- 1Department of Botany, Maitreyi College, University of Delhi, New Delhi, India
- 2Department of Botany, Dyal Singh College, University of Delhi, New Delhi, India
- 3Department of Biosciences and Technology, Dr. Vishwanath Karad MIT World Peace University, Pune, Maharashtra, India
- 4Department of Biotechnology, Faculty of Engineering and Technology, Manav Rachna International Institute of Research and Studies, Faridabad, India
Plant-associated microbes include taxonomically diverse communities of bacteria, archaebacteria, fungi, and viruses, which establish integral ecological relationships with the host plant and constitute the phyto-microbiome. The phyto-microbiome not only contributes in normal growth and development of plants but also plays a vital role in the maintenance of plant homeostasis during abiotic stress conditions. Owing to its immense metabolic potential, the phyto-microbiome provides the host plant with the capability to mitigate the abiotic stress through various mechanisms like production of antioxidants, plant growth hormones, bioactive compounds, detoxification of harmful chemicals and toxins, sequestration of reactive oxygen species and other free radicals. A deeper understanding of the structure and functions of the phyto-microbiome and the complex mechanisms of phyto-microbiome mediated abiotic stress mitigation would enable its utilization for abiotic stress alleviation of crop plants and development of stress-resistant crops. This review aims at exploring the potential of phyto-microbiome to alleviate drought, heat, salinity and heavy metal stress in crop plants and finding sustainable solutions to enhance the agricultural productivity. The mechanistic insights into the role of phytomicrobiome in imparting abiotic stress tolerance to plants have been summarized, that would be helpful in the development of novel bioinoculants. The high-throughput modern approaches involving candidate gene identification and target gene modification such as genomics, metagenomics, transcriptomics, metabolomics, and phyto-microbiome based genetic engineering have been discussed in wake of the ever-increasing demand of climate resilient crop plants.
1. Introduction
Climate change has lead to several perturbations in the environment such as extremes of heat and cold, drought, waterlogging, and changing weather patterns, which are responsible for adverse effects on crop production globally. Various environmental and anthropogenic factors pose abiotic stress on plants such as temperature, salinity, drought, heavy metals (Sandrini et al., 2022), UV radiation (Shourie et al., 2014), and pesticides (Yasmin and D’Souza, 2010). Temperature fluctuations, erratic rainfall and frequent droughts are also attributed to shifts in agricultural cycles. More than 50% of agricultural losses are caused due to heat, salinity, drought and heavy metal stresses, both qualitatively and quantitatively (Salam et al., 2023). It is worth noting that a minor temperature increase of even 1°C can reduce the crop yield of various crops by 3–7% (Zhao et al., 2017). These aspects are also responsible for changes in the edaphic factors like pH, moisture, salinity, ion content, mineral availability and organic content, which directly affect the crop yield. Salinity and heavy metal accumulation in soil has significant impacts on plant health and crop productivity. Salt stress inhibits seed germination and disturbs the homeostasis at cellular and biochemical level. It affects water uptake, exerts osmotic stress and causes nutritional imbalance in plants. Similarly, heavy metals also cause detrimental effects on plants by imposing toxicity and hampering physiological processes that are vital for survival and growth of plants.
Plants adopt various strategies to survive under unfavorable environmental conditions and have remarkable capabilities of enduring and adapting to abiotic stresses through transient and stable gene expression mediated by stress signaling. The microorganisms present in the soil, rhizosphere, and phyllosphere of plants play a crucial role in the maintenance of environmental homeostasis and enable plants to survive under stress conditions (Barea, 2015; Ngumbi and Kloepper, 2016). A plant acquires its microbiome from the parent plant, the soil in which the seed is sown, and the environment to which it is exposed. Plants and their microbiome are in an exquisite symbiotic relationship and mutually promote growth, health, and development. Plant-associated microbes offer numerous benefits to plants such as fixing atmospheric nitrogen, enhancing the bioavailability of minerals, producing organic nutrients, detoxifying pesticides, harmful chemicals and toxins, mitigating plant diseases, and producing plant growth hormones and bioactive compounds (Sagar et al., 2021). The rhizospheric occupants belonging to the genera Azotobacter (Sahoo et al., 2014), Azospirillum (Omar et al., 2009), Rhizobium, Pantoea, Bacillus, Pseudomonas (Sorty et al., 2016), Enterobacter (Nadeem et al., 2007), Bradyrhizobium (Panlada et al., 2013), Methylobacterium (Meena et al., 2012), Burkholderia (Oliveira et al., 2009), Trichoderma (Ahmad P. et al., 2015) and cyanobacteria (Joshi et al., 2020) have been reported to contribute in growth promotion of several crop plants.
Plant microbiome offers an abiotic stress protection mechanism to the host as the metabolic potential of microbiome is immense and it supplements the metabolic capacity of the plants to acquire nutrition and develop tolerance against stress. The phyto-microbiome is dynamic and its organization is sculpted by the degree and duration of the abiotic stress. The plant and its associated microbiome synergistically respond to abiotic stress for mutual survival and growth (Javaid et al., 2022). Under unfavorable environmental conditions, soil-dwelling microorganisms from the genera Achromobacter, Azospirillum, Variovorax, Bacillus, Enterobacter, Azotobacter, Aeromonas, Klebsiella, and Pseudomonas have been demonstrated to promote plant growth (Dardanelli et al., 2008; Belimov et al., 2009; Ortiz et al., 2015; Kaushal and Wani, 2016; Sorty et al., 2016). Burkholderia phytofirmans strain PsJN has been found to reduce salt stress in Arabidopsis (Pinedo et al., 2015) and maize (Naveed et al., 2014b), as well as drought stress in wheat (Naveed et al., 2014a).
The response of the phyto-microbiome to the abiotic stress largely influences the growth, tolerance, adaptation, and evolution of the host plant and microbes both. There is now mounting evidence that plant-associated microbes may prove to be instrumental in the sustenance of agriculture in times of drastic impacts of climate change. The phyto-microbiome architecture could be better utilized for abiotic stress alleviation of plants and the development of stress-tolerant crop plants if the ecological relationships of the plant-associated microbial diversity and mechanisms of their interactions are deeply understood. The potential options for overcoming crop plants’ productivity constraints in stress-prone environments include the selection, screening, and application of stress-tolerant microorganisms. The application of beneficial microorganisms as bioinoculants can be a good alternative for promoting plant growth under various types of abiotic stresses.
In this review paper, the ecology of phyto-microbiome is summarized, focusing on the beneficial microbes and their role during abiotic stress conditions. The physiological and molecular responses of phyto-microbiome against major stressors drought, heat, salinity and heavy metal toxicity are discussed to determine the role of the microbiome in the stress alleviation of plants. Ascertaining the potential of microbes in the development of stress-resistant plants, the paper further emphasizes modern strategies like introducing novel bio-inoculants, application of multi-omics technologies for gene modification, and phyto-microbiome-based genetic engineering as sustainable solutions to enhance agricultural productivity.
2. Ecological structure and function of phyto-microbiome
The phyto-microbiome is composed of taxonomically diverse communities including bacteria, archaebacteria, fungi, and viruses, which establish various ecological relationships with the host plant such as symbiosis, mutualism, and parasitism (Chialva et al., 2022). Microbiomes associated with plants are either epiphytic or endophytic, and colonize both niches–the phylloplane (above ground part) and rhizoplane (below ground part) (Santos and Olivares, 2021). Various plant compartments in which the microbes form their niche and colonize are depicted in Figure 1. Rhizospheric microbiome is selectively attracted and recruited by the host plant though rhizodeposition in which root exudates containing compounds like amino acids, carbohydrates, organic acids, fatty acids, siderophores and flavonoids, are secreted. These root exudates act as signals to establish communication between plant and specific microbes. The type of root exudates varies with plant’s genotype, innate immunity, signaling pathways and response to environmental conditions. The root exudate composition is instrumental in shaping the structure of phyto-microbiome assembly in the rhizosphere. The epiphytic interactions in the rhizoplane and phylloplane provide oppuortunity to the microbes to enter the tissues, systematically spread through vascular system and colonize other compartments as endophytes. The endophytic community structure is mostly substrate-driven and depends upon the allocation of resources in different plant compartments. Besides plant-microbe interactions, the microbe-soil interactions and microbe-microbe interactions significantly affect the plant growth (Santoyo, 2022).
2.1. Rhizobacteria
The soil microenvironment of the root region is rich in microbes because it contains a wide range of nutrients, minerals, and metabolites. The microbial colonization of the rhizosphere is significantly influenced by root exudates or substances that a plant root secretes (Kong and Six, 2012). Some microorganisms of the rhizosphere that aid in reducing abiotic stress include plant-growth-promoting rhizobacteria (PGPRs), act as beneficial microorganisms that adopt several strategies to reduce abiotic stress, including the production of phytohormones, a decrease in ethylene oxide levels, an increase in the dehydration response, and the induction of genes encoding antioxidant enzymes (Yang et al., 2009). Further, these bacteria contribute to the production of plant growth regulators like indole-3-acetic acid (IAA), deaminase, and 1-aminocyclopropane-1-carboxylic acid (ACC) that aid in enhancing plant growth (Glick, 2014). It was observed that the genera Diazotrophicus, Bacillus, Pseudomonas, Azotobacter, Azospirillium, Rhizobium, Burkholderia, Gluconacetobacter and Serratia are the most important rhizospheric inhabitants that help plants mitigate a variety of abiotic stresses (Backer et al., 2018). In order to reduce stress in rice, Trichoderma harzianum was used to increase aquaporin, dehydrin, and malondialdehyde (Pandey et al., 2016). Additionally, T. harzianum was used to increase the oil yield from salinity affected Indian mustard (Brassica juncea) which enhanced antioxidant synthesis, decreased Na + uptake, and improved nutrient uptake in plants (Cho et al., 2008). Rhizobacteria-induced drought endurance and resilience (RIDER) is defined as changes in the levels of phytohormones, defense-related proteins, enzymes, antioxidants, and epoxy polysaccharides on exposure to various stresses (Kaushal and Wani, 2016). These changes increase the plants’ resistance to abiotic stress (Raymond et al., 2004). IAA and ACC-deaminase production in barley and oats appeared to be improved using Pseudomonas sp. and Acinetobacter sp. (Lu et al., 2013). Pseudomonas sp. improved its ability to inhabit roots sideways is due to its capacity to produce exo-polysaccharides (EPS) stimulus, and increased salinity resistance in rice during germination (Rojas-Tapias et al., 2012). Actinomycetes are known to promote plant growth and lessen the damage caused under abiotic stress. They are able to grow under harsh environment such as high salinity, drought and high temperature (Grover et al., 2016). In the rhizosphere the Actinomycetes utilizes the nutrient and water more efficiently in the stressed soil as they possess the ability to cleave the rhizospheric soil particles and hence form strong bonds with the plants (Sandrini et al., 2022). These bacteria follow several mechanisms such as changes in root and cell wall morphology, 1-aminocyclopropane-1-carboxylic acid (ACC) deaminase activity, possess the ability to avoid oxidative damage, phytohormonal alterations, compatible solute production (glycine-betaine and proline) that promotes osmoregulation (Chukwuneme et al., 2020).
2.2. Phyllosphere bacteria
Phyllosphere is an ideal environment for microbes that harbors a huge variety of beneficial microbes belonging to bacteria, fungi and viruses. The performance of the plant is significantly influenced by the phyllospheric microbiome. These microbes also assist plants in purging contaminants. Additionally, they support the preservation of plant health and control the spread of plant pathogens. The long-distance transport process has a significant impact on the microbiota of plant parts that are distant from the soil or in other aerial parts of plants (Arun et al., 2020). When rice plants were stressed by drought, inoculating the plants with the plant growth-promoting, drought-tolerant Bacillus altitudinis FD48 increased relative water content, chlorophyll stability index, and membrane stability index compared to control (uninoculated plants) (Awasthy et al., 2017).
2.3. Fungi
Many fungi inhabiting the rhizospheric soil have remarkable potential of degradation of various pollutants, thereby protecting the plants from abiotic stress (Shourie and Vijayalakshmi, 2022). Some fungi like Arbuscular mycorrhizal fungi (AMF) are obligate mycorrhizal fungi that form symbiotic relationships with vascular plants including halophytes. AMFs can sporulate in the rhizosphere as well as form vesicles and hyphae in roots. Plant growth is increased because of the excellent access to the soil surface area provided by the hyphal network that AMFs create. Through the effective translocation of nutrients, AMFs contribute to an improvement in plant nutrition. Additionally, they aid in enhancing the health of plants and the soil (Compant et al., 2010). Plant productivity is typically reduced by drought stress, in which AMFs assist plants in retaining growth and increasing yield. AMFs aid in increasing water uptake as part of the drought mitigation mechanism and aid the plant in enhancing nutrient uptake, which enables plants to withstand stresses (Jiang et al., 2016). It was found that the plant biomass, fruit yield, and shoot content of P, K, Cu, Fe, and Zn increased when a tomato plant was inoculated with Funneliformis mosseae under saline conditions (Chandrasekaran et al., 2021). Wheat plants inoculated with AMFs performed well under salt stress and the oxidative damage to the plants is significantly reduced (Hayat et al., 2010). Extremely low and high temperatures, however, were reported to inhibit the development of the extra radical hyphal network and AMF fungal activity, and decrease AMF fungal growth (Mathur and Jajoo, 2020). AMF helps plants grow their root system for water absorption at high temperatures to ensure high photosynthetic capacity and prevent damage to the photosynthetic apparatus. The inoculation of barley (Hordeum vulgare L.) with AMF led to improved growth, photosynthesis, osmotic homeostasis, and potassium uptake under low-temperature conditions, and Glomus versiforme was frequently more successful than Rhizophagus irregularis at boosting survival rates (Hajiboland et al., 2019). Vesicular Arbuscular Mycorrhiza (VAM) also alters the physiological, functional, and biochemical makeup of plants in ways that increase their ability to withstand various abiotic stresses. AMF inoculation in vegetables has been shown to boost biomass production and increase yield (Haghighi et al., 2015; Duc et al., 2018). The uptake of greater amounts of nutrients, leaf water potential, and stomatal conductance are all significantly influenced by AMF inoculation (Khan et al., 2013). When lettuce plants were inoculated with AMF, their abscisic acid (ABA) levels decreased, indicating that they were less stressed than uninoculated plants. Therefore, AMF inoculation modified the plant’s hormonal profile and physiology to make it more suited to saline conditions (Aroca et al., 2013).
2.4. Endophytes
Endophytes have symbiotic relationships with plants and live inside them for the entirety of their life cycles. Endophytes typically invade the seeds, roots, leaves, and stems of host plant, establish colonies in plant tissues and promote plant growth by enhancing nitrogen fixation, phytohormone secretion, and nutrient uptake. During periods of abiotic stress, endophytic microbes stimulate plant growth by various mechanisms such as osmolyte accumulation, induced systemic tolerance, production of phytohormones such as ABA, gibberellic acid (GA), cytokinins and IAA, ACC deaminase production for lowering ethylene. The endophytic Arthrobacter strains EZB4, EZB18, and EZB20 inoculation increased the proline content in Capsicum annum L. exposed to abiotic stress (Sziderics et al., 2007). Salinity stress was alleviated by Bacillus firmus and Bacillus sp. in peanut, Curtobacterium sp. in soybean and rice, Enterobacter ludwigii, Bacillus cereus and Micrococcus yunnanensis in rice (Khan et al., 2019, 2020; Pal et al., 2021). The root fungal endophyte Piriformospora indica induced drought tolerance in Chinese cabbage (Sun et al., 2010) and salt tolerance in barley (Baltruschat et al., 2008), by boosting the levels of antioxidants.
3. Physiological and molecular response of phyto-microbiome against abiotic stress
The responses of crop plants against abiotic stresses are manifested as altered phenotypes at morphological, physiological, and biochemical levels. Plants have developed complex signaling mechanisms to counteract stress conditions and enable survival. The plant microbiome further supplements the metabolic capacity of the plants to combat stress conditions. Here, the major stress factors (drought, heat, salinity and heavy metal) are discussed for their impact, plant response, and the role of the microbiome in combating stress.
3.1. Drought stress
Severe drought stress leads to wilting, yellowing, discoloration, and leaf burning in plants. Plants have the inherent capability to respond to drought stress and they try to control the damage by complex mechanisms. They respond and regulate the drought stress by closing stomata, decreasing the surface area of succulent leaves, and increasing the roots. However, prolonged drought stress is known to stunt plant growth with a reduction in leaf size, and stems, production of a greater number of roots, decrease in RubisCO activity and photosynthetic pigments, reduction in seedling vigor, and decrease in seed germination. It reduces membrane potential and increases the concentration of reactive oxygen species (ROS) causing free radical damage and disruption of ATP synthesis (Shaffique et al., 2022).
