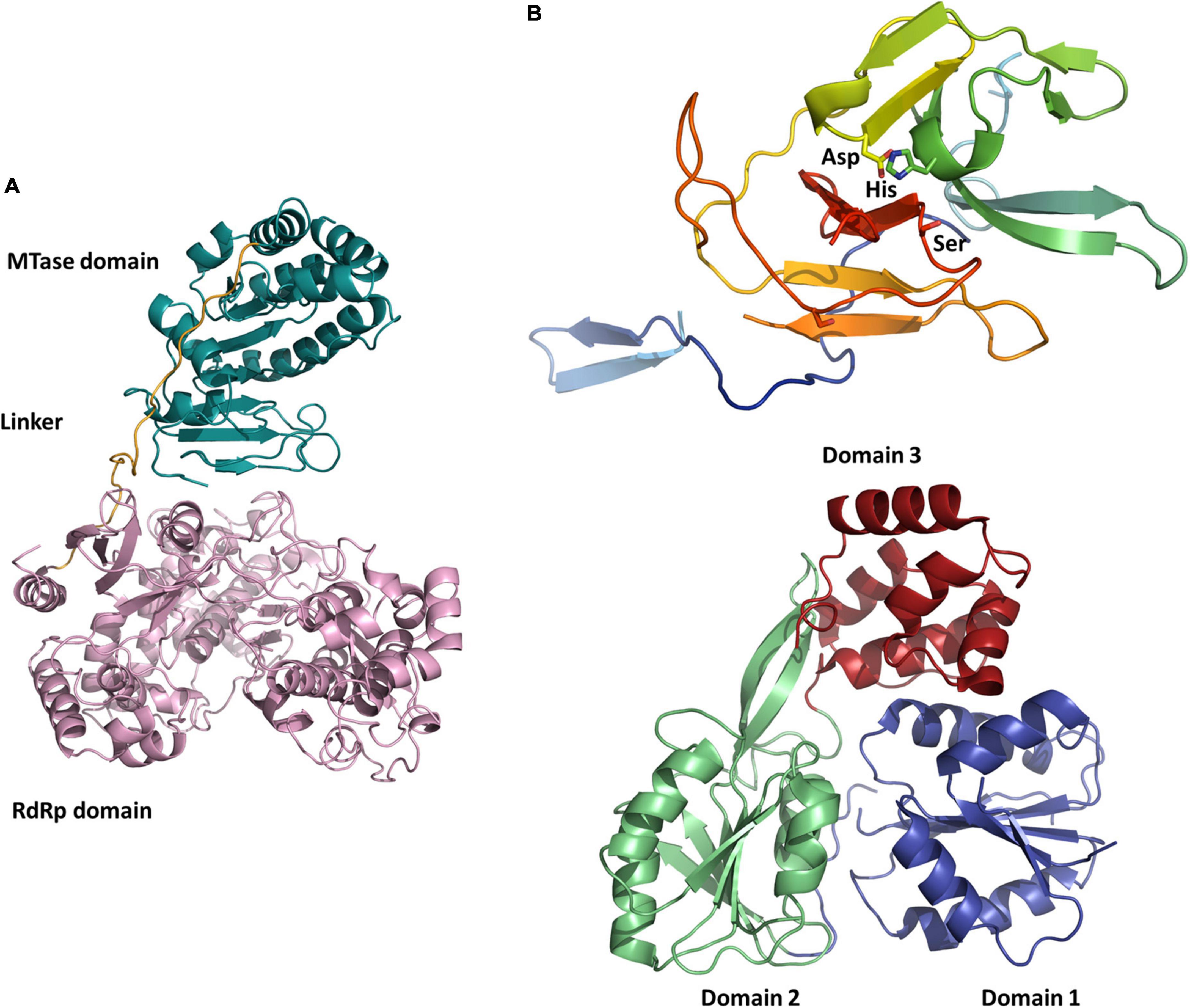
Figure 2. Structures of NSP1 and NSP2 functional domains putatively involved in the replication. (A) Cartoon representation of the NSP1 MTase (turquoise) and RdRp (pink) domains of Guaico Culex virus (homology model). (B) Cartoon representation of the NSP2 protease domain of Jingmen tick virus (homology model) with the canonical Asp-His-Ser catalytic triad (top) and NSP2 helicase domain of Alongshan virus (PDB code 6M40, from X-ray crystallography data in Gao et al., 2020) organized in subdomains 1 (blue), 2 (green), and 3 (ochre) (bottom). All representations were prepared using PyMOL (Schrödinger).
A partial sequence from Brazilian R. microplus was identified in 2013 as part of a flaviviral genome (termed Mogiana tick virus – MGTV), prior to the characterization of JMTV (Maruyama et al., 2014). The sequences were later found to share 93–97% amino acid identity with JMTV, suggesting these are strains of the same virus, but the segmented nature of its genome had not been identified at the time (Maruyama et al., 2014; Villa et al., 2017).
Since the discovery of JMTV, sequences of JMTV strains have been detected from samples originating from four continents: Asia (China, Lao PDR, Japan), Europe (Turkey, Italy, Kosovo, Romania, Russia), the Americas (Brazil, Trinidad and Tobago, French Antilles, Colombia) and Africa (Uganda, Guinea, Kenya) (see Table 1 and Figure 3). To date, the species in which JMTV RNA has been detected most commonly is the tick R. microplus. In fact, JMTV was found in high prevalence in R. microplus from China (53–63%), Brazil (25–67%), Trinidad and Tobago (6–46%), and the French Antilles (24–77%) over the years (Maruyama et al., 2014; Qin et al., 2014; Souza et al., 2018; Pascoal et al., 2019; Sameroff et al., 2019; Temmam et al., 2019; Gondard et al., 2020; Guo J. J. et al., 2020; Kobayashi et al., 2021; Xu et al., 2021). However, the nature of the samples containing JMTV RNA extends beyond this species and includes 25 additional tick species from several genera, Rhipicephalus, Amblyomma, Dermacentor, Haemaphysalis, Hyalomma, and Ixodes (Maruyama et al., 2014; Qin et al., 2014; Souza et al., 2018; Dinçer et al., 2019, 2022; Jia et al., 2019; Meng et al., 2019; Pascoal et al., 2019; Sameroff et al., 2019; Temmam et al., 2019; Gondard et al., 2020; Guo J. J. et al., 2020; Gómez et al., 2020; Ternovoi et al., 2020b; Xu et al., 2020, 2021; Bratuleanu et al., 2021; Li et al., 2021; Shi et al., 2021; Ogola et al., 2022), as well as two mosquito species (Qin et al., 2014; Parry et al., 2021). The local prevalence of JMTV RNA in tick species such as Hae. longicornis, Hae. campanulata, D. nuttalli, Hae. hystricis in different regions of China can be as high as 11–55, 75, 75, and 46% respectively (Qin et al., 2014; Meng et al., 2019; Guo J. J. et al., 2020; Xu et al., 2021). While these numbers are calculated either with individual tick numbers (minimum infection rate) or estimated from pool numbers, it is nonetheless clear that JMTV can be found in high prevalence in ticks with a widespread geographical distribution, particularly in R. microplus. This invasive tick species is considered the most important cattle tick in the world since it can transmit a range of diseases and has spread from Asia to a range of tropical and subtropical regions of Africa, America, and Australasia (Estrada-Peña et al., 2006a,b; Barré and Uilenberg, 2010). The distribution of R. microplus is predicted to expand even further and reach Western Europe due to climate change (Marques et al., 2020). An example of this unstoppable spread is North America, where an eradication program took place over 40 years in the first half of the 20th century, which could not prevent the recent reintroduction of R. microplus or its predicted spread throughout this continent (Giles et al., 2014).
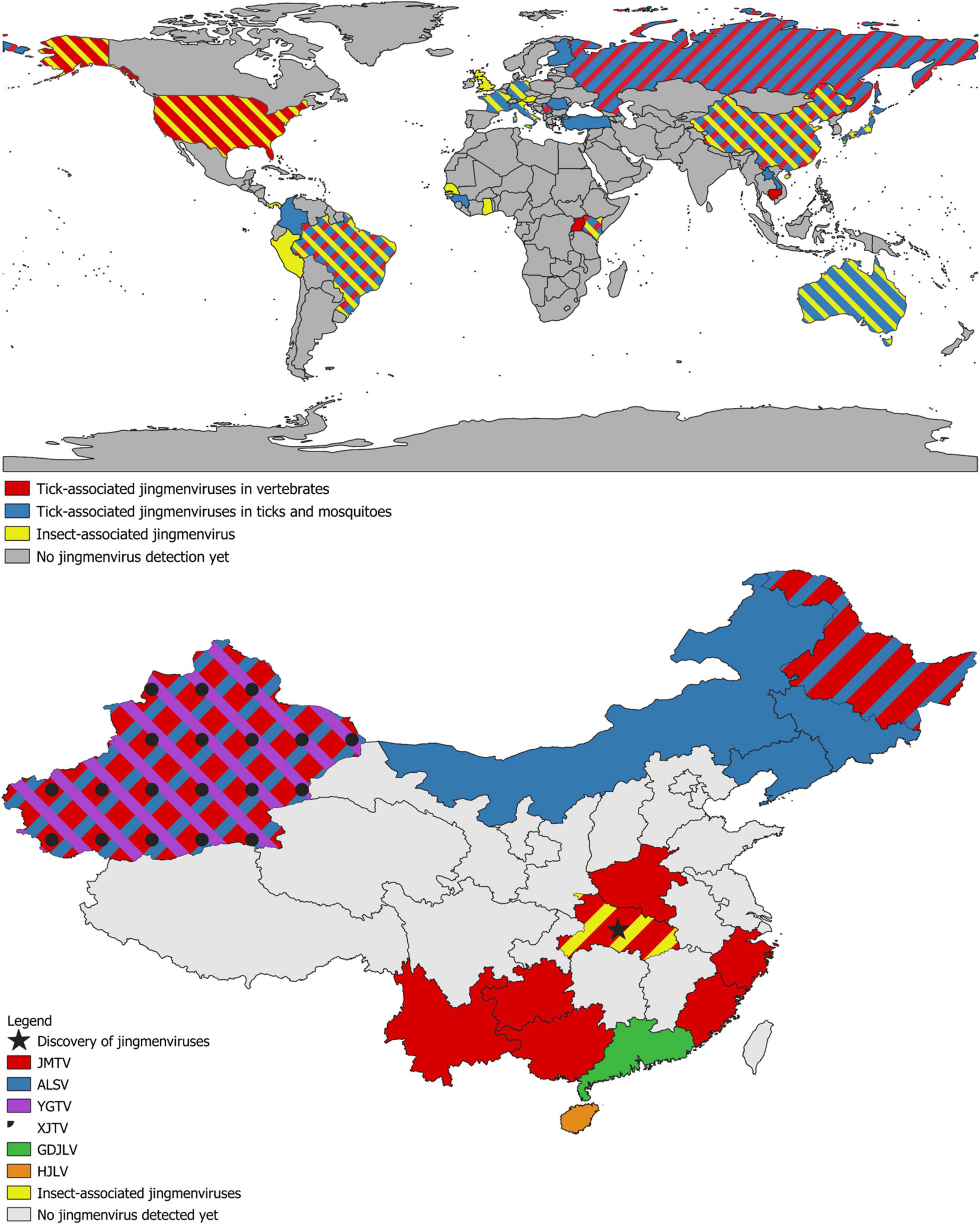
Figure 3. (Top) World map of detections of jingmenvirus sequences. Countries in which tick-associated jingmenviruses have been detected in vertebrates contain red; countries in which tick-associated jingmenviruses have been detected in ticks and mosquitoes contain blue; countries in which insect-associated jingmenviruses have been detected contain yellow. (Bottom) Map of detections of jingmenvirus sequences in Chinese provinces. The province where jingmenviruses were first discovered is highlighted with a black star. Provinces in which Jingmen tick virus (JMTV) has been detected contain red; provinces in which Alongshan virus (ALSV) has been detected contain blue; provinces in which Yanggou tick virus (YGTV) has been detected contain purple; provinces in which Xinjiang tick virus (XJTV) has been detected contain black spots; provinces in which Guangdong jingmen-like virus (GDJLV) has been detected contain green; provinces in which Hainan jingmen-like virus (HJLV) has been detected contain orange; provinces in which insect-associated jingmenviruses have been detected contain yellow. All other provinces are in gray. These maps were drawn using the QGIS software and open-source geographic data.
