- 1Center for Brain Repair and Rehabilitation, Institute of Neuroscience and Physiology, University of Gothenburg, Gothenburg, Sweden
- 2Division of Neonatology, Center for Maternal-Neonatal Care, Nagoya University Hospital, Nagoya, Japan
- 3Department of Obstetrics and Gynecology, Narita Hospital, Nagoya, Japan
- 4Institute of Neuroscience and Physiology, University of Gothenburg, Gothenburg, Sweden
- 5Department of Obstetrics and Gynecology, Mie University, Tsu, Japan
- 6Department of Pediatrics, The Third Affiliated Hospital of Zhengzhou University, Zhengzhou, China
- 7Department of Pediatric Oncology, Karolinska University Hospital, Stockholm, Sweden
- 8Department of Women's and Children's Health, Karolinska Institutet, Stockholm, Sweden
The pool of neural stem and progenitor cells (NSPCs) in the dentate gyrus of the hippocampus is reduced by ionizing radiation. This explains, at least partly, the learning deficits observed in patients after radiotherapy, particularly in pediatric cases. An 8 Gy single irradiation dose was delivered to the whole brains of postnatal day 9 (P9) C57BL/6 mice, and BrdU-labeled, syngeneic NSPCs (1.0 × 105 cells/injection) were grafted into each hippocampus on P21. Three months later, behavior tests were performed. Irradiation impaired novelty-induced exploration, place learning, reversal learning, and sugar preference, and it altered the movement pattern. Grafting of NSPCs ameliorated or even normalized the observed deficits. Less than 4% of grafted cells survived and were found in the dentate gyrus 5 months later. The irradiation-induced loss of endogenous, undifferentiated NSPCs in the dentate gyrus was completely restored by grafted NSPCs in the dorsal, but not the ventral, blade. The grafted NSPCs did not exert appreciable effects on the endogenous NSPCs; however, more than half of the grafted NSPCs differentiated. These results point to novel strategies aimed at ameliorating the debilitating late effects of cranial radiotherapy, particularly in children.
Introduction
The second most common type of childhood cancer is brain tumors, amounting to nearly one-third of all childhood cancers. The survival rates of pediatric brain tumor patients has improved in recent decades, and currently more than 80% of them survive (1). Although neurosurgery techniques and chemotherapy regimens have improved, radiation therapy (RT) is still an essential treatment modality not only for malignant brain tumors, but also for central nervous system (CNS) leukemia/lymphoma. However, RT causes both increased mortality and morbidity, in survivors of pediatric brain tumors (2). Cognitive impairments, as well as perturbed growth and puberty are some of the known late effects observed after RT. Moreover, it has been shown that the cognitive deficits are more severe in younger children after RT (3, 4), and the deficits increase progressively over time. It is unclear whether low doses of ionizing radiation (less than 0.5 Gy) administered to the CNS can cause cognitive impairment. One study claimed to find such effects (5), whereas a similar but larger study failed to do so (6). Ameliorating the late effects of RT will improve the quality of life of cancer survivors, whose prevalence is increasing, and particularly of pediatric cases, whose remaining life expectancy is long.
Irradiation (IR) causes injuries to many brain regions and cell types; however, the underlying pathogenesis is not clear. Neurogenesis persists throughout life in two regions, the subventricular zone (SVZ) and the dentate gyrus of the hippocampus. These regions harbor proliferating cells, and are therefore particularly susceptible to IR (7). Several reports suggest that injury to neural stem and progenitor cells (NSPCs) in the hippocampus can cause some of the late effects observed after IR (8–10), and the depletion of NSPCs induced by IR appears to be long-lasting, even after a single, moderate dose of IR (11, 12). The depletion is even aggravated over time (13). Currently, there are no established interventions after RT, but it was shown that memory training improved the attention and memory performance of children treated for medulloblastoma (14). Voluntary physical exercise increases the number of stem cells and enhances neurogenesis after IR of the young mouse brain, and at least partly normalizes IR-induced behavior changes (8, 15). In one study, human embryonic stem cells grafted into the hippocampus of adult immune-deficient rats improved their performance in a memory task after IR (16). On the other hand, grafting of syngeneic enteric neural stem cells (17) or syngeneic cerebral neural stem cells (18) into the hippocampus of irradiated mice caused local gliosis. Given the importance of inflammation-related signaling in the brain under both normal and pathological conditions, the aim of the present study was to explore whether grafting NSPCs into the hippocampus of immune-competent mice could ameliorate the deficits observed after IR at a young age in the absence of immunosuppressive treatment.
Materials and Methods
Animals
All animal experimental protocols in the present study were approved by the Gothenburg committee of the Swedish Animal Welfare Agency (326-2009). C57BL/6J male pups with dams were purchased from Charles River (Sulzfeld, Germany) and maintained under a 12-h light/dark cycle with access to food and water ad libitum.
Six litters (6 pups/litter), in total 36 mice, were used for the study. Among them, 26 mice received IR on postnatal day 9 (P9). Three mice died during the IR procedure. Twenty-three irradiated mice were allocated into the NSPC (n = 13) or vehicle (n = 10) groups. All mice in the NSPC group were grafted with NSPCs and all in the vehicle group were injected with vehicle. However, 3 of 13 NSPC-grafted mice were excluded from analyses after histological evaluations as they were not grafted correctly (the cells were accidentally injected outside the hippocampus). All non-irradiated mice (n=10) were allocated to the non-irradiated group and injected with vehicle. One IR NSPC mouse died during the IC chip insertion procedure, and one vehicle-treated mouse died before behavioral tests. Therefore, IntelliCage® was started with 13 NSPC-treated mice, 9 vehicle-treated mice and 10 non-irradiated mice. In the “Introduction 2” section in IntelliCage® (Supplementary Figure 2), when doors were closed, 1 NSPC-treated mouse and 2 vehicle-treated mice had not been able to learn how to do a “nosepoke” by the end of the section, and could hence not drink water. Therefore, these three mice were excluded from the “First corner” section. As 2 vehicle-treated mice died between the end of the IntelliCage® and the movement pattern analysis, the subsequent two behavioral tests were performed with 12 NSPC-treated mice, 7 vehicle-treated mice and 10 non-irradiated mice. One non-irradiated mouse died, and the sections for histological evaluations were made from the remaining mice (12 NSPC-treated mice, 7 vehicle-treated mice and 9 non-irradiated mice).
Experimental Procedures
IR was administered to P9 C57BL/6J mice, and NSPCs derived from the same mouse strain were injected into each hippocampus (in the right and left hemispheres) at P21. IR-Vehicle- treated mice received irradiation and were injected with vehicle instead of NSPCs. Non IR- Vehicle mice were only anesthetized, without irradiation, and received injections of vehicle (Supplementary Figure 1). Three months after grafting, the behavior of the mice was examined using three tests: IntelliCage®(P110-129), Movement pattern analysis (P162) and Sugar water (anhedonia) test (P166-170) (Supplementary Figure 1). After these behavioral tests, mice were sacrificed (P173, 5 months after grafting) (Supplementary Figure 1).
