- 1Department of Anesthesiology, Tianjin Medical University General Hospital, Tianjin, China
- 2Tianjin Institute of Anesthesiology, Tianjin, China
Introduction: Sevoflurane is the most commonly used general anesthetic in pediatric surgery, but it has the potential to be neurotoxic. Previous research found that long-term or multiple sevoflurane exposures could cause cognitive deficits in newborn mice but not adult mice, whereas short-term or single inhalations had little effect on cognitive function at both ages. The mechanisms behind these effects, however, are unclear.
Methods: In the current study, 6- and 60-day-old C57bl mice in the sevoflurane groups were given 3% sevoflurane plus 60% oxygen for three consecutive days, each lasting 2 hours, while those in the control group only got 60% oxygen. The cortex tissues were harvested on the 8th or 62nd day. The tandem mass tags (TMT)pro-based quantitative proteomics combined with liquid chromatography-tandem mass spectrometry (LC-MS/MS) analysis, Golgi staining, and western blotting analysis were applied to analyze the influences of multiple sevoflurane anesthesia on the cerebral cortex in mice with various ages. The Morris water maze (MWM) test was performed from postnatal day (P)30 to P36 or P84 to P90 after control or multiple sevoflurane treatment. Sevoflurane anesthesia affected spatial learning and memory and diminished dendritic spines primarily in newborn mice, whereas mature animals exhibited no significant alterations.
Results: A total of 6247 proteins were measured using the combined quantitative proteomics methods of TMTpro-labeled and LC-MS/MS, 443 of which were associated to the age-dependent neurotoxic mechanism of repeated sevoflurane anesthesia. Furthermore, western blotting research revealed that sevoflurane-induced brain damage in newborn mice may be mediated by increasing the levels of protein expression of CHGB, PTEN, MAP2c, or decreasing the level of SOD2 protein expression.
Conclusion: Our findings would help to further the mechanistic study of age-dependent anesthetic neurotoxicity and contribute to seek for effective protection in the developing brain under general anesthesia.
1. Introduction
With rapid advances in anesthetic technology, millions of newborns and children worldwide undergo surgical interventions using general anesthesia, rendering child safety a major public health concern (1). Sevoflurane is the most commonly employed inhalational anesthetic in pediatric surgery (2). It has been reported that repeated or long-term sevoflurane exposure prior to 3–4 years of age can increase the potential for future learning and memory challenges (3–5), although available data remain debatable (6). Furthermore, our previous studies have demonstrated that multiple exposures to inhalational anesthetics, such as sevoflurane, can cause adverse effects, including neuroinflammation, apoptosis, synaptic insufficiency, and cognitive deficits in 6-day-old newborn mice, while 60-day-old adult mice showed no notable damage (7–11). The mechanisms underlying these age-dependent effects remain elusive.
Quantitative proteomics is a precise method for identifying differentially expressed proteins (DEPs) in biological processes or diseases and predicting therapeutic drug targets and underlying mechanisms (12). The continual development and application of molecular-based technologies have allowed researchers to explore the features of complex regulatory systems (13). Tandem mass tag (TMT)pro-based quantitative proteomics, a quantitative proteomics method, allows a large number of samples to be identified concurrently, thereby reducing batch effects (14). To further investigate the mechanism of age-dependent anesthetic neurotoxicity induced by sevoflurane, we used a combination of TMTpro-labeled quantitative proteomics and liquid chromatography-tandem mass spectrometry (LC-MS/MS) to identify DEPs after multiple inhalations of 3% sevoflurane plus 60% oxygen or 60% oxygen alone in neonatal and adult mice.
2. Materials and methods
2.1. Animals and experimental design
Pregnant mice (gestation days 16–17) and 60-day-old female C57BL/6J mice were purchased from Sibeifu Bioscience Company (license number, SCXK 2019-0010; Beijing, China). The mice were housed under 12 h of natural light and 12 h of darkness at a constant temperature (23 ± 1°C), with unrestricted access to food and water. Female and male newborn mouse pups delivered by pregnant mice were selected for experiments, including proteome analysis and western blotting, whereas only neonatal female mice were selected to undergo behavioral testing and Golgi staining. Mice were randomly allocated to postnatal day (P) 6 + Control, P6 + Sevoflurane, P60 + Control, and P60 + Sevoflurane groups. Twenty mice (n = 5 mice/group) were used for cerebral cortex proteomic analysis, 40 were subjected to the Morris water maze (MWM) test (n = 10 mice/group), 12 underwent Golgi staining (n = 3 mice/group), and 20 were used for western blotting analysis (n = 5 mice/group; Figure 1). All experiments were approved by the Animal Experimental Ethics Committee of Tianjin Medical University General Hospital in Tianjin, China (Approval No. IRB2021-DWFL-210). Every effort was made to reduce the suffering of mice and the number of animals used.
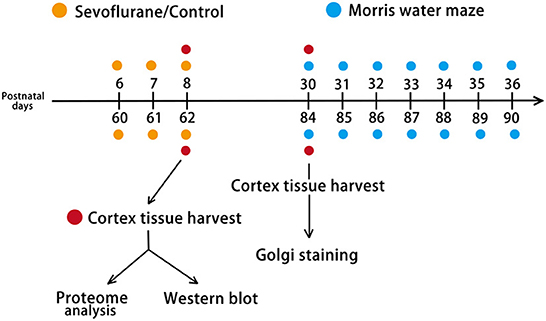
Figure 1. Experimental design. Mice were randomly assigned to four groups: P6 + Control, P6 + Sevoflurane, P60 + Control, and P60 + Sevoflurane. P6 (female plus male) and P60 (female) mice were subjected to 60% O2 + 3% sevoflurane or 60% O2 inhalation for 2 h over 3 consecutive days. Two hours after the last treatment, the cerebral cortex was harvested for TMT-based quantitative proteome analysis and western blotting. After 22 days of treatment, female mice were selected for the Morris water maze (MWM) test and Golgi staining. P6, postnatal day 6; P60, postnatal day 60; TMT, Tandem mass tag.
2.2. Multiple sevoflurane exposures and treatment
As described in our previous study (8), mice in the sevoflurane group were anesthetized using 3% sevoflurane and 60% oxygen at a flow rate of 2 L/min for 2 h per day for three consecutive days; the control group inhalationally administered 60% O2. Mice were exposed to sevoflurane in a transparent chamber (25 × 15 × 10 cm) connected with a sevoflurane-specific vaporizer. During treatment, the sevoflurane concentration was monitored using a gas monitor (Vamos/Vamos Plus; Draeger, Germany). The rectal temperature of mice was maintained at 37 ± 0.5°C using a heating blanket.
2.3. MWM
MWM trials were performed as described in our previous study (9). A circular pool (120 cm diameter × 60 cm height) was filled with opaque water using ~0.5 g/L titanium dioxide powder. The hidden white platform (diameter, 10 cm) was positioned in the center of the first quadrant, submerged 1.0 cm below the water surface. The water temperature in the pool was maintained at 23 ± 1°C. We tested P30 and P84 mice for 7 days (P30–P36; P84–P90), with four trials performed daily in the morning (from 8 to 12 am). The swimming behavior of mice was monitored using an automatic tracking system (Xinruan Information Technology Co. Ltd, Shanghai, China). On successfully locating the hidden platform within 90 s, the stopwatch was automatically stopped, and mice were maintained on the platform for 10 s. If a mouse failed to find the platform within 90 s, the animal was manually guided to the platform and then maintained on the platform for 15 s. To determine the spatial learning and memory performance of mice, we analyzed data related to escape latency during the training stage and platform crossing times in the probe test on P36 or P90. Mice were allowed a 30 min rest interval between each trial for daily training. After each training session, a heating pad was used to maintain a stable body temperature for 10 min.