In addition to arthropods, JMTV RNA was also detected in vertebrates, either in mammals-derived samples such as cattle serum (Qin et al., 2014; Souza et al., 2018; Guo J. J. et al., 2020), primate plasma (Ladner et al., 2016), serum and organs from 11 species of bats from 6 genera and 11 species of rodents from 7 genera (Guo J. J. et al., 2020; Yu et al., 2020); or reptile-derived samples such as tortoise blood (Ogola et al., 2022). Finally, JMTV genomes were recovered from three acute phase serum samples collected from Crimean-Congo hemorrhagic fever orthonairovirus patients as well as from skin biopsies from four patients with tick bites (Emmerich et al., 2018; Jia et al., 2019). While the reported prevalence of JMTV RNA in vertebrates is lower than in ticks, it is still remarkable for cattle from China (3.5–10%) and Brazil (14%), for bats (12%) and rodents (7%) from China and for tortoises from Kenya (67%) (Qin et al., 2014; Souza et al., 2018; Guo J. J. et al., 2020; Yu et al., 2020; Shi et al., 2021; Ogola et al., 2022). Moreover, an in-depth study of rodents from China detected JMTV in all tested organs (liver, kidney, lung, heart, and spleen), preferentially in the liver samples (25%), and particularly in Apodemus uralensis (29%) and Microtus arvalis (24%) (Yu et al., 2020).
In concordance with RNA detection, anti-JMTV antibodies have been found in cattle from three regions of China with 18–37% seroprevalence (Qin et al., 2014; Shi et al., 2021). Moreover, patients seronegative for tick-borne encephalitis virus (TBEV) from China with a history of tick bites were tested for seroconversion to JMTV and 1.6% were positive (Jia et al., 2019). Furthermore, one sporadic detection of anti-JMTV antibodies was reported in a cohort of 70 patients with recorded tick bites from France (Temmam et al., 2019). Taken together with the RNA detections listed above, these data strongly suggest that JMTV could replicate in vertebrates, notably cattle and humans, although the tropism, pathogenesis, and clinical manifestations of the infection remain unknown.
To date, only limited ecological data relate to the transmission cycle of this virus. Indeed, JMTV was detected in adult ticks, both males and females, as well as in nymphs and unfed larvae, suggesting it could be vertically transmitted (Maruyama et al., 2014; Jia et al., 2019; Pascoal et al., 2019; Kobayashi et al., 2021). It has also been detected in the salivary glands of naturally and experimentally infected male ticks, suggesting the virus could be transmitted through an infected tick bite (Maruyama et al., 2014; Jia et al., 2019). Furthermore, the analysis of the codon usage bias of JMTV supports the hypothesis that it is a true arbovirus, cycling between an arthropod vector and a vertebrate host (Maruyama et al., 2014). Finally, sequence analysis revealed that JMTV genetic diversity is more strongly influenced by geographic distance than by the host from which the sequence originated, which is compatible with a vector-borne transmission cycle (Figure 4; Guo J. J. et al., 2020).
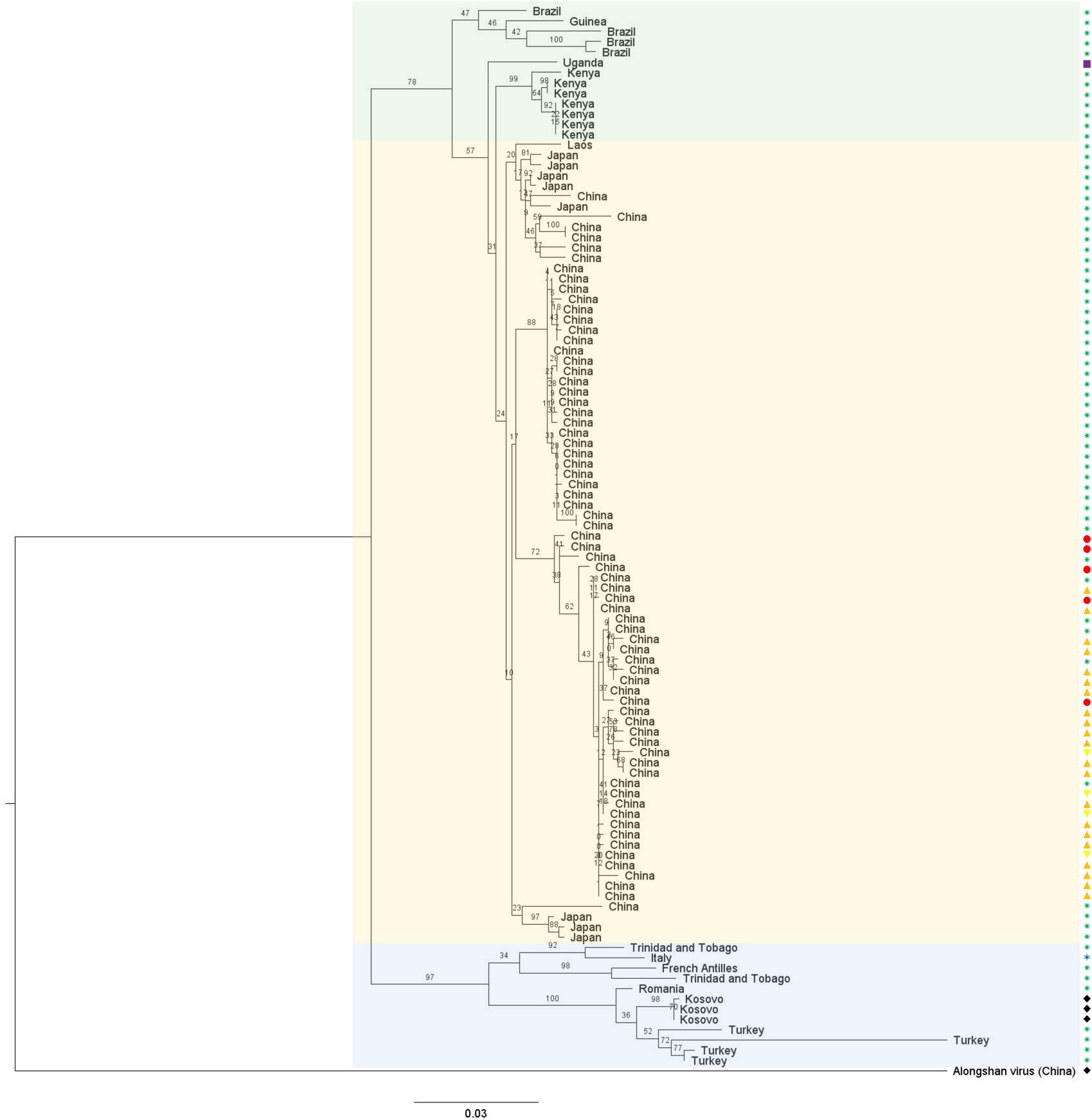
Figure 4. Phylogenetic analysis of all published full-length NSP1 ORF amino acid sequences of JMTV strains, and the prototype strain of ALSV as an outgroup. This tree was built with PhyML with an LG substitution model and midpoint-rooted. The branches are labeled with the bootstrap proportion in percentages (out of 100 bootstraps), the tips are labeled with the sample collection location, and the bar represents 0.03 substitutions per nucleotide position. JMTV strains seem to cluster according to their detection location rather than host with two clear subclades, the European and Central American strains (shaded in blue) on one side and the Asian (shaded yellow) and African and South American (shaded green) strains on the other side. The hosts are represented with symbols on the side of the phylogenetic tree: ticks are green stars with eight branches, mosquitoes are blue stars with six branches, monkeys are purple squares, cattle are red circles, bats are orange triangles pointing up, rodents are yellow triangles pointing down and humans are black diamonds.
Despite a strong presence in environmental samples, the virus does not seem to be fit for replication in the laboratory models tested to date. Indeed, most authors report that isolating the strains they sequenced was unsuccessful, or could only be sustained for a couple of passages, on BME26 cells (R. microplus, tick), C6/36 cells (Aedes albopictus, mosquito), DH82 cells (Canis familiaris, dog) or Vero E6 cells (Cercopithecus aethiops, monkey) at low titers and did not necessarily produce cytopathic effect (CPE) (Maruyama et al., 2014; Qin et al., 2014; Ladner et al., 2016; Souza et al., 2018; Dinçer et al., 2019; Meng et al., 2019; Kobayashi et al., 2021; Shi et al., 2021; Ogola et al., 2022). Jia et al. (2019) reported sustained JMTV replication in the BME/CTVM23 (R. microplus) cell line with a 3-log titer increase by day 7, but the number of positive passages is not mentioned. These limited models enabled the observation of thin sections of infected DH-82 cells and their negatively stained supernatant by transmission electron microscopy which suggested that JMTV particles are enveloped and slightly larger than flaviviruses, with a diameter of 70–80 nm and clear protrusions (Qin et al., 2014). Other, unsuccessful, laboratory models tested were the following cells lines: BHK-21 (Mesocricetus auratus, hamster), LLC-PK1 (Sus scrofa, pig), PK-15 (Sus scrofa, pig), MDBK (Bos taurus, bovine), Vero (Cercopithecus aethiops, monkey), HEK-293 (Homo sapiens, human), DF-1 (Gallus gallus, chicken), and new-born mice (Maruyama et al., 2014; Souza et al., 2018; Meng et al., 2019; Shi et al., 2021). Interestingly, JMTV RNA was reported to be detected in midguts and salivary glands of experimentally infected adult male A. javanense, which suggests live tick models could provide more sustainable and consistent laboratory models than cell culture (Jia et al., 2019). Setting up a laboratory model fitting JMTV replication would be a clear step toward a better fundamental understanding of this virus. Finding and studying virus strains or species that are better suited for replication in vitro could be another solution.
