- 1Laboratorio de Biología Molecular de Bacterias y Levaduras, Departamento de Microbiología, Escuela Nacional de Ciencias Biológicas, Instituto Politécnico Nacional, Ciudad de México, Mexico
- 2Laboratorio de Aprovechamiento Integral de Recursos Bióticos, Universidad Politécnica de Pachuca, Hidalgo, Mexico
- 3Laboratorio de Recursos Genéticos Microbianos, Centro Nacional de Recursos Genéticos, INIFAP, Jalisco, Mexico
Plant growth-promoting bacteria (PGPB) are a source of nutrient supply, stimulate plant growth, and even act in the biocontrol of phytopathogens. However, these phenotypic traits have rarely been explored in culturable bacteria from native maize landraces. In this study, synthetic microbial communities (SynCom) were assembled with a set of PGPB isolated from the Jala maize landrace, some of them with additional abilities for the biocontrol of phytopathogenic fungi and the stimulation of plant-induced systemic resistance (ISR). Three SynCom were designed considering the phenotypic traits of bacterial strains, including Achromobacter xylosoxidans Z2K8, Burkholderia sp. Z1AL11, Klebsiella variicola R3J3HD7, Kosakonia pseudosacchari Z2WD1, Pantoea ananatis E2HD8, Pantoea sp. E2AD2, Phytobacter diazotrophicus Z2WL1, Pseudomonas protegens E1BL2, and P. protegens E2HL9. Plant growth promotion in gnotobiotic and greenhouse seedlings assays was performed with Conejo landrace; meanwhile, open field tests were carried out on hybrid CPL9105W maize. In all experimental models, a significant promotion of plant growth was observed. In gnotobiotic assays, the roots and shoot length of the maize seedlings increased 4.2 and 3.0 times, respectively, compared to the untreated control. Similarly, the sizes and weights of the roots and shoots of the plants increased significantly in the greenhouse assays. In the open field assay performed with hybrid CPL9105W maize, the yield increased from 11 tons/ha for the control to 16 tons/ha inoculated with SynCom 3. In addition, the incidence of rust fungal infections decreased significantly from 12.5% in the control to 8% in the treatment with SynCom 3. All SynCom designs promoted the growth of maize in all assays. However, SynCom 3 formulated with A. xylosoxidans Z2K8, Burkholderia sp. Z1AL11, K. variicola R3J3HD7, P. ananatis E2HD8, P. diazotrophicus Z2WL1, and P. protegens E1BL2 displayed the best results for promoting plant growth, their yield, and the inhibition of fungal rust. This study demonstrated the biotechnological eco-friendly plant growth-promoting potential of SynCom assemblies with culturable bacteria from native maize landraces for more sustainable and economic agriculture.
1. Introduction
Plants and their microbiome are holobionts that coevolve modulating or enhancing the adaptation to the environment, fitness, competitivity, phytopathogen resistance, abiotic stress tolerance, health, and productivity of plants in natural or agricultural ecosystems (Chiu and Gilbert, 2015; Berg et al., 2021; Cesaro et al., 2021). Abundant studies on plant–bacteria interactions are available in the scientific literature, and their potential biotechnological applications to agriculture as bioinoculants have a significant market share (Nehra and Choudhary, 2015; Owen et al., 2015; Saritha and Tollamadugu, 2019; Nosheen et al., 2021). Plant–bacteria holobiont interactions are extraordinarily complex because the flow of metabolites between both components is bidirectional (Selosse et al., 2004; Wani et al., 2015; Sánchez-Cañizares et al., 2017; Hawkes et al., 2020). Bioinoculants and synthetic microbial communities (SynCom) contain microbial species that can synthesize several plant growth-promoting (PGP) and biopesticide metabolites (Bashan, 1998; Johnsen et al., 2001; Cavali et al., 2010; Souza et al., 2020; Kaur et al., 2022; Prigigallo et al., 2022; Shayanthan et al., 2022). The expression of these synergistic activities by specialized microbial species can be continued or alternated to maintain a constant rate of metabolite incorporation even under different environmental conditions and/or stages of the plant life cycle (Maier et al., 2009; Rana et al., 2011; Babu et al., 2015; Emami et al., 2019; Magallon-Servin et al., 2020; Hett et al., 2022).
The main source for bioinoculants or SynCom is the culturable fraction of autochthonous bacteria from the endosphere or rhizosphere of agricultural plants. However, the selection pressures derived from the use of abundant artificial fertilizers, pesticides, improved and genetically homogeneous varieties of plants, and antibiotics and antifungals used to control phytopathogens in seeds may have contributed to the loss of alpha diversity of plant growth-promoting bacteria (PGPB) in the endosphere of domesticated plants used in intensive agriculture (Lynch et al., 2004; Hussain et al., 2009; Zaller et al., 2016; Berg et al., 2017; Ohore et al., 2022). This decrease in microbial biodiversity in the endosphere of commercial plants has some implications for losing their tolerance to abiotic and biotic stress (Gutierrez and Grillo, 2022). This diversity loss has previously been observed in chicory roots (Verdin et al., 2006), soybeans (Vieira et al., 2007), and some legumes (Fox et al., 2007). Futhermore, the diversity of the seed microbiota of several cereal crops and rice has generally been shown to be higher in cultivated cereals than in wild ancestors, while more microbe–microbe interactions have been detected in wild relative species (Kim et al., 2020; Abdullaeva et al., 2021). In any case, the diversity of the endophytic microorganisms of plants used in traditional agriculture with wild, native, and ancestral plants helps maintain a potential pool of novel plant growth-promoting microorganism strains used in sustainable agriculture (Vibha and Neelam, 2012; Berg et al., 2017).
Maize (Zea mays) is the most produced and consumed grain globally, moreover, having the largest planting area worldwide. Maize is a primary consumer product in numerous countries (International Grains Council, 2022un). The enormous world demand for this cereal requires an increase in the area of available arable land as well as the use of all available biotechnological resources such as fertilizers and pesticides, enhanced varieties and transgenic plants, and plant-growth promoting (PGP) microorganisms capable of antagonizing phytopathogens (Compant et al., 2005; Roriz et al., 2020; Al-Tammar and Khalifa, 2022). Currently, a total of 59 landraces of native maize have been described only in México (Hellin et al., 2014). However, relatively few studies have focused on investigating the bacteria from the microbiomes of native maize landraces (Bodhankar et al., 2017; Van Deynze et al., 2018; Chavéz-Díaz et al., 2022; Lund et al., 2022). However, they may offer an opportunity to expand the microbial options for new bioinoculants and SynCom.
In this study, nine endophytic PGP and fungal antagonist bacteria previously isolated from the endosphere of the Jala landrace maize were incorporated into the design of three SynCom (SynCom 1, 2, and 3). Following compatibility tests among strains, the PGP, extracellular enzyme production, the activity of the enzyme phenylalanine ammonia-lyase (PAL), and antifungal in vitro capacities of SynCom were evaluated. All SynCom presented relevant phenotypic traits, but SynCom 3 stood out. Plant growth-promoting gnotobiotic and greenhouse assays performed with Conejo landrace seedlings revealed that SynCom 3 significantly increased the length and dry weight of plant shoots and roots compared to the uninoculated control. In addition, the open field experiment performed with hybrid CPL9105W maize inoculated with SynCom 3 significantly increased the maize grain yield and moderately decreased the incidence of fungal rust. This is the first study exploring the potential of endophytic strains of native Mexican maize breeds to formulate synthetic communities and implement them for the improvement of other native maize and improved hybrid maize. This underscores the significance of utilizing these native resources in the rational design of SynCom to foster sustainable agriculture practices.
2. Materials and methods
2.1. Biological materials
Most of the PGP bacterial strains used in this study were previously isolated from the maize endosphere (steam, root, and seed) of the Jala landrace (Rios-Galicia et al., 2021). Burkholderia sp. Z1AL11, Pantoea sp. E2AD2, and P. ananatis E2HD8 were isolated from a second sampling (Table 1). The strains stored at−70°C were recovered in a solid Luria Bertani (LB) medium and incubated at 28°C for 48 h.
2.2. Compatibility assay
Compatibility was determined by simultaneous inhibition tests to recognize antagonistic interactions among the strains. Overnight cultures of the nine strains were adjusted to an OD600nm of 0.8. A total of 0.1 mL of the suspension was inoculated and distributed on the surface of a Müeller-Hinton (MH) medium. The plates were incubated at 28°C for 48 h. Following the incubation, agar punches were placed on the surface of the MH medium that had previously been massively inoculated with each strain. These plates were pre-incubated at 4°C for 2 h to promote the diffusion of metabolites in the agar and subsequently incubated at 28°C for 24–48 h (Pérez et al., 2014). The inhibition halos represented a negative interaction between each pair of strains. The inhibition pattern among the strains was an important criterion to formulate the SynCom used as bioinoculants.
2.3. SynCom formulation
To create the SynCom formulation, the complementary activities of its constituent strains were deemed crucial. This involved ensuring that pairs of strains that demonstrated inhibition in previous tests (2.2) were not included in the same formulation to ensure compatibility. The final SynCom contained strains with complementary phenotypic traits, including nitrogen fixation capability, phosphate solubilization, metallophore production, indoleacetic acid (IAA) production, and ACC deaminase and antifungal activity. Each bacteria strain was grown individually in LB liquid media to the exponential phase. A viable account was carried out to be able to adjust the population density of each bacteria in the formulation at 1.4 × 107 CFU/mL for SynCom 1.2 × 107 CFU/mL for SynCom 2, and 1.4 × 107 CFU/mL for SynCom 3, so that when mixed in a single liquid formulation in LB media, each SynCom has a final density of 1 × 108 CFU/mL, and for subsequent analysis, aliquots of this bacterial mixtures were taken (Armanhi et al., 2018; Rios-Galicia et al., 2021).
2.4. Biological nitrogen fixation
The nitrogenase activity was indirectly estimated with an acetylene reduction assay using gas chromatography (Hardy et al., 1968). The axenic bacterial strains and SynCom were grown in sealed bottles containing 5 mL of a semisolid BMGM medium with 50 μL of bacterial suspension adjusted to a final 1x108 CFU/mL concentration. The bottles were sealed and incubated for 48 h at 28°C; K. variicola 6A3 was used as a positive control (Rios-Galicia et al., 2021). A volume of 400 μL of the gas contained in the vial was replaced by the same volume of acetylene gas and incubated for up to 6 h, after which the atmosphere of the vial was analyzed by gas chromatography in a Perkin Elmer, Inc. Clarus 580® Ethylene production and residual acetylene (nmol h−1) were estimated by integrating the area under the curve.
