A comparative analysis of Danionella cerebrum and zebrafish (Danio rerio) larval locomotor activity in a light-dark test
- Division of Cellular and Molecular Neurobiology, Zoological Institute, Technische Universität Braunschweig, Braunschweig, Germany
The genus Danionella comprises some of the smallest known vertebrate species and is evolutionary closely related to the zebrafish, Danio rerio. With its optical translucency, rich behavioral repertoire, and a brain volume of just 0.6 mm3, Danionella cerebrum (Dc) holds great promise for whole-brain in vivo imaging analyses with single cell resolution of higher cognitive functions in an adult vertebrate. Little is currently known, however, about the basic locomotor activity of adult and larval Danionella cerebrum and how it compares to the well-established zebrafish model system. Here, we provide a comparative developmental analysis of the larval locomotor activity of Dc and AB wildtype as well as crystal zebrafish in a light-dark test. We find similarities but also differences in both species, most notably a striking startle response of Dc following a sudden dark to light switch, whereas zebrafish respond most strongly to a sudden light to dark switch. We hypothesize that the different startle responses in both species may stem from their different natural habitats and could represent an opportunity to investigate how neural circuits evolve to evoke different behaviors in response to environmental stimuli.
Introduction
With just 10–15 mm in body length, the genus Danionella comprises some of the smallest known extant vertebrate species (Roberts, 1986; Britz et al., 2021). The natural habitats of these miniature cyprinids are the slow-flowing and rather shallow but turbid streams of southern Myanmar and north-eastern India (Roberts, 1986; Britz et al., 2021) that are also home to other members of the subfamily of Danioninae, possibly including the zebrafish, Danio rerio too (Parichy, 2015). In fact, phylogenetic analyses have shown that Danionella are closely related to the zebrafish as they form a sister group of the genus Danio and diverged from a common ancestor about 36 million years ago (Britz et al., 2009; Tang et al., 2010).
Consistent with its miniature body plan, the adult brain of Danionella cerebrum (Dc) [previously erroneously described as Danionella translucida (Britz et al., 2021)] has a volume of just 0.6 mm3 and consists of approximately 6.5 × 105 neurons (Schulze et al., 2018) whereas the adult zebrafish brain has a volume of 2.8 mm3 (Kenney et al., 2021) and consists of approximately 1.0 × 107 cells (Hinsch and Zupanc, 2007). Recently, it has been shown that Dc is amenable to transgenesis, and remains optically translucent during adulthood, particularly in the tyr background (Schulze et al., 2018), thereby enabling the application of whole-brain in vivo imaging techniques (Penalva et al., 2018; Schulze et al., 2018). Larval but not adult zebrafish, particularly in the crystal background (Antinucci and Hindges, 2016), are also optically translucent, and have a brain volume of less than 0.5 mm3 that is made up of 1.0 × 105 neurons (Randlett et al., 2015). The zebrafish larval brain is therefore only slightly smaller than the adult Danionella brain, which is why thus far larval zebrafish have been in the vanguard of whole-brain in vivo imaging analyses (Ahrens et al., 2013; Ahrens and Engert, 2015; Vanwalleghem et al., 2018). However, with their yet immature brains previous studies suggested that larval zebrafish were largely lacking behind their juvenile and adult counterparts in performing associative learning tasks, or emotional and social behaviors thereby pointing toward the possibility that the underlying neural circuits enabling higher cognitive functions and behaviors may not be fully developed and functional at this early developmental stage (Valente et al., 2012; Dreosti et al., 2015; Huang et al., 2020; Stednitz and Washbourne, 2020). More recently though this notion has been challenged as it has been shown, for example, that the performance of 7 days post fertilization (dpf) larval zebrafish equals those of juveniles (21 dpf) and adults (90 dpf) in a spatial discrimination task (Santacà et al., 2020a,b), that 7–10 dpf larvae appear to be capable to learn active avoidance in an operant conditioning task (Yang et al., 2019), or that 10–12 dpf zebrafish can be trained to associate different colors and geometric shapes with a food reward in an appetitive learning task (Santacà et al., 2022). Danionella, therefore, holds great promise for future investigations of whole-brain in vivo imaging analyses of higher cognitive functions and behaviors, in particular during adulthood but also throughout its entire life cycle.
As an emerging neurophysiological model system, little is currently known, however, about the basic locomotor activity of Danionella and how it compares to the well-established zebrafish both at the larval and adult stage. Furthermore, little is currently also known about the larval locomotor activity of the optically translucent pigmentation mutant crystal that is particularly suited for whole-brain in vivo imaging analyses, since it offers an unique access to the forebrain in light sheet microscopy (Antinucci and Hindges, 2016), whereas the locomotor activity of other zebrafish strains, including the pigmentation mutant casper (White et al., 2008), in which forebrain structures are largely inaccessible by conventional light sheet microscopy (Antinucci and Hindges, 2016), has been characterized previously (de Esch et al., 2012; Lange et al., 2013; van den Bos et al., 2017; Audira et al., 2020). Developed for zebrafish larvae, the light-dark test is a high-throughput behavioral paradigm that can be used to analyze locomotor activity during alternating light/dark periods in multi-well plates (Prober et al., 2006; Emran et al., 2008; MacPhail et al., 2008; Irons et al., 2010; Padilla et al., 2011; de Esch et al., 2012; Brun et al., 2019; Fitzgerald et al., 2019; García-González et al., 2021). Locomotor activity of wildtype zebrafish in the light-dark test follows a standardized pattern during both the light and dark periods that can be classified into three phases (Emran et al., 2008; MacPhail et al., 2008; García-González et al., 2021). Immediately after the switch from light to dark, (i) zebrafish larvae respond with a startle response, they then (ii) increase their velocity relative to preceding light period (and higher than baseline level) before (iii) decreasing their velocity again (still higher than baseline level). After the switch from dark to light, (i) zebrafish larvae also respond with a startle response, then (ii) decrease their velocity relative to the preceding dark period [and lower than baseline level (freezing)] before (iii) increasing their velocity again (equal to baseline level). Here, we made use of the light-dark test to analyze and characterize the larval swimming behavior and locomotor activity of 4–6 dpf Danionella cerebrum and compare it with AB wildtype and crystal zebrafish. We found similarities but also differences in both species.
Results
The total duration of the light-dark test is 80 min (4,800 s). In its layout, the test that we used here is identical to the one previously reported by Fitzgerald et al. (2019) and consists of 6 phases: 1 habituation phase (20 min); 1 swimming phase (20 min); 2 dark phases (10 min each); and 2 light phases (10 min each; Figure 1A).
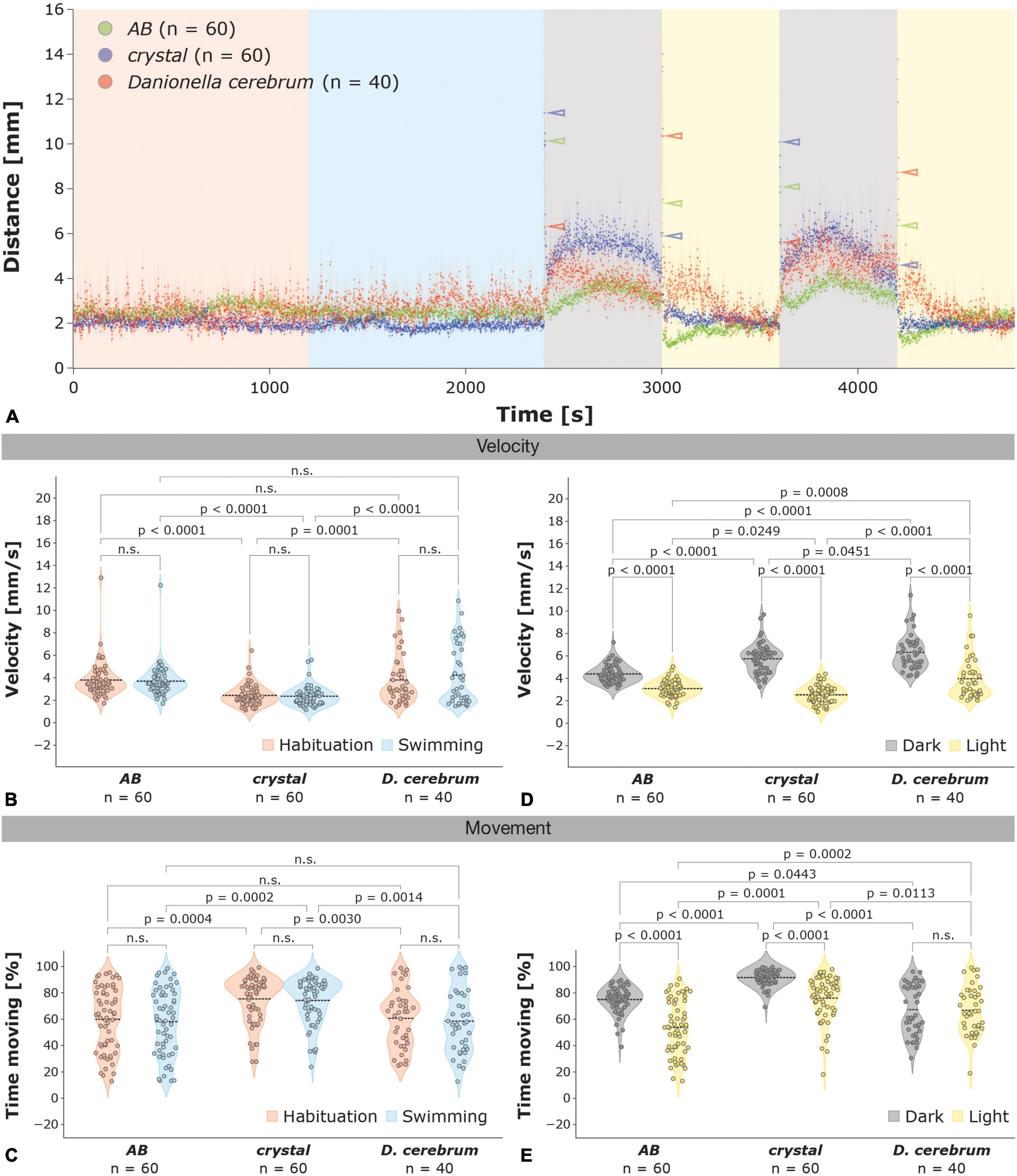
Figure 1. Locomotor activity of 6 dpf zebrafish and Dc larvae in the light-dark test. (A) The light-dark test consists of a habituation (20 min; orange), swimming (20 min; blue) and two alternating dark (10 min each; gray) and light (10 min each; yellow) phases. The average locomotor activity ± the SEM (shaded) per second is shown for zebrafish AB wildtype (green; n = 60), crystal (blue; n = 60), and Dc (red; n = 40) larvae; color-coded arrowheads highlight the increases in locomotor activity 1 s after the illumination switch. (B,C) Violin plots of the velocity during movement (B) and percentage of time spent moving (C) of zebrafish AB wildtype, crystal, and Dc during the habituation (orange) and swimming phase (blue); the mean is indicated by a dotted black line. (D,E) Violin plots of the velocity during movement (D) and percentage of time spent moving (E) of zebrafish AB, crystal, and Dc during the light (yellow) and dark phases (gray); the mean is indicated by a dotted black line. Two-way ANOVA followed by Šídák’s or Tukey’s multiple comparisons test was used to analyze differences in velocity or movement between phases of the light-dark test in and between AB, crystal, and Dc; p > 0.05 is abbreviated as not significant (n.s.).
