- Max Planck Institute for Terrestrial Microbiology, Marburg, Germany
There is virtually no environmental process that is not dependent on temperature. This includes the microbial processes that result in the production of CH4, an important greenhouse gas. Microbial CH4 production is the result of a combination of many different microorganisms and microbial processes, which together achieve the mineralization of organic matter to CO2 and CH4. Temperature dependence applies to each individual step and each individual microbe. This review will discuss the different aspects of temperature dependence including temperature affecting the kinetics and thermodynamics of the various microbial processes, affecting the pathways of organic matter degradation and CH4 production, and affecting the composition of the microbial communities involved. For example, it was found that increasing temperature results in a change of the methanogenic pathway with increasing contribution from mainly acetate to mainly H2/CO2 as immediate CH4 precursor, and with replacement of aceticlastic methanogenic archaea by thermophilic syntrophic acetate-oxidizing bacteria plus thermophilic hydrogenotrophic methanogenic archaea. This shift is consistent with reaction energetics, but it is not obligatory, since high temperature environments exist in which acetate is consumed by thermophilic aceticlastic archaea. Many studies have shown that CH4 production rates increase with temperature displaying a temperature optimum and a characteristic apparent activation energy (Ea). Interestingly, CH4 release from defined microbial cultures, from environmental samples and from wetland field sites all show similar Ea values around 100 kJ mol−1 indicating that CH4 production rates are limited by the methanogenic archaea rather than by hydrolysis of organic matter. Hence, the final rather than the initial step controls the methanogenic degradation of organic matter, which apparently is rarely in steady state.
Introduction
There is virtually no microbial activity that would not be regulated by temperature (Wiegel, 1990). Biological activity is based on chemical reactions. Chemical reactions are controlled by temperature and so is biological activity. Therefore, temperature dependence of biological activity follows the same physical principles as chemical reactions do. In fact, it is predominantly enzyme-based biochemical reactions, which control biological activity. Therefore, biological activity always exhibits a temperature optimum, beyond which enzymes become inactivated (Radmer and Kok, 1979), while purely chemical reactions may tolerate much higher temperatures.
Microbial life is complex. Microorganisms contain many different enzymes, which may react differently upon temperature changes. Microbial populations consist of many different individual microorganisms, each possibly slightly different in its response to temperature. In nature, microbial communities consist of many different microbial populations with different physiologies and life styles and thus, with potentially different features of temperature dependence. Therefore, it is by principle very complex how environmental microbial communities will react to temperature changes and it is hard to make any predictions.
Nevertheless, it is worthwhile to review the literature on temperature dependence of microbial activity to see whether there are any guiding principles. In the following I will primarily (but not exclusively) focus on methanogenic microbial communities living in anoxic environments such as flooded rice fields and aquatic sediments, whose temperature characteristics have frequently been studied over the last 40–50 years. A previous mini-review on this subject (Conrad, 2008) will be updated and expanded. The temperature dependence of methanogenic communities is of particular interest, since methane is an important greenhouse gas, which is partially responsible for past and present climate change and will in turn be affected by the global temperature increase in many respects (Kirschke et al., 2013).
Temperature dependence of methane-producing microbial populations
Methane is produced by methanogenic archaea, which convert simple substrates like acetate, H2 + CO2, formate, trimethylamine, dimethylsulfide, and methanol to CH4. The most common methanogenic substrates in nature are acetate and H2 + CO2 (Conrad, 2020). The rates of CH4 production may be described by Michaelis–Menten kinetics, which account not only for the maximal possible rate, Vmax, but also for the effect of the substrate concentration, S, i.e., v = Vmax S/(Km + S). The Km value is the substrate concentration at Vmax/2. In this way, metabolic rates are modulated by the substrate availability. Both Vmax and Km of enzymatic reactions are expected to increase with increasing temperature (Scopes, 1995). Microbial growth rates, μ, are analogously modulated by substrate concentration using the Monod equation, i.e., μ = μmax S/(KS + S), where KS is the substrate concentration at μmax/2. Note, however, that Km and KS are different values with a different physiological meaning and relevance.
The rates of CH4 production increase with increasing temperature. The increase in rate (v) can be described by the Arrhenius equation, i.e., v = A exp {−Ea/(RT)} with A = Arrhenius constant and Ea = apparent activation energy. In a microbial cell, e.g., a methanogenic archaeon, CH4 is produced as the end product of a catabolic reaction chain, in which the methanogenic substrate is converted to CH4 (Thauer et al., 2008). The rate-limiting step is usually not the reaction producing the CH4, but the first enzymatic step in the entire process chain, since otherwise process intermediates would accumulate (Heinrich and Schuster, 1996). It is generally assumed that the transport of the substrate over the cellular membrane is the step, which limits catabolism and growth (Button, 1985). The cell-specific affinity, which is equal to Vmax/Km, is decisive for substrate uptake and catabolism. It has been argued that substrates with active transport over the cellular membrane (e.g., nitrate) face a cell-specific affinity that increases with temperature, whereas substrates without active transport (e.g., ammonia) face a temperature independent cell-specific affinity (Nedwell, 1999). The methanogenic substrates H2 and CO2 display no active transport. However, determination of Michaelis–Menten parameters of process kinetics in methanogenic microbes showed that both Vmax and Km exhibit temperature dependence (Westermann et al., 1989; Kotsyurbenko et al., 2001). The increasing Vmax is compensated by the also increasing Km thus resulting in fairly constant cell-specific affinities (Vmax/Km), such as predicted by Nedwell (1999). Therefore, we have to assume that CH4 production rates are not limited by the substrate transport of substrates, but by one of the subsequent enzymatic reaction steps. This limiting enzyme reaction may be the same as that defining the kinetic isotope effect of CH4 production (Games et al., 1978). The kinetic isotope effect, which by itself is not influenced by temperature (Penger et al., 2014), is assumed to be influenced by the first irreversible enzymatic steps, which result in a “commitment of reaction” (Northrop, 1981; Thullner et al., 2013). For aceticlastic methanogens it is the activation of acetate by either acetate kinase or acetyl CoA synthase, resulting in stronger isotope effect in Methanosarcina versus Methanothrix (Methanosaeta) species, respectively (Penning et al., 2006; Goevert and Conrad, 2009). These two methanogenic genera typically also display different μmax, Vmax, KS, Km and thresholds for acetate by having either of the two acetate-activating enzyme systems (Jetten et al., 1992). It is likely that these enzyme systems constitute the rate limiting steps for aceticlastic CH4 production displaying temperature dependence.
In any case, the temperature characteristic described by Ea is that of the rate-limiting step of a particular methanogenic archaeon and a particular substrate. The Ea values of different methanogenic archaea are typically 106 kJ mol−1 (89–122 kJ mol−1). These data are from a meta-analysis of literature data, based on 33 different strains of growing or non-growing methanogenic archaea (Yvon-Durocher et al., 2014). These Ea values are relatively high when compared to respiration (Yvon-Durocher et al., 2012), photosynthesis (Allen et al., 2005) and hydrolysis of organic matter (Middelburg et al., 1996; Weston and Joye, 2005).
Temperature dependence of methane-producing microbial communities
A similar range of Ea values was obtained from 47 different methanogenic microbial communities in paddy soils, wetlands and aquatic sediments, i.e., 89 kJ mol−1 (79–99 kJ mol−1; Yvon-Durocher et al., 2014). In these environmental samples CH4 is produced as the final step in a complex microbial community that converts organic matter to CH4 and CO2. Polysaccharides, a common form of organic matter, are hydrolyzed to sugars, which are then fermented by bacteria to fatty acids, alcohols and other small compounds. These are then further fermented to acetate, H2 and CO2 by bacteria living in syntrophic association with methanogenic archaea, which produce CH4 (Zinder, 1993; Schink and Stams, 2013; Figure 1). If the system is in steady state, the first step, i.e., hydrolysis of organic matter, should be the rate-limiting step of CH4 production, and all the subsequent reactions should be substrate-limited (Nedwell, 1984). However, this seems to be rarely the case. Thus, CH4 production increases if the methanogenic substrates acetate and H2 are added showing that temperature limits substrate supply (Westermann, 1993; Nozhevnikova et al., 1997). Addition of polysaccharides or sugars results in enhancement of fermentation reactions and the production of acetate and H2, and consequently also stimulates CH4 production (Kotsyurbenko et al., 1993; Chin et al., 1998). Stimulation of CH4 production by substrate addition usually is stronger at high than at low temperatures, so that Ea values increase. For example, addition of excess H2 to methanogenic paddy soil increased the Ea values from about 70 to 91 kJ mol−1 (Conrad et al., 1987). This response is plausible if the Ea values of the terminal CH4 production step are larger than those of the preceding processes such as polysaccharide hydrolysis or fermentative H2 production (Figure 2). In experiments with marine sediments, Ea values of hydrolysis/fermentation indeed are on the average only 49 kJ mol−1 (Weston and Joye, 2005). In other marine sediments, however, the range of Ea values for hydrolytic processes extends from 54 to 125 kJ mol−1 (Middelburg et al., 1996). After submergence of rice field soils, degradation of labile organic matter usually increases the production of the methanogenic substrates acetate and H2 over several days before CH4 production eventually enters quasi steady state conditions with much lower rates. Values of Ea are typically much lower (more than three times) during steady state than during the phase of excess substrate (Yao and Conrad, 2000). Hence, the relatively narrow range and the relatively high values of Ea for CH4 production obtained by the meta-analysis of Yvon-Durocher et al. (2014) indicate that the microbial communities are not in steady state with the hydrolysis of organic matter. This is even more so, since the mean value (89 kJ mol−1) is close to that of defined methanogenic populations (106 kJ mol−1) suggesting that CH4 production by methanogenic archaea is the rate-limiting step also when organic matter is the primary substrate (Hoehler and Alperin, 2014). This observation suggests that the methanogenic archaea in the complex communities are not limited by supply of their substrates (i.e., acetate, H2 + CO2) when temperature increases. An alternative explanation is that the hydrolysis of organic matter, as the initial rate-limiting step under steady state conditions, has a similar Ea value as the CH4 production by methanogenic archaea (Hoehler and Alperin, 2014). Note that literature data show a rather large range of Ea values from 49 to 125 kJ mol−1 (Middelburg et al., 1996; Weston and Joye, 2005). Also note, that the different hydrolytic enzymes may be differently affected by temperature (Fey and Conrad, 2003; Arnosti and Joergensen, 2006) and that the effective composition of organic matter, which is converted to CH4, changes with incubation time, resulting in a change of the pathway of CH4 formation (Ji et al., 2018).
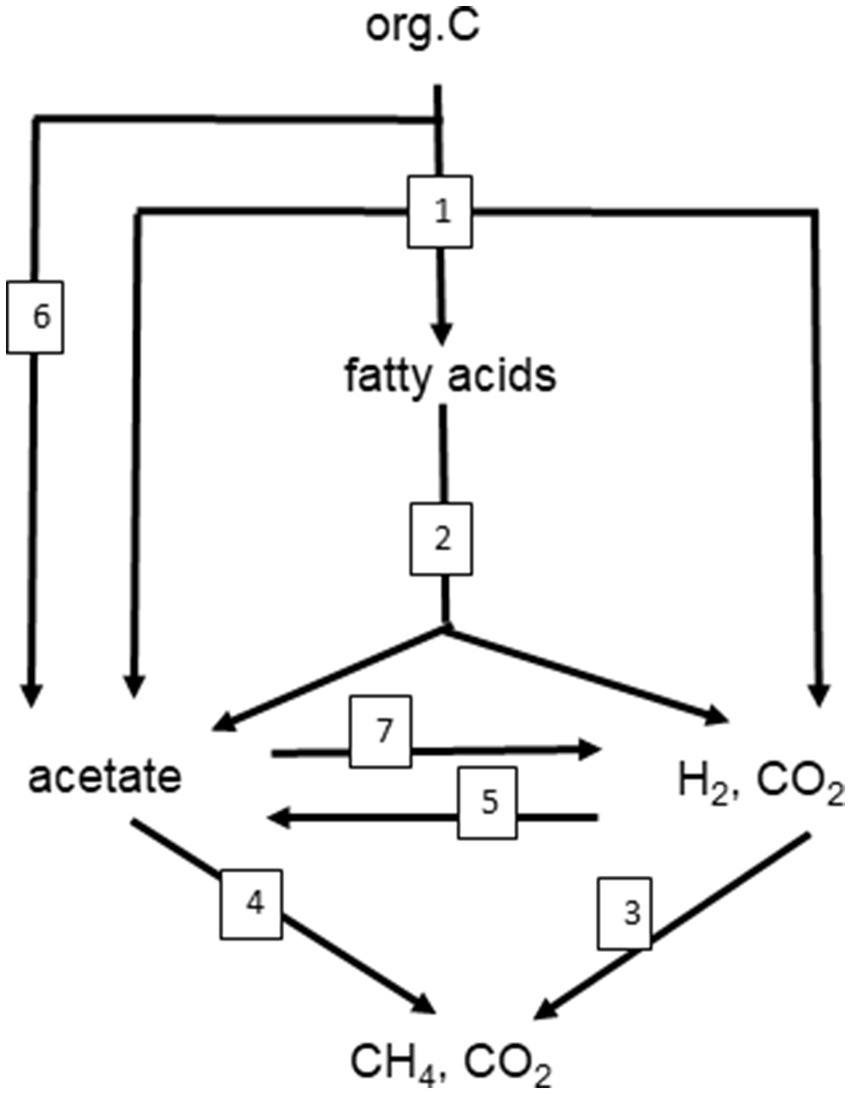
Figure 1. Scheme of the anaerobic degradation of organic matter (e.g., cellulose) to CH4 and CO2. The degradation involves the following functions indicated by numbers: (1) hydrolysis of polymeric organic matter to monomers, followed by fermentation of the monomers to short-chain fatty acids (e.g., lactate, propionate), acetate and H2, CO2; (2) syntrophic conversion of short-chain fatty acids to acetate, H2, CO2; (3) methanogenic conversion of H2, CO2 to CH4 (hydrogenotrophic methanogenesis); (4) methanogenic conversion of acetate to CH4 (aceticlastic methanogenesis); (5) conversion of H2, CO2 to acetate (chemolithotrophic homoacetogenesis); (6) fermentation of monomers to acetate only (heterotrophic homoacetogenesis); (7) syntrophic oxidation of acetate to H2, CO2. Stoichiometries and thermodynamic parameters of examples for the numbered reactions are shown in Table 1.
