- 1Department of Physiology and Galway Neuroscience Centre, School of Medicine, National University of Ireland, Galway, Ireland
- 2CÚRAM–SFI Research Centre for Medical Devices, National University of Ireland Galway, Galway, Ireland
- 3School of Mathematical and Statistical Sciences, National University of Ireland Galway, Galway, Ireland
- 4Cerenovus, Galway, Ireland
- 5Metropolitan Hospital, Stroke Unit, Piraeus, Greece
- 6Second Department of Neurology, National and Kapodistrian University of Athens, “Attikon” University Hospital, Athens, Greece
- 7Department of Neurointerventions, National Institute of Clinical Neurosciences, Budapest, Hungary
- 8Department of Neurology, Sahlgrenska University Hospital, Gothenburg, Sweden
- 9Department of Clinical Neuroscience, Institute of Neuroscience and Physiology, Sahlgrenska Academy at University of Gothenburg, Gothenburg, Sweden
- 10Department of Interventional and Diagnostic Neuroradiology, Sahlgrenska University Hospital, University of Gothenburg, Gothenburg, Sweden
- 11Department of Radiology, Royal College of Surgeons in Ireland, Beaumont Hospital, Dublin, Ireland
Background and Aims: Besides the crucial role in the treatment of acute ischemic stroke (AIS), mechanical thrombectomy represents a unique opportunity for researchers to study the retrieved clots, with the possibility of unveiling biological patterns linked to stroke pathophysiology and etiology. We aimed to develop a shotgun proteomic approach to study and compare the proteome of formalin-fixed paraffin-embedded (FFPE) cardioembolic and large artery atherosclerotic (LAA) clots.
Methods: We used 16 cardioembolic and 15 LAA FFPE thrombi from 31 AIS patients. The thrombus proteome was analyzed by label-free quantitative liquid chromatography-tandem mass spectrometry (LC-MS/MS). MaxQuant v1.5.2.8 and Perseus v.1.6.15.0 were used for bioinformatics analysis. Protein classes were identified using the PANTHER database and the STRING database was used to predict protein interactions.
Results: We identified 1,581 protein groups as part of the AIS thrombus proteome. Fourteen significantly differentially abundant proteins across the two etiologies were identified. Four proteins involved in the ubiquitin-proteasome pathway, blood coagulation or plasminogen activating cascade were identified as significantly abundant in LAA clots. Ten proteins involved in the ubiquitin proteasome-pathway, cytoskeletal remodeling of platelets, platelet adhesion or blood coagulation were identified as significantly abundant in cardioembolic clots.
Conclusion: Our results outlined a set of 14 proteins for a proof-of-principle characterization of cardioembolic and LAA FFPE clots, advancing the proteome profile of AIS human thrombi and understanding the pathophysiology of ischemic stroke.
Introduction
Stroke is a leading cause of mortality and disability worldwide, with 11.9 million stroke accidents recorded in 2017, 6.2 million of which were fatal (1). The majority of strokes (87%) are ischemic events, caused by blockage of a cerebral blood vessel by a blood clot (2). Occlusion of the cerebrovasculature deprives brain tissue of blood supply, resulting in death of brain cells and development of an area of ischemic infarct. Ischemic stroke can be treated in the acute clinical setting by an endovascular intervention called mechanical thrombectomy, in which the clot is retrieved through the vasculature using an aspiration and/or a stentriever device (3). The so-called “omics techniques” can be extremely useful to advance our understanding of the pathophysiology of ischemic stroke through the study of the thrombotic material extracted by mechanical thrombectomy. Fresh/frozen (FF) specimens are the most used for genomics, proteomics, and metabolomics analysis, but there are practical drawbacks to this approach in a busy clinical setting, reducing availability of FF samples for research. Furthermore, FF samples are expensive to store (4) and to transport to other laboratories for analysis. Formalin-fixation followed by paraffin-embedding (FFPE) is a standard procedure for tissue samples, which allows storage of clinical material at ambient temperature over a long period. Application of mass spectrometry techniques to analyse FFPE tissues is more difficult (5) because the fixation process results in cross-links between proteins and other biomolecules present in the tissue, owing to reactivity of formaldehyde with side chains of amino acids (e.g., lysine, arginine, tyrosine, asparagine, glutamine) (6). These cross-links lead to decreased protein immunoreactivity and extraction from the tissue. Another limitation is the difficulty of paraffin removal (which is water insoluble) without damage or loss of targeted compounds. For these reasons, extraction of biomolecules from FFPE material for analyses using mass spectrometry techniques for proteomics and metabolomics remains a challenging task. Nevertheless, there is great interest in optimizing the use of FFPE tissues in retrospective proteomic studies because they are more practical and versatile compared to FF tissues. Several studies have described different methods of protein extraction from archival clinical samples (7–10).
Using an in-house optimized protein extraction protocol, our study used a shotgun proteomics approach to study the FFPE thrombotic material retrieved from 31 acute ischemic stroke (AIS) patients. To advance understanding of the pathophysiology of AIS, we investigated the proteome of 16 clots of cardioembolic (CE) etiology and 15 clots of large artery atherosclerosis (LAA) etiology.
Materials and Methods
AIS Blood Clot Samples
Blood clots were collected from 31 AIS patients as a part of the CÚRAM RESTORE Registry. Samples were collected from four hospitals: Beaumont Hospital, Dublin; Sahlgrenska Hospital, Gothenburg; National Institute of Clinical Neurosciences, Budapest; Metropolitan Hospital, Piraeus. Samples were analyzed by a label-free quantitative liquid chromatography-tandem mass spectrometry (LC-MS/MS) proteomics approach. Prior to this study, approval of the regional hospital ethics committees and National University of Ireland Galway (NUIG) research ethics committees (16-SEPT-08) was obtained in accordance with the ethical standards of the Declaration of Helsinki and its amendments (11). All patients included in the study underwent mechanical thrombectomy between February 2018 and November 2019 for acute occlusion of a large intracranial artery in the anterior or posterior cerebral circulation. The inclusion criteria were: patients >18 years, having undergone mechanical thrombectomy treatment for AIS and having thrombus material available for analysis. Pertinent clinical data were collected from each patient in the form of an anonymized data abstraction form where the etiology of the patient was specified, according to Trial of Org 10172 in Acute Stroke Treatment (TOAST) criteria (12). The TOAST classification system is a widely used subtyping system to classify stroke according to etiology (12). Large artery atherosclerosis and cardioembolism are the two main determined large vessel occlusion stroke subtypes (12). Identifying etiological biomarkers could help in the development of new therapeutics and stroke prevention strategies. Stroke experts performed TOAST classification after complete work-up of the patients in the recruiting hospitals. Thrombi extracted after thrombectomy were collected at the hospital site, stored in pots containing 10% neutral buffered formalin and shipped to the laboratory at NUI Galway for paraffin-embedding and subsequent analysis.
Sample Preparation Protocol
The sample preparation workflow is schematically represented in Figure 1. Samples were stored in formalin for not longer than 4 weeks to minimize adverse impact of prolonged formalin storage on tissue integrity (13). After formalin fixation and paraffin embedding, approximately 60 μm thick sections (6 sections of 10 μm thickness) were sliced from each FFPE tissue block using a microtome and placed in a 1.5 mL Eppendorf tube. Samples were embedded carefully, maximizing longitudinal distal to proximal orientation, thereby ensuring tissue sections for analysis were representative of the whole clot. Wax blocks were trimmed to expose full-face sections, following which the 6 sections of 10 μm thickness were cut for the analysis.
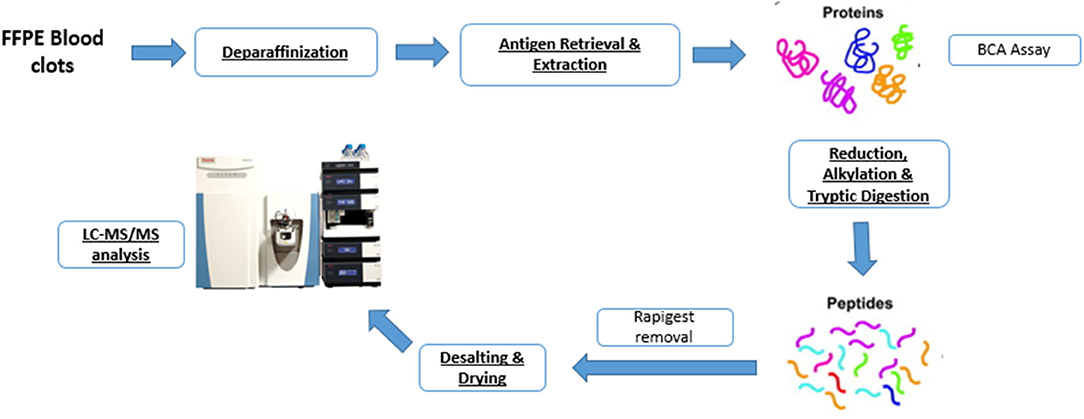
Figure 1. Shotgun proteomics workflow for characterizing the protein content of formalin-fixed paraffin-embedded (FFPE) blood clots.