3.1.1. Drought stress response and signaling
Water scarcity is detected by the leaves and roots. However, the signals are transmitted majorly from roots to shoots. Plants sense and transmit water deficit through signaling activated by osmotic pressure, ROS and mechanical stresses, involving numerous sensing molecules. ABA is an important phytohormone that is produced in response to drought stress and plays a crucial role in adaptation to drought stress. ABA is mostly produced in vascular tissues and is transported via a transporter to various tissues. It induces stomatal closure and activation of stress-related genes that increase drought resilience (Kuromori et al., 2022). Water deficit induces the expression of enzymes of ABA biosynthetic pathway such as ZEP/ABA1, AAO3, cis-epoxy carotenoid dioxygenase (NCED3), and molybdenum cofactor sulfurase (MCSU/LOS5/ABA3). The binding of ABA with ABA receptor proteins PYR/ PYL/ RCAR initiates the ABA-dependent stomatal regulation pathway leading to the activation of Protein Phosphatases 2C (PP2C) and SNF1-Related Protein Kinases 2 (SnRK2). The transcription factors such as ABF, MYC MYB, NAC, ERF, bZIP, and DREB/CBF are activated, and they bind to nuclear targets resulting in the expression of drought stress proteins (Ali S. et al., 2022; Aslam et al., 2022).
Drought stress causes a variety of biochemical changes inside the host, including an excessive build-up of reactive oxygen species (ROS), which can harm different tissues and cellular components like nucleic acids and other biomolecules, leading to programmed cell death (PCD) (Hasanuzzaman et al., 2020). In addition to altering biogeochemical cycles like the nitrogen and carbon cycles and slowing down the breakdown of organic matter, drought stress can also cause a considerable decrease in plant absorption and translocation of macronutrients (K, N, and P). Drought stress also reduces the absorption of cations (Ca2+, K+, and Mg2+) leading to the inhibition of several vital enzymes (Farooq et al., 2009).
3.1.2. Role of phyto-microbiome in drought stress
It has been discovered that plant-associated microbiomes secrete a variety of chemicals during drought, including phytohormones, osmolytes, and antioxidants, which increase plant drought tolerance. Apart from facilitating plant growth, phytohormones such as IAA, cytokinin, and gibberellins, can assist plants in coping with abiotic stresses. Interestingly, the primary mechanism of mitigation of drought stress by plant microbiome is by inducing drought stress-responsive genes and regulating phytohormones (Iqbal et al., 2022).
3.2. Heat stress
Heat stress leads to a decrease in cell water content, cell size, plant size, growth, and biomass. Severe heat stress leads to scorching and discoloration of leaves, fruits, and other plant parts. Heat stress also leads to the alteration of biomolecular composition. It has been seen that heat stress increases the concentration of amino acids while decreasing the concentration of starch, sugars, and lipids. Maltose concentration has been seen to be elevated (Dastogeer et al., 2022). At the molecular level, heat stress leads to protein denaturation and misfolding. The cell membrane fluidity is increased while membrane integrity is compromised (Sehar et al., 2022). The indirect effects are complex to decipher. The increase in temperature can cause previously unknown infections due to the growth of microbial pathogens and the emergence of newer more pathogenic strains (Velásquez et al., 2018). Continuous thermal stress can increase the deposition of reactive oxygen species (ROS) resulting in membrane depolarization and initiation of programmed cell death (Katano et al., 2018).
3.2.1. Plant defense against heat stress
Plants possess inherent thermal tolerance known as basal heat tolerance while thermotolerance can also be acquired. Plants resort to short-lived or long-term adaptation strategies to combat heat stress. Some plants have leaf and bud shedding, annual flowering, or regenerative stage completion in winter as an adaptation to high-temperature habitats. Heat stress leads to the induction or activation of ion transporters, antioxidants, phytohormones and signal transduction elements. Late embryogenesis abundant (LEA) proteins are produced to protect against heat stress. To prevent acute heat injury and mortality, plants contain molecular stress memory states known as short-term acquired tolerance (SAT) and long-term acquired tolerance (LAT). Particularly, C4 and CAM plants adopt a variety of modifications to boost the process of photosynthesis - under heat stress. Intensive transpiration from leaves can prevent damage by lowering the temperature of the leaves by several degrees (Hasanuzzaman et al., 2013).
Heat stress leads to the production of reactive oxygen species and in response the production of antioxidants such as peroxidase (POX), ascorbate peroxidase (APX), glutathione reductase (GR), superoxide dismutase (SOD), and catalase (CAT) is triggered in plants. The superoxide anion radical is changed by SOD into H2O2 and O2, which are subsequently changed into water and oxygen by CAT and APX. In plant cells, GR plays a role in the regeneration of the reduced glutathione which is an essential antioxidant. These detoxification systems maintain cellular homeostasis and promote plant growth and development during heat stress (Zandi and Schnug, 2022). Plants activate a complex signaling system involving heat shock factors (HSFs) that control the transcription of heat shock genes, including the production of heat shock proteins (HSPs). HSPs help protect the plant by promoting the proper folding of proteins, preventing protein denaturation and aggregation, and facilitating the breakdown of defective proteins. Different types of HSPs are produced, such as Hsp60, Hsp70, Hsp90, Hsp100, and sHSPs, each has specific functions in maintaining cellular homeostasis and promoting thermotolerance. Overall, the synthesis and overexpression of HSFs and HSPs play a critical role in enabling plants to cope with high-temperature stress (Ul Haq et al., 2019).
3.2.2. Induction of thermotolerance
A brief pre-exposure to mild heat stress, also called priming, might cause plants to develop thermotolerance. This brief exposure builds a molecular stress memory which allows quicker and higher expression of heat stress transcription factors (HSFs) that control the production of heat shock proteins (HSPs) and antioxidant genes (Khan et al., 2022). Under heat stress, HSPs work as molecular chaperones to preserve the structure and function of proteins (Jacob et al., 2017). As a result, stress memory enables primed plants to respond swiftly to heat stress and recover from the adverse effects of heat. Four isomers of HSFA1A, B, D, and E, are known as master regulators of heat stress. They trigger the expression of HSFA2. A group of heat stress response genes, known as memory genes are in turn amplified by HSFA2 (Friedrich et al., 2021).
3.2.3. Phyto-microbiome in combating heat stress.
Plant growth-promoting microorganisms (PGPM) can induce thermotolerance in plants by the production of heat shock proteins and induction of structural changes in plants. Moreover, phytohormone production, nutrient mobilization, and nitrogen fixation are brought about by the PGPM. Rhizospheric microorganisms produce and secrete phytohormones like IAA, gibberellins, and cytokinins. Endophytic microorganisms modulate the levels of abscisic acid, salicylic acid, and jasmonic acid under multiple stresses. Auxins are required for cell division and differentiation, growth of root and shoot, and seed germination, gibberellins regulate embryogenesis, stem growth, flowering, and fruit ripening, and abscisic acid regulates cell division and fruit ripening. Cytokines are involved in seed germination, root and shoot development, while ethylene is involved in abscission, senescence, and reproductive development. PGPM which produce gibberellins stimulate plant growth and stress tolerance (Hakim et al., 2021). Plant-associated microorganisms known to secrete exopolysaccharide form a biofilm over the plant roots and make a protective barrier and facilitate nutrient supply. Exopolysaccharide-producing Bacillus cereus was found to increase root and shoot length, chlorophyll content, water-intake, flowering, and fruiting in tomatoes (Mukhtar T. et al., 2020).
3.3. Salinity stress
Salinity reduces nutrient and microbial diversity, organic matter, nitrogen, dissolved organic carbon, and microbial carbon biomass in soil. Additionally, it causes osmotic stress, disturbs the nutrient balance, reduces chlorophyll content, leaf area, and photosynthetic efficiency, and negatively impacts intracellular K+ influx. Salinity stress also affects several cellular enzymes involved in nitrogen metabolism and synthesis of amino acids and indirectly induces the accumulation of ROS, which could damage the plant cells.
3.3.1. Salinity stress response and signaling
Salinity stress is perceived by cell surface receptors relaying the signals through secondary messengers like inositol phosphates and ROS, and the activation of proteins like calcium-dependent protein kinase (CDPK) and mitogen-activated protein kinase (MAPK) that regulate the expression and function of numerous genes. Transcription factors play a pivotal role in imparting resilience towards salinity stress through modulation of expression of the salinity stress genes (Hasanuzzaman and Fujita, 2022).
3.3.2. Role of phyto-microbiome in salinity stress
Plant microbiome employs several strategies to survive salinity stress including production of osmolytes, synthesis of extracellular proteases, and activation of Na+/H+ antiporter. They induce the production of plant growth hormones auxins, cytokinins, and gibberellins. Under salinity stress, the hormone ABA production is stimulated which reduces salinity stress by promoting osmolyte build-up in root vacuoles and the uptake of Ca2+ and K+ (Chen et al., 2022). Cytokinins maintain plant totipotent cells in the shoot and root apical meristems. Under abiotic stress, ethylene is known to accumulate in plants. Ethylene is an essential hormone and signaling molecule which plays key role in growth, seed germination and ripening, root hair elongation, and leaf senescence. However, high ethylene concentration has a detrimental effect on plants. Plant growth promoting bacteria (PGPB) produce ACC deaminase which lowers ethylene by converting ethylene precursor to ammonia and ketobutyrate. In response to abiotic stress, microorganisms develop biofilms, which cover the roots and keep them from drying out. They also foster optimal microenvironments for interactions between plants and microbes (Hakim et al., 2021).
Highly soluble organic substances such as sugars, sugar alcohols, glucosyl glycerol, betaines, amino acids, and tetrahydropyrimidine are produced or accumulated by bacteria. These osmolytes help in maintaining the osmotic pressure of the cells under salinity. At the same time, plant cells also assimilate osmolytes such as disaccharides, oligosaccharides, sugar, alcohols, glycine, betaine, proline, and glutamate which in turn help in the survival of plant microbiome during salinity stress (Kumar et al., 2020).
Salinity stress impacts the uptake of Nitrogen (N), Phosphorus (P), Potassium (K), and water, leading to huge reduction in crop yields. PGPB improve nitrogen uptake and bioavailability of phosphorus by acidification and chelation. Similarly, the bioavailability of microelements such as Cu, Fe, Mn, Zn is also increased. Potassium-solubilizing bacteria such as Burkholderia convert potassium into a bioavailable form. Salinity reduces iron availability and exacerbates iron deficiency in plants. Iron is essential for the activity of several plant enzymes and for the synthesis of chlorophyll (Teo et al., 2022). Siderophore-producing PGPB contribute significantly to Fe accumulation in roots and to its transportation to leaves (Yasmin et al., 2020; Sultana et al., 2021). Endophytic Streptomycetes that produce siderophores have been shown to increase root and shoot biomass as a result of improved Fe supply. Siderophore-producing PGPB have been demonstrated to increase salt tolerance (Afzal et al., 2019; Saeed et al., 2021).
Trichoderma harzianum reduced salt stress in plants by upregulating monodehydroascorbate reductase generating ACC-deaminase, as supported by mutant experiments (Brotman et al., 2013). In salty soil, Pseudomonas sp. and Acinetobacter sp. were found to increase IAA and ACC-deaminase synthesis in barley and oats (Chang et al., 2014). Streptomyces sp. strain PGPA39 was found to reduce salt stress and promote development in ‘Micro-Tom’ tomato plants (Palaniyandi et al., 2014). Tolerance in rice was increased against salt stress by inoculation of Pseudomonas sp. (Sen and Chandrasekhar, 2014) and against salt and high boron stress by inoculation of Bacillus pumilus (Khan et al., 2016).
3.4. Heavy metal stress in plants
Heavy metals pollutants such as Mercury (Hg), Arsenic (As), Cobalt (Co), Manganese (Mn), Iron (Fe), Cadmium (Cd), Nickel (Ni), Zinc (Zn), Copper (Cu), Chromium (Cr) and Lead (Pb) are released into the environment thorugh anthropogenic activities like growing industrialization, intensive agriculture, and urbanization (Ayangbenro and Babalola, 2017; Kurniawan et al., 2022). The uptake of an excessive amount of heavy metals by crop plants from the contaminated soil affects plant health due to toxicity and considerably reduces the yield.
Heavy metals impact the growth and physiological processes either directly by inhibiting cytoplasmic enzyme activity and inducing oxidative stress, or indirectly by altering the phyto-microbiome structure and functions (Dotaniya and Saha, 2016).
3.4.1. Heavy metal stress response and signaling
Plants have evolved various strategies to detect and respond to heavy metal stress in their environment through complex stress signaling processes that involve multiple pathways and mechanisms. The uptake and transportation of heavy metals in plants depend upon several transporters and proteins which help in their sequestration, intracellular or tissue compatrmentalization and detoxification. ATP-driven pumps HMA (Heavy Metal ATPases) are found on the plasma membrane and tonoplast (vacuolar membrane). They transport heavy metal ions such as such as Cu, Zn Cd and Pb across membranes and facilitate the sequestration of heavy metals into vacuoles or their extrusion from the cytoplasm. ZIP transporters (Zrt/Irt-like Protein) are involved in the uptake of essential metals such as Zn, Fe, Mn and Cu, but they can also transport toxic metals like Cd and Pb on exposure. ZIP transporters are located in the plasma membrane and are responsible for the uptake of these metals from the soil into the root cells. NRAMP transporters (Natural Resistance-Associated Macrophage Protein) are involved in the uptake and translocation of many divalent metal ions such as Fe, Mn, Zn and Cd. They are found in the plasma membrane and endomembranes of plant cells. NRAMP transporters have been shown to play a role in metal distribution within the plant and in metal detoxification processes. ABC transporters (ATP-Binding Cassette) constitute a large family of proteins involved in various cellular processes, including heavy metal transport. Some ABC transporters are known to transport heavy metals such as Fe, Cu and Zn. They are also present in the plasma membrane and other intracellular membranes.
It has been seen that initial abiotic stress signaling pathways are shared among the different types of abiotic stress. The heavy metal stress signaling involves production of Reactive Oxygen Species (ROS) such as superoxide radicals (O2−) and hydrogen peroxide (H2O2), in response to heavy metal induced cellular damage in plants. They act as secondary messengers in the signaling pathways. Mitogen-Activated Protein Kinase (MAPK) signaling pathway is one of the major pathways activated by heavy metal stress. MAPKs modulate the expression of stress-responsive genes, including those involved in metal detoxification and ROS scavenging. Phytochelatins (PCs) are small peptides synthesized in response to heavy metal stress by the enzyme phytochelatin synthase (PCS), which conjugates glutathione molecules to form PC complexes. PCs play a crucial role in heavy metal detoxification by chelating heavy metals and sequestering them into vacuoles, preventing their toxicity. Metallothioneins (MTs), low molecular weight, cysteine-rich proteins, are induced by heavy metal stress, which have a high affinity for heavy metals and can bind and sequester them, thereby reducing their toxicity. MTs are involved in metal homeostasis and play a protective role against heavy metal stress in plants. Heavy metal stress triggers changes in intracellular calcium (Ca2+) concentrations, leading to calcium signaling. Calcium ions act as secondary messengers and regulate Calcium-dependent protein kinases (CDPKs) are activated by increased calcium levels. CDPKs modulate the expression of stress-responsive genes. Heavy metal stress can also activate the abscisic acid (ABA) signaling pathway in plants, which promotes the expression of stress-responsive genes, thereby improving plant tolerance to heavy metals. Several transcription factors specifically AP2/ERF, MYB, WRKY, and NAC families are involved in regulating the expression of stress-responsive genes under heavy metal stress and regulate the expression of heavy metal response genes (Tiwari and Lata, 2018; Keyster et al., 2020).
3.4.2. Role of phyto-microbiome in heavy metal stress
Phyto-microbiome plays a crucial role in heavy metal stress mitigation in plants. These microorganisms render the protection to plants from harmful effects of heavy metals through many ways, such as heavy metal sequestration or biosorption, nutrient mobilization and solubilization, heavy metal transformation and detoxification, induction of stress tolerance.
Microbes efficiently bind and sequester heavy metals, preventing their accumulation in plant tissues. They promote the immobilization and containment of heavy metals in the soil, reducing their bioavailability to plants. Microbial enzymes can mobilize and solubilize the essential nutrients in the soil, making them more accessible for plant uptake. They can convert insoluble compounds into soluble forms, increasing their bio- availability and reducing heavy metal toxicity. Microbes also transform and detoxify heavy metals through processes like reduction, oxidation, and methylation.
Plant-associated microorganisms induce systemic resistance and enhance the stress tolerance of plants. They can stimulate the production of plant growth-promoting hormones, antioxidants, and other protective compounds, which help plants to cope with heavy metal stress. It is important to note that the effectiveness of plant-associated microorganisms in heavy metal stress mitigation can vary depending on the specific microorganism, plant species, and environmental conditions. Syed et al. (2023) isolated strains of Pseudomonas fluorescence and Trichoderma spp. from heavy metal contaminated soil and improved the growth and yield of chickpea by lowering Cd uptake (; Oubohssaine et al., 2022; Syed et al., 2023) isolated rhizobacterial strains from heavy metal contaminated mining sites and studied their application on growth of Sulla spinosissima L. in a highly multi-polluted toxic soil. They observed that the strain LMR291 (Pseudarthrobacter oxydans), LMR340 (Rhodococcus qingshengii), LMR249 (Pseudarthrobacter phenanthrenivorans), and LMR283 (Pseudomonas brassicacearum) substantially improved all the growth parameters of Sulla plants, their photosynthetic pigments, and their antioxidative enzymatic activities (Oubohssaine et al., 2022).