Danionella and AB wildtype zebrafish larvae have a similar baseline locomotor activity
During the habituation and swimming phase of the light-dark test, 6 dpf Dc and AB wildtype zebrafish showed a similar locomotor activity (total distance over time) and velocity during movement (hereafter referred to as velocity; see Section “Materials and methods” for details; Figures 1A,B) crystal larvae, however, showed a lower locomotor activity and velocity compared to AB zebrafish and Dc (Figures 1A,B). In the swimming phase, the average velocity of Dc and AB zebrafish was 4.24 ± 0.43 and 3.70 ± 0.18 mm/s, respectively, whereas crystal larvae moved with a slower average speed of 2.36 ± 0.11 mm/s [Figure 1B; two-way ANOVA followed by Tukey’s multiple comparisons test F(2,314) = 30.86, AB versus Dc p = 0.2406; AB versus crystal p < 0.0001; crystal versus Dc p < 0.0001]; we did not observe significant differences in locomotor activity or velocity between the habituation and the swimming phase in Dc, AB, and crystal larvae [two-way ANOVA F(1,314) = 0.2000, p = 0.6550; Figures 1A,B]. Similarly, we found no differences in the time spent moving between the habituation and swimming phase in zebrafish AB, crystal, and Dc larvae [Figure 1C; two-way ANOVA F(1,314) = 0.4462, p = 0.5046]. With 58.53 ± 3.81 and 58.25 ± 3.22 percent during the swimming phase, Dc and AB zebrafish, respectively, were found to spent nearly equal amounts of time moving, whereas with 74.25 ± 2.24 percent crystal larvae were found to be moving significantly more [Figure 1C; two-way ANOVA followed by Tukey’s multiple comparisons test F(2,314) = 19.00, AB versus Dc p = 0.9979; AB versus crystal p = 0.0002; crystal versus Dc p = 0.0030].
Danionella and zebrafish larvae show similar increases in locomotor activity during dark periods
Similar to AB and crystal zebrafish, Dc strongly increase their locomotor activity and velocity during the dark relative to the light phases [Figures 1A,D; two-way ANOVA followed by Šídák’s multiple comparisons test F(1,314) = 297.0, dark versus light phases AB p < 0.0001; crystal p < 0.0001; Dc p < 0.0001]. Compared to the light phases, AB showed a 1.4-fold (3.09 ± 0.09 versus 4.39 ± 0.10 mm/s), crystal a 2.3-fold (2.53 ± 0.10 versus 5.75 ± 0.17 mm/s), and Dc larvae a 1.6-fold (3.96 ± 0.28 versus 6.32 ± 0.26 mm/s) increase in their average velocity during the dark phases (Figure 1D). Thus, the average velocity of Dc during both the dark and the light phases was significantly higher than in both AB and crystal zebrafish [Figure 1D; two-way ANOVA followed by Tukey’s post hoc multiple comparison test F(2,314) = 35.33 dark phases AB versus Dc p < 0.0001; crystal versus Dc p < 0.0451; light phases AB versus Dc p < 0.0002; crystal versus Dc p < 0.0001]. In contrast to AB and crystal zebrafish larvae that increased the percentage of their time spent moving from 53.83 ± 2.70 and 76.04 ± 2.12 during the light to 74.99 ± 1.30 and 91.66 ± 0.74, respectively, during the dark phases, Dc spent similar amounts of time moving in the light and dark phases (66.79 ± 2.89 light versus 67.31 ± 3.03 percent dark; Figure 1E).
Danionella and zebrafish larvae show different light-dark and dark-light startle responses
Zebrafish and Dc larvae differ most strikingly in their startle behavior immediately after the switch from light to dark and dark to light (Figures 1A, 2A–D and Supplementary Figure 1). AB and crystal zebrafish strongly increase their locomotor activity immediately after the switch from the swimming to the first dark phase (Figures 1A, 2A) and, similarly, during the switch from the first light to the second dark phase (Figure 1A and Supplementary Figure 1A), and in particular during the first second following the switch (Figure 2A and Supplementary Figure 1A). Dc also show a startle response during the first second of the light to dark switch, however, it is much less pronounced than in zebrafish (Figure 2A and Supplementary Figure 1A). With an average velocity of 11.38 ± 0.77 mm/s the amplitude of the startle response was highest in crystal followed by 10.14 ± 0.45 mm/s in AB but was only 6.32 ± 0.39 mm/s in Dc larvae which was significantly different from both crystal and AB [Figures 1A, 2A,B; one-way ANOVA followed by Tukey’s post hoc multiple comparison test F(2,157) = 16.53, AB versus Dc p = 0.0001; crystal versus Dc p < 0.0001], while there was no difference between AB and crystal zebrafish larvae [one-way ANOVA followed by Tukey’s post hoc multiple comparison test F(2,157) = 16.53, AB versus crystal p = 0.2696]. Differences between zebrafish and Dc in the amplitude of their startle response were even more pronounced when switching the illumination from dark to light albeit now with opposing amplitudes relative to the light to dark switch (Figures 1A, 2C,E and Supplementary Figures 2C,E). Dc showed the highest amplitude in the startle response 3 s after the switch, whereas wildtype AB and crystal zebrafish showed the highest response again after 1 s (Figure 2C). Three seconds after the dark to light switch Dc peaked with an average velocity of 14.01 ± 1.21 mm/s that was significantly higher compared to the average velocity of 3.69 ± 0.35 and 3.31 ± 0.41 mm/s that we observed in AB in crystal larvae, respectively (Figures 2C,D; Kruskal–Wallis test followed by Dunn’s multiple comparison test AB versus Dc p < 0.0001; crystal versus Dc p < 0.0001; AB versus crystal p > 0.9999). Moreover, we did not detect a freezing phase, i.e., a decrease in locomotor activity below baseline levels following the dark > light switch in Dc although it was manifest in AB wildtype and, to a lesser extent, in crystal zebrafish larvae (Figures 1A, 2C and Supplementary Figure 1C).
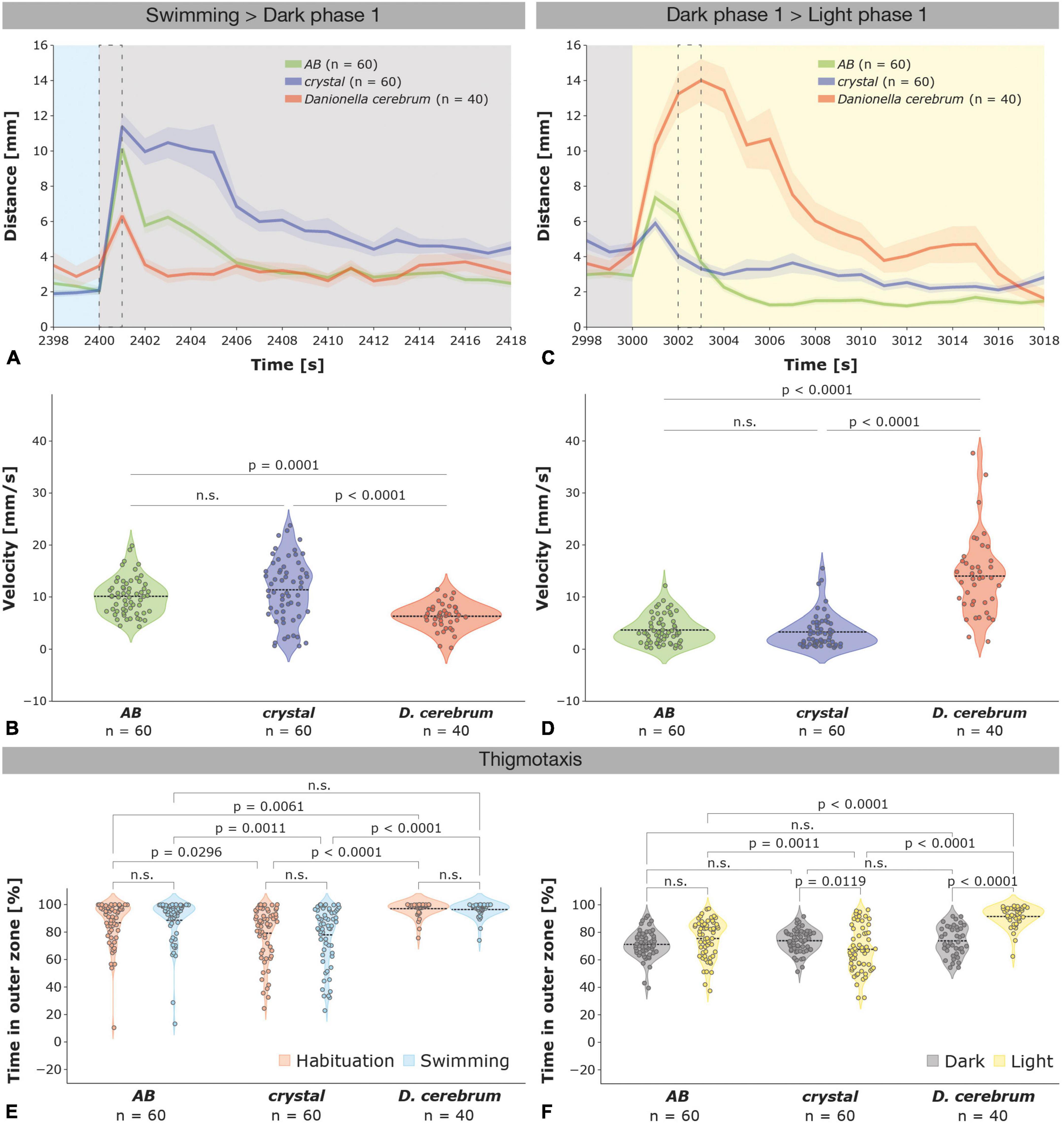
Figure 2. Different startle responses evoked by illumination changes in zebrafish and Dc larvae. (A) Startle responses ± SEM (shaded) of 6 dpf zebrafish AB wildtype (green; n = 60), crystal (blue; n = 60), and Dc (red; n = 40) larvae depicted from 2 s before (2,398 s) to 18 s after (2,418 s) the first light (swimming phase; blue) to dark (dark phase 1; gray) switch (see also Supplementary Figure 1A); a dotted black rectangle indicates the 1 s time interval that was used to compare the velocity of the larvae in (B). (B) Violin plots depicting the velocity of AB, crystal, and Dc larvae during 1 s (2,400–2,401 s) following the first light to dark switch (see also Supplementary Figure 1B). Note that Dc increase their velocity significantly less than AB wildtype and crystal zebrafish. (C) Startle responses of 6 dpf zebrafish AB wildtype (green), crystal (blue), and Dc (red) larvae depicted from 2 s before (2,998 s) to 18 s after (3,018 s) the first dark (gray) to light (yellow) switch (see also Supplementary Figure 1C); a dotted black rectangle indicates the 1 s time interval that was used to compare the velocity of the larvae in (D). (D) Violin plots depicting the velocity of AB, crystal, and Dc larvae during 1 s (3,002–3,003 s) following the first dark to light switch (see also Supplementary Figure 1D). Note that Dc increase their velocity significantly more and during a longer time period than AB wildtype and crystal zebrafish. One-way ANOVA followed by Tukey’s multiple comparisons test or Kruskal–Wallis test followed by Dunn’s multiple comparisons test was used to analyze differences in velocity between AB, crystal, and Dc; p > 0.05 is abbreviated as not significant (n.s.). (E) Violin plots depicting the time spent in the outer zone during the habituation (orange) and swimming (blue) phase is highest in Dc followed by AB, but lower in crystal larvae. (F) Violin plots show that thigmotaxis is increased in Dc relative to both AB and crystal zebrafish larvae during the light but not the dark phases. Two-way ANOVA followed by Šídák’s or Tukey’s multiple comparisons test was used to analyze differences in thigmotaxis between phases of the dark-light test in and between AB, crystal, and Dc; p > 0.05 is abbreviated as not significant (n.s.).
