- Department of Neurology, School of Medicine, Washington University in St. Louis, St. Louis, MO, United States
Apart from common respiratory symptoms, neurological symptoms are prevalent among patients with COVID-19. Research has shown that infection with SARS-CoV-2 accelerated alpha-synuclein aggregation, induced Lewy-body-like pathology, caused dopaminergic neuron senescence, and worsened symptoms in patients with Parkinson's disease (PD). In addition, SARS-CoV-2 infection can induce neuroinflammation and facilitate subsequent neurodegeneration in long COVID, and increase individual vulnerability to PD or parkinsonism. These findings suggest that a post-COVID-19 parkinsonism might follow the COVID-19 pandemic. In order to prevent a possible post-COVID-19 parkinsonism, this paper reviewed neurological symptoms and related findings of COVID-19 and related infectious diseases (influenza and prion disease) and neurodegenerative disorders (Alzheimer's disease, PD and amyotrophic lateral sclerosis), and discussed potential mechanisms underlying the neurological symptoms and the relationship between the infectious diseases and the neurodegenerative disorders, as well as the therapeutic and preventive implications in the neurodegenerative disorders. Infections with a relay of microbes (SARS-CoV-2, influenza A viruses, gut bacteria, etc.) and prion-like alpha-synuclein proteins over time may synergize to induce PD. Therefore, a systematic approach that targets these pathogens and the pathogen-induced neuroinflammation and neurodegeneration may provide cures for neurodegenerative disorders. Further, antiviral/antimicrobial drugs, vaccines, immunotherapies and new therapies (e.g., stem cell therapy) need to work together to treat, manage or prevent these disorders. As medical science and technology advances, it is anticipated that better vaccines for SARS-CoV-2 variants, new antiviral/antimicrobial drugs, effective immunotherapies (alpha-synuclein antibodies, vaccines for PD or parkinsonism, etc.), as well as new therapies will be developed and made available in the near future, which will help prevent a possible post-COVID-19 parkinsonism in the 21st century.
Introduction
It has been reported during the coronavirus disease 2019 (COVID-19) pandemic that around a third of patients with COVID-19 suffered from neuropsychiatric disorders (1), and 82% of patients hospitalized with COVID-19 had neurological symptoms such as headache, loss of sense of smell and/or taste, encephalopathy, cognitive impairment, impaired consciousness and stroke (2). COVID-19 is caused by infection with severe acute respiratory syndrome coronavirus 2 (SARS-CoV-2). Viral infections such as infection with herpes simplex virus 1 (HSV-1), influenza A virus or human immunodeficiency virus (HIV) can trigger neuroinflammation (which initiates neurodegeneration) (3), and increase the risk of Alzheimer's disease (AD) (4), Parkinson's disease (PD) (5), or amyotrophic lateral sclerosis (ALS) (6). SARS-CoV-2 infection has been found to induce neuroinflammation (7), and increase the risk of AD and PD in susceptible individuals (8–11). One of the consequences of SARS-CoV-2 infection is lasting neuroinflammation and subsequent neurodegeneration in long COVID (11–13).
Historically, there was an outbreak of encephalitis lethargica and a global spreading of parkinsonism following the 1918 influenza pandemic, and people who were born during the 1918 influenza pandemic had a 2~3 times higher risk of developing PD than those born before 1888 or after 1924. Reexamination of the 1918 influenza pandemic data revealed that the influenza A virus was involved in PD (14). In addition, a recent study indicated that influenza was related to Parkinson's disease over 10 years following infection (odds ratio: 1.73), i.e., influenza infection was likely to increase the long-term risk of Parkinson's disease (15). These findings have highlighted the need to investigate COVID-19 neurological symptoms, complications and their mechanisms in the COVID-19 pandemic.
A number of cases have been reported that patients developed acute parkinsonism (several days or weeks) after contracting SARS-CoV-2 (16–22), and a large cohort study indicated that COVID-19 survivors had an estimated incidence of 0.11% for parkinsonism in the following 6 months after their COVID diagnosis (1). In addition, recent research found that SARS-CoV-2 nucleocapsid protein accelerated alpha-synuclein aggregation (in vitro) (23), and the presence of SARS-CoV-2 existed in the substantia nigra of 6 COVID-19 patients (24). Further, SARS-CoV-2 infection caused dopaminergic neuron (derived from human pluripotent stem cells) senescence (both in vitro and in vivo) (24), worsened preexisting PD symptoms in around 59% of PD patients (25), and increased susceptibility to parkinsonism induced by oxidative stress (9). These findings suggested that SARS-CoV-2 infection increases individual vulnerability of PD or parkinsonism, leading to the onset or progression of PD (or parkinsonism) or in some cases unmasking preexisting pre-symptomatic PD in susceptible individuals (26).
Searching for possible links between COVID-19 and PD or parkinsonism (9, 26, 27), clinicians and medical researchers have been asking, discussing and debating: Will there be a post-COVID-19 parkinsonism after the COVID-19 pandemic (11, 26, 28–31)? There are currently 10 reported COVID-19 associated acute parkinsonism cases (16–22), but according to the estimated incidence of 0.11% for parkinsonism among COVID-19 survivors in the large-cohort (n = 236,379) long follow-up study (1), 260 of them developed parkinsonism 6 months after COVID onset (1), which provided strong evidence and support for post-COVID-19 parkinsonism. The estimated incidence of 0.20% for parkinsonism among the COVID-19 survivors who were hospitalized due to COVID-19 together with the 0.11% incidence for parkinsonism among all the COVID-19 survivors in the large-cohort (1) further suggested that there would be much more post-COVID-19 parkinsonism cases than COVID-19 associated acute parkinsonism cases globally. Furthermore, this study revealed that there was no significant difference between the probability of developing post-infection parkinsonism in COVID-19 survivors after SARS-CoV-2 infection and that in influenza survivors after influenza infection (1).
There was emerging evidence of a Parkinson's “pandemic” before the COVID-19 pandemic (e.g., the total number of PD cases increased 118% globally from 1990 to 2015 and doubled from around 3 million in 1990 to over 6 million in 2015) and it was estimated that the global burden of Parkinson's disease (accounting for changes in aging, industrialization, etc.) would be over 17 million by 2040 (32). The widespread SARS-CoV-2 infection during the COVID-19 pandemic increased the risk of PD or parkinsonism (9, 24, 26), either triggered parkinsonism (16, 19) or unmasked preexisting prodromal (or pre-symptomatic) PD in COVID-19 patients or survivors [especially in long COVID (1)] around the globe. In addition, since (1) a number of COVID-19 symptoms (loss of sense of smell, taste impairment, dizziness, etc.) and long COVID symptoms (cognitive impairment, tremors, functional mobility impairment, gastrointestinal problems, sleep disorder, etc.) (13, 33–35) overlap with symptoms of PD or parkinsonism, (2) the evidence of the COVID-associated acute parkinsonism cases (16–22), the 260 (0.11%) post-COVID-19 parkinsonism cases (1), high percentages of functional mobility impairment (44%) and mobility decline (20.2%) in long COVID (13), and an increasing trend of motor symptoms like tremors (35) among patients with long COVID has suggested that SARS-CoV-2 infection may trigger (acute or chronic) neural injury in the nigrostriatal system, a long lasting neuroinflammation and subsequent motor-associated neurodegeneration, (3) there are much similarities between SARS-CoV-2 and H1N1 influenza virus [in viral contagiousness/transmission, viral infection fatality/severity, immune system activation, cytokine storm induction, neuroinvasion, cellular aging pathway modulation, association with increased risk/susceptibility to PD or parkinsonism (9, 15), etc.], and the risk factors for the recent Parkinson's “pandemic” (such as aging and industrial/agricultural/chemical/metal pollution) still exist (32), there might be an increased global risk of PD or parkinsonism associated with long COVID and a possible post-COVID-19 parkinsonism (1). Therefore, the further question is: How to prevent a possible post-COVID-19 parkinsonism?
In order to raise public awareness of the neurological damage that SARS-CoV-2 infection induces and to prevent possible post-COVID-19 parkinsonism, this paper reviewed the neurological symptoms and related findings of COVID-19 and 2 other infectious diseases (influenza and prion disease) that are related to PD, parkinsonism or neurodegenerative movement disorder. In addition, since SARS-CoV-2 infection increases the risk of AD and PD neurodegeneration (8–12, 24), neurodegenerative disorders such as AD, PD and ALS were covered in this paper, which like COVID-19, also cause severe patient sufferings, numerous life loss, high healthcare cost and heavy social burden. Further, this paper discussed the potential mechanisms underlying the neurological symptoms of these diseases and the relationship between the infectious diseases and the neurodegenerative disorders as well as their therapeutic and preventive implications in the currently incurable neurodegenerative disorders.
Neurological symptoms and related findings of the infectious diseases and the neurodegenerative disorders
The main neurological symptoms of the infectious diseases (COVID-19, influenza, and prion disease) and the neurodegenerative disorders (AD, PD, and ALS) and possible causes of these diseases are summarized in Table 1. For COVID-19 and PD, their main neurological symptoms and related findings are highlighted in this section.
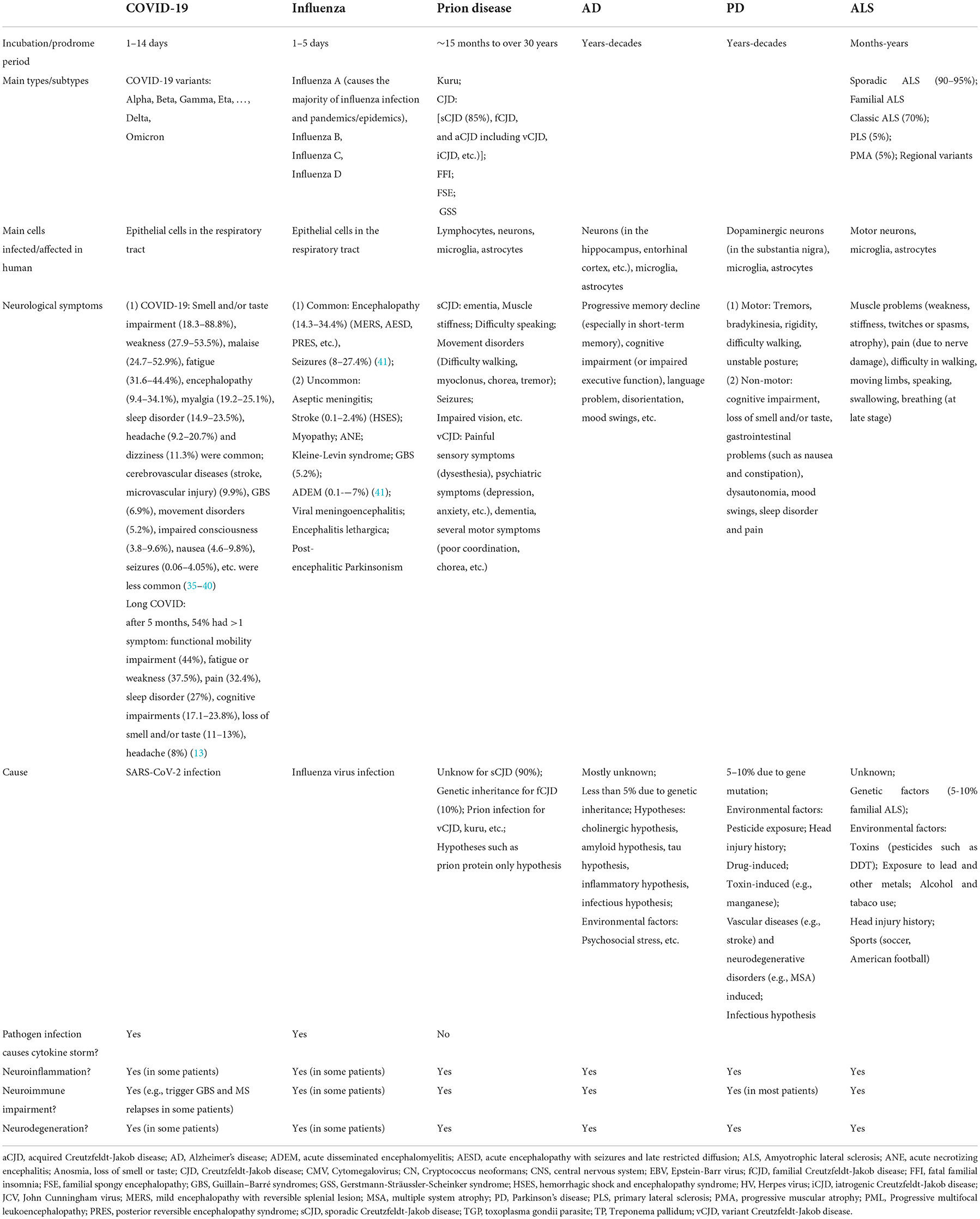
Table 1. Main features (including neurological symptoms) of infectious diseases (COVID-19, influenza, and prion disease), and neurodegenerative disorders (Alzheimer's Disease, Parkinson's Disease, and Amyotrophic Lateral Sclerosis).