Since 2014, segmented flavi-like virus sequences have been detected worldwide. Some of these sequences share over 95% identity with JMTV (Kindia tick virus, Guangxi tick virus, Amblyomma virus, or Manych virus) and are therefore likely to all belong to the same species (Jia et al., 2019; Ternovoi et al., 2020b; Xu et al., 2020). Sequences from novel segmented flavi-like virus species have also been identified and grouped under the putative genus name Jingmenvirus.
Alongshan virus
A novel jingmenvirus tentatively named Alongshan virus (ALSV) was isolated and sequenced from a blood sample taken from a human patient reporting tick bites in China in 2017 (Wang et al., 2019a). The genome organization of ALSV is similar to that of JMTV, except for the fact that segment 2 seems to be at least bicistronic, with two overlapping reading frames VP1a and VP1b, as well as the putative open reading frame nuORF (Figure 1; Wang et al., 2019a). ALSV NSP1 and NSP2 share approximately 80% amino acid similarity with JMTV, the segments are 3 prime polyadenylated, and the segment termini conserved sequences are homologous between the two viruses. The structural proteins share only 25–75% amino acid similarity with their JMTV counterparts, suggesting that ALSV is a novel species in the Jingmenvirus genus (Wang et al., 2019a).
Similarly to JMTV, ALSV was subsequently detected in a number of tick and mosquito hosts in varying prevalence, I. persulcatus, I. ricinus, D. nuttalli, D. reticulatus, Hae. concinna, Hae. longicornis, Anopheles yatsushiroensis, Ae. vexans, Cx. pipiens pallens, and Cx. tritaeniorhynchus, as well as from sheep, cattle, and deer sera, from locations in Eurasia: in China, Finland, Russia, Serbia, Germany, and France (Kuivanen et al., 2019; Temmam et al., 2019; Wang et al., 2019a,b; Boelke et al., 2020; Kholodilov et al., 2020, 2021; Stanojević et al., 2020; Hu, 2022) (Table 1 and Figure 3). Its main host seems to be Ixodes ticks, which are the main vector for TBEV transmission in Europe (Jääskeläinen et al., 2011; European Centre for Disease Prevention and Control [ECDC], and European Food Safety Authority [EFSA], 2018, 2020; Chitimia-Dobler et al., 2019). Ixodes ticks are widely distributed in Asia and Europe, and common hosts include sheep, cattle, horses, dogs, rabbits, and humans (Jääskeläinen et al., 2011; European Centre for Disease Prevention and Control [ECDC], and European Food Safety Authority [EFSA], 2018, 2020; Chitimia-Dobler et al., 2019).
In the north-eastern region of China, 9% of sheep and 5% of cattle were found to have ALSV-reactive antibodies, and 4% of sheep and 2% of cattle had neutralizing antibodies (Wang et al., 2019b). Moreover, a retrospective survey of patients who presented with undiagnosed symptoms and a history of tick bites in the same region as the prototype found evidence of ALSV in 23% of around one hundred tested samples (Wang et al., 2019a). The patients had non-specific clinical symptoms (headache and fever) and all had a complete recovery (Wang et al., 2019a). Seroconversion and seroneutralization against ALSV were detected in all tested positive patients, which, according to the authors, suggests the induction of a humoral immune response (Wang et al., 2019a).
The detection of JMTV and ALSV in human samples is notable, particularly considering that their tropism and pathogenesis remain to be formally elucidated. This could be facilitated by the fact that ALSV seems to be better suited to classical laboratory models than JMTV. Indeed, the virus was isolated from human, cattle, and sheep samples from China and passaged in Vero cells by Wang et al., which produced CPE at earlier time points at every passage, suggesting a certain level of cell adaptation (Wang et al., 2019a,b). However, Kuivanen et al. (2019) have found that their Finnish ALSV isolates did not replicate on Vero cells. Limited ALSV replication was found in a range of human cell lines (SH-SY5Y, WISH, SMMC, THP-1) after inoculation with a concentrated stock of virus (Wang et al., 2019a). Kholodilov et al. (2020, 2021) found that two tick cell lines (IRE/CTVM19 from I. ricinus and HAE/CTVM8 from Hya. anatolicum) can successfully sustain persistent infection by a Russian strain of ALSV, over up to several years, with no CPE. Balb/c mice were inoculated intraperitoneally with cell culture supernatant containing a Chinese strain of virus, with 107 copies of RNA and were found to present pathological changes in the liver, kidneys, and brain 14 days post-injection (Wang et al., 2019a). Viral RNA was detected in the liver, spleen, and lung tissues (107 RNA copies/mL), as well as in the kidney and heart tissues (106 RNA copies/mL) but to a lesser extent in brain tissues and blood (104 RNA copies/mL) 30 days post-infection (Wang et al., 2019a). However, ALSV replication was not detected in BHK-21, Hepa 1-6 (Mus musculus, mouse), or in U-87MG, HFF, Caco-2, SK-N-SH, and CRL-2088 (Homo sapiens, human) cell lines (Kuivanen et al., 2019; Wang et al., 2019a).
This limited replication in laboratory models enabled the imaging of ALSV particles, by transmission electron microscopy of thin sections of infected Vero cells and negatively stained purified particles. The prototype ALSV particles, from China, are enveloped and larger than flaviviruses, with a diameter of 80–100 nm (Wang et al., 2019a). However, Kholodilov et al. (2020) reported that purified ALSV particles from a Russian isolate had a diameter of around 40 nm, accompanied by smaller particles, with a diameter of 13 nm. It is not clear why such a difference in size was observed between these Chinese and Russian isolates of ALSV, but this discrepancy highlights the need for a stable and widely available laboratory replication system for jingmenviruses.
Some ALSV proteins have been characterized despite the lack of a stable replication model. Pending X-ray crystallography and cryo-electron microscopy confirmation, Garry and Garry have used structural models to show that the jingmenvirus glycoproteins share similarities with the glycoproteins of flaviviruses, alphaviruses and bunyaviruses, termed class II viral fusion proteins (Garry and Garry, 2020). The putative fusion peptide loop was identified in ALSV VP1a (amino acids 119–129), and one of the subdomains of VP1a shows high structural similarities with the flaviviral E domain III (Garry and Garry, 2020). An additional, jingmenvirus-specific domain was predicted, as a mucin-like domain which is thought to be modified by O-glycosylation(s). Such post-translational modifications may shield the virus from recognition by the host’s immune system (Garry and Garry, 2020). Despite the difference in organization of the segment coding for the glycoprotein, their models applied to both JMTV VP1 and ALSV VP1a, but not to insect-associated jingmenviruses (see below) (Garry and Garry, 2020).
Besides the models built for the structural proteins, a recombinant version of the ALSV protein NSP2 was used to determine a 2.9 Å-resolution structure of its helicase domain by X-ray crystallography (PDB 6M40) (Figure 2; Gao et al., 2020). The ATPase activity of the recombinant protein was confirmed and it was shown that structural features at the ATPase active site and RNA-binding groove remain conserved between flaviviruses and ALSV (Gao et al., 2020).
Other tick-associated jingmenviruses
Only four other tick-associated jingmenviruses species have been reported to date (Table 1). Yanggou tick virus (YGTV) was first identified from ticks collected in China in 2014 [unpublished data, deposited on Genbank by Shi J. M. (2021)]. Viral sequences were identified from 21 samples in that original collection, 3 from D. nuttalli and 18 from unspecified tick species. YGTV RNA was subsequently detected in two D. marginatus and one D. nuttalli samples during a tick survey in Russia during the period 2011-2019. Although the number of samples remains low, YGTV seems to be associated to Dermacentor spp. ticks (Kholodilov et al., 2021). Its genome organization resembles that of ALSV, with VP1a and VP1b in segment 2. YGTV shares 80% amino acid similarity over NSP1 and NSP2 with both JMTV and ALSV, and 50–60% amino acid similarity over the structural proteins. Similarly to ALSV, YGTV positive samples were used in a successful isolation attempt on IRE/CTVM19 and HAE/CTVM8 tick cells, with a persistent infection displaying no CPE (Kholodilov et al., 2021).
Takachi virus was detected in five pools of Hae. formosensis nymphs collected by flagging in Japan between 2019 and 2020. The virus could not be isolated on Vero or BHK-21 cells, but its genome sequence was determined and shares 55–85% amino acid similarity with JMTV and ALSV (Kobayashi et al., 2021).
Newport Tick virus (also named Ixodes holocyclus jingmenvirus) was identified in a metagenomics study of ticks from New South Wales in Australia (Gofton et al., 2022). The sequences were detected in relatively high prevalence in I. holocylcus ticks from Kioloa and Sydney. According to the authors, the largest contig (2087 nt) encodes the complete putative glycoprotein, which shares approximately 62% homology to JMTV, ALSV, and YGTV (from BLAST results). A MUSCLE alignment using the available glycoprotein sequences of tick- and vertebrate-associated jingmenviruses shows that this sequence shares between 51 and 55% homology with one group of tick- and vertebrate-associated jingmenviruses (ALSV, YGTV, XJTV1, TAKV) and between 27 and 30% with the other group (JMTV, PLJV and GDJLV – see below). This virus could not be included in our phylogenies (Figure 5) since no NSP1 or NSP2 sequences have been published yet. Additional sequences from other segments would be needed to confirm the segmented nature of this virus genome and its position in the phylogenies.
