- 1Key Laboratory of Feed Biotechnology of Ministry of Agriculture and Rural Affairs, Institute of Feed Research, Chinese Academy of Agricultural Sciences (CAAS), Beijing, China
- 2National Engineering Research Center of Biological Feed, Beijin, China
- 3Chengde Academy of Agricultural and Forestry Sciences, Chengde, China
Bacillus licheniformis is considered a potential alternative to antibiotic growth promoters of animal growth and health. However, the effects of Bacillus licheniformis on the foregut and hindgut microbiota, and their relationships with nutrient digestion and health, in broiler chickens remain unclear. In this study, we aimed to identify the effects of Bacillus licheniformis BCG on intestinal digestion and absorption, tight junctions, inflammation, and the fore- and hind-gut microbiota. We randomly assigned 240 1-day-old male AA broilers into three treatment groups: CT (basal diet), BCG1 (basal diet + 1.0 × 108 CFU/kg B. licheniformis BCG), and BCG2 (basal diet + 1.0 × 109 CFU/kg B. licheniformis BCG). On day 42, the jejunal and ileal chyme and mucosa were subjected to analysis of digestive enzyme activity, nutrient transporters, tight junctions, and signaling molecules associated with inflammation. The ileal and cecal chyme were subjected to microbiota analysis. Compared with the CT group, the B. licheniformis BCG group showed significantly greater jejunal and ileal α-amylase, maltase, and sucrase activity; moreover, the α-amylase activity in the BCG2 group was higher than that in the BCG1 group (P < 0.05). The transcript abundance of FABP-1 and FATP-1 in the BCG2 group was significantly greater than that in the CT and BCG1 groups, and the GLUT-2 and LAT-1 relative mRNA levels were greater in the BCG2 group than the CT group (P < 0.05). Dietary B. licheniformis BCG resulted in significantly higher ileal occludin, and lower IL-8 and TLR-4 mRNA levels than observed in the CT group (P < 0.05). B. licheniformis BCG supplementation significantly decreased bacterial community richness and diversity in the ileum (P < 0.05). Dietary B. licheniformis BCG shaped the ileac microbiota by increasing the prevalence of f_Sphingomonadaceae, Sphingomonas, and Limosilactobacillus, and contributed to nutrient digestion and absorption; moreover, it enhanced the intestinal barrier by increasing the prevalence of f_Lactobacillaceae, Lactobacillus, and Limosilactobacillus. Dietary B. licheniformis BCG decreased microbial community diversity by diminishing Desulfovibrio, Alistipes, Campylobacter, Vibrio, Streptococcus, and Escherichia coli-Shigella levels, and down-regulating inflammatory associated molecule expression. Therefore, dietary B. licheniformis BCG contributed to digestion and absorption of nutrients, enhanced the intestinal physical barrier, and decreased intestinal inflammation in broilers by decreasing microbial diversity and optimizing the microbiota structure.
1. Introduction
In poultry production, broiler chickens are generally subjected to harsh and stressful conditions, particularly when they are reared at high stocking density, thus resulting in host stress and immune dysfunction (Kridtayopas et al., 2019). This subhealth status decreases the growth potential and causes intestinal dysfunction in broilers, and increases the economic costs of rearing. The intestines not only digest and absorb nutrients, but also are the largest immune organ (Zhang et al., 2022a). Trillions of microorganisms colonize the gastrointestinal tract; the total microbial number is 10 times the number of host somatic cells, and the collective number of genes is 150 times that in the host genome (Collins et al., 2012; Strandwitz, 2018). The intestinal microbiota co-develops with the host and participates in nutrient digestion, improves intestinal development and health, and regulates the body’s metabolism and immunity function (Li et al., 2019; Han et al., 2020). Gut microbiota disorders have been associated with subhealth and disease. Broilers show a shift in the gut microbial profile when they experience subclinical forms of necrotic enteritis: the relative abundance of Firmicutes, Lactobacillus, and Bacteroides decreases, thus resulting in low host productivity (Antonissen et al., 2016). Other research has indicated that challenge with Salmonella typhimurium decreased Lactobacillus prevalence, damaged intestinal morphology, and subsequently decreased the growth performance of broilers (Jazi et al., 2019). The overall consensus is that the intestinal microbiota is interlinked with intestinal health and poultry growth.
Probiotics have been demonstrated to be an effective means of promoting animal growth and improving body health in the post-antibiotic era (Mingmongkolchai and Panbangred, 2018). Bacillus licheniformis (B. licheniformis) is a Gram-positive bacterium characterized by resistance to stresses such as high acidity and temperature. Because of these characteristics, B. licheniformis can be used in livestock production practices. Bacillus spores are metabolically dormant under harsh conditions including feed pelleting but subsequently grow in the favorable environment of the gastrointestinal tract after ingestion (Konieczka et al., 2018). B. licheniformis shows growth-promoting effects in poultry (Gadde et al., 2017). Moreover, B. licheniformis benefits broilers by protecting against heat stress and preventing necrotic enteritis (Abdelqader et al., 2020; Xu et al., 2021). These benefits might be attributable to a variety of biologically active substances produced by B. licheniformis, which contribute to feed digestibility, immune system regulation, and enhanced intestinal barrier function (Kim et al., 2004; Zhou et al., 2016; Kan et al., 2021). B. licheniformis improves the intestinal mechanical barrier and decreases intestinal permeability by up-regulating the gene expression of mucins and tight junction proteins in laying hens (Wang et al., 2017). Diets containing a mixture of B. licheniformis and B. subtilis have been found to alleviate Escherichia coli-induced enteritis by increasing intestinal epithelial barrier integrity (Yang et al., 2016). The potential underlying mechanism involves regulation of the composition of the intestinal microbiota to restore and maintain intestinal homeostasis (Sanders et al., 2019; Zhao et al., 2022b). For instance, probiotic Bacillus strains have been found to prevent or diminish gut colonization by Chlamydia psittaci, Escherichia coli, Streptococcus, and Salmonella, thus improving intestinal mucosa integrity and gut health (Zuo et al., 2020; Haque et al., 2021). Therefore, probiotic Bacillus appears to prevent disease or stress, and promote growth performance, possibly through an optimized intestinal microbial structure and improved gut health.
Although B. licheniformis has great potential application value in the broiler industry, the efficacy of probiotic Bacillus varies among strains and depends on the exogenous environmental conditions to which animals are exposed (Konieczka et al., 2022). In the present study, we hypothesized that dietary B. licheniformis BCG might alter the ileal and cecal microbiota, and contribute to broiler digestibility and gut health. To this end, we aimed to explore the protective roles of B. licheniformis BCG involving improved nutrient digestion and absorption, a strengthened intestinal barrier, and decreased inflammation, and to understand their relationships with the gut microbiota shifted by B. licheniformis BCG. Our data provided a theoretical basis for application of B. licheniformis BCG in the production of healthy broilers.
2. Materials and methods
The experimental animal protocol for this study was conducted in accordance with the recommendations of “Guidelines on Welfare and Ethical Review for Laboratory Animals” (GB/T 35892-2018), and approved by the Institutional Animal Care and Use Committee of the Institute of Feed Research of Chinese Academy of Agricultural Sciences (FRI-CAAS20210827).
2.1. Animals and experimental design
A total of 240 1-day-old male Arbor Acre broilers (body weight, 42.62 ± 0.82 g) were randomly allocated to three groups. Each group consisted of eight replicates (pens) with 10 broilers per pen. Two phase non-medicated basal diets in mashed form were formulated based on the nutrient requirements of the National Research Council (1994); (Table 1). The three groups included basal diet (CT, n = 8), and basal diet with a dose of 1.0 × 108 CFU/kg (BCG1, n = 8) and 1.0 × 109 CFU/kg (BCG2, n = 8) B. licheniformis BCG, respectively (Wang et al., 2017; Kan et al., 2021; Xu et al., 2021). All broilers were feed in wire-floored cages in a one-level battery on their respective diets. The study lasted 42 days, during which time broilers had ad libitum to feed and fresh water. Broilers were housed in an environmentally controlled room and temperature was gradually reduced from 35°C on day 1 to 26°C at day 21 and then kept roughly constant. A 20 h light-4 h dark cycle was carried out throughout the experimental period. B. licheniformis BCG was isolated from humus soil in the northeast forest area and preserved in the Key Laboratory of Feed Biotechnology of Ministry of Agriculture and Rural Affairs. It presents great biological characteristics in carbohydrate metabolism enzymes and stress tolerance through the whole genome sequencing and in vitro evaluation. B. licheniformis BCG with viable count = 1.08 × 1010 CFU/g was used and mixed in the basal diet, which was prepared in bacterial mashed form after processed in activation, culture, centrifugation, freeze-drying, and grinding.
2.2. Sample collection
At 42 days of age, after fasting overnight, one broiler representing the average weight from each replicate was selected and humanely slaughtered. Jejunum, ileum and cecum segments were divided and fresh ileal and cecal contents were collected for α-amylase and microbiota analysis. Jejunal and ileal mucosa were scraped by autoclaved blade after precooled saline flush for maltase, sucrase and gene expression analysis. All samples were obtained as described previously (Wang et al., 2008), and immediately frozen in liquid nitrogen and stored at −80°C.
2.3. Biochemical analysis
Appropriately 100 mg frozen mucosa and chyme of jejunum and ileum, respectively, were taken and mixed with 1 mL cold buffer (pH7.4), containing 10 mM Tris-HCl, 0.1 EDTA-Na2 and 0.8% (w/v) NaCl, and homogenized using an Ultra-Turrax homogenizer for 30 s. Homogenates were centrifuged at 3,000 × g for 15 min at 4°C and supernatants transferred to new tubes for protein assay and other measurements. The activities of α-amylase, maltase and sucrase were measured by colorimetry using the commercial kits (Nanjing Jiancheng Bioengineering Institute, Nanjing, China) according to the manufacturer’s protocols. Maltase and sucrase were normalized to tissue protein concentrations, which were measured with a bicinchoninic acid commercial kit (Thermo Fisher Scientific, Waltham, MA, USA) following the manufacturer’s instructions.
2.4. Real-time quantitative PCR (RT-qPCR)
Selected mRNA abundance was determined by RT-qPCR, including nutrient transporters genes FABP-1 (fatty acid binding protein 1), FATP-1 (fatty acid transport protein 1), GLUT-2 (glucose transporter 2), LAT-1 (L type amino acid transporter 1), PepT-1 (peptide transporter 1) and SGLT-1 (sodium glucose co-transporter 1), inflammatory molecules’ genes TLR-4 (Toll-like receptor 4), IL-1β (interleukin 1β), IL-8, IL-10, TNF-α (tumor necrosis factor α), TGF-β (Transforming growth factor β) and NF-κB (Nuclear factor kappa B), and tight junction genes Claudin-1, Occludin, ZO-1 and Mucin-2. Total RNA was isolated from ileal mucosa samples (approximately 0.75 mg) using an RNAprep pure tissue kit (Tiangen Biotech Co. Ltd., Beijing, China) under the manufacturer’s instructions. Total RNA concentrations and quality were assessed using a NanoDrop 2000 spectrophotometer (Thermo Fisher Scientific, Waltham, MA, USA). RNA integrity was evaluated using agarose gel (1%) electrophoresis. Then, cDNA was synthesized from 1 μg total RNA using a PrimeScript RT reagent kit (TaKaRa Biotechnology Co., Ltd., Otsu, Japan) following to manufacturer’s protocols. Selected mRNA reactions were detected in 10 μL (Bio-Rad Laboratories, Hercules, CA, USA) using SYBR® Premix Ex TaqTM II (Tli RNaseH Plus) (TaKaRa Biotechnology Co., Otsu, Japan). The primers for nutrient transporters, inflammatory, and tight junction-related and housekeeping genes [glyceraldehyde 3-phosphate dehydrogenase (GAPDH)] were described previously (Wang et al., 2016, 2020). The 2–ΔΔCt method was used for quantification using GAPDH as a reference gene, and relative abundance was normalized to CON group values.
