- 1Graduate School of Life Sciences, Toyo University, Oura-gun, Gunma, Japan
- 2Faculty of Life Sciences, Toyo University, Oura-gun, Gunma, Japan
- 3Department of Quantum-Applied Biosciences, Takasaki Institute for Advanced Quantum Science, Foundational Quantum Technology Research Directorate, National Institutes for Quantum and Radiological Science and Technology, Takasaki, Gunma, Japan
- 4Bio-Resilience Research Project (BRRP), Toyo University, Oura-gun, Gunma, Japan
- 5Bio Nano Electronics Research Centre, Toyo University, Kawagoe, Saitama, Japan
Cesium (Cs) is an alkali metal with radioactive isotopes such as 137Cs and 134Cs. 137Cs, a product of uranium fission, has garnered attention as a radioactive contaminant. Radioactive contamination remediation using microorganisms has been the focus of numerous studies. We investigated the mechanism underlying Cs+ resistance in Microbacterium sp. TS-1 and other representative microorganisms, including Bacillus subtilis. The addition of Mg2+ effectively improved the Cs+ resistance of these microorganisms. When exposed to high concentrations of Cs+, the ribosomes of Cs+-sensitive mutants of TS-1 collapsed. Growth inhibition of B. subtilis in a high-concentration Cs+ environment was because of a drastic decrease in the intracellular potassium ion concentration and not the destabilization of the ribosomal complex. This is the first study demonstrating that the toxic effect of Cs+ on bacterial cells differs based on the presence of a Cs+ efflux mechanism. These results will aid in utilizing high-concentration Cs+-resistant microorganisms for radioactive contamination remediation in the future.
1. Introduction
After the Fukushima nuclear power plant accident in 2011, a large amount of radioactive cesium (Cs), 134Cs and 137Cs, was released into the environment, resulting in serious impacts on human health and the environment (Hirose, 2016; Nakamura et al., 2022; Wu et al., 2022). Since then, studies on contamination of the soil with radioactive Cs ions (Cs+), decontamination and bioremediation efforts, and the quest to identify Cs+-tolerant microorganisms have been prominent. Imparting radio resistance to Cs+-resistant microorganisms would lead to the creation of highly functional radiation-tolerant bacteria, which could efficiently recover radioactive Cs from radioactive Cs-contaminated environments. Therefore, creating highly functional microorganisms to be used for bioremediation in environments contaminated with radioactive Cs is desirable.
Cs, an alkali metal, generally exists as a monovalent cation in solutions. Radioactive isotopes of Cs are water-soluble and can be taken up by the ion uptake system of living organisms. The isotopes 134Cs and 137Cs have long half-lives (134Cs, 2.0652 years; 137Cs, 30.1 years), are discharged into the external environment during nuclear power generation and emit γ- and β-rays when they decay (Hampton et al., 2004; Sanial et al., 2017). In addition, as Cs+ possess physicochemical properties similar to those of potassium ions (K+), Cs+ is transported into the cells through the K+ transport system (Perkins and Gadd, 1995). Since Escherichia coli does not have a Cs+ efflux system, Cs+ accumulates inside its cell. K+ is excreted from the cell by the K+ efflux system instead of Cs+. As a result, intracellular K+ concentration is significantly reduced (Jung et al., 2001). In addition, K+ contributes to the stabilization of ribosomes, and its deficiency results in growth inhibition (Avery, 1995; Hampton et al., 2004).
Microbacterium sp. TS-1 (TS-1), an alkaliphilic bacterium, was isolated from a jumping spider (Salticidae) in our laboratory (Fujinami et al., 2013). It is a gram-positive aerobic bacterium with an optimum pH range of 8.0–9.0. In addition, it is a high-concentration Cs+-resistant bacterium that can grow in media containing 1,200 mM CsCl (Koretsune et al., 2022). Two significant mechanisms of Cs+ resistance in TS-1 have been reported (Koretsune et al., 2022; Ishida et al., 2023). The first mechanism works through the Cs+/H+ antiporter CshA, which excretes Cs+ from the cell and maintains a low intracellular Cs+ concentration. The Cs+-sensitive mutant strain Mut4 is a CshA defective mutant isolated previously (Koretsune et al., 2022). The second mechanism involves maintaining Cs+ resistance through the uptake of magnesium ions (Mg2+) into cells via the magnesium transporter MgtE, even when the Cs+ concentration increases (Ishida et al., 2023). A mutation in the mgtE gene of TS-1 (mutant strain Mut5) leads to Cs+-sensitivity because of the deficiency of intracellular Mg2+ (Ishida et al., 2023), suggesting that Mg2+ plays an essential role in Cs+ resistance in TS-1. Magnesium is a group 2 element and an essential trace element for organisms (Fiorentini et al., 2021). It plays various roles, such as maintaining the structure of ribosomes and enzymatic activity (Akanuma et al., 2014; Pontes et al., 2016). If the Mg2+ transporter MgtE contributes toward improving Cs+ resistance in TS-1, it would be interesting to investigate whether Mg2+ uptake into cells also leads to the enhancement of Cs+ tolerance in other microorganisms. These strains have multiple Mg2+ transporters but no Cs+/H+ antiporter CshA homologous proteins.
Therefore, to verify whether Mg2+ plays an essential role in Cs+ resistance in microorganisms, we used the gram-positive bacteria Bacillus subtilis BR151MA (wild-type), Staphylococcus aureus IAM12544, gram-negative E. coli W3110, and Pseudomonas aeruginosa NBRC13275 as representative microorganisms. In addition, the eukaryote Saccharomyces cerevisiae JCM1499 was used for Cs+-resistant growth testing with MgCl2 added to the medium.
Functional analysis of TS-1 showed that in an environment with a Cs+ concentration of ≤200 mM, Cs+ resistance was maintained by increasing the Mg2+ concentration in cells. Conversely, in a high Cs+ outer environment of >200 mM, Cs+ resistance was maintained with a low Cs+ concentration in cells through CshA. However, the exact mechanism by which the increase in cell Mg2+ concentration affects Cs+ resistance of TS-1 and the underlying physiological functions of CshA maintaining intracellular Cs+ concentration remain unknown.
Mg2+ stabilizes ribosomal complexes (Hsiao et al., 2009; Nierhaus, 2014; Akanuma et al., 2018). In B. subtilis, intracellular Mg2+ is stored in ribosomes and contributes to the stabilization of complex ribosomal formation (Akanuma et al., 2014, 2018). The intracellular accumulation of Cs+ in E. coli reduces intracellular K+ concentrations and inhibits growth (Bossemeyer et al., 1989; Avery, 1995). Based on these findings, in this study, we considered the intracellular actions of the Cs+ resistance mechanism in TS-1 and hypothesized that in an environment with a Cs+ concentration < 200 mM, ribosomes are destabilized when Cs+ is taken up into the cells. As Mg2+ stabilizes the ribosomal complex, Cs+ resistance is acquired by enhancing the Mg2+ uptake by MgtE. In a Cs+ environment >200 mM, Cs+ resistance is achieved by excreting intracellular Cs+ through CshA to prevent intracellular accumulation of Cs+ and decrease intracellular K+ concentration.
Therefore, we analyzed the ribosomal complex to verify the hypothesis regarding the acquisition of Cs+ resistance by incorporating Mg2+ into TS-1. To investigate the role of Mg2+ in Cs+ resistance, we attempted to measure changes in Cs+, K+, and Mg2+ concentrations in the cells. Through these experiments, we attempted to elucidate the effects of Cs+ concentration on TS-1 and also on B. subtilis, in which the relationship between ribosomes and Mg2+ has been well studied (Akanuma et al., 2014; Nierhaus, 2014; Akanuma et al., 2018; Lee et al., 2019; Akanuma, 2021).
