- 1Department of Neurology, Henry Ford Health System, Detroit, MI, United States
- 2Department of Physics, Oakland University, Rochester, MI, United States
Diabetic neuropathy (DN) is the most prevalent chronic complication of diabetes mellitus. The exact pathophysiological mechanisms of DN are unclear; however, communication network dysfunction among axons, Schwann cells, and the microvascular endothelium likely play an important role in the development of DN. Mounting evidence suggests that microRNAs (miRNAs) act as messengers that facilitate intercellular communication and may contribute to the pathogenesis of DN. Deregulation of miRNAs is among the initial molecular alterations observed in diabetics. As such, miRNAs hold promise as biomarkers and therapeutic targets. In preclinical studies, miRNA-based treatment of DN has shown evidence of therapeutic potential. But this therapy has been hampered by miRNA instability, targeting specificity, and potential toxicities. Recent findings reveal that when packaged within extracellular vesicles, miRNAs are resistant to degradation, and their delivery efficiency and therapeutic potential is markedly enhanced. Here, we review the latest research progress on the roles of miRNAs as biomarkers and as potential clinical therapeutic targets in DN. We also discuss the promise of exosomal miRNAs as therapeutics and provide recommendations for future research on miRNA-based medicine.
Introduction
Diabetic neuropathy (DN) is a prevalent complication associated with diabetes mellitus (1). Approximately half of all diabetic patients, either pre-diabetes, type 1 or type 2 diabetes, will go on to develop this condition (2, 3). The symptoms of DN vary according to different stages of the disease. However, the commonality across all stages is the distal-to-proximal gradient of severity (4, 5). DN involves more sensory than motor impairments (6, 7). At the early stage, DN patients mainly experience pain and hyperalgesia. As the disease progresses, patients experience numbness, muscle weakness, loss of balance, and foot ulcers (6, 8, 9). Besides the staggering healthcare costs, DN patients experience poor quality of life and have high rates of ulcerations and amputations (10, 11). Also, long-term physical discomfort may lead to the development of depression and anxiety (12).
To date, the treatment options for DN patients include (1) intensive glycemic control, which slows the progression of the disease, (2) pain relief drugs, anti-seizure medications, and antidepressants, and (3) foot care (13, 14). However, a recent meta-analysis of DN studies has indicated that glycemic control does not benefit the majority of DN patients (15). Additionally, rapid glucose lowering can trigger neuropathic pain, which is known as treatment-induced neuropathy (15). Thus, available treatments for DN are far from sufficient.
Function of microRNAs
Increasing evidence indicates that microRNAs (miRNAs) are involved in the pathogenesis of DN (16). miRNAs are non-coding RNA sequences, and they are composed of 18–24 nucleotides in length (17). miRNAs bind to target mRNAs and induce translational repression and mRNA decay (18). Mature miRNAs can be released into the circulation and body fluids. As they are protected by RNA-binding proteins and lipid-containing vesicles (microvesicles, exosomes, apoptotic bodies, and high-density lipoprotein), miRNAs show good stability and facilitate communication between cells or organs (19). In the context of diabetes, hyperglycemia, hypoxia, and inflammation affect miRNA biogenesis and release. Consequently, these alterations in the miRNA profile are associated with multiple microvascular complications (20–23). Of note, a significant portion of miRNAs was found to be specifically packaged into extracellular vesicles that express cell-type-specific proteins to mediate their different functions (24). These findings support the potential applications of miRNAs in the diagnosis and therapy for DN (25).
In this review, we summarize recent findings on the roles of miRNAs in the progression of DN and their potential as biomarkers and therapeutic targets of DN. In particular, we emphasize the clinical potential of miRNA-based therapy in the treatment of DN.
miRNAs and the Pathophysiology of Diabetic Neuropathy
DN is a multifunctional disorder which is characterized by complex pathogenic mechanisms that have not been fully elucidated. The pathogenic factors which contribute to DN include metabolic dysfunction, inflammation, and oxidative stress, the associated molecular underpinnings of which have been proposed (26, 27). In this subsection, we discuss recent findings regarding the roles that miRNAs play in the regulation of peripheral nervous system (PNS) function and their therapeutic potential in DN (Figure 1).
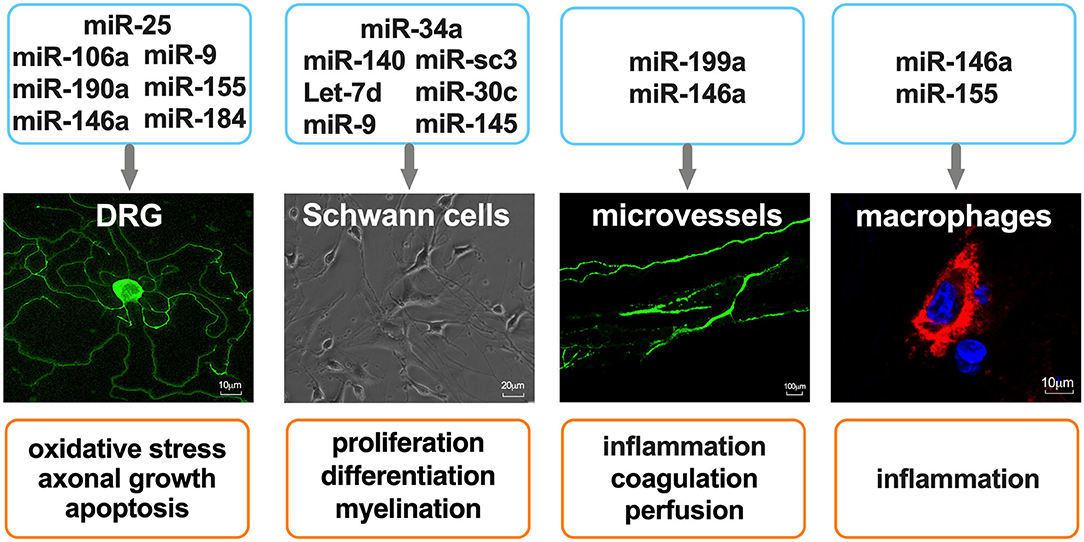
Figure 1. miRNAs as regulators of cell function in the peripheral nervous system. Primary mouse DRG neurons were stained with anti-Neurofilament H. Microvessels in mouse sciatic nerves were perfused with FITC-dextran (molecular mass 2,000 kDa, 500 mg/kg). Macrophages were stained with CD68, and nuclei were stained with 4′,6-diamidino-2-phenylindole.
miRNAs and Metabolic Alteration of Diabetic Neuropathy
The metabolic changes in diabetes that contribute to DN include hyperglycemia, dyslipidemia, and insulin resistance (28–33). These metabolic imbalances promote the activation of protein kinase C (PKC), polyol and hexosamine pathways, advanced glycation end products (AGEs) production, the activity of poly (ADP-ribose) polymerase (PARP), lipoxygenase, and elevated Na+/H+ exchanger-1, as well as suppression of insulin signaling (34–43). Oxidative stress and inflammation are considered as critical common pathways of cellular injury in hyperglycemia (44–46). An increase in oxidative and nitrosative stress in plasma and tissues cause mitochondrial impairment, DNA damage and apoptosis (47–49). Studies have reported the role of miRNAs in oxidative response in diabetes but reports on DN individuals are limited. In db/db mice with DN, overexpression of the miR-25 precursor reduced PKC-α phosphorylation-mediated reactive oxygen species production in diabetic peripheral nerves, leading to improvement of neurological function (50). Wu et al. reported that miR-106a targeted 12/15-Lipoxygenase of oxidative/nitrative stress and hindered apoptosis of dorsal root ganglia (DRG) in DN (51). However, the important role of miRNAs in the regulation of metabolism associated with DN remains to be determined.