Danionella show increased thigmotaxis relative to zebrafish larvae in the light
Thigmotaxis or centrophobism, i.e., the tendency of animals to avoid the center area of an open field or arena and instead to spend more time in its periphery, is a behavioral response that is evolutionary conserved from Drosophila (Besson and Martin, 2005; Mohammad et al., 2016) to zebrafish (Colwill and Creton, 2011; Richendrfer et al., 2012; Schnörr et al., 2012; Pietri et al., 2013; Zhang et al., 2017; Xu and Guo, 2020) and mammals (Hall, 1934; Denenberg, 1969; Treit and Fundytus, 1988; Prut and Belzung, 2003), including humans (Walz et al., 2016; Gromer et al., 2021). Although a natural behavioral tendency across species, it has been suggested that thigmotaxis is indicative of an anxiety-like state in both larval and adult zebrafish (Maximino et al., 2010; Richendrfer et al., 2012; Schnörr et al., 2012; Pietri et al., 2013; Zhang et al., 2017; Abreu et al., 2020; Xu and Guo, 2020).
During the habituation and swimming phase, thigmotaxis was highest in Dc, followed by wildtype AB zebrafish, whereas crystal larvae spent comparatively less time in the outer zone of the wells (Figure 2E); we did not observe significant differences between the habituation and swimming phase in Danionella or zebrafish [two-way ANOVA F(1,314) = 0.0001574, p = 0.9900]. In the swimming phase, Dc were found 96.40 ± 0.81 percent of the time in the outer zone while AB and crystal zebrafish engaged in thigmotactic behavior 88.62 ± 2.16 and 77.95 ± 2.74 percent of the time, respectively. The thigmotactic behavior of crystal larvae was therefore significantly lower compared to both AB zebrafish and Dc whereas the difference between AB and Dc was not [Figure 2E; two-way ANOVA followed by Tukey’s multiple comparison test F(2,314) = 30.45, crystal versus AB p = 0.0011; crystal versus Dc p < 0.0001; AB versus Dc p = 0.0512]. Relative to the light Dc decreased thigmotaxis during the dark phases (from 91.58 ± 1.21 in the light to 73.79 ± 1.70 percent in the dark) but showed significantly increased levels of thigmotactic behavior relative to both wildtype AB (71.21 ± 1.28 in the dark and 75.55 ± 1.78 percent in the light) and crystal zebrafish (73.88 ± 1.07 in the dark and 67.62 ± 2.13 in the light) during the light phases [Figure 2F; two-way ANOVA followed by Tukey’s multiple comparison test F(2,314) = 25.69, Dc versus AB p < 0.0001; Dc versus crystal p < 0.0001]. Thus, the thigmotactic behavior of AB wildtype zebrafish was only mildly altered in the dark versus the light phases, whereas crystal and Dc larvae responded with an opposing behavior, namely by an increase and a decrease in thigmotaxis, respectively, in the dark relative to the light phases (Figure 2F).
Age-dependent locomotor activity and light-dark and dark-light startle responses in 4–6 dpf Dc and AB zebrafish larvae
How does the locomotor activity of Danionella larvae change during development and at what developmental age do larvae respond to changes in illumination in the light-dark test? To answer these questions we investigated the locomotor activity in 4 and 5 dpf Dc and compared it to 6 dpf larvae. We found that the velocity during movement in the swimming phase was lower in 4 dpf Dc (3.09 ± 0.51 mm/s) compared to 5 dpf (4.45 ± 0.55 mm/s) and 6 dpf (4.24 ± 0.43 mm/s) larvae, although this difference was not significant [Figures 3A,B; two-way ANOVA followed by Šídák’s multiple comparisons test F(2,194) = 4.563, 4 dpf versus 5 dpf p = 0.1583; 4 dpf versus 6 dpf p = 0.2180]. However, the percentage of time during the swimming phase that 4 dpf (24.33 ± 4.02%) spent moving was less than half that of 5 dpf (56.21 ± 4.53%) and 6 dpf (58.53 ± 3.81%) larvae [Figure 3C; two-way ANOVA followed by Tukey’s multiple comparisons test F(2,194) = 49.85, 4 dpf versus 5 dpf p < 0.0001; 4 dpf versus 6 dpf p < 0.0001] whereas there was no difference between 5 dpf and 6 dpf [Figure 3C; two-way ANOVA followed by Tukey’s multiple comparisons test F(2,194) = 49.85, 5 dpf versus 6 dpf p = 0.9130]. Increases in the velocity during the dark relative to the light phases were found significant in 5 and 6 dpf Dc larvae whereas in 4 dpf they were not [Figure 3D; two-way ANOVA followed by Šídák’s multiple comparisons test F(1,194) = 28.95, 6 dpf p < 0.0001; 5 dpf p = 0.0383; 4 dpf p = 0.0600]. Similar to the habituation and swimming phases, 5 and 6 dpf generally spent more time moving than 4 dpf during both the dark and the light phases, and 4 and 5 dpf tended to spend a higher percentage of time moving during the dark relative to the light phases whereas 6 dpf Dc larvae did not (Figure 3E).
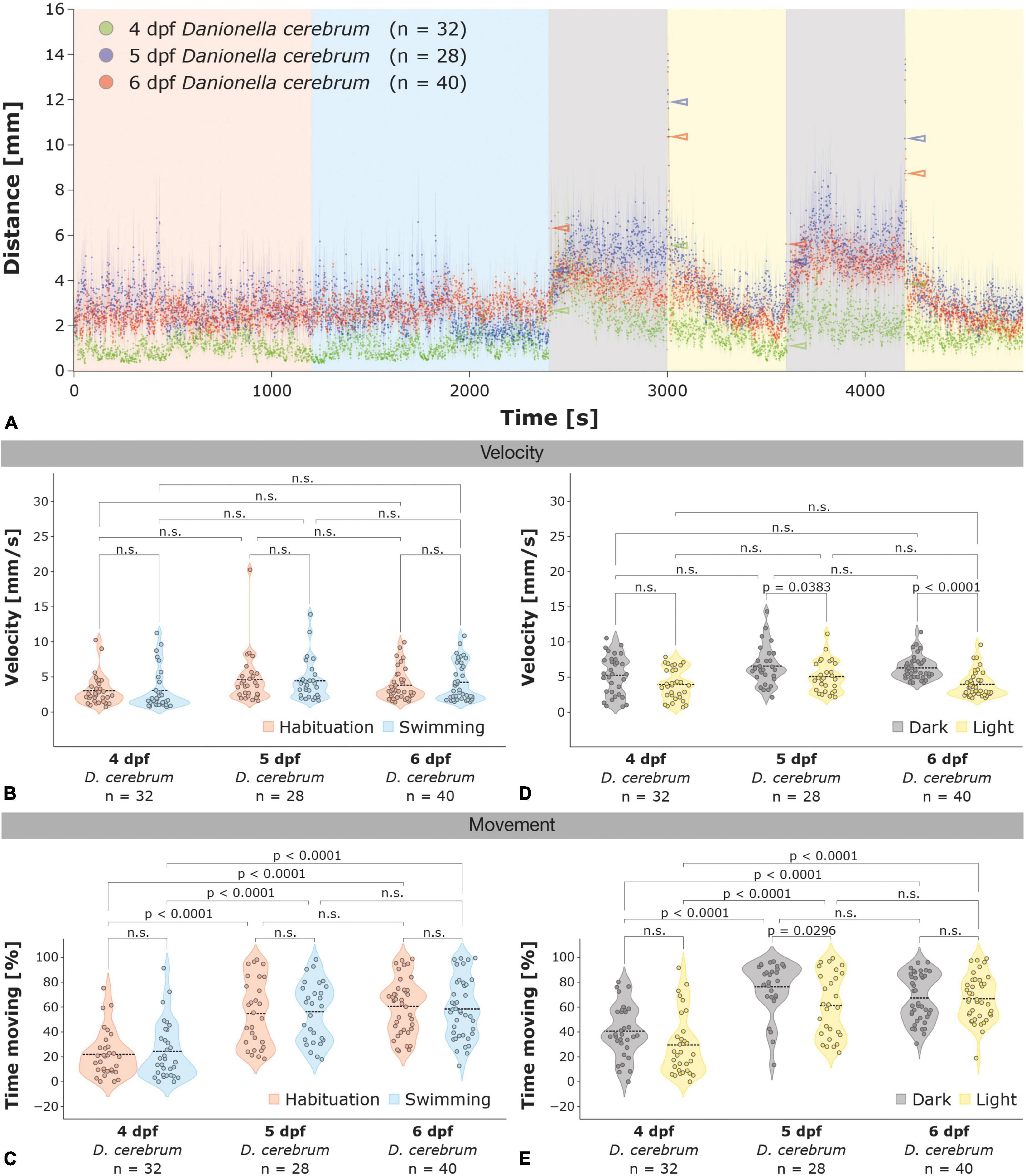
Figure 3. Locomotor activity of 4–6 dpf Dc larvae in the light-dark test. (A) Locomotor activity of 4 dpf (green; n = 32), 5 dpf (blue; n = 28), and 6 dpf (red; n = 40) Dc larvae in the light-dark test; color-coded arrowheads highlight the increases in locomotor activity 1 s after the illumination switch. (B,C) Violin plots of the velocity during movement (B) and the time spent moving (C) for 4–6 dpf Dc larvae in the habituation (red) and swimming (blue) phase. (D,E) Violin plots of the velocity during movement (D) and the time spent moving (E) for 4–6 dpf Dc larvae in the light (yellow) and dark (gray) phases. Note the reduced movement of 4 dpf (C,E) and the lack of increase in velocity during the dark phases (A,D) relative to 5 and 6 dpf Dc larvae. Two-way ANOVA followed by Šídák’s or Tukey’s multiple comparisons test was used to analyze differences in velocity or movement between phases of the light-dark test in and between 4 and 6 dpf Dc; p > 0.05 is abbreviated as not significant (n.s.).
During the light to dark and dark to light switches, 4 dpf Dc exhibited a startle response, however, it was overall less pronounced than in 5 and 6 dpf larvae that showed comparatively similar responses (Figures 4A–D and Supplementary Figure 2). One second after the first light to dark switch 4, 5, and 6 dpf had an average velocity of 2.68 ± 0.65 mm/s, 4.46 ± 0.69 mm/s, and 6.32 ± 0.39 mm/s, respectively (Figures 4A,B; Kruskal–Wallis test followed by Dunn’s multiple comparisons test 4 dpf versus 5 dpf p = 0.0865; 4 versus 6 dpf p < 0.0001; 5 versus 6 dpf p = 0.1678). As previously shown for 6 dpf, the amplitude of the startle response of 4 and 5 dpf Dc larvae in a dark to light switch was 2–3 times higher relative to a light to dark switch peaking at an average of 7.14 ± 2.16 mm/s in 4 dpf, 13.72 ± 2.21 mm/s in 5 dpf, and 14.01 ± 1.21 mm/s in 6 dpf after 3 s following the switch (Figures 4C,D; Kruskal–Wallis test followed by Dunn’s multiple comparisons test 4 dpf versus 5 dpf p = 0.0024; 4 versus 6 dpf p < 0.0001; 5 versus 6 dpf p > 0.9999).