2.5. Ileal and cecal microbiota and analysis
Bacterial genomic DNA was extracted from ileal and cecal chyme samples (Qiagen DNA stool mini kit, Qiagen, Germany). DNA quantity and quality were assessed using a NanoDrop 2000 spectrophotometer (Thermo Fisher Scientific, Waltham, MA, USA) and 1% agarose gels, respectively. The V3–V4 hypervariable region 16S rRNA was amplified using specific primers (forward 5′-ACTCCTACGGGAGGCAGCA-3′ and reverse 5′-GGACTACHVGGGTWTCTAAT-3′), containing unique barcodes. Polymerase chain reaction (PCR) was conducted in a total volume of 20 μL, including 1 × FastPfu buffer, 250 μM dNTP, 0.2 μM each primer, 1 U FastPfu polymerase (Beijing TransGen Biotech, Beijng, China), and 10 ng template DNA. PCR products were electrophoresed on 2% agarose gels and purified using a Qiagen gel extraction kit (Qiagen, Germany). Sequencing libraries were constructed using a TruSeq® DNA PCR-Free Sample Preparation Kit (Illumina, San Diego, CA, USA) based on manufacturer’s instructions, and index codes were added. Library quality was assessed using a Qubit V.2.0 Fluorometer (Thermo Fisher Scientific, Waltham, MA, USA). Qualified DNA libraries were loaded into a NovaSeq platform capable of 2 × 250 bp paired-end sequencing reads (Novogene, Beijing, China).
Paired-end reads were generated and merged using FLASH software (V1.2.7)1. Operational taxonomic units with 97% identity were gathered using Uparse2 (ver. 7.1). Taxonomic annotations were performed using the Mothur algorithm (70% confidence) in the Silva database3. Alpha-diversity was analyzed using Observed_species, Chao1, and Shannon indices. Beta-diversity was visualized using principal coordinate analysis (PCoA) plots based on weighted UniFrac distance. Bacterial biomarkers between groups were displayed using the linear discriminant analysis effect size (LEfSe, linear discriminant analysis (LDA) > 3.5).
2.6. Statistical analysis
Statistical analyses were performed using one-way analysis of variance in SAS 9.4 (SAS Institute, Inc., Cary, NC, USA). Each broiler served as statistical unit. Differences between treatment means for enzyme activity and gene expression were evaluated using Duncan’s multiple-range tests. Wilcox test was used for alpha-diversity index. LEfSe, t-test, and Metastat analyses were used to test for significant differences between microbiota relative abundance. Results were represented as mean with standard error of mean (SEM) in the tables and the mean with standard error (SE) in the figures, while P < 0.05 (*) and P < 0.01 (**) values were considered statistically and extremely significant, respectively. Bar charts were drafted in Graphpad Prism 7.0 software (GraphPad Software Inc., La Jolla, CA, USA).
3. Results
3.1. Effects of B. licheniformis BCG on jejunal and ileal enzyme activity in broilers
The BCG2 group showed significantly greater jejunal and ileal maltase and sucrase activity, and the BCG1 group showed significantly greater ileal sucrase activity, than the CT group (P < 0.05, Table 2). No differences in these two parameters were observed between the BCG1 and BCG2 groups (P > 0.05). Dietary B. licheniformis BCG resulted in significantly greater α-amylase activity than that in the CT group (P < 0.05), and this activity was higher in the BCG2 group than the BCG1 group (P < 0.05).
3.2. Effects of B. licheniformis BCG on nutrient transporter gene mRNA levels in the ileum
FABP-1 and FATP-1 relative mRNA levels in the BCG2 group were significantly higher than those in the CT and BCG1 groups (P < 0.05, Figure 1). The transcript abundance of GLUT-2 and LAT-1 was greater in the BCG2 group than the CT group (P < 0.05), and no significant difference was found between the BCG1 group and the other groups (P > 0.05).
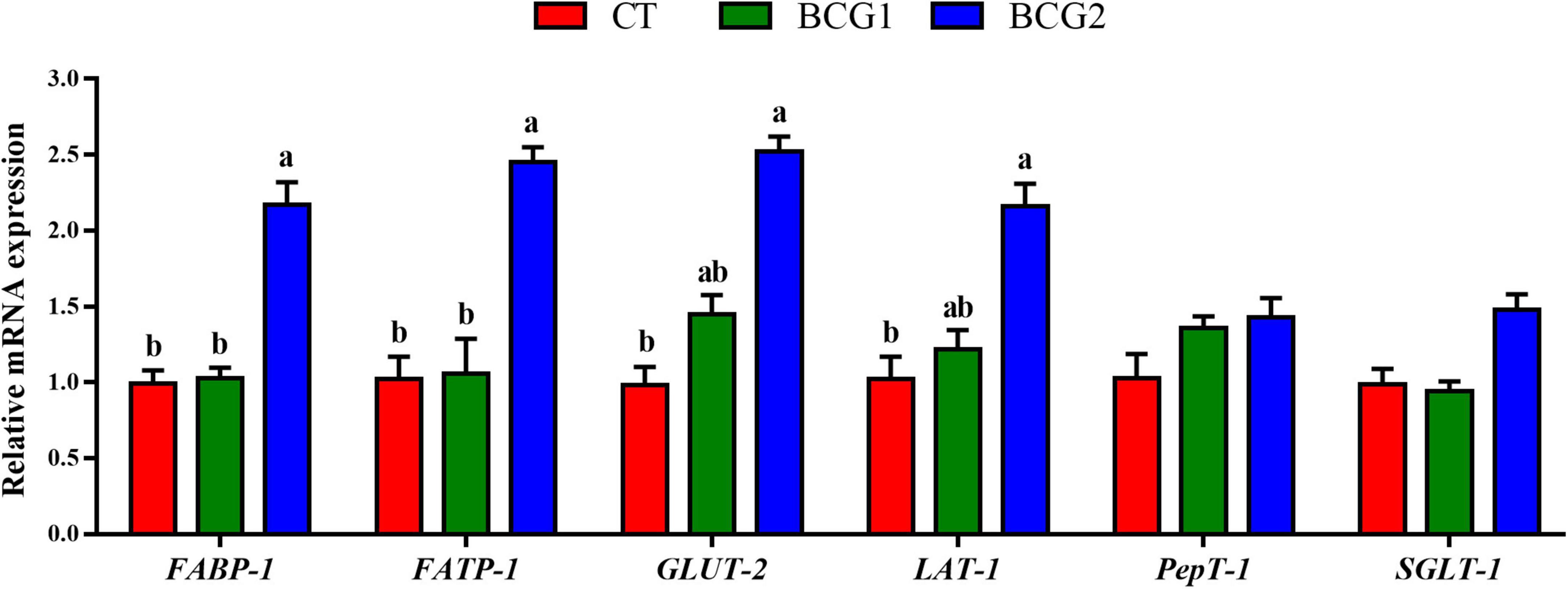
Figure 1. Effects of Bacillus licheniformis BCG on nutrient transporter gene mRNA levels in the ileum. Values are mean ± SE (n = 6). a,bBars with different letters within the same index indicate a significant difference between groups (P < 0.05).
3.3. Effects of B. licheniformis BCG on tissue morphology and the mRNA expression of tight junction and inflammatory molecules in the ileum
Histological examination of the ileum indicated that the villi and epithelium in the CT group, as compared with the BCG1 and BCG2 groups, showed damage; however, no clear infiltration of inflammatory cells was observed among groups (Figure 2A). Dietary B. licheniformis BCG significantly up-regulated ileal occludin mRNA levels, which were higher in the BCG2 group than the CT group (P < 0.05, Figure 2B). The transcript abundance of IL-8 and TLR-4 in the BCG1 and BCG2 groups was lower than that in the CT group (P < 0.05, Figure 2C), whereas no significant difference was observed between the BCG1 and BCG2 groups. No significant difference in claudin-1, ZO-1, mucin-2, IL-1β, TNF-α, NF-κB, IL-10, and TGF-β transcript abundance was observed between groups (P > 0.05).
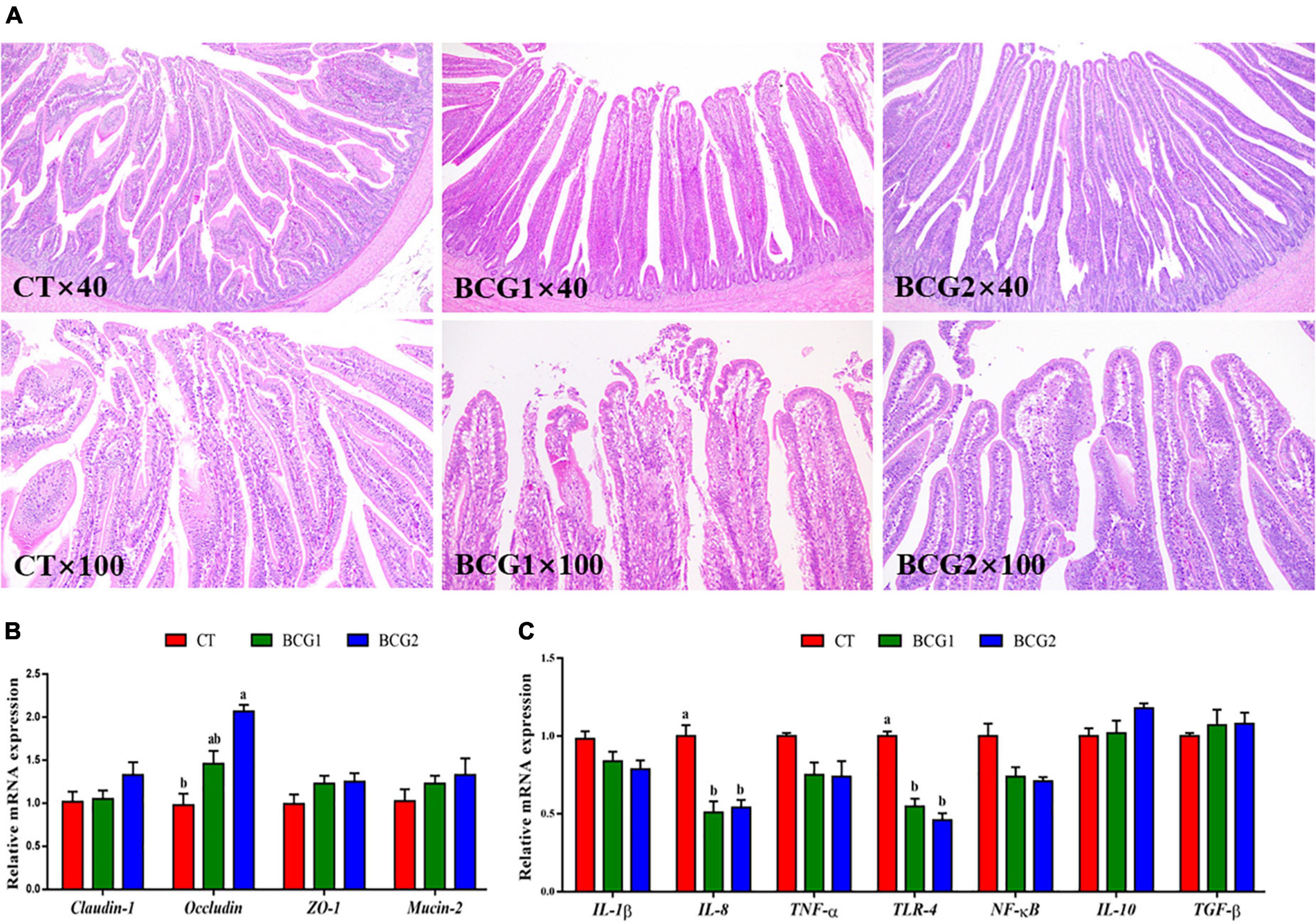
Figure 2. Effects of Bacillus licheniformis BCG on ileal morphology, and tight junction and inflammatory molecule gene mRNA levels in the ileum. (A) Representative ileal histological sections of broilers. (B) mRNA levels of the tight junction genes claudin-1, occludin, ZO-1, and Mucin-2. (C) The mRNA levels of the inflammatory molecule genes TLR-4, IL-1β, IL-8, IL-10, TNF-α, TGF-β, and NF-κB. Values are the mean ± SE (n = 6). a,bBars with different letters within the same index indicate a significant difference between groups (P < 0.05).