miRNAs and Inflammation of Diabetic Neuropathy
DN is closely associated with chronic low-grade inflammation and activation of the innate immune system (27). Inflammation is strongly associated with neuropathic pain, which affects approximately 10–40% of the general population (52). In DN patients, elevated glucose levels increase fibronectin and inflammatory mediators, such as IL-6, vascular endothelial growth factor (VEGF), nuclear factor-kappa B (NF-κB), TNFα, intercellular adhesion molecule-1 (ICAM-1), and transforming growth factor-β (TGF-β). These effects cause an increased sensitization of the central nervous system (53–57). Gong et al. examined the miRNA profiles of the lumbar spinal dorsal horn of mice with DN and found that miR-190a-5p and miR-184-5p were related to inflammation-associated genes that were involved in the pathogenesis of neuropathic pain (58). MiR-9 interacted with calcium homeostasis modulator 1 (CALHM1) and activated the ATP-P2X7R signaling pathway in the spinal cord neurons of rats with DN (59). MiR-155 mediated neuropathic pain by targeting NF-κB activity (60).
Using rodent models, some miRNA-based treatments have been shown to be effective in pain control (Table 1). MiR-190a alleviated pain by targeting solute carrier family 17 member 6 (SLC17A6) and decreasing the IL-1β and IL-6 level in the lumbar spinal dorsal horn (61). In a mouse model of DN, miR-155 mimics suppressed pro-inflammatory cytokines and attenuated DN by targeting tumor necrosis factor receptor-associated factor 2 (TRAF2) and Notch2 (62). Zhang et al., reported that miR-25 decreased reactive oxygen species content in diabetic peripheral nerves by activating NADPH oxidase and upregulating Advanced glycation endproduct (AGE)–RAGE interaction (50), In a diabetic state, reduced miR-146a expression was observed in serum, leukocytes, retina, heart, and PNS (65–67). MiR-146a was also reported to be involved in DN progression (64, 68). Our recent experimental study adds substantially to this body of evidence (63). The administration of miR-146a mimics increased nerve conduction velocity and ameliorated morphological damage in the sciatic nerve and footpad of DN mice. In the circulation and the PNS, miR-146a reduced levels of the inflammatory cytokines TNFα and IL-1β. Further analysis revealed that miR-146a altered the macrophage polarization by suppressing proinflammatory macrophage (M1) activation and increasing anti-inflammatory macrophage (M2) activation (63). These results are consistent with those of other groups, which emphasize the regulatory effects of miR-146a on inflammatory responses in DN (64, 68–70).
Roles of miRNAs in the Neurovascular Dysfunction of Diabetic Neuropathy
miRNAs and Sensory Neurons
Approximately 80% of DN patients develop gradual damage to the distal terminals of sensory neurons, leading to the symptoms of pain or loss of sensation in their toes (4). Diabetes preferentially targets sensory nerves, autonomic nerves and then motor nerves (3). An explanation is that sensory neurons located in the DRG lack the protection of the blood-nerve barrier, while motor neurons located in the ventral horn of spinal cord retain this protection (71). Furthermore, the PNS contains more unmyelinated axons, known as C fibers than myelinated axons. C fibers send afferent impulses in response to thermal and mechanical stimuli (72). Loss of C fibers is an early change that is witness to the development of DN (73). The molecular mechanisms of how diabetes targets peripheral neurons and their axons remain unclear. The prevailing hypotheses include oxidative stress, AGEs accumulation, inflammation, and mitochondrial dysfunction, all of which contribute to the injury of DRG neurons and alteration of mRNA and miRNA expression (74–76). Cheng et al. found that Let-7i and miR-341 were dysregulated in diabetic DRG, while administration of let-7i mimic and anti-miR-341 independently improved structural abnormalities and neurological dysfunction in a mouse model of DN (75). MiR-29b was found to be down-regulated in DRG neurons of STZ-induced diabetic rats. The decreased miR-29b was associated with axonal swelling, apoptosis, and abnormal gene profiles in DRG (77).
In order to reverse the neuropathic deficits associated with DN, another strategy involves the activation of axonal regeneration, which has shown promising results in in vitro and in vivo DN models (78–80). PNS neurons are capable of long-distance axonal regeneration (81). The administration of miR-29b mimic promoted axonogeneration and inhibited neurodegeneration via the TGF-β/Smad3 pathway (77). Previous studies from our group showed that miRNAs mediate the axonal growth of DRG neurons. Diabetic db/db mice showed upregulation of miR-29c in peripheral nervous tissues. When cultured under high glucose condition, DRG neurons showed a substantial reduction in axonal growth. However, when the DRG neurons were transfected with a miR-29c inhibitor, attenuation of endogenous miR-29c increased axonal growth. This effect was mediated by PRKCI, which codes for Protein kinase C iota. When PRKCI was knocked down, the effects of miR-29c mimics or inhibitors were abolished (80).
In another study, our group observed that miR-34a was involved in axonal growth in db/db mice with DN. In cultured DRG neurons, miR-34a targets a seed region in the 3′UTR region of forkhead box protein P2 and vesicle amine transport 1, leading to a reduction in axonal growth (79). Hyperglycemia down-regulates miR-146a, which is involved in the reduction of axonal outgrowth and apoptosis of DRG neurons (78, 82). Axonal miRNAs locally regulate energy metabolism. Using DRG neurons cultured in a microfluidic chamber, high glucose locally reduced miR-146a levels in distal axons. Gain- and loss-of-function of miR-146a regulate axonal growth via its target genes IL-1 receptor-associated kinase 1(IRAK1) and tumor necrosis factor receptor-associated factor 6 (TRAF6) (78).
miRNAs and Schwann Cells
Schwann cells are the most abundant glial cells in the PNS, and they maintain neuronal structure and function, promote survival, and growth after injury (83, 84). Sciatic nerves of DN patients usually show Schwann cell injury. The ultrastructure of Schwann cells shows reactive, degenerative, and proliferative changes with the progression of DN (83).
In a diabetic state, hyperglycemia interrupts Schwann cell function through different mechanisms (85). Redundant glucose is converted to sorbitol by aldose reductase, which is expressed in myelinating Schwann cells, and depletes NADPH, leading to oxidative stress (86). In addition, sorbitol promotes Schwann cell dedifferentiation into an immature phenotype via reducing insulin-like growth factor 1 expression (87). Excess glucose and modified lipoproteins trigger the production of inflammatory cytokines in Schwann cells and thereby enhance immune cell recruitment (88). Diabetes-induced mitochondria dysfunction activate a maladaptive stress response in Schwann cells, which induces lipid oxidation and inhibits lipid synthesis, resulting in the depletion of the lipid myelin components, and thereby causes axonal degeneration (89).