2.5. Phosphate solubilization
The semiquantitative inorganic phosphate solubilization of each axenic bacteria and SynCom were evaluated in plates containing NBRIP medium [10 g/L glucose, 5 g/L Ca3(PO4)2, 5 g/L MgCl2•6H2O, 0.25 g/L MgSO4•6H2O, 0.2 g/L KCl, 0.1 g/L (NH4)2SO4 and 16 g/L agar] (Nautiyal, 1999). After 3–5 days of incubation, the solubilization halos around the colonies were measured, and the solubilization indices were reported as the products of the dividing halo and colony diameters (Rashid et al., 2004). The phosphate solubilization by bacteria was quantitatively evaluated in 10 mL of NBRIP liquid medium supplemented with 0.5% hydroxyapatite inoculated 0.1 mL of the adjusted suspension of each SynCom. After an incubation period at 28°C for 5 days, 0.2 mL of aliquot was taken and centrifuged at 13,000 rpm for 10 min. In total, 64 μL of the supernatant was taken and mixed with 16 μL of 0.01 M NaMoO4, 80 μL of 0.1 M ascorbic acid, and 40 μL of 5% acetic acid. Absorbance was read at 580 nm (He and Honeycutt, 2005; Bashan et al., 2013).
2.6. Indoleacetic acid production
The indoleacetic acid (IAA) production of axenic bacteria and SynCom was evaluated using the colorimetric method employing a Salkowski reagent (0.5 M FeCl3 and 35% HClO4), which reacts with the indole ring of several related compounds giving it a reddish color. A standard curve of IAA was produced at concentrations ranging from 2 and 200 μg/mL using the Salkowski technique and read at 530 nm (Gordon and Weber, 1951; Szkop et al., 2012).
2.7. Metallophore production
The semiquantitative production of metallophores was determined in Chrome Azurol S medium (CAS) plates supplemented with different ion solutions for every metallophore in particular Fe2(SO4)3, CuSO4, Na2MoO4, NaVO3, CaSO4, MgSO4, and ZnSO4 (Rios-Galicia et al., 2021). Spots of 5 μL of each axenic bacteria and SynCom were placed on a medium surface, and the plates were incubated at 28°C for 48 h. The yellow halos around the colonies indicated the production of the chelating metallophores of corresponding metal ions. The chelation indices were reported as the products of the dividing halo and colony diameters (Payne, 1994). The metallophores were quantitatively estimated in a liquid CAS medium. The metallophore concentration was proportional to the reduction in blue color intensity produced by removing metals from the chelation CAS-ion complex (Jikare and Chavan, 2013). Bacterial SynCom was grown in a flask containing 10 mL of 10 g/L peptone and 5 g/L NaCl for 48 h at 28°C. After incubation, 0.1 mL aliquots were centrifuged at 13,000 rpm for 15 min. A total of 50 μL of supernatant were added and mixed with 50 μL of CAS solution in a 96-well plate, and the mixture was left to react for 20 min at 25°C. Subsequently, the absorbance at 630 nm was read. An uninoculated medium was used as a control. The chelation percentages were calculated using the following formula:
where:
AR = Absorbance of the reference (CAS medium without inoculation)
AS = Absorbance of the test sample (CAS medium with SynCom inoculation)
2.8. Aminocyclopropane-1-carboxylate (ACC) deaminase production
The production of the ACC deaminase enzyme was indirectly determined by the bacterial population growth in LGI culture medium (50 g/L sucrose, 0.01 g/L FeCl3•6H2O, 0.8 g/L K2HPO4, 0.2 g/L MgSO4•7H2O, and 0.002 g/L Na2MoO4•2H2O) supplemented with 1 g/L ACC as the sole source of nitrogen. A total of 180 μL of the liquid LGI culture supplemented with 1 g/L of ACC medium were inoculated with 20 μL of the suspension of each SynCom (0.04 OD600nm) in 96-well plates (Johnston-Monje and Raizada, 2011). The plates were incubated at 28°C, and DO600 was determined every 24 h. The negative control was prepared with an LGI medium without ACC.
2.9. Growth inhibition of fungal phytopathogens
The antagonist effect of the axenic bacteria and SynCom were evaluated against several phytopathogenic fungal strains isolated from plant tissues with symptoms including Fusarium oxysporum (wilting), Pestalotia sp. (ear rot), Curvularia sp. (leaf spot), Colletotrichum sp. (anthracnose), Helminthosporium sp. (leaf blight). A 0.8 OD600 streak of each SynCom suspension was placed at the center of the plate, and an agar fragment containing mycelial fungal growth was placed on each side. The plates were incubated at 28°C for 5 days (El-Sayed and Edrees, 2014). The percentage of radial growth inhibition (PRGI) was obtained with the following formula:
where:
R1 = Major radius of phytopathogen growth
R2 = Minor radius of inhibition of phytopathogen growth
A commercial aqueous solution of 400 g/100 L copper chloride oxide (CUPRAVIT®, Bayer) was used as a positive control for the inhibition.
2.10. PAL activity determination for ISR
Germinated fungus-free seeds were placed in 100 g sterile, moistened vermiculite bags. The bags were kept at room temperature for 12 h in light and 12 h in darkness. A total of 5 mL of SynCom or control treatments adjusted to OD600nm of 1 were placed on the base of the shoot on the substrate line. The plants were sampled at vegetative stages VE, V1, and V2 (3, 9, and 12 days, respectively) to determine their height, aerial weight, and root weight. The shoot and leaves were taken separately from the root from these samples and frozen at −20°C until use. To obtain the raw enzyme extracts, the plant tissues were macerated with a lysis solution containing 1% SDS, 3% PVP, and 0.037% EDTA in a ratio of 1:10 P/V until a homogeneous solution was obtained. The tissues were lysed through ten cycles of vortex mixing 1 mL of homogenized tissues and 0.5 g of glass beads in 2 mL cryotubes for 1 min before being placed in an ice bath for 1 min. The lysate was centrifuged at 12,000 rpm at 4°C for 10 min, and the supernatant or cell-free extract was collected. The enzymatic activity of phenylalanine ammonium lyase (PAL) was determined with 200 μL of the cell-free extract and 1,355 μL of 5 mM phenylalanine as a substrate in a 5 mM sodium borate buffer solution having a pH of 8. The mixture was stirred and incubated for 45 min at 25°C. After the incubation, the reaction was stopped with 235 μL of 10% trichloroacetic acid in an ice bath. The samples were read at 290 nm. The PAL unit was defined as the amount of enzyme that allowed the formation of 1 μmol/mL·min of trans-cinnamic acid (Rosler et al., 1997). The Bradford method determined the protein concentration (Bradford, 1976). The specific activity was determined by dividing the volumetric activity by the protein concentration (mg/mL).
2.11. Determination of the effect of the inoculation of bacterial SynCom in maize plants
Once the phenotypic characterization tests of the proposed inoculants had been carried out, the effects of each SynCom on maize plants were evaluated.
2.12. Seed preparation for gnotobiotic, greenhouse, and open-field PGP assays
The maize seeds were rinsed superficially with distilled water and stirred with deionized water for 2 h at room temperature. The seeds were disinfected by a thermal shock in hot water at 50°C for 10 min followed by 10°C for 5 min in cold water. Subsequently, the seeds were washed with 0.3% HgCl2 and rinsed with sterile distilled water ten times. Later, some seeds were placed on a plate with LB agar to verify their proper disinfection (Rios-Galicia et al., 2021).
2.13. Preparation of inoculum
For in planta gnotobiotic and greenhouse assays, each SynCom and isolated bacterial species used as controls were adjusted to OD600nm of 1. In total, 1 mL of this suspension was then added to each germinated seed.
2.14. PGP of maize in gnotobiotic tests
The seeds germinating for 3 days were placed in tubes containing 20 mL of a semi-solid Murashige & Skoog medium (MS) and a suspension of 1 mL of each SynCom; the bacteria were adjusted to OD600nm of 1. The plants inoculated with all the treatments in the mixture were kept at 28°C in darkness for five days and subsequently transferred to a lighting incubator at a room temperature of 24°C, with a photoperiodic lighting of 16 h of light and 8 h of darkness for 20 days (Rekha et al., 2007). The plants were removed from the agar, and the fresh weights and length of the roots and shoots were determined.
The treatments were as follows: seeds inoculated with SynCom 1, SynCom 2, SynCom 3, P. protegens E1BL2 (positive control), and sterile water (negative control).
2.15. PGP of maize in greenhouse tests
Groups of six seeds were inoculated with 1 mL per seed of each treatment, germinated for 3 days, and incubated for 24 h. The seeds were sown in plastic pots with sterile vermiculite and irrigated every third day with a Hoagland nutrient solution to avoid overhydration, dehydration, pests, or pH alterations (Rios-Galicia et al., 2021). Periodic observations of plant conditions were performed over 60 days.
The treatments are as follows: seeds inoculated with SynCom 1, SynCom 2, SynCom 3, P. protegens E1BL2 (positive control), and sterile water (negative control). The shoot and root lengths and dry weights were determined at the end of the 60 days. The dry weights were determined by oven drying at 55°C until a constant weight was reached.
2.16. PGP of maize in open field tests
The field test evaluated the effect of the inoculation of SynCom on the maize crop yield and rust incidence. The experiment was performed in the experimental fields of the Innovation and Technological Development Center Mezquital Valley, located in the Cinta Larga municipality of Mixquiahula Hidalgo, Mexico (20.192457,−99.243521). The sowing was performed during the third week of May 2019. Commercial hybrid seeds CPL9105W from CROPLAN Genetics® were used for the test.
The seeds were washed with tap water to remove the insecticides and fungicides until the wash water had no color. They were then dried with absorbent paper, placed in a plastic bag, and inoculated using a suspension of SynCom and P. protegens E1BL2 used as a PGP positive control at a concentration of 1 × 108 CFU/mL. The bacteria were obtained from a culture grown for 48 h in an LB broth. Carboxymethylcellulose was used as an adherent with 30 g/kg of seeds. The negative controls with distilled water and the LB media were incorporated into the experiment.
The evaluation was completely randomized over five repetitions in experimental plots 5 m long with four furrows and 80 cm of separation between each one. Three seeds were sown and separated by a distance of 17.5 cm. Irrigation was applied monthly. The herbicides Marvel® (3,6-dichloro-2-methoxy benzoic acid and 6-chloro-N-ethyl-N-isopropyl-1,3,5-triazine-2,4-diamine) were applied at a dose of 2 L/ha after the first irrigation. At 30 days, the smaller plants were removed to obtain one plant per hole and a uniform population of 112 plants per row, equivalent to a population density of 72,500 plants/ha. Three applications of the SynCom and control were made monthly per treatment, and 2.5 L of the formulated SynCom and control were sprayed 25 days after the sowing.
To determine the yield, the ears of the plants in the central rows were counted in each plot. A total of 22 ears of each treatment were taken randomly, weighed, and shelled. The kernels were also weighed. Seed moisture was determined using a brand grain moisture tester (John Deere, IL, USA). The shelled factor (SF) was calculated using the following formula:
With this data, the yield (Y) in Ton/ha was calculated using the following formula:
where:
W = Average weight (kg) of 22 ears
T = Total number of ears in central rows
HPG = Humidity percentage of kernels
86 = Standardized yield factor at 14% humidity
SF = Shelled factor
G = Furrow width (0.8 m).