Irradiation Procedure
The IR procedure was performed as described earlier (18, 19). For IR, a linear accelerator (Varian Clinac 600C/D) with 4-MV nominal photon energy and a dose rate of 2.3 Gy/min was used. Nine-day-old mice were anesthetized with an intraperitoneal injection of tribromoethanol (Sigma-Aldrich, Stockholm, Sweden), placed in a prone position (head to gantry) on an expanded polystyrene bed. Both cerebral hemispheres of each animal were irradiated with a 2 × 2-cm asymmetrical radiation field. The source-to-skin distance was ~99.5 cm. The head was covered with a 1-cm tissue equivalent. A single absorbed dose of 8 Gy was administered to each mouse. Dose variation within the target volume was estimated to be ±5%. The entire procedure was completed within 10 min. After IR, pups were returned to their biological dams until weaning. Sham control animals were anesthetized but not subjected to IR. Using the LQ-model (20) and an α:β ratio of 3 for late effects in the normal brain tissue, an acute exposure of 8 Gy is equivalent to approximately 18 Gy when delivered in repeated 2-Gy fractions (21). This represents a clinically relevant dose, equivalent to the total dose used in treatment protocols for prophylactic cranial IR in selected cases of childhood acute lymphatic leukemia.
Culture of NSPCs
NSPCs were kindly provided by Prof. Fred H. Gage (22), which were isolated from the whole brain of adult mice, except the cerebellum or the olfactory bulbs. NSPCs were cultured as described previously (18). Briefly, NSPCs were cultured and expanded as a monolayer in Dulbecco's Modified Eagle medium (DMEM)/nutrient mixture F-12 (1:1) (Invitrogen, San Diego, CA, USA) containing 1% N2 (Invitrogen), 20 ng/mL epidermal growth factor (Sigma-Aldrich, Saint Louis, MO, USA), 20 ng/mL basic fibroblast growth factor-2 (bFGF; BD Biosciences), and 5 μg/mL heparin (Sigma-Aldrich). Two days before grafting, BrdU was added to the medium at a final concentration of 1.25 μM. After collecting and washing, cells were suspended in DMEM containing 300 μg bFGF for grafting. More than 95% of the cells were viable as judged by a trypan blue exclusion test and more than 90% were BrdU-positive, as judged by immunostaining before grafting.
NSPC Grafting
NSPC grafting was performed as described previously (18). Mice were mounted onto a stereotactic head holder (Kopf Instruments, Tujunga, CA, USA) under anesthesia with isoflurane (Isoba® vet; Schering-Plough Corp., NJ, USA; 5% for induction, and 2% to 3% for maintenance) in the flat skull position. In order to graft NSPCs into the hippocampus, a 5-μL 26 gauge syringe (Innovative Labor Systeme, Stuetzerbach, Germany) attached to the holder was inserted according to the following coordinates: body weight < 9 g: 0.45 × (distance from lambda to bregma) mm posterior and ± 1.2 mm lateral to bregma, 3.0 mm deep from the skull surface; body weight ≥ 9 g, 0.42 × (distance from lambda to bregma) mm posterior and ± 1.3 mm lateral to bregma, 3.2 mm deep from the skull surface. Before the needle was inserted, a small hole was drilled in the proper position according to the above coordinates. Then, 1 × 105 NSPCs in 2 μL DMEM were injected very slowly over a 2-min period, with a 4-min delay prior to removal of the syringe, and 2 min were allowed for syringe removal. No immunosuppressive drugs were administered.
Tissue Preparation
Mice were deeply anesthetized at P173 and intracardially perfusion-fixed using 0.9% NaCl followed by buffered formaldehyde (Histofix, Histolab, Göteborg, Sweden). Brains were removed and immersion-fixed in the same solution at 4°C for 24 h, and then, immersed in 30% sucrose for at least 2 days. Brains were cut sagittally at 30 μm on a sliding microtome in dry ice.
Microscopy and Immunohistochemistry
The following antibodies and final dilutions were used: anti-BrdU (1:500; AbD Seortec, Martinsried, Germany), goat anti-Sox2 (1:200; Santa Cruz Biotechnology, Santa Cruz, CA, USA), and rabbit anti-S100b (1:1000; Swant, Bellinzona, Switzerland). Immunoperoxidase detection of BrdU was performed as follows: free-floating sections were rinsed in Tris-buffered saline (TBS; 0.1 M Tris–HCl, pH 7.4/0.9% NaCl), and sections were then treated with 0.6% H2O2/TBS for 30 min, followed by incubation for 2 h in 50% formamide/2 × SSC (0.3 M NaCl, 0.03 M sodium citrate) at 65°C, rinsed in 2 × SSC, incubated for 30 min in 2 N HCl at 37°C, and rinsed in 0.1 M boric acid (pH 8.5). Incubation in TBS/3% donkey serum/0.1% Triton X (TBS++) for 30 min was followed by overnight incubation with mouse anti-BrdU. After rinsing in TBS, sections were incubated for 1 h with donkey anti-mouse-biotin (1:1,000 biotinylated donkey anti-mouse, Jackson ImmunoResearch Laboratories, West Grove, PA, USA) and then with avidin-biotin-peroxidase complex (Vectastain ABC Elite Kit, Vector Laboratories, Burlingame, CA, USA). This was followed by peroxidase detection for 5 min (0.25 mg/mL DAB, 0.01% H2O2, 0.04% NiCl). Immunoperoxidase detection of Sox2 was performed in the same manner with the proper secondary antibody, donkey anti-goat-biotin (1:1,000, Jackson ImmunoResearch Laboratories) as that of BrdU, except treatments for the formamide and HCl.
Triple immunofluorescence was performed as follows: free-floating sections were rinsed in TBS, and sections were incubated for 30 min in 2 N HCl at 37°C and rinsed in 0.1 M boric acid (pH 8.5). After several rinses in TBS, sections were incubated in TBS++ for 30 min, followed by a primary antibody cocktail containing rat anti-BrdU, goat anti-Sox2 and rabbit anti-S100b, for 24 h at 4°C. Sections were then rinsed in TBS, incubated with a cocktail of fluorochrome-labeled secondary antibodies for 2 h (1:1,000 donkey anti-rat Alexa 488, donkey anti-goat Alexa 546 and donkey anti-rabbit Alexa 647, Invitrogen Corporation, Carlsbad, CA, USA), rinsed again in TBS, and mounted on glass slides.
Stereological Quantification of Cells
In each animal, every 12th section (typically 6 sections) containing a dorsal hippocampus was used to determine the total number of BrdU- and Sox2-positive cells in the granule cell layer (GCL) under light microscopy. These numbers were counted using stereology software (StereoInvestigator, version 6; MBF Bioscience, Williston, VT, USA). Cell counts were then multiplied with the series factor (12) to determine the total number of cells per GCL. In triple immunofluorescence, the percentage of each positive cell type (≥50 BrdU- or Sox2 -positive cells per animal) was assessed using a confocal microscope (Leica TCS SP2; Leica Microsystems, Wetzlar, Germany). Resulting percentages of each positive cell type were multiplied with the absolute number of BrdU- or Sox2-positive cells to calculate the absolute number of each cell type (23). GCL volume in P173 mice was calculated by measuring the area in every 12th section throughout the hippocampus, and the total sum of the areas traced for dorsal and ventral blades separately was multiplied by section thickness and series number to produce the entire GCL volume.
Behavioral Tests
IntelliCage®
IntelliCage® is a novel system for unbiased automated monitoring of spontaneous and learning behavior of mice in their home cage environment. Each cage had four corners, and each corner contained two water bottles. A door was located in front of each water bottle, and the door opened when a mouse touched it with its nose. Each cage housed maximum of 11 mice. Mice from the same litters were kept together in the same IntelliCage. Cage 1: 4 NSPC-treated, 3 vehicle-treated and 4 non-irradiated mice. Cage 2: 5 NSPC-treated, 3 vehicle-treated and 2 non-irradiated mice. Cage 3: 2 NSPC-treated, 2 vehicle-treated and 2 non-irradiated mice. Cage 4: 1 NSPC-treated, 1 vehicle-treated and 2 non-irradiated mice. Microchips (DataMars, Bedano, Switzerland) for identification were implanted s.c. in the dorsal neck of the mice on P54 under isoflurane anesthesia.