2.4. Golgi staining and dendritic spine density analysis
The dendritic spine density of cortical neurons was identified using the FD Rapid GolgiStain Kit (Cat#: PK401, FD Neuro Technologies, USA). Mice were well-sedated for 4 min with 3% sevoflurane before decapitation on P30 or P84. Brains were subsequently dissected and immersed in a solution of mercuric chloride, potassium dichromate, and potassium chromate at room temperature for 14 days in the dark. The mixed solution was replaced the next day. Brain tissues were transferred to Solution C at room temperature in the dark for 96–120 h, and Solution C was replaced the following day. The impregnated brains were embedded in 4% agarose and sectioned at 120–150 μm using a Leica Vibratome. The slides were immersed in solutions D and E and Milli-Q water mixes. After careful washing with Milli-Q water, the slices were dehydrated with graded alcohol (50, 75, 95, and 100% ethanol in Milli-Q water) and cleared with xylene. Finally, the slides were sealed with neutral gum and analyzed under a bright field using a Nikon Eclipse TE2000U microscope. Ten pyramidal neurons from each mouse were selected in layers 4/5 of the cerebral cortex, and the dendritic spine density was counted using 10 secondary or third intermediate dendrites of 10-μm length under an oil immersion 100× objective. Thirty dendritic segments from each group were quantified using ImageJ2 (version 2.3, National Institutes of Health, USA). Dendritic spine density was calculated and expressed as the mean number of spines per 10 μm dendritic length.
2.5. Sample collection
Twenty mice were briefly anesthetized with sevoflurane 2 h after the end of treatment and decapitated. The cerebral cortex of each mouse was collected in independent cryogenic vials and stored in liquid nitrogen for rapid freezing. Samples were stored at −80°C until subsequent analysis. We selected TMTpro (16plek) based on 20 samples to reduce the loss of quantification data and counts of experimental batches. Two additional internal standards (IS) were introduced into our experiment as an independent group to reduce the impact of various batches. Both IS were mixtures of TMTpro-labeled peptides from 20 samples. Finally, TMT-based quantitative proteomic analysis with five biological replicates was performed on six groups of 22 samples.
2.6. Cortex homogenization and protein digestion
All tissue samples were homogenized on ice using lysis buffer (4% sodium dodecyl sulfate (SDS), 1 mM DTT, 100 mM Tris-HCl [pH 7.6], and protease inhibitor cocktail), and proteins were measured using the BCA protein assay kit (Bio-Rad Laboratories, USA). Briefly, samples were combined with 30 l SDT buffer (4% SDS, 100 mM DTT, 150 mM Tris-HCl, pH 8.0) for protein digestion. The detergent and DTT were subsequently removed using a mixture of uric acid (UA) buffer (8 M urea, 150 mM Tris-HCl, pH 8.0) and repeated ultrafiltration (Microcon units, 10 kD). Iodoacetamide [IAA; 100 μL iodoacetamide (100 mM IAA in UA buffer)] was added to the samples to block reduced cysteine residues, and samples were incubated in the dark for 30 min. The filters were then thrice washed with 100 μL UA buffer and twice with 100 μL 25 mM NH4HCO3 buffer. The peptides were collected as a filtrate after digestion with 4 g trypsin (Promega) in 40 μL 25 mM NH4HCO3 buffer overnight at 37°C. The peptides were desalted using C18 Cartridges [Sigma-Aldrich, USA, EmporeTM SPE Cartridges C18 (standard density) bed I.D. 7 mm, volume 3 mL], vacuum centrifuged, and reconstituted in 40 μL of 0.1% (v/v) formic acid. The extinction coefficient of 1.1 in the 0.1% (g/l) solution was calculated by considering the frequency of tryptophan and tyrosine in vertebrate proteins to estimate the ultraviolet (UV) light spectral density of peptides at 280 nm.
2.7. TMT labeling
For each sample, 100 μg of the peptide mixture was labeled using the TMTpro 16plek label reagent (Cat#: A44520, Thermo Fisher Scientific, USA) according to the manufacturer's instructions. The experiments were conducted in two batches. One batch comprised a set of peptides from the control group (10 samples) and IS, while the other consisted of peptides from sevoflurane groups (10 samples) and IS. The values of each batch were corrected using batch-specific IS. The samples were labeled using 11 of 16 TMTpro labels, including TMTpro-126, TMTpro-127C, TMTpro-127N, TMTpro-128C, TMTpro-128N, TMTpro-129C, TMTpro-129N, TMTpro-130C, TMTpro-130N, TMTpro-131C, and TMTpro-131N.
2.8. High-pH reversed-phase (RP) fractionation
Labeled peptides were fractionated to increase proteome coverage using a high-pH RP Peptide Fractionation Kit (Cat#:84868, Thermo Fisher Scientific, USA). The peptides were vacuum-dried prior to acidification with 0.1% trifluoroacetic acid (TFA) solution, followed by loading onto an equilibrated, high-pH, RP fractionation spin column. Peptides were bound to the hydrophobic resin under aqueous conditions and then desalted by washing the column with water using low-speed centrifugation at 5,000 × g for 2 min. After removing the solution and packing the resin, the liquid was discarded. To elute bound peptides into 10 separate fractions, a stepwise gradient of increasing acetonitrile (ACN) concentrations in a volatile high-pH elution solution was applied to columns recovered by centrifugation. The recovered fractions were desalted on C18 Cartridges (Cat#: EmporeTM SPE Cartridges C18 (standard density) bed I.D. 7 mm, volume 3 ml, Sigma-Aldrich, MO, USA) and vacuum centrifuged. The lyophilized peptide was re-dissolved in 12 μL of 0.1% formic acid solution (FA). The peptide concentration was measured at an optical density of 280 nm (OD280).
2.9. LC-MS/MS analysis
After loading into a reverse-phase trap column (Thermo Fisher Scientific, 100 m 2 cm) linked to a C18 RP analytical column (10 cm, ID75 μm, 3 μm resin), lyophilized peptides were resuspended in buffer A (0.1% FA). The tagged peptides were separated using an IntelliFlow-controlled linear gradient of buffer B (84% ACN and 0.1% FA) at a flow rate of 30 NL/min.
For LC-MS/MS analysis, we used a Q-Exactive mass spectrometer (Thermo Fisher Scientific) and an Easy nLC system. MS data were collected at 70,000 resolutions and 200 m/z in a scan range of 300–1,800 m/z in positive ion mode to detect intact peptides. The AGC goal was set at 1e6, the maximum inject time was 10 ms, and the dynamic exclusion duration was 40.0 s. In total, 20 MS2 scans were obtained based on the following settings to capture the mass-charge ratios of the polypeptide and polypeptide fragments for each entire scan: MS2 was activated with HCD, the isolation window was set to 2 m/z, the resolution of the HCD spectra was set to 17,500 at 200 m/z, the normalized collision energy was 30 eV, and the underfill ratio was 0.1%.