2. Materials and methods
2.1. Bacterial strains, growth media, and conditions for culture
The bacterial strains used in this study are listed in Table 1. B. subtilis BR151MA (Henkin et al., 1991), S. aureus JCM20624, E. coli W3110, P. aeruginosa IFO13275, and S. cerevisiae JCM1499 were used to assess improvements in Cs+ resistance. Luria-Bertani (LB), Miller medium (2 mL; BD Difco™; BD Biosciences, Franklin Lakes, NJ, United States) (pH 7.5 for B. subtilis, pH 7.0 for others) was dispensed into a 14-mL culture tube, inoculated with each single colony, and cultured with shaking at 37°C and 200 rpm for 8 h. This culture solution was used as the pre-culture. A bioshaker BR-43FM (TAITEC Co., Ltd., Koshigaya, Japan) was used for shaking the culture, and the same equipment was used unless otherwise specified.
Various concentrations of MgCl2 (25–200 mM final concentration) were added to 2 mL of LB medium supplemented with different concentrations of CsCl (100–600 mM final concentration). Ten microliters of the pre-culture solution were inoculated and cultured with shaking at 37°C and 200 rpm, and the OD600 was measured after 16 h. Turbidity was measured using a UV-1800 ultraviolet–visible spectrophotometer (Shimadzu Co., Ltd., Kyoto, Japan). For S. cerevisiae, 2 mL of yeast malt (YM) broth (BD Difco™; BD Biosciences) was dispensed into a 14-mL capacity culture tube, inoculated using a single colony, and cultured with shaking at 25°C and 200 rpm for 24 h. This was used as the pre-culture. Similar to eubacteria, CsCl and MgCl2 were added to the YM broth; approximately 10 μL of the pre-culture was inoculated and cultured with shaking at 25°C and 200 rpm for 24 h, and turbidity was measured at OD600.
Alkaliphilic Microbacterium sp. TS-1 was grown at 30°C in neutral complex (NC medium) and Tris media (Imazawa et al., 2016). Tris medium consisted of 3.63 g L−1 Tris base, 1.47 g L−1 citric acid monohydrate, 0.5 g L−1 yeast extract, 9 g L−1 glucose, and 1% (w/v) trace elements (Cohen-Bazire et al., 1957). Deionized (DI) water was used as the solvent. The pH was adjusted to 8 and 9 using 1 M N-methyl-D-glucamine. The pH was adjusted to 7 using 5 N sulfuric acid (H2SO4). Tris medium was used for the monovalent cation resistance test to avoid underestimation of cation influx. The NC medium consisted of 15.5 g L−1 K2HPO4, 4.5 g L−1 KH2PO4, 0.05 g L−1 MgSO4•7H2O, 0.34 g L−1 citric acid, 5 g L−1 peptone, 2 g L−1 yeast extract, 5 g L−1 glucose, and 11.7 g L−1 NaCl. Deionized water was used as a solvent. The final pH was adjusted to the desired value using KOH or H2SO4 (Fujinami et al., 2011).
2.2. Measurement of intracellular Cs+, K+, and Mg2+ concentration
Intracellular Cs+, K+, and Mg2+ concentrations were measured in TS-1 wild-type, Cs+-sensitive mutants (Mut4 and Mut5), and B. subtilis. As TS-1 Cs+-sensitive mutants and B. subtilis did not grow under high Cs+ concentration conditions, CsCl was added after culturing. Intracellular ion concentrations were measured 1 h after the addition of CsCl.
Strain TS-1 was inoculated from a single colony by dispensing 2 mL of neutral complex medium (pH 8.0) into a 14-mL culture tube and cultured at 30°C and 200 rpm for 18 h. The culture solution (100 μL) was inoculated into a medieval composite agar medium and pre-incubated at 37°C for 18 h. The cultured neutral complex agar medium was inoculated into 200 mL of 30 mM Tris medium (pH 8.0) such that the turbidity at OD600 was 0.1 and cultured at 37°C and 150 rpm. When the turbidity at OD600 reached 0.4, 20-mL portions were dispensed, various concentrations of CsCl (0, 100, 200, 300, and 400 mM) were added, and the cells were further cultured for 1 h. The culture solution was centrifuged at 9,100 × g at 20°C for 3 min to collect the cells. Unless otherwise specified, the TOMY Seiko MX-305 centrifuge (TOMY SEIKO Co., Ltd., Tokyo, Japan) was used. The supernatant was removed, and the cell pellet was suspended in 5 mL of 300 mM sucrose solution. The suspension was centrifuged at 9,000 × g for 3 min at 20°C, and the cell pellet was resuspended in 5 mL of 300 mM sucrose solution. The protein concentration was determined via the Lowry method using a 100-μL aliquot of the suspension. One milligram of protein was used in a volume of 3 μL of bacterial cells. The suspension was then centrifuged at 9,000 × g for 3 min at 20°C. The cell pellet was suspended in 5 mL of 5% trichloroacetic acid (TCA) and incubated at 100°C for 10 min. After that, the mixture was centrifuged at 4°C and 9,000 × g for 5 min, and the supernatant was used for intracellular ion concentration measurement. The Cs+ concentration was measured by taking 1 mL of the intracellular ion concentration measurement sample using an atomic absorption photometer (iCE3400; Thermo Fisher Scientific, Waltham, MA, United States). A 1-mL aliquot was taken from the measurement sample, and the K+ concentration was measured using an ANA-135 flame photometer (Tokyo Koden Co., Ltd., Tokyo, Japan). Mg2+ concentration was measured using a metalloassay magnesium assay LS kit (Metallogenics Co., Ltd., Chiba, Japan) according to the manufacturer’s instructions. First, 4.5 μL of 5 M NaOH was added to 100 μL of the measurement sample to adjust the pH from 2 to 7.
Subsequently, 3 μL of the pH-adjusted measurement sample was added to 250 mL of the coloring solution, vortexed, and allowed to stand at 20°C for 5 min. Absorbance at OD660 was then measured. The Mg2+ concentration of the samples was calculated from the obtained value using the following formula:
2.3. Data analysis
Statistical analysis was performed with BellCurve for Excel (Social Survey Research Information Co., Ltd., Tokyo, Japan). Tukey test data for post hoc analysis of the results in Figures 1, 2 are presented in Supplementary Tables S1, S2, respectively. In Figures 1A–C, multiple comparison analysis was performed between strains after confirming a significant difference by two-way analysis of variance.
