- 1CEMB, University of the Punjab, Lahore, Pakistan
- 2Department of Biological Sciences, National University of Medical Sciences (NUMS), Rawalpindi, Pakistan
- 3Wuhan Institute of Biological Products Co., Ltd., Wuhan, Hubei, China
Hantaviruses are a significant and emerging global public health threat, impacting more than 200,000 individuals worldwide each year. The single-stranded RNA viruses belong to the Hantaviridae family and are responsible for causing two acute febrile diseases in humans: Hantavirus pulmonary syndrome (HPS) and hemorrhagic fever with renal syndrome (HFRS). Currently, there are no licensed treatments or vaccines available globally for HTNV infection. Various candidate drugs have shown efficacy in increasing survival rates during the early stages of HTNV infection. Some of these drugs include lactoferrin, ribavirin, ETAR, favipiravir and vandetanib. Immunotherapy utilizing neutralizing antibodies (NAbs) generated from Hantavirus convalescent patients show efficacy against HTNV. Monoclonal antibodies such as MIB22 and JL16 have demonstrated effectiveness in protecting against HTNV infection. The development of vaccines and antivirals, used independently and/or in combination, is critical for elucidating hantaviral infections and the impact on public health. RNA interference (RNAi) arised as an emerging antiviral therapy, is a highly specific degrades RNA, with post-transcriptional mechanism using eukaryotic cells platform. That has demonstrated efficacy against a wide range of viruses, both in vitro and in vivo. Recent antiviral methods involve using small interfering RNA (siRNA) and other, immune-based therapies to target specific gene segments (S, M, or L) of the Hantavirus. This therapeutic approach enhances viral RNA clearance through the RNA interference process in Vero E6 cells or human lung microvascular endothelial cells. However, the use of siRNAs faces challenges due to their low biological stability and limited in vivo targeting ability. Despite their successful inhibition of Hantavirus replication in host cells, their antiviral efficacy may be hindered. In the current review, we focus on advances in therapeutic strategies, as antiviral medications, immune-based therapies and vaccine candidates aimed at enhancing the body’s ability to control the progression of Hantavirus infections, with the potential to reduce the risk of severe disease.
1. Introduction
Hantaviruses are negative-sense, single-stranded tri-segmented RNA viruses that belong to the order Bunyavirales, family Hantaviridae and genus Orthohantavirus (Kuhn and Schmaljohn, 2023). They exclusively maintain themselves in the population of their natural host and produce a persistent viral infection in them and make a continuous shedding in rodent excreta. They cause two acute febrile diseases in humans: Hantavirus pulmonary syndrome (HPS) and hemorrhagic fever with renal syndrome (HFRS) (Khaiboullina et al., 2005). Hantaviruses pose an emerging global threat to public health causing a devastating effect on human lives, affecting more than 200,000 individuals worldwide annually (Bi et al., 2008). Moreover, the number of cases is significantly increasing day by day in different parts of the world (Watson et al., 2014). Two major outbreaks of Hantavirus disease, reported in the last century, were the first to catch global attention. The first, HFRS outbreak, occurred during the Korean War (1950–1953), affected more than 3,000 US troops (Tian and Stenseth, 2019). The second, HPS outbreak, was documented in the southwestern regions of the US in 1993 (Mills et al., 1999). Pathophysiological studies have revealed that Hantavirus transmits by rodents, mainly through contaminated saliva, feces, urine, and aerosols. It can also be transmitted by bites of affected animals, though it is rarely reported (Brocato and Hooper, 2019). Although the Hantaviruses transmit from natural hosts to humans through a natural ecological process, the outbreak is accelerated by rodent animals like striped field mice and seasonal climatic fluctuations (Tian and Stenseth, 2019).
The clinical presentations of the disease depend on the geographic distribution of the viral strains around the world. In Asia, Hantavirus (HNTV) and Seoul virus (SEOV) primarily infect the human kidney and cause hemorrhagic fever with renal syndrome (HFRS). In North America, Andes virus (ANDV) and Sin Nombre virus (SNV) target the lungs and cause Hantavirus cardiopulmonary syndrome (HCPS) or Hantavirus pulmonary syndrome (HPS), with a high rate of mortality. While in Europe, Puumala virus (PUUV) and Dobrava-Belgarde virus (DOBV) cause a milder form of HFRS, the nephropathia epidemica (Echterdiek et al., 2019). These viruses, like any other enveloped virus, get inactivated when exposed to detergents, UV radiations, hypochlorite solutions, organic solvents, and high temperature (60°C for 30 min). They attach to the host cell surface receptors by glycoprotein and infect a number of cells including dendritic, lymphocyte, and epithelial cells. It has been revealed that integrins, the transmembrane proteins of the host cell, play a pivotal role in viral attachment and entry into the cell. Two types of integrin, β1 and β3-integrin interact with Gn of apathogenic Hantaviruses and glycoprotein of pathogenic Hantaviruses, respectively (Gavrilovskaya et al., 1998; Mackow and Gavrilovskaya, 2009).
2. Hantavirus genome organization
The genome of Hantaviruses contains three segments of single stranded, negative ribonucleic acid (RNA) molecules with a terminal sequence at their 3ˋend (Avšič-Županc et al., 2019) as shown in Figure 1. These genome segments are named according to their nucleotide sequence length as small (S), medium (M), and large (L) as shown in Figure 1 (Singh et al., 2022). Hantaviruses are enveloped viruses with a spherical structure of about 80 to 120 nm length in diameter. The envelope membrane is composed of bilayer of exterior lipids secreted from Golgi complex (Hepojoki et al., 2012). The lipid bilayer is lined with spikes (viral proteins) that protrude from the layer around 10 nm. These spikes appear as heterodimers of Gn and Gc glycoproteins and show a significant binding affinity with oligomers (Huiskonen et al., 2010). The complex and unusual symmetry of the spikes is considered rarely common in enveloped viruses (Huiskonen et al., 2010). The S segment comprises 1,700 to 2,100 bp nucleotides encoding N protein that synthesizes the nucleocapsid of the virion. The M segment containing 3,613–3,707 bp nucleotides encodes the envelop protein of 1,150 amino acids for glycoprotein. It carries a conserved pentapeptide motif “WAASA” at its N-terminus that acts as a target site for co-translational cleavage by the cellular peptidase complex (Löber et al., 2001). The L segment is 6,500 nucleotides wide and encodes about 2,160 amino acids for the biosynthesis of RNA-dependent RNA polymerase and other viral proteins like reverse transcriptase that converts the negative sense RNA into positive sense mRNA for protein synthesis and multiple genome copies. Variation and genetic alterations in M and S segments can disturb the virulence and antigenicity of virus (Du et al., 2014).
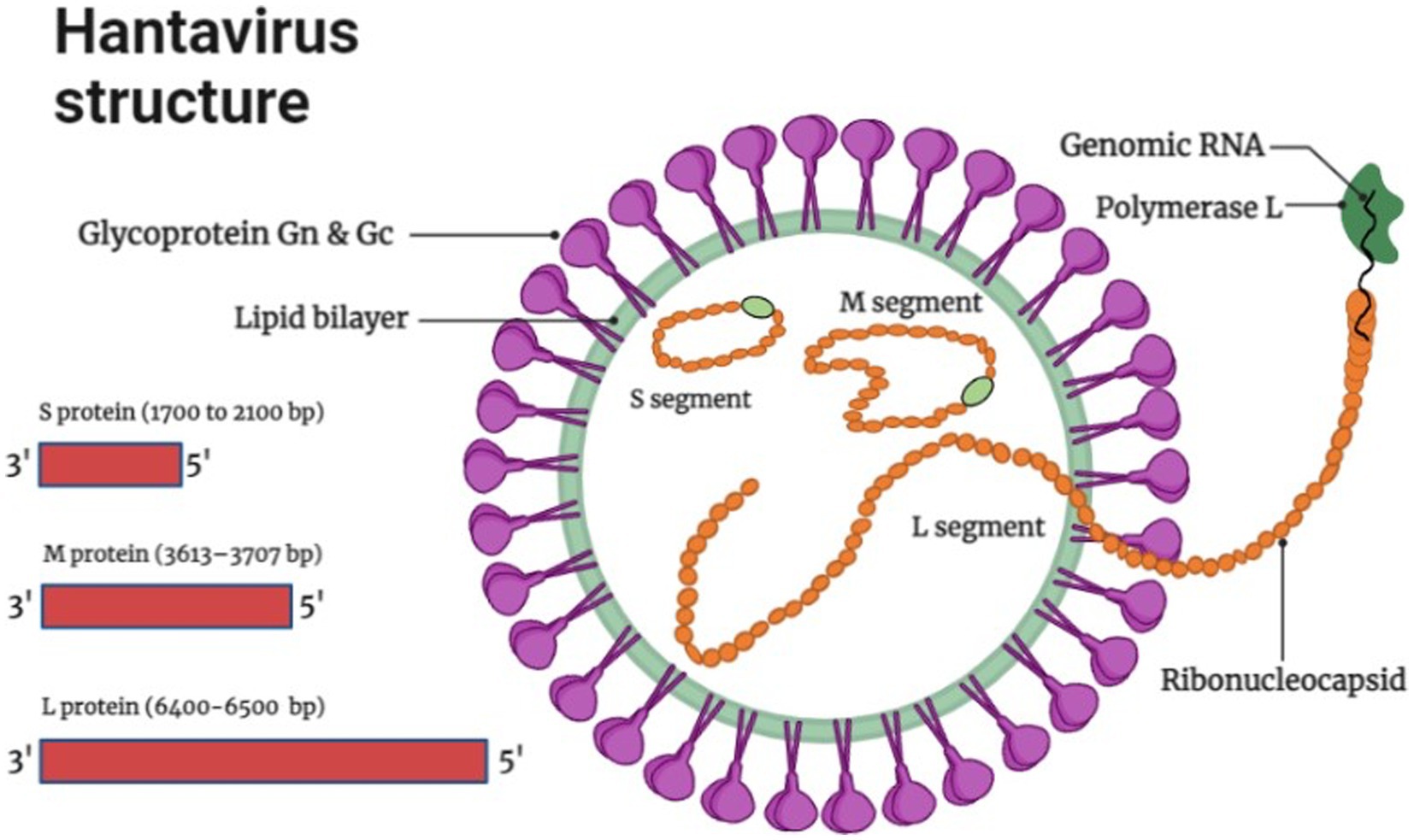
Figure 1. Hantavirus structure and genome organization (Image generated: www.biorender.com).
3. Prevalence
In humans Hantaviruses can cause serious fatal diseases such as hemorrhagic fever renal syndrome (HFRS) in Asia, HFRS and nephropathia epidemica (NE) in Europe and Hantavirus cardiopulmonary syndrome (HCPS) in North and South Americas. In recent years, almost 200,000 people around the world are affected by Hantaviruses annually, with a case fatality of 1–15% for HFRS, 0.1–1% for NE and up to 40–60% for HCPS. Bi et al. (2008) and Singh et al., (2022) two significant outbreaks were documented in the last century which brought Hantavirus disease to worldwide attention. More than 3,000 United Nations forces contracted the HFRS during the Korean War (1950–1953), which was the first time it happened and the second was the outbreak of HCPS in 1993 in the Four Corners area of the United States (Tian and Stenseth, 2019).
3.1. Asia
90% of all the documented cases worldwide are from China, which has the greatest frequency of HFRS, mainly caused by HTNV and SEOV. One of the key HFRS hotspots is the populous central Chinese province of Shaanxi (Yu et al., 2015). According to Chinese Center for Disease Control and Prevention (China CDC) a total of 77,558 cases and 866 fatalities were reported from 2006–2012 possessing a case fatality rate of 1.13%, death rate of 0.01 per 100,000 and a yearly incidence rate of 0.83 per 100,000 cases. HFRS cases have been documented in 30 out of 32 provinces in China as of yet (Zhang et al., 2014). HFRS cases have been mainly documented in autumn-winter and spring seasons (Ke et al., 2016). The incidence of HFRS in China remained low nationwide as compared to the previous five years. As of 18 December 2021, 29 provincial-level administrative divisions (PLDAs) reported 8,502 cases with 54 (0.63%) deaths which was 9.10 and 17.39% more than the 7,793 cases and 46 deaths in 2020 (Aitichou et al., 2005; Wei et al., 2021). Epidemiology of HTNV across asia is shown in Figure 2. The Khabarovsk region reported the first HFRS case in 1934 in Asian Russia. From 1978–1995 a total of 3,145 cases of HFRS in Asian Russia occurred, with a 1.7% morbidity rate (Onishchenko and Ezhlova, 2013). HTNV was first isolated in Korea and an average of 1% mortality rate is recorded for the 300–500 cases reported annually (Lee et al., 2013). A few HFRS have been reported in Vietnam (Huong et al., 2010), Thailand (Suputthamongkol et al., 2005), Singapore (Wong et al., 1985), Sri Lanka (Vitarana et al., 1988), India (Chandy et al., 2009) and Japan (Lokugamage et al., 2004) but more data needs to be recorded from these and other regions in Asia (Heyman et al., 2011).
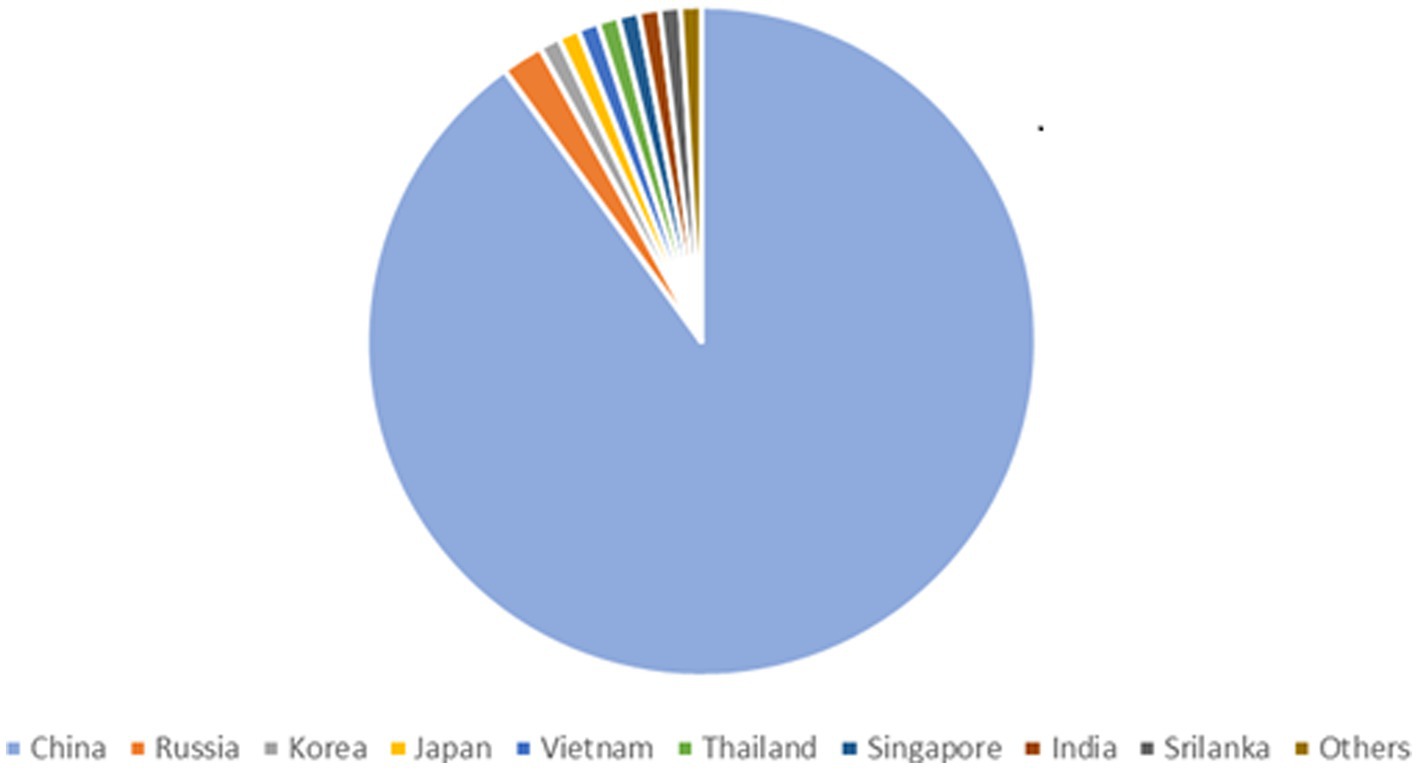
Figure 2. Epidemiology of Hantavirus cases across Asia, showing 90% infections in China, 2% in Russia and 1% in Korea, Japan, Vietnam, Thailand, Singapore, India, Srilanka, Others.
3.2. Europe
Greater than 10,000 HFRS and thousands of NE (nephropathia epidemica) cases are identified on an annual basis all over Europe and are primarily caused by PUUV, DOBV, and SAAV (Vaheri et al., 2013) and (Jiang H. et al., 2017). HFRS cases have been recorded in Finland, Germany, Belgium, France, UK, Poland, the Balkans but in many other areas very few cases are recorded even though the sero-positive prevalence is high in them, which is why more data needs to be documented in Europe because Hantavirus associate infections are high in these areas but remain undocumented (Heyman et al., 2011). Greater than 2,800 cases of Hantavirus caused HFRS were reported in Germany in 2012 (Krüger et al., 2013). In UK SEOV virus associated with acute kidney injury (AKI) in rats was first isolated in Scotland in 1977 and only caused AKI in 1 of 15 rats (Duggan, 2019). Figure 3 No. of HTNV cases across Europe from 2016–2020.
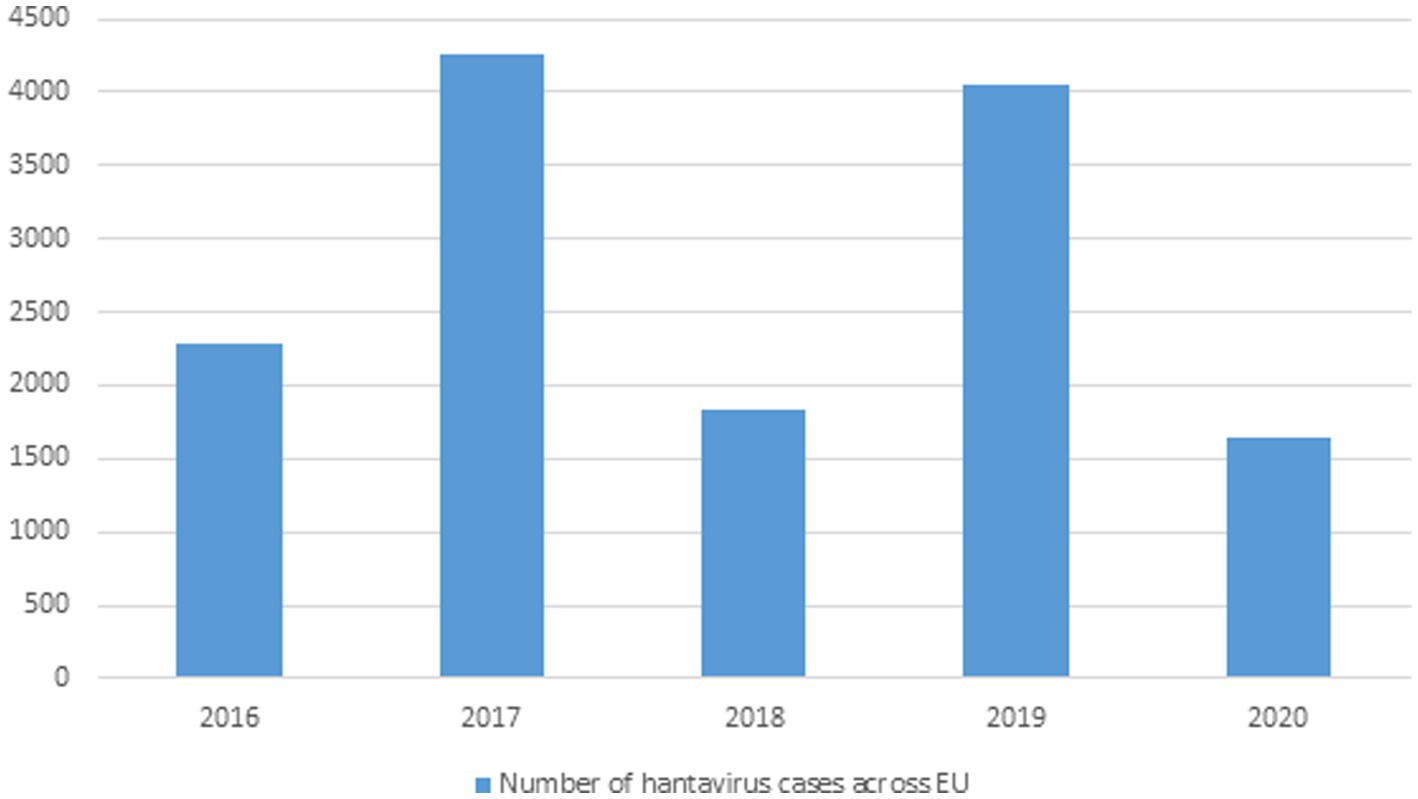
Figure 3. Data showing Hantavirus cases in the EU from 2016–2020 (This data was published by the European Center for Disease Control and Prevention).