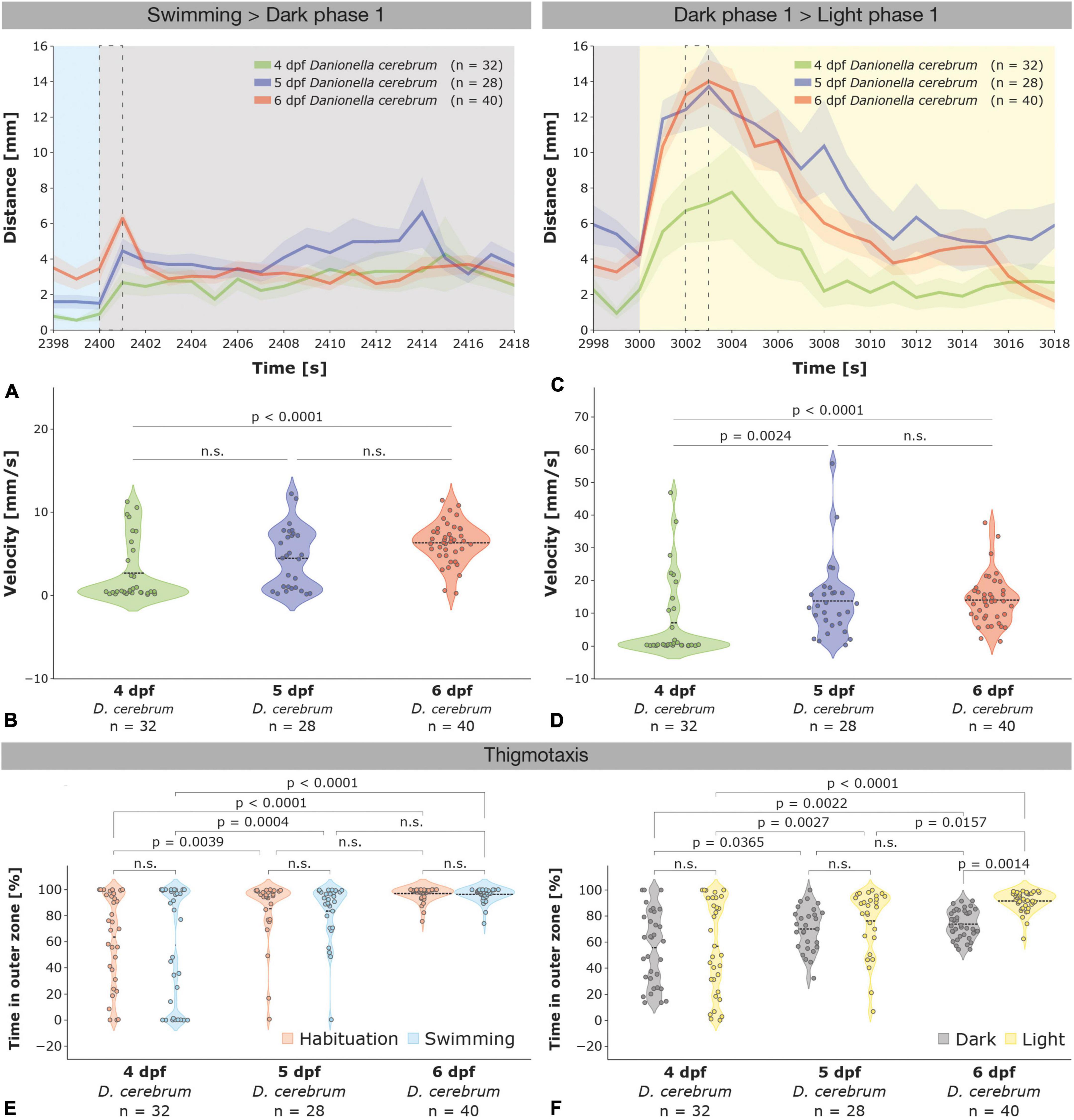
Figure 4. Age-dependent startle responses evoked by illumination changes and thigmotaxis in 4–6 dpf Dc larvae. (A) Startle responses with standard error of the mean (SEM; shaded area) of 4 dpf (green, n = 32), 5 dpf (blue, n = 28), and 6 dpf (red, n = 40) Dc larvae depicted from 2 s before (2,398 s) to 18 s after (2,418 s) the first light (swimming phase; blue) to dark (dark phase 1; gray) switch (see also Supplementary Figure 2A); a dotted black rectangle indicates the 1 s time interval that was used to compare the velocity of the larvae in (B). (B) Violin plots depicting the velocity of 4–6 dpf Dc larvae during 1 s (2,400–2,401 s) following the first light to dark switch (see also Supplementary Figure 2B). (C) Startle responses of 4–6 dpf Dc larvae depicted 2 s before (2,998 s) and 18 s after (3,018 s) the first dark (gray) to light (yellow) switch; see also Supplementary Figure 2C; a dotted black rectangle indicates the 1 s time interval that was used to compare the velocity of the larvae in (D). (D) Violin plots depicting the velocity of 4–6 dpf Dc larvae during 1 s (3,002–3,003 s) following the first dark to light switch (see also Supplementary Figure 2D). Note that 5 and 6 dpf Dc larvae increase their velocity significantly more than 4 dpf. Kruskal–Wallis test followed by Dunn’s multiple comparisons test was used to analyze differences in velocity between 4 and 6 dpf Dc; p > 0.05 is abbreviated as not significant (n.s.). (E,F) Violin plots depicting the time spent in the outer zone of the wells show an age-dependent increase in thigmotaxis in 5 and in 6 dpf compared to 4 dpf Dc larvae during the habituation and swimming (E) and also during the light and dark phases (F). Two-way ANOVA followed by Šídák’s or Tukey’s multiple comparisons test was used to analyze differences in thigmotaxis between phases of the light-dark test in and between 4 and 6 dpf Dc; p > 0.05 is abbreviated as not significant (n.s.).
The described development of locomotor activity in 4–6 dpf Dc is largely similar to the development of locomotor activity in 4–6 dpf AB zebrafish larvae. Four dpf AB also show a lower locomotor activity (Supplementary Figure 3A) and velocity (Supplementary Figures 3B,D) compared to 5 and 6 dpf AB larvae throughout all phases of the dark-light test. Although the time spent moving of 4 dpf AB during the swimming phase (37.77 ± 3.67%) is not less than half of that of 5 (72.68 ± 3.14%) and 6 dpf (58.29 ± 3.22%) AB larvae, as it is the case in 4 versus 5 and 6 dpf Dc (see above), it is still substantially reduced during all but the dark phases (Supplementary Figures 3C,E). The overall similarities in locomotor activity of 5 and 6 dpf AB, but not 4 dpf AB zebrafish, further extend to similarities in their corresponding startle responses during the light to dark and dark to light switches (Supplementary Figures 4A–D, 5A–D). Here, we found the increases in the velocity during the first second following the first (Supplementary Figures 4A,B) and second (Supplementary Figures 5A,B) light to dark switch of 5 and 6 dpf AB zebrafish to be significantly different from 4 dpf but quite similar in 5 and 6 dpf larvae (Supplementary Figure 4B; Kruskal–Wallis test followed by Dunn’s multiple comparisons test 4 dpf versus 5 dpf p = 0.0182; 4 versus 6 dpf p = 0.0339; 5 versus 6 dpf p > 0.9999). More pronounced than 4 dpf but similar in between 5 and 6 dpf AB zebrafish startle responses were also seen in both dark to light switches (Supplementary Figures 4C,D, 5C,D). However, whereas the startle response of 6 dpf AB larvae exhibited a significantly higher amplitude relative to 4 dpf AB during both dark to light switches, the increase in velocity of 5 dpf relative to 4 dpf reached significance only during the second (Supplementary Figure 5D) but not during the first (Supplementary Figure 4D) dark to light switch.
Age-dependent thigmotaxis of 4–6 dpf Danionella versus age-independent thigmotaxis of zebrafish larvae
During all (habituation, swimming, dark, and light) phases of the light-dark test, thigmotaxis was higher in 5 and 6 dpf Danionella compared to 4 dpf larvae (Figures 4E,F). During the swimming phase, for example, 4 dpf larvae spent only slightly more than half of their time (57.34 ± 7.74%) in the outer zone of the wells whereas 5 dpf (83.54 ± 4.23%) and 6 dpf (96.40 ± 0.81%) larvae spent most of their time in the outer zone [Figure 4E; two-way ANOVA followed by Tukey’s multiple comparisons test F(2,194) = 35.37, 4 dpf versus 5 dpf p = 0.0004; 4 dpf versus 6 dpf p < 0.0001; 5 dpf versus 6 dpf p = 0.1107]. Thigmotaxis decreased during the dark phases relative to light phases in all developmental ages of Dc, although this effect was most strongly seen at 6 dpf [Figures 4F; 4 dpf: 56.84 ± 6.36% light phases versus 55.72 ± 5.03% dark phases; 5 dpf: 76.23 ± 4.71% light phases versus 70.06 ± 3.16% dark phases; 6dpf: 91.58 ± 1.21% versus 73.80 ± 1.70% dark phases; two-way ANOVA followed by Šídák’s multiple comparisons test F(1,194) = 6.886, 4 dpf p = 0.9960; 5 dpf p = 0.6584; 6 dpf p = 0.0014]. Thus, Dc exhibit an age-dependent thigmotaxis that increases from 4 to 6 dpf and decreases during the dark relative to light periods. This age-dependent thigmotaxis of Dc is distinct from an age-independent thigmotaxis of AB zebrafish, however, as we found the percentage of time spent in the outer zone of the wells throughout all phases of the light-dark test largely unchanged in 4–6 dpf AB larvae (compare Figures 4E,F for 4–6 dpf Dc with Supplementary Figures 4E,F for 4–6 dpf AB zebrafish). Analogous to 4–6 dpf Dc though, 4–6 dpf AB zebrafish also exhibited a reduced thigmotaxis during the dark relative to the light periods (compare Figure 4F with Supplementary Figure 4F).
Discussion
Making use of a light-dark test, we analyzed and compared the larval locomotor activity of AB wildtype and crystal zebrafish with D. cerebrum, an emerging neurophysiological model species (Britz et al., 2021; Rajan et al., 2022a). Furthermore, we compared and analyzed the development of larval locomotor activity in 4, 5, and 6 dpf Dc and AB zebrafish.
Development of locomotor activity and thigmotaxis in Dc compared to zebrafish larvae
Overall, the ontogenetic development of larval locomotor activity in 4–6 dpf Dc and AB zebrafish appears to be largely similar. In Dc as well as in AB zebrafish, 4 dpf show a lower locomotor activity and spend more time resting compared to 5 and 6 dpf larvae; for zebrafish this has also been previously described by Colwill and Creton (2011), Padilla et al. (2011), and Ingebretson and Masino (2013). Both 4–6 dpf Dc and AB zebrafish decrease their resting time and increase their velocity in the dark relative to the light phases. Dc and AB zebrafish also both exhibit an age-dependent response manifest in how the two species respond to changes in illumination. Here, 5 and 6 dpf Dc as well as 5 and 6 dpf AB zebrafish show a comparable amplitude in their startle responses that is different and less pronounced at 4 dpf in both species. In 4 dpf Dc we occasionally observed Rosetta-like locomotor activity patterns of concentric trajectories (Supplementary Figures 6A–C); such activity patterns, however, were not observed in zebrafish larvae (Supplementary Figure 6D).