COVID-19
Meta-analyses showed that smell impairment (18.3–85.6%), taste impairment (19.6–88.8%), weakness (27.9–53.5%), malaise (24.7–52.9%), fatigue (31.6–44.4%), encephalopathy (9.4–34.1%), myalgia (19.2–25.1%), sleep disorder (14.9–23.5%), headache (9.2–20.7%), and dizziness (11.3%) were common among COVID-19 patients, while cerebrovascular diseases (ischemic or hemorrhagic stroke and microvascular injury) (9.9%), Guillain–Barré syndromes (GBS) (6.9%), movement disorders (5.2%), impaired consciousness (3.8–9.6%), nausea (4.6–9.8%), and seizures (0.06–4.05%) were less common (36–40). The prevalence of neurological symptoms is much higher in hospitalized patients with COVID-19: acute encephalopathy (49%), coma (17%), etc. (2). The estimated incidences in COVID-19 patients at the intensive care unit (ICU) were 9.58% for stroke, 4.24% for nerve (or nerve root) disorders, 3.35% for myoneural (or muscle) disease, 1.74% for dementia, and 0.26% for parkinsonism (1).
In addition, around 31–69% of patients with COVID-19 suffer from long COVID (13), and common post-acute COVID-19 symptoms in long COVID include fatigue, loss of concentration or memory, headache, dizziness, anosmia and gastrointestinal distress (42). A meta-analysis found that after 5 months, 54% of COVID-19 survivors experienced at least one post-acute symptom such as functional mobility impairment, fatigue or muscle weakness, pain, sleep disorder and cognitive impairment (Table 1) (13). Another study further indicated that after 6 months, a number of symptoms remained: fatigue, malaise, cognitive dysfunction, sensorimotor symptoms (including tremors and internal vibrations), headaches, memory problems, muscle (or joint) pain, sleep problems, and gastrointestinal disorders (including nausea and constipation) (34). Moreover, ~30% of patients had persistent symptoms 9 months after the onset of COVID-19 (43).
Further, imaging, lab, and pathological findings confirmed the neurological damage in the nervous system caused by SARS-CoV-2 infection. Imaging studies showed common abnormalities such as acute ischemic stroke lesions, white matter lesions, and hyperintensity and/or microhemorrhages in the olfactory bulb on magnetic resonant imaging (MRI) (44). A meta-analysis revealed that olfactory bulb abnormalities (23.1%) were the most common neuroimaging abnormality in COVID-19 patients, followed by white matter abnormality (17.6%), acute/subacute ischemic infarction (16%), and encephalopathy (13%) (45), while microhemorrhages, acute intracranial hemorrhage, and encephalitis were less common (46). Particularly, microvascular injury was observed in the olfactory bulb, brain stem and basal ganglia (including substantia nigra) in COVID-19 patients (47). In addition, the presence of SARS-CoV-2 was detected in the CSF of 6% patients who had acute neurological symptoms (48), in the brains (frontal lobe, basal ganglia, brain stem, cerebellum, etc.) of 21 (53%) deceased patients (49), and in the substantia nigra of 6 patients (24). Apart from the presence of SARS-CoV-2, post-mortem studies reported inflammation (35.6%), gliosis with diffuse activation of microglia and astrocytes (35.6%), arteriosclerosis (29.5%), hypoxic-ischemic injury (28.1%), diffuse edema (17.1%), intracranial bleed (subarachnoid hemorrhage and punctate hemorrhages) (12.4%) and infarctions (2.7%) in the brains of COVID-19 patients (7) (Table 2).
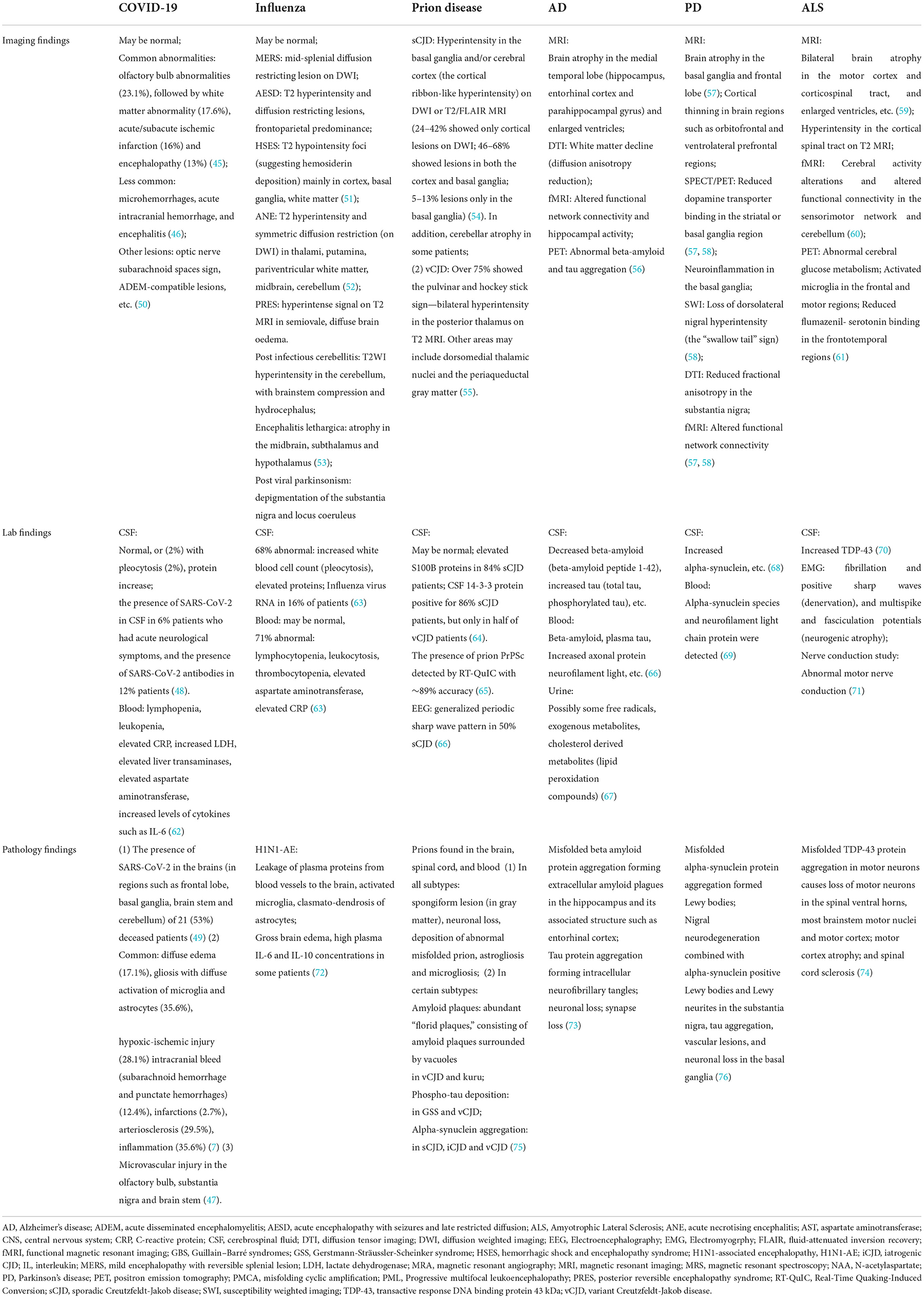
Table 2. Imaging, lab and pathology findings of neurological injury in the infectious diseases (COVID-19, influenza and prion disease) and the neurodegenerative disorders (Alzheimer's Disease, Parkinson's Disease, and Amyotrophic Lateral Sclerosis).
Parkinson's disease
The symptoms of PD are 2-fold: (1) Motor symptoms such as tremors, bradykinesia, rigidity, difficulty walking, and unstable posture; (2) Non-motor symptoms such as cognitive impairment, loss of smell and/or taste, gastrointestinal problems (such as nausea and constipation), dysautonomia, mood swings, rapid eye movement (REM) sleep behavior disorder and pain. Some non-motor symptoms such as hyposmia, constipation and REM sleep behavior disorder often precede motor-symptoms. In addition, cognitive problems (such as dementia and language problems), depression and apathy become common at the late stage of the disease (Table 1).
Imaging showed a loss of hyperintensity in the bilateral substantia nigra, and atrophy in the basal ganglia and frontal lobe on MRI in advanced PD (58), a loss of dorsolateral substantia nigral hyperintensity (the “swallow tail” sign) on susceptibility-weighted imaging (SWI), and reduced dopamine transporter binding in the basal ganglia on dopamine transporter scan imaging SPECT (single-photon emission computed tomography) or PET (position emission tomography) (57, 58). Increased alpha-synuclein was detected in the CSF of patients with PD (68), and alpha-synuclein species and neurofilament light chain protein were detected in the blood of PD patients (69). Autopsy of deceased PD patients revealed neurodegeneration combined with alpha-synuclein positive Lewy bodies and Lewy neurites in the substantia nigra, tau aggregation, vascular lesions, and neuronal loss in the basal ganglia (76) (Table 2).
COVID-19-associated acute and chronic PD (or parkinsonism)
The case reports that patients developed COVID-19-associated acute parkinsonism after contracting SARS-CoV-2 are summarized in Table 3. Clinical evidence suggested that SARS-CoV-2 infection was primarily responsible for such sudden parkinsonism (especially in young or middle-aged patients who had no prodromal PD symptoms) 16–22], but could not rule out the possibility that SARS-CoV-2 infection unmasked pre-symptomatic PD (21). Table 3 shows that (1) COVID-19-associated acute parkinsonism occurred in young, middle-aged and old patients 3–50 days after the onset of their mild, moderate or severe SARS-CoV-2 infection; (2) ~89% (8 of 9) patients did not have prodromal PD symptoms; (3) Among those whose dopaminergic imaging was available, 100% (5 of 5) patients had reduced dopaminergic uptake in the striatum; (4) ~63% (5 of 8) patients responded to dopaminergic medications and had improved motor symptoms after taking levodopa or dopamine agonist; (5) ~22 % (2 of 9) patients' motor symptoms improved spontaneously after either non-treatment (16) or convalescent plasma treatment (19) for SARS-CoV-2 infection. The possibility of SARS-CoV-2 infection unmasking prodromal (or presymptomatic) PD may exist in old patients who developed COVID-19 related acute parkinsonism (or PD) and had prodromal symptoms [e.g., constipation in the Baylor case (21)], but less likely in young or middle-aged patients who had no prodromal symptoms, and unlikely in the 2 cases where patients' motor symptoms improved spontaneously without any dopaminergic medication treatment (16, 19). Further research is needed to clarify the link between SARS-CoV-2 infection and PD.
In addition to COVID-19-associated acute parkinsonism, COVID-19 survivors developed post-COVID chronic parkinsonism with an estimated incidence of 0.11% (1), and Table 4 provides a summary of the incidence and case number details. PD motor symptoms such as tremors (10.8%) and rigidity (5.38%) were reported in COVID-19 patients with motor symptoms (77). In long COVID, a meta-analysis found that 44% of COVID-19 survivors experienced functional mobility impairment 5 months after the disease onset (13). Moreover, sensorimotor symptoms (including tremors and internal vibrations) were prevalent among COVID-19 patients 7 months after the onset of the sickness, and there was an increasing trend of tremors 6–7 months after COVID-19 (34).
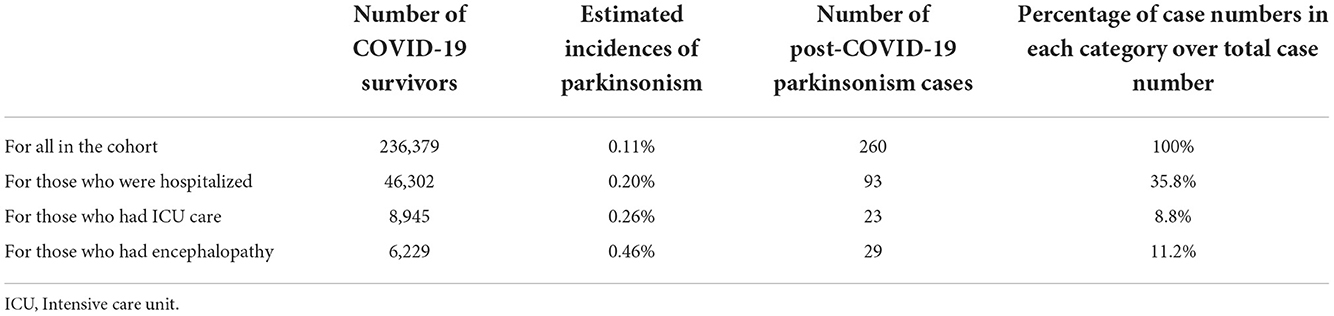
Table 4. Summary of the estimated incidences and numbers of post-COVID-19 parkinsonism reported in Taquet et al. (1).