3.3. Americas
In 1993, HCPS was initially identified as a hantaviral illness during the Four Corners outbreak in the USA. In North and South Americas almost 200 cases are documented annually. HCPS in Americas is primarily caused by ANDV and SNV. HCPS cases have also been reported in Bolivia, Paraguay, Uruguay, Brazil, Argentina, Panama, Chile, and Canada (Jonsson et al., 2010). In Canada 143 confirmed HCPS cases have been recorded as of 2020 (Warner et al., 2020). Each year between 100 and 200 cases of HCPS are recorded in Argentina, mostly in spring and summer (Jiang H. et al., 2017). Chile has the annual mortality rate of 32–35% for HCPS (Martinez-Valdebenito et al., 2014). Brazil recorded 2032 HCPS cases as of 2017. Table 1 shows the Hantavirus reported cases across North and South America (Warner et al., 2020; Armién et al., 2023).
3.4. Africa
No indigenous Hantavirus was recognized in Africa 15 years ago (Kruger et al., 2015). Since then, only a few studies have examined SEOV and other hantaviruses in Africa and their effects on human health. Due to the lack of regionally specific SEOV testing in human blood samples and the paucity of investigations, it appears that SEOV is not a significant public health hazard on the continent (Heyman et al., 2004). However, there are substantial indications that humans and wild rats in 17 different African nations may be infected with organisms similar to SEOV (Clement et al., 2019). In Africa, the SEOV is not seen as a serious hazard to the general population.
3.5. Pathogenesis
Hantaviruses infections cause HFRS, NE, and HCPS in humans, the symptoms associated with them and their mode of transmission is discussed below.
3.6. Symptoms associated with HFRS and NE
Kidneys are primarily affected in both HFRS and NE. NE is a relatively mild version of HFRS. Symptoms include thrombocytopenia, fever, differing degrees of acute renal failure, myalgia some cases have also reported symptoms related to ocular and central nervous systems. Both of them have five clinical phases febrile, hypotensive, oliguric, polyuric and convalescent. Complications can include multiorgan failure, bleedings, severe encephalomyelitis, pituitary hemorrhage, pulmonary edema, shock and fatal outcome in case of NC and all the aforementioned complications in addition to glomerulonephritis, respiratory distress syndrome and disseminated intravascular congestion can occur in HFRS as shown in Table 2 (Jiang et al., 2016; Jiang H. et al., 2017; Hautala et al., 2021).
3.7. Symptoms associated with HCPS
Lungs are mainly affected in HCPS. Three phases are associated with HCPS they are prodromal, cardiopulmonary, convalescent phases. Prodromal phase lasts 1–5 days and symptoms include malaise, fever, gastrointestinal distress and headaches. Cardiopulmonary phase symptoms include cardiopulmonary malfunction, pulmonary edema, cough, dyspnea and hypoxia. Convalescent phase is the recovery phase where all previous symptoms subside except for dyspnea, which can persist up to 1–2 years (Llah et al., 2018).
3.8. Spread of hantaviruses
Hantaviruses basically infect rodents and are also found in small insectivorous mammals and bats. They cause asymptomatic infections in rodents, and are transmitted to humans through rodent bite, inhalation of aerosolized virus particles and via inhalation of dried feces, urine and saliva of rodents. Hantavirus infection in humans mainly affects the endothelial cells in lungs and kidneys and leads to HCPS, NE, and HFRS (Ermonval et al., 2016). Environmental factors such as availability of food, climate change, geographical location also contributes to Hantavirus infection (Guterres and de Lemos, 2018).
3.9. Proposed mechanism of Hantavirus pathogenesis
The infection begins with contact of Gn and Gc surface proteins with β-integrin receptors, yet how hantaviruses spread in the human body is still not fully known. Given that they express 3-integrin receptors and are found close to epithelial cells, immature dendritic cells likely play a crucial role (Gavrilovskaya et al., 1999, 2002). Platelet dysfunction, immunological responses, and the disruption of endothelial cell barrier capabilities likely have a role in the pathological process of Hantavirus (Mackow and Gavrilovskaya, 2009). DCs in humans are extremely mobile, they link innate and adaptive immunity and reside in pathogen-host interface in the respiratory mucosa and lung alveoli. They have the ability to “snorkel” through the epithelial-tight junctions by introducing their dendritic projections into the airway lumen (Jahnsen et al., 2006) and contract Hantavirus in the lungs (Raftery et al., 2002; Marsac et al., 2011). Additionally, monocytes exposed to HTNV transform into cells that resemble DCs (Markotić et al., 2007; Schönrich et al., 2008) that might serve as Trojan horse, assisting viruses to spread across the human body and eventually infect endothelial cells in numerous organs. It has been seen in various studies that EC become stimulated during PUUV infection, increasing the production of chemokines and adhesion molecules such as E-Selectin, intercellular adhesion molecule 1 (ICAM-1) and VCAM-1 (Temonen et al., 1996). Stimulated chemokines include a neutrophil activator and recruiter called IL-8 (interleukin 8) (Klingström et al., 2008; Sadeghi et al., 2011; Libraty et al., 2012; Kyriakidis and Papa, 2013). Production of human leucocyte antigen mainly HLA-E is elevated on EC, which in turn activates nature killer (NK) cells (Kraus et al., 2004; Björkström et al., 2011). Hantavirus-infected EC may be removed by activated immune cell’s cytotoxic action, which could lead to vascular leakage (Hayasaka et al., 2007). HTNV infected EC are somewhat defended from cytotoxic T cells and NK cells (Gupta et al., 2013) uninfected EC’s are however, vulnerable to cytotoxic assault and bystander killing. According to recent studies, neutrophils can generally contribute to the immunopathogenesis (Gupta et al., 2010; Saffarzadeh et al., 2012) by interacting with active EC they go through 2 mechanisms of programmed cell death. Virus-induced 2 integrin signaling causes neutrophils to become activated, which then leads to either NETosis or the production of inflammatory cytokines like TNF-α or VEGF, depending on the 2 integrin ligands involved and possibly additional micro-environmental stimuli, resulting in increase in the vascular leakage, though through different processes (Schönrich et al., 2015). Pentraxin-related protein 3 (PTX3), a humoral pattern-recognizing receptor, activates complement during acute HFRS (Outinen et al., 2012). PTX3 is kept in neutrophil granules and released via integrins in response to signals (Jaillon et al., 2007; Razvina et al., 2015). In addition to cytoskeletal rearrangements in EC, the soluble complement components C3a and C5a produced after complement activation by antibodies and PTX3 also causes IL-8 production (Monsinjon et al., 2003). As a result, PTX3 draws additional neutrophils to the endothelium barrier, escalating the inflammation in the vessels. TNF alpha a pro-inflammatory cytokine is released by Hantavirus-infected macrophages, DC as well as NK cells, neutrophils and CD8+ T cells that have been activated (Raftery et al., 2002; Marsac et al., 2011; Shin et al., 2012). TNF-α (Tumor necrosis factor alpha) eliminates the virus from infected cells by non-cytolytic processes, it may, on the one hand, aid in the control of hantaviral propagation (Khaiboullina et al., 2000; Guidotti and Chisari, 2001). On the other hand, vascular leakage and breathing problems are generated if it is given externally in proportions that are encountered during Hantavirus infection (Tracey and Cerami, 1994; Wimer, 1998). Both direct and indirect methods may cause localized TNF-local release at the EC contact to promote vascular permeability(Schönrich et al., 2015). Figure 4 illustrates the proposed immunological mechanisms that result in endothelial barrier breakdown by the Hantavirus.
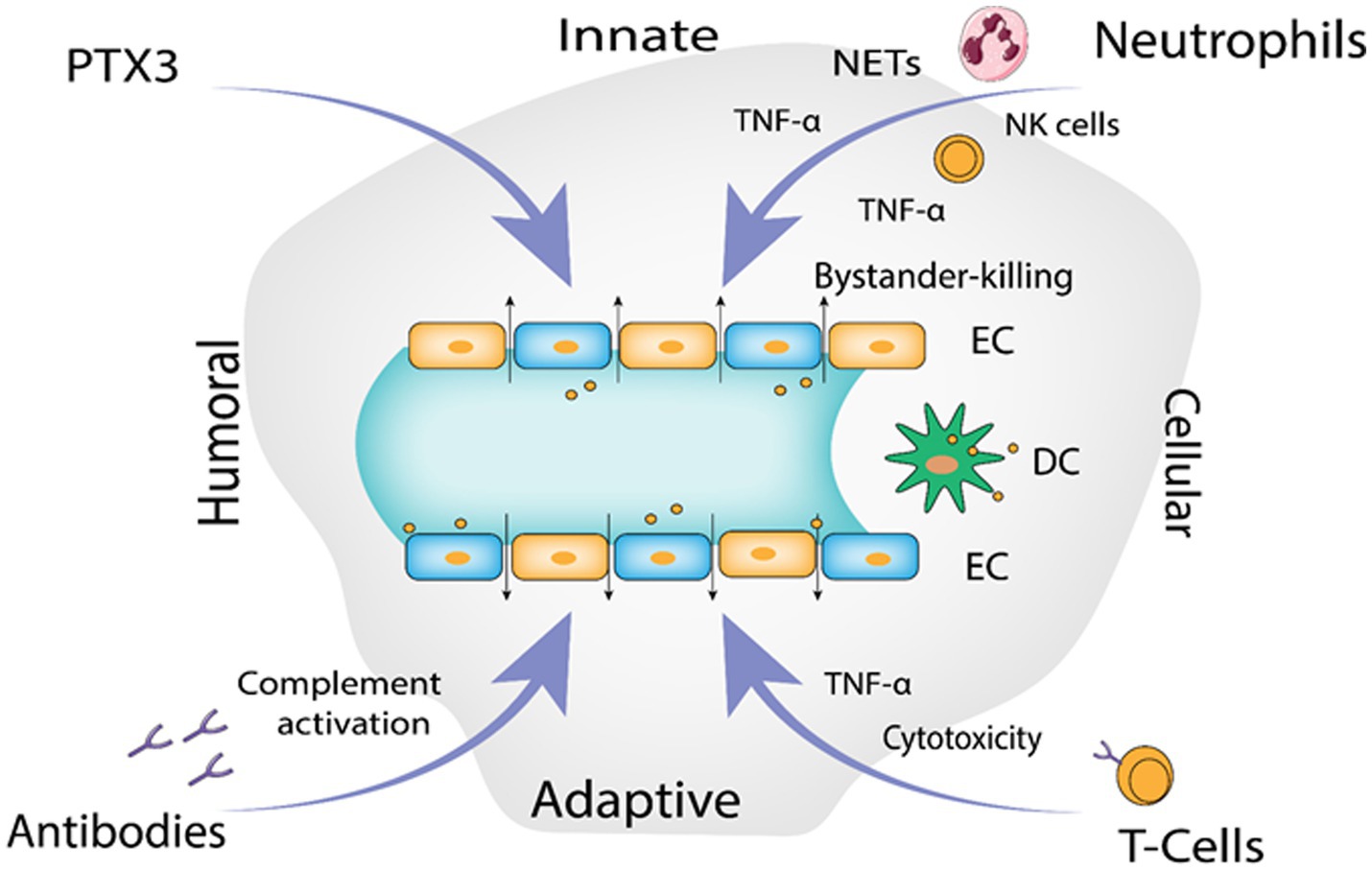
Figure 4. The proposed immunological mechanisms leading to endothelial barrier breakdown by the Hantavirus (Image generated: www.biorender.com).
3.10. Replication cycle of hantaviruses
Macrophages and vascular endothelial cells, particularly those in the lungs and kidneys, are targeted by the Hantavirus (Yanagihara and Silverman, 1990). In order to facilitate binding, Gn protein of the virus engages with integrin receptors that reside on the outer layer of the cell it invades, according to a number of findings (Mir and Panganiban, 2010). A family of heterodimeric proteins called integrins include both alpha and beta chains. Both cell to extracellular matrix and cell to cell interactions are facilitated by it (Takagi and Springer, 2002; Campbell and Humphries, 2011). Virons are eventually carried to lysosomes after binding, which is accomplished via clathrin-coated pits. In the endolysosomal compartment, virions uncoat, releasing three viral nucleocapsids into the cytoplasm (Jin et al., 2002). The virus is engulfed by clathrin-coated vesicle (CCV), which is composed of clathrin-coated cellular membrane (Ramanathan and Jonsson, 2008). Three mRNAs transcribed by RdRp, one from the S, M, and L sections of the viral RNA. Free ribosomes are locations for the translation of the S and L derived mRNAs. Whereas, (RER) rough endoplasmic reticulum is where M-specific mRNAs are converted into proteins. Two glycoproteins, Gn and Gc, are produced as a result of the glycoprotein precursor’s intrinsic cleavage at a highly conserved amino acid sequence (Spiropoulou, 2001). For glycosylation, the Golgi complex receives the glycoproteins Gn and Gc, where hanta virions are thought to develop as illustrated in Figure 5. Followed by exocytosis, migration to the golgi cisternae, then to the outer membrane of secretory vesicles and finally egression through exocytosis. The details of virion egress, however, are mostly unclear (Szabo, 2017). Other possible mechanisms for virus entry include caveola, macropinocytosis, cholesterol-dependent endocytosis, clathrin-independent endocytosis-mediated receptor and micropinocytosis (Ramanathan et al., 2007).
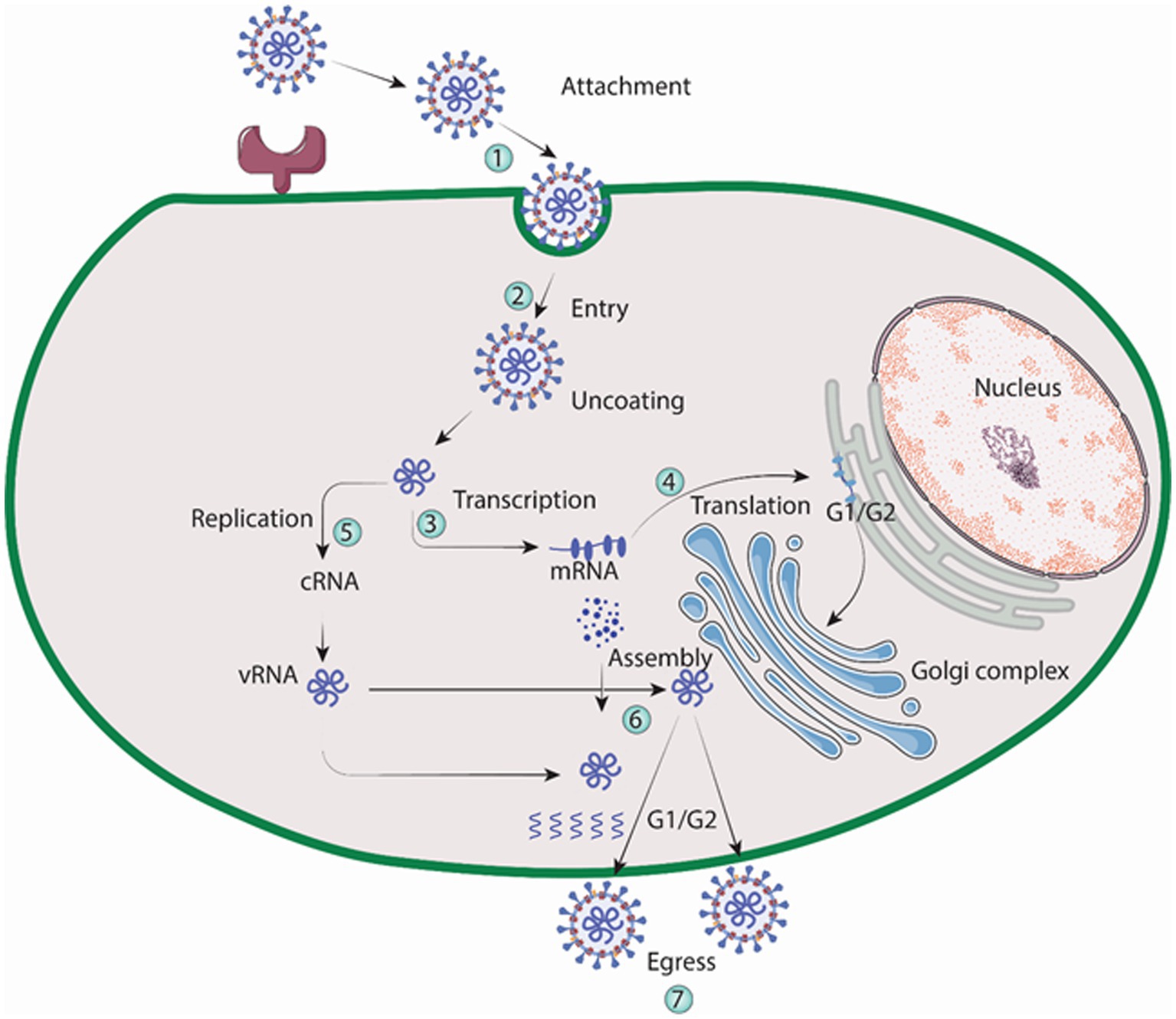
Figure 5. Replication cycle of Hantavirus (Image generated: www.biorender.com).
4. Diagnosis
Initial diagnosis can be performed by observing the symptoms associated with HCPS, HFRS, and NE. Since Hantaviruses are rodent transmitted viruses so clues can be obtained for diagnosis at the time of taking patient’s history by asking if a patient recently traveled to areas infested with rodents or came in contact with rodents or their excretions. Screening tests such as Complete Blood Count (CBC) and peripheral smear can also be used (Dvorscak and Czuchlewski, 2014).
Serological methods available for definite diagnosis of infection caused by Hantaviruses. Serology is used to detect IgG and IgM anti-hantaviral antibodies in the blood of the patient. The IgM antibodies appear extremely early during the course of the infection, whereas IgG antibodies appear later in the process. Enzyme Linked Immunosorbent Assay (ELISA) and the strip immunoblot detects viral antibodies, only disadvantage is the potential for cross reacting with other viral antigens, but are still extremely useful and important for diagnosis purposes. Indirect Immunofluorescence assay is more specific than Elisa but it is laborious. Neutralization tests are the most specific serological tests but are costly, laborious and require BSL-3 conditions. Immunochromatographic tests are readily performed and are cheap but cross reactivity minimizes precision of results obtained (Schubert et al., 2001; Meisel et al., 2006; Navarrete et al., 2007).
Molecular methods include Real Time RT PCR, an important, sensitive and fast technique for detecting viral RNA in blood, blood clots or tissues, its only disadvantage includes that it only yields results during the viremic phase of the Hantavirus caused infection (Aitichou et al., 2005). Microarray technique is fast, sensitive and allows simultaneous detection of thousands of viruses but is costly and the data analysis is complex. Next Generation Sequencing (NGS) is also used for virus detection and complete sequencing but it is expensive and requires complex bioinformatics tools.
Virological methods include isolation in cell cultures which allows extensive functional and virological studies but is laborious and requires specially trained personnel and BSL-3 conditions. Immunohistochemistry by enzyme immunoassay and immunofluorescent test allows diagnosis from infected tissues of organs but laborious preparations are required (Kruger et al., 2015).
5. Treatment and management
Hantavirus infections are managed mostly by managing the symptoms, providing supporting care and admitting patients to the Intensive Care Unit (ICU), providing patients with oxygen therapy, by administrating an antiviral ribavirin, which has showed reduction in death rate of patients still in their initial stages of infection. Treatment for HCPS patients includes respiratory and cardiac monitoring and support which includes mechanical ventilation, hemofiltration and membrane oxygenation. Supportive treatment for HRFS includes electrolyte infusion and hydration to stabilize blood pressure, acute thrombocytopenia is managed with transfusing platelets, uremia is managed with intermittent hemodialysis and continuous renal replacement therapy is given to manage multi-organ pulmonary edema (Sargianou et al., 2012; Liu et al., 2020).
6. Recent therapeutic advances against Hantavirus
6.1. Blocking viral entry
The following candidate drugs have been shown to increase survival rate only in the initial stages of HTNV infection, but are ineffective in later stages.
