- 1Toxicology and Mycotoxin Research Unit, U.S. National Poultry Research Center, Agricultural Research Service, U.S. Department of Agriculture, Athens, GA, United States
- 2Department of Animal and Dairy Science, University of Georgia, Athens, GA, United States
- 3Department of Poultry Science, University of Georgia, Athens, GA, United States
Fusarium toxins are one of the most common contaminants in poultry diets. The co-occurrence of fumonisins (FUM) and deoxynivalenol (DON), even at a subclinical dose, negatively affects the growth performance, intestinal integrity and induce subclinical necrotic enteritis in broiler chickens. Loss of gut integrity can be expected to alter the intestinal microbiota’s composition. The objective of this study was to identify the effects of combined FUM and DON on the cecal microbiome profile and predicted metabolic functions and a short chain fatty acid profile in broilers challenged with Clostridium perfringens. A total of 240 1 day-old chicks were randomly assigned to two treatments: a control diet and the control diet with 3 mg/kg FUM + 4 mg/kg DON each with eight replications. All the birds were received cocci vaccine at d0. All birds in both treatment groups were challenged with C. perfringens 1 × 108 CFU via feed on d 19 and 20 to achieve 5% mortality. On d 35, the FUM and DON contaminated diet numerically (P = 0.06) decreased the body weight gain (BWG) by 84 g compared to the control group. The bacterial compositions of the cecal contents were analyzed by sequencing the V3–V4 region of the 16S rRNA gene. Overall, microbial richness and diversity increased (P < 0.02) during the studied period (d 21–35). Cecal contents of birds in the FUM + DON group had greater (P < 0.05) microbial evenness and diversity (Shannon index) compared to the control group. FUM + DON exposure decreased (P = 0.001) the relative abundance of Proteobacteria in the cecal content, compared to the control group. The combined FUM + DON significantly increased the relative abundance of the Defluviitaleaceae and Lachnospiraceae families (P < 0.05) but decreased the abundances of the Moraxellaceae and Streptococcaceae (P < 0.05) compared to the control group birds. At the genus level, FUM + DON exposure decreased (P < 0.05) Acinetobacter and Pseudomonas abundance and had a tendency (P = 0.08) to decrease Thermincola abundance compared to the control group. In the ileum, no NE-specific microscopic abnormalities were found; however, the tip of the ileal villi were compromised. The present findings showed that dietary FUM and DON contamination, even at subclinical levels, altered cecal microbial composition, dysregulated intestinal functions, and impaired the gut immune response, potentially predisposing the birds to necrotic enteritis.
Introduction
The chicken’s gut microbial community is complex, and the interactions between gut microbiota significantly affect the physiological, immunological, nutritional (Zhao et al., 2013), and health status (Kumar et al., 2018) of the host. The gut microbial community directs the development of the host immune system by interacting with the host innate immune system and plays a role in the development of T-cell or B-cell repertoires (Pan and Yu, 2014; Kogut and Arsenault, 2016). When compared to mammals, the chicken gastrointestinal tract is shorter, with an average feed passage time of less than 3.5 h (Hughes, 2008). However, slow feed retention times in ceca provide an ideal site for some microbial taxa, such as Bacteroidaceae, Ruminococcaceae, Lachnospiraceae, and Clostridiaceae, to thrive in the cecal microenvironment (Oakley et al., 2014).
Mycotoxins are ubiquitously present in both pre- and post-harvest feed and food commodities. The annual estimated loss due to all mycotoxins in agriculture is approximately $1.4 billion (Vardon et al., 2003). Among Fusarium mycotoxins, fumonisins (FUM) and deoxynivalenol (DON) are commonly present in corn and other poultry feed ingredients (Awad et al., 2013). The epithelial cells in the chicken gastrointestinal tract are the very first target cells for the mycotoxins (Grenier and Applegate, 2013). Mycotoxins cause intestinal damage, activate gut inflammation, and eventually disturb gut microbiota homeostasis (Elmassry et al., 2022). Gut microbiota are considered key players and play a pivotal role in the detoxification of orally ingested mycotoxins (Grenier and Applegate, 2013; Liew et al., 2018).
Mycotoxins reduce chicken growth performance by altering gut bacterial composition and interactions between gut microbes and the host (Robert et al., 2017). Chronic subclinical doses of either FUM or DON alone affect the gut microbiome diversity (Antonissen et al., 2015; Lucke et al., 2018). DON contamination at 10 mg/kg diet decreases cecal species richness and evenness in broiler chickens. Similarly, 12 mg/kg of total FUM contamination altered the composition of the bacterial community and decreased the microbial diversity index in pigs (Burel et al., 2013; Mateos et al., 2018).
The current FDA recommendation is that the maximum concentrations of DON and FUM should be 5 and 50 mg/kg in the finished diet of broiler chickens, respectively. However, these concentrations are for individual mycotoxins, and according to a 2021 mycotoxin survey, 58% of the corn samples tested were positive for more than one mycotoxin (Biomin, 2021). Further, Fusarium species such as F. verticillioides and F. graminearum produce multiple mycotoxins and secondary metabolites, including trichothecenes. As a result, the co-occurrence of multiple mycotoxins in poultry finished diets cause a synergistic detrimental effect on poultry health and adversely affects production performance, immunity, and health status.
Necrotic enteritis is a disease with multiple etiologies. Uncontrolled proliferation of Clostridium perfringens in the gut following coccidial pathogen-induced gut damage has been recognized as a major etiological factor that induces necrotic enteritis in broilers (Cooper and Songer, 2009). We earlier reported that continuous exposure to 3 mg/kg of FUM and 4 mg/kg of DON, at doses below FDA tolerance concentrations, induces subclinical necrotic enteritis in chickens even in the absence of coccidial-induced gut damage but in the presence of C. perfringens inoculation. FUM and DON-induced losses in gut integrity and the presence of C. perfringens resulted in subclinical necrotic enteritis (Shanmugasundaram et al., 2022). The objective of this study was to identify if subclinical doses of combined FUM and DON alter cecal microbial composition or their microbial functions and the SCFA profile in broiler chickens challenged with C. perfringens, and thereby explain the occurrence of subclinical necrotic enteritis in birds exposed to FUM and DON.
Materials and methods
Diet formulation
Two strains of Fusarium, F. graminearum strain PH-1 and F. verticillioides strain M3125, were cultured for DON and FB production, respectively, as mentioned previously (Shanmugasundaram et al., 2022). A non-medicated corn–soybean meal-based mash diet was used as a basal diet (Supplementary Table 1). The feeding experiment was divided into two phases: (1) the starter diet (d0–18) and (2) the finisher diet (d 19–35). The final diets were analyzed to determine the actual content of FB, DON, and other major mycotoxins by LC-MS-MS in Romer Labs (Union, MO, United States). The mycotoxin content of the experimental diets is provided in Supplementary Table 2.
Birds and housing
A total of 240 1 day-old Ross x Ross 708 strain broiler chicks (Aviagen, Blairsville, GA, USA) were weighed individually and randomly distributed to either one of the two treatments: control or FUM (3 mg/kg diet) + DON (4 mg/kg diet) contaminated diet. Each treatment was replicated in eight pens with 15 birds per pen, and the study was terminated on d 35. All animal protocols were approved by the Institutional Animal Care and Use Committee at the Southern Poultry Research Group, Athens, GA, and the birds were raised under the supervision of a licensed poultry veterinarian. The animal care practices and use procedures were followed under the Guide for the Care and Use of Agricultural Animals in Research and Teaching (McGlone, 2020). Day-old broiler chicks were raised in 1.5 × 1.5 m floor pens on new shavings or litter, as is standard industry practice in North America, and were kept at room temperature. The floor pens were equipped with nipple-type waterers and thermostatically controlled heaters. Chicks had ad libitum access to feed and water throughout the experimental period. The mortality of birds was recorded daily. All birds were euthanized by cervical dislocation, which was approved by the American Veterinary Medical Association.
Production performances and C. Perfringens challenge
At 19 and 20 days, birds in both treatment groups were challenged with 1 × 108 CFU of C. perfringens (strain #6) via feed, as described earlier (Hofacre et al., 1998). Prior to the C. perfringens challenge, feed and water were withdrawn from the birds for 4 and 2 h, respectively. On d 21, the mortality was reached 5% in the FUM + DON group; hence, both treatment groups had not received C. perfringens on d 21. Bodyweight and feed intake were measured at 7, 14, 20, 27, and 35 days of age. Average feed intake and body weight gain (BWG) were corrected for mortality when calculating the feed conversion ratio (FCR) for each pen.
Total IgA quantification and C. perfringens specific IgA quantification in bile by ELISA
At 21, 28, and 35 days, bile was collected from one bird in each of the eight floor pens per treatment group (n = 8) and stored at –20°C until further use. The bile samples were collected from gallbladder using a 22 G needle. The bile total IgA quantification was measured by indirect ELISA using a chicken IgA ELISA kit following the manufacture’s instructions (Bethyl Laboratories Inc., Montgomery, TX, USA, Catalog # E33-103). Bile samples were also analyzed for anti-Clostridium IgA content using an ELISA as described previously (Shanmugasundaram et al., 2020). The primary and secondary antibody concentrations were confirmed by using checkerboard titrations with dilutions of bile, coating antigens, and secondary antibodies. The C. perfringens antigen for coating was prepared by six consecutive freeze-thaw cycles of pure culture of C. perfringens followed by mechanical lysing. The pure culture was lysed three times by glass beads of sizes 425–600 μm (Sigma-Aldrich, St. Louis, MO, USA) in a TissueLyser LT (Qiagen, Germany) for 5 min at 50 Hz. The lysed cells were centrifuged at 10,000 × g for 10 min, and the resultant supernatant was collected and stored at –70°C until use. Flat-bottomed 96-well microtitration plates (Microlon 600 High Binding, Greiner, NC, USA) were coated with 100 μL of 10 μg/mL of the antigen diluted in 0.1 M carbonate buffer and incubated overnight at 4°C. The plates were washed three times with PBS-Tween 20. To prevent non-specific binding, wells were blocked with 100 μL of SuperBlock™ (PBS) Blocking Buffer (Thermofisher Scientific, Waltham, MA, USA) and incubated for 30 min at 37°C. For IgA analysis, 100 μL of 1:1600 dilution of the bile in SuperBlock™ (PBS) Blocking Buffer was added to the plates in duplicates and incubated for 1 h at room temperature. After washing, 100 μL of 1:100,000 dilutions of horseradish peroxidase-labeled anti-chicken IgA (Novus Biologicals, Littleton, CO, USA) in SuperBlock™ (PBS) Blocking Buffer was added to each well and incubated for 1 h at room temperature. The plates were washed with PBS-Tween 20, and the substrate 3,3,5,5-tetramethylbenzidine (TMB) solution (eBioscience, San Diego, CA, USA) was added to the wells (100 μL/well). The reaction was stopped after 10 min using 1N HCl (100 μL/well), and the plate was read at 450 nm optical density using a microplate ELISA reader. IgA values were reported as the mean optical density.
Ileum and jejunum histology
On d 21, 28, and 35, approximately 4 cm of jejunal and ileal samples were cut proximally and distally to the Meckel’s diverticulum. Samples were collected from 1 bird/pen (n = 8) from each replication post-Clostridium challenge and stored in buffered formalin. The jejunal and ileal samples were processed using a tissue processor (Sakura Finetek USA, Inc., Torrance, CA, USA) and embedded in paraffin. Paraffin blocks were cut into 5 μm cross-sections and mounted on superfrost slides (Thermo Fisher Scientific, Waltham, MA, USA) and stained with hematoxylin and eosin. Cross-sections were viewed using the cellSens Imaging software (Olympus America, Central Valley, PA, USA) to analyze the morphological differences. The NE microlesion score used in this study has previously been reported by Bortoluzzi et al. (2019). The following NE scores were used in this study: (0)- no necrotic lesions, intact intestinal epithelial cells attached to the lamina propria; (1)- mild single cell enterocyte degeneration/necrosis detachment from the lamina propria; (2)- mild necrosis at villi tips; (3)- moderate necrosis affecting half of the villi; (4)- severe necrosis affecting the entire villi and/or submucosa.