The rust incidence percentage in each plot was also evaluated at 90 days after sowing using the Peterson scale (Peterson et al., 1948).
2.17. Statistical analysis
All phenotypic experiments were assayed in triplicate and repeated at least two times, for in planta gnotobiotic and greenhouse were assayed with six plants per treatment and repeated two times. An ANOVA was carried out for the phenotypic experiments. The means of each treatment were compared using Duncan's multiple range test at a 0.05 level. The data are shown as the means and their standard errors. The plant growth data were analyzed using a completely randomized design, and Tukey's Honest Significance Differences test compared the means. Differences were considered significant at a P-value of < 0.05. Statistical analyses were performed with GraphPad Prism version 9.00 (GraphPad Software, La Jolla California, USA).
3. Results
3.1. Compatibility assay and SynCom design
Antagonistic interactions were only detected for Pantoea sp. E2AD2 on Burkholderia sp. Z1AL11, P. protegens E2HL9, P. ananatis E2HD8, and K. pseudosacchari Z2WD1; and for P. ananatis E2HD8 on P. protegens E2HL9 and K. pseudosacchari Z2WD1. The SynCom design included bacterial strains with partially redundant and complementary PGP features, but antagonistic strains were not included (Figure 1). The final formulations were SynCom 1: A. xylosoxidans Z2K8, Burkholderia sp. Z1AL11, K. variicola R3J3HD7, K. pseudosacchari Z2WD1, P. diazotrophicus Z2WL1, P. protegens E1BL2, P. protegens E2HL9; SynCom 2: A. xylosoxidans Z2K8, K. variicola R3J3HD7, P. diazotrophicus Z2WL1, Pantoea sp. E2AD2, P. protegens E1BL2; SynCom 3: A. xylosoxidans Z2K8, Burkholderia sp. Z1AL11, K. variicola R3J3HD7, P. diazotrophicus Z2WL1, P. ananatis E2HD8, P. protegens E1BL2. Each SynCom contained a population of 1 × 108 CFU/mL; each bacterial species was in the same cellular population. These suspensions were used for the in vitro and in planta tests.
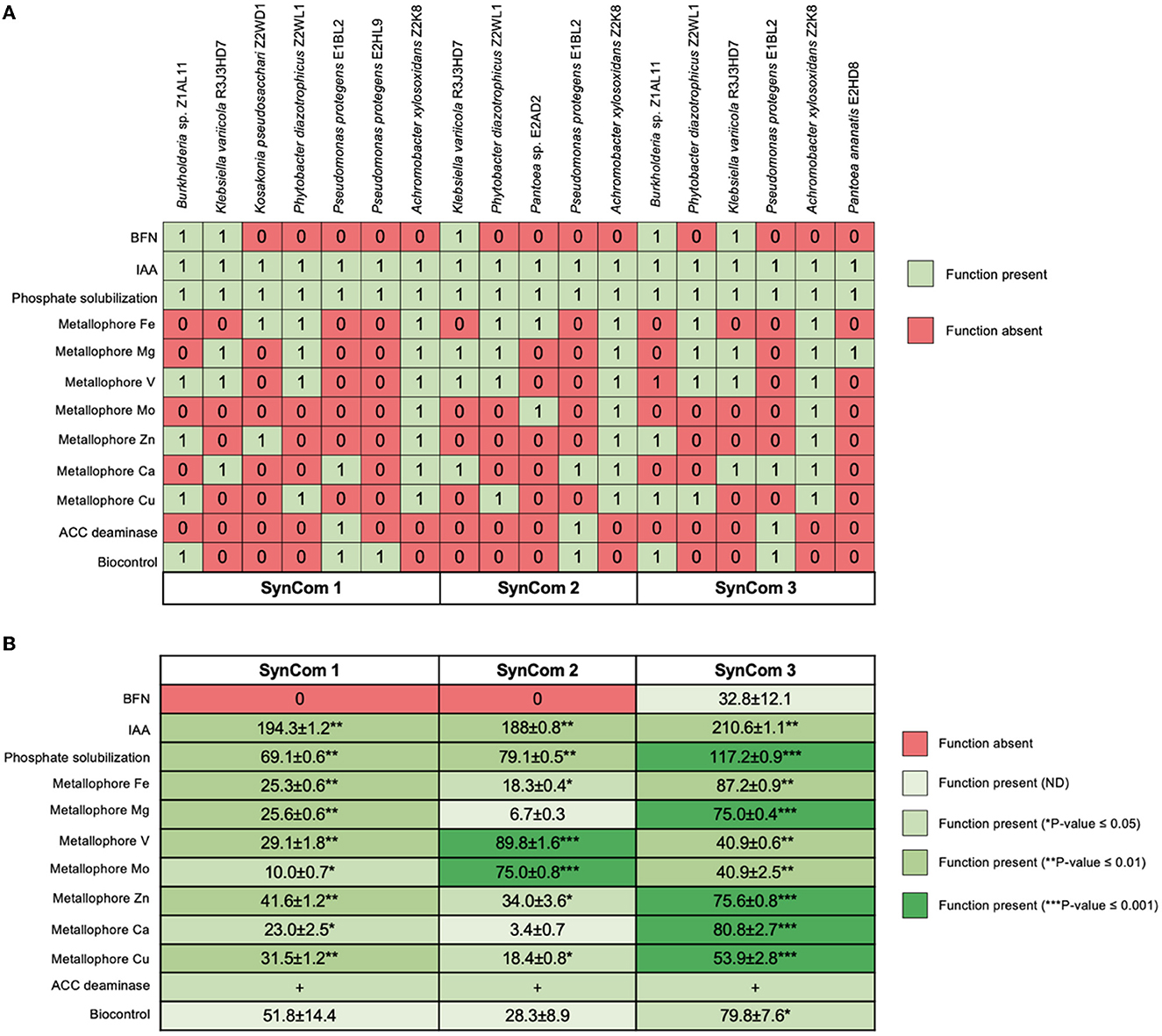
Figure 1. Functional traits of Jala maize endophytes individually and in the synthetic microbial communities (SynCom). The growth promotion and biocontrol (A) capabilities of each of the studied isolates are shown and compared with (B) the formulated SynCom. The colors represent the absence and presence of these activities as well as statistically significant differences according to Duncan's test (*P ≤ 0.05, **P ≤ 0.01, and ***P ≤ 0.001).
3.2. PGP traits of mixed bacterial inoculants
The in vitro phenotypic PGP tests of the axenic bacteria and SynCom are shown in Table 2. The semiquantitative phosphate solubilization test revealed no differences between the SynCom and control strains, but the quantitative assay differences were evident. SynCom 3 accumulated more soluble orthophosphate (117 μg/mL) in 96 h than the other axenic bacteria, including Pantoea sp. E2AD2 (29 μg/mL), SynCom 1 (69 μg/mL), and SynCom2 (79 μg/mL).
All axenic bacteria produced between 3.1 and 51.7 μg/mL of IAA in a culture medium supplemented with 51.7 μg/mL tryptophan. P. ananatis E2HD8 was the strain that produced the highest amount of IAA. All synthetic communities produced from 188 to 210.6 μg/mL of IAA, but SynCom 3 was the most efficient.
Aminocyclopropane-1-carboxylate (ACC) deaminase production was only detected in P. protegens E1BL2; the other P. protegens E2HL9 did not express this enzymatic activity. All SynCom contained the P. protegens E2HL9 strain and expressed the activity. Different profiles of metallophores for different ions (Co2+, Ca2+, Mg2+, Zn2+, Fe3+, Mo2+, and V5+) were observed in both the bacteria and SynCom. Nitrogen fixation was only detected in Burkholderia sp. Z1AL11 and K. variicola R3J3HD7. Although all SynCom had at least one diazotrophic bacterium, only SynCom 3 exhibited evident growth in the BMGM media and reduced acetylene allowing 32.8 ± 12.1 nmol of ethylene h−1.
3.3. Growth inhibition of fungal phytopathogens
The inhibition of fungal growth by PGPB and SynCom is shown in Figure 2. P. protegens E1BL2 and SynCom 3 exhibited the highest inhibition percentages reaching 80% of inhibition. Pestalotia sp. and Helminthosporium sp. were hardly inhibited by P. protegens E1BL2 and SynCom 3, respectively.
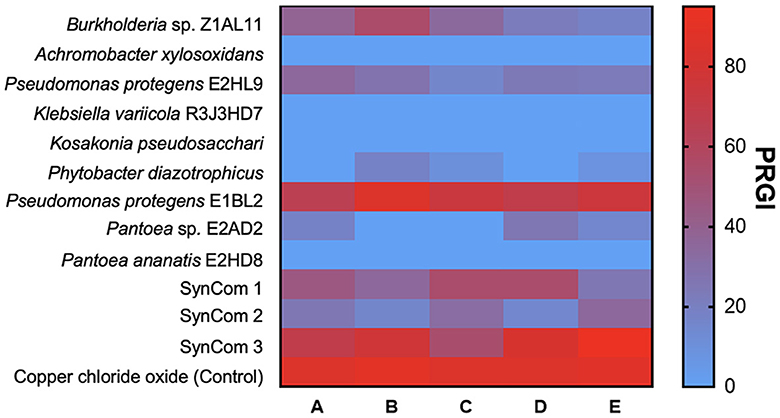
Figure 2. Heat map of the fungal radial growth inhibition by endophytic bacteria. (A) Fusarium oxysporum, (B) Pestalotia sp., (C) Curvularia sp., (D) Colletotrichum sp., and (E) Helminthosporium sp.
3.4. PAL activity determination for ISR
The treatments of the plants with P. protegens E1BL2 and SynCom 3 increased the PAL enzymatic activity of the corn root tissues. However, as shown in Figure 3, only the effect of SynCom 3 was statistically significant as it tripled the activity of the enzyme of the untreated control.
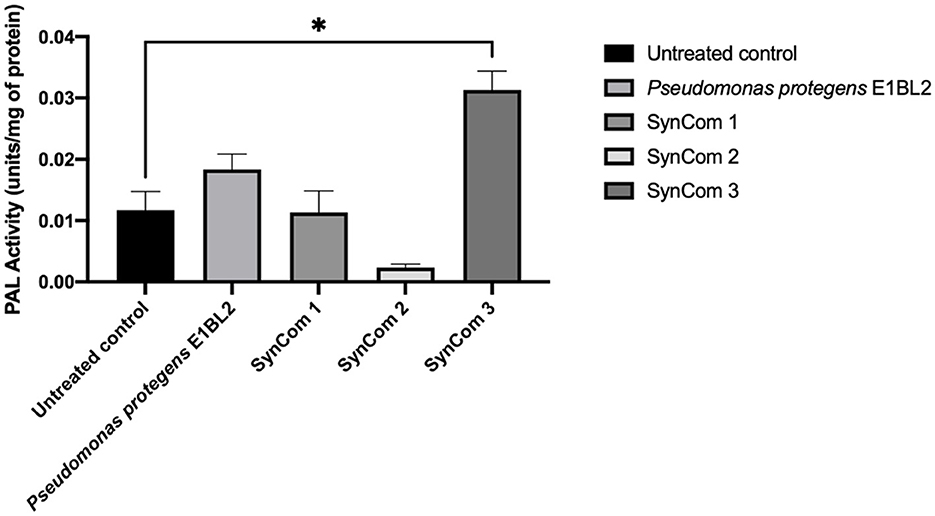
Figure 3. Enzymatic activity of maize roots' phenylalanine ammonium lyase (PAL) treated with inoculants; the P. protegens E1BL2 strain was used as a control. The PAL activity of inoculated and uninoculated plants was compared using Tukey's test (*p ≤ 0.05).