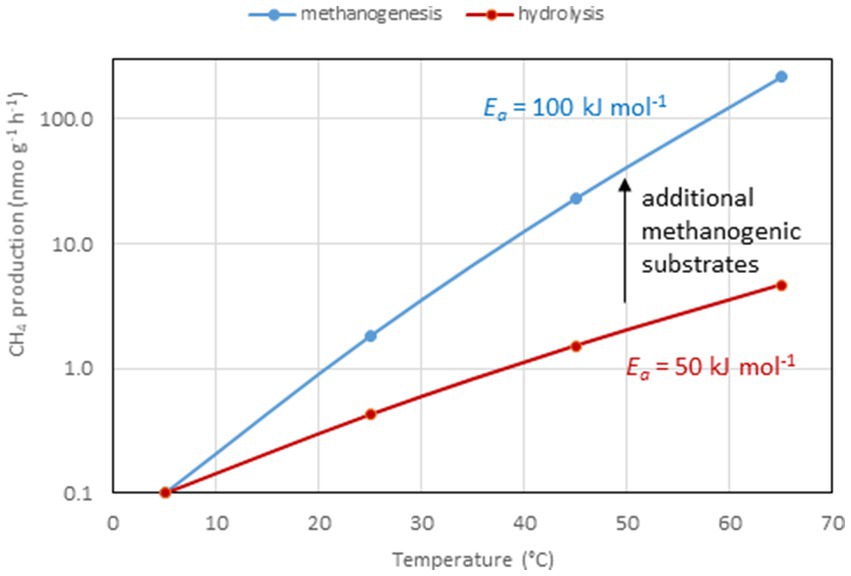
Figure 2. Temperature dependence of rates of CH4 production and hydrolysis of organic matter by assuming Ea values of 100 and 50 kJ mole−1, respectively.
Temperature dependence of methane-producing ecosystems
Meta-analysis of 127 sites of methanogenic ecosystems (marshes, rice fields, peatlands) shows a narrow range of Ea values, i.e., 92 kJ mol−1 (83–103 kJ mol−1; Yvon-Durocher et al., 2014). These values are similar to those found in methanogenic communities and in methanogenic populations, again suggesting that the temperature dependence of CH4-emitting ecosystems is the same as that of methanogenic microbial cultures rather than that of enzymes hydrolyzing organic matter. The Ea values in one Italian rice field exhibited a broad range from 50 to 450 kJ mol−1 (Schütz et al., 1990). The meta analysis of various ecosystems also showed a broad frequency distribution of Ea values (Yvon-Durocher et al., 2014). Nevertheless, the mean Ea values of CH4-emitting ecosystems and CH4-producing methanogenic microbial cultures were statistically indistinguishable. This is a remarkable observation, especially since the Ea values related to CH4 emission are relatively high. They are much higher than those related to respiration (Yvon-Durocher et al., 2012), photosynthesis (Allen et al., 2005) or to the CO2 emission rates observed in the same meta analysis (Yvon-Durocher et al., 2014).
In order to appreciate the coincidence of Ea values of methanogenic archaeal populations with those of entire ecosystems, one should realize that at the level of ecosystems the temperature changes are recorded as daily rhythms and seasonal variations. In addition to the temperature effects, ecosystems are exposed to many other control variables, such as variations in substrate supply [e.g., root exudations in vegetated soil and sediment (Watanabe et al., 1999)], consumption of CH4 at the oxic sediment surface or the rhizosphere (Schütz et al., 1989), or simply by temperature gradients within the ecosystem (sediment depth; Schütz et al., 1990). Usually the microbial community is also exposed to various potential oxidants such as nitrate, ferric iron, and sulfate (Zehnder and Stumm, 1988). These oxidants allow the oxidative catabolism of the organic substrates to CO2 in the community thus suppressing CH4 production. Production of CH4 is suppressed, since the process kinetics and thermodynamics of microorganisms reducing nitrate, ferric iron, or sulfate allow to outcompete methanogenic archaea for their substrates H2 and acetate (Cord-Ruwisch et al., 1988; Lovley and Goodwin, 1988). However, CH4 production is initiated as soon as these oxidants are depleted. Since these oxidation processes are temperature dependent in a similar way as the processes leading to CH4 production, the different phases of sequential reduction of oxidants can strongly affect the Ea values until quasi steady state is reached (Nozhevnikova et al., 1997; VanBodegom and Stams, 1999; vanHulzen et al., 1999; Yao and Conrad, 2000). In summary, CH4 emissions from ecosystems exhibit a temperature dependence that is hardly consistent with the assumption of being in steady state with organic matter degradation in the soil or sediment. Instead, the CH4-producing methanogens must be supplied with additional substrate. In rice fields and vegetated wetlands it may be photosynthetically produced organic substrates, which are supplied as root exudation. In fact, this source of organic carbon seems to be responsible for more than 50% of total CH4 emission (Watanabe et al., 1999; Tokida et al., 2011; Yuan et al., 2012). In lake sediments, fresh organic carbon can be supplied by sedimentation of algae (Schulz and Conrad, 1995; Schwarz et al., 2008). Furthermore, fresh organic matter has a short lifetime in paddy soil so that 80–90% is degraded within 1 year (Neue and Scharpenseel, 1987). The composition of the degradable organic matter in paddy soil changes over time, thus causing a change in the degradation pathway to CH4 (Ji et al., 2018). Seasonal changes in the methanogenic pathway are commonly observed in rice field ecosystems (Bilek et al., 1999; Krüger et al., 2002; Zhang et al., 2013). Hence, it is not surprising that hydrolysis of organic matter is usually not the rate-limiting step of CH4 production in the environment, but other processes forming methane precursors.
The temperature dependence of CH4 production is similar in tropical versus boreal lake sediments (Marotta et al., 2014). Since sediments of low latitude versus high latitude lakes are much warmer, greenhouse gas production is also much higher in those regions despite their much smaller lake area, and they will also respond more strongly to global warming because of the exponential effect of Ea.
Temperature optimum of methane production
Production of CH4 exhibits a temperature optimum beyond which the existing microorganisms and their enzyme systems become inactivated or beyond which methanogenic life does not exist. Such a temperature optimum can be modelled within the framework of the Arrhenius theory by a temperature-dependent change in the heat capacity for enzyme catalysis (Hobbs et al., 2013; Schipper et al., 2014). Whenever methanogenic environmental samples have been incubated at different temperatures, the highest CH4 production rates have generally been observed at mesophilic temperatures around 30°C (Zeikus and Winfrey, 1976; Conrad et al., 1989; Nozhevnikova et al., 1997; Fey and Conrad, 2003; Metje and Frenzel, 2007; Blake et al., 2015). Occasionally, a second optimum has been observed at thermophilic temperatures of 50–70°C (Nozhevnikova et al., 1997; Fey et al., 2001). It is remarkable that the temperature optima are much higher than the average in-situ temperatures. This is especially notable for profundal lake sediments, which are at permanently low 4°C, while temperature optima are around 30°C. The display of temperature optima higher than in-situ indicates the existence of mesophilic microbes that rapidly respond to a temperature increase, thus outcompeting the microorganisms active at in-situ temperature. These mesophilic microorganisms must cover the entire microbial community responsible for CH4 production, including hydrolytic and fermentative bacteria. The difference between in-situ temperature and temperature optimum is not restricted to methanogenesis, but seems to be a general feature of sediment metabolism (Yayanos, 1986; Arnosti et al., 1998).
Nevertheless, microbial and enzymatic activity is also found at the low in-situ temperatures (Schulz and Conrad, 1996; Arnosti and Joergensen, 2003; Metje and Frenzel, 2007; Kolton et al., 2019). This can be interpreted either as psychotolerance of mesophilic microorganisms or as the existence of a hidden psychrophilic microbial community. Psychotolerance seems to be a widely occurring phenomenon (Nozhevnikova et al., 2001, 2003; Saunders et al., 2003; Kotsyurbenko, 2005). However, reports of psychrophilic methanogenic archaea, which are not only tolerant but are especially adapted to life at low temperatures are not very common (Franzmann et al., 1997; Simankova et al., 2001, 2003; Cavicchioli, 2006; Parshina et al., 2014; Zhou et al., 2014). This is in contrast to the abundance of hyperthermophilic methanogenic species (Stetter, 2002), indicating that methanogenic archaea potentially adapt better to high than to low temperatures. This preference may be explained by the specificities of cellular characteristics in Archaea versus Bacteria (Cavicchioli, 2006; Valentine, 2007). For example, the microbial cell membranes of Archaea and Bacteria are ether lipid monolayers and fatty acid ester bilayers, respectively. The temperature dependence of membrane rigidity and flexibility is different for the two types of lipid membranes (Koga, 2012; Siliakus et al., 2017), thus affecting microbial adaptation. Since different microbial populations may display different temperature adaptations, the optimum temperature can be different for different physiological groups and different biogeochemical pathways, e.g., hydrogenotrophic and acetotrophic methanogenesis (Svensson, 1984; Schulz et al., 1997). In fact, the pathway of CH4 production is strongly affected by temperature (see below).
Temperature dependence of process thermodynamics
Besides enzyme kinetics, temperature also affects the thermodynamics of methanogenic processes. The free enthalpy (Gibbs free energy) change of a reaction is given by the changes of the enthalpy and the entropy, i.e., ΔG = ΔH – TΔS. For standard conditions (1 atm, 1 M, 298°K, pH = 0) values of ΔGo, ΔHo, and ΔSo for reactions important in CH4 production pathways can be calculated from tabulated values (e.g., Stumm and Morgan, 1996; Table 1). Values of ΔGo´ give the standard free enthalpy at pH 7 if n protons are produced or consumed in the reaction, i.e., ΔGo´ = ΔGo ± nRT ln(10−7), which is −39.94 kJ mol−1 protons produced. Temperature dependence of ΔGo is basically a function of the magnitude of the process entropies (ΔSo) under standard conditions. The actual ΔG at the given concentrations of reactants and products can be calculated from the Nernst equation, which is also temperature-dependent, i.e., ΔG = ΔGo + RT ln(P/S), where P are the products and S the reactants. P and S represent the product of the activities or partial pressures of the individual products and reactants, respectively. At dilute solutions activities may be replaced by concentrations. The lowest possible concentrations of the reactants are those when the actual ΔG = 0, i.e., the threshold. For example, hydrogenotrophic methanogenesis proceeds until the threshold concentration (partial pressure) of H2 is reached. Below the threshold H2 can no longer be consumed and CH4 production stops (Figure 3). On the other hand, there is also an upper threshold of H2, which may not be surpassed to allow the syntrophic degradation of compounds such as lactate, propionate, or acetate (Figure 3). The degradation of such compounds is endergonic under standard conditions, but becomes exergonic if the H2 partial pressures are sufficiently low (McInerney et al., 2008; Schink and Stams, 2013). The thresholds of H2 consumption by methanogenesis or sulfate reduction are usually a bit higher than predicted by ΔG = 0 (Yao and Conrad, 1999; Hoehler et al., 2001) and those of H2 production by syntrophic fatty acid degradation are usually a bit lower (Scholten and Conrad, 2000; Yao and Conrad, 2001). The deviation is on the order of 3–6 kJ mol−1 H2. It is probably due to the fact that the microorganisms require a minimum of free enthalpy to allow the synthesis of 1/4–1/3 ATP (Thauer and Morris, 1984; Schink, 1997; Lever et al., 2015). The H2 threshold has also been explained by models, e.g., by a combination of the thermodynamic equilibrium constant (K = exp {−ΔGo/(RT)}) with the Michaelis–Menten equation (Hoh and Cord-Ruwisch, 1996), or by including the maintenance energy requirements of the microorganisms (Hoehler, 2004).