Schwann cells can sense axonal loss and dedifferentiate to a proliferative phase and support axonal regeneration (83). The immature-like Schwann cells clear myelin debris and release neurotrophic factors until new axons form. Then, they redifferentiate to form myelin sheaths (84). Using in vitro and in vivo DN models, miRNAs were demonstrated to participate in the response of Schwann cells to injury and Schwann cell–axon interactions (90). A recent study showed that Dicer, which is a critical enzyme for miRNA biogenesis, is important for Schwann cell myelination (91). Viader et al. found that miR-34a was able to regulate dedifferentiation by Notch1 and cyclin D1 (92). MiR-140 was found to regulate the expression of the transcription factor for myelinogenesis, Krox20 (92). Furthermore, Let-7d significantly reduced primary Schwann cell proliferation and migration by directly targeting nerve growth factors (93). MiR-9, miR-sc3, miR-30c were reported to be involved in Schwann cell proliferation and migration (94–96). In cultured rat Schwann cells (RSC96), the inhibition of miR-145-3p by circular RNA ACR alleviated high glucose-induced cell apoptosis, autophagy, and ROS generation by activating the phosphatidylinositol 3-kinase/protein kinase B/mammalian target of rapamycin (PI3K/AKT/mTOR) pathway (97). Therefore, manipulation of Schwann cell miRNAs has the potential to be used therapeutically to reverse the effects of DN.
miRNAs and Microvascular Dysfunction
DN is considered to be a microvascular complication of diabetes (98). Several mechanisms of hyperglycemia-induced cellular injury were described to occur in the vascular endothelium (99, 100). Nerve biopsies from DN patients show thickening of capillary basement membrane and capillary pericyte degeneration (98). Microvasculature dysfunction results in a reduction of nutrition, oxygen, and waste transportation through nerves, and either precedes or parallels nerve dysfunction and demyelination (98, 101). Although the mechanism is not fully understood, there is evidence that the chronic inflammatory state of diabetes contributes to microvascular complications (102).
miRNAs are involved in diabetes-induced vascular dysfunction (103). In a study of 60 patients with DN, miR-199a-3p was significantly higher compared to control volunteers. The authors proposed that miR-199a-3p promotes coagulation of the skin peripheral circulation through down-regulating serine protease inhibitor E2 (SerpinE2) and up-regulating matrix metalloproteinase-13 (MMP-13) in endothelial cells (104). Kamali et al. reported that miR-146a is upregulated in human umbilical vein endothelial cells during the early phase of hyperglycemic state, and possibly regulates the NF-κB activity (105). Our previous data shows that db/db mice exhibited a significant reduction of blood flow in peripheral nerve tissues, which were measured using laser Doppler flowmetry. This reduction in blood flow correlated with nerve dysfunction and axonal demyelination in a sex-dependent manner (106). However, the delivery of miR-146a mimics significantly improved peripheral nerve tissue perfusion (63). These data highlight the role of miRNAs in restoring blood flow which eventually improves the nerve function in the treatment of DN (Table 2).
miRNAs as Biomarkers in the Diagnosis of Diabetic Neuropathy
The diagnosis of DN is not yet standardized but comprises both qualitative and quantitative methods. In addition to being evaluated for any signs of muscle weakness, numbness, and impaired reflexes, patients are usually administered blood tests (vitamin level, diabetes, and immune function), imaging tests [computed tomography (CT) scans, magnetic resonance imaging (MRI), muscle and nerve ultrasound], non-invasive neurological tests (electromyography and nerve conduction velocity test), and invasive tests (including nerve biopsy and skin biopsy) (107–110).
miRNA profiles of diabetic patients have been reported in various studies. Liu et al. measured miRNA profiles in the mononuclear cells collected from 63 diabetic patients with or without complications. The study confirmed that miR-125a-5p, miR-145-3p, miR-99b-5p, and miR-873-5p were enriched in peripheral blood mononuclear cells, and the targeted gene families of these miRs were associated with DN (22). A comprehensive study on the serum miRNA profile of type 1 diabetes patients with DN revealed that serum miR-518d-3p and miR-618 were upregulated compared to diabetic patients without DN (23). Another study of 60 diabetic patients demonstrated a correlation between miR-199a-3p and reduced extracellular serine content (104). Ciccacci et al. reported that patients carrying the rs3746444 GG genotype of the miR-499a had a higher risk of developing DN (111). In a study of DN patients, the expression of miR-21-5p, miR-146a, and miR-155 were aberrant in white blood cells, peripheral nerves and skin tissue (112) (Table 3).
Some groups have identified dysregulated miRNAs in the tissue of animal models of DN. Guo et al. examined microRNA and mRNA expression profiling in the dorsal root ganglia isolated from diabetic rats and built a microRNA-gene network. They found that miR-17-1-3p, miR-330-5p, and miR-346 are potent promoters of DN (113). Xourgia et al. proposed that miR-146, miR-499a, and miR-199a-3p are possible biomarkers of DN (21). In addition, miR-181a-1, miR-541, miR-341, and miR-203 were reported to be dysregulated in an animal model of DN (116). These data suggest that circulating miRNAs may serve as potential biomarkers in the diagnosis of DN.
Extracellular miRNAs and Diabetic Neuropathy
miRNAs and Extracellular Vesicles
Since miRNAs have been detected in biological fluid, their characteristic properties have been investigated in order to identify their potential as biomarkers or therapeutic targets (117). The stability of circulating miRNAs supports its potential as biomarkers. There are two possible means by which miRNAs can be protected from RNase degradation: complexing with Argonaute-protein, and being packaged within extracellular vesicles (EVs) or lipoprotein (118). Exosomes have low immunogenicity, low toxicity, and high nucleic acid loading capacity. Therefore, they hold great promise as vehicles for therapeutic cargos (119). Exosomal miRNAs have been found to regulate target gene expression and thereby mediate physiological responses in the recipient cells (120). Some studies employed silencing of Dicer to generate miRNA-depleted EVs, and the effects of the naïve exosomes were abolished (121, 122). These studies suggest that the miRNA cargo of exosomes largely contribute to their molecular and biological effects. According to www.Clinicaltrail.gov, there are several ongoing clinical trials using exosomal miRNAs to diagnose or to study the pathophysiology of myocardial infarction, diabetic retinopathy, cancer, and sepsis (NCT04127591, NCT03264976, NCT03738319, NCT03911999, NCT02957279).
Extracellular miRNAs in Peripheral Neurovascular Function
In a preclinical study, Lopez-Verrilli et al. reported that Schwann cell-derived exosomes were internalized by DRG axons to promote axonal regeneration (123). Our group showed that exosomes derived from Schwann cells (SC-exos) increased intraepidermal nerve fiber density in the footpad and reduced axonal and myelin damage of the sciatic nerve, leading to a reduction of neuropathic symptoms in DN mice (124). In contrast, exosomes derived from Schwann cells cultured in high-glucose medium facilitated the development of DN by reducing epidermal nerve fibers (125). Naïve SC-exos were enriched in miR-21, miR-27a, and miR-146a. However, the ablation of miR-27a in exosomes abolished their effects on promoting axonal growth of diabetic DRG neurons and migration of Schwann cells compared with naïve SC-exos. Bioinformatics analysis revealed that miR-21, miR-27a, and miR-146a target Semaphorin 6A (SEMA6A), Ras homolog gene family, member A (RhoA), phosphatase and tensin homolog (PTEN), and nuclear factor-κB (NF-κB) genes, respectively, thereby protecting axons and improving axonal growth. Exosomes isolated from Schwann cells stimulated by high glucose were enriched for miR-28, miR-31a, and miR-130, which target DNA methyltransferase-3a (NDNMT3A), NUMB (endocytic adaptor protein), synaptosome associated protein 25 (SNAP25), and growth-associated protein-43 (GAP43), separately and participate in axonal growth (125).
Extracellular miRNA as Therapy Target for Diabetic Neuropathy
Mesenchymal stromal cell (MSC)-derived exosomes have been employed in the study of cancer, stroke and cardiovascular diseases. These studies showed that MSC-exosomes function via their miRNA cargoes (126). In a recent study, we administered MSC-derived exosomes to db/db mice with DN and observed a significant improvement in neurological outcomes (127). Further analysis confirmed that MSC-exosomes inhibit the inflammatory response and promote neurovascular remodeling. Data from miRNA sequence and bioinformatics analysis revealed that MSC-exosomes are enriched in miRNAs that are highly involved in inflammation, cell cycle progression, and apoptosis. Let-7a, miR-23a, and miR-125b, among others, synergistically target the TLR4/NF-κB signaling pathway, thereby regulating macrophage polarization (127).