This behavioral test consisted of five sections (Supplementary Figure 2). The objective of the first two sections was for the mice to learn how to drink water. In the first section, “Introduction 1,” every mouse was permitted to drink in all corners, and cage doors were kept open to aid the mice in locating the water bottles. In the second section, “Introduction 2,” the doors were closed, and the mice, therefore, had to touch them with their noses (“nosepoke”) to open them and drink water. In the third section, “Corner training 1,” one corner was randomly allocated to each mouse. The mouse could open the door only in its allocated corner. The number of times a mouse opened the door in its allocated corner and/or tried to open it in the other corners were automatically recorded. In the next section, “Corner training 2,” the corner allocated in the previous section was changed randomly. Each mouse could access only its own allocated corner. In the final section, “Corner training 3,” the allocated corner was changed again.
Movement Pattern Analysis
The method and variables used for movement pattern analysis have been described in detail elsewhere (24, 25). Briefly, on the day of the experiment, mice were individually introduced into an unfamiliar open field arena and videotaped for 10 min in their inactive phase (lights on). Four arenas were simultaneously videotaped from above with a single CCD Monochrome video camera. The camera was connected to an S-VHS videocassette recorder. The arenas were made of black Plexiglas (l × w × h = 46 × 33 × 35 cm, respectively) rubbed with sandpaper and indirectly illuminated by bright light to avoid reflexes and shadows. The arena floors were covered with light gray gravel that had been previously exposed to other mice. The gravel was used to contrast the black color of the mice, and to act as an absorbent. It was exposed to other mice to saturate it with smells from multiple other individuals in order not to distract them to or from certain areas of the arena more than other areas. After completion of the experiment, videotapes were analyzed with the video tracking program EthoVision 3.1 (Noldus Information Technologies, Wageningen, Netherlands). For each sampling occasion, the program provided data on the position of the mouse as well as the animal's body area viewed by the overhead camera. The analysis resulted in a track record which described the animals' behavioral pattern during the observation period. Video tracking was performed at a sampling frequency of 12.5 Hz. Variables were calculated in a middle zone, defined as the central part located ≥6 cm from the arena walls, as well as in a border zone (< 6 cm from the arena walls). We evaluated the following variables: distance moved, number of stops made, and percent time spent in motion.
Sugar Water (Anhedonia) Test
This test spanned 4 days. Every mouse was housed individually, and normal water (Day 1 and 2) or 3% fructose water (Days 3 and 4) was supplied from the starting time of the night cycle after 6 h of water restriction. We measured the volume (weight) of water/sugar water each mouse drank per night. We then calculated the ratio of consumption (Day 3 + 4/Day 1 + 2) to evaluate preference for the fructose water. A decrease in fructose intake and preference over water is generally taken as a putative sign of anhedonia in rodents (26).
Statistical Analysis
All animal-related data are presented as mean ± standard error. ANOVA followed by Dunnett's post-hoc test was used to compare the three groups. A p-value of < 0.05 was considered to indicate a significant difference between compared mean values.
Results
Mortality and Body Weight Gain
Thirty-six mice were used in the present study, but three mice died during anesthesia/irradiation, and 3 mice were excluded from analyses due to grafting failure. 1 NSPC-treated mouse died during the insertion of a microchip during anesthesia, and three vehicle-treated mice died during the observation period. Therefore, the mortality rates of each group after IR were 1/10 in NSPC-treated mice, 3/10 in vehicle-treated mice, and 1/10 in vehicle-treated non-irradiated mice.
The body weight gains from the point of grafting (P21) to sacrifice (P173) was calculated. The gain was significantly lower in irradiated vehicle-treated mice than in non-irradiated mice (Figure 1, p < 0.01), whereas irradiated, NSPC-grafted mice were not different from controls.
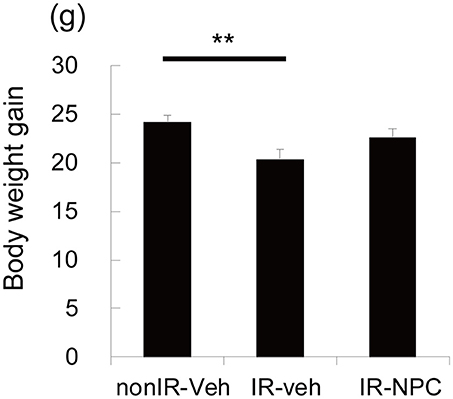
Figure 1. Body weight gain. The body weight gains from the point of grafting (P21) to sacrifice (P173) was significantly lower in vehicle treated mice (but not in NSPC-grafted mice) than those in treated non-irradiated mice (**p < 0.01).
Grafting NSPCs Ameliorated the Negative Effects of IR on Behavior
To examine the effects of grafting on behavior after IR, a single dose of 8 Gy cranial IR was administered at P9, and NSPCs were grafted onto both hippocampi at P21. Beginning 3 months after grafting, we examined the mice's behavior using three tests: IntelliCage®, movement pattern analysis (Open Field test), and sugar water preference (anhedonia test).
IntelliCage® is a system for unbiased automated monitoring of spontaneous activity and learning of mice in their home cage environment (27–34). In the first session, we measured the time it took for each mouse to visit a corner, representing a combined measure of novelty-induced exploratory behavior and anxiety. Irradiated, vehicle-injected mice took four times longer to visit the first corner compared with non-irradiated mice (Figure 2A, p < 0.05), whereas irradiated, NSPC-grafted mice took an intermediate length of time (Figure 2A). The number of times that each mouse tried to open the door in non-allocated corners (errors) was examined. The number of errors in the second corner session (reversal learning) was significantly higher for irradiated, vehicle-injected mice than for irradiated, grafted mice (Figure 2B; Days 1, 2, and 4, p < 0.05) and non-irradiated mice (Figure 2B; Day 2, p < 0.01; Days 1, 3, and 4, p < 0.05). The accumulated number of errors during the second corner session (reversal learning), as registered when a mouse tried to open a door in a non-allocated corner, was higher for the IR + vehicle group than the other two groups (Figure 2C). Irradiated, vehicle-injected mice made several-fold more errors than non-irradiated or irradiated NSPC-grafted mice. Importantly, the number of correct door openings did not differ between groups (Figure 2D). Both this IR-induced learning deficit and the ameliorating effect of NSPC grafting were also apparent in the first and third corners (Supplementary Figures 3A,B). These results indicate that IR significantly impaired place learning and reversal learning, and grafting of NSPCs significantly ameliorated these deficits.