Cecal DNA isolation and 16S rRNA gene amplification
At days 21, 28, and 35, ceca were removed from each of the eight floor pens per treatment group (n = 8), and cecal content was squeezed into 15 ml tubes that were immediately placed on ice before being stored at –20°C until further use. Bacterial genomic DNA was extracted using a combination of mechanical and enzymatic methods. Briefly, approximately 100 mg of cecal content samples were homogenized by using Lysing Matrix E Tube (MP Biomedicals, Solon, OH, USA) with the FastPrep homogenizer (MP Biomedicals, LLC, Irvine, CA, USA) at 6.0 m/s for 40 s, followed by genomic DNA extraction using the QIAamp PowerFecal Pro DNA Kit (Qiagen, Carlsbad, CA, USA) according to the manufacturer’s recommendations. The purified DNA was eluted in a total volume of 75 μL and the DNA concentrations were determined by a Synergy HT Microplate Reader using a Take3 plate (BioTek Instruments, Inc., Winooski, VT, USA). Bacterial genomic DNA samples were sent to LC Sciences (Texas, USA) for sequencing on an Illumina platform. The V3–V4 region of the bacterial 16S rDNA gene was amplified using the S-D-Bact-0341-b-S-17 (5′-CCTACGGGNGGCWGCAG-3′) forward and S-D-Bact-0785-a-A-21 (5′-GACTACHVGGGTATCTAATCC-3′) primer pairs (Klindworth et al., 2013). Each PCR reaction contained the DNA template (12.5 ng), 5 μL forward primer (1 μM), 5 μL reverse primer (1 μM), 12.5 μL 2 × Kapa HiFi Hotstart ready mix (Roche Sequencing and Life Science, Wilmington, MA, USA), and water to a final volume of 25 μL. The DNA was subjected to initial denaturation at 95°C for 3 min. Amplification was then achieved by 25 cycles of denaturation at 95°C for 30 s, annealing at 55°C for 30 s, and extension at 72°C for 30 s. The final extension was at 72°C for 5 min. PCR products were cleaned using AMPure XP magnetic beads (Beckman Coulter Life Sciences, Indianapolis, IN, USA) and 80% ethanol. The PCR products were submitted to another round of PCR to incorporate indexes (Illumina Nextera XT indexing primers, Illumina, Inc., San Diego, CA, USA) into the samples. Each PCR reaction contained 5 μL of each index primer, 25 μL 2 × Kapa HiFi hot start ready-mix (Roche Sequencing and Life Science, Wilmington, MA, USA), and 10 μL water. PCR cycling conditions were as previously described except for the number of amplification cycles, which was set to eight. PCR products were cleaned using AMPure XP beads and 80% ethanol, pooled, and paired ends were sequenced at a read length of 250 nucleotides on a NovaSeq platform (Illumina, Inc., San Diego, CA, USA).
Short chain fatty acid analyses
At d 21, 28, and 35, chicken cecal content samples were analyzed for acetate, propionate, butyrate, valerate, isovalerate, and isobutyrate (SCFA) by using gas chromatography as described earlier (Lourenco et al., 2020). Briefly, the cecal content samples were mixed with three parts water and stored overnight. The samples were thawed and centrifuged at 10,000 × g for 10 min. A total of 1 mL of the supernatant was transferred into a new centrifuge tube, mixed with metaphosphoric acid solution (25% wt/vol) and the samples were frozen overnight. The samples were then thawed and centrifuged at 10,000 × g for 10 min. A total of 1 mL of supernatant was mixed with an internal standard (2-ethylbutyric acid), and ethyl acetate in a 2:1 ratio. After homogenization, samples were allowed to settle for 5 min, and then 0.5 mL of the separated ethyl acetate fraction was analyzed by gas chromatography (Shimadzu GC-2010 Plus, Shimadzu Corporation, Kyoto, Japan) using a flame ionization detector and a capillary column (Zebron ZB-FFAP; 30 m × 0.32 mm × 0.25 μm; Phenomenex Inc., Torrance, CA, USA). The sample injection volume was 1.0 μL, and helium was used as the carrier gas. The column temperature was 110°C and the injector and detector temperatures were 250 and 350°C, respectively. To determine the concentrations of SCFAs in the samples, the peak heights of the samples were compared to those of actual standards.
Bioinformatics analysis
Sequencing data were demultiplexed and converted into FASTQ files, and the paired-end sequences were imported into QIIME 2 (Bolyen et al., 2019). The non-biological nucleotides were removed, and sequences were denoised, dereplicated, and chimera-filtered using DADA2 (Callahan et al., 2016). A pre-trained naive Bayes classifier, trained on the SILVA 138 SSU database, was used to assign taxonomies to the sequences, and reads were classified by taxon using the fitted classifier (Quast et al., 2012). For alpha and beta diversity analyses, all samples were rarefied to a common sequencing depth of 40,000 sequences per sample. The alpha diversity indices computed were the number of observed features (number of ASVs), the Shannon diversity index, and Pielou’s evenness. Beta diversity was assessed using Jaccard distances, applying principal coordinate analysis, and generating visualization plots with EMPeror (Vázquez-Baeza et al., 2013). To make predictions about the metabolic functions of the microbial community, Phylogenetic Investigation of Communities by Reconstruction of Unobserved States (PICRUSt2) was used (Douglas et al., 2020). The MetaCyc pathway database was used to identify the metagenome metabolic functions.
Statistical analysis
A one-way ANOVA was conducted to analyze the effects of the subclinical dose of FUM + DON on dependent variables: body weight gain, FCR, total IgA, C. perfringens-specific IgA, and SCFA, with the pen being considered the experimental unit. When there were significant differences, the means were separated using the Student’s t-test (JMP Pro 15 software, Cary, NC, USA). Relative bacterial abundance and relative predicted microbial function were analyzed in R version 4.2.0 (R Core Team, 2013). Individual taxa and alpha diversity indexes were analyzed using the Kruskal-Wallis H Test. Comparisons were performed for overall differences between the diets and for differences between days, and contrasts between diets within each timepoint were carried out. Results from all statistical tests were corrected by the Benjamini-Hochberg False Discovery Rate (FDR) procedure for multiple comparisons to limit the number of false positive results. The FDR adjusted p-values were statistically significant when P ≤ 0.05; and considered a trend when P-values were between 0.05 and 0.10.
Results
Effect of subclinical dose of FUM + DON on production performances
There were significant (P < 0.05) treatment effects on BWG at d 14 and 21 (Table 1). On d 14 and 21, birds in the FUM + DON treatment group had significantly lower BWG (P < 0.05) compared to the birds in the control group. On 21 days of age, birds in the FUM + DON group had a 55.1 g lower BWG than the birds in the control group. There was a trend on BWG at d 28 (P = 0.07) and d 35 (P = 0.06), and FUM + DON decreased the BWG by 65.8 g on d 28 and 84.3 g on d 35.
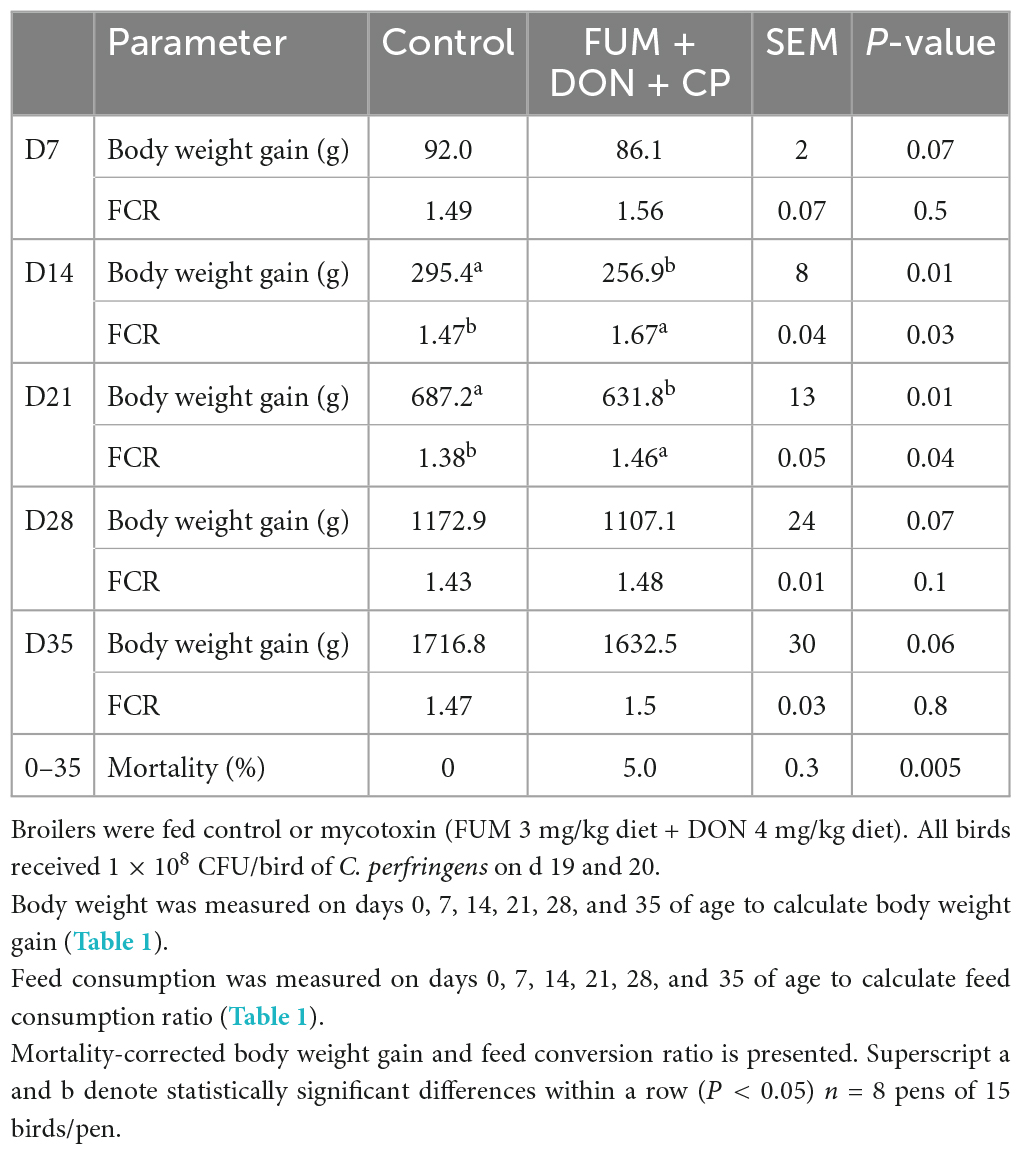
Table 1. Effect of subclinical fumonisins (FUM) and deoxynivalenol (DON) contamination on production parameters.
There were significant (P < 0.05) treatment effects on the FCR on days 14 and 21 (Table 1). On d 14, birds in the FUM + DON group had a 21 point (P = 0.05) higher in FCR compared to the birds in the control group. On d 21, birds in the FUM + DON groups had an eight point significant increase in FCR compared to the birds in the control group.
Effect of subclinical dose of FUM + DON on bile anti-clostridium IgA after C. perfringens challenge
Bile anti-Clostridium-specific IgA antibody concentration were in the detectable range in both the control and treatment groups at d 21 of age (Figure 1A). There were significant differences between the control and FUM + DON treatment groups on anti-Clostridium–specific bile IgA antibody levels at d 28 and 35 after C. perfringens infection. Birds in the FUM + DON group had significantly lower (P < 0.05) antibody titers for anti-Clostridium IgA. There were significant differences between the control and FUM + DON treatment groups on total bile-IgA antibody concentration at d 21, 28, and 35 (Figure 1B). Birds in the FUM + DON group had significantly lower (P < 0.05) antibody concentrations for total bile IgA.