2.10. Protein identification and quantification
The raw data for each sample were processed using Proteome Discover software (version 1.4, Thermo Fisher Scientific) and the MASCOT engine (version 2.2, Matrix Science, London, UK) to match with the Uniport mouse database (“Swissprot_Mus_musculus_17063_20210106.fasta” downloaded from1 on January 6, 2021, and included 17,063 protein sequences). The following search parameters were entered: Mas missed cleavages: 2; peptide mass tolerance: ± 20 ppm; fragment mass tolerance: 0.1 Da; enzyme: Trypsin; Carbamidomethyl (C), TMT 6/10/16 plex (N-term), TMT 6/10/16 plex (K) are fixed modifications, and oxidation (M) is a variable modification. Decoy is a database pattern. Effective peptides had a false discovery rate of <0.01. Protein ratios were determined as the median of distinct peptides used to measure proteins. To account for experimental bias, all protein ratios were standardized using the mean protein ratio. The mass spectrometry proteomics data have been deposited to the ProteomeXchange Consortium via the PRIDE partner repository with the dataset identifier PXD037294.
2.11. Western blot
On completing the sevoflurane treatment, mice were decapitated, and the cerebral cortex was extracted after short-term anesthesia with 3% sevoflurane for 5 min. RIPA buffer (Cat#: R0020, Solarbio, China) and protease inhibitors (Cat#: HY-K0010, MedChemExpress, China) were used for tissue lysis. The extracted proteins were examined to assess the expression of the following proteins and confirm proteomic data: chromogranin B (CHGB), secretogranin-2 (SCG2), phosphatase and tensin homolog (PTEN), microtubule-associated protein 2c (MAP2c), and mitochondrial superoxide dismutase 2 (MSOD2) (SOD2). The protein concentration was measured using a BCA protein assay kit (Cat#: CW0014S, CWBIO, China). Identical protein amounts were loaded onto 4–12% SDS- polyacrylamide gel electrophoresis gels (Cat#: M00652, Gennscript, China), and proteins were transferred to polyvinylidene fluoride membranes (Cat#: IPVH00010, Millipore, US) and blocked with SuperBlock (TBS) Blocking Buffer (Cat#:37536, Thermo Fisher Scientific, USA) for 30 min. Membranes were incubated with the following primary antibodies: CHGB (1:1000; Cat#: ab150354, Abcam, UK), SCG2 (1:800; Cat#: NO.20357-1-AP, ProteinTech, USA), PTEN (1:100; Santa Cruz Biotechnology, USA), MAP2 (1:1000; Cat#: NO. 17490-1-AP, Proteintech, USA), SCG2 (1:1000; Cat#: NO. 24127-1-AP, ProteinTech, USA), and GAPDH (1:5000; Cat#: T0004, Affinity Biosciences, USA) at 4°C overnight. After washing five times with TBST (1× Tris-buffered saline with 0.1% Tween-20), membranes were then incubated with the horseradish peroxidase (HRP)-conjugated goat anti-mouse (1:5000; Cat#: S0002 Affinity Biosciences, USA) or rabbit secondary antibody (1:5000; Cat#: S0001 Affinity Biosciences, USA) at room temperature for 1.5 h, following washing mentioned above. Finally, the protein bands were visualized with ECL solution, and after obtaining images, the primary and secondary antibodies of the membrane were stripped by western blot stripping buffer (Cat#: 21059, ThermoFisher Scientific, USA) for 30–60 min at 37°C, depending on antibody affinity. The next target protein was incubated on the stripped membrane with primary and secondary antibodies, as described previously. ImageJ2 was used to qualify the protein bands (version 2.3, National Institutes of Health, USA). The protein expression levels of CHGB, SCG2, MAP2c, PTEN, and SOD2 were normalized to GAPDH levels. The experiment was performed in triplicate.
2.12. Statistical analysis
Data analyses were performed using GraphPad Prism version 9.0 (GraphPad Software Inc., La Jolla, CA, USA). The results of biochemical experiments are presented as mean ± standard deviation (SD). Values of MWM escape latency are presented as the mean ± standard error of the mean (SEM), and platform crossing numbers are expressed as the median and interquartile range.
A normality test was used to determine whether data values were normally distributed. Two-way repeated-measures ANOVA was used to compare escape latency in behavioral data. The Mann-Whitney test was used to assess the number of platform crossings between the control and sevoflurane anesthesia groups. Two-way ANOVA was performed to examine the influence of age (6-day-old vs. 60-day-old), treatment (control vs. sevoflurane treatment), and the interaction between age and treatment on protein expression levels (CHGB, PTEN, MAP2c, and SOD2). One-way ANOVA was used to examine differences in the relative density of the proteins described above, as well as the density of dendritic spines. P < 0.05 was deemed as a statistically significant difference.
3. Results
3.1. Multiple sevoflurane inhalations induce future spatial learning and memory deficits in newborn but not in adult mice
Data on escape latency and platform crossing numbers were collected throughout the positional navigation training and spatial exploration assessments. Considering the escape latency in the positioning navigation stage, pubertal mice subjected to multiple 3% sevoflurane anesthesia throughout neonatal development exhibited impaired spatial learning (P < 0.05), with no statistical difference observed between the P60 + Control and P60 + Sevoflurane groups (Figure 2A). Furthermore, sevoflurane anesthesia decreased the platform crossing number of adolescent mice when compared with that of control mice (P < 0.05), with no notable difference observed in the adult groups (Figure 2B). These results suggested that multiple sevoflurane doses could impair the spatial learning and memory function of newborn mice during puberty, with no significant effect observed in adult mice.
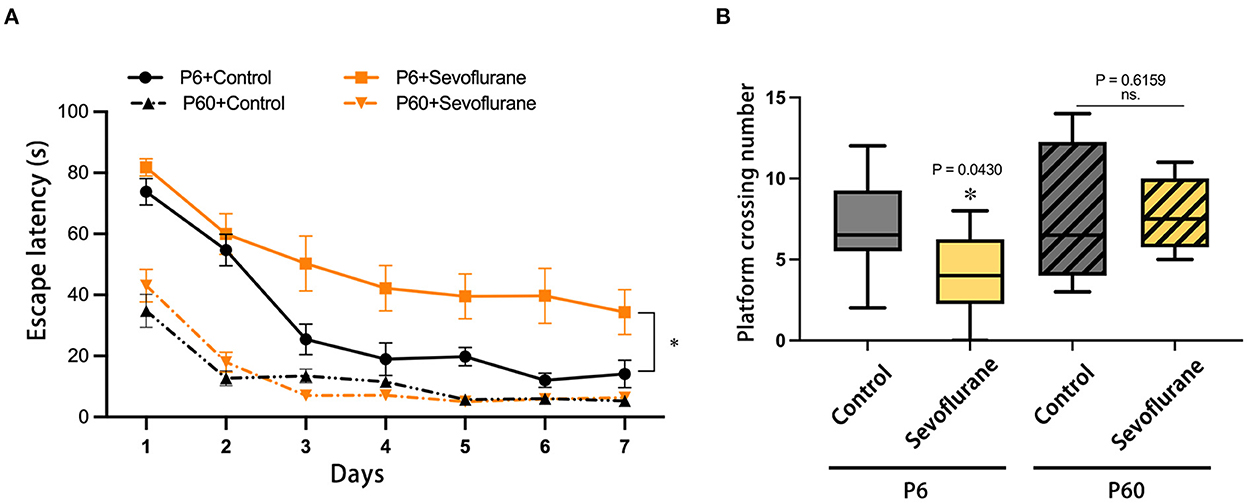
Figure 2. Effects of multiple exposures to sevoflurane anesthesia to neonatal and adult mice on future spatial cognitive function in the test. (A) Swimming escape latency and (B) platform crossing number. n = 10 mice/group. Escape latency results are presented as mean ± standard error of the mean (SEM); the platform crossing number is expressed as median and interquartile range. *P < 0.05 vs. P6 + Control. MWM, Morris water maze; P6, postnatal day 6.