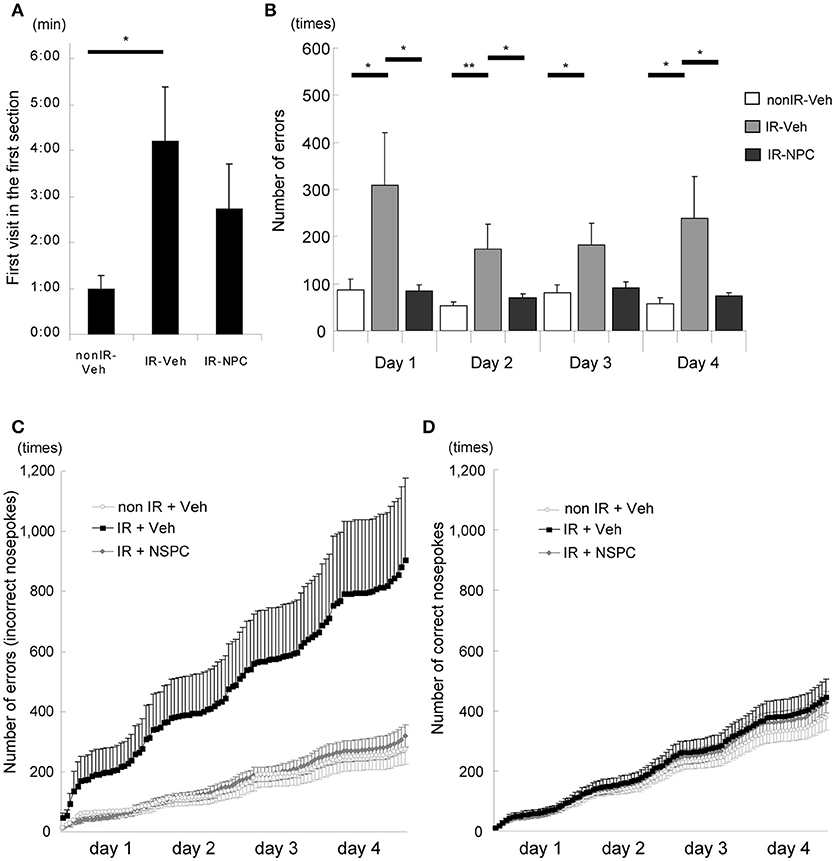
Figure 2. Learning deficit after irradiation (IR) (behavioral evaluation with Intellicage®). (A) First visit in the first section (Introduction 1). The time it took for irradiated mice injected with vehicle (IR-vehicle), but not with neural stem and progenitor cells (NSPCs) (IR-NSPC), to visit a corner was significantly longer than that for non-irradiated mice (non-IR-vehicle; *p < 0.05). (B) Number of errors (second corner). The number of times that each mouse tried to open the door in non-allocated corners (i.e., errors) was significantly higher for irradiated (IR-vehicle) than for irradiated and grafted (IR-NSPC) and non-irradiated (non IR-vehicle) mice (*p < 0.05, **p < 0.01). (C) Accumulated number of errors (second corner). The accumulated number of errors that each mouse made (trying to open a non-allocated door) in the IR-vehicle group was higher than that in the non IR-vehicle group, but that in the IR-NSPC group was almost identical to that in the non-IR-vehicle group. Open circle indicates the non-IR-vehicle group; closed square indicates the IR-vehicle group; and closed diamond indicates the IR-NSPC group. (D) Accumulated number of correct nosepokes (second corner). The accumulated number of correct nosepokes was almost identical between the three groups. Open circle indicates the non-IR-vehicle group; closed square indicates the IR-vehicle group; and closed diamond indicates the IR-NSPC group. Data represent the mean ± S.E.M.
The effect of IR and NSPC grafting on movement pattern in the Open Field test was analyzed with respect to the distance moved, number of stops, and percent time in motion. IR significantly decreased the distance moved (Figure 3A, p < 0.05) and the number of stops made (Figure 3B, p < 0.05), and tended to decrease the time in motion (Figure 3C) in the middle of the arena. On the other hand, there were no significant differences in any variables in the border zone (Figures 3D–F). The irradiated mice appeared more “anxious” and avoided the middle of the arena, but this was normalized by the grafting of NSPCs. (Figures 3A–C, p < 0.05 vs. IR group).
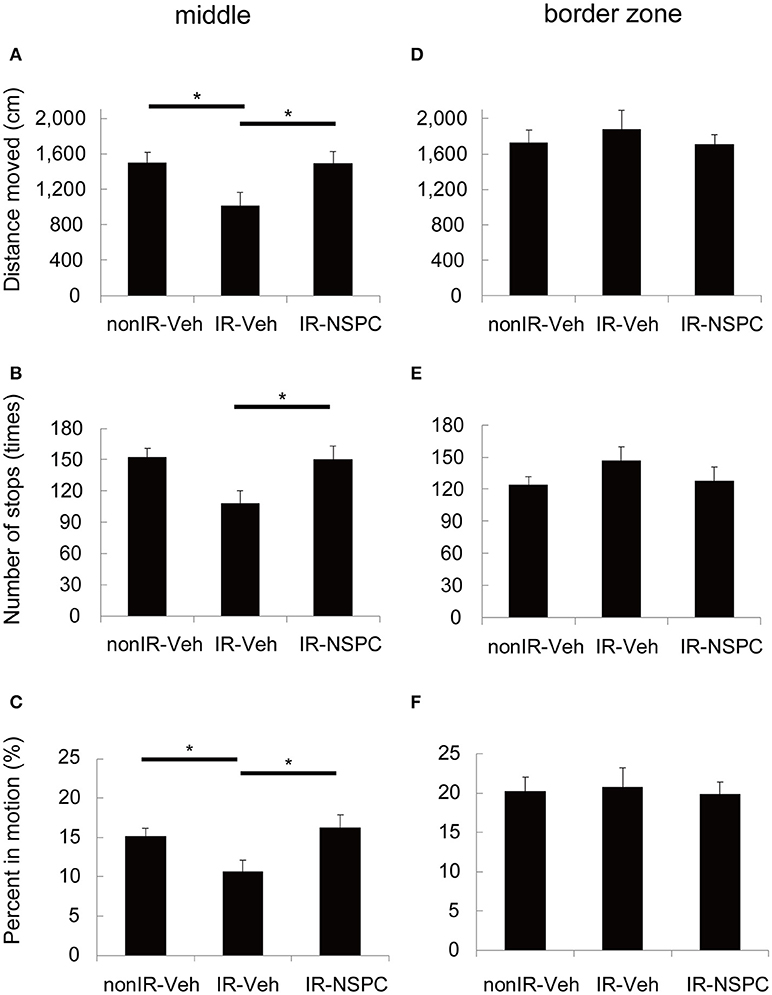
Figure 3. Movement pattern analysis. We evaluated the effect of irradiation (IR) and neural stem and progenitor cell (NSPC) grafting on the movement pattern of mice using the Open Field test. IR significantly decreased the distance moved (A) (*p < 0.05) and the number of stops made (B) (*p < 0.05) and tended to decrease the percent time spent in motion (C) in the middle. NSPCs grafting normalized the effect of IR on these variables (A–C) (*p < 0.05 vs. IR group). Neither IR nor NSPCs grafting changed any variables significantly in the border zone (D–F). Data represent the mean ± S.E.M.
We also applied the sugar water preference or anhedonia test. Irradiated mice treated with vehicle, but not NSPC-grafted mice, tended to consume less sugar water than did non-irradiated mice (Figure 4).
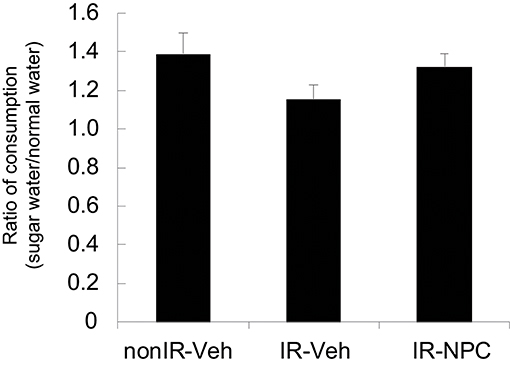
Figure 4. Sugar water preference (anhedonia test). The ratio of consumption [Day 3 + 4 (sugar water)/Day 1 + 2 (normal water)] was examined. Irradiated mice treated with vehicle, but not grafted mice, tended to consume less sugar water than did non-irradiated mice. Data represent the mean ± S.E.M.