3.5. In planta assay in gnotobiotic and greenhouse systems
The effect of SynCom on plant growth in the gnotobiotic system is shown in Figure 4A. The promotion of seedling growth was highlighted by SynCom 3, which promoted the growth of the roots and shoots to sizes 4.2 and 3.0 times greater, respectively, than those of the untreated control. The effect of SynCom on plant growth at the greenhouse level is shown in Figure 4B, with increases of 3.3 and 1.4 times the roots and shoot length and 2.3 and 3 times the dry weights of the shoots and roots, respectively. Examples of in vitro PGP traits and in planta assays are illustrated in Figure 5.
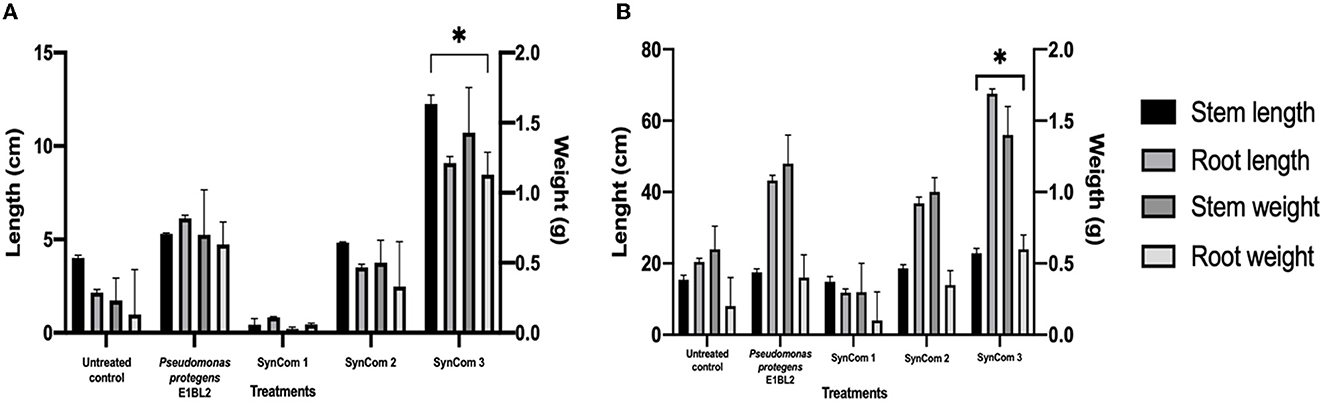
Figure 4. Effect of the inoculation of SynCom on the shoot, root length, and weight of the Conejo landrace. (A) Application of SynCom bioinoculants on the gnotobiotic system and (B) greenhouse system. Tukey's test compared the lengths and weights of inoculated and uninoculated plants (*p ≤ 0.05).
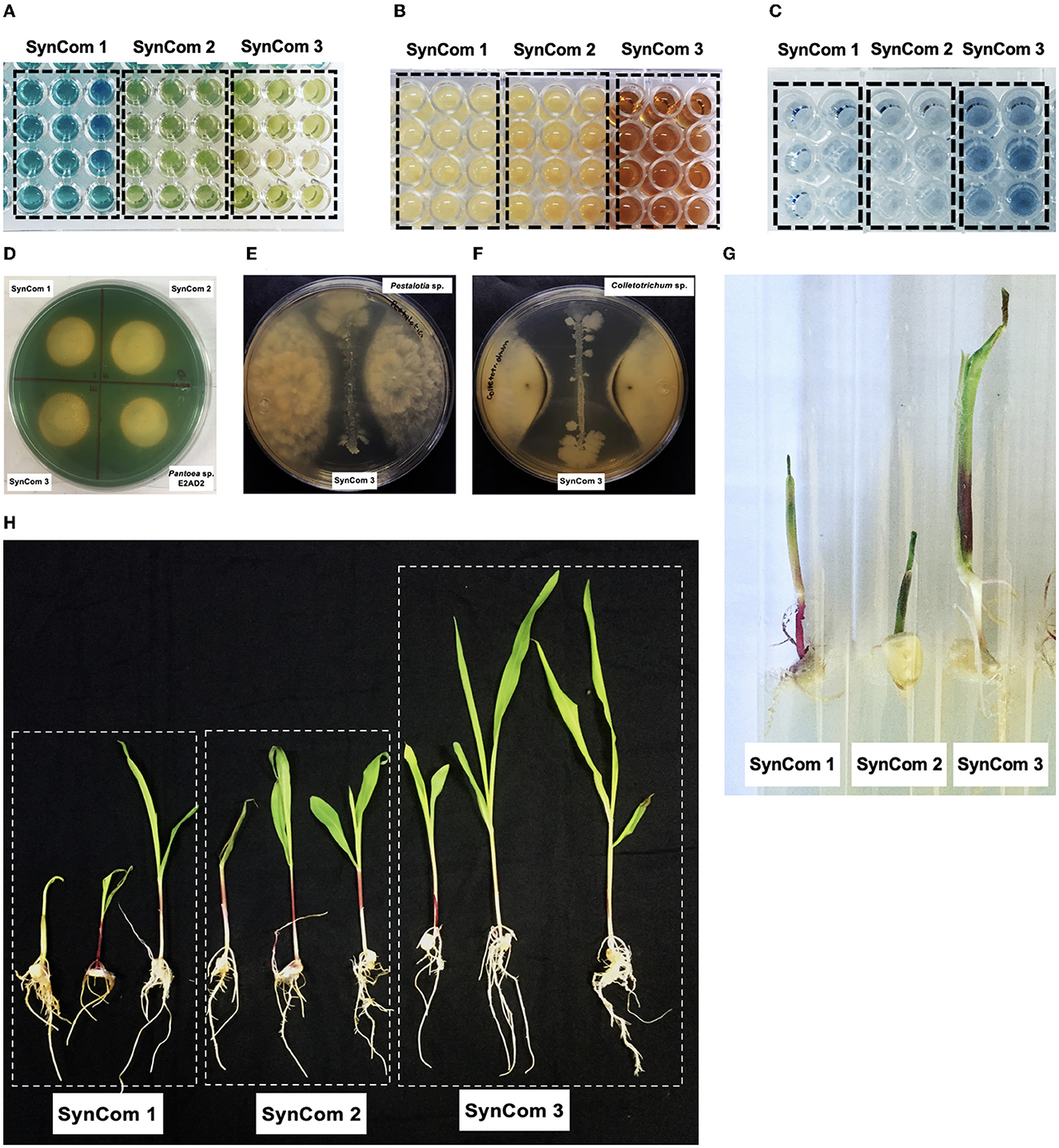
Figure 5. Phenotypic traits of Jala maize SynCom. (A) Quantitative metallophore test in a liquid medium, blue colors represent low production, and green and yellow colors represent high production. (B) In quantitative IAA determination, yellow colors represent low production, and orange and red colors represent high production. (C) In quantitative phosphate solubilization, the intensity of the blue color is proportional to the production of orthophosphate. (D) Metallophore production test in plates, the size of the halos was determined to estimate the production of each particular metallophore. (E) Inhibition of mycelial growth of Pestalotia sp. by SynCom 3. (F) Inhibition of mycelial growth of Colletotrichum sp. by SynCom 3. (G) Plant growth promotion assay in a gnotobiotic system by SynCom. (H) Plant growth promotion in a greenhouse system by SynCom.
3.6. Field test
As shown in Figure 6, the productivity yield of the control treatment was 11 Tons/ha, but P. protegens E1BL2 and the SynCom 3 treatments resulted in yields of 14 and 16 Tons/ha, respectively. Moreover, the incidence of rust infection showed a significant decrease of 12.5% with the SynCom 3 treatment. SynCom 1 and 2 did not show significant increases in productivity or decreases in rust infection concerning the controls.
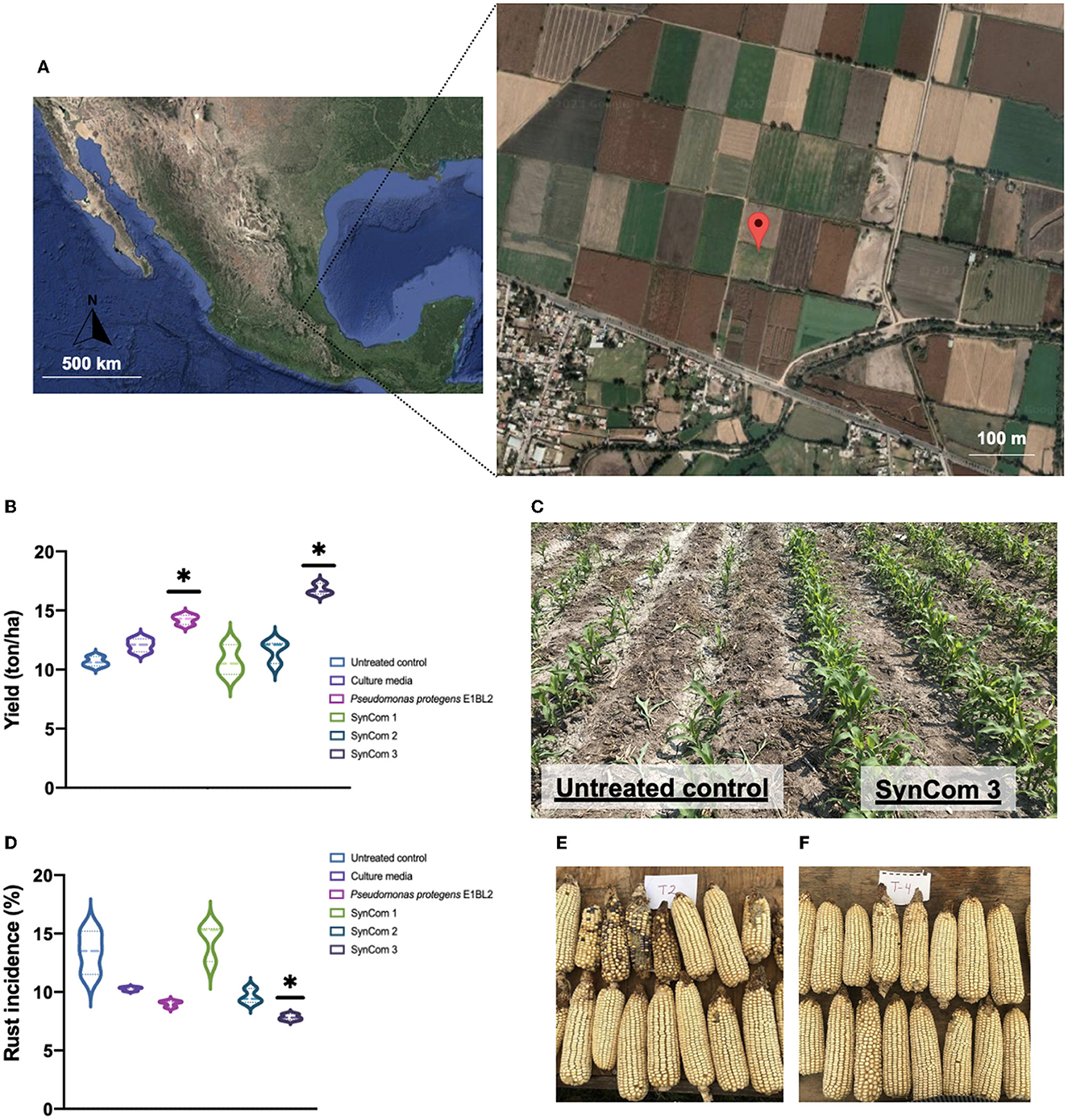
Figure 6. Field test of bacterial SynCom application in maize crop using the commercial hybrid CPL9105W. (A) Geographic localization of the open field in Mixquiahula Hidalgo, Mexico (20.192457,−99.243521). (B) Bacterial SynCom effect in the crop yield (ton/ha). (C) Bacterial SynCom effect in the rust incidence percentage (%). (D) A comparison of untreated control plants and SynCom 3 treatment. (E) Ears or maize without treatment and (F) ears treated with SynCom 3. The test was conducted in the experimental fields from the Innovation and Technological Development Center Mezquital Valley, Mixquiahula Hidalgo, México. Tukey's test compared the yield and rust incidence of inoculated and uninoculated plants (*p ≤ 0.05).