The use of exosomes as therapy represents an innovative and very promising delivery system. It provides an opportunity to address a compelling clinical need. To mimic the property of exosomes, chemically synthesized nanoparticles have been used to package small RNAs in disease treatment (128). In a study on DN, Luo et al. employed imine backbone-based polymer to construct a cationic nanocarrier for miR-146a and demonstrated that nanoparticle–miR-146a-5p alleviated the morphological changes of sciatic nerve in a rat model of DN by regulating the inflammatory response (70).
Conclusion and Future Perspective
The field of miRNAs as a potential therapy for DN is growing. In this review, we summarized recent findings on miRNAs that are involved in the pathophysiology and treatment of DN. Several challenges remain to enable the translation of miRNA-based therapy to the clinic. The biological function of the majority of miRNAs has yet to be investigated. Previous studies have targeted specific dysregulated miRNAs. However, given that DN is caused by multiple factors, the regulation of a single miRNA may not be sufficient to reverse the impairments. A network of miRNAs and mRNAs will likely provide more comprehensive and accurate characterization of DN. There is a paucity of literature interrogating the mechanisms by which miRNAs regulate the development of DN. Most of the miRNA-related studies were performed in different rodent strains utilizing different methods to induce DN. More work is needed to unlock the potential of miRNA therapy for clinical use.
Recent preclinical studies using exogenous double-stranded miRNAs mimic or single-stranded antisense RNAs (antimiRs) have shown promising results in rodent models of DN (50, 59, 63, 77, 79). However, the use of miRNAs as translational agents or pharmaco-targets in DN patients requires future investigations. The mechanisms underlying these beneficial therapies are being elucidated. Additionally, safety considerations need to be fully explored. Exosomes enhance the stability of miRNA delivery with high transduction efficiency. Largely, exosome-based therapy appears to be safe without adverse effects. Standard quality control needs to be established to ensure the consistency of exosomal products and the content of their miRNA cargo.
Author Contributions
BF compose the 1st version of the manuscript. ZZ wrote part 1-2. MC wrote part 3-4. XL is in charge of the overall design and revised the manuscript. All authors contributed to the article and approved the submitted version.
Funding
This work was supported by the National Institutes of Health National Institute of Diabetes and Digestive and Kidney Disease grant RO1 RDK102861A (XL), American Heart Association Grant-in-Aid 14GRNT20460167 (XL), and RO1 NS075156 (ZZ).
Conflict of Interest
The authors declare that the research was conducted in the absence of any commercial or financial relationships that could be construed as a potential conflict of interest.
The handling editor is currently organizing a research topic with one of the authors XL.
References
1. Brownlee M. Biochemistry and molecular cell biology of diabetic complications. Nature. (2001) 414:813–20. doi: 10.1038/414813a
2. Boulton AJ, Vinik AI, Arezzo JC, Bril V, Feldman EL, Freeman R, et al. Diabetic neuropathies: a statement by the American diabetes association. Diabetes Care. (2005) 28:956–62. doi: 10.2337/diacare.28.4.956
3. Feldman EL, Callaghan BC, Pop-Busui R, Zochodne DW, Wright DE, Bennett DL, et al. Diabetic neuropathy. Nat Rev Dis Primers. (2019) 5:41. doi: 10.1038/s41572-019-0092-1
4. Said G. Diabetic neuropathy–a review. Nat Clin Pract Neurol. (2007) 3:331–40. doi: 10.1038/ncpneuro0504
5. Callaghan BC, Cheng HT, Stables CL, Smith AL, Feldman EL. Diabetic neuropathy: clinical manifestations and current treatments. Lancet Neurol. (2012) 11:521–34. doi: 10.1016/S1474-4422(12)70065-0
6. Feldman EL, Nave KA, Jensen TS, Bennett DLH. New horizons in diabetic neuropathy: mechanisms, bioenergetics, and pain. Neuron. (2017) 93:1296–313. doi: 10.1016/j.neuron.2017.02.005
7. Ramdharry G. Peripheral nerve disease. Handb Clin Neurol. (2018) 159:403–15. doi: 10.1016/B978-0-444-63916-5.00026-4
8. Braune HJ. Early detection of diabetic neuropathy: a neurophysiological study on 100 patients. Electromyogr Clin Neurophysiol. (1997) 37:399–407.
9. Kanade RV, Van Deursen RW, Harding KG, Price PE. Investigation of standing balance in patients with diabetic neuropathy at different stages of foot complications. Clin Biomech. (2008) 23:1183–91. doi: 10.1016/j.clinbiomech.2008.06.004
10. Boulton AJ, Kirsner RS, Vileikyte L. Clinical practice. Neuropathic diabetic foot ulcers. N Engl J Med. (2004) 351:48–55. doi: 10.1056/NEJMcp032966
11. Sen S, Barsun A, Romanowski K, Palmieri T, Greenhalgh D. Neuropathy may be an independent risk factor for amputation after lower-extremity burn in adults with diabetes. Clin Diabetes. (2019) 37:352–6. doi: 10.2337/cd18-0066
12. Vileikyte L, Crews RT, Reeves ND. Psychological and biomechanical aspects of patient adaptation to diabetic neuropathy and foot ulceration. Curr Diab Rep. (2017) 17:109. doi: 10.1007/s11892-017-0945-5
13. Donnan J, Ledger S. An update on the treatment and management of diabetic peripheral neuropathy. CANNT J. (2006) 16:32−6; quiz 37–38.