4. Bioinoculants for alleviating abiotic stresses
Bioinoculants are formulations of microorganisms which can be inoculated in crop plants for facilitating growth and enhance production. These comprise of living or quiescent cells of specific microbial strains that benefit host plant by mechanisms such as facilitating nutrient acquisition, releasing plant growth hormones, and other biological activities like pest control. Additionally, bioinoculants may also be used to mitigate the harmful effects of abiotic stress (Benidire et al., 2020). Several theories have been proposed to elucidate the mechanisms of beneficial effects of bioinoculants including production of phytohormones, biofilm, EPS, and ACC deaminase (Tittabutr et al., 2013; Ansari et al., 2019; Goswami and Deka, 2020), production of antioxidants (Singh et al., 2019), cryoprotectants, heat shock proteins, solubilization of minerals such as phosphorus (P), potassium (K), and zinc (Zn), nitrogen (N) fixation, production of siderophores (Ferreira et al., 2019), antibiotics (Jin et al., 2021), hydrolytic enzymes such as proteases, cellulases, chitinases, and β-glucanases (Veliz et al., 2017), and volatile compounds (Harun-Or-Rashid and Chung, 2017). Some microbes also improve the induced systemic resistance (ISR) and systemic acquired resistance (SAR) and thereby help in alleviating multiple stresses in plants. Table 1 summarizes the effects of microbial inoculants on mitigation of abiotic stresses.
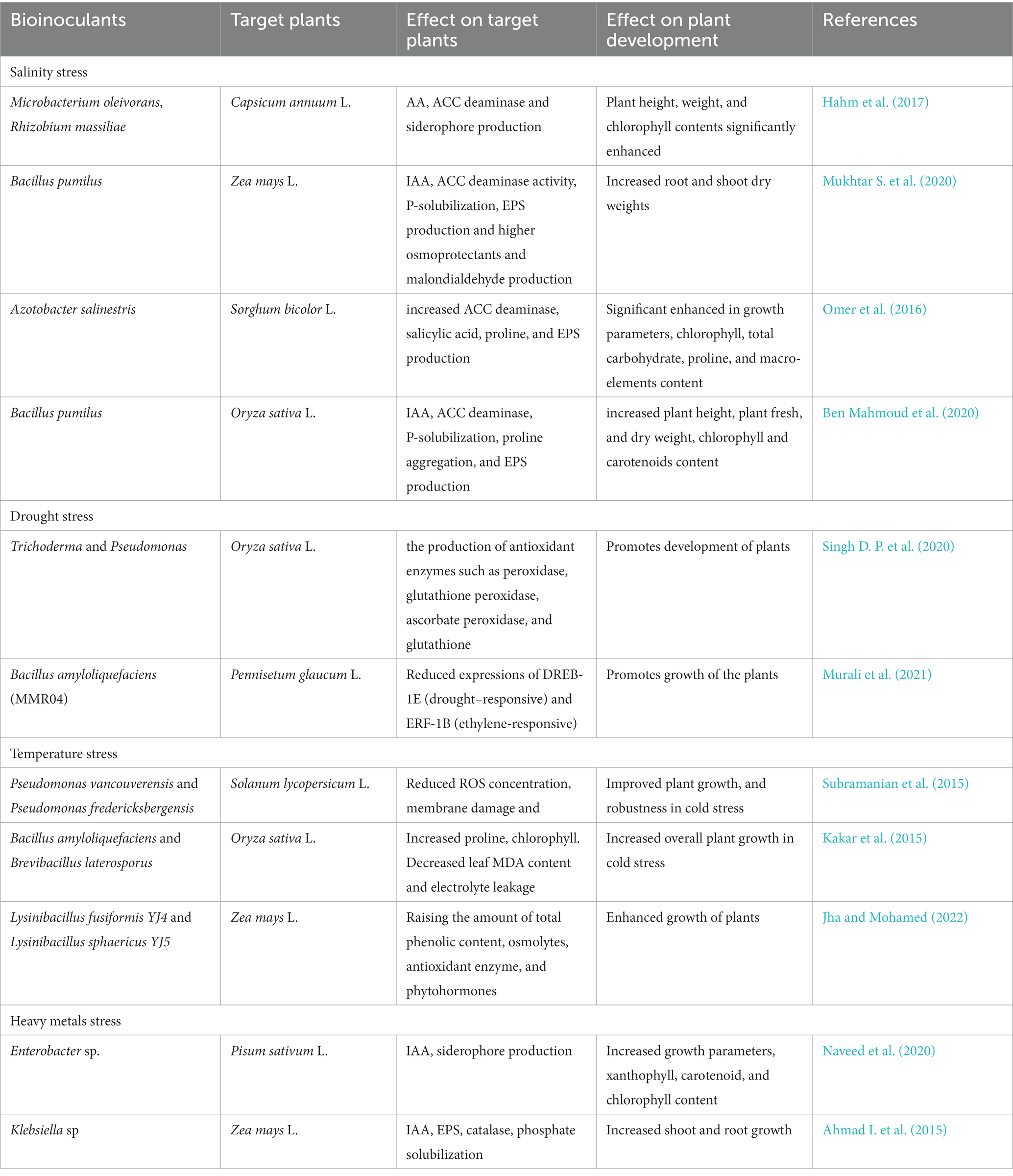
Table 1. Effects of various microbial inoculants in reducing abiotic stress and improving plant stress resistance.
4.1. Bioinoculants for inducing salt stress tolerance in plants
In saline soils, plants experience two forms of stresses- nutrient stress and osmotic stress (Ashrafi et al., 2014). Salinity stress increases the production of ethylene hormone which is damaging and inhibits plant growth. Bioinoculants consist of microbes that produce ACC deaminase, which lowers ethylene concentration and maintain plant growth in saline conditions (Ansari et al., 2019). Numerous studies have shown that the ACC deaminase-producing microbes support plant growth in saline environments such as Bacillus cereus in Vigna radiate L. (mung bean) (Islam et al., 2016), Bacillus pumilus strain TUAT-1 in Oryza sativa L. (rice) (Win et al., 2022) and Enterobacter strain G in Cajanus cajan L. (Anand et al., 2021).
4.2. Bioinoculants for inducing drought stress tolerance in plants
Drought stress directly affects the water relations in plants and exters huge impacts on plant physiology. Bacterial IAA promotes the production of ACC deaminase, which dissociates one of the ethylene precursors and delays the onset of senescence in drought stressed plants (Uzma et al., 2022). Microbially produced ACC deaminase resists plant root drying by degrading ACC and reducing the level of ethylene in the plant cell (Ngumbi and Kloepper, 2016). Like auxin, cytokinin is another plant hormone that is important in preventing early leaf mortality during water shortages. Microbes also increase the synthesis of the endogenous stress hormone ABA, which is crucial for the plant’s drought resistance. Higher levels of endogenous ABA increase root-water conductivity by upregulating the expression of aquaporins (Goswami and Deka, 2020). Microbial inoculation also increases the synthesis of antioxidant enzymes in the plant, which helps the plant to enhance drought tolerance by decreasing ROS and increasing the production of antioxidant enzymes. Batool et al. (2020) showed that inoculating potatoes with Bacillus subtilis HAS31 reduced ROS production and mono-dehydroascorbate (MDA) production while increasing catalase, peroxidase, superoxide dismutase, and total soluble sugar in drought-stressed environments.
4.3. Bioinoculants for inducing temperature stress tolerance in plants
Heat stress has an impact on plants at several growth stages, including seed germination and reproduction, on a physical, physiological, and biochemical level. During high-temperature stress, seed germination rate and stand establishment are reduced due to the disturbed activity of enzymes involved in the breakdown of starch and synthesis of ABA & GA (Begcy et al., 2018). High temperatures have a significant impact on the photosynthetic process because they cause thylakoid disorganization, grana swelling and loss, a decrease in the activity of the electron acceptor and donor sites of photo-system (PS) II, and a decrease in the activity of enzymes such as RuBisCO (Hassan et al., 2020). Several microbes that are utilized as bioinoculants can withstand extremely high and extremely low temperatures. The temperature stress tolerance mechanisms include synthesis of heat and cold shock proteins, biofilm formation, and production of osmo-protective chemicals (Bruno et al., 2020). Inoculating Solanum lycopersicum L. (tomato) under chilling stress with Trichoderma harzianum was shown to increase photosynthesis and growth rate by lowering lipid peroxidation, electrolyte leakage, reducing ROS concentration, increasing leaf water and proline concentration (Ghorbanpour et al., 2018).
4.4. Bioinoculants for inducing heavy metal toxicity tolerance in plants
Heavy metals are absorbed by plants from contaminated soil through roots and are translocated to aerial parts through xylem, where they are bioaccumulated and impose considerable toxicity. Bioinoculants alleviate heavy metal toxicity by producing microbial siderophores for metal chelation, and phytohormones that boost the antioxidative enzymes in plants (Nazli et al., 2020). PGPB that are heavy metal tolerant (HMT) not only lessen the harmful effects of heavy metals but also encourage plant development in such conditions. Inoculation with the HMT-PGPB consortium increased the growth of Sorghum bicolor L. plants while also lowering the bioavailability of heavy metals Cu, Cd, Pb and Zn (El-Meihy et al., 2019). The inoculation of Mucor sp., Klebsiella pneumoniae, Bacillus pumilus, Klebsiella sp., and Enterobacter sp., also considerably reduced heavy metal contamination and improved plant growth (Ma et al., 2015; Karthik et al., 2016; Pramanik et al., 2017; Zahoor et al., 2017; Mitra et al., 2018).
5. Multi-omics approaches to mitigate abiotic stress in plants
Omics refers to the modern day technologies that give a deep insight into the metabolism, genomics and transcriptomics processes occurring in plants and hence the multi-omics approaches are advantageous in plant improvement against abiotic stresses. The understanding and sequencing of the whole plant genome in Arabidopsis thaliana L. have proved the potential benefits of omics tools. Various other plants such as rice, maize and soyabean possess a complicated genome, which have been fully sequenced by the use of omics technology (Ali A. et al., 2022). Numerous studies suggest that under abiotic stress not all genes are turned on or off at the same time, due to which the plant metabolism becomes complicated to understand and hence the phenotype cannot be determined by the genotype (Jha et al., 2019; Singh N. et al., 2020). Therefore, the amalgamation of proteomics, genomics transcriptomics, metabolomics, epigenomics, ionomics, interact omics, phenomics could help in identification of the candidate genes and improve the productivity of various crops under abiotic stress (Kumar et al., 2022).
The plant-microbe interactions are better understood due to the recent development in the various omics tools and sequencing technologies involving the regulation of gene expression and biodiversity (Sandrini et al., 2022). The characterization of beneficial microbes associated with plants and their functions along with the knowledge of rhizospheric science are possible due to the microbiome based multi-omics studies (White et al., 2017). The integrated omics approaches, computational and synthetic biology along with latest advances in high throughput culturing are providing significant knowledge about the structure and function of diverse natural microbiomes and providing avenues for artificially engineering the microbial communities and hence improving the crop growth, protection against pathogens and several abiotic stresses (Trivedi et al., 2021). Plants’ regulatory networks function to induce protective genes while inhibiting the negative regulators activated by abiotic stress factors. These regulations are responsible for the restoration of cellular homeostasis during the stress phase of plant cells. Multi-omics approaches help to integrate cellular processes at different levels based on systems biology knowledge (Katam et al., 2022).
5.1. Transcriptomics in abiotic stress
Transcriptome studies are a novel approach to understand the response of plants to abiotic stresses. Next-generation sequencing (NGS) and parallel RNA sequencing (RNA-Seq) are the two most promising techniques that open a new dimension of biological research to identify the networks among genes that actually respond to stress (Janiak et al., 2016). The advent of high-throughput omics techniques and rapid advancement in post-genomic epoch, specifically the next-generation sequencing (NGS), molecular characterization and modeling have proved beneficial in improving the efficiency and resilience of crop plants under abiotic stress (Pandey et al., 2021). The benefits of plant associated microbes and their communities are well understood by the use of NGS on DNA extracted from soil and rhizosphere and hence lead to better knowledge of their diversity, structure, abundance and important microbes (Alawiye and Babalola, 2019). Also the clustered regularly interspaced short palindromic repeats (CRISPR/Cas9) is an important technology and serves to knockout non-transgenic plant and microbe mutants, characterize symbiosis-related protein, plant traits that sustain beneficial microbiome, various genetic factors and identification of candidate genes responsible stress tolerance and further assigning them specific functions (Levy et al., 2018; Khatabi et al., 2019). The overexpression of transcription factors increases the expression of genes that encodes enzymes and chaperones associated with endoplasmic reticulum stress response, resulting in an increase in rate of photosynthesis and tolerance to drought-like abiotic stress (Wang et al., 2018).
Expressed sequence tags (ESTs), microarray, Affymetrix GeneChip technology, and serial analysis of gene expression (SAGE) have been used to elucidate the function of various genes associated with abiotic stress (Varshney et al., 2009; Deokar et al., 2011; Le et al., 2012). Chickpea genotype microarray study shows 210 differentially expressed genes (DEGs) and numerous differentially expressed unigenes under drought stress (Wang et al., 2012). Global transcriptome profiling of the root tissues of drought-stressed lentils, chickpeas, and ground nuts identified differentially expressed genes (DEGs) involved in different energy metabolism pathways like TCA cycle, glycolysis cycles mediated by transcription factors like WRKY, zinc finger family protein, bHLH, NAC, AP2/ERF and MYB protein domain family (Brasileiro et al., 2015; Singh et al., 2017). Genomics studies showed that ZmWRKY40 and ZmNF-YB2 genes encode a transcription factor that helps in resistance to drought in maize plants (Nelson et al., 2007; Zhang et al., 2008; Gangola and Ramadoss, 2020). In Arabidospsis bZIP was identified and it was associated with drought, salt, and cold tolerance by increasing oxidative enzyme level (Zong et al., 2018).
5.2. Meta-transcriptomics and metaproteomics
Meta-transcriptomics helps in the assessment of expressed genes (Nilsson et al., 2019). Studies related to ecology of microbial communities were possible by the sequencing of transcripts (RNA-seq) (Marcelino et al., 2019). However, interpretation of RNA-seq results is a tedious process but the advancement of databases and the increasing availability of annotated transcriptomes in curated databases as well as development of a robust de novo RNA-seq assembler can help in making the explanation of the result easier (Kuske et al., 2015). Metaproteomics provides functional data and suggests about the complex matrix such as specific soil sample. It emphasizes the study of proteins present in a biomass (Sandrini et al., 2022). It is an important phenomenon that helps in recognition of metabolic pathways, characterization of biological processes, plant-microbe interactions, their structure, function, significance, dynamics, and r egulation of symbiosis and molecular basis of cell communication (Khatabi et al., 2019).
5.3. MiRNA-omics in abiotic stress
Plant produces miRNAs (micro-RNAs) that are post-transcriptional gene-expression regulators, which help them to survive under stress conditions (Zhang and Wang, 2015). By using computational tools like screening of small RNAs library, different drought stress-responsive miRNAs were identified from Arabidopsis, rice, and sugarcane (Liu et al., 2008; Zhou et al., 2010; Gentile et al., 2015). Fourteen different stress-inducible miRNAs were identified from Arabidopsis and among them, miR168, miR171, and miR396 responded to all of the different types of stresses (Liu et al., 2008). In rice 18 cold-responsive miRNAs were identified by (Lv et al., 2010) most of which were downregulated by the cold stress (4°C) and it was supposed that miRNAs were ubiquitous regulators in rice. While on the other hand, due to cold stress sharp increase in the expression of miR812q in rice plants was observed in starting of the reproductive phase (Jeong et al., 2011). High-throughput sequencing revealed that 31 cold stress-induced genes were upregulated and 43 miRNAs were downregulated in tea (Camellia sinensis L.) (Zhang et al., 2014). In Arabidopsis roots enhanced expression of a few miRNAs like miR156g, miR157d, miR158a, miR159a, miR172a,b, miR391, and miR775 were observed, under low oxygen stress situations (Moldovan et al., 2010). In wheat Tae-miR6000, miR156, miR159, miR164, miR167a, miR171 and miR395 were identified as UV-B responsive microRNAs (Wang et al., 2013).
The functions of miRNAs in response to abiotic stresses as well as plants’ development are determined by using artificial miRNAs (amiRNAs), which could be useful to design the strategies for silencing endogenous genes and inhibit the expression of target genes (Zhang F. et al., 2022). Technologies such as Real-Time Quantitative Reverse Transcription Polymerase Chain Reaction (qRT-PCR) and microarrays suggested that abiotic stress conditions induce miRNA expression profiles and are diverse among plant species (Begum, 2022).