Due to their lack of pigmentation, reduced movement, and optical translucency we were unable to reliably detect and track 4 dpf crystal larvae with our system. In general, tracking and, in particular, detection of non-moving crystal larvae proved to be challenging and more difficult than detection and tracking of AB zebrafish or Dc. Even at 5 dpf crystal larvae were moving less than half of the time (<50%) during all test phases except the dark phase, possibly indicating a slightly delayed development compared to AB wildtype, whereas 6 dpf crystal moved significantly more (>74%; Supplementary Figures 7C,E). Aside from differences in movement, 5 and 6 dpf crystal larvae showed an overall similar locomotor activity (Supplementary Figure 7A), velocity (Supplementary Figures 7B,D), and responses to changes in illumination (Supplementary Figures 7A, 8A–D, 9A–D). However, the amplitude of the startle response following the light to dark (Supplementary Figures 8A, 9A) and dark to light switch (Supplementary Figures 8A, 9A) appeared to be more variable and less consistent in 5 dpf in relation to 6 dpf crystal larvae, suggesting comparable behavioral analyses are best performed at 6 dpf (Fitzgerald et al., 2019).
In contrast to 6 dpf AB and Dc that decreased thigmotaxis during the dark relative to the light phases, 6 dpf crystal zebrafish apparently increased their time spent in the outer zone of the wells during the dark (Figure 2F); we did not observe increased thigmotaxis during the dark in 5 dpf crystal larvae though (Supplementary Figure 8F). The transparent nature due to the lack of pigmentation of crystal larvae could possibly bias them to increase thigmotaxis in the dark as a predator avoidance behavior. However, as increased thigmotaxis during the dark was not observed in 5 dpf crystal, it is also possible that thigmotaxis is generally more variable in crystal larvae and that individuals of this pigmentation mutant exhibit a higher individual variability compared to AB zebrafish.
The age-dependent thigmotaxis in 4–6 dpf Dc contrasts with age-independent thigmotaxis that we and others (e.g., Colwill and Creton, 2011) observed for AB zebrafish of the same age. The Rosetta-like locomotor activity pattern, in which 4 dpf Dc swim in concentric trajectories mostly within the center of the wells and which is absent in their evolutionary closely related zebrafish counterparts and also rarely seen in Dc older than 4 dpf, may contribute to this apparent age-dependency; however, since we observe this pattern only occasionally and not in all individuals, it may be a contributing but not a determining factor. In principle, an increase in movement, as it is seen from 4 to 5 and 6 dpf in Dc (Figures 3C,E), coupled with an increase in the ratio of straight paths versus turns could also lead itself to an increase in thigmotaxis when locomotor activity is measured in arenas with concave walls, as has been pointed out by Fero et al. (2010) and Horstick et al. (2016). However, considering the fact that 4 dpf AB zebrafish also show a largely reduced amount of time spent moving compared to their 5 and 6 dpf counterparts (Supplementary Figures 3C,E), but, at the same time, exhibit no major differences in the time spent in the outer zone of the wells (Supplementary Figures 4E,F), makes an explanation relying solely on an increase of straight forward motion at the expenditure of turns rather unlikely, even though we are currently lacking information about how such a glide and turn ratio compares between Dc and zebrafish.
That Dc larvae show an increased thigmotaxis relative to zebrafish during the light (Figure 2F), together with a strong startle response during a dark to light switch (Figures 2C,D and Supplementary Figures 1C,D), and the observation that Dc preferentially occupy the lower zone of a water column (Rajan et al., 2022b, see also below) appears to be indicating that Dc may generally favor a rather dark over a light environment. Since thigmotaxis has also been associated with an anxiety-like behavior (Maximino et al., 2010; Richendrfer et al., 2012; Schnörr et al., 2012; Pietri et al., 2013; Zhang et al., 2017; Abreu et al., 2020; Xu and Guo, 2020), increased thigmotaxis of Dc relative to zebrafish during the light periods could also be indicating increased levels of anxiety in Dc. Although we cannot exclude this possibility, ascribing heightened levels of anxiety to Dc compared to zebrafish based solely on a single behavioral parameter appears to be premature, which is why we currently favor a natural habitat or environmental-based hypothesis as a more plausible explanation for the observed phenomena.
Different natural habitats may be underlying different startle responses in Dc and zebrafish larvae
Although the baseline locomotor activity is comparatively similar in 6 dpf wildtype AB zebrafish and Dc, but not crystal, and both species increase their velocity during the dark relative to the light phases, they differ strikingly in their startle response to sudden changes in illumination (Figures 1A, 2A–D and Supplementary Figure 1). Whereas AB and crystal larvae respond strongly to a light > dark switch but only weakly to a dark > light switch, Dc respond strongly to a dark > light switch and only weakly to a light > dark switch. What may be causing this differential response in the two evolutionary closely related species? One possible explanation may be that D. rerio and D. cerebrum occupy different depths within the water column of the slow flowing streams, pools, and ponds of northeastern India and Myanmar that form their natural habitat and where they may or may not sometimes even co-exist. Indeed, it has been reported that D. cerebrum was found at a depth below 30 cm of the water surface (Britz et al., 2021) and with adults spawning in crevices and small openings at the bottom of laboratory tanks (Schulze et al., 2018) which is in contrast to zebrafish that spawn in shallow and typically clear water near the surface (Parichy, 2015). Reports from observations of the two species in their natural habitats were recently further confirmed in the laboratory by directly showing that 6 dpf Dc predominantly (∼80%) occupy the lower zone (0–12 cm) whereas 6 dpf zebrafish larvae predominantly (∼80%) occupy the upper zone (24–36 cm) of a water column with a total height of 36 cm (Rajan et al., 2022b). Larval zebrafish may thus generally be more accustomed to a brighter environment thereby triggering a strong light > dark but a comparatively weaker dark > light startle response, whereas larval Dc may generally be more accustomed to a darker environment thereby triggering a strong dark > light but a comparatively weaker light > dark startle response. Since both zebrafish, in particular in the crystal background (Antinucci and Hindges, 2016; Mattern et al., 2020), and Dc are uniquely amenable to whole-brain in vivo imaging techniques (Ahrens et al., 2013; Schulze et al., 2018; Rajan et al., 2022b) the different light-dark and dark-light response in both species may possibly represent an interesting opportunity for a comparative neurophysiological analysis of the mechanisms and evolution of neural circuits in two closely related vertebrate species through which they evoke different behavioral responses to similar environmental stimuli.
Materials and methods
Zebrafish and Danionella maintenance
Zebrafish (D. rerio) and D. cerebrum were maintained and raised at 28°C on a 14 h light/10 h dark cycle and bred following standard procedures (Schulze et al., 2018; Aleström et al., 2019; Rajan et al., 2022a). Danionella eggs were collected from spontaneous spawnings, and both species were raised in 30% Danieau solution [17.4 mM NaCl, 0.21 mM KCl, 0.12 mM MgSO4, 0.18 mM Ca(NO3)2, 1.5 mM HEPES, pH 7.0] in 94 mm (diameter) × 16 mm (depth) petri dishes (Greiner Bio-One, Kremsmünster, Austria).
Experimental setup
The experimental setup consisted of custom-made black box with the dimensions 666 mm (length) × 472 mm (width) × 1010 mm (height) fabricated by Noldus (Wageningen, Netherlands) that shielded larvae from external influences. The box was illuminated through light emitting diodes (LEDs) of a white light and infrared (IR; 940–950 nm) backlight unit located at the bottom and contained a Gigabit Ethernet camera (acA1300-60gm; Basler, Ahrensburg, Germany) attached to 12 mm/F1.4 lens (Kowa, Nagoya, Japan) with an 850 nm IR filter (Heliopan, Gräfelfing, Germany) on the top. The white light unit was connected to an USB-IO box Noldus (Wageningen, Netherlands) that was controlled through EthoVision XT software (15.0.1418, Noldus, Wageningen, Netherlands) running under Windows 10 Pro (Microsoft, Redmond, WA, United States) on a Dell (Round Rock, TX, United States) workstation. 12-well plates (Greiner Bio-One, Kremsmünster, Austria) filled with 4 ml of Danieau solution were placed directly on top of the IR (940 nm) and white light illumination unit. Illuminance inside the wells was measured at 1,300 lux with a Panlux electronic 2 photometer [Gossen Metrawatt (previously Gossen) Nürnberg, Germany]. A Fresnel lens (Noldus, Wageningen, Netherlands) was placed in between the Gigabit Ethernet camera and the 12-well plate (at a distance of 20 and 265 mm from the 12-well plate and the Gigabit Ethernet camera, respectively) to reduce distortion of non-centered wells relative to the camera’s position and to increase the contrast within and in particular at the border of the wells in order to optimize IR tracking quality and robustness. The temperature of the room that contained the experimental setup was maintained at 28°C.
Light-dark test and tracking
The light dark test was performed as previously reported by Fitzgerald et al. (2019) with slight modifications. 24 h before the start of the behavioral analysis larvae were transferred from a 94 mm (diameter) × 16 mm (depth) petri dish (Greiner Bio-One, Kremsmünster, Austria) into individual wells [22.2 mm (diameter) × 16.5 mm (depth)] filled with 4 ml Danieau solution on a 12-well plate (Greiner Bio-One, Kremsmünster, Austria) to accustom to the new environment. On the day of the experiment, larvae in the 12-well plates were transferred from the incubator in which they were raised to the experimental setup at 12:00 p.m. to which they were allowed to accustom for 1 h before the light-dark test was started at 1:00 p.m. The light-dark test was thus always carried out at the same time of the day and experimental parameters were kept constant to avoid as much as possible potential effects on locomotor activity as has been reported previously (MacPhail et al., 2008; Ingebretson and Masino, 2013).
Live video tracking was performed with 30 frames per second (fps) at a resolution of 1,280 pixels × 1,024 pixels with EthoVision XT software (15.0.1418, Noldus, Wageningen, Netherlands) that also controlled the light-dark and dark-light illumination switches through an USB-IO box (Noldus, Wageningen, Netherlands) that was connected to the custom-made black box containing the white light and IR illumination unit (Noldus, Wageningen, Netherlands). The total duration of the light-dark test was 4,800 s (80 min) and it was divided into the following phases: 0 – 1,200 s habituation phase; 1,201 – 2,400 s swimming phase; 2,401 – 3,000 s dark phase 1; 3,001 – 3,600 s light phase 1; 3,601 – 4,200 s dark phase 2; and 4,201 – 4,800 s light phase 2. The light was switched off at the end of the swimming phase at 2,400 and light phase 1 at 3,600 s; the light was switched on at the end of dark phase 1 at 3,000 and dark phase 2 at 4,200 s.
To measure thigmotaxis of Danionella and zebrafish larvae in the light-dark test we defined an outer and an inner zone within each well of the 12-well plate with an equal surface area with EthoVision XT software (15.0.1418, Noldus, Wageningen, Netherlands).