6.1.1. Griffithsin
A protein called griffithsin (GRFT), which was first discovered from red algae, has demonstrated potential as a multifunctional antiviral agent. Its capacity to prevent the invasion of several viruses, including HIV and SARS-CoV, has been researched (O'Keefe et al., 2010; Lusvarghi and Bewley, 2016). The spikes of the Hantavirus consist of tetramers formed by Gn-Gc heterodimers, which envelop the entire surface of the virus particle. Gn, one of the viral envelope glycoproteins, contains several N-linked glycosylation sites and is positioned on the virus surface, making it a potential primary target for GRFT. A high-mannose oligosaccharide-binding lectin called Griffithsin (GRFT) is now being tested in phase I clinical trials as a topical microbicide for the defense against several viruses. It is a powerful inhibitor of ANDV infection. The fact that GRFT prevented the entry of pseudo-particles containing ANDV envelope glycoprotein into host cells suggests that it prevents the function of viral envelope protein during entry. To combat ANDV and SNV infection, 3mGRFT (trimeric synthetic tandemer of GRFT) is more effective than GRFT (Shrivastava-Ranjan et al., 2020). In a recent study GFRT prevented the entry and HTNV infection of recombinant vesicular stomatitis virus (VSV) containing HTNV glycoproteins into host cells in vitro by attaching to the viral N-glycans. It also shielded the suckling mice from death brought on by cerebral exposure to HTNV, according to in vivo tests. These findings highlighted the importance of GRFT as a potential HTNV infection inhibitor (Zhao et al., 2022).
6.1.2. Coumarin
Coumarin and its derivatives have been found to have antiviral activity against a variety of viruses, including human immunodeficiency virus (HIV), hepatitis virus, herpes simplex virus, CHIKV, and enterovirus 71. Coumarin derivative’s potential use is as antiviral medicines. The diverse chemical makeup of coumarin derivatives however, meant that these substances had an impact on the many stages of a virus life cycle. Tricyclic coumarin GUT-70 inhibited HIV’s ability to attach and fuse to cellular walls and plasma membranes, while dipyranocoumarin (+)-calanolide A could block reverse transcription (Xu et al., 2021). Coumarin dimmer analogs could also block HIV integrase, and amide coumarin derivatives could affect how HIV was put together. On the basis of molecular structure of coumarin, two of the most common coumarin derivatives, dicoumarin and pyrone-coumarin, were synthesized. It was demonstrated that the tri-fluoro substituent on the benzene ring of the dicoumarin derivatives N6 and N7 had a strong anti-HTNV action (Li et al., 2022a). Dicoumarin demonstrated increased anti-HTNV activity, and adding Cl or CF3 might increase the inhibitory activity and selectivity to the HTNV, according to the structure activity relationship (SAR). To clarify the connection between the chemical structure and the biological action against the HTNV, more research is necessary. N6, a derivative of coumarin, showed both in vivo and in vitro action against HTNV, and AKT1 may have played a role in the molecular mechanism by which N6 combats viral infection (Reguera et al., 2010; Li et al., 2021, 2022b). Therefore, finding novel coumarin compounds that could fight viral infection has significant significance for creating potent drugs.
6.1.3. Lactoferrin
Lactoferrin (LF), a glycoprotein that binds to iron and has been shown to have broad antibacterial, antifungal and antiviral properties, inhibited hantaviral attachment, adsorption (Masson et al., 1969; Buys et al., 2011). Both in vitro and in vivo studies have demonstrated that LF guards against Hantavirus infection (Murphy et al., 2001). Lactoferrin was found partially effective in preventing HFRS by restricting focus formation in suckling mice, but when used in-vivo in combinations with ribavirin, it prevented focus development entirely (Murphy et al., 2001).
In a study, Seoul virus (SEOV) was used to infect Vero E6 cells in order to test the antiviral potency of LF against hantaviruses. The objective of the study was to contrast LF’s antiviral activity with that of Rbv.100 μg/mL of RBV administered after infection reduced the number of foci by 97.5%. Vero E6 cells treated with 400 μg/mL of LF showed a significant 85% decrease in the number of foci as compared to the control group. However, in LF-pretreated cells, the number of foci began to increase by 24 h post-infection (hpi). At 24 hpi LF prevented viral shedding, but not after 48 hpi (Murphy et al., 2001). As shown by another supporting study, the findings suggested that LF may attach to cell surfaces and prevent SEOV from adhering to host cells in the early stages of infection (Murphy et al., 2000). It is interesting to note that, LF improved cell survival rates following hantaviral amplification despite not inhibiting the formation of NP or Gc. This is due to the ability of LF to increase the cytocidal activity of NK cells (Murphy et al., 2001). However, the specific method through which LF block SEOV absorption, affect host immune responses and has an effect on other Hantavirus species are still unknown.
6.1.4. Virus fusion inhibitors (domain III and stem peptides)
Several processes are thought to be involved in virus-cell membrane fusion (Kielian and Rey, 2006; Harrison, 2015). During viral fusion, fusion proteins become activated and insert a fusion peptide or loop into the target membrane. As a result, an intermediate phase is generated in which one end of the fusion protein can join to the viral envelope via transmembrane region, linking the viral and cellular membranes together. The fusion protein undergoes conformational changes which can draw both anchors together, achieving a hairpin-like structure where both domains gather at one end. After that, the outer leaflets of membranes fuse to form a hemi-fusion intermediate, followed by complete membrane fusion once the opposed membranes have been brought together with the help of local membrane curvature. A pore is formed as a result of the fusion, allowing the virus to inject its ribonucleocapsids into the cytoplasm of the cell and begin replication.
On the basis of molecular structures, viral fusion proteins are categorized into three classes: I, II, and III. Alpha helices make up the majority of class I fusion proteins, while beta sheets make up the majority of class II proteins. Class III fusion proteins exhibit characteristics from both class I and class II (Kielian, 2014; Modis, 2014; Harrison, 2015). In silico and in vitro investigations reveal that Hantavirus Gc glycoprotein resembles class II fusion proteins (Cifuentes-Munoz et al., 2011). Three domains (I-III) and a stem connecting the ectodomain to the transmembrane region make up class II fusion proteins (Rey et al., 1995; Kielian, 2006). During fusion, DIII advances toward the fusion loop to adopt a hairpin-like structure (Bressanelli et al., 2004; Gibbons et al., 2004). This movement is followed by stem region which folds against the trimeric core created by the fusion protein (Roman-Sosa and Kielian, 2011; DuBois et al., 2013; Klein et al., 2013). It is possible to intervene and stop the fusion process as a result of these significant conformational changes, thereby inhibiting viral infection. It is possible to delay or block viral entry by selectively binding ligands to a fusion protein’s intermediate form before it assumes its post-fusion conformation (Barriga et al., 2016). It has been demonstrated that protein fragments spanning the stem region and domain III (DIII) are capable of inhibiting these fusion proteins. Due to this, to block viral fusion and entrance into the cell, recombinant ANDV DIII and stem peptides were developed. A 60% reduction in Vero E6 infection by ANDV via the endosomal pathway was achieved by combining DIII with the C-terminal of stem region. Over 95% infection was prevented when ANDV fused at the plasma membrane. These findings indicated that a stem fragment method used against Hantavirus may obviously block the fusion of related viruses belonging to the same genus (Barriga et al., 2016).
6.1.5. Hantavirus-binding receptor inhibitors (cyclic nonapeptides)
Hantaviruses have two transmembrane glycoproteins, Gn and Gc, derived from a single glycoprotein precursor through proteolytic cleavage that occurs during post-translational modifications (Jonsson and Schmaljohn, 2001). The entry of hantaviruses into human endothelial cells is facilitated by the interaction of viral surface glycoproteins with αvβ3 integrins present on the host cell surface (Gavrilovskaya et al., 1999; Raymond et al., 2005). Both Gn and Gc may play a role in the entry of viruses, Gn is involved in the viral attachment process while Gc is thought to drive membrane fusion (Mackow and Gavrilovskaya, 2001; Tischler et al., 2005).
Small molecules like peptide ligands have potential therapeutic applications as they can bind to specific proteins and interfere with particular protein–protein interactions (Mayor et al., 2021). For this purpose, particular peptides can be synthesized either by imitating one of the binding partners or by creating new binding interactions. Novel peptide ligands were created with the ability to block SNV infection. SNV, the causative agent of HCPS, is classified as a category A pathogen by the NIAID. Currently, there is no specific treatment for HCPS and its fatality rate remains high reaching approximately 40%.
Therapeutics that target SNV particularly are still lacking. To tackle this issue, researchers are utilizing phage display technique to discover cyclic nonapeptides that can bind to the cellular receptor αvβ3. By identifying these peptides, they aim to prevent Hantavirus entry including SNV into the human endothelial cells particularly during in vitro experiments with Vero E6 cells (Larson et al., 2005; Hall et al., 2007). These peptides offer therapeutic promise while avoiding the potential adverse effects often associated with conventional mAbs employed as therapeutic agents to inhibit such interaction (Baudouin et al., 2003; Gauvreau et al., 2003). Cyclic nonapeptides were created using peptide sequences from phage that displayed the most potent infection inhibition. However, when tested, the isolated peptides showed lower effectiveness in blocking infection (ranging from 9.0 to 27.6% inhibition) compared to the phage-presented peptides, which achieved inhibition levels of 74.0 to 82.6%. As the phage displayed pentavalent peptides, the focus was on exploring whether presenting the identified peptides in a multivalent manner would lead to enhance inhibition. In order to do this, certain cyclic peptides were bound to multivalent nanoparticles using carboxyl linkages and their ability to suppress infection was examined (Hall et al., 2008).
With a 4:1 nanoparticle-to-virus ratio, SNV infection was reported to be inhibited in vitro by two of the synthetic cyclic nonapeptides CLVRNLAWC and CQATTARNC. CLVRNLAWC inhibited the infection by 9–32.5% while CQATTARNC inhibited it by 27.6–37.6%. At a 20:1 ratio, CQATTARNC decreased infection by 50% (Hall et al., 2008). These findings demonstrate the potential therapeutic value of multivalent inhibitors in the disruption of interactions between proteins, particularly those critical for host cell viral infection. Based on molecular makeup and potential capacity to engage the αvβ3 cell receptor, further peptidomimetic compounds were selected. In the first round of screening, 49 peptidomimetic compounds and in the second round, 68 compounds were found having an anti-Hantavirus action in lower 2,000 μM range. Due to this, a special collection of chemicals were acquired for the subsequent phases of drug development. The antiviral potential of these chemical compounds requires improvement and in vivo research to support it (Hall et al., 2010).
6.2. Blocking viral replication
6.2.1. Ribavirin
When used both in vitro and in vivo, the purine nucleoside analog ribavirin exhibits a wide range of antiviral effects against numerous different RNA, DNA viruses and it works by blocking viral replication. However, its pleiotropic effects, make it difficult to understand how it works (Fernandez-Larsson and Patterson, 1990; Graci and Cameron, 2006). These contain both direct mechanisms, like interfering with RNA capping, inhibiting polymerase activity, and inducing lethal mutagenesis, as well as indirect mechanisms, such as inhibiting inosine monophosphate dehydrogenase and exerting immunomodulatory effects (see Figure 6). The first specialized phase in the cellular production of guanine nucleotides, involves the catalytic activity of enzyme IMPDH. This process relies on NAD+ and involves the transformation of inosine monophosphate to xanthosine monophosphate. Because Ribavirin 5′-monophosphate (RMP) bears structural resemblance to GMP, It serves as a highly effective competitive inhibitor of IMP dehydrogenase (Streeter et al., 1973). Human IMP dehydrogenase (IMPDH) type I and type II isoforms are both potently inhibited by RMP, which reduces de novo GTP production. Because of this disturbance, viral RdRp (RNA-dependent RNA polymerase) cannot function properly. The antiviral effects of ribavirin are assumed to be related to its ability to inhibit IMP dehydrogenase (IMPDH) (Graci and Cameron, 2006).
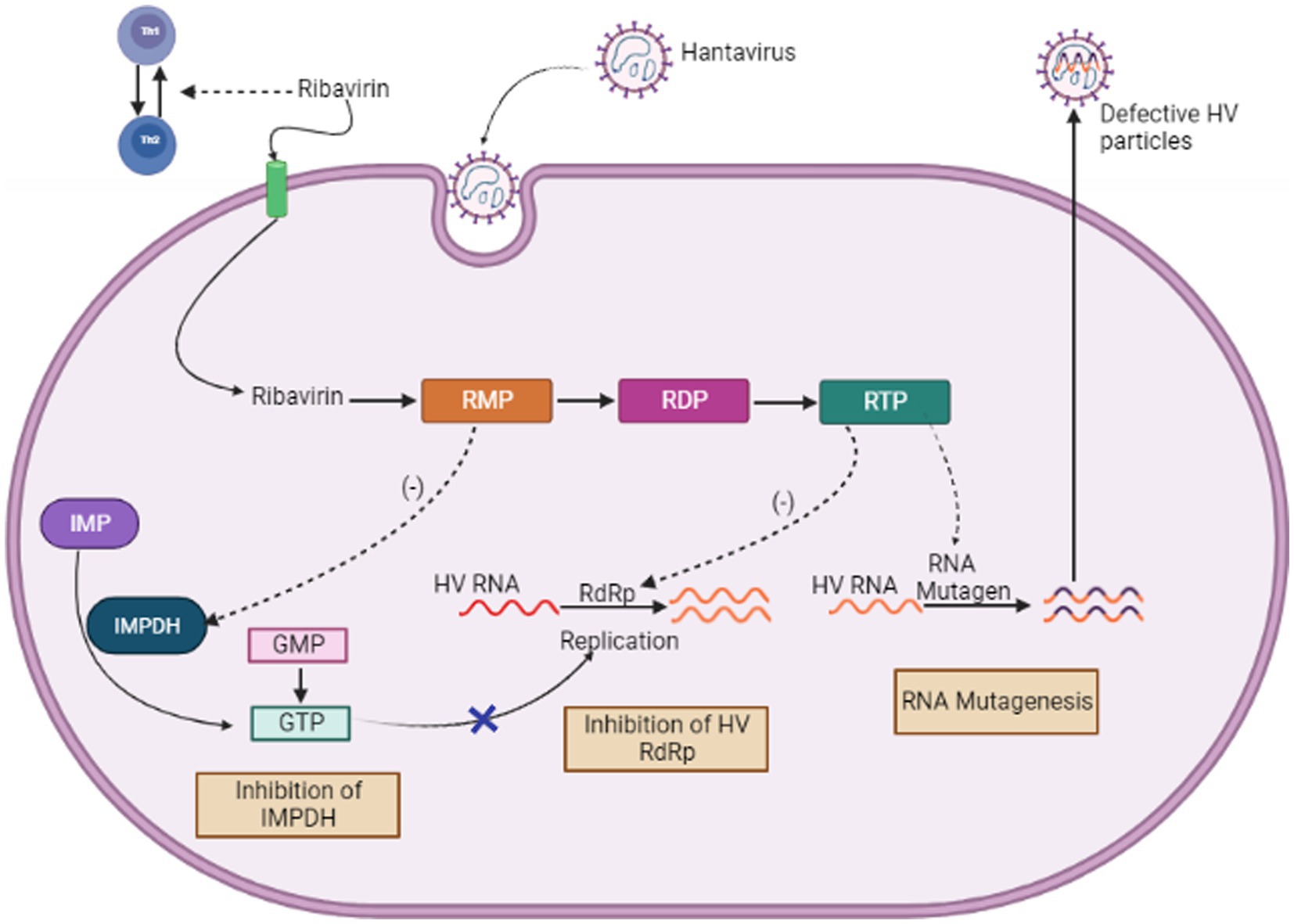
Figure 6. Mechanism of Action of Ribavirin (Image generated: www.biorender.com).
Moreover, RBV was found to control host immunological responses by inhibiting the production of interleukin-10 by regulatory T cells (Kobayashi et al., 2012). The blocking mechanism of ribavirin is believed to target the capping (Goswami et al., 1979) and translation efficiency (Toltzis and Huang, 1986) of viral mRNA, along with the direct reduction of the viral polymerase’s activity (Wray et al., 1985; Fernandez-Larsson and Patterson, 1989). Ribavirin acts by interfering with the accuracy and effectiveness of polymerase replication. As a result, it may induce chain termination more frequently or improper nucleotide insertion, which could result in error catastrophe. Recent investigation showed that ribavirin rises the chances of error made by the Hantavirus polymerase within a cell culture model (Severson et al., 2003). We assumed that this elevated error rate is because of the integration of ribavirin into the viral RNAs via RNA-dependent RNA polymerase. It has been shown that ribavirin causes hantaviruses to replicate in an error-prone manner, which lowers the viral titer (Severson et al., 2003).
Although more investigation is required to completely understand how ribavirin inhibits Hantavirus, the findings of Severson et al. (2003) indicated that ribavirin may function as a mutagen via directly integrating into the S-segment of cRNA or mRNA through the viral RdRp. The amount of error-free mRNA falls when ribavirin is integrated into mRNA, which might lower the amount of viral proteins required to construct infectious viral particles. The amount of mRNA was, however, significantly decreased in these experiments. This finding implies that the ribavirin integration may reduce mRNA stability. This theory appears to be a logical and convincing explanation given the virus life cycle. As a result, the integration of ribavirin could accelerate viral mRNA to become more unstable and break down more quickly within the host cell (Jonsson et al., 2005).
6.2.2. ETAR
ETAR, also known as (1-beta-d-ribofuranosyl-3-ethynyl-[1,2,4] triazole), functions as a nucleoside analog and is similar to ribavirin in that it prevents HV replication by lowering the levels of GTP. Notably, ETAR showed superior efficacy to ribavirin as a therapeutic option in trials on suckling mice infected with HTNV. Due to the absence of pseudo base pairs, ETAR is unlikely to cause mutations (Chung et al., 2008). Furthermore, there is no proof that ETAR has any immunoregulatory effects (Szabo, 2017).
6.2.3. Favipiravir
T-705 is a pyrazine derivative also referred to as favipiravir or 6-fluoro-3-hydroxy-2-pyrazinecarboxamide. It was initially known for its ability to combat influenza, but it is now thought to have antiviral capabilities against a number of other viruses that rely on RdRp for replication (Furuta et al., 2009). Flaviviruses, noroviruses, arenaviruses, and bunyaviruses (Gowen et al., 2007) are some of these viruses. T-705 works as a prodrug that is transformed into T-705-4-ribofuranosyl-5-triphosphate with the help of several intracellular enzymes. When T-705-4-ribofuranosyl-5-triphosphate is created, it functions as a purine nucleotide analog, it is incorporated into the newly synthesized RNA chain, selectively inhibiting RdRp (Furuta et al., 2005). Favipiravir causes the production of non-infectious viral particles when it is employed by the viral polymerase as an alternative nucleoside substrate.
Favipiravir was investigated in both non-fatal and fatal SNV/ANDV hamster models, and the outcome showed a reduction in viral load in hamster serum and different organs. Furthermore, in the lethal ANDV infection model, the use of Favipiravir led to 100% survival (Safronetz et al., 2013). Other studies have shown that after viremia has started, the ANDV/hamster model did not offer protection against delayed antiviral treatment (Munir et al., 2021).
6.2.4. Baloxavir acid
Small molecules also gained importance for their antiviral potential (Deng et al., 2020). Baloxavir, a recently licensed influenza medication derived from BXM, is an endonuclease-targeting small molecule that prevents the translation of the influenza virus and also prevents the virus from replicating. Since the mRNA produced by the viral RdRp lacks a 5′ cap and both hantaviruses and influenza viruses fall within the category of negative-sense RNA viruses, these viruses must acquire a host mRNA cap and apply it to their mRNA (Reguera et al., 2010). BXA specifically targets the PA-PB1-PB2 trimer belonging to the endonuclease domain of the influenza virus. The structures of the RdRp molecules of VSV, a mononegavirus, and another bunyavirus, La Crosse virus (LACV), among others, suggest that the RdRp molecules of negative-sense viruses share certain fundamental characteristics. Particularly within the order Bunyavirales, the endonuclease domain of RdRp appears to be more conserved than the other domains. BXA was incorporated into the active center of the RNA endonuclease domain by utilizing the well-known ANDV LP RNA endonuclease domain structure and computationally modeling the HTNV domain. This novel method revealed a previously unknown relationship with Influenza B virus (IBV), illuminating the potential fitness implications. Modeling outcomes might explain why BXA inhibits Hantavirus replication. Additionally, because of the structural closeness of the endonucleases in these viruses, this approach may also apply to arenaviruses (Ye et al., 2019).
6.2.5. siRNA-based therapy
RNA interference (RNAi) has emerged as a new exciting frontier for antiviral therapies. RNAi is a post-transcriptional, and highly specific cellular mechanism in eukaryotic cells where small non-coding RNA molecules (typically 21–25 nucleotides long) bind to specific mRNA molecules and degrade them, thereby inhibiting the expression of specific genes (Ambesajir et al., 2012). For the degradation and cleavage of the mRNA before translation, the siRNA duplexes are integrated into an RNA-induced silencing complex (RISC) (Fire et al., 1998; Elbashir et al., 2001; MacRae et al., 2006). The siRNAs possess complementary sequences that bind to the target mRNA after transcription and inhibit its translation, as illustrated in Figure 7. The precision and accuracy of RNAi make it an accessible option for gene silencing (Tian et al., 2021). Since the discovery of RNAi in 1900, RNAi technologies have developed rapidly to suppress rogue viruses. So, there is a wide range of viruses that can be inhibited by RNAi-based methods, both in vivo and in vitro (Weinberg and Arbuthnot, 2010).