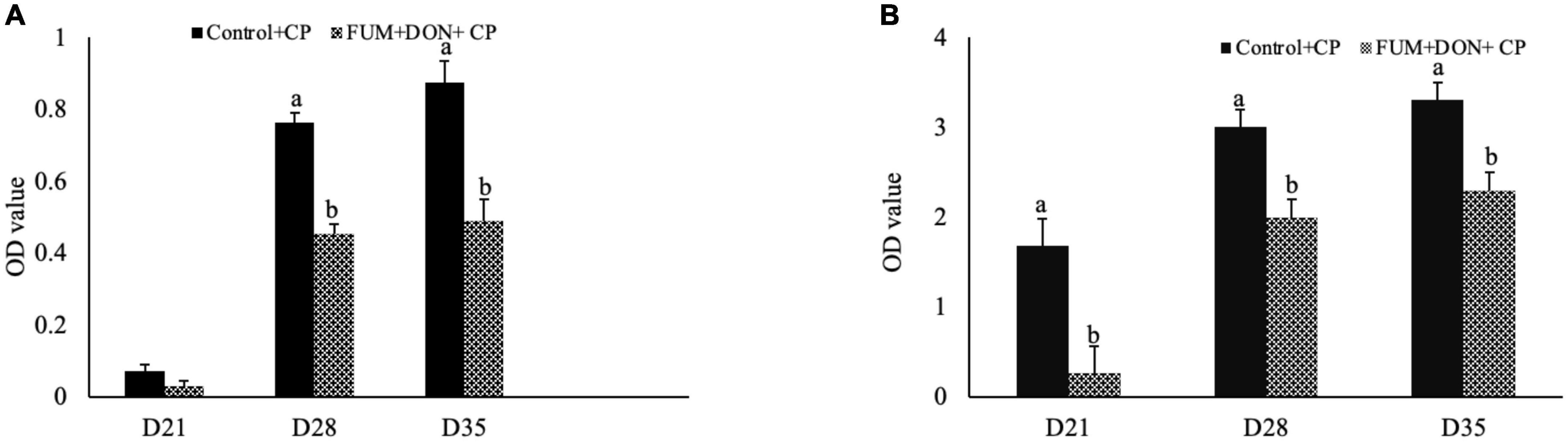
Figure 1. Effect of subclinical fumonisins (FUM) and deoxynivalenol (DON) contamination on bile anti-Clostridium IgA content. Broilers were fed control or mycotoxin (FUM 3 mg/kg diet + DON 4 mg/kg diet). All birds received 1 × 108CFU/bird of C. perfringens on d 19 and 20. At 1, 7, and 14 days after infection, bile was analyzed for anti-C. perfringens specific IgA (A) and total IgA (B) by ELISA and expressed as optical density (OD). Bars (+SEM) with “a” and “b” superscript differ significantly (P < 0.05).
Effect of subclinical dose of FUM + DON on intestinal lesion score and ileum histomorphology
There were significant (p < 0.05) treatment effects on the NE lesion score on day 21 (Table 2). Subclinical dose of FUM + DON increased (p < 0.05) the Wilcoxon/Kruskal–Wallis Score Means for lesion scores compared with the control group on day 21. Additionally combined FUM + DON increased the necrosis at the tip of the villi (Figure 2) and significantly increased the intestinal lesion score (P < 0.05).

Table 2. Effect of subclinical fumonisins (FUM) and deoxynivalenol (DON) contamination on necrotic enteritis lesion scores.
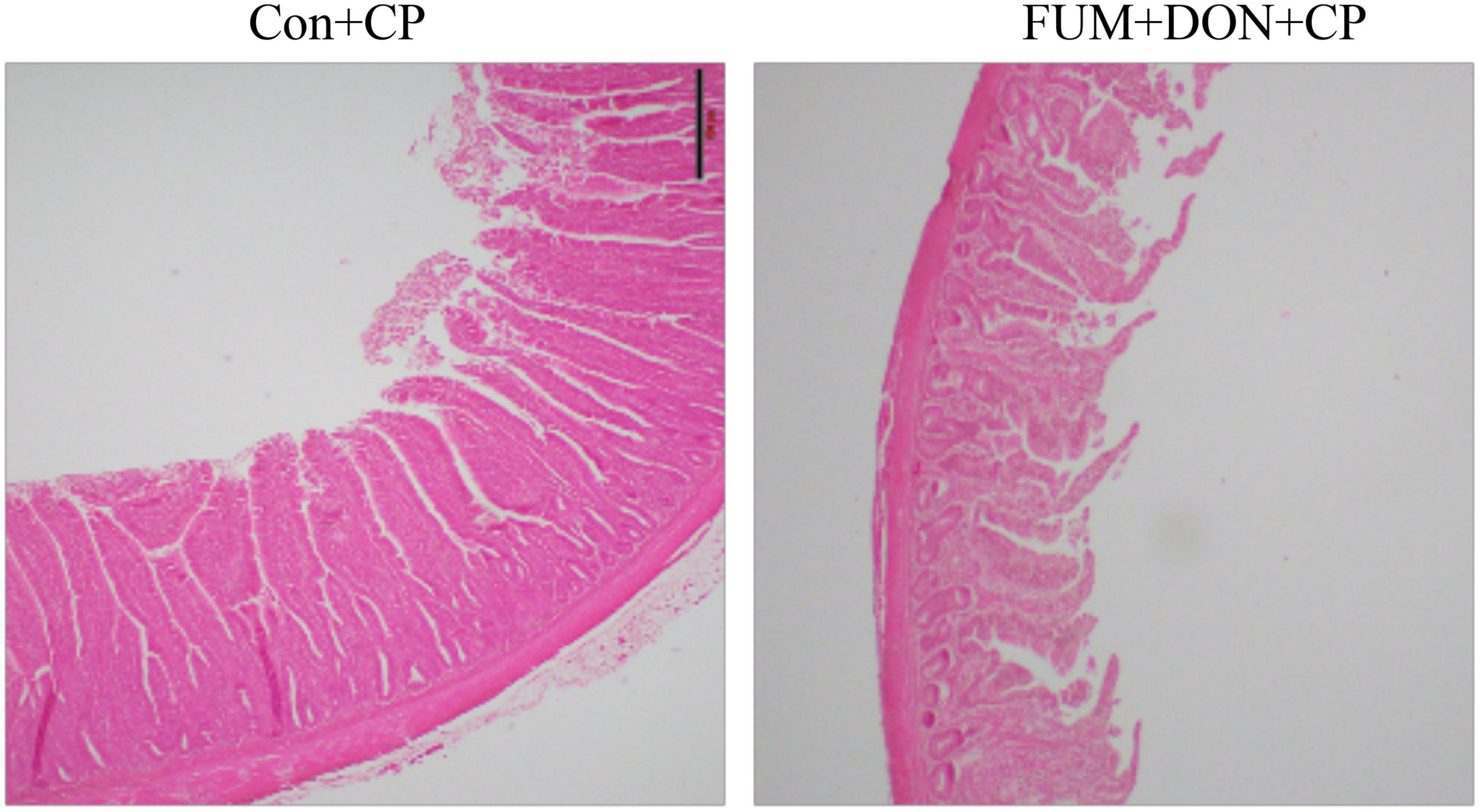
Figure 2. Effect of subclinical fumonisins (FUM) and deoxynivalenol (DON) contamination on Ileum histomorphology at d 21. Broilers were fed control or mycotoxin (FUM 3 mg/kg diet + DON 4 mg/kg diet). All birds received 1 × 108CFU/bird of C. perfringens on d 19 and 20 (n = 8). Haematoxylin and eosin staining; Scale bar = 500 μm.
Effect of subclinical dose of FUM + DON on the cecal microbiota
At the phylum level, Firmicutes (92.0%), Bacteroidota (5.0%), and Proteobacteria (1.7%) were the most predominant phyla in the cecal content (Figure 3). The abundance of Proteobacteria was significantly lower (P = 0.001) in the FUM + DOM group compared to the control. At the family level, the most abundant microbial community was Ruminococcaceae (31.0%), followed by Lachnospiraceae (30.2%), Lactobacillaceae (6.8%), Oscillospiraceae (6.4%), Clostridia UCG-014 (5.4%), and Butyricicoccus (4.3%) (Figure 4). Burkholderiaceae, Carnobacteriaceae, Moraxellaceae, Pseudomonadaceae, Pseudonocardiaceae, Shewanellaceae, Sphingobacteriaceae, Streptococcaceae, and Thermincolaceae relative abundances were all decreased (P 0.05) in the FUM + DON group (Figure 5). Conversely, combined FUM and DON significantly increased the relative abundances of Defluviitaleaceae by 5.3-fold and Lachnospiraceae by 0.15-fold (P = 0.05) compared to the control group on d 35 (Figure 5). At the genus level, the predominating microbial community were Faecalibacterium (19.9%), Ruminococcaceae (13.6%), Lactobacillus (6.8%), and Butyricicoccus (4.2%) (Supplementary Figure 1). On d 35, FUM + DON contamination decreased the Acinetobacter by 0.5-fold and Pseudomonas by 0.8-fold (P ≤ 0.05) in the cecal content, and FUM + DON contamination abundance tended (P = 0.08) to be decreased for Thermincola (Supplementary Figure 2).
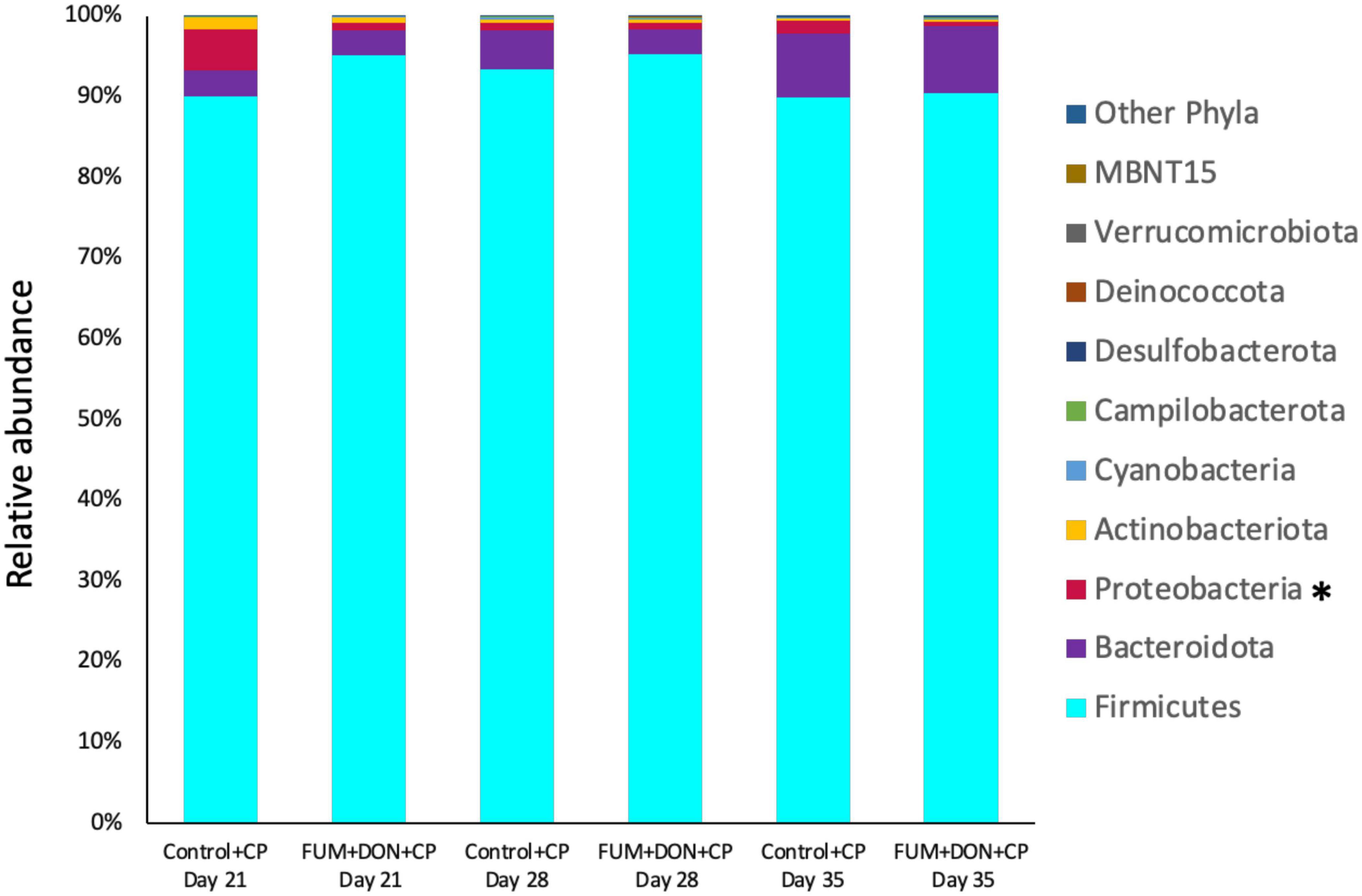
Figure 3. Effect of subclinical fumonisins (FUM) and deoxynivalenol (DON) contamination on relative abundance of microbiome composition at phylum level. Broilers were fed control or mycotoxin (FUM 3 mg/kg diet + DON 4 mg/kg diet). All birds received 1 × 108CFU/bird of C. perfringens on d 19 and 20. Cecal digesta was analyzed for microbiome composition at phyla level using 16S rRNA sequencing at 1, 7, and 14 days post-infection. Asterisk denotes significant difference (*P < 0.05).