3.2. Changes in dendritic spine density in cerebral cortex
Based on Golgi-Cox staining of the cerebral cortex harvested 22 days post-treatment, pyramidal neurons in layer 4/5 of the P6+Sevoflurane group had a lower dendritic spine density than those of the P6+Control group; multiple sevoflurane exposures did not affect future dendritic spine density in adult mice. The above results were compatible with the behavioral test results (*P <0.05, P6 + Control, Figure 3).
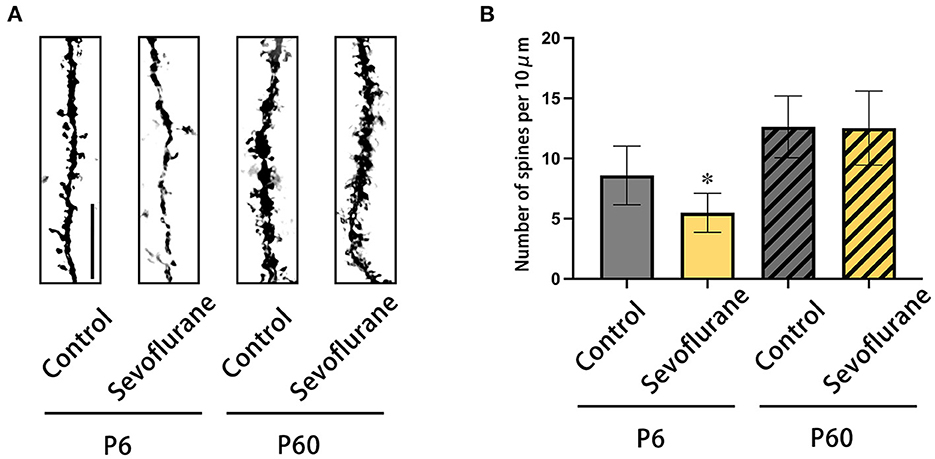
Figure 3. The results of Golgi-Cox staining on the dendrites of pyramidal neurons of cerebral cortex in layers 4/5. (A) Representative photomicrographs of Golgi-Cox-stained dendrites (scale bar, 1 μm). (B) The dendritic spine density (numbers of dendritic spine/10 μm). n = 20 dendrites/mice. *P < 0.05 vs. P6 + Control. P6, postnatal day 6.
3.3. Protein quantitative characterization and DEP screening
A TMT-based quantitative proteomics technique was used to examine the cerebral cortex proteome of different groups. Our findings indicated that 6,861 proteins were discovered, with 6,247 proteins presenting quantitative values and annotation words. In the present study, proteins whose quantitative levels increased or decreased 1.2-fold were deemed DEPs.
Four groups were used for pairwise comparisons. DEPs derived from sevoflurane-induced neurotoxicity in newborn mice met at least one of three criteria: (1) in neonatal mice, sevoflurane caused the differential expression of proteins (DEPs in the P6 + Sevoflurane/P6 + Control), while age induced differential protein expression in control group mice (DEPs in the P60 + Control/P6 + Control). Discrepancies in DEP changes between the two groups implied that sevoflurane anesthesia might suppress age-induced DEPs, therefore promoting neurotoxic vulnerability in young mice (Table 1); (2) sevoflurane caused DEPs in newborn groups (DEPs in the P6 + Sevoflurane/P6 + Control), but age had no effect on the expression of these proteins in the sevoflurane treatment group (non-DEPs in the P60 + Sevoflurane/P6 + Sevoflurane). This finding suggests that these proteins could be intervention targets for sevoflurane-induced developmental neurotoxicity and are not associated with age. The therapeutic potential of these proteins could be exploited using selective inhibitors to prevent damage associated with multiple sevoflurane inhalations at a young age (Table 2); (3) Multiple sevoflurane inhalation induced statistically significant differences in protein expression in the neonatal group (DEPs in the P6 + Sevoflurane/P6 + Control), whereas age could also induce these proteins to produce significant differential expression in the sevoflurane group (DEPs in the P60 + Sevoflurane/P6 + Sevoflurane group). This finding revealed that targets of sevoflurane-induced neurotoxicity in neonatal mice matched those of age-dependent DEPs exposed to several sevoflurane doses (Table 3). Based on the above criteria, 443 proteins were filtered out to evaluate the potential mechanisms of sevoflurane-induced developmental neurotoxicity.
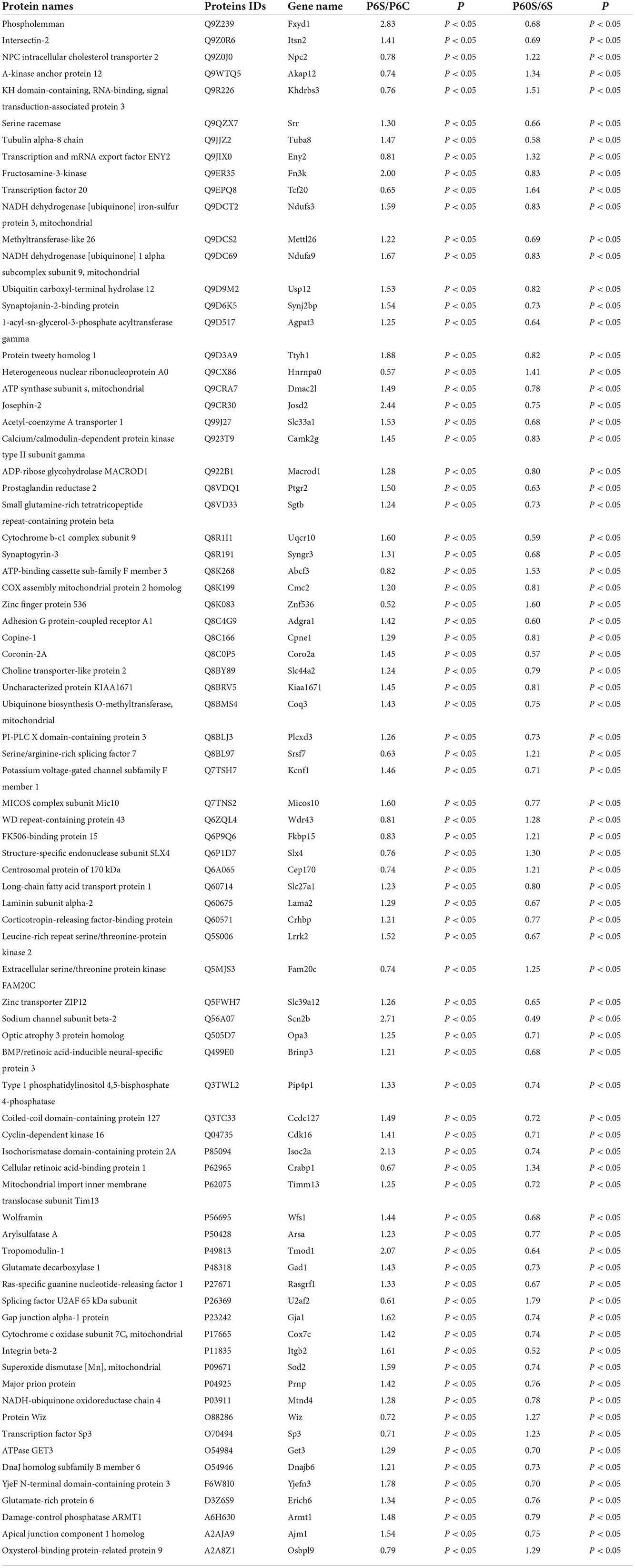
Table 1. List of the several sevoflurane-related significantly differential proteins in condition 1.