4. Discussion
The Tehuacán and Balsas Valleys in Mexico are the biological origin of maize and its domestication, artificial selection, and initial diversification that currently include at least 59 landraces of native maize. The PGP and fungal antagonistic bacteria used in this study were isolated from the endosphere of native maize of the Jala landrace, which is grown in the states of Jalisco and Nayarit in Mexico (Rios-Galicia et al., 2021). However, the PGP test was performed on the Conejo maize landrace because it is a tropical strain prone to fast growth, reaches physiological maturity around 90 days, and is adapted to humid coastal climates and dry tropics (Wilkes, 1977). CPL9105W hybrid maize seeds were used for the field trials due to their better adaptation to the field climate and early maturation time.
All SynCom displayed better in vitro PGP capabilities than the strains used in individual trials. This synergistic effect mainly observed in maize, rice, and wheat assays confirms the advantages of the design of the three SynCom (Olanrewaju and Babalola, 2019; Ngalimat et al., 2021; Cherif et al., 2022). However, any comparison of the quantitative results obtained in this study with previous data must be made with caution and considering the context because many methods and conditions for PGP and antifungal assays have been reported in the literature.
Phosphorus is an essential component of molecules such as DNA and ATP and it is vital for photosynthesis, energy transfer, and cell reproduction. In addition, phosphorus is necessary for the growth and production of strong and resistant roots, which is important for disease resistance and soil nutrient intake; thus, applying soluble phosphate microorganisms is a cost-effective alternative for producing this mineral (Rawat et al., 2021; Elhaissoufi et al., 2022). Although the strains in this study did not reach the levels of phosphate solubilization of Serratia sp. S119 (70 μg/mL) (Ludueña et al., 2018), all the SynCom generated similar or higher yields.
Indoleacetic acid (IAA) is an auxin phytohormone that has complex effects on the growth of different plant organs depending on its concentration, life cycle stage, the affected tissue, and an endogenous or exogenous origin (Duca et al., 2014; Kunkel, 2021). The constant exogenous supply of this hormone in quantities not exceeding 10 nM (growth-inhibiting concentration in many plant species) ensures the development of the roots (Eliasson et al., 1989). The axenic bacterial strains produced IAA similar to those of Azospirillum brasiliense strains (21 to 102 μg/mL) (Meza et al., 2015), but all SynCom synthesized significantly higher IAA final concentrations. The explanation for this phenomenon is beyond the scope of this study. Still, it could partly explain the shoot and root growth promotion documented in the trials under gnotobiotic, greenhouse, and open field conditions.
Metallophores, particularly siderophores, are secondary metabolites with metal ion chelating activity secreted by bacteria. In addition, to supply Fe and other essential metals to plants and promote their growth, metallophores provide additional benefits such as limiting the development of some phytopathogen fungi and bacteria and binding and detoxifying toxic heavy metals in heavy metal-contaminated soils (Timofeeva et al., 2022). The production capacity of metallophores is widely distributed among bacteria, and the species used in this study are no exception (McRose et al., 2018). The tests could not discern if the strains produced a single metallophore with several chelating capabilities or if several metallophores with specific affinities were excreted. Still, it seems to be a frequent phenomenon in bacteria (McRose et al., 2018; Reitz, 2022). The SynCom included bacteria species with a vast display of siderophores.
The aminocyclopropane-1-carboxylate (ACC) deamination ability of bacteria provides plants with an additional nitrogen source of ammonium, which plants can also use. Both IAA and ACC can be synthesized by plants and bacteria cells. Under stress conditions, IAA and ACC increase and induce a stress response via ethylene, a compound that can inhibit plant growth. The excess ACC is exported to associated bacteria, and ACC deaminase metabolizes to ammonia and a-ketobutirate. A bioinoculant with both characteristics would help regulate the plant synthesis of ethylene under stress conditions (Glick, 2014). SynCom 3 contained bacteria with multiple phenotypic traits probably responsible for increased plant growth observed in the assays. In part, this growth resulted from ethylene levels that have remained low during the plant's life cycle since endogenous IAA synthesis was inactivated thanks to an external supply (Etesami et al., 2014). In addition, the production of the ACC synthase and oxidase enzymes in response to stress is regulated by the intervention of the bacterial deaminase ACC (Glick, 2005; Van de Poel and Van Der Straeten, 2014). Thus, while the entry of exogenous IAA promotes growth and contributes to diminishing its endogenous synthesis, the enzyme ACC-deaminase reduces the impact of the wave of endogenous ethylene synthesis that occurs in response to stress by acting as an exhaust valve that prevents ethylene from reaching harmful levels (Singh et al., 2015; Nascimento et al., 2018; Mou et al., 2020).
All formulated SynCom contained the strain of K. variicola R3J3HD7, but only SynCom 3 reduced acetylene and possibly fixed nitrogen in vitro. The free-living nitrogen-fixing ability of K. variicola has previously been reported (Haahtela et al., 1983; Qin et al., 2014), and its importance in open-field experiments has been recognized (Guerrieri et al., 2021; Kusale et al., 2021). The abundant polysaccharides of K. variicola probably generated a physicochemical barrier to molecular oxygen to protect oxygen-sensitive nitrogenase (Fourmond and Léger, 2017; Kubas et al., 2017). In addition, the Burkholderia sp. Z1AL11 diazotrophic strain was included in the SynCom 3 design. The nitrogen fixation capability is distributed among some species of the Burkholderia cepacia complex (Sandanakirouchenane et al., 2017; Li et al., 2022). The specific contribution to the final nitrogen fixation of each species in the SynCom is unknown, but this PGP feature is essential in the formulation.
SynCom 3 contained Burkholderia sp. Z1AL11 and P. protegens E2HL9 and exhibited an apparent antagonistic effect on several phytopathogenic fungi in vitro, in addition to decreasing the incidence of rust in the open-field experiment. This antifungal effect may have been due to nutrient competition and the production of siderophores and soluble or volatile antifungals (Mannaa and Kim, 2018; Pellicciaro et al., 2021). P. protegens produces biocontrol compounds such as 2,4-diacetyl phloroglucinol, pyoluteorin, pyrrolnitrin, phenazines, and hydrogen cyanide (Haas and Keel, 2003; Haas and Défago, 2005; Raaijmakers et al., 2009; Ramette et al., 2011; Zhang et al., 2020). The antifungal ability is also widely distributed among the Burkholderia genus (Depoorter et al., 2016; Elshafie and Camele, 2021).
Plants colonized with endophytic bacteria can induce an efficient and rapid systemic resistance to phytopathogens. In the absence of challenge trials with phytopathogen fungi, the PAL activity of SynCom was used as an indicator of this adaptive response. Numerous papers have described this response; for example, Citrullus lanatus and Cucumis sativus exposed to a non-pathogenic strain of Colletotrichum magna showed high levels of lignin deposition, peroxidase activity, and PAL activity and protection against diseases caused by Colletotrichum orbiculare and Fusarium oxysporum (Fu-kang et al., 2010; Hassan et al., 2014; Elsharkawy et al., 2015). More detailed study is needed, but SynCom 3 decreased the frequency of fungal rust in the open-field experiment.
The set of experiments proposed in this study did not allow us to determine the contribution of each of the bacteria and their phenotypic traits in the observed PGP at the gnotobiotic, greenhouse, or open field levels. However, the rational design of SynCom suggests that it is an efficient strategy that can be evaluated experimentally at various levels. The “Theory of Multiple Mechanisms” could explain the effects on PGP,” where the impact of individual mechanisms, operating simultaneously or consecutively, will result in a more significant effect on the plant (Mendoza and Cruz, 2012). The SynCom are new alternatives for an ecological and environmentally friendly approach as they arise from and are applied to the corn environment, making use of the same natural resources in an optimized manner and considering the plant-microorganisms' holobiont relationship (Chiu and Gilbert, 2015; Sánchez-Cañizares et al., 2017; Cesaro et al., 2021). The increase in grain productivity and reduction of fungal infections, even when modest, offer alternatives to the indiscriminate use of chemical fertilizers and recalcitrant pesticides and should thus certainly be investigated further (Xue et al., 2016; Jat et al., 2021; Kiani et al., 2021).
5. Conclusion
The inoculation of a SynCom of growth-promoting bacteria composed of the strains Burkholderia. sp. Z1AL11, A. xylosoxidans Z2K8, K. variicola R3J3HD7, P. diazotrophicus Z2WL1, P. protegens E1BL2, and P. ananatis E2HD8 promoted the growth of Conejo landrace maize plants in gnotobiotic and greenhouse trials. Treatments of maize using this synthetic community also increased productivity and decreased the rust incidence in hybrid CPL9105W crops.
The studied SynCom also inhibited the growth of some phytopathogenic fungi, making it a potential biofertilizer and bio fungicide that could be applied in the field.
Bacterial SynCom promotes the defense activity of plants by providing them with an ISR is one of the strategies that should be used as an alternative to recalcitrant pesticides and environmentally harmful contaminants.
Data availability statement
The raw data supporting the conclusions of this article will be made available by the authors, without undue reservation.