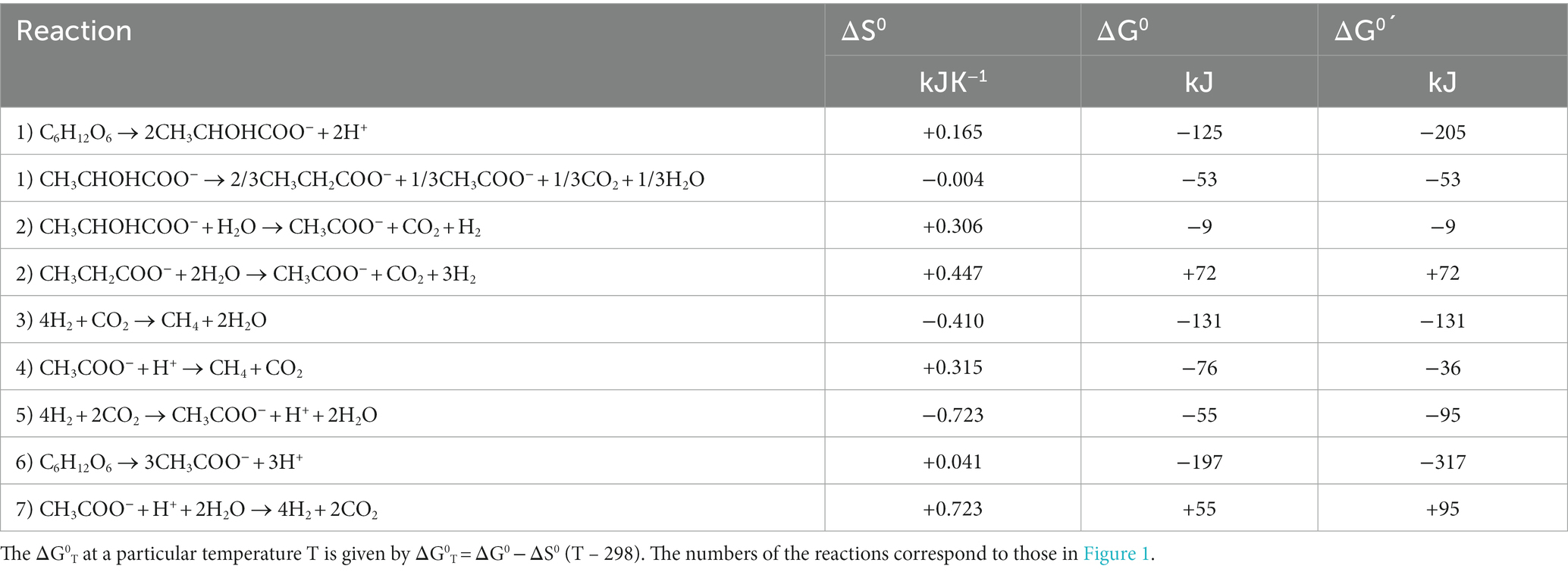
Table 1. Entropies (ΔS0) and free enthalpies (ΔG0) under standard conditions (1 bar, 1 M, 298°K, pH = 0) and at pH = 7 (ΔG0´) for different reactions involved in the degradation of hexose to CH4.
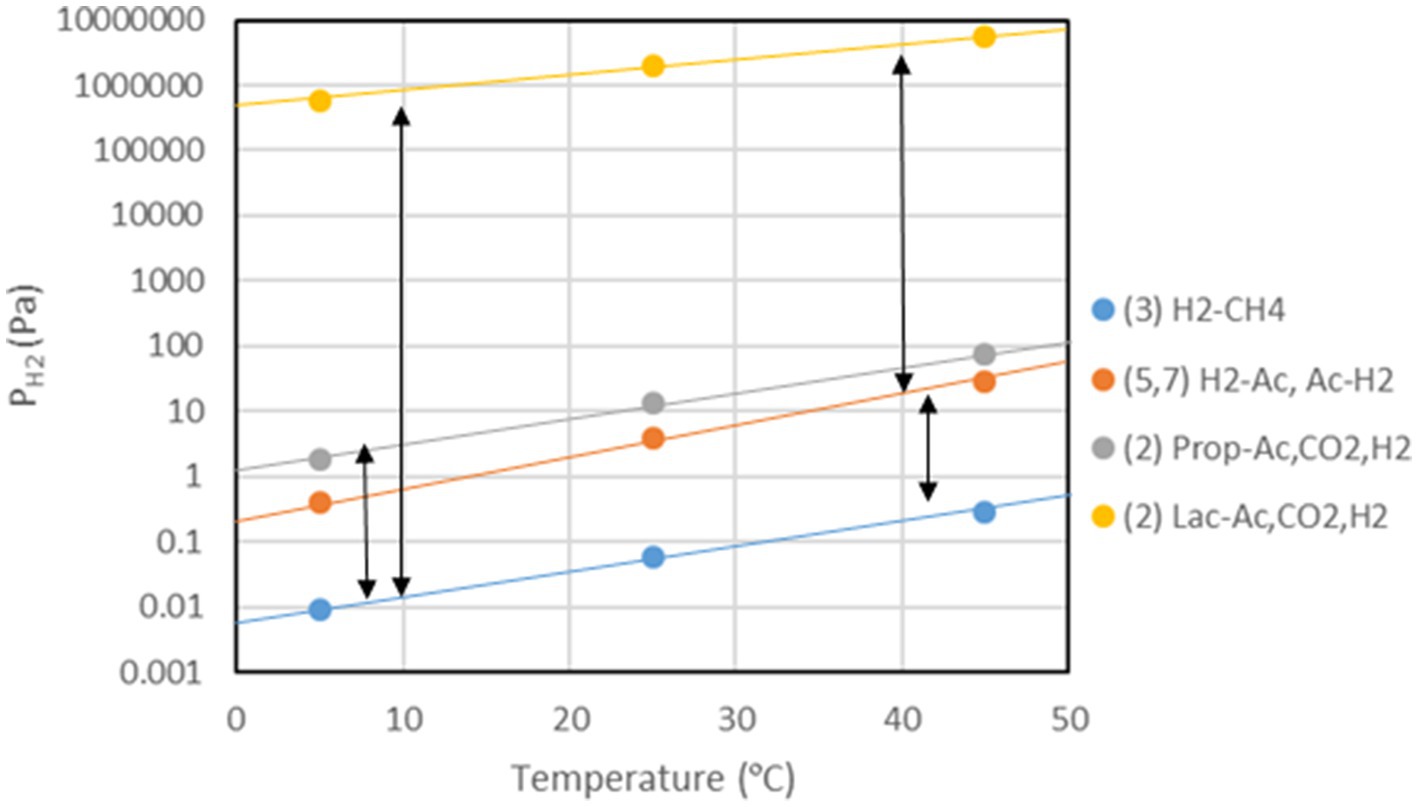
Figure 3. Temperature dependence of H2 thresholds of H2-producing and H2-consuming processes. The double arrows indicate the window of H2 partial pressures in which syntrophic interaction between the H2-producing and H2-consuming processes is possible. The H2-thresholds were calculated for ΔG’T = 0 using the Nernst equation with CH4 = 0.001 bar, CO2 = 0.1 bar, and lactate, propionate and acetate = 1 mM. The numbers indicate the processes listed in Table 1.
Thresholds of H2 partial pressures are a function of the standard free enthalpies of anaerobic H2-consuming processes, and generally decrease with the useful energy (more negative ΔGo). Hence, the H2 threshold is for example lower in hydrogenotrophic ferric iron reducers < sulfate reducers < methanogens < homoacetogens. This has been found in microbial populations (Lovley, 1985; Cord-Ruwisch et al., 1988; Seitz et al., 1990; Caccavo et al., 1992) and in methanogenic environments (Conrad et al., 1986; Lovley and Goodwin, 1988; Hoehler et al., 1998). In the environment, CH4 production from H2/CO2 only operates, if the H2 partial pressures are higher than the threshold (Rothfuss and Conrad, 1993; Yao and Conrad, 1999; Hoehler et al., 2001). By contrast, generation of H2 from ethanol or fatty acids only operates, if H2 partial pressures are lower than the threshold (Seitz et al., 1990; Westermann, 1994; Scholten and Conrad, 2000; Yao and Conrad, 2001). Hence, metabolism and growth of both H2-producing and H2-consuming microorganisms is thermodynamically restricted in opposite ways. The satisfaction of the upper and the lower thresholds creates a window of permissive H2 partial pressures. Only within this window is the syntrophic conversion of ethanol, fatty acids and aromatic compounds to CH4 possible. The size of this window depends on the thermodynamics of the actual H2-producing and H2-consuming physiologies (Zinder, 1993; Dolfing, 2013).
The thresholds of H2 consumption increase with increasing temperature in a way that is characteristic for the underlying process, i.e., differently for sulfate reduction < methanogenesis < homoacetogenesis (Conrad and Wetter, 1990; Hoehler et al., 1998; Fey and Conrad, 2000; Kotsyurbenko et al., 2001). The thresholds of syntrophic H2 production also increase with increasing temperature. However, syntrophy between H2 production and H2 consumption is only possible if the threshold of production is higher than that of consumption (Figure 3). The resulting window of permissive H2 partial pressures gradually shifts to higher values when temperature increases (Westermann, 1996; Schink, 1997; Hattori, 2008). Alternatively to H2, syntrophy can also be maintained with formate acting as electron shuttle (Schink et al., 2017) or even with direct electron transfer (Shrestha and Rotaru, 2014; Li et al., 2015). Formate and H2/CO2 are largely at equilibrium and thus energetically equivalent in most methanogenic environments (Schink et al., 2017; Montag and Schink, 2018).
Acetate in methanogenic environments also displays a characteristic threshold that changes with temperature (Fey and Conrad, 2000). Like H2, acetate is an intermediate in the anaerobic degradation of organic matter to CH4. It is produced by many fermentation processes, notably by homoacetogenesis both from carbohydrates and from H2 + CO2 (Drake, 1994), and by many syntrophic degradation processes (Schink, 1997; McInerney et al., 2008). The syntrophic degradation processes can be subject to a decisive thermodynamic sensitivity for increased acetate concentrations, which may become inhibitory if too high (Ahring and Westermann, 1988; Dolfing and Tiedje, 1988; Beaty and McInerney, 1989; Platen et al., 1994). In methanogenic systems acetate is degraded to CH4 and CO2 by the genera Methanosarcina and Methanothrix only. Thermodynamics predict that the concentration of acetate, which is permissive for aceticlastic methanogenesis, decreases with temperature (ΔSo > 0; Table 1), which indeed was observed in methanogenic paddy soil (Fey and Conrad, 2000). However, acetate concentrations are usually sufficiently high to allow exergonic CH4 production in methanogenic ecosystems (Klüber and Conrad, 1998; Yao and Conrad, 1999; Beer and Blodau, 2007). The decrease of acetate concentrations observed by Fey and Conrad (2000) was consistent with a change in the active methanogenic populations from a predominance of Methanosarcina to Methanothrix with relatively high versus low acetate thresholds (Jetten et al., 1992).
Temperature dependence of carbon flow
Methane production from organic carbon follows characteristic degradation pathways that depend on which substrate the methanogenic archaea utilize. The major substrates of methanogenic archaea are acetate, H2 + CO2 and methyl compounds such as methanol, trimethyl amine or dimethyl sulfide. In most natural environments it is acetate and H2 + CO2 that dominate (Conrad, 1999; Conrad, 2020). The pathway of carbon flow providing acetate and H2 + CO2 from the degradation of organic matter may vary considerably between 100% acetate and 100% H2 + CO2 and any mixture in between (Conrad, 2020). Polysaccharides are an important class of organic matter. Cellulose and xylane are the major forms of dead plant material. Complete methanogenic degradation of cellulose occurs in four major steps, i.e., (1) hydrolysis and primary fermentation, (2) syntrophic secondary fermentation, (3) hydrogenotrophic methanogenesis, and (4) aceticlastic methanogenesis. Because of simplicity the following stoichiometries present lactate as sole product of primary fermentation. In methanogenic environments, other primary fermentation products, such as propionate, are actually more important. However, the principles of degradation are the same (Figure 1):
1: C6H12O6 → 2CH3CHOHCOOH
2: 2CH3CHOHCOOH + 2H2O → 2CH3COOH + 2CO2 + 4H2
3: 4H2 + CO2 → CH4 + 2H2O
4: 2CH3COOH → 2CH4 + 2CO2
Sum: C6H12O2 → 3CH4 + 3CO2
The pathway starts with primary fermentation (1) of sugar to lactic acid, followed by secondary syntrophic fermentation (2) of lactic acid to acetate, CO2 and H2. There are alternative pathways, e.g., via ethanol, butyrate or propionate, which all eventually result in the formation of 2 acetate, 2 CO2 and 4 H2. In such ‘syntrophic’ pathways aceticlastic and hydrogenotrophic methanogenesis contribute 67% (2 CH4) and 33% (1 CH4) to total CH4 production (3 CH4), respectively. The ‘syntrophic’ pathway is quite common for many methanogenic environments including anaerobic digestors (Zehnder, 1978; McCarty and Smith, 1986), rice field soils (Conrad and Frenzel, 2002; Conrad, 2007), peat bogs (Drake et al., 2009; Lai, 2009) and lake sediment (Ward and Winfrey, 1985; Capone and Kiene, 1988), especially at moderate temperatures (20–35°C).
Many studies have shown that the anaerobic degradation pathway can change with the in-situ temperature (Conrad, 2020). At low temperatures, in particular, the contribution of acetate can increase to 100%. This may happen when hydrogenotrophic methanogenesis (3) is replaced by with chemolithotrophic homoacetogenesis (5) (Figure 1):
1: C6H12O6 → 2CH3CHOHCOOH
2: 2CH3CHOHCOOH + 2H2O → 2CH3COOH + 2CO2 + 4H2
5: 4H2 + 2CO2 → CH3COOH + 2H2O
4: 3CH3COOH → 3CH4 + 3CO2
Sum: C6H12O2 → 3CH4 + 3CO2
Or it may happen when sugar fermentation to lactic acid (1) is replaced by homoacetogenic sugar fermentation (6):
6: C6H12O6 → 3CH3COOH
4: CH3COOH → 3CH4 + 3CO2
Sum: C6H12O2 → 3CH4 + 3CO2
It has been shown for many anoxic environments that homoacetogenesis is a favored process at low (<15°C) temperatures (Conrad et al., 1989; Nozhevnikova et al., 1994), so that hydrogenotrophic methanogenesis decreases relative to aceticlastic methanogenesis. A dominance of aceticlastic methanogenesis at low temperatures has been observed in rice paddy fields (Chin and Conrad, 1995; Fey and Conrad, 2000; Fu et al., 2018), lake sediments (Schulz and Conrad, 1996; Nozhevnikova et al., 1997; Glissmann et al., 2004) and soils (Küsel and Drake, 1995; Fu et al., 2015). However, it has not been observed in anaerobic marine sediments (Roussel et al., 2015) and in many boreal and arctic peat bogs (Kotsyurbenko et al., 2004; Metje and Frenzel, 2007; Tveit et al., 2015). In an Alaskan bog, CH4 is not produced from acetate, although it is the dominant product of organic matter degradation. Instead, it is only degraded by oxic respiration and ferric iron reduction (Duddleston et al., 2002). Hence, although acetogenesis and acetoclastic methanogenesis seems to be enhanced at low temperatures, the degradation of organic matter via both acetate and H2 + CO2 is not excluded.