5.4. Metabolomics in abiotic stress
Metabolomics technology provides a chemical profile of thousands of compounds and involves the use of high-pressure liquid chromatography along with high resolution mass spectrometry (LC–MS), gas chromatography and mass spectroscopy (GC–MS) and nuclear magnetic resonance (NMR) spectroscopy for characterization of stress induced metabolites (Crandall et al., 2020). The suitability of the selection of the technique depends on the speed, sensitivity, and accuracy of the method used (Ghatak et al., 2018). Due to stress conditions plants can adapt to the stress or tolerate the stress. Mostly metabolomics studies focused on the comparative analysis of stress-susceptible and stress-tolerant responses of plants. Along with amino acids other metabolites like sugar, phenolic compounds, and organic acid also play an important role in plant abiotic stress (Dawid and Hille, 2018; Ghatak et al., 2018). The metabolomics and other -omics technologies, allowed a detailed and in-depth analysis of plant stress as the result of the alteration of metabolites and gene expressions (Anzano et al., 2022). It was observed that under stress conditions plants activate high proline production but under the stress recovery phase, it undergoes proline catabolism (Krasensky and Jonak, 2012). Metabolomics was used to study phytohormone response against salt stress as a targeted approach in roots and shoots in Arabidopsis seedlings (Šimura et al., 2018) and non-targeted approach in maize (Richter et al., 2015). Metabolomics revealed the contribution of overexpression of GmGSTU4, responsible for production of glutathione transferases (GSTs) and increase the glutathione biosynthesis under salt stress in transgenic tobacco (Kissoudis et al., 2015). In mycorrhizal roots the variations in the metabolomics profile have been observed leading to the identification of potential primed compounds that are involved in improved stress tolerance in mycorrhizal plants (Rivero et al., 2018; Bernardo et al., 2019). Metabolomics play an important role in studying the exudates released by the roots in the rhizosphere which serve as a significant feed source for the microbes that are associated with the roots as compared to the surrounding soil that is poor in nutrients (Escudero-Martinez and Bulgarelli, 2019). A major issue in metabolomics is that a huge variety of potential metabolites are present in any given sample and due to the limited extent of public metabolite reference databases assigning a measured metabolite to a specific organism or condition, it becomes difficult to correlate the metabolite production to a particular stress (Khatabi et al., 2019).
5.5. Genomics in abiotic stress
Abiotic stress studies on plants include many cellular processes like sensing, signaling, transcription, transcript processing, translation, and post-translational protein modifications. These studies ultimately boost crop productivity and agricultural sustainability through genetic, chemical, and microbial approaches (Zhang H. et al., 2022). The effect of different abiotic stress in plants was monitored quantitatively by using imaging technology along with the support of information technology. Various phenotypic expressions of plants are useful quantitative phenotypic tools, as genotypic changes lead to the expression of phenotypes. By using a combination of genomics and data science, we can analyze the plant stress responses under different combinations of environmental stress (Zandalinas and Mittler, 2022). In this method target sequences can be designed and introduced into the most appropriate vectors. DNA, RNA, or RNPs like genetic cargo is selected for further delivery by (i) modifying the targeted sequence, (ii) regenerating the edited calli, and (iii) producing the edited plants (Farooqi et al., 2022).
5.6. Metagenomics
Metagenomics identifies the genomic diversity and functions of microbial genes (Solden et al., 2016) and suggests about the relative abundance and taxonomic composition (Singer et al., 2016). The plant-microbe complexity can be studied through next-generation DNA sequencing methods like 454 pyrosequencing and second and third generation sequencing platforms such as PacBio RSII Sequel, GridION, Illumina MiSeq, NovaSeq, GeneStudio, Oxford Nanopore MinION, PrometION, Ion Torrent PGM (Nilsson et al., 2019). Two key methods such as shortgun metagenomics and metabarcoding are employed to identify the microbial communities and compare them on the basis of composition, richness, evenness and assembly (Sharma et al., 2020).
5.7. Phenomics-manipulating plant root-associated microbiomes
The plant root-associated microbiome influences numerous plant traits, primary and secondary metabolites that act as growth substrate for few microbes and have antagonistic effect on the others, they act as signals that regulate the plant microbe interactions. Although few rhizospheric microbial species act as symbionts or growth promoting rhizobacteria are beneficial for the plants and helps in enhancing the plant pathogen defense and nutrition, few microbes may be parasitic and commensal (Lareen et al., 2016; Pascale et al., 2020; Chen et al., 2021). Therefore the study and classification of the complex interaction of plant microbiome and soil rhizosphere is important for developing novel approaches towards crop resilence against pathogens and environmental stresses (Zenda et al., 2021).
6. Dynamic role of fauna in plant microbiome functions
Some of the useful fungi serve as plant parasitic nematode hunters such as A. avenae is effective against Ditylenchus (plant-parasitic nematode) propagation and is an active bio-controlling agent in many parasitic nematodes and pathogenic fungi (Haraguchi and Yoshiga, 2020). Members of Protista are abundantly present in the plant rhizosphere and regulates various mechanism such as nutrient recycling, and interactions in the food web, promotes productivity (Hünninghaus et al., 2017), and lowers the total bacterial biomass (Krome et al., 2010). It also suppresses plant pathogens and boosts immunity of plants against various pathogens. They represent diverse modes of nutrition, wide range of prey interactions, and chemical communication (Mahmud et al., 2021; Solanki et al., 2022). Due to their numerous interactions, the protists cause significant changes in the structure and function of the microbiome. Also regulates auxin and cytokinin levels and hence possesses a significant effect on plant microbiome linked to several hormonal fluctuations (Krome et al., 2010). Earthworms also possess a strong impact on the soil microbiome. It is present in the soil rhizosphere and significantly adds to about 80% of the biomass in the soil macrofauna (Yasmin and D’Souza, 2010). Depending on the earthworm type and the micro-habitat, its impact can be positive, negative, or neutral on the diversity and enrichment of the microbial population (Afridi et al., 2022b). The activities of microorganisms are activated due to the release of acutaneous mucus (glycoprotein) by the earthworm that enhances the interactions. Due to the significant interaction between the microorganisms and the earthworm, there is improved microbial activity in the soil, increased availability of nutrients and increased carbon turnover (Bedano et al., 2019).
7. Plant microbiome engineering to combat abiotic stress
Plant microbiome engineering (PME) is an important approach to promoting plant health, growth, and productivity under adverse environmental conditions (Afridi et al., 2022a). According to Parnell et al. (2016) soil microbiome is the next green revolution as it is serving as a promising tool that will meet the future global food demands. PME has been used to improve nitrogen use efficiency (NUE) in plants (Lau et al., 2022). Also, it is a beneficial technology in plants as it regulates the mechanism of hormones and specific antagonistic metabolite (rhizobitoxine) production that provides resistance against several pathogens, suppresses soil-borne diseases, and regulates nutrient availability in the rhizosphere (Rodríguez et al., 2020; Figure 2). Various approaches used for engineering the phyto-microbiome for developing abiotic stress tolerance in crop plants are summarized in (Table 2). The steps involved in engineering the plant microbiome include selection and engineering of the host-mediated multi-generation microbiome, inoculation of microbial communities as bioinoculants in the rhizosphere, soil, seedling/ seeds, mixed strain inoculation, tissue atomization, and direct injection in the plant tissues.
7.1. Selection and engineering of the host-mediated multi-generation microbiome
The selection and engineering of the microbiome are important as it selects the microbial communities through the host and influences and modifies the traits of the host plant which further influences the microbiome (Mueller and Sachs, 2015). Hence there is a synergistic relationship between host-mediated microbial communities and contributes significantly to agricultural yield, biodiversity, and food security (Kaul et al., 2021). The “artificial selection of the ecosystem” was done to screen the plant biomass of Arabidopsis thaliana L.with the lowest (low selection lines) and highest (high selection lines) plant biomass which was earlier improved by the microbial community and their interactions with the plants for over 16 generations (Swenson et al., 2000). Different studies in Arabidopsis thaliana L. and Brassica rapa L. (Panke-Buisse et al., 2015) suggest a positive correlation between plant biomass and increase activity of microbial extracellular enzymes leading to soil nitrogen mineralization and suggesting the dynamic role of microbiomes to deal with numerous environmental and agronomic issues (Orozco-Mosqueda et al., 2018).
7.2. Inoculation of microbial communities as bioinoculants in the rhizosphere, soil, seedlings/seeds
Using microbial communities as bioinoculants finds wide applications such as plant growth, enhanced nutrient mobilization, stress resilience (Al Kahtani et al., 2020; Alok et al., 2020). The inoculation of different external strains from the rhizospheric soil can alter the structure of the microbiome. Different studies suggest their significant role. For example, the healthy oilseed crop was grown by using the bioinoculants on oil palm seedlings (Elaeis guineensis Jacq.), it modified the enzymatic and dynamic potential of rhizospheric microbes (Veeramachaneni and Ramachandrudu, 2020). Inoculation of Agrobacterium sp. 10C2 in Phaseolus vulgaris enhanced plant biomass and nodule formation by increasing antioxidant level, flavonoids, polyphenols, and phosphorus content in the beans, and also promoted colonization of beneficial rhizobacteria Brevibacterium, Paenibacillus koreensis, Bacillus pumilus and Actinomyces (Chihaoui et al., 2015). A group of biocompatible microbial communities enhanced the growth of maize seedlings under a greenhouse with low-phosphorus soil. They are the group of engineered bacteria that were studied for biofilm formation, phosphate solubilization, and root colonization (Magallon-Servin et al., 2020). In orchids, the inoculation of Klebsiella oxytoca and Pseudomonas fluorescens into Dendrobium nobile Lindl. promoted the vigor, growth, germination, and adaptability (Pavlova et al., 2017). Growth of tomato seedlings (Lycopersicon esculentum L. cv. Saladette) was significantly enhanced with the co-inoculation of two endophytic strains Pseudomonas stutzeri E25 and Stenotrophomonas maltophilia CR71 in the rhizosphere as compared to single inoculation (Rojas-Solís et al., 2018).
7.3. Mixed strain inoculation and tissue atomization
The significant effect of the microbiome depends upon its interaction, multiple mechanisms, and functions carried out by the microbial community. Mixed strain inoculation proved beneficial compared to single or no inoculation in Populus plants where additive incorporation of strains of Pseudomonas and Burkholderia isolated from Populus deltoids L. significantly improved the plant’s photosynthetic capacity and root biomass (Timm et al., 2016). Also, the response was analyzed by transcriptomics, and specific genes for Pseudomonas and Burkholderia were turned on through inoculation of each strain and mixture including genes that encode for stress (temperature & salinity) and regulate plant hormone (ethylene). The mixed inoculation was studied in various other genes involved in the synthesis of lipids, sulfate, and thiamine and also the comparison of mixed and single inoculation was studied on the metabolic profiling of the leaf (Timm et al., 2016). The tissue atomization technique proved successful in improving plant development through the bioengineering of plant microbiomes without any genetic manipulation. This technique was exploited by using an endophytic bacterium Paraburkholderia phytofirmans PsJN in the flowers of dicot and monocot plants and significant improvements were observed in the seed microbiome by vertical inheritance as well as growth parameters (Mitter et al., 2017).
7.4. Direct injection in the plant tissues
This technique helped in incorporating the antimicrobial properties in the plants susceptible to attack by pathogens. For example, Manuka (Leptospermum scoparium L.), a medicinal plant produces anti-microbial oil that is having a potential effect against pathogenic bacteria (Pseudomonas syringae; Orozco-Mosqueda et al., 2018). Bacteria were able to colonize and survive in the plant through direct inoculation of biocontrol agent and PGPB (Arthrobacter agilis UMCV2) which is given a direct injection in the stem of Medicago trancatula L. plant (Avilés-García et al., 2016). More efficient colonization will occur depending on the bioavailability of nutrients (Avilés-García et al., 2016). Certain plants for example Zea mays L., and teocinte have shown direct injection techniques using bacterial endophytes (Johnston-Monje and Raizada, 2011).
8. Conclusion
Microbial interactions with plants have multifaceted functions; on one hand, microbes help plants to maintain their growth and development by fixing, mobilizing, and producing nutrients, hormones, and organic phyto-stimulant compounds, while on the other hand, they induce local or systemic stress alleviation response mechanisms in plants to survive under abiotic stress conditions. Phyto-microbiome essentially helps crop plants in their adaptation and survival on exposure to abiotic stress via induced systemic tolerance. It plays a key role in determining the varying levels of phytohormones, defense-related proteins, enzymes, antioxidants, and secondary metabolites, which mediate the stress-signaling processes. Growth-promoting microbes use various mechanisms to enhance plant growth under stress conditions, which include the production of plant growth regulators, iron and zinc sequestration, phosphorus and potassium solubilization, siderophore production, atmospheric nitrogen fixation, secondary metabolite production, as well as facilitation of uptake of other essential macro- and micronutrients from the soil. Microbial communities exhibit excellent resilience towards environmental challenges. The microbiome also displays functional redundancy by which, in the wake of environmental stresses, one microbial taxon can be replaced by another that can survive the stress. In addition to enhancing the microbial community structure, the introduction of advantageous stress-tolerant microorganisms can help improve plant and soil health when exposed to abiotic stress.
Recent agricultural practices have provided evidence that microbial bio-inoculants such as PGPRs not only aid in reducing environmental stresses but also increase the production of a variety of crop plants including rice, maize, barley, and soybean. The bio-inoculants not only enhance crop yield by bolstering the plant’s defense mechanism and protecting it from abiotic stress such as drought, and salinity, but they also improve soil health. Recently, the use of a consortium of microorganisms in crop production has been promoted because a single bioinoculant might not be sufficient to protect plants from various stresses. The development of a novel and effective bio-inoculant formulation must be centered on the selection of effective native strains for improved outcomes and the bioinoculants must be tested at multiple sites prior to commercialization to avoid failure at field level.
Multi-omics tools and technologies have revolutionized crop improvement research for the development of abiotic stress-tolerant varieties. The potential of multi-omics approaches can be utilized to decipher the stress tolerance mechanisms governed by phyto-microbiome. Metagenomics and meta-transcriptomics offer huge potential to identify the complex microbial networks implicated in stress signaling and tolerance development. Genomics technologies enable high-throughput screening of beneficial microbes, leveraging gene modification and genetic engineering approaches for introducing abiotic stress resistance in plants. Plant microbiome engineering could be immensely beneficial in the development of strategies to improve plant health, enhance crop productivity, improve resistance against abiotic and biotic stresses, and achieve sustainable agriculture in an eco-friendly manner.
Author contributions
AbS did the conception, design, editing, finalization, and submission of manuscript. AnS, SM, SC, PG, and AbS did literature survey, analysis and preparation of draft of the manuscript. AnS, SM, SC, and PG did revision and redrafting. All authors contributed to the article and approved the submitted version.
Conflict of interest
The authors declare that the research was conducted in the absence of any commercial or financial relationships that could be construed as a potential conflict of interest.
Publisher’s note
All claims expressed in this article are solely those of the authors and do not necessarily represent those of their affiliated organizations, or those of the publisher, the editors and the reviewers. Any product that may be evaluated in this article, or claim that may be made by its manufacturer, is not guaranteed or endorsed by the publisher.