Further, smell impairment (olfactory dysfunction) is an early non-motor symptom of PD, while taste impairment can appear in early PD but more frequently in advanced PD (78). In addition, cognitive impairment (including memory problems), gastrointestinal problems and sleep disorders are common non-motor symptoms of PD. Since SARS-CoV-2 infection induces neuroinflammation and PD neurodegeneration and increases individual vulnerability to PD or parkinsonism (1, 7, 26, 47), the high prevalence of olfactory dysfunction and taste disorder in COVID-19 patients and the frequently reported cognitive impairment, functional mobility impairment, tremors, internal vibrations, gastrointestinal problems and sleep disorder in long COVID (13, 34, 35) suggest possible future development of PD or parkinsonism in patients with long COVID.
The relatively low incidence of parkinsonism in COVID-19 patients or survivors (1) may be because the nigrostriatal damage caused by SARS-CoV-2 infection (77) in some patients (especially those with long COVID) or COVID-19 survivors was not severe enough to manifest PD or parkinsonism symptoms (i.e., they were at the prodromal or presymptomatic stage of PD or parkinsonism) and thus they remained undiagnosed/unreported (13, 28, 35). Therefore, long-term following up on COVID-19 patients who have non-motor and/or motor symptoms of PD (or parkinsonism) will help reveal the true incidence of PD (or parkinsonism) in patients with long COVID (1, 27, 28, 77).
Mechanisms of neurological injury in the infectious diseases and neurodegenerative disorders and treatments of these diseases
Mechanisms of neurological injuries in the diseases
The mechanisms of neurological injury caused by infectious diseases (COVID-19, influenza, and prion disease), and the neurodegenerative disorders are summarized in Table 5. For COVID-19 and PD, the mechanisms underlying their neurological symptoms are highlighted in this section.
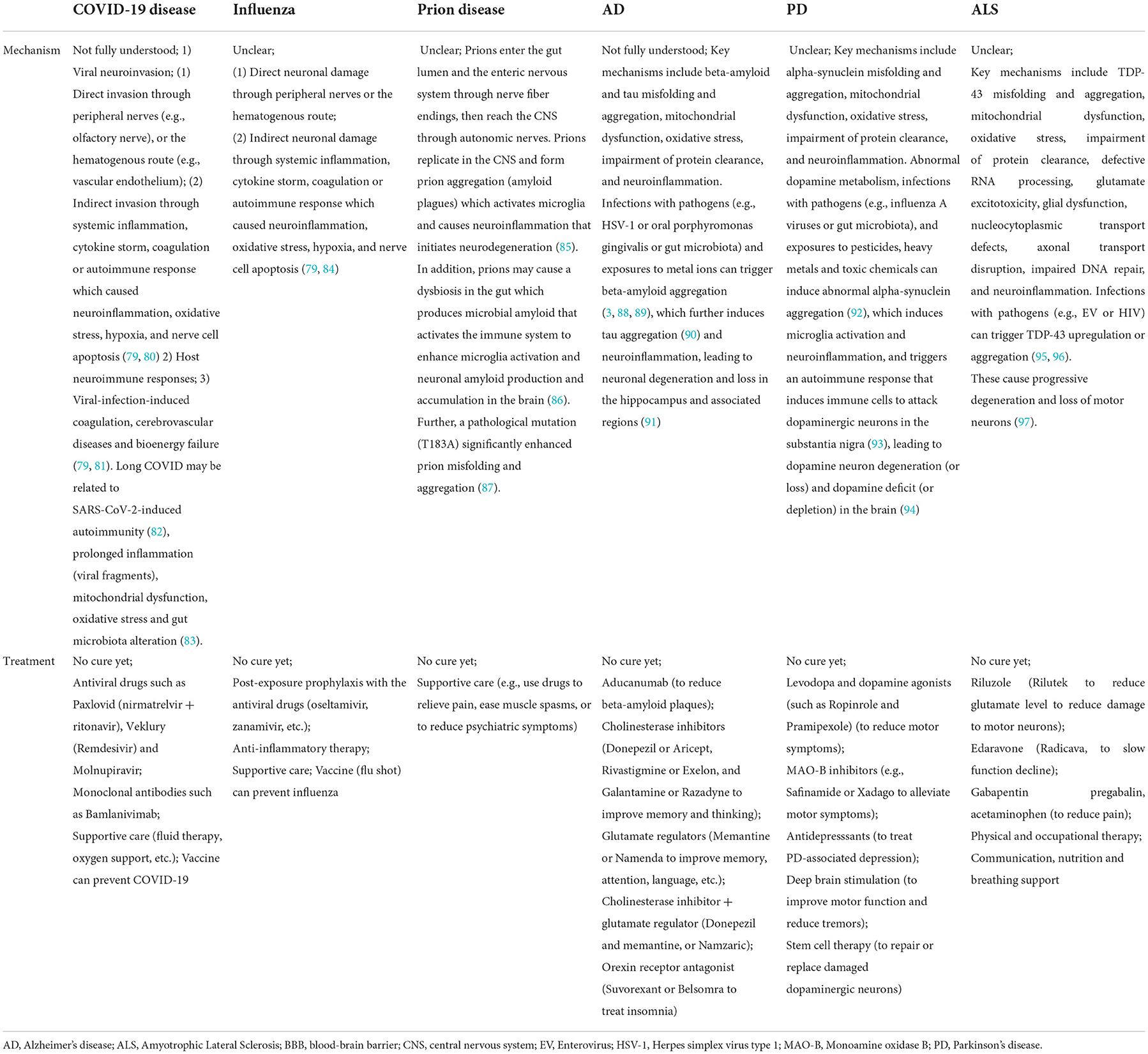
Table 5. Mechanisms and treatments of neorlogical injury in the infectious diseases (COVID-19, influenza and prion disease), and the neurodegenerative disorders (Alzheimer's Disease, Parkinson's Disease, and Amyotrophic Lateral Sclerosis).
In general, there are several ways that pathogens in infectious diseases can cause neuronal damage which leads to nervous system impairment and neurological symptoms. (1) Direct invasion which can lead to neuroinflammation, encephalitis, meningitis and myelitis; (2) Indirect invasion or para-infectious complications such as sepsis, metabolic dysfunction and coagulopathy, which can lead to encephalopathy, seizures, vasculitis and stroke; (3) Infection-triggered immune-mediated attacks in the nervous system which can lead to acute disseminated encephalomyelitis (ADEM), acute motor axonal neuropathy, acute inflammatory demyelinating polyneuropathy, and Guillain–Barré syndromes (GBS); (4) Persistent or latent infection (due to, e.g., viral mutation) and/or interplay with other pathogens, which can lead to potential late reactivation, neurodegeneration, neurocognitive disorders and subacute sclerosing encephalitis (79).
In COVID-19, the mechanisms of the neurological symptoms have not been fully established and more research is needed to unfold them. Potential mechanisms include viral neuroinvasion, host neuroimmune responses, viral-infection-induced coagulopathy, cerebrovascular diseases (such as microvascular injury, vasculitis, and ischemic or hemorrhagic stroke), and bioenergy failure (30, 79–81). There are mainly 2 ways of SARS-CoV-2 neuroinvasion that causes damage to the human nervous system: (1) Direct neuronal damage that SARS-CoV-2 virus may travel to the brain through peripheral nerves such as the olfactory nerve and the vagus nerve, or through the hematogenous route to cross the blood-brain-barrier (BBB) (80, 98). (2) Indirect neuronal damage through systemic inflammation or cytokine storm or autoimmune response triggered by a viral infection, which caused metabolic dysfunction, oxidative stress, hypoxia, neuroinflammation and neuronal death (79, 80, 99, 100). As to long COVID, potential mechanisms may include SARS-CoV-2-induced autoimmunity (82), prolonged inflammation (due to unresolved viral fragments and delayed viral clearance), mitochondrial dysfunction, oxidative stress and gut microbiota alteration (83). In addition, it has been found that factors such as SARS-CoV-2 RNA level in the blood, the presence of autoantibodies and reactivation of the Epstein-Barr virus (EBV) increase the risk for long COVID (101).
The mechanisms of PD have not been fully understood, but key molecular mechanisms include alpha-synuclein misfolding and aggregation, mitochondrial dysfunction, oxidative stress, impairment of protein clearance, and neuroinflammation (94). Excessive oxidation of dopamine can induce mitochondrial dysfunction and oxidative stress, which leads to misfolded alpha-synuclein aggregation (102). In addition, infections with pathogens (e.g., influenza A viruses or gut bacteria), and exposures to pesticides, heavy metals, and chemicals such as 1-methy1-4-phenyl-1,2,3,6-tetrahydropyridine (MPTP) can also cause abnormal alpha-synuclein aggregation (5, 92). which activates microglia and may trigger an autoimmune response that induces immune cells to attack dopaminergic neurons in the substantia nigra (93). Alpha-synuclein pathology and microglial activation, along with other abnormalities such as impairment of protein degradation and clearance, mitochondrial dysfunction, oxidative stress and glutaminergic excitotoxicity, lead to neuroinflammation in the substantia nigra. Neuroinflammation initiates neurodegeneration, which further causes neuronal dysfunction and death of dopamine neurons in the substantia nigra, resulting in dopamine deficit (or depletion) and motor symptoms in PD patients (94). Further, alpha-synuclein aggregates in the olfactory system and the enteric nervous system propagate to the brain through the olfactory nerve and the vagus nerve in a prion-like manner, while short-chain fatty acids and bacteria metabolites (produced by gut microbiota) may reach the brain through the endocrine route to induce alpha-synuclein aggregation and microglia activation in the brain (92, 103). Based on pathological findings, the Braak hypothesis (104, 105) and prion-like mechanisms in PD have been proposed (106), and they are discussed in the Discussion section.
During the COVID-19 pandemic, it has been found that SARS-CoV-2 infection increases individual susceptibility to PD or parkinsonism (9, 24), and the potential mechanism is illustrated in Figure 1. Briefly, SARS-CoV-2 infection can cause blood-brain-barrier (BBB) disruption, cerebrovascular damage, neuronal lysis, neuroinflammation, etc. (via the hematogenous route), and hyposmia or anosmia (via the olfactory route) (80, 98). SARS-CoV-2 infection triggers a cytokine storm that induces oxidative stress and neuroinflammation, which further initiated neurodegeneration (80, 99, 100). At the cellular level, SARS-CoV-2 infection causes bioenergetic stress, alpha-synuclein upregulation, antiviral alpha-synuclein accumulation, and proteostasis impairment in the infected dopaminergic neurons in the substantia nigra, which induces mitochondrial dysfunction, oxidative stress and hypoxia (24, 107–111). In addition, SARS-CoV-2 infection triggers microvascular injury (such as perivascular activated microglia, astrocytosis, and CD8+ T cell infiltration) in the basal ganglia and brain stem (47, 49) and (activated microglia and neuronophagia) in the substantia nigra (47) which results in hypoperfusion, neuroinflammation and subsequent neurodegeneration. These damages to the nervous system induce alpha-synuclein (or Lewy-body-like) pathology (110, 112), trigger neuroinflammation and increase the dopaminergic neurons' vulnerability to neuroinflammation, which leads to dopaminergic neuron senescence and neurodegeneration (9, 24, 108). SARS-CoV-2 nucleocapsid protein accelerated alpha-synuclein aggregation, which induced neuroinflammation and subsequent neurodegeneration, and increased cell death (9, 23). At the neuroimmune level, SARS-CoV-2 infection can activate microglia by causing alpha-synuclein pathology, trigger neuroinflammation through immune mediation, and induce neurodegeneration and dopaminergic neuron loss in the substantia nigra, which increases individual susceptibility to PD or parkinsonism, leading to acute or chronic parkinsonism (1, 9, 26, 31). Further, aging, infections with other pathogens (e.g., flu virus), genetic factors and/or environmental factors (such as toxic chemicals and metals) can synergize to worsen nigral neurodegeneration and dopaminergic neuronal loss, and induce or exacerbate PD or parkinsonism (25, 32, 113).