Data analysis and processing
Data were analyzed and processed with EthoVision XT software (15.0.1418, Noldus, Wageningen, Netherlands) and exported to Microsoft Excel (Microsoft, Redmond, WA, United States). Graphs were generated with plotly in Python1 and assembled with Adobe Illustrator (24.3, San Jose, CA, United States). For the analysis of locomotor activity (Figures 1A, 2A,C, 3A, 4A,C and Supplementary Figures 1A,C, 2A,C) the average total distance of AB, crystal and Danionella larvae per second time interval recorded with 30 fps was first exported from EthoVision XT to Microsoft Excel and organized into data sheets. The standard error of the mean (SEM) was then calculated in Python for each time point per second, and the data were visualized with plotly. Similarly, data for velocity (Figures 1B,D, 3B,D), movement (Figures 1C,E, 3C,E), and thigmotaxis (Figures 2E,F, 4E,F) during the swimming, habituation, both light and both dark phases of the light-dark test was also exported from EthoVision XT to Microsoft Excel and visualized with plotly.
A threshold setting of 0.84 and 0.42 mm/s with an averaging interval of 3 frames (100 ms) was defined with EthoVision XT for moving versus non-moving larvae, respectively. This threshold was defined based on the average larval body length of 4.2 mm (and our observations of resting versus moving larvae) that we measured in AB, crystal, and Danionella at 6 dpf (Supplementary Figure 10; see also the section body length measurements below). Thus, larvae moving less than 1/10 of their body length per second were considered not-moving whereas larvae moving more than 1/5 per second were considered moving. Based on this definition we analyzed the velocity in moving larvae to which we also applied an averaging interval across 3 frames (100 ms); no averaging interval, however, was applied for the analysis of the velocity during the 1 s time intervals of the startle responses (Figures 2B,D, 4B,D and Supplementary Figures 1B,D, 2B,D).
Body length measurements
Body (snout to tail including the fin) and standard length (snout to tail excluding the fin; Parichy et al., 2009) were measured in 6 dpf AB wildtype and crystal zebrafish and in 4–6 dpf Danionella larvae after the light-dark test (Supplementary Figure 10). Single larvae were anesthetized with MS-222 [Merck (previously Sigma-Aldrich) Darmstadt, Germany] in the wells of the 12-well plate and imaged with a Leica M205 FA stereomicroscope (Leica Microsystems, Wetzlar, Germany) controlled by LAS X software (3.4.2.18368; Leica Microsystems, Wetzlar, Germany). Body and standard length were measured with the scale bar tool of LAS X on the acquired images.
Statistics
Statistical analysis was performed with Prism (9.1.2, GraphPad, La Jolla, CA, United States). A D’Agostino and Pearson and an Anderson-Darling test was used to determine whether the data followed a Normal (Gaussian) distribution. Parametric statistical analysis was performed by one-way (Figures 2B, 4B,D and Supplementary Figures 1B,D, 2B,D, 5B,D, 10) or two-way analysis of variance (ANOVA) (Figures 1B–E, 2E,F, 3B–E, 4E,F and Supplementary Figures 3B–E, 4E,F, 7B–E, 8E,F) followed by Tukey’s or Šídák’s multiple comparisons test as appropriate or an unpaired Student’s t-test; (Supplementary Figure 8B); non-parametric statistical analysis was performed by a Kruskal–Wallis test (Figures 2D, 4B,D and Supplementary Figures 2B,D, 4B,D) followed by Dunn’s multiple comparisons test or a Mann–Whitney U test (Supplementary Figures 8B,D). P-values are shown in the graphs for all values with p < 0.05 that was considered significant; p-values with p > 0.05 were considered as not significant and are abbreviated in the graphs as n.s.
Data availability statement
The original contributions presented in the study are included in the article/Supplementary material, further inquiries can be directed to the corresponding author.
Ethics statement
All animal procedures and experiments were conducted in accordance with the European Union Directive 2010/63/EU to reduce and minimize animal suffering and were reviewed and approved by the Lower Saxony State Office for Consumer Protection and Food Safety (33.19-42502-04-21/3827).
Author contributions
NL, LK, JP, RS, and TK performed the light-dark test under the supervision of JT and contributed to data analysis. JT conceived the project, analyzed the data, and wrote the manuscript together with RK. All authors contributed to the article and approved the submitted version.
Acknowledgments
We are grateful to Benjamin Judkewitz and Nahid Hakiy for providing us with and their advice on Danionella and to Paride Antinucci for providing us with crystal fish. We thank all members of the Köster group for discussions and their scientific input along this project, Janine Fichtner for a critical reading of the manuscript, and Timo Fritsch for excellent animal care. We acknowledge support by the Open Access Publication Funds of the Technische Universität Braunschweig.
Conflict of interest
The authors declare that the research was conducted in the absence of any commercial or financial relationships that could be construed as a potential conflict of interest.
Publisher’s note
All claims expressed in this article are solely those of the authors and do not necessarily represent those of their affiliated organizations, or those of the publisher, the editors and the reviewers. Any product that may be evaluated in this article, or claim that may be made by its manufacturer, is not guaranteed or endorsed by the publisher.
Supplementary material
The Supplementary Material for this article can be found online at: https://www.frontiersin.org/articles/10.3389/fnbeh.2022.885775/full#supplementary-material
Supplementary Figure 1 | (Related to Figures 2A–D). Different startle responses evoked by illumination changes in zebrafish and Dc larvae. (A) Startle responses ± SEM (shaded) of 6 dpf zebrafish AB wildtype (green; n = 60), crystal (blue; n = 60), and Dc (red; n = 40) larvae depicted from 2 s before (3,598 s) to 18 s after (3,618 s) the second light (yellow) to dark (gray) switch; a dotted black rectangle indicates the 1 s time interval that was used to compare the velocity of the larvae in (B). Note the similar responses of AB, crystal, and Dc larvae compared to the first light to dark switch depicted in Figure 2A. (B) Violin plots depicting the velocity of AB, crystal, and Dc larvae during 1 s (3,600–3,601 s) following the second light to dark switch (see also Figure 2B). (C) Startle responses of 6 dpf zebrafish AB wildtype (green), crystal (blue), and Dc (red) larvae depicted from 2 s before (4,198 s) to 18 s after (4,218 s) the second dark (gray) to light (yellow) switch; a dotted black rectangle indicates the 1 s time interval that was used to compare the velocity of the larvae in (D). Note the similar responses of AB, crystal, and Dc larvae compared to the first dark to light switch depicted in Figure 2C. (D) Violin plots depicting the velocity of AB, crystal, and Dc larvae during 1 s (4,202–4,203 s) following the second dark to light switch (see also Figure 2D). One-way ANOVA followed by Tukey’s multiple comparisons test or Kruskal–Wallis test followed by Dunn’s multiple comparisons test was used to analyze differences in velocity between AB, crystal, and Dc; p > 0.05 is abbreviated as not significant (n.s.).
Supplementary Figure 2 | (Related to Figures 4A–D). Age-dependent startle responses evoked by illumination changes in 4–6 dpf Dc larvae. (A) Startle responses with standard error of the mean (SEM; shaded area) of 4 dpf (green, n = 32), 5 dpf (blue, n = 28), and 6 dpf (red, n = 40) Dc larvae depicted from 2 s before (3,598 s) to 18 s after (3,618 s) the second light to dark switch (compare with Figure 4A); a dotted black rectangle indicates the 1 s time interval that was used to compare the velocity of the larvae in (B). (B) Violin plots depicting the velocity of 4–6 dpf Dc larvae during 1 s (3,600–3,601 s) following the second light to dark switch (compare with Figure 4B). (C) Startle responses of 4–6 dpf Dc larvae depicted 2 s before (4,198 s) and 18 s after (4,218 s) the second dark (gray) to light (yellow) switch; a dotted black rectangle indicates the 1 s time interval that was used to compare the velocity of the larvae in (D). (D) Violin plots depicting the velocity of 4–6 dpf Dc larvae during 1 s (4,202–4,203 s) following the second dark to light switch. Kruskal–Wallis test followed by Dunn’s multiple comparisons test was used to analyze differences in velocity between 4 and 6 dpf Dc; p > 0.05 is abbreviated as not significant (n.s.).
Supplementary Figure 3 | Locomotor activity of 4–6 dpf AB larvae in the light-dark test. (A) Locomotor activity of 4 dpf (green; n = 48), 5 dpf (blue; n = 48), and 6 dpf (red; n = 60) AB larvae in the light-dark test; color-coded arrowheads highlight the increases in locomotor activity 1 s after the illumination switch. (B,C) Violin plots of the velocity during movement (B) and the time spent moving (C) for 4–6 dpf AB larvae in the habituation (red) and swimming (blue) phase. (D,E) Violin plots of the velocity during movement (D) and the time spent moving (E) for 4–6 dpf AB larvae in the light (yellow) and dark (gray) phases. Note the reduced velocity (B,D) and time spent moving (C,E) of 4 dpf particularly during the habituation, swimming and light phases relative to 5 and 6 dpf AB larvae. Two-way ANOVA followed by Šídák’s or Tukey’s multiple comparisons test was used to analyze differences in velocity or movement between phases of the light-dark test in and between 4 and 6 dpf AB; p > 0.05 is abbreviated as not significant (n.s.).
Supplementary Figure 4 | Age-dependent startle responses evoked by illumination changes and age-independent thigmotaxis in 4–6 dpf AB larvae. (A) Startle responses with standard error of the mean (SEM; shaded area) of 4 dpf (green, n = 48), 5 dpf (blue, n = 48), and 6 dpf (red, n = 60) AB larvae depicted from 2 s before (2,398 s) to 18 s after (2,418 s) the first light to dark switch (see also Supplementary Figure 5A); a dotted black rectangle indicates the 1 s time interval that was used to compare the velocity of the larvae in (B). (B) Violin plots depicting the velocity of 4–6 dpf AB larvae during 1 s (2,400–2,401 s) following the first light to dark switch (see also Supplementary Figure 5B). (C) Startle responses of 4–6 dpf AB larvae depicted 2 s before (2,998 s) and 18 s after (3,018 s) the first dark (gray) to light (yellow) switch; (see also Supplementary Figure 5C); a dotted black rectangle indicates the 1 s time interval that was used to compare the velocity of the larvae in (D). (D) Violin plots depicting the velocity of 4–6 dpf AB larvae during 1 s (3,000–3,001 s) following the first dark to light switch (see also Supplementary Figure 5D). Note the relatively similar startle responses of 5 and 6 dpf AB larvae during the illumination changes. Kruskal–Wallis test followed by Dunn’s multiple comparisons test was used to analyze differences in velocity between 4 and 6 dpf AB; p > 0.05 is abbreviated as not significant (n.s.). (E,F) Violin plots depicting the time spent in the outer zone of the wells show age-independent levels of thigmotaxis in 4–6 dpf AB throughout all phases of the test and a significant decrease during the dark phases in 4 dpf (E). Two-way ANOVA followed by Šídák’s or Tukey’s multiple comparisons test was used to analyze differences in thigmotaxis between phases of the light-dark test in and between 4 and 6 dpf AB; p > 0.05 is abbreviated as not significant (n.s.).