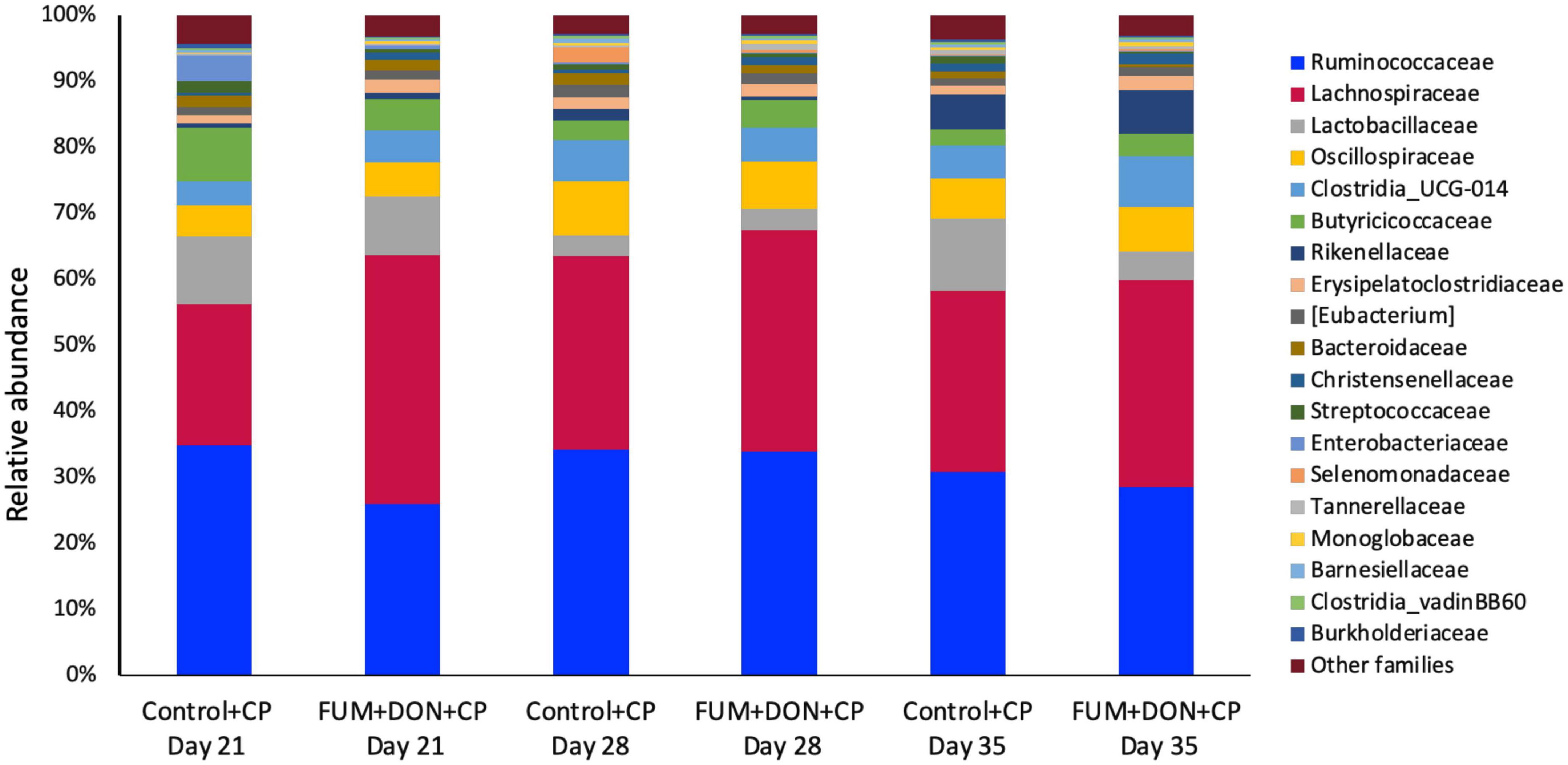
Figure 4. Relative abundance of microbiome at the family level. Broilers were fed control or mycotoxin (FUM 3 mg/kg diet + DON 4 mg/kg diet). All birds received 1 × 108CFU/bird of C. perfringens on d 19 and 20. Cecal digesta was analyzed for relative abundance of microbiome using 16S rRNA sequencing at 1, 7, and 14 days post-infection.
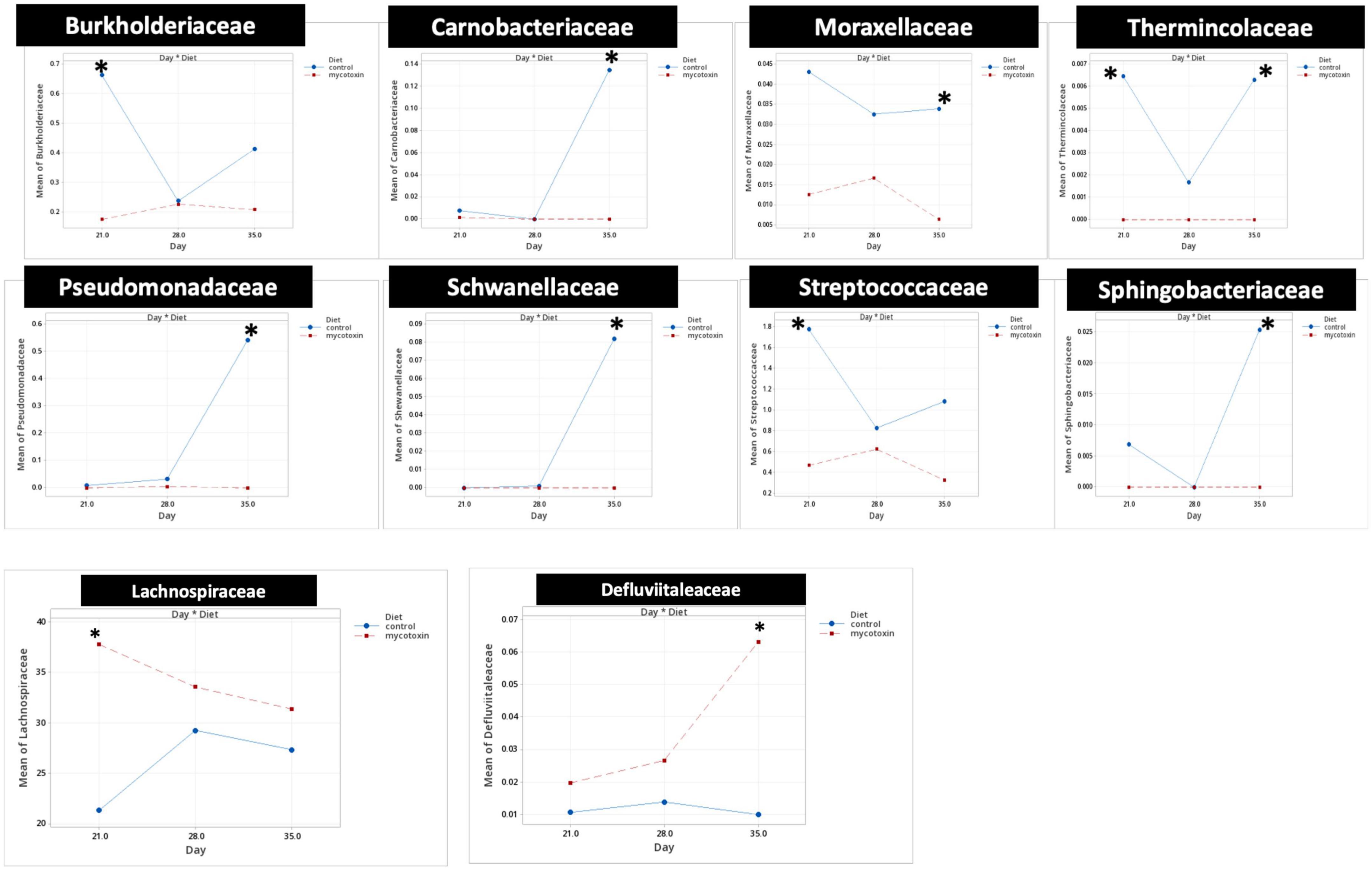
Figure 5. Bacterial families found to be significantly different (P < 0.05) between treatments during the study. Broilers were fed control or mycotoxin (FUM 3 mg/kg diet + DON 4 mg/kg diet). All birds received 1 × 108CFU/bird of C. perfringens on d 19 and 20. Cecal digesta was analyzed for microbiome composition at family level using 16S rRNA sequencing at 1, 7, and 14 days post-infection. Values are presented as least squares means n = 8 (Kruskal-Wallis tests corrected by FDR). Asterisks denote significant difference. *P < 0.05.
For both diets, microbial richness and diversity increased (P ≤ 0.05) from d 21 to 35 (Figure 6). There were no differences between treatments regarding the number of observed features (ASVs) (P = 0.85); however, FUM + DON significantly increased the microbial diversity (P ≤ 0.05) and evenness (P ≤ 0.05) compared to the control groups.

Figure 6. Effect of subclinical fumonisins (FUM) and deoxynivalenol (DON) contamination on Alpha-Diversity indexes: Number of observed features (A), Shannon diversity (B), and Pielou’s evenness index (C). Broilers were fed control or mycotoxin (FUM 3 mg/kg diet + DON 4 mg/kg diet). All birds received 1 × 108 CFU/bird of C. perfringens on d 19 and 20. Cecal digesta was analyzed for microbiome composition using 16S rRNA sequencing at 1, 7, and 14 days post-infection. Significance of day for number of observed features (P < 0.001), Shannon diversity (P = 0.02), Pielou’s evenness (P = 0.29). Significance of diet for number of observed features (P = 0.85), Shannon diversity (P = 0.04), Pielou’s evenness (P = 0.003). Asterisks denote significant difference (*P < 0.05).
The distribution of the stabilization control and the FUM + DON samples were explored via Jaccard distances (Figure 7A) and Principal Coordinates Analysis (PCoA) with Bray–Curtis distance (Figure 7B). As depicted Figure 7, cecal microbiome profile from FUM + DON groups were well-separated on d 21.
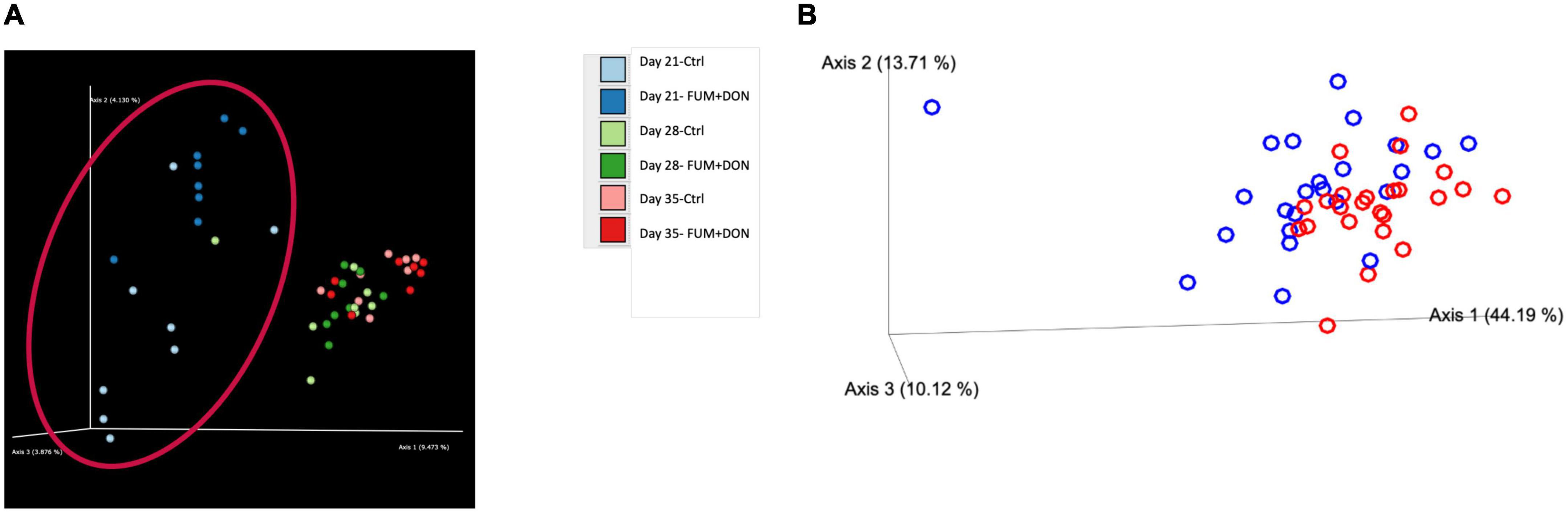
Figure 7. Effect of subclinical fumonisins (FUM) and deoxynivalenol (DON) contamination on (A). Beta-diversity [based on Jaccard distances and (B)]. Principal coordinate analysis with Bray-Curtis dissimilarity of the gut microbial community structure. Broilers were fed control or mycotoxin (FUM 3 mg/kg diet + DON 4 mg/kg diet). All birds received 1 × 108CFU/bird of C. perfringens on d 19 and 20. Cecal digesta was analyzed for microbiome composition using 16S rRNA sequencing at 1, 7, and 14 days post-infection. Significant differences were observed between two groups by PERMANOVA test (P = 0.001).