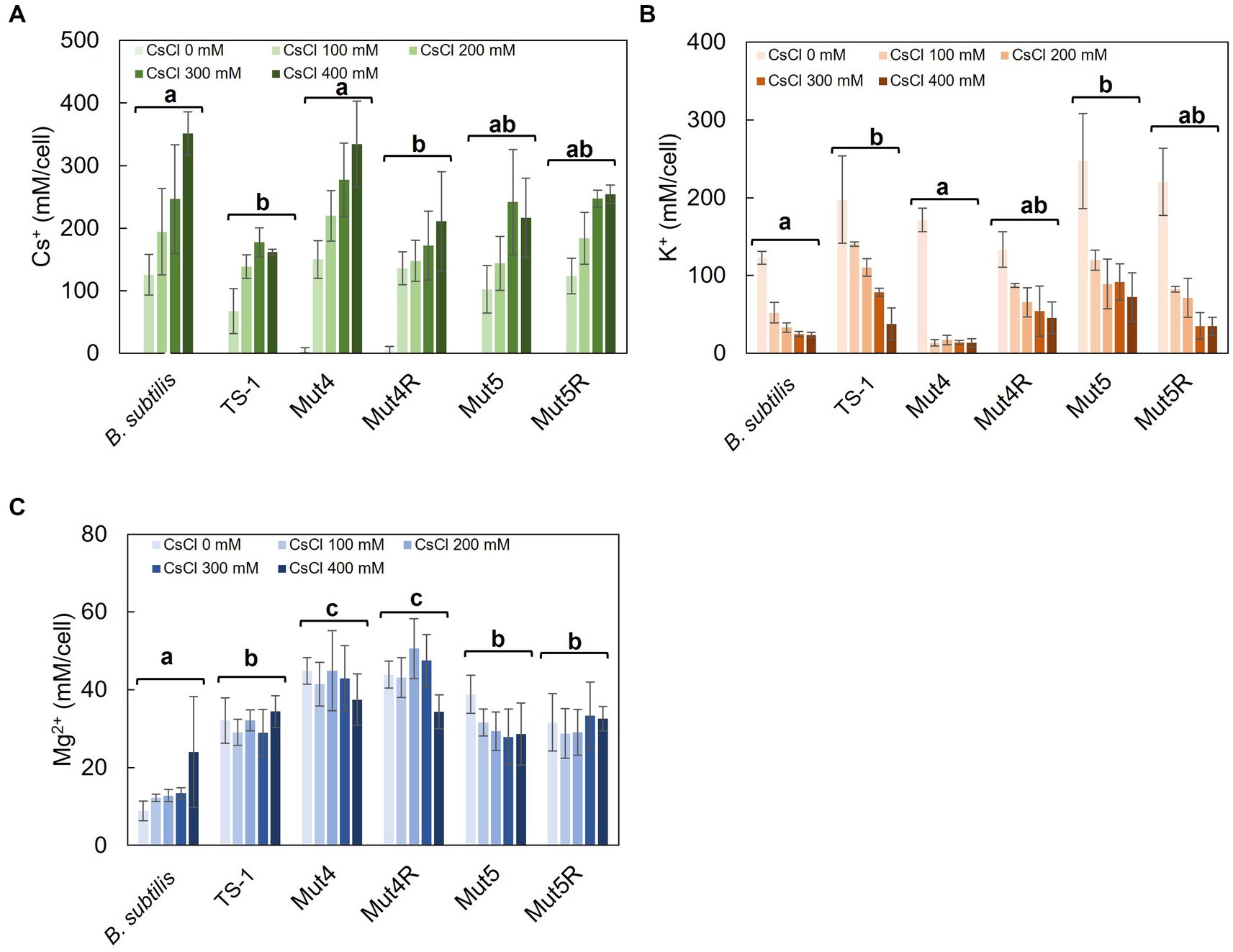
Figure 1. Intracellular Cs+, K+, and Mg2+ concentrations after CsCl treatment in strain TS-1, its mutants, and B. subtilis. Strain TS-1 and its derivative mutants were cultured in Tris medium (pH 8.0) and B. subtilis in LB medium (pH 7.5) until turbidity reached 0.4. Subsequently, the cells were treated with CsCl for 1 h, collected, and treated with 5% TCA, and the intracellular Cs+ concentration (A), intracellular K+ concentration (B), and intracellular Mg2+ concentration (C) were measured. The vertical axis indicates the intracellular concentration (mM/cell), and the series shows the results when each strain was treated with CsCl (0, 100, 200, 300, and 400 mM). Error bars indicate the standard deviation of at least three independent experiments. Different lowercase letters indicate significant differences. In panels A–C, multiple comparison analysis was performed between strains after confirming a significant difference by two-way analysis of variance. Different superscript letters (a, b, c) denote significant differences from each other in all combinations.
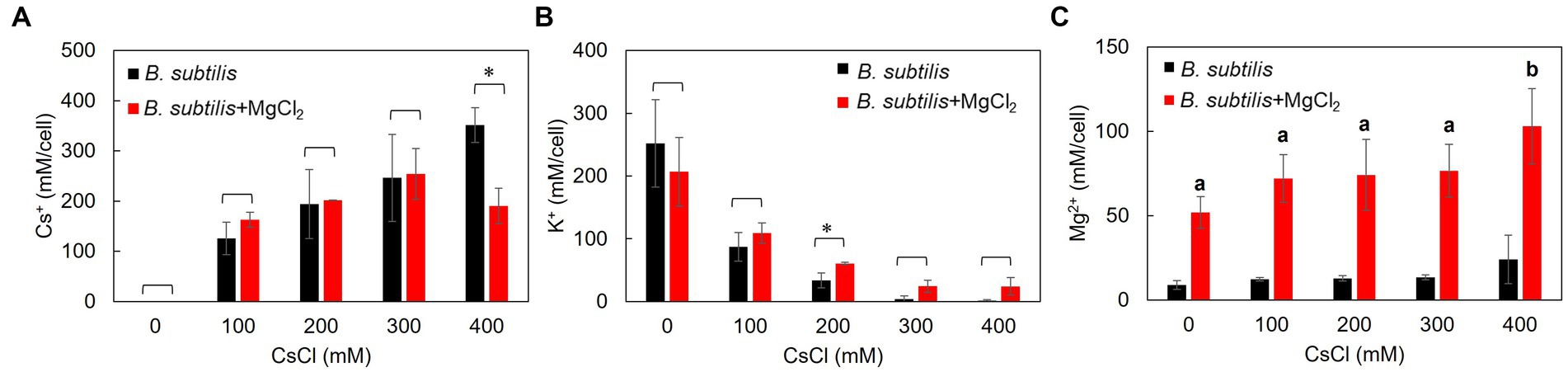
Figure 2. Intracellular Cs+, K+, and Mg2+ concentrations of B. subtilis with the addition of CsCl and MgCl2. After culturing in LB medium (pH 7.5) to a turbidity of 0.4, the cells were treated with CsCl and MgCl2 for 1 h, collected, and suspended in 5% TCA, and the Cs+ (A), K+ (B), and Mg2+ (C) concentrations were measured. The vertical axis indicates the intracellular Cs+ concentration (mM/cell), and the series shows the results when each strain was treated with CsCl (0, 100, 200, 300, and 400 mM). Error bars indicate the standard deviation of three independent experiments. Asterisk (*) indicates p < 0.05, indicating a significant difference. Different superscript letters (a, b) denote significant differences from each other in all combinations.
2.4. Ribosome preparation
Ribosome preparation and fractionation were based on a previously reported method (Takada et al., 2021). Wild-type strain TS-1 and its Cs+-sensitive mutants Mut4 and Mut5 were streaked on a pH 8.5 neutral composite agar medium and used as pre-culture (37°C, 18 h). Using an inoculation loop, cells grown on the plate were collected and inoculated (37°C, 200 rpm) in 1,000 mL of Tris medium (pH 8.0). This was used as the culture medium. Cultivation was started at an initial turbidity (OD600 = 0.1), and after 4 h of culture initiation, 25 and 50 mL of 8 M CsCl were added. To collect the bacteria, centrifugation (5,000 × g, 4°C, 10 min) was performed using an NA-600C rotor (TOMY SEIKO Co. Ltd.) The cells were resuspended in 10 mL Buffer I and subjected to a French press operation twice at 8,000 psi to disrupt the cells. Buffer I (pH 7.6) consisted of 2.42 g L−1 tris base, 2.15 g L−1 magnesium acetate tetrahydrate, 7.7 g L−1 ammonium acetate, 0.015 g L−1 ammonium acetate, and 8 mL of 0.1 M phenylmethylsulfonyl fluoride (PMSF). The pH was adjusted to 7.6 with 6 N HCl. Undisrupted cells were removed via centrifugation (12,000 × g, 4°C, 15 min), and crude cell debris containing ribosomes in the supernatant was collected. The absorbance of the cell debris at 260 nm was measured using a spectrophotometer and stored at 4°C until ultracentrifugation. Cell lysates were subjected to ultracentrifugation within 3 days of preparation. The same experiment was performed twice to confirm reproducibility.
For B. subtilis, 2 mL of LB medium (pH 7.5) was dispensed into a 14-mL culture tube, inoculated using a single colony, and cultured at 37°C and 200 rpm for 8 h. The culture solution (100 μL) was inoculated onto LB agar medium (pH 7.5) and pre-cultured at 37°C for 16 h. The cultured LB agar medium was inoculated into 1 L of LB medium (pH 7.5) such that the turbidity at OD600 was 0.03 and cultured at 37°C and 150 rpm. After 2.5 h of culture initiation, CsCl (0, 200, and 400 mM) and 50 mM MgCl2 were added, and the mixture was further cultured for 1 h. A crude cell extract was obtained from the culture solution in the same manner as described for strain TS-1.