Author contributions
ED, JS-A, and BR-G performed the bacterial isolation and characterization and conducted the greenhouse experiments. YM-F provided the seed samples and coordinated the field test. RA-G, LV-T, and CH-R provided the samples and designed and coordinated the study. ED wrote the first draft of the manuscript. ED, JS-A, BR-G, YM-F, RA-G, LV-T, and CH-R contributed to the editing of the manuscript. All authors read and approved the final manuscript.
Funding
This study was supported by the Secretaría de Investigación y Posgrado-IPN (SIP-20211032, 20220795, and 20231480) and Instituto Nacional de Investigaciones Forestales, Agrícolas y Pecuarias (13395732205 Estudio de la diversidad microbiana asociada a suelos rizosférico de 10 variedades de maiz).
Acknowledgments
Proof-Reading-Service.com Ltd. reviewed the manuscript. All authors thank Dr. Paulina Estrada-De Los Santos and Dr. Erika Yanet Tapia-García for their support in developing BFN assays. ED, JS-A, and BR-G are grateful to the Consejo Nacional de Ciencia y Tecnología (CONACYT) and PIFI/BEIFI-IPN for granting them scholarships. LV-T and CH-R are fellows of EDI-IPN, COFAA-IPN, and SNI-CONACYT.
Conflict of interest
The authors declare that the research was conducted in the absence of any commercial or financial relationships that could be construed as a potential conflict of interest.
Publisher's note
All claims expressed in this article are solely those of the authors and do not necessarily represent those of their affiliated organizations, or those of the publisher, the editors and the reviewers. Any product that may be evaluated in this article, or claim that may be made by its manufacturer, is not guaranteed or endorsed by the publisher.
References
Abdullaeva, Y., Manirajan, B. A., Honermeier, B., Schnella, S., and Cardinale, M. (2021). Domestication affects the composition, diversity, and co-occurrence of the cereal seed microbiota. J. Adv. Res. 31, 75–86. doi: 10.1016/j.jare.2020.12.008
Al-Tammar, F. K., and Khalifa, A. Y. Z. (2022). Plant growth promoting bacteria drive food security. Braz. J. Biol. 82, e267257. doi: 10.1590/1519-6984.267257
Armanhi, J. S. L., Souza, D., Damasceno, R. S. C., de Araújo, N., and Imperial, L. M. J. (2018). A community-based culture collection for targeting novel plant growth-promoting bacteria from the sugarcane microbiome. Front. Plant Sci. 8, 2191. doi: 10.3389/fpls.2017.02191
Babu, S., Prasanna, R., Bidyarani, N., Nain, L., and Shivay, Y. S. (2015). Synergistic action of PGP agents and Rhizobium spp. for improved plant growth, nutrient mobilization and yields in different leguminous crops. ISBAB 4, 456–464. doi: 10.1016/j.bcab.2015.09.004
Bashan, Y. (1998). Inoculants of plant growth-promoting bacteria for use in agriculture. Biotechnol. Adv. 16, 729–770. doi: 10.1016/S0734-9750(98)00003-2
Bashan, Y., Kamnev, A. A., and de-Bashan, L. E. (2013). A proposal for isolating and testing phosphate-solubilizing bacteria that enhance plant growth. Biol. Fertil. Soils 49, 1–2. doi: 10.1007/s00374-012-0756-4
Berg, G., Köberl, M., Rybakova, D., Müller, H., Grosch, R., Smalla, K., et al. (2017). Plant microbial diversity is suggested as the key to future biocontrol and health trends. FEMS Microbiol. Ecol. 93, 1–6. doi: 10.1093/femsec/fix050
Berg, G., Kusstatscher, P., Abdelfattah, A., Cernava, T., and Smalla, K. (2021). Microbiome modulation—toward a better understanding of plant microbiome response to microbial inoculants. Front. Microbiol. 12, 650610. doi: 10.3389/fmicb.2021.650610
Bodhankar, S., Grover, M., Hemanth, S., Reddy, G., Rasul, S., Yadav, S. K., et al. (2017). Maize seed endophytic bacteria: dominance of antagonistic, lytic enzyme-producing Bacillus spp. Biotech. 7, 1–13. doi: 10.1007/s13205-017-0860-0
Bradford, M. M. (1976). A rapid and sensitive method for the quantitation of microgram quantities of protein utilizing the principle of protein-dye binding. Anal. Biochem. 72, 248. doi: 10.1016/0003-2697(76)90527-3
Cavali, J., Pereira, O. G., Valadares Filho, S. D. C., Porto, M. O., Fernandes, F. E. P., Garcia, R., et al. (2010). Mixed sugarcane and elephant grass silages with or without bacterial inoculant. Rev. Bras. Zootec. 39, 462–470. doi: 10.1590/S1516-35982010000300003
Cesaro, P., Gamalero, E., Zhang, J., and Pivato, B. (2021). the plant holobiont volume i: microbiota as part of the holobiont; challenges for agriculture. Front. Plant Sci. 12, 1–3. doi: 10.3389/fpls.2021.799168
Chavéz-Díaz, I. F., Cruz-Cárdenas, C. I., Sandoval-Cancino, G., Calvillo-Aguilar, F. F., Ruíz-Ramírez, S., Blanco-Camarillo, M., et al. (2022). Seedling growth promotion and potential biocontrol against phytopathogenic Fusarium by native rhizospheric Pseudomonas spp. strains from Amarillo Zamorano maize landrace. Rhizosphere 24, 100601. doi: 10.1016/j.rhisph.2022.100601
Cherif, H., Sghaier, I., and Hassen, W. (2022). Halomonas desertis G11, Pseudomonas rhizophila S211 and Oceanobacillus iheyensis E9 as biological control agents against wheat fungal pathogens: PGPB consorcia optimization through mixture design and response surface analysis. Int. Clin. Pathol. J. 9, 20–28. doi: 10.15406/icpjl.2022.09.00204
Chiu, L., and Gilbert, S. F. (2015). The birth of the holobiont: multi-species birthing through mutual scaffolding and niche construction. Biosemiotics 82, 191–210. doi: 10.1007/s12304-015-9232-5
Compant, S., Duffy, B., Nowak, J., Clément, C., and Barka, E. A. (2005). Use of plant growth-promoting bacteria for biocontrol of plant diseases: principles, mechanisms of action, and future prospects. Appl. Environ. Microbiol. 719, 4951–4959. doi: 10.1128/AEM.71.9.4951-4959.2005
Depoorter, E., Bull, M. J., Peeters, C., Coenye, T., Vandamme, P., Mahenthiralingam, E., et al. (2016). Burkholderia: an update on taxonomy and biotechnological potential as antibiotic producers. Appl. Microbiol. Biotecnol. 100, 5215–5229. doi: 10.1007/s00253-016-7520-x
Duca, D., Lorv, J., Patten, C. L., Rose, D., and Glick, B. R. (2014). Indole-3-acetic acid in plant–microbe interactions. Antonie Leeuwenhoek. 106, 85–125. doi: 10.1007/s10482-013-0095-y
Elhaissoufi, W., Ghoulam, C., Barakat, A., Zeroual, Y., and Bargaz, A. (2022). Phosphate bacterial solubilization: A key rhizosphere driving force enabling higher P use efficiency and crop productivity. J. Adv. Res. 38, 13–28. doi: 10.1016/j.jare.2021.08.014
Eliasson, L., Bertell, G., and Bolander, E. (1989). Inhibitory action of auxin on root elongation not mediated by ethylene. Plant Physiol. 91, 310–314. doi: 10.1104/pp.91.1.310
El-Sayed, I. A., and Edrees, N. O. (2014). Using of plant growth promoting rhizobacteria as biocontrol agent for root-knot nematode under greenhouse. Nat. Sci. 12, 41–49. doi: 10.13140/RG.2.1.2610.9603
Elshafie, H. S., and Camele, I. (2021). An overview of metabolic activity, beneficial and pathogenic aspects of Burkholderia Spp. Metabolites 11, 321. doi: 10.3390/metabo11050321
Elsharkawy, M. M., Shivanna, M. B., Meera, M. S., and Hyakumachi, M. (2015). Mechanism of induced systemic resistance against anthracnose disease in cucumber by plant growth-promoting fungi. Acta Agric. Scand. -B Soil Plant Sci. 65, 287–299. doi: 10.1080/09064710.2014.1003248
Emami, S., Alikhani, H. A., Pourbabaei, A. A., Etesami, H., Sarmadian, F., Motessharezadeh, B., et al. (2019). Effect of rhizospheric and endophytic bacteria with multiple plant growth promoting traits on wheat growth. Environ. Sci. Pollut. Res. 26, 19804–19813. doi: 10.1007/s11356-019-05284-x
Etesami, H., Hosseini, H. M., Alikhani, H. A., and Mohammadi, L. (2014). Bacterial biosynthesis of 1-aminocyclopropane-1-carboxylate (ACC) deaminase and indole-3-acetic acid (IAA) as endophytic preferential selection traits by rice plant seedlings. J. Plant Growth Regul. 33, 654–670. doi: 10.1007/s00344-014-9415-3
Fourmond, V., and Léger, C. (2017). Dinitrogen reduction: Interfacing the enzyme nitrogenase with electrodes. Angew. Chem. Int. Ed. 56, 4388–4390. doi: 10.1002/anie.201701179
Fox, J. E., Gulledge, J., Engelhaupt, E., Burow, M. E., and McLachlan, J. A. (2007). Pesticides reduce symbiotic efficiency of nitrogen-fixing rhizobia and host plants. Pro. Natl. Acad. Sci. U.S.A. 104, 10282–10287. doi: 10.1073/pnas.0611710104
Fu-kang, G., Chuan-chao, D., and Xiao-zhen, L. (2010). Mechanisms of fungal endophytes in plant protection against pathogens. Afr. J. Microbiol. Res. 4, 1346–1351.
