- College of Animal Science, Guizhou University, Guiyang, China
Dried distillers’ grains with solubles (DDGS) are rich in nutrients, and partially alternative feeding of DDGS effectively reduces cost of feed and improves animals’ growth. We used 16S rDNA gene sequencing and LC/MS-based metabolomics to explore the effect of feeding cattle with a basal diet (BD) and a Jiang-flavor DDGS diet (replaces 25% concentrate of the diet) on microbiome and metabolome of ruminal and cecal contents in Guanling yellow cattle. The results showed that the ruminal and cecal contents shared the same dominance of Bacteroidetes, Firmicutes and Proteobacteria in two groups. The ruminal dominant genera were Prevotella_1, Rikenellaceae_RC9_gut_group, and Ruminococcaceae_UCG-010; and the cecal dominant genera were Ruminococcaceae_UCG-005, Ruminococcaceae_UCG-010, and Rikenellaceae_RC9_gut_group. Linear discriminant analysis effect size analysis (LDA > 2, P < 0.05) revealed the significantly differential bacteria enriched in the DDGS group, including Ruminococcaceae_UCG_012, Prevotellaceae_UCG_004 and Anaerococcus in the ruminal contents, which was associated with degradation of plant polysaccharides. Besides, Anaerosporobacter, Anaerovibrio, and Caproiciproducens in the cecal contents were involved in fatty acid metabolism. Compared with the BD group, 20 significantly different metabolites obtained in the ruminal contents of DDGS group were down-regulated (P < 0.05), and based on them, 4 significantly different metabolic pathways (P < 0.05) were enriched including “Linoleic acid metabolism,” “Biosynthesis of unsaturated fatty acids,” “Taste transduction,” and “Carbohydrate digestion and absorption.” There were 65 significantly different metabolites (47 were upregulated, 18 were downregulated) in the cecal contents of DDGS group when compared with the BD group, and 4 significantly different metabolic pathways (P < 0.05) were enriched including “Longevity regulating pathway,” “Bile secretion,” “Choline metabolism in cancer,” and “HIF-1 signaling pathway.” Spearman analysis revealed close negative relationships between the top 20 significantly differential metabolites and Anaerococcus in the ruminal contents. Bacteria with high relevance to cecal differential metabolites were Erysipelotrichaceae_UCG-003, Dielma, and Solobacterium that affect specific metabolic pathways in cattle. Collectively, our results suggest that feeding cattle with a DDGS diet improves the microbial structure and the metabolic patterns of lipids and carbohydrates, thus contributing to the utilization efficiency of nutrients and physical health to some extent. Our findings will provide scientific reference for the utilization of DDGS as feed in cattle industry.
1. Introduction
Guizhou Moutai liquor is a well-known Jiang-flavor Chinese spirits made from local high-quality glutinous sorghum. The output of Moutai distillers’ grains (DG) exceeds 150,000 tons per year, which contain high water content with perishable properties, easily causing environmental pollution without proper disposal (Li M. Y. et al., 2022). DG is rich in crude protein, crude fat, crude fiber, vitamins, essential amino acids, and other nutrients (Zhang P. G. et al., 2022). Evidences have revealed that partially alternative feeding of DG effectively reduces environmental pollution and feed costs. Fresh DG are dried by the drying process to made into dried distillers’ grains with solubles (DDGS), which effectively improve their nutritional properties and extend their shelf life, and eventually can be added to livestock feed (Wang et al., 2021).
Current studies on the feed utilization of DG are mainly focused on the improvement of animal growth and the promotion of milk production and quality. It has been reported that the substitution of forage and soy protein with high-protein DDG effectively improves feed conversion ratio and milk production in Holstein cows (Hubbard et al., 2009). A recent study reports that feeding cattle with a mixed diet containing yellow wine lees both reduces oxidative stress in heat-stressed cattle and increases the antioxidant capacity of milk (Yao et al., 2021). Another study evaluating the effect of a mixed ration with corn DDGS replacing cornmeal on intake, animal performance, and carcass traits in Nellore cattle demonstrates that DDGS increase average daily gain and carcass gain in cattle (Da et al., 2022). And such an effect was also seen in fattening pigs (Li H. et al., 2022). These studies show that feeding the DDGS diets is beneficial to animals’ growth and improves the quality of animals’ products.
The genome of gastrointestinal microorganisms is rich in genes that regulate the metabolism of carbohydrates, amino acids, vitamins, and short-chain fatty acids. The changes of metabolites can reflect the differential expression of relevant genes, and these changes can be used to assess the physiological or pathological status of the organisms (Gill et al., 2006; Paganelli et al., 2022). With the rapid development of multi-omics in recent years, microbiome and metabolomics have been widely applied in animal-associated studies, such as investigation of the responses to ration-induced milk fat inhibition in dairy cattle and demonstration of the effect of dandelion on the rumen metabolome and microbiota in lactating cows (Zeng et al., 2019; Li Y. et al., 2021). However, there are few reports on the effects of a diet of DDGS on the gastrointestinal microbiome and metabolome in cattle. Guanling yellow cattle is a famous local cattle breed of Guizhou Province, China, and it was approved by the Ministry of Agriculture of China to implement geographical indication registration and protection of agricultural products (No. AGI2016-03-1987) in 2016. In this study, 16S rDNA gene sequencing and LC/MS-based metabolomics technology were adopted to explore the effect of feeding with a basal diet and mixed diet with 25% of concentrate replaced by DDGS on microbiome and metabolome of ruminal and cecal contents in Guanling yellow cattle, which will to some extent provide some theoretical basis for further utilization of Moutai liquor DG as a feed resource in livestock production.
2. Materials and methods
2.1. Ethics statement
Animal breeding, care, and use, as well as sample collection were performed according to the guidelines of the Experimental Animal Ethics Subcommittee of Guizhou University (No. EAE-GZu-2020-E018). All standard procedures concerning animal care and management were taken, and all efforts were made to minimize their suffering throughout the experiment.
2.2. Origin and preparation of DDGS
The DGs used in this study were obtained from the Kweichow Moutai Group in Moutai Town, Renhuai, Guizhou, China. The main ingredients of Moutai DG are distilled sorghum and wheat, that are a byproduct of the brewing processes. A drum dryer was used for the drying treatment before feeding. After centrifugation of fresh Moutai DG, the filter residues and filtrate were separated, and the filtrate was evaporated and concentrated, and then mixed and dried together with the filter residues to obtain DDGS with a moisture content of 10–15%.
2.3. Animal experiment
Twelve healthy Guanling yellow cattle (227 ± 18 kg, 18 months old) selected from Guizhou Cattle Industry Group Co., Ltd. were used in this study and were pre-tested without Brucella and foot-and-mouth disease virus-O/-A infection. Two experimental groups (containing 6 cattle each) were randomly assigned to one of the two dietary treatments: a basal diet (BD) and a Jiang-flavor DDGS diet replacing 25% of the concentrate by DDGS. The BD was formulated with reference to the nutritional requirement of 300 kg body weight and 1 kg/d average daily gain according to China’s Beef Feeding Standard (NY/T 815-2004), with a forage: concentrate ratio of 60:40 on a dry matter basis. And Pennisetum sinese Roxb was added as a forage in the diet. The composition of experimental concentrate is shown in the Supplementary Table 1, and the nutritional levels of two diets are shown in Supplementary Table 2. The cattle were fed at 9:00 and 16:30 every day for 75 days (15 days for adaptation to the experimental diet and 60 days for formal feeding of the experimental diet). During the entire experiment, the cattle drank water freely, and hygiene and daily management were carried out as a matter of routine. At the end of the experiment, 3 experimental cattle in each group were randomly selected for sacrificed via electrical stunning, and the ruminal contents (BD-R and DDGS-R) and cecal contents (BD-C and DDGS-C) were collected. All samples were collected and frozen in liquid nitrogen for 12 h and then transferred to −80°C for storage until subsequent microbiomic and metabonomic analyses.
2.4. 16s rDNA gene amplification, sequencing, and data mining
Total genomic DNA was extracted from ruminal and cecal contents of each cattle using a DNeasy PowerSoil kit (QIAGEN NO12888, New York, USA). We used universal primers to augment the V3 and V4 regions of the 16S rDNA gene, and the primer sequences were 343F (5′-TACGGRAGGCAGCAG-3′) and 798R (5′-AGGGTATCTAATCCT-3′). All the polymerase chain reactions (PCR) were performed using a Tks Gflex DNA Polymerase kit (Takara 580BR10905, Kyoto, Japan). Each run included 2 × Gflex PCR Buffer 15 μL, Forward primer (5 pmol/μL) 1 μL, Reverse primer (5 pmol/μL) 1 μL, Template DNA 1 μL, Tks Gflex DNA Polymerase (1.25 U/μL) 0.6 μL, and ddH2O 11.4 μL. Amplification condition was set as follows: the pre-degeneration was performed at 94°C for 5 min, then 26 cycles of denaturation (94°C, 30 s), annealing (56°C, 30 s) and elongation (72°C, 20 s) were performed, followed by extension at 72°C for 5 min.