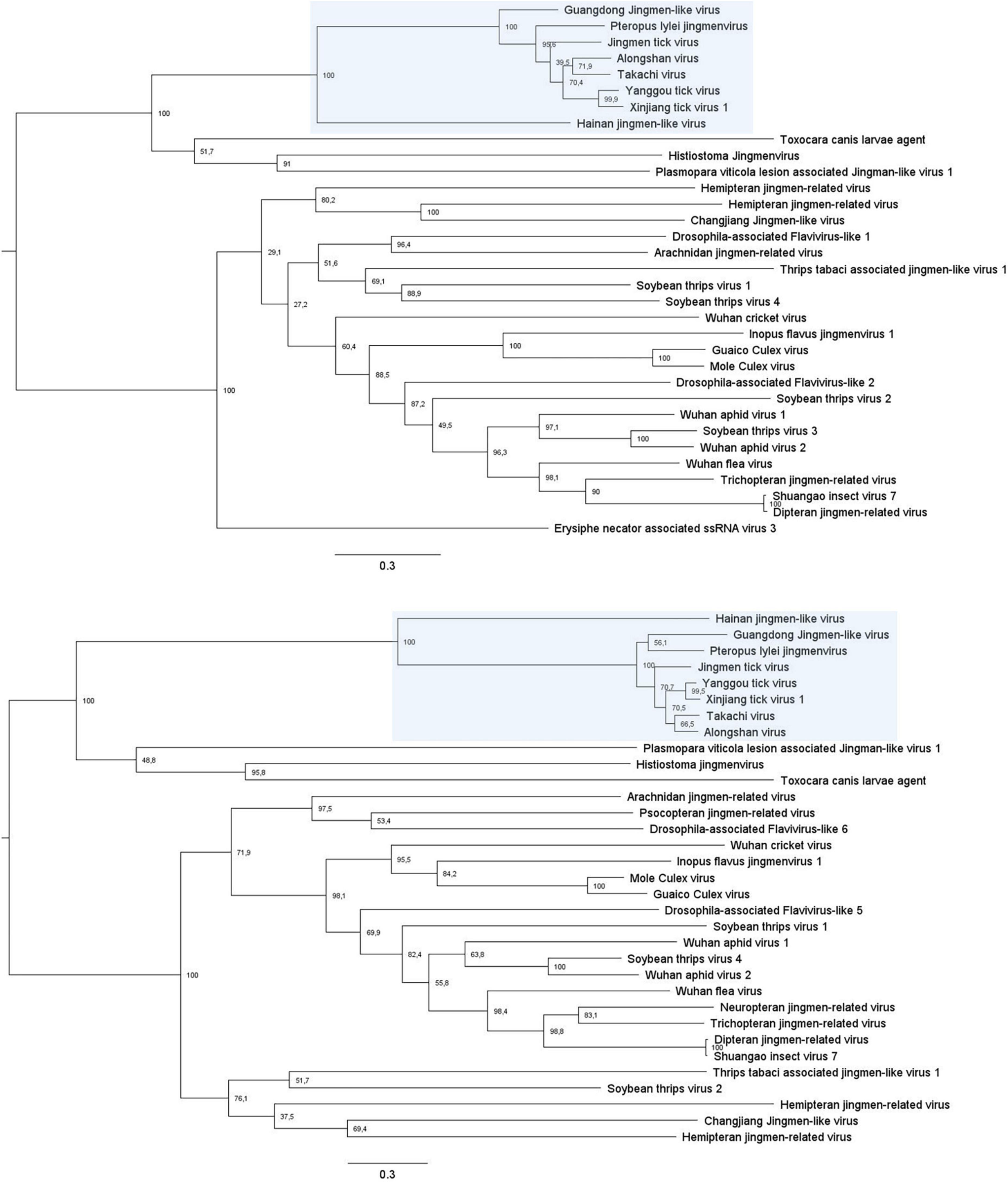
Figure 5. Phylogenetic analysis of all known jingmenvirus species with full-length NSP1 (Top) and NSP2 (Bottom) ORF amino acid sequences. These trees were built with PhyML with an LG substitution model and midpoint rooted. The branches are labeled with the bootstrap proportion in percentages (out of 1000 bootstraps) and the bars represent 0.3 substitutions per nucleotide position. Jingmenviruses cluster in two main clades, on the one hand the tick- and vertebrate-associated jingmenviruses (shaded in blue) and on the other hand, insect-associated jingmenviruses.
Finally, Xinjiang tick virus 1 was detected from unspecified ticks from China [unpublished data, deposited on Genbank by Shi J. M. (2021)]. At this time, no additional information is available on these sequences. An analysis using NCBI BLASTx shows that the virus with the most similar sequences is YGTV and pairwise MUSCLE alignments show that their ORFs share 75–91% amino acid similarity (National Library Medicine [NIH], 2022).
Jingmenvirus sequences related to tick-associated jingmenviruses
A complete coding sequence for a novel segmented flavi-like virus was detected in urine specimens from Pteropus lylei bats from Cambodia (Table 1; Temmam et al., 2019). The viral sequences share 70–80% similarity with JMTV and ALSV over the non-structural proteins NSP1 and NSP2 and 40–55% similarity over proteins encoded by segments 2 and 4. The genome organization is similar to that of JMTV with a single VP1 ORF in segment 2 (Figure 1).
A partial novel jingmenvirus sequence was detected in serum samples collected from Peromyscus leucopus mice in the USA in 2011–2017 (Vandegrift et al., 2020). These sequences were not included in our phylogenies (Figure 5) since they are partial sequences, covering part of NSP2 and most of NSP1, but an alignment showed that these sequences shares under 70% amino acid similarity with JMTV and ALSV.
Two full genome sequences have been identified by metatranscriptomics of environmental samples, namely cattle feces and soil from China (Chen et al., 2022). They have been putatively named Guangdong jingmen-like virus and Hainan jingmen-like virus, respectively. Their host remains to be identified but the presence of jingmenvirus sequences in the environment is another proof that these viruses are ubiquitous and need to be thoroughly characterized.
These jingmenvirus sequences detected from mammals and the environment cluster with the tick-associated jingmenviruses in both non-structural protein-derived phylogenies (Figure 5), which could suggest these viruses follow a classical arbovirus horizontal transmission cycle (Colmant et al., 2022). The optimal models for the phylogenies presented here were selected using the server Smart Model Selection in PhyML (Lefort et al., 2017).
Insect- and other host-associated jingmenviruses
Sequences for 40 other putative segmented flavi-like virus species have been detected from a range of hosts, as described below and listed in Table 2. As mentioned above, the sequences seem to phylogenetically cluster in two separate groups (Figure 5), with, broadly, on the one hand the tick- and vertebrate-associated jingmenviruses, and on the other hand the insect-associated jingmenviruses, in a way that is remindful of vertebrate-infecting and insect-specific flaviviruses being separated in separate clades (Blitvich and Firth, 2015; Vandegrift et al., 2020; Zhang et al., 2020).
Mosquito-associated jingmenviruses
Jingmenviruses have also been found in other arthropods, including mosquitoes. The most studied insect-associated jingmenvirus is Guaico Culex virus (GCXV), which was isolated from six Culex mosquito pools (Cx. declarator, Cx. Coronator, and Cx. interrogator) collected from Trinidad, Peru, and Panama between 2008 and 2013, and subsequently from two Culex spp. mosquito pools collected from Brazil in 2010 (Ladner et al., 2016; Pauvolid-Corrêa et al., 2016; Table 2). The genome sequence was shown to include four to five non-polyadenylated segments, depending on the isolate, comprising conserved termini sequences (Ladner et al., 2016). Segment 1 codes for NSP1, related to the flaviviral NS5, segment 2 codes for NSP2, related to the flaviviral NS3, while segments 3 and 4 each code for three ORFs, most likely structural proteins (VP1 to VP6), and segment 5 codes VP7 (Figures 1, 2; Ladner et al., 2016). Zhang et al. (2021) have confirmed that GCXV NSP2 is an RNA helicase that can unwind RNA structures in both 3 prime and 5 prime directions in an ATP-dependent manner. NSP2 also has an RNA chaperone activity that can remodel structured RNA and facilitate RNA strand annealing, independently of the presence and catalysis of ATP (Zhang et al., 2021).
Viral replication was detected in three mosquito cell lines (C6/36 Ae. albopictus, CT Cx. tarsalis, Aag2 Ae. aegypti) and intrathoracically inoculated female mosquitoes (Ae. albopictus and Cx. quinquefasciatus), but not in tick (ISE6 I. scapularis), sand-fly (LL-5 Lutzomyia longipalpis) or vertebrate (Vero, BHK-21, DF-1) cell lines, or in intracranially inoculated new-born mice. No significant vertical transmission was detected in mosquitoes. Ladner et al. (2016) developed a reverse genetics system to show that replication could occur with and without segment 5. The authors demonstrated that, remarkably, each segment can be packaged separately, in a multicomponent viral system. Purified enveloped virions were found to be 30–35 nm in diameter.
Another Culex-associated jingmenvirus tentatively named Mole Culex virus (MoCV) was isolated from three pools of Culex mosquitoes collected from Ghana in 2016 and caused CPE on C6/36 cells (Amoa-Bosompem et al., 2020). Its genome organization is similar to that of GCXV and the sequences for NSP1 and NSP2 share 80 and 70% amino acid similarity with those of GCXV respectively, while the two viruses share between 50 and 80% amino acid similarity over VP1 to VP6 (VP1: 70%, VP2: 50%, VP3: 70%, VP4: 80%, VP5: 60%, VP6: 70%).
Contrary to their tick-associated counterparts, GCXV and MoCV were found in low prevalence in their arthropod host and there is no evidence of any association with vertebrates.
Other host-associated jingmenvirus sequences
Sequences for jingmenvirus-related putative viruses have been found in various arthropods including insects such as fleas, flies, aphids, crickets, biting midges, drosophilae, plant lice, thrips, as well as mites, scorpions, and crayfish (Webster et al., 2015; Ladner et al., 2016; Shi et al., 2016a,b; Temmam et al., 2016; Guo L. et al., 2020; Kobayashi et al., 2020; Kondo et al., 2020; Thekke-Veetil et al., 2020; Wu et al., 2020; Chiapello et al., 2021; Paraskevopoulou et al., 2021; Colmant et al., 2022). These “insect-associated” jingmenvirus sequences do not contain poly(A) tails, the second segment is bicistronic (VP4 before VP1, see Figure 1) and was reported to be expressed in much higher numbers than the other three segments, and compared to JMTV (Shi et al., 2016b). None of these have been isolated and all remain putative viruses.
Jingmenvirus-related sequences have also been detected worldwide in a range of non-arthropod- and non-vertebrate-derived samples, including nematodes, fungus, and plants (Table 2 and Figure 3) (Tetteh et al., 1999; Callister et al., 2008; Qin et al., 2014; Chiapello et al., 2020; Gaafar and Ziebell, 2020). The classification of some of these short partial sequences as genomic sequences from putative viruses is purely hypothetical, until more segment sequences are elucidated (Matsumura et al., 2017; Rodriguez-Romero et al., 2021). In particular, Qin et al. (2014) identified jingmenvirus-related sequences in previously published sequencing data, based on similarities with JMTV (Tetteh et al., 1999; Callister et al., 2008). These sequences originating from the nematode Toxocara canis were originally attributed to a putative Toxocara canis larvae agent (TCLA). Of note is the fact that human patients were found to be seropositive against recombinant proteins from the putative structural ORFs (Tetteh et al., 1999; Callister et al., 2008).
The phylogeny of insect-associated jingmenvirus sequences does not follow the phylogeny of their putative identified insect hosts, which is different from what has been found for insect-specific flaviviruses (Figure 5; Colmant et al., 2017, 2022). This is corroborated by the presence of crayfish-, scorpion-, fungus- and plant-associated jingmenvirus sequences within the phylogeny. This suggests these putative viruses have not co-evolved with their hosts, which could be due to multiple reasons. The hosts could have been incorrectly assigned, due to data originating from metagenomics studies, which cannot differentiate sequences from an insect or from its previous meal, or a contaminating parasite or fungus (Colmant et al., 2022). Jingmenviruses could also exist in non-arthropod reservoirs, such as plants or fungi, which would allow independent evolution. Remarkably, the complete genome sequence of putative virus Wuhan aphid virus 2, originally detected in two aphid species in China, was detected in peas (Pisum sativum) from France, Germany, and Austria (Shi et al., 2016b; Gaafar and Ziebell, 2020). The novel sequences share between 90 and 97% amino acid identity with the prototype strain, depending on the segment (Gaafar and Ziebell, 2020). This close relationship could suggest that the virus can be transmitted horizontally between aphids and plants. This would support the lack of co-evolution between insect and insect-associated jingmenvirus sequence phylogenies.