The Fate of Grafted Cells and the Effect of Irradiation and/or NSPC Grafting on Morphological Change and Endogenous Neural Stem Cells
We evaluated the number and fate of the grafted cells, the GCL size after grafting, and the effect of grafted NSPCs on endogenous neural stem cells. Five months after IR, 7,222 ± 455 BrdU-positive grafted cells per brain survived in the GCL; i.e., 3.6% of the total number of cells injected. Figure 5A shows a representative image of the hippocampus 5 months after grafting.
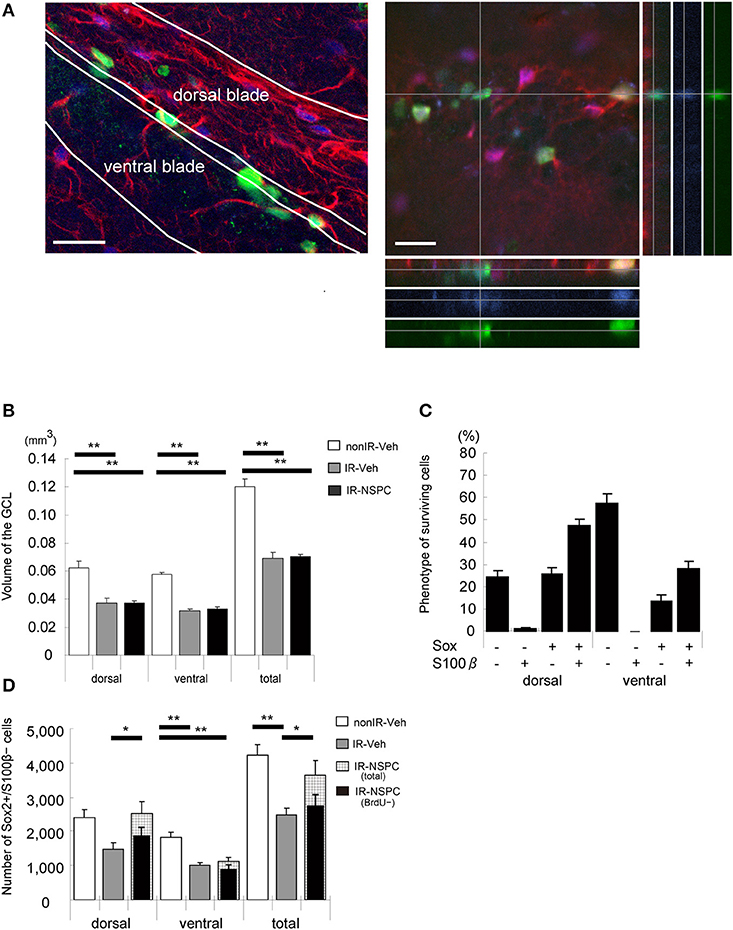
Figure 5. The effect of irradiation (IR) and grafting on the morphology and endogenous neural stem and progenitor cells (NSPCs) of the GCL and the fate of surviving cells (5 months after grafting). (A) Representative microphotograph of the hippocampus 5 months after grafting. Stained for BrdU (green), Sox2 (blue), and S100β (red). Note that surviving grafted cells remained in the whole GCL, and gliosis-like change existed in the dorsal blade of the GCL. A Z-stack was created to analyze BrdU+/Sox2+/S100β+ cells to verify that they were truly double- or triple-positive. Scale bar = 25 μm. (B) Volume of the GCL. There was a significant decrease in irradiated, vehicle-injected and grafted mice compared with non-IR mice (**p < 0.01). Volume of grafted mice was almost identical to that of vehicle-injected mice. (C) Phenotype of surviving cells. We evaluated the phenotype of surviving cells by staining for BrdU, Sox2, and S100β. Only 14% of BrdU-positive cells in the lower blade and 25% in the upper blade of the GCL were positive for Sox2 and negative for S100 β. (D) Total number of stem cells. We evaluated the number of stem cells by staining for Sox2 (positive) and S100β (negative). Total number of stem cells of irradiated vehicle-injected mice was significantly lower than that of non-irradiated mice. Grafted mice had almost the same number of stem cells in the upper blade as non-irradiated mice, but the number in the lower blade was reduced to that of irradiated vehicle-injected mice (**P < 0.01, *P < 0.05). We also evaluated the number of endogenous stem cells by staining for BrdU (negative), Sox2 (positive), and S100β (negative). Number of grafted mice was almost the same as that of irradiated vehicle-injected mice. Volume measurement and positive cell counting were conducted as described in the Materials and Methods section. Data represent the mean ± S.E.M.
IR induces apoptosis of endogenous NSPCs and thereby arrests subsequent growth of the GCL in irradiated developing and juvenile brains (7). NSPC grafting did not increase the GCL volume in irradiated brains, in the dorsal or ventral blades (Figure 5B). The GCL consists mainly of tightly packed granule neurons, and the grafted cells hence did not seem to replace the granule cells that failed to develop after IR.
We estimated how many grafted NSPCs remained undifferentiated, using Sox2 and S100β staining, assuming that Sox2+/S100β− cells represent undifferentiated neural stem cells (double positive cells were astrocytes, whereas double negative cells could be neurons or oligodendrocytes) (35). In the ventral blade of the GCL, 14% of the grafted cells were Sox2+/S100β−, whereas 58% were negative for both Sox2 and S100β. In the dorsal blade, however, 26% of grafted cells were Sox2+/S100β−, and only 25% were negative for both Sox2 and S100β (Figure 5C). The total number of undifferentiated (Sox2+/S100β−) cells were counted in each GCL; i.e., both grafted and endogenous undifferentiated NSPCs (Figure 5D). IR reduced the number of undifferentiated NSPCs to almost half that observed in non-IR hippocampi in both the dorsal and ventral blades (Figure 5D, p < 0.01). Interestingly, NSPC grafting restored the number of undifferentiated NSPCs to control levels but only in the dorsal blade (Figure 5D). In the ventral blade, grafting did not affect the number of undifferentiated neural stem cells (Figure 5D). The normalized number of undifferentiated cells in the dorsal blade could be the result of grafted cells populating the subgranular zone (SGZ), grafted cells stimulating the proliferation and/or survival of endogenous neural stem cells, or a combination of the two. To differentiate between endogenous and grafted undifferentiated neural stem cells, we counted the number of BrdU-negative, undifferentiated (BrdU–/Sox2+/S100β−) cells; i.e., the number of endogenous, undifferentiated cells (Figure 5D). The number of endogenous, undifferentiated cells in irradiated, grafted mice was virtually identical to that of irradiated, non-grafted mice, although there was a tendency toward higher numbers in the dorsal blade of grafted mice (Figure 5D). Therefore, the normalized number of NSPCs in the dorsal blade of irradiated, grafted mice seems to result mainly from the grafted cells populating the SGZ and remaining undifferentiated.
Discussion
In the present study, we have demonstrated that grafted NSPCs can survive in the GCL for at least 5 months, that IR caused behavioral abnormalities, including place learning deficits, and that NSPC grafting into the hippocampus could ameliorate or normalize the behavioral abnormalities induced by IR.
In a previous study, we showed that the survival of grafted NSPCs was significantly impaired and that neuronal differentiation was lower in irradiated than in non-irradiated brains when grafting was performed 24 h after IR (53% in irradiated brains vs. 84% in non-irradiated brains), whereas there were no significant differences when grafting occurred 1 week (64 vs. 66%) or 6 weeks (29 vs. 38%) after IR (18). These findings indicate that we should not graft NSPCs in this acute phase, soon after IR. Grafting cells before IR was deemed not useful, considering that proliferating neural stem cells are very susceptible to IR (7, 11, 19, 36), and a large portion of the grafted NSPCs would be killed by IR and handling the dead and injured cells would add to the burden of the tissue. Based on these findings, we grafted the NSPCs after the acute phase and observed an ameliorating effect of grafting on CNS complications after IR. However, it remains unknown whether grafting before or in the acute phase after IR also would exert such an effect.