After PCR amplification, all amplicon libraries were sequenced using an Illumina MiSeq PE 300 platform (Illumina, California, USA). The quality control of each dataset was performed using Trimmomatic v0.35 software to trim the 3′-end of reads and 5′-end of reads, cut low-quality bases (quality scores < 20), and remove short reads (< 50 bp) and “N” records. Paired-end reads were merged into tags using FLASH v1.2.11 with a minimum overlap of a 10-base sequence. UCHIME v2.4.2 was used to remove the chimera sequence in the sequence to obtain final clean reads. Tags were clustered into operational taxonomic units (OTU) at a 97% similarity threshold using Vsearch v1.9.6 software. Species annotation analysis was conducted using RDP classifier v2.2 software (the threshold value was set as 0.7∼1.0). The alpha(α) diversity, including Chao1, Shannon, and Simpson indices was used to determine the richness and diversity of bacterial community. The beta (β)-diversity calculations were performed using QIIME v1.8.0 and displayed with R software v2.15.3. β-diversity was estimated by computing the unweighted UniFrac distance and visualized using principal coordinate analysis (PCoA). Based on Kruskal–Wallis (KW) sum-rank test, the linear discriminant analysis effect size (LEfSe) method was used to identify the most differentially abundant taxonomic features at genus levels. For this analysis, the significance threshold for the Kruskal–Wallis (KW) test was set to 0.05, and the logarithmic linear discriminant analysis (LDA) score cut-off was set to 2.0.
2.5. LC/MS-based metabonomic determination and data analysis
The metabonomic analysis was done using an ACQUITY ultra-performance liquid-chromatography (UPLC) I-Class ultra-high performance liquid chromatography tandem VION IMS Q-Tof high-resolution mass spectrometer (MS). 30 mg samples were mixed with L-2-chlorophenylalanine (20 μL, 0.3 mg/mL) and 600 μL cold 20% methanol/water and then vortex-mixed for 2 min. The solution was ultrasonically extracted on ice for 3 min, incubated at −20°C for 30 min and then centrifuged at 13,000×g at 4°C for 10 min. A total of 150 μL supernatant was used for LC/MS analysis. Chromatographic separation was carried out with an Acquity uplc BEH C-18 column (100 mm × 2.1 mm, 1.7 μm) with a temperature of 45°C. The UPLC mobile phases were (A) 0.1% formic acid-water and (B) 0.1% formic acid-acetonitrile. The injection volume was 1 μL, and the flow rate was 0.4 mL/min. Mass spectrometry was performed in both positive and negative mode. The ion source temperature was 115°C and the capillary voltage 2.5 kV, the injection voltage was 40 V, the collision voltage was 4 eV, the desolvation temperature was 450 m, the desolvation gas flow was 900 L/h, the mass spectrometry scan range was 50–1,000 amu, scan time was 0.2 s, interscan time 0.02 s. Quality control (QC) sample was prepared by mixing aliquots of each fluid sample. The QC was performed by running four samples as technical replicates in positive and negative mode, respectively, to verify the stability and reproducibility of the UPLC/MS system.
The raw data were used for peak exacting, data baseline filtering and calibration of the baseline, peak alignment, deconvolution analysis, peak identification, and integration of the peak area by the Progenesis QI v2.3. Multidimensional statistical analysis is performed, including the unsupervised Principal Component Analysis (PCA) method and orthogonal partial least squares discriminant analysis (OPLS-DA) with supervised regression modeling to identify the significantly differential metabolites. The differential metabolites were filtered and confirmed by combining the results of the variable importance in the projection (VIP) generated in OPLS-DA, t-test (P < 0.05) and fold change (FC) of the peak intensities (mean value of peak intensity obtained from DDGS group/mean value of peak intensity obtained from BD group). The screening standard was FC > 1, P value < 0.05 and VIP > 1. and KEGG functional annotation and enrichment analysis were performed for differential metabolites. Correlations between differential metabolites (top20) and genus-level microbes were explored with Spearman’s correlation analysis using the OECloud tools in https://cloud.oebiotech.cn. For all analyses, FDR-corrected P-values below 0.05 were considered statistically significant.
3. Results
3.1. 16S rDNA sequencing results and diversity estimates
A total of 624,283 clean reads were obtained from 6 rumen fluid samples, and 457,238 clean reads were obtained from 6 cecal samples via Illumina sequencing. Read shear filtration and quality control obtained an average of 66,212 ± 2,976 and 65,266 ± 1,829 in the rumen and cecum, respectively, and the quality control effective rate was over 81%. The Venn diagram showed that the intersection number of OTU was 6,371 in the BD-R and DDGS-R groups (Figure 1A), and the intersection number of OTU was 6,013 in the BD-C and DDGS-C groups (Figure 1B). 4,598 and 4,374 specific OTUs were observed in the BD-R group and DDGS-R group, respectively (Figure 1A). 4,616 and 3,190 specific OTUs were observed in the BD-C group and DDGS-C group, respectively (Figure 1B). As shown in the Supplementary Table 3, no significant differences in Chao1 richness, Shannon diversity index, and Simpson index of rumen and cecum between the BD and DDGS groups was observed. However, the Good’s coverages for all samples exceeded 96%, indicating the accuracy and reproducibility of the sequencing. Based on bacterial species, the PcoA results showed separations between the two treatments in the rumen (Figure 1C) and cecum (Figure 1D), respectively.
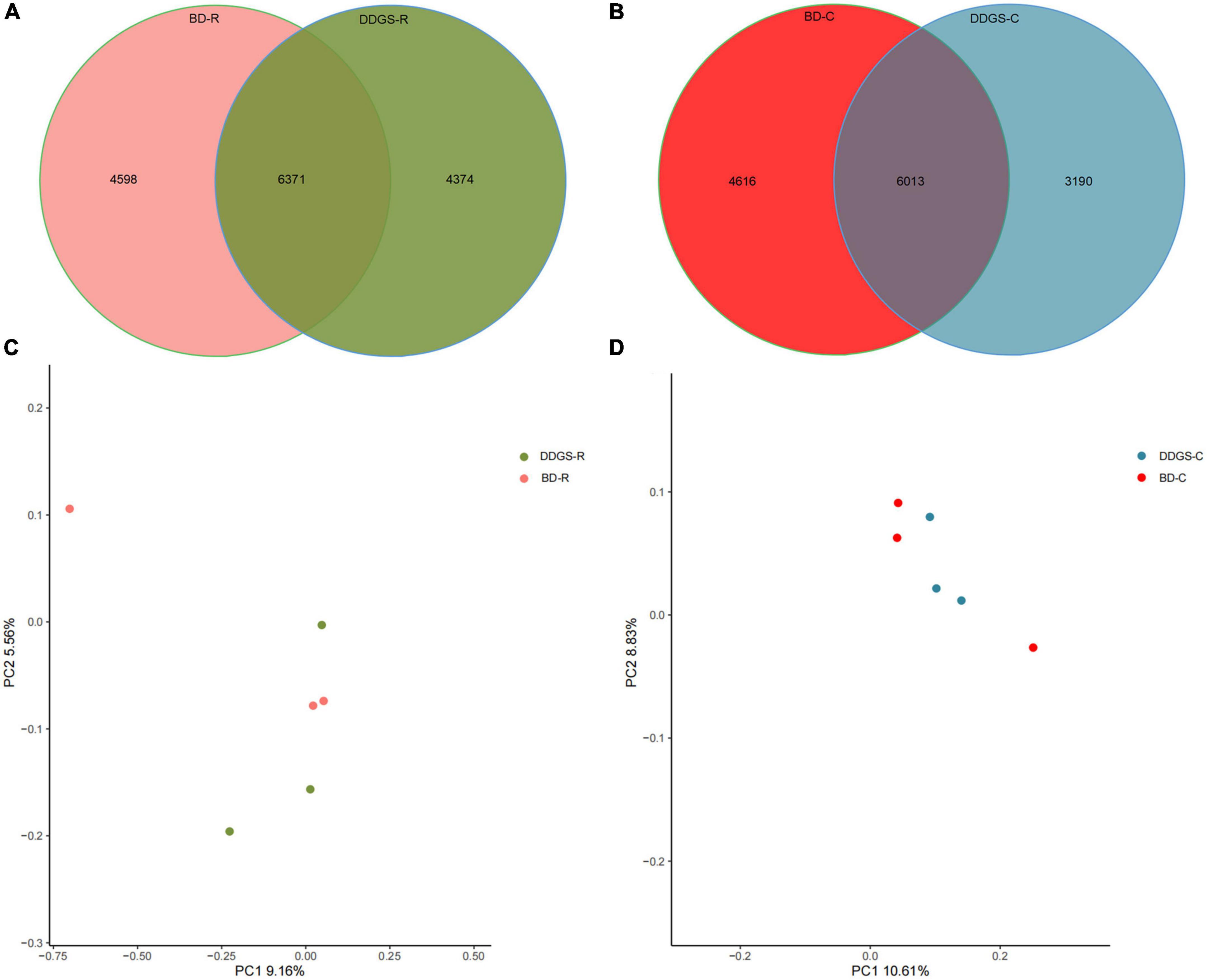
Figure 1. Bacterial diversity analysis. Venn diagram was drawn to illustrate the overlap of microbial OTUs in the ruminal (A) and cecal (B) contents between the BD and DDGS groups. β-diversity changes in ruminal (C) and cecal (D) microbiota across BD and DDGS groups were assessed by the principal coordinate analysis (PCoA).