Discussion
Taken together, these data clearly show that the vast majority of sequences published are identified as putative jingmenvirus genomes rather than sequences from isolated jingmenviruses. While metagenomics provides essential information on sequence diversity, these efforts should be combined with classical virology to identify and characterize the putative viruses, their hosts, and the interactions between the two. Indeed, Taniguchi wrote in 2019 that “the establishment of JMTV cultivating systems in mammalian cell lines and of a reverse genetics system is the next challenge” to study JMTV, but these milestones have not been reached to date (Taniguchi, 2019). The mechanisms involved in jingmenvirus replication restriction in laboratory models are not yet clear. It was shown that Inopus flavus jingmenvirus 1 can elicit an immune response from its host via the RNA interference pathways (Colmant et al., 2022). However, a non-permissive cell line tested for this virus has a dysfunctional RNAi response, so this mechanism is unlikely to be preventing virus replication in vitro (Brackney et al., 2010).
A stable laboratory replication model for jingmenviruses will be essential to investigate the viral tropism, replication mechanisms, host restriction, and potential pathogenicity in different hosts. The elucidation of the 3D structures, in particular that of the variable structural proteins, would provide insights into the mechanisms involved in binding and entry into host cells, potential immune response evasion mechanisms, and contribute to the development of vaccines and therapies in the context of emergence preparedness. Reverse genetics systems would enable functional studies and help elucidate replication mechanisms as well as reassortment potential. Once these are tools in place, it would also be possible to perform more applied and translational research to uncover potential biotechnological applications of these divergent flavi-like viruses, as it was seen for insect-specific flaviviruses (Hobson-Peters et al., 2019; Hardy et al., 2021; Harrison et al., 2021). In addition to the mechanistic and evolutionary aspects, the technologies mentioned above will be necessary to understand whether jingmenviruses can be responsible for febrile illnesses as it has been reported by some (Emmerich et al., 2018; Jia et al., 2019; Wang et al., 2019a), by fulfilling (virus-adapted) Koch’s postulates (Rivers, 1937; Antonelli and Cutler, 2016).
The mode of transmission of jingmenviruses is another complex aspect of their fundamental characterization that remains to be formally demonstrated. Indeed, jingmenviruses are associated with a range of different hosts. To date, it is considered that jingmenviruses fall into two main clades, insect-associated versus tick- and vertebrate-associated jingmenviruses. The first subgroup includes sequences detected in insects, but also in other arthropods such as scorpions or crayfish, and in plants. The mode of transmission of these putative viruses is unknown so far and could vary wildly from one host to another. It would be interesting to establish whether vertical transmission plays a part, as it was seen for insect-specific flaviviruses (Lutomiah et al., 2007; Bolling et al., 2012; Hall-Mendelin et al., 2016; Auguste et al., 2021; McLean et al., 2021; Peinado et al., 2022). Interestingly, some suggest that the transmission could occur horizontally between insects and plants, even though this remains to be demonstrated. This route of transmission has also been hypothesized for a number of insect viruses from other families, and some plant viruses are known to be transmitted by insects (Cui and Holmes, 2012; Vasilakis et al., 2013; Whitfield et al., 2015; Dietzgen et al., 2016; Dáder et al., 2017; Nunes et al., 2017; Newton et al., 2020).
In the case of tick- and vertebrate-associated jingmenviruses, the main hypothesis seems to be horizontal transmission with a tick vector feeding on a vertebrate host, since the same viruses have been found in both types of samples. However, this has not been demonstrated experimentally. Moreover, horizontal transmission vectored by ticks can be very complex, in particular due to the fact that different tick species can have different types of lifecycle. For example, R. microplus have a monophasic life cycle, which means that they feed as larvae, molt, feed as nymphs, molt, and feed as adults on a single host individual (Leal et al., 2018). In contrast, I. persulcatus have a triphasic life cycle, where they feed on a host as a larva, drop to the ground and molt, seek a new host as nymphs, drop to the ground and molt, and seek a new host again as adults to feed for reproduction (Grigoryeva and Stanyukovich, 2016). We must elucidate the stages at which virus transmission can occur from the tick vector to the vertebrate host. It should be noted that while JMTV and ALSV are mainly associated with R. microplus and I. persulcatus, respectively, their RNA has been detected in a multitude of other tick species and genera, suggesting that they could replicate in and/or be transmitted by ticks with all types of life cycles. The differences in vector lifecycle could, however, result in differences in transmission efficiency.
In addition to the transmission occurring from a tick vector to a vertebrate host, it is essential to study the transmission routes from ticks to ticks. Indeed, the tick-borne flavivirus TBEV can be transmitted from ticks to ticks horizontally by co-feeding on a non-viremic host, or via the sexual route, or vertically via the transovarial route, and is known to be transmitted transtadially from one lifecycle stage to the next (egg, larva, nymph, adult) (Labuda et al., 1993; Nuttall et al., 1994; Danielová et al., 2002; Mansfield et al., 2009; Pettersson et al., 2014). All of these routes of transmission need to be explored for jingmenviruses, first to understand ecology of jingmenviruses and the risk associated with their transmission, but also to elucidate the vector competence of different tick species (Labuda et al., 1993). Studying the transmission cycle of jingmenviruses would also confirm current host assignments, attributed using metagenomics methods, which cannot differentiate between a virus actively infecting an insect or present in its last meal or in a parasiting species.
The discovery of segmented flavi-like viruses was a real breakthrough in virus research as they were the first examples of a segmented genome at least partly derived from an unsegmented genome (Bennett, 2014). The evolutionary costs and benefits of segmentation are poorly understood, and the existence of segmented and unsegmented viruses in the same genus raises questions related to their emergence. Are segmented flavi-like viruses more, or as likely to emerge as pathogens as their unsegmented counterparts? What evolutionary or host-adaptation mechanisms would facilitate their emergence? Could reassortments or recombination events be involved (McDonald et al., 2016; Lucía-Sanz and Manrubia, 2017)? Reassortment happens when different strains or species of segmented viruses exchange genetic material (segments) when co-infecting the same cells to produce virus progeny with novel genome combinations (Vijaykrishna et al., 2015). Recombination does not necessitate the existence of a segmented genome, the genetic exchange takes place during RNA replication that can happen by template switching based on sequence homology (Pérez-Losada et al., 2015). In both cases, the genetic diversity of the replicating viruses is enriched, creating new opportunities for viruses to overcome selective pressures and to adapt to new environments and hosts. In the context of evaluating jingmenvirus potential for emergence, this is particularly relevant, considering that evidence of reassortment and recombination events was found by analyzing phylogenetic clustering of JMTV and ALSV isolates, in all segments, as well as several insect-associated jingmenvirus sequences (Qin et al., 2014; Dinçer et al., 2019; Kholodilov et al., 2020; Paraskevopoulou et al., 2021).
The discovery of GCXV and its characterization as a multicomponent flavi-like virus was a second breakthrough for jingmenvirus-associated virus research since it was the first report of a multicomponent viral system in an animal virus (Attar, 2016). Indeed, GCXV is thought to package each segment individually and to require at least three different particles to form a plaque in infected cells (Ladner et al., 2016). Interestingly, GCXV is the only jingmenvirus shown to be multicomponent, and is one of the only jingmenviruses that has been successfully isolated and cultured stably in laboratory models in vitro and in vivo. The influence of this characteristic on the host restriction displayed by others jingmenviruses in laboratory models should be evaluated. The fact that GCXV branches within the insect-associated jingmenvirus subgroup suggests that it shares a common ancestor with a number of other jingmenviruses. This could suggest that many other jingmenviruses are multicomponent, but this remains to be formally demonstrated. The current knowledge on the structural organization of jingmenviruses is somewhat contradictory, since JMTV was measured to be 70–80 nm, ALSV was found to be 80–100 nm for the Chinese strain and 40 nm with 13 nm smaller particles for the Russian strain, and GCVX was measured at 30–35 nm (Qin et al., 2014; Ladner et al., 2016; Wang et al., 2019a; Kholodilov et al., 2020). The reason for the discrepancy between these sizes is not yet clear, but it could potentially be linked to the multicomponent nature or not of the viral system. What is clear is that these considerations need to be pursued in order to better understand the replication and transmission of jingmenviruses.
In conclusion, jingmenvirus research should be prioritized research topic as they have the potential to emerge as human or veterinary pathogens. Jingmenviruses are found worldwide, including in tick species that are widespread, in close contact with vertebrates, and known to be vectors for other diseases. They can be associated with ticks and vertebrates and could follow a vector-borne transmission cycle. Tick-associated jingmenviruses have been associated with febrile illness in humans. Finally, they are under-studied and therefore the scientific and clinical communities need better preparedness to face a potential future epidemic.
Author contributions
AC, BC, and RC: conceptualization and writing – review and editing. AC: investigation and writing – original draft. BC and RC: resources. AC and BC: visualization. All authors contributed to the article and approved the submitted version.
Funding
This work was supported by the European Virus Archive GLOBAL (EVA-GLOBAL) project that has received funding from the European Union’s Horizon 2020 Research and Innovation Program under grant agreement no. 871029.
Conflict of interest
The authors declare that the research was conducted in the absence of any commercial or financial relationships that could be construed as a potential conflict of interest.
Publisher’s note
All claims expressed in this article are solely those of the authors and do not necessarily represent those of their affiliated organizations, or those of the publisher, the editors and the reviewers. Any product that may be evaluated in this article, or claim that may be made by its manufacturer, is not guaranteed or endorsed by the publisher.
References
Amoa-Bosompem, M., Kobayashi, D., Murota, K., Faizah, A. N., Itokawa, K., Fujita, R., et al. (2020). Entomological Assessment of the Status and Risk of Mosquito-borne Arboviral Transmission in Ghana. Viruses. 12, E147. doi: 10.3390/v12020147
Antonelli, G., and Cutler, S. (2016). Evolution of the Koch postulates: towards a 21st-century understanding of microbial infection. Clin Microbiol Infect 22, 583-4. doi: 10.1016/j.cmi.2016.03.030
Attar, N. (2016). Viral evolution: Animal viruses in pieces. Nat Rev Microbiol 14, 606-7. doi: 10.1038/nrmicro.2016.133
Auguste, A. J., Langsjoen, R. M., Porier, D. L., Erasmus, J. H., Bergren, N. A., Bolling, B. G., et al. (2021). Isolation of a novel insect-specific flavivirus with immunomodulatory effects in vertebrate systems. Virology 562, 50-62. doi: 10.1016/j.virol.2021.07.004
Barré, N., and Uilenberg, G. (2010). Spread of parasites transported with their hosts: case study of two species of cattle tick. Rev Sci Tech 29, 149-60,135-47. doi: 10.20506/rst.29.1.1969
Bennett, H. M. (2014). Split reality for novel tick virus. Nat Rev Microbiol 12, 464-464. doi: 10.1038/nrmicro3297
Blitvich, B. J., and Firth, A. E. (2015). Insect-specific flaviviruses: a systematic review of their discovery, host range, mode of transmission, superinfection exclusion potential and genomic organization. Viruses 7, 1927-59. doi: 10.3390/v7041927
Boelke, M., Kubinski, M., Dümmer, K., Oelke, A., Glanz, J., Gregersen, E., et al. (2020). “Harz mountain virus, a new member of the zoonotic Alongshan virus group, is prevalent in ticks, tick saliva and game animals from Lower Saxony/Germany,” in Proceedings of the 5 Süddeutscher Zeckenkongress: Tagungsband, 46. **City publisher Q.