Glick, B. R. (2005). Modulation of plant ethylene levels by the bacterial enzyme ACC deaminase. FEMS Microbiol. Lett. 251, 1–7. doi: 10.1016/j.femsle.2005.07.030
Glick, B. R. (2014). Bacteria with ACC deaminase can promote plant growth and help to feed the world. Microbiol. Res. 169, 30–39. doi: 10.1016/j.micres.2013.09.009
Gordon, S. A., and Weber, R. P. (1951). Colorimetric estimation of indoleacetic acid. Plant Physiol. 26, 192–195. doi: 10.1104/pp.26.1.192
Guerrieri, M. C., Fiorini, A., Fanfoni, E., Tabaglio, V., Cocconcelli, P. S., Trevisan, M., et al. (2021). Integrated genomic and greenhouse assessment of a novel plant growth-promoting rhizobacterium for tomato plant. Front. Plant Sci. 12, 660620. doi: 10.3389/fpls.2021.660620
Gutierrez, A., and Grillo, M. A. (2022). Effects of Domestication on plant-microbiome interactions. Plant Cell Physiol. 63, 1654–1666. doi: 10.1093/pcp/pcac108
Haahtela, K., Kari, K., and Sundman, V. (1983). Nitrogenase activity (acetylene reduction) of root-associated, cold-climate Azospirillum, Enterobacter, Klebsiella, and Pseudomonas species during growth on various carbon sources and at various partial pressures of oxygen. Appl. Environ. Microbiol. 45, 563–570. doi: 10.1128/aem.45.2.563-570.1983
Haas, D., and Défago, G. (2005). Biological control of soil-borne pathogens by fluorescent pseudomonads. Nat. Rev. Microbiol. 3, 307–319. doi: 10.1038/nrmicro1129
Haas, D., and Keel, C. (2003). Regulation of antibiotic production in root-colonizing Pseudomonas spp. and relevance for biological control of plant disease. Annu. Rev. Phytopathol. 41, 117–153. doi: 10.1146/annurev.phyto.41.052002.095656
Hardy, R. W., Holsten, R. D., Jackson, E. K., and Burns, R. C. (1968). The acetylene-ethylene assay for N2 fixation: laboratory and field evaluation. Plant Physiol. 43, 1185–1207. doi: 10.1104/pp.43.8.1185
Hassan, N., Elsharkawy, M. M., Shivanna, M. B., Meera, M. S., and Hyakumachi, M. (2014). Elevated expression of hydrolases, oxidase, and lyase in susceptible and resistant cucumber cultivars systemically induced with plant growth-promoting fungi against anthracnose. Acta Agric. Scand. -B Soil Plant Sci. 64, 155–164. doi: 10.1080/09064710.2014.898783
Hawkes, C. V., Bull, J. J., and Lau, J. A. (2020). Symbiosis and stress: how plant microbiomes affect host evolution. Philos. Trans. R. Soc. B 375, 20190590. doi: 10.1098/rstb.2019.0590
He, Z., and Honeycutt, C. W. (2005). A modified molybdenum blue method for orthophosphate determination suitable for investigating enzymatic hydrolysis of organic phosphates. Commun. Soil Sci. Plant Anal. 36, 1373–1383. doi: 10.1081/CSS-200056954
Hellin, J., Bellon, M. R., and Hearne, S. J. (2014). Maize landraces and adaptation to climate change in Mexico. J. Crop Improv. 28, 484–501. doi: 10.1080/15427528.2014.921800
Hett, J., Neuhoff, D., Döring, T. F., Masoero, G., Ercole, E., Bevivino, A., et al. (2022). Effects of multi-species microbial inoculants on early wheat growth and litterbag microbial activity. Agronomy 12, 899. doi: 10.3390/agronomy12040899
Hussain, S., Siddique, T., Saleem, M., Arshad, M., and Khalid, A. (2009). Impact of pesticides on soil microbial diversity, enzymes, and biochemical reactions. Adv. Agron. 102, 159–200. doi: 10.1016/S0065-2113(09)01005-0
International Grains Council (2022). Available online at: https://www.igc.int/es/ (accessed January 23, 2023)
Jat, S. L., Suby, S. B., Parihar, C. M., Gambhir, G., Kumar, N., Rakshit, S., et al. (2021). Microbiome for sustainable agriculture: a review with special reference to the corn production system. Arch. Microbiol. 203, 2771–2793. doi: 10.1007/s00203-021-02320-8
Jikare, A. M., and Chavan, M. D. (2013). Siderophore produced by Bacillus shackletonii. Gn-09 and showed its plant growth promoting activity. Int. J. Pharm. Biol. Sci. 3, 198–202.
Johnsen, K., Jacobsen, C. S., Torsvik, V., and Sørensen, J. (2001). Pesticide effects on bacterial diversity in agricultural soils–a review. Biol. Fertil. Soils. 33, 443–453. doi: 10.1007/s003740100351
Johnston-Monje, D., and Raizada, M. N. (2011). Conservation and diversity of seed-associated endophytes in Zea across boundaries of evolution, ethnography and ecology. PLoS ONE 6, e20396. doi: 10.1371/journal.pone.0020396
Kaur, S., Egidi, E., Qiu, Z., Macdonald, C. A., Verma, J. P., Trivedi, P., et al. (2022). Synthetic community improves crop performance and alters rhizosphere microbial communities. J. Sustain. Agric. Environ. 1, 118–131. doi: 10.1002/sae2.12017
Kiani, T., Mehboob, F., Hyder, M. Z., Zainy, Z., Xu, L., Huang, L., et al. (2021). Control of stripe rust of wheat using indigenous endophytic bacteria at seedling and adult plant stage. Sci. Rep. 11, 1–14. doi: 10.1038/s41598-021-93939-6
Kim, H., Lee, K. K., Jeon, J., Harris, W. A., and Lee, Y. H. (2020). Domestication of Oryza species eco-evolutionarily shapes bacterial and fungal communities in rice seed. Microbiome 8, 20. doi: 10.1186/s40168-020-00805-0
Kubas, A., Orain, C., Sancho, D. De., Saujet, L., Sensi, M., Gauquelin, C., et al. (2017). Mechanism of O2 diffusion and reduction in FeFe hydrogenases. Nat. Chem. 9, 88–95. doi: 10.1038/nchem.2592
Kunkel, B. N. (2021). Auxin Plays Multiple Roles during Plant–Pathogen Interactions. Cold Spring Harb. Perspect. Biol. 9, a040022. doi: 10.1101/cshperspect.a040022
Kusale, S. P., Attar, Y. C., Sayyed, R. Z., El Enshasy, H., Hanapi, S. Z., Ilyas, N., et al. (2021). Inoculation of Klebsiella variicola alleviated salt stress and improved growth and nutrients in wheat and maize. Agronomy 11, 927. doi: 10.3390/agronomy11050927
Li, Z., Henawy, A. R., Halema, A. A., Fan, Q., Duanmu, D., Huang, R., et al. (2022). A wild rice rhizobacterium Burkholderia cepacia BRDJ enhances nitrogen use efficiency in rice. Int. J. Mol. Sci. 23, 10769. doi: 10.3390/ijms231810769
Ludueña, L. M., Anzuay, M. S., Angelini, J. G., McIntosh, M., Becker, A., Rupp, O., et al. (2018). Strain Serratia sp. S119: a potential biofertilizer for peanut and maize and a model bacterium to study phosphate solubilization mechanisms. Appl. Soil Ecol. 126, 107–112. doi: 10.1016/j.apsoil.2017.12.024
Lund, M., Agerbo Rasmussen, J., Ramos-Madrigal, J., Sawers, R., Gilbert, M. T. P., and Barnes, C. J. (2022). Rhizosphere bacterial communities differ among traditional maize landraces. Environ. DNA. 4, 1241–1249. doi: 10.1002/edn3.333
Lynch, J. M., Benedetti, A., Insam, H., Nuti, M. P., Smalla, K., Torsvik, V., et al. (2004). Microbial diversity in soil: ecological theories, the contribution of molecular techniques and the impact of transgenic plants and transgenic microorganisms. Biol. Fertil. Soils. 40, 363–385. doi: 10.1007/s00374-004-0784-9
Magallon-Servin, P., Antoun, H., Taktek, S., and de-Bashan, L. E. (2020). Designing a multi-species inoculant of phosphate rock-solubilizing bacteria compatible with arbuscular mycorrhizae for plant growth promotion in low-P soil amended with PR. Biol. Fertil. Soils. 56, 521–536. doi: 10.1007/s00374-020-01452-1
Maier, R. M., Pepper, I. L., and Gerba, C. P. (2009). Introduction to Environmental Microbiology in Environmental Microbiolog. London: Arizona, Press, 3–7.
Mannaa, M., and Kim, K. D. (2018). Biocontrol activity of volatile-producing Bacillus megaterium and Pseudomonas protegens against Aspergillus and Penicillium spp. predominant in stored rice grains: study II. Mycobiology 46, 52–63. doi: 10.1080/12298093.2018.1454015
McRose, D. L., Seyedsayamdost, M. R., and Morel, F. M. M. (2018). Multiple siderophores: bug or feature? J. Biol. Inorg. Chem. 23, 983–993. doi: 10.1007/s00775-018-1617-x
Mendoza, A., and Cruz, M. (2012). Empleo de Azospirillum Como Biofertilizante. In Introducción al uso y Manejo de Biofertilizantes en la Agricultura. México: México Press, 176–188.
Meza, B., Luz, E., and Bashan, Y. (2015). Involvement of indole-3-acetic acid produced by Azospirillum brasilense in accumulating intracellular ammonium in Chlorella vulgaris. Res. Microbiol. 166, 72–83. doi: 10.1016/j.resmic.2014.12.010
Mou, W., Kao, Y. T., Michard, E., Simon, A. A., Li, D., Wudick, M. M., et al. (2020). Ethylene-independent signaling by the ethylene precursor ACC in Arabidopsis ovular pollen tube attraction. Nat. Commun. 11, 1–11. doi: 10.1038/s41467-020-17819-9
Nascimento, F. X., Rossi, M. J., and Glick, B. R. (2018). Ethylene and 1-Aminocyclopropane-1-carboxylate (ACC) in plant–bacterial interactions. Front. Plant Sci. 9, 114. doi: 10.3389/fpls.2018.00114
Nautiyal, C. S. (1999). An efficient microbiological growth medium for screening phosphate solubilizing microorganisms. FEMS Microbiol. Lett. 170, 265–270. doi: 10.1111/j.1574-6968.1999.tb13383.x
Nehra, V., and Choudhary, M. (2015). A review on plant growth promoting rhizobacteria acting as bioinoculants and their biological approach towards the production of sustainable agriculture. J. Appl. Nat. Sci. 7, 540–556. doi: 10.31018/jans.v7i1.642
Ngalimat, M. S., Mohd Hata, E., Zulperi, D., Ismail, S. I., Ismail, M. R., Mohd Zainudin, N. A. I., et al. (2021). Plant growth-promoting bacteria as an emerging tool to manage bacterial rice pathogens. Microorganisms 9, 682. doi: 10.3390/microorganisms9040682
Nosheen, S., Ajmal, I., and Song, Y. (2021). Microbes as biofertilizers, a potential approach for sustainable crop production. Sustainability 134, 1868. doi: 10.3390/su13041868
Ohore, O. E., Qin, Z., Sanganyado, E., Wang, Y., Jiao, X., Liu, W., et al. (2022). Ecological impact of antibiotics on bioremediation performance of constructed wetlands: Microbial and plant dynamics, and potential antibiotic resistance genes hotspots. J. Hazard. Mater. 424, 127495. doi: 10.1016/j.jhazmat.2021.127495
Olanrewaju, O. S., and Babalola, O. O. (2019). Bacterial consortium for improved maize (Zea mays L.) production. Microorganisms 7, 519. doi: 10.3390/microorganisms7110519
Owen, D., Williams, A. P., Griffith, G. W., and Withers, P. J. (2015). Use of commercial bio-inoculants to increase agricultural production through improved phosphorus acquisition. Appl. Soil Ecol. 86, 41–54. doi: 10.1016/j.apsoil.2014.09.012
Payne, S. M. (1994). Detection, isolation, and characterization of siderophores. Meth. Enzymol. 235, 329–344. doi: 10.1016/0076-6879(94)35151-1
Pellicciaro, M., Lione, G., Giordano, L., and Gonthier, P. (2021). Biocontrol potential of Pseudomonas protegens against Heterobasidion species attacking conifers in Europe. Biol. Control. 157, 104583. doi: 10.1016/j.biocontrol.2021.104583
Pérez, R., Gonzalez, T., and Muñoz, J. (2014). Antagonismo microbiano asociado a cepas bacterianas provenientes de jitomate (Lycopersicum esculentum Mill) y maíz (Zea mays). Rev. Iberoam. Cienc. 1, 53–60.