3.2. Bacterial community composition analyses
Taxonomic analysis of the reads revealed that Bacteroidetes, Firmicutes and Proteobacteria were the dominant phyla of the ruminal and cecal contents in two groups (Figures 2A, C). Feeding DDGS increased the abundance of Bacteroidetes (P = 0.67) and Firmicutes (P = 0.46) and decreased the abundance of Proteobacteria (P = 0.35) in the ruminal contents (Figure 2A). However, feeding DDGS decreased the abundance of Bacteroidetes (P = 0.50) and increased the abundance of Proteobacteria (P = 0.29) in the cecal contents (Figure 2C). At the genus level, Prevotella_1 and Rikenellaceae_RC9_gut_group were the dominant genera in the ruminal contents (Figure 2B), and Prevotella_1 (P = 0.46), Erysipelotrichaceae_UCG-004 (P = 0.26) and Ruminococcaceae_UCG-010 (P = 0.0.28) were enriched in DDGS-R group. The dominant genera in the cecal contents were Ruminococcaceae_UCG-010, Rikenellaceae_RC9_gut_group, Bacteroides and Ruminococcaceae_UCG-005, and Rikenellaceae_RC9_gut_group were enriched in DDGS-C group (Figure 2D).
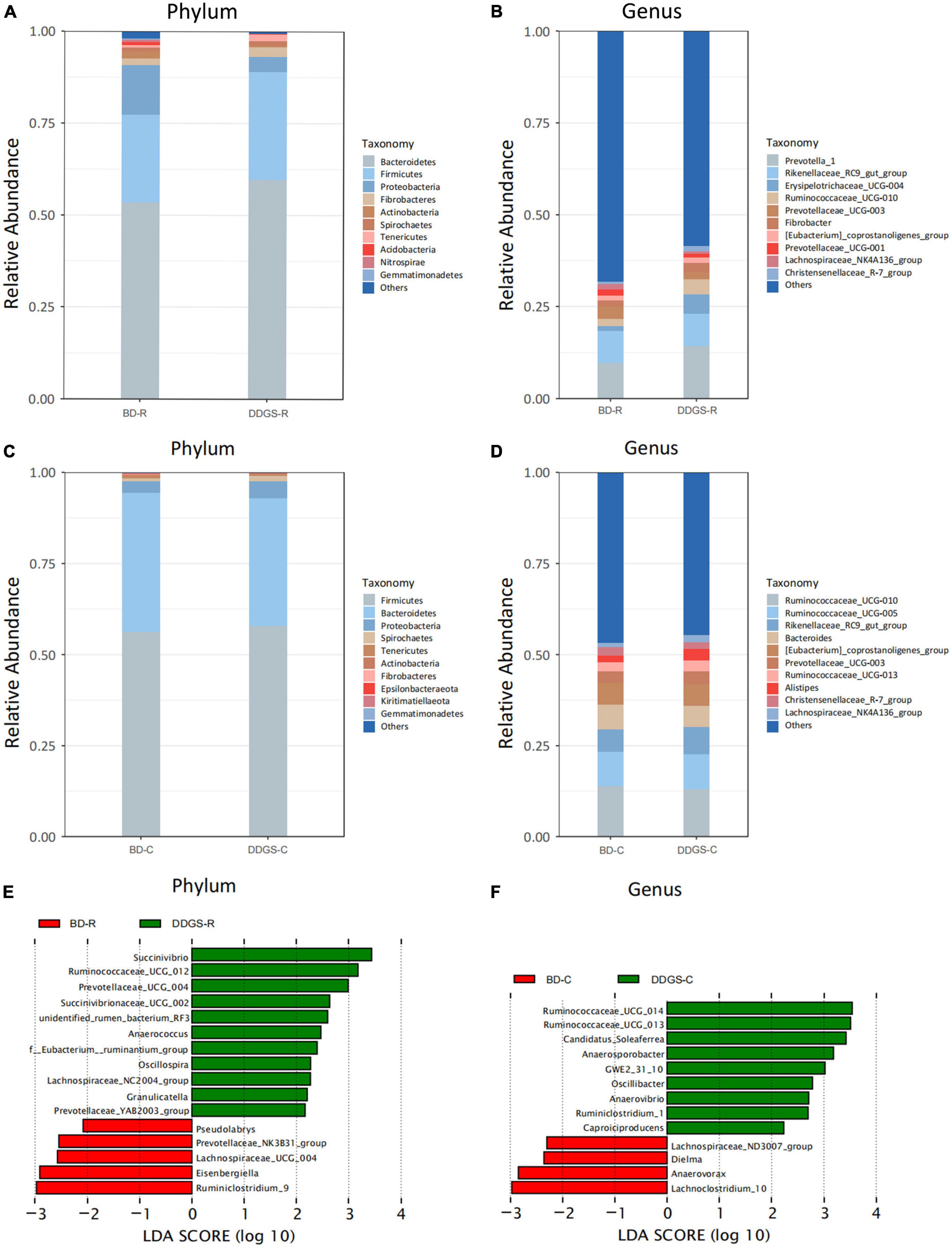
Figure 2. Microbial compositional profiles of the ruminal and cecal contents in the BD and DDGS group. Column chart showing the bacterial composition of the ruminal and cecal contents at the phylum (A,C) and genus level (B,D), respectively. Differential microbial genera in the ruminal (E) and cecal (F) contents of the BD and DDGS groups were tested by linear discriminant analysis effect size (LEfSe) analysis, with linear discriminant analysis (LDA) score of > 2 and P-value of < 0.05.
The LEfSe analysis (LDA > 2, P < 0.05) performs a non-parametric Kruskal–Wallis (KW) sum-rank test to assess the effect size of each differentially abundant bacterium. At the genus level, the relative abundance of Succinivibrio, Ruminococcaceae_UCG_012, Prevotellaceae_UCG_004, Anaerococcus, Oscillosp-ira, Succinivibrionaceae_UCG_002, unidentified_rumen_bacterium_RF3, f__Eubacterium__ ruminantium_group, Lachnospiraceae_NC2004_group, Granul-icatella, and Prevotellaceae_YAB2003_ group were increased in the DDGS-C group compared with the BD-C group, while the relative abundance of Ruminiclostridium_9, Eisenbergiella, Lachnospiraceae_UCG_004, Prevotellaceae_NK3B31_group, and Pseudolabrys were decreased (Figure 2E). The relative abundance of Ruminococcaceae_ UCG_014, Ruminococcaceae_UCG_013, Candidatus_Soleaferrea, Anaerosporobacter, GWE2_31_10, Oscillibacter, Anaerovibrio, Ruminiclostridium_1, and Caproiciproducens were increased in DDGS-C group, however, Lachnoclostridium_10, Anaerovorax, Dielma, and Lachnospiraceae_ND3007_group were decreased (Figure 2F).
3.3. Metabolomics profiling of ruminal and cecal contents
In this study, the overlay of the QC samples in the PCA plot indicated that this model was stable, reproducible and consistent for all the samples (Supplementary Figure 1). PCA plots of the ruminal and cecal contents samples provided a less satisfactory separation between the two sets of data (Supplementary Figure 2). Further evaluation of the parameters of the OPLS-DA model presented that the ruminal and cecal fluids of BD and DDGS group had significantly differential metabolite compositions (Figures 3A, B), which indicated that metabolites obtained in the ruminal and cecal fluid samples of two treatments were markedly distinct.
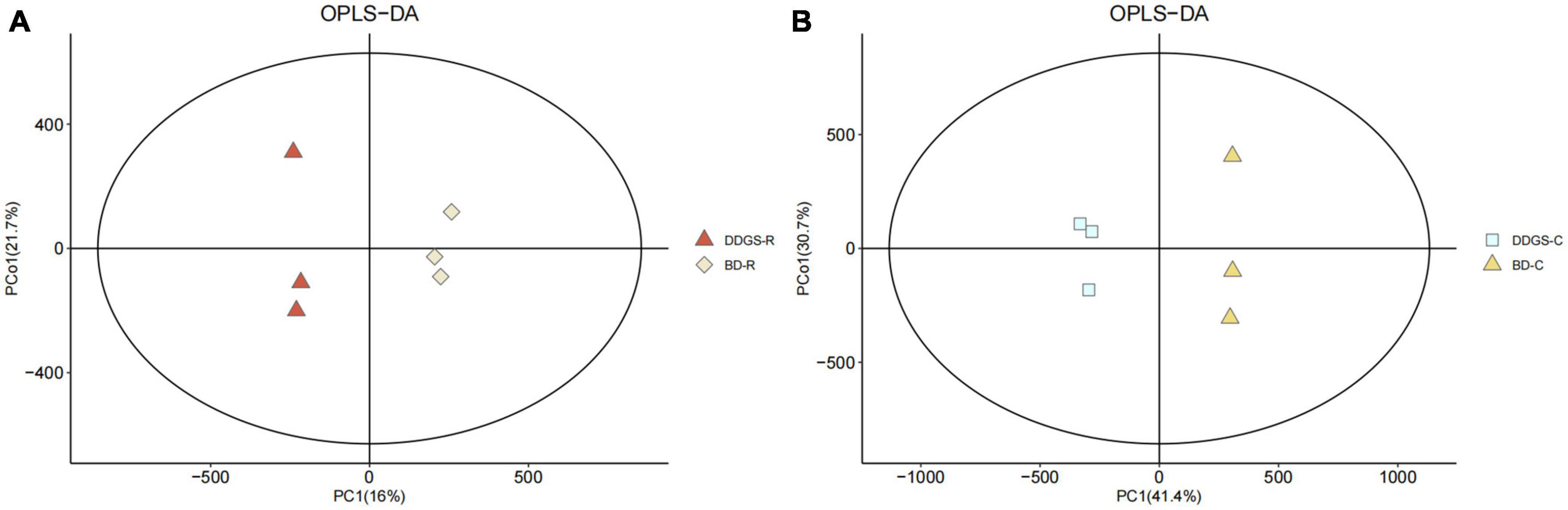
Figure 3. Orthogonal partial least squares discriminant analysis (OPLS-DA) plot of ruminal (A) and cecal (B) contents metabolites in comparisons between BD and DDGS groups.