Also at high temperature (>45°C), aceticlastic and hydrogenotrophic methanogenesis can operate producing 67 and 33% of the CH4, respectively, as observed in anaerobic digestors (Zinder et al., 1984; Vanlier et al., 1993; Gehring et al., 2015) and in rice field soils (Liu et al., 2018). This is the case, since thermophilic or thermotolerant microbes exist, which act as syntrophic secondary fermenters, as aceticlastic methanogens and as hydrogenotrophic methanogens (Vanlier et al., 1996; Imachi et al., 2000). Frequently, however, the contribution of H2 + CO2 increases at high temperatures to 100%, as acetate is consumed by syntrophic acetate oxidation (7) coupled to hydrogenotrophic methanogenesis (3) (Figure 1):
1: C6H12O6 → 2CH3CHOHCOOH
2: 2CH3CHOHCOOH + 2H2O → 2CH3COOH + 2CO2 + 4H2
7: 2CH3COOH + 4H2O → 8H2 + 4CO2
3: 12H2 + 3CO2 → 3CH4 + 6H2O
Sum: C6H12O2 → 3CH4 + 3CO2
The existence of syntrophic acetate oxidizing bacteria (7) and their general preference for high temperatures has been shown in anaerobic sludge digestors (Lee and Zinder, 1988a; Ahring, 1995; Hattori, 2008; Tang et al., 2008; Ho et al., 2013) and rice paddy fields (Fey et al., 2001; Conrad et al., 2009; Liu and Conrad, 2010; Rui et al., 2011; Liu et al., 2018). Evidence for syntrophic acetate oxidation at elevated temperature has also been found in an alkaline wetland soil in Tibet (Deng et al., 2019).
Although empirical studies have frequently shown that environments at intermediate temperatures (mesophilic) are dominated by the ‘syntrophic’ pathway (67% acetate, 33% H2/CO2), low temperatures (psychrophilic) by the acetogenic pathway (<67% acetate), and high temperatures (thermophilic) by the hydrogenotrophic pathway (100% H2/CO2), such preferences are by no means obligatory. The mechanistic reasons for these preferences are not quite clear. A look at the thermodynamics of the degradation pathways may be helpful. The values of ΔGo are constrained by the stoichiometries of the pathways. The values change with temperature according to the magnitude of ΔSo (Table 1). Therefore, the ΔGoT values of the different degradation steps also change with temperature. The ΔGoT of the entire process (cellulose → 3CH4 + 3CO2) is always −405, −426, and −445 kJ at 5, 25, and 45°C, thus moderately decreasing (becoming more exergonic) with temperature. The ΔGo’T values of the individual reactions at 5, 25, and 45°C are shown in Figure 4. The data demonstrate that syntrophic secondary fermentation reactions producing H2 become increasingly less endergonic or more exergonic, if temperature increases. Figure 4 only shows the syntrophic degradation of lactate and acetate, but that of ethanol, butyrate, and propionate would be in-between. As a consequence, thermodynamics suggest that hydrogenotrophic and ‘syntrophic’ pathways are favored at increasing temperature. Since the acetogenic pathway from sugars (reaction 6 in Figure 1) is thermodynamically feasible at every temperature (small ΔSo, Table 1) this may be the reason why it predominates at low temperatures where the other degradation pathways are thermodynamically restricted. Although homoacetogenesis from H2 + CO2 is thermodynamically less favorable than hydrogenotrophic methanogenesis, and results in relatively higher H2 thresholds (Figure 3), the former can possibly prevail since psychrophilic homoacetogens seem to exist in many environments while psychrophilic methanogens are missing (Conrad et al., 1989; Kotsyurbenko et al., 2001). In addition homoacetogens can operate in a mixotrophic way. Homoacetogenic bacteria are able to use many different substrates. Therefore, thresholds for H2 can be lower with two substrates than with H2 alone (Peters et al., 1998).
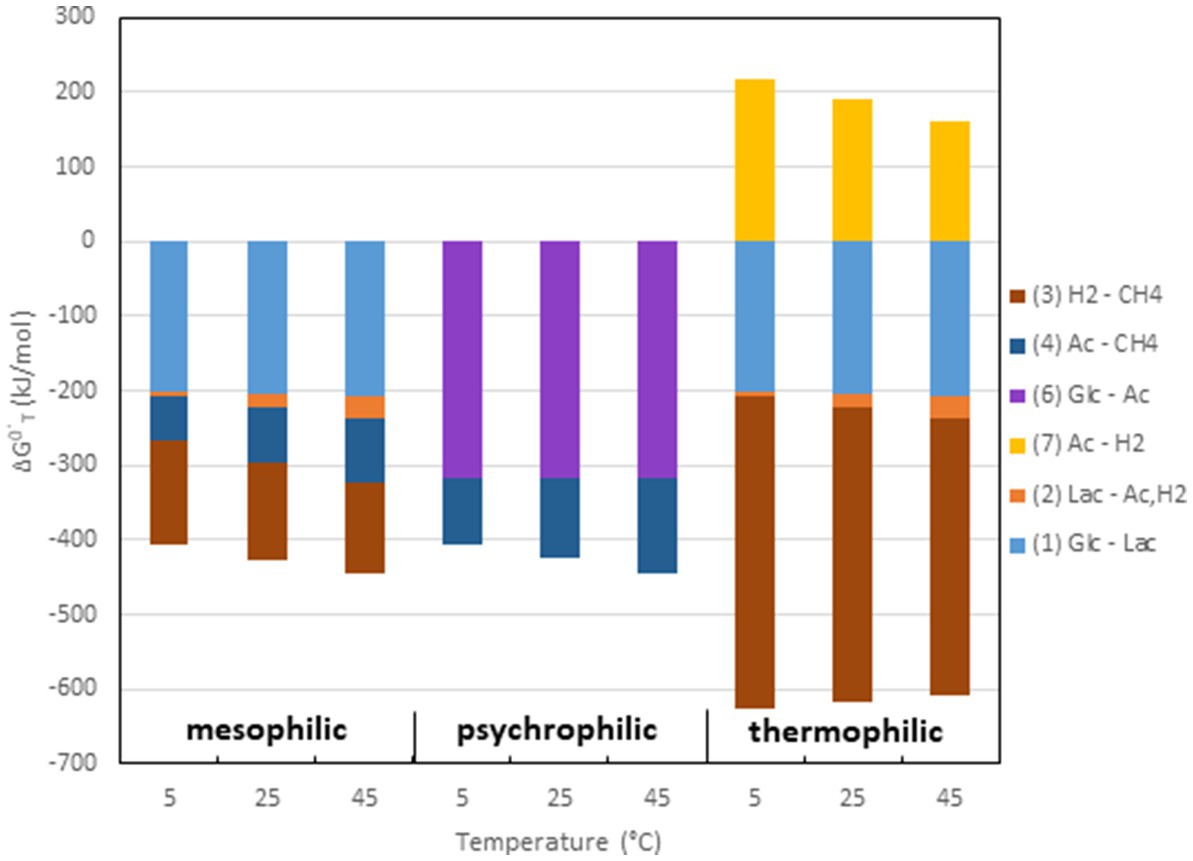
Figure 4. Values of ΔG0’T at 5, 25, and 45°C of the different processes shown in Figure 1 and listed in Table 1. The processes are combined for typical reaction chains of mesophilic, psychrophilic and thermophilic degradation of organic matter to CH4 + CO2.
However, the dominance of the hydrogenotrophic pathway at high temperatures requires different explanations. In fact, syntrophic degradation of lactate (or ethanol, butyrate, propionate) and aceticlastic methanogenesis both become thermodynamically more favorable when temperature increases (Figure 4). Thus, why should aceticlastic methanogenesis be replaced by syntrophic acetate oxidation and hydrogenotrophic methanogenesis? The minimum threshold concentration of acetate can be calculated from the respective ΔGoT values using he Nernst equation. However, the ΔGoT values are the same independently by which mechanism the conversion of acetate is achieved and so are the minimum thresholds of acetate. At the time being, one can only speculate why syntrophic acetate oxidation is dominant at high temperature. One possibility is that cell integrity of aceticlastic methanogens is worse at high temperatures than that of hydrogenotrophic methanogens. However, thermophilic species do exist for both aceticlastic genera Methanothrix and Methanosarcina (Zinder and Mah, 1979; Zinder et al., 1987; Kamagata et al., 1992; Mladenovska and Ahring, 2000). Another possibility is that it is preferable that the available energy is shared by two rather than one organism. Such sharing is not uncommon, e.g., oxidation of ammonia to nitrate by either one (comammox; Daims et al., 2015) or more commonly by two bacterial species (e.g., Nitrosomonas, Nitrobacter). Sharing reduces the length of the pathway (number of enzymatic steps) for the individual species, which may be favorable for energetic reasons (Costa et al., 2006). Since thermodynamics become more favorable at increasing temperatures, the syntrophic sharing option may become more attractive.
In fact, energy sharing among different physiological groups seems to be common for anaerobic breakdown of organic matter, even for simple sugars. This is seen in the multiple syntrophic degradation processes (e.g., of lactate, ethanol, butyrate, propionate), in which energy is usually shared by at least three different microbes, (1) the secondary fermenters producing H2, acetate and CO2 as final products, (2) hydrogenotrophic methanogens and (3) aceticlastic methanogens (Schink, 1997). Three partners have to share the energy content of substrate degradation. It is amazing, that degradation is not simply achieved by a single microbe, i.e., a methanogen, which would convert the substrate to CH4 + CO2 + H2O without having to share the energy. However, such methanogens are unknown, probably since their evolution was not competitive against the shared mode of metabolism.
Temperature dependence of microbial community composition
The change of the pathway of organic matter degradation with increasing temperature makes it likely, that the microbial community responsible for the methanogenic degradation also changes. This is indeed the case. Many studies exist for rice field soils (Figure 5). Thus, the communities of methanogenic archaea are different at low, medium and elevated temperatures (Chin et al., 1999; Fey and Conrad, 2000; Wu et al., 2002; Rui et al., 2009; Peng et al., 2018; Liu et al., 2019). Most notable is the change from mesophilic to moderately thermophilic conditions, which shows a decrease of aceticlastic Methanosarcina and Methanothrix species and an increase of thermophilic Methanocella species (formerly RC-I), which are hydrogenotrophic (Conrad et al., 2009; Lu et al., 2015; Liu et al., 2018; Peng et al., 2018). Hence hydrogenotrophic methanogens are most likely involved in the syntrophic degradation of acetate under thermophilic conditions. The population size of putative syntrophic acetate oxidizers is also enhanced under thermophilic conditions, namely Thermoanaerobacter species relatively increase (Liu and Conrad, 2010; Rui et al., 2011; Liu et al., 2018; Peng et al., 2018). However, moderately thermophilic methanogens are ubiquitous in the environment, members of the hydrogenotrophic genus Methanocella (Fey et al., 2001), but also other hydrogenotrophic genera [e.g., Methanobacterium, Methanoregula (Peng et al., 2008; Deng et al., 2019)] and aceticlastic Methanosarcina and Methanothrix as well (Wu et al., 2006). Therefore, it is not surprising that rice fields exist in which thermophilic degradation of acetate is achieved by canonical aceticlastic methanogenesis (Liu et al., 2018). Nevertheless, even with aceticlastic methanogenesis the methanogenic microbial communities form a different network at high versus moderate temperatures (Peng et al., 2018; Liu et al., 2019).