Peterson, R. F., Campbell, A. B., and Hannah, A. E. (1948). A diagrammatic scale for estimating rust intensity on leaves and stems of cereals. Can. J. Res. 26, 496–500. doi: 10.1139/cjr48c-033
Prigigallo, M. I., Cabanás, C. G. L., Mercado-Blanco, J., and Bubici, G. (2022). Designing a synthetic microbial community devoted to biological control: the case study of Fusarium wilt of banana. Front. Microbiol. 13, 967885. doi: 10.3389/fmicb.2022.967885
Qin, S., Zhang, Y. J., Yuan, B., Xu, P. Y., Xing, K., Wang, J., et al. (2014). Isolation of ACC deaminase-producing habitat-adapted symbiotic bacteria associated with halophyte Limonium sinense (Girard) Kuntze and evaluating their plant growth-promoting activity under salt stress. Plant Soil 374, 753.– 766. doi: 10.1007/s11104-013-1918-3
Raaijmakers, J. M., Paulitz, T. C., Steinberg, C., Alabouvette, C., and Moënne-Loccoz, Y. (2009). The rhizosphere: a playground and battlefield for soilborne pathogens and beneficial microorganisms. Plant Soil 321, 341–361. doi: 10.1007/s11104-008-9568-6
Ramette, A., Frapolli, M., Fischer-Le Saux, M., Gruffaz, C., Meyer, J. M., Défago, G., et al. (2011). Pseudomonas protegens sp. nov., widespread plant-protecting bacteria producing the biocontrol compounds 2, 4.-diacetylphloroglucinol and pyoluteorin. Syst. Appl. Microbiol. 34, 180-188. doi: 10.1016/j.syapm.2010.10.005
Rana, A., Saharan, B., Joshi, M., Prasanna, R., Kumar, K., Nain, L., et al. (2011). Identification of multi-trait PGPR isolates and evaluating their potential as inoculants for wheat. Ann. Microbiol. 61, 893–900. doi: 10.1007/s13213-011-0211-z
Rashid, M., Khalil, S., Ayub, N., Alam, S., and Latif, F. (2004). Organic acids production and phosphate solubilization by phosphate solubilizing microorganisms (PSM) under in vitro conditions. Pak. J. Biol. Sci. 7, 187–196. doi: 10.3923/pjbs.2004.187.196
Rawat, P., Das, S., and Shankhdhar, D. (2021). Phosphate-solubilizing microorganisms: Mechanism and their role in phosphate solubilization and uptake. J. Soil Sci. Plant Nutr. 21, 49–68. doi: 10.1007/s42729-020-00342-7
Reitz, Z. L. (2022). Genome mining strategies for metallophore discovery. Curr. Op. Biotechnol., 77, 102757. doi: 10.1016/j.copbio.2022.102757
Rekha, P. D., Lai, W. A., Arun, A. B., and Young, C. C. (2007). Effect of free and encapsulated Pseudomonas putida CC-FR2-4 and Bacillus subtilis CC-pg104 on plant growth under gnotobiotic conditions. Bioresour. Technol. 98, 447–451. doi: 10.1016/j.biortech.2006.01.009
Rios-Galicia, B., Villagómez-Garfias, C., De la Vega-Camarillo, E., Guerra-Camacho, J. E., Medina-Jaritz, N., Arteaga-Garibay, R. I., et al. (2021). The Mexican giant maize of Jala landrace harbour plant-growth-promoting rhizospheric and endophytic bacteria. 11, 447. doi: 10.1007/s13205-021-02983-6
Roriz, M., Carvalho, S. M., Castro, P. M., and Vasconcelos, M. W. (2020). Legume biofortification and the role of plant growth-promoting bacteria in a sustainable agricultural era. Agronomy 10, 435. doi: 10.3390/agronomy10030435
Rosler, J., Krekel, F., Amrhein, N., and Schmid, J. (1997). Maize phenylalanine ammonia-lyase has tyrosine ammonia-lyase activity. Plant Physiol. 113, 175–179. doi: 10.1104/pp.113.1.175
Sánchez-Cañizares, C., Jorrín, B., Poole, P. S., and Tkacz, A. (2017). Understanding the holobiont: the interdependence of plants and their microbiome. Curr. Opin. Microbiol. 38, 188–196. doi: 10.1016/j.mib.2017.07.001
Sandanakirouchenane, A., Haque, E., and Geetha, T. (2017). Recent studies on N2 fixing Burkholderia isolates as a biofertilizer for the sustainable agriculture. Int. J. Curr. Microbiol. Appl. Sci. 6, 2780–2796. doi: 10.20546/ijcmas.2017.611.329
Saritha, M., and Tollamadugu, N. P. (2019). The Status of Research and Application of Biofertilizers and Biopesticides: Global Scenario Recent Developments in Applied Microbiology and Biochemistry. New York, NY: Academic Press, 195–207.
Selosse, M. A., Baudoin, E., and Vandenkoornhuyse, P. (2004). Symbiotic microorganisms, a key for ecological success and protection of plants. C. R. Biol. 327, 639–648. doi: 10.1016/j.crvi.2003.12.008
Shayanthan, A., Ordoñez, P. A. C., and Oresnik, I. J. (2022). The role of synthetic microbial communities (SynCom) in sustainable agriculture. Front. Agron. 30, 58. doi: 10.3389/fagro.2022.896307
Singh, R. P., Shelke, G. M., Kumar, A., and Jha, P. N. (2015). Biochemistry and genetics of ACC deaminase: a weapon to “stress ethylene” produced in plants. Front. Microbiol. 6, 937. doi: 10.3389/fmicb.2015.00937
Souza, D., Armanhi, R. S. C. J. S. L., and Arruda, P. (2020). From microbiome to traits: designing synthetic microbial communities for improved crop resiliency. Front. Plant Sci. 11, 1179. doi: 10.3389/fpls.2020.01179
Szkop, M., Sikora, P., and Orzechowski, S. (2012). A novel, simple, and sensitive colorimetric method to determine aromatic amino acid aminotransferase activity using the Salkowski reagent. Folia Microbiol. 57, 1–4. doi: 10.1007/s12223-011-0089-y
Timofeeva, A. M., Galyamova, M. R., and Sedykh, S. E. (2022). Bacterial siderophores: Classification, biosynthesis, perspectives of use in agriculture. Plants 11, 3065. doi: 10.3390/plants11223065
Van de Poel, B., and Van Der Straeten, D. (2014), 1.-aminocyclopropane-1-carboxylic acid (ACC) in plants: more than just the precursor of ethylene! Front. Plant Sci. 5:640. doi: 10.3389/fpls.2014.00640
Van Deynze, A., Zamora, P., Delaux, P. M., Heitmann, C., Jayaraman, D., Rajasekar, S., et al. (2018). Nitrogen fixation in a landrace of maize is supported by a mucilage-associated diazotrophic microbiota. PLoS Biol. 16, e2006352. doi: 10.1371/journal.pbio.2006352
Verdin, A., Loune‘s-Hadj, S. A., Fontaine, J., Grandmougin-Ferjani, A., and Durand, R. (2006). Effects of anthracene on development of an arbuscular mycorrhizal fungus and contribution of the symbiotic association to pollutant dissipation. Mycorrhiza 16, 397–405. doi: 10.1007/s00572-006-0055-8
Vibha, B., and Neelam, G. (2012). Importance of exploration of microbial biodiversity. Int. Res. J. Biol. Sci. 1, 78–83.
Vieira, R. F., Silva, C. M. M. S., and Silveira, A. P. D. (2007). Soil microbial biomass C and symbiotic processes associated with soybean after sulfentrazone herbicide application. Plant Soil 300, 95–103. doi: 10.1007/s11104-007-9392-4
Wani, Z. A., Ashraf, N., Mohiuddin, T., and Riyaz-Ul-Hassan, S. (2015). Plant-endophyte symbiosis, an ecological perspective. Appl. Microbiol. Biotechnol. 99, 2955–2965. doi: 10.1007/s00253-015-6487-3
Wilkes, H. G. (1977). Hybridization of maize and teosinte, in Mexico and Guatemala and the improvement of maize. Econ. Bot. 31, 254–293. doi: 10.1007/BF02866877
Xue, S., Miao, L., Ma, Y., Du, Y., and Yan, H. (2016). Optimizing Bacillus circulans Xue-113168 for biofertilizer production and its effects on crops. Afr. J. Biotechnol. 15, 2795–2803. doi: 10.5897/AJB2016.15255
Zaller, J. G., König, N., Tiefenbacher, A., Muraoka, Y., Querner, P., Ratzenböck, A., et al. (2016). Pesticide seed dressings can affect the activity of various soil organisms and reduce decomposition of plant material. BMC Ecol. 16, 1–11. doi: 10.1186/s12898-016-0092-x
Keywords: endophytic bacteria, plant growth-promoting bacteria (PGPB), Jala maize, synthetic microbial communities (SynCom), plant-microbe interaction, induced systemic resistance (ISR), biocontrol
Citation: De la Vega-Camarillo E, Sotelo-Aguilar J, Rios-Galicia B, Mercado-Flores Y, Arteaga-Garibay R, Villa-Tanaca L and Hernández-Rodríguez C (2023) Promotion of the growth and yield of Zea mays by synthetic microbial communities from Jala maize. Front. Microbiol. 14:1167839. doi: 10.3389/fmicb.2023.1167839
Received: 16 February 2023; Accepted: 02 May 2023;
Published: 19 May 2023.
Edited by:
Eric Altermann, Massey University, New ZealandReviewed by:
Pratibha Vyas, Punjab Agricultural University, IndiaNI Luh Suriani, Udayana University, Indonesia
Copyright © 2023 De la Vega-Camarillo, Sotelo-Aguilar, Rios-Galicia, Mercado-Flores, Arteaga-Garibay, Villa-Tanaca and Hernández-Rodríguez. This is an open-access article distributed under the terms of the Creative Commons Attribution License (CC BY). The use, distribution or reproduction in other forums is permitted, provided the original author(s) and the copyright owner(s) are credited and that the original publication in this journal is cited, in accordance with accepted academic practice. No use, distribution or reproduction is permitted which does not comply with these terms.
*Correspondence: César Hernández-Rodríguez, chdez38@hotmail.com
†Present address: Bibiana Rios-Galicia, Institute of Animal Science, University of Hohenheim, Stuttgart, Germany