14. Zilliox LA, Russell JW. Physical activity and dietary interventions in diabetic neuropathy: a systematic review. Clin Auton Res. (2019) 29:443–55. doi: 10.1007/s10286-019-00607-x
15. Callaghan BC, Little AA, Feldman EL, Hughes RA. Enhanced glucose control for preventing and treating diabetic neuropathy. Cochrane Database Syst Rev. (2012) 6:CD007543. doi: 10.1002/14651858.CD007543.pub2
16. Simeoli R, Fierabracci, A. Insights into the role of microRNAs in the onset and development of diabetic neuropathy. Int J Mol Sci. (2019) 20:4627. doi: 10.3390/ijms20184627
17. Bartel DP. MicroRNAs: target recognition and regulatory functions. Cell. (2009) 136:215–33. doi: 10.1016/j.cell.2009.01.002
18. Iwakawa HO, Tomari Y. The functions of microRNAs: mRNA decay and translational repression. Trends Cell Biol. (2015) 25:651–65. doi: 10.1016/j.tcb.2015.07.011
19. Chen X, Liang H, Zhang J, Zen K, Zhang CY. Secreted microRNAs: a new form of intercellular communication. Trends Cell Biol. (2012) 22:125–32. doi: 10.1016/j.tcb.2011.12.001
20. Joglekar MV, Januszewski AS, Jenkins AJ, Hardikar AA. Circulating microRNA biomarkers of diabetic retinopathy. Diabetes. (2016) 65:22–4. doi: 10.2337/dbi15-0028
21. Xourgia E, Papazafiropoulou A, Melidonis A. Circulating microRNAs as biomarkers for diabetic neuropathy: a novel approach. World J Exp Med. (2018) 8:18–23. doi: 10.5493/wjem.v8.i3.18
22. Massaro JD, Polli CD, Costa ESM, Alves CC, Passos GA, Sakamoto-Hojo ET, et al. Post-transcriptional markers associated with clinical complications in Type 1 and Type 2 diabetes mellitus. Mol Cell Endocrinol. (2019) 490:1–14. doi: 10.1016/j.mce.2019.03.008
23. Santos-Bezerra DP, Santos AS, Guimaraes GC, Admoni SN, Perez RV, Machado CG, et al. Micro-RNAs 518d-3p and 618 are upregulated in individuals with type 1 diabetes with multiple microvascular complications. Front Endocrinol. (2019) 10:385. doi: 10.3389/fendo.2019.00385
24. Thery C, Zitvogel L, Amigorena S. Exosomes: composition, biogenesis and function. Nat Rev Immunol. (2002) 2:569–79. doi: 10.1038/nri855
25. Rayner KJ, Hennessy EJ. Extracellular communication via microRNA: lipid particles have a new message. J Lipid Res. (2013) 54:1174–81. doi: 10.1194/jlr.R034991
26. Zhou J, Zhou S. Inflammation: therapeutic targets for diabetic neuropathy. Mol Neurobiol. (2014) 49:536–46. doi: 10.1007/s12035-013-8537-0
27. Pop-Busui R, Ang L, Holmes C, Gallagher K, Feldman EL. Inflammation as a therapeutic target for diabetic neuropathies. Curr Diab Rep. (2016) 16:29. doi: 10.1007/s11892-016-0727-5
28. Greene DA, Lattimer S, Ulbrecht J, Carroll P. Glucose-induced alterations in nerve metabolism: current perspective on the pathogenesis of diabetic neuropathy and future directions for research and therapy. Diabetes Care. (1985) 8:290–9. doi: 10.2337/diacare.8.3.290
29. Fried LF, Forrest KY, Ellis D, Chang Y, Silvers N, Orchard TJ. Lipid modulation in insulin-dependent diabetes mellitus: effect on microvascular outcomes. J Diabetes Complications. (2001) 15:113–9. doi: 10.1016/S1056-8727(01)00140-4
30. Hamada Y. Metabolic factors in the pathogenesis of diabetic neuropathies. Nihon Rinsho. (2005) 63(Suppl. 6):504–8.
31. Dobretsov M, Romanovsky D, Stimers JR. Early diabetic neuropathy: triggers and mechanisms. World J Gastroenterol. (2007) 13:175–91. doi: 10.3748/wjg.v13.i2.175
32. Vincent AM, Hinder LM, Pop-Busui R, Feldman EL. Hyperlipidemia: a new therapeutic target for diabetic neuropathy. J Peripher Nerv Syst. (2009) 14:257–67. doi: 10.1111/j.1529-8027.2009.00237.x
33. Perkins BA, Dholasania A, Buchanan RA, Bril V. Short-term metabolic change is associated with improvement in measures of diabetic neuropathy: a 1-year placebo cohort analysis. Diabet Med. (2010) 27:1271–9. doi: 10.1111/j.1464-5491.2010.03110.x
34. Boulton AJ, Knight G, Drury J, Ward JD. The prevalence of symptomatic, diabetic neuropathy in an insulin-treated population. Diabetes Care. (1985) 8:125–8. doi: 10.2337/diacare.8.2.125
35. Eichberg J. Protein kinase C changes in diabetes: is the concept relevant to neuropathy? Int Rev Neurobiol. (2002) 50:61–82. doi: 10.1016/S0074-7742(02)50073-8
36. Brussee V, Cunningham FA, Zochodne DW. Direct insulin signaling of neurons reverses diabetic neuropathy. Diabetes. (2004) 53:1824–30. doi: 10.2337/diabetes.53.7.1824
37. Obrosova IG, Drel VR, Pacher P, Ilnytska O, Wang ZQ, Stevens MJ, et al. Oxidative-nitrosative stress and poly(ADP-ribose) polymerase (PARP) activation in experimental diabetic neuropathy: the relation is revisited. Diabetes. (2005) 54:3435–41. doi: 10.2337/diabetes.54.12.3435
38. Wada R, Yagihashi S. Role of advanced glycation end products and their receptors in development of diabetic neuropathy. Ann N Y Acad Sci. (2005) 1043:598–604. doi: 10.1196/annals.1338.067
39. Sugimoto K, Yasujima M, Yagihashi S. Role of advanced glycation end products in diabetic neuropathy. Curr Pharm Des. (2008) 14:953–61. doi: 10.2174/138161208784139774
40. Obrosova IG, Stavniichuk R, Drel VR, Shevalye H, Vareniuk I, Nadler JL, et al. Different roles of 12/15-lipoxygenase in diabetic large and small fiber peripheral and autonomic neuropathies. Am J Pathol. (2010) 177:1436–47. doi: 10.2353/ajpath.2010.100178
41. Stavniichuk R, Drel VR, Shevalye H, Vareniuk I, Stevens MJ, Nadler JL, et al. Role of 12/15-lipoxygenase in nitrosative stress and peripheral prediabetic and diabetic neuropathies. Free Radic Biol Med. (2010) 49:1036–45. doi: 10.1016/j.freeradbiomed.2010.06.016
42. Hosseini A, Abdollahi M. Diabetic neuropathy and oxidative stress: therapeutic perspectives. Oxid Med Cell Longev. (2013) 2013:168039. doi: 10.1155/2013/168039
43. Lupachyk S, Watcho P, Shevalye H, Vareniuk I, Obrosov A, Obrosova IG, et al. Na+/H+ exchanger 1 inhibition reverses manifestation of peripheral diabetic neuropathy in type 1 diabetic rats. Am J Physiol Endocrinol Metab. (2013) 305:E396–404. doi: 10.1152/ajpendo.00186.2013
44. Baynes JW, Thorpe SR. Role of oxidative stress in diabetic complications: a new perspective on an old paradigm. Diabetes. (1999) 48:1–9. doi: 10.2337/diabetes.48.1.1
45. Du XL, Edelstein D, Rossetti L, Fantus IG, Goldberg H, Ziyadeh F, et al. Hyperglycemia-induced mitochondrial superoxide overproduction activates the hexosamine pathway and induces plasminogen activator inhibitor-1 expression by increasing Sp1 glycosylation. Proc Natl Acad Sci USA. (2000) 97:12222–6. doi: 10.1073/pnas.97.22.12222