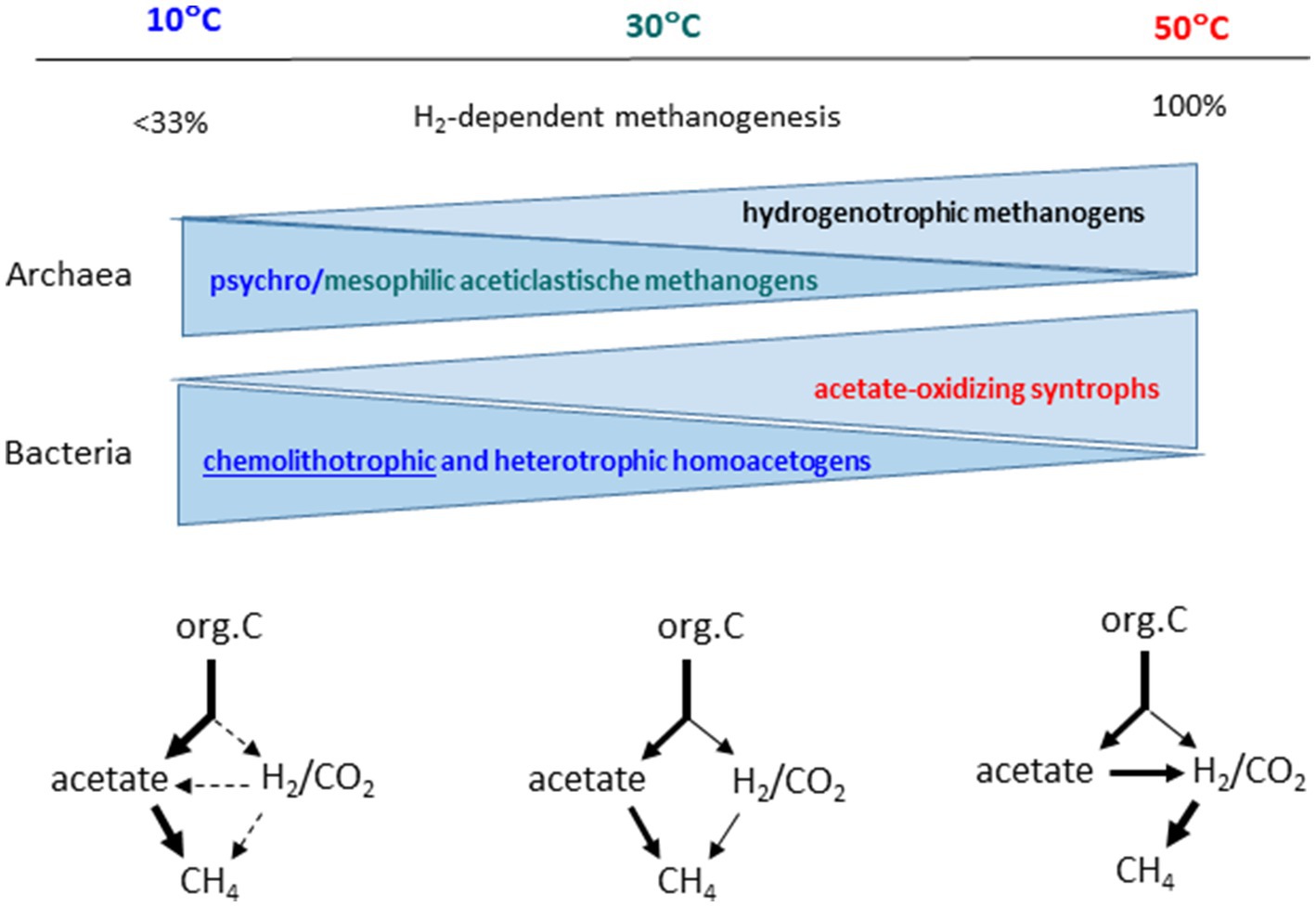
Figure 5. Relative contribution of hydrogenotrophic methanogenesis, composition of the microbial communities, and pathways of methanogenic degradation of organic matter as being characteristic for psychrophilic (10°C), mesophilic (30°C) and thermophilic (50°C) conditions.
Thermophilic syntrophic acetate conversion also has frequently been observed in anaerobic digestor systems usually fed with waste or waste water. The microbial communities under mesophilic versus thermophilic conditions are generally different. However, the taxa of putative thermophilic syntrophic acetate oxidizers are quite diverse in different digestors. Early enrichments resulted in an unnamed homoacetogenic bacterium (Lee and Zinder, 1988b) and in Thermoacetogenium phaeum (Hattori et al., 2000; Hattori, 2008). Later analyses of digestor communities using molecular tools indicate the operation of various taxa of syntrophic acetate oxidizers, such as Thermotogae, Dethiobacteraceae, Clostridium, Hydrogenophaga, Fervidobacterium, Spirochaeta, Limnohabitans, Rhodococcus, Thermoacetogenium, Tepidiphilus, Petrobacter (Leven et al., 2007; Tang et al., 2008; Hao et al., 2011; Sun et al., 2018; Dyksma et al., 2020). Thermophilic methanogens include the genera Methanobacterium, Methanoculleus, Methanothermobacter, and Methanosarcina (Leven et al., 2007; Tang et al., 2008; Hao et al., 2011; Ho et al., 2013; Dyksma et al., 2020). Note, however, that mesophilic syntrophic acetate oxidizers also exist, e.g., Clostridium ultunense (Schnürer et al., 1996) or Syntrophaceticus schinkii (Westerholm et al., 2010).
Psychrophilic conditions also affect the methanogenic microbial communities in rice paddy soils, but the effects are more delicate than the difference between mesophilic and thermophilic conditions. Usually, homoactogenic activities are enhanced at low temperature (Figure 5). The acetogenic populations seem to belong to the Clostridium cluster I and Peptococcaceae (Liu and Conrad, 2011). Enhanced acetate production at low temperature is followed by consumption by aceticlastic methanogens of the genera Methanosarcina and Methanothrix (Chin et al., 1999; Wu et al., 2002; Peng et al., 2008). The overall diversity of methanogenic archaea seems to increase at low temperature, Methanothrix in particular (Chin et al., 1999; Wu et al., 2002).
Different microbial communities at psychrophilic versus mesophilic conditions were also observed in peat bogs and arctic wetlands (Hoej et al., 2008; Blake et al., 2015; Schmidt et al., 2015, 2016; Kolton et al., 2019). The taxa Methanosarcina, Methanothrix, Methanobacteriaceae, Methanoregulaceae, and Methanocella are generally common, but the aceticlastic genera (Methanosarcina, Methanothrix) are especially abundant at low temperatures. These taxa have also been found in a boreal mire, but with little changes in community composition over a seasonal temperature gradient between 0 and 14°C (Juottonen et al., 2008). The bacterial communities in peat lands have been found to be dominated by Clostridiaceae (Kolton et al., 2019) and by Pelobacter, Syntrophobacteraceae, Syntrophaceae and Syntrophorhabdaceae as potential secondary fermenters (Schmidt et al., 2015, 2016).
Conclusion
Methanogenic microbial communities catalyze the anaerobic degradation of organic matter to CO2 and CH4. This process is basically the same in various environments, such as rice paddy fields, wetlands, lake sediments, peat bogs, anaerobic digestors, or the intestinal tract of animals. The pathway of the process is also basically the same. Irrespective of the particular environment, the degradation steps and the metabolic classes of the microrganisms involved are basically the same (Figure 1). Nevertheless, the exact composition of the methanogenic microbial communities can be quite different in the different environments. Furthermore, the composition can change with temperature, thus resulting in a change of the pathway of the degradation process. Thus, it is frequently observed that the pathway and the responsible methanogenic microbial community changes from psychrophilic to mesophilic to thermophilic conditions, with dominance of aceticlastic methanogenesis at low and hydrogenotrophic methanogenesis at high temperatures (Figure 5). Such behavior is consistent with thermodynamics of the critical steps in organic matter degradation, but is nevertheless not obligatory. Thus, environments exist, in which CH4 production is dominated by hydrogenotrophic methanogenesis despite low temperatures and aceticlastic methanogenesis despite high temperatures, simply because these environments contain the respective psychrophilic and thermophilic species.
Microorganisms proliferate within and tolerate a more or less wide range of temperatures. This is observed in environments that experience only small temperature fluctuations (lake sediments, technical digesters, hot springs) and also in others that experience dramatic temperature changes on a daily or seasonal range (littoral sediments, rice paddies, peatlands). The microorganisms generally display characteristic temperature optima and a characteristic increase of reaction kinetics with increasing temperature, which can be modelled by the Arrhenius equation using a characteristic apparent activation energy (Ea). Interestingly, methanogenic archaea were found to exhibit a rather high range of Ea values of >100 kJ mol−1. In the environment, hydrolysis of complex organic matter is the first step of the methanogenic degradation processes, and this process can exhibit markedly lower Ea values of about 60 kJ mol−1. However, analysis of various methanogenic environments with complex microbial communities display Ea values, which are not similar to those of hydrolysis but to those of the methanogenic archaea. This observation indicates that hydrolysis of organic matter is not the rate limiting step of CH4 production in most environments, meaning that the microbial community is frequently supplied with pulses of easily degradable substrates.
Author contributions
The author confirms being the sole contributor of this work and has approved it for publication.
Acknowledgments
I thank Rolf Thauer for encouragement to write this review and for valuable discussion.
Conflict of interest
The author declares that the research was conducted in the absence of any commercial or financial relationships that could be construed as a potential conflict of interest.
Publisher’s note
All claims expressed in this article are solely those of the authors and do not necessarily represent those of their affiliated organizations, or those of the publisher, the editors and the reviewers. Any product that may be evaluated in this article, or claim that may be made by its manufacturer, is not guaranteed or endorsed by the publisher.
References
Ahring, B. K. (1995). Methanogenesis in thermophilic biogas reactors. Ant. Leeuwenhoek 67, 91–102. doi: 10.1007/BF00872197
Ahring, B. K., and Westermann, P. (1988). Product inhibition of butyrate metabolism by acetate and hydrogen in a thermophilic coculture. Appl. Environ. Microbiol. 54, 2393–2397. doi: 10.1128/aem.54.10.2393-2397.1988
Allen, A. P., Gillooly, J. F., and Brown, J. H. (2005). Linking the global carbon cycle to individual metabolism. Funct. Ecol. 19, 202–213. doi: 10.1111/j.1365-2435.2005.00952.x
Arnosti, C., and Joergensen, B. B. (2003). High activity and low temperature optima of extracellular enzymes in Arctic sediments: implications for carbon cycling by heterotrophic microbial communities. Mar. Ecol. Prog. Ser. 249, 15–24. doi: 10.3354/meps249015
Arnosti, C., and Joergensen, B. B. (2006). Organic carbon degradation in arctic marine sediments, Svalbard: A comparison of initial and terminal steps. Geomicrobiol J. 23, 551–563. doi: 10.1080/01490450600897336
Arnosti, C., Joergensen, B. B., Sagemann, J., and Thamdrup, B. (1998). Temperature dependence of microbial degradation of organic matter in marine sediments - polysaccharide hydrolysis, oxygen consumption, and sulfate reduction. Mar. Ecol. Prog. Ser. 165, 59–70. doi: 10.3354/meps165059
Beaty, P. S., and McInerney, M. J. (1989). Effects of organic acid anions on the growth and metabolism of Syntrophomonas wolfei in pure culture and in defined consortia. Appl. Environ. Microbiol. 55, 977–983. doi: 10.1128/aem.55.4.977-983.1989
Beer, J., and Blodau, C. (2007). Transport and thermodynamics constrain belowground carbon turnover in a northern peatland. Geochim. Cosmochim. Acta 71, 2989–3002. doi: 10.1016/j.gca.2007.03.010
Bilek, R. S., Tyler, S. C., Sass, R. L., and Fisher, F. M. (1999). Differences in CH4 oxidation and pathways of production between rice cultivars deduced from measurements of CH4 flux and δ13C of CH4 and CO2. Glob. Biogeochem. Cycles 13, 1029–1044. doi: 10.1029/1999GB900040
Blake, L. I., Tveit, A., Oevreas, L., Head, I. M., and Gray, N. D. (2015). Response of methanogens in arctic sediments to temperature and methanogenic substrate availability. PLoS One 10:e0129733. doi: 10.1371/journal.pone.0129733
Button, D. K. (1985). Kinetics of nutrient-limited transport and microbial growth. Microbiol. Rev. 49, 270–297. doi: 10.1128/mr.49.3.270-297.1985
Caccavo, F., Blakemore, R. P., and Lovley, D. R. (1992). A hydrogen-oxidizing, Fe(III)-reducing microorganism from the Great Bay estuary, New-Hampshire. Appl. Environ. Microbiol. 58, 3211–3216. doi: 10.1128/aem.58.10.3211-3216.1992
Capone, D. G., and Kiene, R. P. (1988). Comparison of microbial dynamics in marine and freshwater sediments: contrasts in anaerobic carbon catabolism. Limnol. Oceanogr. 33, 725–749. doi: 10.4319/lo.1988.33.4_part_2.0725
Cavicchioli, R. (2006). Cold-adapted archaea. Nat. Rev. Microbiol. 4, 331–343. doi: 10.1038/nrmicro1390
Chin, K. J., and Conrad, R. (1995). Intermediary metabolism in methanogenic paddy soil and the influence of temperature. FEMS Microbiol. Ecol. 18, 85–102. doi: 10.1111/j.1574-6941.1995.tb00166.x
Chin, K. J., Lukow, T., and Conrad, R. (1999). Effect of temperature on structure and function of the methanogenic archaeal community in an anoxic rice field soil. Appl. Environ. Microbiol. 65, 2341–2349. doi: 10.1128/AEM.65.6.2341-2349.1999
Chin, K. J., Rainey, F. A., Janssen, P. H., and Conrad, R. (1998). Methanogenic degradation of polysaccharides and the characterization of polysaccharolytic clostridia from anoxic rice field soil. Syst. Appl. Microbiol. 21, 185–200. doi: 10.1016/S0723-2020(98)80023-4
Conrad, R. (1999). Contribution of hydrogen to methane production and control of hydrogen concentrations in methanogenic soils and sediments. FEMS Microbiol. Ecol. 28, 193–202. doi: 10.1111/j.1574-6941.1999.tb00575.x
Conrad, R. (2007). Microbial ecology of methanogens and methanotrophs. Adv. Agron. 96, 1–63. doi: 10.1016/S0065-2113(07)96005-8
Conrad, R. (2008). “Temperature effects on methanogenic microbial communities” in Microbes and the environment: Perspectives and challenges. eds. S. J. Liu and H. L. Drake (Beijing: Science Press), 35–40.
Conrad, R. (2020). Importance of hydrogenotrophic, aceticlastic and methylotrophic methanogenesis for methane production in terrestrial, aquatic and other anoxic environments: A mini review. Pedosphere 30, 25–39. doi: 10.1016/S1002-0160(18)60052-9
Conrad, R., Bak, F., Seitz, H. J., Thebrath, B., Mayer, H. P., and Schütz, H. (1989). Hydrogen turnover by psychrotrophic homoacetogenic and mesophilic methanogenic bacteria in anoxic paddy soil and lake sediment. FEMS Microbiol. Ecol. 62, 285–293. doi: 10.1111/j.1574-6968.1989.tb03382.x
Conrad, R., and Frenzel, P. (2002). “Flooded soils” in Encyclopedia of environmental microbiology. ed. G. Bitton (New York: John Wiley & Sons, Inc), 1316–1333.