2.5. Ribosome analysis
For strain TS-1 and its Cs+-sensitive mutants Mut4 and Mut5, an Ultra-Clear™ centrifuge tube (14 mL; Beckman Coulter, Brea, CA, United States) was used for sucrose density gradient ultracentrifugation with 4 mL of 10% sucrose in Buffer I. Thereafter, 4 mL of 35% sucrose in Buffer I was added to the bottom of the tube using a syringe. The upper lid of the tube was covered with parafilm, and the tube was allowed to stand tilted to form a sucrose density gradient at 20°C for 2 h, after which the tube was left at 4°C for 1 h. Crude ribosomal cell extract prepared as described above was layered on top of the sucrose density gradient. An OptimaTML-80XP Ultracentrifuge (Beckman Coulter) was used for ultracentrifugation (222,000 × g, 4°C, 3 h) using an SW40Ti rotor (6 × 14 mL; Beckman Coulter). Fractions of 200 μL each were taken from the upper layer of the tube (45 fractions), and the A260 of each fraction was measured using a Thermo Nano Drop200C (Thermo Fisher Scientific K.K., Tokyo, Japan). The sucrose concentration was measured using an Atago handheld refractometer (MASTER-PM, ATAGO Co., Ltd., Tokyo, Japan). After the measurements, a separation profile diagram was constructed.
For B. subtilis, 4.5 mL of 10% sucrose in Buffer I was poured into a 14-mL Ultra-Clear™ centrifuge tube, after which the 4.5 mL of 35% sucrose in Buffer I was added to the bottom of the tube using a syringe. Subsequent experiments were performed as described above for the TS-1 to create a ribosome profile.
3. Results
3.1. Effect of treatment with or without Mg2+ addition on the growth of the representative Cs+-resistant microorganisms
Using B. subtilis, S. aureus, E. coli, P. aeruginosa, and S. cerevisiae as representative microorganisms, the effect of Mg2+ addition on Cs+ resistance was investigated. Cs+ resistance improved upon the addition of Mg2+ to the culture media for all microorganisms, although there was a difference in the degree of Cs+ resistance observed between them. B. subtilis showed only a slight increase in Cs+ tolerance with the addition of Mg2+ when the pH of LB medium was 7.0 (data not shown). Therefore, the experiment was performed at pH 7.5, where a more pronounced effect was observed. In B. subtilis, Cs+ resistance improved to 300 mM (Figure 3A) when 50 mM MgCl2 was added to the medium compared with 100 mM without Mg2+. Regarding the Cs+ resistance of S. aureus, growth inhibition was observed at 300 mM CsCl without Mg2+. However, growth was observed even with 600 mM CsCl when 100 mM MgCl2 was added (Figure 3B). E. coli cultures were resistant to 400 mM CsCl when 50 mM MgCl2 was added compared with 300 mM without Mg2+ (Figure 3C). P. aeruginosa grew in the presence of 200 mM CsCl when 125 mM MgCl2 was added, compared with only 100 mM CsCl without Mg2+ (Figure 3D). S. cerevisiae grew in the presence of 200 mM CsCl when 150 mM MgCl2 was added, compared to its growth in the absence of Mg2+ (Figure 3E).
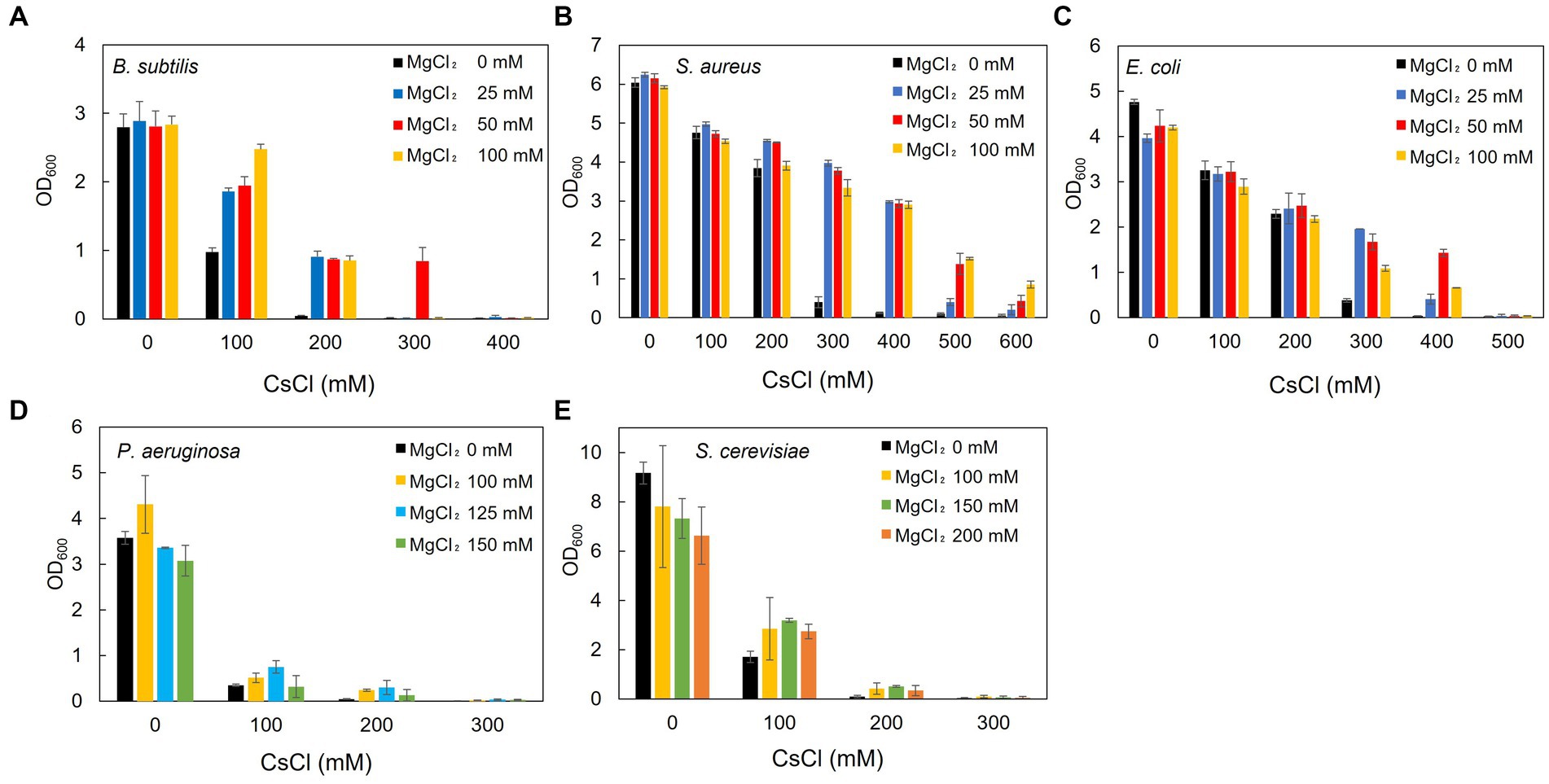
Figure 3. Effect of Mg2+ addition on the growth of the representative Cs+-resistant microorganisms. B. subtilis BR151MA (A), S. aureus IAM12544 (B), E. coli W3110 (C), and P. aeruginosa IFO13275 (D) were cultured in LB medium for 16 h, and turbidity at OD600 was measured. B. subtilis was cultured in an LB medium at pH 7.5 and the others at pH 7.0. S. cerevisiae JCM1499 (E) was cultured in YM broth for 24 h, and turbidity at OD600 was measured. The vertical axis indicates turbidity, and the horizontal axis indicates the CsCl concentration of the medium. Error bars indicate the standard deviation of three independent experiments.
3.2. Comparison of changes in intracellular Cs+, K+, and Mg2+ concentrations in strain TS-1, its Cs+-sensitive mutants, its revertants, and B. subtilis with and without CsCl treatment
Intracellular Cs+ concentrations were measured in TS-1 wild-type, Cs+-sensitive mutants (Mut4 and Mut5), revertants (Mut4R and Mut5R), and B. subtilis with and without CsCl treatment (Figure 1A). In B. subtilis, intracellular Cs+ concentration increased with increasing CsCl concentration. Conversely, the intracellular Cs+ concentration was maintained at <200 mM in the TS-1 wild-type. In Mut4, the intracellular Cs+ concentration increased with increasing CsCl concentration, similar to the trend seen in B. subtilis. Mut4R had a lower Cs+ concentration than that of Mut4, and this was comparable to the wild-type. Strains Mut5 and Mut5R exhibited intracellular Cs+ concentrations intermediate to those of TS-1 wild-type and B. subtilis.