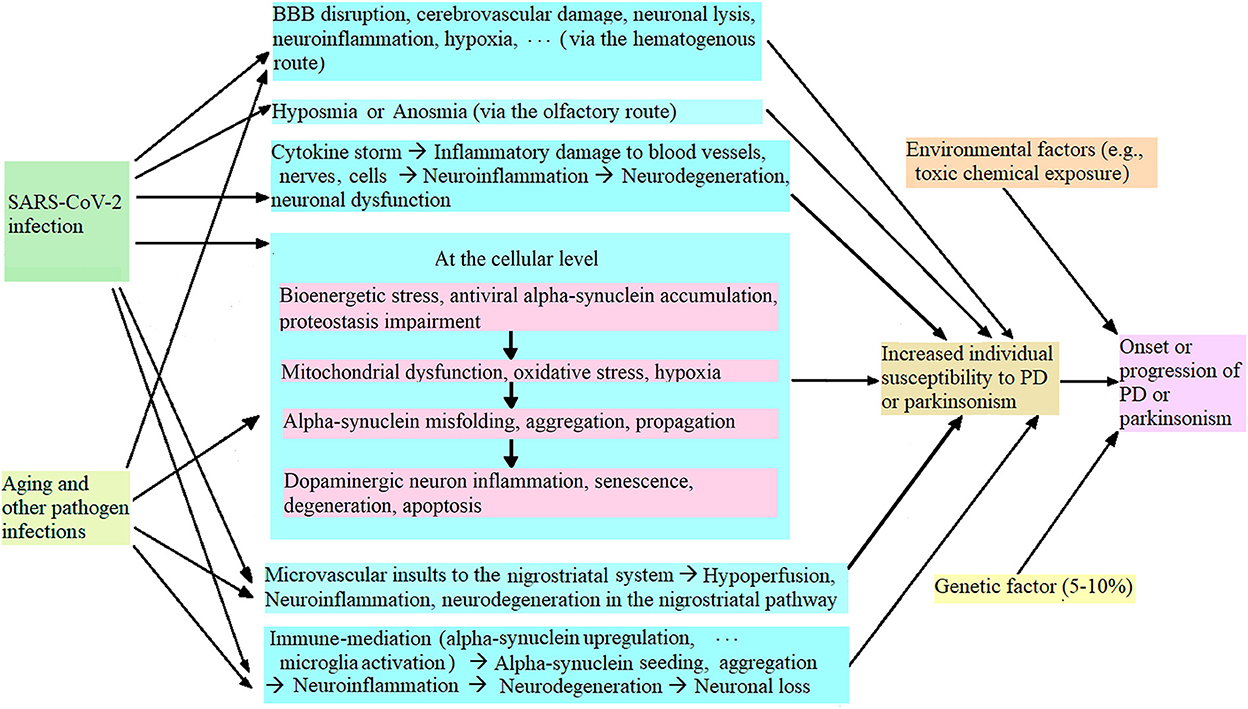
Figure 1. Potential mechanism of SARS-CoV-2 infection leading to increased individual vulnerability to PD or parkinsonism.
Bioinformatics analysis has confirmed that SARS-CoV-2 (spike and nucleocapsid) proteins have a favorable binding affinity to alpha-synuclein, and a cell line study has verified that SARS-CoV-2 proteins upregulate alpha-synuclein expression and induce alpha-synuclein aggregation and Lewy-body-like pathology (110). Therefore, SARS-CoV-2 infection could change normal alpha-synuclein proteins and turn them into pathological (misfolded, aggregated) alpha-synuclein that seeding and spreading like prions. This is further discussed in the Discussion section.
Treatments of the diseases
Currently, there is no cure for the six diseases covered in this paper (infectious diseases: COVID-19, influenza and prion diseases; and neurodegenerative disorders: AD, PD and ALS), but vaccines for COVID-19 and influenza are available. Table 5 outlines the treatments and prevention methods of these diseases. Treatments for these incurable diseases are mainly medications (to manage symptoms or slow down disease progression) and supportive care. For example, in COVID-19, new antiviral drugs (such as Paxlovid), monoclonal antibodies (such as Bamlanivimab), and supportive care have been used to treat COVID-19 symptoms, relieve patients from suffering, and save their lives; while in PD, medications such as levodopa can reduce motor symptoms, and surgical procedures (e.g., deep brain stimulation) are effective in reducing tremors and improving motor function. Further, new therapies such as stem cell therapy are promising in repairing or replacing damaged dopaminergic neurons in PD (114).
Discussion
Infectious diseases such as COVID-19 and influenza are very different from neurodegenerative disorders such as AD, PD and ALS, but some common neurological symptoms (e.g., viral infection with SARS-CoV-2 or influenza A viruses can induce symptoms of AD, PD, or ALS) bring the two groups of diseases together. Examining the common neurological symptoms and their mechanisms reveals interconnections between the two groups of diseases.
The relationship between infectious diseases and neurodegenerative disorders
Since pathological proteins such as beta-amyloid, tau, alpha-synuclein and transactive response DNA binding protein 43 (TDP-43) in AD, PD or ALS act like prions in misfolding, aggregating, seeding, and spreading in the brain, these neurodegenerative disorders have been called prion-like diseases (115–117). In addition, prion diseases (such as Kuru and Creutzfeldt-Jakob disease) are both infectious diseases and neurodegenerative disorders. Thus, prion diseases and prions are the links between infectious diseases and neurodegenerative disorders.
Traditionally, pathological hallmarks of AD, PD, and ALS such as senile plaques (beta-amyloid aggregates), neurofibrillary tangles (tau aggregates), Lewy bodies (alpha-synuclein aggregates), and Bunina bodies (TDP-43 aggregates) were considered as non-transmittable. Recent research findings have changed the traditional opinion (115, 118–121). The transmissibility of pathological proteins such as alpha-synuclein, beta-amyloid, tau and TDP-43 has been reported, and these proteins can be transmitted between cells in the central nervous system (CNS), propagate across species (e.g., transmitted PD-associated α-synuclein seeds could propagate in new mammal host) (119), and even spread between individuals (e.g., AD-associated pathological beta-amyloid) (122), like prions in prion diseases (122–126).
Further, recent findings indicated that proteins such as beta-amyloid and alpha-synuclein are antimicrobial peptides protecting neurons against microbes (bacteria, fungi, viruses, etc.) (127), restricting viral infections in the brain (128), and acting like chemoattractants to immune cells (e.g., monocytes) (129). In addition, hyperphosphorylation of certain residue of tau can protect against beta-amyloid-induced excitotoxicity (e.g., due to antiviral beta-amyloid accumulation) and spine loss (130), while TDP-43 has a positive role in antiviral response (131) and infection with enterovirus (EV) or HIV can induce TDP-43 upregulation or aggregation (96, 132). These findings suggest that pathogen infection can trigger the upregulation, accumulation, misfolding and aggregation of the antimicrobial proteins such as beta-amyloid, alpha-synuclein and TDP-43, which consequently activates microglia in the brain. Namely, pathogen infections can induce the accumulation of the antimicrobial proteins and initiate the formation of “prions” that turns these normal proteins into pathological prion-like proteins, which further evokes neuroinflammation and subsequent neurodegeneration in the neurodegenerative disorders.
Compelling evidence has shown that in AD, infections with pathogens such as herpes simplex virus 1, oral porphyromonas gingivalis, and abnormal gut microbiota can induce anti-pathogen accumulation of beta-amyloid, cause beta-amyloid to misfold and aggregate which triggers tau aggregation (90), and thus these pathogen infections evoke the formation of “prions” that conforms normal beta-amyloid and tau proteins to pathological prion-like beta-amyloid and tau proteins (3, 88, 89). In PD, infections with pathogens such as influenza A virus, SARS-CoV-2, West Nile virus, and gut bacteria (e.g., Desulfovibrio bacteria) can trigger an anti-pathogen accumulation of alpha-synuclein and alpha-synuclein pathology (misfolding, aggregation, etc.), and evoke “prion” formation that conforms normal alpha-synuclein proteins to pathological prion-like alpha-synuclein proteins (5, 23, 92, 109, 111, 133, 134). In ALS, infections with pathogens such as EV and HIV can induce TDP-43 upregulation, misfolding and aggregation, and turn normal TDP-43 into pathological prion-like TDP-43 proteins (6, 95, 96, 132) and infection with gut microbiota could modulate ALS (135). Further, in prion diseases, infection with prions (PrPSc) may cause a gut dysbiosis which produces microbial amyloid that activates the immune system, triggers misfolding and aggregation of prion protein PrPC in the brain, conforms normal PrPC to pathological prions (PrPSc), activates microglia, and enhances neuronal amyloid production and accumulation (136).
Interestingly, gut microbiota abnormality and gut-brain axis alterations have been implicated in the pathogenesis of neurodegenerative disorders such as AD, PD, ALS, and prion diseases (88, 92, 135, 136). In addition, a pathogen-infection-evoked autoimmune response has been found in AD, PD, ALS, and prion diseases that cause the immune system to treat pathological prions (or “prions”) as pathogens and attack neurons that have the presence or aggregation of these pathogens (137–140). Therefore, the pathogenesis of neurodegenerative disorders may be a 2-step relay process: First, one or multiple pathogen infections trigger an anti-pathogen accumulation of antimicrobial proteins (beta-amyloid, alpha-synuclein, TDP-43, etc.) which evokes the formation of pathological prions (or prion-like proteins), and induces neuroinflammation; Second, the pathological prions or prion-like proteins keep seeding, spreading and conforming (normal proteins to prions or prion-like proteins), which triggers persistent neuroinflammation and subsequent neurodegeneration in these neurodegenerative disorders.
The unknown pathogen in the Braak hypothesis in sporadic PD
Braak et al. have hypothesized that sporadic PD is caused by an unknown pathogen that enters the body via the respiratory route, is swallowed subsequently, and reaches the gut, initiating Lewy pathology in the olfactory and the digestive tracts (104). Further, Braak staging has been developed for AD (141, 142) and PD (105).
Over the years, mounting evidence from clinical research has supported the Braak hypothesis (143). In AD, it has been found that tau-PET and Braak staging for AD are prognostic markers of cognitive decline in patients with mild cognitive impairment or dementia including AD (144). In PD, Lewy bodies have been found in the neurons of the olfactory tract and the enteric nervous system in patients with PD (which leads to olfactory/smell impairment and digestive/gastrointestinal disorder at the early stage of PD), and the presence of Lewy bodies in the olfactory tract and the enteric nervous system precedes the onset of PD motor symptoms (145, 146).
The Braak hypothesis has also been supported by animal studies with influenza viruses (5, 113). Jang et al. showed that (1) the influenza A virus H5N1 traveled to the brain (of mice) through the vagus nerve, induced alpha-synuclein phosphorylation and aggregation, and persistent microglial activation (neuroinflammation), which triggered neurodegeneration, dopaminergic neuronal loss in the substantia nigra and parkinsonism, and (2) the spread of the influenza virus in the brain was similar to the alpha-synuclein progression pattern described in the Braak staging in sporadic PD (5). Influenza A virus H1N1 also infected dopaminergic neurons and its replication in neurons can induce seeding of aggregated alpha-synuclein, leading to alpha-synuclein aggregates and synucleinopathies (147). In addition, influenza A virus H1N1 activated the immune system that further triggered neuroinflammation, and thus infection with influenza A virus H1N1 made dopaminergic neurons susceptible to toxic chemicals such as MPTP (113). Therefore, multiple exposures to influenza A viruses (H1N1, H5N1, etc.), other pathogens (SARS-CoV-2, gut microbes, etc.) and toxic chemicals over years or decades can synergize to induce PD (113).
The unknown pathogen that causes sporadic PD in the Braak hypothesis is not one pathogen, but a relay of pathogens such as microbes (influenza A viruses, SARS-CoV-2, Desulfovibrio bacteria in gut microbiota, etc.) and prion-like alpha-synuclein proteins. Like a relay, infections with the microbes initiate the formation of alpha-synuclein “prions” which keep seeding, spreading and conforming (normal alpha-synuclein proteins to pathological prion-like alpha-synuclein proteins), inducing neuroinflammation and subsequent neurodegeneration, and causing damage and neuronal loss to dopaminergic neurons in the substantia nigra in sporadic PD.
Therapeutic and preventive implications in the incurable neurodegenerative disorders
Since infections with a relay of pathogens (microbes and prions or prion-like proteins) over time (months, years or decades) can synergize to induce devastating neurodegenerative disorders such as sporadic PD and AD, a new direction has emerged for the treatment and prevention of these neurodegenerative diseases, i.e., treating or preventing these diseases by treating or preventing the infections caused by the pathogens. This insight has brought new light and hopes to the field, which has suggested that neurodegenerative disorders may be curable using a systematic approach that treatments targeting a relay of pathogens including microbes and prions or prion-like proteins need to work together in a systematic, orderly and harmonious way in order to provide effective cures for these disorders. Thus, the treatment or prevention of these diseases may be two-fold.