A total of 227 metabolites were identified in the ruminal and cecal metabolome. After t-test and VIP filtering for the relative concentrations of metabolites, 20 significantly differential metabolites were downregulated in the DDGS-R group in comparison with the BD-R group (P < 0.05, VIP > 1; Table 1). Among them, 11, 2, 2, 2, and 3 metabolites were super-classified into “Lipids and lipid-like molecules,” “Organic oxygen compounds,” “Phenylpropanoids and polyketides,” “Organoheterocyclic compounds,” and “Unclassified,” respectively. These significantly differential metabolites in the rumen were enriched into 10 metabolic pathways, among which “Linoleic acid metabolism,” “Biosynthesis of unsaturated fatty acids,” “Taste transduction,” and “Carbohydrate digestion and absorption” were the significantly differential pathways (P < 0.05, Figure 4A).
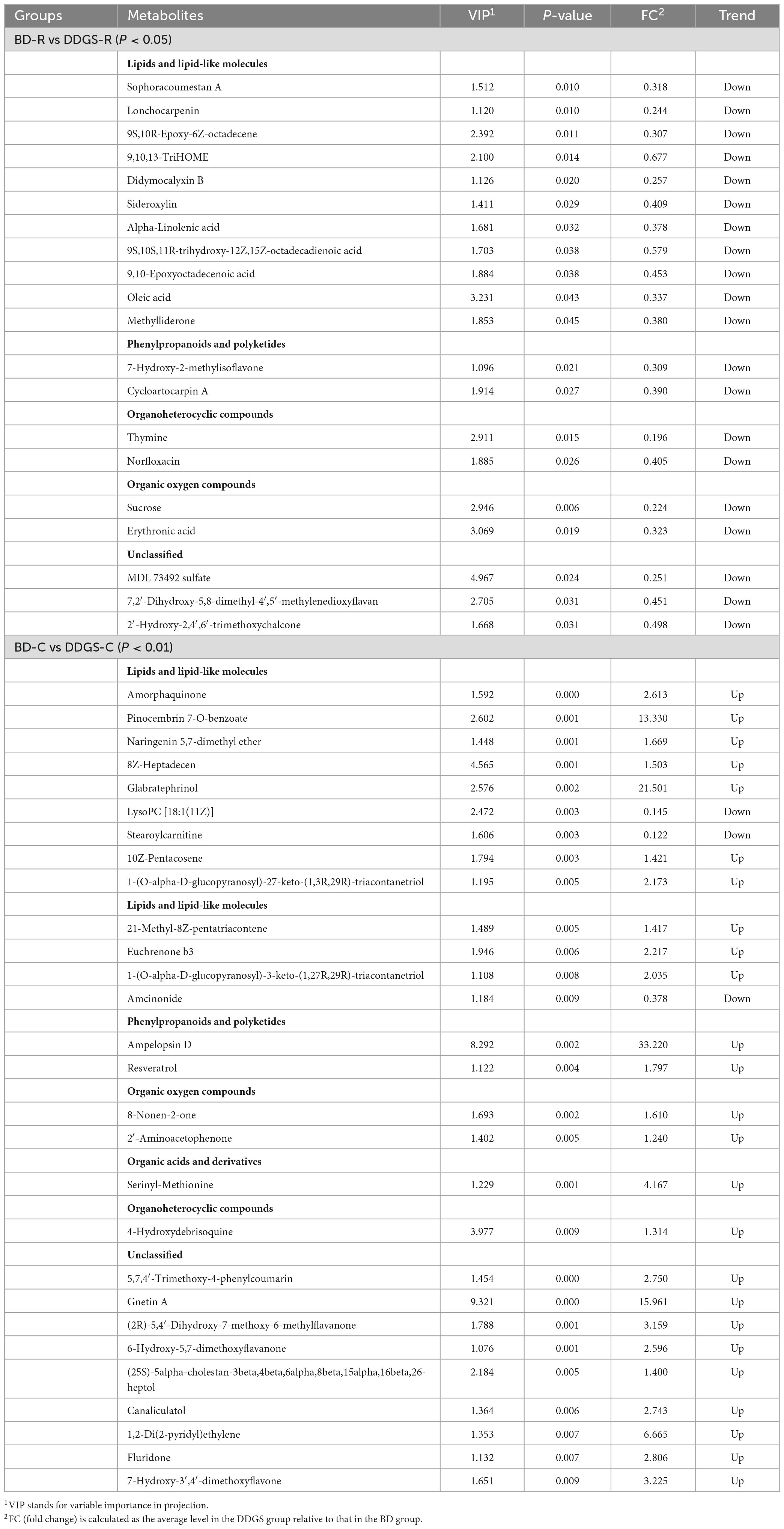
Table 1. List of ruminal and cecal fluid metabolites that showed difference between BD and DDGS groups (P < 0.05 or P < 0.01).
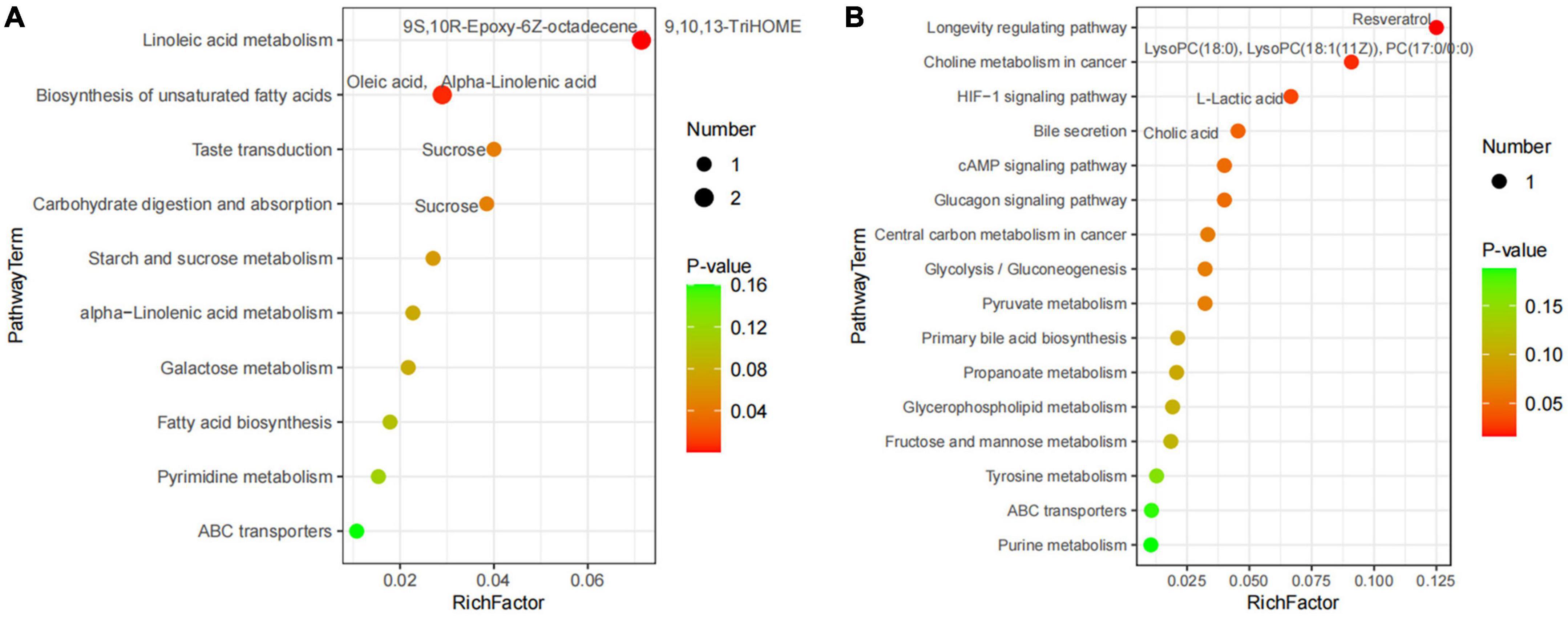
Figure 4. Pathway impact resulting from the differential ruminal (A) and cecal (B) metabolites in BD and DDGS groups. The X-axis represents pathway impact and the Y-axis represents the pathway enrichment. The larger size of the circle indicates greater pathway enrichment and the darker color indicates higher pathway impact values. The closer the color is to red, the smaller the P-value is. Significantly different metabolic pathways are marked around each dot with differential metabolites.