Since proliferating neural stem cells are highly susceptible to IR and since a reduced number of proliferating NSPCs in the GCL of the dentate gyrus of the hippocampus can lead to learning/behavioral abnormalities later in life, we hypothesized that exogenous NSPCs could ameliorate the side effects of IR therapy by compensating for the lost cells. In addition, the younger the brain, the higher the number of differentiated neuronal cells after grafting (18). NSPC grafting may therefore be more effective in developing brains than in adult brains. In the present study, we showed that the NSPC grafting into the hippocampi of developing brains could ameliorate learning deficits and behavioral abnormalities. It is known that NSPCs secrete various neurotrophic factors and exert neuroprotective effects (37, 38). In several animal models of stem cell grafting for neurodegenerative diseases, including stroke, both morphological and functional recovery have been observed, even when only a few grafted cells survived. It was reported that grafted cells did not replace degenerated neuronal cells but provided trophic factors and/or suppressed the immune/inflammatory response that induces angiogenesis, neurogenesis, and/or neuroprotection (39). Grafted NSPCs or mesenchymal stem cells enhance proliferation of endogenous cells and neurogenesis in neurogenic regions including the hippocampus and SVZ (40). To elucidate the mechanism of the ameliorating effect of NSPC grafting after IR in the present study, we evaluated the trophic effect of the grafted NSPCs. Specifically, these effects were the volume of the GCL of the hippocampus, which is reduced after IR due to reduced growth (19, 31), and the number of endogenous neural stem cells, based on the number of Sox2-positive and S100β-negative cells and BrdU-negative cells. However, no trophic effects were observed in the present study, as judged by the virtually identical size of the GCL and number of endogenous neural stem cells in irradiated grafted mice were compared to those in irradiated vehicle-injected mice. On the other hand, in our previous study, 5 weeks after grafting at P21, more than 60% of surviving cells were neurons (18). The present study also showed that at 5 months after grafting, more than 50% of surviving cells in the ventral blade were negative for both Sox2 and S100β, which indicated that they adopted a neuronal or oligodendrocytic phenotype, although it was not confirmed with a neuronal marker like NeuN. Although the functionality of the surviving neurons differentiated from grafted NSPCs remains to be evaluated, it is possible that grafted cells replaced, to some extent, the IR-induced loss of NSPCs. This in turn may have led to amelioration of abnormalities after IR. Another possible explanation could be continuous production of growth and trophic factors by NSPCs, which over time leads to changes in connectivity and other functional aspects not related to the actual numbers of cells or their phenotypes.
Toward clinical applications of NSPC grafting for IR-induced learning and behavioral deficits, several issues remain to be addressed. One of these is grafting-induced astrogliosis. We previously showed that injection of NSPCs, but not the vehicle, into the hippocampus induced astrogliosis and reduced thickness of the dorsal blade of the GCL (18). Cell grafting can induce and promote glial scarring (17, 41) and upregulation of expression of chondroitin sulfate proteoglycans around the injection site (42). These cause impaired migration and neuritogenesis of grafted cells, leading to abortive host-graft integration. In a clinical application of NSPC grafting, some modification [e.g., inhibition of reactive astrocytes (43), enzymatic removal of chondroitin sulfate (44)], may be needed to circumvent this problem and enhance the therapeutic effect.
In the present study, we administered NSPCs directly into the hippocampus. In other studies of neuronal diseases, intravenous administration has also been used (45–47). Systemic administration may be advantageous, especially for diseases with multifocal and/or disseminated lesions such as multiple sclerosis. Although NSPCs administrated intravenously can migrate into lesions to some degree, the number of cells is limited, up to 13% (45), and NSPCs accumulate in organs without lesions (47). More recently, an intranasal route for administration of NSPCs has been developed (48–50). It was shown that intranasally administered stem cells can migrate into the brain. These alternative administration routes are less invasive than intracranial grafting but also less effective, and for clinical applications a balance between safety and efficacy must be established.
It may be argued that using postnatal day 9 mice corresponds to a very young human brain, developmentally (51), an age when cranial radiotherapy wouldn't be considered in a clinical setting, but the numbers of endogenous NSPCs and the rate of neurogenesis are higher at this relatively early age, so for the purpose of studying the effects of IR and NSPC grafting, it is useful for proof of principle, as demonstrated in earlier studies in both rats and mice (8, 11, 19). Another reason is that the survival of grafted cells is better in a younger brain, as noted in our previous study (18). Further studies are needed to show the treatment effect of grafting NSPCs with older mice.
In conclusion, our results indicate that NSPCs grafting into the hippocampus can ameliorate or normalize the impaired place learning as well as the altered movement pattern induced by IR. Although ethical, technical and other issues remain to be addressed prior to its clinical application, NSPC grafting is promising as a therapeutic technique. Further studies are needed to optimize the treatment and clarify the underlying mechanisms.
Author Contributions
YS, MS, and KO were actively involved in experiments. NS cultured NSPCs for experiments. MN and YS performed open field test. YS, CZ, HK, MP, and KB conceptualized and designed this study. YS, CZ, HK, and KB interpreted data in this study. YS drafted an initial manuscript, and NS, MS, KO, CZ, MP, HK, and KB revised it critically. All authors approved the final manuscript as submitted and agree to be accountable for all aspects of the work.
Conflict of Interest Statement
The authors declare that the research was conducted in the absence of any commercial or financial relationships that could be construed as a potential conflict of interest.
Acknowledgments
We thank Dr. Niklas Karlsson for help with the use and interpretation of Intellicage® experiments, and Mrs. Rita Grandér for excellent technical assistance. This work was supported by the Swedish Childhood Cancer Foundation (Barncancerfonden), the Swedish Research Council (Vetenskapsrådet), the Swedish Cancer Foundatio (Cancerfonden), grants provided by the Stockholm County Council and the Västra Götaland Region (ALF projects), the Frimurare Barnhus Foundations of Gothenburg and Stockholm, the Märta and Gunnar V. Philipson Foundation, the Swedish Brain Foundation (Hjärnfonden), the Swedish Radiation Safety Authority, the Aina Wallström's and Mary-Ann Sjöblom's Foundation, and the Ulla and Rune Amlöv Foundations. The funding agencies had no influence on the study design.
Supplementary Material
The Supplementary Material for this article can be found online at: https://www.frontiersin.org/articles/10.3389/fneur.2018.00715/full#supplementary-material
References
1. Gustafsson G, Kogner P, Heyman M. Childhood Cancer Incidence and Survival in Sweden 1984-2010. Report 2013. Karolinska Institutet (2013).