First, treat or prevent the infections and replications of the microbes in the human body to remove the source of “prion” production in these neurodegenerative disorders.
In AD, a recent study showed that antiviral treatment (such as acyclovir and valaciclovir) for infection of herpes viruses such as HSV-1 was associated with a lower long-term risk of dementia (including AD), while untreated herpes infection was related to a higher risk (148). A clinical trial is also investigating whether the antiviral drug valaciclovir can slow the progression of AD in patients with HSV-1 infection (149). In addition, recent research has found that oral porphyromonas gingivalis infection secreted toxic protease gingipains and increased beta-amyloid production, and gingipains inhibitor reduced the infection, beta-amyloid aggregation and neuroinflammation (89). Further, vaccines against these microbes (e.g., HSV-1) are promising to prevent AD induced by such microbe infections.
In PD, it has been reported that treating or preventing infections caused by microbes such as influenza A viruses, SARS-CoV-2, and gut microbiota can protect dopaminergic neurons or reduce the risk of PD induced by such infections (86, 113, 150). Research showed that influenza vaccine (flu shot) or anti-viral medication treatment could protect against dopaminergic neuronal loss in the substantia nigra (caused by influenza A virus infection or MPTP toxicity) (113). Similarly, since COVID-19 vaccines protect against SARS-CoV-2 infection, they may help prevent COVID-19-associated parkinsonism by preventing COVID-19 or SARS-CoV-2 infection. Further, treatment for COVID-19 varied in patients with COVID-19-associated acute parkinsonism: one patient had spontaneously improved motor symptoms after treatment with convalescent plasma for SARS-CoV-2 infection (19), while another patient (who did not respond to dopaminergic medication) achieved significant spontaneous improvement in motor symptoms without any specific treatment, suggesting that treatment for SARS-CoV-2 infection in this case came from the immune system of the patient (16).
In addition, research has shown that since the introduction of highly active antiretroviral therapy (HAART), the overall prevalence of neurological symptoms including HIV-associated dementia dropped by over 60% (151, 152). Antiretroviral therapy also reversed HIV-induced parkinsonism (153) and HIV-induced ALS (6). Similarly, antiviral drug ribavirin could reverse EV-infection-induced ALS symptoms in mice (132).
Second, treat or prevent propagation of prions or prion-like proteins by reducing or removing prions or prion-like proteins and mediators of neuroinflammation and neurodegeneration in the neurodegenerative disorders, and providing neuroprotection and immuno-modulation to prevent or reverse the neurodegeneration process and restore normal protein homeostasis.
Immunotherapy has been introduced to neurodegenerative disorders in recent years. It uses either passive immunotherapy monoclonal antibodies, or active immunotherapy therapeutic vaccines (that induce adaptive immune responses to produce antibodies) to reduce or remove prions or prion-like proteins and/or mediators of neuroinflammation and neurodegeneration in the neurodegenerative disorders in order to manage or prevent the diseases.
In AD, a beta-amyloid immunotherapy Aducanumab or Aduhelm (an anti-beta-amyloid human antibody) that can reduce beta-amyloid plaques has been approved by the FDA, and other similar therapeutics such as gantenerumab are in clinical trials (154). In addition, therapeutic beta-amyloid vaccines have been developed to train the immune system to produce beta-amyloid antibodies, and beta-amyloid vaccines (such as ABvac 40 by Araclon Biotech) have entered clinical trials (154). As to tau, clinical trials of tau immunotherapies that use tau-targeted antibodies (such as semorinemab) to reduce phosphorylated tau levels in AD have started, and tau vaccines such as ACI-35 (by AC Immune) that stimulate the body to develop tau antibodies have also entered clinical trials (154, 155). Currently, the clinical efficacy of beta-amyloid or tau immunotherapies in restoring cognition (e.g., memory improvement) in AD patients is limited. Rather than target pathological beta-amyloid or tau alone, another strategy is to address the pathological imbalance in pathways that created the abnormal beta-amyloid and tau aggregation and modulate the immune system to reverse the AD neurodegeneration process and restore normal protein homeostasis. AD immune system-modulating vaccines or medications such as GV1001 (by GemVax and KAEL Bio) can stimulate the immune system to clear beta-amyloid plaques and tau tangles, protect neurons, and improve cognition in AD, which are being studied in clinical trials (154).
In PD, ENT-01 (by Enterin Inc.) reduces alpha-synuclein accumulation in the neurons in the gut, restores enteric neuron functions, and repairs the dysfunctional gut-brain axis in PD, and a clinical trial was completed in 2018 which treated constipation in over 80% patients, and revealed the reversal of a neurodegenerative process in PD patients for their symptoms (156). In addition, alpha-synuclein immunotherapy using alpha-synuclein antibodies (such as BIIB054) has entered clinical trials, and alpha-synuclein vaccines (that prompt the body to produce alpha-synuclein antibodies) such as PD01A (by AFFiRiS), ACI-7104 (by AC Immune), and UB312 (by Vaxxinity) are being studied in clinical trials (157, 158).
In ALS, a recent study found that thioridazine enhanced the clearance of TDP-43 aggregates which recovered TDP-43 functionality (159). In addition, targeting inflammatory or neurodegenerative mediators, immunotherapy using drugs such as granulocyte-macrophage colony-stimulating factor (GM-CSF) is promising to reduce TDP-43-induced neuroinflammation and motor neuron death, which may slow ALS progression and enhance patient survival (160).
Conclusion
In summary, the neurological symptoms of the infectious diseases (COVID-19, influenza and prion disease) and the neurodegenerative disorders (AD, PD, and ALS), the potential mechanisms underlying these symptoms, and the relationship between the two groups of diseases shed light on the treatment and prevention of the currently incurable neurodegenerative disorders. For example, SARS-CoV-2 infection can increase individual vulnerability to PD or parkinsonism, and infections with a relay of microbes (influenza A viruses, SARS-CoV-2, gut microbiota, etc.) and prion-like alpha-synuclein proteins over time can synergize to induce PD or parkinsonism. Therefore, a systematic approach that targets these pathogens and the pathogen-induced neuroinflammation and neurodegeneration may provide cures for the neurodegenerative disorders like PD. This approach includes: (1) treating or preventing the infections and replications of the microbes, and (2) treating or preventing propagation of prions or prion-like proteins as well as the neuroinflammation and subsequent neurodegeneration induced by the pathology of prions or prion-like proteins, and restoring normal protein homeostasis. Further, antiviral/antimicrobial medications, vaccines, immunotherapies and new therapies (such as stem cell therapy) need to work together to treat or prevent devastating neurodegenerative disorders. Effective immunotherapies for AD, PD, and ALS (including monoclonal antibodies and therapeutic vaccines for prion-like proteins such as beta-amyloid, tau, alpha-synuclein and TDP-43) are on the horizon. As medical science and technology advance rapidly, better COVID-19 vaccines for SARS-CoV-2 variants, effective antiviral or antimicrobial drugs, innovative immunotherapies (such as monoclonal antibodies for prion-like alpha-synuclein proteins and therapeutic vaccines for PD or parkinsonism), as well as new (or unconventional) therapies will be developed and made available in the near future, which will help prevent a possible epidemic or global spreading of post-COVID-19 parkinsonism in the 21st century.
Author contributions
JZ contributed to the research design, data collection and analysis, and drafting of the manuscript.
Acknowledgments
The author thanks Dr. David Holtzman and Dr. Joel Perlmutter for their inspiring work in Alzheimer's Disease and Parkinson's Disease research at the Washington University in St. Louis, and thanks Dr. Robert Paul for his inspiring work in NeuroHIV research at the University of Missouri in St. Louis.
Conflict of interest
The author declares that the research was conducted in the absence of any commercial or financial relationships that could be construed as a potential conflict of interest.
Publisher's note
All claims expressed in this article are solely those of the authors and do not necessarily represent those of their affiliated organizations, or those of the publisher, the editors and the reviewers. Any product that may be evaluated in this article, or claim that may be made by its manufacturer, is not guaranteed or endorsed by the publisher.
References
1. Taquet M, Geddes JR, Husain M, Luciano S, Harrison PJ. 6-month neurological and psychiatric outcomes in 236379 survivors of COVID-19: a retrospective cohort study using electronic health records. Lancet Psychiatry. (2021) 8:416–27. doi: 10.1016/S2215-0366(21)00084-5
2. Chou SHY, Beghi E, Helbok R, Moro E, Sampson J, Altamirano V, et al. Global incidence of neurological manifestations among patients hospitalized with COVID-19—a report for GCS-NeuroCOVID Consortium and the ENERGY Consortium. JAMA Netw Open. (2021) 4:e2112131. doi: 10.1001/jamanetworkopen.2021.12131
3. Zhou L, Miranda-Saksena M, Saksena NK. Viruses and neurodegeneration. Virol J. (2013) 10:172. doi: 10.1186/1743-422X-10-172
4. Itzhaki RF. Herpes simplex virus type 1 and Alzheimer's disease: increasing evidence for a major role of the virus. Front Aging Neurosci. (2014) 6:202. doi: 10.3389/fnagi.2014.00202
5. Jang H, Boltzc D, Sturm-Ramirezc K, Shepherda KR, Jiaoa Y, Websterc R, et al. Highly pathogenic H5N1 influenza virus can enter the central nervous system and induce neuroinflammation and neurodegeneration. Proc Natl Acad Sci USA. (2009) 106:14063–8. doi: 10.1073/pnas.0900096106
6. Bowen LN, Tyagi R, LiW, Alfahad T, Smith B, WrightM, et al. HIV-associated motor neuron disease: HERV-K activation and response to antiretroviral therapy. Neurology. (2016) 87:1756–62. doi: 10.1212/WNL.0000000000003258
7. Pajo AT, Espiritu AI, Apor ADAO, Jamora RDG. Neuropathological findings of patients with COVID-19: a systematic review. Neurol Sci. (2021) 43:1255–66. doi: 10.1007/s10072-021-05068-7
8. Zarifkar P, Peinkhofer C, BenrosME, Kondziella D. Frequency of neurological diseases after COVID-19, Influenza A/B and Bacterial Pneumonial. Front Neurol. (2022) 13:904796. doi: 10.3389/fneur.2022.904796
9. Smeyne RJ, Eells J, Chatterjee D, Byrne M, Akula SM, Sriramula S, et al. COVID-19 infection enhances susceptibility to oxidative stress-induced parkinsonism. Mov Disord. (2022) 37:1394–1404. doi: 10.1002/mds.29116