A total of 65 differential metabolites (47 upregulated and 18 downregulated) were obtained in the DDGS-C group compared with the BD-C group (P < 0.05, VIP > 1; Supplementary Table 4). Of these, 28 highly significantly differential metabolites were shown in Table 1 (P < 0.01 and VIP > 1). Among these 65 differential metabolites, 31, 5, 7, 2, 2, 1, and 17 metabolites were super-classified into “Lipids and lipid-like molecules,” “Phenylpropanoids and polyketides,” “Organoheterocyclic compounds,” “Organic oxygen compounds,” “Organic acids and derivatives,” “Benzenoids,” and “Unclassified,” respectively. These significantly differential metabolites in the cecal contents were enriched into 16 metabolic pathways, among which “Longevity regulating pathway,” “Bile secretion,” “choline metabolism in cancer,” and “HIF-1 signaling pathway” were significantly differential pathways (P < 0.05, Figure 4B). These results suggest that the addition of DDGS to the diet of Guanling yellow cattle causes some changes in metabolites and metabolic pathways in the ruminal and cecal contents.
3.4. Correlation between the microbiome and metabolome in ruminal and cecal contents
To explore the potential relationship between microbiota and metabolites of DDGS-induced changes in the rumen and cecum of cattle. The correlation network between discrepant microbiota and top20 differential metabolites in the BD and DDGS groups were assessed based on Spearman’s correlation coefficients (| r| > 0.88, P < 0.05). The correlation network of the ruminal consisted of 23 nodes and 38 edges, including 18 positive correlations and 20 negative correlations (Figure 5A). Top 20 differential metabolites, including MDL 73492 sulfate, 9,10,13-TriHOME, Oleic acid, Sucrose, and Erythronic acid were negatively correlated with the relative abundance of Anaerococcus, and 9 of them were positively correlated with both Lachnospiraceae_UCG-004 and Eisenbergiella.
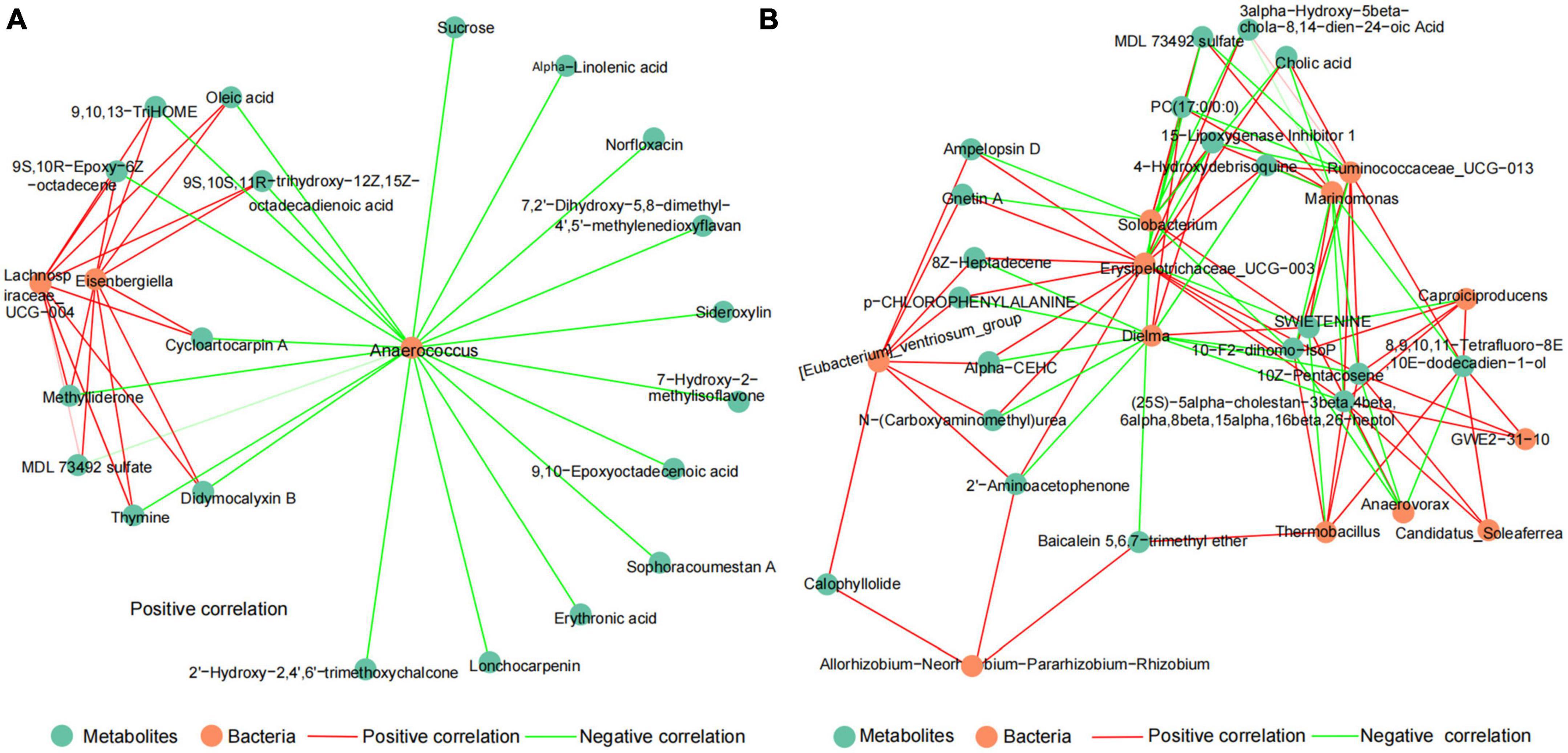
Figure 5. The spearman correlation networks between top20 different metabolites and discrepant bacteria of the ruminal (A) and cecal (B) contents between BD and DDGS groups. The correlation coefficients with a statistical P value < 0.05 and the absolute value > 0.8 were used to build the network graph. Green nodes represent metabolites, orange nodes indicate bacteria. Red lines denote positive correlations, while green lines denote negative correlations.
Likewise, the correlation network of the cecal contents consisted of 32 nodes and 94 edges, including 58 positive correlations and 36 negative correlations (Figure 5B). It was found that top 20 significantly differential metabolites had highly correlation with the relative abundance of 6 bacteria, including Erysipelotrichaceae_UCG-003 (13 positive relationships, 4 negative relationships), Dielma (3 positive relationships, 9 negative relationships), Marinomonas (4 positive relationships, 7 negative relationships), Ruminococcaceae_UCG-013 (7 positive relationships, 4 negative relationships), Solobacterium (4 positive relationships, 6 negative relationships), and [Eubacterium]_ventriosum_group (8 positive relationships). Furthermore, we identified 12 hub metabolites such as 10-F2-dihomo-IsoP, 10Z-Pentacosene, PC (17:0/0:0), and Cholic acid. These metabolites mainly belong to “Lipids and lipid-like molecules,” and some of these metabolites were “Organic oxygen compounds” and “Unclassified.” Based on correlation network analysis, we found that the abundance of Anaerococcus and Erysipelotrichaceae_UCG-003 may be important for changes in the rumen and cecum metabolites, respectively.
4. Discussion
Complex microbiota in gastrointestinal tract of ruminants play critical roles in maintaining homeostasis, regulating energy metabolism, and activating intestinal immunity (Zhang Y. et al., 2021), which can be regulated by changing the diet to improve feed utilization rate, the growth and development of animals (Dill-McFarland et al., 2019; Cui et al., 2022). Our study suggested that DDGS diets have a potent ability to reshape the ruminal and cecal microbial communities. Similar to other studies (Kim et al., 2022), Bacteroidetes, Firmicutes, and Proteobacteria were the dominant phyla in all samples, and the abundance of Firmicutes and Bacteroidetes were increased in the DDGS-R group. Both Firmicutes and Bacteroidetes are key participants in fiber fermentation and carbohydrate degradation, producing multiple cellulases to hydrolyze macromolecular compounds such as cellulose and sugars (Chen et al., 2019; Li X. et al., 2021), which facilitates fat deposition, milk fat production and nutrient digestion and absorption (Spence et al., 2006; Brulc et al., 2009; Jami et al., 2014). Proteobacteria, whose abundance was increased in DDGS-C group, has been evidenced to be involved in the degradation and fermentation of biopolymers (Zhang Z. et al., 2021). Saccharolytic and anaerobic microbiota (such as Prevotella_1, Ruminococcaceae_UCG-010 and Fibrobacter) can especially aid in the degradation of host indigestible carbohydrates (such as cellulose and resistant polysaccharides) into monomeric or dimeric sugars, and subsequently ferment them into short-chain fatty acids (SCFAs) (Tremaroli and Backhed, 2012; Koh et al., 2016). We observed an increase in these bacterial genera in the DDGS-R group. In addition, fiber-degrading bacteria with increased abundance in the DDGS-C group included Firmicutes, Ruminococcaceae_UCG-005, Alistipes, Ruminococcaceae_UCG-013, Rikenellaceae_RC9_gut_group, and Prevotellaceae_UCG-003, which exert important fermentative and degradative effects on the indigestible dietary fiber in the foregut (Macfarlane and Macfarlane, 2012; Williams et al., 2016). Similar to other studies (Zou et al., 2020), our study found that the diversity, structure, and composition of the microbial communities were distinct between the rumen and cecum of cattle fed DDGS diet, which also leads to differences in metabolome of rumen and cecum. Similar to ruminal fermentation, in the ruminant’s cecum feed digestion is also performed by a specialized consortia of microorganisms. Fermentable substrates arriving in the cecum are different to those in the rumen, which may result in compositional or structural differences in the microbiota of these two compartments (Popova et al., 2017). These results showed that DDGS diets increased the abundance of bacteria associated with glycolysis and fiber degradation, which is beneficial to the digestion and absorption of polysaccharides in cattle.