3.4. Effects of B. licheniformis BCG on microbiota diversity in the ileum and cecum
A total of 3,917,657 high quality sequencing reads were generated from 47 broiler gut samples, with an average of 69,233 effective sequences/sample. Alpha diversity analyses indicated varying community richness and diversity among groups in the ileal but not the cecal bacterial communities. Both the BCG1 and BCG2 groups showed significantly lower ileal Observed_species, Chao1, and Shannon indexes than the CT group (P < 0.05, Figures 3A–C). Differences in the microbial structure among groups and niches were evaluated with PCoA analysis based on weighted UniFrac distance. Microbial communities were well separated between the ileal microbiota and counterparts colonizing the cecum, and between groups in the ileum (Figures 3D–F). ANOSIMs also confirmed the structural dissimilarity between the ileum and cecum, and between the CT group and the two BCG groups in the ileum or cecum (R > 0, P < 0.05, Table 3).
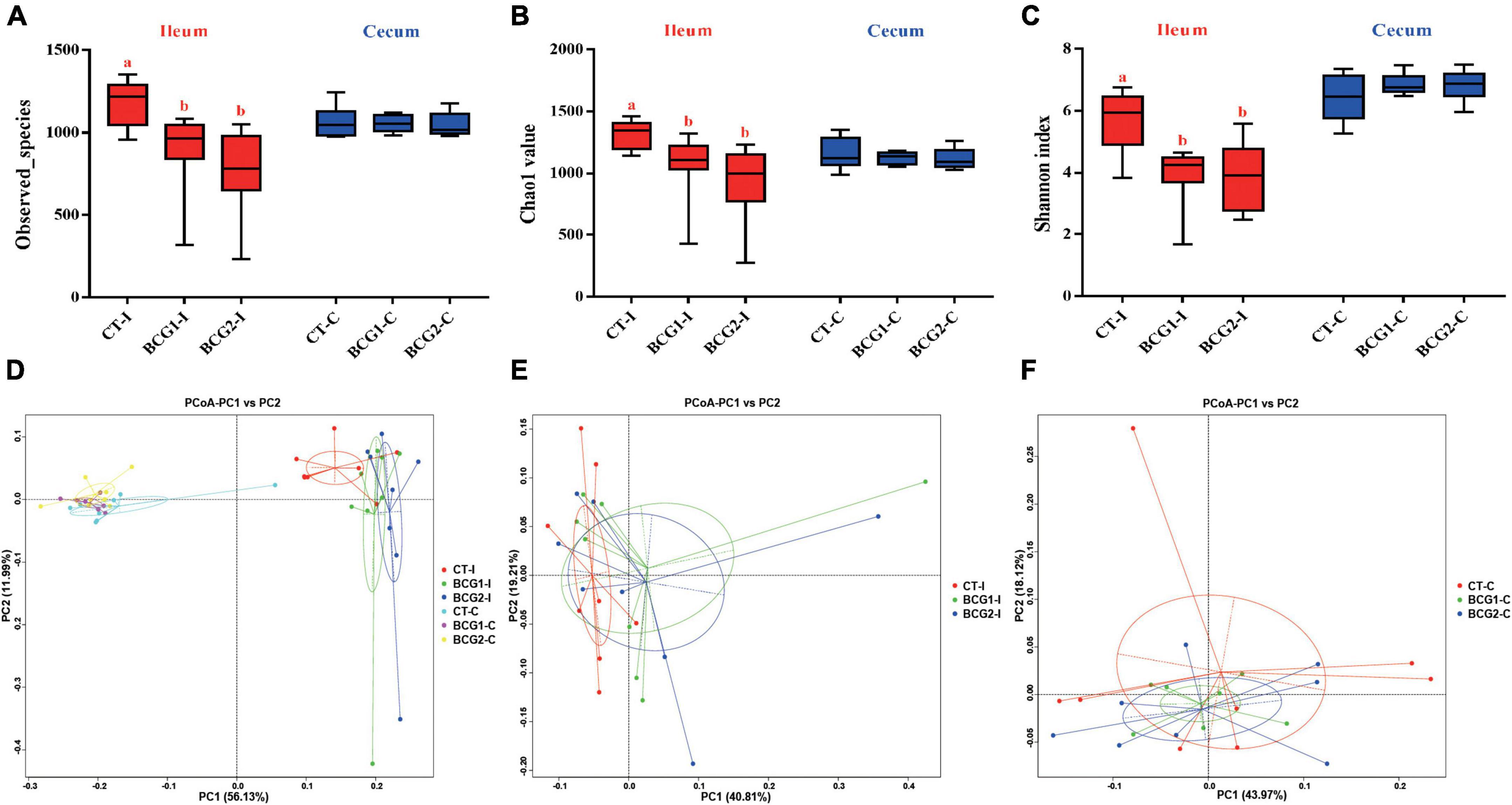
Figure 3. Effects of Bacillus licheniformis BCG on alpha- and beta-diversity of the microbiota inhabiting the ileum and cecum in broilers. (A–C) Bacterial richness and diversity, estimated with Observed_species, Chao1 value, and Shannon index. (D–F) Principal coordinate analysis based on weighted UniFrac distances, showing separation in the microbiota between the ileum and cecum, and between groups in the ileum or cecum. Values are mean ± SE (n = 8; BCG1-C, n = 7). a,bBoxes with different letters within the same index indicate a significant difference between groups (P < 0.05).
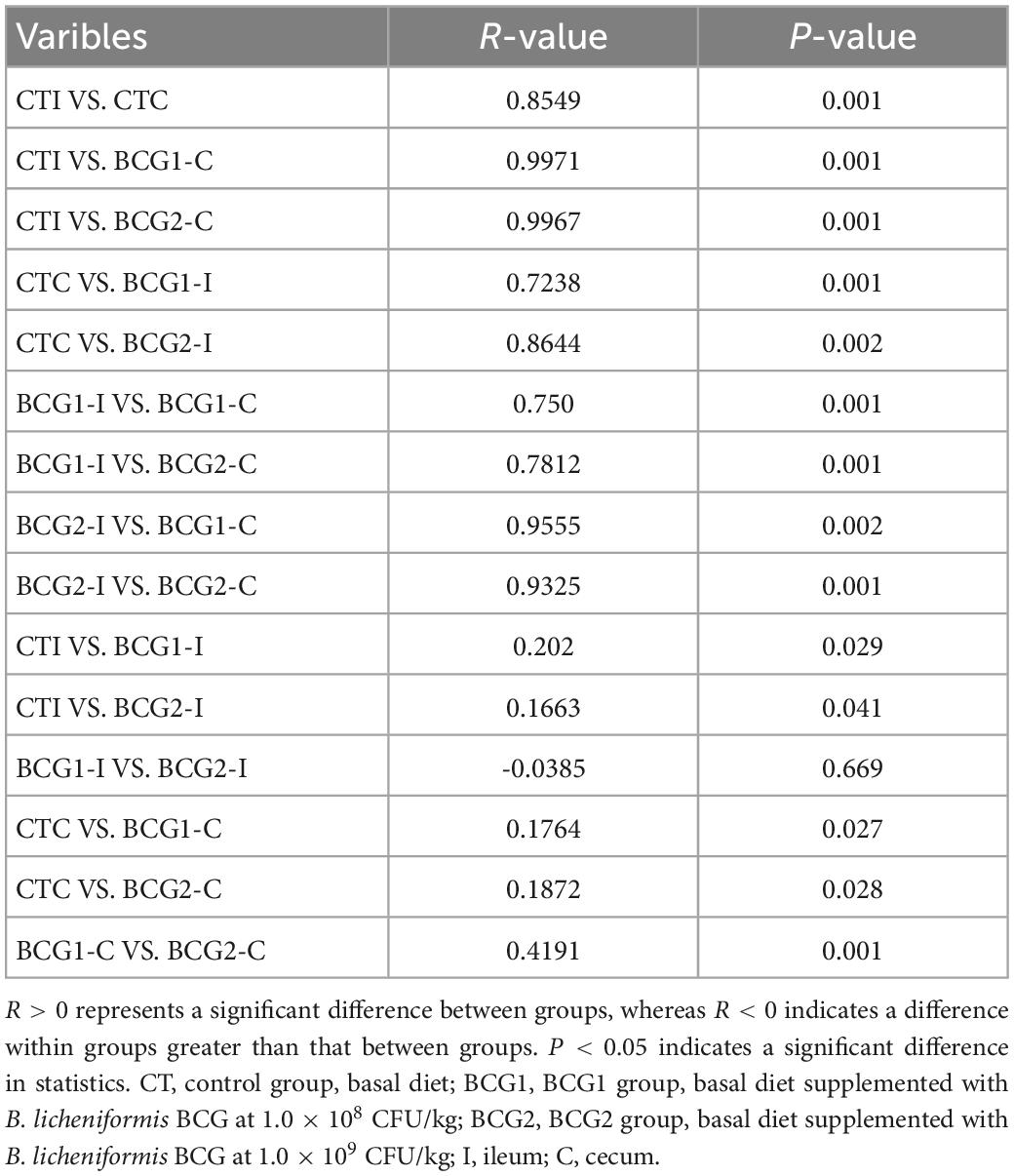
Table 3. ANOSIM analysis of differences in bacterial structure between variables, on the basis of Bray-Curtis distances.
3.5. Effects of B. licheniformis BCG on ileal and cecal bacterial structures
In the ileum, Proteobacteria, Firmicutes, Bacteroidota, and Campylobacterota were the dominant bacterial phyla, with a relative abundance accounting for > 95% of the total ileal bacterial communities (Figure 4A). In contrast to the CT-ileal (CT-I) group, the BCG1-I and BCG2-I groups showed an increase in the relative abundance of Proteobacteria and Firmicutes, from 11.79 to 17.44 (16.81%), and from 64.11 to 69.37 (73.94%), respectively (P > 0.05). However, the BCG1-I and BCG2-I groups showed a decrease in the relative abundance of Bacteroidota and Campylobacterota, from 10.25 to 4.19 (4.62%), and from 9.08 to 6.42 (1.98%), respectively (P > 0.05). At the family level, in contrast to the CT group, the BCG1 and BCG2 groups showed an increase in the relative abundance of Peptostreptococcaceae, Sphingomonadaceae, and Lactobacillaceae, from 17.6 to 26.67 (26.92%), from 0.04 to 6.89 (5.39%), and from 12.18 to 23.17 (22.97%), respectively (P > 0.05). The relative abundance of Campylobacteraceae in the BCG1-I and BCG2-I groups was, respectively, 0.68 and 1.44%, and lower than the 7.31% in the CT-I group (P > 0.05, Figure 4B). Diets with BCG1 and BCG2 significantly decreased several low abundance bacteria at the family level (P < 0.05, Figures 4C, D). For the 35 most dominant ileal genera, the BCG1 and BCG2 diet groups showed significantly lower relative abundance of Clostridiales bacterium CHKCI001, Enterococcus, Clostridia_vadinBB60_group, Faecalibacterium, Phascolarctobacterium, Barnesiella, Alistipes, and Ruminococcaceae UCG-005 than that in the CT group (P < 0.05, Figure 4E). The BCG1 diet group showed significantly lower relative abundance of Lactobacillus, Vibrio, Pseudomonas, Bacteroides, Streptococcus, Staphylococcus, and Bacillus than that in the CT group (P < 0.05). The relative abundance of Helicobacter in the BCG2-I group was significantly lower than that in the CT-I and BCG1-I groups (P < 0.05). In contrast to the CT-I group, the BCG1-I and BCG2-I groups showed an increase in the relative abundance of Sphingomonas from 0.03 to 6.34 (11.55%), whereas Campylobacter levels showed a decrease from 7.31 to 0.68 (1.44%) (P > 0.05).