Conrad, R., Klose, M., and Noll, M. (2009). Functional and structural response of the methanogenic microbial community in rice field soil to temperature change. Environ. Microbiol. 11, 1844–1853. doi: 10.1111/j.1462-2920.2009.01909.x
Conrad, R., Schink, B., and Phelps, T. J. (1986). Thermodynamics of H2-producing and H2-consuming metabolic reactions in diverse methanogenic environments under in-situ conditions. FEMS Microbiol. Ecol. 38, 353–360.
Conrad, R., Schütz, H., and Babbel, M. (1987). Temperature limitation of hydrogen turnover and methanogenesis in anoxic paddy soil. FEMS Microbiol. Ecol. 45, 281–289. doi: 10.1111/j.1574-6968.1987.tb02378.x
Conrad, R., and Wetter, B. (1990). Influence of temperature on energetics of hydrogen metabolism in homoacetogenic, methanogenic, and other anaerobic bacteria. Arch. Microbiol. 155, 94–98. doi: 10.1007/BF00291281
Cord-Ruwisch, R., Seitz, H. J., and Conrad, R. (1988). The capacity of hydrogenotrophic anaerobic bacteria to compete for traces of hydrogen depends on the redox potential of the terminal electron acceptor. Arch. Microbiol. 149, 350–357. doi: 10.1007/BF00411655
Costa, E., Perez, J., and Kreft, J. U. (2006). Why is metabolic labour divided in nitrification? Trends Microbiol. 14, 213–219. doi: 10.1016/j.tim.2006.03.006
Daims, H., Lebedeva, E. V., Pjevac, P., Han, P., Herbold, C., Albertsen, M., et al. (2015). Complete nitrification by Nitrospira bacteria. Nature 528, 504–509. doi: 10.1038/nature16461
Deng, Y., Liu, P., and Conrad, R. (2019). Effect of temperature on the microbial community responsible for methane production in alkaline NamCo wetland soil. Soil Biol. Biochem. 132, 69–79. doi: 10.1016/j.soilbio.2019.01.024
Dolfing, J. (2013). Syntrophic propionate oxidation via butyrate: a novel window of opportunity under methanogenic conditions. Appl. Environ. Microbiol. 79, 4515–4516. doi: 10.1128/AEM.00111-13
Dolfing, J., and Tiedje, J. M. (1988). Acetate inhibition of methanogenic, syntrophic benzoate degradation. Appl. Environ. Microbiol. 54, 1871–1873. doi: 10.1128/aem.54.7.1871-1873.1988
Drake, H. L. (1994). “Acetogenesis, acetogenic bacteria, and the acetyl-CoA "Wood/Ljungdahl" pathway: past and current perspectives” in Acetogenesis. ed. H. L. Drake (New York: Chapman & Hall), 3–60.
Drake, H. L., Horn, M. A., and Wüst, P. K. (2009). Intermediary ecosystem metabolism as a main driver of methanogenesis in acidic wetland soil. Environ. Microbiol. Rep. 1, 307–318. doi: 10.1111/j.1758-2229.2009.00050.x
Duddleston, K. N., Kinney, M. A., Kiene, R. P., and Hines, M. E. (2002). Anaerobic microbial biogeochemistry in a northern bog: acetate as a dominant metabolic end product. Glob. Biogeochem. Cycles 16:11-1. doi: 10.1029/2001GB001402
Dyksma, S., Jansen, L., and Gallert, C. (2020). Syntrophic acetate oxidation replaces acetoclastic methanogenesis during thermophilic digestion of biowaste. Microbiome 8:105. doi: 10.1186/s40168-020-00862-5
Fey, A., Chin, K. J., and Conrad, R. (2001). Thermophilic methanogens in rice field soil. Environ. Microbiol. 3, 295–303. doi: 10.1046/j.1462-2920.2001.00195.x
Fey, A., and Conrad, R. (2000). Effect of temperature on carbon and electron flow and on the archaeal community in methanogenic rice field soil. Appl. Environ. Microbiol. 66, 4790–4797. doi: 10.1128/AEM.66.11.4790-4797.2000
Fey, A., and Conrad, R. (2003). Effect of temperature on the rate limiting step in the methanogenic degradation pathway in rice field soil. Soil Biol. Biochem. 35, 1–8. doi: 10.1016/S0038-0717(02)00175-X
Franzmann, P. D., Liu, Y. T., Balkwill, D. L., Aldrich, H. C., ConwayDeMacario, E., and Boone, D. R. (1997). Methanogenium frigidum sp. nov., a psychrophilic, H2-using methanogen from Ace Lake, Antarctica. Int. J. Syst. Bacteriol. 47, 1068–1072. doi: 10.1099/00207713-47-4-1068
Fu, B., Conrad, R., and Blaser, M. (2018). Potential contribution of acetogenesis to anaerobic degradation in methanogenic rice field soils. Soil Biol. Biochem. 119, 1–10. doi: 10.1016/j.soilbio.2017.10.034
Fu, L., Song, T., and Lu, Y. (2015). Snapshot of methanogen sensitivity to temperature in Zoige wetland from Tibetan plateau. Front. Microbiol. 6:131. doi: 10.3389/fmicb.2015.00131
Games, L. M., Hayes, J. M., and Gunsalus, R. P. (1978). Methane-producing bacteria: natural fractionations of the stable carbon isotopes. Geochim. Cosmochim. Acta 42, 1295–1297. doi: 10.1016/0016-7037(78)90123-0
Gehring, T., Klang, J., Niedermayr, A., Berzio, S., Immenhauser, A., Klocke, M., et al. (2015). Determination of methanogenic pathways through carbon isotope (δ13C) analysis for the two-stage anaerobic digestion of high-solids substrates. Environ. Sci. Technol. 49, 4705–4714. doi: 10.1021/es505665z
Glissmann, K., Chin, K. J., Casper, P., and Conrad, R. (2004). Methanogenic pathway and archaeal community structure in the sediment of eutrophic Lake Dagow: effect of temperature. Microb. Ecol. 48, 389–399. doi: 10.1007/s00248-003-2027-2
Goevert, D., and Conrad, R. (2009). Effect of substrate concentration on carbon isotope fractionation during acetoclastic methanogenesis by Methanosarcina barkeri and M. acetivorans and in rice field soil. Appl. Environ. Microbiol. 75, 2605–2612. doi: 10.1128/AEM.02680-08
Hao, L. P., Lu, F., He, P. J., Li, L., and Shao, L. M. (2011). Predominant contribution of syntrophic acetate oxidation to thermophilic methane formation at high acetate concentrations. Environ. Sci. Technol. 45, 508–513. doi: 10.1021/es102228v
Hattori, S. (2008). Syntrophic acetate-oxidizing microbes in methanogenic environments. Microbes Environ. 23, 118–127. doi: 10.1264/jsme2.23.118
Hattori, S., Kamagata, Y., Hanada, S., and Shoun, H. (2000). Thermoacetogenium phaeum gen. nov., sp nov., a strictly anaerobic, thermophilic, syntrophic acetate-oxidizing bacterium. Int. J. Syst. Evol. Microbiol. 50, 1601–1609. doi: 10.1099/00207713-50-4-1601
Ho, D. P., Jensen, P. D., and Batstone, D. J. (2013). Methanosarcinaceae and acetate-oxidizing pathways dominate in high-rate thermophilic anaerobic digestion of waste-activated sludge. Appl. Environ. Microbiol. 79, 6491–6500. doi: 10.1128/AEM.01730-13
Hobbs, J. K., Jiao, W., Easter, A. D., Parker, E. J., Schipper, L. A., and Arcus, V. L. (2013). Change in heat capacity for enzyme catalysis determines temperature dependence of enzyme catalyzed rates. ACS Chem. Biol. 8, 2388–2393. doi: 10.1021/cb4005029
Hoehler, T. M. (2004). Biological energy requirements as quantitative boundary conditions for life in the subsurface. Geobiology 2, 205–215. doi: 10.1111/j.1472-4677.2004.00033.x
Hoehler, T. M., and Alperin, M. J. (2014). Methane minimalism. Nature 507, 436–437. doi: 10.1038/nature13215
Hoehler, T. M., Alperin, M. J., Albert, D. B., and Martens, C. S. (1998). Thermodynamic control on hydrogen concentrations in anoxic sediments. Geochim. Cosmochim. Acta 62, 1745–1756.
Hoehler, T. M., Alperin, M. J., Albert, D. B., and Martens, C. S. (2001). Apparent minimum free energy requirements for methanogenic Archaea and sulfate-reducing bacteria in an anoxic marine sediment. FEMS Microbiol. Ecol. 38, 33–41. doi: 10.1111/j.1574-6941.2001.tb00879.x
Hoej, L., Olsen, R. A., and Torsvik, V. L. (2008). Effects of temperature on the diversity and community structure of known methanogenic groups and other archaea in high Arctic peat. ISME J. 2, 37–48. doi: 10.1038/ismej.2007.84
Hoh, C. Y., and Cord-Ruwisch, R. (1996). A practical kinetic model that considers endproduct inhibition in anaerobic digestion processes by including the equilibrium constant. Biotechnol. Bioeng. 51, 597–604. doi: 10.1002/(SICI)1097-0290(19960905)51:5<597::AID-BIT12>3.0.CO;2-F
Imachi, H., Sekiguchi, Y., Kamagata, Y., Ohashi, A., and Harada, H. (2000). Cultivation and in situ detection of a thermophilic bacterium capable of oxidizing propionate in syntrophic association with hydrogenotrophic methanogens in a thermophilic methanogenic granular sludge. Appl. Environ. Microbiol. 66, 3608–3615. doi: 10.1128/AEM.66.8.3608-3615.2000
Jetten, M. S. M., Stams, A. J. M., and Zehnder, A. J. B. (1992). Methanogenesis from acetate - A comparison of the acetate metabolism in Methanothrix soehngenii and Methanosarcina spp. FEMS Microbiol. Rev. 88, 181–197.
Ji, Y., Liu, P., and Conrad, R. (2018). Change of the pathway of methane production with progressing anoxic incubation of paddy soil. Soil Biol. Biochem. 121, 177–184. doi: 10.1016/j.soilbio.2018.03.014
Juottonen, H., Tuittila, E. S., Juutinen, S., Fritze, H., and Yrjälä, K. (2008). Seasonality of rDNA- and rRNA-derived archaeal communities and methanogenic potential in a boreal mire. ISME J. 2, 1157–1168. doi: 10.1038/ismej.2008.66
Kamagata, Y., Kawasaki, H., Oyaizu, H., Nakamura, K., Mikami, E., Endo, G., et al. (1992). Characterization of three thermophilic strains of Methanothrix ("Methanosaeta") thermophila sp. nov. and rejection of Methanothrix ("Methanosaeta") thermoacetophila. Int. J. Syst. Bacteriol. 42, 463–468. doi: 10.1099/00207713-42-3-463
Kirschke, S., Bousquet, P., Ciais, P., Saunois, M., Canadell, J. G., Dlugokencky, E. J., et al. (2013). Three decades of global methane sources and sinks. Nat. Geosci. 6, 813–823. doi: 10.1038/ngeo1955
Klüber, H. D., and Conrad, R. (1998). Effects of nitrate, nitrite, NO and N2O on methanogenesis and other redox processes in anoxic rice field soil. FEMS Microbiol. Ecol. 25, 301–319. doi: 10.1016/S0168-6496(98)00011-7
Koga, Y. (2012). Thermal adaptation of the archaeal and bacterial lipid membranes. Archaea 789652-doi:10.1155/2012/789652 2012, 1–6. doi: 10.1155/2012/789652
Kolton, M., Marks, A., Wilson, R. M., Chanton, J. P., and Kostka, J. E. (2019). Impact of warming on greenhouse gas production and microbial diversity in anoxic peat from a Sphagnum-dominated bog (Grand Rapids, Minnesota, United States). Front. Microbiol. 10:870. doi: 10.3389/fmicb.2019.00870
Kotsyurbenko, O. R. (2005). Trophic interactions in the methanogenic microbial community of low-temperature terrestrial ecosystems. FEMS Microbiol. Ecol. 53, 3–13. doi: 10.1016/j.femsec.2004.12.009
Kotsyurbenko, O. R., Chin, K. J., Glagolev, M. V., Stubner, S., Simankova, M. V., Nozhevnikova, A. N., et al. (2004). Acetoclastic and hydrogenotrophic methane production and methanogenic populations in an acidic West-Siberian peat bog. Environ. Microbiol. 6, 1159–1173. doi: 10.1111/j.1462-2920.2004.00634.x
Kotsyurbenko, O. R., Glagolev, M. V., Nozhevnikova, A. N., and Conrad, R. (2001). Competition between homoacetogenic bacteria and methanogenic archaea for hydrogen at low temperature. FEMS Microbiol. Ecol. 38, 153–159. doi: 10.1111/j.1574-6941.2001.tb00893.x
Kotsyurbenko, O. R., Nozhevnikova, A. N., and Zavarzin, G. A. (1993). Methanogenic degradation of organic matter by anaerobic bacteria at low temperature. Chemosphere 27, 1745–1761. doi: 10.1016/0045-6535(93)90155-X
Krüger, M., Eller, G., Conrad, R., and Frenzel, P. (2002). Seasonal variation in pathways of CH4 production and in CH4 oxidation in rice fields determined by stable carbon isotopes and specific inhibitors. Glob. Chang. Biol. 8, 265–280. doi: 10.1046/j.1365-2486.2002.00476.x
Küsel, K., and Drake, H. L. (1995). Effects of environmental parameters on the formation and turnover of acetate by forest soils. Appl. Environ. Microbiol. 61, 3667–3675. doi: 10.1128/aem.61.10.3667-3675.1995
Lai, D. Y. F. (2009). Methane dynamics in northern peatlands: A review. Pedosphere 19, 409–421. doi: 10.1016/S1002-0160(09)00003-4
Lee, M. J., and Zinder, S. H. (1988a). Hydrogen partial pressures in a thermophilic acetate-oxidizing methanogenic coculture. Appl. Environ. Microbiol. 54, 1457–1461.