Our protein extraction protocol followed the method described by Foll et al. (9) with one main adaptation in the deparaffinization/rehydration step. Before protein extraction, deparaffinization was performed by pipetting 1 mL of xylene in the Eppendorf tube containing the sample. After 20 min incubation at room temperature, the tube was then vortexed, centrifuged and the supernatant discarded. The procedure was repeated 2 more times and then, for rehydration, the tissue was incubated twice for 10 min in 100% ethanol, twice for 10 min in 95% ethanol, following 10 min incubation, respectively, with 70 and 50% ethanol. Tissues were then stored in 1 mL water (Liquid Chromatography Mass Spectrometry (LCMS) grade; Sigma-Aldrich, St. Louis, MO, USA) overnight, until further processing.
Extraction was performed by pipetting 200 μL of aqueous buffer containing 0.1% w/v RapiGest (Waters, Milford, MA, USA), 50 mM ammonium bicarbonate (Sigma-Aldrich) pH 8 and 1 mM Dithiothreitol (DTT, Sigma-Aldrich) in each tube containing the sample. The buffered samples were incubated in a thermomixer at 95°C, 750 rpm for 4 h for antigen retrieval and protein extraction. Then, samples were sonicated for 30 min using a waterbath sonicator at room temperature and centrifuged for 20 min at 4°C. The supernatant was collected and the Bicinchoninic acid assay (BCA, Sigma-Aldrich) was used to estimate protein concentration. Samples were then reduced with 3 μL of Tris(2-carboxyethyl)phosphine hydrochloride, (TCEP, Sigma-Aldrich), incubated for 1 h at 37°C and alkylated with 3 μL of 200 mM Iodoacetamide (IAA, Sigma-Aldrich), by incubation for 30 min at room temperature in the dark. Enzymatic digestion was performed by adding 5 μL of 1:1 w/v sequencing grade modified trypsin (Promega, Mybio Ltd) and samples were incubated overnight at 37°C. Trifluoroacetic acid (TFA, Sigma-Aldrich) up to a final concentration of 0.5% v/v (pH <2) was added to stop digestion and precipitate the RapiGest. Samples were then incubated for 1 h at 37°C and centrifuged at 13,000 rpm for 15 min. The supernatant was collected and 5 μg of peptides were desalted by using self-packed C18 zip-tips (Merk Millipore). A speed vacuum concentrator was used to dry the purified peptides, which were then stored at −80°C until shipment to NUI Maynooth for measurement by LC-MS/MS.
LC-MS/MS Analysis
Vacuum dried samples were dissolved in 20 μL of loading buffer (3% acetonitrile, 97% LC-MS water, and 0.05% trifluoroacetic acid) giving a final concentration of 250 ng/μL. For each run, 4 μL of sample was injected and analyzed by Orbitrap Q-Exactive (Thermo Scientific) coupled to an Easy nano LC 1000 (Thermo Scientific) with 0.3 mL/min flow rate. Peptides were separated by a Thermo RSLC C18 analytical column (2 μm 100A 75 μm 50 cm) using the mobile phases A (97% LC-MS water, 3% LC-MS acetonitrile, 0.1% LC-MS Formic acid) and B (20% LC-MS water, 80% LC-MS acetonitrile, 0.1% LC-MS formic acid). The elution gradient was: 0–5 min 3% [B]; 5–10 min 10% [B]; 10–100 min 40% [B]; 100–105 min 90% [B]; 105–112 min 90% [B]; 112–113 min 5% [B]; 113–133 min 3% [B]. The acquisition was carried out in positive ionization mode (ESI+) and MS was operated in data-dependent mode (Full MS/dd-MS2).
Bioinformatics Analysis
Protein identification and label-free quantification (LFQ) normalization of MS/MS data was performed using MaxQuant v1.5.2.8 (http://www.maxquant.org) (14). Variable modifications selected were Acetyl (Protein N-term) and Oxidation (M), while trypsin was selected as the digestion enzyme and the maximum number of allowed missed cleavage was 2. MS/MS data were correlated against the Homo sapiens reference proteome database from Uniprot using the Andromeda search algorithm incorporated in MaxQuant software, including a contaminant sequence set. Data analysis, processing and visualization were performed using Perseus v.1.6.15.0 (www.maxquant.org/) following standard steps for clinical proteomics as described in literature (15). Briefly, normalized peptide intensity values were used to quantify protein abundance for the analysis. Data were filtered to remove protein groups that are only identified by peptides that carry one or more modified amino acids, matching to the reverse database and contaminants. Then log2 transformation was performed, with subsequent grouping of samples according to etiology. Further filtering based on 70% valid values was carried out and a multisample t-test was performed. Statistical testing was done at the 2-tailed α level of 0.05 (p < 0.05) to identify significantly differentially abundant proteins across the two etiologies. We also used the commonly used permutation based false discovery rate test to correct p-values for multiple hypothesis testing. Protein classes were identified using publicly available software programs such as the PANTHER database of protein families (http://pantherdb.org/http://pantherdb.org/) (16) and the STRING database (http://string-db.org) (17) of known and predicted protein interactions that include direct physical and indirect functional protein associations.
Results
General Proteomic Profiling of AIS Clots
The general proteome profiling of the analyzed clots was examined to identify differences in protein presence or abundance according to etiology. We identified 1,581 protein groups as part of AIS thrombus proteome (Supplementary Table 1), after removing proteins identified by site, matching to the reverse database and contaminants. After further filtering for at least 70% of valid values (i.e., removing samples and protein groups with <70% valid values), 4 samples were excluded. A total of 179 protein groups (Supplementary Table 2) in the remaining 27 samples (14 LAA and 13 CE) were further analyzed. In 15 cases (8 LAA and 7 CE) recombinant tissue plasminogen activator (rtPA) was administered before mechanical thrombectomy, while in 12 cases (6 LAA and 6 CE) mechanical thrombectomy alone was performed (Supplementary Figure 2). Principal Component Analysis (PCA) was performed based on the protein abundance values from LC-MS/MS to obtain Principal Components (PCs) that explained 48.7% (27.2% PC1 and 21.5% PC2) of data variation among the 27 samples belonging to the two different etiologies (Figure 2A). In Supplementary Figure 1 the PCA has additional labeling identifying the samples by hospital site. No evidence of clustering by collection center is observed.
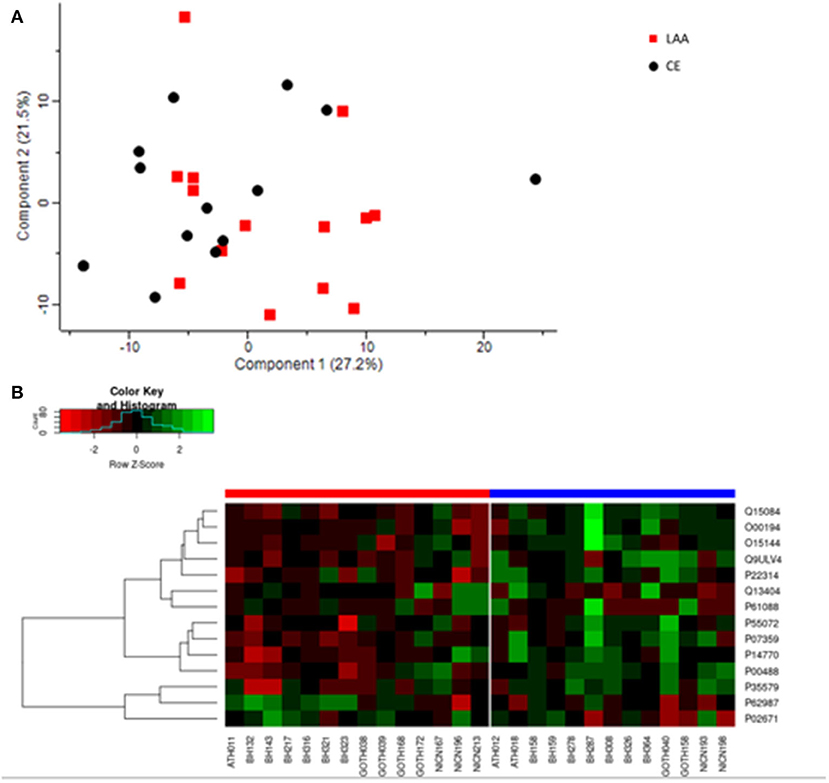
Figure 2. (A) Principal component analysis of the general proteome of FFPE AIS clots. LAA clots are indicated with red squares and Cardioembolic (CE) clots are represented by black circles. Each shape indicates individual samples. Component 1 and 2 explain the 27.2 and 21.5% of the sample variation, respectively. (B) Heatmap depicting differential expression of the significantly abundant protein groups in LAA (red bar on the left) and CE (blue bar on the right) clots. Protein groups are on the right and indicated with their UniProt ID. In the heatmap, red indicates a decrease in protein abundance, while green indicates an increase in protein abundance.