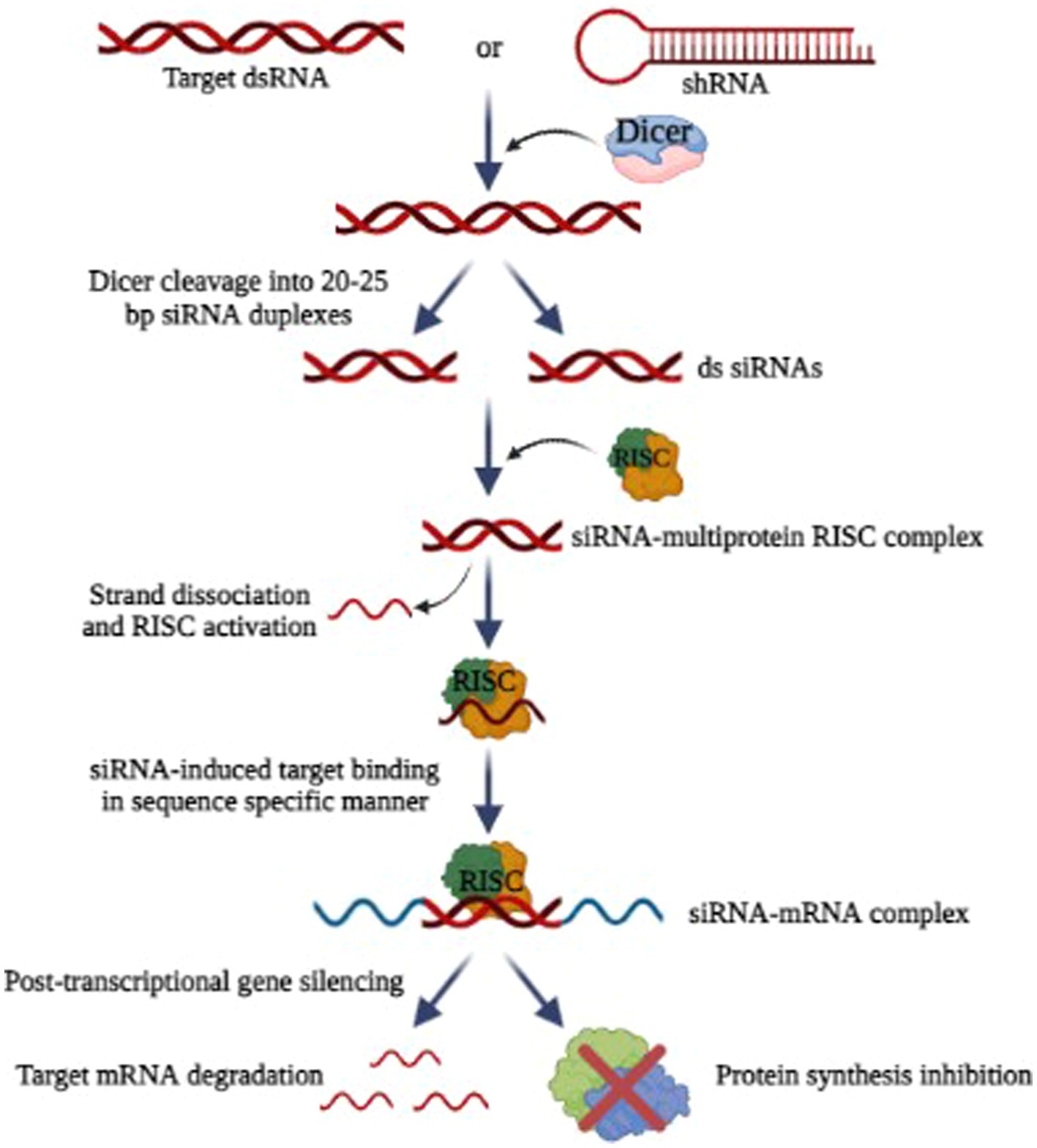
Figure 7. Mechanism of RNA interference (Image generated: www.biorender.com).
Research has shown that HIV-1, polioviruses, nairoviruses, and Lassa viruses can be eradicated using RNAi as a strategy in vitro by inhibiting viral replication (Flusin et al., 2011). Researchers found that RNAi can be used to inhibit SARS-CoV-2 replication (Ricci et al., 2020). For some other viruses that affect humans and animals, RNAi-based therapies effectively reduce viral loads and increase the chances of survival (DeVincenzo et al., 2010). But the problem with RNAi technology is its delivery and most of the studies are only limited to in vitro. Scientists are working to find carriers to safely deliver the siRNA (Bobbin and Rossi, 2016). In this way, RNAi combinations can avoid problems associated with multidrug sensitivities and toxicity. By targeting rapidly evolving viral sequences, RNAi-based therapies prevent the emergence of drug-resistant viruses (Chen et al., 2018). It is also possible for RNAi-based drugs to produce a sustained therapeutic response since genes can be introduced.
Hantaviral replication can be prevented most directly and effectively by targeting viral RNAs. A prospective antiviral method has been evaluated using siRNA against targeted hantaviral genes, which may enhance virus RNA clearance based on RNA interfering (RNAi) processes. In Vero E6 cells or human lung microvascular endothelial cells, it has been demonstrated that siRNAs targeting the S, M, or L segments of the ANDV may decrease viral replication. In Vero E6 cells, an S-targeted siRNA pool appeared to be more efficient in inhibiting viral transcription and replication than an M- or L-targeted siRNA pool (Chiang et al., 2014). Significantly, even if administered after infection, these siRNAs may prevent ANDV replication.
However, since siRNAs are low in biological stability and in vivo targeting ability, their antiviral efficacy may be severely hindered despite their successful inhibition of Hantavirus amplification in host cells. One method of treating in a mouse model of HTNV-induced encephalitis is intraperitoneal administration of recombinant antibodies that recognize HTNV Gc (3G1-Ck-tP). These antibodies were combined with siRNAs that target the encoding regions of the HTNV genome. This resulted in siRNAs being delivered precisely to HTNV-infected brain cells and HTNV intracranial infection being prevented (Yang et al., 2017). Moreover, the shRNA expression showed promising results by inhibiting HTNV infection in both in vitro and in vivo (Liu et al., 2016). To ensure the stability and selectivity of siRNAs, innovative delivery mechanisms should be created; however, it is yet unknown how effective and safe these systems will be in the treatment of HFRS or HCPS.
6.3. Host-targeting antiviral
6.3.1. Vandetanib
Hantavirus-induced increased endothelial microvascular cell permeability was checked through in-vitro techniques. The expression of the cellular adhesion molecules including VE-cadherin and VEGF were both increased and decreased as a result of ANDV infection, which also increased the phosphorylation of the VEGF-receptor 2 (VEGFR2) (Gorbunova et al., 2010; Bird et al., 2016). As a result of ANDV infection, the VEGF-A expression was shown to be enhanced in the 3D model of human lungs tissue (Sundström et al., 2016).
The activation of SFK (Src family kinases) signaling may result from the binding of VEGF to VEGFR2, which has the ability to cause the dissociation, internalization, and destruction of VE-cadherin. The structural integrity of adherent junctions was damaged as a result of changes in VE-cadherin expression and localization, which led to an increase in cellular permeability (Jiang et al., 2016). According to studies, the connection between β3 integrin and VEGFR2 can be disrupted by HTNV or ANDV infection, which causes excessive phosphorylation of VEGFR2. Because of this disturbance, infected endothelium cells may become more permeable by becoming more VEGF-responsive (Wang et al., 2012).
In one investigation, it was discovered that the use of a VEGFR2 kinase inhibitor and SFK inhibitors significantly reduced the increased endovascular permeability brought on by ANDV. Particularly successful were the SFK inhibitors dasatinib and pazopanib, which prevented VE-cadherin separation by more than 90% (Gorbunova et al., 2011). Another study demonstrated that Vandetanib, a tyrosine kinase inhibitor specifically target VEGFR2, has a capacity to inhibit in vitro phosphorylation of VEGFR2, leading to the reduction of VE cadherin degradation (Bird et al., 2016). However, Vandetanib showed signs of potential side effects during human studies, including hypertension, dermatologic responses, and other cardiorespiratory consequences (Grande et al., 2013).
In the ANDV/hamster model, giving the therapy at doses of 10, 25 and 50 mg/kg/day, beginning 5 days prior the ANDV threat, resulted in a slowed mortality and raised overall survival rate by 23%. The same tiny therapeutic molecules, however, failed to protect the hamsters from a lethal ANDV challenge when given once viremia had started in the hamster model infected with ANDV (Munir et al., 2021).
6.3.2. Bradykinin B2 receptor antagonists
Using bradykinin receptor antagonists as a treatment for Hantavirus infections is another interesting strategy. All Hantavirus infections commonly cause vascular leakage and increased capillary permeability. The underlying mechanisms that result in alterations in vascular permeability following Hantavirus infection are yet unknown. Hantaviruses have been reported to be the cause of enhanced stimulation of kinin-kallikrein system following endothelial cells infection, which leads to release of bradykinin (Golias et al., 2007). Bradykinin is a nonapeptide-binding bradykinin B2 receptor that functions as an inflammatory mediator that causes vessels to dilate, increases vascular leakage and lowers blood pressure in Hantavirus infection.
It is acknowledged as the main facilitator of vascular leakage by destroying inter-endothelial connections. Additionally, the synthesis of interleukin-1 and tumor necrosis factor alpha is also induced (Maurer et al., 2011). Icatibant, a synthetic polypeptide that resembles bradykinin structurally, works as a strong, focused, and aggressive antagonist of the bradykinin B2 receptor. Icatibant binds to the bradykinin B2 receptor, preventing bradykinin from attaching to this receptor (Taylor et al., 2013). It stops vasodilatation brought on by bradykinin in humans and in C1 esterase inhibitor-knockout animals, it reverses enhanced vascular permeability, as well as inhibiting bradykinin-induced effects in vivo with dose- and time-dependent inhibition (Cockcroft et al., 1994; Cicardi et al., 2010). Following subcutaneous injection, Icatibant is almost completely bioavailable. Many people just need a single 30-mg dose because the drug is well tolerated (Cicardi et al., 2010; Deeks, 2010).
6.4. Corticosteroid therapy/anti-inflammatory agents
As discussed already, Hantavirus affects endothelium cells and causes the host to produce pro-inflammatory cytokines especially TNF-α during the course of infection. Although not categorized as an antiviral, restricting this aspect of the immunological response to virus infection was hypothesized to offer potential clinical advantages. An immunomodulatory treatment was firstly performed in which only intramuscular injection or oral administration of cortisone was permitted. Although there was no reduction in mortality with this therapeutic approach, fatality was decreased to shock. An additional method to administer methylprednisolone for the treatment of HCPS was used but the outcome offered no clinical advantages (Priya and Priya, 2020).
6.5. Immunotherapy
Immunotherapy could be done against HTNV by utilizing neutralizing antibodies (NAbs) generated as a result of Hantavirus infection. It has been established that human convalescent plasma offers protective benefits to animals in both infection and lethal disease models of SNV/mouse (Medina et al., 2007) and ANDV/hamster (Brocato et al., 2012), respectively. These findings determine the sufficiency of neutralizing antibodies in preventing infection and disease (Brocato and Hooper, 2019).
Studies have only been done with animal models (suckling mice, hamsters, infant rats) where antibody efficacy was measured at cellular level using focus reduction neutralization test (FRNT) and hemagglutination test (HI) (Manigold and Vial, 2014). Although presently no particular effective treatment has been found against hantaviruses that cause HPS, according to numerous studies neutralizing antibodies can block HPS in vivo. An open trial has been carried out in Chile to assess the effectiveness of using human immunological sera as an HPS therapy (Vial et al., 2015). When compared to the 32% case fatality rates in the rest of the nation over the course of the study, the results showed a borderline statistical significance (Vial et al., 2015; Dheerasekara et al., 2020).
It was hypothesized that treatment at an earlier stage of HTNV infection could further enhance results. However, the practical implementation of immunotherapy is restricted by the requirement for ABO blood typing of convalescent plasma and the absence of a standardized product, which prevents its widespread use (Brocato and Hooper, 2019).
6.5.1. Monoclonal antibodies
Antibodies have been employed on a global scale to prevent and treat viral infectious diseases (Casadevall, 1999). Researchers are currently working on generating antibodies targeting various viruses responsible for causing hemorrhagic fevers. One instance involves the development of a group of engineered human monoclonal antibodies (MAbs) designed to combat the Ebola virus (Maruyama et al., 1999). These specialized antibodies have shown efficacy not only in treating infections but also in providing protection before and after exposure to various viruses, including cytomegalovirus (CMV) (Aulitzky et al., 1991) and respiratory syncytial virus (RSV) (Malley et al., 1998). Up until now, there has not been a successful therapeutic antibody available for clinical use in treating and preventing Hantavirus infection. As a result, the development of Hantavirus-neutralizing MAbs is of utmost importance to establish effective immunotherapy and prophylaxis against Hantavirus infection (Xu et al., 2002).
During the 1980s, researchers extensively studied monoclonal antibodies targeting HTNV, detailing their interactions with the glycoproteins, Gn and Gc, and identifying specific neutralization sites (Arikawa et al., 1989). In an attempt to establish a link between particular viral epitopes and protection against HTNV infection, researchers conducted assessments on monoclonal antibodies using passive transfer techniques (Schmaljohn et al., 1990). This significant experiment provided compelling evidence that out of the 15 tested monoclonal antibodies, a neutralizing immune response to either Gn or Gc alone is adequate to prevent HTNV infection in hamsters. Following the HTNV/hamster experiment, the effectiveness of the recombinant Human GP monoclonal antibody HCO2 in providing protection was verified (Liang et al., 1996). This protective potential of neutralizing monoclonal antibodies was further validated in a later study utilizing a suckling mouse/HTNV model (Arikawa et al., 1992).
In a recent development, two genetically engineered monoclonal antibodies demonstrated their ability to protect hamsters from fatal ANDV-HPS (Garrido et al., 2018). One source patient with high antibody titers was chosen after screening 27 ANDV convalescent HCPS sera. Utilizing recombinant DNA technology, researchers generated recombinant monoclonal antibodies from memory B cells specific to the ANDV glycoprotein. The resulting candidates, JL16 and MIB22, displayed effective neutralization of ANDV in vitro (Garrido et al., 2018; Dheerasekara et al., 2020). When passively transferred antibodies on days 3 and 8 after infection, the administered antibodies successfully prevented lethality in hamsters infected with ANDV via intranasal exposure, whether given individually or in combination (Brocato and Hooper, 2019).
In another investigation, researchers produced and analyzed 18 murine monoclonal antibodies (MAbs) targeting HTNV strain Chen. Out of these, 13 MAbs were directed at the viral nucleocapsid protein (NP), four identified the viral envelope glycoprotein G2, and one MAb responded with both NP and G2. Only the monoclonal antibodies (MAbs) that specifically targeted epitopes on G2 exhibited positive results in the hemagglutination inhibition (HI) test. Additionally, these MAbs demonstrated in vitro virus-neutralizing activity and provided in vivo protection against HTNV infection in susceptible mice. Because the mice received virus-neutralizing MAbs one day before and two days after being exposed to HTNV, all of them were protected. This suggests that these particular neutralizing MAbs could be helpful for both pre- and post-exposure preventive measures, as well as potential immunotherapy against HTNV infection. In endemic regions of China, Phase II clinical trials are being conducted to evaluate the efficacy of these neutralizing MAbs as an emergent treatment for patients in the early stages of HRFS (Xu et al., 2002).
Anti-Hantaan Virus murine monoclonal antibody was developed for treating HFRS and a single dose of 2.5-20 mg was administered to healthy Chinese volunteers intravenously and the results indicated that it was nicely received (Xu et al., 2009). The cross-reactivity of novel and previously created MAbs effective against N protein of TULV, TMPV, DOBV and PUUV were assessed contrary to N proteins of fifteen shrew and rodent borne hantaviruses using various immunological techniques in order to have a large collection of well-described Hantavirus-specific MAbs. The results indicated that all MAbs, with the exception of those that are exclusive to TPMV, displayed various cross-reactivity patterns with Hantavirus N proteins and recognized native viral antigens in infected mammalian cells (Avižinienė et al., 2023). A recently discovered widely neutralizing antibody was structurally analyzed by Mittler et al. (2023) on a patient who had recovered from Puumala virus (Old world Hantavirus) infection. The authors created an improved variant of this patient-extracted antibody that could defend against Andes (New world Hantavirus) and Puumala virus, in rodent models using functional research, structural data along with complementary binding and neutralization. The therapeutic candidate ADI-65534, which is a broadly neutralizing antibody possesses a potential to treat Hantavirus infections.
6.5.2. Polyclonal antibodies
In a recent study, polyclonal immunotherapy in which purified IgG polyclonal antibodies produced in DNA immunized alpacas (ANDV M/SNV M) were given to Syrian hamsters, which protected them completely against HPS (Sroga et al., 2021). Studies have demonstrated the immunogenicity of Hantavirus DNA vaccines in various animal species, such as geese, rabbits and ducks (Hooper et al., 1999; Brocato et al., 2012, 2013). A substantial step forward was recently achieved with the development of a fully human polyclonal antibody using trans-chromosomal bovine that had received ANDV and SNV DNA vaccines. This product has demonstrated positive protection against two deadly HPS animal models (Hooper et al., 2014). Presently, researchers are actively working to broaden their investigation and develop a standardized polyclonal antibody capable of targeting a variety of Hantaviruses, including HTNV, ANDV, SNV, and PUUV. The objective is to advance this product through preclinical testing and ultimately carry out a Phase 1 clinical study, paving the path for potential therapeutic applications. Table 3 (Vilcek, 1991; Murphy et al., 2000, 2001; Sundstrom et al., 2001; Glass et al., 2003; Maes et al., 2004; Chung et al., 2008, 2013; Hall et al., 2008; Antonen et al., 2013; Ogg et al., 2013; Safronetz et al., 2013; Vial et al., 2013, 2015; Laine et al., 2015; Barriga et al., 2016; Bird et al., 2016; Garrido et al., 2018; Brocato and Hooper, 2019).
7. Vaccines and immunotherapy
As viruses are constantly emerging from zoonotic origin, vaccines seem to be the most effective therapeutic option to reduce the incidence of disease (Ahmed et al., 2022). Different vaccines are under development against hantaviruses to improve protective efficacy and safety profiles (Saavedra et al., 2021). Attenuated and killed vaccines are the most common and primitive method of vaccine development that is injected into the animal or human body to elicit a protective immune response (Naeem et al., 2021). These vaccines are prepared by growing the isolated viral strain on the Vero cell line followed by inactivation through physical and chemical means. Similarly, the formalin-inactivated vaccine, Hantavax, was the first developed vaccine to prevent hantaviral infection in South Korea, it was developed using the HTNV strain ROK 84/105, which multiplies in lactating mice’s brains. Its clinical trial proved that it was well endured in human volunteers and successfully lowered the incidence of HFRS patients (Cho et al., 2002; Dheerasekara et al., 2020). However, the neutralizing antibody response was poor after two doses, therefore 3rd dose was injected to attain protective immune response in the host that lasts for 3–4 year (Dheerasekara et al., 2020). A bivalent inactivated vaccine against infection caused by SEOV and HTNV was developed in 1994 and was approved for use in China in 2005. It was found effective against HTNV and SEOV infections (Cho et al., 2002).
The success achieved by Hantavax is to decrease the incidence of hantaviral infection (Munir et al., 2021) but still needs a more effective and safe vaccine against Hantavirus which becomes possible to improve through modernization in virology and molecular biology with the development of more applied biological techniques. Hantavax is less efficient for long-term immunity and negligible cell-mediated immunity which can be overcome by immunizing the individual multiple times. Therefore, a vaccine is required that induces more effective and long-lasting immunity against the Hantavirus (Mohsen et al., 2017). Virus-Like Particles are considered efficient with better safety profiles, and prolonged immunity with the production of high titers of antibodies in humans (Mohsen and Bachmann, 2022). VLPs of Hantavirus are constructed using the M and S gene segment or only the M segment that interacts with each other to form virus-like particles in vitro similar to Hantavirus virion (Acuña et al., 2014; Brocato and Hooper, 2019) along with the incorporation of CD40L or GMCSF gene segment in vectors that stimulates activation of macrophages and dendritic cells (Ying et al., 2016; Dong et al., 2019). CD40L or GMCSF decorated VLPs provide prolonged immunity with elevated humoral and cell-mediated immune response than undecorated VLPs (Ying et al., 2016; Dong et al., 2019). Another approach involves the insertion of gene segments from nucleocapsid protein from DOBV, HTNV, and PUUV into HBV core particles, it is shown to be highly immunogenic with or without adjuvant that stimulates the generation of all classes of IgG antibodies (Brocato and Hooper, 2019).