2. Bandopadhayay P, Bergthold G, London WB, Goumnerova LC, Morales La Madrid A, Marcus KJ, et al. Long-term outcome of 4,040 children diagnosed with pediatric low-grade gliomas: an analysis of the Surveillance Epidemiology and End Results (SEER) database. Pediatr Blood Cancer (2014) 61:1173–9. doi: 10.1002/pbc.24958
3. Roddy E, Mueller S. Late effects of treatment of pediatric central nervous system tumors. J Child Neurol. (2016) 31:237–54. doi: 10.1177/0883073815587944
4. Gupta P, Jalali R. Long-term survivors of childhood brain tumors: impact on general health and quality of life. Curr Neurol Neurosci Rep. (2017) 17:99. doi: 10.1007/s11910-017-0808-0
5. Hall P, Adami HO, Trichopoulos D, Pedersen NL, Lagiou P, Ekbom A, et al. Effect of low doses of ionising radiation in infancy on cognitive function in adulthood: Swedish population based cohort study. BMJ (2004) 328:19. doi: 10.1136/bmj.328.7430.19
6. Blomstrand M, Holmberg E, Aberg MA, Lundell M, Bjork-Eriksson T, Karlsson P, et al. No clinically relevant effect on cognitive outcomes after low-dose radiation to the infant brain: a population-based cohort study in Sweden. Acta Oncol. (2014) 53:1143–50. doi: 10.3109/0284186X.2014.899434
7. Zhou K, Xie C, Wickstrom M, Dolga AM, Zhang Y, Li T, et al. Lithium protects hippocampal progenitors, cognitive performance and hypothalamus-pituitary function after irradiation to the juvenile rat brain. Oncotarget (2017) 8:34111–27. doi: 10.18632/oncotarget.16292
8. Naylor AS, Bull C, Nilsson MK, Zhu C, Bjork-Eriksson T, Eriksson PS, et al. Voluntary running rescues adult hippocampal neurogenesis after irradiation of the young mouse brain. Proc Natl Acad Sci USA. (2008) 105:14632–7. doi: 10.1073/pnas.0711128105
9. Georg Kuhn H, Blomgren K. Developmental dysregulation of adult neurogenesis. Eur J Neurosci. (2011) 33:1115–22. doi: 10.1111/j.1460-9568.2011.07610.x
10. Redmond KJ, Mahone EM, Terezakis S, Ishaq O, Ford E, McNutt T, et al. Association between radiation dose to neuronal progenitor cell niches and temporal lobes and performance on neuropsychological testing in children: a prospective study. Neuro Oncol. (2013) 15:360–9. doi: 10.1093/neuonc/nos303
11. Fukuda A, Fukuda H, Swanpalmer J, Hertzman S, Lannering B, Marky I, et al. Age-dependent sensitivity of the developing brain to irradiation is correlated with the number and vulnerability of progenitor cells. J Neurochem. (2005) 92:569–84. doi: 10.1111/j.1471-4159.2004.02894.x
12. Hellstrom NA, Bjork-Eriksson T, Blomgren K, Kuhn HG. Differential recovery of neural stem cells in the subventricular zone and dentate gyrus after ionizing radiation. Stem Cells (2009) 27:634–41. doi: 10.1634/stemcells.2008-0732
13. Bostrom M, Kalm M, Karlsson N, Hellstrom Erkenstam N, Blomgren K. Irradiation to the young mouse brain caused long-term, progressive depletion of neurogenesis but did not disrupt the neurovascular niche. J Cereb Blood Flow Metab. (2013) 33:935–43. doi: 10.1038/jcbfm.2013.34
14. van't Hooft I, Norberg AL. SMART cognitive training combined with a parental coaching programme for three children treated for medulloblastoma. Neurorehabilitation (2010) 26, 105–13. doi: 10.3233/NRE-2010-0541
15. Ji JF, Ji SJ, Sun R, Li K, Zhang Y, Zhang LY, et al. Forced running exercise attenuates hippocampal neurogenesis impairment and the neurocognitive deficits induced by whole-brain irradiation via the BDNF-mediated pathway. Biochem Biophys Res Commun. (2014) 443:646–51. doi: 10.1016/j.bbrc.2013.12.031
16. Acharya MM, Christie LA, Lan ML, Donovan PJ, Cotman CW, Fike JR, et al. Rescue of radiation-induced cognitive impairment through cranial transplantation of human embryonic stem cells. Proc Natl Acad Sci USA. (2009) 106:19150–5. doi: 10.1073/pnas.0909293106
17. Osman AM, Zhou K, Zhu C, Blomgren K. Transplantation of enteric neural stem/progenitor cells into the irradiated young mouse hippocampus. Cell Transplant. (2014) 23:1657–71. doi: 10.3727/096368913X674648
18. Sato Y, Shinjyo N, Sato M, Osato K, Zhu C, Pekna M, et al. Grafting of neural stem and progenitor cells to the hippocampus of young, irradiated mice causes gliosis and disrupts the granule cell layer. Cell Death Dis. (2013) 4:e591. doi: 10.1038/cddis.2013.92
19. Fukuda H, Fukuda A, Zhu C, Korhonen L, Swanpalmer J, Hertzman S, et al. Irradiation-induced progenitor cell death in the developing brain is resistant to erythropoietin treatment and caspase inhibition. Cell Death Differ. (2004) 11:1166–78. doi: 10.1038/sj.cdd.4401472
20. Fowler JF. The linear-quadratic formula and progress in fractionated radiotherapy. Br J Radiol. (1989) 62:679–94. doi: 10.1259/0007-1285-62-740-679
21. Pui C-H, Howard SC. Current management and challenges of malignant disease in the CNS in paediatric leukaemia. Lancet Oncol. (2008) 9:257–68. doi: 10.1016/S1470-2045(08)70070-6
22. Ray J, Gage FH. Differential properties of adult rat and mouse brain-derived neural stem/progenitor cells. Mol Cell Neurosci. (2006) 31:560–73. doi: 10.1016/j.mcn.2005.11.010
23. Zhu C, Huang Z, Gao J, Zhang Y, Wang X, Karlsson N, et al. Irradiation to the immature brain attenuates neurogenesis and exacerbates subsequent hypoxic-ischemic brain injury in the adult. J Neurochem. (2009) 111:1447–56. doi: 10.1111/j.1471-4159.2009.06413.x
24. Nilsson M, Waters S, Waters N, Carlsson A, Carlsson ML. A behavioural pattern analysis of hypoglutamatergic mice–effects of four different antipsychotic agents. J Neural Transm. (2001) 108:1181–96. doi: 10.1007/s007020170008
25. Nilsson M, Markinhuhta KR, Carlsson ML. Differential effects of classical neuroleptics and a newer generation antipsychotics on the MK-801 induced behavioural primitivization in mouse. Prog Neuropsychopharmacol Biol Psychiatr. (2006) 30:521–30. doi: 10.1016/j.pnpbp.2005.11.010
26. Porsolt R, Papp M. CNS – Psychiatric models of disease: depression. In: Williams M, Enna SJ, Kenakin T, Ferkany JW, editors. Current Protocols in Pharmacology, NewYork, NY: Wiley (1998). p. 591–8.