Supplementary Figure 5 | (Related to Supplementary Figures 4A–D). Age-dependent startle responses evoked by illumination changes in 4–6 dpf AB larvae. (A) Startle responses with standard error of the mean (SEM; shaded area) of 4 dpf (green, n = 48), 5 dpf (blue, n = 48), and 6 dpf (red, n = 60) AB larvae depicted from 2 s before (3,598 s) to 18 s after (3,618 s) the second light to dark switch (compare with Supplementary Figure 4A); a dotted black rectangle indicates the 1 s time interval that was used to compare the velocity of the larvae in (B). (B) Violin plots depicting the velocity of 4–6 dpf AB larvae during 1 s (3,600–3,601 s) following the second light to dark switch (compare with Supplementary Figure 4B). (C) Startle responses of 4–6 dpf AB larvae depicted 2 s before (4,198 s) and 18 s after (4,218 s) the second dark (gray) to light (yellow) switch; a dotted black rectangle indicates the 1 s time interval that was used to compare the velocity of the larvae in (D). (D) Violin plots depicting the velocity of 4–6 dpf AB larvae during 1 s (4,200–4,201 s) following the second dark to light switch (see also Supplementary Figure 4D). Kruskal–Wallis test followed by Dunn’s multiple comparisons test was used to analyze differences in velocity between 4 and 6 dpf AB; p > 0.05 is abbreviated as not significant (n.s.).
Supplementary Figure 6 | Rosetta-like locomotor activity patterns in 4 dpf Dc. Some 4 dpf Dc swim in what resembles concentric-like pathways (black) during the swimming (A), first dark (B), and first light (C) phase, resulting in Rosetta-like structures when depicted in a 300 s time interval; examples in (A–C) are from 3 different larvae. Such a peculiar locomotor activity was not observed in 4 dpf AB larvae [D; example pathway (black) during the swimming phase with a 300 s time interval as in A–C]. Each arena consist of a center (yellow) and outer (magenta) zone that are equal in area.
Supplementary Figure 7 | Locomotor activity of 5 and 6 dpf crystal larvae in the light-dark test. (A) Locomotor activity of 5 dpf (green; n = 25) and 6 dpf (blue; n = 60) crystal larvae in the light-dark test; color-coded arrowheads highlight the increases in locomotor activity 1 s after the illumination switch. (B,C) Violin plots of the velocity during movement (B) and the time spent moving (C) for 5–6 dpf crystal larvae in the habituation (red) and swimming (blue) phase. (D,E) Violin plots of the velocity during movement (D) and the time spent moving (E) for 5–6 dpf crystal larvae in the light (yellow) and dark (gray) phases. Note the reduced time spent moving (C,E) of 5 dpf particularly during the habituation, swimming and light phases relative to 6 dpf crystal larvae. Two-way ANOVA followed by Šídák’s or Tukey’s multiple comparisons test was used to analyze differences in velocity or movement between phases of the light-dark test in and between 5 and 6 dpf crystal; p > 0.05 is abbreviated as not significant (n.s.).
Supplementary Figure 8 | Startle responses evoked by illumination changes and thigmotaxis in 5 and 6 dpf crystal larvae. (A) Startle responses with standard error of the mean (SEM; shaded area) of 5 dpf (green, n = 25) and 6 dpf (blue, n = 60) crystal larvae depicted from 2 s before (2,398 s) to 18 s after (2,418 s) the first light to dark switch; a dotted black rectangle indicates the 1 s time interval that was used to compare the velocity of the larvae in (B). (B) Violin plots depicting the velocity of 5 and 6 dpf crystal larvae during 1 s (2,400–2,401 s) following the first light (blue) to dark (gray) switch. (C) Startle responses of 5 and 6 dpf crystal larvae depicted 2 s before (2,998 s) and 18 s after (3,018 s) the first dark (gray) to light (yellow) switch; a dotted black rectangle indicates the 1 s time interval that was used to compare the velocity of the larvae in (D). (D) Violin plots depicting the velocity of 5 and 6 dpf crystal larvae during 1 s (3,000–3,001 s) following the first dark to light switch. Student’s t or Mann–Whitney U test was used to analyze differences in velocity between 5 and 6 dpf crystal; p > 0.05 is abbreviated as not significant (n.s.). (E,F) Violin plots depicting the time spent in the outer zone of the wells show age-independent levels of thigmotaxis in 5 and 6 dpf crystal in all except the light phases of the test. Two-way ANOVA followed by Šídák’s or Tukey’s multiple comparisons test was used to analyze differences in thigmotaxis between phases of the light-dark test in and between 5 and 6 dpf crystal; p > 0.05 is abbreviated as not significant (n.s.).
Supplementary Figure 9 | (Related to Supplementary Figures 8A–D). Startle responses evoked by illumination changes in 5 and 6 dpf crystal larvae. (A) Startle responses with standard error of the mean (SEM; shaded area) of 5 dpf (green, n = 25) and 6 dpf (blue, n = 60) crystal larvae depicted from 2 s before (3,598 s) to 18 s after (3,618 s) the second light to dark switch (compare with Supplementary Figure 8A); a dotted black rectangle indicates the 1 s time interval that was used to compare the velocity of the larvae in (B). (B) Violin plots depicting the velocity of 5 and 6 dpf crystal larvae during 1 s (3,600–3,601 s) following the second light to dark switch (compare with Supplementary Figure 8B). (C) Startle responses of 5 and 6 dpf crystal larvae depicted 2 s before (4,198 s) and 18 s after (4,218 s) the second dark (gray) to light (yellow) switch; a dotted black rectangle indicates the 1 s time interval that was used to compare the velocity of the larvae in (D). (D) Violin plots depicting the velocity of 5 and 6 dpf crystal larvae during 1 s (4,200–4,201 s) following the second dark to light switch. Mann–Whitney U test was used to analyze differences in velocity between 5 and 6 dpf crystal; p > 0.05 is abbreviated as not significant (n.s.).
Supplementary Figure 10 | Body length of zebrafish and Dc larvae. (A) Violin plots depicting the body length of AB wildtype (green) and crystal (blue) zebrafish, and Dc (red) at 6 dpf show that both species are similar in size at this developmental age. (B) Violin plots depicting the body length of 4–6 dpf Dc show that 4 dpf (green) are significantly smaller than 5 dpf (blue) and 6 dpf (red) larvae. One-way ANOVA followed by Tukey’s multiple comparisons test was used to analyze differences in body length between AB, crystal, and Dc, and 4–6 dpf Dc; p > 0.05 is abbreviated as not significant (n.s.).
Footnotes
References
Abreu, M. S., Maximino, C., Banha, F., Anastácio, P. M., Demin, K. A., Kalueff, A. V., et al. (2020). Emotional behavior in aquatic organisms? lessons from crayfish and zebrafish. J. Neurosci. Res. 98, 764–779. doi: 10.1002/jnr.24550
Ahrens, M. B., and Engert, F. (2015). Large-scale imaging in small brains. Curr. Opin. Neurobiol. 32C, 78–86. doi: 10.1016/j.conb.2015.01.007
Ahrens, M. B., Orger, M. B., Robson, D. N., Li, J. M., and Keller, P. J. (2013). Whole-brain functional imaging at cellular resolution using light-sheet microscopy. Nat. Methods 10, 413–420. doi: 10.1038/nmeth.2434
Aleström, P., D’Angelo, L., Midtlyng, P. J., Schorderet, D. F., Schulte-Merker, S., Sohm, F., et al. (2019). Zebrafish: housing and husbandry recommendations. Lab. Anim. 54, 213–224. doi: 10.1177/0023677219869037
Antinucci, P., and Hindges, R. (2016). A crystal-clear zebrafish for in vivo imaging. Sci. Rep. 6:29490. doi: 10.1038/srep29490
Audira, G., Siregar, P., Strungaru, S.-A., Huang, J.-C., and Hsiao, C.-D. (2020). Which zebrafish strains are more suitable to perform behavioral studies? a comprehensive comparison by phenomic approach. Biology 9:200. doi: 10.3390/biology9080200
Besson, M., and Martin, J. (2005). Centrophobism/thigmotaxis, a new role for the mushroom bodies in Drosophila. J. Neurobiol. 62, 386–396. doi: 10.1002/neu.20111
Britz, R., Conway, K. W., and Rüber, L. (2009). Spectacular morphological novelty in a miniature cyprinid fish. Danionella dracula n. sp. Proc. R. Soc. B Biol. Sci. 276, 2179–2186. doi: 10.1098/rspb.2009.0141
Britz, R., Conway, K. W., and Rüber, L. (2021). The emerging vertebrate model species for neurophysiological studies is Danionella cerebrum, new species (Teleostei: Cyprinidae). Sci. Rep. 11:18942. doi: 10.1038/s41598-021-97600-97600
Brun, N. R., Hage, P., van, Hunting, E. R., Haramis, A.-P. G., Vink, S. C., et al. (2019). Polystyrene nanoplastics disrupt glucose metabolism and cortisol levels with a possible link to behavioural changes in larval zebrafish. Commun. Biol. 2:382. doi: 10.1038/s42003-019-0629-626
Colwill, R. M., and Creton, R. (2011). Locomotor behaviors in zebrafish (Danio rerio) larvae. Behav. Process 86, 222–229. doi: 10.1016/j.beproc.2010.12.003
de Esch, C., Linde, H., van der, Slieker, R., Willemsen, R., Wolterbeek, A., et al. (2012). Locomotor activity assay in zebrafish larvae: influence of age, strain and ethanol. Neurotoxicol. Teratol. 34, 425–433. doi: 10.1016/j.ntt.2012.03.002
Denenberg, V. H. (1969). Open-Field behavior in the rat: what does it mean?*. Ann. Ny. Acad. Sci. 159, 852–859. doi: 10.1111/j.1749-6632.1969.tb12983.x
Dreosti, E., Lopes, G., Kampff, A. R., and Wilson, S. W. (2015). Development of social behavior in young zebrafish. Front. Neural Circuits 9:39. doi: 10.3389/fncir.2015.00039
Emran, F., Rihel, J., and Dowling, J. E. (2008). A behavioral assay to measure responsiveness of zebrafish to changes in light intensities. J. Vis. Exp. e923. doi: 10.3791/923
Fero, K., Yokogawa, T., and Burgess, H. A. (2010). “The behavioral repertoire of larval zebrafish,” in Zebrafish Models in Neurobehavioral, eds A. V. Kalueff and J. M. Cachat (Totowa, NJ: Humana Press:), 249–291. doi: 10.1007/978-1-60761-922-2_12
Fitzgerald, J. A., Kirla, K. T., Zinner, C. P., and vom Berg, C. M. (2019). Emergence of consistent intra-individual locomotor patterns during zebrafish development. Sci. Rep. 9:13647. doi: 10.1038/s41598-019-49614-y