Recombinant vaccines are constructed using Glycoprotein C (Gc), Glycoprotein N (Gn), or Nucleocapsid protein that shows high immunogenicity and antigenicity, bearing the ability to induce protection against Hantavirus (Dheerasekara et al., 2020). The baculovirus expression system was used to develop the more efficient recombinant vaccines using Gc, Gn, or N protein (Dheerasekara et al., 2020) followed by immunization in hamsters which develop partial protection from infection when used solely either Gc or Gn and complete protection when Gc/Gn used in combination or immunized with N protein (Brocato and Hooper, 2019). The nucleocapsid protein is more conserved among different hantaviral species, therefore, the immune response produced against N protein induces highly cross-reactive antibody responses to PUUV, DOBV, and ANDV produced by E. coli (Krüger et al., 2011). Moreover, the use of adjuvants enhances the immunogenicity and protective efficacy of the vaccine in humans (Porter et al., 2012).
The S and M cDNA segment of HTNV was inserted into the vaccinia virus to develop a molecular virus vector-based vaccine (Brocato and Hooper, 2019). Protective efficacy was evaluated in Syrian hamsters by inoculating two doses of VACV-vectored HTNV vaccine which resulted in protection from HTNV or SEOV but not PUUV (Munir et al., 2021). Cross-reactive antibodies of HTNV protected against SEOV but were unable to protect from PUUV (Malinin and Platonov, 2017). Clinical trials were conducted to further evaluate the vaccine efficacy (McClain et al., 2000; Brocato and Hooper, 2019) and it was confirmed that the vaccine was safe to inoculate and resulted in the production of neutralizing antibodies against both VACV and HTNV, and the subcutaneous route was preferred to administer the vaccine (Perley et al., 2020). The efficacy of the vaccine was also evaluated in vaccinated and non-vaccinated individuals and 72% efficacy was observed in non-vaccinated and 26% in vaccinated individuals (Dheerasekara et al., 2020). Moreover, non-replicating adenovirus vectors were used to provoke vigorous cytolytic response when ANDV N protein, Gn, Gc, or Gc and Gn in combination was expressed (Safronetz et al., 2009). Similarly, the Vesicular stomatitis virus (VSV) pseudo-type virus containing Gn and Gc of Hantavirus and ANDV glycoprotein precursor (GPC)was separately inoculated in mice and hamsters and it produced robust neutralizing antibody response (Saavedra et al., 2021). However, it required 3 doses to produce long-term immunity in an individual (Brocato and Hooper, 2019; Saavedra et al., 2021).
All vaccines have shown their efficacy against hantaviruses but the safety and efficacy was varied between primitive and modern-generation vaccines. DNA vaccine encoding the HTNV Gn and lysosomal-associated membrane protein 1 which directed to major histocompatibility complex II (MHC) and processed as an exogenous antigen resulting in robust humoral and cell-mediated immune response (Jiang D.-B. et al., 2017). Normally, inactivated vaccines have poor immunological memory but DNA vaccines have much-improved memory profiles. The S and M segments of SEOV were cloned into an expression vector pWRG7077 or Sindbis replicon vector (Munir et al., 2021). The results depicted that the M segment had shown improved protection from SEOV infection in Syrian hamsters (Brocato et al., 2021). Therefore, the M segment of HTNV, PUUV, ANDV, SNV, SEOV, and DOBV is used for vaccination in non-human primates that elicits an elevated level of antibody response (Liu et al., 2020). Several DNA vaccines against HTNV associated infections are currently undergoing clinical trials. Hopper’s group have developed several vaccines against the envelope glycoprotein gene of hantaviruses and further studies have confirmed the ability of these vaccines to produce neutralizing antibodies against HFRS in multiple animal species and even protected hamsters against HFRS (Schmaljohn et al., 2014). Apart from vaccine development, delivery methods and routes are much more significant to achieve desired efficacy. Multiple administration routes are studied and found gene gun inoculation is more effective than any other route (Dheerasekara et al., 2020). Moreover, a combination of different DNA vaccines of hantaviruses is much more efficient to provoke an immune response rather than a single vaccine administration (Brocato et al., 2013). Currently no FDA approved treatment and vaccines are available against HTNV worldwide. There are still many unresolved issues regarding mass production, safety and efficacy and no significant effect in lowering the severity of the disease. However, more research is required in vaccine delivery routes to improve the immunogenicity of the vaccine.
Apart from vaccines, monoclonal and polyclonal antibodies are used to eliminate hantaviral pathogens in the human or animal body against Gc and Gn (Duehr et al., 2020). Furthermore, DNA vaccination of ANDV, SNV, HTNV, and PUUV in bovines produces purified polyclonal human IgG antibodies exhibiting high neutralization activity and provided effective protection against lethal HCPS (Liu et al., 2020). Therefore, it is worldwide tested and used as a promising prophylactic therapy. The administration of neutralizing antibodies during the acute phase of HPS is considered an effective treatment for hantaviral infection (Iglesias et al., 2022). The patients with low titers of neutralizing antibodies often had a severe disease while mild disease cases were present in individuals with higher antibody titers (Iglesias et al., 2022; Iheozor-Ejiofor et al., 2022). Therefore, different clinicians and scientists speculated that a strong neutralizing antibody response or passive immunization can efficiently reduce the severity of the disease by reducing viremia (Shah et al., 2020).
8. Future therapeutic developments
8.1. Hantavirus-induced cytokine and chemokine response
One of the main contributors to HPS and HFRS symptoms during the course of Hantavirus infection may be the cytokine production. Cytokines, particularly TNF-α, IL-1, and IL-6 play a vital role in inducing fever and septic shock. TNF-α is produced by Hantavirus infected neutrophils, NK cells, CD8+ T cells, DC and macrophages (Schönrich et al., 2015). The excessive production of TNF-α may cause systemic toxicity (Maes et al., 2004). Although the precise mechanism is yet unknown, these cytokines also play a significant part in the vascular permeability seen in HPS and HFRS (Sundstrom et al., 2001). One of the most significant pro-inflammatory cytokines is TNF-α. Monocytes and macrophages infiltrate the area of inflammation and release TNF-α (Vilcek, 1991). Patients with severe NE were found to have significantly higher plasma levels of TNF-α (Linderholm et al., 1996). The kidney biopsies from NE patients revealed that TNF-α expression was elevated in the peritubular regions (Temonen et al., 1996). Patients with HTNV infection have higher serum levels of soluble IL-2 receptor (sIL-2R) and soluble IL-6 receptor (sIL-6R). sIL-2R and sIL-6R serum levels increased two days and six days after the onset of HFRS, respectively (Markotic et al., 2002). The infected monolayers of endothelial cells remained irreversibly permeable while uninfected monolayers fully restored their function (Niikura et al., 2004). Chemokines serve as inflammatory mediators and are responsible for the regulation of viral infections. HTNV infected endothelial cells in vitro, resulted in the production of significant amount of interferon-inducible protein (IP-10) and regulated upon activation, normal T cell expressed and secreted (RANTES), also called CCL5 (Sundstrom et al., 2001). Another study showed production of chemokines like RANTES, IP-10, IL-6, and IL-8 by HTNV but NYV failed to generate the majority of these cellular chemokines (Geimonen et al., 2002). Inflammatory chemokines like RANTES and monocyte chemotactic protein-1 (MCP-1), usually produced by acute respiratory viruses, can increase inflammatory responses giving rise to virus immunopathology (Glass et al., 2003). All supernatant cell lines harboring hantaviruses have a substantial increase in RANTES mRNA (Khaiboullina and St. Jeor, 2002). The conclusion that chemokines play a significant role in virus pathogenesis, which has been drawn for other viruses, is supported by this data. Due to the dual functions that these chemokines play in viral infections, inhibiting them or using them as immunomodulators may be important strategies to treat or reduce viral illness, depending on the type of virus. During HTNV infection, production of IP-10 and RANTES can lead to an increased effector immune response directed against the infected vascular endothelial cells (Sundstrom et al., 2001). Consideration should be given to a general therapeutic strategy for Hantavirus infections that combine antiviral and anti-chemokine therapy.
8.2. Potential vaccines undergoing development against Hantavirus
Hantaviruses enter human societies by zoonotic transmission via inhaling contaminated aerosols. Normally, half a million people are infected worldwide annually with a mortality rate of up to 40%. The HFRS and HPS seem to be serious health threats in endemic areas due to their cryptic transmission and unpredictable nature of disease occurrence in healthy adults with elevated case fatality rates. The inappropriate commercialization of therapeutics in endemic areas constantly increases the prevalence of the disease. Moreover, no special antiviral drugs were found efficient to be used in hantaviral infection except ribavirin. However, during HPS and HFRS cases, the ribavirin had been found effective but its efficacy could not prevent the severity of the disease.
Since there are so many cases of hantaviral infection each year, medicinal countermeasures to prevent infection from these viruses must be developed. Animal models have repeatedly shown that antivirals are not effective if administered after the onset of viremia. Therefore, the development of vaccination and an antiviral that can be used separately or in combination is much necessary for public health. Immunization may provide long-lasting immunity while an antiviral, such as polyclonal antibody treatment, would provide immediate immunity. So, vaccines and passive immunotherapy can effectively prevent and treat hantaviral infections in endemic regions of the world. The transmission from person to person becomes limited by vaccination and some vaccines are currently undergoing clinical trials (see Table 4) (Hooper et al., 2001, 2006, 2013; de Carvalho et al., 2002; Geldmacher et al., 2004; Maes et al., 2006, 2008; Safronetz et al., 2009; Brocato et al., 2013; Prescott et al., 2014; Jiang D.-B. et al., 2017; Dong et al., 2019; Khan et al., 2019; Warner et al., 2019).
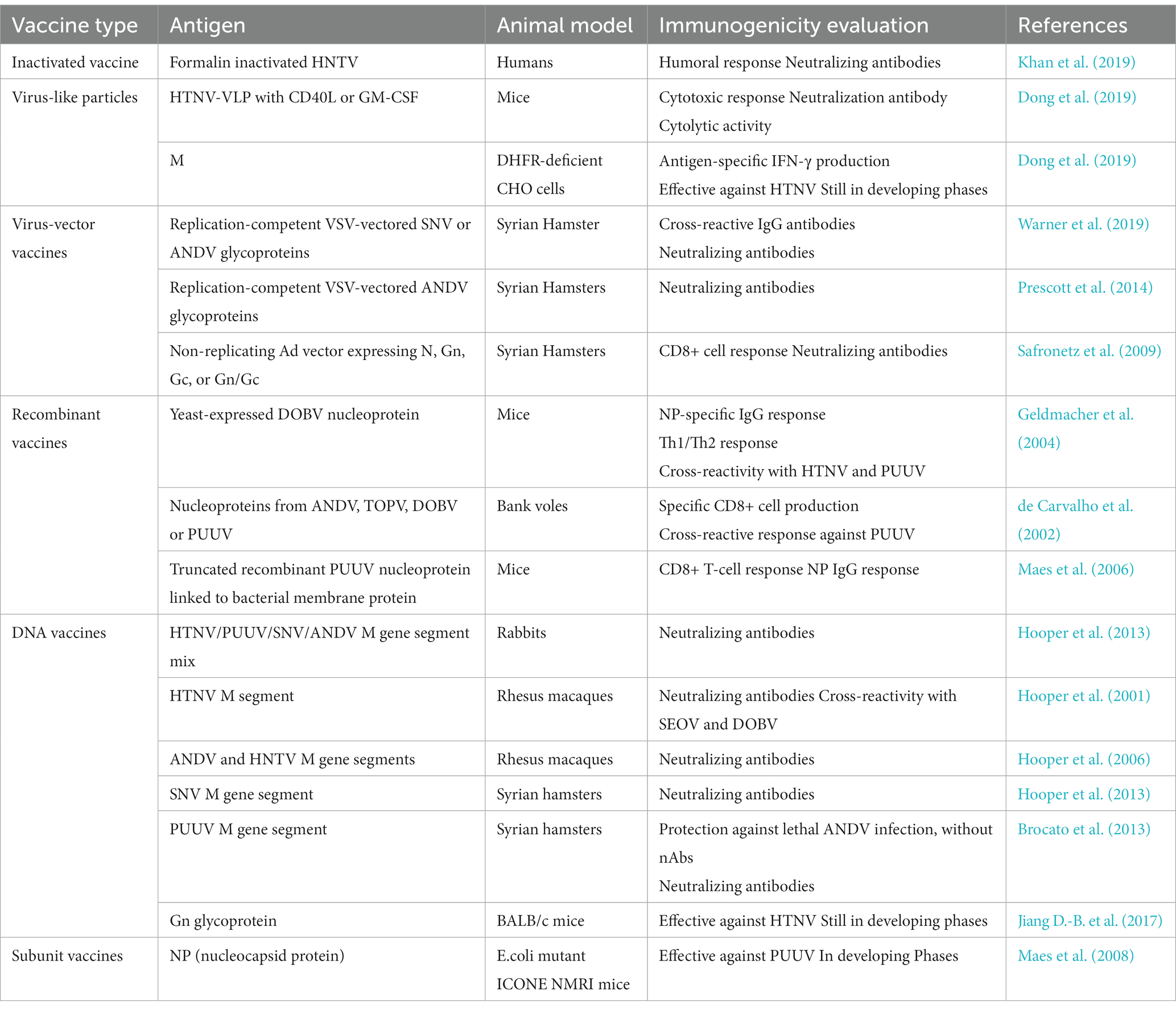
Table 4. Describing evaluation of Hantavirus vaccines in various animal models and some vaccines currently undergoing clinical trials.
Author contributions
LA and SA conceived the study. LA, MA, MH, MS, AA, AB, JY, and NK searched the literature and drafted the manuscript. LA, SA, AB, NK, and JY critically reviewed the manuscript. All authors contributed to the article and approved the submitted version.
Conflict of interest
JY was employed by Wuhan Institute of Biological Products Co., Ltd.
The remaining authors declare that the research was conducted in the absence of any commercial or financial relationships that could be construed as a potential conflict of interest.
Publisher’s note
All claims expressed in this article are solely those of the authors and do not necessarily represent those of their affiliated organizations, or those of the publisher, the editors and the reviewers. Any product that may be evaluated in this article, or claim that may be made by its manufacturer, is not guaranteed or endorsed by the publisher.
References
Acuña, R., Cifuentes-Muñoz, N., Márquez, C. L., Bulling, M., Klingström, J., Mancini, R., et al. (2014). Hantavirus gn and gc glycoproteins self-assemble into virus-like particles. J. Virol. 88, 2344–2348. doi: 10.1128/JVI.03118-13
Ahmed, A., Safdar, M., Sardar, S., Yousaf, S., Farooq, F., Raza, A., et al. (2022). Modern vaccine strategies for emerging zoonotic viruses. Expert Rev. Vaccines 21, 1711–1725. doi: 10.1080/14760584.2022.2148660
Aitichou, M., Saleh, S. S., McElroy, A. K., Schmaljohn, C., and Ibrahim, M. S. (2005). Identification of Dobrava, Hantaan, Seoul, and Puumala viruses by one-step real-time RT-PCR. J. Virol. Methods 124, 21–26. doi: 10.1016/j.jviromet.2004.10.004
Ambesajir, A., Kaushik, A., Kaushik, J. J., and Petros, S. T. (2012). RNA interference: a futuristic tool and its therapeutic applications. Saudi J. Biol. Sci. 19, 395–403. doi: 10.1016/j.sjbs.2012.08.001
Antonen, J., Leppänen, I., Tenhunen, J., Arvola, P., Mäkelä, S., Vaheri, A., et al. (2013). A severe case of Puumala Hantavirus infection successfully treated with bradykinin receptor antagonist icatibant. Scand. J. Infect. Dis. 45, 494–496. doi: 10.3109/00365548.2012.755268
Arikawa, J., Schmaljohn, A. L., Dalrymple, J. M., and Schmaljohn, C. S. (1989). Characterization of Hantaan virus envelope glycoprotein antigenic determinants defined by monoclonal antibodies. J. Gen. Virol. 70, 615–624. doi: 10.1099/0022-1317-70-3-615
Arikawa, J., Yao, J.-S., Yoshimatsu, K., Takashima, I., and Hashimoto, N. (1992). Protective role of antigenic sites on the envelope protein of Hantaan virus defined by monoclonal antibodies. Arch. Virol. 126, 271–281. doi: 10.1007/BF01309700
Armién, B., Muñoz, C., Cedeño, H., Salazar, J. R., Salinas, T. P., González, P., et al. (2023). Hantavirus in Panama: twenty years of epidemiological surveillance experience. Viruses 15:1395. doi: 10.3390/v15061395
Aulitzky, W., Schulz, T., Tilg, H., Niederwieser, D., Larcher, K., Ostberg, L., et al. (1991). Human monoclonal antibodies neutralizing cytomegalovirus (CMV) for prophylaxis of CMV disease: report of a phase I trial in bone marrow transplant recipients. J. Infect. Dis. 163, 1344–1347. doi: 10.1093/infdis/163.6.1344
Avižinienė, A., Kučinskaitė-Kodzė, I., Petraitytė-Burneikienė, R., Žvirblienė, A., Mertens, M. L., Schmidt, S., et al. (2023). Characterization of a panel of cross-reactive Hantavirus Nucleocapsid protein-specific monoclonal antibodies. Viruses 15:532. doi: 10.3390/v15020532
Avšič-Županc, T., Saksida, A., and Korva, M. (2019). Hantavirus infections. Clin. Microbiol. Infect. 21s, e6–e16. doi: 10.1111/1469-0691.12291
Barriga, G. P., Villalón-Letelier, F., Márquez, C. L., Bignon, E. A., Acuña, R., Ross, B. H., et al. (2016). Inhibition of the Hantavirus fusion process by predicted domain III and stem peptides from glycoprotein Gc. PLoS Negl. Trop. Dis. 10:e0004799. doi: 10.1371/journal.pntd.0004799
Baudouin, V., Crusiaux, A., Haddad, E., Schandene, L., Goldman, M., Loirat, C., et al. (2003). Anaphylactic shock caused by immunoglobulin E sensitization after retreatment with the chimeric anti-interleukin-2 receptor monoclonal antibody basiliximab. Transplantation 76, 459–463. doi: 10.1097/01.TP.0000073809.65502.8F
Bi, Z., Formenty, P. B., and Roth, C. E. (2008). Hantavirus infection: a review and global update. J. Infect. Dev. Countries 2, 003–023. doi: 10.3855/jidc.317
Bird, B. H., Shrivastava-Ranjan, P., Dodd, K. A., Erickson, B. R., and Spiropoulou, C. F. (2016). Effect of Vandetanib on Andes virus survival in the hamster model of Hantavirus pulmonary syndrome. Antivir. Res. 132, 66–69. doi: 10.1016/j.antiviral.2016.05.014
Björkström, N. K., Lindgren, T., Stoltz, M., Fauriat, C., Braun, M., Evander, M., et al. (2011). Rapid expansion and long-term persistence of elevated NK cell numbers in humans infected with Hantavirus. J. Exp. Med. 208, 13–21. doi: 10.1084/jem.20100762
Bobbin, M. L., and Rossi, J. J. (2016). RNA interference (RNAi)-based therapeutics: delivering on the promise? Annu. Rev. Pharmacol. Toxicol. 56, 103–122. doi: 10.1146/annurev-pharmtox-010715-103633
Bressanelli, S., Stiasny, K., Allison, S. L., Stura, E. A., Duquerroy, S., Lescar, J., et al. (2004). Structure of a flavivirus envelope glycoprotein in its low-pH-induced membrane fusion conformation. EMBO J. 23, 728–738. doi: 10.1038/sj.emboj.7600064
Brocato, R. L., and Hooper, J. W. (2019). Progress on the prevention and treatment of Hantavirus disease. Viruses 11:610. doi: 10.3390/v11070610
Brocato, R., Josleyn, M., Ballantyne, J., Vial, P., and Hooper, J. W. (2012). DNA vaccine-generated duck polyclonal antibodies as a postexposure prophylactic to prevent Hantavirus pulmonary syndrome (HPS). PLoS One 7:e35996. doi: 10.1371/journal.pone.0035996
Brocato, R., Josleyn, M., Wahl-Jensen, V., Schmaljohn, C., and Hooper, J. (2013). Construction and nonclinical testing of a Puumala virus synthetic M gene-based DNA vaccine. Clin. Vaccine Immunol. 20, 218–226. doi: 10.1128/CVI.00546-12