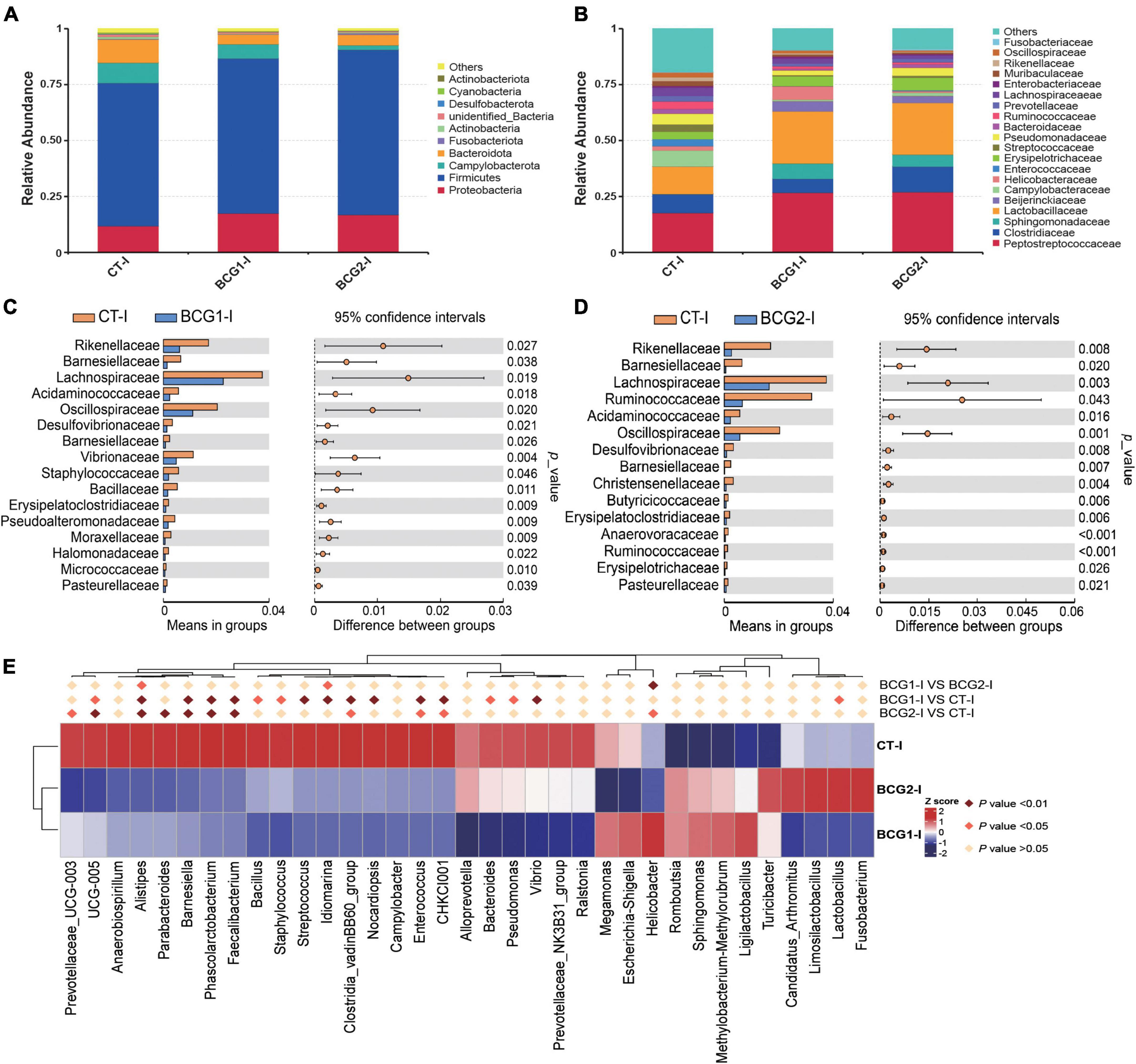
Figure 4. Effects of Bacillus licheniformis BCG on bacterial composition in the ileum. (A,B) Distribution of ileal bacteria at the phylum and family levels. (C,D) The t-tests were used to assess significant differences at the family level; P < 0.05 indicates a significant difference. (E) Statistical analysis of differences in the relative abundance of the top 35 genera; Metastat was used to test for significant differences; light pink diamonds indicate P < 0.05, and dark pink diamonds indicate P < 0.01 between groups (n = 8).
In the cecum, Bacteroidota, Firmicutes, Proteobacteria, Campylobacterota, and Fusobacteriota were the major bacterial phyla, and their relative abundance was seldom affected by BCG treatments (P > 0.05, Figure 5A). On the basis of T-test and LEfSe results, CT-C broilers had a higher relative abundance of f_Erysipelotrichaceae, f_Peptostreptococcaceae, and g_Romboutsia than that in the BCG1-C and BCG2-C groups, and f_Erysipelotrichaceae and f_Peptostreptococcaceae levels in the BCG2-C group were significantly higher than those in the BCG1-C group (P < 0.05, Figures 5B–F). f-Lactobacillaceae and g_Lactobacillus were the dominant bacteria in the BCG2-C group, in contrast to the BCG1-C and CT-C groups, whereas f_Enterococcaceae, f_Campylobacteraceae, f_Tannerellaceae, g_Escherichia-Shigella, g_Campylobacter, and g_Parabacteroides were the dominant bacteria in the BCG1-C group, in contrast to the CT-C and BCG2-C groups. The f_Campylobacteraceae level in the BCG2-C group was significantly lower than that in the BCG1-C group (P < 0.05).
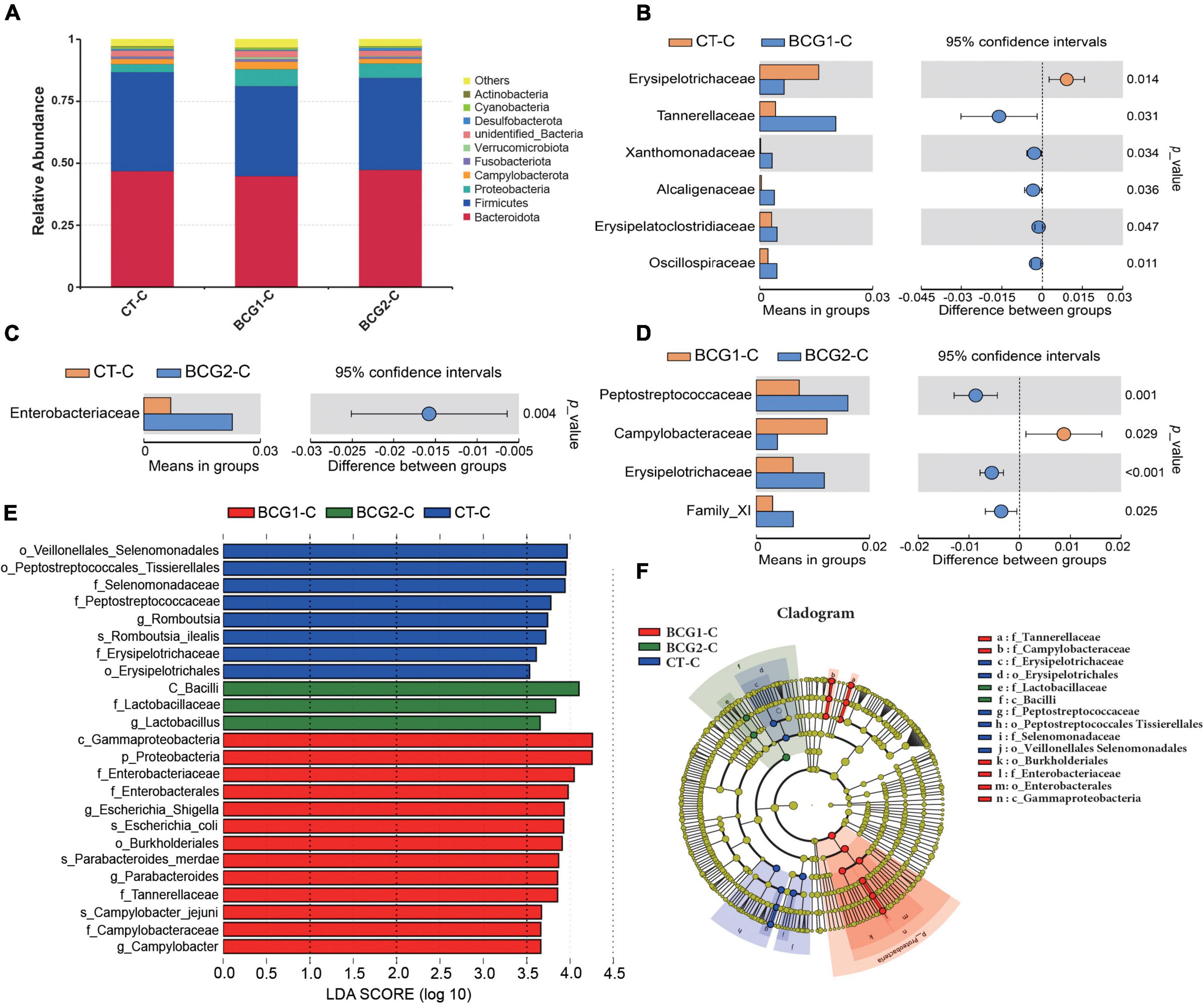
Figure 5. Effects of Bacillus licheniformis BCG on bacterial composition in the cecum. (A) Distribution of cecal bacteria at the phylum level. (B–D) The t-tests were used to assess significant differences at the family level; P < 0.05 indicates a significant difference. (E) LEfSe analysis of differences in taxa enrichment in microbial communities between groups; bacterial taxa with a logarithmic LDA score > 3.5 were biomarker taxa. (F) Cladogram showing bacteria with significant differences between groups (n = 8; BCG1-C, n = 7).
3.6. Correlations between ileal microbiota and enzyme activity and nutrient transporters or inflammatory and barrier parameters
A Spearman’s correlation analysis was performed to explore the relationships of predominant ileal phyla, families, and genera with the nutrient digestion and absorption, or inflammatory and barrier parameters (Figure 6). Proteobacteria, f_Sphingomonadaceae, and Sphingomonas were significantly positively correlated with FATP-1 expression, whereas Alistipes and Barnesiella were significantly negatively correlated with α-amylase activity (P < 0.05, Figure 6A). Bacteroides and Limosilactobacillus showed a significant positive correlation with SGLT-1 expression and α-amylase activity (P < 0.05). Helicobacter was significantly positively correlated with maltase activity but negatively correlated with SGLT-1 expression (P < 0.05). Firmicutes, f_Lactobacillaceae, Lactobacillus, Fusobacterium, and Limosilactobacillus showed significant positive correlations with ZO-1 expression. f_Lactobacillaceae and Lactobacillus were significantly positively correlated with occludin and mucin-2 expression. f_Lactobacillaceae showed a significantly positive correlation with claudin-1 expression (P < 0.05, Figure 6B). Bacteroidota, Bacteroides, and Phascolarctobacterium were significantly correlated with TLR 4 and IL-10 expression, and Ruminococcaceae UCG-005 was significantly correlated with TNF-α, NF-κB, and IL-8 expression (P < 0.05). Proteobacteria, f_Sphingomonadaceae, and Sphingomonas showed significant negative correlations with IL-1β and NF-κB expression (P < 0.05).