46. Kasznicki J, Kosmalski M, Sliwinska A, Mrowicka M, Stanczyk M, Majsterek I, et al. Evaluation of oxidative stress markers in pathogenesis of diabetic neuropathy. Mol Biol Rep. (2012) 39:8669–78. doi: 10.1007/s11033-012-1722-9
47. Hinokio Y, Suzuki S, Hirai M, Chiba M, Hirai A, Toyota T. Oxidative DNA damage in diabetes mellitus: its association with diabetic complications. Diabetologia. (1999) 42:995–8. doi: 10.1007/s001250051258
48. Vincent AM, Russell JW, Low P, Feldman EL. Oxidative stress in the pathogenesis of diabetic neuropathy. Endocr Rev. (2004) 25:612–28. doi: 10.1210/er.2003-0019
49. Pop-Busui R, Sima A, Stevens M. Diabetic neuropathy and oxidative stress. Diabetes Metab Res Rev. (2006) 22:257–73. doi: 10.1002/dmrr.625
50. Zhang Y, Song C, Liu J, Bi Y, Li H. Inhibition of miR-25 aggravates diabetic peripheral neuropathy. Neuroreport. (2018) 29:945–53. doi: 10.1097/WNR.0000000000001058
51. Wu Y, Xu D, Zhu X, Yang G, Ren M. MiR-106a associated with diabetic peripheral neuropathy through the regulation of 12/15-LOX-meidiated oxidative/nitrative stress. Curr Neurovasc Res. (2017) 14:117–24. doi: 10.2174/1567202614666170404115912
52. Finnerup NB, Sindrup SH, Jensen TS. The evidence for pharmacological treatment of neuropathic pain. Pain. (2010) 150:573–81. doi: 10.1016/j.pain.2010.06.019
53. Andriambeloson E, Baillet C, Vitte PA, Garotta G, Dreano M, Callizot N. Interleukin-6 attenuates the development of experimental diabetes-related neuropathy. Neuropathology. (2006) 26:32–42. doi: 10.1111/j.1440-1789.2006.00651.x
54. Wang Y, Schmeichel AM, Iida H, Schmelzer JD, Low PA. Enhanced inflammatory response via activation of NF-kappaB in acute experimental diabetic neuropathy subjected to ischemia-reperfusion injury. J Neurol Sci. (2006) 247:47–52. doi: 10.1016/j.jns.2006.03.011
55. Hur J, Sullivan KA, Pande M, Hong Y, Sima AA, Jagadish HV, et al. The identification of gene expression profiles associated with progression of human diabetic neuropathy. Brain. (2011) 134:3222–35. doi: 10.1093/brain/awr228
56. Hussain G, Rizvi SA, Singhal S, Zubair M, Ahmad, J. Serum levels of TGF-beta1 in patients of diabetic peripheral neuropathy and its correlation with nerve conduction velocity in type 2 diabetes mellitus. Diabetes Metab Syndr. (2016) 10(1 Suppl. 1):S135–9. doi: 10.1016/j.dsx.2015.10.011
57. Herder C, Kannenberg JM, Huth C, Carstensen-Kirberg M, Rathmann W, Koenig W, et al. Proinflammatory cytokines predict the incidence and progression of distal sensorimotor polyneuropathy: KORA F4/FF4 study. Diabetes Care. (2017) 40:569–76. doi: 10.2337/dc16-2259
58. Gong Q, Lu Z, Huang Q, Ruan L, Chen J, Liang Y, et al. Altered microRNAs expression profiling in mice with diabetic neuropathic pain. Biochem Biophys Res Commun. (2015) 456:615–20. doi: 10.1016/j.bbrc.2014.12.004
59. Liu W, Ao Q, Guo Q, He W, Peng L, Jiang J, et al. miR-9 mediates CALHM1-Activated ATP-P2X7R signal in painful diabetic neuropathy rats. Mol Neurobiol. (2017) 54:922–9. doi: 10.1007/s12035-016-9700-1
60. Khamaneh AM, Alipour MR, Sheikhzadeh Hesari F, Ghadiri Soufi F. A signature of microRNA-155 in the pathogenesis of diabetic complications. J Physiol Biochem. (2015) 71:301–9. doi: 10.1007/s13105-015-0413-0
61. Yang D, Yang Q, Wei X, Liu Y, Ma D, Li J, et al. The role of miR-190a-5p contributes to diabetic neuropathic pain via targeting SLC17A6. J Pain Res. (2017) 10:2395–403. doi: 10.2147/JPR.S133755
62. Chen J, Liu W, Yi H, Hu X, Peng L, Yang F. MicroRNA-155 mimics ameliorates nerve conduction velocities and suppresses hyperglycemia-induced pro-inflammatory genes in diabetic peripheral neuropathic mice. Am J Transl Res. (2019) 11:3905–18.
63. Liu XS, Fan B, Szalad A, Jia L, Wang L, Wang X, et al. MicroRNA-146a mimics reduce the peripheral neuropathy in type 2 diabetic mice. Diabetes. (2017) 66:3111–21. doi: 10.2337/db16-1182
64. Feng Y, Chen L, Luo Q, Wu M, Chen Y, Shi X. Involvement of microRNA-146a in diabetic peripheral neuropathy through the regulation of inflammation. Drug Des Devel Ther. (2018) 12:171–7. doi: 10.2147/DDDT.S157109
65. Balasubramanyam M, Aravind S, Gokulakrishnan K, Prabu P, Sathishkumar C, Ranjani H, et al. Impaired miR-146a expression links subclinical inflammation and insulin resistance in Type 2 diabetes. Mol Cell Biochem. (2011) 351:197–205. doi: 10.1007/s11010-011-0727-3
66. Baldeon RL, Weigelt K, de Wit H, Ozcan B, van Oudenaren A, Sempertegui F, et al. Decreased serum level of miR-146a as sign of chronic inflammation in type 2 diabetic patients. PLoS ONE. (2014) 9:e115209. doi: 10.1371/journal.pone.0115209
67. Feng B, Chen S, Gordon AD, Chakrabarti S. miR-146a mediates inflammatory changes and fibrosis in the heart in diabetes. J Mol Cell Cardiol. (2017) 105:70–6. doi: 10.1016/j.yjmcc.2017.03.002
68. Yousefzadeh N, Alipour MR, Soufi FG. Deregulation of NF-small ka, CyrillicB-miR-146a negative feedback loop may be involved in the pathogenesis of diabetic neuropathy. J Physiol Biochem. (2015) 71:51–8. doi: 10.1007/s13105-014-0378-4
69. Lu Y, Cao DL, Jiang BC, Yang T, Gao YJ. MicroRNA-146a-5p attenuates neuropathic pain via suppressing TRAF6 signaling in the spinal cord. Brain Behav Immun. (2015) 49:119–29. doi: 10.1016/j.bbi.2015.04.018
70. Luo Q, Feng Y, Xie Y, Shao Y, Wu M, Deng X, et al. Nanoparticle-microRNA-146a-5p polyplexes ameliorate diabetic peripheral neuropathy by modulating inflammation and apoptosis. Nanomedicine. (2019) 17:188–97. doi: 10.1016/j.nano.2019.01.007
71. Sasaki H, Schmelzer JD, Zollman PJ, Low PA. Neuropathology and blood flow of nerve, spinal roots and dorsal root ganglia in longstanding diabetic rats. Acta Neuropathol. (1997) 93:118–28. doi: 10.1007/s004010050592
72. Cavanaugh DJ, Lee H, Lo L, Shields SD, Zylka MJ, Basbaum AI, et al. Distinct subsets of unmyelinated primary sensory fibers mediate behavioral responses to noxious thermal and mechanical stimuli. Proc Natl Acad Sci USA. (2009) 106:9075–80. doi: 10.1073/pnas.0901507106
73. Holland NR, Stocks A, Hauer P, Cornblath DR, Griffin JW, McArthur JC. Intraepidermal nerve fiber density in patients with painful sensory neuropathy. Neurology. (1997) 48:708–11. doi: 10.1212/WNL.48.3.708