Bolling, B. G., Olea-Popelka, F. J., Eisen, L., Moore, C. G., and Blair, C. D. (2012). Transmission dynamics of an insect-specific flavivirus in a naturally infected Culex pipiens laboratory colony and effects of co-infection on vector competence for West Nile virus. Virology 427, 90-7. doi: 10.1016/j.virol.2012.02.016
Brackney, D. E., Scott, J. C., Sagawa, F., Woodward, J. E., Miller, N. A., Schilkey, F. D., et al. (2010). C6/36 Aedes albopictus cells have a dysfunctional antiviral RNA interference response. PLoS Negl Trop Dis. 4:e856. doi: 10.1371/journal.pntd.0000856
Bratuleanu, B. E., Temmam, S., Chrétien, D., Regnault, B., Pérot, P., Bouchier, C., et al. (2021). The virome of Rhipicephalus, Dermacentor and Haemaphysalis ticks from Eastern Romania includes novel viruses with potential relevance for public health. Transbound Emerg Dis 69, 1387–1403. doi: 10.1111/tbed.14105
Cai, X. (2020). Alongshan virus isolate HLJ1 NS3-like protein gene, partial cds. Available online at: http://www.ncbi.nlm.nih.gov/nuccore/MT246198.1
Callister, D. M., Winter, A. D., Page, A. P., and Maizels, R. M. (2008). Four abundant novel transcript genes from Toxocara canis with unrelated coding sequences share untranslated region tracts implicated in the control of gene expression. Mol Biochem Parasitol 162, 60-70. doi: 10.1016/j.molbiopara.2008.07.004
Chen, Y. M., Sadiq, S., Tian, J. H., Chen, X., Lin, X. D., Shen, J. J., et al. (2022). RNA viromes from terrestrial sites across China expand environmental viral diversity. Nat. Microbiol. 7, 1312–1323. doi: 10.1038/s41564-022-01180-2
Chitimia-Dobler, L., Lemhöfer, G., Król, N., Bestehorn, M., Dobler, G., and Pfeffer, M. (2019). Repeated isolation of tick-borne encephalitis virus from adult Dermacentor reticulatus ticks in an endemic area in Germany. Parasit Vectors. 12, 90. doi: 10.1186/s13071-019-3346-6
Chiapello, M., Bosco, L., Ciuffo, M., Ottati, S., Salem, N., Rosa, C., et al. (2021). Complexity and Local Specificity of the Virome Associated with Tospovirus-Transmitting Thrips Species. J Virol. 95, e0059721. doi: 10.1128/JVI.00597-21
Chiapello, M., Rodríguez-Romero, J., Ayllón, M. A., and Turina, M. (2020). Analysis of the virome associated to grapevine downy mildew lesions reveals new mycovirus lineages. Virus Evol. 6, veaa058. doi: 10.1093/ve/veaa058
Colmant, A. M. G., Furlong, M. J., and Etebari, K. (2022). Discovery of a Novel Jingmenvirus in Australian Sugarcane Soldier Fly (Inopus flavus) Larvae. Viruses. 14, 1140. doi: 10.3390/v14061140
Colmant, A. M. G., Hobson-Peters, J., Bielefeldt-Ohmann, H., van den Hurk, A. F., Hall-Mendelin, S., Chow, W. K., et al. (2017). A New Clade of Insect-Specific Flaviviruses from Australian Anopheles Mosquitoes Displays Species-Specific Host Restriction. mSphere. 2, e262–e217. doi: 10.1128/mSphere.00262-17
Cui, J., and Holmes, E. C. (2012). Endogenous RNA viruses of plants in insect genomes. Virology 427, 77-9. doi: 10.1016/j.virol.2012.02.014
Dáder, B., Then, C., Berthelot, E., Ducousso, M., Ng, J. C. K., and Drucker, M. (2017). Insect transmission of plant viruses: Multilayered interactions optimize viral propagation. Insect Sci 24, 929-46. doi: 10.1111/1744-7917.12470
Danielová, V., Holubová, J., Pejcoch, M., and Daniel, M. (2002). Potential significance of transovarial transmission in the circulation of tick-borne encephalitis virus. Folia Parasitol (Praha) 49, 323-5. doi: 10.14411/fp.2002.060
Dietzgen, R. G., Mann, K. S., and Johnson, K. N. (2016). Plant Virus-Insect Vector Interactions: Current and Potential Future Research Directions. Viruses. 8, E303. doi: 10.3390/v8110303
Dinçer, E., Hacıoğlu, S., Kar, S., Emanet, N., Brinkmann, A., Nitsche, A., et al. (2019). Survey and Characterization of Jingmen Tick Virus Variants. Viruses. 11, E1071. doi: 10.3390/v11111071
Dinçer, E., Timurkan, M. Ö, Oğuz, B., şahindokuyucu, Ý, şahan, A., Ekinci, M., et al. (2022). Several Tick-Borne Pathogenic Viruses in Circulation in Anatolia, Turkey. Vector Borne Zoonotic Dis 22, 148-58. doi: 10.1089/vbz.2021.0082
Emmerich, P., Jakupi, X., von Possel, R., Berisha, L., Halili, B., Günther, S., et al. (2018). Viral metagenomics, genetic and evolutionary characteristics of Crimean-Congo hemorrhagic fever orthonairovirus in humans, Kosovo. Infect Genet Evol J Mol Epidemiol Evol Genet Infect Dis 65, 6-11. doi: 10.1016/j.meegid.2018.07.010
Estrada-Peña, A., Bouattour, A., Camicas, J. L., Guglielmone, A., Horak, I., Jongejan, F., et al. (2006a). The known distribution and ecological preferences of the tick subgenus Boophilus (Acari: Ixodidae) in Africa and Latin America. Exp Appl Acarol 38, 219-35. doi: 10.1007/s10493-006-0003-5
Estrada-Peña, A., García, Z., and Sánchez, H. F. (2006b). The distribution and ecological preferences of Boophilus microplus (Acari: Ixodidae) in Mexico. Exp Appl Acarol 38, 307-16. doi: 10.1007/s10493-006-7251-2
European Centre for Disease Prevention and Control [ECDC], and European Food Safety Authority [EFSA]. (2020). Ixodes ricinus – current known distribution: May 2020. Solna: European Centre for Disease Prevention and Control [ECDC].
European Centre for Disease Prevention and Control [ECDC], and European Food Safety Authority [EFSA]. (2018). Ixodes persulcatus – current known distribution, May 2018. Solna: European Centre for Disease Prevention and Control [ECDC].
Gaafar, Y. Z. A., and Ziebell, H. (2020). Comparative study on three viral enrichment approaches based on RNA extraction for plant virus/viroid detection using high-throughput sequencing. PloS One. 15:e0237951. doi: 10.1371/journal.pone.0237951
Gao, X., Zhu, K., Wojdyla, J. A., Chen, P., Qin, B., Li, Z., et al. (2020). Crystal structure of the NS3-like helicase from Alongshan virus. IUCrJ 7(Pt 3), 375-82. doi: 10.1107/S2052252520003632
Garry, C. E., and Garry, R. F. (2020). Proteomics Computational Analyses Suggest That the Envelope Glycoproteins of Segmented Jingmen Flavi-Like Viruses are Class II Viral Fusion Proteins (b-Penetrenes) with Mucin-Like Domains. Viruses. 12, E260. doi: 10.3390/v12030260
Giles, J. R., Peterson, A. T., Busch, J. D., Olafson, P. U., Scoles, G. A., Davey, R. B., et al. (2014). Invasive potential of cattle fever ticks in the southern United States. Parasit Vectors. 7, 189. doi: 10.1186/1756-3305-7-189
Gofton, A. W., Blasdell, K. R., Taylor, C., Banks, P. B., Michie, M., Roy-Dufresne, E., et al. (2022). Metatranscriptomic profiling reveals diverse tick-borne bacteria, protozoans and viruses in ticks and wildlife from Australia. Transbound Emerg Dis. doi: 10.1111/tbed.14581
Gómez, G. F., Isaza, J. P., Segura, J. A., Alzate, J. F., and Gutiérrez, L. A. (2020). Metatranscriptomic virome assessment of Rhipicephalus microplus from Colombia. Ticks Tick-Borne Dis. 11, 101426. doi: 10.1016/j.ttbdis.2020.101426
Gondard, M., Temmam, S., Devillers, E., Pinarello, V., Bigot, T., Chrétien, D., et al. (2020). RNA Viruses of Amblyomma variegatum and Rhipicephalus microplus and Cattle Susceptibility in the French Antilles. Viruses. 12, E144. doi: 10.3390/v12020144
Grigoryeva, L. A., and Stanyukovich, M. K. (2016). Life cycle of the taiga tick Ixodes persulcatus (Acari: Ixodidae) in the North-West of Russia. Exp Appl Acarol 69, 347-57. doi: 10.1007/s10493-016-0038-1
Guo, J. J., Lin, X. D., Chen, Y. M., Hao, Z. Y., Wang, Z. X., Yu, Z. M., et al. (2020). Diversity and circulation of Jingmen tick virus in ticks and mammals. Virus Evol. 6, veaa051. doi: 10.1093/ve/veaa051
Guo, L., Lu, X., Liu, X., Li, P., Wu, J., Xing, F., et al. (2020). Meta-Transcriptomic Analysis Reveals the Virome and Viral Genomic Evolution of Medically Important Mites. J Virol 95, JVI.1686–JVI.1620. doi: 10.1128/JVI.01686-20
Hall-Mendelin, S., McLean, B. J., Bielefeldt-Ohmann, H., Hobson-Peters, J., Hall, R. A., and van den Hurk, A. F. (2016). The insect-specific Palm Creek virus modulates West Nile virus infection in and transmission by Australian mosquitoes. Parasit Vectors. 9, 414. doi: 10.1186/s13071-016-1683-2