References
Afridi, M. S., Ali, S., Salam, A., César Terra, W., Hafeez, A., Sumaira, A., et al. (2022b). Plant microbiome engineering: hopes or hypes. Biol. 11:1782. doi: 10.3390/biology11121782
Afridi, M. S., Javed, M. A., Ali, S., de Medeiros, F. H. V., Ali, B., Salam, A., et al. (2022a). New opportunities in plant microbiome engineering for increasing agricultural sustainability under stressful conditions. Front. Plant Sci. 13:899464. doi: 10.3389/fpls.2022.899464
Afzal, I., Shinwari, Z. K., Sikandar, S., and Shahzad, S. (2019). Plant beneficial endophytic bacteria: mechanisms, diversity, host range and genetic determinants. Microbiol. Res. 221, 36–49. doi: 10.1016/j.micres.2019.02.001
Ahmad, I., Akhtar, M., Asghar, H., Ghafoor, U., and Shahid, M. (2015). Differential effects of plant growth-promoting Rhizobacteria on maize growth and cadmium uptake. J. Plant Growth Regul. 35, 303–315. doi: 10.1007/s00344-015-9534-5
Ahmad, S., Chaudhary, H. J., and Damalas, C. A. (2022). Microbial detoxification of dimethoate through mediated hydrolysis by Brucella sp. PS4: molecular profiling and plant growth-promoting traits. Environ. Sci. Pollut. Res. 29, 2420–2431. doi: 10.1007/s11356-021-15806-1
Ahmad, P., Hashem, A., Abd-Allah, E. F., Alqarawi, A. A., John, R., Egamberdieva, D., et al. (2015). Role of Trichoderma harzianum in mitigating NaCl stress in Indian mustard (Brassica juncea L.) through antioxidative defense system. Front. Plant Sci. 6:868. doi: 10.3389/fpls.2015.00868
Al Kahtani, M. D. F., Fouda, A., Attia, K. A., Al-Otaibi, F., Eid, A. M., Ewais, E. D., et al. (2020). Isolation and characterization of plant growth promoting endophytic bacteria from desert plants and their application as bioinoculants for sustainable agriculture. Agronomy 10:1325. doi: 10.3390/agronomy10091325
Alawiye, T. T., and Babalola, O. O. (2019). Bacterial diversity and community structure in typical plant rhizosphere. Diversity 11:179. doi: 10.3390/d11100179
Ali, A., Altaf, M. T., Nadeem, M. A., Karaköy, T., Shah, A. N., Azeem, H., et al. (2022). Recent advancement in OMICS approaches to enhance abiotic stress tolerance in legumes. Front. Plant Sci. 13:952759. doi: 10.3389/fpls.2022.952759
Ali, S., Tyagi, A., Park, S., Mir, R. A., Mushtaq, M., Bhat, B., et al. (2022). Deciphering the plant microbiome to improve drought tolerance: mechanisms and perspectives. Environ. Exp. Bot. 201:104933. doi: 10.1016/j.envexpbot.2022.104933
Alok, D., Annapragada, H., Singh, S., Ghosh, P., Sengupta, A., Basu, D., et al. (2020). Symbiotic nitrogen fixation and endophytic bacterial community structure in Bt-transgenic chickpea (Cicer arietinum L). Sci. Rep. 10:5453. doi: 10.1038/s41598-020-62199-1
Anand, G., Bhattacharjee, A., Shrivas, V. L., Dubey, S., and Sharma, S. (2021). ACC deaminase positive Enterobacter-mediated mitigation of salinity stress, and plant growth promotion of Cajanus cajan: a lab to field study. Physiol. Mol. Biol. Plants 27, 1547–1557. doi: 10.1007/s12298-021-01031-0
Ansari, F. A., Ahmad, I., and Pichtel, J. (2019). Growth stimulation and alleviation of salinity stress to wheat by the biofilm forming Bacillus pumilus strain FAB10. Appl. Soil Ecol. 143, 45–54. doi: 10.1016/j.apsoil.2019.05.023
Anzano, A., Bonanomi, G., Mazzoleni, S., and Lanzotti, V. (2022). Plant metabolomics in biotic and abiotic stress: a critical overview. Phytochem. Rev. 21, 503–524. doi: 10.1007/s11101-021-09786-w
Aroca, R., Luiz-Lozano, J. M., Zamarreño, Á. M., Paz, J. A., García-Mina, J. M., Pozo, M. J., et al. (2013). Arbuscular mycorrhizal symbiosis influences strigolactone production under salinity and alleviates salt stress in lettuce plants. J. Plant Physiol. 170, 47–55. doi: 10.1016/j.jplph.2012.08.020
Arun, K. D., Sabarinathan, K. G., Gomathy, M., Kannan, R., and Balachandar, D. (2020). Mitigation of drought stress in rice crop with plant growth-promoting abiotic stress-tolerant rice phyllosphere bacteria. J. Basic Microbiol. 60, 768–786. doi: 10.1002/jobm.202000011
Ashrafi, E., Zahedi, M., and Razmjoo, J. (2014). Co-inoculations of arbuscular mycorrhizal fungi and rhizobia under salinity in alfalfa. Soil Sci. Plant Nutr. 60, 619–629. doi: 10.1080/00380768.2014.936037
Aslam, M. M., Waseem, M., Jakada, B. H., Okal, E. J., Lei, Z., Sohaib, H., et al. (2022). Mechanisms of abscisic acid-mdiated drought stress responses in plants. Int. J.Mol Sci. 19, 23:1084. doi: 10.3390/ijms23031084
Avilés-García, M. E., Flores-Cortez, I., Hernández-Soberano, C., Santoyo, G., and Valencia-Cantero, E. (2016). La rizobacteria promotora del crecimiento vegeta: Arthrobacter agilis UMCV2 coloniza endofíticamente a Medicago truncatula. Rev. Argent. Microbiol. 48, 342–346. doi: 10.1016/j.ram.2016.07.004
Awasthy, S., Kumar, S. R., and Sivakumar, U. (2017). Mitigation of drought in rice by a phyllosphere bacterium Bacillus altitudinis FD48. Afr. J. Microbiol. Res. 11, 1614–1625. doi: 10.5897/AJMR2017.8610
Ayangbenro, A. S., and Babalola, O. O. (2017). A new strategy for heavy metal polluted environments: a review of microbial biosorbents. Int. J. Environ. Res. Public Health 14:94. doi: 10.3390/ijerph14010094
Backer, R., Rokem, J. S., Ilangumaran, G., Lamont, J., Praslickova, D., Ricci, E., et al. (2018). Plant growth-promoting rhizobacteria: context, mechanisms of action, and roadmap to commercialization of biostimulants for sustainable agriculture. Front. Plant Sci. 9:1473. doi: 10.3389/fpls.2018.01473
Baltruschat, H., Fodor, J., Harrach, B. D., Niemczyk, E., Barna, B., Gullner, G., et al. (2008). Salt tolerance of barley induced by the root endophyte Piriformospora indica is associated with a strong increase in anti-oxidants. New Phytol. 180, 501–510. doi: 10.1111/j.1469-8137.2008.02583.x
Barea, J. M. (2015). Future challenges and perspectives for applying microbial biotechnology in sustainable agriculture based on a better understanding of plant-microbiome interactions. J. Soil Sci. and Plant Nutr. 15, 261–282. doi: 10.4067/S0718-95162015005000021
Batool, T., Ali, S., Seleiman, M. F., Naveed, N. H., Ali, A., Ahmed, K., et al. (2020). Plant growth promoting rhizobacteria alleviates drought stress in potato in response to suppressive oxidative stress and antioxidant enzymes activities. Sci. Rep. 10:16975. doi: 10.1038/s41598-020-73489-z
Bedano, J. C., Vaquero, F., Domínguez, A., Rodríguez, M. P., Wall, L., and Lavelle, P. (2019). Earthworms contribute to ecosystem process in no-till Systems with high crop rotation intensity in Argentina. Acta Oecol. 98, 14–24. doi: 10.1016/j.actao.2019.05.003
Begcy, K., Sandhu, J., and Walia, H. (2018). Transient heat stress during early seed development primes germination and seedling establishment in rice. Front. Plant Sci. 9:1768. doi: 10.3389/fpls.2018.01768
Begum, Y. (2022). Regulatory role of microRNAs (miRNAs) in the recent development of abiotic stress tolerance of plants. Gene 821:146283. doi: 10.1016/j.gene.2022.146283
Belimov, A. A., Dodd, I. C., Hontzeas, N., Theobald, J. C., Safronova, V. I., and Davies, W. J. (2009). Rhizosphere bacteria containing 1-aminocyclopropane1-carboxylate deaminase increase yield of plants grown in drying soil via both local and systemic hormone signalling. New Phytol. 181, 413–423. doi: 10.1111/j.1469-8137.2008.02657.x
Ben Mahmoud, O. M., Hidri, R., Talbi-Zribi, O., Taamalli, W., Abdelly, C., and Djébali, N. (2020). Auxin and proline producing rhizobacteria mitigate salt-induced growth inhibition of barley plants by enhancing water and nutrient status. S. Afr. J. Bot. 128, 209–217. doi: 10.1016/j.sajb.2019.10.023
Benidire, L., El Khalloufi, F., Oufdou, K., Barakat, M., Tulumello, J., Ortet, P., et al. (2020). Phytobeneficial bacteria improve saline stress tolerance in Vicia faba and modulate microbial interaction network. Sci. Total Environ. 729:139020. doi: 10.1016/j.scitotenv.2020.139020
Bernardo, L., Carletti, P., Badeck, F. W., Rizza, F., Morcia, C., Ghizzoni, R., et al. (2019). Metabolomic responses triggered by arbuscular mycorrhiza enhance tolerance to water stress in wheat cultivars. Plant Physiol. Biochem. 137, 203–212. doi: 10.1016/j.plaphy.2019.02.007
Brasileiro, A. C. M., Morgante, C. V., Araujo, A. C. G., Leal-Bertioli, S. C. M., Silva, A. K., Martins, A. C. Q., et al. (2015). Transcriptome profiling of wild Arachis from water-limited environments uncovers drought tolerance candidate genes. Plant Mol. Biol. Rep. 33, 1876–1892. doi: 10.1007/s11105-015-0882-x
Brotman, Y., Landau, U., Cuadros-Inostroza, Á., Takayuki, T., Fernie, A. R., Chet, I., et al. (2013). Trichoderma-plant root colonization: escaping early plant defense responses and activation of the antioxidant machinery for saline stress tolerance. PLoS Pathog. 9:e1003221. doi: 10.1371/journal.ppat.1003221
Bruno, L. B., Karthik, C., Ma, Y., Kadirvelu, K., Freitas, H., and Rajkumar, M. (2020). Amelioration of chromium and heat stresses in Sorghum bicolor by Cr(6þ) reducing thermos tolerant plant growth promoting bacteria. Chemosphere 244:125521. doi: 10.1016/j.chemosphere.2019.125521
Cabral, C., Sabine, R., Ivanka, T., and Bernd, W. (2016). Arbuscular mycorrhizal fungi modify nutrient allocation and composition in wheat (Triticum aestivum L.) subjected to heat-stress. Plant Soil 408, 385–399. doi: 10.1007/s11104-016-2942-x
Chandrasekaran, M., Boopathi, T., and Manivannan, P. (2021). Comprehensive assessment of ameliorative effects of AMF in alleviating abiotic stress in tomato plants. J Fungi 7:303. doi: 10.3390/jof7040303
Chang, P., Gerhardt, K. E., Huang, X.-D., Yu, X.-M., Glick, B. R., Gerwing, P. D., et al. (2014). Plant growthpromoting bacteria facilitate the growth of barley and oats in saltimpacted soil: implications for phytoremediation of saline soils. Int. J. Phytoremediation 16, 1133–1147. doi: 10.1080/15226514.2013.821447
Chen, L., Schwier, M., Krumbach, J., Kopriva, S., and Jacoby, R. P. (2021). Metabolomics in plant-microbe interactions in the roots. Adv. Bot. Res. 98, 133–161. doi: 10.1016/bs.abr.2020.09.018
Chen, G., Zheng, D., Feng, N., Zhou, H., Mu, D., Zhao, L., et al. (2022). Physiological mechanisms of ABA-induced salinity tolerance in leaves and roots of rice. Sci. Rep. 12:8228. doi: 10.1038/s41598-022-11408-0
Chialva, M., Lanfranco, L., and Bonfante, P. (2022). The plant microbiota: composition, functions, and engineering Curr. Opin. Biotechnol. 73, 135–142. doi: 10.1016/j.copbio.2021.07.003
Chihaoui, S. A., Trabelsi, D., Jdey, A., Mhadhbi, H., and Mhamdi, R. (2015). Inoculation of Phaseolus vulgaris with the nodule-endophyte agrobacterium sp. 10C2 affects richness and structure of rhizosphere bacterial communities and enhances nodulation and growth. Arch. Microbiol. 197, 805–813. doi: 10.1007/s00203-015-1118-z
Cho, S. M., Kang, B. R., Han, S. H., Anderson, A. J., Park, J. Y., Lee, Y. H., et al. (2008). 2R, 3R-butanediol, a bacterial volatile produced by Pseudomonas chlororaphis O6, is involved in induction of systemic tolerance to drought in Arabidopsis thaliana. Mol. Plant-Microbe Interact. 21, 1067–1075. doi: 10.1094/MPMI-21-8-1067
Chukwuneme, C. F., Babalola, O. O., Kutu, F. R., and Ojuederie, O. B. (2020). Characterization of actinomycetes isolates for plant growth promoting traits and their effects on drought tolerance in maize. J. Plant Interact. 15, 93–105. doi: 10.1080/17429145.2020.1752833
Compant, S., Marcel, G. A., Heijden, V. D., and Sessitsch, A. (2010). Climate change effects on beneficial plant–microorganism interactions. FEMS Microbiol. Ecol. 73, 197–214. doi: 10.1111/j.1574-6941.2010.00900.x
Crandall, S. G., Gold, K. M., Jiménez-Gasco, M. D. M., Filgueiras, C. C., and Willett, D. S. (2020). A multi-omics approach to solving problems in plant disease ecology. PLoS One 15:e0237975. doi: 10.1371/journal.pone.0237975
Dardanelli, M. S., Fernández de Córdoba, F. J., Espuny, M. R., Rodríguez Carvajal, M. A., Soria Díaz, M. E., Gil Serrano, A. M., et al. (2008). Effect of Azospirillum brasilense coinoculated with rhizobium on Phaseolus vulgaris flavonoids and nod factor production under salt stress. Soil Biol. Biochem. 40, 2713–2721. doi: 10.1016/j.soilbio.2008.06.016
Dastogeer, K. M. G., Zahan, M. I., Rhaman, M. S., Sarker, M. S. A., and Chakraborty, A. (2022). Microbe-mediated Thermotolerance in plants and pertinent mechanisms- a meta-analysis and review. Front. Microbiol. 13:511. doi: 10.3389/fmicb.2022.833566
Dawid, C., and Hille, K. (2018). Functional metabolomics–a useful tool to characterize stress-induced Metabolome alterations opening new avenues towards tailoring food crop quality. Agronomy 8:138. doi: 10.3390/agronomy8080138
Deokar, A. A., Kondawar, V., Jain, P. K., Karuppayil, M., Raju, N. L., Vadez, V., et al. (2011). Comparative analysis of expressed sequence tags (ESTs) between drought-tolerant and susceptible genotypes of chickpea under terminal drought stress. BMC Plant Biol. 11:70. doi: 10.1186/1471-2229-11-70
Dotaniya, M., and Saha, J. (2016). Heavy metal polluted soils in India: status and countermeasures. JNKVV Res. J. 49, 320–337.
Duc, N. H., Csintalan, Z., and Posta, K. (2018). Arbuscular mycorrhizal fungi mitigate negative effects of combined drought and heat stress on tomato plants. Plant Physiol. Biochem. 132, 297–307. doi: 10.1016/j.plaphy.2018.09.011
El-Esawi, M. A., Alaraidh, I. A., Alsahli, A. A., Alamri, S. A., Ali, H. M., and Alayafi, A. A. (2018). Bacillus firmus (SW5) augments salt tolerance in soybean (Glycine max L.) by modulating root system architecture, antioxidant defense systems and stress-responsive genes expression. Plant Physiol. Biochem. 132, 375–384. doi: 10.1016/j.plaphy.2018.09.026
El-Meihy, R., Abou-Aly, H., Youssef, A., Tewfike, T., and Elakshar, E. (2019). Efficiency of heavy metals-tolerant plant growth promoting bacteria for alleviating heavy metals toxicity on sorghum. Environ. Exp. Bot. 162, 295–301. doi: 10.1016/j.envexpbot.2019.03.005
Escudero-Martinez, C., and Bulgarelli, D. (2019). Tracing the evolutionary routes of plant-microbiota interactions. Curr. Opin. Microbiol. 49, 34–40. doi: 10.1016/j.mib.2019.09.013
Farooq, M., Wahid, A., Kobayashi, N., Fujita, D., and Basra, S. M. A. (2009). Plant drought stress: effects, mechanisms and management. Agron. Sustain. Dev. 29, 185–212. doi: 10.1051/agro:2008021
Farooqi, M. Q. U., Nawaz, G., Wani, S. H., Choudhary, J. R., Rana, M., Sah, R. P., et al. (2022). Recent developments in multi-omics and breeding strategies for abiotic stress tolerance in maize (Zea mays L.). Front. Plant Sci. 13:965878. doi: 10.3389/fpls.2022.965878
Ferreira, M. J., Silva, H., and Cunha, A. (2019). Siderophore-producing rhizobacteria as a promising tool for empowering plants to cope with iron limitation in saline soils: a review. Pedosphere 29, 409–420. doi: 10.1016/S1002-0160(19)60810-6
Friedrich, T., Oberkofler, V., Trindade, I., Altmann, S., Brzezinka, K., Lämke, J., et al. (2021). Heteromeric HSFA2/HSFA3 complexes drive transcriptional memory after heat stress in Arabidopsis. Nat. Commun. 12:23786. doi: 10.1038/s41467-021-23786-6
Gangola, M. P., and Ramadoss, B. R. (2020). “WRKY transcription factors for biotic and abiotic stress tolerance in plants” in Transcription factors for abiotic stress tolerance in plants. ed. S. H. Wani (Amsterdam: Elsevier), 15–28.
Gentile, A., Dias, L. I., Mattos, R. S., Ferreira, T. H., and Menossi, M. (2015). MicroRNAs and drought responses in sugarcane. Front. Plant Sci. 6:58. doi: 10.3389/fpls.2015.00058
Ghatak, A., Chaturvedi, P., and Weckwerth, W. (2018). Metabolomics in plant stress physiology. Adv. Biochem. Eng. Biotechnol. 164, 187–236. doi: 10.1007/10_2017_55
Ghorbanpour, A., Salimi, A., Ghanbary, M. A. T., Pirdashti, H., and Dehestani, A. J. S. H. (2018). The effect of Trichoderma harzianum in mitigating low temperature stress in tomato (Solanum lycopersicum L.) plants. Sci. Hortic. 230, 134–141. doi: 10.1016/j.scienta.2017.11.028
Glick, B. R. (2014). Bacteria with ACC deaminase can promote plant growth and help to feed the world. Microbiol. Res. 169, 30–39. doi: 10.1016/j.micres.2013.09.009
Goswami, M., and Deka, S. (2020). Plant growth-promoting rhizobacteria-alleviators of abiotic stresses in soil: a review. Pedosphere 30, 40–61. doi: 10.1016/S1002-0160(19)60839-8
Grover, M., Bodhankar, S., Maheswari, M., and Srinivasarao, C. (2016). “Actinomycetes as mitigators of climate change and abiotic stress” in Plant growth promoting Actinobacteria. eds. G. Subramaniam, S. Arumugam, and V. Rajendran (Springer: Singapore), 203–212.