Lee, M. J., and Zinder, S. H. (1988b). Isolation and characterization of a thermophilic bacterium which oxidizes acetate in syntrophic association with a methanogen and which grows acetogenically on H2-CO2. Appl. Environ. Microbiol. 54, 124–129. doi: 10.1128/aem.54.1.124-129.1988
Leven, L., Eriksson, A. R., and Schnürer, A. (2007). Effect of process temperature on bacterial and archaeal communities in two methanogenic bioreactors treating organic household waste. FEMS Microbiol. Ecol. 59, 683–693. doi: 10.1111/j.1574-6941.2006.00263.x
Lever, M. A., Rogers, K. L., Lloyd, K. G., Overmann, J., Schink, B., Thauer, R. K., et al. (2015). Life under extreme energy limitation: a synthesis of laboratory- and field-based investigations. FEMS Microbiol. Rev. 39, 688–728. doi: 10.1093/femsre/fuv020
Li, H., Chang, J., Liu, P., Fu, L., Ding, D., and Lu, Y. (2015). Direct interspecies electron transfer accelerates syntrophic oxidation of butyrate in paddy soil enrichments. Environ. Microbiol. 17, 1533–1547. doi: 10.1111/1462-2920.12576
Liu, F. H., and Conrad, R. (2010). Thermoanaerobacteriaceae oxidize acetate in methanogenic rice field soil at 50°C. Environ. Microbiol. 12, 2341–2354. doi: 10.1111/j.1462-2920.2010.02289.x
Liu, F., and Conrad, R. (2011). Chemolithotrophic acetogenic H2/CO2 utilization in Italian rice field soil. ISME J. 5, 1526–1539. doi: 10.1038/ismej.2011.17
Liu, P. F., Klose, M., and Conrad, R. (2018). Temperature effects on structure and function of the methanogenic microbial communities in two paddy soils and one desert soil. Soil Biol. Biochem. 124, 236–244. doi: 10.1016/j.soilbio.2018.06.024
Liu, P., Klose, M., and Conrad, R. (2019). Temperature-dependent network modules of soil methanogenic bacterial and archaeal communities. Front. Microbiol. 10:496. doi: 10.3389/fmicb.2019.00496
Lovley, D. R. (1985). Minimum threshold for hydrogen metabolism in methanogenic bacteria. Appl. Environ. Microbiol. 49, 1530–1531. doi: 10.1128/aem.49.6.1530-1531.1985
Lovley, D. R., and Goodwin, S. (1988). Hydrogen concentrations as an indicator of the predominant terminal electron-accepting reactions in aquatic sediments. Geochim. Cosmochim. Acta 52, 2993–3003. doi: 10.1016/0016-7037(88)90163-9
Lu, Y., Fu, L., Lu, Y., Hugenholtz, F., and Ma, K. (2015). Effect of temperature on the structure and activity of a methanogenic archaeal community during rice straw decomposition. Soil Biol. Biochem. 81, 17–27. doi: 10.1016/j.soilbio.2014.10.031
Marotta, H., Pinho, L., Gudasz, C., Bastviken, D., Tranvik, L., and Enrich-Prast, A. (2014). Greenhouse gas production in low-latitude lake sediments responds strongly to warming. Nat. Clim. Chang. 4, 467–470. doi: 10.1038/nclimate2222
McCarty, P. L., and Smith, D. P. (1986). Anaerobic wastewater treatment. Environ. Sci. Technol. 20, 1200–1206. doi: 10.1021/es00154a002
McInerney, M. J., Struchtemeyer, C. G., Sieber, J., Mouttaki, H., Stams, A. J. M., Schink, B., et al. (2008). Physiology, ecology, phylogeny, and genomics of microorganisms capable of syntrophic metabolism. Ann. N. Y. Acad. Sci. 1125, 58–72. doi: 10.1196/annals.1419.005
Metje, M., and Frenzel, P. (2007). Methanogenesis and methanogenic pathways in a peat from subarctic permafrost. Environ. Microbiol. 9, 954–964. doi: 10.1111/j.1462-2920.2006.01217.x
Middelburg, J. J., Klaver, G., Nieuwenhuize, J., Wielemaker, A., DeHaas, W., Vlug, T., et al. (1996). Organic matter mineralization in intertidal sediments along an estuarine gradient. Mar. Ecol. Prog. Ser. 132, 157–168. doi: 10.3354/meps132157
Mladenovska, Z., and Ahring, B. K. (2000). Growth kinetics of thermophilic Methanosarcina spp. isolated from full-scale biogas plants treating animal manures. FEMS Microbiol. Ecol. 31, 225–229. doi: 10.1111/j.1574-6941.2000.tb00687.x
Montag, D., and Schink, B. (2018). Formate and hydrogen as electron shuttles in terminal fermentations in an oligotrophic freshwater lake sediment. Appl. Environ. Microbiol. 84, e01572–e01518. doi: 10.1128/AEM.01572-18
Nedwell, D. B. (1984). The input and mineralization of organic carbon in anaerobic aquatic sediments. Adv. Microb. Ecol. 7, 93–131. doi: 10.1007/978-1-4684-8989-7_3
Nedwell, D. B. (1999). Effect of low temperature on microbial growth: lowered affinity for substrates limits growth at low temperature. FEMS Microbiol. Ecol. 30, 101–111. doi: 10.1111/j.1574-6941.1999.tb00639.x
Neue, H. U., and Scharpenseel, H. W. (1987). Decomposition pattern of 14C-labeled rice straw in aerobic and submerged rice soils of the Philippines. Sci. Total Environ. 62, 431–434.
Northrop, D. B. (1981). The expression of isotope effects on enzyme-catalyzed reactions. Annu. Rev. Biochem. 50, 103–131. doi: 10.1146/annurev.bi.50.070181.000535
Nozhevnikova, A. N., Holliger, C., Ammann, A., and Zehnder, A. J. B. (1997). Methanogenesis in sediments from deep lakes at different temperatures (12-70oC). Water Sci. Technol. 36, 57–64. doi: 10.2166/wst.1997.0575
Nozhevnikova, A. N., Kotsyurbenko, O. R., and Simankova, M. V. (1994). “Acetogenesis at low temperature” in Acetogenesis. ed. H. L. Drake (New York: Chapman & Hall), 416–431.
Nozhevnikova, A. N., Simankova, M. V., Parshina, S. N., and Kotsyurbenko, O. R. (2001). Temperature characteristics of methanogenic archaea and acetogenic bacteria isolated from cold environments. Water Sci. Technol. 44, 41–48. doi: 10.2166/wst.2001.0460
Nozhevnikova, A. N., Zepp, K., Vazquez, F., Zehnder, A. J. B., and Holliger, C. (2003). Evidence for the existence of psychrophilic methanogenic communities in anoxic sediments of deep lakes. Appl. Environ. Microbiol. 69, 1832–1835. doi: 10.1128/AEM.69.3.1832-1835.2003
Parshina, S. N., Ermakova, A. V., Bomberg, M., and Detkova, E. N. (2014). Methanospirillum stamsii sp. nov., a psychrotolerant, hydrogenotrophic, methanogenic archaeon isolated from an anaerobic expanded granular sludge bed bioreactor operated at low temperature. Int. J. Syst. Evol. Microbiol. 64, 180–186. doi: 10.1099/ijs.0.056218-0
Peng, J., Lü, Z., Rui, J., and Lu, Y. (2008). Dynamics of the methanogenic archaeal community during plant residue decomposition in an anoxic rice field soil. Appl. Environ. Microbiol. 74, 2894–2901. doi: 10.1128/AEM.00070-08
Peng, J. J., Wegner, C. E., Bei, Q. C., Liu, P. F., and Liesack, W. (2018). Metatranscriptomics reveals a differential temperature effect on the structural and functional organization of the anaerobic food web in rice field soil. Microbiome 6:169. doi: 10.1186/s40168-018-0546-9
Penger, J., Conrad, R., and Blaser, M. (2014). Stable carbon isotope fractionation of six strongly fractionating microorganisms is not affected by growth temperature under laboratory conditions. Geochim. Cosmochim. Acta 140, 95–105. doi: 10.1016/j.gca.2014.05.015
Penning, H., Claus, P., Casper, P., and Conrad, R. (2006). Carbon isotope fractionation during acetoclastic methanogenesis by Methanosaeta concilii in culture and a lake sediment. Appl. Environ. Microbiol. 72, 5648–5652. doi: 10.1128/AEM.00727-06
Peters, V., Janssen, P. H., and Conrad, R. (1998). Efficiency of hydrogen utilization during unitrophic and mixotrophic growth of Acetobacterium woodii on hydrogen and lactate in the chemostat. FEMS Microbiol. Ecol. 26, 317–324. doi: 10.1111/j.1574-6941.1998.tb00516.x
Platen, H., Janssen, P. H., and Schink, B. (1994). Fermentative degradation of acetone by an enrichment culture in membrane-separated culture devices and in cell suspension. FEMS Microbiol. Lett. 122, 27–32. doi: 10.1111/j.1574-6968.1994.tb07138.x
Radmer, R. J., and Kok, B. (1979). Rate-temperature curves as an unambiguous indicator of biological activity in soil. Appl. Environ. Microbiol. 38, 224–228. doi: 10.1128/aem.38.2.224-228.1979
Rothfuss, F., and Conrad, R. (1993). Thermodynamics of methanogenic intermediary metabolism in littoral sediment of Lake Constance. FEMS Microbiol. Ecol. 12, 265–276. doi: 10.1111/j.1574-6941.1993.tb00039.x
Roussel, E. G., Cragg, B. A., Webster, G., Sass, H., Tang, X., Williams, A. S., et al. (2015). Complex coupled metabolic and prokaryotic community responses to increasing temperatures in anaerobic marine sediments: critical temperatures and substrate changes. FEMS. Microb. Ecol. 91:fiv084. doi: 10.1093/femsec/fiv084
Rui, J., Peng, J., and Lu, Y. (2009). Succession of bacterial populations during plant residue decomposition in rice field soil. Appl. Environ. Microbiol. 75, 4879–4886. doi: 10.1128/AEM.00702-09
Rui, J., Qiu, Q., and Lu, Y. (2011). Syntrophic acetate oxidation under thermophilic methanogenic condition in Chinese paddy field soil. FEMS Microbiol. Ecol. 77, 264–273. doi: 10.1111/j.1574-6941.2011.01104.x
Saunders, N. F. W., Thomas, T., Curmi, P. M. G., Mattick, J. S., Kuczek, E., Slade, R., et al. (2003). Mechanisms of thermal adaptation revealed from the genomes of the Antarctic Archaea Methanogenium frigidum and Methanococcoides burtonii. Genome Res. 13, 1580–1588. doi: 10.1101/gr.1180903
Schink, B. (1997). Energetics of syntrophic cooperation in methanogenic degradation. Microbiol. Mol. Biol. Rev. 61, 262–280.
Schink, B., Montag, D., Keller, A., and Müller, N. (2017). Hydrogen or formate: alternative key players in methanogenic degradation. Environ. Microbiol. Rep. 9, 189–202. doi: 10.1111/1758-2229.12524
Schink, B., and Stams, A. J. M. (2013). “Syntrophism among prokaryotes” in The prokaryotes: prokaryotic communities and ecophysiology. eds. E. Rosenberg, E. F. DeLong, S. Lory, E. Stackebrandt, and F. Thompson (Berlin: Springer), 471–493.
Schipper, L. A., Hobbs, J. K., Rutledge, S., and Arcus, V. L. (2014). Thermodynamic theory explains the temperature optima of soil microbial processes and high Q(10) values at low temperatures. Glob. Chang. Biol. 20, 3578–3586. doi: 10.1111/gcb.12596
Schmidt, O., Hink, L., Horn, M. A., and Drake, H. L. (2016). Peat: home to novel syntrophic species that feed acetate- and hydrogen-scavenging methanogens. ISME J. 10, 1954–1966. doi: 10.1038/ismej.2015.256
Schmidt, O., Horn, M. A., Kolb, S., and Drake, H. L. (2015). Temperature impacts differentially on the methanogenic food web of cellulose-supplemented peatland soil. Environ. Microbiol. 17, 720–734. doi: 10.1111/1462-2920.12507
Schnürer, A., Schink, B., and Svensson, B. H. (1996). Clostridium ultunense sp.nov., a mesophilic bacterium oxidizing acetate in syntrophic association with a hydrogenotrophic methanogenic bacterium. Int. J. Syst. Bacteriol. 46, 1145–1152. doi: 10.1099/00207713-46-4-1145
Scholten, J. C. M., and Conrad, R. (2000). Energetics of syntrophic propionate oxidation in defined batch and chemostat cocultures. Appl. Environ. Microbiol. 66, 2934–2942. doi: 10.1128/AEM.66.7.2934-2942.2000
Schulz, S., and Conrad, R. (1995). Effect of algal deposition on acetate and methane concentrations in the profundal sediment of a deep lake (Lake Constance). FEMS Microbiol. Ecol. 16, 251–260. doi: 10.1111/j.1574-6941.1995.tb00289.x
Schulz, S., and Conrad, R. (1996). Influence of temperature on pathways to methane production in the permanently cold profundal sediment of Lake Constance. FEMS Microbiol. Ecol. 20, 1–14. doi: 10.1111/j.1574-6941.1996.tb00299.x
Schulz, S., Matsuyama, H., and Conrad, R. (1997). Temperature dependence of methane production from different precursors in a profundal sediment (Lake Constance). FEMS Microbiol. Ecol. 22, 207–213. doi: 10.1111/j.1574-6941.1997.tb00372.x
Schütz, H., Seiler, W., and Conrad, R. (1989). Processes involved in formation and emission of methane in rice paddies. Biogeochemistry 7, 33–53.