Comparative Profiling by Etiology
Adjusted p-values did not indicate any significant difference in abundance between CE and LAA clots. However, considering the non-adjusted p-values, the comparative proteomic profiling of LAA vs. CE clots resulted in the identification of 14 significantly differentially abundant protein groups between the two different etiologies, four of which were identified as significantly different abundant for LAA stroke etiology (Table 1) and the other ten were identified as significantly different abundant for CE stroke etiology (Table 1). The heatmap in Figure 2B shows the differential expression of the 14 statistically significantly differentially abundant proteins across the 27 samples.
Biological Functions and Interaction Patterns of the Significantly Different Abundant Proteins for LAA Stroke Etiology
Figure 3A shows biological pathways and Figure 3B shows interaction patterns associated with the four significantly different abundant proteins for LAA stroke etiology. The protein groups are involved in four biological pathways: blood coagulation, plasminogen activating cascade, Toll receptor signaling pathway and ubiquitin-proteasome pathway (Figure 3A). Ubiquitin-60S ribosomal protein L40 is part of Ubiquitin-proteasome system (UPS), encoded by the gene UBA52 and its levels increase in response to oxidative stress (18). Its role is closely connected to the proteins Ubiquitin-conjugating enzyme E2, also called Ubc13, and Ubiquitin-conjugating enzyme E2 variant 1 (respectively encoded by genes UBE2N and UBE2V1), which are also significantly more abundant in LAA clots and involved in Toll-like receptor signaling pathway (19) as shown in Figure 3B. The fourth identified significantly different abundant protein, fibrinogen alpha chain (encoded by gene FGA), does not directly interact with the other three protein groups (Figure 3B). The fibrinogen alpha chain is one of the three chains forming the soluble protein fibrinogen and it is involved in both the blood coagulation and plasminogen activation cascade pathways (20).
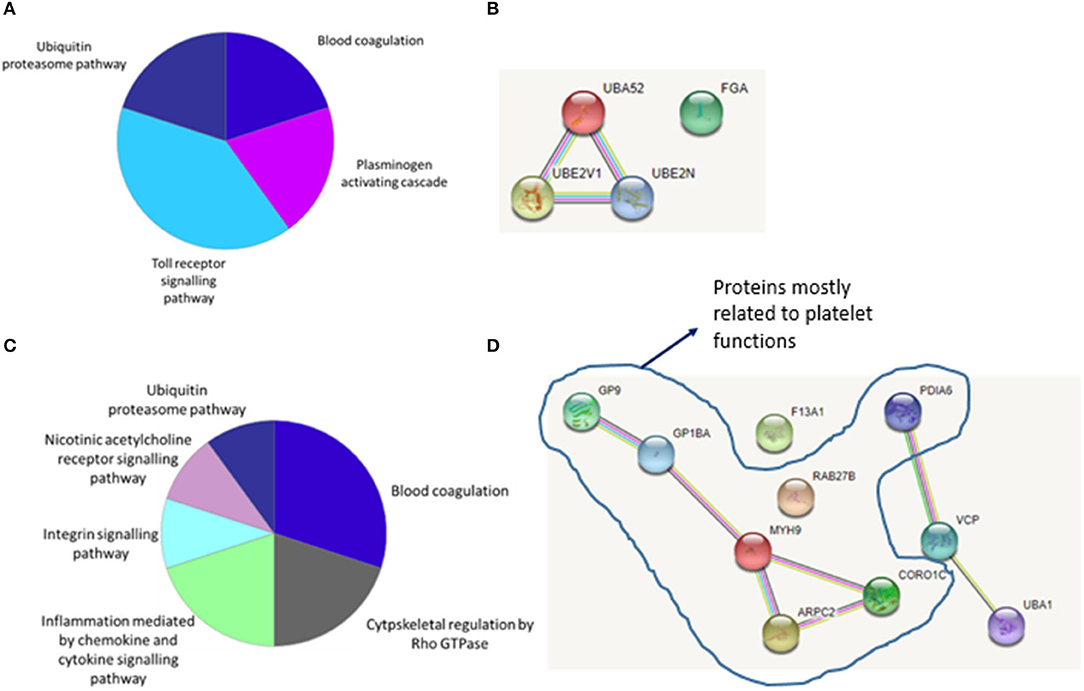
Figure 3. (A) Biological pathways and interaction patterns associated with LAA specific proteins. Figure depicts a summary of the biological pathways associated with the specific protein groups identified as having an increased abundance in LAA samples. (B) Bioinformatic analysis of the statistically significant proteins using the STRING database identified the interaction patterns with known and predicted protein associations including physical and indirect functional protein linkages. (C) Biological pathways and interaction patterns associated with CE specific proteins. Figure depicts a summary of the biological pathways associated with the specific protein groups identified as having an increased abundance in CE samples. (D) Bioinformatic analysis of the statistically significant proteins using the STRING database identified the interaction patterns with known and predicted protein associations including physical and indirect functional protein linkages.
Biological Functions and Interaction Patterns of Significantly Different Abundant Proteins for CE Stroke Etiology
Figure 3C shows identified biological pathways and Figure 3D shows interaction patterns for the ten protein groups with significantly different abundant proteins for CE stroke etiology. An increase in proteins related to six biological pathways was observed: blood coagulation, cytoskeletal regulation by Rho GTPase, cytokine and chemokine mediated inflammation, integrin signaling, nicotinic acetylcholine receptor signaling and the ubiquitin-proteasome pathway (Figure 3C). Figure 3D shows the interaction patterns of the significantly different abundant proteins for cardioembolic stroke etiology, with two main clusters, respectively, of five and three protein groups closely interacting and two protein groups not showing any interaction.
One of the main clusters observed consisted of the protein groups coded by the genes GP9, GP1BA, MYH9, ARPC2, and CORO1C. MYH9, ARPC2, and CORO1C are involved in cytoskeletal remodeling of platelets (21–23). GP1BA and GP9 code for proteins that are part of a complex that works as the von Willebrand factor (vWF) receptor and mediates vWF-dependent platelet adhesion to blood vessels, which is a critical initiating event in haemostasis (24).
A second cluster is formed by the protein groups encoded by genes PDIA6, VCP and UBA1, which are associated with the endoplasmic reticulum (ER) and play a role in the ER stress response (25, 26). Finally, although ras-related protein Rab-27B (encoded by gene RAB-27B) and coagulation factor XIII A chain (encoded by gene F13A1) do not show an interaction pattern with the rest of the protein groups, their functions are related to blood coagulation. Ras-related protein Rab-27B is involved in platelet secretion (27) and coagulation factor XIII A chain stabilizes the fibrin mesh in the clot (28).
Discussion
The rapid development of proteomic techniques in recent years has boosted novel approaches to detect and identify specific proteomes with potential impact in clinical research (29).
In clinical fields, proteomics is used to gain insights into pathological mechanisms and to identify new therapeutic targets by unveiling molecular pathways involved in disease progression. In stroke research, proteomic techniques have the potential to identify biomarkers useful for diagnosis and for secondary stroke prevention. In our study, the primary separation based on sample group in the PCA (component 1) shows some separation of LAA and CE etiologies, which is encouraging. Why for some of the samples there is some level of clustering, while for others there is some overlap is still unclear. It is possible that the presence of other pre-existing conditions, not always diagnosed, which might be common for both CE and LAA etiologies could explain this phenomenon and is worthy of further investigation. Although adjusted p-values did not indicate any significant difference in abundance between CE and LAA clots, significant differences in protein abundances between CE and LAA clots were observed in an analysis in which p-values were not corrected for multiple hypothesis testing. Adjusted p-values are related to sample size, which is quite small in our case. To moderate this limitation, we used the stringent filter of 70% valid values in our analysis workflow producing the non-adjusted p-values which show clear statistically significance differences between clots of CE or LAA etiology. Future work with larger sample numbers, with differences in abundance corrected for multiple hypothesis testing is required. However, the present study is encouraging and suggests that FFPE samples analyzed by MS are suitable for biomarker discovery.