Brocato, R. L., Kwilas, S. A., Josleyn, M. D., Long, S., Zeng, X., Perley, C. C., et al. (2021). Small animal jet injection technique results in enhanced immunogenicity of Hantavirus DNA vaccines. Vaccine 39, 1101–1110. doi: 10.1016/j.vaccine.2021.01.002
Buys, K. K., Jung, K.-H., Smee, D. F., Furuta, Y., and Gowen, B. B. (2011). Maporal virus as a surrogate for pathogenic New World hantaviruses and its inhibition by favipiravir. Antivir. Chem. Chemother. 21, 193–200. doi: 10.3851/IMP1729
Campbell, I. D., and Humphries, M. J. (2011). Integrin structure, activation, and interactions. Cold Spring Harb. Perspect. Biol. 3:a004994. doi: 10.1101/cshperspect.a004994
Casadevall, A. (1999). Passive antibody therapies: progress and continuing challenges. Clin. Immunol. 93, 5–15. doi: 10.1006/clim.1999.4768
Chandy, S., Boorugu, H., Chrispal, A., Thomas, K., Abraham, P., and Sridharan, G. (2009). Hantavirus infection: a case report from India. Indian J. Med. Microbiol. 27:267. doi: 10.4103/0255-0857.53215
Chen, X., Mangala, L. S., Rodriguez-Aguayo, C., Kong, X., Lopez-Berestein, G., and Sood, A. K. (2018). RNA interference-based therapy and its delivery systems. Cancer Metastasis Rev. 37, 107–124. doi: 10.1007/s10555-017-9717-6
Chiang, C.-F., Albariňo, C. G., Lo, M. K., and Spiropoulou, C. F. (2014). Small interfering RNA inhibition of Andes virus replication. PLoS One 9:e99764. doi: 10.1371/journal.pone.0099764
Cho, H.-W., Howard, C. R., and Lee, H.-W. (2002). Review of an inactivated vaccine against hantaviruses. Intervirology 45, 328–333. doi: 10.1159/000067925
Chung, D.-H., Kumarapperuma, S. C., Sun, Y., Li, Q., Chu, Y.-K., Arterburn, J. B., et al. (2008). Synthesis of 1-β-d-ribofuranosyl-3-ethynyl-[1, 2, 4] triazole and its in vitro and in vivo efficacy against Hantavirus. Antivir. Res. 79, 19–27. doi: 10.1016/j.antiviral.2008.02.003
Chung, D.-H., Västermark, Å., Camp, J. V., McAllister, R., Remold, S. K., Chu, Y.-K., et al. (2013). The murine model for Hantaan virus-induced lethal disease shows two distinct paths in viral evolutionary trajectory with and without ribavirin treatment. J. Virol. 87, 10997–11007. doi: 10.1128/JVI.01394-13
Cicardi, M., Banerji, A., Bracho, F., Malbrán, A., Rosenkranz, B., Riedl, M., et al. (2010). Icatibant, a new bradykinin-receptor antagonist, in hereditary angioedema. N. Engl. J. Med. 363, 532–541. doi: 10.1056/NEJMoa0906393
Cifuentes-Munoz, N., Barriga, G. P., Valenzuela, P. D., and Tischler, N. D. (2011). Aromatic and polar residues spanning the candidate fusion peptide of the Andes virus Gc protein are essential for membrane fusion and infection. J. Gen. Virol. 92, 552–563. doi: 10.1099/vir.0.027235-0
Clement, J., LeDuc, J. W., Lloyd, G., Reynes, J.-M., McElhinney, L., Van Ranst, M., et al. (2019). Wild rats, laboratory rats, pet rats: global Seoul Hantavirus disease revisited. Viruses 11:652. doi: 10.3390/v11070652
Cockcroft, J., Chowienczyk, P., Brett, S., Bender, N., and Ritter, J. (1994). Inhibition of bradykinin-induced vasodilation in human forearm vasculature by icatibant, a potent B2-receptor antagonist. Br. J. Clin. Pharmacol. 38, 317–321. doi: 10.1111/j.1365-2125.1994.tb04360.x
de Carvalho, N., Gonzalez Delia Valle, M., Padula, P., Bjorling, E., Plyusnin, A., and Lundkvist, A. (2002). Cross-protection against challenge with Puumala virus after immunization with nucleocapsid proteins from different hantaviruses. J. Virol. 76, 6669–6677. doi: 10.1128/jvi.76.13.6669-6677.2002
Deng, X., Tian, S., Yu, Z., Wang, L., Liang, R., Li, Y., et al. (2020). Development of small-molecule inhibitors against hantaviruses. Microbes Infect. 22, 272–277. doi: 10.1016/j.micinf.2020.05.011
DeVincenzo, J., Lambkin-Williams, R., Wilkinson, T., Cehelsky, J., Nochur, S., Walsh, E., et al. (2010). A randomized, double-blind, placebo-controlled study of an RNAi-based therapy directed against respiratory syncytial virus. Proc. Natl. Acad. Sci. 107, 8800–8805. doi: 10.1073/pnas.0912186107
Dheerasekara, K., Sumathipala, S., and Muthugala, R. (2020). Hantavirus infections—treatment and prevention. Curr. Treat. Options Infect. Dis. 12, 410–421. doi: 10.1007/s40506-020-00236-3
Dong, Y., Ma, T., Zhang, X., Ying, Q., Han, M., Zhang, M., et al. (2019). Incorporation of CD40 ligand or granulocyte-macrophage colony stimulating factor into Hantaan virus (HTNV) virus-like particles significantly enhances the long-term immunity potency against HTNV infection. J. Med. Microbiol. 68, 480–492. doi: 10.1099/jmm.0.000897
Du, H., Li, J., Yu, H.-T., Jiang, W., Zhang, Y., Wang, J.-N., et al. (2014). Early indicators of severity and construction of a risk model for prognosis based upon laboratory parameters in patients with hemorrhagic fever with renal syndrome. Clin. Chem. Lab. Med. 52, 1667–1675. doi: 10.1515/cclm-2014-0016
DuBois, R. M., Vaney, M.-C., Tortorici, M. A., Kurdi, R. A., Barba-Spaeth, G., Krey, T., et al. (2013). Functional and evolutionary insight from the crystal structure of rubella virus protein E1. Nature 493, 552–556. doi: 10.1038/nature11741
Duehr, J., McMahon, M., Williamson, B., Amanat, F., Durbin, A., Hawman, D. W., et al. (2020). Neutralizing monoclonal antibodies against the Gn and the Gc of the Andes virus glycoprotein spike complex protect from virus challenge in a preclinical hamster model. MBio 11, e00028–e00020. doi: 10.1128/mBio.00028-20
Duggan, J. M. (2019). Prevalence of Seoul Hantavirus in UK wild rats: an emerging public health problem? Vet. Rec. 184:523. doi: 10.1136/vr.l1163
Dvorscak, L., and Czuchlewski, D. R. (2014). Successful triage of suspected Hantavirus cardiopulmonary syndrome by peripheral blood smear review. Am. J. Clin. Pathol. 142, 196–201. doi: 10.1309/AJCPNFVWG46NUHED
Echterdiek, F., Kitterer, D., Alscher, M. D., Schwenger, V., Ruckenbrod, B., Bald, M., et al. (2019). Clinical course of Hantavirus-induced nephropathia epidemica in children compared to adults in Germany—analysis of 317 patients. Pediatr. Nephrol. 34, 1247–1252. doi: 10.1007/s00467-019-04215-9
Elbashir, S. M., Harborth, J., Lendeckel, W., Yalcin, A., Weber, K., and Tuschl, T. (2001). Duplexes of 21-nucleotide RNAs mediate RNA interference in cultured mammalian cells. Nature 411, 494–498. doi: 10.1038/35078107
Ermonval, M., Baychelier, F., and Tordo, N. (2016). What do we know about how hantaviruses interact with their different hosts? Viruses 8:223. doi: 10.3390/v8080223
Fernandez-Larsson, R., and Patterson, J. L. (1989). Altered ATP function of a vesicular stomatitis virus mutant detected by kinetic analysis of the transcriptase using phosphorylated ribavirin. J. Gen. Virol. 70, 2791–2797. doi: 10.1099/0022-1317-70-10-2791
Fernandez-Larsson, R., and Patterson, J. L. (1990). Ribavirin is an inhibitor of human immunodeficiency virus reverse transcriptase. Mol. Pharmacol. 38, 766–770.
Fire, A., Xu, S., Montgomery, M. K., Kostas, S. A., Driver, S. E., and Mello, C. C. (1998). Potent and specific genetic interference by double-stranded RNA in Caenorhabditis elegans. Nature 391, 806–811. doi: 10.1038/35888
Flusin, O., Vigne, S., Peyrefitte, C. N., Bouloy, M., Crance, J.-M., and Iseni, F. (2011). Inhibition of Hazara nairovirus replication by small interfering RNAs and their combination with ribavirin. Virol. J. 8, 1–11. doi: 10.1186/1743-422X-8-249
Furuta, Y., Takahashi, K., Kuno-Maekawa, M., Sangawa, H., Uehara, S., Kozaki, K., et al. (2005). Mechanism of action of T-705 against influenza virus. Antimicrob. Agents Chemother. 49, 981–986. doi: 10.1128/AAC.49.3.981-986.2005
Furuta, Y., Takahashi, K., Shiraki, K., Sakamoto, K., Smee, D. F., Barnard, D. L., et al. (2009). T-705 (favipiravir) and related compounds: novel broad-spectrum inhibitors of RNA viral infections. Antivir. Res. 82, 95–102. doi: 10.1016/j.antiviral.2009.02.198
Garrido, J. L., Prescott, J., Calvo, M., Bravo, F., Alvarez, R., Salas, A., et al. (2018). Two recombinant human monoclonal antibodies that protect against lethal Andes Hantavirus infection in vivo. Sci. Transl. Med. 10:eaat6420. doi: 10.1126/scitranslmed.aat6420
Gauvreau, G. M., Becker, A. B., Boulet, L. P., Chakir, J., Fick, R. B., Greene, W. L., et al. (2003). The effects of an anti-CD11a mAb, efalizumab, on allergen-induced airway responses and airway inflammation in subjects with atopic asthma. J. Allergy Clin. Immunol. 112, 331–338. doi: 10.1067/mai.2003.1689
Gavrilovskaya, I. N., Brown, E. J., Ginsberg, M. H., and Mackow, E. R. (1999). Cellular entry of hantaviruses which cause hemorrhagic fever with renal syndrome is mediated by β3 integrins. J. Virol. 73, 3951–3959. doi: 10.1128/JVI.73.5.3951-3959.1999
Gavrilovskaya, I. N., Peresleni, T., Geimonen, E., and Mackow, E. R. (2002). Pathogenic hantaviruses selectively inhibit β 3 integrin directed endothelial cell migration. Arch. Virol. 147, 1913–1931. doi: 10.1007/s00705-002-0852-0
Gavrilovskaya, I. N., Shepley, M., Shaw, R., Ginsberg, M. H., and Mackow, E. R. (1998). β3 integrins mediate the cellular entry of hantaviruses that cause respiratory failure. Proc. Natl. Acad. Sci. 95, 7074–7079. doi: 10.1073/pnas.95.12.7074
Geimonen, E., Neff, S., Raymond, T., Kocer, S. S., Gavrilovskaya, I. N., and Mackow, E. R. (2002). Pathogenic and nonpathogenic hantaviruses differentially regulate endothelial cell responses. Proc. Natl. Acad. Sci. 99, 13837–13842. doi: 10.1073/pnas.192298899
Geldmacher, A., Skrastina, D., Petrovskis, I., Borisova, G., Berriman, J. A., Roseman, A. M., et al. (2004). An amino-terminal segment of Hantavirus nucleocapsid protein presented on hepatitis B virus core particles induces a strong and highly cross-reactive antibody response in mice. Virology 323, 108–119. doi: 10.1016/j.virol.2004.02.022
Gibbons, D. L., Vaney, M.-C., Roussel, A., Vigouroux, A., Reilly, B., Lepault, J., et al. (2004). Conformational change and protein–protein interactions of the fusion protein of Semliki Forest virus. Nature 427, 320–325. doi: 10.1038/nature02239
Glass, W. G., Rosenberg, H. F., and Murphy, P. M. (2003). Chemokine regulation of inflammation during acute viral infection. Curr. Opin. Allergy Clin. Immunol. 3, 467–473. doi: 10.1097/00130832-200312000-00008
Golias, C., Charalabopoulos, A., Stagikas, D., Charalabopoulos, K., and Batistatou, A. (2007). The kinin system-bradykinin: biological effects and clinical implications. Multiple role of the kinin system-bradykinin. Hippokratia 11, 124–128.
Gorbunova, E., Gavrilovskaya, I. N., and Mackow, E. R. (2010). Pathogenic hantaviruses Andes virus and Hantaan virus induce adherens junction disassembly by directing vascular endothelial cadherin internalization in human endothelial cells. J. Virol. 84, 7405–7411. doi: 10.1128/JVI.00576-10
Gorbunova, E. E., Gavrilovskaya, I. N., Pepini, T., and Mackow, E. R. (2011). VEGFR2 and Src kinase inhibitors suppress Andes virus-induced endothelial cell permeability. J. Virol. 85, 2296–2303. doi: 10.1128/JVI.02319-10
Goswami, B. B., Borek, E., Sharma, O. K., Fujitaki, J., and Smith, R. A. (1979). The broad spectrum antiviral agent ribavirin inhibits capping of mRNA. Biochem. Biophys. Res. Commun. 89, 830–836. doi: 10.1016/0006-291X(79)91853-9
Gowen, B. B., Wong, M.-H., Jung, K.-H., Sanders, A. B., Mendenhall, M., Bailey, K. W., et al. (2007). In vitro and in vivo activities of T-705 against arenavirus and bunyavirus infections. Antimicrob. Agents Chemother. 51, 3168–3176. doi: 10.1128/AAC.00356-07
Graci, J., and Cameron, C. E. (2006). Mechanisms of action of ribavirin against distinct viruses. Rev. Med. Virol. 16, 37–48. doi: 10.1002/rmv.483
Grande, E., Kreissl, M. C., Filetti, S., Newbold, K., Reinisch, W., Robert, C., et al. (2013). Vandetanib in advanced medullary thyroid cancer: review of adverse event management strategies. Adv. Ther. 30, 945–966. doi: 10.1007/s12325-013-0069-5
Guidotti, L. G., and Chisari, F. V. (2001). Noncytolytic control of viral infections by the innate and adaptive immuneresponse. Annu. Rev. Immunol. 19, 65–91. doi: 10.1146/annurev.immunol.19.1.65
Gupta, S., Braun, M., Tischler, N. D., Stoltz, M., Sundström, K. B., Björkström, N. K., et al. (2013). Hantavirus-infection confers resistance to cytotoxic lymphocyte-mediated apoptosis. PLoS Pathog. 9:e1003272. doi: 10.1371/journal.ppat.1003272
Gupta, A. K., Joshi, M. B., Philippova, M., Erne, P., Hasler, P., Hahn, S., et al. (2010). Activated endothelial cells induce neutrophil extracellular traps and are susceptible to NETosis-mediated cell death. FEBS Lett. 584, 3193–3197. doi: 10.1016/j.febslet.2010.06.006
Guterres, A., and de Lemos, E. R. S. (2018). Hantaviruses and a neglected environmental determinant. One Health 5, 27–33. doi: 10.1016/j.onehlt.2017.12.002
Hall, P. R., Hjelle, B., Brown, D. C., Ye, C., Bondu-Hawkins, V., Kilpatrick, K. A., et al. (2008). Multivalent presentation of antihantavirus peptides on nanoparticles enhances infection blockade. Antimicrob. Agents Chemother. 52, 2079–2088. doi: 10.1128/AAC.01415-07
Hall, P. R., Leitão, A., Ye, C., Kilpatrick, K., Hjelle, B., Oprea, T. I., et al. (2010). Small molecule inhibitors of Hantavirus infection. Bioorg. Med. Chem. Lett. 20, 7085–7091. doi: 10.1016/j.bmcl.2010.09.092
Hall, P. R., Malone, L., Sillerud, L. O., Ye, C., Hjelle, B. L., and Larson, R. S. (2007). Characterization and NMR solution structure of a novel cyclic pentapeptide inhibitor of pathogenic hantaviruses. Chem. Biol. Drug Des. 69, 180–190. doi: 10.1111/j.1747-0285.2007.00489.x
Harrison, S. C. (2015). Viral membrane fusion. Virology 479, 498–507. doi: 10.1016/j.virol.2015.03.043
Hautala, N., Partanen, T., Kubin, A.-M., Kauma, H., and Hautala, T. (2021). Central nervous system and ocular manifestations in Puumala Hantavirus infection. Viruses 13:1040. doi: 10.3390/v13061040
Hayasaka, D., Maeda, K., Ennis, F. A., and Terajima, M. (2007). Increased permeability of human endothelial cell line EA. hy926 induced by Hantavirus-specific cytotoxic T lymphocytes. Virus Res. 123, 120–127. doi: 10.1016/j.virusres.2006.08.006
Hepojoki, J., Strandin, T., Lankinen, H., and Vaheri, A. (2012). Hantavirus structure–molecular interactions behind the scene. J. Gen. Virol. 93, 1631–1644. doi: 10.1099/vir.0.042218-0
Heyman, P., Ceianu, C., Christova, I., Tordo, N., Beersma, M., Alves, M. J., et al. (2011). A five-year perspective on the situation of haemorrhagic fever with renal syndrome and status of the Hantavirus reservoirs in Europe, 2005-2010. Eur. Secur. 16:19961. doi: 10.2807/ese.16.36.19961-en
Heyman, P., Plyusnina, A., Berny, P., Cochez, C., Artois, M., Zizi, M., et al. (2004). Seoul Hantavirus in Europe: first demonstration of the virus genome in wild Rattus norvegicus captured in France. Eur. J. Clin. Microbiol. Infect. Dis. 23, 711–717. doi: 10.1007/s10096-004-1196-3
Hooper, J. W., Brocato, R. L., Kwilas, S. A., Hammerbeck, C. D., Josleyn, M. D., Royals, M., et al. (2014). DNA vaccine–derived human IgG produced in transchromosomal bovines protect in lethal models of Hantavirus pulmonary syndrome. Sci. Transl. Med. 6:264ra162. doi: 10.1126/scitranslmed.3010082
Hooper, J. W., Custer, D. M., Smith, J., and Wahl-Jensen, V. (2006). Hantaan/Andes virus DNA vaccine elicits a broadly cross-reactive neutralizing antibody response in nonhuman primates. Virology 347, 208–216. doi: 10.1016/j.virol.2005.11.035
Hooper, J., Custer, D., Thompson, E., and Schmaljohn, C. (2001). DNA vaccination with the Hantaan virus M gene protects hamsters against three of four HFRS hantaviruses and elicits a high-titer neutralizing antibody response in Rhesus monkeys. J. Virol. 75, 8469–8477. doi: 10.1128/JVI.75.18.8469-8477.2001
Hooper, J. W., Josleyn, M., Ballantyne, J., and Brocato, R. (2013). A novel sin Nombre virus DNA vaccine and its inclusion in a candidate pan-Hantavirus vaccine against Hantavirus pulmonary syndrome (HPS) and hemorrhagic fever with renal syndrome (HFRS). Vaccine 31, 4314–4321. doi: 10.1016/j.vaccine.2013.07.025
Hooper, J., Kamrud, K., Elgh, F., Custer, D., and Schmaljohn, C. (1999). DNA vaccination with Hantavirus M segment elicits neutralizing antibodies and protects against Seoul virus infection. Virology 255, 269–278. doi: 10.1006/viro.1998.9586
Huiskonen, J. T., Hepojoki, J., Laurinmaki, P., Vaheri, A., Lankinen, H., Butcher, S. J., et al. (2010). Electron cryotomography of Tula Hantavirus suggests a unique assembly paradigm for enveloped viruses. J. Virol. 84, 4889–4897. doi: 10.1128/JVI.00057-10
Huong, V. T. Q., Yoshimatsu, K., Luan, V. D., Nhi, L., Arikawa, J., and Nguyen, T. M. N. (2010). Hemorrhagic fever with renal syndrome, Vietnam. Emerg. Infect. Dis. 16:363. doi: 10.3201/eid1602.091204
Iglesias, A. A., Períolo, N., Bellomo, C. M., Lewis, L. C., Olivera, C. P., Anselmo, C. R., et al. (2022). Delayed viral clearance despite high number of activated T cells during the acute phase in Argentinean patients with Hantavirus pulmonary syndrome. EBioMedicine 75:103765. doi: 10.1016/j.ebiom.2021.103765
Iheozor-Ejiofor, R., Vapalahti, K., Sironen, T., Levanov, L., Hepojoki, J., Lundkvist, Å., et al. (2022). Neutralizing antibody titers in hospitalized patients with acute Puumala Orthohantavirus infection do not associate with disease severity. Viruses 14:901. doi: 10.3390/v14050901