Intracellular K+ concentrations were measured in TS-1 wild-type, Cs+-sensitive mutants, revertants, and B. subtilis with and without Cs+ treatment (Figure 1B). The addition of CsCl to B. subtilis significantly decreased intracellular K+ concentration. The TS-1 wild-type showed a more gradual decrease in intracellular K+ concentration than that of B. subtilis following CsCl addition, showing a significant difference (p < 0.05). Similar to B. subtilis, Mut4 showed a drastic reduction in the intracellular K+ concentration when CsCl was added. Mut4R, Mut5, and Mut5R exhibited approximately similar behaviors as the wild-type strain.
Intracellular Mg2+ concentrations were measured in TS-1 wild-type, Cs+-sensitive mutants, revertants, and B. subtilis with and without Cs+ treatment (Figure 1C). There was no significant difference in intracellular Mg2+ concentrations between TS-1 wild-type, Mut5, and Mut5R. In contrast, Mut4 and Mut4R showed high intracellular Mg2+ concentrations.
Tukey test raw data for post hoc analysis of the results in Figure 1 are presented in Supplementary Table S1.
3.3. Comparison of Cs+ resistance in B. subtilis with Mg2+ addition and changes in intracellular Cs+, K+, and Mg2+ concentrations
Intracellular Cs+ concentrations in B. subtilis, representing common microorganisms, were measured with and without CsCl and MgCl2 treatments (Figure 2A). At CsCl concentrations of 0–300 mM, adding 50 mM MgCl2 did not change the intracellular Cs+ concentration, but at 400 mM CsCl, intracellular Cs+ concentration decreased by approximately 50%.
Similarly, the intracellular K+ concentration in B. subtilis was also measured (Figure 2B). There was no significant difference in the intracellular K+ concentrations with the addition of 50 mM MgCl2 at CsCl concentrations of 0, 100, 300, and 400 mM. However, intracellular K+ concentrations significantly increased at a CsCl concentration of 200 mM.
Likewise, intracellular Mg2+ concentrations were also measured in B. subtilis (Figure 2C). Without CsCl treatment, adding 50 mM MgCl2 increased the intracellular Mg2+ concentration by approximately 40 mM. As the amount of CsCl increased, the intracellular Mg2+ concentration increased slightly, and a significant difference (p < 0.05) was observed at a CsCl concentration of 400 mM.
Tukey test raw data for post hoc analysis of the results in Figure 2 are presented in Supplementary Table S2.
3.4. Effects of CsCl treatment on the growth of TS-1 and B. subtilis
TS-1 wild-type, Mut4, and Mut5 were cultured, treated with CsCl after 4 h of culturing, and harvested after 1 h to prepare ribosomes. The doubling times of TS-1 wild-type, Mut4, and Mut5 up to 4 h of culture were approximately 2.6, 2.5, and 2.5 h, respectively. The growth of the TS-1 wild-type continued regardless of the presence or absence of CsCl treatment (Figure 4A). In contrast, growth inhibition of both Mut4 and Mut5 was observed after the addition of CsCl (Figures 4B,C). Next, B. subtilis was cultured, CsCl treatment was performed after 2.5 h of culture, and the cells were harvested after 1 h to prepare ribosomes. The doubling time of B. subtilis up to 2.5 h before culture was approximately 37 min, and growth inhibition was observed after the addition of CsCl (Figure 4D).
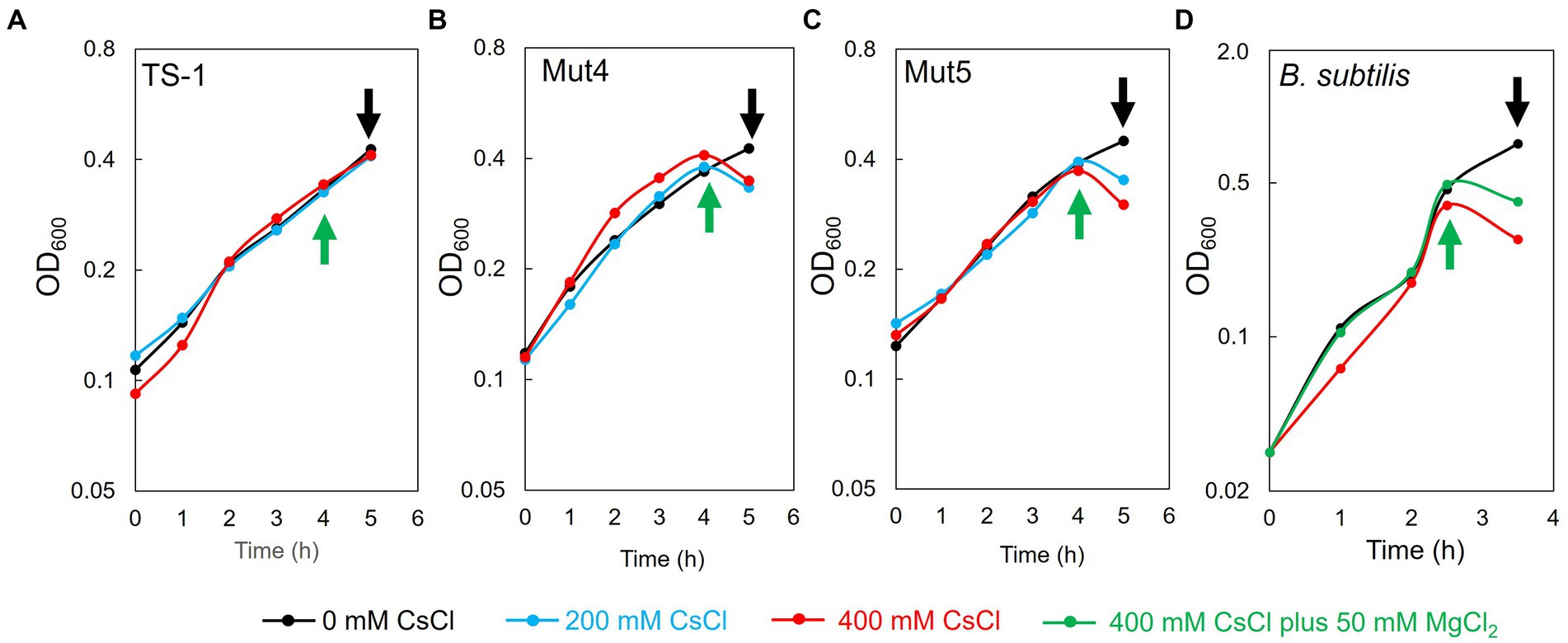
Figure 4. Effects of CsCl treatment on the growth of TS-1 and B. subtilis. The horizontal axis indicates culture time (h), and the vertical axis indicates turbidity (OD600). For TS-1 (A), Mut4 (B), Mut5 (C), and B. subtilis (D), CsCl was added at the time points indicated by the green upward arrow and collected at the time points indicated by the black downward arrow.
3.5. Effects of CsCl treatment in TS-1 on ribosomal complexes
To investigate the effect of Cs+ treatment on ribosome complex formation in the TS-1 wild-type and its mutants, crude cell extracts were prepared from cultured cells, and the ribosome complexes were separated using sucrose density gradient ultracentrifugation (Figure 5A). In the TS-1 wild-type, no effect was observed on the formation of ribosomal complexes, regardless of the presence or absence of CsCl treatment. Next, the ribosome complexes were analyzed for the Cs+-sensitive mutants, Mut4 and Mut5, in the same manner as the wild-type (Figures 5B,C). Both mutants were treated with CsCl at 200 and 400 mM, upon which the 70S ribosomes collapsed, and a finer peak than that of 30S ribosomes was observed.