García-González, J., Quadros, B., de, Havelange, W., Brock, A. J., and Brennan, C. H. (2021). Behavioral effects of developmental exposure to JWH-018 in wild-type and disrupted in schizophrenia 1 (disc1) mutant zebrafish. Biomolecules 11:319. doi: 10.3390/biom11020319
Gromer, D., Kiser, D. P., and Pauli, P. (2021). Thigmotaxis in a virtual human open field test. Sci. Rep. 11:6670. doi: 10.1038/s41598-021-85678-85675
Hall, C. S. (1934). Emotional behavior in the rat. I. defecation and urination as measures of individual differences in emotionality. J. Comp. Psychol. 18, 385–403. doi: 10.1037/h0071444
Hinsch, K., and Zupanc, G. K. H. (2007). Generation and long-term persistence of new neurons in the adult zebrafish brain: a quantitative analysis. Neuroscience 146, 679–696. doi: 10.1016/j.neuroscience.2007.01.071
Horstick, E. J., Mueller, T., and Burgess, H. A. (2016). Motivated state control in larval zebrafish: behavioral paradigms and anatomical substrates. J. Neurogenet. 30, 122–132. doi: 10.1080/01677063.2016.1177048
Huang, K.-H., Rupprecht, P., Frank, T., Kawakami, K., Bouwmeester, T., and Friedrich, R. W. (2020). A virtual reality system to analyze neural activity and behavior in adult zebrafish. Nat. Methods 17, 343–351. doi: 10.1038/s41592-020-0759-752
Ingebretson, J. J., and Masino, M. A. (2013). Quantification of locomotor activity in larval zebrafish: considerations for the design of high-throughput behavioral studies. Front. Neural Circuit 7:109. doi: 10.3389/fncir.2013.00109
Irons, T. D., MacPhail, R. C., Hunter, D. L., and Padilla, S. (2010). Acute neuroactive drug exposures alter locomotor activity in larval zebrafish. Neurotoxicol. Teratol. 32, 84–90. doi: 10.1016/j.ntt.2009.04.066
Kenney, J. W., Steadman, P. E., Young, O., Shi, M. T., Polanco, M., Dubaishi, S., et al. (2021). A 3D adult zebrafish brain atlas (AZBA) for the digital age. eLife 10:e69988. doi: 10.7554/elife.69988
Lange, M., Neuzeret, F., Fabreges, B., Froc, C., Bedu, S., Bally-Cuif, L., et al. (2013). Inter-individual and inter-strain variations in zebrafish locomotor ontogeny. PLoS One 8:e70172. doi: 10.1371/journal.pone.0070172
MacPhail, R. C., Brooks, J., Hunter, D. L., Padnos, B., Irons, T. D., and Padilla, S. (2008). Locomotion in larval zebrafish: influence of time of day, lighting and ethanol. Neurotoxicology 30, 52–58. doi: 10.1016/j.neuro.2008.09.011
Mattern, K., von Trotha, J. W., Erfle, P., Köster, R. W., and Dietzel, A. (2020). NeuroExaminer: an all-glass microfluidic device for whole-brain in vivo imaging in zebrafish. Commun. Biol. 3:311. doi: 10.1038/s42003-020-1029-1027
Maximino, C., Brito, T. M., de, Batista, A. W., da, S., Herculano, A. M., et al. (2010). Measuring anxiety in zebrafish: a critical review. Behav. Brain Res. 214, 157–171. doi: 10.1016/j.bbr.2010.05.031
Mohammad, F., Aryal, S., Ho, J., Stewart, J. C., Norman, N. A., Tan, T. L., et al. (2016). Ancient anxiety pathways influence drosophila defense behaviors. Curr. Biol. 26, 981–986. doi: 10.1016/j.cub.2016.02.031
Padilla, S., Hunter, D. L., Padnos, B., Frady, S., and MacPhail, R. C. (2011). Assessing locomotor activity in larval zebrafish: influence of extrinsic and intrinsic variables. Neurotoxicol. Teratol. 33, 624–630. doi: 10.1016/j.ntt.2011.08.005
Parichy, D. M. (2015). Advancing biology through a deeper understanding of zebrafish ecology and evolution. eLife 4:e05635. doi: 10.7554/elife.05635
Parichy, D. M., Elizondo, M. R., Mills, M. G., Gordon, T. N., and Engeszer, R. E. (2009). Normal table of postembryonic zebrafish development: staging by externally visible anatomy of the living fish. Dev. Dynam. 238, 2975–3015. doi: 10.1002/dvdy.22113
Penalva, A., Bedke, J., Cook, E. S. B., Barrios, J. P., Bertram, E. P. L., and Douglass, A. D. (2018). Establishment of the miniature fish species Danionella translucida as a genetically and optically tractable neuroscience model. bioRxiv [prperint] doi: 10.1101/444026
Pietri, T., Roman, A.-C., Guyon, N., Romano, S. A., Washbourne, P., Moens, C. B., et al. (2013). The first mecp2-null zebrafish model shows altered motor behaviors. Front. Neural Circuits 7:118. doi: 10.3389/fncir.2013.00118
Prober, D. A., Rihel, J., Onah, A. A., Sung, R.-J., and Schier, A. F. (2006). Hypocretin/orexin overexpression induces an insomnia-like phenotype in zebrafish. J. Neurosci. 26, 13400–13410. doi: 10.1523/jneurosci.4332-06.2006
Prut, L., and Belzung, C. (2003). The open field as a paradigm to measure the effects of drugs on anxiety-like behaviors: a review. Eur. J. Pharmacol. 463, 3–33. doi: 10.1016/s0014-2999(03)01272-x
Rajan, G., Duroure, K., and Del Bene, F. (2022a). “Danionella translucida, a tankful of new opportunities,” in Laboratory Fish in Biomedical Research, eds L. D’Angelo and P. de Girolamo (Cambridge, MA: Academic Press), 409–418. doi: 10.1016/b978-0-12-821099-4.00017-1
Rajan, G., Lafaye, J., Faini, G., Carbo-Tano, M., Duroure, K., Tanese, D., et al. (2022b). Evolutionary divergence of locomotion in two related vertebrate species. Cell Rep. 38:110585. doi: 10.1016/j.celrep.2022.110585
Randlett, O., Wee, C. L., Naumann, E. A., Nnaemeka, O., Schoppik, D., Fitzgerald, J. E., et al. (2015). Whole-brain activity mapping onto a zebrafish brain atlas. Nat. Methods 12, 1039–1046. doi: 10.1038/nmeth.3581
Richendrfer, H., Pelkowski, S. D., Colwill, R. M., and Creton, R. (2012). On the edge: pharmacological evidence for anxiety-related behavior in zebrafish larvae. Behav. Brain Res. 228, 99–106. doi: 10.1016/j.bbr.2011.11.041
Roberts, T. R. (1986). Danionella translucida, a new genus and species of cyprinid fish from Burma, one of the smallest living vertebrates. Environ. Biol. Fish 16, 231–241. doi: 10.1007/bf00842977
Santacà, M., Agrillo, C., Petrazzini, M. E. M., and Bisazza, A. (2020a). The ontogeny of continuous quantity discrimination in zebrafish larvae (Danio rerio). Anim. Cogn. 23, 731–739. doi: 10.1007/s10071-020-01384-1381
Santacà, M., Caja, T., Petrazzini, M. E. M., Agrillo, C., and Bisazza, A. (2020b). Size discrimination in adult zebrafish (Danio rerio): normative data and individual variation. Sci. Rep. 10:1164. doi: 10.1038/s41598-020-57813-57811
Santacà, M., Dadda, M., Valle, L. D., Fontana, C., Gjinaj, G., and Bisazza, A. (2022). Learning and visual discrimination in newly hatched zebrafish. iscience 25:104283. doi: 10.1016/j.isci.2022.104283
Schnörr, S. J., Steenbergen, P. J., Richardson, M. K., and Champagne, D. L. (2012). Measuring thigmotaxis in larval zebrafish. Behav. Brain Res. 228, 367–374. doi: 10.1016/j.bbr.2011.12.016
Schulze, L., Henninger, J., Kadobianskyi, M., Chaigne, T., Faustino, A. I., Hakiy, N., et al. (2018). Transparent Danionella translucida as a genetically tractable vertebrate brain model. Nat. Methods 15, 977–983. doi: 10.1038/s41592-018-0144-146
Stednitz, S. J., and Washbourne, P. (2020). Rapid progressive social development of zebrafish. Zebrafish 17, 11–17. doi: 10.1089/zeb.2019.1815
Tang, K. L., Agnew, M. K., Hirt, M. V., Sado, T., Schneider, L. M., Freyhof, J., et al. (2010). Systematics of the subfamily danioninae (Teleostei: Cypriniformes: Cyprinidae). Mol. Phylogenet. Evol. 57, 189–214. doi: 10.1016/j.ympev.2010.05.021
Treit, D., and Fundytus, M. (1988). Thigmotaxis as a test for anxiolytic activity in rats. Pharmacol. Biochem. Be 31, 959–962. doi: 10.1016/0091-3057(88)90413-90413
Valente, A., Huang, K. H., Portugues, R., and Engert, F. (2012). Ontogeny of classical and operant learning behaviors in zebrafish. Learn. Memory 19, 170–177. doi: 10.1101/lm.025668.112
van den Bos, R., Mes, W., Galligani, P., Heil, A., Zethof, J., Flik, G., et al. (2017). Further characterisation of differences between TL and AB zebrafish (Danio rerio): gene expression, physiology and behaviour at day 5 of the larval stage. PLoS One 12:e0175420. doi: 10.1371/journal.pone.0175420
Vanwalleghem, G. C., Ahrens, M. B., and Scott, E. K. (2018). Integrative whole-brain neuroscience in larval zebrafish. Curr. Opin. Neurobiol. 50, 136–145. doi: 10.1016/j.conb.2018.02.004
Walz, N., Mühlberger, A., and Pauli, P. (2016). A human open field test reveals thigmotaxis related to agoraphobic fear. Biol. Psychiat. 80, 390–397. doi: 10.1016/j.biopsych.2015.12.016
White, R. M., Sessa, A., Burke, C., Bowman, T., LeBlanc, J., Ceol, C., et al. (2008). Transparent adult zebrafish as a tool for in vivo transplantation analysis. Cell Stem Cell 2, 183–189. doi: 10.1016/j.stem.2007.11.002
Xu, J., and Guo, S. (2020). “Molecular genetic approaches to dissect complex behaviors in zebrafish,” in Behavioral and Neural Genetics of Zebrafish, ed. R. T. Gerlai (Cambridge, MA: Academic Press), 223–244. doi: 10.1016/b978-0-12-817528-6.00014-0
Yang, W., Meng, Y., Li, D., and Wen, Q. (2019). Visual contrast modulates operant learning responses in larval zebrafish. Front. Behav. Neurosci. 13:4. doi: 10.3389/fnbeh.2019.00004
Keywords: Danionella cerebrum, Danio rerio (zebrafish), crystal, locomotor activity, light-dark test
Citation: Lindemann N, Kalix L, Possiel J, Stasch R, Kusian T, Köster RW and von Trotha JW (2022) A comparative analysis of Danionella cerebrum and zebrafish (Danio rerio) larval locomotor activity in a light-dark test. Front. Behav. Neurosci. 16:885775. doi: 10.3389/fnbeh.2022.885775
Received: 28 February 2022; Accepted: 05 July 2022;
Published: 04 August 2022.
Edited by:
Giorgio Vallortigara, University of Trento, ItalyReviewed by:
Maria Elena Miletto Petrazzini, University of Padua, ItalyAndrea Messina, University of Trento, Italy
Copyright © 2022 Lindemann, Kalix, Possiel, Stasch, Kusian, Köster and von Trotha. This is an open-access article distributed under the terms of the Creative Commons Attribution License (CC BY). The use, distribution or reproduction in other forums is permitted, provided the original author(s) and the copyright owner(s) are credited and that the original publication in this journal is cited, in accordance with accepted academic practice. No use, distribution or reproduction is permitted which does not comply with these terms.
*Correspondence: Jakob William von Trotha, j.von-trotha@tu-braunschweig.de
†These authors have contributed equally to this work