Effect of subclinical dose of FUM + DON on short chain fatty acids
Subclinical doses of FUM + DON significantly decreased the acetate, propionate butyrate concentration on d 28 (P < 0.05, Figure 8) and iso butyrate concentration on d 35. Additionally, feeding FUM + DON tended to decrease the iso-valerate concentration in the cecal content on d 35 (P = 0.08).
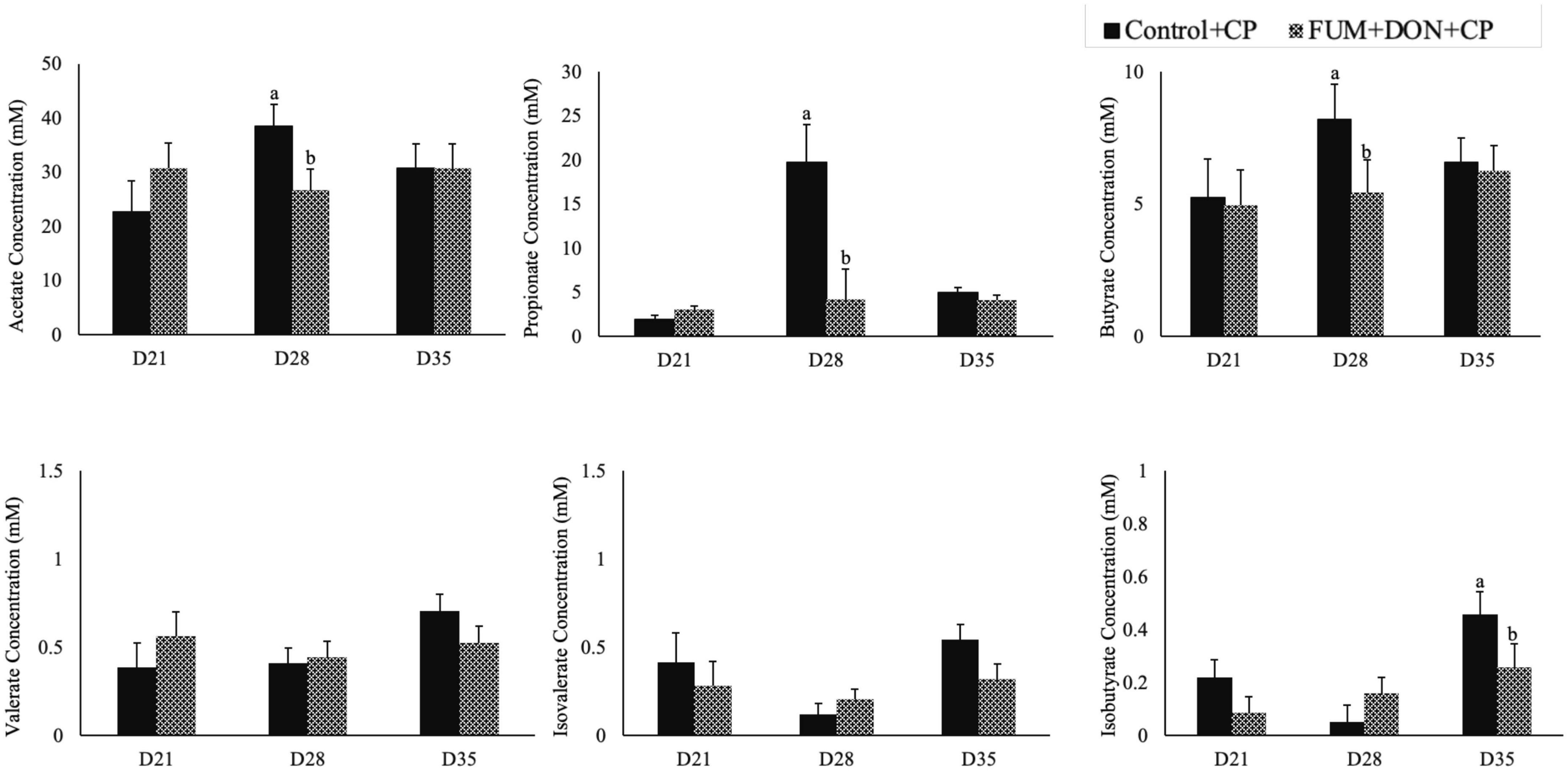
Figure 8. Effect of subclinical fumonisins (FUM) and deoxynivalenol (DON) contamination on of short chain fatty acids (SCFA). Broilers were fed control or mycotoxin (FUM 3 mg/kg diet + DON 4 mg/kg diet). All birds received 1 × 108CFU/bird of C. perfringens on d 19 and 20. Cecal digesta was analyzed SCFA at 1, 7, and 14 days post-infection. Bars (+SEM) with “a” and “b” superscripts indicate statistical differences within an age (P < 0.05).
Correlation analysis
Pearson’s correlation was used to understand the correlation between bacterial abundances, and SCFA concentrations in cecal digesta. Proteobacteria had a positive correlation with isovalerate (r = 0.52) and isobutyrate (r = 0.36) (Figure 9).
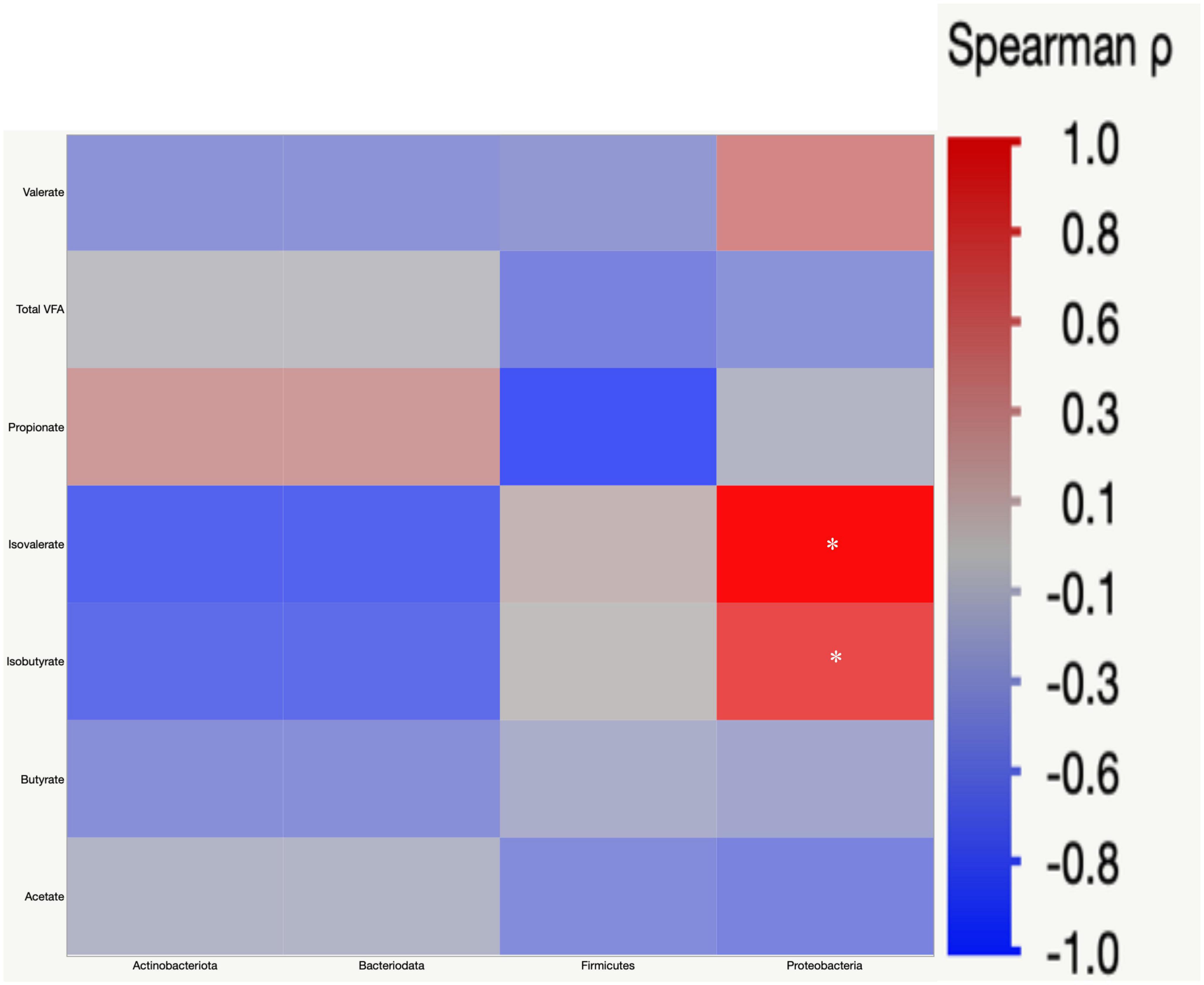
Figure 9. Spearman’s rank correlation matrix of the four main phyla and short chain fatty acids (SCFA). The colors of the scale bar denote the nature of the correlation with one indicating a perfect positive correlation (dark red) and –1 indicating perfect negative correlation (dark blue). Significant correlations are indicated by asterisk (*P < 0.05).
Effect of subclinical dose of FUM + DON on functional metagenome prediction
To evaluate potential modifications in bacterial metabolism driven by dietary FUM + DON, functional metagenome prediction was performed. FUM and DON exposure decreased the metabolic pathways of TCA cycle degradation, fatty acid and lipid biosynthesis, cofactor and vitamin biosynthesis, amino-acid synthesis and secondary metabolites degradation (P ≤ 0.05) (Figure 10).