10. Li C, Liu J, Lin J, Shang H. COVID-19 and risk of neurodegenerative disorders: a Mendelian randomization study. Transl Psychiatry. (2022) 12:238. doi: 10.1038/s41398-022-02052-3
11. Krey L, Huber MK, Hoglinger GU, Wegner F. Can SARS-CoV-2 infection lead to neurodegeneration and Parkinson's disease? Brain Sci. (2021) 11:1654. doi: 10.3390/brainsci11121654
12. Ferini-Strambi L, Salsone M. COVID-19 and neurological disorders: are neurodegenerative or neuroimmunological diseases more vulnerable? J Neurol. (2021) 21:1–11. doi: 10.1007/s00415-020-10070-8
13. Groff D, Sun A, Ssentongo AE, Ba DM, Parsons N, Poudel GR, et al. Short-term and long-term rates of postacute sequelae of SARS-CoV-2 infection: a systematic review. JAMA Netw Open. (2021) 4:e2128568. doi: 10.1001/jamanetworkopen.2021.28568
14. Hayase Y, Tobita K. Influenza virus and neurological diseases. Psychiatry Clin Nerrrosci. (l997) 51:181–4. doi: 10.1111/j.1440-1819.1997.tb02580.x
15. Cocoros NM, Svensson E, Szepligeti SK, Vestergaard SV, Szentkuti P, Thomsen RW, et al. Long-term risk of Parkinson disease following influenza and other infections. JAMA Neurol. (2021) 78:1461–70. doi: 10.1001/jamaneurol.2021.3895
16. Mendez-Guerrero A, Laespada-Garcia MI, Gomez-Grande A, Ruiz-Ortiz M, Blanco-Palmero VA, Azcarate-Diaz FJ, et al. Acute hypokineticrigid syndrome following SARS-CoV-2 infection. Neurology. (2020) 95:e2109–118. doi: 10.1212/WNL.0000000000010282
17. Faber I, Brandao PRP, Menegatti F, de Carvalho Bispo DDD, Maluf FB, Cardoso F. Coronavirus disease 2019 and parkinsonism: a non-post-encephalitic case. Mov Disord. (2020) 35:1721–2. doi: 10.1002/mds.28277
18. Cohen ME, Eichel R, Steiner-Birmanns B, Janah A, Ioshpa M, Bar-Shalom R, et al. A case of probable Parkinson's disease after SARS-CoV-2 infection. Lancet Neurol. (2020) 19:804–5. doi: 10.1016/S1474-4422(20)30305-7
19. Akilli NB, Yosunkaya A. Part of the Covid-19 puzzle: acute parkinsonism. Am J Emerg Med. (2021) 47:333. e1–333.e3. doi: 10.1016/j.ajem.2021.02.050
20. Rao AR, Hidayathullah SM, Hegde K, Adhikaria P. Parkinsonism: an emerging post COVID sequelae. IDCases. (2022) 27:e01388. doi: 10.1016/j.idcr.2022.e01388
21. Makhoul K, Jankovic J. Parkinson's disease after COVID-19. J Neurol Sci. (2021) 422:117331. doi: 10.1016/j.jns.2021.117331
22. Morassi M, Palmerini F, Nici S, Magni E, Savelli G, Guerra UP, et al. SARS-CoV-2-related encephalitis with prominent parkinsonism: clinical and FDG-PET correlates in two patients. J Neurol. (2021) 268:3980–7. doi: 10.1007/s00415-021-10560-3
23. Semerdzhiev SA, Fakhree MAA, Segers-Nolten I, Blum C, Claessens MMAE. Interactions between SARS-CoV-2 N-Protein and alpha-Synuclein accelerate amyloid formation. ACS Chem Neurosci. (2022) 13:143–50. doi: 10.1021/acschemneuro.1c00666
24. Chen S, Han Y, Yang L Kim T, Nair M, Harschnitz O, et al. SARSCoV-2 infection causes dopaminergic neuron senescence. Res Sq. (2021) 5.21:rs.3.rs-513461. doi: 10.21203/rs.3.rs-513461/v1
25. Kubota T, Kuroda N. Exacerbation of neurological symptoms and COVID-19 severity in patients with preexisting neurological disorders and COVID-19: a systematic review. Clin Neurol Neurosurg. (2021) 200:106349–55. doi: 10.1016/j.clineuro.2020.106349
26. Bouali-Benazzouz R, Benazzouz A. COVID-19 infection and parkinsonism: is there a link? Mov Disord. (2021) 36:1737–43. doi: 10.1002/mds.28680
27. Sulzer D, Antonini A, Leta V, Nordvig A, Smeyne RJ, Goldman JE, et al. COVID-19 and possible links with Parkinson's disease and parkinsonism: from bench to bedside. npj Parkinsons Dis. (2020) 6:18. doi: 10.1038/s41531-020-00123-0
28. Beauchamp LC, Finkelstein DI, Bush AI, Evansd AH, Barnham KJ. Parkinsonism as a third wave of the COVID-19 pandemic? J Parkinsons Dis. (2020) 10:1343–53. doi: 10.3233/JPD-202211
29. Baazaoui N, Iqbal K. COVID-19 and neurodegenerative diseases: prion-like spread and long-term consequences. J Alzheimers Dis. (2022) 88:399–416. doi: 10.3233/JAD-220105
30. Merello M, Bhatia KP, Obeso JA. SARS-CoV-2 and the risk of Parkinson's disease: facts and fantasy. Lancet Neurol. (2021) 20:94–95. doi: 10.1016/S1474-4422(20)30442-7
31. Awogbindin IO, Ben-Azu B, Olusola BA, Akinluyi ET, Adeniyi PA, Paolo TD, et al. Microglial implications in SARS-CoV-2 infection and COVID-19: lessons from viral RNA neurotropism and possible relevance to Parkinson's Disease. Front Cell Neurosci. (2021) 15:670298. doi: 10.3389/fncel.2021.670298
32. Dorsey ER, Sherer T, Okun MS, Bloem BR. The emerging evidence of the Parkinson pandemic. J Parkinsons Dis. (2018) 8:S3–8. doi: 10.3233/JPD-181474
33. Massey D Baker AD Berrent DZ Güthe N Shidlovsky SP Fisher L. Internal tremors and vibration symptoms among people with postacute sequelae of SARS-CoV-2: a narrative review of patient reports. medRxiv. (2021) 12:12.03.21267146. doi: 10.1101/2021.12.03.21267146
34. Davis HE, Assaf GS, McCorkell L, Weia H, Lowa RJ, Re'em Y, et al. Characterizing long COVID in an international cohort: 7 months of symptoms and their impact. EClinicalMedicine. (2021) 38:101019. doi: 10.1016/j.eclinm.2021.101019
35. Yusuf F, Fahriani M, Mamada SS, Frediansyah A, Abubakar A, Maghfirah D, et al. Global prevalence of prolonged gastrointestinal symptoms in COVID-19 survivors and potential pathogenesis: a systematic review and meta-analysis. F1000Research. (2021) 10:301–19. doi: 10.12688/f1000research.52216.1
36. Wang L, Shen Y, Li M, Chuang H, Ye Y, Zhao H, et al. Clinical manifestations and evidence of neurological involvement in 2019 novel coronavirus SARS-CoV-2: a systematic review and meta-analysis. J Neurol. (2020) 267:2777–89. doi: 10.1007/s00415-020-09974-2
37. Favas TT, Dev P, Chaurasis RN, Chakravarty K, Mishra R, Joshi D, et al. Neurological manifestations of COVID-19: a systematic review and meta-analysis of proportions. Neurological Sci. (2020) 41:3437–70. doi: 10.1007/s10072-020-04801-y
38. Rogers JP, Watson CJ, Badenoch J, Cross B, ButlerM, Song J, et al. Neurology and Neuropsychiatry of COVID-19: a systematic review and meta-analysis of the early literature reveals frequent CNS manifestations and key emerging narratives. J Neurol Neurosurg Psychiatry. (2021) 92:932–41. doi: 10.1136/jnnp-2021-326405
39. Vitalakumar D, Sharma A, Jumar A, Flora SJS. Neurological manifestations in COVID-19 patients: a meta-analysis. ACS Chem Neurosci. (2021) 12:2776–97. doi: 10.1021/acschemneuro.1c00353
40. Yassin A, Nawaiseh M, Shaban A, Alsherbini K, El-Salem K, Soudah O, et al. Neurological manifestations and complications of coronavirus disease (2019) (COVID-19): a systematic review and meta-analysis. BMC Neurol. (2021) 21:138–55. doi: 10.1186/s12883-021-02161-4
41. Cardenas G, Soto-Hernandez JL, Diaz-Alba A, Ugalde Y, Merida-Puga J, Rosetti M, et al. Neurological events related to influenza (H1N1) pdm09. Influenza Other Respir Viruses. (2014) 8:339–46. doi: 10.1111/irv.12241
42. Proal AD, VanElzakkerMB. Long COVID or Post-acute sequelae of COVID-19 (PASC): an overview of biological factors that may contribute to persistent symptoms. Front Microbiol. (2021) 12:698169. doi: 10.3389/fmicb.2021.698169
43. Logue JK, Franko NM, McCulloch DJ, McDonald D, Magedson A, Wolf CR, et al. Sequelae in adults at 6 months after COVID-19 infection. JAMA Netw Open. (2021) 4:210830. doi: 10.1001/jamanetworkopen.2021.0830
44. Sklinda K, Dorobek M, Wasilewski PG, Drezewski K, Debicka M, Walecki J, et al. Radiological manifestation of neurological complications in the course of SARS-CoV-2 infection. Front Neurol. (2021) 12:711026. doi: 10.3389/fneur.2021.711026
45. Kim PW, Kim M, Suh CH, Chung SR, Park JE, Kim SC, et al. Neuroimaging findings in patients with COVID-19: a systematic review andmeta-analysis. Korean J Radiol. (2021) 22:1875–85. doi: 10.3348/kjr.2021.0127
46. Choi Y, Lee MK. Neuroimaging findings of brain MRI and CT in patients with COVID-19: a systematic review and meta-analysis. Eur J Radiol. (2020) 133. 109393. doi: 10.1016/j.ejrad.2020.109393
47. Lee MH, Perl DP, Nair G, Li W, Maric D, Murray H, et al. Microvascular injury in the brains of patients with COVID-19. N Engl J Med. (2021) 384:481–3. doi: 10.1056/NEJMc2033369
48. Lewis A, Frontera J, Placantonakis DG, Lighter J, Galetta S, Balcer L, et al. Cerebrospinal fluid in COVID-19: a systematic review of the literature. J Neurol Sci. (2021). doi: 10.1016/j.jns.2021.117316
49. Matschke J, Lutgehetmann M, Hagel C, Sperhake JP, Schröder AS, Edler C, et al. Neuropathology of patients with COVID-19 in Germany: a post-mortemcase series. Lancet Neurol. (2020) 19:919–29. doi: 10.1016/S1474-4422(20)30308-2
50. Zanin L, Saraceno G, Panciani PP, Renisi G, Signorini L, Migliorati K, Fontanella MM. SARS-CoV-2 can induce brain and spine demyelinating lesions. Acta Neurochir. (2020) 162:1491–4. doi: 10.1007/s00701-020-04374-x
51. Tsai JP, Baker AJ. Influenza-associated neurological complications. Neurocritical Care. (2013) 18:118–30. doi: 10.1007/s12028-012-9796-8
52. Davis LE, Koster F, Cawthon A. Neurologic aspects of influenza viruses. Handb Clin Neurol. (2014) 123:619–45. doi: 10.1016/B978-0-444-53488-0.00030-4
53. Akins PT, Belko J, Uyeki TM, Axelrod Y, Lee KK, Silverthorn J. H1N1 encephalitis with malignant edema and review of neurologic complications from influenza. Neurocrit Care. (2010) 13:396–406. doi: 10.1007/s12028-010-9436-0
54. Macfarlane RG, Wroe SJ, Collinge J, Yousry TA, Jager HR. Neuroimaging findings in human prion disease. J Neurol Neurosurg Psychiatry. (2007) 78:664–70. doi: 10.1136/jnnp.2006.094821
55. Fragoso DC, Goncalves Filho AL, Pacheco FT, Barros BR, Littig IA, Nunes RH, et al. Imaging of Creutzfeldt-Jakob disease: imaging patterns and their differential diagnosis. Radiographics. (2017) 37:234–57. doi: 10.1148/rg.2017160075
56. Marquez F, YassaMA. Neuroimaging biomarkers for Alzheimer's disease. Mol Neurodegen. (2019) 14:21–35. doi: 10.1186/s13024-019-0325-5
57. Mitchel T, Lehericy S, Chiu SY, Strafella AP, Stoessl AJ, Vaillancourt DE. Emerging neuroimaging biomarkers across disease stage in Parkinson's disease: a review. JAMA Neurol. (2021) 78:1262–72. doi: 10.1001/jamaneurol.2021.1312
58. Saeed U, Lang AE, Masellis M. Neuroimaging advances in Parkinson's disease and atypical Parkinsonian syndromes. Front Neurol. (2020) 11:572976. doi: 10.3389/fneur.2020.572976
59. Dadar M, Manera AL, Zinman L, Korngut L, Genge A, Graham SJ, et al. Cerebral atrophy in amyotrophic lateral sclerosis parellels the pathological distribution of TDP43. Brain Commun. (2020) 2:fcaa061. doi: 10.1093/braincomms/fcaa061
60. Mazon M, Vazquez Costa JF, Ten-Esteve A, Martí-Bonmatí L. Imaging biomarkers for the diagnosis and prognosis of neurodegenerative diseases. The example of amyotrophic lateral sclerosis. Front Neurosci. (2018) 12:784. doi: 10.3389/fnins.2018.00784
61. Menke RAL, Agosta F, Grosskreutz J, Filippi M, Turner MR. Neuroimaging endpoints in amyotrophic lateral sclerosis. Neurotherapeutics. (2017) 14:11–23. doi: 10.1007/s13311-016-0484-9
62. ACEP (American College of Emergency Physicians). Available online at: https://www.acep.org/corona/covid-19-field-guide/assessment/laboratory-abnormalities (accessed February 23, 2022).