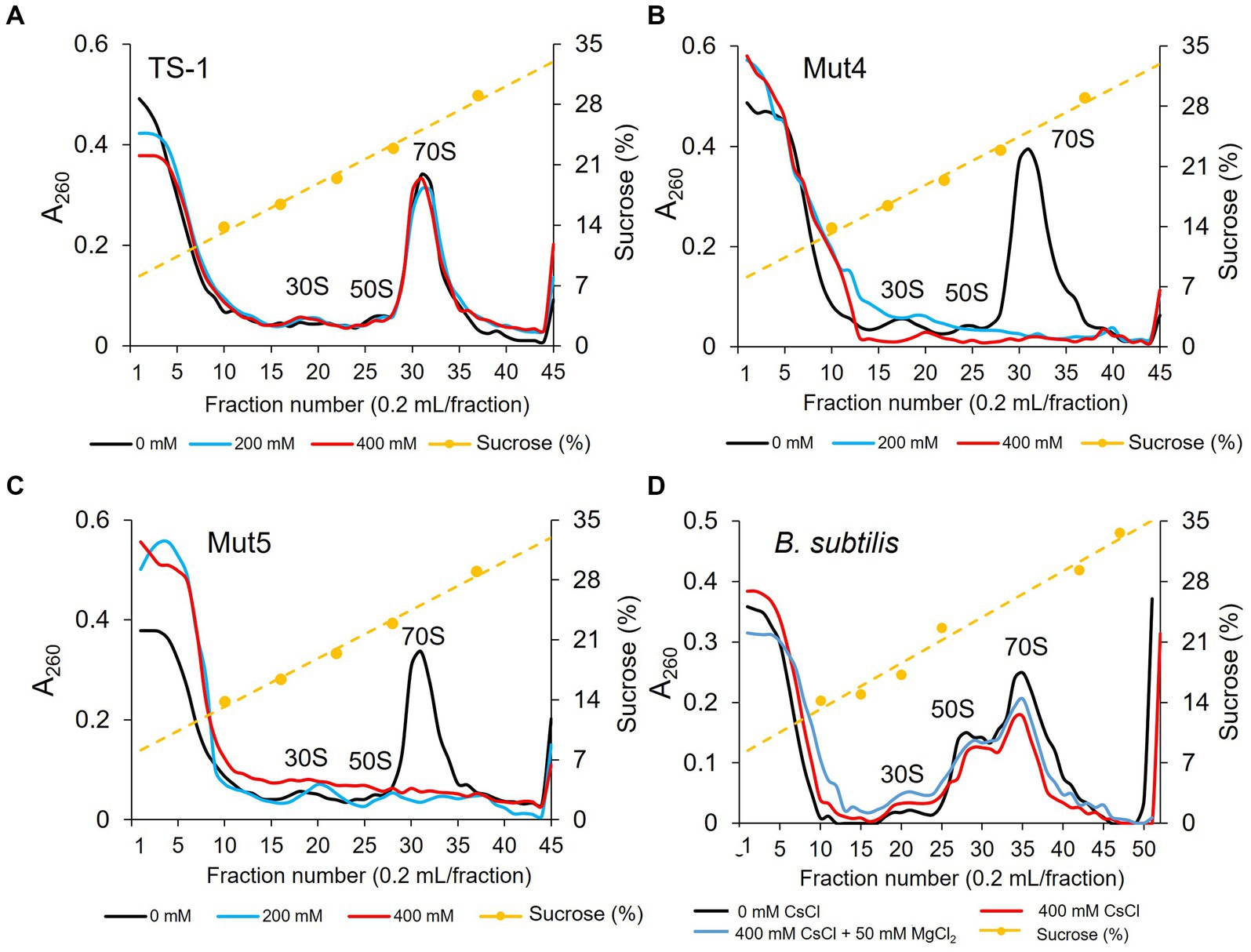
Figure 5. Ribosomal complex profiles with and without CsCl treatment in strain TS-1, its mutants, and B. subtilis. The effects of CsCl treatment on complex ribosome formation in strain TS-1 (A), its mutant strains Mut4 (B) and Mut5 (C), and B. subtilis (D) were investigated. Each crude cell extract was obtained and analyzed for ribosomal complexes using sucrose density gradient ultracentrifugation. The first vertical axis indicates the absorbance at A260, the second vertical axis shows the sucrose concentration, and the horizontal axis indicates the fraction number. Two independent experiments were performed to confirm reproducibility.
3.6. Effects of CsCl and MgCl2 treatment in B. subtilis on ribosomal complexes
The effect of CsCl treatment on ribosome complex formation was investigated in B. subtilis. We also investigated whether ribosome complex formation was affected by the addition of MgCl2. Ribosome complexes were analyzed using sucrose density gradient ultracentrifugation in the same manner as that described for the TS-1 strain (Figure 5D). Even when B. subtilis was treated with CsCl, 70S ribosomes tended to decrease slightly; however, ribosomes were not significantly affected. In addition, no effect on the ribosome complex was observed when MgCl2 was added to the culture medium.
4. Discussion
4.1. Effect of treatment with and without Mg2+ addition on the growth of representative microorganisms showing Cs+ resistance
Examining the effect of Mg2+ addition on Cs+ resistance in B. subtilis, P. aeruginosa, E. coli, S. aureus, and S. cerevisiae as representative microorganisms showed that Cs+ resistance was improved by adding Mg2+ to the medium for all the five strains tested in this study. Furthermore, it was effective not only for TS-1 but also for microorganisms in general.
Mg2+ plays various essential roles in many organisms, including maintaining ribosome structure, genome stabilization, and enzyme activation (Akanuma et al., 2018). It has been reported that increasing the intracellular Mg2+ concentration stabilizes ribosomes in B. subtilis, in which the structure of the ribosome is destabilized due to the deletion of a part of the ribosomal protein (Akanuma et al., 2018). Therefore, we hypothesized that Mg2+ enhanced Cs+ tolerance. Cs+ uptake by cells affected ribosome complex formation. As Mg2+ stabilized the structure of ribosomes, the upper limit of Cs+ concentrations tolerated by the strain was considered to be improved.
Differences were found in the improvement in Cs+ tolerance and the amount of Mg2+ required for the outcome obtained by adding Mg2+ to each microbial species culture. It was speculated that the increase in intracellular Mg2+ could counteract the drastic decrease in intracellular K+ concentration to some extent or that the stability of ribosomes might differ among microorganisms or both.
4.2. Comparison of changes in intracellular Cs+, K+, and Mg2+ concentrations in strain TS-1, its Cs+-sensitive mutants, its revertants, and B. subtilis with and without CsCl treatment
Changes in intracellular Cs+, K+, and Mg2+ concentrations in strains TS-1 and B. subtilis with and without CsCl treatment were measured.
In B. subtilis, intracellular Cs+ concentration increased with increasing CsCl concentration in the medium, and the K+ concentration decreased sharply. From the Cs+-tolerant growth test results, the intracellular K+ concentration decreased to approximately 13% of that in the absence of Cs+ at 200 mM CsCl, at which B. subtilis could not grow. It was speculated that the extreme decrease in intracellular K+ concentration in B. subtilis impeded the maintenance of vital functions. This was consistent with results reported for E. coli (Avery, 1995). B. subtilis and E. coli do not harbor a Cs+ efflux mechanism. Thus, it was inferred that a decrease in intracellular K+ concentration was the leading cause of growth inhibition in these bacteria. CsCl treatment slightly increased intracellular Mg2+ but did not make a significant difference. Contrastingly, in strain TS-1, the intracellular Cs+ concentration remained lower than that in B. subtilis even when the CsCl concentration increased, and the concentration was maintained at approximately <200 mM. Intracellular K+ concentration was moderately decreased in TS-1 following Cs+ treatment compared to that in B. subtilis, and intracellular K+ concentration was high compared to that in B. subtilis. This may be because TS-1 harbors a Cs+ efflux system, CshA (Koretsune et al., 2022). The apparent Km value of CshA at pH 8.0 was approximately 250 mM, indicating that TS-1 prevented the intracellular Cs+ concentration from increasing and K+ deficiency.