Haghighi, M., Mozafariyan, M., and Abdolahipour, B. (2015). Effect of cucumber mycorrhiza inoculation under low and high root temperature grown on hydroponic conditions. J. Crop. Sci. Biotechnol. 18, 89–96. doi: 10.1007/s12892-014-0083-4
Hahm, M. S., Son, J. S., Hwang, Y. J., Kwon, D. K., and Ghim, S. Y. (2017). Alleviation of salt stress in pepper (Capsicum annum L.) plants by plant growth-promoting rhizobacteria. J. Microbiol. Biotechnol. 27, 1790–1797. doi: 10.4014/jmb.1609.09042
Hajiboland, R., Joudmand, A., Aliasgharzad, N., Tolrá, R., and Poschenrieder, C. (2019). Arbuscular mycorrhizal fungi alleviate low-temperature stress and increase freezing resistance as a substitute for acclimation treatment in barley. Crop Pasture Sci. 70, 218–233. doi: 10.1071/CP18385
Hakim, S., Naqqash, T., Nawaz, M. S., Laraib, I., Siddique, M. J., Zia, R., et al. (2021). Rhizosphere engineering with plant growth-promoting microorganisms for agriculture and ecological sustainability. Front. Sustain. Food Syst. 5:617157. doi: 10.3389/fsufs.2021.617157
Haraguchi, S., and Yoshiga, T. (2020). Potential of the fungal feeding nematode Aphelenchus avenae to control fungi and the plant parasitic nematode Ditylenchus destructor associated with garlic. Biol. Control 143:104203. doi: 10.1016/j.biocontrol.2020.104203
Harun-Or-Rashid, M., and Chung, Y. R. (2017). Induction of systemic resistance against insect herbivores in plants by beneficial soil microbes. Front. Plant Sci. 8:816. doi: 10.3389/fpls.2017.01816
Hasanuzzaman, M., Bhuyan, M. H. M. B., Zulfiqar, F., Raza, A., Mohsin, S. M., Al Mahmud, J., et al. (2020). Reactive oxygen species and antioxidant defense in plants under abiotic stress: revisiting the crucial role of a universal defense regulator. Antioxidants 9, 1–52. doi: 10.3390/antiox9080681
Hasanuzzaman, M., and Fujita, M. (2022). Plant responses and tolerance to salt stress: physiological and molecular interventions. Int. J. Mol. Sci. 23:4810. doi: 10.3390/ijms23094810
Hasanuzzaman, M., Nahar, K., Alam, M. M., Roychowdhury, R., and Fujita, M. (2013). Physiological, biochemical, and molecular mechanisms of heat stress tolerance in plants. Int. J. Mol. Sci. 14, 9643–9684. doi: 10.3390/ijms14059643
Hashem, A., Alqarawi, A. A., Radhakrishnan, R., Al-Arjani, A. F., Aldehaish, H. A., Egamberdieva, D., et al. (2018). Arbuscular mycorrhizal fungi regulate the oxidative system, hormones and ionic equilibrium to trigger salt stress tolerance in Cucumis sativus L. Saudi J. Biol. Sci. 25, 1102–1114. doi: 10.1016/j.sjbs.2018.03.009
Hassan, M., Chattha, M., Khan, I., Chattha, M., Barbanti, L., Aamer, M., et al. (2020). Heat stress in cultivated plants: nature, impact, mechanisms, and mitigation strategies - a review. Plant Biosyst. 155, 1–56. doi: 10.1080/11263504.2020.1727987
Hayat, R., Ali, S., Amara, U., Ali, U., Amara, R., Khalid, I., et al. (2010). Soil beneficial bacteria and their role in plant growth promotion: a review. Ann. Microbiol. 60, 579–598. doi: 10.1007/s13213-010-0117-1
Hünninghaus, M., Koller, R., Kramer, S., Marhan, S., Kandeler, E., and Bonkowski, M. (2017). Changes in bacterial community composition and soil respiration indicate rapid successions of protist grazers during mineralization of maize crop residues. Pedobiologia 62, 1–8. doi: 10.1016/j.pedobi.2017.03.002
Iqbal, S., Wang, X., Mubeen, I., Kamran, M., Kanwal, I., Díaz, G. A., et al. (2022). Phytohormones trigger drought tolerance in crop plants: outlook and future perspectives. Front. Plant Sci. 12:799318. doi: 10.3389/fpls.2021.799318
Islam, F., Yasmeen, T., Ali, S., Ali, D., Hameed, S., Zhou, W., et al. (2016). Plant growth promoting bacteria confer salt tolerance in Vigna radiata by up-regulating antioxidant defense and biological soil fertility. Plant Growth Regul. 80, 23–26. doi: 10.1007/s10725-015-0142-y
Jaber, L. R., and Alananbeh, K. M. (2018). Fungal entomopathogens as endophytes reduce several species of Fusarium causing crown and root rot in sweet pepper (Capsicum annuum L.). Biol. Control 126, 117–126. doi: 10.1016/j.biocontrol.2018.08.007
Jacob, P., Hirt, H., and Bendahmane, A. (2017). The heat-shock protein/ chaperone network and multiple stress resistance. Plant Biotechnol. J. 15, 405–414. doi: 10.1111/pbi.12659
Janiak, A., Kwaśniewsk, M., and Szarejko, I. (2016). Gene expression regulation in roots under drought. J. Exp. Bot. 67, 1003–1014. doi: 10.1093/jxb/erv512
Javaid, M. M., Florentine, S., Mahmood, A., Wasaya, A., Javed, T., Sattar, A., et al. (2022). Interactive effect of elevated CO2 and drought on physiological traits of Datura stramonium. Front. Plant Sci. 13:929378. doi: 10.3389/fpls.2022.929378
Jeong, D. H., Park, S., Zhai, J., Gurazada, S. G., De Paoli, E., Meyers, B. C., et al. (2011). Massive analysis of rice small RNAs: mechanistic implications of regulated microRNAs and variants for differential target RNA cleavage. Plant Cell 23, 4185–4207. doi: 10.1105/tpc.111.089045
Jha, U. C., Bohra, A., Jha, R., and Parida, S. K. (2019). Salinity stress response and ‘omics’ approaches for improving salinity stress tolerance in major grain legumes. Plant Cell Rep. 38, 255–277. doi: 10.1007/s00299-019-02374-5
Jha, Y., and Mohamed, H. I. (2022). Inoculation with Lysinibacillus fusiformis strain YJ4 and Lysinibacillus sphaericus strain YJ5 alleviates the effects of cold stress in maize plants. Gesunde Pflanz. 75, 77–95. doi: 10.1007/s10343-022-00666-7
Jiang, Q. Y., Zhuo, F., Long, S. H., Zhao, H. D., Yang, D. J., Ye, Z. H., et al. (2016). Can arbuscular mycorrhizal fungi reduce cd uptake and alleviate cd toxicity of Lonicera japonica grown in cd-added soils? Sci. Rep. 6:21805. doi: 10.1038/srep21805
Jin, P., Tan, Z., Wang, H., Liu, W., and Miao, W. (2021). Antimicrobial effect of Bacillus licheniformis HN-5 bacitracin a on rice pathogen Pantoea ananatis. Biol. Control 66, 249–257. doi: 10.1007/s10526-020-10052-9
Johnston-Monje, D., and Raizada, M. N. (2011). Conservation and diversity of seed associated endophytes in Zea across boundaries of evolution, ethnography and ecology. PLoS One 6:e20396. doi: 10.1371/journal.pone.0020396
Joshi, H., Shourie, A., and Singh, A. (2020). Cyanobacteria as a source of biofertilizers for sustainable agriculture. In Singh, P. K., Kumar, A., Singh, V. K., and Shrivistava, A. K., Advances in cyanobacterial biology (pp. 385–396). Cambrigge, MA: Academic Press.
Kakar, K., Ren, X. L., Nawaz, Z., Cui, Z., Li, B., Xie, G., et al. (2015). Consortium of Rhizobacterial strains and biochemical growth elicitors improve cold and drought stress tolerance in rice (Oryza Sativa L.). Plant Biol. 3, 471–483. doi: 10.1111/plb.12427
Karthik, C., Oves, M., Thangabalu, R., Sharma, R., Santhosh, S. B., and Indra Arulselvi, P. (2016). Cellulosimicrobium funkei-like enhances the growth of Phaseolus vulgaris by modulating oxidative damage under chromium (VI) toxicity. J. Adv. Res. 7, 839–850. doi: 10.1016/j.jare.2016.08.007
Katam, R., Lin, C., Grant, K., Katam, C. S., and Chen, S. (2022). Advances in plant metabolomics and its applications in stress and single-cell biology. Int. J. Mol. Sci. 23:6985. doi: 10.3390/ijms23136985
Katano, K., Honda, K., and Suzuki, N. (2018). Integration between ROS regulatory systems and other signals in the regulation of various types of heat responses in plants. Int. J. Mol. Sci. 19:3370. doi: 10.3390/ijms19113370
Kaul, S., Choudhary, M., Gupta, S., and Dhar, M. K. (2021). Engineering host microbiome for crop improvement and sustainable agriculture. Front. Microbiol. 28:635917. doi: 10.3389/fmicb.2021.635917
Kaushal, M., and Wani, S. P. (2016). Plant-growth-promoting rhizobacteria: drought stress alleviators to ameliorate crop production in drylands. Ann. Microbiol. 66, 35–42. doi: 10.1007/s13213-015-1112-3
Keyster, M., Niekerk, L. A., Basson, G., Carelse, M., Bakare, O., Ludidi, N., et al. (2020). Decoding heavy metal stress signalling in plants: towards improved food security and safety. Plan. Theory 9, 1–26. doi: 10.3390/plants9121781
Khan, M. A., Asaf, S., Khan, A. L., Adhikari, A., Jan, R., Ali, S., et al. (2020). Plant growth-promoting endophytic bacteria augment growth and salinity tolerance in rice plants. Plant Biol. 22, 850–862. doi: 10.1111/plb.13124
Khan, M. A., Asaf, S., Khan, A. L., Ullah, I., Ali, S., Kang, S. M., et al. (2019). Alleviation of salt stress response in soybean plants with the endophytic bacterial isolate Curtobacterium sp. SAK1. Ann. Microbiol. 69, 797–808. doi: 10.1007/s13213-019-01470-x
Khan, A. L., Kang, S. M., Dhakal, K. H., Hussain, J., Adnan, M., Kim, J. G., et al. (2013). Flavonoids and amino acid regulation in Capsicum annuum L. by endophytic fungi under different heat stress regimes. Sci. Hortic. 155, 1–7. doi: 10.1016/j.scienta.2013.02.028
Khan, A., Khan, V., Pandey, K., Sopory, S. K., and Sanan-Mishra, N. (2022). Thermo-priming mediated cellular networks for abiotic stress management in plants. Front. Plant Sci. 13:866409. doi: 10.3389/fpls.2022.866409
Khan, A., Sirajuddin, Z., Zhao, X. Q., Javed, M. T., Khan, K. S., Bano, A., et al. (2016). Bacillus pumilus enhances tolerance in rice (Oryza sativa L.) to combined stresses of NaCl and high boron due to limited uptake of Na+. Environ. Exp. Bot. 124, 120–129. doi: 10.1016/j.envexpbot.2015.12.011
Khatabi, B., Gharechahi, J., Ghaffari, M. R., Liu, D., Haynes, P. A., McKay, M. J., et al. (2019). Plant-microbe symbiosis: what has proteomics taught us? Proteomics 19:e1800105. doi: 10.1002/pmic.201800105
Kissoudis, C., Kalloniati, C., Flemetakis, E., Madesis, P., Labrou, N. E., Tsaftaris, A., et al. (2015). Stress-inducible GmGSTU4 shapes transgenic tobacco plants metabolome towards increased salinity tolerance. Acta Physiol. Plant. 37, 1–11. doi: 10.1007/s11738-015-1852-5
Köhl, J., Medeiros, F. H., Lombaers-van der Plas, C., Groenenboom-de Haas, L., and van den Bosch, T. (2020). Efficacies of bacterial and fungal isolates in biocontrol of Botrytis cinerea and Pseudomonas syringae pv. Tomato and growth promotion in tomato do not correlate. Biol. Control 150:104375. doi: 10.1016/j.biocontrol.2020.104375
Kong, A. Y. Y., and Six, J. (2012). Microbial community assimilation of cover crop rhizodeposition within soil microenvironments in alternative and conventional cropping systems. Plant Soil 356, 315–330. doi: 10.1007/s11104-011-1120-4
Krasensky, J., and Jonak, C. (2012). Drought, salt, and temperature stress-induced metabolic rearrangements and regulatory networks. J. Exp. Bot. 63, 1593–1608. doi: 10.1093/jxb/err460
Krome, K., Rosenberg, K., Dickler, C., Kreuzer, K., Ludwig-Müller, J., Ullrich-Eberius, C., et al. (2010). Soil bacteria and protozoa affect root branching via effects on the Auxin and Cytokinin balance in plants. Plant Soil 328, 191–201. doi: 10.1007/s11104-009-0101-3
Kumar, P., Choudhary, M., Halder, T., Prakash, N. R., Singh, V., Sheoran, S., et al. (2022). Salinity stress tolerance and omics approaches: revisiting the progress and achievements in major cereal crops. Heredity 128, 497–518. doi: 10.1038/s41437-022-00516-2
Kumar, P., and Sharma, P. K. (2020). Soil salinity and food security in India. Front. Sustain. Food Syst. 4:533781. doi: 10.3389/fsufs.2020.533781
Kumar, A., Singh, S., Gaurav, A. K., Srivastava, S., and Verma, J. P. (2020). Plant growth-promoting bacteria: biological tools for the mitigation of salinity stress in plants. Front. Microbiol. 11:1216. doi: 10.3389/fmicb.2020.01216
Kurniawan, S. B., Ramli, N. N., Said, N. S. M., Alias, J., Imron, M. F., Abdullah, S. R. S., et al. (2022). Practical limitations of bioaugmentation in treating heavy metal contaminated soil and role of plant growth promoting bacteria in phytoremediation as a promising alternative approach. Heliyon 8:e08995. doi: 10.1016/j.heliyon.2022.e08995
Kuromori, T., Fujita, M., Takahashi, F., Yamaguchi-Shinozaki, K., and Shinozaki, K. (2022). Inter-tissue and inter-organ signaling in drought stress response and phenotyping of drought tolerance. Plant J. 109, 342–358. doi: 10.1111/tpj.15619
Kuske, C. R., Hesse, C. N., Challacombe, J. F., Cullen, D., Herr, J. R., Mueller, R. C., et al. (2015). Prospects and challenges for fungal metatranscriptomics of complex communities. Fungal Ecol. 14, 133–137. doi: 10.1016/j.funeco.2014.12.005
Lareen, A., Burton, F., and Schäfer, P. (2016). Plant root-microbe communication in shaping root microbiomes. Plant Mol. Biol. 90, 575–587. doi: 10.1007/s11103-015-0417-8
Lau, E. T., Tani, A., Khew, C. Y., Chua, Y. Q., and San Hwang, S. (2020). Plant growth-promoting bacteria as potential bio-inoculants and biocontrol agents to promote black pepper plant cultivation. Microbiol. Res. 240:126549. doi: 10.1016/j.micres.2020.126549
Lau, S. E., Teo, W. F. A., Teoh, E. Y., and Tan, B. C. (2022). Microbiome engineering and plant biostimulants for sustainable crop improvement and mitigation of biotic and abiotic stresses. Discover Food 2:9. doi: 10.1007/s44187-022-00009-5
Le, D. T., Nishiyama, R., Watanabe, Y., Tanaka, M., Seki, M., Ham, L. H., et al. (2012). Differential gene expression in soybean leaf tissues at late developmental stages under drought stress revealed by genome-wide transcriptome analysis. PLoS One 7:e49522. doi: 10.1371/journal.pone.0049522
Levy, A., Conway, J. M., Dangl, J. L., and Woyke, T. (2018). Elucidating bacterial gene functions in the plant microbiome. Cell Host Microbe 24, 475–485. doi: 10.1016/j.chom.2018.09.005
Liu, H. H., Tian, X., Li, Y. J., Wu, C. A., and Zheng, C. C. (2008). Microarray-based analysis of stress-regulated microRNAs in Arabidopsis thaliana. RNA 14, 836–843. doi: 10.1261/rna.895308
Lu, X., Jin, C., Yang, J., Liu, Q., Wu, S., Li, D., et al. (2013). Prenatal and lactational Lead exposure enhanced oxidative stress and altered apoptosis status in offspring rats’ hippocampus. Biol. Trace Elem. Res. 151, 75–84. doi: 10.1007/s12011-012-9531-5
Lv, D. K., Bai, X., Li, Y., Ding, X. D., Ge, Y., Cai, H., et al. (2010). Profiling of cold-stress-responsive miRNAs in rice by microarrays. Gene 459, 39–47. doi: 10.1016/j.gene.2010.03.011
Ma, Y., Rajkumar, M., Rocha, I., Oliveira, R. S., and Freitas, H. (2015). Serpentine bacteria influence metal translocation and bioconcentration of Brassica juncea and Ricinus communis grown in multi-metal polluted soils. Front. Plant Sci. 5:757. doi: 10.3389/fpls.2014.00757
Magallon-Servin, P., Antoun, H., Taktek, S., Taktek, S., and De-Bashan, L. E. (2020). Designing a multi-species inoculant of phosphate rock-solubilizing bacteria compatible with arbuscular mycorrhizae for plant growth promotion in low-P soil amended with PR. Biol. Fertil. Soils 56, 521–536. doi: 10.1007/s00374-020-01452-1