74. Leinninger GM, Edwards JL, Lipshaw MJ, Feldman EL. Mechanisms of disease: mitochondria as new therapeutic targets in diabetic neuropathy. Nat Clin Pract Neurol. (2006) 2:620–8. doi: 10.1038/ncpneuro0320
75. Cheng C, Kobayashi M, Martinez JA, Ng H, Moser JJ, Wang X, et al. Evidence for epigenetic regulation of gene expression and function in chronic experimental diabetic neuropathy. J Neuropathol Exp Neurol. (2015) 74:804–17. doi: 10.1097/NEN.0000000000000219
76. Hur J, O'Brien PD, Nair V, Hinder LM, McGregor BA, Jagadish HV, et al. Transcriptional networks of murine diabetic peripheral neuropathy and nephropathy: common and distinct gene expression patterns. Diabetologia. (2016) 59:1297–306. doi: 10.1007/s00125-016-3913-8
77. Zhang X, Gong X, Han S, Zhang Y. MiR-29b protects dorsal root ganglia neurons from diabetic rat. Cell Biochem Biophys. (2014) 70:1105–11. doi: 10.1007/s12013-014-0029-y
78. Jia L, Wang L, Chopp M, Zhang Y, Szalad A, Zhang ZG. MicroRNA 146a locally mediates distal axonal growth of dorsal root ganglia neurons under high glucose and sildenafil conditions. Neuroscience. (2016) 329:43–53. doi: 10.1016/j.neuroscience.2016.05.005
79. Jia L, Chopp M, Wang L, Lu X, Zhang Y, Szalad A, et al. MiR-34a regulates axonal growth of dorsal root ganglia neurons by targeting FOXP2 and VAT1 in postnatal and adult mouse. Mol Neurobiol. (2018) 55:9089–99. doi: 10.1007/s12035-018-1047-3
80. Jia L, Wang L, Chopp M, Li C, Zhang Y, Szalad A, et al. MiR-29c/PRKCI regulates axonal growth of dorsal root ganglia neurons under hyperglycemia. Mol Neurobiol. (2018) 55:851–8. doi: 10.1007/s12035-016-0374-5
81. Huebner EA, Strittmatter SM. Axon regeneration in the peripheral and central nervous systems. Results Probl Cell Differ. (2009) 48:339–51. doi: 10.1007/400_2009_19
82. Wang L, Chopp M, Szalad A, Zhang Y, Wang X, Zhang RL, et al. The role of miR-146a in dorsal root ganglia neurons of experimental diabetic peripheral neuropathy. Neuroscience. (2014) 259:155–63. doi: 10.1016/j.neuroscience.2013.11.057
83. Goncalves NP, Vaegter CB, Andersen H, Ostergaard L, Calcutt NA, Jensen TS. Schwann cell interactions with axons and microvessels in diabetic neuropathy. Nat Rev Neurol. (2017) 13:135–47. doi: 10.1038/nrneurol.2016.201
84. Jessen KR, Mirsky R, Lloyd AC. Schwann cells: development and role in nerve repair. Cold Spring Harb Perspect Biol. (2015) 7:a020487. doi: 10.1101/cshperspect.a020487
85. Tomlinson DR, Gardiner NJ. Glucose neurotoxicity. Nat Rev Neurosci. (2008) 9:36–45. doi: 10.1038/nrn2294
86. Goncalves NP, Vaegter CB, Pallesen LT. peripheral glial cells in the development of diabetic neuropathy. Front Neurol. (2018) 9:268. doi: 10.3389/fneur.2018.00268
87. Hao W, Tashiro S, Hasegawa T, Sato Y, Kobayashi T, Tando T, et al. Hyperglycemia promotes schwann cell de-differentiation and de-myelination via sorbitol accumulation and igf1 protein down-regulation. J Biol Chem. (2015) 290:17106–15. doi: 10.1074/jbc.M114.631291
88. Tang W, Lv Q, Chen XF, Zou JJ, Liu ZM, Shi YQ. CD8(+) T cell-mediated cytotoxicity toward Schwann cells promotes diabetic peripheral neuropathy. Cell Physiol Biochem. (2013) 32:827–37. doi: 10.1159/000354485
89. Viader A, Sasaki Y, Kim S, Strickland A, Workman CS, Yang K, et al. Aberrant schwann cell lipid metabolism linked to mitochondrial deficits leads to axon degeneration and neuropathy. Neuron. (2013) 77:886–98. doi: 10.1016/j.neuron.2013.01.012
90. Sohn EJ, Park HT. MicroRNA mediated regulation of schwann cell migration and proliferation in peripheral nerve injury. Biomed Res Int. (2018) 2018:8198365. doi: 10.1155/2018/8198365
91. Pereira JA, Baumann R, norrmen c, somandin c, miehe m, jacob c, et al. dicer in schwann cells is required for myelination and axonal integrity. J Neurosci. (2010) 30:6763–75. doi: 10.1523/JNEUROSCI.0801-10.2010
92. Viader A, Chang LW, Fahrner T, Nagarajan R, Milbrandt J. MicroRNAs modulate schwann cell response to nerve injury by reinforcing transcriptional silencing of dedifferentiation-related genes. J Neurosci. (2011) 31:17358–69. doi: 10.1523/JNEUROSCI.3931-11.2011
93. Li S, Wang X, Gu Y, Chen C, Wang Y, Liu J, et al. Let-7 microRNAs regenerate peripheral nerve regeneration by targeting nerve growth factor. Mol Ther. (2015) 23:423–33. doi: 10.1038/mt.2014.220
94. Zhou S, Gao R, Hu W, Qian T, Wang N, Ding G, et al. MiR-9 inhibits schwann cell migration by targeting cthrc1 following sciatic nerve injury. J Cell Sci. (2014) 127:967–76. doi: 10.1242/jcs.131672
95. Yi S, Wang S, Zhao Q, Yao C, Gu Y, Liu J, et al. miR-sc3, a novel microRNA, promotes schwann cell proliferation and migration by targeting astn1. Cell Transplant. (2016) 25:973–82. doi: 10.3727/096368916X690520
96. Yi S, Wang QH, Zhao LL, Qin J, Wang YX, Yu B, et al. miR-30c promotes schwann cell remyelination following peripheral nerve injury. Neural Regen Res. (2017) 12:1708–15. doi: 10.4103/1673-5374.217351
97. Liu Y, Chen X, Yao J, Kang J. Circular RNA ACR relieves high glucose-aroused RSC96 cell apoptosis and autophagy via declining microRNA-145-3p. J Cell Biochem. (2019). 1–10. doi: 10.1002/jcb.29568
98. Cameron NE, Eaton SE, Cotter MA, Tesfaye S. Vascular factors and metabolic interactions in the pathogenesis of diabetic neuropathy. Diabetologia. (2001) 44:1973–88. doi: 10.1007/s001250100001
99. Cameron NE, Cotter MA. The relationship of vascular changes to metabolic factors in diabetes mellitus and their role in the development of peripheral nerve complications. Diabetes Metab Rev. (1994) 10:189–224. doi: 10.1002/dmr.5610100302
100. Sinnreich M, Taylor BV, Dyck PJ. Diabetic neuropathies. Classification, clinical features, and pathophysiological basis. Neurologist. (2005) 11:63–79. doi: 10.1097/01.nrl.0000156314.24508.ed
101. Lim TK, Shi XQ, Johnson JM, Rone MB, Antel JP, David S, et al. Peripheral nerve injury induces persistent vascular dysfunction and endoneurial hypoxia, contributing to the genesis of neuropathic pain. J Neurosci. (2015) 35:3346–59. doi: 10.1523/JNEUROSCI.4040-14.2015
102. Doupis J, Lyons TE, Wu S, Gnardellis C, Dinh T, Veves A. Microvascular reactivity and inflammatory cytokines in painful and painless peripheral diabetic neuropathy. J Clin Endocrinol Metab. (2009) 94:2157–63. doi: 10.1210/jc.2008-2385
103. Zhang Y, Sun X, Icli B, Feinberg MW. Emerging roles for microRNAs in diabetic microvascular disease: novel targets for therapy. Endocr Rev. (2017) 38:145–68. doi: 10.1210/er.2016-1122