Analysis of the changes in intracellular Cs+, K+, and Mg2+ concentrations with and without Cs+ treatment in the TS-1 Cs+-sensitive mutants and its revertants revealed that CsCl treatment of Mut4 resulted in an influx of Cs+ into cells, and the K+ concentration was significantly reduced. This was similar to the results obtained for B. subtilis, which does not possess a Cs+ efflux system. This suggested that Cs+ excretion by CshA was essential for Cs+ resistance in TS-1 cells. In addition, the intracellular Mg2+ concentration in Mut4 was maintained at higher levels than those in the wild-type strain. This suggested that Mg2+ was incorporated into cells to resist the toxicity caused by the influx of a large amount of Cs+ into the cells. Since CshA in the Cs+ efflux system is active in Mut5, which is a MgtE mutant, Cs+ and K+ concentrations were similar to those of the wild-type even after Cs+ treatment. No significant difference was observed in the intracellular Mg2+ concentration. This suggested the possibility that another Mg2+ transporter performed the uptake of Mg2+ into the cell and functioned when Cs+ entered the cells of Mut5.
Mg2+ has been shown to improve Cs+ resistance in several microorganisms. We investigated changes in intracellular Cs+, K+, and Mg2+ concentrations when MgCl2 was added simultaneously with CsCl in B. subtilis. The addition of 50 mM of MgCl2 decreased the intracellular Cs+ concentration at a CsCl concentration of 400 mM. The E. coli Cs+ uptake system, Kup, becomes less active when the cell is filled with K+ (Dosch et al., 1991). Although the details of the Cs+ uptake system of B. subtilis have not been elucidated, the K+ uptake system is expected to take up Cs+. A significant increase in the intracellular K+ concentration was observed when Mg2+ was added at a CsCl concentration of 200 mM (p = 0.03). Although there was no significant difference in this experiment, the intracellular K+ concentration increased at CsCl concentrations of 300 and 400 mM at a level close to the CsCl concentration of 200 mM (300 mM: p = 0.06, 400 mM: p = 0.08). This was presumed to be because the addition of high concentrations of CsCl and MgCl2 enhanced the uptake of K+ to adapt to the high osmotic pressure environment.
4.3. Effects of CsCl treatment of TS-1, its Cs+-sensitive mutants, and B. subtilis on ribosomal complexes
To elucidate the mechanism of Cs+ resistance through Mg2+ uptake in TS-1, we investigated whether the presence or absence of CsCl treatment in TS-1, its Cs+-sensitive mutants, and B. subtilis affected the formation of ribosomal complexes. In addition, we investigated whether the ribosomal complex was affected when Mg2+ was added to B. subtilis cultures to improve Cs+ tolerance. We observed that Cs+ treatment did not affect the ribosomal complex in the TS-1 wild-type. However, in the Cs+-sensitive strains Mut4 and Mut5, the 70S ribosomes collapsed following CsCl treatment. However, CsCl treatment did not affect the ribosomal complex of B. subtilis.
Mut4 is a CshA mutant, and a large amount of Cs+ accumulated in the cells based on the measurement results of intracellular Cs+ concentration. Therefore, it is suggested that high concentrations of intracellular Cs+ destabilize ribosomes. Strain Mut5 is an MgtE mutant, and although it has no issue with Mg2+ uptake, it is presumed that Mg2+ uptake cannot be enhanced when Mg2+ is required. Therefore, it was speculated that ribosomes destabilized by Cs+ could be stabilized owing to the lack of Mg2+. High concentrations of K+ inhibit ribosome complex formation by competing with Mg2+ (Nierhaus, 2014; Senbayram et al., 2015). Because Cs+ has physicochemical properties similar to those of K+, it was speculated that Cs+ destabilized ribosomes by competing with Mg2+.
TS-1 wild-type suppressed intracellular Cs+ to ≤200 mM in a high-concentration Cs+ environment by excreting intracellular Cs+ using CshA. In a Cs+ environment of ≤200 mM, MgtE is considered to enhance Mg2+ uptake and stabilize the ribosomal complex, thereby adapting to the Cs+ environment.
Bacillus subtilis did not exhibit Cs+ resistance, and when treated with CsCl, Cs+ accumulated in the cells, similar to Mut4. Thus, the ribosome complex is expected to be similarly destroyed by CsCl treatment. However, CsCl treatment did not have a significant effect on ribosomal complexes. The impact of improving Cs+ tolerance by adding Mg2+ differed depending on the microbial species. Hence, the stability of ribosomes to Cs+ was expected to vary depending on the microbial species. Certain ribosomal proteins function to replace Mg2+ for ribosomal stabilization (Hsiao et al., 2009; Akanuma et al., 2014). Although Cs+ competes with Mg2+ to destabilize ribosomes, it does not affect ribosome stabilization by ribosomal proteins, suggesting that B. subtilis ribosomes are not affected by Cs+. However, it has been suggested that the influx of Cs+ into the cells of B. subtilis leads to an extreme decrease in K+ and that a shortage of K+ interferes with life support.
5. Conclusion
In the present study, it was found that Cs+ treatment of B. subtilis increased the intracellular Cs+ concentration, similar to the trend observed in Mut4; therefore, it was presumed that the ribosomal complex would also be disrupted, but no significant effect was observed. This suggests that the degree of cell dependence on Mg2+ in the context of the strength of the ribosomal complex differs depending on the microbial species.
In TS-1, when the intracellular Cs+ concentration is ≤200 mM, the Cs+ that flows into the cell competes with Mg2+ and destabilizes the ribosome. Therefore, we inferred that the stability of the ribosomal complex was enhanced by maintaining a high intracellular Mg2+ concentration.
Prospects for these results include the application of the Cs+/H+ antiporter CshA in bioremediation using Mg2+ as an additive. Because CshA is a low-affinity antiporter (apparent Km value of 250 mM for Cs+), it is not suitable for recovering low concentrations of Cs+. Therefore, its affinity should be further improved. We plan to elevate the affinity of CshA for Cs+ by molecular evolution engineering, such as error-prone PCR. In addition, when using microorganisms that accumulate Cs+ but have low Cs+ resistance for bioremediation, using Mg2+ as an additive is expected to enhance the Cs+ resistance of microorganisms and increase the efficiency of their recovery.
Data availability statement
The original contributions presented in the study are included in the article/Supplementary material, further inquiries can be directed to the corresponding author.
Author contributions
MI designed the research and wrote the manuscript. YI, CZ, KS, and MI conducted the research. YI, CZ, and MI analyzed the data. All authors contributed to the article and approved the submitted version.
Funding
This work was supported by a grant for the Toyo University Top Priority Research Promotion Program and the Toyo University intellectual property practical application promotion program.
Acknowledgments
We would like to thank Editage (www.editage.com) for the English language editing of the manuscript.
Conflict of interest
The authors declare that the research was conducted in the absence of any commercial or financial relationships that could be construed as a potential conflict of interest.
Publisher’s note
All claims expressed in this article are solely those of the authors and do not necessarily represent those of their affiliated organizations, or those of the publisher, the editors and the reviewers. Any product that may be evaluated in this article, or claim that may be made by its manufacturer, is not guaranteed or endorsed by the publisher.