Table 2. List of the several sevoflurane-related significantly differential proteins in condition 2.

Table 3. List of the several sevoflurane-related significantly differential proteins in condition 3.
3.4. Gene ontology and Kyoto Encyclopedia of Genes and Genomes (KEGG) functional enrichment analysis of sevoflurane-related proteins
We used the DAVID database (https://david.ncifcrf.gov/home.jsp) for bioinformatics research, including GO and KEGG, to further determine the role of DEPs. The GO term is a collection of three primary ontologies: biological process (BP), molecular function (MF), and cellular component (CC), and GO functions of all DEPs have been annotated. We also performed a KEGG pathway analysis to identify the most important biochemical functions of identified DEPs.
For BP terms, the first three terms were lipid metabolic process (7.2%), translation (4.3%), and response to oxidative (2.3%), according to the percentage. The top 11 significantly enriched BP terms were translation, lipid metabolic process, cytoplasmic translation, regulation of neuron projection development, regulation of Golgi organization, response to oxidative stress, regulation of protein kinase A signaling, positive regulation of protein kinase activity, mitochondrial translation, modulation of synaptic transmission, and aerobic respiration (Figure 4A).
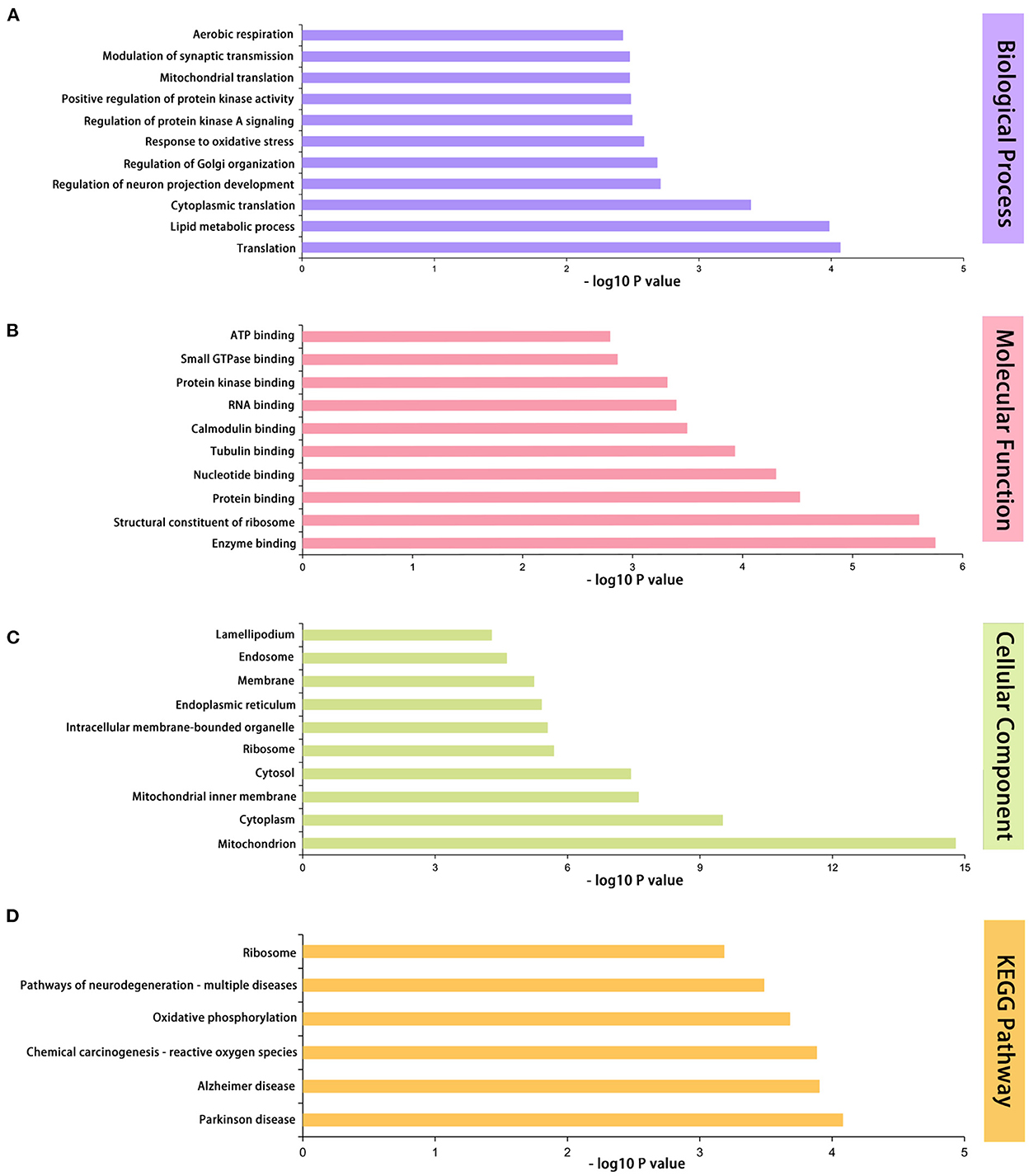
Figure 4. GO and KEGG functional enrichment analyses of 433 differentially expressed proteins. (A) The first 11 enriched GO terms of biological process. (B) The first 10 enriched GO terms of molecular functions. (C) The first 10 enriched GO terms of cellular components. (D) Differentially expressed protein pathway enriched by KEGG pathway analysis. n = 5 mice/group. GO, Gene Ontology; KEGG, Kyoto Encyclopedia of Genes and Genomes.
For MF terms, protein binding (34.3%), nucleotide binding (13.9%), and RNA binding (8.3%) were ranked first. The top 10 significantly enriched MF terms were enzyme binding, structural constituent of ribosome, protein binding, nucleotide binding, tubulin binding, calmodulin binding, RNA binding, protein kinase binding, small GTPase binding, and ATP binding (Figure 4B).
For CC terms, we discovered that cytoplasm (47.6%), membrane (40.6%), and cytosol (29.7%) were the three most significant ratios. The top 10 CC terms that were significantly enriched were as follows: mitochondrion, cytoplasm, mitochondrial inner membrane, cytosol, ribosome, intracellular membrane-bounded organelle, endoplasmic reticulum, membrane, endosome, and lamellipodium (Figure 4C).
The top six enriched pathways with significant differences were as follows: Parkinson's disease, Alzheimer's disease, chemical carcinogenesis, reactive oxygen species (ROS), oxidative phosphorylation, pathways of neurodegeneration, multiple diseases, and ribosomes (Figure 4D).