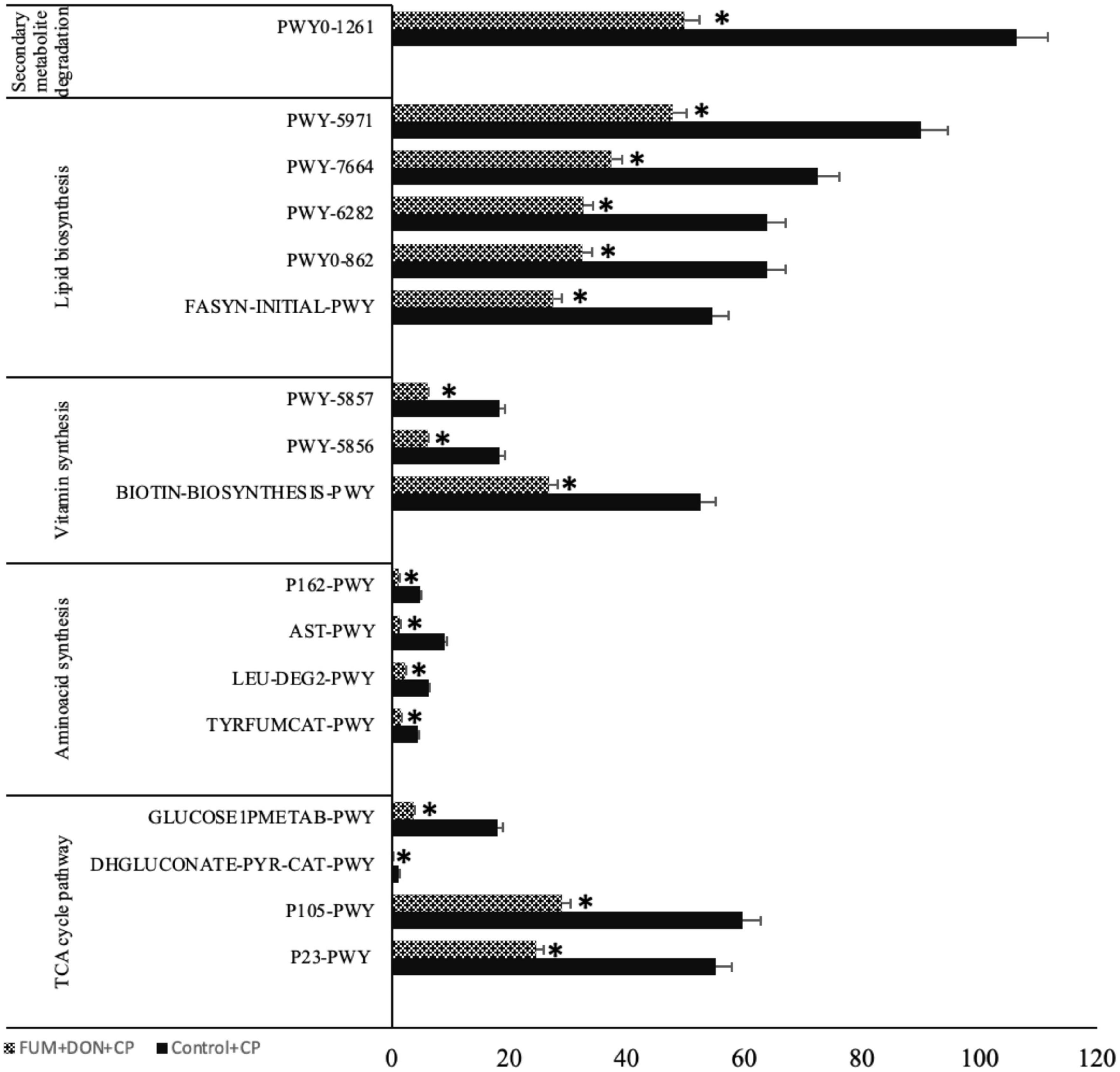
Figure 10. Microbial function analysis: Broilers were fed control or mycotoxin (FUM 3 mg/kg diet + DON 4 mg/kg diet). All birds received 1 × 108CFU/bird of C. perfringens on d 19 and 20. Cecal digesta was analyzed SCFA at 1, 7, and 14 days post-infection. P23-PWY: Reductive TCA cycle I; P105-PWY: TCA cycle IV (2-oxoglutarate decarboxylase); DHGLUCONATE-PYR-CAT-PWY: Glucose degradation (oxidative); GLUCOSE1PMETAB-PWY: Glucose and glucose-1-phosphate degradation; TYRFUMCAT-PWY: L-tyrosine degradation I; LEU-DEG2-PWY: L-leucine degradation I; AST-PWY: L-arginine degradation II (AST pathway) (AST-PWY); P162-PWY: L-glutamate degradation V (via hydroxyglutarate); BIOTIN-BIOSYNTHESIS-PWY: Biotin Biosynthesis I; PWY-5856: Cofactor, Carrier, and Vitamin Biosynthesis; PWY-5857: Cofactor, Carrier, and Vitamin Biosynthesis; PWY-5971: Palmitate Biosynthesis; PWY-6282: Fatty Acid and Lipid Biosynthesis; PWY-7664: Fatty Acid and Lipid Biosynthesis; FASYN-INITIAL-PWY: Fatty Acid and Lipid Biosynthesis; PWY0-1261: Secondary metabolite degradation. Bars (+SEM) with no common superscript differ significantly. Asterisks denote significant difference (*P < 0.05) n = 8.
Discussion
The most frequent mycotoxins found in poultry feed ingredients are FUM and DON (Weaver et al., 2021). Recent research from our lab has demonstrated that broiler productivity and health are adversely impacted by exposure to combined subclinical doses of 3 mg/kg FUM and 4 mg/kg DON (Shanmugasundaram et al., 2022). Because FUM and DON are not efficiently absorbed from the intestine, gut epithelial cells are typically exposed to the ingested toxins at the highest concentration (Grenier et al., 2016). DON and FUM alter intestinal homeostasis and increase the leakage of plasma amino acids into the intestinal lumen, providing a suitable environment for C. perfringens proliferation (Antonissen et al., 2014). As a result, several key intestinal functions, like intestinal barrier functions, are compromised, ultimately leading to subclinical necrotic enteritis (Antonissen et al., 2014, 2015). These changes in intestinal physiological functions affect the gut microbiome and can cause bacterial translocation and the onset of infectious diseases corresponding to changes in the health status of chickens (Hills et al., 2019). However, very limited information is available on the impacts of the chicken gut microbiota on combined mycotoxins and the effects of mycotoxins on the gut microbiome. The objective of this study was to determine if combined FUM and DON cause cecal microbiota dysbiosis and, hence, can explain the induction of subclinical necrotic enteritis.
In the present study, the combined FUM and DON significantly decreased the chicken body weight gain by 39 g and increased the feed conversion ratio by 21 points on d 14. Persistent exposure to multiple mycotoxins at subclinical doses decreased the growth performance of broilers (Young et al., 2007). Similarly, chickens fed contaminated feed containing 20 mg/kg FUM and 1.5 mg/kg DON or 20 mg/kg FUM and 5 mg/kg DON had lower BWG (Guerre, 2020). However, feed contaminated with 5 mg/kg DON alone (Eriksen et al., 2002; Guerre, 2020) or 20 mg/kg (Guerre, 2020) or 50 mg/kg (Zhai et al., 2019) of FUM alone did not negatively affect the body weight gain in broilers. Previous research has identified that DON activates the mitogen-activated protein kinase pathways to inhibit the synthesis of tight junction proteins in enterocytes, resulting in a leaky gut and lower production performance in broilers (Lucioli et al., 2013). In our study, histomorphology analysis of ileum samples on d 21 showed that the combined FUM and DON synergistically damaged the villi structure, resulting in decreased production performance. Villi damage caused by FUM + DON (Figure 3) is likely to reduce nutrient absorption, resulting in decreased production performance (Grenier et al., 2016).
The FUM and DON-induced decrease in body weight was more pronounced at 21 days compared to the decrease in body weight at 35 days. The negative effect of mycotoxins on growth depends on the growth phase of the animals, with younger animals being more susceptible than older animals. A meta-analysis study with pigs identified that including the age of pigs as a covariable improved the estimation of mycotoxin effects and suggested that the increased sensitivity of young animals to mycotoxins is likely because of the reduced detoxification enzymes in the liver of young animals (Andretta et al., 2012). In addition, younger birds have a lower microbial alfa diversity (Lundberg et al., 2021), which can be expected to decrease the ability of gut microbes to detoxify mycotoxin in young broilers.
In broilers, exposure to DON and FUM can compromise mucosal immunity (Bracarense et al., 2012). In the current study, the combined DON and FUM decreased the concentration of total IgA and C. perfringens-specific IgA in bile. These results are in agreement with the previous study: 10 mg DON/kg suppressed the production of antibodies against the infectious bronchitis vaccine (IBV) (Ghareeb et al., 2012) and to the Newcastle disease virus (NDV) in broilers (Danicke et al., 2002). Given that B cells and T cells are highly proliferative cells with high protein turnover, combined FUM and DON can be expected to target B cells and reduce antibody production.
The exposure to a combined subclinical dose of FUM and DON increased microbial evenness and diversity at days 21 and 35. In the current study, FUM and DON increased the alpha-diversity of the cecal microbiome despite having a negative impact on body weight, feed consumption, and intestinal lesion scores. It is possible that some taxa within the bacterial community may have had growth benefits leading to an increase in the diversity of the microbial species, as indicated by the greater Shannon index values in the FUM + DON group. Chickens were exposed to FUM and DON from their very first day of life, thereby modifying the initial bacterial colonization population in the ceca (Lucke et al., 2018). Even though it is believed that a microbiome with a high species diversity is more stable and less susceptible to disease invasion, a bacterial population that is less diversified and more specialized would still be beneficial to the host because it would make better use of the available resources and promote the host’s synthesis of energy (Hibbing et al., 2010). It can therefore be concluded that even though FUM and DON increased the species evenness and diversity in the cecal microbiota, the microbiota as a whole failed to prevent the induction of subclinical necrotic enteritis. Further significant differences on Jaccard dissimilarity and Principal coordinates analysis (PCoA) with Bray-Curtis dissimilarity of the gut microbial community structure indicated that combined FUM + DON, significantly affected the gut microbiome diversity.
Gut microbiota are the host’s first line of defense against toxic metabolites in feed (Apajalahti and Vienola, 2016). The host gut microbes have the ability to detoxify or transform mycotoxins by secreting enzymes into the gut. Most of the transformation involved the hydrolysis of mycotoxins and the formation of metabolites that are less toxic than the parent compound (Coleman et al., 1997). In chickens, gut microbiota can de-epoxidation DON to DOM-1 (Young et al., 2007); however, this mechanism is less efficient in chickens. Further, the recovery rate of high concentrations of fumonisins from the different parts of the intestinal tract and the excreta of birds fed a FB-contaminated diet indicates that chicken gut microbes are less efficient at hydrolyzing the fumonisins (Guerre, 2015; Grenier et al., 2017). Several bacterial species belonging to the phylum Proteobacteria have been recognized for their mycotoxin-detoxifying ability (Zhai et al., 2019). In this study, exposure to FUM and DON decreased (P = 0.001) the relative abundance of Proteobacteria. Similar results were observed when continuous exposure to 10 mg/kg DON decreased the relative abundance of Proteobacteria (Lucke et al., 2018). The decrease in the phyla of Proteobacteria was reflected in the reduction in the relative abundance of Pseudomonadaceae, Moraxellaceae, and Shewanellaceae at day 35. The decrease in the relative abundance of Proteobacteria was accompanied by an increase in Firmicutes at d 21 and 35. Combined FUM and DON increased the Firmicutes to Proteobacteria ratio toward more Firmicutes, indicating that combined doses of FUM and DON, even at subclinical doses, may contribute to intestinal dysbiosis.
The decrease in the relative abundance of the Pseudomonadaceae family was reflected in the decrease in the relative abundance of the Pseudomonas genera at day 35. Pseudomonas spp. are non-pathogenic gut bacteria that play an essential role in producing vitamin B12 (Balabanova et al., 2021) and biodegrading toxic compounds such as mycotoxins (Wang et al., 2020). In this study, FUM and DON exposure decreased the Pseudomonas spp. Thus, the reduction in the relative abundance of the Pseudomonas genus might weaken the gut microbiota’s ability to detoxify toxic mycotoxins, a potential cause of necrotic enteritis.
Fumonisins and DON decreased the relative abundance of the Moraxellaceae family, which includes the genus Acinetobacter. Acinetobacter species produce extracellular enzymes that oxidize zearalenone (Yu et al., 2011) and convert DON into the less harmful 3-epimer-DON metabolite (Wilson et al., 2017). The reduction in the relative abundance of the Acinetobacter genus might increase the broiler’s susceptibility to a wide array of mycotoxins. Similarly, the Shewanellaceae family, which contains Shewanella as the only genus (MacDonell and Colwell, 1985), decreased in broilers exposed to FUM and DON. Shewanella bacteria is a facultative anaerobe that can utilize heavy metals and toxic compounds as electron donors and detoxify heavy metals and toxic compounds (Thorell et al., 2019). Furthermore, Shewanella algae strain YM8 produces antifungal compounds to inhibit the growth of Aspergillus and the production of aflatoxins (Gong et al., 2015). The decrease in the relative abundance of the Shewanellaceae family likely led to a decrease in the mycotoxin-detoxifying ability of the cecal microbiota, thereby leading to the induction of intestinal dysbiosis.
Fumonisins and DON decreased the relative abundance of Streptococcaceae and Carnobacteriaceae. The Streptococcaceae family consists of gram-positive bacteria within the order Lactobacillales (Lory, 2014; Rosenberg et al., 2014). Several members of the Streptococcaceae family are recognized for their probiotic potential (Lory, 2014). Pigs exposed to combined DON and ZEN for seven days had a significantly decreased relative abundance of Streptococcaceae (Le Sciellour et al., 2020). Similarly, mice exposed to 1.5 mg/kg body weight ochratoxin had a decrease in Streptococcaceae relative abundance (Izco et al., 2021). Lactic acid bacteria, including Streptococcus thermophilus, can bind and neutralize FUM and DON (Niderkorn et al., 2006). The reduction in the relative abundance of the Streptococcaceae family can lead to a higher accumulation of mycotoxin, predisposing birds to necrotic enteritis.