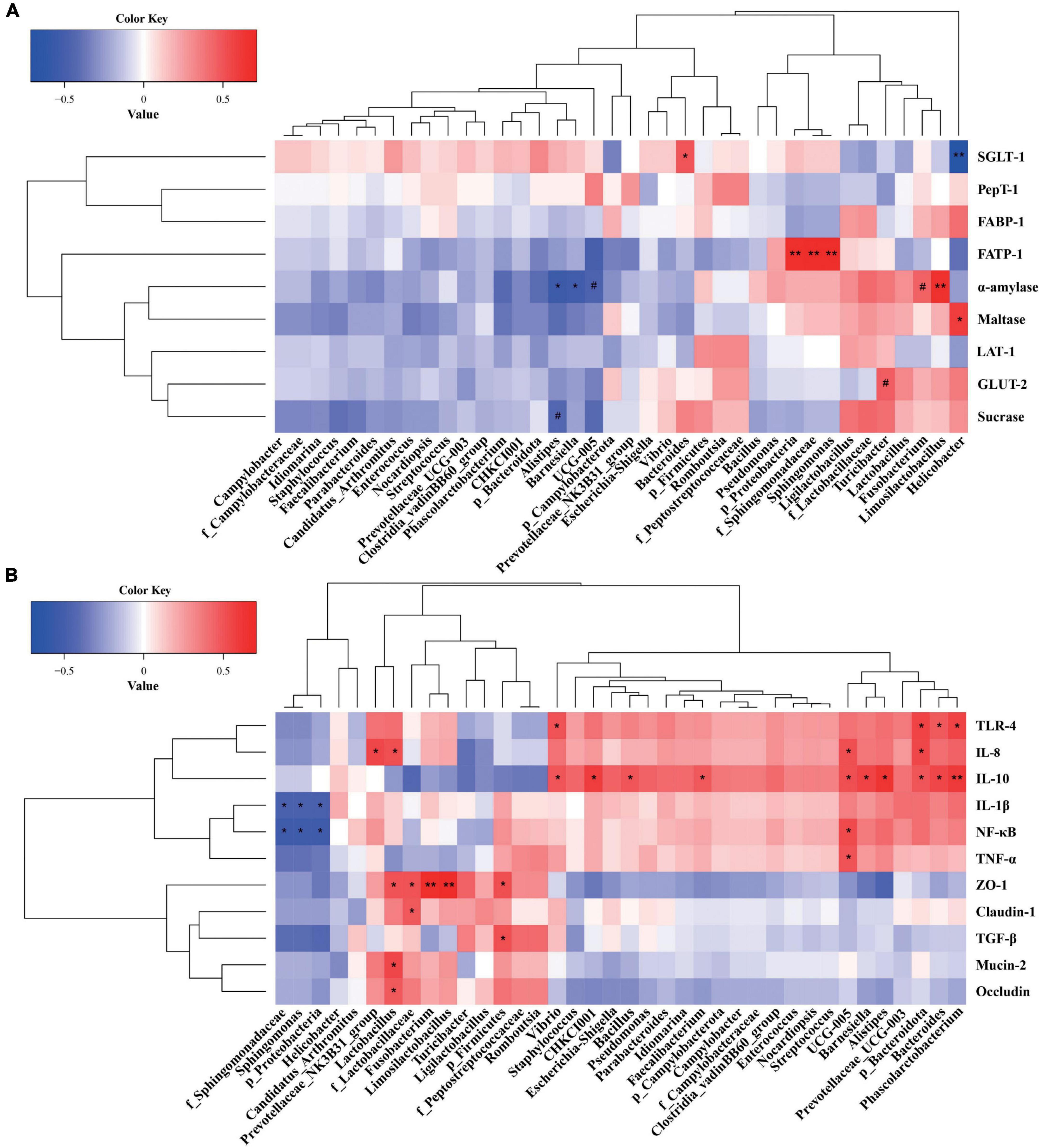
Figure 6. (A) Correlations between the ileal microbiota and enzyme activity and nutrient transporters. (B) Correlations between the ileal microbiota and inflammatory and barrier parameters. Red represents a positive correlation, and blue represents a negative correlation. *P < 0.05 and **P < 0.01 indicate significant and extremely significant correlations.
4. Discussion
Bacillus spp. bacteria serve as a potential alternative to antibiotic growth promoters in livestock production, owing to their stress resistance and probiotic characteristics. Previous studies have shown that dietary supplementation with B. licheniformis significantly promoted broiler growth by increasing body weight and decreasing the feed to weight ratio (Liu et al., 2012; Chen and Yu, 2020; Xu et al., 2021). In addition, several studies have indicated that B. licheniformis administration promoted broiler growth under heat stress and Clostridium perfringens challenge conditions (Song et al., 2014; Zhou et al., 2016; Musa et al., 2019). These findings were consistent with those in our previous study indicating that diets with B. licheniformis at a dose of 1.0 × 109 CFU/kg significantly increases broiler body weight and average daily gain (Wang et al., 2022). Thus, B. licheniformis improved broiler growth performance under both normal and stress conditions. The mechanism underlying the improvements in nutrient digestion and host health were likely to involve the formation of beneficial metabolites such as extracellular digestive enzymes, organic acids, and antibacterial peptides (Kim et al., 2004; Knap et al., 2010; Xu et al., 2021). Thus, the activity of α-amylase, maltase, and sucrase in the jejunum and ileum was further determined, because these enzymes participated in the digestion of nutrients.
In poultry, feed starch is generally degraded by α-amylase into smaller molecular oligomers after initial hydrolyzation by the microbiota, and is finally hydrolyzed into maltose and maltotriose in the small intestine (Dhital et al., 2017). Subsequently, maltose and maltotriose are hydrolyzed into glucose by sucrase, and maltase resides at the surfaces of the intestinal villi (Zhou et al., 2021). B. licheniformis secretes extracellular enzymes such as α-amylase and proteases (Kaewtapee et al., 2017). In the present study, dietary B. licheniformis BCG, compared with the CT diet, significantly increased jejunal and ileal α-amylase, maltase, and sucrase activity, in partial agreement with findings reported by Yang et al. (2021). The α-amylase activity is an important rate-determining factor in starch digestion, because endogenous α-amylase activity is low in broilers (Zhou et al., 2021). The increased α-amylase activity may increase the nutrient digestibility coefficients of starch and organic matter and consequently increase feed metabolizable energy, thus contributing to broiler growth performance (Jiang et al., 2008; Kaczmarek et al., 2014). In addition, nutrient transporters are crucial for nutrient absorption at the brush border membrane in the small intestine. Both FABP-1 and FATP-1 are important for intestinal absorption of lipids and fatty acids, particularly in long-chain fatty acid metabolism and intracellular transportation (Coe et al., 1999; Richieri et al., 1999). GLUT-2 is an Na+-independent transporter responsible for the basolateral exit of glucose from the intestinal mucosa into the portal circulation (Wang et al., 2020). Intestinal LAT-1 participates in branched-chain and aromatic amino acid transport in an Na+-independent manner (Broer, 2008). As previously reported, probiotic supplementation enhances expression of some types of nutrient transporters in the small intestines in animals (Faseleh Jahromi et al., 2016; Duan et al., 2018). In agreement with previous findings, the present study indicated that B. licheniformis BCG administration significantly increased FABP-1, FATP-1, GLUT-2, and LAT-1 expression, to a greater extent in the BCG2 group than the BCG1 group. Thus, dietary supplementation with B. licheniformis BCG may aid in starch digestion and the absorption of glucose, amino acids, and fatty acids, thereby resulting in higher growth performance of broilers.
To determine whether B. licheniformis BCG contributed to intestinal health, we assessed the gene expression of TLR4 signaling pathway related molecules, tight junction proteins, and mucin-2. TLR4, as a pathogen-associated molecular pattern, mediates downstream inflammatory signals through the linker protein MyD88, thereby activating NF-kB, which then translocates into the nucleus and elicits pro-inflammatory cytokine secretion and cellular responses of immune-associated cells (Huebener and Schwabe, 2013; Kan et al., 2021). B. licheniformis has been demonstrated to decrease secretion of the pro-inflammatory cytokines IL-8 and IL-6 in vivo (Deng et al., 2012; Roselli et al., 2017). Pretreatment with B. licheniformis has been found to decrease the serum TNF-α and IL-1β levels in an acetaminophen-induced acute liver injury rat model (Neag et al., 2020). Similarly, B. licheniformis also decreases the inflammatory response in an LPS-induced acute inflammation rat model (Deng et al., 2017). Moreover, B. licheniformis treatment markedly counteracts the increase in IL-6, IL-8 and TNF-α inflammatory gene expression induced by enterotoxigenic Escherichia coli F4 in vitro. The immunity homeostasis of HT-29 cells is improved by treatment with B. licheniformis MCC 2514, on the basis of downregulation of IL-1α, IL-6, IL-8, IL-12, and TNF-α, and upregulation of IL-4, IL-10, TGF-2, and TGF-3 (Rohith and Halami, 2021a). These results are consistent with our observation that the ileal TLR 4 and IL-8 expression in the BCG1 and BCG2 groups was significantly lower than that in the CT group. The underlying mechanism was associated with B. licheniformis bacteriostasis. As previously reported, B. licheniformis inhibited the growth of pathogenic bacteria such as Kocuria rhizophila and enterotoxigenic Escherichia coli by adhering to the intestines (Rohith and Halami, 2021b; Li et al., 2022a). Another key mechanism through which B. licheniformis attenuates inflammation might involve intestinal barrier improvement.
The integrated intestinal barrier plays an important role in maintaining epithelial cell function, because the epithelium is permeable to feed-associated antigens, and luminal pathogens and their toxins (Awad et al., 2017). The transmembrane proteins claudins, occludins, and zonula occludens involved in tight junctions are responsible for connecting epithelial cells and regulating paracellular and intracellular permeability (Aijaz et al., 2006). The regular permeability and integrity of tight junctions are generally negatively affected by stress factors, such as heat stress and pathogen invasion (Song et al., 2014; Musa et al., 2019; Li et al., 2022a). Under Clostridium perfringens challenge, dietary B. licheniformis significantly increases the gene expression of claudin-1 and ZO-1 in the duodenum in broilers at post challenge days 7 and 21 (Musa et al., 2019). In laying hens, B. licheniformis administration enhances the intestinal mechanical barrier by upregulating the gene expression of mucin-2 and tight junction proteins (Wang et al., 2017). In in vitro experiments, B. licheniformis PF9 application has been found to reverse the decrease in ZO-1 and occludin expression in the cell membrane after challenge with enterotoxigenic Escherichia coli (Li et al., 2022a). In agreement with previous studies, the present study indicated that a diet with a high dose of B. licheniformis BCG resulted in significantly higher occludin expression than that in the CT group. Thus, B. licheniformis BCG alleviates ileal inflammation partly by enhancing the physical barrier. The improved barrier function resulting from B. licheniformis BCG might be attributable to its biologically active substances including bacteriocin and antibacterial peptides, which suppress pathogenic bacterial colonization and balance the intestinal microbiota (Zhou et al., 2016; Kan et al., 2021).
The gut microbiota provides a broad range of functions for hosts, for example, the digestion of complex dietary nutrients, defense against pathogens, enhancement of the intestinal barrier, and promotion of immune maturation (Koh et al., 2016). Owing to differences in histology and function, the broiler intestinal tract is generally divided into the fore- and hindgut. Previous studies in broilers have focused primarily on the hindgut microbiota and their functions, whereas studies on the microbiota in the foregut and their interactions with the host have been limited (Oakley et al., 2014; Huang et al., 2018). In the current study, we analyzed ileal and cecal microbial diversity and composition, and their effects under B. licheniformis BCG treatment. The PCoA revealed a clear separation between the ileum and cecum compartments, thus indicating a large difference between them, in agreement with findings from a previous study (van der Wielen et al., 2002). In addition, piglets fed Bacillus species probiotics show diminished microbial richness in feces (Kaewtapee et al., 2017; Poulsen et al., 2018). These findings are partially consistent with those in the current study indicating diminished bacterial richness and diversity in the ileum but not cecum in broilers in response to B. licheniformis BCG. Our findings indicated that dietary supplementation with B. licheniformis BCG shifted the ileal bacterial community structure in broilers. Theoretically, gut bacterial diversification is a gradual process that increases with age, and high diversity is considered a sign of gut bacterial maturity (Micah et al., 2007). Premature formation of an adult-type bacterial community negatively affects host gut immunity (Nylund et al., 2013). Dietary supplementation with B. licheniformis BCG significantly decreased ileal inflammation in the present study, a result partially attributed to the decrease in bacterial diversity.
Bacterial composition is also closely associated with host physiology, including digestion, metabolism, and immunity. In the present study, considerable variations in Proteobacteria, Firmicutes, Campylobacterota, and Bacteroidota were found between the ileum and cecum. Oxygen-sensitive Bacteroidota markedly increased from the ileum to the cecum, because it is adapted to a low oxygen environment. However, oxygen-tolerant Proteobacteria and Campylobacterota, such as Enterococcaceae and Campylobacteraceae, decreased from the ileum to the cecum. Our results are consistent with previous observations that spatial shifts in bacterial composition depend on microenvironment change (Huang et al., 2018; Li et al., 2022b). Firmicutes include Peptostreptococcaceae, Clostridiaceae, and Lactobacillaceae, which are positively associated with energy intake and have been found to provide an additional 628 kJ of energy when their abundance increases by 20% (Hildebrandt et al., 2009; Jumpertz et al., 2011). This causal relationship has also been found in piglet models (Mach et al., 2015; Li et al., 2022a). Firmicutes fermentation is a more suitable energy source than Bacteroidota because it produces more short chain fatty acids, thus enhancing efficient heat absorption (Krajmalnik-Brown et al., 2012; Pan et al., 2022). We observed that a diet with B. licheniformis increased ileal Firmicutes levels but decreased Bacteroidota levels, a finding partially explained by our previous results indicating that B. licheniformis administration promoted broiler growth (Wang et al., 2022). In recent studies, Sphingomonas involved in the f_Sphingomonadaceae has been observed as an abundant bacterium in chicken intestines (Chen et al., 2018; Zhang et al., 2022b). The abundance of Sphingomonas is significantly positively correlated with fat catabolism in the liver, serum, and muscle (Li et al., 2020; Zhang et al., 2022b). In addition, Sphingomonas participates in lipid metabolism across the entire process of chicken embryonic development (Akinyemi et al., 2020). Diets with B. licheniformis resulted in significantly greater ileal f_Sphingomonadaceae and Sphingomonas levels than those in the CT group; these levels were significantly positively correlated with FATP-1 expression but significantly negatively correlated with IL-1β and NF-κB expression. These findings suggested that the shifts in the prevalence of f_Sphingomonadaceae and Sphingomonas after B. licheniformis BCG treatment contributed to the intestinal absorption and oxidative decomposition of lipids and fatty acids, and alleviation of intestinal inflammation.