Proteomic analysis of FFPE samples is more challenging than using fresh frozen tissues. However, if conditions for proteomic analysis with FFPE samples could be optimized and become routine, this could lead to improved availability of clinical samples for research enabling quicker discovery. Another advantage of using FFPE samples is that tissue could be used e.g., mass spectrometry analysis and histopathologic analysis in tandem.
Many research groups have successfully analyzed the proteome of a various tissue types and disease states using FFPE tissue (7–10), and have shown similarity in the fresh frozen and FFPE proteome (30). We successfully analyzed the proteome of FFPE samples in the current study, identifying 1,581 protein groups as part of the proteome of AIS thrombi using LC-MS/MS. Our results are in line with previous studies performed on FF samples, such as the study of Dargazanli et al. who identified 2,455 proteins in 50 samples (31) and the earlier study by Munoz et al., which used four samples and identified a proteome in the order of 1,600 proteins (32).
Both of these earlier studies found a set of proteins of interest helpful to advance AIS thrombus proteome characterization (31, 32). We acknowledge that our use of FFPE samples allowed us to identify less proteins than previous studies which used FF samples. However, it is encouraging that many of the identified proteins and functional cascades highlighted in this and previous studies using FF tissue are similar. To further advance the feasibility of using FFPE samples in studies such as this, it would be of interest to carry out a direct comparison of the fresh frozen and FFPE proteome of the same sample halved upon collection.
Some of the most significantly enriched pathways found by Munoz et al. in the thrombus proteome included remodeling of epithelial adherents junctions, protein ubiquitination, mitochondrial dysfunction, platelet activation and aggregation and acute inflammatory phase response signaling, including interleukin 6 (IL-6) and Tumor Necrosis Factor alpha (TNF-α) (32). Furthermore, the interactome characterization of the subset of proteins that were common in the four clots, revealed several protein networks that may be involved in thrombus formation, such as the interaction of fibronectin with 14-3-3 proteins. 14-3-3 protein in platelets interacts with several phosphoserine-dependent binding sites in the major platelet adhesion receptor, the glycoprotein Ib-IX complex, regulating its interaction with vWF and initiating thrombus formation (33). Dargazanli et al. compared the proteome of AIS clots of cardioembolic and atheroembolic etiology, and identified seven key proteins that could be indicative of cardioembolic etiology, in particular, coagulation factor XIII and proteins involved in cellular cytoskeletal assembly such as myosin light chain kinase and F-actin capping protein (31). Our results are in line with these findings, since coagulation factor XIII A chain is one of the most abundant proteins we found in CE clots. Additionally, we identified other proteins involved in cytoskeletal activity such as Actin-related protein 2/3 (Arp 2/3) complex subunit 2, Myosin-9, and Coronin-1C.
In our study, we found 14 proteins differentially abundant in clots of the two different etiologies. The protein ubiquitination pathway has been previously related to stroke (34) and our study confirms that several protein groups involved in this pathway are key factors for both CE and LAA etiology. Among the significantly different abundant proteins for LAA stroke etiology there were proteins involved in ubiquitin-proteasome pathway and the coagulation and plasminogen activating cascade. The significantly different abundant proteins for cardioembolic stroke etiology were also related to the ubiquitin proteasome pathway, and the coagulation cascade, but additionally, we identified proteins related to platelet structure and function. Also, a previous study evaluated the proteome of 20 AIS clots from different stroke etiology (35). The study from Rao et al. identified 81 common proteins in all 20 thrombi, while the presence of proteins associated with inflammation (e.g., Integrin Alpha-M) was observed in emboli from patients with high LDL (35). Overall, our findings support the small number of previous studies in the literature demonstrating the potential of mass spectrometry in discriminating thrombi of different stroke etiologies (31, 32, 35). Untargeted proteomics can help researchers to unveil the thrombus proteome, which could provide insights into triggers for thrombus formation, although more studies and targeted analyses are needed. Our findings are in line with our previous findings that CE clots are significantly more platelet and fibrin rich than LAA clots (36). Interestingly, a recent study investigating the proteome of coronary thrombus in patients with ST-segment elevation myocardial infarction, identified five focal adhesion proteins implicated in platelet activation as characteristic of coronary thrombi (37), acknowledging also the important role of platelets in atherosclerotic pathophysiology.
Furthermore, it would be interesting to compare the AIS clot proteome with thrombi retrieved from patients with myocardial infarction and venous thromboembolism, potentially leading to broad and targeted new therapeutic targets.
Proteins Involved in Ubiquitin-Proteasome System and ER Stress Unfolded Protein Response
Most stroke thrombi are formed in the periphery and subsequently embolise to the brain. The proteins identified reflect the molecular environment of the cells that are part of the thrombus or involved in thrombus development. The results from our study suggest that the ubiquitin-proteasome system (UPS) and ER stress may be part of an inflammatory pathway leading to thrombus formation and subsequently, to stroke. The UPS is a major protein degradation system in eukaryotes (38). A major task of the UPS is to identify and degrade aberrant proteins (misfolded proteins or normal short-lived proteins) which are recognized by the presence of degradation signals, such as small domains or motifs that recruit specific ubiquitin ligases resulting in ubiquitination and degradation of the target protein by the proteasome (38). Although ubiquitin/proteasome-dependent protein degradation is carried out in the nucleus and cytosol, there is a close connection between protein quality control in the endoplasmic reticulum (ER) and the UPS (39). Proteins that fail the ER quality control are transported back to the cytosol where they are rapidly destroyed by the UPS, in a process called Endoplasmic Reticulum Associated Degradation (ERAD) (40). The increase in misfolded/unfolded proteins in the ER lumen triggers the activation of the Unfolded Protein Response (UPR) (41). Our results suggest that both the UPS and the UPR are involved in the molecular mechanisms leading to stroke, since several protein groups identified as most abundant in LAA and CE clots are directly connected with these pathways.
Three out of four protein groups identified as most abundant in LAA clots are part of the UPS, Ubiquitin-60S ribosomal protein L40, Ubiquitin-conjugating enzyme E2 (also called Ubc13), and Ubiquitin-conjugating enzyme E2 variant 1. Ubiquitin-60S ribosomal protein L40, coded by the gene UBA52, is a single ubiquitin fused at the C-terminus to ribosomal protein (RP) L40 (42). It has been shown that the UPS can be directly activated by oxidative stress, both increasing the expression of UBA52 and the ubiquitin-conjugating activity to numerous endogenous substrates (18). The overexpression of UBA52 leads, in turn, to the expression of other antioxidant genes via nuclear translocation of the nuclear factor-like 2 (Nrf2) and to the modulation of P52 toward cell repairing or apoptosis when the stress situation is beyond repair (43). Furthermore, it also influences the activity of FOXO, a transcription factor family whose dysregulation is involved in several cardiovascular diseases (44) and of the pRb pathway, which is a key system of the cell-cycle machinery (45). Interestingly, overexpression of UBA52 was found significantly altered in advanced carotid atherosclerotic plaque in humans (46), which is in line with the findings reported in the present study.
Ubiquitin E2 conjugating enzyme, (Ubc13, and its variant coded by UBE2V1), is another member of the UPS that participates with many different E3 ligases to form lysine 63-linked (Lys63) ubiquitin chains, which are critical to inflammatory signaling and DNA damage response pathways (47). Ubc13 is responsible for non-canonical ubiquitination of TNF receptor-associated factor (TRAF)-family adapter proteins involved in Toll-like receptor and TNF-family cytokine receptor signaling, promoting inflammatory responses (48). It has been suggested that agents reducing Ubc13 activity could have therapeutic utility for treatment of inflammatory and autoimmune disorders (48).
We also found that the significantly different abundant proteins for CE stroke etiology are related to UPS and/or ER stress: Ubiquitin-like modifier-activating enzyme 1 (UBA1), protein disulphide-isomerase A6 (PDIA6) and Valosin-containing protein (VCP).
UBA1 is the E1 enzyme at the apex of ubiquitin signaling in the DNA Damage Response (DDR) (49). This signal transduction pathway that detects lesions in DNA and ensures cell and organismal survival through coordination of DNA repair and DNA replication with physiological processes, including cell cycle progression and transcription (50). Furthermore, UBA1 is also a key component of the UPS, since it catalyzes the first step in ubiquitin conjugation to mark cellular proteins for degradation via the proteasome (51) and has been implicated in the development of cancer and neurodegenerative diseases (51, 52).
PDIA6 is a protein disulphide isomerase present in the eukaryotic ER (53) that negatively regulates the UPR following ER stress (25). An in-vivo study in mice showed that PDIA6 can protect cardiac myocytes against simulated ischemia/reperfusion-induced death in a manner that is dependent on the catalytic activity of the enzyme (54). Furthermore, PDIA6 plays a role in a number of pathways related to platelet activities, such as platelet aggregation, secretion and fibrinogen binding (55).