Fumonisins and DON exposure increased the relative abundance of the Lachnospiraceae family. However, FB1 or combined DON and ZEN have been identified to decrease Lachnospiraceae and increase Lactobacillaceae (Mateos et al., 2018). The role of the Lachnospiraceae family in gut health is not clearly defined (Vacca et al., 2020). Lachnospiraceae, Lactobacillaceae, and Ruminococcaceae species of Firmicutes hydrolyze starch and other carbohydrates to produce butyrate and other SCFAs, which can be expected to improve gut health in birds. Even though FUM and DON significantly increased the Lachnospiraceae abundance at days 21, 28, and 35, the predicted metagenomic pathway analysis identified that FUM and DON impaired carbohydrate metabolism, potentially contributing to necrotic enteritis. High relative abundances of Lachnospiraceae were positively correlated with impaired glucose and lipid metabolism and decreased SCFA, indicating metabolic disturbance in this study. Bacterial strains belonging to the family Lachnospiraceae are associated with metabolic diseases such as IBD, obesity, and liver diseases (Vacca et al., 2020). However, several members of the Lachnospiraceae family play a significant role in maintaining the health and homeostasis of the gut. Roseburia intestinalis, a member of the Lachnospiraceae family, produces butyrate to improve the integrity of the intestinal barrier (Kasahara et al., 2018). It is likely that the increase in the Lachnospiraceae family in FUM and DON-exposed birds was a gut bacteria-host interaction response to aid the recovery of damaged intestinal cells by producing SCFAs to promote the growth performance.
On d 35, exposure to FUM and DON decreased the relative abundance of the Lactobacillus and Faecalibacterium genera while increasing the Clostridia (uncultured genus level group) UCG-014. In contrast, piglets exposed to fumonisin B1 increased Lactobacillus and decreased Faecalibacterium (Mateos et al., 2018). In pigs, 47% of fumonisins were hydrolyzed into less toxic metabolites, as evidenced by the increased relative abundance of the Lactobacillus genus (Schertz et al., 2018). Lactobacillus produces numerous proteolytic enzymes that play a critical role in the detoxification of mycotoxins. The Lactobacillus metabolizes the FUM by using polysaccharides and peptidoglycans on their bacterial cell wall to bind to the mycotoxins and increase their physical adsorption (Zhao et al., 2016). Therefore, the reduction in the relative abundance of Lactobacillus in chickens is most likely related to their disintegrated binding sites for FUM and, as a result, their decreased ability to metabolize the toxins. The relative abundances of Faecalibacterium and Clostridiaceae indicate that FUM and DON enhanced the inflammation and provided a favorable environment for Clostridia UCG-014 as a consequence of intestinal dysbiosis, and this most likely contributes to subclinical necrotic enteritis.
One of the limitations of this study is that all birds received C. perfringens inoculation, and hence the interaction between C. perfringens and FUM and DON could be a confounding factor in this study. Given that C. perfringens is commensal in the poultry intestine and ubiquitous in the poultry environment, this interaction could represent a real-world scenario in which feed FUM, and DON interact with toxins produced by C. perfringens, exacerbating gut damage.
In conclusion, our data suggest that exposure to FUM and DON alters the cecal microbiota in birds and induces intestinal dysbiosis, and ultimately increasing the risk of subclinical necrotic enteritis. The decrease in the relative abundance of bacterial families involved in the biodegradation of FUM and DON exacerbates the bird’s susceptibility to the toxins produced by C. perfringens. Our study demonstrated that there is a direct relationship between microbiota, mycotoxins, and host performance. Continuous exposure to multiple mycotoxins, even at subclinical doses, had a negative effect on microbiota composition and microbiota-related metabolic functions. FUM and DON, even at a subclinical dose, dysregulate intestinal functions, impairing the gut immune response, which eventually has the potential to predispose the birds to necrotic enteritis. The effect of mycotoxin within the gut varies considerably due to bioavailability, excretion, the effect of the microbiota on mycotoxins, and which segment of the gut is being analyzed. Future studies analyzing the different parts of the gut microbiome profile in response to FUM, and DON exposure would provide a clear picture of the impact of mycotoxins on the gut microbiome.
Data availability statement
The sequencing data is publicly available on the MG-RAST website (www.mg-rast.org) under accession number: mgm4991309.3.
Ethics statement
This animal study was reviewed and approved by Institutional Animal Care and Use Committee at the Southern Poultry Research Group, Athens, GA.
Author contributions
RS: conceptualization, investigation, methodology, data curation, writing—original draft preparation, and funding acquisition. JL: methodology, data curation, and writing—review and editing. WH and MD: methodology and writing—review and editing. TA: writing—review and editing. All authors contributed to the article and approved the submitted version.
Funding
This work was funded by USDA ARS award number: 6040-42000-046-000D.
Acknowledgments
We acknowledge Glenn (USDA) for providing FUM and DON fungal culture material; T. Mitchell (USDA) for quantifying FUM and DON in culture materials; Romer Labs Inc., for independent quantification of mycotoxins in the poultry feed; and A. Pokoo-Aikins (USDA), C. McDonough (USDA), S. Cunningham (USDA), and H. Yeh (USDA) for their assistance in sampling. The authors thank C. Hofacre and M. Jones from (Southern Poultry Research Group) (SPRG) and other SPRG crew members for assistance in animal trials.
Conflict of interest
The authors declare that the research was conducted in the absence of any commercial or financial relationships that could be construed as a potential conflict of interest.
Publisher’s note
All claims expressed in this article are solely those of the authors and do not necessarily represent those of their affiliated organizations, or those of the publisher, the editors and the reviewers. Any product that may be evaluated in this article, or claim that may be made by its manufacturer, is not guaranteed or endorsed by the publisher.
Supplementary material
The Supplementary Material for this article can be found online at: https://www.frontiersin.org/articles/10.3389/fmicb.2023.1106604/full#supplementary-material
References
Andretta, I., Kipper, M., Lehnen, C. R., Hauschild, L., Vale, M. M., and Lovatto, P. A. (2012). Meta-analytical study of productive and nutritional interactions of mycotoxins in growing pigs. Animal 6, 1476–1482. doi: 10.1017/S1751731111002278
Antonissen, G., Croubels, S., Pasmans, F., Ducatelle, R., Eeckhaut, V., Devreese, M., et al. (2015). Fumonisins affect the intestinal microbial homeostasis in broiler chickens, predisposing to necrotic enteritis. Vet. Res. 46:98. doi: 10.1186/s13567-015-0234-8
Antonissen, G., Van Immerseel, F., Pasmans, F., Ducatelle, R., Haesebrouck, F., Timbermont, L., et al. (2014). The mycotoxin deoxynivalenol predisposes for the development of Clostridium perfringens-induced necrotic enteritis in broiler chickens. PLoS One 9:e108775. doi: 10.1371/journal.pone.0108775
Apajalahti, J., and Vienola, K. (2016). Interaction between chicken intestinal microbiota and protein digestion. Anim. Feed Sci. Technol. 221, 323–330.
Awad, W., Ghareeb, K., Böhm, J., and Zentek, J. (2013). The toxicological impacts of the Fusarium mycotoxin, deoxynivalenol, in poultry flocks with special reference to immunotoxicity. Toxins 5, 912–925. doi: 10.3390/toxins5050912
Balabanova, L., Averianova, L., Marchenok, M., Son, O., and Tekutyeva, L. (2021). Microbial and genetic resources for cobalamin (Vitamin B12) biosynthesis: From ecosystems to industrial biotechnology. Int. J. Mol. Sci. 22:4522. doi: 10.3390/ijms22094522
Biomin, (2021). World mycotoxin survey impact 2021. Available online at: https://www.biomin.net/science-hub/world-mycotoxin-survey-impact-2021 (accessed November 22, 2022).
Bolyen, E., Rideout, J. R., Dillon, M. R., Bokulich, N. A., Abnet, C. C., Al-Ghalith, G. A., et al. (2019). Reproducible, interactive, scalable and extensible microbiome data science using QIIME 2. Nat. Biotechnol. 37, 852–857.
Bortoluzzi, C., Lumpkins, B., Mathis, G., França, M., King, W., Graugnard, D., et al. (2019). Zinc source modulates intestinal inflammation and intestinal integrity of broiler chickens challenged with coccidia and Clostridium perfringens. Poult. Sci. 98, 2211–2219. doi: 10.3382/ps/pey587
Bracarense, A.-P. F., Lucioli, J., Grenier, B., Pacheco, G. D., Moll, W.-D., Schatzmayr, G., et al. (2012). Chronic ingestion of deoxynivalenol and fumonisin, alone or in interaction, induces morphological and immunological changes in the intestine of piglets. Br. J. Nutr. 107, 1776–1786. doi: 10.1017/S0007114511004946
Burel, C., Tanguy, M., Guerre, P., Boilletot, E., Cariolet, R., Queguiner, M., et al. (2013). Effect of low dose of fumonisins on pig health: Immune status, intestinal microbiota and sensitivity to Salmonella. Toxins 5, 841–864. doi: 10.3390/toxins5040841
Callahan, B. J., McMurdie, P. J., Rosen, M. J., Han, A. W., Johnson, A. J. A., and Holmes, S. P. (2016). DADA2: High-resolution sample inference from Illumina amplicon data. Nat. Methods 13, 581–583. doi: 10.1038/nmeth.3869
Coleman, J., Blake-Kalff, M., and Davies, E. (1997). Detoxification of xenobiotics by plants: Chemical modification and vacuolar compartmentation. Trends Plant Sci. 2, 144–151.
Cooper, K. K., and Songer, J. G. (2009). Necrotic enteritis in chickens: A paradigm of enteric infection by Clostridium perfringens type A. Anaerobe 15, 55–60. doi: 10.1016/j.anaerobe.2009.01.006
Danicke, S., Ueberschar, K., Halle, I., Matthes, S., Valenta, H., and Flachowsky, G. (2002). Effect of addition of a detoxifying agent to laying hen diets containing uncontaminated or Fusarium toxin-contaminated maize on performance of hens and on carryover of zearalenone. Poult. Sci. 81, 1671–1680. doi: 10.1093/ps/81.11.1671
Douglas, G. M., Maffei, V. J., Zaneveld, J. R., Yurgel, S. N., Brown, J. R., Taylor, C. M., et al. (2020). PICRUSt2 for prediction of metagenome functions. Nat. Biotechnol. 38, 685–688.
Elmassry, M. M., Zayed, A., and Farag, M. A. (2022). Gut homeostasis and microbiota under attack: Impact of the different types of food contaminants on gut health. Crit. Rev. Food Sci. Nutr. 62, 738–763. doi: 10.1080/10408398.2020.1828263
Eriksen, G. S., Pettersson, H., Johnsen, K., and Lindberg, J. (2002). Transformation of trichothecenes in ileal digesta and faeces from pigs. Arch. Anim. Nutr. 56, 263–274. doi: 10.1080/00039420214343
Ghareeb, K., Awad, W., and Böhm, J. (2012). Ameliorative effect of a microbial feed additive on infectious bronchitis virus antibody titer and stress index in broiler chicks fed deoxynivalenol. Poult. Sci. 91, 800–807. doi: 10.3382/ps.2011-01741
Gong, A.-D., Li, H.-P., Shen, L., Zhang, J.-B., Wu, A.-B., He, W.-J., et al. (2015). The Shewanella algae strain YM8 produces volatiles with strong inhibition activity against Aspergillus pathogens and aflatoxins. Front. Microbiol. 6:1091. doi: 10.3389/fmicb.2015.01091
Grenier, B., and Applegate, T. J. (2013). Modulation of intestinal functions following mycotoxin ingestion: Meta-analysis of published experiments in animals. Toxins 5, 396–430. doi: 10.3390/toxins5020396
Grenier, B., Dohnal, I., Shanmugasundaram, R., Eicher, S. D., Selvaraj, R. K., Schatzmayr, G., et al. (2016). Susceptibility of broiler chickens to coccidiosis when fed subclinical doses of deoxynivalenol and fumonisins—special emphasis on the immunological response and the mycotoxin interaction. Toxins 8:231. doi: 10.3390/toxins8080231
Grenier, B., Schwartz-Zimmermann, H., Gruber-Dorninger, C., Dohnal, I., Aleschko, M., Schatzmayr, G., et al. (2017). Enzymatic hydrolysis of fumonisins in the gastrointestinal tract of broiler chickens. Poult. Sci. 96, 4342–4351. doi: 10.3382/ps/pex280
Guerre, P. (2015). Fusariotoxins in avian species: Toxicokinetics, metabolism and persistence in tissues. Toxins 7, 2289–2305. doi: 10.3390/toxins7062289
Hibbing, M. E., Fuqua, C., Parsek, M. R., and Peterson, S. B. (2010). Bacterial competition: Surviving and thriving in the microbial jungle. Nat. Rev. Microbiol. 8, 15–25. doi: 10.1038/nrmicro2259
Hills, R. D. Jr., Pontefract, B. A., Mishcon, H. R., Black, C. A., Sutton, S. C., and Theberge, C. R. (2019). Gut microbiome: Profound implications for diet and disease. Nutrients 11:1613.