Simultaneously, increasing evidence confirms that gut microbes (for instance, Prevotellaceae_UCG_004, Prevotellaceae_YAB2003_group, Anaerovibrio, Lachnospiraceae_ NC2004_group, Anaerosporobacter and Caproiciproducens) affect lipid deposition and fatty acid content by altering lipid metabolism (Tremlett et al., 2021; Tang et al., 2022; Zhu et al., 2022). LEfSe analysis in our study also showed that these bacteria were enriched in the DDGS group, and we hypothesized that this may affect fat metabolism in the gastrointestinal tract of experimental cattle in the DDGS group and has a positive role in weight gain of cattle. Lachnospiraceae_NC2004_group and Anaerococcus play critical roles in fermenting and degrading carbohydrates such as cellulose, pectin, and xylan, as well as producing short-chain fatty acids (Murphy and Frick, 2013; Vacca et al., 2020). Both of them were enriched in the DDGS-R group. In addition, another bacterium Candidatus_Soleaferrea up-regulated by DDGS diet has been reported to be involved in intestinal immunity (Zou et al., 2022), suggesting that DDGS diet has the potential to promote gut immunity and health. To sum up, DDGS diet changes the gastrointestinal microbiota of experimental Guanling yellow cattle and subsequently enhances the ability of fermenting and degrading macromolecular compounds, including carbohydrates and lipids, altering lipid metabolism, thereby improving the digestion and absorption of nutrients and promoting intestinal health and weight gain in cattle.
Accumulating evidence shows that changes in structure and function of the intestinal microbiota affect host metabolism and immunity (Muller et al., 2021). Similar to the results of previous studies related to dietary modifications (Tang et al., 2021; Zhang X. et al., 2022), our results showed that metabolites affected by DDGS diet were mainly “Lipids and lipid-like molecules,” “Phenylpropanoids and polyketides,” “Organoheterocyclic compounds,” and “Organic oxygen compounds,” which are all important participants in glycolipid metabolisms and redox reactions (Cohain et al., 2021; Hao et al., 2022). It has been reported that Anaerococcus participate in glycolysis and produce butyrate (Donohoe et al., 2012; Rivera-Chávez et al., 2016). Interestingly, we found that the Anaerococcus was enriched and negatively correlated with 20 downregulated differential metabolites in the DDGS-R group compared with the BD-R group in the spearman analysis. This finding suggests that abundant Anaerococcus may improve the metabolism of certain lipids and carbohydrates. Some previous studies reported that Erysipelotrichaceae and [Eubacterium]_ventriosum_group are the key bacteria for butyrate production to regulate intestinal immunity (Wei et al., 2018; Liu et al., 2019), and Dielma and Solobacterium may be involved in the synthesis of short-chain fatty acids and biohydrogenation of fatty acids (Salami et al., 2021; Qiu et al., 2022). In the spearman analysis, we found these bacteria had strong correlation with cecal top20 differential metabolites, which mainly belong to “Lipids and lipid-like molecules” and “Organic oxygen compounds.” These findings indicate that DDGS diet changes the gastrointestinal microbiota, metabolism of lipid and organic oxygen compounds in experimental Guanling yellow cattle.
It is well known that sucrose is broken down into glucose in the rumen and absorbed in small intestine. Here we found that sucrose was downregulated in the rumen of the DDGS group and DDGS diet also affected sugar-related metabolic pathways including “Taste transduction” and “Carbohydrate digestion and absorption.” Lactic acid has been evidenced to be the main gluconeogenic precursor and the major fuel for mitochondrial respiration (Brooks, 2020). Interestingly, L-lactic acid is also induced by DDGS to downregulate cecal sugar-related pathways, such as “Glycolysis/Gluconeogenesis,” “Pyruvate metabolism,” and “Central carbon metabolism in cancer.” This suggests that the DDGS diet may promote the process of gastrointestinal glycolysis and glycosylation, thus facilitating the digestion, absorption and utilization of carbohydrates in the experimental cattle. Rumen microorganisms have been reported to promote the hydrogenation of oleic and α-linolenic acid to stearic acid, a saturated fatty acid and one of the main components of solid fat (Vargas et al., 2020; Van Rooijen et al., 2021). In our study, oleic and α-linolenic acids, which were negatively correlated with Anaerococcus, were reduced in the rumen of the DDGS group and affected the “Biosynthesis of unsaturated fatty acids” pathway, indicating that Anaerococcus affected by DDGS diet promoted the biohydrogenation of oleic and α-linolenic acids, downregulated the synthesis of unsaturated fatty acids, and promoted fat deposition. Cholic acid, a component of digestive juices, is a key signaling molecule that regulates lipid metabolism and immune function in animals (Zhang H. et al., 2022). Our results showed that cholic acid was upregulated by DDGS diet in the cecal “Bile secretion” and “Primary bile acid biosynthesis” pathways, which indicates that DDGS diet promotes the secretion of cholic acid, thereby facilitating the digestion and absorption of lipids.
Linoleic acid, an essential amino acid, has a positive regulatory effect on the body’s growth and development, and can reduce the risk of cardiovascular and cerebrovascular diseases, and it also has beneficial effects on inflammation and degenerative diseases (Marangoni et al., 2020). 9S,10R-Epoxy-6Z-octadecene and 9,10,13-TriHOME are derivatives of linoleic acid (Hamberg, 1991; Fuchs et al., 2018), and they are reduced in the “Linoleic acid metabolism” pathway in the rumen of the DDGS group, indicating that the DDGS diet inhibits the conversion of linoleic acid, which is potentially beneficial to the growth, development, and physical health of the experimental cattle. Evidence has showed that resveratrol plays a beneficial role in preventing chronic diseases associated with inflammation (Malaguarnera, 2019). Our results showed that resveratrol was upregulated by DDGS diet in the “Longevity regulating pathway,” and may have a positive effect on the prevention of intestinal inflammation. Lysophosphatidylcholine (LysoPC) is a pro-inflammatory lipid and is considered as a key marker in pathological conditions (Ren et al., 2022). Another choline metabolite phosphatidylcholine (PC) can mediates proliferative growth and programmed cell death (Saito et al., 2022). Our study showed that LysoPC (18:0), LysoPC [18:1(11Z)], and PC (17:0/0:0) were downregulated in the DDGS group, and they were involved in “Choline metabolism in cancer” and may have a positive effect on preventing the development of cancer and maintaining physical health.
5. Conclusion
In summary, our results suggest that diets containing DDGS modify the microbial structure and specific metabolic patterns of the gastrointestinal tract in Guanling yellow cattle, demonstrated by increasing the abundance of bacteria which can promote glycolysis and fibers-degradable, and by modulating the metabolic patterns of rumen and cecum, including alteration of the compositions of “Lipids and lipid-like molecules,” “Phenylpropanoids and polyketides,” “Organoheterocyclic compounds,” and “Organic oxygen compounds,” as well as changes in the metabolic pathways of glucose metabolism, lipid metabolism, and organic oxygen compounds metabolism. These changes may improve digestion and absorption of the nutrients such as carbohydrates and lipids, and facilitate fat deposition and weight gain of beef cattle. In addition, some microorganisms and metabolic pathways affected by DDGS diet are beneficial to intestinal immunity, potentially have a beneficial effect on growth, development, and physical health of cattle. In summary, our findings will provide some theoretical basis for further utilization of Moutai liquor DG as a feed resource in livestock production.
Data availability statement
All datasets generated for this study are included in the article/Supplementary material.
Ethics statement
The animal study was approved by the Experimental Animal Ethics Subcommittee of Guizhou University (No. EAE-GZu-2020-E018). The study was conducted in accordance with the local legislation and institutional requirements.
Author contributions
CS, EZ, and ZC conceived the study. CS, TZ, DX, and MZ performed the experiments. CS, EZ, ZC, and CC analyzed experimental results and data. BZ, KW, CC, and SM assisted with the animal experiments. CS wrote the manuscript. All authors read and approved the final manuscript.