104. Li YB, Wu Q, Liu J, Fan YZ, Yu KF, Cai Y. miR199a3p is involved in the pathogenesis and progression of diabetic neuropathy through downregulation of SerpinE2. Mol Med Rep. (2017) 16:2417–24. doi: 10.3892/mmr.2017.6874
105. Kamali K, Korjan ES, Eftekhar E, Malekzadeh K, Soufi FG. The role of miR-146a on NF-kappaB expression level in human umbilical vein endothelial cells under hyperglycemic condition. Bratisl Lek Listy. (2016) 117:376–80. doi: 10.4149/BLL_2016_074
106. Fan B, Liu XS, Szalad A, Wang L, Zhang R, Chopp M, et al. Influence of sex on cognition and peripheral neurovascular function in diabetic mice. Front Neurosci. (2018) 12:795. doi: 10.3389/fnins.2018.00795
107. Perkins BA, Bril V. Diabetic neuropathy: a review emphasizing diagnostic methods. Clin Neurophysiol. (2003) 114:1167–75. doi: 10.1016/S1388-2457(03)00025-7
108. Onde ME, Ozge A, Senol MG, Togrol E, Ozdag F, Saracoglu M, et al. The sensitivity of clinical diagnostic methods in the diagnosis of diabetic neuropathy. J Int Med Res. (2008) 36:63–70. doi: 10.1177/147323000803600109
109. Iqbal Z, Azmi S, Yadav R, Ferdousi M, Kumar M, Cuthbertson DJ, et al. Diabetic peripheral neuropathy: epidemiology, diagnosis, and pharmacotherapy. Clin Ther. (2018) 40:828–49. doi: 10.1016/j.clinthera.2018.04.001
110. Shabeeb D, Najafi M, Hasanzadeh G, Hadian MR, Musa AE, Shirazi A. Electrophysiological measurements of diabetic peripheral neuropathy: a systematic review. Diabetes Metab Syndr. (2018) 12:591–600. doi: 10.1016/j.dsx.2018.03.026
111. Ciccacci C, Latini A, Greco C, Politi C, D'Amato C, Lauro D, et al. Association between a MIR499A polymorphism and diabetic neuropathy in type 2 diabetes. J Diabetes Complicat. (2018) 32:11–7. doi: 10.1016/j.jdiacomp.2017.10.011
112. Leinders M, Uceyler N, Thomann A, Sommer C. Aberrant microRNA expression in patients with painful peripheral neuropathies. J Neurol Sci. (2017) 380:242–9. doi: 10.1016/j.jns.2017.07.041
113. Guo G, Liu Y, Ren S, Kang Y, Duscher D, Machens HG, et al. Comprehensive analysis of differentially expressed microRNAs and mRNAs in dorsal root ganglia from streptozotocin-induced diabetic rats. PLoS ONE. (2018) 13:e0202696. doi: 10.1371/journal.pone.0202696
114. Chien HY, Chen CY, Chiu YH, Lin YC, Li WC. Differential microRNA profiles predict diabetic nephropathy progression in Taiwan. Int J Med Sci. (2016) 13:457–65. doi: 10.7150/ijms.15548
115. Wang G-F, Xu N. Expression and clinical significance of microRNA 146a in peripheral blood mononuclear cells from type 2 diabetic neuropathy patients. Int J Clin Exp Pathol. (2018) 11:7156–64.
116. Li H, Shen L, Ma C, Huang Y. Differential expression of miRNAs in the nervous system of a rat model of bilateral sciatic nerve chronic constriction injury. Int J Mol Med. (2013) 32:219–26. doi: 10.3892/ijmm.2013.1381
117. Wang J, Chen J, Sen S. MicroRNA as biomarkers and diagnostics. J Cell Physiol. (2016) 231:25–30. doi: 10.1002/jcp.25056
118. Arroyo JD, Chevillet JR, Kroh EM, Ruf IK, Pritchard CC, Gibson DF, et al. Argonaute2 complexes carry a population of circulating microRNAs independent of vesicles in human plasma. Proc Natl Acad Sci USA. (2011) 108:5003–8. doi: 10.1073/pnas.1019055108
119. Escudier B, Dorval T, Chaput N, Andre F, Caby MP, Novault S, et al. Vaccination of metastatic melanoma patients with autologous dendritic cell (DC) derived-exosomes: results of thefirst phase I clinical trial. J Transl Med. (2005) 3:10. doi: 10.1186/1479-5876-3-10
120. Kalluri R, LeBleu VS. The biology, function, and biomedical applications of exosomes. Science. (2020) 367:eaau6977. doi: 10.1126/science.aau6977
121. Cantaluppi V, Gatti S, Medica D, Figliolini F, Bruno S, Deregibus MC, et al. Microvesicles derived from endothelial progenitor cells protect the kidney from ischemia-reperfusion injury by microRNA-dependent reprogramming of resident renal cells. Kidney Int. (2012) 82:412–27. doi: 10.1038/ki.2012.105
122. Zhang Y, Chopp M, Liu XS, Katakowski M, Wang X, Tian X, et al. Exosomes derived from mesenchymal stromal cells promote axonal growth of cortical neurons. Mol Neurobiol. (2017) 54:2659–73. doi: 10.1007/s12035-016-9851-0
123. Lopez-Verrilli MA, Picou F, Court FA. Schwann cell-derived exosomes enhance axonal regeneration in the peripheral nervous system. Glia. (2013) 61:1795–806. doi: 10.1002/glia.22558
124. Wang L, Chopp M, Szalad A, Lu X, Zhang Y, Wang X, et al. Exosomes derived from schwann cells ameliorate peripheral neuropathy in type II diabetic mice. Diabetes. (2020) 69:749–59. doi: 10.2337/db19-0432
125. Jia L, Chopp M, Wang L, Lu X, Szalad A, Zhang G, et al. Exosomes derived from high-glucose-stimulated schwann cells promote development of diabetic peripheral neuropathy. FASEB J. (2018) 32:fj201800597R. doi: 10.1096/fj.201800597R
126. Ferguson SW, Wang J, Lee CJ, Liu M, Neelamegham S, Canty JM, et al. The microRNA regulatory landscape of MSC-derived exosomes: a systems view. Sci Rep. (2018) 8:1419. doi: 10.1038/s41598-018-19581-x
127. Fan B, Li C, Szalad A, Wang L, Pan W, Zhang R, et al. Mesenchymal stromal cell-derived exosomes ameliorate peripheral neuropathy in a mouse model of diabetes. Diabetologia. (2020) 63:431–43. doi: 10.1007/s00125-019-05043-0
Keywords: diabetic neuropathy, microRNA, neurovascular dysfunction, extracellular vesicle (EV), exosome
Citation: Fan B, Chopp M, Zhang ZG and Liu XS (2020) Emerging Roles of microRNAs as Biomarkers and Therapeutic Targets for Diabetic Neuropathy. Front. Neurol. 11:558758. doi: 10.3389/fneur.2020.558758
Received: 04 May 2020; Accepted: 16 September 2020;
Published: 22 October 2020.
Edited by:
Hsiuying Wang, National Chiao Tung University, TaiwanReviewed by:
Manabu Funayama, Juntendo University, JapanGanesh Chauhan, Indian Institute of Science, India
Copyright © 2020 Fan, Chopp, Zhang and Liu. This is an open-access article distributed under the terms of the Creative Commons Attribution License (CC BY). The use, distribution or reproduction in other forums is permitted, provided the original author(s) and the copyright owner(s) are credited and that the original publication in this journal is cited, in accordance with accepted academic practice. No use, distribution or reproduction is permitted which does not comply with these terms.
*Correspondence: Xian Shuang Liu, eGxpdTJAaGZocy5vcmc=