On the basis of the analysis of the abundance of bacteria in the present study, Lactobacillus, Bacteroides, Alistipes, Escherichia-Shigella, Desulfovibrio, Streptococcus, Ruminococcaceae_UCG-005, Fusobacterium, and Campylobacter predominated in broilers. In general, the presence of Lactobacillus is considered beneficial for intestinal health and animal health, owing to their immunomodulation, pathogen inhibition and bacteriocin production ability (Kaewtapee et al., 2017; Zhao et al., 2022b). In the current study, broilers in the BCG2 group had higher Lactobacillus levels in both the ileum and cecum than those in the CT or BCG1 group, in agreement with findings from previous studies (Hung et al., 2019; Zhao et al., 2022b). An increase in Lactobacillus might have resulted from B. licheniformis BCG supplementation, which creates a suitable environment for the colonization of Lactobacillus anaerobic bacteria by consumption of oxygen in the gut (Fazelnia et al., 2021). In previous studies, Lactobacillus administration has been found to contribute to nutrient absorption by significantly increasing the expression of sugar transporter genes, including GLUT-2, GLUT-5, SGLT1, and SGLT4 (Faseleh Jahromi et al., 2016). Lactobacillus enhances occludin levels and suppresses Escherichia coli invasion in intestinal epithelial cells (Resta-Lenert and Barrett, 2003). Moreover, Lactobacillus reverses LPS-induced disruption in tight junction proteins, such as occludin, claudin-1, and ZO-1 (Zhou et al., 2014; Roselli et al., 2017). These findings were consistent with our observations indicating that f_Lactobacillaceae and Lactobacillus were significantly positively correlated with the expression of ZO-1, claudin-1, mucin-2, and occludin, and the occludin expression in the BCG2 group was significantly higher than that in the CT group. In addition, Limosilactobacillus spp. and Lactobacillus spp. have been found to significantly decrease the abundance of Helicobacter, one of the most common pathogens globally associated with gastritis and cancer, by 90% and 83%, respectively, in an infected mouse model (Zhao et al., 2022a). Moreover, Limosilactobacillus spp. administration significantly increased the prevalence of Lactobacillus spp. but decreased the abundance of Desulfovibrio. These relationships among Limosilactobacillus, Lactobacillus, Helicobacter, and Desulfovibrio were consistent with the microbial structure shift induced by B. licheniformis BCG administration. Limosilactobacillus showed a significantly positive correlation with ZO-1 expression and α-amylase activity. These findings indicated that the increase in f_Lactobacillaceae, Lactobacillus, and Limosilactobacillus levels attributed to B. licheniformis BCG treatment improved intestinal barrier function through interaction with epithelial cells in broilers.
Desulfovibrionaceae is a family of opportunistic pathogens such as Desulfovibrio, which is a major sulfate-reducing bacterium that is ubiquitous in human intestines (Ichiishi et al., 2010). These sulfate-reducing bacteria destroy intestinal epithelial cells through generating large amounts of hydrogen sulfide (Zhang-Sun et al., 2015). In agreement with the current findings, B. licheniformis DSM5749 administration sustains intestinal health in laying hens by decreasing the Desulfovibrio level (Pan et al., 2022). Alistipes has been isolated from the appendicular, abdominal, perirectal, and brain abscesses, thus indicating a potential opportunistic pathogenic role in humans (Parker et al., 2020). A high relative abundance of Alistipes is strongly associated with gut disorders and host diseases, such as liver fibrosis and non-alcoholic steatohepatitis, which is inhibited by Bacillus licheniformis H2 administration (Parker et al., 2020; Zhao et al., 2022b). Campylobacter is well known as a major cause of acute bacterial enteritis in humans. Poultry is considered a major reservoir of Campylobacter and generally colonized by Campylobacter at the age of 2 weeks; therefore, Campylobacter may be as a principal vehicle of transmission to humans (Evans and Sayers, 2000; Lamb-Rosteski et al., 2008). Furthermore, Vibrio, a potential pathogen, should be harmful for host health (Richards et al., 2016). Streptococcus and Escherichia coli are pathogens that metabolize proteins in the small intestine (Ma et al., 2017). The above bacteria decreased after B. licheniformis BCG treatment, particularly in the BCG2 group. Dietary supplementation with B. licheniformis BCG significantly decreased the expression of pro-inflammatory molecules, and thus might decrease the risk of bacterial enteritis in broilers and its associated postinfectious sequelae in humans. However, in the present study, the relative abundance of Bacteroides, Prevotellaceae_UCG-003, and Prevotellaceae_NK3B31_group and Ruminococcaceae_UCG-005 were lower in the BCG1 and BCG2 groups than the CT group. As reported, Prevotella can metabolize plant cell walls and produce short chain fatty acids that benefit intestinal immunity homeostasis (Ramayo-Caldas et al., 2016). Prevotella and Prevotella_9 show significantly negative correlations with levels of the pro-inflammatory cytokines IL-6 and IL-12 in the jejunum (Han et al., 2022). Bacteroides shows a significantly negative correlation with serum IL-6 but a positive correlation with IL-4 (Zhang et al., 2022a). The abundance of Ruminococcaceae, beneficial bacteria that are more commonly found in healthy people, is correlated with the production of short chain fatty acids (Koh et al., 2016; Feng et al., 2022). These reports are contrary to those from a present study reporting that bacteria whose abundance decreased after B. licheniformis BCG administration are positively correlated with the pro-inflammatory molecules IL-8, TLR-4, NF-κB, or TNF-α expression; therefore, these findings must be verified in further study.
5. Conclusion
The present study indicated that dietary B. licheniformis BCG significantly increased jejunal and ileal α-amylase, maltase, and sucrase activity; up-regulated ileal occludin mRNA levels; and decreased the transcript abundance of IL-8 and TLR-4 in the ileum in broilers. Diets with B. licheniformis BCG significantly decreased bacterial community richness and diversity in the ileum but not the cecum. Dietary B. licheniformis BCG shaped the ileac microbiota; increased the prevalence of f_Sphingomonadaceae, Sphingomonas, and Limosilactobacillus; contributed to nutrient digestion and absorption; increased the prevalence of f_Lactobacillaceae, Lactobacillus, and Limosilactobacillus; and enhanced intestinal barrier function. In addition, dietary B. licheniformis BCG decreased microbial community diversity; decreased the abundance of Desulfovibrio, Alistipes Campylobacter, Vibrio, Streptococcus, and Escherichia coli-Shigella; and down-regulated expression of inflammatory molecules. Therefore, diets with B. licheniformis BCG contributed to broiler digestion and absorption of nutrients; enhanced the intestinal physical barrier; and decreased intestinal inflammation by decreasing microbial diversity and optimizing the microbiota structure. Our data provided a theoretical basis for B. licheniformis BCG application in broilers.
Data availability statement
The datasets presented in this study can be found in online repositories. The names of the repository/repositories and accession number(s) can be found below: NCBI - PRJNA648691.
Ethics statement
The animal study was reviewed and approved by the Institutional Animal Care and Use Committee of the Institute of Feed Research of Chinese Academy of Agricultural Sciences (FRI-CAAS20210827).
Author contributions
YH, JW, and PY designed the experiments. XX, DL, HZ, and YH conducted experiments and collected samples. JW, XX, and HC performed sample analysis. YH analyzed the data. YH, PY, and KM wrote and revised the manuscript. All authors contributed to the article and approved the submitted version.
Funding
This research was funded by the National Key Research and Development Program of China (2022YFD1300601), the Special Basic Research Fund for Central Public Research Institutes (1610382022010), and the Hebei Province Key Research and Development Program of China (22326624D).
Acknowledgments
We gratefully acknowledge all professors and students in the Institute of Feed Research, Chinese Academy of Agricultural Sciences for their assistance in conducting experiments and revising the article.
Conflict of interest
The authors declare that the research was conducted in the absence of any commercial or financial relationships that could be construed as a potential conflict of interest.
Publisher’s note
All claims expressed in this article are solely those of the authors and do not necessarily represent those of their affiliated organizations, or those of the publisher, the editors and the reviewers. Any product that may be evaluated in this article, or claim that may be made by its manufacturer, is not guaranteed or endorsed by the publisher.
Footnotes
References
Abdelqader, A., Abuajamieh, M., Hayajneh, F., and Al-Fataftah, A. R. (2020). Probiotic bacteria maintain normal growth mechanisms of heat stressed broiler chickens. J. Therm. Biol. 92:102654. doi: 10.1016/j.jtherbio.2020.102654
Aijaz, S., Balda, M. S., and Matter, K. (2006). Tight junctions: Molecular architecture and function. Int. Rev. Cytol. 248, 261–298. doi: 10.1016/s0074-7696(06)48005-0
Akinyemi, F. T., Ding, J., Zhou, H., Xu, K., He, C., Han, C., et al. (2020). Dynamic distribution of gut microbiota during embryonic development in chicken. Poult. Sci. 99, 5079–5090. doi: 10.1016/j.psj.2020.06.016
Antonissen, G., Eeckhaut, V., Van Driessche, K., Onrust, L., Haesebrouck, F., Ducatelle, R., et al. (2016). Microbial shifts associated with necrotic enteritis. Avian. Pathol. 45, 308–312. doi: 10.1080/03079457.2016.1152625
Awad, W. A., Hess, C., and Hess, M. (2017). Enteric pathogens and their toxin-induced disruption of the intestinal barrier through alteration of tight junctions in chickens. Toxins 9:60. doi: 10.3390/toxins9020060
Broer, S. (2008). Amino acid transport across mammalian intestinal and renal epithelia. Physiol. Rev. 88, 249–286. doi: 10.1152/physrev.00018.2006
Chen, Y. C., and Yu, Y. H. (2020). Bacillus licheniformis-fermented products improve growth performance and the fecal microbiota community in broilers. Poult. Sci. 99, 1432–1443. doi: 10.1016/j.psj.2019.10.061
Chen, Z. M., Chang, W. H., Zheng, A. J., Zhang, S., Cai, H. Y., and Liu, G. H. (2018). Comparison of gut microbial diversity in Beijing oil and arbor acres chickens. Rev. Bras. Cienc. Avic. 20, 37–44. doi: 10.1590/1806-9061-2017-0549
Coe, N. R., Smith, A. J., Frohnert, B. I., Watkins, P. A., and Bernlohr, D. A. (1999). The fatty acid transport protein (FATP1) is a very long chain acyl-CoA synthetase. J. Biol. Chem. 274, 36300–36304. doi: 10.1074/jbc.274.51.36300
Collins, S. M., Surette, M., and Bercik, P. (2012). The interplay between the intestinal microbiota and the brain. Nat. Rev. Microbiol. 10, 735–742. doi: 10.1038/nrmicro2876
Council, N. R. (1994). Nutrient requirements of poultry: Ninth revised edition. Washington, DC: The National Academies Press.