Jahnsen, F. L., Strickland, D. H., Thomas, J. A., Tobagus, I. T., Napoli, S., Zosky, G. R., et al. (2006). Accelerated antigen sampling and transport by airway mucosal dendritic cells following inhalation of a bacterial stimulus. J. Immunol. 177, 5861–5867. doi: 10.4049/jimmunol.177.9.5861
Jaillon, S., Peri, G., Delneste, Y., Frémaux, I., Doni, A., Moalli, F., et al. (2007). The humoral pattern recognition receptor PTX3 is stored in neutrophil granules and localizes in extracellular traps. J. Exp. Med. 204, 793–804. doi: 10.1084/jem.20061301
Jiang, H., Du, H., Wang, L. M., Wang, P. Z., and Bai, X. F. (2016). Hemorrhagic fever with renal syndrome: pathogenesis and clinical picture. Front. Cell. Infect. Microbiol. 6:1. doi: 10.3389/fcimb.2016.00001
Jiang, D.-B., Sun, L.-J., Cheng, L.-F., Zhang, J.-P., Xiao, S.-B., Sun, Y.-J., et al. (2017). Recombinant DNA vaccine of Hantavirus Gn and LAMP1 induced long-term immune protection in mice. Antivir. Res. 138, 32–39. doi: 10.1016/j.antiviral.2016.12.001
Jiang, H., Zheng, X., Wang, L., Du, H., Wang, P., and Bai, X. (2017). Hantavirus infection: a global zoonotic challenge. Virol. Sin. 32, 32–43. doi: 10.1007/s12250-016-3899-x
Jin, M., Park, J., Lee, S., Park, B., Shin, J., Song, K.-J., et al. (2002). Hantaan virus enters cells by clathrin-dependent receptor-mediated endocytosis. Virology 294, 60–69. doi: 10.1006/viro.2001.1303
Jonsson, C. B., Figueiredo, L. T. M., and Vapalahti, O. (2010). A global perspective on Hantavirus ecology, epidemiology, and disease. Clin. Microbiol. Rev. 23, 412–441. doi: 10.1128/CMR.00062-09
Jonsson, C. B., Milligan, B. G., and Arterburn, J. B. (2005). Potential importance of error catastrophe to the development of antiviral strategies for hantaviruses. Virus Res. 107, 195–205. doi: 10.1016/j.virusres.2004.11.009
Jonsson, C. B., and Schmaljohn, C. S. (2001). Replication of hantaviruses. Curr. Top. Microbiol. Immunol. 256, 15–32. doi: 10.1007/978-3-642-56753-7_2
Ke, G., Hu, Y., Huang, X., Peng, X., Lei, M., Huang, C., et al. (2016). Epidemiological analysis of hemorrhagic fever with renal syndrome in China with the seasonal-trend decomposition method and the exponential smoothing model. Sci. Rep. 6, 1–7. doi: 10.1038/srep39350
Khaiboullina, S. F., Morzunov, S., and St Jeor, S. C. (2005). Hantaviruses: molecular biology, evolution and pathogenesis. Curr. Mol. Med. 5, 773–790. doi: 10.2174/156652405774962317
Khaiboullina, S. F., Netski, D. M., Krumpe, P., and St. Jeor, S. C. (2000). Effects of tumor necrosis factor alpha on sin Nombre virus infection in vitro. J. Virol. 74, 11966–11971. doi: 10.1128/JVI.74.24.11966-11971.2000
Khaiboullina, S. F., and St. Jeor, S. C. (2002). Hantavirus immunology. Viral Immunol. 15, 609–625. doi: 10.1089/088282402320914548
Khan, A., Shin, O. S., Na, J., Kim, J. K., Seong, R.-K., Park, M.-S., et al. (2019). A systems vaccinology approach reveals the mechanisms of immunogenic responses to hantavax vaccination in humans. Sci. Rep. 9:4760. doi: 10.1038/s41598-019-41205-1
Kielian, M. (2006). Class II virus membrane fusion proteins. Virology 344, 38–47. doi: 10.1016/j.virol.2005.09.036
Kielian, M. (2014). Mechanisms of virus membrane fusion proteins. Ann. Rev. Virol. 1, 171–189. doi: 10.1146/annurev-virology-031413-085521
Kielian, M., and Rey, F. A. (2006). Virus membrane-fusion proteins: more than one way to make a hairpin. Nat. Rev. Microbiol. 4, 67–76. doi: 10.1038/nrmicro1326
Klein, D. E., Choi, J. L., and Harrison, S. C. (2013). Structure of a dengue virus envelope protein late-stage fusion intermediate. J. Virol. 87, 2287–2293. doi: 10.1128/JVI.02957-12
Klingström, J., Lindgren, T., and Ahlm, C. (2008). Sex-dependent differences in plasma cytokine responses to Hantavirus infection. Clin. Vaccine Immunol. 15, 885–887. doi: 10.1128/CVI.00035-08
Kobayashi, T., Nakatsuka, K., Shimizu, M., Tamura, H., Shinya, E., Atsukawa, M., et al. (2012). Ribavirin modulates the conversion of human CD 4+ CD 25− T cell to CD 4+ CD 25+ FOXP 3+ T cell via suppressing interleukin-10-producing regulatory T cell. Immunology 137, 259–270. doi: 10.1111/imm.12005
Kraus, A. A., Raftery, M. J., Giese, T., Ulrich, R., Zawatzky, R., Hippenstiel, S., et al. (2004). Differential antiviral response of endothelial cells after infection with pathogenic and nonpathogenic hantaviruses. J. Virol. 78, 6143–6150. doi: 10.1128/JVI.78.12.6143-6150.2004
Kruger, D. H., Figueiredo, L. T. M., Song, J.-W., and Klempa, B. (2015). Hantaviruses—globally emerging pathogens. J. Clin. Virol. 64, 128–136. doi: 10.1016/j.jcv.2014.08.033
Krüger, D. H., Schönrich, G., and Klempa, B. (2011). Human pathogenic hantaviruses and prevention of infection. Hum. Vaccin. 7, 685–693. doi: 10.4161/hv.7.6.15197
Krüger, D. H., Ulrich, R. G., and Hofmann, J. (2013). Hantaviruses as zoonotic pathogens in Germany. Dtsch. Arztebl. Int. 110:461. doi: 10.3238/arztebl.2013.0461
Kuhn, J. H., and Schmaljohn, C. S. (2023). A brief history of bunyaviral family Hantaviridae. Diseases 11:38. doi: 10.3390/diseases11010038
Kyriakidis, I., and Papa, A. (2013). Serum TNF-α, sTNFR1, IL-6, IL-8 and IL-10 levels in hemorrhagic fever with renal syndrome. Virus Res. 175, 91–94. doi: 10.1016/j.virusres.2013.03.020
Laine, O., Leppänen, I., Koskela, S., Antonen, J., Mäkelä, S., Sinisalo, M., et al. (2015). Severe Puumala virus infection in a patient with a lymphoproliferative disease treated with icatibant. Infect. Dis. 47, 107–111. doi: 10.3109/00365548.2014.969304
Larson, R. S., Brown, D. C., Ye, C., and Hjelle, B. (2005). Peptide antagonists that inhibit sin Nombre virus and hantaan virus entry through the beta3-integrin receptor. J. Virol. 79, 7319–7326. doi: 10.1128/JVI.79.12.7319-7326.2005
Lee, S.-H., Chung, B.-H., Lee, W.-C., and Choi, I.-S. (2013). Epidemiology of hemorrhagic fever with renal syndrome in Korea, 2001–2010. J. Korean Med. Sci. 28, 1552–1554. doi: 10.3346/jkms.2013.28.10.1552
Li, Z., Kong, D., Liu, Y., and Li, M. (2022a). Pharmacological perspectives and molecular mechanisms of coumarin derivatives against virus disease. Genes Dis. 9, 80–94. doi: 10.1016/j.gendis.2021.03.007
Li, Z., Wang, F., Liu, Y., Zhai, D., Zhang, X., Ying, Q., et al. (2021). Coumarin derivative N6 as a novel anti-Hantavirus infection agent targeting Akt. Front. Pharmacol. 12:745646. doi: 10.3389/fphar.2021.745646
Li, Z., Wang, F., Ying, Q., Kong, D., Zhang, X., Dong, Y., et al. (2022b). In vitro anti-Hantavirus activity of protein kinase inhibitor 8G1 targeting AKT/mTOR/eIF4E signaling pathway. Front. Microbiol. 13:880258. doi: 10.3389/fmicb.2022.1086239
Liang, M., Chu, Y.-K., and Schmaljohn, C. (1996). Bacterial expression of neutralizing mouse monoclonal antibody fab fragments to Hantaan virus. Virology 217, 262–271. doi: 10.1006/viro.1996.0113
Libraty, D. H., Mäkelä, S., Vlk, J., Hurme, M., Vaheri, A., Ennis, F. A., et al. (2012). The degree of leukocytosis and urine GATA-3 mRNA levels are risk factors for severe acute kidney injury in Puumala virus nephropathia epidemica. PLoS One 7:e35402. doi: 10.1371/journal.pone.0035402
Linderholm, M., Ahlm, C., Settergren, B., Waage, A., and Tärnvik, A. (1996). Elevated plasma levels of tumor necrosis factor (TNF)-α, soluble TNF receptors, interleukin (IL)-6, and IL-IO in patients with hemorrhagic fever with renal syndrome. J. Infect. Dis. 173, 38–43. doi: 10.1093/infdis/173.1.38
Liu, Y.-y., Chen, L.-j., Zhong, Y., Shen, M.-x., Ma, N., Liu, B.-y., et al. (2016). Specific interference shRNA-expressing plasmids inhibit Hantaan virus infection in vitro and in vivo. Acta Pharmacol. Sin. 37, 497–504. doi: 10.1038/aps.2015.165
Liu, R., Ma, H., Shu, J., Zhang, Q., Han, M., Liu, Z., et al. (2020). Vaccines and therapeutics against hantaviruses. Front. Microbiol. 10:2989. doi: 10.3389/fmicb.2019.02989
Llah, S. T., Mir, S., Sharif, S., Khan, S., and Mir, M. A. (2018). Hantavirus induced cardiopulmonary syndrome: a public health concern. J. Med. Virol. 90, 1003–1009. doi: 10.1002/jmv.25054
Löber, C., Anheier, B., Lindow, S., Klenk, H.-D., and Feldmann, H. (2001). The Hantaan virus glycoprotein precursor is cleaved at the conserved pentapeptide WAASA. Virology 289, 224–229. doi: 10.1006/viro.2001.1171
Lokugamage, N., Kariwa, H., Lokugamage, K., Iwasa, M. A., Hagiya, T., Yoshii, K., et al. (2004). Epizootiological and epidemiological study of Hantavirus infection in Japan. Microbiol. Immunol. 48, 843–851. doi: 10.1111/j.1348-0421.2004.tb03616.x
Lusvarghi, S., and Bewley, C. A. (2016). Griffithsin: an antiviral lectin with outstanding therapeutic potential. Viruses 8:296. doi: 10.3390/v8100296
Mackow, E. R., and Gavrilovskaya, I. N. (2001). Cellular receptors and Hantavirus pathogenesis. Curr. Top. Microbiol. Immunol. 256, 91–115. doi: 10.1007/978-3-642-56753-7_6
Mackow, E. R., and Gavrilovskaya, I. N. (2009). Hantavirus regulation of endothelial cell functions. Thromb. Haemost. 102, 1030–1041. doi: 10.1160/TH09-09-0640
MacRae, I. J., Zhou, K., Li, F., Repic, A., Brooks, A. N., Cande, W. Z., et al. (2006). Structural basis for double-stranded RNA processing by dicer. Science 311, 195–198. doi: 10.1126/science.1121638
Maes, P., Clement, J., Cauwe, B., Bonnet, V., Keyaerts, E., Robert, A., et al. (2008). Truncated recombinant puumala virus nucleocapsid proteins protect mice against challenge in vivo. Viral Immunol. 21, 49–60. doi: 10.1089/vim.2007.0059
Maes, P., Clement, J., Gavrilovskaya, I., and Van Ranst, M. (2004). Hantaviruses: immunology, treatment, and prevention. Viral Immunol. 17, 481–497. doi: 10.1089/vim.2004.17.481
Maes, P., Keyaerts, E., Bonnet, V., Clement, J., Avsic-Zupanc, T., Robert, A., et al. (2006). Truncated recombinant Dobrava Hantavirus nucleocapsid proteins induce strong, long-lasting immune responses in mice. Intervirology 49, 253–260. doi: 10.1159/000093454
Malinin, O. V., and Platonov, A. E. (2017). Insufficient efficacy and safety of intravenous ribavirin in treatment of haemorrhagic fever with renal syndrome caused by Puumala virus. Infect. Dis. 49, 514–520. doi: 10.1080/23744235.2017.1293841
Malley, R., DeVincenzo, J., Ramilo, O., Dennehy, P. H., Meissner, H. C., Gruber, W. C., et al. (1998). Reduction of respiratory syncytial virus (RSV) in tracheal aspirates in intubated infants by use of humanized monoclonal antibody to RSV F protein. J. Infect. Dis. 178, 1555–1561. doi: 10.1086/314523
Manigold, T., and Vial, P. (2014). Human Hantavirus infections: epidemiology, clinical features, pathogenesis and immunology. Swiss Med. Wkly. 144, w13937–w. doi: 10.4414/smw.2014.13937
Markotic, A., Gagro, A., Dasic, G., Kuzman, I., Lukas, D., Nichol, S., et al. (2002). Immune parameters in hemorrhagic fever with renal syndrome during the incubation and acute disease: case report. Croat. Med. J. 43, 587–590.
Markotić, A., Hensley, L., Daddario, K., Spik, K., Anderson, K., and Schmaljohn, C. (2007). Pathogenic hantaviruses elicit different immunoreactions in THP-1 cells and primary monocytes and induce differentiation of human monocytes to dendritic-like cells. Coll. Antropol. 31, 1159–1167.
Marsac, D., García, S., Fournet, A., Aguirre, A., Pino, K., Ferres, M., et al. (2011). Infection of human monocyte-derived dendritic cells by ANDES Hantavirus enhances pro-inflammatory state, the secretion of active MMP-9 and indirectly enhances endothelial permeability. Virol. J. 8, 1–9. doi: 10.1186/1743-422X-8-223
Martinez-Valdebenito, C., Calvo, M., Vial, C., Mansilla, R., Marco, C., Palma, R. E., et al. (2014). Person-to-person household and nosocomial transmission of Andes Hantavirus, southern Chile, 2011. Emerg. Infect. Dis. 20:1629. doi: 10.3201/eid2010.140353
Maruyama, T., Rodriguez, L. L., Jahrling, P. B., Sanchez, A., Khan, A. S., Nichol, S. T., et al. (1999). Ebola virus can be effectively neutralized by antibody produced in natural human infection. J. Virol. 73, 6024–6030. doi: 10.1128/JVI.73.7.6024-6030.1999
Masson, P., Heremans, J., and Schonne, E. (1969). Lactoferrin, an iron-binbing protein Ni neutrophilic leukocytes. J. Exp. Med. 130, 643–658. doi: 10.1084/jem.130.3.643
Maurer, M., Bader, M., Bas, M., Bossi, F., Cicardi, M., Cugno, M., et al. (2011). New topics in bradykinin research. Allergy 66, 1397–1406. doi: 10.1111/j.1398-9995.2011.02686.x
Mayor, J., Torriani, G., Engler, O., and Rothenberger, S. (2021). Identification of novel antiviral compounds targeting entry of hantaviruses. Viruses 13:685. doi: 10.3390/v13040685
McClain, D. J., Summers, P. L., Harrison, S. A., Schmaljohn, A. L., and Schmaljohn, C. S. (2000). Clinical evaluation of a vaccinia-vectored Hantaan virus vaccine. J. Med. Virol. 60, 77–85. doi: 10.1002/(SICI)1096-9071(200001)60:1<77::AID-JMV13>3.0.CO;2-S
Medina, R. A., Mirowsky-Garcia, K., Hutt, J., and Hjelle, B. (2007). Ribavirin, human convalescent plasma and anti-β 3 integrin antibody inhibit infection by sin Nombre virus in the deer mouse model. J. Gen. Virol. 88, 493–505. doi: 10.1099/vir.0.82459-0
Meisel, H., Wolbert, A., Razanskiene, A., Marg, A., Kazaks, A., Sasnauskas, K., et al. (2006). Development of novel immunoglobulin G (IgG), IgA, and IgM enzyme immunoassays based on recombinant Puumala and Dobrava Hantavirus nucleocapsid proteins. Clin. Vaccine Immunol. 13, 1349–1357. doi: 10.1128/CVI.00208-06
Mills, J. N., Ksiazek, T. G., Peters, C., and Childs, J. E. (1999). Long-term studies of Hantavirus reservoir populations in the southwestern United States: a synthesis. Emerg. Infect. Dis. 5:135. doi: 10.3201/eid0501.990116
Mir, M. A., and Panganiban, A. T. (2010). The triplet repeats of the sin Nombre Hantavirus 5′ untranslated region are sufficient in cis for nucleocapsid-mediated translation initiation. J. Virol. 84, 8937–8944. doi: 10.1128/JVI.02720-09
Mittler, E., Serris, A., Esterman, E. S., Florez, C., Polanco, L. C., O’Brien, C. M., et al. (2023). Structural and mechanistic basis of neutralization by a pan-Hantavirus protective antibody. Sci. Transl. Med. 15:eadg1855. doi: 10.1126/scitranslmed.adg1855
Modis, Y. (2014). Relating structure to evolution in class II viral membrane fusion proteins. Curr. Opin. Virol. 5, 34–41. doi: 10.1016/j.coviro.2014.01.009
Mohsen, M. O., and Bachmann, M. F. (2022). Virus-like particle vaccinology, from bench to bedside. Cell. Mol. Immunol. 19, 993–1011. doi: 10.1038/s41423-022-00897-8
Mohsen, M. O., Zha, L., Cabral-Miranda, G., and Bachmann, M. F.. Major findings and recent advances in virus–like particle (VLP)-based vaccines. Seminars in immunology. (2017). Amsterdam: Elsevier.
Monsinjon, T., Gasque, P., Chan, P., Ischenko, A., Brady, J. J., and Fontaine, M. (2003). Regulation by complement C3a and C5a anaphylatoxins of cytokine production in human umbilical vein endothelial cells. FASEB J. 17, 1003–1014. doi: 10.1096/fj.02-0737com
Munir, N., Jahangeer, M., Hussain, S., Mahmood, Z., Ashiq, M., Ehsan, F., et al. (2021). Hantavirus diseases pathophysiology, their diagnostic strategies and therapeutic approaches: a review. Clin. Exp. Pharmacol. Physiol. 48, 20–34. doi: 10.1111/1440-1681.13403
Murphy, M. E., Kariwa, H., Mizutani, T., Tanabe, H., Yoshimatsu, K., Arikawa, J., et al. (2001). Characterization of in vitro and in vivo antiviral activity of lactoferrin and ribavirin upon Hantavirus. J. Vet. Med. Sci. 63, 637–645. doi: 10.1292/jvms.63.637
Murphy, M., Kariwa, H., Mizutani, T., Yoshimatsu, K., Arikawa, J., and Takashima, I. (2000). In vitro antiviral activity of lactoferrin and ribavirin upon Hantavirus. Arch. Virol. 145, 1571–1582. doi: 10.1007/s007050070077
Naeem, M. A., Adeel, M. M., Kanwal, A., Ahmad, S., Ahmad, W., Akram, Q., et al. (2021). Reconnoitering Mycobacterium tuberculosis lipoproteins to design subunit vaccine by immunoinformatics approach. Adv. Life Sci. 8, 300–306.
Navarrete, M., Barrera, C., Zaror, L., and Otth, C. (2007). Rapid immunochromatographic test for Hantavirus Andes contrasted with capture-IgM ELISA for detection of Andes-specific IgM antibodies. J. Med. Virol. 79, 41–44. doi: 10.1002/jmv.20759
Niikura, M., Maeda, A., Ikegami, T., Saijo, M., Kurane, I., and Morikawa, S. (2004). Modification of endothelial cell functions by Hantaan virus infection: prolonged hyper-permeability induced by TNF-alpha of hantaan virus-infected endothelial cell monolayers. Arch. Virol. 149, 1279–1292. doi: 10.1007/s00705-004-0306-y
Ogg, M., Jonsson, C. B., Camp, J. V., and Hooper, J. W. (2013). Ribavirin protects Syrian hamsters against lethal Hantavirus pulmonary syndrome—after intranasal exposure to Andes virus. Viruses 5, 2704–2720. doi: 10.3390/v5112704
O'Keefe, B. R., Giomarelli, B., Barnard, D. L., Shenoy, S. R., Chan, P. K., McMahon, J. B., et al. (2010). Broad-spectrum in vitro activity and in vivo efficacy of the antiviral protein griffithsin against emerging viruses of the family Coronaviridae. J. Virol. 84, 2511–2521. doi: 10.1128/JVI.02322-09
Onishchenko, G., and Ezhlova, E. (2013). Epidemiologic surveillance and prophylaxis of hemorrhagic fever with renal syndrome in Russian Federation. Zh. Mikrobiol. Epidemiol. Immunobiol. 4, 23–32.