Mahmud, K., Missaoui, A., Lee, K., Ghimire, B., Presley, H. W., and Makaju, S. (2021). Rhizosphere microbiome manipulation for sustainable crop production. Curr. Plant Biol. 27:100210. doi: 10.1016/j.cpb.2021.100210
Marcelino, V. R., Irinyi, L., Eden, J. S., Meyer, W., Holmes, E. C., and Sorrell, T. C. (2019). Metatranscriptomics as a tool to identify fungal species and subspecies in mixed communities–a proof of concept under laboratory conditions. IMA Fungus. 10:12. doi: 10.1186/s43008-019-0012-8
Mathur, S., and Jajoo, A. (2020). Arbuscular mycorrhizal fungi protects maize plants from high temperature stress by regulating photosystem II heterogeneity. Ind. Crop. Prod. 143:111934. doi: 10.1016/j.indcrop.2019.111934
Meena, K. K., Kumar, M., Kalyuzhnaya, M. G., Yandigeri, M. S., Singh, D. P., Saxena, A. K., et al. (2012). Epiphytic pink-pigmented methylotrophic bacteria enhance germination and seedling growth of wheat (Triticum aestivum) by producing phytohormone. Anton. Van Leeuw. 101, 777–786. doi: 10.1007/s10482-011-9692-9
Mehmood, S., Khatoon, Z., Ahmad, I. A., Muneer, M. A., Kamran, M. A., et al. (2021). Bacillus sp. PM31 harboring various plant growth-promoting activities regulates Fusarium dry rot and wilt tolerance in potato. Arch. Agron. Soil Sci. 2021, 1–15. doi: 10.1080/03650340.2021.1971654
Mitra, S., Pramanik, K., Sarkar, A., Ghosh, P., Soren, T., and Maiti, T. (2018). Bioaccumulation of cadmium by Enterobacter sp. and enhancement of rice seedling growth under cadmium stress. Ecotoxicol. Environ. Saf. 156, 183–196. doi: 10.1016/j.ecoenv.2018.03.001
Mitter, B. N., Pfaffenbichler, R., Flavell, S., Compant, L., Antonielli, A., Petric, A., et al. (2017). A new approach to modify plant microbiomes and traits by introducing beneficial bacteria at flowering into progeny seeds. Front. Microbiol. 8:11. doi: 10.3389/fmicb.2017.00011
Moldovan, D., Spriggs, A., Yang, J., Pogson, B. J., Dennis, E. S., and Wilson, I. W. (2010). Hypoxia-responsive microRNAs and trans-acting small interfering RNAs in Arabidopsis. J. Exp. Bot. 61, 165–177. doi: 10.1093/jxb/erp296
Mueller, U. G., and Sachs, J. L. (2015). Engineering microbiomes to improve plant and animal health. Trends Microbiol. 23, 606–617. doi: 10.1016/j.tim.2015.07.009
Mukherjee, P., Mitra, A., and Roy, M. (2019). Halomonas rhizobacteria of Avicennia marina of Indian Sundarbans promote rice growth under saline and heavy metal stresses through exopolysaccharide production. Front. Microbiol. 10:1207. doi: 10.3389/fmicb.2019.01207
Mukhtar, T., Ali, F., Rafique, M., Ali, J., Afridi, M. S., Smith, D., et al. (2022). Biochemical characterization and potential of Bacillus safensis strain SCAL1 to mitigate heat stress in Solanum lycopersicum L. J. Plant Growth Regul. 139, 569–577. doi: 10.1007/s00344-021-10571-4
Mukhtar, T., Ur Rehman, S., Smith, D., Sultan, T., Seleiman, M. F., Alsadon, A. A., et al. (2020). Mitigation of heat stress in Solanum lycopersicum L. by ACC-deaminase and exopolysaccharide producing Bacillus cereus: effects on biochemical profiling. Sustainability. 12:2159. doi: 10.3390/su12062159
Mukhtar, S., Zareen, M., Khaliq, Z., Mehnaz, S., and Malik, K. A. (2020). Phylogenetic analysis of halophyte-associated rhizobacteria and effect of halotolerant and halophilic phosphate-solubilizing biofertilizers on maize growth under salinity stress conditions. J. Appl. Microbiol. 128, 556–573. doi: 10.1111/jam.14497
Murali, M., Singh, S. B., Gowtham, H. G., Shilpa, N., Prasad, M., Aiyaz, M., et al. (2021). Induction of drought tolerance in Pennisetum glaucum by ACC deaminase producing PGPR- Bacillus amyloliquefaciens through antioxidant defence system. Microbiol. Res. 253:126891. doi: 10.1016/j.micres.2021.126891
Nadeem, S. M., Zahir, Z. A., Naveed, M., and Arshad, M. (2007). Preliminary investigations on inducing salt tolerance in maize through inoculation with rhizobacteria containing ACC deaminase activity. Can. J. Microbiol. 53, 1141–1149. doi: 10.1139/W07-081
Naveed, M., Hussain, M. B., Zahir, Z. A., Mitter, B., and Sessitsch, A. (2014a). Drought stress amelioration in wheat through inoculation with Burkholderia phytofirmans strain PsJN. Plant Growth Regul. 73, 121–131. doi: 10.1007/s10725-013-9874-8
Naveed, M., Mitter, B., Reichenauer, T. G., Wieczorek, K., and Sessitsch, A. (2014b). Increased drought stress resilience of maize through endophytic colonization by Burkholderia phytofirmans PsJN and Enterobacter sp FD17. Environ. Exp. Bot. 97, 30–39. doi: 10.1016/j.envexpbot.2013.09.014
Naveed, M., Mustafa, A., Majeed, S., Naseem, Z., Saeed, Q., Khan, A., et al. (2020). Enhancing cadmium tolerance and pea plant health through Enterobacter sp. MN17 inoculation together with biochar and gravel sand. Plan. Theory 9:530. doi: 10.3390/plants9040530
Nazli, F., Mustafa, A., Ahmad, M., Hussain, A., Jamil, M., Wang, X., et al. (2020). A review on practical application and potentials of phytohormone-producing plant growthpromoting rhizobacteria for inducing heavy metal tolerance in crops. Sustainability 12:9056. doi: 10.3390/su12219056
Nelson, D. E., Repetti, P. P., Adams, T. R., Creelman, R. A., Wu, J., Warner, D. C., et al. (2007). Plant nuclear factor Y (NF-Y) B subunits confer drought tolerance and lead to improved corn yields on water-limited acres. Proc. Natl. Acad. Sci. U. S. A. 104, 16450–16455. doi: 10.1073/pnas.0707193104
Ngumbi, E., and Kloepper, J. (2016). Bacterial-mediated drought tolerance: current and future prospects. Appl. Soil Ecol. 105, 109–125. doi: 10.1016/j.apsoil.2016.04.009
Nilsson, R. H., Anslan, S., Bahram, M., Wurzbacher, C., Baldrian, P., and Tedersoo, L. (2019). Mycobiome diversity: high-throughput sequencing and identification of fungi. Nat. Rev. Microbiol. 17, 95–109. doi: 10.1038/s41579-018-0116-y
Oliveira, C. A., Alves, V. M. C., Marriel, I. E., Gomes, E. A., Scotti, M. R., Carneiro, N. P., et al. (2009). Phosphate solubilizing microorganisms isolated from rhizosphere of maize cultivated in an oxisol of the Brazilian Cerrado biome. Soil Biol. Biochem. 41, 1782–1787. doi: 10.1016/j.soilbio.2008.01.012
Omar, M. N. A., Osman, M. E. H., Kasim, W. A., and Abd El-Daim, I. A. (2009). Improvement of salt tolerance mechanisms of barley cultivated under salt stress using Azospirillum brasiliense. Tasks Veg. Sci. 44, 133–147. doi: 10.1007/978-1-4020-9065-3_15
Omer, A. M., Emara, H. M., Zaghloul, R. A., Abdel-Monem, M. O., and Dawwam, G. E. (2016). Potential of Azotobacter salinestris as plant growth promoting rhizobacteria under saline stress conditions. Res. J. Pharm. Biol. Chem. Sci. 7, 2572–2583.
Orozco-Mosqueda, M. D. C., Rocha-Granados, M. D. C., Bernard, R. G., and Santoyo, G. (2018). Microbiome engineering to improve biocontrol and plant growth-promoting mechanisms. Microbiol. Res. 208, 25–31. doi: 10.1016/j.micres.2018.01.005
Ortiz, N., Armadaa, E., Duque, E., Roldánc, A., and Azcóna, R. (2015). Contribution of arbuscular mycorrhizal fungi and/or bacteria to enhancing plant drought tolerance under natural soil conditions: effectiveness of autochthonous or allochthonous strains. J. Plant Physiol. 174, 87–96. doi: 10.1016/j.jplph.2014.08.019
Oubohssaine, M., Sbabou, L., and Aurag, J. (2022). Native heavy metal-tolerant plant growth promoting rhizobacteria improves Sulla spinosissima (L.) growth in post-mining contaminated soils. Microorganisms. 10:50838. doi: 10.3390/microorganisms10050838
Pal, K. K., Dey, R., Sherathia, D. N., Devidayal, M., Mangalassery, S., Kumar, A., et al. (2021). Alleviation of salinity stress in peanut by application of endophytic bacteria. Front. Microbiol. 12:650771. doi: 10.3389/fmicb.2021.650771
Palaniyandi, S. A., Damodharan, K., Yang, S. H., and Suh, J. W. (2014). Streptomyces sp. strain PGPA39 alleviates salt stress and promotes growth of ‘micro tom’ tomato plants. J. Appl. Microbiol. 117, 766–773. doi: 10.1111/jam.12563
Pandey, V., Ansari, M. W., Tula, S., Yadav, S., Sahoo, R. K., Shukla, N., et al. (2016). Dose-dependent response of Trichoderma harzianum in improving drought tolerance in rice genotypes. Planta 243, 1251–1264. doi: 10.1007/s00425-016-2482-x
Pandey, A. K., Rubiales, D., Wang, Y., Fang, P., Sun, T., Liu, N., et al. (2021). Omics resources and omics-enabled approaches for achieving high productivity and improved quality in pea (Pisum Sativum L.). Theor. Appl. Genet. 134, 755–776. doi: 10.1007/s00122-020-03751-5
Panke-Buisse, K., Poole, A. C., Goodrich, J. K., Ley, R. E., and Kao-Kniffin, J. (2015). Selection on soil microbiomes reveals reproducible impacts on plant function. ISME J. 9, 980–989. doi: 10.1038/ismej.2014.196
Panlada, T., Pongdet, P., Aphakorn, L., Rujirek, N.-N., Nantakorn, B., and Neung, T. (2013). Alleviation of the effect of environmental stresses using co-inoculation of mungbean by Bradyrhizobium and rhizobacteria containing stress-induced ACC deaminase enzyme. Soil Sci. Plant Nutr. 59, 559–571. doi: 10.1080/00380768.2013.804391
Parnell, J. J., Berka, R., Young, H. A., Sturino, J. M., Kang, Y., Barnhart, D. M., et al. (2016). From the lab to the farm: an industrial perspective of plant beneficial microorganisms. Front. Plant Sci. 7:1110. doi: 10.3389/fpls.2016.01110
Pascale, A., Proietti, S., Pantelides, I. S., and Stringlis, I. A. (2020). Modulation of the root microbiome by plant molecules: the basis for targeted disease suppression and plant growth promotion. Front. Plant Sci. 2020:1741. doi: 10.3389/fpls.2019.01741
Pavlova, A. S., Leontieva, M. R., Smirnova, T. A., Kolomeitseva, G. L., Netrusov, A. I., and Tsavkelova, E. A. (2017). Colonization strategy of the endophytic plant growth promoting strains of Pseudomonas fluorescens and Klebsiella oxytoca on the seeds, seedlings and roots of the epiphytic orchid, Dendrobium nobile Lindl. J. Appl. Microbiol. 123, 217–232. doi: 10.1111/jam.13481
Pinedo, I., Ledger, T., Greve, M., and Poupin, M. J. (2015). Burkholderia phytofirmans PsJN induces long-term metabolic and transcriptional changes involved in Arabidopsis thaliana salt tolerance. Front. Plant Sci. 6:466. doi: 10.3389/fpls.2015.00466
Pramanik, K., Mitra, S., Sarkar, A., Soren, T., and Maiti, T. K. (2017). Characterization of cadmium-resistant Klebsiella pneumoniae MCC 3091 promoted rice seedling growth by alleviating phytotoxicity of cadmium. Environ. Sci. Pollut. Control Ser. 24, 24419–24437. doi: 10.1007/s11356-017-0033-z
Rani, B. A., Madan, S., Pooja, K. S., Sharma, K. D., Kumari, N., and Kumar, A. (2018). Mitigating the effect of drought stress on yield in wheat (Triticum aestivum) using arbuscular mycorrhiza fungi (Glomus mosseae). Indian J. Agric. Sci. 88, 95–100. doi: 10.56093/ijas.v88i12.85444
Raymond, J., Siefart, J. L., Staples, C. R., and Blakenship, R. E. (2004). The natural history of nitrogen fixation. Mol. Biol. Evol. 21, 541–554. doi: 10.1093/molbev/msh047
Richter, J. A., Erban, A., Kopka, J., and Zörb, C. (2015). Metabolic contribution to salt stress in two maize hybrids with contrasting resistance. Plant Sci. 233, 107–115. doi: 10.1016/j.plantsci.2015.01.006
Rivero, J., Álvarez, D., Flors, V., Azcón-Aguilar, C., and Pozo, M. J. (2018). Root metabolic plasticity underlies functional diversity in mycorrhiza-enhanced stress tolerance in tomato. New Phytol. 220, 1322–1336. doi: 10.1111/nph.15295
Rodríguez, M., Torres, M., Blanco, L., Béjar, V., Sampedro, I., and Llamas, I. (2020). Plant growth-promoting activity and quorum quenching-mediated biocontrol of bacterial phytopathogens by Pseudomonas segetis strain P6. Sci. Rep. 10:4121. doi: 10.1038/s41598-020-61084-1
Rojas-Solís, D., Zetter-Salmón, E., Contreras-Pérez, M., del Carmen Rocha-Granados, M., Macías-Rodríguez, L., and Santoyo, G. (2018). Pseudomonas stutzeri E25 and Stenotrophomonas maltophilia CR71 endophytes produce antifungal volatile organic compounds and exhibit additive plant growth-promoting effects. Biocatal. Agric. Biotechnol. 13, 46–52. doi: 10.1016/j.bcab.2017.11.007
Rojas-Tapias, D., Moreno-Galvan, A., Pardo-Diaz, S., Obando, M., Riveria, D., and Bonilla, R. (2012). Effect of inoculation with plant growth-promoting bacteria (PGPB) on amelioration of saline stress in maize (Zea mays). Appl. Soil Ecol. 61, 264–272. doi: 10.1016/j.apsoil.2012.01.006
Saeed, Q., Xiukang, W., Haider, F. U., Kučerik, J., Mumtaz, M. Z., Holatko, J., et al. (2021). Rhizosphere bacteria in plant growth promotion, biocontrol, and bioremediation of contaminated sites: a comprehensive review of effects and mechanisms. Int. J. Mol. Sci. 22:10529. doi: 10.3390/ijms221910529
Sagar, A., Rathore, P., Ramteke, P. W., Ramakrishna, W., Reddy, M. S., and Pecoraro, L. (2021). Plant growth promoting rhizobacteria, arbuscular mycorrhizal fungi and their synergistic interactions to counteract the negative effects of saline soil on agriculture: key macromolecules and mechanisms. Microorganisms 9:1491. doi: 10.3390/microorganisms9071491
Sahoo, R. K., Ansari, M. W., Dangar, T. K., Mohanty, S., and Tuteja, N. (2014). Phenotypic and molecular characterisation of efficient nitrogen-fixing Azotobacter strains from rice fields for crop improvement. Protoplasma 251, 511–523. doi: 10.1007/s00709-013-0547-2
Salam, U., Ullah, S., Tang, Z. H., Elateeq, A. A., Khan, Y., Khan, J., et al. (2023). Plant metabolomics: an overview of the role of primary and secondary metabolites against different environmental stress factors. Life 13:706. doi: 10.3390/life13030706
Sandrini, M., Nerva, L., Sillo, F., Balestrini, R., Chitarra, W., and Zampieri, E. (2022). Abiotic stress and belowground microbiome: the potential of omics approaches. Int. J. Mol. Sci. 23:1091. doi: 10.3390/ijms23031091
Santos, L. F., and Olivares, F. L. (2021). Plant microbiome structure and benefits for sustainable agriculture. Current Plant Biology. 26:100198. doi: 10.1016/j.cpb.2021.100198
Santoyo, G. (2022). How plants recruit their microbiome? New insights into beneficial interactions. J. Adv. Res. 40, 45–58. doi: 10.1016/j.jare.2021.11.020
Sehar, Z., Gautam, H., Iqbal, N., Alvi, A. F., Jahan, B., Fatma, M., et al. (2022). The functional interplay between ethylene, hydrogen sulfide, and sulfur in plant heat stress tolerance. Biomol. Ther. 12:678. doi: 10.3390/biom12050678
Sen, S., and Chandrasekhar, C. N. (2014). Effect of PGPR on growth promotion of rice (Oryza sativa L.) under salt stress. Asian J. Plant Sci. Res. 4, 62–67.