Deng, B., Wu, J., Li, X., Men, X., and Xu, Z. (2017). Probiotics and probiotic metabolic product improved intestinal function and ameliorated LPS-induced injury in rats. Curr. Microbiol. 74, 1306–1315. doi: 10.1007/s00284-017-1318-7
Deng, W., Dong, X. F., Tong, J. M., and Zhang, Q. (2012). The probiotic Bacillus licheniformis ameliorates heat stress-induced impairment of egg production, gut morphology, and intestinal mucosal immunity in laying hens. Poult. Sci. 91, 575–582. doi: 10.3382/ps.2010-01293
Dhital, S., Warren, F. J., Butterworth, P. J., Ellis, P. R., and Gidley, M. J. (2017). Mechanisms of starch digestion by alpha-amylase-structural basis for kinetic properties. Crit. Rev. Food Sci. Nutr. 57, 875–892. doi: 10.1080/10408398.2014.922043
Duan, Y., Wang, Y., Dong, H., Ding, X., Liu, Q., Li, H., et al. (2018). Changes in the intestine microbial, digestive, and immune-related genes of litopenaeus vannamei in response to dietary probiotic Clostridium butyricum supplementation. Front. Microbiol. 9:2191. doi: 10.3389/fmicb.2018.02191
Evans, S. J., and Sayers, A. R. (2000). A longitudinal study of Campylobacter infection of broiler flocks in Great Britain. Prev. Vet. Med. 46, 209–223. doi: 10.1016/s0167-5877(00)00143-4
Faseleh Jahromi, M., Wesam Altaher, Y., Shokryazdan, P., Ebrahimi, R., Ebrahimi, M., Idrus, Z., et al. (2016). Dietary supplementation of a mixture of Lactobacillus strains enhances performance of broiler chickens raised under heat stress conditions. Int. J. Biometeorol. 60, 1099–1110. doi: 10.1007/s00484-015-1103-x
Fazelnia, K., Fakhraei, J., Yarahmadi, H. M., and Amini, K. (2021). Dietary supplementation of potential probiotics Bacillus subtilis, Bacillus licheniformis, and Saccharomyces cerevisiae and synbiotic improves growth performance and immune responses by modulation in intestinal system in broiler chicks challenged with Salmonella typhimurium. Probiotics Antimicro 13, 1081–1092. doi: 10.1007/s12602-020-09737-5
Feng, S., Meng, C., Hao, Z., and Liu, H. (2022). Bacillus licheniformis reshapes the gut microbiota to alleviate the subhealth. Nutrients 14:1642. doi: 10.3390/nu14081642
Gadde, U., Oh, S. T., Lee, Y. S., Davis, E., Zimmerman, N., Rehberger, T., et al. (2017). The effects of direct-fed microbial supplementation, as an alternative to antibiotics, on growth performance, intestinal immune status, and epithelial barrier gene expression in broiler chickens. Probiotics Antimicro 9, 397–405. doi: 10.1007/s12602-017-9275-9
Han, Y., Tang, C., Zhao, Q., Fan, S., Yang, P., and Zhang, J. (2022). Butyrate mitigates lipopolysaccharide-induced intestinal morphological changes in weanling piglets by regulating the microbiota and energy metabolism, and alleviating inflammation and apoptosis. Microorganisms 10:2001. doi: 10.3390/microorganisms10102001
Han, Y., Zhao, Q., Tang, C., Li, Y., Zhang, K., Li, F., et al. (2020). Butyrate mitigates weanling piglets from lipopolysaccharide-induced colitis by regulating microbiota and energy metabolism of the gut-liver axis. Front. Microbiol. 11:588666. doi: 10.3389/fmicb.2020.588666
Haque, M. A., Wang, F., Chen, Y., Hossen, F., Islam, M. A., Hossain, M. A., et al. (2021). Bacillus spp. contamination: A novel risk originated from animal feed to human food chains in south-eastern Bangladesh. Front. Microbiol. 12:783103. doi: 10.3389/fmicb.2021.783103
Hildebrandt, M. A., Hoffmann, C., Sherrill-Mix, S. A., Keilbaugh, S. A., Hamady, M., Chen, Y. Y., et al. (2009). High-fat diet determines the composition of the murine gut microbiome independently of obesity. Gastroenterology 137, 1716–1724. doi: 10.1053/j.gastro.2009.08.042
Huang, P., Zhang, Y., Xiao, K., Jiang, F., Wang, H., Tang, D., et al. (2018). The chicken gut metagenome and the modulatory effects of plant-derived benzylisoquinoline alkaloids. Microbiome 6:211. doi: 10.1186/s40168-018-0590-5
Huebener, P., and Schwabe, R. F. (2013). Regulation of wound healing and organ fibrosis by toll-like receptors. Biochim. Biophys. Acta. 1832, 1005–1017. doi: 10.1016/j.bbadis.2012.11.017
Hung, D., Cheng, Y., Chen, W., Hua, K., Pietruszka, A., Dybus, A., et al. (2019). Bacillus licheniformis-fermented products reduce diarrhea incidence and alter the fecal microbiota community in weaning piglets. Animals 9:1145. doi: 10.3390/ani9121145
Ichiishi, S., Tanaka, K., Nakao, K., Izumi, K., Mikamo, H., and Watanabe, K. (2010). First isolation of Desulfovibrio from the human vaginal flora. Anaerobe 16, 229–233. doi: 10.1016/j.anaerobe.2010.02.002
Jazi, V., Mohebodini, H., Ashayerizadeh, A., Shabani, A., and Barekatain, R. (2019). Fermented soybean meal ameliorates Salmonella typhimurium infection in young broiler chickens. Poult. Sci. 98, 5648–5660. doi: 10.3382/ps/pez338
Jiang, Z., Zhou, Y., Lu, F., Han, Z., and Wang, T. (2008). Effects of different levels of supplementary alpha-amylase on digestive enzyme activities and pancreatic amylase mRNA expression of young broilers. Asian-Austral J. Anim. Sci. 21, 97–102. doi: 10.5713/ajas.2008.70110
Jumpertz, R., Le, D. S., Turnbaugh, P. J., Trinidad, C., Bogardus, C., Gordon, J. I., et al. (2011). Energy-balance studies reveal associations between gut microbes, caloric load, and nutrient absorption in humans. Am. J. Clin. Nutr. 94, 58–65. doi: 10.3945/ajcn.110.010132
Kaczmarek, S. A., Rogiewicz, A., Mogielnicka, M., Rutkowski, A., Jones, R. O., and Slominski, B. A. (2014). The effect of protease, amylase, and nonstarch polysaccharide-degrading enzyme supplementation on nutrient utilization and growth performance of broiler chickens fed corn-soybean meal-based diets. Poult. Sci. 93, 1745–1753. doi: 10.3382/ps.2013-03739
Kaewtapee, C., Burbach, K., Tomforde, G., Hartinger, T., Camarinha-Silva, A., Heinritz, S., et al. (2017). Effect of Bacillus subtilis and Bacillus licheniformis supplementation in diets with low- and high-protein content on ileal crude protein and amino acid digestibility and intestinal microbiota composition of growing pigs. J. Anim. Sci. Biotechnol. 8:37. doi: 10.1186/s40104-017-0168-2
Kan, L., Guo, F., Liu, Y., Pham, V. H., Guo, Y., and Wang, Z. (2021). Probiotics Bacillus licheniformis improves intestinal health of subclinical necrotic enteritis-challenged broilers. Front. Microbiol. 12:623739. doi: 10.3389/fmicb.2021.623739
Kim, Y., Cho, J. Y., Kuk, J. H., Moon, J. H., Cho, J. I., Kim, Y. C., et al. (2004). Identification and antimicrobial activity of phenylacetic acid produced by Bacillus licheniformis isolated from fermented soybean, chungkook-jang. Curr. Microbiol. 48, 312–317. doi: 10.1007/s00284-003-4193-3
Knap, I., Lund, B., Kehlet, A. B., Hofacre, C., and Mathis, G. (2010). Bacillus licheniformis prevents necrotic enteritis in broiler chickens. Avian. Dis. 54, 931–935. doi: 10.1637/9106-101509-ResNote.1
Koh, A., De Vadder, F., Kovatcheva-Datchary, P., and Backhed, F. (2016). From dietary fiber to host physiology: Short-chain fatty acids as key bacterial metabolites. Cell 165, 1332–1345. doi: 10.1016/j.cell.2016.05.041
Konieczka, P., Nowicka, K., Madar, M., Taciak, M., and Smulikowska, S. (2018). Effects of pea extrusion and enzyme and probiotic supplementation on performance, microbiota activity and biofilm formation in the broiler gastrointestinal tract. Br. Poult. Sci. 59, 654–662. doi: 10.1080/00071668.2018.1507017
Konieczka, P., Sandvang, D., Kinsner, M., Szkopek, D., Szyrynska, N., and Jankowski, J. (2022). Bacillus-based probiotics affect gut barrier integrity in different ways in chickens subjected to optimal or challenge conditions. Vet. Microbiol. 265:109323. doi: 10.1016/j.vetmic.2021.109323
Krajmalnik-Brown, R., Ilhan, Z. E., Kang, D. W., and DiBaise, J. K. (2012). Effects of gut microbes on nutrient absorption and energy regulation. Nutr. Clin. Pract. 27, 201–214. doi: 10.1177/0884533611436116
Kridtayopas, C., Rakangtong, C., Bunchasak, C., and Loongyai, W. (2019). Effect of prebiotic and synbiotic supplementation in diet on growth performance, small intestinal morphology, stress, and bacterial population under high stocking density condition of broiler chickens. Poult. Sci. 98, 4595–4605. doi: 10.3382/ps/pez152
Lamb-Rosteski, J. M., Kalischuk, L. D., Inglis, G. D., and Buret, A. G. (2008). Epidermal growth factor inhibits Campylobacter jejuni-induced claudin-4 disruption, loss of epithelial barrier function, and Escherichia coli translocation. Infect. Immun. 76, 3390–3398. doi: 10.1128/IAI.01698-07
Li, Q., Li, L., Chen, Y., Yu, C., Azevedo, P., Gong, J., et al. (2022a). Bacillus licheniformis PF9 improves barrier function and alleviates inflammatory responses against enterotoxigenic Escherichia coli F4 infection in the porcine intestinal epithelial cells. J. Anim. Sci. Biotechnol. 13:86. doi: 10.1186/s40104-022-00746-8
Li, Y., Han, Y., Zhao, Q., Tang, C., Zhang, J., and Qin, Y. (2022b). Fermented soy and fish protein dietary sources shape ileal and colonic microbiota, improving nutrient digestibility and host health in a piglet model. Front. Microbiol. 13:911500. doi: 10.3389/fmicb.2022.911500
Li, R., Hou, G., Jiang, X., Song, Z., Fan, Z., Hou, D. X., et al. (2019). Different dietary protein sources in low protein diets regulate colonic microbiota and barrier function in a piglet model. Food Funct. 10, 6417–6428. doi: 10.1039/c9fo01154d
Li, S., Yan, C., Liu, T., Xu, C., Wen, K., Liu, L., et al. (2020). Research note: Increase of bad bacteria and decrease of good bacteria in the gut of layers with vs. without hepatic steatosis. Poult. Sci. 99, 5074–5078. doi: 10.1016/j.psj.2020.07.007
Liu, X., Yan, H., Lv, L., Xu, Q., Yin, C., Zhang, K., et al. (2012). Growth performance and meat quality of broiler chickens supplemented with Bacillus licheniformis in drinking water. Asian-Australas J. Anim. Sci. 25, 682–689. doi: 10.5713/ajas.2011.11334
Ma, N., Tian, Y., Wu, Y., and Ma, X. (2017). Contributions of the interaction between dietary protein and gut microbiota to intestinal health. Curr. Protein Pept. Sci. 18, 795–808. doi: 10.2174/1389203718666170216153505
Mach, N., Berri, M., Estelle, J., Levenez, F., Lemonnier, G., Denis, C., et al. (2015). Early-life establishment of the swine gut microbiome and impact on host phenotypes. Environ. Microbiol. Rep. 7, 554–569. doi: 10.1111/1758-2229.12285
Micah, H., Claire, F.-L., Rob, K., and Gordon Jeffrey, I. (2007). The human microbiome project: Exploring the microbial part of ourselves in a changing world. Nature 449, 804–810. doi: 10.1038/nature06244
Mingmongkolchai, S., and Panbangred, W. (2018). Bacillus probiotics: An alternative to antibiotics for livestock production. J. Appl. Microbiol. 124, 1334–1346. doi: 10.1111/jam.13690
Musa, B. B., Duan, Y., Khawar, H., Sun, Q., Ren, Z., Elsiddig Mohamed, M. A., et al. (2019). Bacillus subtilis B21 and Bacillus licheniformis B26 improve intestinal health and performance of broiler chickens with Clostridium perfringens-induced necrotic enteritis. J. Anim. Physiol. Anim. Nutr. 103, 1039–1049. doi: 10.1111/jpn.13082
Neag, M. A., Catinean, A., Muntean, D. M., Pop, M. R., Bocsan, C. I., Botan, E. C., et al. (2020). Probiotic Bacillus spores protect against acetaminophen induced acute liver injury in rats. Nutrients 12:632. doi: 10.3390/nu12030632
Nylund, L., Satokari, R., Nikkilä, J., Rajilić-Stojanović, M., Kalliomäki, M., Isolauri, E., et al. (2013). Microarray analysis reveals marked intestinal microbiota aberrancy in infants having eczema compared to healthy children in at-risk for atopic disease. BMC microbiol. 13:12. doi: 10.1186/1471-2180-13-12
Oakley, B. B., Lillehoj, H. S., Kogut, M. H., Kim, W. K., Maurer, J. J., Pedroso, A., et al. (2014). The chicken gastrointestinal microbiome. FEMS Microbiol. Lett. 360, 100–112. doi: 10.1111/1574-6968.12608
Pan, X., Cai, Y., Kong, L., Xiao, C., Zhu, Q., and Song, Z. (2022). Probiotic effects of Bacillus licheniformis DSM5749 on growth performance and intestinal microecological balance of laying hens. Front. Nutr. 9:868093. doi: 10.3389/fnut.2022.868093
Parker, B. J., Wearsch, P. A., Veloo, A. C. M., and Rodriguez-Palacios, A. (2020). The genus Alistipes: Gut bacteria with emerging implications to inflammation, cancer, and mental health. Front. Immunol. 11:906. doi: 10.3389/fimmu.2020.00906
Poulsen, A. R., Jonge, N., Nielsen, J. L., Hojberg, O., Lauridsen, C., Cutting, S. M., et al. (2018). Impact of Bacillus spp. spores and gentamicin on the gastrointestinal microbiota of suckling and newly weaned piglets. PLoS One 13:e0207382. doi: 10.1371/journal.pone.0207382
Ramayo-Caldas, Y., Mach, N., Lepage, P., Levenez, F., Denis, C., Lemonnier, G., et al. (2016). Phylogenetic network analysis applied to pig gut microbiota identifies an ecosystem structure linked with growth traits. ISME J. 10, 2973–2977. doi: 10.1038/ismej.2016.77
Resta-Lenert, S., and Barrett, K. (2003). Live probiotics protect intestinal epithelial cells from the effects of infection with enteroinvasive Escherichia coli (EIEC). Gut 52, 988–997. doi: 10.1136/gut.52.7.988
Richards, G. P., Fay, J. P., Uknalis, J., Olanya, O. M., and Watson, M. A. (2016). Purification and host specificity of predatory Halobacteriovorax isolates from seawater. Appl. Environ. Microbiol. 82, 922–927. doi: 10.1128/AEM.03136-15
Richieri, G., Ogata, R., and Kleinfeld, A. (1999). Fatty acid interactions with native and mutant fatty acid binding proteins. Mol. Cell Biochem. 192, 77–85. doi: 10.1007/978-1-4615-4929-1_9
Rohith, H. S., and Halami, P. M. (2021a). The combined effect of potential probiotic Bacillus licheniformis MCC 2514 and Bifidobacterium breve NCIM 5671 towards anti-inflammatory activity on HT-29 cell lines. Probiotics Antimicro doi: 10.1007/s12602-021-09851-y [Epub ahead of print].