Hardy, J. M., Newton, N. D., Modhiran, N., Scott, C. A. P., Venugopal, H., Vet, L. J., et al. (2021). A unified route for flavivirus structures uncovers essential pocket factors conserved across pathogenic viruses. Nat Commun. 12, 3266. doi: 10.1038/s41467-021-22773-1
Harrison, J. J., Hobson-Peters, J., Bielefeldt-Ohmann, H., and Hall, R. A. (2021). Chimeric Vaccines Based on Novel Insect-Specific Flaviviruses. Vaccines. 9, 1230. doi: 10.3390/vaccines9111230
Hobson-Peters, J., Harrison, J. J., Watterson, D., Hazlewood, J. E., Vet, L. J., Newton, N. D., et al. (2019). A recombinant platform for flavivirus vaccines and diagnostics using chimeras of a new insect-specific virus. Sci Transl Med. 11, eaax7888. doi: 10.1126/scitranslmed.aax7888
Hu, H. X. (2022). Alongshan virus isolate Liaoning glycoprotein gene, complete cds. Available online at: http://www.ncbi.nlm.nih.gov/nuccore/MZ676705.1
International Committee on Taxonomy of Viruses [ICTV]. (2022). Genus: Flavivirus – Flaviviridae – Positive-sense RNA Viruses – ICTV [Internet]. Available online at: https://talk.ictvonline.org/ictv-reports/ictv_online_report/positive-sense-rna-viruses/w/flaviviridae/360/genus-flavivirus
Jääskeläinen, A. E., Tonteri, E., Sironen, T., Pakarinen, L., Vaheri, A., and Vapalahti, O. (2011). European subtype tick-borne encephalitis virus in Ixodes persulcatus ticks. Emerg Infect Dis 17, 323-5. doi: 10.3201/eid1702.101487
Jia, N., Liu, H. B., Ni, X. B., Bell-Sakyi, L., Zheng, Y. C., Song, J. L., et al. (2019). Emergence of human infection with Jingmen tick virus in China: A retrospective study. EBioMedicine 43, 317-24. doi: 10.1016/j.ebiom.2019.04.004
Kholodilov, I. S., Belova, O. A., Morozkin, E. S., Litov, A. G., Ivannikova, A. Y., Makenov, M. T., et al. (2021). Geographical and Tick-Dependent Distribution of Flavi-Like Alongshan and Yanggou Tick Viruses in Russia. Viruses. 13, 458. doi: 10.3390/v13030458
Kholodilov, I. S., Litov, A. G., Klimentov, A. S., Belova, O. A., Polienko, A. E., Nikitin, N. A., et al. (2020). Isolation and Characterisation of Alongshan Virus in Russia. Viruses. 12, E362. doi: 10.3390/v12040362
Kobayashi, D., Murota, K., Faizah, A. N., Amoa-Bosompem, M., Higa, Y., Hayashi, T., et al. (2020). RNA virome analysis of hematophagous Chironomoidea flies (Diptera: Ceratopogonidae and Simuliidae) collected in Tokyo, Japan. Med Entomol Zool 71, 225-43. doi: 10.7601/mez.71.225
Kobayashi, D., Kuwata, R., Kimura, T., Shimoda, H., Fujita, R., Faizah, A. N., et al. (2021). Detection of Jingmenviruses in Japan with Evidence of Vertical Transmission in Ticks. Viruses. 13, 2547. doi: 10.3390/v13122547
Kondo, H., Fujita, M., Hisano, H., Hyodo, K., Andika, I. B., and Suzuki, N. (2020). Virome Analysis of Aphid Populations That Infest the Barley Field: The Discovery of Two Novel Groups of Nege/Kita-Like Viruses and Other Novel RNA Viruses. Front Microbiol. 11:509. doi: 10.3389/fmicb.2020.00509
Kuivanen, S., Levanov, L., Kareinen, L., Sironen, T., Jääskeläinen, A. J., Plyusnin, I., et al. (2019). Detection of novel tick-borne pathogen, Alongshan virus, in Ixodes ricinus ticks, south-eastern Finland, 2019. Euro Surveill 24, 1900394. doi: 10.2807/1560-7917.ES.2019.24.27.1900394
Labuda, M., Nuttall, P. A., Kozuch, O., Elecková, E., Williams, T., Zuffová, E., et al. (1993). Non-viraemic transmission of tick-borne encephalitis virus: a mechanism for arbovirus survival in nature. Experientia 49, 802-5. doi: 10.1007/BF01923553
Ladner, J. T., Wiley, M. R., Beitzel, B., Auguste, A. J., Dupuis, A. P., Lindquist, M. E., et al. (2016). A Multicomponent Animal Virus Isolated from Mosquitoes. Cell Host Microbe 20, 357-67. doi: 10.1016/j.chom.2016.07.011
Leal, B., Thomas, D. B., and Dearth, R. K. (2018). Population Dynamics of Off-Host Rhipicephalus (Boophilus) microplus (Acari: Ixodidae) Larvae in Response to Habitat and Seasonality in South Texas. Vet Sci. 5, E33. doi: 10.3390/vetsci5020033
Lefort, V., Longueville, J. E., and Gascuel, O. (2017). SMS: Smart Model Selection in PhyML. Mol Biol Evol 34, 2422-4. doi: 10.1093/molbev/msx149
Li, L. J., Ning, N. Z., Zheng, Y. C., Chu, Y. L., Cui, X. M., Zhang, M. Z., et al. (2021). Virome and Blood Meal-Associated Host Responses in Ixodes persulcatus Naturally Fed on Patients. Front Microbiol. 12:728996. doi: 10.3389/fmicb.2021.728996
Liu, L., Dong, H., Chen, H., Zhang, J., Ling, H., Li, Z., et al. (2010). Flavivirus RNA cap methyltransferase: structure, function, and inhibition. Front Biol 5:286-303. doi: 10.1007/s11515-010-0660-y
Lucía-Sanz, A., and Manrubia, S. (2017). Multipartite viruses: adaptive trick or evolutionary treat? NPJ Syst Biol Appl. 3, 34. doi: 10.1038/s41540-017-0035-y
Lutomiah, J. J. L., Mwandawiro, C., Magambo, J., and Sang, R. C. (2007). Infection and vertical transmission of Kamiti river virus in laboratory bred Aedes aegypti mosquitoes. J Insect Sci Online 7, 1-7. doi: 10.1673/031.007.5501
Mansfield, K. L., Johnson, N., Phipps, L. P., Stephenson, J. R., Fooks, A. R., and Solomon, T. (2009). Tick-borne encephalitis virus – a review of an emerging zoonosis. J Gen Virol 90(Pt 8), 1781-94. doi: 10.1099/vir.0.011437-0
Marques, R., Krüger, R. F., Peterson, A. T., de Melo, L. F., Vicenzi, N., and Jiménez-García, D. (2020). Climate change implications for the distribution of the babesiosis and anaplasmosis tick vector, Rhipicephalus (Boophilus) microplus. Vet Res. 51, 81. doi: 10.1186/s13567-020-00802-z
Maruyama, S. R., Castro-Jorge, L. A., Ribeiro, J. M. C., Gardinassi, L. G., Garcia, G. R., Brandão, L. G., et al. (2014). Characterisation of divergent flavivirus NS3 and NS5 protein sequences detected in Rhipicephalus microplus ticks from Brazil. Mem Inst Oswaldo Cruz 109, 38-50. doi: 10.1590/0074-0276130166
Matsumura, E. E., Coletta-Filho, H. D., Nouri, S., Falk, B. W., Nerva, L., Oliveira, T. S., et al. (2017). Deep Sequencing Analysis of RNAs from Citrus Plants Grown in a Citrus Sudden Death-Affected Area Reveals Diverse Known and Putative Novel Viruses. Viruses. 9, E92. doi: 10.3390/v9040092
McDonald, S. M., Nelson, M. I., Turner, P. E., and Patton, J. T. (2016). Reassortment in segmented RNA viruses: mechanisms and outcomes. Nat Rev Microbiol 14, 448-60. doi: 10.1038/nrmicro.2016.46
McLean, B. J., Hall-Mendelin, S., Webb, C. E., Bielefeldt-Ohmann, H., Ritchie, S. A., Hobson-Peters, J., et al. (2021). The Insect-Specific Parramatta River Virus Is Vertically Transmitted by Aedes vigilax Mosquitoes and Suppresses Replication of Pathogenic Flaviviruses In Vitro. Vector Borne Zoonotic Dis 21, 208-15. doi: 10.1089/vbz.2020.2692
Meng, F., Ding, M., Tan, Z., Zhao, Z., Xu, L., Wu, J., et al. (2019). Virome analysis of tick-borne viruses in Heilongjiang Province, China. Ticks Tick-Borne Dis 10, 412-20. doi: 10.1016/j.ttbdis.2018.12.002
National Library Medicine [NIH]. (2022). BLAST: Basic Local Alignment Search Tool. Bethesda, MD: National Library Medicine [NIH].
Newton, N. D., Colmant, A. M. G., O’Brien, C. A., Ledger, E., Paramitha, D., Bielefeldt-Ohmann, H., et al. (2020). Genetic, Morphological and Antigenic Relationships between Mesonivirus Isolates from Australian Mosquitoes and Evidence for Their Horizontal Transmission. Viruses. 12, E1159. doi: 10.3390/v12101159
Niu, G., Pang, Z., and Liu, L. (2022). Jingmen tick virus isolate WS3 segment 1, complete sequence. Available online at: http://www.ncbi.nlm.nih.gov/nuccore/OM459837.1
Nunes, M. R. T., Contreras-Gutierrez, M. A., Guzman, H., Martins, L. C., Barbirato, M. F., Savit, C., et al. (2017). Genetic characterization, molecular epidemiology, and phylogenetic relationships of insect-specific viruses in the taxon Negevirus. Virology 504, 152-67. doi: 10.1016/j.virol.2017.01.022
Nuttall, P. A., Jones, L. D., Labuda, M., and Kaufman, W. R. (1994). Adaptations of arboviruses to ticks. J Med Entomol 31, 1-9.