Schütz, H., Seiler, W., and Conrad, R. (1990). Influence of soil temperature on methane emission from rice paddy fields. Biogeochemistry 11, 77–95. doi: 10.1007/BF00002060
Schwarz, J. I. K., Eckert, W., and Conrad, R. (2008). Response of the methanogenic microbial community of a profundal Lake sediment (Lake Kinneret, Israel) to algal deposition. Limnol. Oceanogr. 53, 113–121. doi: 10.4319/lo.2008.53.1.0113
Scopes, R. K. (1995). The effect of temperature on enzymes used in diagnostics. Clin. Chim. Acta 237, 17–23. doi: 10.1016/0009-8981(95)06060-Q
Seitz, H. J., Schink, B., Pfennig, N., and Conrad, R. (1990). Energetics of syntrophic ethanol oxidation in defined chemostat cocultures. 1. Energy requirement for H2 production and H2 oxidation. Arch. Microbiol. 155, 82–88. doi: 10.1007/BF00291279
Shrestha, P. M., and Rotaru, A. E. (2014). Plugging in or going wireless: strategies for interspecies electron transfer. Front. Microbiol. 5:237. doi: 10.3389/fmicb.2014.00237
Siliakus, M. F., Vander Oost, J., and Kengen, S. W. M. (2017). Adaptations of archaeal and bacterial membranes to variations in temperature, pH and pressure. Extremophiles 21, 651–670. doi: 10.1007/s00792-017-0939-x
Simankova, M. V., Kotsyurbenko, O. R., Lueders, T., Nozhevnikova, A. N., Wagner, B., Conrad, R., et al. (2003). Isolation and characterization of new strains of methanogens from cold terrestrial habitats. Syst. Appl. Microbiol. 26, 312–318. doi: 10.1078/072320203322346173
Simankova, M. V., Parshina, S. N., Tourova, T. P., Kolganova, T. V., Zehnder, A. J. B., and Nozhevnikova, A. N. (2001). Methanosarcina lacustris sp. nov., a new psychrotolerant methanogenic archaeon from anoxic lake sediments. Syst. Appl. Microbiol. 24, 362–367. doi: 10.1078/0723-2020-00058
Stetter, K. O. (2002) in Hyperthermophilic microorganisms. In astrobiology. The quest for the conditions of life. eds. G. Horneck and C. Baumstark-Kahn (Berlin: Springer), 169–184.
Sun, W., Krumins, V., Dong, Y., Gao, P., Ma, C., Hu, M., et al. (2018). A combination of stable isotope probing, Illumina sequencing, and co-occurrence network to investigate thermophilic acetate- and lactate-utilizing bacteria. Microb. Ecol. 75, 113–122. doi: 10.1007/s00248-017-1017-8
Svensson, B. H. (1984). Different temperature optima for methane formation when enrichments from acid peat are supplemented with acetate or hydrogen. Appl. Environ. Microbiol. 48, 389–394. doi: 10.1128/aem.48.2.389-394.1984
Tang, Y. Q., Matsui, T., Morimura, S., Wu, X. L., and Kida, K. (2008). Effect of temperature on microbial community of a glucose-degrading methanogenic consortium under hyperthermophilic chemostat cultivation. J. Biosci. Bioeng. 106, 180–187. doi: 10.1263/jbb.106.180
Thauer, R. K., Kaster, A. K., Seedorf, H., Buckel, W., and Hedderich, R. (2008). Methanogenic archaea: ecologically relevant differences in energy conservation. Nat. Rev. Microbiol. 6, 579–591. doi: 10.1038/nrmicro1931
Thauer, R. K., and Morris, J. G. (1984) in Metabolism of chemotrophic anaerobes: Old views and new aspects. ed. N. G. A. Carr (Cambridge: Cambridge University Press), 123–168.
Thullner, M., Fischer, A., Richnow, H. H., and Wick, L. Y. (2013). Influence of mass transfer on stable isotope fractionation. Appl. Microbiol. Biotechnol. 97, 441–452. doi: 10.1007/s00253-012-4537-7
Tokida, T., Adachi, M., Cheng, W., Nakajima, Y., Fumoto, T., Matsushima, M., et al. (2011). Methane and soil CO2 production from current-season photosynthates in a rice paddy exposed to elevated CO2 concentration and soil temperature. Glob. Chang. Biol. 17, 3327–3337. doi: 10.1111/j.1365-2486.2011.02475.x
Tveit, A. T., Urich, T., Frenzel, P., and Svenning, M. M. (2015). Metabolic and trophic interactions modulate methane production by Arctic peat microbiota in response to warming. Proc. Natl. Acad. Sci. U. S. A. 112, E2507–E2516. doi: 10.1073/pnas.1420797112
Valentine, D. L. (2007). Adaptations to energy stress dictate the ecology and evolution of the Archaea. Nat. Rev. Microbiol. 5, 316–323. doi: 10.1038/nrmicro1619
VanBodegom, P. M., and Stams, A. J. M. (1999). Effects of alternative electron acceptors and temperature on methanogenesis in rice paddy soils. Chemosphere 39, 167–182.
vanHulzen, J. B., Segers, R., VanBodegom, P. M., and Leffelaar, P. A. (1999). Temperature effects on soil methane production: an explanation for observed variability. Soil Biol. Biochem. 31, 1919–1929. doi: 10.1016/S0038-0717(99)00109-1
Vanlier, J. B., Grolle, K. C. F., Frijters, C. T. M. J., Stams, A. J. M., and Lettinga, G. (1993). Effects of acetate, propionate, and butyrate on the thermophilic anaerobic degradation of propionate by methanogenic sludge and defined cultures. Appl. Environ. Microbiol. 59, 1003–1011. doi: 10.1128/aem.59.4.1003-1011.1993
Vanlier, J. B., Martin, J. L. S., and Lettinga, G. (1996). Effect of temperature on the anaerobic thermophilic conversion of volatile fatty acids by dispersed and granular sludge. Water Res. 30, 199–207. doi: 10.1016/0043-1354(95)00107-V
Ward, D. M., and Winfrey, M. R. (1985). Interactions between methanogenic and sulfate-reducing bacteria in sediments. Adv. Aquat. Microbiol. 3, 141–179.
Watanabe, A., Takeda, T., and Kimura, M. (1999). Evaluation of origins of CH4 carbon emitted from rice paddies. J. Geophys. Res. 104, 23623–23629. doi: 10.1029/1999JD900467
Westerholm, M., Roos, S., and Schnürer, A. (2010). Syntrophaceticus schinkii gen. Nov., sp. nov., an anaerobic, syntrophic acetate-oxidizing bacterium isolated from a mesophilic anaerobic filter. FEMS Microbiol. Lett. 309, 100–104. doi: 10.1111/j.1574-6968.2010.02023.x
Westermann, P. (1993). Temperature regulation of methanogenesis in wetlands. Chemosphere 26, 321–328. doi: 10.1016/0045-6535(93)90428-8
Westermann, P. (1994). The effect of incubation temperature on steady-state concentrations of hydrogen and volatile fatty acids during anaerobic degradation in slurries from wetland sediments. FEMS Microbiol. Ecol. 13, 295–302. doi: 10.1111/j.1574-6941.1994.tb00076.x
Westermann, P. (1996). Temperature regulation of anaerobic degradation of organic matter. World J. Microbiol. Biotechnol. 12, 497–503. doi: 10.1007/BF00419463
Westermann, P., Ahring, B. K., and Mah, R. A. (1989). Temperature compensation in Methanosarcina barkeri by modulation of hydrogen and acetate affinity. Appl. Environ. Microbiol. 55, 1262–1266. doi: 10.1128/aem.55.5.1262-1266.1989
Weston, N. B., and Joye, S. B. (2005). Temperature-driven decoupling of key phases of organic matter degradation in marine sediments. Proc. Natl. Acad. Sci. U. S. A. 102, 17036–17040. doi: 10.1073/pnas.0508798102
Wiegel, J. (1990). Temperature spans for growth: hypothesis and discussion. FEMS Microbiol. Rev. 75, 155–169. doi: 10.1111/j.1574-6968.1990.tb04092.x
Wu, X. L., Chin, K. J., and Conrad, R. (2002). Effect of temperature stress on structure and function of the methanogenic archaeal community in a rice field soil. FEMS Microbiol. Ecol. 39, 211–218. doi: 10.1111/j.1574-6941.2002.tb00923.x
Wu, X. L., Friedrich, M. W., and Conrad, R. (2006). Diversity and ubiquity of thermophilic methanogenic archaea in temperate anoxic soils. Environ. Microbiol. 8, 394–404. doi: 10.1111/j.1462-2920.2005.00904.x
Yao, H., and Conrad, R. (1999). Thermodynamics of methane production in different rice paddy soils from China, the Philippines and Italy. Soil Biol. Biochem. 31, 463–473. doi: 10.1016/S0038-0717(98)00152-7
Yao, H., and Conrad, R. (2000). Effect of temperature on reduction of iron and production of carbon dioxide and methane in anoxic wetland rice soils. Biol. Fertil. Soils 32, 135–141. doi: 10.1007/s003740000227
Yao, H., and Conrad, R. (2001). Thermodynamics of propionate degradation in anoxic paddy soil from different rice-growing regions. Soil Biol. Biochem. 33, 359–364. doi: 10.1016/S0038-0717(00)00148-6
Yayanos, A. A. (1986). Evolutional and ecological implication of the properties of deep-sea barophilic bacteria. Proc. Natl. Acad. Sci. U. S. A. 83, 9542–9546. doi: 10.1073/pnas.83.24.9542
Yuan, Q., Pump, J., and Conrad, R. (2012). Partitioning of CH4 and CO2 production originating from rice straw, soil and root organic carbon in rice microcosms. PLoS One 7:e49073. doi: 10.1371/journal.pone.0049073
Yvon-Durocher, G., Allen, A. P., Bastviken, D., Conrad, R., Gudasz, C., St-Pierre, A., et al. (2014). Methane fluxes show consistent temperature dependence across microbial to ecosystem scales. Nature 507, 488–491. doi: 10.1038/nature13164
Yvon-Durocher, G., Caffrey, J. M., Cescatti, A., Dossena, M., DelGiorgio, P., Gasol, J. M., et al. (2012). Reconciling the temperature dependence of respiration across timescales and ecosystem types. Nature 487, 472–476. doi: 10.1038/nature11205
Zehnder, A. J. B. (1978). “Ecology of methane formation” in Water pollution microbiology. ed. R. Mitchell (New York: Wiley), 349–376.
Zehnder, A. J. B., and Stumm, W. (1988). “Geochemistry and biogeochemistry of anaerobic habitats” in Biology of anaerobic microorganisms. ed. A. J. B. Zehnder (New York: Wiley), 1–38.
Zeikus, J. G., and Winfrey, M. R. (1976). Temperature limitation of methanogenesis in aquatic sediments. Appl. Environ. Microbiol. 31, 99–107. doi: 10.1128/aem.31.1.99-107.1976
Zhang, G., Liu, G., Zhang, Y., Ma, J., Xu, H., and Yagi, K. (2013). Methanogenic pathway and fraction of CH4 oxidized in paddy fields: seasonal variation and effect of water management in winter fallow season. PLoS One 8:e73982. doi: 10.1371/journal.pone.0073982
Zhou, L., Liu, X., and Dong, X. (2014). Methanospirillum psychrodurum sp. nov., isolated from wetland soil. Int. J. Syst. Evol. Microbiol. 64, 638–641. doi: 10.1099/ijs.0.057299-0
Zinder, S. H. (1993). “Physiological ecology of methanogens” in Methanogenesis. Ecology, Physiology, Biochemistry and Genetics. ed. J. G. Ferry (New York: Chapman & Hall), 128–206.
Zinder, S. H., Anguish, T., and Lobo, A. L. (1987). Isolation and characterization of a thermophilic acetotrophic strain of Methanothrix. Arch. Microbiol. 146, 315–322. doi: 10.1007/BF00410928
Zinder, S. H., Cardwell, S. C., Anguish, T., Lee, M., and Koch, M. (1984). Methanogenesis in a thermophilic (58°C) anaerobic digestor: Methanothrix sp. as an important aceticlastic methanogen. Appl. Environ. Microbiol. 47, 796–807. doi: 10.1128/aem.47.4.796-807.1984
Keywords: methanogenesis, activation energy, temperature optima, thermodynamic, microbial community
Citation: Conrad R (2023) Complexity of temperature dependence in methanogenic microbial environments. Front. Microbiol. 14:1232946. doi: 10.3389/fmicb.2023.1232946
Edited by:
Zhe Lyu, North Carolina State University, United StatesReviewed by:
Xiuzhu Dong, Chinese Academy of Sciences (CAS), ChinaBernhard Schink, University of Konstanz, Germany
Copyright © 2023 Conrad. This is an open-access article distributed under the terms of the Creative Commons Attribution License (CC BY). The use, distribution or reproduction in other forums is permitted, provided the original author(s) and the copyright owner(s) are credited and that the original publication in this journal is cited, in accordance with accepted academic practice. No use, distribution or reproduction is permitted which does not comply with these terms.
*Correspondence: Ralf Conrad, Y29ucmFkQG1waS1tYXJidXJnLm1wZy5kZQ==