Funding
This research was supported by grants from the Guizhou Provincial Support Fund of Science and Technology (No. [2021]5646), the Guizhou Provincial Department of Agriculture project (No. [2022] 163), the Guizhou Provincial Science and Technology Projects (QKHJC-ZK [2022] YB158), the Cultivating Project of Guizhou University (GDPY[2020]85), and the Scientific Research Fund for recruiting the talents of Guizhou University (GDRJHZ [2020]63).
Acknowledgments
We kindly acknowledge the Kweichow Moutai Group for providing the distillers’ grains with solubles, and the Guizhou Cattle Industry Group Co., Ltd. for providing us with Guanling cattle.
Conflict of interest
The authors declare that the research was conducted in the absence of any commercial or financial relationships that could be construed as a potential conflict of interest.
Publisher’s note
All claims expressed in this article are solely those of the authors and do not necessarily represent those of their affiliated organizations, or those of the publisher, the editors and the reviewers. Any product that may be evaluated in this article, or claim that may be made by its manufacturer, is not guaranteed or endorsed by the publisher.
Supplementary material
The Supplementary Material for this article can be found online at: https://www.frontiersin.org/articles/10.3389/fmicb.2023.1171563/full#supplementary-material
References
Brooks, G. A. (2020). Lactate as a fulcrum of metabolism. Redox Biol. 35:101454. doi: 10.1016/j.redox.2020.101454
Brulc, J. M., Antonopoulos, D. A., Miller, M. E., Wilson, M. K., Yannarell, A. C., Dinsdale, E. A., et al. (2009). Gene-centric metagenomics of the fiber-adherent bovine rumen microbiome reveals forage specific glycoside hydrolases. Proc. Natl. Acad. Sci. U.S.A. 106, 1948–1953. doi: 10.1073/pnas.0806191105
Chen, S. Y., Deng, F., Jia, X., Liu, H., Zhang, G. W., and Lai, S. J. (2019). Gut microbiota profiling with differential tolerance against the reduced dietary fibre level in rabbit. Sci. Rep. 9:288. doi: 10.1038/s41598-018-36534-6
Cohain, A. T., Barrington, W. T., Jordan, D. M., Beckmann, N. D., Argmann, C. A., Houten, S. M., et al. (2021). An integrative multiomic network model links lipid metabolism to glucose regulation in coronary artery disease. Nat. Commun. 12:547. doi: 10.1038/s41467-020-20750-8
Cui, Y., Liu, H., Gao, Z., Xu, J., Liu, B., Guo, M., et al. (2022). Whole-plant corn silage improves rumen fermentation and growth performance of beef cattle by altering rumen microbiota. Appl. Microbiol. Biotechnol. 106, 4187–4198. doi: 10.1007/s00253-022-11956-5
Da, R. E. S. P., Vilas, B. E. S. Y., Paulino, P., de Paula, S. D., Possamai, A. J., Da, F. L., et al. (2022). Dried distiller’s grains for feedlot nellore cattle fed non-forage-based diets. Trop. Anim. Health Prod. 54:230. doi: 10.1007/s11250-022-03225-4
Dill-McFarland, K. A., Weimer, P. J., Breaker, J. D., and Suen, G. (2019). Diet influences early microbiota development in dairy calves without long-term impacts on milk production. Appl. Environ. Microbiol. 85:e02141-18. doi: 10.1128/AEM.02141-18
Donohoe, D. R., Wali, A., Brylawski, B. P., and Bultman, S. J. (2012). Microbial regulation of glucose metabolism and cell-cycle progression in mammalian colonocytes. PLoS One 7:e46589. doi: 10.1371/journal.pone.0046589
Fuchs, D., Hamberg, M., Skold, C. M., Wheelock, A. M., and Wheelock, C. E. (2018). An lc-ms/ms workflow to characterize 16 regio- and stereoisomeric trihydroxyoctadecenoic acids. J. Lipid Res. 59, 2025–2033. doi: 10.1194/jlr.D087429
Gill, S. R., Pop, M., Deboy, R. T., Eckburg, P. B., Turnbaugh, P. J., Samuel, B. S., et al. (2006). Metagenomic analysis of the human distal gut microbiome. Science 312, 1355–1359. doi: 10.1126/science.1124234
Hamberg, M. (1991). Regio- and stereochemical analysis of trihydroxyoctadecenoic acids derived from linoleic acid 9- and 13-hydroperoxides. Lipids 26, 407–415. doi: 10.1007/BF02536065
Hao, M., Deng, J., Huang, X., Li, H., Ou, H., Cai, X., et al. (2022). Metabonomic characteristics of myocardial diastolic dysfunction in type 2 diabetic cardiomyopathy patients. Front. Physiol. 13:863347. doi: 10.3389/fphys.2022.863347
Hubbard, K. J., Kononoff, P. J., Gehman, A. M., Kelzer, J. M., Karges, K., and Gibson, M. L. (2009). Short communication: The effect of feeding high protein distillers dried grains on milk production of Holstein cows. J. Dairy Sci. 92, 2911–2914. doi: 10.3168/jds.2008-1955
Jami, E., White, B. A., and Mizrahi, I. (2014). Potential role of the bovine rumen microbiome in modulating milk composition and feed efficiency. PLoS One 9:e85423. doi: 10.1371/journal.pone.0085423
Kim, D. Y., Jung, D. H., Song, E. J., Jang, A. R., Park, J. Y., Ahn, J. H., et al. (2022). D-galactose intake alleviates atopic dermatitis in mice by modulating intestinal microbiota. Front. Nutr. 9:895837. doi: 10.3389/fnut.2022.895837
Koh, A., De Vadder, F., Kovatcheva-Datchary, P., and Backhed, F. (2016). From dietary fiber to host physiology: Short-chain fatty acids as key bacterial metabolites. Cell 165, 1332–1345. doi: 10.1016/j.cell.2016.05.041
Li, H., Duan, Y., Yin, F., Zhu, Q., Hu, C., Wu, L., et al. (2022). Dietary addition of fermented sorghum distiller’s dried grains with soluble improves carcass traits and meat quality in growing-finishing pigs. Trop. Anim. Health Prod. 54:97. doi: 10.1007/s11250-022-03089-8
Li, M. Y., Cheng, Q. M., Li, P., Fan, X. Y., Chen, Y. L., Long, J. H., et al. (2022). Effects of mixed silage of Pennisetum sinese Roxb and moutai Distiller’s grains on its nutrient contents, fermentation quality and microbial diversity. Chin. J. Anim. Nutr. 34, 4071–4080. doi: 10.3969/j.issn.1006-267x.2022.06.066
Li, X., Hui, Y., Leng, B., Ren, J., Song, Y., Che, L., et al. (2021). Millet-based supplement restored gut microbial diversity of acute malnourished pigs. PLoS One 16:e0250423. doi: 10.1371/journal.pone.0250423
Li, Y., Lv, M., Wang, J., Tian, Z., Yu, B., Wang, B., et al. (2021). Dandelion (Taraxacum mongolicum hand.-Mazz.) Supplementation-enhanced rumen fermentation through the interaction between ruminal microbiome and metabolome. Microorganisms 9:83. doi: 10.3390/microorganisms9010083
Liu, S., Li, E., Sun, Z., Fu, D., Duan, G., Jiang, M., et al. (2019). Altered gut microbiota and short chain fatty acids in Chinese children with autism spectrum disorder. Sci. Rep. 9:287. doi: 10.1038/s41598-018-36430-z
Macfarlane, G. T., and Macfarlane, S. (2012). Bacteria, colonic fermentation, and gastrointestinal health. J. AOAC Int. 95, 50–60. doi: 10.5740/jaoacint.sge_macfarlane
Malaguarnera, L. (2019). Influence of resveratrol on the immune response. Nutrients 11:946. doi: 10.3390/nu11050946
Marangoni, F., Agostoni, C., Borghi, C., Catapano, A. L., Cena, H., Ghiselli, A., et al. (2020). Dietary linoleic acid and human health: Focus on cardiovascular and cardiometabolic effects. Atherosclerosis 292, 90–98. doi: 10.1016/j.atherosclerosis.2019.11.018
Muller, E., Algavi, Y. M., and Borenstein, E. (2021). A meta-analysis study of the robustness and universality of gut microbiome-metabolome associations. Microbiome 9:203. doi: 10.1186/s40168-021-01149-z
Murphy, E. C., and Frick, I. M. (2013). Gram-positive anaerobic cocci–commensals and opportunistic pathogens. FEMS Microbiol. Rev. 37, 520–553. doi: 10.1111/1574-6976.12005
Paganelli, A., Righi, V., Tarentini, E., and Magnoni, C. (2022). Current knowledge in skin metabolomics: Updates from literature review. Int. J. Mol. Sci. 23:8776. doi: 10.3390/ijms23158776
Popova, M., McGovern, E., McCabe, M. S., Martin, C., Doreau, M., Arbre, M., et al. (2017). The structural and functional capacity of ruminal and cecal microbiota in growing cattle was unaffected by dietary supplementation of linseed oil and nitrate. Front. Microbiol. 8:937. doi: 10.3389/fmicb.2017.00937