63. Meijer WJ, Linn FH, Wensing AM, Wensing AMJ, Leavis HL, van Riel D, et al. Acute influenza virus-associated encephalitis and encephalopathy in adults: a challenging diagnosis. JMM Case Rep. (2016) 3:e005076. doi: 10.1099/jmmcr.0.005076
64. Rudge P, Hyare H, Green A, Collinge J, Mead S. Imaging and CSF analyses effectively distinguish CJD from its mimics. J Neurol Neurosurg Psychiatry. (2018) 89:461–6. doi: 10.1136/jnnp-2017-316853
65. Mead S, Rudge P. CJD mimics and chameleons. Pract Neurol. (2017) 17:113–21. doi: 10.1136/practneurol-2016-001571
66. Gabelli C. Blood and cerebrospinal fluid biomarkers for Alzheimer's disease. J Lab Precis Med. (2020) 5:15. doi: 10.21037/jlpm.2019.12.04
67. Kurbatova N, GargM, Whiley L, Chekmeneva E, Jiménez B, Gómez-Romero M, et al. Urinary metabolic phenotyping for Alzheimer's disease. Sci Rep. (2020) 10:21745. doi: 10.1038/s41598-020-78031-9
68. Gao L, Tang H, Nie K, Wang L, Zhao J, Gan R, et al. Cerebrospinal fluid alpha-synuclein as a biomarker for Parkinson's disease diagnosis: a systematic review and meta-analysis. Int J Neurosci. (2015) 125:645–54. doi: 10.3109/00207454.2014.961454
69. Parnetti L, Gaetani L, Eusebi P, Paciotti S, Hansson O, El-Agnaf O, et al. CSF and blood biomarkers for Parkinson's disease. Lancet Neurol. (2019) 18:573–86. doi: 10.1016/S1474-4422(19)30024-9
70. Majumder V, Gregory JM, Barria MA, Green A, Pal S. TDP-43 as a potential biomarker for amyotrophic lateral sclerosis: a systematic review and meta-analysis. BMC Neurol. (2018) 18:90. doi: 10.1186/s12883-018-1091-7
71. de Carvalho M. Eletrodiagnosis of Amyotrophic lateral sclerosis: a review of existing guidelines. J Clin Neurophysiol. (2020) 37:294–8. doi: 10.1097/WNP.0000000000000682
72. Nakajima N, Sato Y, Katano H, Hasegawa H, Kumasaka T, Hata S, et al. Histopathological and immunohistochemical findings of 20 autopsy cases with (2009) H1N1 virus infection. Mod Pathol. (2012) 25:1–3. doi: 10.1038/modpathol.2011.180
73. DeTure MA, Dickson DW. The neuropathological diagnosis of Alzheimer's disease. Mol Neurodegen. (2019) 14:32. doi: 10.1186/s13024-019-0333-5
74. Saberi S, Stauffer JE, Schulte DJ, Ravits J. Neuropathology of amyotrophic lateral sclerosis and its variants. Neurol Clin. (2015) 33:855–76. doi: 10.1016/j.ncl.2015.07.012
75. Kovacs GG, Budka H. Molecular pathology of human prion diseases. Int J Mol Sci. (2009) 10:976–99. doi: 10.3390/ijms10030976
76. Geut H, Hepp DH, Foncke E, Berendse HW, Rozemuller JM, Huitinga I, et al. Neuropathological correlates of parkinsonian disorders in a large Dutch autopsy series. Acta Neuropathol Communic. (2020) 8:39–53. doi: 10.1186/s40478-020-00914-9
77. Brandao PRP, Grippe TC, Pereira DA, Munhoz RP, Cardoso F. New-onset movement disorders associated with COVID-19. Tremor Other Hyperkinetic Mov. (2021) 11:1–17. doi: 10.5334/tohm.595
78. Oppo V, Melis M, Melis M, Barbarossa LT, Cossu G. “Smelling and Tasting” Parkinson's disease: using senses to improve the knowledge of the disease. Front Aging Neurosci. (2020) 12:43. doi: 10.3389/fnagi.2020.00043
79. Valerio F, Whitehouse DP, Menon DK, Newcombe VFJ. The neurological sequelae of pandemics and epidemics. J Neurol. (2021) 268:2629. doi: 10.1007/s00415-020-10261-3
80. Wan D, Du T, Hong W, Chen L, Que H, Lu S, et al. Neurological complications and infection mechanism of SARS-CoV-2. Sig Transduct Target Ther. (2021) 6:406–22. doi: 10.1038/s41392-021-00818-7
81. Balcom EF, Nath A, Power C. Acute and chronic neurological disorders in COVID-19: potential mechanisms of disease. Brain. (2021) 144:3576–88. doi: 10.1093/brain/awab302
82. Gracia-Ramos AE, Martin-Nares E, Hernandez-Molina G. New onset of autoimmune diseases following COVID-19 diagnosis. Cells. (2021) 10:3592. doi: 10.3390/cells10123592
83. Ramakrishnan RK, Kashour T, Hamid Q, Halwani R, Tleyjeh IM. Unraveling the mystery surrounding post-acute sequelae of COVID-19. Front Immunol. (2021) 12:686029. doi: 10.3389/fimmu.2021.686029
84. Bohmwald K, Galvez MNS, Rios M, Kalergis AM. Neurologic alterations due to respiratory virus infections. Front Cell Neurosci. (2018) 12:386. doi: 10.3389/fncel.2018.00386
85. Saa P, Harris DA, Cervenakova L. Mechanisms of prion-induced neurodegeneration. Expert Rev Mol Med. (2016) 18:e5. doi: 10.1017/erm.2016.8
86. Liu J, Xu F, Nie Z, Shao L. Gut microbiota approach—a new strategy to treat Parkinson's disease. Front Cell Infect Microbiol. (2021) 10:570658. doi: 10.3389/fcimb.2020.570658
87. Sanz-Hernandez M, Barritt JD, Sobek J, Hornemannc S, Aguzzic A, Simone AD. Mechanism of misfolding of the human prion protein revealed by a pathological mutation. Proc Natl Acad Sci USA. (2021) 118:e2019631118. doi: 10.1073/pnas.2019631118
88. Sun Y, Sommerville NR, Liu JYH, Ngan MP, Poon D, Ponomarev ED, et al. Intra-gastrointestinal amyloid-beta1–42 oligomers perturb enteric function and induce Alzheimer's disease pathology. J Physiol. (2020) 598:4209–23. doi: 10.1113/JP279919
89. Dominy SS, Lynch C, Ermini F, Benedyk M, Marczyk A, Konradi A, et al. Porphyromonas gingivalis in Alzheimer's disease brains: evidence for disease causation and treatment with small-molecule inhibitors. Sci Adv. (2019) 5:eaau3333. doi: 10.1126/sciadv.aau3333
90. Shin WS, Di J, Cao Q, Li B, Seidler PM, Murray KA, et al. Amyloid-beta protein oligomers promote the uptake of tau fibril seeds potentiating intracellular tau aggregation. Alz Res Ther. (2019) 11:86. doi: 10.1186/s13195-019-0541-9
91. Guo T, Zhang D, Zehng Y, Huang TY, Xu H, Zhao Y. Molecular and cellular mechanisms underlying the pathogenesis of Alzheimer's disease. Mol Neurodegen. (2020) 15:40. doi: 10.1186/s13024-020-00391-7
92. Fitzgerald E, Murphy S, Martinson HA. Alpha-synuclein pathology and the role of the microbiota in Parkinson's disease. Front Neurosci. (2019) 13:369. doi: 10.3389/fnins.2019.00369
93. Garretti F, Agalliu D, Lindestam Arlehamn CS, Sette A, Sulzer D. Autoimmunity in Parkinson's disease: the role of alpha-synuclein-specific T cells. Front Immunol. (2019) 10:303. doi: 10.3389/fimmu.2019.00303
94. Maiti P, Manna J, Dunbar GL. Current understanding of the molecular mechanisms in Parkinson's disease: targets for potential treatments. Transl Neurodegener. (2017) 6:28–63. doi: 10.1186/s40035-017-0099-z
95. Xue YC, Ruller CM, Fung G, Mohamud Y, Deng H, Liu H, et al. Enteroviral infection leads to transactive response DNA-binding protein 43 pathology in vivo. Immunopathol Infect Dis. (2018) 188:2853–62. doi: 10.1016/j.ajpath.2018.08.013
96. Doubille RN, Nath A. Human endogenous retrovirus-K and TDP-43 expression bridges ALS and HIV neuropathology. Front Microbil. (2017) 8:1986. doi: 10.3389/fmicb.2017.01986
97. Mejzini R, Flynn LL, Pitout IL, Fletcher S, Wilton SD, Akkari AP. ALS genetics, mechanisms, and therapeutics: where are we now? Front Neurosci. (2019) 13:1310. doi: 10.3389/fnins.2019.01310
98. Yachou Y, Idrissi AE, Belapasov V, Benali SA. Neuroinvasion, neurotropic, and neuroinflammatory events of SARS-CoV-2: understanding the neurological manifestations in COVID-19 patients. Neurol Sci. (2020) 28:1–13. doi: 10.1007/s10072-020-04575-3
99. Mishra R, Banerjea AC. Neurological damage by coronaviruses: a catastrophe in the queue! Front Immunol. (2020) 11:565521. doi: 10.3389/fimmu.2020.565521
100. Li Z, Liu T, Yang N, Han D, Mi X, Li Y, et al. Neurological manifestations of patients with COVID-19: potential routes of SARS-CoV-2 neuroinvasion from the periphery to the brain. Front Med. (2020) 14:533–41. doi: 10.1007/s11684-020-0786-5
101. Su Y, Yuan D, Chen DG, Ng RH, Wang K, Choi J, et al. Multiple early factors anticipate post-acute COVID-19 sequelae. Cell. (2022) 185:881–95.e20. doi: 10.1016/j.cell.2022.01.014
102. Zeng XS, Geng WS, Jia JJ, Chen L, Zhang P. Cellular and molecular basis of neurodegeneration in Parkinson's disease. Front Aging Neurosci. (2018) 10:109–25. doi: 10.3389/fnagi.2018.00109
103. Ubeda-Banon I, Saiz-Sanchez D, dela Rosa-Prieto C, Martinez-Marcos A. Alpha-synuclein in the olfactory system in Parkinson's disease: role of neural connections on spreading pathology. Brain Struct Funct. (2014) 219:1513–26. doi: 10.1007/s00429-013-0651-2
104. Braak H, Rub U, Gai WP, Del Tredici K. Idiopathic Parkinson's disease: possible routes by which vulnerable neuronal types may be subject to neuroinvasion by an unknown pathogen. J Neural Transm. (2003) 110:517–36. doi: 10.1007/s00702-002-0808-2
105. Braak H, Del Tredici K, Rub U, de Vos RAI, Steur ENHJ, Braak E. Staging of brain pathology related to sporadic Parkinson's disease. Neurobiol Aging. (2003) 24:197–211. doi: 10.1016/S0197-4580(02)00065-9
106. Ma J, Gao J, Wang J. Prion-like mechanisms in Parkinson's disease. Front Neurosci. (2019) 13:552. doi: 10.3389/fnins.2019.00552
107. Jana AK, Lander CW, Cheney AD, Hansmann UHE. Effect of an amyloidogenic SARS-CoV-2 protein fragment on alpha-synuclein monomers and fibrils. bioRxiv. (2022) 2:02.21.481360. doi: 10.1101/2022.02.21.481360
108. Pavel A, Murray DK, Stoessl AJ. COVID-19 and selective vulnerability of Parkinson's disease. Lancet Neurol. (2020) 19:719. doi: 10.1016/S1474-4422(20)30269-6
109. Idrees D, Kumar V. SARS-CoV-2 spike protein interactions with amyloidogenic proteins: potential clues to neurodegeneration. Biochem Biophys Res Commun. (2021) 554:94–8. doi: 10.1016/j.bbrc.2021.03.100
110. Wu Z, Zhang X, Huang Z, Ma K. SARS-CoV-2 proteins interact with alpha synuclein and induce Lewy Body-like pathology in vitro. Int J Mol Sci. (2022) 23:3394. doi: 10.3390/ijms23063394
111. Chaná-Cuevas P, Salles-Gándara P, Rojas-Fernandez A, Salinas-Rebolledo C, Milán-Solé A. The potential role of SARS-CoV-2 in the pathogenesis of Parkinson's disease. Front Neurol. (2020) 11:1044. doi: 10.3389/fneur.2020.01044
112. Szabo MP, Iba M, Nath A, Masliah E, Kim C. Does SARS-CoV-2 affect neurodegenerative disorders? TLR2, a potential receptor for SARS-CoV-2 in the CNS. Exp Mol Med. (2022) 54:447–54. doi: 10.1038/s12276-022-00755-7
113. Sadasivan S, Sharp, B, Stacey SC, Smeyne RJ. Synergistic effects of influenza and 1-methyl-4-phenyl-1,2,3,6-tetrapydropyridine (MPTP) can be eliminated by the use of influenza therapeutics: experimental evidence for the multi-hit hypothesis. Npj Parkinsons Dis. (2017) 3:18. doi: 10.1038/s41531-017-0019-z
114. Gravitz L. The promise and potential of stem cells in Parkinson's disease. Nature. (2021) 597:S8–10. doi: 10.1038/d41586-021-02622-3