3.5. Validation of CHGB, PTEN, MAP2c, and SOD2
We performed western blotting to validate the findings of the quantitative proteomics analysis (Figure 4). CHGB, PTEN, MAP2c, and SOD2 were selected based on their biological function (Supplementary Tables 1–4) and antibody availability. Compared with neonatal mice treated with oxygen, newborn mice subjected to multiple exposures of sevoflurane anesthesia exhibited elevated expression levels of CHGB, PTEN, and MAP2c protein in the cortex, whereas that of SOD2 was notably reduced (*P < 0.05, vs. P6 + control group). No statistically significant difference was detected between the adult groups (Figure 5).
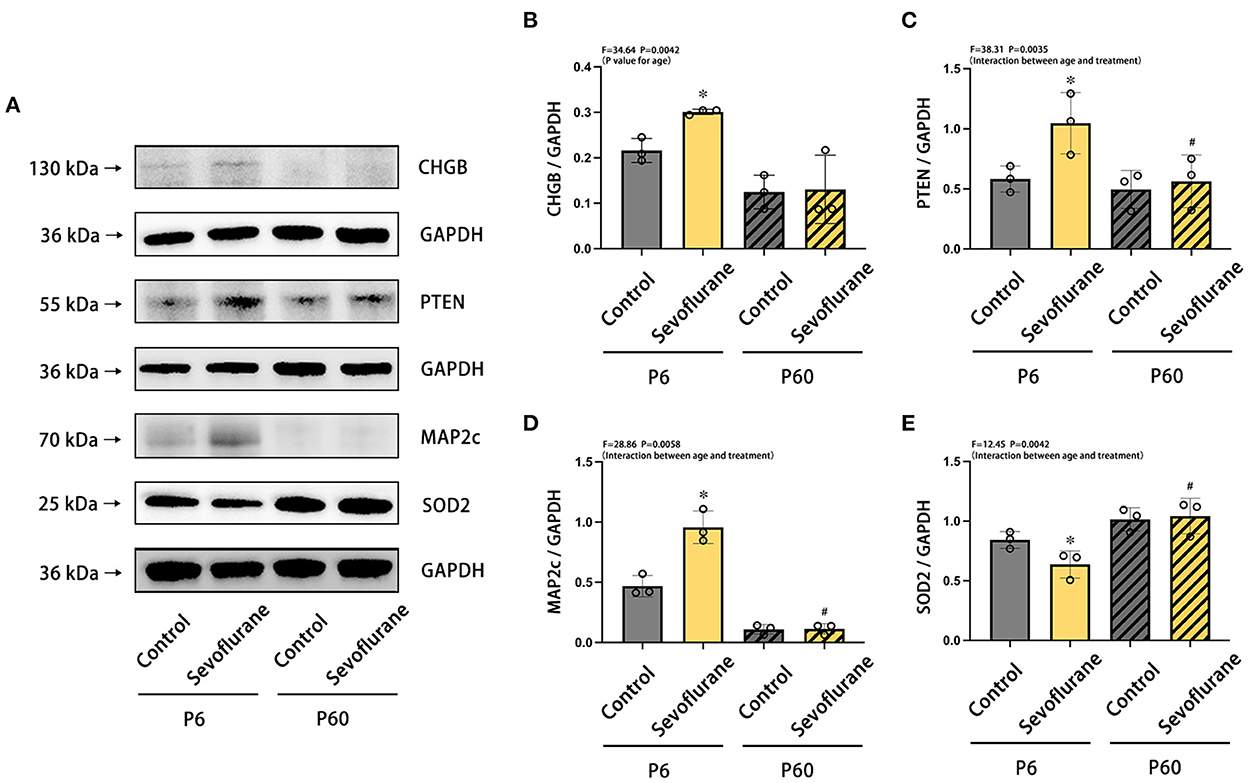
Figure 5. Differences in the expression of CHGB, PTEN, MAP2c, and SOD2 proteins in the cerebral cortex of neonatal and adult mice after multiple exposures to sevoflurane anesthesia. (A) Differences in the relative expression levels of (B) CHGB, (C) PTEN, (D) MAP2c, and (E) SOD2 in mice cerebral cortex at various ages and treatments. n = 5 mice/group. Results are expressed as mean ± standard deviation (SD). *P < 0.05 vs. P6 + control group, #P < 0.05 vs. P6 + Sevoflurane group. P6, postnatal day 6.
4. Discussion
Based on previous research (7–11), we discovered that newborn mice exhibit neurotoxicity after multiple exposures to sevoflurane anesthesia. In the current study, we employed quantitative proteomic analysis using TMTpro(16-plek) tagging and LC-MS/MS to identify 443 DEPs. Moreover, we confirmed that these DEPs are related to unique mechanisms induced following multiple sevoflurane exposure-induced neurotoxicity during development. It is important to emphasize that we selected TMTpro as a label owing to the benefit of concurrent measurement across numerous samples, which markedly minimized batch effects (15).
The DAVID database was used to annotate and evaluate the functions and pathways of the DEPs. Herein, sevoflurane could induce neurotoxicity by influencing major mechanisms of mitochondrial energy metabolism (16), tau phosphorylation (8), and neuroinflammation (17). Our results corroborate those of previous reports, as determined using the GO/KEGG analysis. KEGG pathway analysis revealed that pathways of neurodegenerative diseases, including Parkinson's disease and Alzheimer's disease, were significantly enriched. Moreover, ROS- and oxidative phosphorylation-related pathways play a pivotal role in developmental neurotoxicity induced by multiple exposures to sevoflurane anesthesia. ROS is a toxic byproduct of aerobic metabolism and an indicator of oxidative stress-induced cellular damage. Typically, ROS are produced by mitochondria within the cell, and mitochondrial dysfunction elevates ROS levels to enhance inflammatory processes. Electron transport in the respiratory chain mediates oxidative phosphorylation. Sevoflurane inhibits the mitochondrial complex of the electron transport chain (18), facilitating the generation of oversized ROS. Using the GO analysis, we also identified mitochondrion-related enriched terms. These results indicate that the disrupted mitochondrial energy metabolism plays a significant role in sevoflurane-induced developmental neurotoxicity.
Considering the availability of antibodies and the biological functions of the protein (Supplementary Table 1), we selected four DEPs for verification: CHGB, PTEN, MAP2c, and SOD2. Herein, we noted that expression levels of CHGB, PTEN, and MAP2c increased, whereas those of SOD2 decreased. The results of the western blotting analysis were consistent with the trends predicted by proteome analysis, which demonstrated the reliability of TMT-based quantitative proteomics analysis.