Ogola, E. O., Kopp, A., Bastos, A. D. S., Slothouwer, I., Marklewitz, M., Omoga, D., et al. (2022). Jingmen Tick Virus in Ticks from Kenya. Viruses. 14, 1041. doi: 10.3390/v14051041
Paraskevopoulou, S., Käfer, S., Zirkel, F., Donath, A., Petersen, M., Liu, S., et al. (2021). Viromics of extant insect orders unveil the evolution of the flavi-like superfamily. Virus Evol. 7, veab030. doi: 10.1093/ve/veab030
Parry, R., James, M. E., and Asgari, S. (2021). Uncovering the Worldwide Diversity and Evolution of the Virome of the Mosquitoes Aedes aegypti and Aedes albopictus. Microorganisms. 9, 1653. doi: 10.3390/microorganisms9081653
Pascoal, J. O., Siqueira, S. M., Maia, R. D. C., Szabó, M. P., and Yokosawa, J. (2019). Detection and molecular characterization of Mogiana tick virus (MGTV) in Rhipicephalus microplus collected from cattle in a savannah area, Uberlândia, Brazil. Ticks Tick-Borne Dis 10, 162-5. doi: 10.1016/j.ttbdis.2018.10.002
Pauvolid-Corrêa, A., Solberg, O., Couto-Lima, D., Nogueira, R. M., Langevin, S., and Komar, N. (2016). Novel Viruses Isolated from Mosquitoes in Pantanal, Brazil. Genome Announc. 4, e1195–e1116. doi: 10.1128/genomeA.01195-16
Peinado, S. A., Aliota, M. T., Blitvich, B. J., and Bartholomay, L. C. (2022). Biology and Transmission Dynamics of Aedes flavivirus. J Med Entomol 59, tjab197. doi: 10.1093/jme/tjab197
Pérez-Losada, M., Arenas, M., Galán, J. C., Palero, F., and González-Candelas, F. (2015). Recombination in viruses: mechanisms, methods of study, and evolutionary consequences. Infect Genet Evol 30, 296-307. doi: 10.1016/j.meegid.2014.12.022
Pettersson, J. H. O., Golovljova, I., Vene, S., and Jaenson, T. G. T. (2014). Prevalence of tick-borne encephalitis virus in Ixodes ricinus ticks in northern Europe with particular reference to Southern Sweden. Parasit Vectors. 7, 102. doi: 10.1186/1756-3305-7-102
Pierson, T. C., and Diamond, M. S. (2020). The continued threat of emerging flaviviruses. Nat Microbiol 5, 796-812. doi: 10.1038/s41564-020-0714-0
Qin, X. C., Shi, M., Tian, J. H., Lin, X. D., Gao, D. Y., He, J. R., et al. (2014).. A tick-borne segmented RNA virus contains genome segments derived from unsegmented viral ancestors. Proc Natl Acad Sci U S A 111, 6744-9. doi: 10.1073/pnas.1324194111
Rivers, T. M. (1937). Viruses and Koch’s Postulates. J Bacteriol 33, 1-12. doi: 10.1128/jb.33.1.1-12.1937
Rodriguez-Romero, J., Chiapello, M., Cordoba, L., Turina, M., and Ayllon, M. A. (2021). The miscellaneous mycovirome associated to the plant pathogenic fungus Erysiphe necator. Available online at: http://www.ncbi.nlm.nih.gov/nuccore/MN558700.1
Sameroff, S., Tokarz, R., Charles, R. A., Jain, K., Oleynik, A., Che, X., et al. (2019). Viral Diversity of Tick Species Parasitizing Cattle and Dogs in Trinidad and Tobago. Sci Rep. 9, 10421. doi: 10.1038/s41598-019-46914-1
Shen, S., Moming, A., Luo, T., Chang, C., Fang, Y., Wang, J., et al. (2019). Viromes of Hyalomma asiaticum, Hyalomma detritum and Dermacentor nuttalli ticks from Xinjiang Uygur Autonomous Region, China. Available online at: http://www.ncbi.nlm.nih.gov/nuccore/MH688529.1
Shi, J. M. (2021). Yanggou tick virus isolate XJ-YGTV-1 segment 4, complete sequence. Available online at: http://www.ncbi.nlm.nih.gov/nuccore/MT248421.1
Shi, J., Shen, S., Wu, H., Zhang, Y., and Deng, F. (2021). Metagenomic Profiling of Viruses Associated with Rhipicephalus microplus Ticks in Yunnan Province, China. Virol Sin 36, 623-35. doi: 10.1007/s12250-020-00319-x
Shi, M., Lin, X. D., Tian, J. H., Chen, L. J., Chen, X., Li, C. X., et al. (2016a). Redefining the invertebrate RNA virosphere. Nature 540, 539-43. doi: 10.1038/nature20167
Shi, M., Lin, X. D., Vasilakis, N., Tian, J. H., Li, C. X., Chen, L. J., et al. (2016b). Divergent Viruses Discovered in Arthropods and Vertebrates Revise the Evolutionary History of the Flaviviridae and Related Viruses. J Virol 90, 659-69. doi: 10.1128/JVI.02036-15
Souza, W. M., Fumagalli, M. J., Torres Carrasco, A. O., Romeiro, M. F., Modha, S., Seki, M. C., et al. (2018). Viral diversity of Rhipicephalus microplus parasitizing cattle in southern Brazil. Sci Rep 8, 16315. doi: 10.1038/s41598-018-34630-1
Stanojević, M., Li, K., Stamenković, G., Ilić, B., Paunović, M., Pešić, B., et al. (2020). Depicting the RNA Virome of Hematophagous Arthropods from Belgrade. Serbia. Viruses. 12, E975. doi: 10.3390/v12090975
Taniguchi, S. (2019). Detection of Jingmen tick virus in human patient specimens: Emergence of a new tick-borne human disease? EBioMedicine 43, 18-9. doi: 10.1016/j.ebiom.2019.04.034
Temmam, S., Monteil-Bouchard, S., Robert, C., Baudoin, J. P., Sambou, M., Aubadie-Ladrix, M., et al. (2016). Characterization of Viral Communities of Biting Midges and Identification of Novel Thogotovirus Species and Rhabdovirus Genus. Viruses. 8, 77. doi: 10.3390/v8030077
Temmam, S., Bigot, T., Chrétien, D., Gondard, M., Pérot, P., Pommelet, V., et al. (2019). Insights into the Host Range, Genetic Diversity, and Geographical Distributionof Jingmenviruses. mSphere. 4, e645–e619. doi: 10.1128/mSphere.00645-19
Ternovoi, V. A., Gladysheva, A. V., Sementsova, A. O., Zaykovskaya, A. V., Volynkina, A. S., Kotenev, E. S., et al. (2020a). Novel segmented RNA Jingmenvirus group isolated from human. Available online at: http://www.ncbi.nlm.nih.gov/nuccore/MN218697.1
Ternovoi, V. A., Protopopova, E. V., Shvalov, A. N., Kartashov, M. Y., Bayandin, R. B., Tregubchak, T. V., et al. (2020b). Complete Coding Genome Sequence for a Novel Multicomponent Kindia Tick Virus Detected from Ticks Collected in Guinea. Biorxiv doi: 10.1101/2020.04.11.036723
Tetteh, K. K., Loukas, A., Tripp, C., and Maizels, R. M. (1999). Identification of abundantly expressed novel and conserved genes from the infective larval stage of Toxocara canis by an expressed sequence tag strategy. Infect Immun 67, 4771-9. doi: 10.1128/IAI.67.9.4771-4779.1999
Thekke-Veetil, T., Lagos-Kutz, D., McCoppin, N. K., Hartman, G. L., Ju, H. K., Lim, H. S., et al. (2020). Soybean Thrips (Thysanoptera: Thripidae) Harbor Highly Diverse Populations of Arthropod, Fungal and Plant Viruses. Viruses. 12, E1376. doi: 10.3390/v12121376
Vandegrift, K. J., Kumar, A., Sharma, H., Murthy, S., Kramer, L. D., Ostfeld, R., et al. (2020). Presence of Segmented Flavivirus Infections in North America. Emerg Infect Dis 26, 1810-7. doi: 10.3201/eid2608.190986
Vasilakis, N., Forrester, N. L., Palacios, G., Nasar, F., Savji, N., Rossi, S. L., et al. (2013). Negevirus: a proposed new taxon of insect-specific viruses with wide geographic distribution. J Virol 87, 2475-88. doi: 10.1128/JVI.00776-12
Vijaykrishna, D., Mukerji, R., and Smith, G. J. D. R. N. A. (2015). Virus Reassortment: An Evolutionary Mechanism for Host Jumps and Immune Evasion. PLoS Pathog. 11:e1004902. doi: 10.1371/journal.ppat.1004902
Villa, E. C., Maruyama, S. R., de Miranda-Santos, I. K. F., Palacios, G., and Ladner, J. T. (2017). Complete Coding Genome Sequence for Mogiana Tick Virus, a Jingmenvirus Isolated from Ticks in Brazil. Genome Announc. 5, e232–e217. doi: 10.1128/genomeA.00232-17
Wang, Z. D., Wang, B., Wei, F., Han, S. Z., Zhang, L., Yang, Z. T., et al. (2019a). A New Segmented Virus Associated with Human Febrile Illness in China. N Engl J Med 380, 2116-25. doi: 10.1056/NEJMoa1805068
Wang, Z. D., Wang, W., Wang, N. N., Qiu, K., Zhang, X., Tana, G., et al. (2019b). Prevalence of the emerging novel Alongshan virus infection in sheep and cattle in Inner Mongolia, northeastern China. Parasit Vectors. 12, 450. doi: 10.1186/s13071-019-3707-1
Webster, C. L., Waldron, F. M., Robertson, S., Crowson, D., Ferrari, G., Quintana, J. F., et al. (2015). The Discovery, Distribution, and Evolution of Viruses Associated with Drosophila melanogaster. PLoS Biol. 13:e1002210. doi: 10.1371/journal.pbio.1002210
Westaway, E. G., Brinton, M. A., Gaidamovich, S. Y. a., Horzinek, M. C., Igarashi, A., Kääriäinen, L., et al. (1985). Flaviviridae. Intervirology 24, 183-92. doi: 10.1159/000149642
Whitfield, A. E., Falk, B. W., and Rotenberg, D. (2015). Insect vector-mediated transmission of plant viruses. Virology 479-480, 278-89. doi: 10.1016/j.virol.2015.03.026
Wu, H., Pang, R., Cheng, T., Xue, L., Zeng, H., Lei, T., et al. (2020). Abundant and Diverse RNA Viruses in Insects Revealed by RNA-Seq Analysis: Ecological and Evolutionary Implications. mSystems. 5, e39–e20. doi: 10.1128/mSystems.00039-20
Xu, L., Guo, M., Hu, B., Zhou, H., Yang, W., Hui, L., et al. (2021). Tick virome diversity in Hubei Province, China, and the influence of host ecology. Virus Evol. 7, veab089. doi: 10.1093/ve/veab089
Xu, X., Bei, J., Xuan, Y., Chen, J., Chen, D., Barker, S. C., et al. (2020). Full-length genome sequence of segmented RNA virus from ticks was obtained using small RNA sequencing data. BMC Genomics. 21:641. doi: 10.1186/s12864-020-07060-5
Yang, Z., and Zhang, W. (2021). Xinjiang tick virus 1 isolate XJO381flaviVG1G2 G1 protein and G2 protein genes, complete cds. Available online at: http://www.ncbi.nlm.nih.gov/nuccore/MZ244285.1
Yu, Z. M., Chen, J. T., Qin, J., Guo, J. J., Li, K., Xu, Q. Y., et al. (2020). Identification and characterization of Jingmen tick virus in rodents from Xinjiang, China. Infect Genet Evol J Mol Epidemiol Evol Genet Infect Dis. 84, 104411. doi: 10.1016/j.meegid.2020.104411
Zhang, X., Wang, N., Wang, Z., and Liu, Q. (2020). The discovery of segmented flaviviruses: implications for viral emergence. Curr Opin Virol 40, 11-8. doi: 10.1016/j.coviro.2020.02.001
Keywords: jingmenvirus, arbovirus, tick-borne disease, segmented flavivirus, emerging virus
Citation: Colmant AMG, Charrel RN and Coutard B (2022) Jingmenviruses: Ubiquitous, understudied, segmented flavi-like viruses. Front. Microbiol. 13:997058. doi: 10.3389/fmicb.2022.997058
Received: 18 July 2022; Accepted: 22 September 2022;
Published: 10 October 2022.
Edited by:
Chengming Wang, Auburn University, United StatesReviewed by:
Ryosuke Fujita, Kyushu University, JapanMie Kobayashi-Ishihara, Keio University School of Medicine, Japan
Minakshi – Prasad, Lala Lajpat Rai University of Veterinary and Animal Sciences, India
Copyright © 2022 Colmant, Charrel and Coutard. This is an open-access article distributed under the terms of the Creative Commons Attribution License (CC BY). The use, distribution or reproduction in other forums is permitted, provided the original author(s) and the copyright owner(s) are credited and that the original publication in this journal is cited, in accordance with accepted academic practice. No use, distribution or reproduction is permitted which does not comply with these terms.
*Correspondence: Agathe M. G. Colmant, YWdhdGhlLmNvbG1hbnRAdW5pdi1hbXUuZnI=