VCP activity is required for maintenance of normal ER structure and function (26) and it helps in the extraction of terminally misfolded polypeptides from the ER into the cytosol for degradation by the UPS in the ERAD process (56).
It has also been reported that ER stress and the activation of UPR might be a driving force behind the formation of proplatelets that generate platelets (57). A recent study observed that megakaryocyte differentiation is associated with caspase activation and can be inducted by ER stress (58).
Proteins Involved in Blood Coagulation
Fibrinogen alpha chain is one of the three chains forming the soluble protein fibrinogen and one of the most abundant proteins in LAA clots. Apart from its crucial role in coagulation (20), several studies highlight that the C-terminal domain of the fibrinogen α chain (αC domain) is implicated in different clotting abnormalities (59), amyloid deposits in Alzheimer's disease (60) and familiar renal amyloidosis (61). For example, there is some evidence that the α-fibrinogen T312A variant might influence clot structure through increased factor XIII cross-linking, leading to the formation of fibrin clots that could predispose to embolization (62, 63). Furthermore, it seems that the fibrinogen associated with Aβ peptides might contribute to the development of Alzheimer's disease by accumulating around or inside brain blood vessels (64), provoking the degeneration of vessel wall components, adversely affecting cerebral blood flow and worsening cognitive decline (65).
Seven of the significantly different abundant proteins for CE stroke etiology are related to platelet function.
The proteins myosin-9, coronin-1C and actin-related protein (Arp) 2/3 complex subunit 2, for example, are in involved cytoskeletal remodeling of platelets (21–23), which undergo profound shape changes during activation and adhesion (66). Myosin-9 plays a crucial role in organelle distribution and F-actin organization in megakaryocytes and platelets (21) and mutations in the MYH9 gene have been related to several coagulation disorders (67). A recent case report described a correlation between MYH9-related thrombocytopenia and Moyamoya-like vasculopathy, a disorder defined by progressive occlusion or stenosis of the intracranial distal segments of the internal carotid arteries and the arteries of the circle of Willis, provoking ischemic stroke events (68). Coronin-1C works as a regulator of the actin cytoskeleton turnover (69) together with actin-related protein 2/3 complex (23, 70). There are several studies indicating the relevance of members of the evolutionarily conserved coronin family of proteins in stroke and thrombus formation, since they are crucial for platelet function (23), but also for controlling lipoprotein uptake and degradation in macrophages (71). Furthermore, coronins, specifically overexpression of coronin-1C, has been implicated in several cancers (72–75), therefore, it may be an interesting starting point for the study of stroke-related tumors.
The platelet glycoproteins Ib alpha chain (GPIbα) and IX are two other protein groups found significantly different abundant for CE stroke etiology. Their role is extremely interconnected since they are part of the glycoprotein Ib-IX-V complex (24), which is a crucial platelet receptor for initial tethering and adhesion at sites of vascular injury. In particular, upon vascular damage, the vWF protein is exposed and binds to GPIbα, which is responsible for the initial platelet adhesion, as shown by the bleeding disorders Bernard Soulier syndrome (76) and von Willebrand disease (77). Studies in mice demonstrated that GPIbα is an important mediator of ischemic stroke, suggesting that targeted inhibition of this receptor may open new approaches for stroke treatment (78), especially in view of the thromboinflammatory axis played by VWF-GPIbα interaction in acute ischemic stroke (79).
The coagulation factor XIII A chain is part of coagulation factor XIII, a heterotetrameric protein complex consisting of a dimer of A subunits (FXIIIA2), and a dimer of B subunits (FXIIIB2) (80, 81). The role of this protein in blood clotting is extremely important since its activation, by thrombin and Ca2+ represents the last step of the coagulation cascade (82). The FXIIIA2 subunit is the catalytic subunit and it has transglutaminase activity that covalently cross-links fibrin polymers to confer stability to the blood clot and resistance against premature fibrinolysis (83). Apart from its physiological activity, there are several studies that showed out how polymorphisms in coagulation factor XIII (especially related to the A subunit) might be linked to thrombotic diseases (84–86) and possibly to an increased risk of acute ischemic stroke, especially in patients with coronary artery disease and/or myocardial infarction (86, 87). These findings are in line with our results and suggest that coagulation factor XIII A should be further investigated as a possible biomarker for AIS, possibly related to cardioembolic etiology.
Finally, Ras-related protein Rab-27b (encoded by the gene RAB27B) is another protein we found as significantly different abundant proteins for CE stroke etiology. Like the majority of the protein groups most abundant in CE clots, its function is related to platelets, as highlighted by a previous study investigating the platelet proteome (88). There is evidence that this protein is a key regulator of dense granule secretion in platelets (27). In addition, ras-related protein Rab-27b belongs to the class of the Rab proteins, which are gaining recognition as important in the biogenesis and membrane structure of exosomes and may have therapeutic and diagnostic potential for a number of pathologies, included cancer, stroke and neurological disorders (89, 90).
Study Strengths and Limitations
As previously mentioned, extraction of biomolecules from FFPE material for mass spectrometry analysis remains a challenging task. However, we obtained a number of proteins comparable to FF samples used in previous studies present in literature, which is the major strength of our study. We acknowledge some limitations, such as the relatively small number of samples we included in our study. We also acknowledge that pre-stroke anticoagulant therapies and the treatment with recombinant tissue plasminogen activator before mechanical thrombectomy might also modify the proteome of the thrombus. However, as in our cohort, the percentages of rtPA yes and rtPA no patients are similar across the two etiologies, this makes it unlikely that thrombolysis is a confounder in our study. However, it would be valuable to probe in more depth the impact of potential confounders such as thrombolysis, prior medication, age, sex, comorbidities and stroke risk factors on the thrombus proteome in future studies.
Conclusion
This proof-of-principle study demonstrates the feasibility of mass spectrometry to characterize the proteome of FFPE blood clots as well as the possibility to identify specific proteins that may differentiate between CE and LAA etiology. Further studies with larger cohorts of patients are needed. Our results confirm that quantitative proteomic studies performed on the clots that cause stroke in AIS patients can unveil important information to enrich our understanding of stroke pathophysiology.
Data Availability Statement
The datasets presented in this study can be found in online repositories. The name of the repository and accession number can be found below: Mass Spectrometry Interactive Virtual Environment (MassIVE), https://massive.ucsd.edu/ProteoSAFe/static/massive.jsp, MSV000088792'.
Ethics Statement
The studies involving human participants were reviewed and approved by prior to this study, approval of the Regional Hospital Ethics Committees and National University of Ireland Galway (NUIG) Research Ethics Committees (16-SEPT-08) was obtained in accordance with the ethical standards of the Declaration of Helsinki and its amendments. The patients/participants provided their written informed consent to participate in this study.
Author Contributions
KD obtained the funds for the study, coordinated the implementation of the study, and supervised the writing of the manuscript. RR participated in samples collection, developed the study design, developed the proteome extraction method, performed the bioinformatic analysis, composed the manuscript, and wrote the results and discussion. OM participated to the development of the extraction protocol and helped in sample collection. MB, SO'C, and CT participated in performing the bioinformatic analysis. SM and AD participated in samples collection, analysis, and interpretation of results. KP, GT, AR, and JT performed the thrombectomy at the several hospitals, evaluated stroke etiology, and collected samples and procedural data. KP, GT, IS, TT, AR, and JT contributed to the study design and were responsible thrombus collection at the relevant stroke center. AP, MG, and RM contributed to develop the study design and funding acquisition. PÓ supervised the bioinformatic analysis. All authors have read and reviewed the manuscript.
Funding
This publication has emanated from research conducted with the financial support of Science Foundation Ireland (SFI) and is co-funded under the European Regional Development Fund under Grant No. 13/RC/2073_2. Furthermore, the authors declare that this study received funding from Cerenovus. The funder was not involved in the study design, collection, analysis, interpretation of data, the writing of this article or the decision to submit it for publication.
Conflict of Interest
MG and RM were employed by company Cerenovus.
The remaining authors declare that the research was conducted in the absence of any commercial or financial relationships that could be construed as a potential conflict of interest.
Publisher's Note
All claims expressed in this article are solely those of the authors and do not necessarily represent those of their affiliated organizations, or those of the publisher, the editors and the reviewers. Any product that may be evaluated in this article, or claim that may be made by its manufacturer, is not guaranteed or endorsed by the publisher.
Acknowledgments
The authors wish to acknowledge the invaluable contributions made by the Interventional/Nursing and Clinical coordination teams at each of the sites included in the RESTORE registry. Special acknowledgments to Ms. Caroline Batchelor for the Mass Spectrometric analysis of the samples at Maynooth University.