CHGB is known to be associated with neurodegenerative diseases, including schizophrenia and Parkinson's disease (19, 20). As a type of neuroendocrine secretory granule protein, CHGB plays a key role in the regulated secretory pathway, impacting the secretion of trophic factors that modulate synaptic maturation of developing neurons (21), and is involved in the regulation of synaptic plasticity, which is related to memory formation (22). Combined with our findings exhibited in the BP of enriched GO terms (Figure 4A), multiple exposures to sevoflurane anesthesia could induce dysfunctional synaptic transmission in neonatal mice by increasing CHGB expression. PTEN protein is known to play a potential role in regulating the structure and plasticity of neurons, which hinders the Akt/mTOR signaling pathway to decrease the growth and proliferation of neurons and the activation of autophagy. In neonatal mice exposed to sevoflurane anesthesia, elevated PTEN expression may indicate neuronal loss in key brain regions that mediate cognitive function during neurodevelopment, and excessive activation of autophagy leads to autophagic programmed cell death (23, 24). The observed alterations in PTEN expression are consistent with several previous studies (25, 26). MAP2 exhibits microtubule stabilization activities that involve neurogenesis, morphogenesis, and migration for the development of axons and dendrites. MAP2 has three phenotypes: MAP2c (70 kDa), MAP2a, and MAP2b (both 280 kDa). MAP2a and MAP2b are expressed in the brains of adult mice but not newborn pups, and neonatal mice exhibit low MAP2b expression and abundant levels of MAP2c. MAP2c continues to decrease in abundance until adulthood (27). We selected MAP2c to verify quantitative proteomics owing to its specific expression during the newborn stage. Tau is expressed abundantly in neonatal mice when compared with that in adult mice, and some key phosphorylated-tau sites that detach from microtubules are increased in newborn mice exposed to sevoflurane anesthesia (8, 28). MAP2c and tau compete for binding sites, which is mediated by several factors (29). Therefore, under sevoflurane anesthesia, increased MAP2c brain expression may be influenced by elevated tau in neonatal mice, which could be a compensatory mechanism to maintain microtubule stability. SOD2, an enzyme belonging to the iron/manganese superoxide dismutase family and involved in the mitochondrial catabolic pathway, converts the superoxide anion to hydrogen peroxide, and the former is a potentially damaging product to the brain. The loss of SOD2 plays a critical role in the progression of neurodegenerative diseases (30, 31). Herein (32), the protein expression of SOD2 was attenuated in the hippocampus of neonatal mice exposed to 3% sevoflurane for 4 h. In addition, mitochondrion-related mechanisms are highly associated with sevoflurane-induced developmental neurotoxicity. Consequently, reduced SOD2 protein expression in newborn pups with multiple exposures to sevoflurane may result in elevated superoxide anion oxidation and oxidative stress damage in the brain.
In summary, we examined the mechanism underlying sevoflurane-induced neurotoxicity in newborn mice using TMT labeling and LS-MS/MS. Bioinformatic analysis was applied to identify DEPs, and we underlined the possibility of DNA damage through the mRNA surveillance pathway as a mechanism of sevoflurane-induced developmental neurotoxicity. Multiple sevoflurane exposures can cause brain damage and cognitive deficits in newborn mice, mediated via elevated levels of CHGB, PTEN, and MAP2c protein expression and reduced SOD2 expression. Our findings shed light on the mechanisms underlying the neurotoxicity induced by multiple exposures to sevoflurane anesthesia during development.
Data availability statement
The original contributions presented in the study are publicly available. This data can be found at: ProteomeXchange, http://www.proteomexchange.org/, PXD037294.
Ethics statement
All studies were approved by the Animal Experimental Ethics Committee at Tianjin Medical University General Hospital in Tianjin, China (Approval No. IRB2021-DWFL-210).
Author contributions
JF, HL, and YZ performing all experiments, analyzing the data, and writing the original draft. YYa and XZ prepared the figures. YaYu conceived the study and reviewed the manuscript. YoYu supervised the study. All authors have read and approved the final version of the manuscript.
Funding
This study was supported by the National Natural Science Foundation of China (Grant Numbers 82001149 and 82072150), Tianjin Natural Science Foundation (Grant Number 20JCQNJC01050), Tianjin Education Commission for Higher Education Science and Technology Development Fund (Grant Number 2019KJ201), and Tianjin Research Innovation Project for Postgraduate Students (Grant Number 2021YJSS158).
Acknowledgments
We would like to give our sincere gratitude to the editor and reviewers for their constructive comments.
Conflict of interest
The authors declare that the research was conducted in the absence of any commercial or financial relationships that could be construed as a potential conflict of interest.
Publisher's note
All claims expressed in this article are solely those of the authors and do not necessarily represent those of their affiliated organizations, or those of the publisher, the editors and the reviewers. Any product that may be evaluated in this article, or claim that may be made by its manufacturer, is not guaranteed or endorsed by the publisher.
Supplementary material
The Supplementary Material for this article can be found online at: https://www.frontiersin.org/articles/10.3389/fneur.2022.1056947/full#supplementary-material
Footnotes
References
1. Albayram O, Herbert MK, Kondo A, Tsai CY, Baxley S, Lian X, et al. Function and regulation of tau conformations in the development and treatment of traumatic brain injury and neurodegeneration. Cell Biosci. (2016) 6:59. doi: 10.1186/s13578-016-0124-4
2. Sun M, Xie Z, Zhang J, Leng Y. Mechanistic insight into sevoflurane-associated developmental neurotoxicity. Cell Biol Toxicol. (2021) 21:9677. doi: 10.1007/s10565-021-09677-y
3. DiMaggio C, Sun LS, Kakavouli A, Byrne MW, Li G. A retrospective cohort study of the association of anesthesia and hernia repair surgery with behavioral and developmental disorders in young children. J Neurosurg Anesthesiol. (2009) 21:286–91. doi: 10.1097/ANA.0b013e3181a71f11
4. Flick RP, Katusic SK, Colligan RC, Wilder RT, Voigt RG, Olson MD, et al. Cognitive and behavioral outcomes after early exposure to anesthesia and surgery. Pediatrics. (2011) 128:e1053–61. doi: 10.1542/peds.2011-0351
5. Wilder RT, Flick RP, Sprung J, Katusic SK, Barbaresi WJ, Mickelson C, et al. Early exposure to anesthesia and learning disabilities in a population-based birth cohort. Anesthesiology. (2009) 110:796–804. doi: 10.1097/01.anes.0000344728.34332.5d
6. McCann ME, de Graaff JC, Dorris L, Disma N, Withington D, Bell G, et al. Neurodevelopmental outcome at 5 years of age after general anaesthesia or awake-regional anaesthesia in infancy (GAS): an international, multicentre, randomised, controlled equivalence trial. Lancet. (2019) 393:664–77. doi: 10.1016/S0140-6736(18)32485-1