Outinen, T., Mäkelä, S., Huhtala, H., Hurme, M., Meri, S., Pörsti, I., et al. (2012). High pentraxin-3 plasma levels associate with thrombocytopenia in acute Puumala Hantavirus-induced nephropathia epidemica. Eur. J. Clin. Microbiol. Infect. Dis. 31, 957–963. doi: 10.1007/s10096-011-1392-x
Perley, C. C., Brocato, R. L., Wu, H., Bausch, C., Karmali, P. P., Vega, J. B., et al. (2020). Anti-HFRS human IgG produced in transchromosomic bovines has potent Hantavirus neutralizing activity and is protective in animal models. Front. Microbiol. 11:832. doi: 10.3389/fmicb.2020.00832
Porter, K. R., Ewing, D., Chen, L., Wu, S.-J., Hayes, C. G., Ferrari, M., et al. (2012). Immunogenicity and protective efficacy of a vaxfectin-adjuvanted tetravalent dengue DNA vaccine. Vaccine 30, 336–341. doi: 10.1016/j.vaccine.2011.10.085
Prescott, J., DeBuysscher, B. L., Brown, K. S., and Feldmann, H. (2014). Long-term single-dose efficacy of a vesicular stomatitis virus-based Andes virus vaccine in Syrian hamsters. Viruses 6, 516–523. doi: 10.3390/v6020516
Priya, A. J., and Priya, V. V. (2020). Drug therapy of Hantavirus-a review. PalArch's J. Archaeol. Egypt 17, 345–354.
Raftery, M. J., Kraus, A. A., Ulrich, R., Krüger, D. H., and Schönrich, G. N. (2002). Hantavirus infection of dendritic cells. J. Virol. 76, 10724–10733. doi: 10.1128/JVI.76.21.10724-10733.2002
Ramanathan, H. N., Chung, D.-H., Plane, S. J., Sztul, E., Chu, Y.-k., Guttieri, M. C., et al. (2007). Dynein-dependent transport of the hantaan virus nucleocapsid protein to the endoplasmic reticulum-Golgi intermediate compartment. J. Virol. 81, 8634–8647. doi: 10.1128/JVI.00418-07
Ramanathan, H. N., and Jonsson, C. B. (2008). New and Old World hantaviruses differentially utilize host cytoskeletal components during their life cycles. Virology 374, 138–150. doi: 10.1016/j.virol.2007.12.030
Raymond, T., Gorbunova, E., Gavrilovskaya, I. N., and Mackow, E. R. (2005). Pathogenic hantaviruses bind plexin-semaphorin-integrin domains present at the apex of inactive, bent alphavbeta3 integrin conformers. Proc. Natl. Acad. Sci. U. S. A. 102, 1163–1168. Epub 20050118. doi: 10.1073/pnas.0406743102
Razvina, O., Jiang, S., Matsubara, K., Ohashi, R., Hasegawa, G., Aoyama, T., et al. (2015). Differential expression of pentraxin 3 in neutrophils. Exp. Mol. Pathol. 98, 33–40. doi: 10.1016/j.yexmp.2014.11.009
Reguera, J., Weber, F., and Cusack, S. (2010). Bunyaviridae RNA polymerases (L-protein) have an N-terminal, influenza-like endonuclease domain, essential for viral cap-dependent transcription. PLoS Pathog. 6:e1001101. doi: 10.1371/journal.ppat.1001101
Rey, F. A., Heinz, F. X., Mandl, C., Kunz, C., and Harrison, S. C. (1995). The envelope glycoprotein from tick-borne encephalitis virus at 2 Å resolution. Nature 375, 291–298. doi: 10.1038/375291a0
Ricci, F., Izzicupo, P., Moscucci, F., Sciomer, S., Maffei, S., Di Baldassarre, A., et al. (2020). Recommendations for physical inactivity and sedentary behavior during the coronavirus disease (COVID-19) pandemic. Front. Public Health 8:199. doi: 10.3389/fpubh.2020.00199
Roman-Sosa, G., and Kielian, M. (2011). The interaction of alphavirus E1 protein with exogenous domain III defines stages in virus-membrane fusion. J. Virol. 85, 12271–12279. doi: 10.1128/JVI.05902-11
Saavedra, F., Diaz, F. E., Retamal-Díaz, A., Covian, C., González, P. A., and Kalergis, A. M. (2021). Immune response during Hantavirus diseases: implications for immunotherapies and vaccine design. Immunology 163, 262–277. doi: 10.1111/imm.13322
Sadeghi, M., Eckerle, I., Daniel, V., Burkhardt, U., Opelz, G., and Schnitzler, P. (2011). Cytokine expression during early and late phase of acute Puumala Hantavirus infection. BMC Immunol. 12, 1–10. doi: 10.1186/1471-2172-12-65
Saffarzadeh, M., Juenemann, C., Queisser, M. A., Lochnit, G., Barreto, G., Galuska, S. P., et al. (2012). Neutrophil extracellular traps directly induce epithelial and endothelial cell death: a predominant role of histones. PLoS One 7:e32366. doi: 10.1371/journal.pone.0032366
Safronetz, D., Falzarano, D., Scott, D. P., Furuta, Y., Feldmann, H., and Gowen, B. B. (2013). Antiviral efficacy of favipiravir against two prominent etiological agents of Hantavirus pulmonary syndrome. Antimicrob. Agents Chemother. 57, 4673–4680. doi: 10.1128/AAC.00886-13
Safronetz, D., Hegde, N. R., Ebihara, H., Denton, M., Kobinger, G. P., St. Jeor, S., et al. (2009). Adenovirus vectors expressing Hantavirus proteins protect hamsters against lethal challenge with Andes virus. J. Virol. 83, 7285–7295. doi: 10.1128/JVI.00373-09
Sargianou, M., Watson, D. C., Chra, P., Papa, A., Starakis, I., Gogos, C., et al. (2012). Hantavirus infections for the clinician: from case presentation to diagnosis and treatment. Crit. Rev. Microbiol. 38, 317–329. doi: 10.3109/1040841X.2012.673553
Schmaljohn, C. S., Chu, Y.-K., Schmaljohn, A. L., and Dalrymple, J. M. (1990). Antigenic subunits of Hantaan virus expressed by baculovirus and vaccinia virus recombinants. J. Virol. 64, 3162–3170. doi: 10.1128/jvi.64.7.3162-3170.1990
Schmaljohn, C. S., Spik, K. W., and Hooper, J. W. (2014). DNA vaccines for HFRS: laboratory and clinical studies. Virus Res. 187, 91–96. doi: 10.1016/j.virusres.2013.12.020
Schönrich, G., Krüger, D. H., and Raftery, M. J. (2015). Hantavirus-induced disruption of the endothelial barrier: neutrophils are on the payroll. Front. Microbiol. 6:222. doi: 10.3389/fmicb.2015.00222
Schönrich, G., Rang, A., Lütteke, N., Raftery, M. J., Charbonnel, N., and Ulrich, R. G. (2008). Hantavirus-induced immunity in rodent reservoirs and humans. Immunol. Rev. 225, 163–189. doi: 10.1111/j.1600-065X.2008.00694.x
Schubert, J., Tollmann, F., and Weissbrich, B. (2001). Evaluation of a pan-reactive Hantavirus enzyme immunoassay and of a Hantavirus immunoblot for the diagnosis of nephropathia epidemica. J. Clin. Virol. 21, 63–74. doi: 10.1016/S1386-6532(00)00187-6
Severson, W. E., Schmaljohn, C. S., Javadian, A., and Jonsson, C. B. (2003). Ribavirin causes error catastrophe during Hantaan virus replication. J. Virol. 77, 481–488. doi: 10.1128/JVI.77.1.481-488.2003
Shah, V., Firmal, P., Alam, A., Ganguly, D., and Chattopadhyay, S. (2020). Overview of immune response during SARS-CoV-2 infection: lessons from the past. Front. Immunol. 11:1949. doi: 10.3389/fimmu.2020.01949
Shin, O. S., Yanagihara, R., and Song, J.-W. (2012). Distinct innate immune responses in human macrophages and endothelial cells infected with shrew-borne hantaviruses. Virology 434, 43–49. doi: 10.1016/j.virol.2012.08.004
Shrivastava-Ranjan, P., Lo, M. K., Chatterjee, P., Flint, M., Nichol, S. T., Montgomery, J. M., et al. (2020). Hantavirus infection is inhibited by griffithsin in cell culture. Front. Cell. Infect. Microbiol. 10:561502. doi: 10.3389/fcimb.2020.561502
Singh, S., Numan, A., Sharma, D., Shukla, R., Alexander, A., Jain, G. K., et al. (2022). Epidemiology, virology and clinical aspects of Hantavirus infections: an overview. Int. J. Environ. Health Res. 32, 1815–1826. doi: 10.1080/09603123.2021.1917527
Spiropoulou, C. (2001). Hantavirus maturation. Hantaviruses, 256, 33–46. doi: 10.1007/978-3-642-56753-7_3
Sroga, P., Sloan, A., Warner, B. M., Tierney, K., Lew, J., Liu, G., et al. (2021). Polyclonal alpaca antibodies protect against Hantavirus pulmonary syndrome in a lethal Syrian hamster model. Sci. Rep. 11:17440. doi: 10.1038/s41598-021-96884-6
Streeter, D. G., Witkowski, J., Khare, G. P., Sidwell, R. W., Bauer, R. J., Robins, R. K., et al. (1973). Mechanism of action of 1-β-D-ribofuranosyl-1, 2, 4-triazole-3-carboxamide (Virazole), a new broad-spectrum antiviral agent. Proc. Natl. Acad. Sci. 70, 1174–1178. doi: 10.1073/pnas.70.4.1174
Sundstrom, J. B., McMullan, L. K., Spiropoulou, C. F., Hooper, W. C., Ansari, A. A., Peters, C. J., et al. (2001). Hantavirus infection induces the expression of RANTES and IP-10 without causing increased permeability in human lung microvascular endothelial cells. J. Virol. 75, 6070–6085. doi: 10.1128/JVI.75.13.6070-6085.2001
Sundström, K. B., Nguyen Hoang, A. T., Gupta, S., Ahlm, C., Svensson, M., and Klingström, J. (2016). Andes Hantavirus-infection of a 3D human lung tissue model reveals a late peak in progeny virus production followed by increased levels of proinflammatory cytokines and VEGF-A. PLoS One 11:e0149354. doi: 10.1371/journal.pone.0149354
Suputthamongkol, Y., Nitatpattana, N., Chayakulkeeree, M., and Palabodeewat, S. (2005). Hantavirus infection in Thailand: first clinical case report. Southeast Asian J. Trop. Med. Public Health 36, 700–703.
Szabo, R. (2017). Antiviral therapy and prevention against Hantavirus infections. Acta Virol. 61, 3–12. doi: 10.4149/av_2017_01_3
Takagi, J., and Springer, T. A. (2002). Integrin activation and structural rearrangement. Immunol. Rev. 186, 141–163. doi: 10.1034/j.1600-065X.2002.18613.x
Taylor, S. L., Wahl-Jensen, V., Copeland, A. M., Jahrling, P. B., and Schmaljohn, C. S. (2013). Endothelial cell permeability during Hantavirus infection involves factor XII-dependent increased activation of the kallikrein-kinin system. PLoS Pathog. 9:e1003470. doi: 10.1371/journal.ppat.1003470
Temonen, M., Mustonen, J., Helin, H., Pasternack, A., Vaheri, A., and Holthöfer, H. (1996). Cytokines, adhesion molecules, and cellular infiltration in nephropathia epidemica kidneys: an immunohistochemical study. Clin. Immunol. Immunopathol. 78, 47–55. doi: 10.1006/clin.1996.0007
Tian, Z., Liang, G., Cui, K., Liang, Y., Wang, Q., Lv, S., et al. (2021). Insight into the prospects for RNAi therapy of cancer. Front. Pharmacol. 12:644718. doi: 10.3389/fphar.2021.644718
Tian, H., and Stenseth, N. C. (2019). The ecological dynamics of Hantavirus diseases: from environmental variability to disease prevention largely based on data from China. PLoS Negl. Trop. Dis. 13:e0006901. doi: 10.1371/journal.pntd.0006901
Tischler, N. D., Gonzalez, A., Perez-Acle, T., Rosemblatt, M., and Valenzuela, P. D. T. (2005). Hantavirus Gc glycoprotein: evidence for a class II fusion protein. J. Gen. Virol. 86, 2937–2947. doi: 10.1099/vir.0.81083-0
Toltzis, P., and Huang, A. (1986). Effect of ribavirin on macromolecular synthesis in vesicular stomatitis virus-infected cells. Antimicrob. Agents Chemother. 29, 1010–1016. doi: 10.1128/AAC.29.6.1010
Tracey, K. J., and Cerami, A. (1994). Tumor necrosis factor: a pleiotropic cytokine and therapuetic target. Annu. Rev. Med. 45, 491–503. doi: 10.1146/annurev.med.45.1.491
Vaheri, A., Henttonen, H., Voutilainen, L., Mustonen, J., Sironen, T., and Vapalahti, O. (2013). Hantavirus infections in Europe and their impact on public health. Rev. Med. Virol. 23, 35–49. doi: 10.1002/rmv.1722
Vial, P. A., Valdivieso, F., Calvo, M., Rioseco, M. L., Riquelme, R., Araneda, A., et al. (2015). A non-randomized multicentre trial of human immune plasma for treatment of Hantavirus cardiopulmonary syndrome caused by Andes virus. Antivir. Ther. 20, 377–386. doi: 10.3851/IMP2875
Vial, P., Valdivieso, F., Ferres, M., Riquelme, R., Rioseco, M., Calvo, M., et al. (2013). Hantavirus study Group in Chile High-dose intravenous methylprednisolone for Hantavirus cardiopulmonary syndrome in Chile: a double-blind, randomized controlled clinical trial. Clin. Infect. Dis. 57, 943–951. doi: 10.1093/cid/cit394
Vilcek, J. (1991). Tumor necrosis factor-new insights into the molecular mechanisms of its multiple actions. J. Biol. Chem. 266:7313. doi: 10.1016/S0021-9258(20)89445-9
Vitarana, T., Colombage, G., Bandaranayake, V., and Lee, H. (1988). Hantavirus disease in Sri Lanka. Lancet (London, England) 2:1263.
Wang, W., Zhang, Y., Li, Y., Pan, L., Bai, L., Zhuang, Y., et al. (2012). Dysregulation of the β3 integrin-VEGFR2 complex in Hantaan virus–directed hyperpermeability upon treatment with VEGF. Arch. Virol. 157, 1051–1061. doi: 10.1007/s00705-012-1245-7
Warner, B. M., Dowhanik, S., Audet, J., Grolla, A., Dick, D., Strong, J. E., et al. (2020). Hantavirus cardiopulmonary syndrome in Canada. Emerg. Infect. Dis. 26:3020. doi: 10.3201/eid2612.202808
Warner, B. M., Stein, D. R., Jangra, R. K., Slough, M. M., Sroga, P., Sloan, A., et al. (2019). Vesicular stomatitis virus-based vaccines provide cross-protection against Andes and sin nombre viruses. Viruses 11:645. doi: 10.3390/v11070645
Watson, D. C., Sargianou, M., Papa, A., Chra, P., Starakis, I., and Panos, G. (2014). Epidemiology of Hantavirus infections in humans: a comprehensive, global overview. Crit. Rev. Microbiol. 40, 261–272. doi: 10.3109/1040841X.2013.783555
Wei, J., Huang, X., Li, S., Du, S., Yu, P., and Li, J. (2021). A Total of 2,657 reported cases and 14 deaths due to hemorrhagic fever with renal syndrome—Shaanxi Province, China, January 1–December 19, 2021. China CDC Weekly 3:1143. doi: 10.46234/ccdcw2021.272
Weinberg, M. S., and Arbuthnot, P. (2010). Progress in the use of RNA interference as a therapy for chronic hepatitis B virus infection. Genome Med. 2, 1–7.
Wimer, B. M. (1998). Implications of the analogy between recombinant cytokine toxicities and manifestations of Hantavirus infections. Cancer Biother. Radiopharm. 13, 193–207. doi: 10.1089/cbr.1998.13.193
Wong, T., Chan, Y., and Lee, H. (1985). Haemorrhagic fever with renal syndrome in Singapore: a case report. Southeast Asian J. Trop. Med. Public Health 16, 525–527.
Wray, S. K., Gilbert, B. E., Noall, M. W., and Knight, V. (1985). Mode of action of ribavirin: effect of nucleotide pool alterations on influenza virus ribonucleoprotein synthesis. Antivir. Res. 5, 29–37. doi: 10.1016/0166-3542(85)90012-9
Xu, Z., Chen, Q., Zhang, Y., and Liang, C. (2021). Coumarin-based derivatives with potential anti-HIV activity. Fitoterapia 150:104863. doi: 10.1016/j.fitote.2021.104863
Xu, Z., Wei, L., Wang, L., Wang, H., and Jiang, S. (2002). The in vitro and in vivo protective activity of monoclonal antibodies directed against Hantaan virus: potential application for immunotherapy and passive immunization. Biochem. Biophys. Res. Commun. 298, 552–558. doi: 10.1016/S0006-291X(02)02491-9
Xu, R., Yang, X. Y., Yang, D. F., Zou, C. Y., Gong, P. L., and Zeng, F. D. (2009). Phase I evaluation of the safety and pharmacokinetics of a single-dose intravenous injection of a murine monoclonal antibody against Hantaan virus in healthy volunteers. Antimicrob. Agents Chemother. 53, 5055–5059. doi: 10.1128/AAC.00728-09
Yanagihara, R., and Silverman, D. J. (1990). Experimental infection of human vascular endothelial cells by pathogenic and nonpathogenic hantaviruses. Arch. Virol. 111, 281–286. doi: 10.1007/BF01311063
Yang, J., Sun, J.-F., Wang, T.-T., Guo, X.-H., Wei, J.-X., Jia, L.-T., et al. (2017). Targeted inhibition of Hantavirus replication and intracranial pathogenesis by a chimeric protein-delivered siRNA. Antivir. Res. 147, 107–115. doi: 10.1016/j.antiviral.2017.10.005
Ye, C., Wang, D., Liu, H., Ma, H., Dong, Y., Yao, M., et al. (2019). An improved enzyme-linked focus formation assay revealed baloxavir acid as a potential antiviral therapeutic against Hantavirus infection. Front. Pharmacol. 10:1203. doi: 10.3389/fphar.2019.01203
Ying, Q., Ma, T., Cheng, L., Zhang, X., Truax, A. D., Ma, R., et al. (2016). Construction and immunological characterization of CD40L or GM-CSF incorporated Hantaan virus like particle. Oncotarget 7:63488. doi: 10.18632/oncotarget.11329
Yu, P., Tian, H., Ma, C., Ma, C., Wei, J., Lu, X., et al. (2015). Hantavirus infection in rodents and haemorrhagic fever with renal syndrome in Shaanxi province, China, 1984–2012. Epidemiol. Infect. 143, 405–411. doi: 10.1017/S0950268814001009
Zhang, S., Wang, S., Yin, W., Liang, M., Li, J., Zhang, Q., et al. (2014). Epidemic characteristics of hemorrhagic fever with renal syndrome in China, 2006–2012. BMC Infect. Dis. 14, 1–10. doi: 10.1186/1471-2334-14-384
Keywords: Hantavirus, HFRS, HPS, immunotherapy, siRNA
Citation: Afzal S, Ali L, Batool A, Afzal M, Kanwal N, Hassan M, Safdar M, Ahmad A and Yang J (2023) Hantavirus: an overview and advancements in therapeutic approaches for infection. Front. Microbiol. 14:1233433. doi: 10.3389/fmicb.2023.1233433
Edited by:
Gaëtan Ligat, Université Toulouse III Paul Sabatier, FranceReviewed by:
Manoj Baranwal, Thapar Institute of Engineering and Technology, IndiaWei Ye, Air Force Medical University, China
Copyright © 2023 Afzal, Ali, Batool, Afzal, Kanwal, Hassan, Safdar, Ahmad and Yang. This is an open-access article distributed under the terms of the Creative Commons Attribution License (CC BY). The use, distribution or reproduction in other forums is permitted, provided the original author(s) and the copyright owner(s) are credited and that the original publication in this journal is cited, in accordance with accepted academic practice. No use, distribution or reproduction is permitted which does not comply with these terms.
*Correspondence: Liaqat Ali, bGlhcWF0YmlvdGVjaEBnbWFpbC5jb20=; Jing Yang, V0lCUEpZYW5nQDEyNi5jb20=