Supplementary Material
The Supplementary Material for this article can be found online at: https://www.frontiersin.org/articles/10.3389/fneur.2022.854846/full#supplementary-material
References
1. Krishnamurthi RV, Ikeda T, Feigin VL. Global, regional and country-specific burden of ischaemic stroke, intracerebral haemorrhage and subarachnoid haemorrhage: a systematic analysis of the global burden of disease study 2017. Neuroepidemiology. (2020) 54:171–9. doi: 10.1159/000506396
2. Virani SS, Alonso A, Benjamin EJ, Bittencourt MS, Callaway CW, Carson AP, et al. Heart disease and stroke statistics-2020 update: A report from the american heart association. Circulation. (2020) 141:e139–e596. doi: 10.1161/CIR.0000000000000757
3. Turc G, Bhogal P, Fischer U, Khatri P, Lobotesis K, Mazighi M, et al. European Stroke Organisation (ESO)-European Society for Minimally Invasive Neurological Therapy (ESMINT) guidelines on mechanical thrombectomy in acute ischemic stroke. J Neurointerv Surg. (2019) 4:6–12. doi: 10.1136/neurintsurg-2018-014569
4. Tanca A, Pagnozzi D, Burrai GP, Polinas M, Uzzau S, Antuofermo E, et al. Comparability of differential proteomics data generated from paired archival fresh-frozen and formalin-fixed samples by gelc–ms/ms and spectral counting. J Proteomics. (2012) 77:561–76. doi: 10.1016/j.jprot.2012.09.033
5. Giusti L, Lucacchini A. Proteomic studies of formalin-fixed paraffin-embedded tissues. Expert Rev Proteomics. (2013) 10:165–77. doi: 10.1586/epr.13.3
6. Fox CH, Johnson FB, Whiting J, Roller PP. Formaldehyde fixation. J Histochem Cytochem. (1985) 33:845–53. doi: 10.1177/33.8.3894502
7. Paulo JA, Lee LS, Banks PA, Steen H, Conwell DL. Proteomic analysis of formalin-fixed paraffin-embedded pancreatic tissue using liquid chromatography tandem mass spectrometry. Pancreas. (2012) 41:175–85. doi: 10.1097/MPA.0b013e318227a6b7
8. Buck A, Heijs B, Beine B, Schepers J, Cassese A, Heeren RMA, et al. Round robin study of formalin-fixed paraffin-embedded tissues in mass spectrometry imaging. Anal Bioanal Chem. (2018) 410:5969–80. doi: 10.1007/s00216-018-1216-2
9. Foll MC, Fahrner M, Oria VO, Kuhs M, Biniossek ML, Werner M, et al. Reproducible proteomics sample preparation for single ffpe tissue slices using acid-labile surfactant and direct trypsinization. Clin Proteomics. (2018) 15:11. doi: 10.1186/s12014-018-9188-y
10. Lai X, Schneider BP. Integrated and convenient procedure for protein extraction from formalin-fixed, paraffin-embedded tissues for lc-ms/ms analysis. Proteomics. (2014) 14:2623–7. doi: 10.1002/pmic.201400110
11. [the helsinki declaration of the world medical association (wma). Ethical principles of medical research involving human subjects]. Pol Merkur Lekarski. (2014). 36:298–301
12. Adams HP, Bendixen BH, Kappelle LJ, Biller J, Love BB, Gordon DL, et al. Classification of subtype of acute ischemic stroke. Definitions for use in a multicenter clinical trial. Toast. Trial of org 10172 in acute stroke treatment. Stroke. (1993) 24:35–41. doi: 10.1161/01.STR.24.1.35
13. Douglas A, Fitzgerald S, Pandit A, Doyle KM. Storage of blood clots for histological analysis: how long is too long in saline and paraformaldehyde? Histol Histopathol. (2020) 35:313–20. doi: 10.14670/HH-18-154
14. Tyanova S, Temu T, Cox J. The maxquant computational platform for mass spectrometry-based shotgun proteomics. Nat Protoc. (2016) 11:2301–19. doi: 10.1038/nprot.2016.136
15. Tyanova S, Cox J. Perseus: a bioinformatics platform for integrative analysis of proteomics data in cancer research. Methods Mol Biol. (2018) 1711:133–48. doi: 10.1007/978-1-4939-7493-1_7
16. Thomas PD, Campbell MJ, Kejariwal A, Mi H, Karlak B, Daverman R, et al. Panther: a library of protein families and subfamilies indexed by function. Genome Res. (2003) 13:2129–41. doi: 10.1101/gr.772403
17. Szklarczyk D, Gable AL, Nastou KC, Lyon D, Kirsch R, Pyysalo S, et al. The string database in 2021: Customizable protein-protein networks, and functional characterization of user-uploaded gene/measurement sets. Nucleic Acids Res. (2021) 49:D605-d612. doi: 10.1093/nar/gkab835
18. Fernandes R, Ramalho J, Pereira P. Oxidative stress upregulates ubiquitin proteasome pathway in retinal endothelial cells. Mol Vis. (2006) 12:1526–35. Available online at: http://www.molvis.org/molvis/v12/a175/
19. Deng L, Wang C, Spencer E, Yang L, Braun A, You J, et al. Activation of the ikappab kinase complex by traf6 requires a dimeric ubiquitin-conjugating enzyme complex and a unique polyubiquitin chain. Cell. (2000) 103:351–61. doi: 10.1016/S0092-8674(00)00126-4
20. Mosesson MW. Fibrinogen and fibrin structure and functions. J Thromb Haemost. (2005) 3:1894–904. doi: 10.1111/j.1538-7836.2005.01365.x
21. Pertuy F, Eckly A, Weber J, Proamer F, Rinckel JY, Lanza F, et al. Myosin iia is critical for organelle distribution and f-actin organization in megakaryocytes and platelets. Blood. (2014) 123:1261–9. doi: 10.1182/blood-2013-06-508168
22. Li Z, Kim ES, Bearer EL. Arp2/3 complex is required for actin polymerization during platelet shape change. Blood. (2002) 99:4466–74. doi: 10.1182/blood.V99.12.4466
23. Riley DRJ, Khalil JS, Pieters J, Naseem KM, Rivero F. Coronin 1 is required for integrin β2 translocation in platelets. Int J Mol Sci. (2020) 21:356. doi: 10.3390/ijms21010356
24. Bergmeier W, Chauhan AK, Wagner DD. Glycoprotein ibalpha and von willebrand factor in primary platelet adhesion and thrombus formation: lessons from mutant mice. Thromb Haemost. (2008) 99:264–70. doi: 10.1160/TH07-10-0638
25. Eletto D, Eletto D, Dersh D, Gidalevitz T, Argon Y. Protein disulfide isomerase a6 controls the decay of ire1α signaling via disulfide-dependent association. Mol Cell. (2014) 53:562–76. doi: 10.1016/j.molcel.2014.01.004
26. Wójcik C, Rowicka M, Kudlicki A, Nowis D, McConnell E, Kujawa M, et al. Valosin-containing protein (p97) is a regulator of endoplasmic reticulum stress and of the degradation of n-end rule and ubiquitin-fusion degradation pathway substrates in mammalian cells. Mol Biol Cell. (2006) 17:4606–18. doi: 10.1091/mbc.e06-05-0432
27. Tolmachova T, Abrink M, Futter CE, Authi KS, Seabra MC. Rab27b regulates number and secretion of platelet dense granules. Proc Natl Acad Sci USA. (2007) 104:5872–7. doi: 10.1073/pnas.0609879104
28. Ariëns RA, Philippou H, Nagaswami C, Weisel JW, Lane DA, Grant PJ. The factor xiii v34l polymorphism accelerates thrombin activation of factor xiii and affects cross-linked fibrin structure. Blood. (2000) 96:988–95. doi: 10.1182/blood.V96.3.988
29. Aebersold R, Mann M. Mass-spectrometric exploration of proteome structure and function. Nature. (2016) 537:347–55. doi: 10.1038/nature19949
30. Zhu Y, Weiss T, Zhang Q, Sun R, Wang B, Yi X, et al. High-throughput proteomic analysis of ffpe tissue samples facilitates tumor stratification. Mol Oncol. (2019) 13:2305–28. doi: 10.1002/1878-0261.12570
31. Dargazanli C, Zub E, Deverdun J, Decourcelle M, de Bock F, Labreuche J, et al. Machine learning analysis of the cerebrovascular thrombi proteome in human ischemic stroke: an exploratory study. Front Neurol. (2020) 11:575376. doi: 10.3389/fneur.2020.575376