Sexton, W. K., Fidero, M., Spain, J. C., Jiang, L., Bucalo, K., Cruse-Sanders, J. M., et al. (2019). Characterization of endophytic bacterial communities within greenhouse and field-grown rhizomes of three rare pitcher plant species (Sarracenia oreophila, S. leucophylla, and S. purpurea spp. venosa) with an emphasis on nitrogen-fixing bacteria. Plant Soil 447, 257–279. doi: 10.1007/s11104-019-04372-8
Shaffique, S., Khan, M. A., Imran, M., Kang, S. M., Park, Y. S., Wani, S. H., et al. (2022). Research progress in the field of microbial mitigation of drought stress in plants. Front. Plant Sci. 13:870626. doi: 10.3389/fpls.2022.870626
Sharma, M., Sudheer, S., Usmani, Z., Rani, R., and Gupta, P. (2020). Deciphering the omics of plant-microbe interaction: perspectives and new insights. Curr. Genomics 21, 343–362. doi: 10.2174/1389202921999200515140420
Shourie, A., Tomar, P., Srivastava, D., and Chauhan, R. (2014). Enhanced biosynthesis of quercetin occurs as a photoprotective measure in Lycopersicon esculentum mill. Under acute UV-B exposure. Braz. Arch. Biol. Technol. 57, 317–325. doi: 10.1590/S1516-8913201401678
Shourie, A., and Vijayalakshmi, U. (2022). Fungal diversity and its role in mycoremediation. Geomicrobiol J. 39, 426–444. doi: 10.1080/01490451.2022.2032883
Šimura, J., Antoniadi, I., Široká, J., Tarkowská, D., Strnad, M., Ljung, K., et al. (2018). Plant Hormonomics: multiple phytohormone profiling by targeted metabolomics. Plant Physiol. 177, 476–489. doi: 10.1104/pp.18.00293
Singer, E., Bushnell, B., Coleman-Derr, D., Bowman, B., Bowers, R. M., Levy, A., et al. (2016). High-resolution phylogenetic microbial community profiling. ISME J. 10, 2020–2032. doi: 10.1038/ismej.2015.249
Singh, U., Khemka, N., Rajkumar, M. S., Garg, R., and Jain, M. (2017). PLncPRO for prediction of long non-coding RNAs (lncRNAs) in plants and its application for discovery of abiotic stress-responsive lncRNAs in rice and chickpea. Nucleic Acids Res. 45:e183. doi: 10.1093/nar/gkx866
Singh, U. B., Malviya, D., Singh, S., Kumar, M., Sahu, P. K., Singh, H. V., et al. (2019). Trichoderma harzianum- and methyl jasmonate-induced resistance to Bipolaris sorokiniana through enhanced phenylpropanoid activities in bread wheat (Triticum aestivum L.). Front. Microbiol. 10:1697. doi: 10.3389/fmicb.2019.01697
Singh, N., Rai, V., and Singh, N. K. (2020). Multi-omics strategies and prospects to enhance seed quality and nutritional traits in pigeonpea. Nucleus 63, 249–256. doi: 10.1007/s13237-020-00341-0
Singh, D. P., Singh, V., Gupta, V. K., Shukla, R., Prabha, R., Sarma, B. K., et al. (2020). Microbial inoculation in rice regulates antioxidative reactions and defence related genes to mitigate drought stress. Sci. Rep. 10:4818. doi: 10.1038/s41598-020-61140-w
Solanki, M. K., Solanki, A. C., Rai, S., Srivastava, S., Kashyap, B. K., Divvela, P. K., et al. (2022). Functional interplay between antagonistic bacteria and Rhizoctonia solani in the tomato plant Rhizosphere. Front. Microbiol. 13:990850. doi: 10.3389/fmicb.2022.990850
Solden, L., Lloyd, K., and Wrighton, K. (2016). The bright side of microbial dark matter: lessons learned from the uncultivated majority. Curr. Opin. Microbiol. 31, 217–226. doi: 10.1016/j.mib.2016.04.020
Sorty, A. M., Meena, K. K., Choudhary, K., Bitla, U. M., Minhas, P. S., and Krishnani, K. K. (2016). Effect of plant growth promoting bacteria associated with halophytic weed (Psoralea corylifolia L.) on germination and seedling growth of wheat under saline conditions. Appl. Biochem. Biotechnol. 180, 872–882. doi: 10.1007/s12010-016-2139-z
Subramanian, P., Mageswari, A., Kim, K., Lee, Y., and Sa, T. (2015). Psychrotolerant endophytic pseudomonas sp. strains OB155 and OS261 induced chilling resistance in tomato plants (Solanum lycopersicum mill.) by activation of their antioxidant capacity. Mol. Plant-Microbe Interact. 28, 1073–1081. doi: 10.1094/MPMI-01-15-0021-R
Sultana, S., Alam, S., and Karim, M. M. (2021). Screening of siderophore-producing salt-tolerant rhizobacteria suitable for supporting plant growth in saline soils with iron limitation. J. Agric. Food Res. 4:100150. doi: 10.1016/j.jafr.2021.100150
Sun, C., Johnson, J. M., Cai, D., Sherameti, I., Oelmüller, R., and Lou, B. (2010). Piriformospora indica confers drought tolerance in Chinese cabbage leaves by stimulating antioxidant enzymes, the expression of drought-related genes and the plastid-localized CAS protein. J. Plant Physiol. 167, 1009–1017. doi: 10.1016/j.jplph.2010.02.013
Swenson, W., Wilson, D. S., and Elias, R. (2000). Artificial ecosystem selection. Proc. Natl. Acad. Sci. U. S. A. 97, 9110–9114. doi: 10.1073/pnas.150237597
Syed, A., Elgorban, A. M., Bahkali, A. H., Eswaramoorthy, R., Iqbal, R. K., and Danish, S. (2023). Metal-tolerant and siderophore producing pseudomonas fluorescence and Trichoderma spp. improved the growth, biochemical features and yield attributes of chickpea by lowering cd uptake. Sci. Rep. 13:4471. doi: 10.1038/s41598-023-31330-3
Sziderics, A. H., Rasche, F., Trognitz, F., Sessitsch, A., and Wilhelm, E. (2007). Bacterial endophytes contribute to abiotic stress adaptation in pepper plants (Capsicum annuum L.). Canad. J. Microbiol. 53, 1195–1202. doi: 10.1139/W07-082
Teo, H. M., Aziz, A., Wahizatul, A. A., Bhubalan, K., Siti, N. M. S., Muhamad, S. C. I., et al. (2022). Setting a plausible route for saline soil-based crop cultivations by application of beneficial halophyte-associated bacteria: a review. Microorganisms. 10:657. doi: 10.3390/microorganisms10030657
Timm, C. M., Pelletier, D. A., Jawdy, S. S., Gunter, L. E., Henning, J. A., Engle, N., et al. (2016). Two poplar-associated bacterial isolates induce additive favorable responses in a constructed plant-microbiome system. Front. Plant Sci. 7:497. doi: 10.3389/fpls.2016.00497
Tittabutr, P., Piromyou, P., Longtonglang, A., Noisa-Ngiam, R., Boonkerd, N., and Teaumroong, N. (2013). Alleviation of the effect of environmental stresses using coinoculation of mungbean by Bradyrhizobium and rhizobacteria containing stress induced ACC deaminase enzyme. Soil Sci. Plant Nutr. 59, 559–571. doi: 10.1080/00380768.2013.804391
Tiwari, S., and Lata, C. (2018). Heavy metal stress, signaling, and tolerance due to plant-associated microbes: an overview. Front. Plant Sci. 9:452. doi: 10.3389/fpls.2018.00452
Trivedi, P., Mattupalli, C., Eversole, K., and Leach, J. E. (2021). Enabling sustainable agriculture through understanding and enhancement of microbiomes. New Phytol. 230, 2129–2147. doi: 10.1111/nph.17319
Ul Haq, S., Khan, A., Ali, M., Khattak, A. M., Gai, W. X., Zhang, H. X., et al. (2019). Heat shock proteins: dynamic biomolecules to counter plant biotic and abiotic stresses. Int. J. Mol. Sci. 20:5321. doi: 10.3390/ijms20215321
Uzma, M., Iqbal, A., and Hasnain, S. (2022). Drought tolerance induction and growth promotion by indole acetic acid producing Pseudomonas aeruginosa in Vigna radiata. PLoS One 17:e0262932. doi: 10.1371/journal.pone.0262932
Varshney, R. K., Hiremath, P. J., Lekha, P., Kashiwagi, J., Balaji, J., Deokar, A. A., et al. (2009). A comprehensive resource of drought-and salinity responsive ESTs for gene discovery and marker development in chickpea (Cicer arietinum L.). BMC Genomics 10:523. doi: 10.1186/1471-2164-10-523
Veeramachaneni, S., and Ramachandrudu, K. (2020). Changes in growth, microbial and enzyme activities in oil palm nursery in response to bioinoculants and chemical fertilizers. Arch. Agron. Soil Sci. 66, 545–558. doi: 10.1080/03650340.2019.1628343
Velásquez, A. C., Castroverde, C. D. M., and He, S. Y. (2018). Plant–pathogen warfare under changing climate conditions. Curr. Biol. 28, R619–R634. doi: 10.1016/j.cub.2018.03.054
Veliz, E. A., Martínez-Hidalgo, P., and Hirsch, A. M. (2017). Chitinase-producing bacteria and their role in biocontrol. AIMS Microbiol. 3, 689–705. doi: 10.3934/microbiol.2017.3.689
Vinayarani, G., and Prakash, H. (2018). Growth promoting rhizospheric and endophytic bacteria from Curcuma longa L. as biocontrol agents against rhizome rot and leaf blight diseases. Plant Pathol. J. 34:218. doi: 10.5423/PPJ.OA.11.2017.0225
Wang, B., Li, Z., Ran, Q., Li, P., Peng, Z., and Zhang, J. (2018). ZmNF-YB16 overexpression improves drought resistance and yield by enhancing photosynthesis and the antioxidant capacity of maize plants. Front. Plant Sci. 9:709. doi: 10.3389/fpls.2018.00709
Wang, X., Liu, Y., Jia, Y., Gu, H., Ma, H., Yu, T., et al. (2012). Transcriptional responses to drought stress in root and leaf of chickpea seedling. Plant Mol. Biol. Report. 39, 8147–8158. doi: 10.1007/s11033-012-1662-4
Wang, B., Sun, Y. F., Song, N., Wang, X. J., Feng, H., Huang, L. L., et al. (2013). Identification of UV-B-induced microRNAs in wheat. Genet. Mol. Res. 12, 4213–4221. doi: 10.4238/2013.October.7.7
Wang, B., Zhai, H., He, S., Zhang, H., Ren, Z., Zhang, D., et al. (2016). A vacuolar Na+/H+ antiporter gene, IbNHX2, enhances salt and drought tolerance in transgenic sweet potato. Sci. Hortic. 201, 153–166. doi: 10.1016/j.scienta.2016.01.027
White, R. A., Rivas-Ubach, A., Borkum, M. I., Köberl, M., Bilbao, A., Colby, S. M., et al. (2017). The state of rhizospheric science in the era of multi-omics: a practical guide to omics technologies. Rhizosphere. 3, 212–221. doi: 10.1016/j.rhisph.2017.05.003
Win, K. T., Oo, A. Z., and Yokoyama, T. (2022). Plant growth and yield response to salinity stress of Rice grown under the application of different nitrogen levels and Bacillus pumilus strain TUAT-1. Crops 2, 435–444. doi: 10.3390/crops2040031
Yang, J., Kloepper, J. W., and Ryu, C. M. (2009). Rhizosphere bacteria help plants tolerate abiotic stress. Trends in plant Sci. 14, 1–4. doi: 10.1016/j.tplants.2008.10.004
Yasmin, S., and D’Souza, D. (2010). Effects of pesticides on the growth and reproduction of earthworm: a review. Appl. Environ. Soil Sci. 2010:e678360. doi: 10.1155/2010/678360
Yasmin, H., Naeem, S., Bakhtawar, M., Jabeen, Z., Nosheen, A., Naz, R., et al. (2020). Halotolerant rhizobacteria pseudomonas pseudoalcaligenes and Bacillus subtilis mediate systemic tolerance in hydroponically grown soybean (Glycine max L.) against salinity stress. PLoS One 15:e0231348. doi: 10.1371/journal.pone.0231348
Zahoor, M., Irshad, M., Rahman, H., Qasim, M., Afridi, S. G., Qadir, M., et al. (2017). Alleviation of heavy metal toxicity and phytostimulation of Brassica campestris L. by endophytic Mucor sp. MHR-7. Ecotoxicol. Environ. Saf. 142, 139–149. doi: 10.1016/j.ecoenv.2017.04.005
Zainab, N., Khan, A. A., Azeem, M. A., Ali, B., Wang, T., Shi, F., et al. (2021). PGPR-mediated plant growth attributes and metal extraction ability of Sesbania sesban L. in industrially contaminated soils. Agronomy 11:1820. doi: 10.3390/agronomy11091820
Zandalinas, S. I., and Mittler, R. (2022). Plant responses to multifactorial stress combination. New Phytol. 234, 1161–1167. doi: 10.1111/nph.18087
Zandi, P., and Schnug, E. (2022). Reactive oxygen species, antioxidant responses and implications from a microbial modulation perspective. Biology (Basel) 11:155. doi: 10.3390/biology11020155
Zenda, T., Liu, S., Dong, A., Li, J., Wang, Y., Liu, X., et al. (2021). Omics-facilitated crop improvement for climate resilience and superior nutritive value. Front. Plant Sci. 12:774994. doi: 10.3389/fpls.2021.774994
Zhang, B., and Wang, Q. (2015). MicroRNA-based biotechnology for plant improvement. J. Cell. Physiol. 230, 1–15. doi: 10.1002/jcp.24685
Zhang, Z., Wei, L., Zou, X., Tao, Y., Liu, Z., and Zheng, Y. (2008). Submergence-responsive MicroRNAs are potentially involved in the regulation of morphological and metabolic adaptations in maize root cells. Ann. Bot. 102, 509–519. doi: 10.1093/aob/mcn129
Zhang, F., Yang, J., Zhang, N., Wu, J., and Si, H. (2022). Roles of microRNAs in abiotic stress response and characteristics regulation of plant. Front. Plant Sci. 13:919243. doi: 10.3389/fpls.2022.919243
Zhang, Y., Zhu, X., Chen, X., Song, C., Zou, Z., Wang, Y., et al. (2014). Identification and characterization of cold-responsive microRNAs in tea plant (Camellia sinensis) and their targets using high-throughput sequencing and degradome analysis. BMC Plant Biol. 14:271. doi: 10.1186/s12870-014-0271-x
Zhang, H., Zhu, J., Gong, Z., and Zhu, J. K. (2022). Abiotic stress responses in plants. Nat. Rev. Genet. 23, 104–119. doi: 10.1038/s41576-021-00413-0
Zhao, C., Liu, B., Piao, S., Wang, X., Lobell, D. B., Huang, Y., et al. (2017). Temperature increase reduces global yields of major crops in four independent estimates. Proc. Natl. Acad. Sci. 114, 9326–9331. doi: 10.1073/pnas.1701762114
Zhou, L., Liu, Y., Liu, Z., Kong, D., Duan, M., and Luo, L. (2010). Genome-wide identification and analysis of drought-responsive microRNAs in Oryza sativa. J. Exp. Bot. 61, 4157–4168. doi: 10.1093/jxb/erq237
Keywords: phyto-microbiome, abiotic stress alleviation, bio-inoculants, multi-omics, plant microbiome engineering
Citation: Singh A, Mazahar S, Chapadgaonkar SS, Giri P and Shourie A (2023) Phyto-microbiome to mitigate abiotic stress in crop plants. Front. Microbiol. 14:1210890. doi: 10.3389/fmicb.2023.1210890
Edited by:
Anukool Vaishnav, Agroscope, SwitzerlandReviewed by:
Mahendra Vikram Singh Rajawat, Prabhat Fertilizers and Chemical Works, IndiaKhan Mohd. Sarim, Rudjer Boskovic Institute, Croatia
Copyright © 2023 Singh, Mazahar, Chapadgaonkar, Giri and Shourie. This is an open-access article distributed under the terms of the Creative Commons Attribution License (CC BY). The use, distribution or reproduction in other forums is permitted, provided the original author(s) and the copyright owner(s) are credited and that the original publication in this journal is cited, in accordance with accepted academic practice. No use, distribution or reproduction is permitted which does not comply with these terms.
*Correspondence: Abhilasha Shourie, YWFzaG91cmllQGdtYWlsLmNvbQ==
†ORCID: Abhilasha Shourie, https://orcid.org/0000-0002-3659-1143