7. Yu Y, Yang M, Zhuang X, Pan J, Zhao Y, Yu Y. Effects of toxic apolipoprotein E fragments on Tau phosphorylation and cognitive impairment in neonatal mice under sevoflurane anesthesia. Brain Behav. (2022) 12:e2702. doi: 10.1002/brb3.2702
8. Yu Y, Yang Y, Tan H, Boukhali M, Khatri A, Yu Y, et al. Tau contributes to sevoflurane-induced neurocognitive impairment in neonatal mice. Anesthesiology. (2020) 133:595–610. doi: 10.1097/ALN.0000000000003452
9. Yang M, Tan H, Zhang K, Lian N, Yu Y, Yu Y. Protective effects of Coenzyme Q10 against sevoflurane-induced cognitive impairment through regulating apolipoprotein E and phosphorylated Tau expression in young mice. Int J Dev Neurosci. (2020) 2020:jdn.10041. doi: 10.1002/jdn.10041
10. Li Y, Zhang L, Wang C, Tang X, Chen Y, Wang X, et al. Sevoflurane-induced learning deficits and spine loss via nectin-1/corticotrophin-releasing hormone receptor type 1 signaling. Brain Res. (2019) 1710:188–98. doi: 10.1016/j.brainres.2018.12.010
11. Yang Y, Liang F, Gao J, Dong Y, Zhang Y, Yang G, et al. Testosterone attenuates sevoflurane-induced tau phosphorylation and cognitive impairment in neonatal male mice. Br J Anaesth. (2021) 127:929–41. doi: 10.1016/j.bja.2021.08.028
12. Tang W, Dong M, Teng F, Cui J, Zhu X, Wang W, et al. TMT-based quantitative proteomics reveals suppression of SLC3A2 and ATP1A3 expression contributes to the inhibitory role of acupuncture on airway inflammation in an OVA-induced mouse asthma model. Biomed Pharmacother. (2021) 134:111001. doi: 10.1016/j.biopha.2020.111001
13. Lian N, Shen M, Zhang K, Pan J, Jiang Y, Yu Y, et al. Drinking hydrogen-rich water alleviates chemotherapy-induced neuropathic pain through the regulation of gut microbiota. J Pain Res. (2021) 14:681–91. doi: 10.2147/JPR.S288289
14. Park TJ, Park JH, Lee GS, Lee JY, Shin JH, Kim MW, et al. Quantitative proteomic analyses reveal that GPX4 downregulation during myocardial infarction contributes to ferroptosis in cardiomyocytes. Cell Death Dis. (2019) 10:835. doi: 10.1038/s41419-019-2061-8
15. Li J, Van Vranken JG, Pontano Vaites L, Schweppe DK, Huttlin EL, Etienne C, et al. TMTpro reagents: a set of isobaric labeling mass tags enables simultaneous proteome-wide measurements across 16 samples. Nat Methods. (2020) 17:399–404. doi: 10.1038/s41592-020-0781-4
16. Li M, Guo J, Wang H, Li Y. Involvement of mitochondrial dynamics and mitophagy in sevoflurane-induced cell toxicity. Oxid Med Cell Longev. (2021) 2021:6685468. doi: 10.1155/2021/6685468
17. Huang H, Hu C, Xu L, Zhu X, Zhao L, Min J. The effects of hesperidin on neuronal apoptosis and cognitive impairment in the sevoflurane anesthetized rat are mediated through the PI3/Akt/PTEN and nuclear factor-kappaB (NF-kappaB) signaling pathways. Med Sci Monit. (2020) 26:e920522. doi: 10.12659/MSM.920522
18. Hanley PJ, Ray J, Brandt U, Daut J. Halothane, isoflurane and sevoflurane inhibit NADH:ubiquinone oxidoreductase (complex I) of cardiac mitochondria. J Physiol. (2002) 544:687–93. doi: 10.1113/jphysiol.2002.025015
19. Shin JG, Kim JH, Park CS, Kim BJ, Kim JW, Choi IG, et al. Gender-specific associations between CHGB genetic variants and schizophrenia in a Korean population. Yonsei Med J. (2017) 58:619–25. doi: 10.3349/ymj.2017.58.3.619
20. Wen G, Pang H, Wu X, Jiang E, Zhang X, Zhan X. Proteomic characterization of secretory granules in dopaminergic neurons indicates chromogranin/secretogranin-mediated protein processing impairment in Parkinson's disease. Aging. (2021) 13:20335–58. doi: 10.18632/aging.203415
21. Dominguez N, van Weering JRT, Borges R, Toonen RFG, Verhage M. Dense-core vesicle biogenesis and exocytosis in neurons lacking chromogranins A and B. J Neurochem. (2018) 144:241–54. doi: 10.1111/jnc.14263
22. Tiwari NK, Sathyanesan M, Kumar V, Newton SS. A comparative analysis of erythropoietin and carbamoylated erythropoietin proteome profiles. Life. (2021) 11:359. doi: 10.3390/life11040359
23. Chen CY, Chen J, He L, Stiles BL. PTEN: tumor suppressor and metabolic regulator. Front Endocrinol. (2018) 9:338. doi: 10.3389/fendo.2018.00338
24. Xue H, Xu Y, Wang S, Wu ZY, Li XY, Zhang YH, et al. Sevoflurane post-conditioning alleviates neonatal rat hypoxic-ischemic cerebral injury via Ezh2-regulated autophagy. Drug Des Devel Ther. (2019) 13:1691–706. doi: 10.2147/DDDT.S197325
25. Liu T, Dong X, Wang B, Zhang S, Bai J, Ma W, et al. Silencing of PTEN inhibits the oxidative stress damage and hippocampal cell apoptosis induced by Sevoflurane through activating MEK1/ERK signaling pathway in infant rats. Cell Cycle. (2020) 19:684–96. doi: 10.1080/15384101.2020.1717041
26. Li X, Wu Z, Zhang Y, Xu Y, Han G, Zhao P. Activation of autophagy contributes to sevoflurane-induced neurotoxicity in fetal rats. Front Mol Neurosci. (2017) 10:432. doi: 10.3389/fnmol.2017.00432
27. Garner CC, Matus A. Different forms of microtubule-associated protein 2 are encoded by separate mRNA transcripts. J Cell Biol. (1988) 106:779–83. doi: 10.1083/jcb.106.3.779
28. Dong Y, Liang F, Huang L, Fang F, Yang G, Tanzi RE, et al. The anesthetic sevoflurane induces tau trafficking from neurons to microglia. Commun Biol. (2021) 4:560. doi: 10.1038/s42003-021-02047-8
29. Sontag JM, Nunbhakdi-Craig V, White CL 3rd, Halpain S, Sontag E. The protein phosphatase PP2A/Balpha binds to the microtubule-associated proteins Tau and MAP2 at a motif also recognized by the kinase Fyn: implications for tauopathies. J Biol Chem. (2012) 287:14984–93. doi: 10.1074/jbc.M111.338681
30. Fracassi A, Marcatti M, Zolochevska O, Tabor N, Woltjer R, Moreno S, et al. Oxidative damage and antioxidant response in frontal cortex of demented and nondemented individuals with Alzheimer's neuropathology. J Neurosci. (2021) 41:538–54. doi: 10.1523/JNEUROSCI.0295-20.2020
31. Lin C-H, Wei P-C, Chen C-M, Huang Y-T, Lin J-L, Lo Y-S, et al. Lactulose and melibiose attenuate MPTP-induced Parkinson's disease in mice by inhibition of oxidative stress, reduction of neuroinflammation and up-regulation of autophagy. Front Aging Neurosci. (2020) 12:226. doi: 10.3389/fnagi.2020.00226
Keywords: sevoflurane, developing brain, neurotoxicity, TMT-based quantitative proteomic analysis, anesthetic toxicity
Citation: Feng J, Lin H, Zhao Y, Yang Y, Zhuang X, Yu Y and Yu Y (2022) Tandem mass tag-based quantitative proteomic analysis of effects of multiple sevoflurane exposures on the cerebral cortex of neonatal and adult mice. Front. Neurol. 13:1056947. doi: 10.3389/fneur.2022.1056947
Received: 29 September 2022; Accepted: 28 November 2022;
Published: 13 December 2022.
Edited by:
Hong Ni, Children's Hospital of Soochow University, ChinaReviewed by:
Jia Yan, Shanghai Jiao Tong University, ChinaXueyuan Hu, Qingdao Agricultural University, China
Copyright © 2022 Feng, Lin, Zhao, Yang, Zhuang, Yu and Yu. This is an open-access article distributed under the terms of the Creative Commons Attribution License (CC BY). The use, distribution or reproduction in other forums is permitted, provided the original author(s) and the copyright owner(s) are credited and that the original publication in this journal is cited, in accordance with accepted academic practice. No use, distribution or reproduction is permitted which does not comply with these terms.
*Correspondence: Yang Yu, c2V2ZW55dTE5ODdAb3V0bG9vay5jb20=; Yonghao Yu, eXV5b25naGFvQDEyNi5jb20=
†These authors have contributed equally to this work