32. Munoz R, Santamaria E, Rubio I, Ausin K, Ostolaza A, Labarga A, et al. Mass spectrometry-based proteomic profiling of thrombotic material obtained by endovascular thrombectomy in patients with ischemic stroke. Int J Mol Sci. (2018) 19:498. doi: 10.3390/ijms19020498
33. Chen Y, Ruggeri ZM, Du X. 14–3-3 proteins in platelet biology and glycoprotein ib-ix signaling. Blood. (2018). 131:2436–48. doi: 10.1182/blood-2017-09-742650
34. Meller R. The role of the ubiquitin proteasome system in ischemia and ischemic tolerance. Neuroscientist. (2009) 15:243–60. doi: 10.1177/1073858408327809
35. Rao NM, Capri J, Cohn W, Abdaljaleel M, Restrepo L, Gornbein JA, et al. Peptide composition of stroke causing emboli correlate with serum markers of atherosclerosis and inflammation. Front Neurol. (2017) 8:427. doi: 10.3389/fneur.2017.00427
36. Fitzgerald S, Rossi R, Mereuta OM, Jabrah D, Okolo A, Douglas A, et al. Per-pass analysis of acute ischemic stroke clots: impact of stroke etiology on extracted clot area and histological composition. J Neurointerv Surg. (2020) 13:1111–6. doi: 10.1136/neurintsurg-2020-016966
37. Alonso-Orgaz S, Moreno-Luna R, López JA, Gil-Dones F, Padial LR, Moreu J, et al. Proteomic characterization of human coronary thrombus in patients with st-segment elevation acute myocardial infarction. J Proteomics. (2014) 109:368–81. doi: 10.1016/j.jprot.2014.07.016
38. Hershko A, Ciechanover A. The ubiquitin system. Annu Rev Biochem. (1998) 67:425–79. doi: 10.1146/annurev.biochem.67.1.425
39. Menéndez-Benito V, Verhoef LGGC, Masucci MG, Dantuma NP. Endoplasmic reticulum stress compromises the ubiquitin–proteasome system. Hum Mol Genet. (2005) 14:2787–99. doi: 10.1093/hmg/ddi312
40. Plemper RK, Wolf DH. Retrograde protein translocation: Eradication of secretory proteins in health and disease. Trends Biochem Sci. (1999) 24:266–70. doi: 10.1016/S0968-0004(99)01420-6
41. Bravo R, Parra V, Gatica D, Rodriguez AE, Torrealba N, Paredes F, et al. Endoplasmic reticulum and the unfolded protein response: dynamics and metabolic integration. Int Rev Cell Mol Biol. (2013) 301:215–90. doi: 10.1016/B978-0-12-407704-1.00005-1
42. Baker RT, Board PG. The human ubiquitin-52 amino acid fusion protein gene shares several structural features with mammalian ribosomal protein genes. Nucleic Acids Res. (1991) 19:1035–40. doi: 10.1093/nar/19.5.1035
43. Fasanaro P, Capogrossi MC, Martelli F. Regulation of the endothelial cell cycle by the ubiquitin-proteasome system. Cardiovasc Res. (2010) 85:272–80. doi: 10.1093/cvr/cvp244
44. Maiese K, Chong ZZ, Shang YC, Hou J. Foxo proteins: cunning concepts and considerations for the cardiovascular system. Clin Sci. (2009) 116:191–203. doi: 10.1042/CS20080113
45. Delston RB, Harbour JW. Rb at the interface between cell cycle and apoptotic decisions. Curr Mol Med. (2006) 6:713–8. doi: 10.2174/1566524010606070713
46. Tan X, Zhang X, Pan L, Tian X, Dong P. Identification of key pathways and genes in advanced coronary atherosclerosis using bioinformatics analysis. Biomed Res Int. (2017) 2017:4323496. doi: 10.1155/2017/4323496
47. Hodge CD, Spyracopoulos L, Glover JN. Ubc13: the lys63 ubiquitin chain building machine. Oncotarget. (2016) 7:64471–504. doi: 10.18632/oncotarget.10948
48. Fukushima T, Matsuzawa S, Kress CL, Bruey JM, Krajewska M, Lefebvre S, et al. Ubiquitin-conjugating enzyme ubc13 is a critical component of tnf receptor-associated factor (traf)-mediated inflammatory responses. Proc Natl Acad Sci USA. (2007) 104:6371–6. doi: 10.1073/pnas.0700548104
49. Moudry P, Lukas C, Macurek L, Hanzlikova H, Hodny Z, Lukas J, et al. Ubiquitin-activating enzyme uba1 is required for cellular response to DNA damage. Cell Cycle. (2012) 11:1573–82. doi: 10.4161/cc.19978
50. Ciccia A, Elledge SJ. The DNA damage response: making it safe to play with knives. Mol Cell. (2010) 40:179–204. doi: 10.1016/j.molcel.2010.09.019
51. Groen EJN, Gillingwater TH. Uba1: at the crossroads of ubiquitin homeostasis and neurodegeneration. Trends Mol Med. (2015) 21:622–32. doi: 10.1016/j.molmed.2015.08.003
52. Xu W, Lukkarila JL, da Silva SR, Paiva SL, Gunning PT, Schimmer AD. Targeting the ubiquitin e1 as a novel anti-cancer strategy. Curr Pharm Des. (2013) 19:3201–9. doi: 10.2174/1381612811319180004
53. Hatahet F, Ruddock LW. Protein disulfide isomerase: a critical evaluation of its function in disulfide bond formation. Antioxid Redox Signal. (2009) 11:2807–50. doi: 10.1089/ars.2009.2466
54. Vekich JA, Belmont PJ, Thuerauf DJ, Glembotski CC. Protein disulfide isomerase-associated 6 is an atf6-inducible er stress response protein that protects cardiac myocytes from ischemia/reperfusion-mediated cell death. J Mol Cell Cardiol. (2012) 53:259–67. doi: 10.1016/j.yjmcc.2012.05.005
55. Jordan PA, Stevens JM, Hubbard GP, Barrett NE, Sage T, Authi KS, et al. A role for the thiol isomerase protein erp5 in platelet function. Blood. (2005) 105:1500–7. doi: 10.1182/blood-2004-02-0608
56. DeLaBarre B, Christianson JC, Kopito RR, Brunger AT. Central pore residues mediate the p97/vcp activity required for erad. Mol Cell. (2006) 22:451–62. doi: 10.1016/j.molcel.2006.03.036
57. Morishima N, Nakanishi K. Proplatelet formation in megakaryocytes is associated with endoplasmic reticulum stress. Genes Cells. (2016) 21:798–806. doi: 10.1111/gtc.12384
58. Kovuru N, Raghuwanshi S, Sharma DS, Dahariya S, Pallepati A, Gutti RK. Endoplasmic reticulum stress induced apoptosis and caspase activation is mediated through mitochondria during megakaryocyte differentiation. Mitochondrion. (2020) 50:115–20. doi: 10.1016/j.mito.2019.10.009
59. Soria J, Mirshahi S, Mirshahi SQ, Varin R, Pritchard LL, Soria C, et al. Fibrinogen αc domain: Its importance in physiopathology. Res Pract Thromb Haemost. (2019) 3:173–83. doi: 10.1002/rth2.12183
60. Cortes-Canteli M, Paul J, Norris EH, Bronstein R, Ahn HJ, Zamolodchikov D, et al. Fibrinogen and beta-amyloid association alters thrombosis and fibrinolysis: a possible contributing factor to alzheimer's disease. Neuron. (2010) 66:695–709. doi: 10.1016/j.neuron.2010.05.014
61. Dember LM. Amyloidosis-associated kidney disease. J Am Soc Nephrol. (2006) 17:3458–71. doi: 10.1681/ASN.2006050460
62. Li JF, Lin Y, Yang YH, Gan HL, Liang Y, Liu J, et al. Fibrinogen aα thr312ala polymorphism specifically contributes to chronic thromboembolic pulmonary hypertension by increasing fibrin resistance. PLoS ONE. (2013) 8:e69635. doi: 10.1371/journal.pone.0069635
63. Standeven KF, Grant PJ, Carter AM, Scheiner T, Weisel JW, Ariëns RA. Functional analysis of the fibrinogen aalpha thr312ala polymorphism: effects on fibrin structure and function. Circulation. (2003) 107:2326–30. doi: 10.1161/01.CIR.0000066690.89407.CE
64. Ahn HJ, Chen ZL, Zamolodchikov D, Norris EH, Strickland S. Interactions of β-amyloid peptide with fibrinogen and coagulation factor xii may contribute to alzheimer's disease. Curr Opin Hematol. (2017) 24:427–31. doi: 10.1097/MOH.0000000000000368