115. Cushman M, Johnson BS, King OD, Gitler AD, Shorter J. Prion-like disorders: blurring the divide between transmissibility and infectivity. J Cell Sci. (2010) 123:1191–201. doi: 10.1242/jcs.051672
116. Goedert M. NEURODEGENERATION. Alzheimer's and Parkinson's diseases: the prion concept in relation to assembled Ab, tau, and a-synuclein. Science. (2015) 349:1255555. doi: 10.1126/science.1255555
117. Lee S, Kim HJ. Prion-like mechanism in amyotrophic lateral sclerosis: are protein aggregates the key? Exp Neurobiol. (2015) 24:1–7. doi: 10.5607/en.2015.24.1.1
118. Bu XL, Li WW, Wang YJ. Is Alzheimer's Disease transmissible in humans? Neurosci Bull. (2019) 35:1113–5. doi: 10.1007/s12264-019-00382-9
119. Thomzig A, Wagenfuhr K, Pinder P, Joncic M, Schulz-Schaeffer WJ, Beekes M. Transmissible alpha-synuclein seeding activity in brain and stomach of patients with Parkinson's disease. Acta Neuropathol. (2021) 141:861–79. doi: 10.1007/s00401-021-02312-4
120. Lam S, Petit F, Herard, AS, Boluda S, Eddarkaoui S, et al. Transmission of amyloid-beta and tau pathologies is associated with cognitive impairments in a primate. Acta Neuropathol Commun. (2021) 9:165. doi: 10.1186/s40478-021-01266-8
121. Ding X, Xiang Z, Qin C, Yongkang Chen, Haiyan Tian, Lin Meng, et al. Spreading of TDP-43 pathology via pyramidal tract induces ALS-like phenotypesin TDP-43 transgenic mice. Acta Neuropathol Commun. (2021) 9:15. doi: 10.1186/s40478-020-01112-3
122. Jaunmuktane Z, Mead S, Ellis M, Wadsworth JDF, Nicoll AJ, Kenny J, et al. Evidence for human transmission of amyloid-beta pathology and cerebral amyloid angiopathy. Nature. (2015) 525:247–50. doi: 10.1038/nature15369
123. Masuda-Suzukake M, Nonaka T, Hosokawa M, Oikawa T, Arai T, Akiyama H, et al. Prion-like spreading of pathological alpha-synuclein in brain. Brain. (2013) 136:1128–38. doi: 10.1093/brain/awt037
124. Goedert M, Eisenberg DS, Crowther RA. Propagation of Tau aggregates and neurodegeneration. Annu Rev Neurosci. (2017) 40:189–210. doi: 10.1146/annurev-neuro-072116-031153
125. Watts JC, Giles K, Oehler A, Middleton L, Dexter DT, Gentleman SM, et al. Transmission ofmultiple systematrophy prions to transgenicmice. Proc Natl Acad Sci USA. (2013) 110:19555–60. doi: 10.1073/pnas.1318268110
126. Carlson GA, Prusiner SB. How an infection of sheep revealed prion mechanisms in Alzheimer's Disease and other neurodegenerative disorders. Int J Mol Sci. (2021) 22:4861–83. doi: 10.3390/ijms22094861
127. Soscia SJ, Kirby JE, Washicosky KJ, Tucker SM, Ingelsson M, Hyman B, et al. The Alzheimer's disease-associated amyloid beta-protein is an antimicrobial peptide. PLoS ONE. (2010) 5:e9505. doi: 10.1371/journal.pone.0009505
128. Beatman EL, Massey A, Shives KD, Burrack KS, Chamanian M, Morrison TE, et al. Alpha-synuclein expression restricts RNA viral infections in the brain. J Virol. (2015) 90:2767–82. doi: 10.1128/JVI.02949-15
129. Harms AS, Thome AD, Yan Z, Schonhoff AM, Williams GP, Li X, et al. Peripheral monocyte entry is required for alpha-synuclein induced inflammation and neurodegeneration in a model of Parkinson disease. Exp Neurol. (2018) 300:179–87. doi: 10.1016/j.expneurol.2017.11.010
130. Ittner A, Chua SW, Berta J, Volkerling A, van der Hoven J, Gladbach A, et al. Site-specific phosphorylation of tau inhibits amyloid-beta toxicity in Alzheimer's mice. Science. (2016) 354:904–8. doi: 10.1126/science.aah6205
131. Liu W, Wang Z, Liu L, Yanga Z, Liua S, Ma Z, et al. LncRNA Malat1 inhibition of TDP-43 cleavage suppresses IRF3-initiated antiviral innate immunity. Proc Natl Acad Sci USA. (2020) 117:23695–706. doi: 10.1073/pnas.2003932117
132. Xue YC, Liu H, Mohamud Y, Bahreyni A, Zhang J, Cashman NR, et al. Sublethal enteroviral infection exacerbates disease progression in an ALS mouse model. J Neuroinflamm. (2022) 19:16–31. doi: 10.1186/s12974-022-02380-7
133. Munoz-Pinto MF, Empadinhas N, Cardoso SM. The neuromicrobiology of Parkinson's disease: a unifying theory. Aging Res Rev. (2021) 70:101396. doi: 10.1016/j.arr.2021.101396
134. Toh TS, Chong CW, L Shen-Yang, Shao L. Gut microbiome in Parkinson's disease: new insights from meta-analysis. Parkinson Relat Disord. (2022) 94:1–9. doi: 10.1016/j.parkreldis.2021.11.017
135. Blacher E Bashiardes S, Shapiro H, Rothschild D, Mor U, Dori-Bachash M, et al. Potential roles of gut microbiome and metabolites in modulating ALS in mice. Nature. (2019) 572:474–80. doi: 10.1038/s41586-019-1443-5
136. D'Argenio V, Sarnataro D. Microbiome influence in the pathogenesis of prion and Alzheimer's diseases. Int J Mol Sci. (2019) 20:4704. doi: 10.3390/ijms20194704
137. Lim B, Prassas I, Diamandis EP. Alzheimer disease pathogenesis: the role of autoimmunity. J Applied Lab Med. (2021) 6:756–764. doi: 10.1093/jalm/jfaa171
138. Bonam SR, Muller S. Parkinson's disease is an autoimmune disease: a reappraisal. Autoimmune Rev. (2020) 19:102684. doi: 10.1016/j.autrev.2020.102684
139. Pagani MF, Gonzalez LE, Uchitel OD. Autoimmunity in amyotrophic lateral sclerosis: past and present. Neurol Res Int. (2011) 2011:497080. doi: 10.1155/2011/497080
140. Zhu BT. Human and animal spongiform encephalopathies are the result of chronic autoimmune attack in the CNS: a novel medical theory supported by overwhelming experimental evidence. Hostol Histopathol. (2005) 20:575–92. doi: 10.14670/HH-20.575
141. Braak H, Braak E. Neuropathological staging of Alzheimer-related changes. Acta Neuropathol. (1991) 82:239–59. doi: 10.1007/BF00308809
142. Braak H, Alafuzoff I, Arzberger T, Kretzschmer H, Tredici KD. Staging of Alzheimer disease-associated neurofibrillary pathology using paraffin sections and immunocytochemistry. Acta Neuropathol. (2006) 112:389–404. doi: 10.1007/s00401-006-0127-z
143. Rietdijk CD, Perez-Pardo P, Garssen J, van Wezel RJA, Kraneveld AD. Exploring Braak's hypothesis of Parkinson's disease. Front Neurol. (2017) 8:37. doi: 10.3389/fneur.2017.00037
144. Biel D, Brendel M, Rubinski A, Buerger K, Janowitz D, Dichgans M, et al. Tau-PET and in vivo Braak-staging as prognostic markers of future cognitive decline in cognitively normal to demented individuals. Alz Res Ther. (2021) 13:137. doi: 10.1186/s13195-021-00880-x
145. Shannon KM, Keshavarzian A, Mutlu E, Dodiya HB, Daian D, Jaglin JA, et al. Alpha-synuclein in colonic submucosa in early untreated Parkinson's disease. Mov Disord. (2012) 27:709–15. doi: 10.1002/mds.23838
146. Dickson DW, Fujishiro H, DelleDonne A, Menka J, Ahmed Z, Klos KJ, et al. Evidence that incidental Lewy body disease is pre-symptomatic Parkinson's disease. Acta Neuropathol. (2008) 115:437–44. doi: 10.1007/s00401-008-0345-7
147. Marreiros R, Muller-Schiffmann A, Trossbach SV, Prikulisa I, Hänschb S, Weidtkamp-Peters S, et al. Disruption of cellular proteostasis by H1N1 influenza A virus causes alpha-synuclein aggregation. Proc Natl Acad Sci USA. (2020) 117:6741–51. doi: 10.1073/pnas.1906466117
148. Lindman KL, Hemmingsson ES, Weidung B, Brännström J, Josefsson M, Olsson J, et al. Herpesvirus infections, antiviral treatment, and the risk of dementia registry-based cohort study in Sweden. Alzhe Dement Transl Res Clin Interv. (2021) 7:e12119. doi: 10.1002/trc2.12119
149. Wainberg M, Luquez T, Koelle DM, Readhead B, Johnston C, Darvas M, et al. The viral hypothesis: how herpesviruses may contribute to Alzheimer's disease. Mol Psychiatry. (2021) 26:5476–80. doi: 10.1038/s41380-021-01138-6
150. Caggiu E, Arru G, Hosseini S, Niegowska M, Sechi G, Zarbo IR, et al. Inflammation, infectious triggers and Parkinson's disease. Front Neurol. (2019) 10:122. doi: 10.3389/fneur.2019.00122
151. Habib AG, Yakasai AM, Owolabi LF, Ibrahim A, Habib ZG, Gudaji M, et al. Neurocognitive impairment in HIV-1-infected adults in Sub-Saharan Africa: a systematic review and meta-analysis. Int J Infect Dis. (2013) 17:820–31. doi: 10.1016/j.ijid.2013.06.011
152. Maschke M, Kastrup O, Esser S, Ross B, Hengge U, Hufnagel A. Incidence and prevalence of neurological disorders associated with HIV since the introduction of highly active antiretroviral therapy (HAART). J Neurol Neurosurg Psychiatry. (2000) 69:376–80. doi: 10.1136/jnnp.69.3.376
153. Cheng YW, Lin CH, Wu RM. HIV-associated parkinsonism reversed with antiretroviral therapy. Reurol Asia. (2014) 19:199–203.
154. Beingpatient.com. Available online at: https://www.beingpatient.com/there-are-9-alzheimers-vaccines-in-trials-right-now/ (assessed February 20, 2022).
155. Soeda Y and Takashima A. New insights into drug discovery targeting tau protein. Front Mol Neurosci. (2020) 13:590896. doi: 10.3389/fnmol.2020.5.90896
156. Barbut D, Stolzenberg E, Zasloff M. Gastrointestinal immunity and alphasynuclein. J Parkinsons Dis. (2019) 9:313–22. doi: 10.3233/JPD-191702
157. Jamal F. Immunotherapies targeting alpha-synuclein in Parkinson disease. Fec Pract. (2020) 37:375–9. doi: 10.12788/fp.0026
158. Fleming SM, Davis, A, Simons E. Targeting alpha-synuclein via the immune system in Parkinson's disease: current vaccine therapies. Neuropharmacology. (2022) 202:108870. doi: 10.1016/j.neuropharm.2021.108870
159. Cragnaz L, Spinelli G, Conti LD, Bureau EA, Brownlees J, Feiguin F, et al. Thioridazine reverts the phenotype in cellular and Drosophila models of amyotrophic lateral sclerosis by enhancing TDP-43 aggregate clearance. Neurobiol Dis. (2021) 160:105515. doi: 10.1016/j.nbd.2021.105515
Keywords: COVID-19, Parkinson's disease, neurological symptoms, neurodegenerative disorders, post-COVID-19 parkinsonism management and prevention
Citation: Zhang J (2022) Investigating neurological symptoms of infectious diseases like COVID-19 leading to a deeper understanding of neurodegenerative disorders such as Parkinson's disease. Front. Neurol. 13:968193. doi: 10.3389/fneur.2022.968193
Received: 13 June 2022; Accepted: 08 August 2022;
Published: 07 December 2022.
Edited by:
Antonio Emanuele Elia, IRCCS Carlo Besta Neurological Institute Foundation, ItalyReviewed by:
Tsepo Goerttler, Essen University Hospital, GermanyCao Chen, National Institute for Viral Disease Control and Prevention (China CDC), China
Copyright © 2022 Zhang. This is an open-access article distributed under the terms of the Creative Commons Attribution License (CC BY). The use, distribution or reproduction in other forums is permitted, provided the original author(s) and the copyright owner(s) are credited and that the original publication in this journal is cited, in accordance with accepted academic practice. No use, distribution or reproduction is permitted which does not comply with these terms.
*Correspondence: Jing Zhang, jzhang0000@gmail.com