Hofacre, C., Froyman, R., Gautrias, B., George, B., Goodwin, M., and Brown, J. (1998). Use of Aviguard and other intestinal bioproducts in experimental Clostridium perfringens-associated necrotizing enteritis in broiler chickens. Avian Dis. 42, 579–584.
Hughes, R. (2008). Relationship between digesta transit time and apparent metabolisable energy value of wheat in chickens. Br. Poult. Sci. 49, 716–720. doi: 10.1080/00071660802449145
Izco, M., Vettorazzi, A., de Toro, M., Sáenz, Y., and Alvarez-Erviti, L. (2021). Oral sub-chronic ochratoxin a exposure induces gut microbiota alterations in mice. Toxins 13:106. doi: 10.3390/toxins13020106
Kasahara, K., Krautkramer, K. A., Org, E., Romano, K. A., Kerby, R. L., Vivas, E. I., et al. (2018). Interactions between Roseburia intestinalis and diet modulate atherogenesis in a murine model. Nat. Microbiol. 3, 1461–1471. doi: 10.1038/s41564-018-0272-x
Klindworth, A., Pruesse, E., Schweer, T., Peplies, J., Quast, C., Horn, M., et al. (2013). Evaluation of general 16S ribosomal RNA gene PCR primers for classical and next-generation sequencing-based diversity studies. Nucleic Acids Res. 41:e1. doi: 10.1093/nar/gks808
Kogut, M. H., and Arsenault, R. J. (2016). Gut health: The new paradigm in food animal production. Lausanne: Frontiers Media SA.
Kumar, S., Chen, C., Indugu, N., Werlang, G. O., Singh, M., Kim, W. K., et al. (2018). Effect of antibiotic withdrawal in feed on chicken gut microbial dynamics, immunity, growth performance and prevalence of foodborne pathogens. PLoS One 13:e0192450. doi: 10.1371/journal.pone.0192450
Le Sciellour, M., Zemb, O., Serviento, A.-M., and Renaudeau, D. (2020). Transient effect of single or repeated acute deoxynivalenol and zearalenone dietary challenge on fecal microbiota composition in female finishing pigs. Animal 14, 2277–2287.
Liew, W.-P.-P., Nurul-Adilah, Z., Than, L. T., and Mohd-Redzwan, S. (2018). The binding efficiency and interaction of Lactobacillus casei Shirota toward aflatoxin B1. Front. Microbiol. 9:1503. doi: 10.3389/fmicb.2018.01503
Lory, S. (2014). “The family Streptococcaceae,” in The prokaryotes, eds E. DeLong, S. Lory, E. Stackebrandt, and F. Thompson (Berlin: Springer), 367–370.
Lourenco, J. M., Kieran, T. J., Seidel, D. S., Glenn, T. C., da Silveira, M. F., Callaway, T. R., et al. (2020). Comparison of the ruminal and fecal microbiotas in beef calves supplemented or not with concentrate. PLoS One 15:e0231533. doi: 10.1371/journal.pone.0231533
Lucioli, J., Pinton, P., Callu, P., Laffitte, J., Grosjean, F., Kolf-Clauw, M., et al. (2013). The food contaminant deoxynivalenol activates the mitogen activated protein kinases in the intestine: Interest of ex vivo models as an alternative to in vivo experiments. Toxicon 66, 31–36. doi: 10.1016/j.toxicon.2013.01.024
Lucke, A., Böhm, J., Zebeli, Q., and Metzler-Zebeli, B. U. (2018). Dietary deoxynivalenol contamination and oral lipopolysaccharide challenge alters the cecal microbiota of broiler chickens. Front. Microbiol. 9:804. doi: 10.3389/fmicb.2018.00804
Lundberg, R., Scharch, C., and Sandvang, D. (2021). The link between broiler flock heterogeneity and cecal microbiome composition. Anim. Microbiome 3:54. doi: 10.1186/s42523-021-00110-7
MacDonell, M., and Colwell, R. (1985). Phylogeny of the vibrionaceae, and recommendation for two new genera, Listonella and Shewanella. Syst. Appl. Microbiol. 6, 171–182.
Mateos, I., Combes, S., Pascal, G., Cauquil, L., Barilly, C., Cossalter, A.-M., et al. (2018). Fumonisin-exposure impairs age-related ecological succession of bacterial species in weaned pig gut microbiota. Toxins 10:230. doi: 10.3390/toxins10060230
McGlone, J. (2020). Guide for the care and use of agricultural animals in research and teaching. Ann Arbor, MI: University of Michigan.
Niderkorn, V., Boudra, H., and Morgavi, D. (2006). Binding of Fusarium mycotoxins by fermentative bacteria in vitro. J. Appl. Microbiol. 101, 849–856.
NRC (1994). Nutrient requirements of poultry, 9th Revised Edn. Washington, DC: National Academy Press, 19–34.
Oakley, B. B., Lillehoj, H. S., Kogut, M. H., Kim, W. K., Maurer, J. J., Pedroso, A., et al. (2014). The chicken gastrointestinal microbiome. FEMS Microbiol. Lett. 360, 100–112.
Pan, D., and Yu, Z. (2014). Intestinal microbiome of poultry and its interaction with host and diet. Gut Microbes 5, 108–119.
Quast, C., Pruesse, E., Yilmaz, P., Gerken, J., Schweer, T., Yarza, P., et al. (2012). The SILVA ribosomal RNA gene database project: Improved data processing and web-based tools. Nucleic Acids Res. 41, D590–D596. doi: 10.1093/nar/gks1219
R Core Team (2013). R: A language and environment for statistical computing. Vienna: R Foundation for Statistical Computing.
Robert, H., Payros, D., Pinton, P., Theodorou, V., Mercier-Bonin, M., and Oswald, I. P. (2017). Impact of mycotoxins on the intestine: Are mucus and microbiota new targets? J. Toxicol. Environ. Health B 20, 249–275. doi: 10.1080/10937404.2017.1326071
Rosenberg, E., DeLong, E. F., Lory, S., Stackebrandt, E., and Thompson, F. (2014). The prokaryotes: Firmicutes and tenericutes. Berlin: Springer.
Schertz, H., Kluess, J., Frahm, J., Schatzmayr, D., Dohnal, I., Bichl, G., et al. (2018). Oral and intravenous fumonisin exposure in pigs—a single-dose treatment experiment evaluating toxicokinetics and detoxification. Toxins 10:150. doi: 10.3390/toxins10040150
Shanmugasundaram, R., Adams, D., Ramirez, S., Murugesan, G., Applegate, T. J., Cunningham, S., et al. (2022). Subclinical doses of combined fumonisins and deoxynivalenol predispose Clostridium perfringens–inoculated broilers to necrotic enteritis. Front. Physiol. 13:934660. doi: 10.3389/fphys.2022.934660
Shanmugasundaram, R., Markazi, A., Mortada, M., Ng, T. T., Applegate, T. J., Bielke, L. R., et al. (2020). Research note: Effect of synbiotic supplementation on caecal Clostridium perfringens load in broiler chickens with different necrotic enteritis challenge models. Poult. Sci. 99, 2452–2458. doi: 10.1016/j.psj.2019.10.081
Thorell, K., Meier-Kolthoff, J. P., Sjöling, Å., and Martín-Rodríguez, A. J. (2019). Whole-genome sequencing redefines Shewanella taxonomy. Front. Microbiol. 10:1861. doi: 10.3389/fmicb.2019.01861
Vacca, M., Celano, G., Calabrese, F. M., Portincasa, P., Gobbetti, M., and De Angelis, M. (2020). The controversial role of human gut lachnospiraceae. Microorganisms 8:573.
Vardon, P., McLaughlin, C., and Nardinelli, C. (eds). (2003). “Mycotoxins: Risks in plant, animal, and human systems,” in Potential economic costs of mycotoxins in the United States (Ames, IA: Council for Agricultural Science and Technology), 136–142.
Vázquez-Baeza, Y., Pirrung, M., Gonzalez, A., and Knight, R. (2013). EMPeror: A tool for visualizing high-throughput microbial community data. Gigascience 2:16. doi: 10.1186/2047-217X-2-16
Wang, Y., Wang, G., Dai, Y., Wang, Y., Lee, Y.-W., Shi, J., et al. (2020). Biodegradation of deoxynivalenol by a novel microbial consortium. Front. Microbiol. 10:2964. doi: 10.3389/fmicb.2019.02964
Weaver, A. C., Weaver, D. M., Adams, N., and Yiannikouris, A. (2021). Co-occurrence of 35 mycotoxins: A seven-year survey of corn grain and corn silage in the United States. Toxins 13:516. doi: 10.3390/toxins13080516
Wilson, N. M., McMaster, N., Gantulga, D., Soyars, C., McCormick, S. P., Knott, K., et al. (2017). Modification of the mycotoxin deoxynivalenol using microorganisms isolated from environmental samples. Toxins 9:141. doi: 10.3390/toxins9040141
Young, J. C., Zhou, T., Yu, H., Zhu, H., and Gong, J. (2007). Degradation of trichothecene mycotoxins by chicken intestinal microbes. Food Chem. Toxicol. 45, 136–143.
Yu, Y., Qiu, L., Wu, H., Tang, Y., Lai, F., and Yu, Y. (2011). Oxidation of zearalenone by extracellular enzymes from Acinetobacter sp. SM04 into smaller estrogenic products. World J. Microbiol. Biotechnol. 27, 2675–2681.
Zhai, Y., Zhong, L., Gao, H., Lu, Z., Bie, X., Zhao, H., et al. (2019). Detoxification of deoxynivalenol by a mixed culture of soil bacteria with 3-epi-deoxynivalenol as the main intermediate. Front. Microbiol. 10:2172. doi: 10.3389/fmicb.2019.02172
Zhao, H., Wang, X., Zhang, J., Zhang, J., and Zhang, B. (2016). The mechanism of Lactobacillus strains for their ability to remove fumonisins B1 and B2. Food Chem. Toxicol. 97, 40–46. doi: 10.1016/j.fct.2016.08.028
Keywords: fumonisins (FB1, FB2), deoxynivalenol (DON), cecal microbiome, Clostridium perfringens, necrotic enteritis, poultry
Citation: Shanmugasundaram R, Lourenco J, Hakeem WA, Dycus MM and Applegate TJ (2023) Subclinical doses of dietary fumonisins and deoxynivalenol cause cecal microbiota dysbiosis in broiler chickens challenged with Clostridium perfringens. Front. Microbiol. 14:1106604. doi: 10.3389/fmicb.2023.1106604
Received: 23 November 2022; Accepted: 13 March 2023;
Published: 03 April 2023.
Edited by:
Aldo Corsetti, University of Teramo, ItalyReviewed by:
Gita Cherian, Oregon State University, United StatesBrooke Humphrey, Phibro Animal Health Corporation, United States
Copyright © 2023 Shanmugasundaram, Lourenco, Hakeem, Dycus and Applegate. This is an open-access article distributed under the terms of the Creative Commons Attribution License (CC BY). The use, distribution or reproduction in other forums is permitted, provided the original author(s) and the copyright owner(s) are credited and that the original publication in this journal is cited, in accordance with accepted academic practice. No use, distribution or reproduction is permitted which does not comply with these terms.
*Correspondence: Revathi Shanmugasundaram, cmV2YXRoaS5zaGFuQHVzZGEuZ292; Jeferson Lourenco, amVmYW9AdWdhLmVkdQ==