65. Thal DR, Capetillo-Zarate E, Larionov S, Staufenbiel M, Zurbruegg S, Beckmann N. Capillary cerebral amyloid angiopathy is associated with vessel occlusion and cerebral blood flow disturbances. Neurobiol Aging. (2009) 30:1936–48. doi: 10.1016/j.neurobiolaging.2008.01.017
66. Hartwig JH, Barkalow K, Azim A, Italiano J. The elegant platelet: Signals controlling actin assembly. Thromb Haemost. (1999) 82:392–8. doi: 10.1055/s-0037-1615858
67. Althaus K, Greinacher A. Myh9-related platelet disorders. Semin Thromb Hemost. (2009) 35:189–203. doi: 10.1055/s-0029-1220327
68. Ribigan AC, Badea RS, Ciocan A, Stefan D, Casaru B, Ioan P, et al. Moyamoya-like vasculopathy associated to myh9-related thrombocytopenia manifested by multiple cerebral ischemic lesions: a case report. BMC Neurol. (2020) 20:352. doi: 10.1186/s12883-020-01927-6
69. Chan KT, Creed SJ, Bear JE. Unraveling the enigma: progress towards understanding the coronin family of actin regulators. Trends Cell Biol. (2011) 21:481–8. doi: 10.1016/j.tcb.2011.04.004
70. Sokolova OS, Chemeris A, Guo S, Alioto SL, Gandhi M, Padrick S, et al. Structural basis of arp2/3 complex inhibition by gmf, coronin, and arpin. J Mol Biol. (2017) 429:237–48. doi: 10.1016/j.jmb.2016.11.030
71. Hölttä-Vuori M, Vainio S, Kauppi M, Van Eck M, Jokitalo E, Ikonen E. Endosomal actin remodeling by coronin-1a controls lipoprotein uptake and degradation in macrophages. Circ Res. (2012) 110:450–5. doi: 10.1161/CIRCRESAHA.111.256842
72. Thal D, Xavier CP, Rosentreter A, Linder S, Friedrichs B, Waha A, et al. Expression of coronin-3 (coronin-1c) in diffuse gliomas is related to malignancy. J Pathol. (2008) 214:415–24. doi: 10.1002/path.2308
73. Luan SL, Boulanger E, Ye H, Chanudet E, Johnson N, Hamoudi RA, et al. Primary effusion lymphoma: genomic profiling revealed amplification of selplg and coro1c encoding for proteins important for cell migration. J Pathol. (2010) 222:166–79. doi: 10.1002/path.2752
74. Wu L, Peng CW, Hou JX, Zhang YH, Chen C, Chen LD, et al. Coronin-1c is a novel biomarker for hepatocellular carcinoma invasive progression identified by proteomics analysis and clinical validation. J Exp Clin Cancer Res. (2010) 29:17. doi: 10.1186/1756-9966-29-17
75. Tagliatela AC, Hempstead SC, Hibshman PS, Hockenberry MA, Brighton HE, Pecot CV, et al. Coronin 1c inhibits melanoma metastasis through regulation of mt1-mmp-containing extracellular vesicle secretion. Sci Rep. (2020) 10:11958. doi: 10.1038/s41598-020-67465-w
76. Salles II, Feys HB, Iserbyt BF, De Meyer SF, Vanhoorelbeke K, Deckmyn H. Inherited traits affecting platelet function. Blood Rev. (2008) 22:155–72. doi: 10.1016/j.blre.2007.11.002
77. De Meyer SF, Deckmyn H, Vanhoorelbeke K. Von willebrand factor to the rescue. Blood. (2009) 113:5049–57. doi: 10.1182/blood-2008-10-165621
78. De Meyer SF, Schwarz T, Schatzberg D, Wagner DD. Platelet glycoprotein ibα is an important mediator of ischemic stroke in mice. Exp Transl Stroke Med. (2011) 3:9. doi: 10.1186/2040-7378-3-9
79. Denorme F, Vanhoorelbeke K, De Meyer SF. Von willebrand factor and platelet glycoprotein ib: a thromboinflammatory axis in stroke. Front Immunol. (2019) 10:2884. doi: 10.3389/fimmu.2019.02884
80. Schwartz ML, Pizzo SV, Hill RL, McKee PA. Human factor xiii from plasma and platelets. Molecular weights, subunit structures, proteolytic activation, and cross-linking of fibrinogen and fibrin. J Biol Chem. (1973) 248:1395–407. doi: 10.1016/S0021-9258(19)44312-3
81. Muszbek L, Ariëns RA, Ichinose A. Factor xiii: recommended terms and abbreviations. J Thromb Haemost. (2007) 5:181–3. doi: 10.1111/j.1538-7836.2006.02182.x
82. Komáromi I, Bagoly Z, Muszbek L. Factor xiii: novel structural and functional aspects. J Thromb Haemost. (2011) 9:9–20. doi: 10.1111/j.1538-7836.2010.04070.x
83. Klöck C, Khosla C. Regulation of the activities of the mammalian transglutaminase family of enzymes. Protein Sci. (2012) 21:1781–91. doi: 10.1002/pro.2162
84. Muszbek L, Bagoly Z, Bereczky Z, Katona E. The involvement of blood coagulation factor xiii in fibrinolysis and thrombosis. Cardiovasc Hematol Agents Med Chem. (2008) 6:190–205. doi: 10.2174/187152508784871990
85. Muszbek L, Bereczky Z, Bagoly Z, Komáromi I, Katona É. Factor xiii: a coagulation factor with multiple plasmatic and cellular functions. Physiol Rev. (2011) 91:931–72. doi: 10.1152/physrev.00016.2010
86. Bagoly Z, Koncz Z, Hársfalvi J, Muszbek L. Factor xiii, clot structure, thrombosis. Thromb Res. (2012) 129:382–7. doi: 10.1016/j.thromres.2011.11.040
87. Kohler HP, Ariëns RA, Catto AJ, Carter AM, Miller GJ, Cooper JA, et al. Factor xiii a-subunit concentration predicts outcome in stroke subjects and vascular outcome in healthy, middle-aged men. Br J Haematol. (2002) 118:825–32. doi: 10.1046/j.1365-2141.2002.03707.x
88. O'Neill EE, Brock CJ, von Kriegsheim AF, Pearce AC, Dwek RA, Watson SP, et al. Towards complete analysis of the platelet proteome. Proteomics. (2002) 2:288–305. doi: 10.1002/1615-9861(200203)2:3<288::aid-prot288>3.0.co;2-0
89. Agarwal S, Agarwal V, Agarwal M, Singh M. Exosomes: structure, biogenesis, types and application in diagnosis and gene and drug delivery. Curr Gene Ther. (2020) 20:195–206. doi: 10.2174/1566523220999200731011702
Keywords: biomarkers stroke etiology, stroke biomarkers, mass spectrometry proteomics, FFPE proteomics, thrombus proteome
Citation: Rossi R, Mereuta OM, Barbachan e Silva M, Molina Gil S, Douglas A, Pandit A, Gilvarry M, McCarthy R, O'Connell S, Tierney C, Psychogios K, Tsivgoulis G, Szikora I, Tatlisumak T, Rentzos A, Thornton J, Ó Broin P and Doyle KM (2022) Potential Biomarkers of Acute Ischemic Stroke Etiology Revealed by Mass Spectrometry-Based Proteomic Characterization of Formalin-Fixed Paraffin-Embedded Blood Clots. Front. Neurol. 13:854846. doi: 10.3389/fneur.2022.854846
Received: 14 January 2022; Accepted: 25 March 2022;
Published: 19 April 2022.
Edited by:
Steffen Tiedt, LMU Munich University Hospital, GermanyReviewed by:
Ali Azimi, The University of Sydney, AustraliaAurora Semerano, San Raffaele Scientific Institute (IRCCS), Italy
Frederik Denorme, The University of Utah, United States
Teresa Wölfer, LMU Munich University Hospital, Germany
Copyright © 2022 Rossi, Mereuta, Barbachan e Silva, Molina Gil, Douglas, Pandit, Gilvarry, McCarthy, O'Connell, Tierney, Psychogios, Tsivgoulis, Szikora, Tatlisumak, Rentzos, Thornton, Ó Broin and Doyle. This is an open-access article distributed under the terms of the Creative Commons Attribution License (CC BY). The use, distribution or reproduction in other forums is permitted, provided the original author(s) and the copyright owner(s) are credited and that the original publication in this journal is cited, in accordance with accepted academic practice. No use, distribution or reproduction is permitted which does not comply with these terms.
*Correspondence: Rosanna Rossi, rosanna.rossi@nuigalway.ie; Karen M. Doyle, karen.doyle@nuigalway.ie