27. Barlind A, Karlsson N, Bjork-Eriksson T, Isgaard J, Blomgren K. Decreased cytogenesis in the granule cell layer of the hippocampus and impaired place learning after irradiation of the young mouse brain evaluated using the IntelliCage platform. Exp Brain Res. (2010) 201:781–7. doi: 10.1007/s00221-009-2095-8
28. Zhu C, Gao J, Karlsson N, Li Q, Zhang Y, Huang Z, et al. Isoflurane anesthesia induced persistent, progressive memory impairment, caused a loss of neural stem cells, and reduced neurogenesis in young, but not adult, rodents. J Cereb Blood Flow Metab. (2010) 30:1017–30. doi: 10.1038/jcbfm.2009.274
29. Karlsson N, Kalm M, Nilsson MKL, Mallard C, Björk-Eriksson T, Blomgren K. Learning and activity after irradiation of the young mouse brain analyzed in adulthood using unbiased monitoring in a home cage environment. Radiat Res. (2011) 175:336–46. doi: 10.1667/RR2231.1
30. Huo K, Sun Y, Li H, Du X, Wang X, Karlsson N, et al. Lithium reduced neural progenitor apoptosis in the hippocampus and ameliorated functional deficits after irradiation to the immature mouse brain. Mol Cell Neurosci. (2012) 51:32–42. doi: 10.1016/j.mcn.2012.07.002
31. Roughton K, Kalm M, Blomgren K. Sex-dependent differences in behavior and hippocampal neurogenesis after irradiation to the young mouse brain. Eur J Neurosci. (2012) 36:2763–72. doi: 10.1111/j.1460-9568.2012.08197.x
32. Kalm M, Karlsson N, Nilsson MK, Blomgren K. Loss of hippocampal neurogenesis, increased novelty-induced activity, decreased home cage activity, and impaired reversal learning one year after irradiation of the young mouse brain. Exp Neurol. (2013) 247:402–9. doi: 10.1016/j.expneurol.2013.01.006
33. Perez-Alcazar M, Daborg J, Stokowska A, Wasling P, Bjorefeldt A, Kalm M, et al. Altered cognitive performance and synaptic function in the hippocampus of mice lacking C3. Exp Neurol. (2014) 253:154–64. doi: 10.1016/j.expneurol.2013.12.013
34. Kalm M, Andreasson U, Bjork-Eriksson T, Zetterberg H, Pekny M, Blennow K, et al. C3 deficiency ameliorates the negative effects of irradiation of the young brain on hippocampal development and learning. Oncotarget (2016) 7:19382–94. doi: 10.18632/oncotarget.8400
35. Suh H, Consiglio A, Ray J, Sawai T, D'Amour KA, Gage FH. In vivo fate analysis reveals the multipotent and self-renewal capacities of Sox2+ neural stem cells in the adult hippocampus. Cell Stem Cell (2007) 1:515–28. doi: 10.1016/j.stem.2007.09.002
36. Fukuda A, Fukuda H, Jonsson M, Swanpalmer J, Hertzman S, Lannering B, et al. Progenitor cell injury after irradiation to the developing brain can be modulated by mild hypothermia or hyperthermia. J Neurochem. (2005) 94:1604–19. doi: 10.1111/j.1471-4159.2005.03313.x
37. Lu P, Jones LL, Snyder EY, Tuszynski MH. Neural stem cells constitutively secrete neurotrophic factors and promote extensive host axonal growth after spinal cord injury. Exp Neurol. (2003) 181:115–29. doi: 10.1016/S0014-4886(03)00037-2
38. Llado J, Haenggeli C, Maragakis NJ, Snyder EY, Rothstein JD. Neural stem cells protect against glutamate-induced excitotoxicity and promote survival of injured motor neurons through the secretion of neurotrophic factors. Mol Cell Neurosci. (2004) 27:322–31. doi: 10.1016/j.mcn.2004.07.010
39. Ropper AE, Thakor DK, Han I, Yu D, Zeng X, Anderson JE, et al. Defining recovery neurobiology of injured spinal cord by synthetic matrix-assisted hMSC implantation. Proc Natl Acad Sci USA. (2017) 114:E820–9. doi: 10.1073/pnas.1616340114
40. Gobshtis N, Tfilin M, Wolfson M, Fraifeld VE, Turgeman G. Transplantation of mesenchymal stem cells reverses behavioural deficits and impaired neurogenesis caused by prenatal exposure to valproic acid. Oncotarget (2017) 8:17443–52. doi: 10.18632/oncotarget.15245
41. Kinouchi R, Takeda M, Yang L, Wilhelmsson U, Lundkvist A, Pekny M, et al. Robust neural integration from retinal transplants in mice deficient in GFAP and vimentin. Nat Neurosci. (2003) 6:863–8. doi: 10.1038/nn1088
42. Suzuki T, Akimoto M, Imai H, Ueda Y, Mandai M, Yoshimura N, et al. Chondroitinase ABC treatment enhances synaptogenesis between transplant and host neurons in model of retinal degeneration. Cell Transplant. (2007) 16:493–503. doi: 10.3727/000000007783464966
43. Widestrand A, Faijerson J, Wilhelmsson U, Smith PL, Li L, Sihlbom C, et al. Increased neurogenesis and astrogenesis from neural progenitor cells grafted in the hippocampus of GFAP-/- Vim-/- mice. Stem Cells (2007) 25:2619–27. doi: 10.1634/stemcells.2007-0122
44. Singhal S, Lawrence JM, Bhatia B, Ellis JS, Kwan AS, Macneil A, et al. Chondroitin sulfate proteoglycans and microglia prevent migration and integration of grafted muller stem cells into degenerating retina. Stem Cells (2008) 26:1074–82. doi: 10.1634/stemcells.2007-0898
45. Mitrecic D, Nicaise C, Gajovic S, Pochet R. Distribution, differentiation, and survival of intravenously administered neural stem cells in a rat model of amyotrophic lateral sclerosis. Cell Transplant. (2010) 19:537–48. doi: 10.3727/096368910X498269
46. Mochizuki N, Moriyama Y, Takagi N, Takeo S, Tanonaka K. Intravenous injection of neural progenitor cells improves cerebral ischemia-induced learning dysfunction. Biol Pharm Bull. (2011) 34:260–5. doi: 10.1248/bpb.34.260
47. Donega M, Giusto E, Cossetti C, Schaeffer J, Pluchino S. Systemic injection of neural stem/progenitor cells in mice with chronic EAE. J Vis Exp. (2014) e51154. doi: 10.3791/51154
48. Wu S, Li K, Yan Y, Gran B, Han Y, Zhou F, et al. Intranasal delivery of neural stem cells: a cns-specific, non-invasive cell-based therapy for experimental autoimmune encephalomyelitis. J Clin Cell Immunol. (2013) 4:142. doi: 10.4172/2155-9899.1000142
49. Donega V, Nijboer CH, van Tilborg G, Dijkhuizen RM, Kavelaars A, Heijnen CJ. Intranasally administered mesenchymal stem cells promote a regenerative niche for repair of neonatal ischemic brain injury. Exp Neurol. (2014) 261:53–64. doi: 10.1016/j.expneurol.2014.06.009
50. Ji G, Liu M, Zhao XF, Liu XY, Guo QL, Guan ZF, et al. NF-kappaB signaling is involved in the effects of intranasally engrafted human neural stem cells on neurofunctional improvements in neonatal rat hypoxic-ischemic encephalopathy. CNS Neurosci Ther. (2015) 21:926–35. doi: 10.1111/cns.12441
Keywords: neural stem progenitor cells, irradiation, transplantation, grafting, learning deficits, developing brain, late effects
Citation: Sato Y, Shinjyo N, Sato M, Nilsson MKL, Osato K, Zhu C, Pekna M, Kuhn HG and Blomgren K (2018) Grafting Neural Stem and Progenitor Cells Into the Hippocampus of Juvenile, Irradiated Mice Normalizes Behavior Deficits. Front. Neurol. 9:715. doi: 10.3389/fneur.2018.00715
Received: 31 January 2018; Accepted: 08 August 2018;
Published: 11 September 2018.
Edited by:
Olivier Baud, Geneva University Hospitals (HUG), SwitzerlandReviewed by:
Ivo Bendix, Universitätsklinikum Essen, GermanyRyan J. Felling, Johns Hopkins University, United States
Copyright © 2018 Sato, Shinjyo, Sato, Nilsson, Osato, Zhu, Pekna, Kuhn and Blomgren. This is an open-access article distributed under the terms of the Creative Commons Attribution License (CC BY). The use, distribution or reproduction in other forums is permitted, provided the original author(s) and the copyright owner(s) are credited and that the original publication in this journal is cited, in accordance with accepted academic practice. No use, distribution or reproduction is permitted which does not comply with these terms.
*Correspondence: Klas Blomgren, a2xhcy5ibG9tZ3JlbkBraS5zZQ==