Supplementary material
The Supplementary material for this article can be found online at: https://www.frontiersin.org/articles/10.3389/fmicb.2023.1201121/full#supplementary-material
References
Akanuma, G. (2021). Diverse relationships between metal ions and the ribosome. Biosci. Biotechnol. Biochem. 85, 1582–1593. doi: 10.1093/bbb/zbab070
Akanuma, G., Kobayashi, A., Suzuki, S., Kawamura, F., Shiwa, Y., Watanabe, S., et al. (2014). Defect in the formation of 70S ribosomes caused by lack of ribosomal protein L34 can be suppressed by magnesium. J. Bacteriol. 196, 3820–3830. doi: 10.1128/JB.01896-14
Akanuma, G., Yamazaki, K., Yagishi, Y., Iizuka, Y., Ishizuka, M., Kawamura, F., et al. (2018). Magnesium suppresses defects in the formation of 70S ribosomes as well as in sporulation caused by lack of several individual ribosomal proteins. J. Bacteriol. 200:e00212-18. doi: 10.1128/JB.00212-18
Avery, S. V. (1995). Caesium accumulation by microorganisms: uptake mechanisms, cation competition, compartmentalization and toxicity. J. Ind. Microbiol. 14, 76–84. doi: 10.1007/BF01569888
Bossemeyer, D., Schlosser, A., and Bakker, E. P. (1989). Specific cesium transport via the Escherichia coli Kup (TrkD) K+ uptake system. J. Bacteriol. 171, 2219–2221. doi: 10.1128/jb.171.4.2219-2221.1989
Cohen-Bazire, G., Sistrom, W. R., and Stanier, R. Y. (1957). Kinetic studies of pigment synthesis by non-sulfur purple bacteria. J. Cell. Comp. Physiol. 49, 25–68. doi: 10.1002/jcp.1030490104
Dosch, D. C., Helmer, G. L., Sutton, S. H., Salvacion, F. F., and Epstein, W. (1991). Genetic analysis of potassium transport loci in Escherichia coli: evidence for three constitutive systems mediating uptake potassium. J. Bacteriol. 173, 687–696. doi: 10.1128/jb.173.2.687-696.1991
Fiorentini, D., Cappadone, C., Farruggia, G., and Prata, C. (2021). Magnesium: biochemistry, nutrition, detection, and social impact of diseases linked to its deficiency. Nutrients 13:1136. doi: 10.3390/nu13041136
Fujinami, S., Sato, T., and Ito, M. (2011). The relationship between a coiled morphology and Mbl in alkaliphilic Bacillus halodurans C-125 at neutral pH values. Extremophiles 15, 587–596. doi: 10.1007/s00792-011-0389-9
Fujinami, S., Takeda, K., Onodera, T., Satoh, K., Sano, M., Narumi, I., et al. (2013). Draft genome sequence of sodium-independent alkaliphilic Microbacterium sp. strain TS-1. Genome Announc. 1:e01043-13. doi: 10.1128/genomeA.01043-13
Grundy, F. J., Turinsky, A. J., and Henkin, T. M. (1994). Catabolite regulation of Bacillus subtilis acetate and acetoin utilization genes by CcpA. J. Bacteriol. 176, 4527–4533. doi: 10.1128/jb.176.15.4527-4533.1994
Hampton, C. R., Bowen, H. C., Broadley, M. R., Hammond, J. P., Mead, A., Payne, K. A., et al. (2004). Cesium toxicity in Arabidopsis. Plant Physiol. 136, 3824–3837. doi: 10.1104/pp.104.046672
Henkin, T. M., Grundy, F. J., Nicholson, W. L., and Chambliss, G. H. (1991). Catabolite repression of alpha-amylase gene expression in Bacillus subtilis involves a trans-acting gene product homologous to the Escherichia coli lacl and galR repressors. Mol. Microbiol. 5, 575–584. doi: 10.1111/j.1365-2958.1991.tb00728.x
Hirose, K. (2016). Fukushima Daiichi nuclear plant accident: atmospheric and oceanic impacts over the five years. J. Environ. Radioact. 157, 113–130. doi: 10.1016/j.jenvrad.2016.01.011
Hsiao, C., Mohan, S., Kalahar, B. K., and Williams, L. D. (2009). Peeling the onion: ribosomes are ancient molecular fossils. Mol. Biol. Evol. 26, 2415–2425. doi: 10.1093/molbev/msp163
Imazawa, R., Takahashi, Y., Aoki, W., Sano, M., and Ito, M. (2016). A novel type bacterial flagellar motor that can use divalent cations as a coupling ion. Sci. Rep. 6:19773. doi: 10.1038/srep19773
Ishida, Y., Koretsune, T., Ishiuchi, E., Teshima, M., and Ito, M. (2023). A magnesium transporter is involved in the cesium ion resistance of the high-concentration cesium ion-resistant bacterium Microbacterium sp. TS-1. Front. Microbiol. 14:1136514. doi: 10.3389/fmicb.2023.1136514
Jung, K., Krabusch, M., and Altendorf, K. (2001). Cs+ induces the kdp operon of Escherichia coli by lowering the intracellular K+ concentration. J. Bacteriol. 183, 3800–3803. doi: 10.1128/JB.183.12.3800-3803.2001
Koretsune, T., Ishida, Y., Kaneda, Y., Ishiuchi, E., Teshima, M., Marubashi, N., et al. (2022). Novel cesium resistance mechanism of alkaliphilic bacterium isolated from jumping spider ground extract. Front. Microbiol. 13:841821. doi: 10.3389/fmicb.2022.841821
Lee, D. D., Galera-Laporta, L., Bialecka-Fornal, M., Moon, E. C., Shen, Z., Briggs, S. P., et al. (2019). Magnesium flux modulates ribosomes to increase bacterial survival. Cells 177, 352–360.e13. doi: 10.1016/j.cell.2019.01.042
Nakamura, K., Chiba, S., Kiuchi, T., Nabeshi, H., Tsutsumi, T., Akiyama, H., et al. (2022). Comprehensive analysis of a decade of cumulative radiocesium testing data for foodstuffs throughout Japan after the 2011 Fukushima Daiichi nuclear power plant accident. PLoS One 17:e0274070. doi: 10.1371/journal.pone.0274070
Nierhaus, K. H. (2014). Mg2+, K+, and the ribosome. J. Bacteriol. 196, 3817–3819. doi: 10.1128/JB.02297-14
Perkins, J., and Gadd, G. M. (1995). The influence of pH and external K+ concentration on caesium toxicity and accumulation in Escherichia coli and Bacillus subtilis. J. Ind. Microbiol. 14, 218–225. doi: 10.1007/BF01569931
Pontes, M. H., Yeom, J., and Groisman, E. A. (2016). Reducing ribosome biosynthesis promotes translation during low mg(2+) stress. Mol. Cell 64, 480–492. doi: 10.1016/j.molcel.2016.05.008
Sanial, V., Buesseler, K. O., Charette, M. A., and Nagao, S. (2017). Unexpected source of Fukushima-derived radiocesium to the coastal ocean of Japan. Proc. Natl. Acad. Sci. U. S. A. 114, 11092–11096. doi: 10.1073/pnas.1708659114
Senbayram, M., Gransee, A., Wahle, V., and Thiel, H. (2015). Role of magnesium fertilisers in agriculture: plant-soil continuum. Crop Pasture Sci. 66, 1219–1229. doi: 10.1071/cp15104
Takada, H., Roghanian, M., Caballero-Montes, J., Van Nerom, K., Jimmy, S., Kudrin, P., et al. (2021). Ribosome association primes the stringent factor Rel for tRNA-dependent locking in the A-site and activation of (p)ppGpp synthesis. Nucleic Acids Res. 49, 444–457. doi: 10.1093/nar/gkaa1187
Wu, J., Zheng, X., Chen, J., Yang, G., Zheng, J., and Aono, T. (2022). Distributions and impacts of plutonium in the environment originating from the Fukushima Daiichi nuclear power plant accident: an overview of a decade of studies. J. Environ. Radioact. 248:106884. doi: 10.1016/j.jenvrad.2022.106884
Keywords: cesium-resistant microorganism, magnesium, Microbacterium, Bacillus subtilis, ribosome
Citation: Ishida Y, Zhang C, Satoh K and Ito M (2023) Physiological importance and role of Mg2+ in improving bacterial resistance to cesium. Front. Microbiol. 14:1201121. doi: 10.3389/fmicb.2023.1201121
Edited by:
Philippe M. Oger, Adaptation et Pathogenie (MAP), FranceReviewed by:
Christoph Engl, Queen Mary University of London, United KingdomSomnath Chakravorty, Bharat Serums and Vaccines, India
Copyright © 2023 Ishida, Zhang, Satoh and Ito. This is an open-access article distributed under the terms of the Creative Commons Attribution License (CC BY). The use, distribution or reproduction in other forums is permitted, provided the original author(s) and the copyright owner(s) are credited and that the original publication in this journal is cited, in accordance with accepted academic practice. No use, distribution or reproduction is permitted which does not comply with these terms.
*Correspondence: Masahiro Ito, masahiro.ito@toyo.jp