Rohith, H. S., and Halami, P. M. (2021b). In vitro validation studies for adhesion factor and adhesion efficiency of probiotic Bacillus licheniformis MCC 2514 and Bifidobacterium breve NCIM 5671 on HT-29 cell lines. Arch. Microbiol. 203, 2989–2998. doi: 10.1007/s00203-021-02257-y
Roselli, M., Pieper, R., Rogel-Gaillard, C., de Vries, H., Bailey, M., Smidt, H., et al. (2017). Immunomodulating effects of probiotics for microbiota modulation, gut health and disease resistance in pigs. Anim. Feed Sci. Tech. 233, 104–119. doi: 10.1016/j.anifeedsci.2017.07.011
Sanders, M. E., Merenstein, D. J., Reid, G., Gibson, G. R., and Rastall, R. A. (2019). Probiotics and prebiotics in intestinal health and disease: From biology to the clinic. Nat. Rev. Gastroenterol Hepatol. 16, 605–616. doi: 10.1038/s41575-019-0199-6
Song, J., Xiao, K., Ke, Y. L., Jiao, L. F., Hu, C. H., Diao, Q. Y., et al. (2014). Effect of a probiotic mixture on intestinal microflora, morphology, and barrier integrity of broilers subjected to heat stress. Poult. Sci. 93, 581–588. doi: 10.3382/ps.2013-03455
Strandwitz, P. (2018). Neurotransmitter modulation by the gut microbiota. Brain Res. 1693, 128–133. doi: 10.1016/j.brainres.2018.03.015
van der Wielen, P. W., Keuzenkamp, D. A., Lipman, L. J., van Knapen, F., and Biesterveld, S. (2002). Spatial and temporal variation of the intestinal bacterial community in commercially raised broiler chickens during growth. Microb. Ecol. 44, 286–293. doi: 10.1007/s00248-002-2015-y
Wang, J., Chen, L., Li, D., Yin, Y., Wang, X., Li, P., et al. (2008). Intrauterine growth restriction affects the proteomes of the small intestine, liver, and skeletal muscle in newborn pigs. J. Nutr. 138, 60–66. doi: 10.1093/jn/138.1.60
Wang, J., Han, Y., Cai, H., Wen, Z., Xu, X., Zhao, Z., et al. (2022). Effects of different levels of Bacillus licheniformis on growth performance, immune and intestinal morphology of broilers. China Anim. Husb. Vet. Med. 49, 4593–4603. doi: 10.16431/j.cnki.1671-7236.2022.12.000
Wang, W., Li, Z., Han, Q., Guo, Y., Zhang, B., and D’Inca, R. (2016). Dietary live yeast and mannan-oligosaccharide supplementation attenuate intestinal inflammation and barrier dysfunction induced by Escherichia coli in broilers. Br. J. Nutr. 116, 1878–1888. doi: 10.1017/S0007114516004116
Wang, W., Wang, J., Zhang, H., Wu, S., and Qi, G. (2020). Effects of Clostridium butyricum on production performance and intestinal absorption function of laying hens in the late phase of production. Anim. Feed Sci. Tech. 264:114476. doi: 10.1016/j.anifeedsci.2020.114476
Wang, Y., Du, W., Lei, K., Wang, B., Wang, Y., Zhou, Y., et al. (2017). Effects of dietary Bacillus licheniformis on gut physical barrier, immunity, and reproductive hormones of laying hens. Probiotics Antimicro. 9, 292–299. doi: 10.1007/s12602-017-9252-3
Xu, Y., Yu, Y., Shen, Y., Li, Q., Lan, J., Wu, Y., et al. (2021). Effects of Bacillus subtilis and Bacillus licheniformis on growth performance, immunity, short chain fatty acid production, antioxidant capacity, and cecal microflora in broilers. Poult. Sci. 100:101358. doi: 10.1016/j.psj.2021.101358
Yang, G. Y., Zhu, Y. H., Zhang, W., Zhou, D., Zhai, C. C., and Wang, J. F. (2016). Influence of orally fed a select mixture of Bacillus probiotics on intestinal T-cell migration in weaned MUC4 resistant pigs following Escherichia coli challenge. Vet. Res. 47:71. doi: 10.1186/s13567-016-0355-8
Yang, J., Huang, K., Wang, J., Wu, D., Liu, Z., Yu, P., et al. (2021). Combined use of Bacillus subtilis yb-114,246 and Bacillus licheniformis yb-214,245 improves body growth performance of chinese huainan partridge shank chickens by enhancing intestinal digestive profiles. Probiotics Antimicro. 13, 327–342. doi: 10.1007/s12602-020-09691-2
Zhang, R., Shi, X., Chen, Y., Liu, J., Wu, Y., and Xu, Y. (2022a). Multi-omics revealed the protective effects of phamnolipids in lipopolysaccharide challenged broilers. Front. Immunol. 13:824664. doi: 10.3389/fimmu.2022.824664
Zhang, X., Hu, Y., Ansari, A. R., Akhtar, M., Chen, Y., Cheng, R., et al. (2022b). Caecal microbiota could effectively increase chicken growth performance by regulating fat metabolism. Microb. Biotechnol. 15, 844–861. doi: 10.1111/1751-7915.13841
Zhang-Sun, W., Augusto, L. A., Zhao, L., and Caroff, M. (2015). Desulfovibrio desulfuricans isolates from the gut of a single individual: Structural and biological lipid A characterization. FEBS Lett. 589, 165–171. doi: 10.1016/j.febslet.2014.11.042
Zhao, Y., Li, Z., Zhao, L., Wang, J., Wang, F., Zhang, Q., et al. (2022a). Two novel lactic acid bacteria, Limosilactobacillus fermentum MN-LF23 and Lactobacillus gasseri MN-LG80, inhibited Helicobacter pylori infection in C57BL/6 mice. Food Funct. 13, 11061–11069. doi: 10.1039/d2fo02034c
Zhao, Y., Zeng, Y., Zeng, D., Wang, H., Sun, N., Xin, J., et al. (2022b). Dietary probiotic supplementation suppresses subclinical necrotic enteritis in broiler chickens in a microbiota-dependent manner. Front. Immunol. 13:855426. doi: 10.3389/fimmu.2022.855426
Zhou, H., Wu, Y., Sun, X., Yin, D., Wang, Y., Mahmood, T., et al. (2021). Effects of exogenous alpha-(1,4)-amylase on the utilisation of corn starch and glucose metabolism in broiler chickens. Animal 15:100396. doi: 10.1016/j.animal.2021.100396
Zhou, M., Zeng, D., Ni, X., Tu, T., Yin, Z., Pan, K., et al. (2016). Effects of Bacillus licheniformis on the growth performance and expression of lipid metabolism-related genes in broiler chickens challenged with Clostridium perfringens-induced necrotic enteritis. Lipids Health Dis. 15:48. doi: 10.1186/s12944-016-0219-2
Zhou, M., Zhu, J., Yu, H., Yin, X., Sabour, P. M., Zhao, L., et al. (2014). Investigation into in vitro and in vivo models using intestinal epithelial IPEC-J2 cells and Caenorhabditis elegans for selecting probiotic candidates to control porcine enterotoxigenic Escherichia coli. J. Appl. Microbiol. 117, 217–226. doi: 10.1111/jam.12505
Keywords: Bacillus licheniformis BCG, broiler, digestive enzyme activity, nutrient transporter, ileac and cecum microbiota, intestinal inflammation, tight junction
Citation: Han Y, Xu X, Wang J, Cai H, Li D, Zhang H, Yang P and Meng K (2023) Dietary Bacillus licheniformis shapes the foregut microbiota, improving nutrient digestibility and intestinal health in broiler chickens. Front. Microbiol. 14:1113072. doi: 10.3389/fmicb.2023.1113072
Received: 01 December 2022; Accepted: 23 January 2023;
Published: 10 February 2023.
Edited by:
Jinxin Liu, Nanjing Agricultural University, ChinaReviewed by:
Shiyu Tao, Huazhong Agricultural University, ChinaLiang Chen, Institute of Animal Sciences (CAAS), China
Copyright © 2023 Han, Xu, Wang, Cai, Li, Zhang, Yang and Meng. This is an open-access article distributed under the terms of the Creative Commons Attribution License (CC BY). The use, distribution or reproduction in other forums is permitted, provided the original author(s) and the copyright owner(s) are credited and that the original publication in this journal is cited, in accordance with accepted academic practice. No use, distribution or reproduction is permitted which does not comply with these terms.
*Correspondence: Kun Meng, bWVuZ2t1bkBjYWFzLmNu; Peilong Yang,
eWFuZ3BlaWxvbmdAY2Fhcy5jbg==