Qiu, M., Hu, J., Peng, H., Li, B., Xu, J., Song, X., et al. (2022). Research note: The gut microbiota varies with dietary fiber levels in broilers. Poult. Sci. 101:101922. doi: 10.1016/j.psj.2022.101922
Ren, J., Lin, J., Yu, L., and Yan, M. (2022). Lysophosphatidylcholine: Potential target for the treatment of chronic pain. Int. J. Mol. Sci. 23:8274. doi: 10.3390/ijms23158274
Rivera-Chávez, F., Zhang, L. F., Faber, F., Lopez, C. A., Byndloss, M. X., Olsan, E. E., et al. (2016). Depletion of butyrate-producing clostridia from the gut microbiota drives an aerobic luminal expansion of Salmonella. Cell Host Microbe 19, 443–454. doi: 10.1016/j.chom.2016.03.004
Saito, R. F., Andrade, L. N. S., Bustos, S. O., and Chammas, R. (2022). Phosphatidylcholine-derived lipid mediators: The crosstalk between cancer cells and immune cells. Front. Immunol. 13:768606. doi: 10.3389/fimmu.2022.768606
Salami, S. A., Valenti, B., Luciano, G., Lanza, M., Umezurike-Amahah, N. M., Kerry, J. P., et al. (2021). Dietary cardoon meal modulates rumen biohydrogenation and bacterial community in lambs. Sci. Rep. 11:16180. doi: 10.1038/s41598-021-95691-3
Spence, C., Wells, W. G., and Smith, C. J. (2006). Characterization of the primary starch utilization operon in the obligate anaerobe bacteroides fragilis: Regulation by carbon source and oxygen. J. Bacteriol. 188, 4663–4672. doi: 10.1128/JB.00125-06
Tang, J., Dai, K., Wang, Q. T., Zheng, S. J., Hong, S. D., Jianxiong, Z. R., et al. (2022). Caproate production from xylose via the fatty acid biosynthesis pathway by genus Caproiciproducens dominated mixed culture fermentation. Bioresour. Technol. 351:126978. doi: 10.1016/j.biortech.2022.126978
Tang, Z., Song, B., Zheng, C., Zheng, J., Yin, Y., and Chen, J. (2021). Dietary beta-hydroxy-beta-methyl butyrate supplementation affects growth, carcass characteristics, meat quality, and serum metabolomics profile in broiler chickens. Front. Physiol. 12:633964. doi: 10.3389/fphys.2021.633964
Tremaroli, V., and Backhed, F. (2012). Functional interactions between the gut microbiota and host metabolism. Nature 489, 242–249. doi: 10.1038/nature11552
Tremlett, H., Zhu, F., Arnold, D., Bar-Or, A., Bernstein, C. N., Bonner, C., et al. (2021). The gut microbiota in pediatric multiple sclerosis and demyelinating syndromes. Ann. Clin. Transl. Neurol. 8, 2252–2269. doi: 10.1002/acn3.51476
Vacca, M., Celano, G., Calabrese, F. M., Portincasa, P., Gobbetti, M., and De Angelis, M. (2020). The controversial role of human gut lachnospiraceae. Microorganisms 8:573. doi: 10.3390/microorganisms8040573
Van Rooijen, M. A., Plat, J., Zock, P. L., Blom, W., and Mensink, R. P. (2021). Effects of two consecutive mixed meals high in palmitic acid or stearic acid on 8-h postprandial lipemia and glycemia in healthy-weight and overweight men and postmenopausal women: A randomized controlled trial. Eur. J. Nutr. 60, 3659–3667. doi: 10.1007/s00394-021-02530-2
Vargas, J. E., Andrés, S., López-Ferreras, L., and López, S. (2020). Effects of supplemental plant oils on rumen bacterial community profile and digesta fatty acid composition in a continuous culture system (RUSITEC). Anaerobe 61:102143. doi: 10.1016/j.anaerobe.2019.102143
Wang, C., Wang, Z. S., Hu, R., Ma, J., Cao, G., Yao, X. H., et al. (2021). Effects of different types of white Distiller’s grains on growth performance, nutrient apparent digestibility, serum biochemical indexes and rumen fermentation parameters of simmental crossbred cattle. Chin. J. Anim. Nutr. 33, 913–922. doi: 10.3969/j.issn.1006-267x.2021.02.032
Wei, X., Tao, J., Xiao, S., Jiang, S., Shang, E., Zhu, Z., et al. (2018). Xiexin tang improves the symptom of type 2 diabetic rats by modulation of the gut microbiota. Sci. Rep. 8:3685. doi: 10.1038/s41598-018-22094-2
Williams, B. A., Zhang, D., Lisle, A. T., Mikkelsen, D., McSweeney, C. S., Kang, S., et al. (2016). Soluble arabinoxylan enhances large intestinal microbial health biomarkers in pigs fed a red meat-containing diet. Nutrition 32, 491–497. doi: 10.1016/j.nut.2015.10.008
Yao, K., Jiang, L., Liu, J., Wang, D., Liu, H., and Ren, D. (2021). Effect of yellow wine lees supplementation on milk antioxidant capacity and hematological parameters in lactating cows under heat stress. Animals (Basel) 11:2643. doi: 10.3390/ani11092643
Zeng, H., Guo, C., Sun, D., Seddik, H., and Mao, S. (2019). The ruminal microbiome and metabolome alterations associated with diet-induced milk fat depression in dairy cows. Metabolites 9:154. doi: 10.3390/metabo9070154
Zhang, H., Guan, W., Li, L., Guo, D., Zhang, X., Guan, J., et al. (2022). Dietary carbon loaded with nano-zno alters the gut microbiota community to mediate bile acid metabolism and potentiate intestinal immune function in fattening beef cattle. BMC Vet. Res. 18:425. doi: 10.1186/s12917-022-03483-2
Zhang, P. G., Zhang, X., Deng, L., and Yin, J. D. (2022). Effects of distillers dried grains with solubles on carcass quality and meat quality of pigs. Chin. J. Anim. Nutr. 34, 2097–2104. doi: 10.3969/j.issn.1006-267x.2022.04.005
Zhang, X., Hou, Z., Tian, X., Wu, D., and Dai, Q. (2022). Multi-omics reveals host metabolism associated with the gut microbiota composition in mice with dietary epsilon-polylysine. Food Funct. 13, 4069–4085. doi: 10.1039/d1fo04227k
Zhang, Y., Choi, S. H., Nogoy, K. M., and Liang, S. (2021). Review: The development of the gastrointestinal tract microbiota and intervention in neonatal ruminants. Animal 15:100316. doi: 10.1016/j.animal.2021.100316
Zhang, Z., Shahzad, K., Shen, S., Dai, R., Lu, Y., Lu, Z., et al. (2021). Altering dietary soluble protein levels with decreasing crude protein may be a potential strategy to improve nitrogen efficiency in hu sheep based on rumen microbiome and metabolomics. Front. Nutr. 8:815358. doi: 10.3389/fnut.2021.815358
Zhu, Y., Cidan-Yangji, Sun, G., Luo, C., Duan, J., Shi, B., et al. (2022). Different feeding patterns affect meat quality of tibetan pigs associated with intestinal microbiota alterations. Front. Microbiol. 13:1076123. doi: 10.3389/fmicb.2022.1076123
Zou, X. Y., Zhang, M., Tu, W. J., Zhang, Q., Jin, M. L., Fang, R. D., et al. (2022). Bacillus subtilis inhibits intestinal inflammation and oxidative stress by regulating gut flora and related metabolites in laying hens. Animal 16:100474. doi: 10.1016/j.animal.2022.100474
Keywords: dried distillers’ grains with solubles, Guanling yellow cattle, rumen, cecum, 16S rDNA gene sequencing, metabolomics
Citation: Song C, Zhang T, Xu D, Zhu M, Mei S, Zhou B, Wang K, Chen C, Zhu E and Cheng Z (2023) Impact of feeding dried distillers’ grains with solubles diet on microbiome and metabolome of ruminal and cecal contents in Guanling yellow cattle. Front. Microbiol. 14:1171563. doi: 10.3389/fmicb.2023.1171563
Received: 15 March 2023; Accepted: 29 August 2023;
Published: 18 September 2023.
Edited by:
Ahmed Elolimy, National Research Centre, EgyptReviewed by:
Linshu Jiang, Beijing University of Agriculture, ChinaMaghsoud Besharati, University of Tabriz, Iran
Copyright © 2023 Song, Zhang, Xu, Zhu, Mei, Zhou, Wang, Chen, Zhu and Cheng. This is an open-access article distributed under the terms of the Creative Commons Attribution License (CC BY). The use, distribution or reproduction in other forums is permitted, provided the original author(s) and the copyright owner(s) are credited and that the original publication in this journal is cited, in accordance with accepted academic practice. No use, distribution or reproduction is permitted which does not comply with these terms.
*Correspondence: Zhentao Cheng, chengzhentao@sohu.com; Erpeng Zhu, zhu13782701756@126.com